- Department of Molecular Endocrinology and Metabolism, Tokyo Medical and Dental University, Bunkyo, Japan
Mammals have two types of thermogenic adipocytes: brown adipocytes and beige adipocytes. Thermogenic adipocytes express high levels of uncoupling protein 1 (UCP1) to dissipates energy in the form of heat by uncoupling the mitochondrial proton gradient from mitochondrial respiration. There is much evidence that UCP1 is the center of BAT thermogenesis and systemic energy homeostasis. Recently, UCP1 independent thermogenic pathway identified in thermogenic adipocytes. Importantly, the thermogenic pathways are different in brown and beige adipocytes. Ca2+-ATPase 2b calcium cycling mechanism is selective to beige adipocytes. It remains unknown how the multiple thermogenic mechanisms are coordinately regulated. The discovery of UCP1-independent thermogenic mechanisms potential offer new opportunities for improving obesity and type 2 diabetes particularly in groups such as elderly and obese populations who do not possess UCP1 positive adipocytes.
Thermogenic Fat: Brown and Beige Adipocytes
Mammals have brown and beige thermogenic adipocytes, which are both rich in mitochondria and express uncoupling protein 1 (UCP1). However, brown and beige adipocytes play distinct developmental and anatomical roles in rodents and humans. Brown adipocytes are located in the interscapular and perirenal regions of rodents and infants. By contrast, beige adipocytes (or brite adipocytes) are induced thermogenic adipocytes found sporadically within the white adipose tissue (WAT). The development of beige adipocytes is called “browning” or “beiging.” Beige adipocytes are induced by environmental stimuli, such as chronic cold, β3-adrenergic receptor agonists, peroxisome proliferator-activated receptor gamma (PPARγ) agonists, exercise (1), and cachexia (2). Since the emergence of evidence that adult humans have brown adipose tissue (BAT) (3–7), the debate over whether adult humans have beige adipocytes has been crucial to the metabolic field.
The function of BAT is to regulate the systemic energy balance through non-shivering thermogenesis (NST). Transcriptional analysis of adult human BAT revealed expression of molecular markers specific for murine beige adipocytes (8–10). By contrast, the deep neck region in adult humans possesses thermogenic fat that is similar to the brown fat of mice (11). Of note, clonal analysis of adipose tissue from adult humans revealed evidence that humans have beige adipocytes (12). Even adults who do not exhibit brown fat by 18F-labeled fluorodeoxyglucose positron emission tomography/computerized tomography (18F-FDG-PET/CT) develop thermogenic fat upon prolonged cold stimulation (13–15).
Many studies have reported that the amount of cold-induced thermogenic fat positively correlates with the degree of NST and improvements in insulin sensitivity in humans (13–16). Recently, a study showed a much wider distribution of BAT, including in the abdominal subcutaneous regions, in adult humans by refined 18F-FDG-PET/CT imaging (17). These results support the existence of thermogenic adipocytes in adult humans. Thermogenic adipocytes have the characteristics of the beige-like inducible adipocytes that contribute to whole-body energy homeostasis. Based on these findings, researchers have hypothesized that beige fat may be a promising new therapeutic target to increase energy expenditure and treat obesity and type 2 diabetes. Future studies will determine the function and distinct, essential characteristics of beige fat in humans. While brown and beige adipocytes share many characteristics such as express UCP1, enriched mitochondria, and differentiation mechanisms-transcriptional factor PR domain-containing protein 16 (PRDM16), PPARγ. In contrast to this, recent studies, mainly in mice, suggest discrete thermogenic mechanisms in the two cell types (Figure 1). In this review, we discuss thermogenic mechanisms and pathways in thermogenic fat.
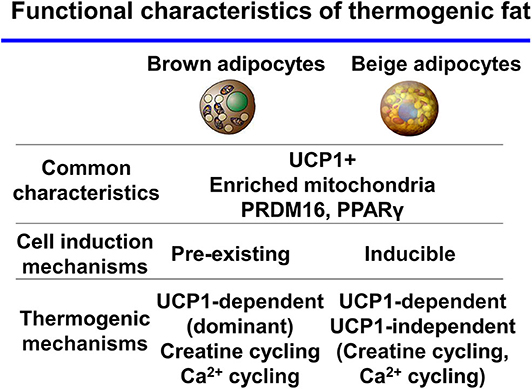
Figure 1. Functional characteristics of thermogenic fat brown and beige adipocytes share many characteristics. In contrast, thermogenic mechanisms are discrete between the two cell types.
UCP1-Dependent Thermogenesis in Thermogenic Fat: Brown and Beige Adipocytes
UCP1 localizes to the mitochondrial inner membrane. It generates heat by dissipating the energy proton gradient from the electron transport chain in mitochondrial respiration. There is considerable evidence that UCP1 is at the center of BAT thermogenesis and systemic energy homeostasis. Many studies have investigated if UCP1 is essential to thermogenesis in thermogenic adipocytes. Ucp1 knockout (KO) mice are unable to maintain their body temperature and develop hypothermia upon acute cold challenge (18). In addition, BAT-deficient mice created by transgenic expression of diphtheria toxin showed diabetic and obese phenotypes in room-temperature environments (19).
The re-synthesis of triacylglycerols after lipolysis is a thermogenic process that is dependent on the amount of ATP needed for triacylglycerol synthesis. Fatty acid synthesis and oxidation are both stimulated and tightly regulated by adrenergic activation (20). Upon adrenergic stimulation, brown adipocyte lipolysis and mitochondrial respiration are activated in a UCP1-dependent manner (21).
However, recent studies in mice with BAT-specific deficiencies in the lipolysis enzyme adipose triglyceride lipase (ATGL) or the ATGL-activating protein comparative gene identification-58 (CGI-58) revealed that the absence of lipolysis in BAT does not change NST (22, 23). These results suggest the existence of compensatory pathways that require further investigation.
UCP1 Is Dispensable for Thermogenesis in Thermogenic Fat
UCP1, often called thermogenin, had been thought to be the only thermogenic protein responsible for NST in thermogenic fat (24, 25). However, the Kozak group demonstrated that mixed strain F1 Ucp1 KO mice were able to adapt to cold exposure with gradual acclimation (26–30). Recently, our study revealed that, in an increased beige fat-enriched mouse model, fatty acid-binding protein (aP2)-promoter Prdm16 transgenic mice (aP2-PRDM16) transgenic × Ucp1 KO mice could maintain their temperature in a cold environment although mice totally lacking Ucp1 could not (31). This finding suggests the existence of physiologically relevant UCP1-independent thermogenesis in adipocytes.
Intriguingly, Ucp1 KO mice fed a high-fat diet (HFD) were resistant to the development of obesity at room temperature, suggesting the activation of a UCP1-independent thermogenic pathway (18, 32). Skeletal muscle has mainly been thought to contribute to UCP1-independent thermogenesis via increased capacity for shivering thermogenesis caused by chronic contractile activity (24). However, the findings of the studies on skeletal muscle UCP1-independent thermogenesis are inconsistent and the mechanism requires further investigation (24, 30, 33–36).
UCP1-Independent Thermogenic Mechanisms in Thermogenic Fat
UCP1 has been thought to be responsible for regulating the energy expenditure and glucose homeostasis of brown and beige fat. The beige fat-deficient adipocyte-specific Prdm16 KO and adipocyte-specific euchromatic histone-lysine N-methyltransferase 1 (EHMT1) KO mice have obese and diabetic phenotypes at room temperature due to insulin resistance (37, 38). However, Ucp1 KO mice do not have a diabetic phenotype and only develop an obese phenotype under thermoneutral conditions (18, 39). This discrepancy in the metabolic phenotypes of Ucp1 KO and beige fat-deficient mice suggests that brown and beige fat have UCP1-independent metabolic mechanisms that contribute to systemic energy and glucose homeostasis.
Many observations support the existence of UCP1-independent metabolic mechanisms. The inguinal WAT of Ucp1 KO mice maintained in a chronic cold environment showed greater respiration than that of Ucp1 KO mice maintained under thermoneutrality (30). In addition, chronic β3 adrenergic agonist treatment increased oxygen consumption in the epididymal WAT of Ucp1 KO mice (20). Recently, creatine-substrate cycling (28, 40, 41) and Ca2+ cycling have been identified as UCP1-independent thermogenic pathways.
Creatine-Substrate Cycling
A decline in creatine levels has been linked to the inhibition of thermal responses through unknown mechanisms in rodent models (42, 43). Kazak et al. recently found that creatine substrate cycling stimulates mitochondrial respiration and serves as a thermogenic pathway in thermogenic adipocytes (28, 41). This pathway was discovered in the mitochondria of murine beige fat. Recently, the creatine thermogenic pathway has been suggested to exist in other adipocytes because fat-specific deletion of the creatine synthesis rate-limiting enzyme glycine amidinotransferase (Gatm) reduced creatine levels in BAT and conferred mild cold intolerance (40). Global creatine transporter (Slc6a8) KO mice had similar levels of creatine reduction as the adipocyte-specific Gatm KO mice, and had an obese phenotype compared to controls (44). Similarly, creatine enzyme Ckmt1 and Ckb double KO mice showed cold intolerance and reduced norepinephrine responses to activate thermogenic respiration (45). These data support the role of the creatine substrate cycling pathway as an adaptive thermogenesis pathway in vivo.
UCP1-Independent Thermogenesis: Ca2+-Dependent ATP Hydrolysis in Brown Adipocytes and Muscle
Calcium transport contributes to NST in both BAT and muscle through sarco-endoplasmic reticulum ATPase (SERCA) activity (46–48). In muscle, Ca2+ cycling pathways involving SERCA drive thermogenesis such as malignant hyperthermia. Ca2+ cycling in the extraocular heater muscle cells of fish suggests the process may be evolutionarily conserved (49, 50).
Sarcolipin (SLN) is a direct peptide-binding SERCA that localizes to the sarcoplasmic reticulum of skeletal muscle. SLN regulates SERCA-mediated ATP turnover in muscle via Ca2+ cycling without affecting ATPase activity (51). SLN may function as an uncoupler of calcium transport from ATP hydrolysis via SERCA, which would be elevated in NST (52). The physiological role of SLN in NST is supported by several mouse studies. Sln KO animals are mildly cold-intolerant (53). In a mouse model of surgical intrascapular BAT ablation, the removal of BAT was tolerated in the setting of acute cold exposure. By contrast, intrascapular BAT ablation in Sln KO mice resulted in cold intolerance, despite the maintenance of skeletal muscle shivering (53). Moreover, Sln KO mice fed a HFD had an obese phenotype, whereas mice with muscle-specific transgenic expression of Sln fed a HFD had an obesity-resistant phenotype (54, 55). These data support the role of SLN in regulating systemic energy expenditure via calcium uncoupling (53).
UCP1-Independent Calcium Cycling Thermogenic Mechanisms in Beige Adipocytes
Our recent study revealed a new thermogenic mechanism in beige adipocytes. The newly identified UCP1-independent thermogenic mechanism depends on ATP-dependent Ca2+ cycling via SERCA2b and the Ca2+ release channel ryanodine receptor 2 (RyR2) (Figure 2) (31). Adipocyte-specific Serca2 KO mice have impaired beige adipocyte thermogenesis. Intriguingly, the SERCA2b-mediated Ca2+ cycling thermogenic mechanism is necessary for beige adipocyte thermogenesis, but dispensable in brown adipocytes. The selectivity of this pathway for beige fat relate to the ability of beige adipocytes to produce ATP due to their high expression of ATP synthase. Thus, beige adipocytes can generate heat in an ATP-dependent manner through SERCA2b-mediated Ca2+ cycling even in the absence of UCP1. Brown adipocytes express low levels of ATP synthase and cannot produce ATP due to their low ATP synthesis capacity (56).
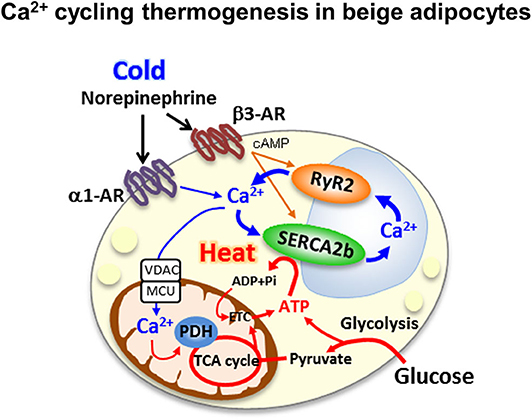
Figure 2. Ca2+ cycling thermogenesis in beige adipocytes the newly identified UCP1-independent thermogenic mechanism depends on ATP-dependent Ca2+ cycling via sacro-endoplasmic reticulumn ATPase 2b(SERCA2b) and the Ca2+ release channel ryanodine receptor 2 (RyR2).
Furthermore, our study showed that beige fat dramatically contributes to whole-body energy and glucose homeostasis via UCP1-independent metabolic mechanisms. Mice with beige fat-specific overexpression of Prdm16 driven by the aP2 promoter were protected from diet-induced obesity compared to littermate control mice (57). Furthermore, aP2-Prdm16 transgenic × Ucp1 KO mice fed a HFD were resistant to obesity, even in the absence of UCP1 (31). Importantly, both aP2-Prdm16 transgenic mice and aP2-Prdm16 transgenic × Ucp1 KO mice showed dramatically better glucose homeostasis on a HFD than mice with normal Prdm16 expression. These data strongly support the existence of a UCP1-independent metabolic mechanism in beige fat that contributes to systemic energy and glucose homeostasis. Therefore, Ca2+ cycling mediated by SERCA2–RyR2 signaling in beige adipocytes may be a potential therapeutic target for obesity and type 2 diabetes. For example, S107, a pharmacological RyR2 stabilizer that minimizes Ca2+ leak from RyR2 and increases Ca2+ loading from the endoplasmic reticulum (58), enhances Ca2+ cycling thermogenesis. S107 treatment of Ucp1 KO mice induced resistance to hypothermia upon cold exposure by activating UCP1-independent thermogenesis (31).
Nevertheless, there is concern that activation of Ca2+ cycling in vivo may have potentially harmful effects on skeletal muscle and the heart. Ryr1 mutation causes malignant hyperthermia (50), and human RYR2 gene mutations cause arrhythmogenic right ventricular cardiomyopathy type 2 and lethal arrhythmia due to catecholaminergic polymorphic ventricular tachycardia (59, 60). Given that activating systemic Ca2+ cycling may be harmful, it may be promising to activate Ca2+ cycling selectively in beige fat to treat obesity and type 2 diabetes while avoiding harmful effects on the muscle and heart.
Beyond Thermogenesis in Thermogenic Adipocytes
Recently, some studies shed light on the physiological function of beige fat to repress adipose tissue fibrosis; these findings are likely to extend beyond thermogenesis (61). Chronic cold-acclimated mice or mice with adipocyte-specific Prdm16 overexpression markedly repress adipose tissue fibrosis. Of note, this repressive effect was independent of UCP1 and independent of body weight reduction (62). The repression of adipose tissue fibrosis caused notable improvements in systemic glucose homeostasis via a UCP1-independent mechanism (62). Although the findings need to be supported by further work, it appears that beige fat can repress adipose tissue fibrosis and control whole-body glucose homeostasis. Brown and beige fat release several physiological agents, known as “batokines,” to control systemic glucose homeostasis (63–66). These data suggest that thermogenic fat has an important physiological function beyond thermogenesis.
Discussion
As thermogenic adipocytes exert multiple thermogenic mechanisms, it will be critical to determine how the regulation of the multiple mechanisms is orchestrated. SLN, a crucial calcium uncoupler, is not expressed in beige adipocytes (31); beige adipocytes must utilize an unknown regulator of the calcium system. Further work is needed to determine the regulator of SERCA2B activity and calcium uncoupler in beige adipocytes.
Brown adipocytes and beige adipocytes have common characteristics, but recent evidence indicates that they have distinct thermogenic mechanisms and functions. Of note, UCP1 is still the main regulator of thermogenesis in BAT, as revealed by numerous studies. However, emerging evidence suggests that beige fat uses UCP1-independent thermogenic pathways, which substantially contribute to systemic energy homeostasis. It will be essential to determine the coordination and contribution of the canonical (UCP1-dependent) and non-canonical (UCP1-independent) thermogenic mechanisms in adipose tissue to whole-body energy homeostasis. In particular, the newly identified UCP1-independent thermogenic pathways creatine substrate cycling and Ca2+ cycling should be evaluated as non-canonical mechanisms of thermogenesis.
Intriguingly, Ca2+ cycling-related thermogenesis seems to be evolutionarily conserved in humans and mice, and even in species that lack functional UCP1, such as pigs (31). These data suggest that UCP1-independent Ca2+ cycling thermogenesis may be the fundamental thermogenic system. Importantly, fibroblast growth factor 21 (FGF21) signaling increases intracellular Ca2+ levels in adipocytes (67) and induces browning (68). Recent evidence indicates that the anti-obesity and anti-diabetic activities of FGF21 are UCP1-independent (69, 70). Furthermore, FGF21 and UCP1 are not required for cold environment acclimation in mice (71). These findings suggest that at least some of the metabolic actions of FGF21 are mediated via UCP1-independent thermogenesis in adipose tissue.
It is of high clinical importance to determine the regulator of UCP1-independent thermogenesis because understanding the mechanism may lead to the development of new treatments for obesity and type 2 diabetes. This may be promising for treating obese and elderly populations who do not possess UCP1-positive adipocytes. The current literature suggests that it may be possible to selectively activate UCP1-independent thermogenesis, such as that mediated by Ca2+ cycling, to treat patients who lack UCP1-positive adipocytes. New tools such as “designer receptors exclusively activated by designer drugs” (DREADD) and the optogenetic tool channel rhodopsin 2 (ChR2) (72) may modulate intracellular calcium signaling pathways in adipocytes and lead to novel treatments for obesity and type 2 diabetes.
Author Contributions
KI and TY wrote the manuscript and edited the manuscript. All authors contributed to the article and approved the submitted version.
Funding
This work was supported by AMED under Grant Number JP19gm6210011, JSPS KAKENHI Grant Number 19K23745 and to KI. KI was supported Boehringer/Lilly Diabetes Research Grant in Japan Diabetes Foundation, The 2020 Inamori Research Grant Program, Kanae Foundation for the Promotion of Medical Science, the Naito Foundation, Ono Medical Research Foundation, Novartis Foundation(Japan) for the Promotion of Science, The Uehara Memorial Foundation, MSD Life Science Foundation, Public Interest Incorporated Foundation, research grant of Astellas Foundation for Research on Metabolic Disorders, Mochida Memorial Foundation for Medical and Pharmaceutical Research, The Ichiro Kanehara Foundation.
Conflict of Interest
The authors declare that the research was conducted in the absence of any commercial or financial relationships that could be construed as a potential conflict of interest.
References
1. Stanford KI, Middelbeek RJ, Goodyear LJ. Exercise effects on white adipose tissue: beiging and metabolic adaptations. Diabetes. (2015) 64:2361–8. doi: 10.2337/db15-0227
2. Petruzzelli M, Schweiger M, Schreiber R, Campos-Olivas R, Tsoli M, Allen J, et al. A switch from white to brown fat increases energy expenditure in cancer-associated cachexia. Cell Metab. (2014) 20:433–47. doi: 10.1016/j.cmet.2014.06.011
3. van Marken Lichtenbelt WD, Vanhommerig JW, Smulders NM, Drossaerts JM, Kemerink GJ, Bouvy ND, et al. Cold-activated brown adipose tissue in healthy men. N Engl J Med. (2009) 360:1500–8. doi: 10.1056/NEJMoa0808718
4. Saito M, Okamatsu-Ogura Y, Matsushita M, Watanabe K, Yoneshiro T, Nio-Kobayashi J, et al. High incidence of metabolically active brown adipose tissue in healthy adult humans: effects of cold exposure and adiposity. Diabetes. (2009) 58:1526–31. doi: 10.2337/db09-0530
5. Cypess AM, Lehman S, Williams G, Tal I, Rodman D, Goldfine AB, et al. Identification and importance of brown adipose tissue in adult humans. N Engl J Med. (2009) 360:1509–17. doi: 10.1056/NEJMoa0810780
6. Virtanen KA, Lidell ME, Orava J, Heglind M, Westergren R, Niemi T, et al. Functional brown adipose tissue in healthy adults. N Engl J Med. (2009) 360:1518–25. doi: 10.1056/NEJMoa0808949
7. Nedergaard J, Bengtsson T, Cannon B. Unexpected evidence for active brown adipose tissue in adult humans. Am J Phys Endocrinol Metab. (2007) 293:E444–52. doi: 10.1152/ajpendo.00691.2006
8. Lidell ME, Betz MJ, Dahlqvist Leinhard O, Heglind M, Elander L, Slawik M, et al. Evidence for two types of brown adipose tissue in humans. Nat Med. (2013) 19:631–4. doi: 10.1038/nm.3017
9. Wu J, Bostrom P, Sparks LM, Ye L, Choi JH, Giang AH, et al. Beige adipocytes are a distinct type of thermogenic fat cell in mouse and human. Cell. (2012) 150:366–76. doi: 10.1016/j.cell.2012.05.016
10. Sharp LZ, Shinoda K, Ohno H, Scheel DW, Tomoda E, Ruiz L, et al. Human BAT possesses molecular signatures that resemble beige/brite cells. PLoS ONE. (2012) 7:e49452. doi: 10.1371/journal.pone.0049452
11. Cypess AM, White AP, Vernochet C, Schulz TJ, Xue R, Sass CA, et al. Anatomical localization, gene expression profiling and functional characterization of adult human neck brown fat. Nat Med. (2013) 19:635–9. doi: 10.1038/nm.3112
12. Shinoda K, Luijten IH, Hasegawa Y, Hong H, Sonne SB, Kim M, et al. Genetic and functional characterization of clonally derived adult human brown adipocytes. Nat Med. (2015) 21:389–94. doi: 10.1038/nm.3819
13. Lee P, Smith S, Linderman J, Courville AB, Brychta RJ, Dieckmann W, et al. Temperature-acclimated brown adipose tissue modulates insulin sensitivity in humans. Diabetes. (2014) 63:3686–98. doi: 10.2337/db14-0513
14. van der Lans AA, Hoeks J, Brans B, Vijgen GH, Visser MG, Vosselman MJ, et al. Cold acclimation recruits human brown fat and increases nonshivering thermogenesis. J Clin Invest. (2013) 123:3395–403. doi: 10.1172/JCI68993
15. Yoneshiro T, Aita S, Matsushita M, Kayahara T, Kameya T, Kawai Y, et al. Recruited brown adipose tissue as an antiobesity agent in humans. J Clin Invest. (2013) 123:3404–8. doi: 10.1172/JCI67803
16. Hanssen MJ, Hoeks J, Brans B, van der Lans AA, Schaart G, van den Driessche JJ, et al. Short-term cold acclimation improves insulin sensitivity in patients with type 2 diabetes mellitus. Nat Med. (2015) 21:863–5. doi: 10.1038/nm.3891
17. Leitner BP, Huang S, Brychta RJ, Duckworth CJ, Baskin AS, McGehee S, et al. Mapping of human brown adipose tissue in lean and obese young men. Proc Natl Acad Sci USA. (2017) 114:8649–54. doi: 10.1073/pnas.1705287114
18. Enerback S, Jacobsson A, Simpson EM, Guerra C, Yamashita H, Harper ME, et al. Mice lacking mitochondrial uncoupling protein are cold-sensitive but not obese. Nature. (1997) 387:90–4. doi: 10.1038/387090a0
19. Lowell BB, V SS, Hamann A, Lawitts JA, Himms-Hagen J, Boyer BB, et al. Development of obesity in transgenic mice after genetic ablation of brown adipose tissue. Nature. (1993) 366:740–2. doi: 10.1038/366740a0
20. Granneman JG, Burnazi M, Zhu Z, Schwamb LA. White adipose tissue contributes to UCP1-independent thermogenesis. Am J Physiol Endocrinol Metab. (2003) 285:E1230–6. doi: 10.1152/ajpendo.00197.2003
21. Li Y, Fromme T, Schweizer S, Schottl T, Klingenspor M. Taking control over intracellular fatty acid levels is essential for the analysis of thermogenic function in cultured primary brown and brite/beige adipocytes. EMBO Rep. (2014) 15:1069–76. doi: 10.15252/embr.201438775
22. Schreiber R, Diwoky C, Schoiswohl G, Feiler U, Wongsiriroj N, Abdellatif M, et al. Cold-induced thermogenesis depends on ATGL-mediated lipolysis in cardiac muscle, but not brown adipose tissue. Cell Metab. (2017) 26:753–63 e7. doi: 10.1016/j.cmet.2017.09.004
23. Shin H, Ma Y, Chanturiya T, Cao Q, Wang Y, Kadegowda AKG, et al. Lipolysis in brown adipocytes is not essential for cold-induced thermogenesis in mice. Cell Metab. (2017) 26:764–77 e5. doi: 10.1016/j.cmet.2017.09.002
24. Golozoubova V, Hohtola E, Matthias A, Jacobsson A, Cannon B, Nedergaard J. Only UCP1 can mediate adaptive nonshivering thermogenesis in the cold. FASEB J. (2001) 15:2048–50. doi: 10.1096/fj.00-0536fje
25. Nedergaard J, Golozoubova V, Matthias A, Asadi A, Jacobsson A, Cannon B. UCP1: the only protein able to mediate adaptive non-shivering thermogenesis and metabolic inefficiency. Biochim Biophys Acta. (2001) 1504:82–106. doi: 10.1016/S0005-2728(00)00247-4
26. Hofmann WE, Liu X, Bearden CM, Harper ME, Kozak LP. Effects of genetic background on thermoregulation and fatty acid-induced uncoupling of mitochondria in UCP1-deficient mice. J Biol Chem. (2001) 276:12460–5. doi: 10.1074/jbc.M100466200
27. Golozoubova V, Cannon B, Nedergaard J. UCP1 is essential for adaptive adrenergic nonshivering thermogenesis. Am J Physiol Endocrinol Metab. (2006) 291:E350–7. doi: 10.1152/ajpendo.00387.2005
28. Kazak L, Chouchani ET, Jedrychowski MP, Erickson BK, Shinoda K, Cohen P, et al. A creatine-driven substrate cycle enhances energy expenditure and thermogenesis in beige fat. Cell. (2015) 163:643–55. doi: 10.1016/j.cell.2015.09.035
29. Keipert S, Kutschke M, Lamp D, Brachthauser L, Neff F, Meyer CW, et al. Genetic disruption of uncoupling protein 1 in mice renders brown adipose tissue a significant source of FGF21 secretion. Mol Metab. (2015) 4:537–42. doi: 10.1016/j.molmet.2015.04.006
30. Ukropec J, Anunciado RP, Ravussin Y, Hulver MW, Kozak LP. UCP1-independent thermogenesis in white adipose tissue of cold-acclimated Ucp1-/- mice. J Biol Chem. (2006) 281:31894–908. doi: 10.1074/jbc.M606114200
31. Ikeda K, Kang Q, Yoneshiro T, Camporez JP, Maki H, Homma M, et al. UCP1-independent signaling involving SERCA2b-mediated calcium cycling regulates beige fat thermogenesis and systemic glucose homeostasis. Nat Med. (2017) 23:1454–65. doi: 10.1038/nm.4429
32. Liu X, Rossmeisl M, McClaine J, Riachi M, Harper ME, Kozak LP. Paradoxical resistance to diet-induced obesity in UCP1-deficient mice. J Clin Invest. (2003) 111:399–407. doi: 10.1172/JCI200315737
33. Meyer CW, Willershauser M, Jastroch M, Rourke BC, Fromme T, Oelkrug R, et al. Adaptive thermogenesis and thermal conductance in wild-type and UCP1-KO mice. Am J Physiol Regul Integr Comp Physiol. (2010) 299:R1396–406. doi: 10.1152/ajpregu.00021.2009
34. Mineo PM, Cassell EA, Roberts ME, Schaeffer PJ. Chronic cold acclimation increases thermogenic capacity, non-shivering thermogenesis and muscle citrate synthase activity in both wild-type and brown adipose tissue deficient mice. Comp Biochem Physiol A, Mol Integr Physiol. (2012) 161:395–400. doi: 10.1016/j.cbpa.2011.12.012
35. Monemdjou S, Hofmann WE, Kozak LP, Harper ME. Increased mitochondrial proton leak in skeletal muscle mitochondria of UCP1-deficient mice. Am J Physiol Endocrinol Metab. (2000) 279:E941–6. doi: 10.1152/ajpendo.2000.279.4.E941
36. Shabalina IG, Hoeks J, Kramarova TV, Schrauwen P, Cannon B, Nedergaard J. Cold tolerance of UCP1-ablated mice: a skeletal muscle mitochondria switch toward lipid oxidation with marked UCP3 up-regulation not associated with increased basal, fatty acid- or ROS-induced uncoupling or enhanced GDP effects. Biochim Biophys Acta. (2010) 1797:968–80. doi: 10.1016/j.bbabio.2010.02.033
37. Ohno H, Shinoda K, Ohyama K, Sharp LZ, Kajimura S. EHMT1 controls brown adipose cell fate and thermogenesis through the PRDM16 complex. Nature. (2013) 504:163–7. doi: 10.1038/nature12652
38. Cohen P, Levy JD, Zhang Y, Frontini A, Kolodin DP, Svensson KJ, et al. Ablation of PRDM16 and beige adipose causes metabolic dysfunction and a subcutaneous to visceral fat switch. Cell. (2014) 156:304–16. doi: 10.1016/j.cell.2013.12.021
39. Feldmann HM, Golozoubova V, Cannon B, Nedergaard J. UCP1 ablation induces obesity and abolishes diet-induced thermogenesis in mice exempt from thermal stress by living at thermoneutrality. Cell Metab. (2009) 9:203–9. doi: 10.1016/j.cmet.2008.12.014
40. Kazak L, Chouchani ET, Lu GZ, Jedrychowski MP, Bare CJ, Mina AI, et al. Genetic depletion of adipocyte creatine metabolism inhibits diet-induced thermogenesis and drives obesity. Cell Metab. (2017) 26:693. doi: 10.1016/j.cmet.2017.09.007
41. Bertholet AM, Kazak L, Chouchani ET, Bogaczynska MG, Paranjpe I, Wainwright GL, et al. Mitochondrial patch clamp of beige adipocytes reveals UCP1-positive and UCP1-negative cells both exhibiting futile creatine cycling. Cell Metab. (2017) 25:811–22.e4. doi: 10.1016/j.cmet.2017.03.002
42. Wakatsuki T, Hirata F, Ohno H, Yamamoto M, Sato Y, Ohira Y. Thermogenic responses to high-energy phosphate contents and/or hindlimb suspension in rats. Japan J Physiol. (1996) 46:171–5. doi: 10.2170/jjphysiol.46.171
43. Yamashita H, Ohira Y, Wakatsuki T, Yamamoto M, Kizaki T, Oh-ishi S, et al. Increased growth of brown adipose tissue but its reduced thermogenic activity in creatine-depleted rats fed beta-guanidinopropionic acid. Biochim Biophys Acta. (1995) 1230:69–73. doi: 10.1016/0005-2728(95)00067-S
44. Perna MK, Kokenge AN, Miles KN, Udobi KC, Clark JF, Pyne-Geithman GJ, et al. Creatine transporter deficiency leads to increased whole body and cellular metabolism. Amino acids. (2016) 48:2057–65. doi: 10.1007/s00726-016-2291-3
45. Streijger F, Pluk H, Oerlemans F, Beckers G, Bianco AC, Ribeiro MO, et al. Mice lacking brain-type creatine kinase activity show defective thermoregulation. Physiol Behav. (2009) 97:76–86. doi: 10.1016/j.physbeh.2009.02.003
46. de Meis L. Brown adipose tissue Ca2+-ATPase: uncoupled ATP hydrolysis and thermogenic activity. J Biol Chem. (2003) 278:41856–61. doi: 10.1074/jbc.M308280200
47. de Meis L, Arruda AP, da Costa RM, Benchimol M. Identification of a Ca2+-ATPase in brown adipose tissue mitochondria: regulation of thermogenesis by ATP and Ca2+. J Biol Chem. (2006) 281:16384–90. doi: 10.1074/jbc.M600678200
48. Periasamy M, Maurya SK, Sahoo SK, Singh S, Sahoo SK, Reis FCG, et al. Role of SERCA pump in muscle thermogenesis and metabolism. Compreh Physiol. (2017) 7:879–90. doi: 10.1002/cphy.c160030
49. Block BA. Thermogenesis in muscle. Annu Rev Physiol. (1994) 56:535–77. doi: 10.1146/annurev.ph.56.030194.002535
50. Rosenberg H, Pollock N, Schiemann A, Bulger T, Stowell K. Malignant hyperthermia: a review. Orphanet J Rare Dis. (2015) 10:93. doi: 10.1186/s13023-015-0310-1
51. Smith WS, Broadbridge R, East JM, Lee AG. Sarcolipin uncouples hydrolysis of ATP from accumulation of Ca2+ by the Ca2+-ATPase of skeletal-muscle sarcoplasmic reticulum. Biochem J. (2002) 361(Pt 2):277–86. doi: 10.1042/bj3610277
52. Sahoo SK, Shaikh SA, Sopariwala DH, Bal NC, Periasamy M. Sarcolipin protein interaction with sarco(endo)plasmic reticulum Ca2+ ATPase (SERCA) is distinct from phospholamban protein, and only sarcolipin can promote uncoupling of the SERCA pump. J Biol Chem. (2013) 288:6881–9. doi: 10.1074/jbc.M112.436915
53. Bal NC, Maurya SK, Sopariwala DH, Sahoo SK, Gupta SC, Shaikh SA, et al. Sarcolipin is a newly identified regulator of muscle-based thermogenesis in mammals. Nat Med. (2012) 18:1575–9. doi: 10.1038/nm.2897
54. Rowland LA, Maurya SK, Bal NC, Kozak L, Periasamy M. Sarcolipin and uncoupling protein 1 play distinct roles in diet-induced thermogenesis and do not compensate for one another. Obesity (Silver Spring). (2016) 24:1430–3. doi: 10.1002/oby.21542
55. Maurya SK, Bal NC, Sopariwala DH, Pant M, Rowland LA, Shaikh SA, et al. Sarcolipin is a key determinant of the basal metabolic rate, and its overexpression enhances energy expenditure and resistance against diet-induced obesity. J Biol Chem. (2015) 290:10840–9. doi: 10.1074/jbc.M115.636878
56. Kramarova TV, Shabalina IG, Andersson U, Westerberg R, Carlberg I, Houstek J, et al. Mitochondrial ATP synthase levels in brown adipose tissue are governed by the c-Fo subunit P1 isoform. FASEB J. (2008) 22:55–63. doi: 10.1096/fj.07-8581com
57. Seale P, Conroe HM, Estall J, Kajimura S, Frontini A, Ishibashi J, et al. Prdm16 determines the thermogenic program of subcutaneous white adipose tissue in mice. J Clin Invest. (2011) 121:96–105. doi: 10.1172/JCI44271
58. Andersson DC, Marks AR. Fixing ryanodine receptor Ca leak - a novel therapeutic strategy for contractile failure in heart and skeletal muscle. Drug Disc Today Dis Mech. (2010) 7:e151–e7. doi: 10.1016/j.ddmec.2010.09.009
59. Tiso N, Stephan DA, Nava A, Bagattin A, Devaney JM, Stanchi F, et al. Identification of mutations in the cardiac ryanodine receptor gene in families affected with arrhythmogenic right ventricular cardiomyopathy type 2 (ARVD2). Hum Mol Genet. (2001) 10:189–94. doi: 10.1093/hmg/10.3.189
60. Marks AR, Priori S, Memmi M, Kontula K, Laitinen PJ. Involvement of the cardiac ryanodine receptor/calcium release channel in catecholaminergic polymorphic ventricular tachycardia. J Cell Physiol. (2002) 190:1–6. doi: 10.1002/jcp.10031
61. Kajimura S, Spiegelman BM, Seale P. Brown and beige fat: physiological roles beyond heat generation. Cell Metab. (2015) 22:546–59. doi: 10.1016/j.cmet.2015.09.007
62. Hasegawa Y, Ikeda K, Chen Y, Alba DL, Stifler D, Shinoda K, et al. Repression of adipose tissue fibrosis through a PRDM16-GTF2IRD1 complex improves systemic glucose homeostasis. Cell Metab. (2018) 27:180–94 e6. doi: 10.1016/j.cmet.2017.12.005
63. Svensson KJ, Long JZ, Jedrychowski MP, Cohen P, Lo JC, Serag S, et al. A secreted Slit2 fragment regulates adipose tissue thermogenesis and metabolic function. Cell Metab. (2016) 23:454–66. doi: 10.1016/j.cmet.2016.01.008
64. Long JZ, Svensson KJ, Bateman LA, Lin H, Kamenecka T, Lokurkar IA, et al. The secreted enzyme PM20D1 regulates lipidated amino acid uncouplers of Mitochondria. Cell. (2016) 166:424–35. doi: 10.1016/j.cell.2016.05.071
65. Wang GX, Zhao XY, Meng ZX, Kern M, Dietrich A, Chen Z, et al. The brown fat-enriched secreted factor Nrg4 preserves metabolic homeostasis through attenuation of hepatic lipogenesis. Nat Med. (2014) 20:1436–43. doi: 10.1038/nm.3713
66. Leiria LO, Wang CH, Lynes MD, Yang K, Shamsi F, Sato M, et al. 12-lipoxygenase regulates cold adaptation and glucose metabolism by producing the omega-3 lipid 12-HEPE from brown fat. Cell Metab. (2019). 30:768–83 e7. doi: 10.1016/j.cmet.2019.07.001
67. Moyers JS, Shiyanova TL, Mehrbod F, Dunbar JD, Noblitt TW, Otto KA, et al. Molecular determinants of FGF-21 activity-synergy and cross-talk with PPARgamma signaling. J Cell Physiol. (2007) 210:1–6. doi: 10.1002/jcp.20847
68. Fisher FM, Kleiner S, Douris N, Fox EC, Mepani RJ, Verdeguer F, et al. FGF21 regulates PGC-1alpha and browning of white adipose tissues in adaptive thermogenesis. Genes Dev. (2012) 26:271–81. doi: 10.1101/gad.177857.111
69. Veniant MM, Sivits G, Helmering J, Komorowski R, Lee J, Fan W, et al. Pharmacologic effects of FGF21 are independent of the “browning” of white adipose tissue. Cell Metab. (2015) 21:731–8. doi: 10.1016/j.cmet.2015.04.019
70. Samms RJ, Smith DP, Cheng CC, Antonellis PP, Perfield JW, 2nd, Kharitonenkov A, et al. Discrete aspects of FGF21 in vivo pharmacology do not require UCP1. Cell Rep. (2015) 11:991–9. doi: 10.1016/j.celrep.2015.04.046
71. Keipert S, Kutschke M, Ost M, Schwarzmayr T, van Schothorst EM, Lamp D, et al. Long-term cold adaptation does not require FGF21 or UCP1. Cell Metab. (2017) 26:437–46.e5. doi: 10.1016/j.cmet.2017.07.016
Keywords: thermogenic fat, brown adipocyte, beige adipocyte, adipogenesis, uncoupling protein 1
Citation: Ikeda K and Yamada T (2020) UCP1 Dependent and Independent Thermogenesis in Brown and Beige Adipocytes. Front. Endocrinol. 11:498. doi: 10.3389/fendo.2020.00498
Received: 20 March 2020; Accepted: 23 June 2020;
Published: 28 July 2020.
Edited by:
Matthias Johannes Betz, University Hospital of Basel, SwitzerlandReviewed by:
Vibha Singhal, Massachusetts General Hospital, United StatesMarco Infante, University of Miami, United States
Copyright © 2020 Ikeda and Yamada. This is an open-access article distributed under the terms of the Creative Commons Attribution License (CC BY). The use, distribution or reproduction in other forums is permitted, provided the original author(s) and the copyright owner(s) are credited and that the original publication in this journal is cited, in accordance with accepted academic practice. No use, distribution or reproduction is permitted which does not comply with these terms.
*Correspondence: Kenji Ikeda, kikeda.mem@tmd.ac.jp