Kinetic energy harvesting based sensing and IoT systems: A review
- 1School of Information Science and Technology, ShanghaiTech University, Shanghai, China
- 2Guangdong Provincial Key Lab of Robotics and Intelligent System, Guangdong-Hong Kong-Macao Joint Laboratory of Human-Machine Intelligence-Synergy Systems, Shenzhen Institute of Advanced Technology, Chinese Academy of Sciences, Shenzhen, China
The rapid advance of the Internet of Things (IoT) has attracted growing interest in academia and industry toward pervasive sensing and everlasting IoT. As the IoT nodes exponentially increase, replacing and recharging their batteries proves an incredible waste of labor and resources. Kinetic energy harvesting (KEH), converting the wasted ambient kinetic energy into usable electrical energy, is an emerging research field where various working mechanisms and designs have been developed for improved performance. Leveraging the KEH technologies, many motion-powered sensors, where changes in the external environment are directly converted into corresponding self-generated electrical signals, are developed and prove promising for multiple self-sensing applications. Furthermore, some recent studies focus on utilizing the generated energy to power a whole IoT sensing system. These systems comprehensively consider the mechanical, electrical, and cyber parts, which lead a further step to truly self-sustaining and maintenance-free IoT systems. Here, this review starts with a brief introduction of KEH from the ambient environment and human motion. Furthermore, the cutting-edge KEH-based sensors are reviewed in detail. Subsequently, divided into two aspects, KEH-based battery-free sensing systems toward IoT are highlighted. Moreover, there are remarks in every chapter for summarizing. The concept of self-powered sensing is clarified, and advanced studies of KEH-based sensing in different fields are introduced. It is expected that this review can provide valuable references for future pervasive sensing and ubiquitous IoT.
1 Introduction
To date, the Internet of Things (IoT) has kept bridging the cyber and physical worlds with cutting-edge monitoring, control, and management capabilities (Atzori et al., 2010; Yang et al., 2022). The countless IoT devices are placed in all manner of locations, and many of them are remote or inaccessible. In this case, replacing and recharging the batteries of sensors leads to monitoring interruptions and waste of labor and resources (Hester and Sorber, 2017). In addition, the threat of global warming and energy crises makes pursuing renewable and green energy sources with lower carbon emissions one of the most urgent matters of the moment (King, 2004; Chu and Majumdar, 2012; Chu et al., 2016). Therefore, the chemical batteries are not in line with the future trends and prove incompatible with ubiquitous sensing and IoT.
Energy harvesting (EH), reclaiming the wasted ambient energy, proves a thriving and promising environment-friendly technology that prolongs the life of sensing nodes, facilitating the development of battery-free IoT (Shaikh and Zeadally, 2016; Liu et al., 2018b; Preimesberger et al., 2020). Utilizing solar cells or radio frequency to capture ambient energy can be widely seen in multiple current applications (Zhao et al., 2015; Jo and Park, 2021; Sandhu et al., 2021), but a heavy reliance on temporal and spatial conditions significantly reduces the feasibility of these battery-free devices. For such case, the kinetic energy harvesting (KEH), a rapidly growing technology using kinetic energy mostly converted from ambient environment (Platt et al., 2005; Yang B. et al., 2009; Yang et al., 2017, Yang et al., 2009 Y.; Khameneifar et al., 2012; Wang, 2013; Zhu et al., 2015, 2014; Bae et al., 2014; Haroun et al., 2015; Zhang Y. et al., 2016; Tao et al., 2016; Abdelkareem et al., 2018; Zhou et al., 2019) or human motion (Rome et al., 2005; Bowers and Arnold, 2009; Bai et al., 2013; Hou et al., 2013; Chen et al., 2016; Liu et al., 2018a; Bai et al., 2018; Li et al., 2018; Singh et al., 2018; Wang et al., 2018; Yan et al., 2018; Li C. et al., 2019; Wang J. et al., 2019; Zhao L.-C. et al., 2019; Cai et al., 2019; Chen et al., 2019; Fan et al., 2019; Gao et al., 2019; Khan et al., 2019; Xie et al., 2019), becomes more reliable for motion-powered devices. The state-of-the-art mechanisms of KEH are limited to transductions based on electromagnetic effect (Rome et al., 2005), piezoelectric effect (Wang, 2012), and electrostatic effect (Mitcheson et al., 2004). Recently, to sufficiently power battery-free devices, using multiple types of energy harvested by hybrid EH mechanisms has become the trend (Ryu et al., 2019; Jiang et al., 2020).
With the rapid advance of the IoT, there is a growing demand for systems with many sensor nodes in a range of application areas for sensing. However, the sensing functions of traditional devices rely on active sensors powered by batteries, which proves incompatible for scenarios with constrained resources (Khalifa et al., 2015a). Achieving sensing with ultra-low power consumption is a key problem for battery-free devices. Under the advancement of KEH, harvesters based on different mechanisms can actively function as self-powered sensors to detect, monitor, and respond to the ambient changes induced by ambient environment or human motion (De Pasquale and Somà, 2013; Hu et al., 2013; Lin et al., 2013, 2021; Bai et al., 2014; Zhao and You, 2014; Khalifa et al., 2015b; Fan et al., 2015; Wu et al., 2015; Yi et al., 2015; Yu et al., 2015; Proto et al., 2016a; Proto et al., 2016b; Asadi et al., 2017; Chen et al., 2017; Askari et al., 2018; Chen et al., 2018; Ma et al., 2018; Shi et al., 2019; Zhu et al., 2019). Implementing self-powered sensors has greatly contributed to the development of pervasive sensing and ubiquitous IoT.
Although the KEH-based sensors can effectively convert mechanical energy into electrical energy, the alternating and intermittent output is insufficient for the whole system (Sandhu et al., 2020). In other words, in the system of signal recognition, only the part of the signal generation is passive, while all other parts are powered by batteries. For a genuinely self-sustaining and maintenance-free sensing system, there still exist many issues to tackle. Thanks to the contributions of many scholars, some cyber-electro-mechanical co-designs have been proposed for self-powered sensing IoT (Kymissis et al., 1998; Kuang and Zhu, 2016; Kuang et al., 2017; Li et al., 2021a; Li, 2021). The pace of self-powered sensing devices and systems development is brisk, and the co-design between mechanical dynamics, electrical power conversion, and information processing is urgent.
In this paper, we comprehensively review the state-of-the-art motion-powered sensors and IoT sensing systems based on piezoelectric, electromagnetic, and triboelectric energy harvesting. First, the fundamentals of KEH are briefly introduced, and multiple classical studies based on three mechanisms from the ambient environment and human motion are discussed. Then, the concept and cutting-edge studies of KEH-based sensors and IoT sensing systems are focused on and proposed in detail. To the best of our knowledge, a systematic review paper focusing on the aforementioned two parts remains unexplored. Hopefully, this review can provide valuable references for future self-powered sensing and battery-free IoT fields.
2 Kinetic energy harvesting
Kinetic energy, as a ubiquitous energy source, is one of the most common and easily accessible energies in our lives. KEH proves the superior technology that converts the wasted mechanical energy from the ambiance into useful green electric energy, whose transduction mechanisms typically include piezoelectric, electromagnetic, and triboelectric phenomena and their combinations (Fu et al., 2021).
1) Piezoelectricity, also known as the piezoelectric effect, was first identified by the Curie brothers in 1880 (Katzir, 2006). It generates electrical energy due to the inherent polarization characteristics of certain crystals, i,e., piezoelectricity results from the deformation of an external object on a piezoelectric material (Kim et al., 2009).
2) Electromagnetic effect-based generator has long been the dominant method for large-scale KEH, which requires interaction between a hard magnet (i.e., NdFeB) and conductive coils (i.e., copper wires) (Beeby et al., 2007). Kinetic energy results in the relative movement between the magnet and coils, producing electricity.
3) In the last decade, triboelectric nanogenerator (TENG), a fundamentally new energy technology invented in 2012, has attracted widespread interest (Fan et al., 2012; Yang et al., 2012). Relying on a coupling effect of contact electrification and electrostatic induction, the unique working mechanism with broad applicability, especially the flexible feature, distinguishes the TENG as a reliable, cost-effective, and efficient method for high-performance vibration energy harvesting, especially in the low-frequency range (Yang et al., 2013a; Wang, 2013; Jing et al., 2014a; Guo et al., 2015b; Kim et al., 2015; Chen and Wang, 2017).
In addition, electrostatic EH, achieved by utilizing a parallel plate capacitor with one plate fixed and the other one movable, mainly harvests the vibration energy in the machines (Tang et al., 2018). Triboelectric EH proves an emerging type of electrostatic EH that without a bias-voltage for normal operation (Liang et al., 2021). Since the decreasing number of works based on traditional electrostatic EH in recent years, this paper will not focus on this part.
The two most common occasions of kinetic energy in life, KEH from the ambient environment and KEH from human motion, are presented below. The three perspectives of piezoelectricity, magnetism, and triboelectricity, respectively, are used to review the typical studies.
2.1 KEH from ambient environment
Vibration, a type of common mechanical motion, exists widely in industrial machinery equipment and mainly lies below a few hundred hertz. The typical designs based on piezoelectric, electromagnetic, and triboelectric EH from ambient vibration are briefly shown in Figures 1A–L, respectively.
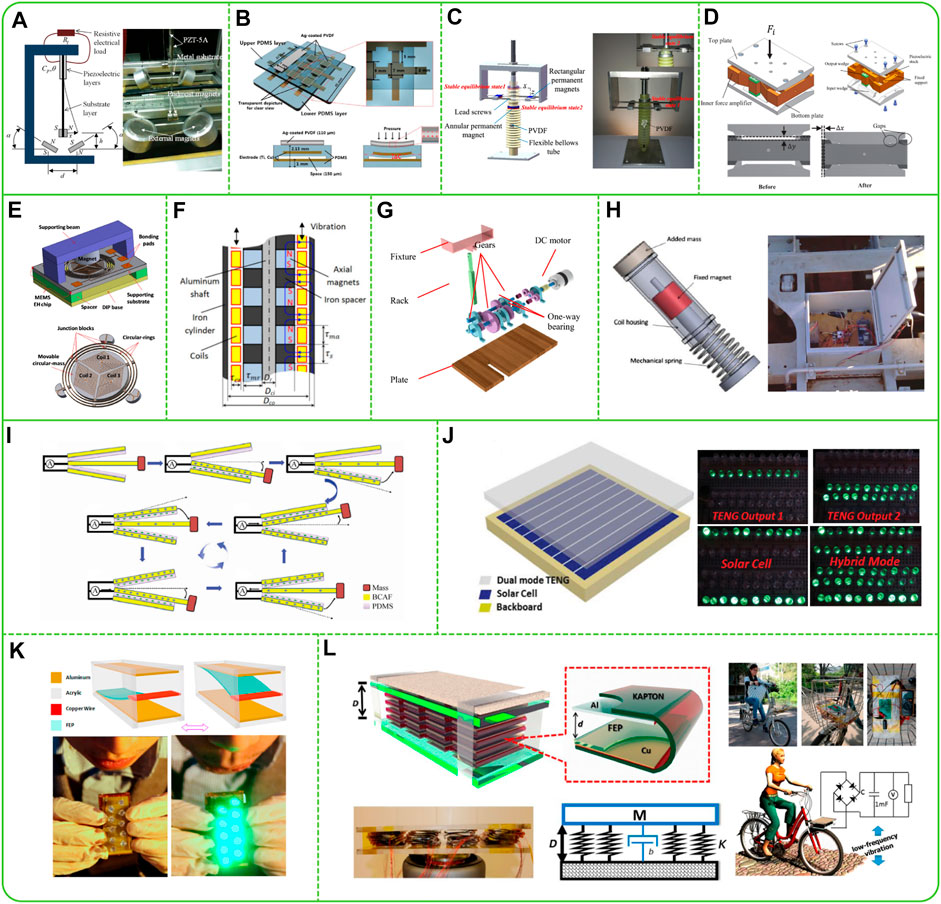
FIGURE 1. Different KEH technologies from the ambient environment. (A) Schematic diagram and close-up view of BPEH (Zhou et al., 2013). (B) Schematic diagram of the flexible energy harvester (Eun et al., 2014). (C) Schematic and prototype of NBEH (Deng et al., 2019). (D) Isometric, sectional and side views of the integrated multistage piezoelectric energy harvester (Wen and Xu, 2019). (E) Schematically drawing and perspective view of the 3D harvester (Liu et al., 2012). (F) Dimensions and parameters of LETs (Tang et al., 2014). (G) Design of the high-efficiency electromagnetic energy harvester (Zhang X. et al., 2016). (H) Mechanical design and field test photograph of the electromagnetic vibration converter (Bradai et al., 2018). (I) A cycle of the electricity generation process of the triple-cantilever based TENG (Yang et al., 2013a). (J) Schematic diagram of the as-fabricated hybridized power pane and the photograph of 50 commercial LED bulbs driven by the hybridized power pane (Zheng et al., 2015). (K) Schematic diagram of the TENG and the photograph showing that the proposed TENG was used to harvest the human mouth blowing induced wind energy (Yang Y. et al., 2013). (L) Schematic diagram, prototype, detailed structure, equivalent mechanical model, and field test photograph of the elastic multiunit TENG (Wang et al., 2017).
2.1.1 Piezoelectric-based KEH
To begin with, some piezoelectric designs are introduced as follows. The authors of (Zhou et al., 2013) investigate an alternative nonlinear Broadband Piezoelectric Energy Harvester (BPEH) with rotatable magnets for enhanced broadband frequency response. The schematic and close-up view of BPEH are shown in Figure 1A. In addition, a new design of flexible energy harvester was proposed to utilize piezoelectric and electrostatic EH concurrently from a single mechanical energy source (Eun et al., 2014), whose schematic diagram is illustrated in Figure 1B. The work in (Deng et al., 2019) presents a nonbeam-type bistable vibrational energy harvester (NBEH) based on a novel bellows structure with permanent magnets that exploits nonlinear bistability to enhance its performance at low frequencies. The schematic and prototype of NBEH are depicted in Figure 1C. In (Wen and Xu, 2019), a novel compact integrated multistage piezoelectric energy harvester has been designed to improve the safety issue of the force amplifiers under large loading situations, whose isometric, sectional, and side views are shown in Figure 1D.
2.1.2 Electromagnetic-based KEH
Furthermore, some electromagnetic-based studies are shown below. The authors of (Liu et al., 2012) develop and characterize a novel electromagnetic energy harvester with multiple modes using three-dimensional (3D) excitation at different frequencies. The schematically drawing and perspective view of the 3D harvester are shown in Figure 1E. In addition, the work in (Tang et al., 2014) proposes the study of tubular linear electromagnetic transducers (LETs) for large-scale vibration energy harvesting applications from vehicle suspensions, tall buildings, or long-span bridges. The dimensions and parameters of LETs are depicted in Figure 1F. A portable high-efficiency electromagnetic energy harvesting system with supercapacitors that converts the energy of track vibrations into electricity was developed in (Zhang X. et al., 2016), and the design of the harvester is illustrated in Figure 1G. With a similar function, in (Bradai et al., 2018), the authors propose an electromagnetic vibration converter specially developed for railway application, whose mechanical design and field test photograph are shown in Figure 1H.
2.1.3 Triboelectric-based KEH
Lastly, TENG-based research are reported underneath. The authors of (Yang et al., 2013a) demonstrate a rationally designed triple-cantilever-based TENG for harvesting vibration energy. A cycle of the electricity generation process of the triple-cantilever-based TENG is illustrated in Figure 1I. In addition, a hybridized power panel has been proposed for simultaneously generating energy from sunlight, raindrop, and wind (Zheng et al., 2015). The schematic diagram of the as-fabricated hybridized power pane and the photograph of 50 commercial LED bulbs driven by the hybridized power pane are shown in Figure 1J. Moreover, in (Yang Y. et al., 2013), a TENG based on wind energy is designed. The schematic diagram of the TENG and the photograph showing that the proposed TENG was used to harvest the human mouth blowing induced wind energy are shown in Figure 1K. The work in (Wang et al., 2017) designs an elastic multiunit TENG to harvest low-frequency vibration energy efficiently. The schematic diagram, prototype, detailed structure, equivalent mechanical model, and field test photograph of the produced TENG are shown in Figure 1L.
2.2 KEH from human motion
The human body is an energy warehouse, and EH from human motion is explored to provide a promising embedded sustainable power supply for wearable devices (Verhelst et al., 2017; Cai et al., 2020b). The typical designs based on piezoelectric, electromagnetic, and triboelectric EH from human motion are briefly shown in Figures 2–4, respectively.
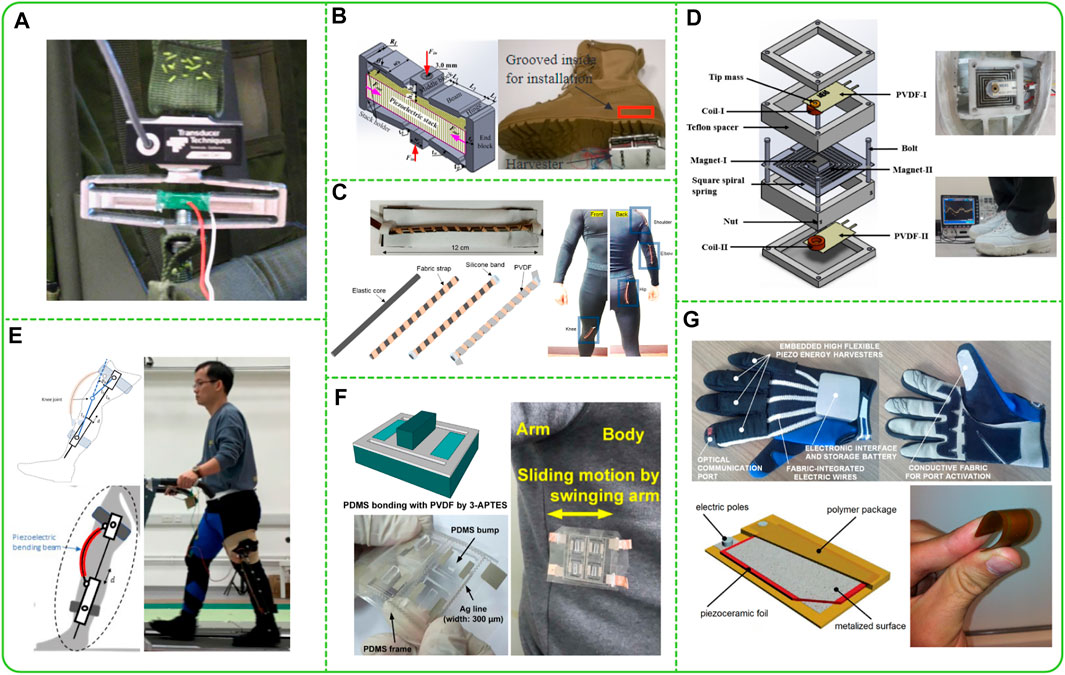
FIGURE 2. Piezoelectric EH from human motion. (A) Field test photograph of the energy harvesting backpack (Feenstra et al., 2008). (B) Force amplification frame and field test photograph of the proposed piezoelectric footwear harvester (Qian et al., 2018). (C) Fabrication process, prototype, and front and back views of HPEH (Iqbal et al., 2020). (D) Exploded view and Photographs of the HIEH incorporated into the sole of a commercial shoe, and the experiment setup (Kim and Yun, 2017). (E) Schematic diagram of the piezoelectric bending beambased energy harvester when being equipped on the human body and field test photograph (Kim et al., 2018). (F) Schematic diagram, fabricated flexible harvester array and implementation of the proposed harvester (Gao et al., 2022). (G) Prototype and piezoelectric material of GoldFinger (De Pasquale et al., 2016).
2.2.1 Piezoelectric-based KEH
To begin with, some piezoelectric designs are introduced as follows. The work in (Feenstra et al., 2008) has developed a novel energy harvesting backpack based on piezoelectric EH that can generate electrical energy from the differential forces between the wearer and the pack. The field test photograph of the energy harvesting backpack is shown in Figure 2A. In addition, the authors of (Qian et al., 2018) present the design, optimization, modeling, and testing of an embedded piezoelectric footwear harvester for energy scavenging from human walking. The force amplification frame and field test photograph are shown in Figure 2B. With the same target, the authors of (Iqbal et al., 2020) report a hybrid insole energy harvester (HIEH), capable of harvesting energy from low-frequency walking step motion to supply power to wearable sensors. The exploded view and photographs of the HIEH incorporated into the sole of a commercial shoe, and the experiment setup are shown in Figure 2D. Moreover, a helical piezoelectric energy harvester (HPEH) is proposed for energy harvesting garment (Kim and Yun, 2017), whose fabrication process, prototype, and front and back views are illustrated in Figure 2C. Coincidentally, the work in (Kim et al., 2018) proposes a flexible piezoelectric strain energy harvester responsive to multi-directional input forces produced by various human motions. The schematic diagram, fabricated flexible harvester array, and photograph of the proposed harvester are shown in Figure 2F. Toward EH from human keen motion, the authors in (Gao et al., 2022) develop a bimorph piezoelectric bending beam-based energy harvester for powering body-worn electronics. A schematic diagram of the proposed harvester when being equipped on the human body and field test photograph can be seen in Figure 2E. GoldFinger (De Pasquale et al., 2016), the human–machine interfacing HMI glove, integrates the advanced materials, miniaturization of components and electronics, and power generation through biomechanical energy harvesting. Photographs of the prototype and piezoelectric material of GoldFinger are shown in Figure 2G.
2.2.2 Electromagnetic-based KEH
Furthermore, some electromagnetic-based studies are shown below. Toward self-powered smart wristband, the authors in (Cai et al., 2020a) report an electromagnetic generator with a coaxial topology that efficiently captures the motion of arm swing to produce electricity. The design and exploded view of the proposed harvester are shown in Figure 3A. To further improve the high-power density of electromagnetic energy harvester, the work in (Cai and Liao, 2021) proposes an inertial energy harvester without additional proof mass to scavenge the kinetic energy of human limb swing efficiently. The architecture of the proposed inertial energy harvester is illustrated in Figure 3C. Targeted for harvesting energy from a shoe, an in-shoe electromagnetic harvester is developed for scavenging mechanical energy from human foot strike for conversion into electricity (Xie and Cai, 2015). The harvester model is depicted in Figure 3D. With a similar function, a type of multipolar linear permanent magnet generator (MLPMG) is developed (Gui et al., 2018). The photograph of MLPMG is shown in Figure 3B. Toward EH from human keen motion, a lightweight human knee energy harvester is designed, where a variable radius drum-cable mechanism with variable transmission ratio is employed (Chan et al., 2021). The components of the proposed harvester are shown in Figure 3E. In addition, the authors of (Delnavaz and Voix, 2014) creatively study the possibility of using energy harvesting from ear canal dynamic motion as a source of power.
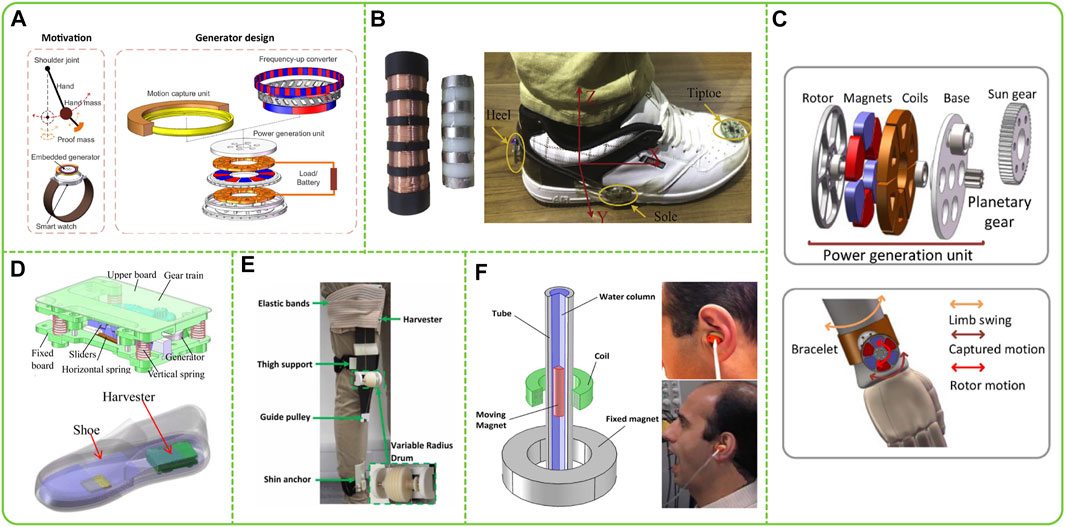
FIGURE 3. Electromagnetic EH from human motion. (A) Design and exploded view of the electromagnetic generator (Cai et al., 2020a). (B) Photograph of MLPMG (Cai and Liao, 2021). (C) Architecture of the inertial energy harvester (Xie and Cai, 2015). (D) 3-D model of the in-shoe energy harvester (Gui et al., 2018). (E) The components of the proposed human knee energy harvester (Chan et al., 2021). (F) Model and experimental setup of the in-ear energy harvester (Delnavaz and Voix, 2014).
2.2.3 Triboelectric-based KEH
Lastly, TENG-based research are reported underneath. The authors of (Tian et al., 2017) demonstrate a high-performance double-layer–stacked triboelectric textile (DTET) for harvesting human motion energy. The model and photograph of DTET are shown in Figure 4A. Toward harvesting energy from the wrist, a hybridized nanogenerator including a TENG and six electromagnetic generators that can effectively scavenge biomechanical energy is proposed for sustainably powering an electronic watch (Quan et al., 2015). The schematic diagram and photograph of the hybridized nanogenerator are illustrated in Figure 4B. In (Zhu et al., 2013), using the insole as a direct power source, the authors develop a fully packaged self-lighting shoe that has broad applications for display and entertainment purposes. The structural design and implementation of the in-shoe harvester are depicted in Figure 4C. In addition, the work in (Yang et al., 2013b) demonstrates a rationally designed TENG with integrated rhombic gridding, significantly improving the total current output. The sketch and photograph of the proposed self-powered backpack are shown in Figure 4D.
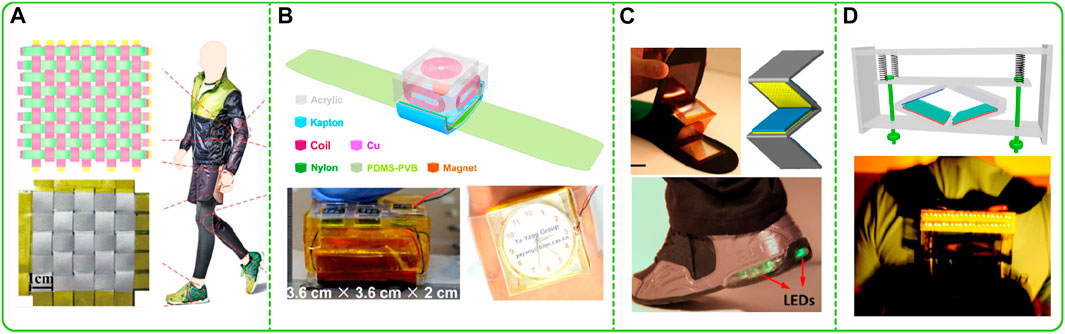
FIGURE 4. Triboelectric EH from human motion. (A) Model and photograph of DTET (Tian et al., 2017). (B) Schematic diagram and photograph of the hybridized nanogenerator (Quan et al., 2015). (C) Structural design and implementation of the in-shoe TENG (Zhu et al., 2013). (D) Sketch and photograph of the proposed self-powered backpack (Yang et al., 2013b).
2.3 Remarks
As previously stated, KEH from environmental vibration and human motion mainly comprises piezoelectric EH, electromagnetic EH, and triboelectric EH. Device size, layout, and application features dictate the most effective transduction mechanism. The comparison of these technologies is summarized in Table 1. For small-scale energy harvesters, the piezoelectric effect is widely used owing to the advantages of high power density, simple structure, and compact size. In addition, piezoelectric transducers have a relatively broader frequency operating range and better compatibility with micro-fabrication. For large-scale energy harvesters, the electromagnetic effect is generally used for the merits of high output current, easy to scale up, low-cost design, and robustness. Moreover, electromagnetic conversion is ideal for high-frequency conditions, and it usually needs a relatively large size, weight, and velocity to deliver a decent output power. TENG is suitable for harvesting irregular, randomly distributed, and wasted low-frequency mechanical energy. Furthermore, TENG has been applied to revive the vibration energy harvesting and self-powered sensing field, especially for low-frequency vibrations such as human motion, automobile, machine, and bridge vibrations (Chen and Wang, 2017). After further addressing the low output power issue, TENG would surely be more applied in real-life scenarios. Nowadays, hybrid energy harvester has attracted increasing interest since combining different technologies may enhance their merits and overcome shortfalls. However, a hybrid energy harvester should have a multiple-input power converter to obtain a regulated desired voltage (Harb, 2011).
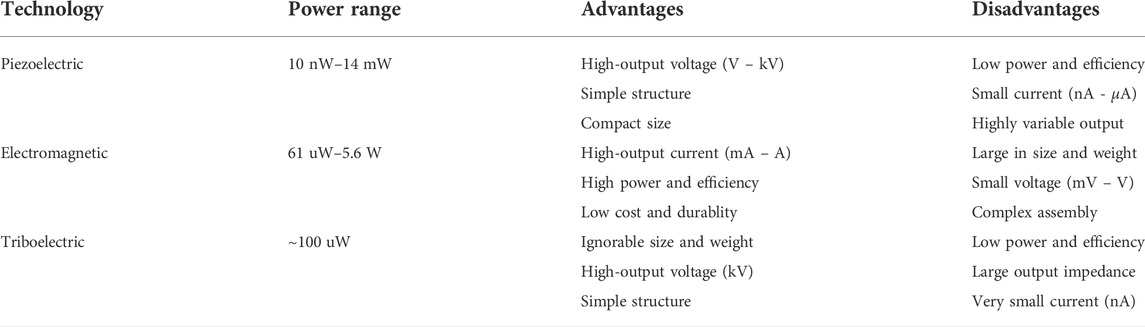
TABLE 1. Comparison of three KEH technologies (Liang et al., 2021; Liu et al., 2022).
Although the available ambient kinetic energy is usually tiny, harvesting energy from nearby sources makes onsite power generation more convenient. With the development of science and technology, KEH will facilitate more and more long-lasting, extensively-deployed, and maintenance-free applications, such as the self-powered sensors and IoT sensing systems to be introduced as follows.
3 Self-powered sensors
A sensor is a device to detect events or changes in the ambiance while transmitting information to other electronic devices, usually a computer processor. Sensors can be divided into two categories, active and passive. Active sensors are the common sensors on the market that require additional battery power, such as electronic temperature sensors, image sensors, accelerometers, gyroscopes, etc. Although the current active sensors are low-cost and low-power, the existence of batteries negatively impacts the lifespan and the performance of the entire network they are utilized in. Passive sensors are applied without a power supply, whose changes of characteristics directly reflect the changes in the external environment. Traditionally, the sensing capability is realized via two primary transducing mechanisms, resistive and capacitive. Some simple sensors intuitively reflect reality without complex mechanical structures, such as wind vane and mercurial thermometer.
In addition, KEH aims to convert kinetic power into electrical signals. On the contrary, after analyzing the acquired voltage and current signals, both static and dynamic processes of the mechanical excitation are also detected, which means the energy harvester can act as a sensor. Changes in the external environment are directly converted into corresponding self-generated electrical signals by such self-powered sensors (Dong et al., 2021). As KEH advances, KEH-based sensors have attracted tremendous interest from both academia and industry. State-of-the-art KEH-based sensors are based on piezoelectric, electromagnetic, and triboelectric energy harvesting. The recently developed self-powered sensing mechanisms lead to a novel method that uses the harvester as a power source as well as a sensor simultaneously. These sensors solve the source problem in sensing systems and represent a revolutionary convergence of renewable energy and industrial applications (Jing et al., 2014b; Guo et al., 2015a). They open up a variety of innovative sensing applications, which have been reviewed as follows.
3.1 TENG-based sensors and AI
The past decade has witnessed the remarkable progress of TENG as both power sources and self-powered sensors (Wang et al., 2014; Yang et al., 2014, 2015; Ma et al., 2016; Cao et al., 2022; Zhang et al., 2022).
Toward vibration amplitude measurement, the authors of (Wang et al., 2014) use the contact-mode freestanding triboelectric nanogenerator (CF-TENG) as a self-powered sensor for quantitative measurement of vibration amplitude. The triboelectric-charged resonator can be agitated to vibrate between two stacked stationary electrodes, thus CF-TENG can work as both a power source and motion-powered sensor that gives complete quantitative information about ambient vibration. The photographs showing the implementation of prototyped CF-TENG and the relation between the output voltage and vibration amplitude are shown in Figure 5A.
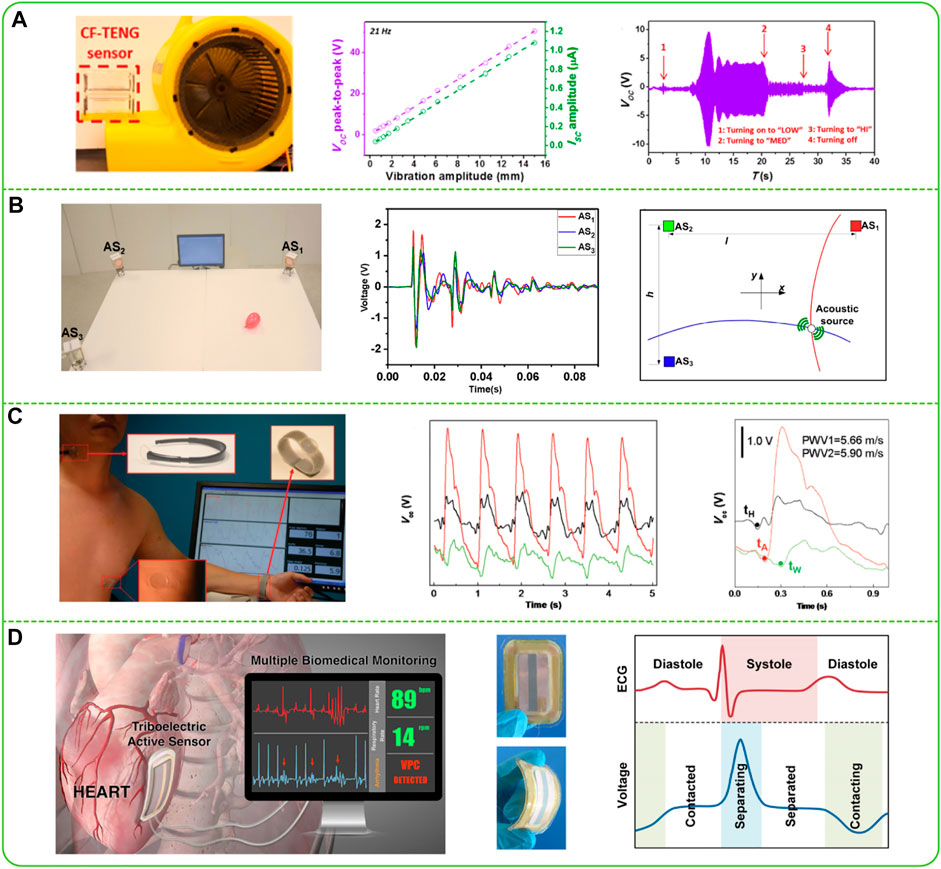
FIGURE 5. TENG-based self-powered sensors. (A) Photograph showing the demonstration of using the CF-TENG to monitor the vibration of a wind blower during its operation, the output voltage and current from the CF-TENG triggered by vibrations with different amplitudes, the output voltage from the CF-TENG when the wind blower was sequentially switched to different speeds (Wang et al., 2014). (B) Photograph and schematic illustration showing the working mechanism of nanogenerators for sound localization, acquired acoustic signals from the three nanogenerators when a balloon burst (Yang et al., 2014). (C) Implementation of BMS, the pulse waves acquired from three different sites of a participant: carotid artery, left wrist, and the chest, and an enlarged view of a cycle of output signals corresponding to three positions (Yang et al., 2015). (D) Schematic diagram and photograph of iTEAS and the schematic correspondence between iTEAS and different phases of heart motion (Ma et al., 2016).
Acoustic wave, as a clean and sustainable energy source, is a specific vibration form ubiquitously surrounding us. The authors of (Yang et al., 2014) develop a triboelectrification-based thin-film nanogenerator for harvesting acoustic energy from the ambient environment, which proves mobile, adaptable, and cost-efficient. The proposed nanogenerator acts as a motion-powered sensor for automatically detecting the location of an acoustic source with an error of less than 7 cm. The demonstration of the organic film nanogenerator acting as an active sensor for acoustic source localization is shown in Figure 5B.
Arterial pulse waves prove one of the most widely used essential signals for human healthcare monitoring and diagnosis. In (Yang et al., 2015), the first motion-powered bionic membrane sensor (BMS) is reported, which is wearable, lightweight, cost-effective, easy to fabricate, and capable of working in a multi-modal manner for either health monitoring or authentication purposes. The BMS can be utilized to measure rapidly changing pressure over a vast frequency range from 0.1 to 3.2 kHz for continuous and noninvasive monitoring. The demonstrations of the proposed bionic membrane sensor for measuring arterial pulse waves are shown in Figure 5C.
More than efficiently scavenging the mechanical energy of internal organs, the study in (Ma et al., 2016) proposes a self-powered, flexible, and one-stop implantable triboelectric active sensor (iTEAS) that can provide continuous monitoring of multiple physiological and pathological signs. As demonstrated in human-scale animals, the iTEAS can monitor heart rates, reaching an accuracy of 99%, which holds great potential for healthcare sensors without an external power supply. Figure 5D shows the schematic diagram and photograph of iTEAS and the schematic correspondence between iTEAS and different phases of heart motion.
The future of intelligent society will be all about sensor technology and AI algorithms (Zhang et al., 2017; Plageras et al., 2018; Syafrudin et al., 2018). With the rapid advance of AI technologies, more comprehensive and complicated information can be extracted and analyzed from different self-powered sensors, leading to more revolutionary applications (Wang C. et al., 2019; Li J. A. et al., 2019).
The authors of (Luo et al., 2019) report a flexible and durable wood-based triboelectric nanogenerator (W-TENG) for self-powered sensing in athletic big data analytics. A high-performance triboelectric material with excellent mechanical properties is designed from natural wood, whose output performance is enhanced by more than 70%. Moreover, a self-powered falling point distribution statistical system and an edge ball judgment system are further developed to provide real-time monitoring. The fabrication and schematic of the flexible wood-based TENG and smart ping-pong table and the schematic diagram of the W-TENG-based self-powered falling point distribution statistical system are illustrated in Figure 6A.
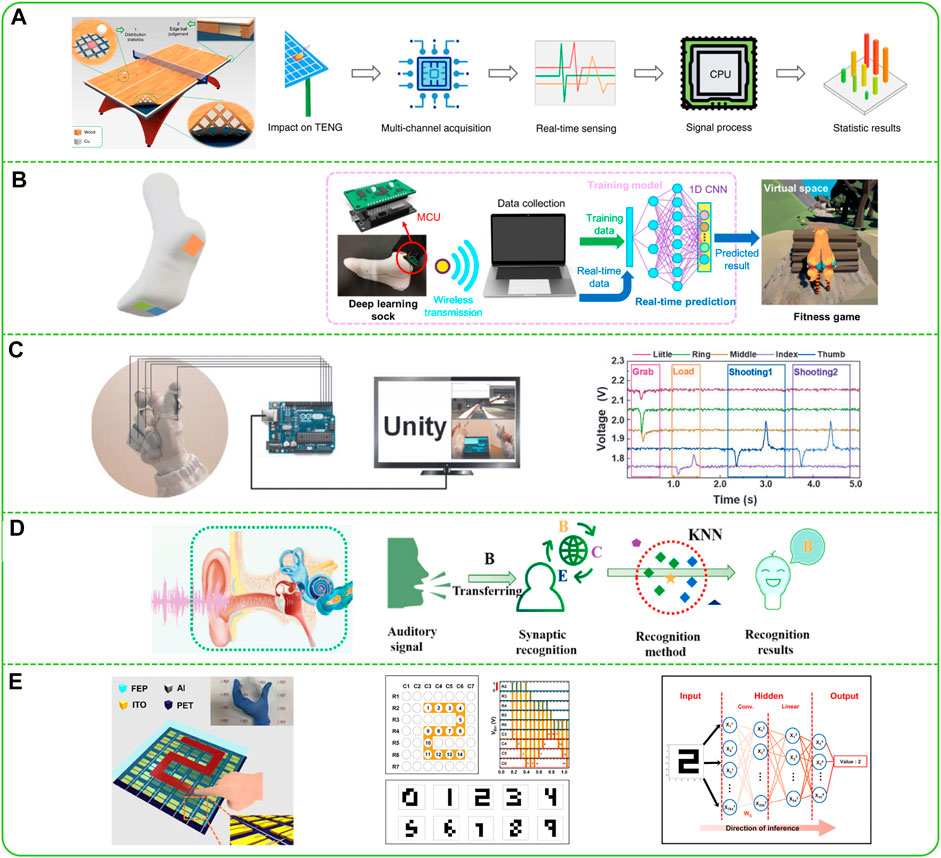
FIGURE 6. TENG-based self-powered sensors toward AI. (A) Fabrication and schematic of the flexible wood-based TENG and smart ping-pong table and the schematic diagram of the W-TENG based self-powered falling point distribution statistical system (Luo et al., 2019). (B) Schematic of the triple-sensor socks and the process flow from sensory information collection to the real-time prediction in VR fitness game (Zhang et al., 2020). (C) Schematic diagram of the control system and signal patterns of grabbing, loading gun, and shooting (Wen et al., 2020). (D) Schematic illustration of the human ear and the acoustic signal recognition process by the artificial auditory pathway (Liu et al., 2020). (E) Schematic illustration of the proposed touchpad, various hand-written number patterns with binarized data, and the interference process of hand-written number patterns with a pre-trained neural network (Yun et al., 2020).
Toward virtual intelligence, the work in (Zhang et al., 2020) develops low-cost triboelectric intelligent socks for harvesting waste energy from low-frequency body motions to transmit wireless sensory data. An optimized deep learning model with an end-to-end structure on the socks signals for the gait analysis is proposed. The physical signals collected through the socks in the virtual space are mapped to establish a digital human system for real-time monitoring. The schematic of the triple-sensor socks and the process flow from sensory information collection to the real-time prediction in the VR fitness game are shown in Figure 6B.
To recognize different human gestures, a facile carbon nanotubes/thermoplastic elastomer (CNTs/TPE) coating approach is investigated in detail to achieve superhydrophobicity of the triboelectric textile for performance improvement (Wen et al., 2020). The proposed glove using the superhydrophobic textile realizes a low-cost, self-powered interface for gesture recognition. By leveraging machine learning technologies, various gesture recognition tasks are done in real-time with high performance. The schematic diagram of the control system and signal patterns of grabbing, loading gun, and shooting are illustrated in Figure 6C.
Focusing on artificial simulation of the sensory nervous system, a TENG actuated self-powered artificial auditory pathway is proposed to emulate the biological auditory functionalities, demonstrating its application in intelligent neuromorphic computing and sound detection (Liu et al., 2020). A self-adaptation artificial neuromorphic circuit with noise-adjustable behavior is demonstrated to improve the efficiency and accuracy of the proposed system. The schematic illustration of the human ear and the acoustic signal recognition process by the artificial auditory pathway are shown in Figure 6D.
Human-machine interface (HMI) research uses various techniques to design and realize interfaces between users and machines, attracting tremendous interest from academia and industry. The study in (Yun et al., 2020) suggests the self-powered triboelectricity-based touchpad (TTP), which combines with artificial intelligence consisting of a TENG array (49 pixels) constructed on a thin, transparent, and flexible substrate, as well as this TTP works based on two major kinds of TENG mechanisms in tapping and sliding modes. With the remarkable applicability of the next-generation HMI in IoT, the TTP is implemented as a intelligent calculator using graphical user interface modeling. The schematic illustration of the proposed touchpad, various hand-written number patterns with binarized data, and the interference process of hand-written number patterns with a pre-trained neural network are illustrated in Figure 6E.
3.2 Electromagnetic and piezoelectric-based sensors and AI
Not just triboelectric-based sensors, piezoelectric and electromagnetic-based motion-powered sensors have a wide assortment of promising applications. The authors in (Khalifa et al., 2018) aim to quantify the potential of human activity recognition from kinetic energy harvesting (HARKE). The results show that HARKE achieves an accuracy of 80–95 percent, depending on the dataset and the placement of the device on the human body, saving 79 percent of the overall system power consumption. The proposed architecture, sensing signals, prototype, and data collection process of HARKE are shown in Figure 7A.
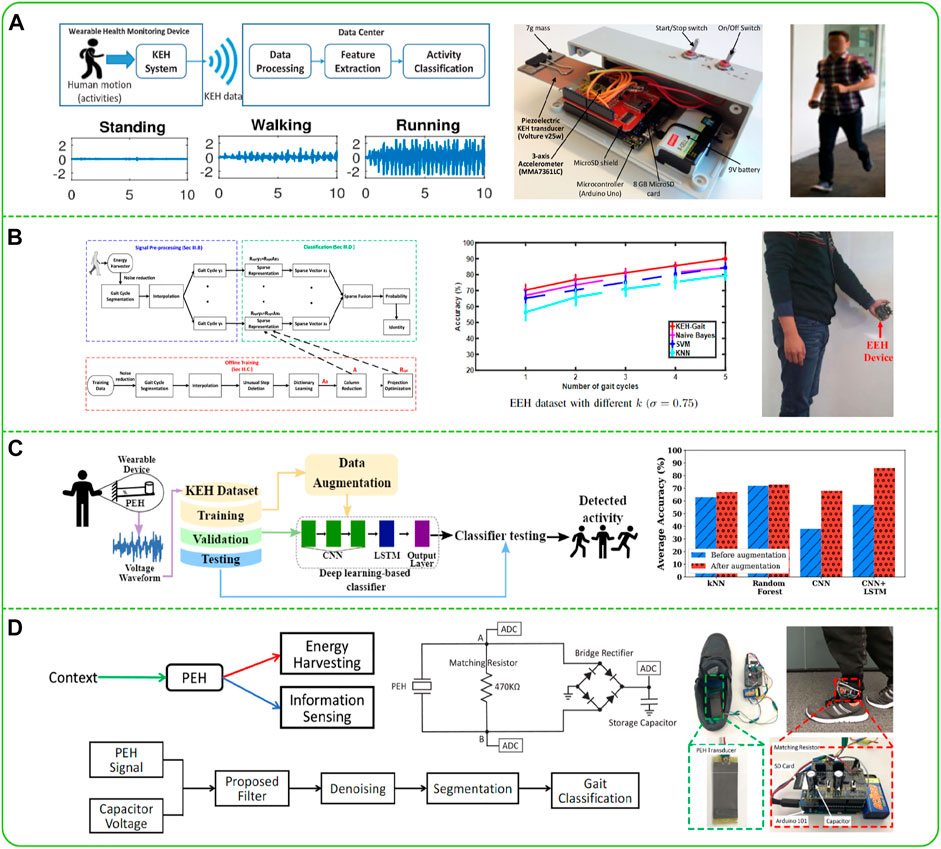
FIGURE 7. Piezoelectric and electromagnetic-based self-powered sensors. (A) Proposed architecture, sensing signals, prototype, and data collection process of HARKE (Khalifa et al., 2018). (B) System flowchart of KEH-Gait, Comparison with classification methods on EEH dataset, photograph of EEH (Xu et al., 2019). (C) Pipeline of the proposed HAR system and the average accuracy before and after applying data augmentation on the piezoelectric-based training set (Manjarrés et al., 2021). (D) Proposed gait-recognition system using SEHS and the prototype of SEHS (Ma et al., 2020).
Combining both electromagnetic energy harvester (EEH) and piezoelectric energy harvester (PEH), the study in (Xu et al., 2019) presents KEH-Gait, which advocates the use of output voltage signal from kinetic energy harvester (KEH) as the source for gait recognition. Compared to conventional accelerometer-based gait detection, KEH-Gait can reduce energy consumption by 78.15%. Moreover, the accuracy can be increased by the proposed Multi-Step Sparse Representation Classification (MSSRC). The system flowchart of KEH-Gait, Comparison with classification methods on EEH dataset, and photographs of EEH are shown in Figure 7B.
Focusing on deep learning, a deep learning architecture is presented to leverage the feature extraction capability of the convolutional neural networks and the construction of the temporal sequences of recurrent neural networks to improve existing classification results (Manjarrés et al., 2021). The proposed architecture outperforms existing approaches of kinetic harvesting-based human activity recognition by 13% of accuracy when the training data is augmented with the proposed methods. The pipeline of the proposed HAR system and the average accuracy before and after applying data augmentation on the piezoelectric-based training set are shown in Figure 7C.
Beyond treating the energy harvester as a power source or a sensor, the authors of (Ma et al., 2020) leverage the potential for an energy harvester to harvest energy and sense simultaneously, i.e., simultaneously energy harvesting and sensing (SEHS). A preprocessing algorithm to filter out the effect of energy storage on piezoelectric signal and long short-term memory (LSTM) network-based classifier is designed. The results show that the proposed architecture detects human gait with 12% higher recall and harvests up to 127% more energy while consuming 38% less power than the state-of-the-art. The proposed gait-recognition system using SEHS and the prototype of SEHS are shown in Figure 7D.
3.3 Remarks
A transducer is a device transforming energy from one form to another, which provides output response to specific input measured. Most transducers are now utilized to convert various forms of energy into electrical energy. Sensors measure and convert the physical quantities into readable signals for an observer or instrument. These two are closely related.
The flow of energy is accompanied by information, and the transmission of information is caused by energy. Therefore, after deriving the relationship between environmental information and energy in specific applications, transducers can all be regarded as sensors. The KEH-based sensors mentioned above are essentially transducers based on different EH mechanisms. And from Table 1, we can see this sensing mode is truly feasible. In different applications, we exploit the known relationship between the energy generated by the environmental vibration or human activity and the information they reflect, thus deducing information directly from energy without the need for additional active sensors, making the sensing process more energy-efficient. We are optimistic that sensors based on KEH, whether piezoelectric, electromagnetic, or triboelectric EH, will be increasingly available in the future and further promote the development of multiple applications.
Yet, the information needed to be transferred and analyzed, or it would be meaningless. Although the self-powered sensor can effectively generate electrical energy from the ambiance, the power proves insufficient for the whole system. In the system of signal recognition, only the part of the signal generation is passive while all other parts are active. In other words, the acquisition and analysis of produced signals are based on battery-powered devices, such as microcontrollers and processors. For a genuinely self-sustaining and maintenance-free sensing system, there are still many challenges to address. The KEH-based battery-free IoT sensing systems introduced below further fulfill the concept of integrated sensing systems by solving the problem of self-powering the generation and transmission of information.
4 KEH-based battery-free IoT sensing systems
Beyond using the ambient kinetic energy to power the load for scientific research, some recent studies focus on utilizing the converted electrical energy to power a whole IoT system (Bonisoli et al., 2017; Zhao C. et al., 2019; Mao et al., 2021), which generally comprises the energy harvester, the energy management circuit, sensors, and the BLE-based SOC. Moreover, to further reduce the power consumption and promote the system performance, some IoT co-designs using self-powered sensors, i.e., treating the harvester as a sensor as well as a power source simultaneously, have been proposed (Li et al., 2021b; Zhang et al., 2021; Hailiang Yang, 2022). Sensing is just the beginning. How to transmit and identify edge information in real-time is the focus of many applications in our lives. The KEH-IoT architecture is introduced first, then the most advanced designs of sensor-based as well as sensor-free self-powered IoT systems are reviewed in detail to thoroughly demonstrate the latest research status and necessity of KEH-based synergies.
A KEH-based IoT system must be self-contained and energy-autonomous. It has to be sustainable against environmental uncertainties while executing the sensing, computing, and communication functions correctly and timely according to the information demand (Liang et al., 2021). The block diagram of a KEH-IoT system is illustrated in Figure 8. Mechanical, electrical, and cyber parts are three critical domains for an all-around KEH-IoT system. In general, the ambient kinetic energy is scavenged and converted into electrical energy by the mechanical part, then the alternating and intermittent energy is stored and managed by the electrical part. A well-rounded energy-aware circuit, incorporating rectification, energy storage, and voltage regulation functions, is usually chosen as an energy management unit to provide a stable and reliable voltage level for powering digital electronics (Li Teng, 2022). For the cyber part, low-power hardware, programming, and wireless communication protocol are significant developing directions. Table 2 lists the low-power devices that are capable of being powered by energy harvesters. Wifi, ZigBee, and Bluetooth low-energy (BLE) are three mainstream wireless transmission technologies for low-power IoT devices, as listed in Table 3. BLE proves the relatively most energy-efficient transmission method for achieving remote and real-time condition monitoring by evaluating data rate and power consumption (Ali et al., 2017; Tang et al., 2018). From a comprehensive perspective, balancing supply and demand by matching the required energy from the IoT part with the supplied energy from the electromechanical part is critical for battery-free IoT systems.
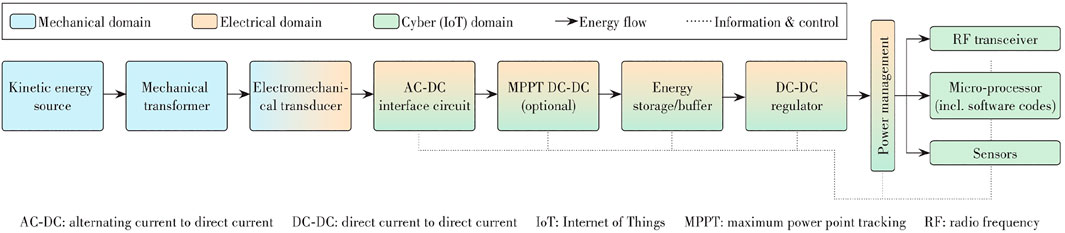
FIGURE 8. Block diagram of a kinetic energy harvesting (KEH)-IoT system (Liang et al., 2021).
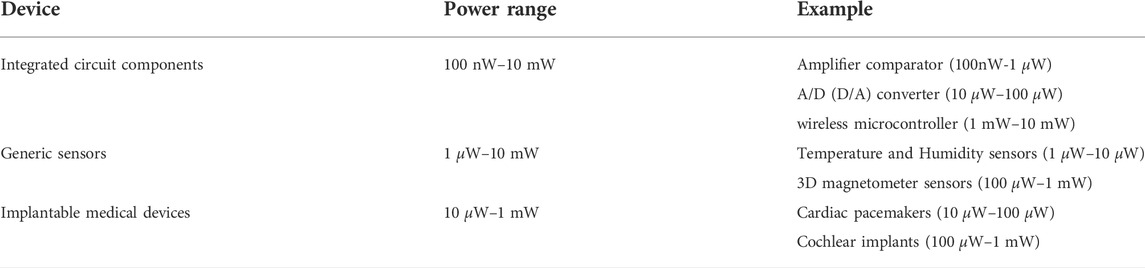
TABLE 2. Power consumption of low-power devices (Proto et al., 2017; Liu et al., 2022).

TABLE 3. Comparison of traditional low-power wireless transmission technologies (Tang et al., 2018).
4.1 Sensor-based self-powered IoT systems
To begin with, some traditional sensor based IoT systems are introduced as follows. Toward supplying a Bluetooth step-counter placed in the sole of a training shoe, a complete system is presented (Bonisoli et al., 2017). To better validate the feasibility, a compact prototype containing the transducer, the electronic interface, the step-counter electronics, and the protective shell is developed. The experimental results show the mean energy recovery over a complete run is 644 μJ/footstep, which means the energy generated at each footstep is larger than the required and allows the step-counter wirelessly to transmit the information. The self-supplied step-counter’s layout, implementation, and system architecture are shown in Figure 9A. Coincidently, a self-powered portable TENG was developed (Mao et al., 2021), which is used in human motion energy collection and monitoring mobile gait. The presented prototype uses a low-frequency stabilized voltage output system to provide power for the wearable miniature electronic device while stabilizing the voltage output. The schematic diagram, working modules, and implementation of the proposed portable mobile gait monitor system can be seen in Figure 9B. In addition, the authors in (Zhao et al., 2019a) present a hybrid piezo/triboelectric nanogenerator (H-P/TENG) for highly efficient mechanical rotation-energy harvesting. By integrating an energy managing circuit with the H-P/TENG, they develop a DC power source to sustainably drive RF wireless temperature sensing networks and commercial electronics. The schematic illustration, circuit diagram, and experimental setup of the H-P/TENG are depicted in Figure 9C.
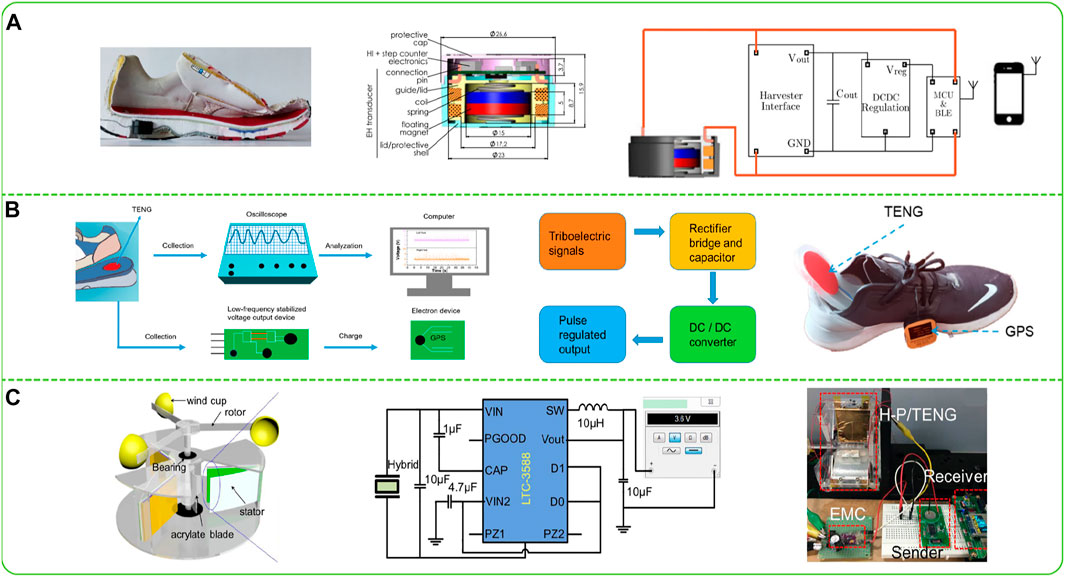
FIGURE 9. Traditional sensor based self-powered IoT systems. (A) Layout, implementation, and system architecture of the self-supplied step-counter (Bonisoli et al., 2017). (B) Schematic diagram, working modules, and implementation of the proposed portable mobile gait monitor system (Mao et al., 2021). (C) Schematic illustration, circuit diagram, and experimental setup of the H-P/TENG (Zhao C. et al., 2019).
4.2 Sensor-free self-powered IoT systems
As a cyber-electro-mechanical co-design, leveraging the energy harvester as a power source and a sensor has recently attracted increasing interest. Toward smart agriculture, the study in (Zhang et al., 2021) proposes a method based on triboelectric nanogenerators and electromagnetic generators to realize self-powered IoT nodes and self-powered sensors simultaneously. An electromagnetic-triboelectric hybrid generator (ES-ETHG) is designed to measure wind direction and speed. Moreover, the proposed motion-powered IoT sensing system based on ES-ETHG can realize the remote collection of wind speed and direction. The schematic configuration of ES-ETHG, conceptual diagram, and systematic structure of the proposed system are shown in Figure 10A. Moreover, in (Li et al., 2021b), a robust design of the transient-motion-powered motion detector is designed, called ViPSN-pluck. ViPSN-pluck can efficiently harvest energy from a transient motion, then effectively carry out motion detection and BLE wireless communication without an active sensor. By skillfully correlating the motion energy generation and motion direction detection and making a cyber-electromechanical co-design, ViPSN-pluck introduces a novel motion-powered IoT sensing architecture. The architecture and performance of ViPSN-pluck are illustrated in Figure 10B. Furthermore, a battery-free pavement roughness estimation system (BF-PRES) is proposed (Hailiang Yang, 2022), which provides road roughness information by linking the driving vibration and wireless packet count. Instead of using a commercial accelerometer, BF-PRES simply sends out a BLE Beacon packet when the energy is sufficient. The theoretical and experimental results demonstrate the proportional relation between packets’ transmission intervals and harmonic base excitation conditions, which is shown in Figure 10C and can be formulated as follows:
where th is the charging interval, X is the relative beam displacement (deflection), Cp is the piezoelectric clamped capacitance, Csto is the storage capacitor, Von and Voff are the threshold voltages, η is the constant conversion efficient, f is the vibration frequency, and αe is the voltage-to-force coupling factor. The experimental setup and results of BF-PRES are shown in Figure 10C.
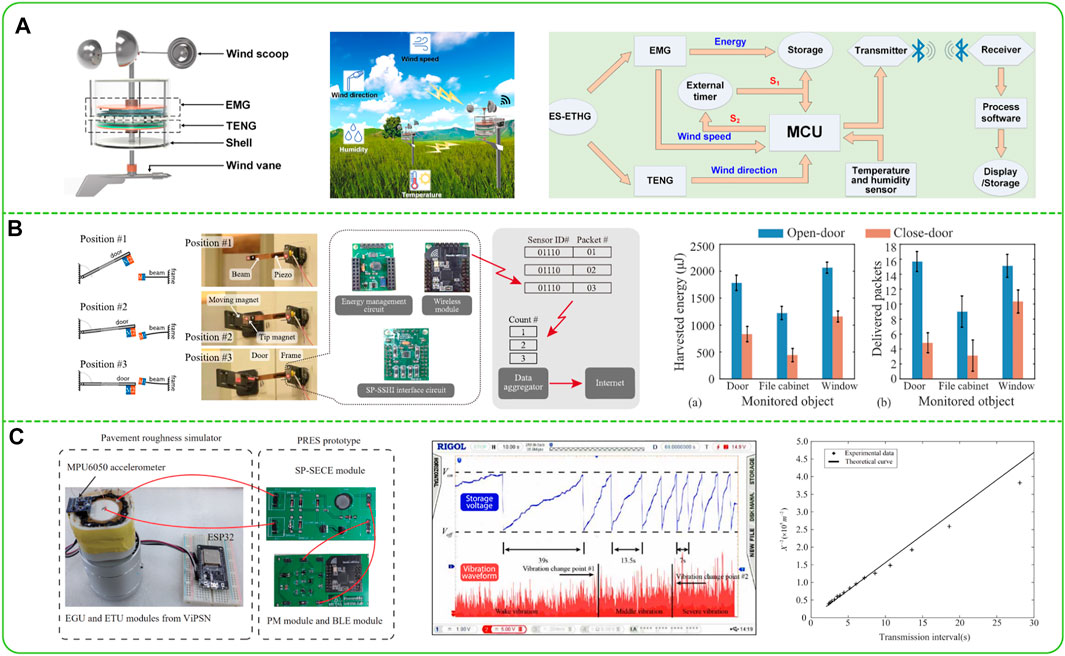
FIGURE 10. Self-powered sensor based battery-free IoT systems. (A) Schematic configuration of ES-ETHG, conceptual diagram, and systematic structure of the proposed system (Zhang et al., 2021). (B) Architecture and performance of ViPSN-pluck (Li et al., 2021b). (C) Experimental setup and results of BF-PRES (Hailiang Yang, 2022).
4.3 Remarks
The aforementioned studies focus on integrating the mechanical, electrical, and cyber parts from a global perspective, i.e., making a KEH-based IoT sensing system. Tables 1–3 prove the feasibility of exploiting energy harvesting technologies to self-sustain low-power IoT devices. After well-designed low-power-oriented programming, i.e., removal of redundant code, the power consumption of IoT devices sending signals can be further reduced, thus making the systems more reliable. Table 4 lists the energy consumption of one BLE beacon packet transmission after dedicated programming, from which we can see the energy cost is ultra-low. The low power consumption of one round of sensing, computing, and transmitting prove crucial for any battery-free IoT device working on an intermittent mode.
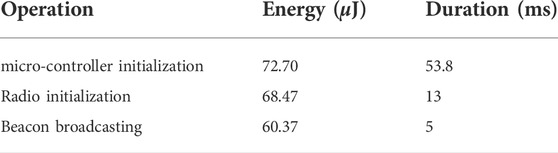
TABLE 4. Energy statistics of one BLE beacon packet broadcasting (Li et al., 2021b).
Beyond combining energy management circuitry with a low-cost SOC and low-power wireless transmission protocol, some battery-IoT systems utilize self-powered sensors and the relation between energy and information flow. They further fulfill the concept of pervasive sensing and ubiquitous IoT and facilitate various intelligent applications, such as smart cities and wearable devices for healthcare and environmental monitoring. As energy harvesting technology improves and low-power IoT technology advances, we remain highly optimistic about the future of more and broader IoT applications based on KEH, whether piezoelectric, electromagnetic, or triboelectric EH, and even self-powered intelligent devices toward ubiquitous AI based on TinyML (Tiny Machine Learning).
Yet, since the signal generation, sampling, and transmission of a self-powered system are all passive, the sampling frequency for the sensor signal is significantly reduced. The sensing, computing, and communication tasks carried out by a KEH-IoT system must take sufficient and comprehensive consideration of the fluctuating feature of most kinetic energy sources, the energy-aware circuit design, the scarce power output capability, and the ultralow-power wireless transmission. In fact, the relationship between the output power of the KEHs and the power consumption of the IoT sensing systems has not been clearly discussed by now. Utilizing the known energy consumption of different IoT applications to reversely design the electromechanical part, realizing the balance between supply and demand, proves significant for battery-free systems.
In a word, exploring the co-design among the mechanical, electrical, and cyber parts is necessary for such an interdisciplinary and holistic co-design (Liang et al., 2021). In the future, it is expected that more related comprehensive studies can be conducted to further promote the development of battery-free IoT and pervasive sensing related academic research and industrial applications.
5 Conclusion
This paper provided a comprehensive review of the state-of-the-art progress on KEH-based sensors and IoT sensing systems. These two research directions are relatively cutting-edge in the EH field and have been drawing substantial and increasing attention from both academia and industry over the past decade. As KEH advances, piezoelectric, electromagnetic, and triboelectric-based EH methods evolve along with diversification and maturation. Combining hybrid energy harvesting technologies into one system also proves a thriving direction to overcome the limitations of different EH mechanisms. KEH can be seen as one of the promising technology to achieve a self-sustaining and maintenance-free sensing system. Self-powered sensors based on KEH mechanisms have great potential to be widely integrated with current leading applications, especially human motion recognition, environmental monitoring, and AIoT. From a global perspective, a KEH-based self-powered IoT sensing system incorporating mechanical, electrical, and cyber parts is urgent, whether it is traditional sensor based or self-powered sensor based. Given the preceding discussion, we believe that more endeavors on cross-domain knowledge and cyber-electro-mechanical synergy are required and highly desired to advance pervasive sensing and ubiquitous IoT further.
Author contributions
All authors contributed to the idea and structure of the review. ZC carried out the literature search and drafted the manuscript. FG and JL contributed to manuscript revision, read, and approved the submitted version.
Funding
This work was supported in part by the Natural Science Foundation of Shanghai under Grant 21ZR1442300; in part by the National Natural Science Foundation of China under Grant U21B2002; in part by the General project of Natural Science Foundation of Guangdong Province (Project No.: 2022A1515011467).
Conflict of interest
The authors declare that the research was conducted in the absence of any commercial or financial relationships that could be construed as a potential conflict of interest.
Publisher’s note
All claims expressed in this article are solely those of the authors and do not necessarily represent those of their affiliated organizations, or those of the publisher, the editors and the reviewers. Any product that may be evaluated in this article, or claim that may be made by its manufacturer, is not guaranteed or endorsed by the publisher.
References
Abdelkareem, M. A., Xu, L., Ali, M. K. A., Elagouz, A., Mi, J., Guo, S., et al. (2018). Vibration energy harvesting in automotive suspension system: A detailed review. Appl. energy 229, 672–699. doi:10.1016/j.apenergy.2018.08.030
Ali, A., Shah, G. A., Farooq, M. O., and Ghani, U. (2017). Technologies and challenges in developing machine-to-machine applications: A survey. J. Netw. Comput. Appl. 83, 124–139. doi:10.1016/j.jnca.2017.02.002
Asadi, E., Askari, H., Behrad Khamesee, M., and Khajepour, A. (2017). High frequency nano electromagnetic self-powered sensor: Concept, modelling and analysis. Measurement 107, 31–40. doi:10.1016/j.measurement.2017.04.019
Askari, H., Saadatnia, Z., Asadi, E., Khajepour, A., and Zu, J. (2018). A flexible hybridized electromagnetic-triboelectric multi-purpose self-powered sensor. Nano Energy 45, 319–329. doi:10.1016/j.nanoen.2018.01.011
Atzori, L., Iera, A., and Morabito, G. (2010). The internet of things: A survey. Comput. Netw. 54, 2787–2805. doi:10.1016/j.comnet.2010.05.010
Bae, J., Lee, J., Kim, S., Ha, J., Lee, B.-S., Park, Y., et al. (2014). Flutter-driven triboelectrification for harvesting wind energy. Nat. Commun. 5, 4929–9. doi:10.1038/ncomms5929
Bai, P., Zhu, G., Jing, Q., Yang, J., Chen, J., Su, Y., et al. (2014). Membrane-based self-powered triboelectric sensors for pressure change detection and its uses in security surveillance and healthcare monitoring. Adv. Funct. Mat. 24, 5807–5813. doi:10.1002/adfm.201401267
Bai, P., Zhu, G., Lin, Z.-H., Jing, Q., Chen, J., Zhang, G., et al. (2013). Integrated multilayered triboelectric nanogenerator for harvesting biomechanical energy from human motions. ACS Nano 7, 3713–3719. doi:10.1021/nn4007708
Bai, Y., Tofel, P., Hadas, Z., Smilek, J., Losak, P., Skarvada, P., et al. (2018). Investigation of a cantilever structured piezoelectric energy harvester used for wearable devices with random vibration input. Mech. Syst. Signal Process. 106, 303–318. doi:10.1016/j.ymssp.2018.01.006
Beeby, S. P., Torah, R. N., Tudor, M. J., Glynne-Jones, P., O’Donnell, T., Saha, C. R., et al. (2007). A micro electromagnetic generator for vibration energy harvesting. J. Micromech. Microeng. 17, 1257–1265. doi:10.1088/0960-1317/17/7/007
Bonisoli, E., Repetto, M., Manca, N., and Gasparini, A. (2017). Electromechanical and electronic integrated harvester for shoes application. IEEE/ASME Trans. Mechatron. 22, 1921–1932. doi:10.1109/tmech.2017.2667401
Bowers, B. J., and Arnold, D. P. (2009). Spherical, rolling magnet generators for passive energy harvesting from human motion. J. Micromech. Microeng. 19, 094008. doi:10.1088/0960-1317/19/9/094008
Bradai, S., Naifar, S., Viehweger, C., and Kanoun, O. (2018). Electromagnetic vibration energy harvesting for railway applications. MATEC Web Conf. 148, 12004. doi:10.1051/matecconf/201814812004
Cai, M., Liao, W.-H., and Cao, J. (2019). A smart harvester for capturing energy from human ankle dorsiflexion with reduced user effort. Smart Mat. Struct. 28, 015026. doi:10.1088/1361-665x/aaed66
Cai, M., and Liao, W.-H. (2021). High-Power density inertial energy harvester without additional proof mass for wearables. IEEE Internet Things J. 8, 297–308. doi:10.1109/jiot.2020.3003262
Cai, M., Wang, J., and Liao, W.-H. (2020a). Self-powered smart watch and wristband enabled by embedded generator. Appl. Energy 263, 114682. doi:10.1016/j.apenergy.2020.114682
Cai, M., Yang, Z., Cao, J., and Liao, W.-H. (2020b). Recent advances in human motion excited energy harvesting systems for wearables. Energy Technol. 8, 2000533. doi:10.1002/ente.202000533
Cao, Y., Yang, Y., Qu, X., Shi, B., Xu, L., Xue, J., et al. (2022). A self-powered triboelectric hybrid coder for human–machine interaction. Small Methods 6, 2101529. doi:10.1002/smtd.202101529
Chan, H. H.-T., Gao, F., Chung, B. L.-H., Liao, W.-H., and Cao, J. (2021). Knee energy harvester with variable transmission to reduce the effect on the walking gait. Smart Mat. Struct. 30, 085024. doi:10.1088/1361-665x/ac0bfe
Chen, F., He, M., Wang, S., Zhong, X., and Guan, M. (2019). Study of an inertial piezoelectric energy harvester from a backpack. Ferroelectrics 550, 233–243. doi:10.1080/00150193.2019.1652513
Chen, J., Huang, Y., Zhang, N., Zou, H., Liu, R., Tao, C., et al. (2016). Micro-cable structured textile for simultaneously harvesting solar and mechanical energy. Nat. Energy 1, 16138–8. doi:10.1038/nenergy.2016.138
Chen, J., and Wang, Z. L. (2017). Reviving vibration energy harvesting and self-powered sensing by a triboelectric nanogenerator. Joule 1, 480–521. doi:10.1016/j.joule.2017.09.004
Chen, X., Miao, L., Guo, H., Chen, H., Song, Y., Su, Z., et al. (2018). Waterproof and stretchable triboelectric nanogenerator for biomechanical energy harvesting and self-powered sensing. Appl. Phys. Lett. 112, 203902. doi:10.1063/1.5028478
Chen, X., Song, Y., Chen, H., Zhang, J., and Zhang, H. (2017). An ultrathin stretchable triboelectric nanogenerator with coplanar electrode for energy harvesting and gesture sensing. J. Mat. Chem. A Mat. 5, 12361–12368. doi:10.1039/c7ta03092d
Chu, S., Cui, Y., and Liu, N. (2016). The path towards sustainable energy. Nat. Mat. 16, 16–22. doi:10.1038/nmat4834
Chu, S., and Majumdar, A. (2012). Opportunities and challenges for a sustainable energy future. Nature 488, 294–303. doi:10.1038/nature11475
De Pasquale, G., Kim, S.-G., and De Pasquale, D. (2016). GoldFinger: Wireless Human–Machine interface with dedicated software and biomechanical energy harvesting system. IEEE/ASME Trans. Mechatron. 21, 565–575.
De Pasquale, G., and Somà, A. (2013). “Energy harvesting from human motion with piezo fibers for the body monitoring by mems sensors,” in 2013 Symposium on Design, Test, Integration and Packaging of MEMS/MOEMS (DTIP) (Barcelona, Spain: IEEE), 1–6.
Delnavaz, A., and Voix, J. (2014). Energy harvesting for In-Ear devices using ear canal dynamic motion. IEEE Trans. Ind. Electron. 61, 583–590. doi:10.1109/tie.2013.2242656
Deng, H., Wang, Z., Du, Y., Zhang, J., Ma, M., and Zhong, X. (2019). A compact and flexible Nonbeam-Type vibrational energy harvesting device with bistable characteristics. IEEE/ASME Trans. Mechatron. 24, 282–292. doi:10.1109/tmech.2019.2891289
Dong, B., Shi, Q., Yang, Y., Wen, F., Zhang, Z., and Lee, C. (2021). Technology evolution from self-powered sensors to aiot enabled smart homes. Nano Energy 79, 105414. doi:10.1016/j.nanoen.2020.105414
Eun, Y., Kwon, D.-S., Kim, M.-O., Yoo, I., Sim, J., Ko, H.-J., et al. (2014). A flexible hybrid strain energy harvester using piezoelectric and electrostatic conversion. Smart Mat. Struct. 23, 045040. doi:10.1088/0964-1726/23/4/045040
Fan, F.-R., Tian, Z.-Q., and Wang, Z. L. (2012). Flexible triboelectric generator. Nano energy 1, 328–334. doi:10.1016/j.nanoen.2012.01.004
Fan, K., Zhang, Y., Liu, H., Cai, M., and Tan, Q. (2019). A nonlinear two-degree-of-freedom electromagnetic energy harvester for ultra-low frequency vibrations and human body motions. Renew. Energy 138, 292–302. doi:10.1016/j.renene.2019.01.105
Fan, X., Chen, J., Yang, J., Bai, P., Li, Z., and Wang, Z. L. (2015). Ultrathin, rollable, paper-based triboelectric nanogenerator for acoustic energy harvesting and self-powered sound recording. ACS Nano 9, 4236–4243. doi:10.1021/acsnano.5b00618
Feenstra, J., Granstrom, J., and Sodano, H. (2008). Energy harvesting through a backpack employing a mechanically amplified piezoelectric stack. Mech. Syst. Signal Process. 22, 721–734. doi:10.1016/j.ymssp.2007.09.015
Fu, H., Mei, X., Yurchenko, D., Zhou, S., Theodossiades, S., Nakano, K., et al. (2021). Rotational energy harvesting for self-powered sensing. Joule 5, 1074–1118. doi:10.1016/j.joule.2021.03.006
Gao, F., Liu, G., Chung, B. L.-H., Chan, H. H.-T., and Liao, W.-H. (2019). Macro fiber composite-based energy harvester for human knee. Appl. Phys. Lett. 115, 033901. doi:10.1063/1.5098962
Gao, F., Liu, G., Fu, X., Li, L., and Liao, W.-H. (2022). Lightweight piezoelectric bending Beam-Based energy harvester for capturing energy from human knee motion. IEEE/ASME Trans. Mechatron. 27, 1256–1266. doi:10.1109/tmech.2021.3098719
Gui, P., Deng, F., Liang, Z., Cai, Y., and Chen, J. (2018). Micro linear generator for harvesting mechanical energy from the human gait. Energy 154, 365–373. doi:10.1016/j.energy.2018.04.123
Guo, H., Chen, J., Leng, Q., Xi, Y., Wang, M., He, X., et al. (2015a). Spiral-interdigital-electrode-based multifunctional device: Dual-functional triboelectric generator and dual-functional self-powered sensor. Nano Energy 12, 626–635. doi:10.1016/j.nanoen.2014.09.021
Guo, H., Leng, Q., He, X., Wang, M., Chen, J., Hu, C., et al. (2015b). A triboelectric generator based on checker-like interdigital electrodes with a sandwiched pet thin film for harvesting sliding energy in all directions. Adv. Energy Mat. 5, 1400790. doi:10.1002/aenm.201400790
Hailiang Yang, J. L., and Teng, Li (2022). “A battery-free pavement roughness estimation system based on kinetic energy harvesting,” in Proceedings of the 2022 IEEE International Symposium on Circuits and Systems.
Harb, A. (2011). Energy harvesting: State-of-the-art. Renew. Energy 36, 2641–2654. doi:10.1016/j.renene.2010.06.014
Haroun, A., Yamada, I., and Warisawa, S. (2015). Study of electromagnetic vibration energy harvesting with free/impact motion for low frequency operation. J. Sound Vib. 349, 389–402. doi:10.1016/j.jsv.2015.03.048
Hester, J., and Sorber, J. (2017). “The future of sensing is batteryless, intermittent, and awesome,” in Proceedings of the 15th ACM Conference on.
Hou, T.-C., Yang, Y., Zhang, H., Chen, J., Chen, L.-J., and Lin Wang, Z. (2013). Triboelectric nanogenerator built inside shoe insole for harvesting walking energy. Nano Energy 2, 856–862. doi:10.1016/j.nanoen.2013.03.001
Hu, Y., Yang, J., Jing, Q., Niu, S., Wu, W., and Wang, Z. L. (2013). Triboelectric nanogenerator built on suspended 3D spiral structure as vibration and positioning sensor and wave energy harvester. ACS Nano 7, 10424–10432. doi:10.1021/nn405209u
Iqbal, M., Nauman, M. M., Khan, F. U., Abas, P. E., Cheok, Q., Iqbal, A., et al. (2020). Multimodal hybrid Piezoelectric-Electromagnetic insole energy harvester using PVDF generators. Electronics 9, 635. doi:10.3390/electronics9040635
Jiang, D., Ouyang, H., Shi, B., Zou, Y., Tan, P., Qu, X., et al. (2020). A wearable noncontact free-rotating hybrid nanogenerator for self-powered electronics. InfoMat 2, 1191–1200. doi:10.1002/inf2.12103
Jing, Q., Zhu, G., Bai, P., Xie, Y., Chen, J., Han, R. P., et al. (2014a). Case-encapsulated triboelectric nanogenerator for harvesting energy from reciprocating sliding motion. ACS Nano 8, 3836–3842. doi:10.1021/nn500694y
Jing, Q., Zhu, G., Wu, W., Bai, P., Xie, Y., Han, R. P. S., et al. (2014b). Self-powered triboelectric velocity sensor for dual-mode sensing of rectified linear and rotary motions. Nano Energy 10, 305–312. doi:10.1016/j.nanoen.2014.09.018
Jo, J., and Park, H. (2021). “Rfinsole: Batteryless gait-monitoring smart insole based on passive rfid tags,” in 2021 International Symposium on Wearable Computers, 141–143.
Katzir, S. (2006). “The discovery of the piezoelectric effect,” in The beginnings of piezoelectricity (Berlin, Germany: Springer), 15–64.
Khalifa, S., Hassan, M., Seneviratne, A., and Das, S. K. (2015b). Energy-Harvesting wearables for Activity-Aware services. IEEE Internet Comput. 19, 8–16. doi:10.1109/mic.2015.115
Khalifa, S., Hassan, M., and Seneviratne, A. (2015a). “Pervasive self-powered human activity recognition without the accelerometer,” in 2015 IEEE International Conference on Pervasive Computing and Communications (PerCom) (St. Louis, MO, USA: IEEE), 79–86.
Khalifa, S., Lan, G., Hassan, M., Seneviratne, A., and Das, S. K. (2018). Harke: Human activity recognition from kinetic energy harvesting data in wearable devices. IEEE Trans. Mob. Comput. 17, 1353–1368. doi:10.1109/tmc.2017.2761744
Khameneifar, F., Arzanpour, S., and Moallem, M. (2012). A piezoelectric energy harvester for rotary motion applications: Design and experiments. Ieee. ASME. Trans. Mechatron. 18, 1527–1534. doi:10.1109/tmech.2012.2205266
Khan, M. B., Kim, D. H., Han, J. H., Saif, H., Lee, H., Lee, Y., et al. (2019). Performance improvement of flexible piezoelectric energy harvester for irregular human motion with energy extraction enhancement circuit. Nano Energy 58, 211–219. doi:10.1016/j.nanoen.2019.01.049
Kim, D., Jeon, S.-B., Kim, J. Y., Seol, M.-L., Kim, S. O., and Choi, Y.-K. (2015). High-performance nanopattern triboelectric generator by block copolymer lithography. Nano Energy 12, 331–338. doi:10.1016/j.nanoen.2015.01.008
Kim, H., Tadesse, Y., and Priya, S. (2009). “Piezoelectric energy harvesting,” in Energy harvesting technologies (Berlin, Germany: Springer), 3–39.
Kim, M.-O., Pyo, S., Oh, Y., Kang, Y., Cho, K.-H., Choi, J., et al. (2018). Flexible and multi-directional piezoelectric energy harvester for self-powered human motion sensor. Smart Mat. Struct. 27, 035001. doi:10.1088/1361-665x/aaa722
Kim, M., and Yun, K.-S. (2017). Helical piezoelectric energy harvester and its application to energy harvesting garments. Micromachines 8, 115. doi:10.3390/mi8040115
King, D. A. (2004). Environment. Climate change science: Adapt, mitigate, or ignore? Science 303, 176–177. doi:10.1126/science.1094329
Kuang, Y., Ruan, T., Chew, Z. J., and Zhu, M. (2017). Energy harvesting during human walking to power a wireless sensor node. Sensors Actuators A Phys. 254, 69–77. doi:10.1016/j.sna.2016.11.035
Kuang, Y., and Zhu, M. (2016). Characterisation of a knee-joint energy harvester powering a wireless communication sensing node. Smart Mat. Struct. 25, 055013. doi:10.1088/0964-1726/25/5/055013
Kymissis, J., Kendall, C., Paradiso, J., and Gershenfeld, N. (1998). “Parasitic power harvesting in shoes,” in Digest of Papers. Second International Symposium on Wearable Computers (Cat. No.98EX215) (ieeexplore.ieee.org), 132–139.
Li, C., Wu, S., Luk, P. C. K., Gu, M., and Jiao, Z. (2019a). Enhanced bandwidth nonlinear resonance electromagnetic human motion energy harvester using magnetic springs and ferrofluid. IEEE/ASME Trans. Mechatron. 24, 710–717. doi:10.1109/tmech.2019.2898405
Li, J. A., Ma, Z., Wang, H. T., Gao, X. X., Zhou, Z., Tao, R. W., et al. (2019b). Skin-inspired electronics and its applications in advanced intelligent systems. Adv. Intell. Syst. 1, 1970060. doi:10.1002/aisy.201970060
Li Teng, S. D., and Liang, J. (2022). “A nano-power wake-up circuit for energy-driven iot applications,” in Proceedings of the 2022 IEEE International Symposium on Circuits and Systems.
Li, X., Tang, H., Hu, G., and Liang, J. (2021a). “Live demo of a transient-motion-powered human motion detector,” in 2021 IEEE International Symposium on Circuits and Systems (ISCAS) (Daegu, Korea: IEEE).
Li, X., Tang, H., Hu, G., Zhao, B., and Liang, J. (2021b). ViPSN-pluck: A transient-motion-powered motion detector. IEEE Internet Things J. 2021, 3372–3382. doi:10.1109/jiot.2021.3098238
Li, Z., Yang, Z., Naguib, H., and Zu, J. (2018). Design and studies on a Low-Frequency Truss-Based Compressive-Mode piezoelectric energy harvester. IEEE/ASME Trans. Mechatron. 23, 2849–2858. doi:10.1109/tmech.2018.2871781
Liang, L., and Yang, H. (2021). Kinetic energy harvesting toward Battery-Free IoT: Fundamentals, Co-Design necessity and prospects. Shenzhen, China: ZTE Communications.
Lin, L., Hu, Y., Xu, C., Zhang, Y., Zhang, R., Wen, X., et al. (2013). Transparent flexible nanogenerator as self-powered sensor for transportation monitoring. Nano Energy 2, 75–81. doi:10.1016/j.nanoen.2012.07.019
Lin, W., Wang, B., Peng, G., Shan, Y., Hu, H., and Yang, Z. (2021). Skin-inspired piezoelectric tactile sensor array with crosstalk-free row+column electrodes for spatiotemporally distinguishing diverse stimuli. Adv. Sci. 8, 2002817. doi:10.1002/advs.202002817
Liu, H., Hou, C., Lin, J., Li, Y., Shi, Q., Chen, T., et al. (2018a). A non-resonant rotational electromagnetic energy harvester for low-frequency and irregular human motion. Appl. Phys. Lett. 113, 203901. doi:10.1063/1.5053945
Liu, H., Soon, B. W., Wang, N., Tay, C. J., Quan, C., and Lee, C. (2012). Feasibility study of a 3D vibration-driven electromagnetic MEMS energy harvester with multiple vibration modes. J. Micromech. Microeng. 22, 125020. doi:10.1088/0960-1317/22/12/125020
Liu, H., Zhong, J., Lee, C., Lee, S.-W., and Lin, L. (2018b). A comprehensive review on piezoelectric energy harvesting technology: Materials, mechanisms, and applications. Appl. Phys. Rev. 5, 041306. doi:10.1063/1.5074184
Liu, M., Qian, F., Mi, J., and Zuo, L. (2022). Biomechanical energy harvesting for wearable and mobile devices: State-of-the-art and future directions. Appl. Energy 321, 119379. doi:10.1016/j.apenergy.2022.119379
Liu, Y., Li, E., Wang, X., Chen, Q., Zhou, Y., Hu, Y., et al. (2020). Self-powered artificial auditory pathway for intelligent neuromorphic computing and sound detection. Nano Energy 78, 105403. doi:10.1016/j.nanoen.2020.105403
Luo, J., Wang, Z., Xu, L., Wang, A. C., Han, K., Jiang, T., et al. (2019). Flexible and durable wood-based triboelectric nanogenerators for self-powered sensing in athletic big data analytics. Nat. Commun. 10, 5147. doi:10.1038/s41467-019-13166-6
Ma, D., Lan, G., Xu, W., Hassan, M., and Hu, W. (2018). “Sehs: Simultaneous energy harvesting and sensing using piezoelectric energy harvester,” in 2018 IEEE/ACM Third International Conference on Internet-of-Things Design and Implementation (IoTDI) (ieeexplore.ieee.org), 201–212.
Ma, D., Lan, G., Xu, W., Hassan, M., and Hu, W. (2020). Simultaneous energy harvesting and gait recognition using piezoelectric energy harvester. IEEE Trans. Mob. Comput. 2020, 1.
Ma, Y., Zheng, Q., Liu, Y., Shi, B., Xue, X., Ji, W., et al. (2016). Self-Powered, One-Stop, and multifunctional implantable triboelectric active sensor for Real-Time biomedical monitoring. Nano Lett. 16, 6042–6051. doi:10.1021/acs.nanolett.6b01968
Manjarrés, J., Lan, G., Gorlatova, M., Hassan, M., and Pardo, M. (2021). Enhancing kinetic energy harvesting-based human activity recognition with deep learning and data augmentation. IEEE Internet Things J. 2021, 1.
Mao, Y., Zhu, Y., Zhao, T., Jia, C., Wang, X., and Wang, Q. (2021). Portable mobile gait monitor system based on triboelectric nanogenerator for monitoring gait and powering electronics. Energies 14, 4996. doi:10.3390/en14164996
Mitcheson, P. D., Miao, P., Stark, B. H., Yeatman, E., Holmes, A., and Green, T. (2004). Mems electrostatic micropower generator for low frequency operation. Sensors Actuators A Phys. 115, 523–529. doi:10.1016/j.sna.2004.04.026
Plageras, A. P., Psannis, K. E., Stergiou, C., Wang, H., and Gupta, B. B. (2018). Efficient iot-based sensor big data collection–processing and analysis in smart buildings. Future Gener. Comput. Syst. 82, 349–357. doi:10.1016/j.future.2017.09.082
Platt, S. R., Farritor, S., and Haider, H. (2005). On low-frequency electric power generation with pzt ceramics. IEEE/ASME Trans. Mechatron. 10, 240–252. doi:10.1109/tmech.2005.844704
Preimesberger, J. I., Kang, S., and Arnold, C. B. (2020). Figures of merit for piezoelectrochemical energy-harvesting systems. Joule 4, 1893–1906. doi:10.1016/j.joule.2020.07.019
Proto, A., Fida, B., Bernabucci, I., Bibbo, D., Conforto, S., Schmid, M., et al. (2016a). “Wearable pvdf transducer for biomechanical energy harvesting and gait cycle detection,” in 2016 IEEE EMBS Conference on Biomedical Engineering and Sciences (IECBES) (Kuala Lumpur, Malaysia: IEEE), 62–66.
Proto, A., Penhaker, M., Bibbo, D., Vala, D., Conforto, S., and Schmid, M. (2016b). Measurements of generated energy/electrical quantities from locomotion activities using piezoelectric wearable sensors for body motion energy harvesting. Sensors 16, 524. doi:10.3390/s16040524
Proto, A., Penhaker, M., Conforto, S., and Schmid, M. (2017). Nanogenerators for human body energy harvesting. Trends Biotechnol. 35, 610–624. doi:10.1016/j.tibtech.2017.04.005
Qian, F., Xu, T.-B., and Zuo, L. (2018). Design, optimization, modeling and testing of a piezoelectric footwear energy harvester. Energy Convers. Manag. 171, 1352–1364. doi:10.1016/j.enconman.2018.06.069
Quan, T., Wang, X., Wang, Z. L., and Yang, Y. (2015). Hybridized electromagnetic–triboelectric nanogenerator for a self-powered electronic watch. ACS Nano 9, 12301–12310. doi:10.1021/acsnano.5b05598
Rome, L. C., Flynn, L., Goldman, E. M., and Yoo, T. D. (2005). Generating electricity while walking with loads. Science 309, 1725–1728. doi:10.1126/science.1111063
Ryu, H., Yoon, H.-J., and Kim, S.-W. (2019). Energy harvesters: Hybrid energy harvesters: Toward sustainable energy harvesting (adv. Mater. 34/2019). Adv. Mat. 31, 1970244. doi:10.1002/adma.201970244
Sandhu, M. M., Geissdoerfer, K., Khalifa, S., Jurdak, R., Portmann, M., and Kusy, B. (2020). “Towards energy positive sensing using kinetic energy harvesters,” in 2020 IEEE International Conference on Pervasive Computing and Communications (PerCom) (ieeexplore.ieee.org), 1–10.
Sandhu, M. M., Khalifa, S., Geissdoerfer, K., Jurdak, R., and Portmann, M. (2021). “Solar: Energy positive human activity recognition using solar cells,” in 2021 IEEE International Conference on Pervasive Computing and Communications (PerCom) (Kassel, Germany: IEEE), 1–10.
Shaikh, F. K., and Zeadally, S. (2016). Energy harvesting in wireless sensor networks: A comprehensive review. Renew. Sustain. Energy Rev. 55, 1041–1054. doi:10.1016/j.rser.2015.11.010
Shi, Q., He, T., and Lee, C. (2019). More than energy harvesting–combining triboelectric nanogenerator and flexible electronics technology for enabling novel micro-/nano-systems. Nano Energy 57, 851–871. doi:10.1016/j.nanoen.2019.01.002
Singh, S., Kumar Gupta, V., and Mukherjee, S. (2018). Piezoelectric based energy harvester embedded in shoe for wearable electronics. Mat. phy. Mech. 37, 159. (
Syafrudin, M., Alfian, G., Fitriyani, N. L., and Rhee, J. (2018). Performance analysis of iot-based sensor, big data processing, and machine learning model for real-time monitoring system in automotive manufacturing. Sensors 18, 2946. doi:10.3390/s18092946
Tang, X., Lin, T., and Zuo, L. (2014). Design and optimization of a tubular linear electromagnetic vibration energy harvester. IEEE/ASME Trans. Mechatron. 19, 615–622. doi:10.1109/tmech.2013.2249666
Tang, X., Wang, X., Cattley, R., Gu, F., and Ball, A. D. (2018). Energy harvesting technologies for achieving self-powered wireless sensor networks in machine condition monitoring: A review. Sensors 18, 4113. doi:10.3390/s18124113
Tao, K., Wu, J., Tang, L., Xia, X., Lye, S. W., Miao, J., et al. (2016). A novel two-degree-of-freedom mems electromagnetic vibration energy harvester. J. Micromech. Microeng. 26, 035020. doi:10.1088/0960-1317/26/3/035020
Tian, Z., He, J., Chen, X., Zhang, Z., Wen, T., Zhai, C., et al. (2017). Performance-boosted triboelectric textile for harvesting human motion energy. Nano Energy 39, 562–570. doi:10.1016/j.nanoen.2017.06.018
Verhelst, J., Van Ham, G., Saelens, D., and Helsen, L. (2017). Model selection for continuous commissioning of hvac-systems in office buildings: A review. Renew. Sustain. Energy Rev. 76, 673–686. doi:10.1016/j.rser.2017.01.119
Wang, C., Dong, L., Peng, D., and Pan, C. (2019a). Tactile sensors for advanced intelligent systems. Adv. Intell. Syst. 1, 1900090. doi:10.1002/aisy.201900090
Wang, J., Wang, H., Thakor, N. V., and Lee, C. (2019b). Self-Powered direct muscle stimulation using a triboelectric nanogenerator (TENG) integrated with a flexible Multiple-Channel intramuscular electrode. ACS Nano 13, 3589–3599. doi:10.1021/acsnano.9b00140
Wang, S., Niu, S., Yang, J., Lin, L., and Wang, Z. L. (2014). Quantitative measurements of vibration amplitude using a contact-mode freestanding triboelectric nanogenerator. ACS Nano 8, 12004–12013. doi:10.1021/nn5054365
Wang, W., Cao, J., Bowen, C. R., Inman, D. J., and Lin, J. (2018). Performance enhancement of nonlinear asymmetric bistable energy harvesting from harmonic, random and human motion excitations. Appl. Phys. Lett. 112, 213903. doi:10.1063/1.5027555
Wang, X., Niu, S., Yi, F., Yin, Y., Hao, C., Dai, K., et al. (2017). Harvesting ambient vibration energy over a wide frequency range for Self-Powered electronics. ACS Nano 11, 1728–1735. doi:10.1021/acsnano.6b07633
Wang, X. (2012). Piezoelectric nanogenerators—Harvesting ambient mechanical energy at the nanometer scale. Nano Energy 1, 13–24. doi:10.1016/j.nanoen.2011.09.001
Wang, Z. L. (2013). Triboelectric nanogenerators as new energy technology for self-powered systems and as active mechanical and chemical sensors. ACS Nano 7, 9533–9557. doi:10.1021/nn404614z
Wen, F., Sun, Z., He, T., Shi, Q., Zhu, M., Zhang, Z., et al. (2020). Machine learning glove using self-powered conductive superhydrophobic triboelectric textile for gesture recognition in VR/AR applications. Adv. Sci. 7, 2000261. doi:10.1002/advs.202000261
Wen, S., and Xu, Q. (2019). Design of a novel piezoelectric energy harvester based on integrated multistage force amplification frame. IEEE/ASME Trans. Mechatron. 24, 1228–1237. doi:10.1109/tmech.2019.2906999
Wu, N., Cheng, X., Zhong, Q., Zhong, J., Li, W., Wang, B., et al. (2015). Cellular polypropylene piezoelectret for human body energy harvesting and health monitoring. Adv. Funct. Mat. 25, 4788–4794. doi:10.1002/adfm.201501695
Xie, L., and Cai, M. (2015). An In-Shoe harvester with motion magnification for scavenging energy from human foot strike. IEEE/ASME Trans. Mechatron. 20, 3264–3268. doi:10.1109/tmech.2015.2428618
Xie, L., Huang, G., Huang, L., Cai, S., and Li, X. (2019). An unpowered flexible lower limb exoskeleton: Walking assisting and energy harvesting. IEEE/ASME Trans. Mechatron. 24, 2236–2247. doi:10.1109/tmech.2019.2933983
Xu, W., Lan, G., Lin, Q., Khalifa, S., Hassan, M., Bergmann, N., et al. (2019). KEH-Gait: Using kinetic energy harvesting for gait-based user authentication systems. IEEE Trans. Mob. Comput. 18, 139–152. doi:10.1109/tmc.2018.2828816
Yan, B., Zhang, C., and Li, L. (2018). Magnetostrictive energy generator for harvesting the rotation of human knee joint. AIP Adv. 8, 056730. doi:10.1063/1.5007195
Yang, B., Lee, C., Xiang, W., Xie, J., He, J. H., Kotlanka, R. K., et al. (2009a). Electromagnetic energy harvesting from vibrations of multiple frequencies. J. Micromech. Microeng. 19, 035001. doi:10.1088/0960-1317/19/3/035001
Yang, C. H., Song, Y., Woo, M. S., Eom, J. H., Song, G. J., Kim, J. H., et al. (2017). Feasibility study of impact-based piezoelectric road energy harvester for wireless sensor networks in smart highways. Sensors Actuators A Phys. 261, 317–324. doi:10.1016/j.sna.2017.04.025
Yang, J., Chen, J., Liu, Y., Yang, W., Su, Y., and Wang, Z. L. (2014). Triboelectrification-based organic film nanogenerator for acoustic energy harvesting and self-powered active acoustic sensing. ACS Nano 8, 2649–2657. doi:10.1021/nn4063616
Yang, J., Chen, J., Su, Y., Jing, Q., Li, Z., Yi, F., et al. (2015). Eardrum-inspired active sensors for self-powered cardiovascular system characterization and throat-attached anti-interference voice recognition. Adv. Mat. 27, 1316–1326. doi:10.1002/adma.201404794
Yang, W., Chen, J., Zhu, G., Wen, X., Bai, P., Su, Y., et al. (2013a). Harvesting vibration energy by a triple-cantilever based triboelectric nanogenerator. Nano Res. 6, 880–886. doi:10.1007/s12274-013-0364-0
Yang, W., Chen, J., Zhu, G., Yang, J., Bai, P., Su, Y., et al. (2013b). Harvesting energy from the natural vibration of human walking. ACS Nano 7, 11317–11324. doi:10.1021/nn405175z
Yang, Y., Lin, L., Zhang, Y., Jing, Q., Hou, T.-C., and Wang, Z. L. (2012). Self-powered magnetic sensor based on a triboelectric nanogenerator. ACS Nano 6, 10378–10383. doi:10.1021/nn304374m
Yang, Y., Tang, L., and Li, H. (2009b). Vibration energy harvesting using macro-fiber composites. Smart Mat. Struct. 18, 115025. doi:10.1088/0964-1726/18/11/115025
Yang, Y., Wang, H., Jiang, R., Guo, X., Cheng, J., and Chen, Y. (2022). A review of IoT-enabled mobile healthcare: Technologies, challenges, and future trends. IEEE Internet Things J. 2022, 9478–9502. doi:10.1109/jiot.2022.3144400
Yang, Y., Zhu, G., Zhang, H., Chen, J., Zhong, X., Lin, Z.-H., et al. (2013). Triboelectric nanogenerator for harvesting wind energy and as self-powered wind vector sensor system. ACS Nano 7, 9461–9468. doi:10.1021/nn4043157
Yi, F., Lin, L., Niu, S., Yang, P. K., Wang, Z., Chen, J., et al. (2015). Stretchable-rubber-based triboelectric nanogenerator and its application as self-powered body motion sensors. Adv. Funct. Mat. 25, 3688–3696. doi:10.1002/adfm.201500428
Yu, A., Song, M., Zhang, Y., Zhang, Y., Chen, L., Zhai, J., et al. (2015). Self-powered acoustic source locator in underwater environment based on organic film triboelectric nanogenerator. Nano Res. 8, 765–773. doi:10.1007/s12274-014-0559-z
Yun, J., Jayababu, N., and Kim, D. (2020). Self-powered transparent and flexible touchpad based on triboelectricity towards artificial intelligence. Nano Energy 78, 105325. doi:10.1016/j.nanoen.2020.105325
Zhang, B., Zhang, S., Li, W., Gao, Q., Zhao, D., Wang, Z. L., et al. (2021). Self-Powered sensing for smart agriculture by Electromagnetic–Triboelectric hybrid generator. ACS Nano 15, 20278–20286. doi:10.1021/acsnano.1c08417
Zhang, W., Xi, Y., Wang, E., Qu, X., Yang, Y., Fan, Y., et al. (2022). Self-powered force sensors for multidimensional tactile sensing. ACS Appl. Mat. Interfaces 14, 20122–20131. doi:10.1021/acsami.2c03812
Zhang, X., Zhang, Z., Pan, H., Salman, W., Yuan, Y., and Liu, Y. (2016a). A portable high-efficiency electromagnetic energy harvesting system using supercapacitors for renewable energy applications in railroads. Energy Convers. Manag. 118, 287–294. doi:10.1016/j.enconman.2016.04.012
Zhang, Y. S., Aleman, J., Shin, S. R., Kilic, T., Kim, D., Mousavi Shaegh, S. A., et al. (2017). Multisensor-integrated organs-on-chips platform for automated and continual in situ monitoring of organoid behaviors. Proc. Natl. Acad. Sci. U. S. A. 114, E2293–E2302. doi:10.1073/pnas.1612906114
Zhang, Y., Zheng, R., Shimono, K., Kaizuka, T., and Nakano, K. (2016b). Effectiveness testing of a piezoelectric energy harvester for an automobile wheel using stochastic resonance. Sensors 16, 1727. doi:10.3390/s16101727
Zhang, Z., He, T., Zhu, M., Sun, Z., Shi, Q., Zhu, J., et al. (2020). Deep learning-enabled triboelectric smart socks for IoT-based gait analysis and VR applications. npj Flex. Electron. 4, 29–12. doi:10.1038/s41528-020-00092-7
Zhao, C., Zhang, Q., Zhang, W., Du, X., Zhang, Y., Gong, S., et al. (2019a). Hybrid piezo/triboelectric nanogenerator for highly efficient and stable rotation energy harvesting. Nano Energy 57, 440–449. doi:10.1016/j.nanoen.2018.12.062
Zhao, J., and You, Z. (2014). A shoe-embedded piezoelectric energy harvester for wearable sensors. Sensors 14, 12497–12510. doi:10.3390/s140712497
Zhao, L.-C., Zou, H.-X., Gao, Q.-H., Yan, G., Liu, F.-R., Tan, T., et al. (2019b). Magnetically modulated orbit for human motion energy harvesting. Appl. Phys. Lett. 115, 263902. doi:10.1063/1.5131193
Zhao, Y., Smith, J. R., and Sample, A. (2015). “Nfc-wisp: A sensing and computationally enhanced near-field rfid platform,” in 2015 IEEE International Conference on RFID (RFID) (San Diego, CA, USA: IEEE), 174–181.
Zheng, L., Cheng, G., Chen, J., Lin, L., Wang, J., Liu, Y., et al. (2015). A hybridized power panel to simultaneously generate electricity from sunlight, raindrops, and wind around the clock. Adv. Energy Mat. 5, 1501152. doi:10.1002/aenm.201501152
Zhou, G., Li, Z., Zhu, Z., Hao, B., and Tang, C. (2019). A new piezoelectric bimorph energy harvester based on the vortex-induced-vibration applied in rotational machinery. Ieee. ASME. Trans. Mechatron. 24, 700–709. doi:10.1109/tmech.2019.2892387
Zhou, S., Cao, J., Erturk, A., and Lin, J. (2013). Enhanced broadband piezoelectric energy harvesting using rotatable magnets. Appl. Phys. Lett. 102, 173901. doi:10.1063/1.4803445
Zhu, G., Bai, P., Chen, J., and Lin Wang, Z. (2013). Power-generating shoe insole based on triboelectric nanogenerators for self-powered consumer electronics. Nano Energy 2, 688–692. doi:10.1016/j.nanoen.2013.08.002
Zhu, G., Peng, B., Chen, J., Jing, Q., and Wang, Z. L. (2015). Triboelectric nanogenerators as a new energy technology: From fundamentals, devices, to applications. Nano Energy 14, 126–138. doi:10.1016/j.nanoen.2014.11.050
Zhu, G., Su, Y., Bai, P., Chen, J., Jing, Q., Yang, W., et al. (2014). Harvesting water wave energy by asymmetric screening of electrostatic charges on a nanostructured hydrophobic thin-film surface. ACS Nano 8, 6031–6037. doi:10.1021/nn5012732
Keywords: energy harvesting, human motion, self-powered sensor, simultaneously energy harvesting and sensing, motionpowered IoT sensing
Citation: Chen Z, Gao F and Liang J (2022) Kinetic energy harvesting based sensing and IoT systems: A review. Front. Electron. 3:1017511. doi: 10.3389/felec.2022.1017511
Received: 12 August 2022; Accepted: 21 September 2022;
Published: 06 October 2022.
Edited by:
Mengying Xie, Tianjin University, ChinaReviewed by:
Bojing Shi, Beihang University, ChinaKean C Aw, The University of Auckland, New Zealand
Yunjia Li, Xi’an Jiaotong University, China
Copyright © 2022 Chen, Gao and Liang. This is an open-access article distributed under the terms of the Creative Commons Attribution License (CC BY). The use, distribution or reproduction in other forums is permitted, provided the original author(s) and the copyright owner(s) are credited and that the original publication in this journal is cited, in accordance with accepted academic practice. No use, distribution or reproduction is permitted which does not comply with these terms.
*Correspondence: Fei Gao, gaofeicsu104@gmail.com; Junrui Liang, liangjr@shanghaitech.edu.cn