Evolution of magnetic properties in iron-based superconductor Eu-doped CaFe2As2
- 1Department of Chemistry and Physics, West Texas A&M University, Canyon, TX, United States
- 2Texas Center for Superconductivity and Department of Physics, University of Houston, Houston, TX, United States
- 3Department of Physics, The University of Texas at Dallas, Richardson, TX, United States
- 4Lawrence Berkeley National Laboratory, Berkeley, CA, United States
This work presents the evolution of magnetic properties of EuxCa1−xFe2As2 (0 ≤ x ≤ 1, ECFA) samples. Unlike the resistivity data, that for magnetic susceptibility χ (T) does not show any clear evidence of the spin density wave (SDW) transition. When the Curie-Weiss contribution is subtracted, a weak anomaly appears at a temperature close to the SDW transition temperature (TSDW) determined from the resistivity data. To understand the magnetic orders arising from Fe-moments and Eu2+ spins order, we have studied the doping dependence of TSDW and Eu2+ antiferromagnetic order TN. It is found that TSDW increases almost linearly with increasing x and remains nearly unchanged above x ∼ 0.4, whereas TN first appears at x ∼ 0.4 and varies almost linearly with further increasing x. These observations suggest that magnetic orders due to two sublattices are coupled to each other. The results discussed here are helpful for understanding the magnetic properties of ECFA and other iron-based superconductors.
Introduction
Iron-based superconductors (IBSs) have continuously served as a platform for exploring novel experimental tools and theoretical approaches since their discovery, especially in studies on the impact of magnetic fluctuations and quantum criticality (Chen et al., 2008a; Hsu et al., 2008; Kamihara et al., 2008; Wang et al., 2012; Deng et al., 2014; Lei et al., 2016; Komedera et al., 2018; Deng et al., 2021; Fernandes et al., 2022). The Fe-magnetic moments appear as a spin density wave (SDW) and, in general, superconductivity emerges when the SDW order is suppressed either by appropriate chemical doping or by applying external pressure (Chen et al., 2008b; Rotter et al., 2008; Takahashi et al., 2008). Thin-film FeSe/STO grown by molecular beam epitaxy (MBE) has exhibited interface-enhanced superconductivity with a Tc above 40 K (Wang et al., 2012; Deng et al., 2014). More recently, a Tc of 38 K was retained in FeSe single crystals at ambient pressure via pressure-quenching (Deng et al., 2021). Defects have also been reported to play an important role in the superconductivity of IBSs (Deng et al., 2016).
CaFe2As2 (Ca122) and EuFe2As2 (Eu122) are two members of the “122” IBS family [(AE)Fe2As2 (AE = Ca, Ba, Sr, and Eu)] and show SDW transitions at TSDW ∼ 165 K and 190 K, respectively (Park et al., 2008; Torikachvili et al., 2008; Lee et al., 2009). At the SDW transition, which appears as a sharp upturn in the resistivity data (Park et al., 2008; Lee et al., 2009; Lv et al., 2011; Shrestha et al., 2020), the material undergoes structural (from the tetragonal to the orthorhombic phase) and magnetic phase (antiferromagnetic ordering of Fe-moments) transitions simultaneously. In Ca122, there exists an additional structural transition near 100 K to the “collapsed-tetragonal” (cT) phase under a moderate pressure of ∼ 0.4 GPa before the superconductivity transition occurs. However, under truly hydrostatic pressure, no superconductivity is observed in Ca122 (Yu et al., 2009). A higher Tc up to 49 K was achieved in this system by chemical doping (Lv et al., 2011) with rare-earth elements. Our group recently reported (Zhao et al., 2016) that the Tc of Ca122 can be raised as high as 25 K using a proper annealing procedure. Another 122 IBS, Eu122, in addition to having a high-temperature SDW transition, exhibits an anomaly near TN ∼ 20 K due to its Eu2+ antiferromagnetic (AFM) order (Ren et al., 2008). It does not show any signature of superconductivity at ambient condition, but it can be turned into a superconductor either by chemical doping [with K (Jeevan et al., 2008) (Tc ∼ 32 K), with Na (Qi et al., 2008) (Tc ∼ 35 K), through Co or La substitutions (He et al., 2010; Ying et al., 2010; Zhang et al., 2012), etc.] or by the application of external high pressure (Terashima et al., 2009; Kurita et al., 2011).
Here, we explore the evolution of the electrical transport and magnetic properties of Eu-doped Ca122 [EuxCa1−xFe2As2 (0 ≤ x ≤ 1, ECFA)]. We recently reported (Shrestha et al., 2020) detailed electrical transport properties of ECFA at ambient and under high-pressure. Here we focus on how its magnetic properties evolve with varied Eu-doping. We observe that, unlike in the measurement of resistivity, the magnetic susceptibility data does not show a visible anomaly resulting from the SDW transition. We find that a tiny anomaly near the SDW transition appears only after subtracting the Curie-Weiss (CW) contribution of the Eu+2 moments.
Experimental details
Large, high-quality single crystals of ECFA were grown using the FeAs flux technique. Starting materials were placed in an alumina crucible that was sealed inside a small silica tube under a reduced Ar atmosphere. The small silica tube was subsequently sealed inside a larger silica tube under vacuum. The assembly was first heated at a temperature of 1,100°C and then slowly cooled down at a rate of 2°C/h. The sample preparation and preliminary characterization details are provided in our previous report (Shrestha et al., 2020). Electrical transport measurement were carried out in a physical property measurement system (PPMS, Quantum Design). A shiny crystal was selected and four platinum wires were attached to its flat surface using silver paint. Magnetic measurements from room temperature down to 2 K were carried out using a magnetic property measurement system (MPMS, Quantum Design). A plate-like sample was placed inside a gelatin capsule that was subsequently attached to a plastic straw. A small magnetic field value of 0.1 T was applied along the c-axis to induce a magnetic moment in the sample.
Results and discussion
Figure 1 shows the temperature-dependent electrical resistance of Ca122 and Eu122. The resistance is normalized to the room-temperature value. Each sample shows a clear upturn anomaly near TSDW ∼ 160 K (Ca122) or 190 K (Eu122) that arises due to the SDW transition, as indicated by the dashed arrows. These TSDW values are consistent with previously reported data (Park et al., 2008; Torikachvili et al., 2008; Lee et al., 2009; Lv et al., 2011). Moreover, there is a tiny kink near TN ∼ 19 K in the Eu122 results (indicated by the solid arrow), which can be more clearly observed in the magnified view shown in the upper inset to Figure 1. This anomaly arises due to the AFM ordering of Eu2+ spin moments (Shrestha et al., 2020). For Ca122, there is a small drop in the resistance value near 10 K, indicated by an asterisk and more clearly visible in the magnified view shown in the lower inset to Figure 1. Under application of external pressure, Ca122 enters into the superconducting state with a transition temperature Tc ∼ 10 K (Park et al., 2008; Torikachvili et al., 2008; Lee et al., 2009). Therefore, this tiny drop in resistance could be due to the onset of the superconducting transition.
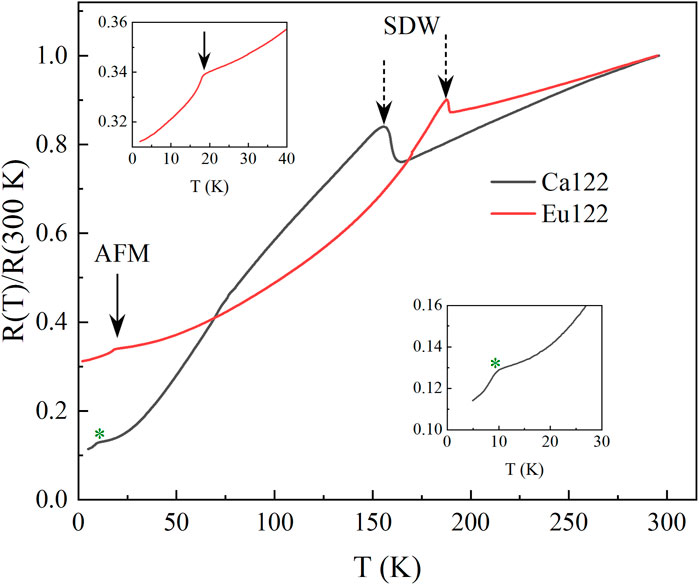
FIGURE 1. Electrical Resistance. Normalized electrical resistance vs. temperature for Ca122 and Eu122. Both samples show a clear anomaly, near 160 K and 190 K for Ca122 and Eu122, respectively, and indicated by dashed arrows, that arises due the SDW transition. The tiny kink near 19 K for Eu122, as indicated by the solid arrow, is due to the Eu2+ AFM order. A small drop in resistance for Ca122 near 10 K, as denoted by an asterisk, is likely due to the superconducting transition (see the text). Upper inset: magnified view of the Eu2+ AFM transition in Eu122. Lower inset: magnified view of the low-temperature drop in resistance for Ca122.
Figure 2 shows the temperature-dependent magnetic susceptibility (χ) of ECFA samples with different amounts of Eu doping (x). χ (T) increases gradually with decreasing temperature and exhibits a distinct anomaly near TN ∼ 10–19 K, as indicated by the arrow. This transition arises due to the Eu2+ AFM order (Ren et al., 2008; Zhang et al., 2012; Maiwald and Gegenwart, 2017). TN is prominent at higher x, and it shifts toward lower temperatures at lower x. For x = 1, TN ∼ 19 K, which is consistent with previously reported TN values (Ren et al., 2008; Zhang et al., 2012; Maiwald and Gegenwart, 2017). Our recent rigorous electrical transport and magnetic studies (Shrestha et al., 2020) showed that TN varies linearly with x. Here, the temperature dependence of χ (T) can be described by the Curie-Weiss (CW) law Eq. 1 as shown in the inset to Figure 2 for the x = 1 sample. The CW law (Ashcroft and Mermin, 1976; Kittel, 2006) is expressed as
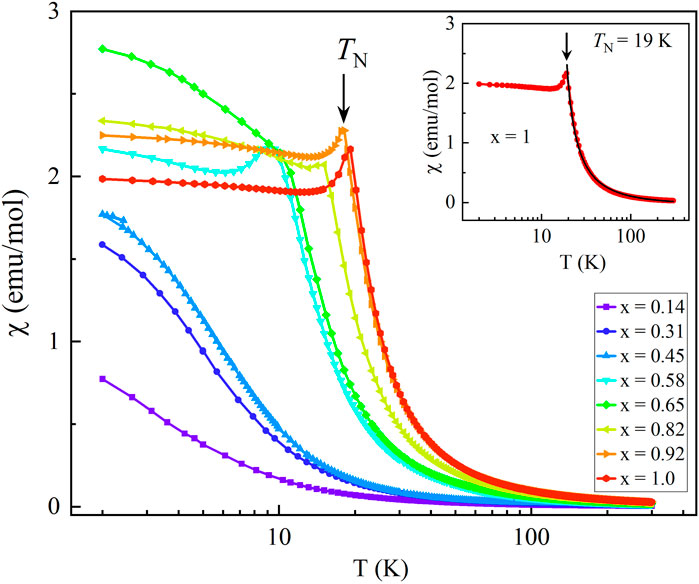
FIGURE 2. Magnetic susceptibility. Magnetic susceptibility (χ) vs. T for ECFA samples with different amounts of Eu doping (0.14 ≤ x ≤ 1. χ (T) increases gradually with decreasing temperature. The low-temperature anomalies, TN ∼ 10–19 K, arise due to the Eu2+ AFM order, as indicated by the arrow. Inset: χ (T) data for EuFe2As2 (x = 1). The solid black curve is the best-fit curve using the Curie-Weiss model Eq. 1. The x-axis is in the logarithmic scale for better visibility of TN.
where C and θ are the Curie-Weiss constant and the Weiss temperature, respectively. Here, C is directly proportional to the effective magnetic moment (μeff) of the sample. Therefore, by fitting the temperature-dependent χ (T) data, we can estimate μeff and θ of the ECFA samples.
Figures 3A–C shows the 1/χ (T) plots for selected ECFA samples. 1/χ (T) varies linearly at high temperatures, but anomalies corresponding to the Eu2+ AFM order occur at low temperatures, as indicated by arrows. The solid lines represent the best-fit to the CW law using Eq. 1. As seen in Figures 3A–C, the temperature-dependent susceptibility data can be well explained by the CW law. It is important to note that there is no clear evidence of the SDW transition in any of the ECFA samples studied [neither in Figure 2 nor in Figures 3A–C] due to Fe-moments in our susceptibility data. In previous studies (Ren et al., 2008; Jiang et al., 2009), the same issue of being unable to observe a clear SDW transition in the magnetization data was reported, but it could be observed after subtracting the CW contribution of the Eu2+ moments. Therefore, we have followed the same approach and calculated Δχ (T) by subtracting the CW contribution of the Eu2+ moments. As shown in the insets to Figures 3A–C, there is a tiny but distinguishable anomaly at TSDW ∼ 165–185 K. These TSDW values are in close agreement with those previously reported based on resistivity and magnetization measurements (Jiang et al., 2009; Harnagea et al., 2018; Tran et al., 2018; Shrestha et al., 2020). We subsequently determined TSDW for various ECFA samples and plotted it as a function of Eu doping. As shown in Figure 3D, TSDW (x) initially increases rapidly with increasing x, gradually increases with further increasing in x above x ∼ 0.4, and then saturates to the value of 190 K. As mentioned in the Introduction, ECFA serves as a special platform that has two magnetic orders, one from Fe-moments (SDW order) and another due to Eu2+ spins (AFM order). To confirm the interaction between these orders, we have also plotted TN vs. x on the right y-axis of Figure 3D. As seen in Figure 3D, with decreasing x, TSDW remains almost unchanged until the Eu2+ moment order is destroyed at x ∼ 0.4. This suggests that the magnetic orders of the two sublattices are coupled to one another.
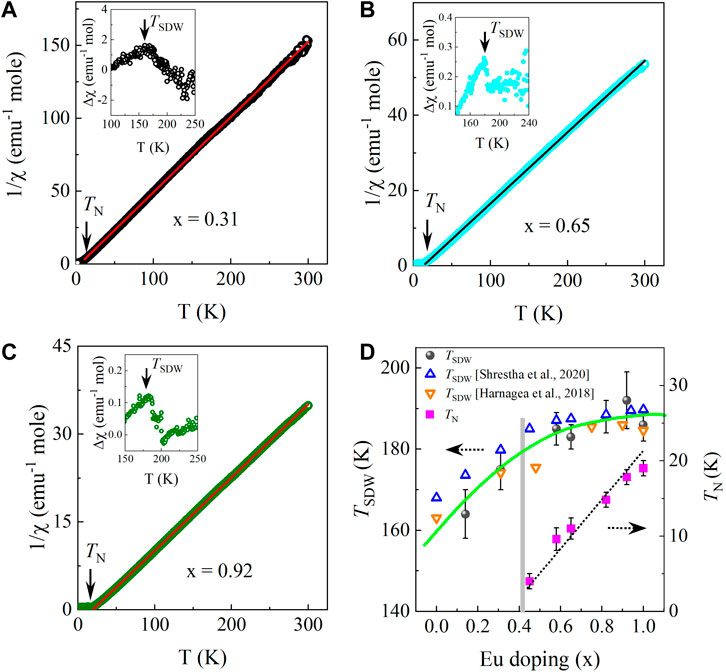
FIGURE 3. Curie-Weiss fit. Inverse susceptibility, 1/χ, vs. T for ECFA samples at (A) x = 0.31, (B) x = 0.65, and (C) x = 0.92.1/χ increases linearly with T, consistent with Curie-Weiss behavior. The arrows show the AFM due to the Eu2+ spins. The solid lines are the best-fit lines using the Curie-Weiss law Eq. 1. The insets in (A)–(C) display the respective Δχ vs. T plots showing the TSDW transitions, as indicated by the arrow in each. (D) TSDW (solid spheres) and TN (solid squares) vs. x for ECFA samples. Open upright and inverted triangles are TSDW data from Refs (Shrestha et al., 2020). and (Harnagea et al., 2018), respectively. TSDW increases rapidly with increasing x and then slowly saturates to TSDW ∼ 190 K above x ∼ 0.4. The error bars are taken as the half-width of the transition. The vertical solid stripe and solid curve are guides to the eye. TN first appears at x ∼ 0.4 and increases almost linearly with further increasing x, as indicated by the dotted line.
From the CW-fitting above, we have obtained the best-fit parameters C = (7.94 ± 0.001) emu K/mole and θ = (19.36 ± 0.13) K for x = 0.92. From the C value obtained, we have estimated the effective magnetic moment of Eu2+, μeff = (7.97 ± 0.10) μB. These values are comparable with those previously reported (Jiang et al., 2009; Harnagea et al., 2018; Tran et al., 2018) for a similar Eu-doping level. The positive θ value implies ferromagnetic (FM) interaction between Eu2+ moments. Resonant X-ray scattering (Herrero-Martín et al., 2009) and neutron diffraction data (Xiao et al., 2009; Xiao et al., 2010) have also shown that Eu2+ spins on the same plane have FM interaction, whereas they have weak AFM coupling across the plane. The Eu-doping-dependent best-fit parameters were calculated for various ECFA samples and are presented in Figure 4.
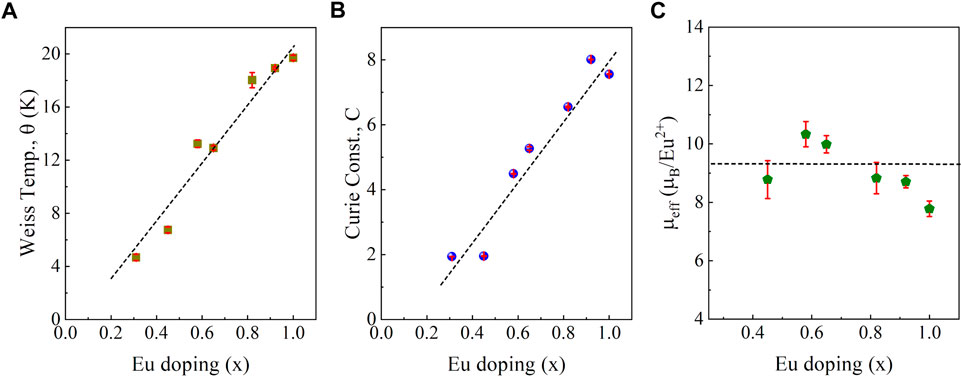
FIGURE 4. Weiss temperature, Curie-constant, and effective moment. The best-fit parameters (A) θ (K) and (B) C plotted as functions of Eu doping (x). Both θ and C vary nearly linearly with Eu doping, as shown by the dashed lines. (C) μeff per Eu2+ ion at various Eu content. Although C increases linearly with increasing x, μeff per Eu2+ ion does not show a clear dependence on x, and its value varies between ∼ 8–10 μB).
Figure 4A shows the variation of the best-fit of parameter θ with x. For x = 0.31, θ = (4.68 ± 0.23) K, and it increases almost linearly with further increasing Eu doping. At x = 1, θ = (19.71 ± 0.19). This θ value is comparable with those of 20.3 K reported by Harnagea et al. (2018) and 19.7 K reported by Jiang et al. (2009). Similarly, the Eu-doping-dependent best fit of C is shown in Figure 4B and has nearly linear dependence on x. Figure 4C displays μeff per Eu2+ ion at various Eu content and shows that, although C increases linearly with x, μeff per Eu2+ ion does not exhibit any clear Eu-doping dependence. Its value changes between ∼ 8–10 μB.
The doping effect on 122 IBSs has been studied extensively using both rare-earth metals (RE = La, Pr, Nd, etc.) (Qi et al., 2008; Gao et al., 2011; Lv et al., 2011; Saha et al., 2012; Ying et al., 2012) and alkali metals (A = K and Na) (Rotter et al., 2008; Sasmal et al., 2008; Zhao et al., 2010). Replacing a divalent AE2+ ion with a trivalent RE3+ (or a univalent A+) in a 122 IBS serves as electron doping (or hole doping) and tunes the electronic properties of the system. In both cases (electron and hole doping), the SDW order is gradually suppressed, and the superconducting phase has been stabilized in the 122 system with a maximum Tc of 49 K (for electron doping) (Gao et al., 2011; Qi et al., 2012; Saha et al., 2012; Ying et al., 2012) and 38 K (for hole doping) (Rotter et al., 2008; Sasmal et al., 2008; Zhao et al., 2010). The superconducting phase in the RE-doped 122 (or A-doped 122 IBS) arises by the suppression of the SDW order via chemical pressure, electron (or hole) doping, or the combination of both (Saha et al., 2012). However, isovalent substitution of Ca2+ with Eu2+ neither adds any charge carriers in the system nor suppresses the SDW order. As a result, no superconductivity has been observed in ECFA samples here. Nonetheless, ECFA provides a unique platform for studying magnetic orders arising from Eu2+ and Fe2+ sublattices. The doping dependence of TSDW and TN, as shown in Figure 3D, suggests that there exists a weak coupling between the SDW order of FeAs and Eu2+ localized moments. This result is consistent with NMR (Guguchia et al., 2011), neutron diffraction (Xiao et al., 2009), and ARPES (Zhou et al., 2010) studies on Eu122.
Summary
Magnetic properties of EuxCa1−xFe2As2 (0 ≤ x ≤ 1, ECFA) samples were presented. The resistivity data show clear anomalies due to the SDW and Eu2+ AFM transitions. The magnetic susceptibility (χ) of ECFA samples exhibits 1/T behavior, which can explained using the Curie-Weiss law. The χ (T) data provide evidence of the Eu2+ AFM order but do not indicate the SDW transition due to the Fe-moments. A weak transition near TSDW appears only after the subtraction of the Curie-Weiss contribution of the Eu2+ moments. With increasing Eu doping, TSDW initially increases rapidly up to x ∼ 0.4, and it then gradually saturates to a value of 190 K at x = 1. The best-fits of the Curie-Weiss law parameters, the Curie constant (C), and the Weiss-temperature (θ), increase linearly with increasing Eu doping, while the effective magnetic moment, μeff, per Eu2 + ion remains nearly constant. Our results provide detailed information about how the magnetic properties evolve in the Eu-doped Ca122 system, which will eventually help in understanding superconductivity in ECFA.
Data availability statement
The raw data supporting the conclusion of this article will be made available by the authors, without undue reservation.
Author contributions
BL synthesized high-quality samples of Eu-doped CaFe2As2. KS and LD carried out measurements. KS analyzed experimental data and wrote the manuscript. CC supervised the project.
Funding
The research at West Texas A&M University was supported by the Killgore Faculty Research program and the Welch Foundation (Grant No. AE-0025). The work done at the University of Houston was supported in part by U.S. Air Force Office of Scientific Research Grants FA9550-15-1-0236 and FA9550-20-1-0068, the T. L. L. Temple Foundation, the John J. and Rebecca Moores Endowment, and the State of Texas through the Texas Center for Superconductivity at the University of Houston. BL acknowledges financial support by U. S. Air Force Office of Scientific Research Grant FA9550-19-1-0037.
Conflict of interest
The authors declare that the research was conducted in the absence of any commercial or financial relationships that could be construed as a potential conflict of interest.
Publisher’s note
All claims expressed in this article are solely those of the authors and do not necessarily represent those of their affiliated organizations, or those of the publisher, the editors and the reviewers. Any product that may be evaluated in this article, or claim that may be made by its manufacturer, is not guaranteed or endorsed by the publisher.
References
Chen, G. F., Li, Z., Wu, D., Li, G., Hu, W. Z., Dong, J., et al. (2008b). Superconductivity at 41 K and its competition with spin-density-wave instability in layered CeO1−xFxFeAs. Phys. Rev. Lett. 100, 247002. doi:10.1103/physrevlett.100.247002
Chen, X. H., Wu, T., Wu, G., Liu, R. H., Chen, H., and Fang, D. F. (2008a). Superconductivity at 43 K in SmFeAsO1−xFx. Nature 453, 761.
Deng, L., Bontke, T., Dahal, R., Xie, Y., Bin Gaoe, K. Y., Xue, Li, et al. (2021). Pressure-induced high-temperature superconductivity retained without pressure in FeSe single crystals. Proc. Natl. Acad. Sci. U. S. A. 118, e2108938118. doi:10.1073/pnas.2108938118
Deng, L. Z., Lv, B., Zhao, K., Wei, F. Y., Xue, Y. Y., Wu, Z., et al. (2016). Evidence for defect-induced superconductivity up to 49 K in Ca1−x RxFe2As2. Phys. Rev. B 93, 054513. doi:10.1103/physrevb.93.054513
Deng, L. Z., Lv, Z. W. B., Xue, Y. Y., Zhang, W. H., Li, F. S., Wang, L. L., et al. (2014). Meissner and mesoscopic superconducting states in 1–4 unit-cell FeSe films. Phys. Rev. B 90, 214513. doi:10.1103/physrevb.90.214513
Fernandes, R. M., Coldea, A. I., Ding, H., Fisher, I. R., Hirschfeld, P. J., and Kotliar, G. (2022). Iron pnictides and chalcogenides: A new paradigm for superconductivity. Nature 601, 35–44. doi:10.1038/s41586-021-04073-2
Gao, Z., Qi, Y., Wang, L., Wang, D., Zhang, X., Yao, C., et al. (2011). Synthesis and properties of La-doped CaFe2As2 single crystals with Tc = 42.7 K. EPL 95, 67002. doi:10.1209/0295-5075/95/67002
Guguchia, Z., Roos, J., Shengelaya, A., Katrych, S., Bukowski, Z., Weyeneth, S., et al. (2011). Strong coupling between Eu2+ spins and Fe2As2 layers in EuFe1.9Co0.1As2 observed with NMR. Phys. Rev. B 83, 144516. doi:10.1103/physrevb.83.144516
Harnagea, L., Kumar, R., Singh, S., Wurmehl, S., Wolter, A. U. B., and Buchner, B. (2018). Evolution of the magnetic order of fe and eu sublattices in Eu1−xCaxFe2As2 (0 ≤ x ≤ 1) single crystals. J. Phys. Condens. Matter 30, 415601. doi:10.1088/1361-648x/aadea6
He, Y., Wu, T., Wu, G., Zheng, Q. J., Liu, Y. Z., Chen, H., et al. (2010). Evidence for competing magnetic and superconducting phases in superconducting Eu1−xSrxFe2−yCoyAs2 single crystals. J. Phys. Condens. Matter 22, 235701. doi:10.1088/0953-8984/22/23/235701
Herrero-Martín, J., Scagnoli, V., Mazzoli, C., Su, Y., Mittal, R., Xiao, Y., et al. (2009). Magnetic structure of EuFe2As2 as determined by resonant x-ray scattering. Phys. Rev. B 80, 134411. doi:10.1103/physrevb.80.134411
Hsu, F. C., Luo, J. Y., Yeh, K. W., Chen, T. K., Huang, T. W., Wu, P. M., et al. (2008). Superconductivity in the PbO-type structure alpha-FeSe. Proc. Natl. Acad. Sci. U. S. A. 105, 14262–14264. doi:10.1073/pnas.0807325105
Jeevan, H. S., Hossain, Z., Kasinathan, D., Rosner, H., Geibel, C., and Gegenwart, P. (2008). High-temperature superconductivity in Eu0.5K0.5Fe2As2. Phys. Rev. B 78, 092406. doi:10.1103/physrevb.78.092406
Jiang, S., Luo, Y., Ren, Z., Zhu, Z., Wang, C., Xu, X., et al. (2009). Metamagnetic transition in EuFe2As2 single crystals. New J. Phys. 11, 025007. doi:10.1088/1367-2630/11/2/025007
Kamihara, Y., Watanabe, T., Hirano, M., and Hosono, H. (2008). Iron-based layered superconductor La[O1−xFx]Fe2As2, x = 0.05 - 0.12 with Tc = 26 K. J. Am. Chem. Soc. 130, 3296.
Komedera, K., Blachowski, A., Ruebenbauer, K., Zukrowski, J., Dubiel, S., Tran, L., et al. (2018). Mössbauer study of Eu0.57Ca0.43Fe2As2 and Eu0.73Ca0.27(Fe0.87Co0.13)2 As2: A comparison to “122” iron-based superconductors parent compounds EuFe2As2 and CaFe2As2. J. Magn. Magn. Mat. 457, 1–7. doi:10.1016/j.jmmm.2018.02.079
Kurita, N., Kimata, M., Kodama, K., Harada, A., Tomita, M., Suzuki, H. S., et al. (2011). Phase diagram of pressure-induced superconductivity in EuFe2As2 probed by high-pressure resistivity up to 3.2 GPa. Phys. Rev. B 83, 214513. doi:10.1103/physrevb.83.214513
Lee, H., Park, E., Park, T., Sidorov, V. A., Ronning, F., Bauer, E. D., et al. (2009). Pressure-induced superconducting state of antiferromagnetic CaFe2As2. Phys. Rev. B 80, 024519. doi:10.1103/physrevb.80.024519
Lei, B., Cui, J. H., Xiang, Z. J., Shang, C., Wang, N. Z., Ye, G. J., et al. (2016). Evolution of high-temperature superconductivity from a low-Tc phase tuned by carrier concentration in FeSe thin flakes. Phys. Rev. Lett. 116, 077002. doi:10.1103/physrevlett.116.077002
Lv, B., Deng, L., Gooch, M., Wei, F., Sun, Y., Meen, J. K., et al. (2011). Unusual superconducting state at 49 K in electron-doped CaFe2As2 at ambient pressure. Proc. Natl. Acad. Sci. U. S. A. 108, 15705–15709. doi:10.1073/pnas.1112150108
Maiwald, J., and Gegenwart, P. (2017). Interplay of 4f and 3d moments in EuFe2As2 iron pnictides. Phys. Status Solidi B 254, 1600150. doi:10.1002/pssb.201600150
Park, T., Park, E., Lee, H., Klimczuk, T., Bauer, E. D., Ronning, F., et al. (2008). Pressure-induced superconductivity in CaFe2As2. J. Phys. Condens. Matter 20, 322204. doi:10.1088/0953-8984/20/32/322204
Qi, Y., Gao, Z., Wang, L., Wang, D., Zhang, X., and Ma, Y. (2008). Superconductivity at 34.7 K in the iron arsenide Eu0.7Na0.3Fe2As2. New J. Phys. 10, 123003. doi:10.1088/1367-2630/10/12/123003
Qi, Y., Gao, Z., Wang, L., Wang, D., Zhang, X., Yao, C., et al. (2012). Transport properties and anisotropy in rare-earth doped CaFe2As2 single crystals with Tc above 40 K. Supercond. Sci. Technol. 25, 045007. doi:10.1088/0953-2048/25/4/045007
Ren, Z., Zhu, Z., Jiang, S., Xu, X., Tao, Q., Wang, C., et al. (2008). Antiferromagnetic transition in EuFe2As2: A possible parent compound for superconductors. Phys. Rev. B 78, 052501. doi:10.1103/physrevb.78.052501
Rotter, M., Tegel, M., and Johrendt, D. (2008). Superconductivity at 38 K in the iron arsenide(Ba1−xKx)Fe2As2. Phys. Rev. Lett. 101, 107006. doi:10.1103/physrevlett.101.107006
Saha, S. R., Butch, N. P., Drye, T., Magill, J., Ziemak, S., Kirshenbaum, K., et al. (2012). Structural collapse and superconductivity in rare-earth-doped CaFe2As2. Phys. Rev. B 85, 024525. doi:10.1103/physrevb.85.024525
Sasmal, K., Lv, B., Lorenz, B., Guloy, A. M., Chen, F., Xue, Y.-Y., et al. (2008). Superconducting Fe-based compounds(A1−xSrx)Fe2As2withA=Kand Cs with transition temperatures up to 37 K. Phys. Rev. Lett. 101, 107007. doi:10.1103/physrevlett.101.107007
Shrestha, K., Deng, L. Z., Zhao, K., Jawdat, B. I., Lv, B., Lorenz, B., et al. (2020). Doping dependence and high-pressure studies on EuxCa1−xFe2As2 (0 ≤ x ≤ 1). Supercond. Sci. Technol. 33, 095010. doi:10.1088/1361-6668/ab9e7e
Takahashi, H., Igawa, K., Arii, K., Kamihara, Y., Hirano, M., and Hosono, H. (2008). Superconductivity at 43 K in an iron-based layered compound Lao(1−x)F(x)FeAs. Nature 453, 376–378. doi:10.1038/nature06972
Terashima, T., Kimata, M., Satsukawa, H., Harada, A., Hazama, K., Uji, S., et al. (2009). EuFe2As2 under high pressure: An antiferromagnetic bulk superconductor. J. Phys. Soc. Jpn. 78, 083701. doi:10.1143/jpsj.78.083701
Torikachvili, M. S., Bud’ko, S. L., Ni, N., and Canfield, P. C. (2008). Pressure induced superconductivity in CaFe2As2. Phys. Rev. Lett. 101, 057006. doi:10.1103/physrevlett.101.057006
Tran, L. M., Babij, M., Korosec, L., Shang, T., Bukowski, Z., and Shiroka, T. (2018). Magnetic phase diagram of ca-substituted EuFe2As2. Phys. Rev. B 98, 104412. doi:10.1103/physrevb.98.104412
Wang, Q.-Y., Li, Z., Zhang, W.-H., Zhang, Z.-C., Zhang, J.-S., Li, W., et al. (2012). Interface-induced high-temperature superconductivity in single unit-cell FeSe films on SrTiO3. Chin. Phys. Lett. 29, 037402. doi:10.1088/0256-307x/29/3/037402
Xiao, Y., Su, Y., Meven, M., Mittal, R., Kumar, C. M. N., Chatterji, T., et al. (2009). Magnetic structure of EuFe2As2 determined by single-crystal neutron diffraction. Phys. Rev. B 80, 174424. doi:10.1103/physrevb.80.174424
Xiao, Y., Su, Y., Schmidt, W., Schmalzl, K., Kumar, C. M. N., Price, S., et al. (2010). Field-induced spin reorientation and giant spin-lattice coupling in EuFe2As2. Phys. Rev. B 81, 220406. doi:10.1103/physrevb.81.220406
Ying, J. J., Liang, J. C., Luo, X. G., Wang, X. F., Yan, Y. J., Zhang, M., et al. (2012). Transport and magnetic properties of La-doped CaFe2As2. Phys. Rev. B 85, 144514. doi:10.1103/physrevb.85.144514
Ying, J. J., Wu, T., Zheng, Q. J., He, Y., Wu, G., Li, Q. J., et al. (2010). Electron spin resonance in EuFe2−xCoxAs2 single crystals. Phys. Rev. B 81, 052503. doi:10.1103/physrevb.81.052503
Yu, W., Aczel, A. A., Williams, T. J., Bud’ko, S. L., Ni, N., Canfield, P. C., et al. (2009). Absence of superconductivity in single-phase CaFe2As2 under hydrostatic pressure. Phys. Rev. B 79, 020511. doi:10.1103/physrevb.79.020511
Zhang, M., Ying, J. J., Yan, Y. J., Wang, A. F., Wang, X. F., Xiang, Z. J., et al. (2012). Phase diagram as a function of doping level and pressure in the Eu1−xLaxFe2As2 system. Phys. Rev. B 85, 092503. doi:10.1103/physrevb.85.092503
Zhao, K., Liu, Q. Q., Wang, X. C., Deng, Z., Lv, Y. X., Zhu, J. L., et al. (2010). Superconductivity above 33 k in (Ca1−xNax)Fe2As2. J. Phys. Condens. Matter 22, 222203. doi:10.1088/0953-8984/22/22/222203
Zhao, K., Lv, B., Deng, L., Huyan, S.-Y., Xue, Y.-Y., and Chu, C.-W. (2016). Interface-induced superconductivity at 25 K at ambient pressure in undoped CaFe2As2 single crystals. Proc. Natl. Acad. Sci. U. S. A. 113, 12968–12973. doi:10.1073/pnas.1616264113
Keywords: iron-based superconductors, spin-density wave order, antiferromagnetic order, Curie-Weiss law, magnetic susceptability
Citation: Shrestha K, Deng LZ, Lv B and Chu CW (2022) Evolution of magnetic properties in iron-based superconductor Eu-doped CaFe2As2. Front. Electron. Mater. 2:1044620. doi: 10.3389/femat.2022.1044620
Received: 14 September 2022; Accepted: 23 November 2022;
Published: 13 December 2022.
Edited by:
Audrey Grockowiak, National Center for Research in Energy and Materials, BrazilCopyright © 2022 Shrestha, Deng, Lv and Chu. This is an open-access article distributed under the terms of the Creative Commons Attribution License (CC BY). The use, distribution or reproduction in other forums is permitted, provided the original author(s) and the copyright owner(s) are credited and that the original publication in this journal is cited, in accordance with accepted academic practice. No use, distribution or reproduction is permitted which does not comply with these terms.
*Correspondence: K. Shrestha, kshrestha@wtamu.edu