- 1Section on Cellular Signaling, The Eunice Kennedy Shriver National Institute of Child Health and Human Development, National Institutes of Health, Bethesda, MD, United States
- 2Laboratory of Biological Modeling, National Institute of Diabetes, Digestive and Kidney Diseases, National Institutes of Health (NIH), Bethesda, MD, United States
- 3Section on Molecular Signal Transduction, The Eunice Kennedy Shriver National Institute of Child Health and Human Development, National Institutes of Health, Bethesda, MD, United States
The role of calcium, but not of other intracellular signaling molecules, in the release of pituitary hormones by exocytosis is well established. Here, we analyzed the contribution of phosphatidylinositol kinases (PIKs) to calcium-driven prolactin (PRL) release in pituitary lactotrophs: PI4Ks - which control PI4P production, PIP5Ks - which synthesize PI(4, 5)P2 by phosphorylating the D-5 position of the inositol ring of PI4P, and PI3KCs – which phosphorylate PI(4, 5)P2 to generate PI(3, 4, 5)P3. We used common and PIK-specific inhibitors to evaluate the strength of calcium-secretion coupling in rat lactotrophs. Gene expression was analyzed by single-cell RNA sequencing and qRT-PCR analysis; intracellular and released hormones were assessed by radioimmunoassay and ELISA; and single-cell calcium signaling was recorded by Fura 2 imaging. Single-cell RNA sequencing revealed the expression of Pi4ka, Pi4kb, Pi4k2a, Pi4k2b, Pip5k1a, Pip5k1c, and Pik3ca, as well as Pikfyve and Pip4k2c, in lactotrophs. Wortmannin, a PI3K and PI4K inhibitor, but not LY294002, a PI3K inhibitor, blocked spontaneous action potential driven PRL release with a half-time of ~20 min when applied in 10 µM concentration, leading to accumulation of intracellular PRL content. Wortmannin also inhibited increase in PRL release by high potassium, the calcium channel agonist Bay K8644, and calcium mobilizing thyrotropin-releasing hormone without affecting accompanying calcium signaling. GSK-A1, a specific inhibitor of PI4KA, also inhibited calcium-driven PRL secretion without affecting calcium signaling and Prl expression. In contrast, PIK93, a specific inhibitor of PI4KB, and ISA2011B and UNC3230, specific inhibitors of PIP5K1A and PIP5K1C, respectively, did not affect PRL release. These experiments revealed a key role of PI4KA in calcium-secretion coupling in pituitary lactotrophs downstream of voltage-gated and PI(4, 5)P2-dependent calcium signaling.
Introduction
Elevation in cytoplasmic calcium concentrations ([Ca2+]i) is the primary intracellular signal that controls the fusion of secretory vesicles with the plasma membrane to release hormones and neurotransmitters. This process, called regulated exocytosis, is also controlled by a complex protein system, which is preserved in organisms ranging from yeast to mammals. These proteins participate in docking, ATP-dependent priming, and fusion of secretory vesicle membranes via molecular interactions that have not yet been fully characterized (1, 2). Both calcium influx and calcium mobilization pathways can trigger the secretion of hormones and neurotransmitters, and the strength of the calcium-secretion coupling depends on local and global [Ca2+]i, as determined by the proximity of the secretory vesicles to calcium influx and/or intracellular calcium release channels (3).
Phosphoinositides are low abundance cellular membrane lipids that can influence regulated exocytosis (4, 5). They are generated by phosphorylation on the inositol headgroup of phosphatidylinositol (PI), to yield PI4P, PI(4, 5)P2, and PI(3, 4, 5)P3. PI4P is produced by four different PI4-kinases (PI4KA, PI4KB, PI4K2A, and PI4K2B), and each of these enzymes is associated with a different aspect of cell physiology (6). Three PI4P 5-kinases (PIP5K1A, PIP5K1B, PIP5K1C) account for the conversion of PI4P into PI(4, 5)P2 (7). An additional route for the production of PI(4, 5)P2 includes the phosphorylation of PI5P by PI5P4-kinases (8, 9). PI(4, 5)P2 is well known to contribute to calcium-secretion coupling as being the substrate for receptor activated phospholipase C to yield inositol 1,4,5 trisphosphate (IP3) and thereby controlling calcium mobilization. PI(4, 5)P2 also directly modulates ion channels and transporters and the accompanying calcium influx (10), and augments calcium-secretion coupling downstream of calcium signaling (11, 12). Class I PI3 kinases (PI3KCA, PI3KCB, PI3KCG, and PI3KCD) may also influence the level of PI(4, 5)P2 in the plasma membrane, using it as a substrate for the synthesis of PI(3, 4, 5)P3 (13), which has been implicated in calcium-secretion coupling as well (14).
Endocrine pituitary cells secrete hormones using both calcium signaling pathways: spontaneous voltage-gated calcium influx and receptor-controlled IP3-dependent calcium mobilization coupled with facilitated voltage-gated calcium influx (15). Pituitary lactotrophs, which are the subject of the present study, exhibit spontaneous plateau-bursting of electrical activity resulting in calcium transients that have sufficient amplitude to trigger basal prolactin (PRL) secretion (16). In these cells, the physiological mechanism for control of PRL release is via dopamine suppression of basal secretion (17). Furthermore, thyrotropin-releasing hormone (TRH) triggers IP3-dependent calcium mobilization from intracellular stores and induces additional hormone secretion (18). However, the contribution of phosphoinositides beyond the role of PI(4, 5)P2 supporting IP3 production in pituitary cell exocytosis remains poorly understood. This reflects the difficulties to up- or down-regulate the expression of enzymes that control phosphoinositide levels in cultured pituitary cells, due to resistance of these cells to conventional transfection procedures. The use of immortalized pituitary cells, such as GH3 lacto-somatotrophs, is also limited, because these cells predominantly secrete hormones in a constitutive manner.
This prompted us to use a pharmacological approach in this initial study of the role of phosphoinositides in calcium-secretion coupling in cultured pituitary lactotrophs, focusing on PI4P, PI(4, 5)P2, and PI(3, 4, 5)P3. We used wortmannin (Wm), a common PI3K inhibitor, which is effective against Class I PI3Ks in the low nanomolar concentration range (19), and against PI4KA and PI4KB in the low to high micromolar concentration range (20). Another inhibitor of a different chemical class, LY294002, also inhibits PI3K activity (21, 22). PIK93 used below 1 µM inhibits PI4KB but not PI4KA (23), while several other compounds, including GSK-A1, are potent and selective PI4KA inhibitors developed by GSK (Glaxo-Smith-Kline) using quinazolinone 28 as the starting compound (24) and characterized by Bojjireddy et al. (25). We also used IS2011B and UNC3230, reported inhibitors of PIP5K1A (26) and PIP5K1C (27), respectively. Our results revealed that inhibition of PI4Ks, but not other kinases, block PRL release downstream of calcium signaling. Additional experiments with PI4K-specific inhibitors, revealed that PI4KA is a key enzyme in establishing calcium-secretion coupling in pituitary lactotrophs.
Material And Methods
Materials
Phosphate buffered saline (PBS), medium-199, rat ELISA kits for GH, horse serum, penicillin, and streptomycin were purchased from Life Technologies (Grand Island, NY, USA). Fura 2-AM, the secondary Alexa Fluor 568 donkey anti-rabbit antibody, and 4′,6-diamidino-2-phenylindole (DAPI) were from Invitrogen (Eugene, OR). Rodent LH ELISA Kit was from Endocrine Technologies (Newark, CA). The primary antibody and standard for the PRL radioimmunoassay as well as the rabbit anti-PRL antibody for immunocytochemistry were from the National Pituitary Agency and Dr. A. F. Parlow (Harbor-UCLA Medical Center, Torrance, CA). 125I-Prolactin was from Perkin Elmer Life Sciences (Boston, MA). Bovine serum albumin (BSA) fraction V and saponin were from MP Biomedicals (Solon, OH). The transcriptor first strand cDNA synthesis kit was from Roche Applied Sciences (Indianapolis, IN), Formaldehyde solution was from Thermo Fisher Scientific (Rockford, IL); Bay K8644, PIK93, UNC3230, and Wm were from Tocris (Bristol, UK). ISA2011B was from Med Chem Express (Monmouth Junction, NJ). TRH and dopamine were from Bachem Americas (Vista, CA). Unless stated otherwise, all other chemicals were obtained from Sigma (St. Louis, MO, USA).
Animals, Cell Preparation/Culturing and Treatments
Experiments were performed on cultured anterior pituitary cells from normal 75-day-old female Sprague-Dawley rats obtained from Taconic Farms (Germantown, NY). Animals were housed under constant conditions of temperature and humidity, with lights on between 6 AM and 8 PM and water and food ad libitum. Euthanasia was performed by asphyxiation with CO2, and the anterior pituitary glands were removed after decapitation. Pituitary tissue was cut into 0.5 x 0.5 mm pieces and treated with trypsin, followed by trypsin inhibitor, and mechanically dispersed as previously described (28). Freshly dispersed pituitary cells were cultured in medium 199 containing Earle’s salts, sodium bicarbonate and supplemented with 10% heat-inactivated horse serum, penicillin (100 units/ml), and streptomycin (100 µg/ml). For immunocytochemistry, 50,000 cells/well were plated on poly-L-lysine-coated eight-well multitest slides (MP Biomedicals, Aurora, OH). For secretory studies, cells were cultured in biocoated 24-well plates (Corning, Kennebunk, ME), 0.25 million per well or in 60 mm petri dishes (Falcon/Becton Dickinson, Franklin lakes, NJ) containing pre-swollen Cytodex-1 beads, 12 million per dish. For RNA extraction, cells were seeded on poly-L-lysine-coated 24-well plates, 1.5 million per well. For calcium experiments, cells were plated at a density of 0.7 million per 25 mm glass coverslip (Thomas Scientific, Logan Township, NJ) coated with poly-D-lysine. In all cases, cells were cultured for approximatively 20 h, washed and treated with several PI4K, PIP5K, and PI3K inhibitors or solvents (Figure 1A). All experimental procedures were in accordance with the National Institutes of Health Policy Manual 3040-2: Animal Care and Use in the Intramural Program and were approved by the National Institute of Child Health and Human Development, Animal Care and Use Committee (Animal Protocol 19-041).
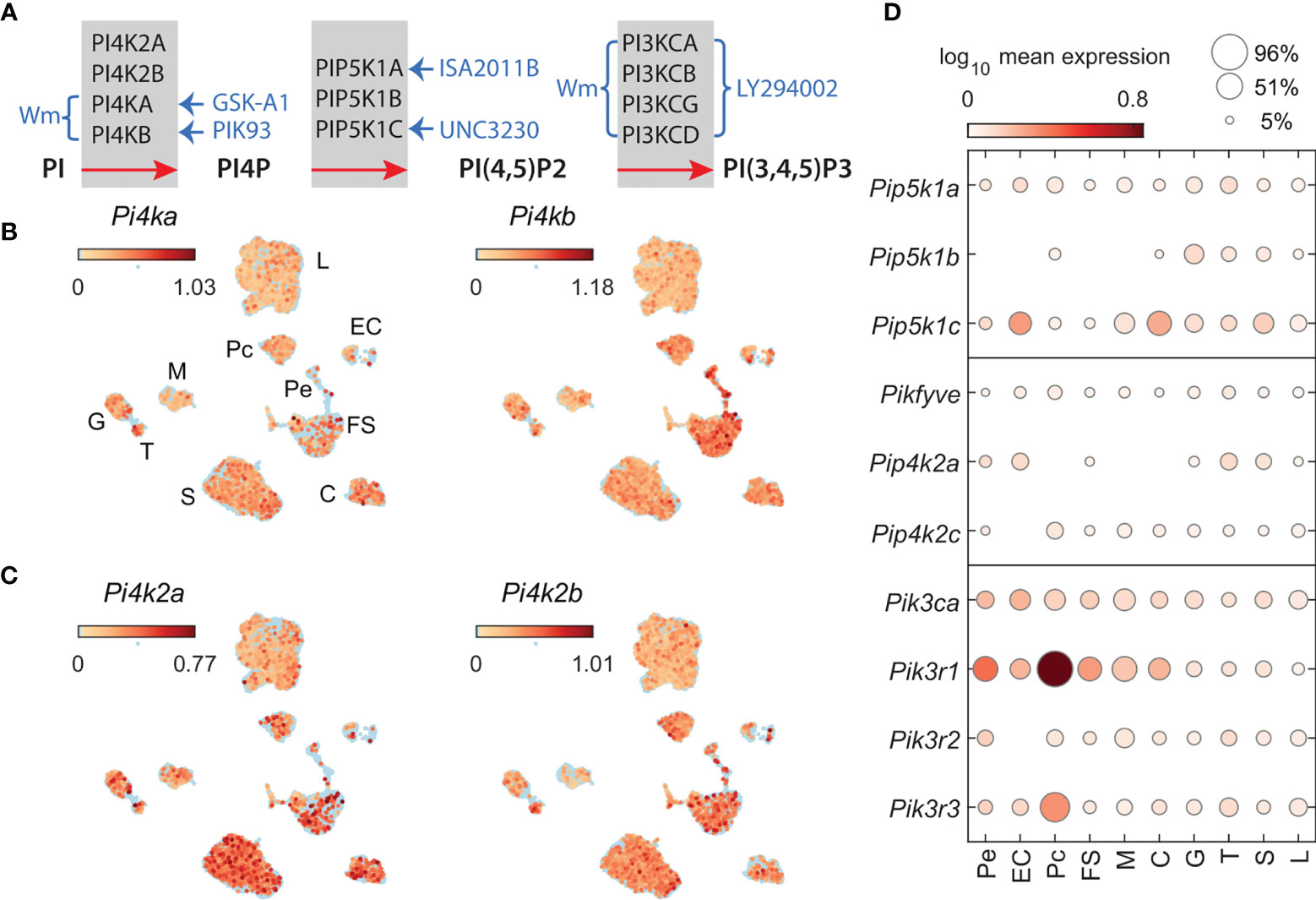
Figure 1 Phosphatidylinositol kinases involved in the generation of PI4P, PI (4,5)P2, and PI (3,4,5)P3 and kinase inhibitors used in this study. (A) Inhibition of PIKs by drugs; braces and arrows indicate the enzymes inhibited by inhibitors (blue text). Wortmannin (Wm), a fungal metabolite, is a cell-permeable, irreversible inhibitor of phosphatidylinositol-4-kinases PI4KA and PI4KB in a micromolar concentration range (20) and phosphadidylinositol-3-kinases (PI3Ks) in a nanomolar concentration range (29). PI3KCs are also inhibited by LY294002 in a micromolar concentration range (30). PI4KA is inhibited by GSK-A1 (25) and PI4KB by PIK93 (31), both in a nanomolar concentration range. ISA2011B blocks PIP5K1A (26), whereas UNC3230 inhibits PIP5K1C (27). If not otherwise indicated, we used 10 µM Wm, 100 nM GSK-A1, 100 nM PIK93, 10 µM ISA2011B, 1 µM UNC3230, and 1 and 50 µM LY2394002. Enzymes involved in PI5P production, PIKfyve, a FYVE finger-containing PIK, and PI5P-dependent PI (4,5)P2 production, PIP4K2, phosphatidylinositol-5-phosphate 4-kinases type-2, and their inhibitors, are not shown. (B, C) The expression pattern of PIK genes in scRNAseq of freshly dispersed rat pituitary cells. Uniform manifold approximation and projection (UMAP) plots showing the expression of P4ka and Pi4kb (B) and Pi4k2a and Pi4k2b (C) in pituitary cells: L, lactotrophs; G, gonadotrophs; T, thyrotrophs; S, somatotrophs; C, corticotrophs; M, melanotrophs; FS, folliculostellate cells; Pe, pericytes; Pc, pituicytes; and EC, endothelial cells. (D), Cell-type-specific expression of Pip5k1a-c (top panel); Pikfyve, Pip4k2a, Pip4k2c (middle panel); Pik3ca, Pik3r1, Pik3r2, and Pik3r3 (bottom panel), shown as mean expression level (color code) and percentage of pituitary cell types expressing these genes (area of circles).
Single-Cell RNA Sequencing Analysis
Dispersed cells from two cell preparations were used for single-cell RNA sequencing (scRNAseq) analysis, as described in Fletcher et al. (32); one preparation was done by dispersion of 30 whole pituitary glands, and the other dispersion was done with 30 separate anterior and intermediate/posterior lobes of the pituitary gland. The whole pituitary sample was run in duplicate, and the anterior and posterior samples were run in separate lanes on a 10X Genomics chromium controller according to manufacturer instructions. The resulting libraries were sequenced on an Illumina HiSeq 2500, and the Cellranger pipeline (10X Genomics) was used for read mapping and transcript counting. A total of 32,455 droplets were recovered from the four lanes. A custom reference genome, cell filtering, differential expression analysis, and ambient RNA removal by SoupX were used as previously described (33). The final data set contained 15,876 cells, for which cell type clusters were identified using known genetic markers: Lum, Col3a1, and C7 for pericytes; Plvap, Kdr, and Flt1 for endothelial cells; Lhx2, Col25a1, and Fgf10 for pituicytes, Mt2A, Aldh3a1, and Capn6 for folliculostellate cells; Pomc for melanotrophs and corticotrophs, with melanotroph-specific Oacyl, Pax7, and Pcsk2, and corticotroph-specific Doc2g, Avpr1b, and Crhr1; Lhb, Gnrhr, and Nr5a1 for gonadotrophs; Pou1f1 for thyrotroph, somatotrophs and lactotrophs, Trhr for thyrotrophs and lactotrophs, with thyrotroph-specific Tshb and Kcnk9, somatotroph-specific Gh1, Ghrhr, and Arhgap36 for somatotrophs, and Prl, Drd2, and Agtr1b for lactotrophs. As expected, the majority of pituicytes and melanotrophs were detected in the posterior pituitary preparation, and pericytes and endothelial cells were present in both the anterior and posterior pituitary preparations. In this study, we use cell-type-specific clustering to examine the expression levels of selected PI3K, PI4K, and PIP5K genes and the percentage of cells of each cell type expressing these genes. Expression of genes of interest was plotted using uniform manifold approximation and projection (UMAP, 34) and percent-expression dot plots using MATLAB (R2018b; The MathWorks, Natick, MA). Single cell RNA sequencing data are accessible at NCBI Gene Expression Omnibus database, accession GSE184319.
Immunocytochemistry
After overnight incubation, 8-well multitest slides were washed, cells were treated with Wm or 0.1% DMSO for 1, 2, or 3 h, washed twice with PBS, and fixed with cold 4% formaldehyde solution for 10 min. From this point, every step of immunostaining protocol was followed by washing cells three times with PBS. Cells were incubated with rabbit anti-PRL antibody (1:2000), followed by subsequent incubation with secondary antibody (1:1000) for 30 min at room temperature. All antibodies were diluted in PBS containing 0.2% saponin and 0.5% BSA. Cells were mounted with Flouromount-G, with DAPI. All images were acquired on an inverted confocal laser-scanning microscope (LSM 780; Carl Zeiss GmbH, Jena, Germany), using a 63x oil objective. Micrographs were sized, and their brightness and contrast levels adjusted in Fiji image processing software (35). Cells were counted on 8 tile-scan images from 2 independent experiments (3x3).
qRT-PCR Analysis
Total RNA was extracted from dispersed anterior pituitary cells using RNeasy Plus Mini Kit and reverse transcribed with the Transcriptor First Strand cDNA Synthesis Kit. qRT-PCR was performed in the presence of cDNA (2 ng), TaqMan™ Fast Advanced Master Mix, and TaqMan Gene Expression Assays using the Quantum Studio 6 Flex Real Time PCR System. Target gene expression levels were determined by the comparative 2^-(delta Ct) quantification method using Gapdh as the reference gene, which was previously established to be suitable for anterior pituitary tissue (36). Applied Biosystems pre-designed TaqMan Gene Expression Assays were used: prolactin (Prl, 00561791_m1) and glyceraldehyde 3-phosphate dehydrogenase gene (Gapdh; Rn01462662_g1).
Single Cell Calcium Measurements
For measurements of intracellular calcium concentration ([Ca2+]i), cultured pituitary cells were washed and bathed in Krebs-Ringer-like medium containing 2.5 μM Fura 2 AM for 1 h at room temperature. The coverslips were washed in Krebs-Ringer-like medium and mounted on the stage of an inverted Observer-D1 microscope (Carl Zeiss, Oberkochen, Germany) with an attached ORCA-ER camera (Hamamatsu Photonics, Hamamatsu City, Japan) and a Lambda DG-4 wavelength switcher (Sutter, Novato, CA). Hardware control and image analysis were performed using Metafluor software (Molecular Devices, Downingtown, PA). Experiments were performed under a 40X oil-immersion objective during exposure to alternating 340 and 380 nm excitation beams, and the intensity of light emission at 520 nm was followed simultaneously in approximately 20 single cells. Changes in [Ca2+]i are represented by the ratio of fluorescence intensities F340/F380. Single lactotrophs in the mixed population of pituitary cells were identified by their responses to both TRH (100 nM) and dopamine (1 µM), in contrast to thyrotrophs responding only to TRH (100 nM).
Secretory Studies
Two types of secretory experiments were performed: static incubations and perfused pituitary cells. After overnight incubation, static cultures were washed with medium 199 supplemented with warm HEPES-containing medium-199 supplemented with 0.1% BSA and penicillin and streptomycin to estimate basal PRL release in the presence of phosphatidylinositol kinase (PIK) inhibitors or solvents used as controls. Cells were incubated at 37°C for 1 h, or in time-course studies for 1-3 h; afterwards, medium was removed to measure secreted hormones. To analyze changes in the intracellular content of PRL in these experiments, 0.5 ml of ice-cold 20 mM sodium carbonate buffer was added to wells, and plates were frozen at -80°C. Next, plates were scraped using a 1 ml pipette tip and contents transferred to individual tubes. The process of scraping was repeated with 0.5 ml of fresh buffer or ethanol and added to the tube containing the first extract. Cell extracts were centrifuged at 3,000 rpm to remove cell debris. Samples were stored at -20°C until analysis. In perfusion experiments, both basal and stimulated (25 mM KCl, 1 µM Bay K8644, or 100 nM TRH) release of PRL were studied. After overnight incubation, beads with attached pituitary cells were transferred to 37°C-heated chambers and continuously perfused with warm HEPES-containing medium-199 supplemented with 0.1% BSA and penicillin and streptomycin for 2 h at a flow rate of 0.5 ml/min to establish stable basal secretion. Basal and stimulated PRL release was studied in the presence of PIK inhibitors or solvents, which were continuously applied to cells, and 1- or 3-min fractions of perfused medium were collected and stored at -20°C until hormone measurements. In most experiments, PRL (released and cell content) was measured by radioimmunoassay (RIA) as previously described (37). In some experiments, released PRL, LH, and GH were measured by ELISA, following the manufacturers’ instructions. Released hormones were expressed as ng/ml and cell content of hormones as µg/well.
Data and Statistical Analysis
Results are presented as representative traces (perfusion experiments) and mean ± SEM values (static incubations and selected perfusion experiments). Statistical analysis was performed by Kruskal-Wallis and Wilcoxon tests, with at least P < 0.01 deemed as statistically significant. Mean and SEM values, statistical analysis, half-maximal inhibitory concentrations (IC50) and time to half-maximal inhibition (τ50) values were calculated using the KaleidaGraph program (Synergy Software, Reading, PA).
Results
Expression of PIK Genes in Anterior Pituitary Cells
To characterize the expression of PIK genes in lactotrophs and other pituitary cell types, we examined the expression levels of these genes using scRNAseq of freshly dispersed anterior and posterior pituitary cells of adult female rats. The UMAP plots shown in Figure 1 indicate that four PI4K genes, Pi4ka, Pi4kb (B) Pi4k2a, and Pi4k2b (C), are expressed in lactotrophs and other hormone-producing cells (somatotrophs, gonadotrophs, thyrotrophs, corticotrophs and melanotrophs) as well as in non-hormonal pituitary cell types (folliculostellate cells, pituicytes, pericytes, and endothelial cells). Among cell types, the percentage of cells expressing these genes varied between 3 and 40, with a mean ± SEM value for all cell types of 18 ± 1.4%. In the lactotroph population, these mRNAs were detected in 14-27% of cells. Among PIP5K1 genes, Pip5k1c was expressed at the highest level, followed by Pip5k1a and Pip5k1b in all cell types, including lactotrophs (Figure 1D, top panel). Pikfyve and Pip4k2c genes, encoding proteins that can support an alternative synthetic pathway of PI(4, 5)P2, are also expressed in lactotrophs and other pituitary cell types (Figure 1D, middle panel). Among PI3KC genes, only Pik3ca reached the threshold for detection by scRNAseq, whereas all three regulatory subunit genes (Pik3r1, Pik3r2, and Pik3r3) were detected in lactotrophs and other cell types (Figure 1D, bottom panel). PI3KCA is tightly associated with a regulatory subunit encoded by the p85alpha gene with three splice forms: p85alpha, p55alpha, and p50alpha (38). However, our scRNAseq system does not discriminate between the various splice forms.
Wortmannin Inhibits Basal PRL Release
Basal pituitary hormone release comprises regulated exocytosis driven by spontaneous action potential (AP) driven calcium signaling and hormone released by constitutive exocytosis. To study basal hormone release, pituitary cells isolated from adult female rats attached to beads were perfused for 2 h without treatment to wash out previously released hormones, followed by collection of perfusate every 3 min during a 25 min period. This was followed by continuous application of Wm (10 µM in 0.1% DMSO) or solvent (0.1% DMSO) till the end of the experiment (Figure 2). Basal PRL, GH, and LH release, measured from the same samples by ELISA, was: 69 ± 3, 18 ± 2, and 5 ± 0.5 ng/ml, respectively. Application of 10 µM Wm caused a rapid decline in PRL release, with an estimated τ50 of ~20 min (Figure 2A), whereas basal GH (B) and LH (C) release were not affected by this inhibitor.
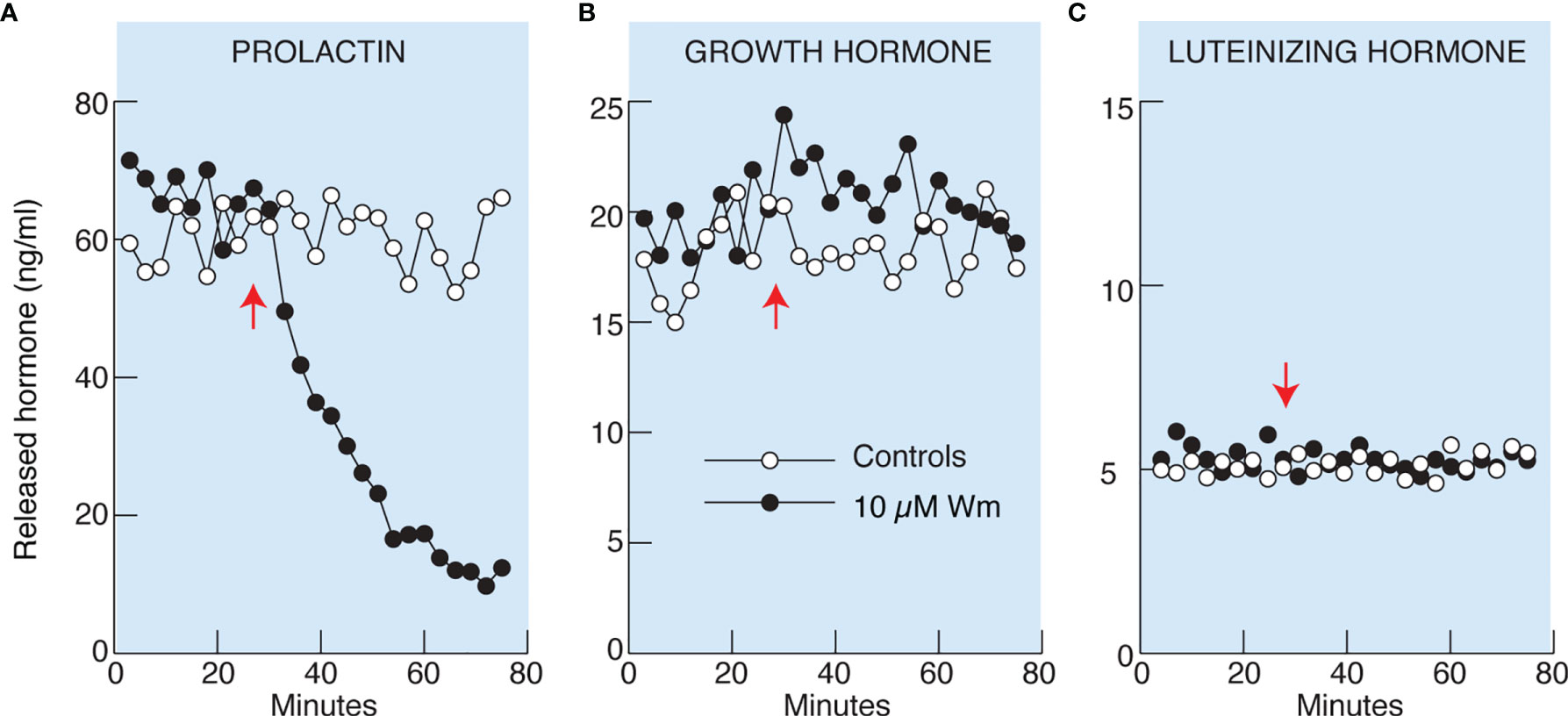
Figure 2 Wortmannin (Wm) inhibited basal prolactin (A), but not growth hormone (B) and luteinizing hormone (C), secretion in perfused rat anterior pituitary cells. Freshly dispersed pituitary cells were attached to beads and cultured for 20 hours before the experiments. Perfusion was performed in 0.5 ml volume chambers at a flow rate of 0.5 ml/min and the cells were washed for 2 hours before collecting samples. After the wash period, samples were collected every three minutes for 76 minutes. Vertical arrows indicate the time of application of 10 µM Wm (black circles) or solvent (white circles). Hormone content of the effluent was measured by ELISA. Data shown are representative from three (A) and two (B, C) similar experiments. For 10 µM Wm-induced inhibition of basal PRL release see also Figures 4C, 5B, C, 7C, D, 8B, and 9D.
Immunofluorescence analysis revealed no significant differences in the percentage of lactotrophs during 3 h incubation with 10 µM Wm, when compared to cultures treated with solvent: controls, 1-3 h = 33.7 ± 2.1 (n = 22); Wm, 1 h = 35.7 ± 1.77 (n = 7), Wm, 2 h = 34.9 ± 3.2 (n = 7), and Wm, 3 h = 32.1 ± 2.7 (n = 8). Figure 3 shows PRL-positive cells in controls and cells treated with Wm for 3 h. Therefore, the cell-type specific action of Wm on basal hormone release in cultured pituitary cells was not caused by selective loss of lactotrophs to Wm but was a genuine effect on PRL secretion.
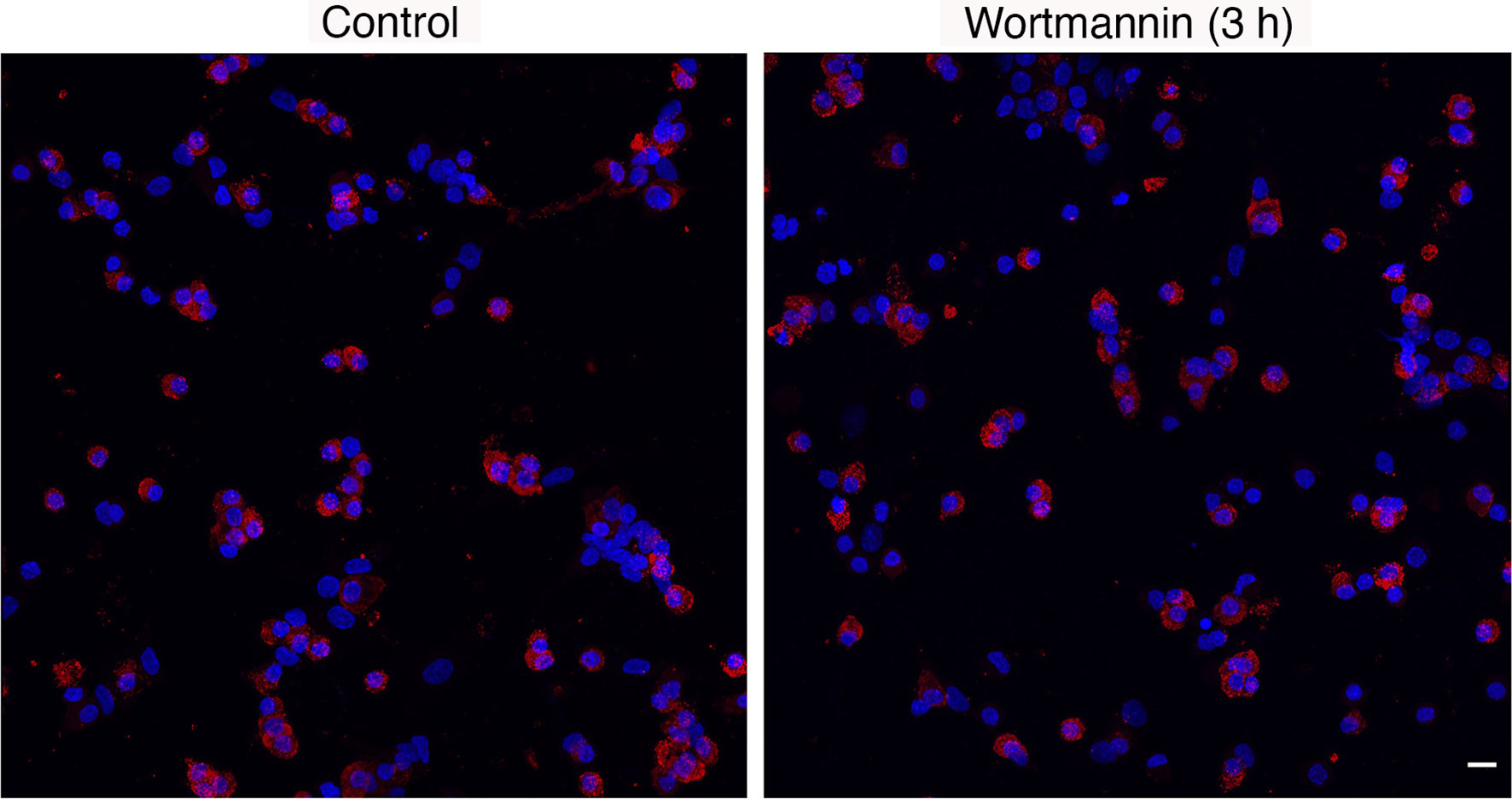
Figure 3 Immunofluorescence analysis of prolactin protein expression in control and Wm (10 µM/3h)-treated pituitary cells. Red indicates prolactin-positive cells; blue indicates cell nuclei stained with DAPI. Scale bar applies to both images: 10 µM. The mean ± SEM values of the percentage of PRL-positive cells in controls was 33.7 ± 2.1 (n = 8) and in Wm-treated cultures was 32.1 ± 2.7 (n = 8).
PI4KA Mediates the Effect of Wm on Basal PRL Release
In further experiments, cultured cells in static incubations were treated with various Wm concentrations for 3 h, and PRL was measured in medium by RIA. Figure 4A illustrates that Wm inhibits basal PRL release in the 1 – 10 µM concentration range, with an estimated IC50 of ~5.5 µM, and was ineffective in the nanomolar concentration range. In perfused pituitary cells, Wm was ineffective at 0.1 µM but inhibited basal PRL release when administrated at 1 and 10 µM (Figure 4B). Therefore, inhibition of basal PRL release induced by Wm at concentration of 10 µM was detected by both methods, ELISA and RIA, confirming the specificity of PRL measurements. The inhibitory Wm action on the basal PRL release was consistently observed, as shown in Figures 4C, 5B, C, 7C, D, and 9D. Basal PRL release in pituitary cells derived from adult male rats was also inhibited by 10 µM Wm (data not shown).
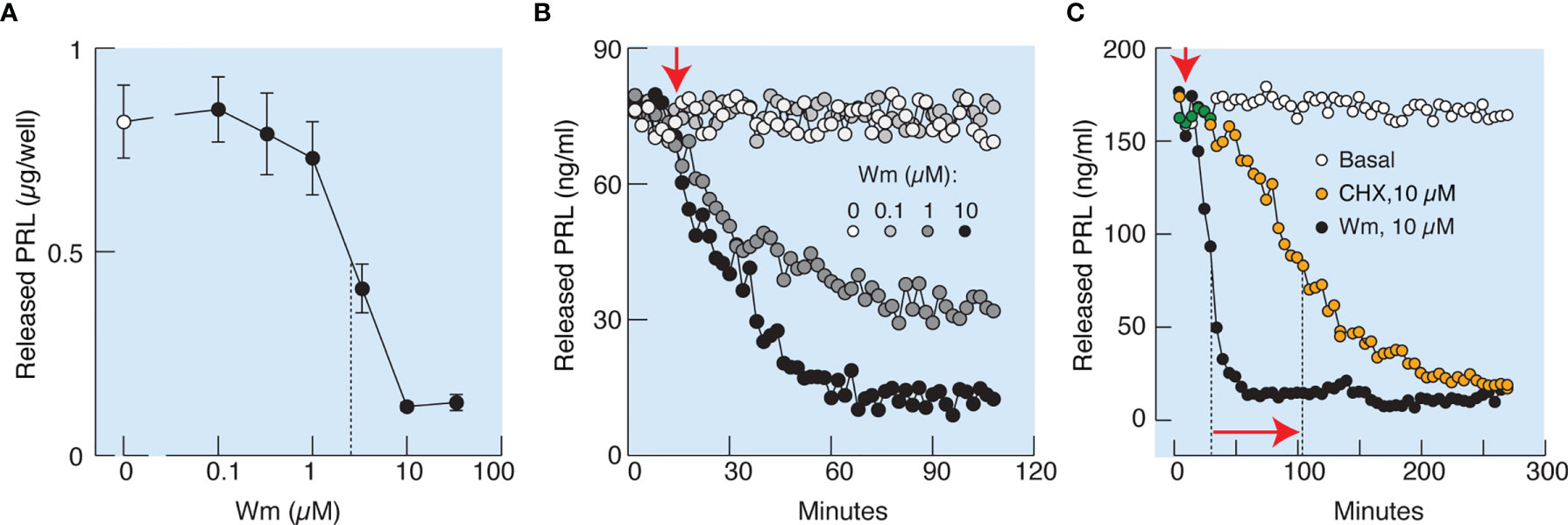
Figure 4 Characterization of Wm-inhibition of basal prolactin secretion in cultured anterior pituitary cells. (A, B) Concentration-dependence of Wm effect on prolactin (PRL) release in pituitary cells in static cultures during 3 h incubation (A) and in perfused pituitary cells (B). Vertical dotted line in (A) indicates the IC50 value. In subsequent experiments, Wm was used at 10 µM concentration. (C) Comparison of the time-course of effects of Wm (black circles) and cycloheximide (CHX), a protein synthesis inhibitor (orange circles), on basal PRL release. Note the different time scale of this experiment vs. panel (B). Vertical dotted lines indicate time points when 50% decrease of hormone secretion was reached, horizontal arrow indicates the difference in half-times, and vertical arrow indicates the beginning of continuous treatments with Wm and CHX. Data points shown in panel A are mean ± SEM of sextuplicate incubation and data shown in panels (B, C) are representative from two experiments. In this and following figures, PRL was measured by radioimmunoassay, unless stated otherwise.
Wm inhibits Class I PI3Ks in the low nanomolar concentration range (19) and PI4Ks in the low to high micromolar concentration range (20). Among the four known PI4Ks, only PI4KA and PI4KB are inhibited by Wm (20). PI4KA is specifically inhibited by GSK-A1 and PI4KB by PIK93 (Figure 1A). In static cultures of anterior pituitary cells, GSK-A1 inhibited basal PRL release in a concentration-dependent manner, with an IC50 of ~10 nM (Figure 5A). In perfused pituitary cells, the rate and level of inhibition of basal PRL release by 100 nM GSK-A1 was highly comparable to the inhibition induced by 10 µM Wm (Figure 5B). In contrast, 1 µM PIK93 was not able to replicate the inhibitory action of Wm on basal PRL release (Figure 5C). Likewise, the PI3K inhibitor LY294002, did not affect basal PRL release in perfused pituitary cells when applied in 1 and 50 µM for 35 min (Figure 5D). Furthermore, ISA2011B (10 µM) and UNC3230 (1 µM), inhibitors of PIP5K1A and PIP5K1C, respectively (26, 27), were ineffective to inhibit basal PRL release when applied alone or together during 2 h (Figure 5E) and 3h (Figure 5F) incubations. In the same experiments, GSK-A1 significantly (P < 0.01) inhibited PRL release during 2 and 3 h of incubation. Therefore, the inhibitory effects of Wm and GSK-A1 at such concentration ranges suggest a role of PI4KA, but not PI4KB, PI3Ks or PIP5Ks, in basal PRL release, reflecting the effects of the drug on the exocytotic pathway and/or on de novo PRL synthesis.
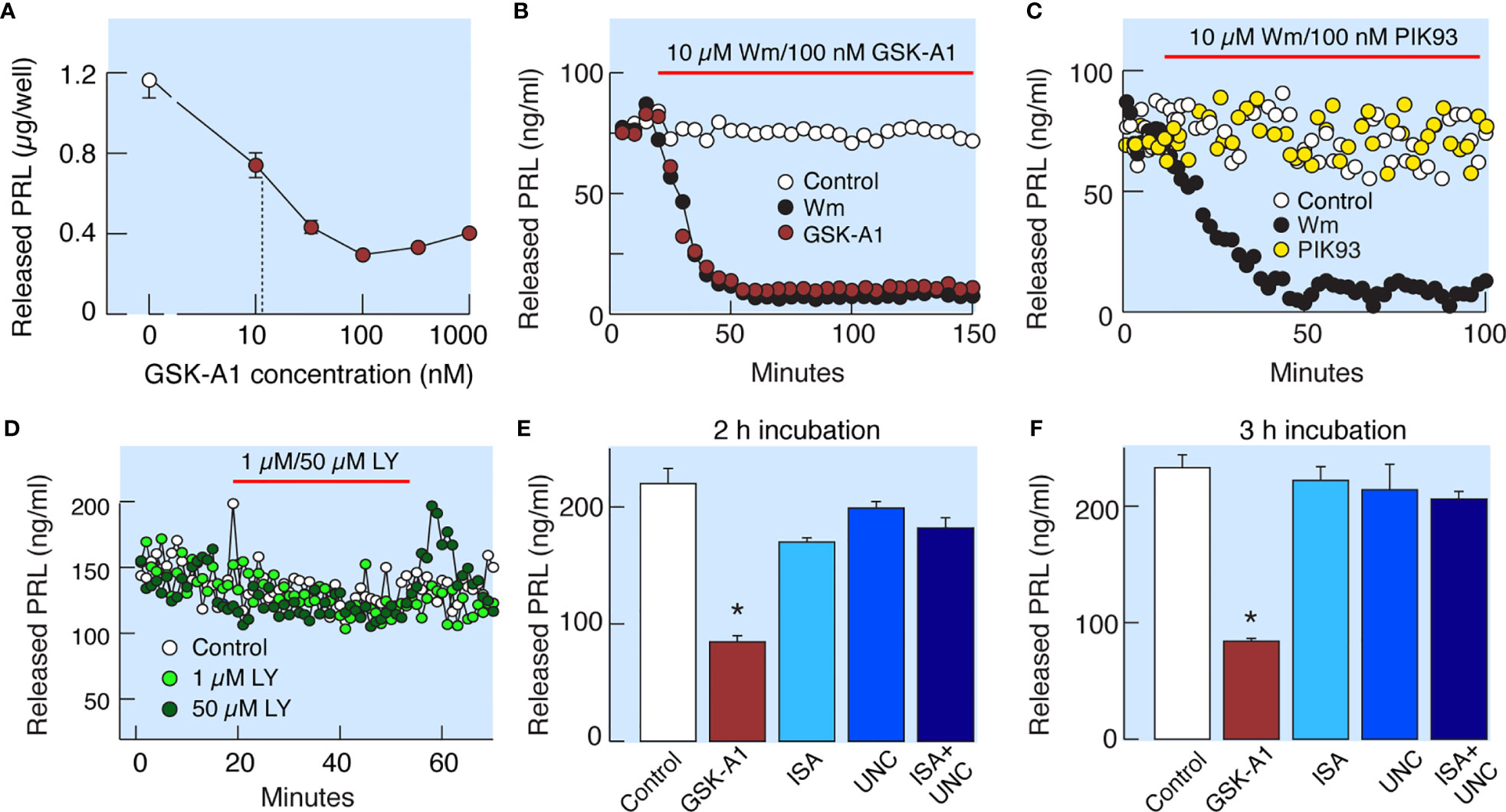
Figure 5 Wm-induced inhibition of PI4KA accounts for inhibition of basal PRL release by Wm in anterior pituitary cells. (A) Concentration-dependence of GSK-A1, a PI4KA-sprecific inhibitor, on basal PRL release in static cultures of pituitary cells during 3-h incubation. In subsequent experiments, GSK-A1 was used at a concentration of 100 nM. (B, C) In perfused pituitary cells, the inhibitory effect of Wm on PRL release was fully mimicked by application of GSK-A1 (B), but not by PIK93, a PI4KB-specific inhibitor (C). (D) Lack of effects of LY294002 (LY), an inhibitor of PI3KC and other PI3Ks, on basal PRL release. In this and following figures, horizontal red bars indicate the duration of treatments with inhibitors and solvents (controls). (E, F) Decrease in basal PRL release by addition of PI4KA but not PIP5K1 inhibitors. Cultured cells were incubated with 100 nM GSK-A1, 10 µM ISA2011B (ISA), 1 µM UNC3230 (UNC), and 10 µM ISA2011B plus 1 µM UNC3230 for 2 h (E) and 3 h (F). Data points shown in panels (A, E, F) are mean ± SEM of sextuplicate incubation; *P < 0.002 vs control. The released PRL was measured by RIA (A–D) and ELISA (E, F). For time course of GSK-A1 effects on basal PRL release see also Figure 8B, left, and 9C. The lack of effects of PIK93 on basal PRL release is also shown in Figures 9C, D.
PI4KA Controls Basal PRL Release Without Affecting De Novo PRL Synthesis
To elucidate whether the effects of Wm and GSK-A1 on basal PRL release reflect a direct action on the exocytotic pathway or an indirect action through blockade of de novo PRL synthesis, we performed three experiments. In the first experiment, we compared the rates of effects of Wm and cycloheximide, a protein synthesis inhibitor, on basal PRL release in perfused pituitary cells. These experiments, shown in Figure 4C, illustrate that the rate of Wm-induced reduction in basal PRL release was faster than the rate of reduction induced by cycloheximide, treatment, suggesting that the exocytotic pathway, rather than PRL synthesis, was blocked by Wm. In the second experiment, we examined the time-course of 10 µM Wm and 100 nM GSK-A1 effects on PRL release (Figure 6A) and intracellular PRL content (Figure 6B) in static incubation. Both compounds inhibited basal PRL release in a similar time-dependent manner, but the blockade of PRL release was accompanied by increase in intracellular PRL cell content. In third experiment, we examined Prl expression (Figure 6C) in Wm- (top) and GSK-A1 (bottom) treated cells. Neither treatment significantly affected the expression of this gene during 1, 2, or 3 h incubation. These results indicate that inhibition of PI4KA blocks the exocytotic pathway without affecting de novo PRL synthesis.
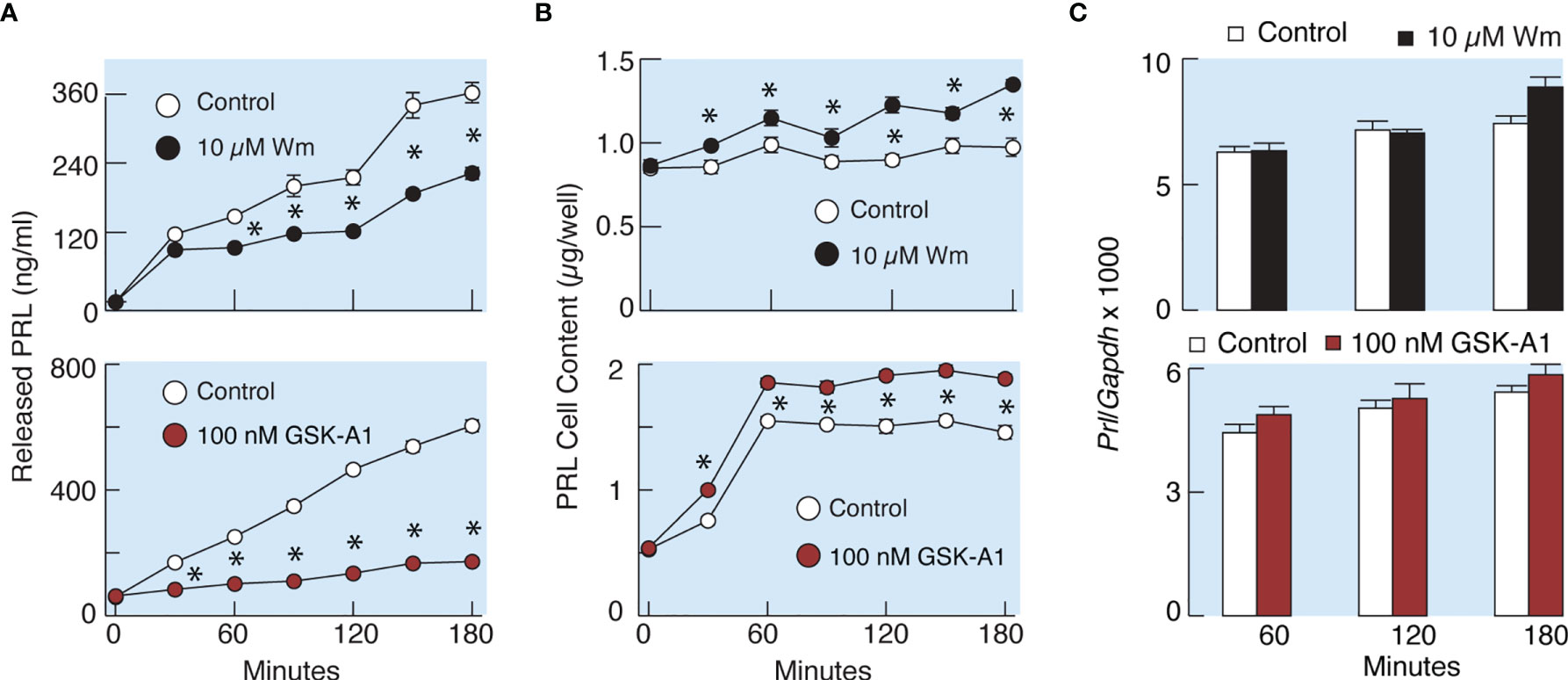
Figure 6 Wm and GSK-A1 induced inhibition of PRL release are not caused by loss of intracellular PRL content and inhibition of PRL gene (Prl) expression. (A) Time course of Wm- (top) and GSK-A1 (bottom) induced inhibition of basal PRL release. (B) Time course of Wm (top) and GSK-A1 (bottom) induced accumulation of intracellular PRL content. (C) Lack of effects of Wm (top) and GSK-A1 (bottom) on Prl expression in cultured anterior pituitary cells. The experiments were performed in static incubation of cultured anterior pituitary cells: 0.25 million/well (A, B) and 1.5. million/well (C). Data points shown are mean ± SEM of sextuplicate incubation; *P < 0.002 between pairs.
PI4KA Controls Stimulated PRL Release Downstream of Calcium Signaling
In theory, inhibition of PI4KA could cause blockade of spontaneous voltage-gated calcium influx, leading to inhibition of PRL release, or may inhibit the exocytotic pathway downstream of voltage-gated calcium signaling. To clarify this issue, we facilitated voltage-gated calcium influx by the addition of 1 µM Bay K8644, an L-type calcium channel agonist (Figure 7A), or by depolarization of the cells by 25 mM KCl (Figure 7B) in control cells (left panels) and cells pretreated with 10 µM Wm for 45 min (right panels). In the presence of Bay K8644 or high potassium, 100 nM TRH was added at the end of recording to estimate the potential effects of Wm on calcium mobilizing action of this agonist, which is dependent on the presence of PI (4,5)P2 in the plasma membrane (39). These experiments revealed that stimulation of calcium influx was not affected by 45 min application of 10 µM Wm. The experiments further showed no difference in the pattern of TRH-induced calcium mobilization in Wm-treated cells (Figures 7A, B). This is an important finding, as it indicates that Wm treatment did not decrease PI (4,5)P2 levels, in agreement with findings in other cell types that Wm and GSK-A1 treatments do not decrease PI (4,5)P2 levels in the absence of phospholipase C activation (40).
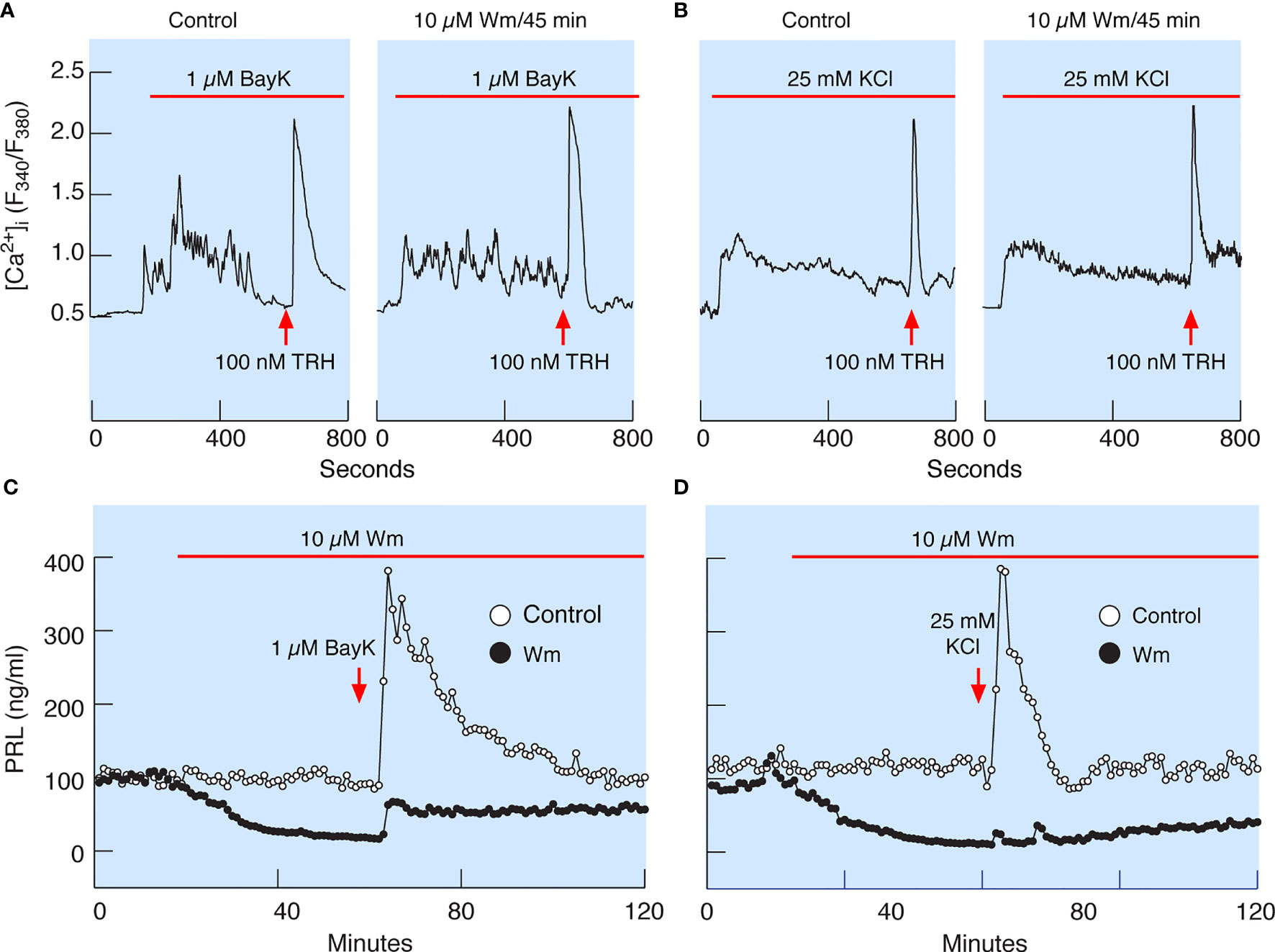
Figure 7 Wm-induced inhibition of PRL release occurs downstream of spontaneous and augmented voltage-gated calcium influx. (A) Bay K8644 (BayK), an L-type calcium channel agonist, induced rise in cytosolic calcium concentration ([Ca2+]i), which was not affected by treatment of cells with 10 µM Wm for 45 min. (B) Facilitation of voltage-gated calcium influx by high K+-induced depolarization of cells was also not affected by treating the cells with 10 µM Wm for 45 min. Horizontal red bars indicate the duration of BayK and high K+ treatments, and the arrows indicate the time of application of thyrotropin-releasing hormone (TRH) in the absence or presence of Wm. In addition to TRH, cells were stimulated with 1 µM dopamine, to identify lactotrophs (not shown). Traces shown are representative from three independent experiments. (C, D) Although Wm pretreatment did not affect the facilitated voltage-gated calcium influx, the stimulatory effect of BayK (C) and high K+ (D) on PRL release was dramatically reduced in pituitary cells perfused with Wm-containing medium for 45 minutes. The mean ± SEM values for PRL release (ng/ml) during the first 20 min of BayK treatments in the absence and presence of Wm in four experiments were: 210 ± 13 vs. 42 ± 2.4; 146 ± 10 vs. 31 ± 1; 184 ± 11 vs. 35 ± 2; and 481 ± 24 vs. 193 ± 11. The mean ± SEM values for two high potassium experiments in the absence and presence of Wm were 173 ± 21 vs. 18 ± 1; and 255 ± 172 vs. 5 ± 3. In all cases, P < 0.0001.
Consistent with calcium-secretion coupling in lactotrophs, facilitation of voltage-gated calcium influx by Bay K8644 rapidly increased PRL release in perfused control cells, followed by a prolonged decay in secretion to basal levels (Figure 7C). Depolarization of control cells by high potassium also increased PRL release but was followed by a faster return to basal secretion levels (Figure 7D). However, in cells treated with 10 µM Wm there was a progressive decay in PRL release, and application of Bay K8644 only slightly recovered PRL release, with a peak in response below the basal levels in controls (Figure 7C). High potassium was practically ineffective in elevating PRL release in Wm-treated cells (Figure 7D).
Similarly, 100 nM GSK-A1 administrated over 45 min did not affect Bay K8644-stimulated voltage-gated calcium influx and TRH-induced calcium mobilization. (Figure 8A). TRH receptors are expressed in lactotrophs and thyrotrophs, but only lactotrophs respond to application of dopamine administration (Figure 8A, left). In contrast to calcium signaling, GSK-A1 dramatically reduced Bay K8644-stimulated PRL release in perfused pituitary cells (Figure 8B, left). When Wm and Bay K8644 were added at the same time, however, no inhibition of the early spike of PRL release was observed (Figure 8B, right), suggesting that blockade of calcium-secretion coupling takes time to develop, likely because it takes time for PI4P levels to decrease after blockade of PI4KA (41).
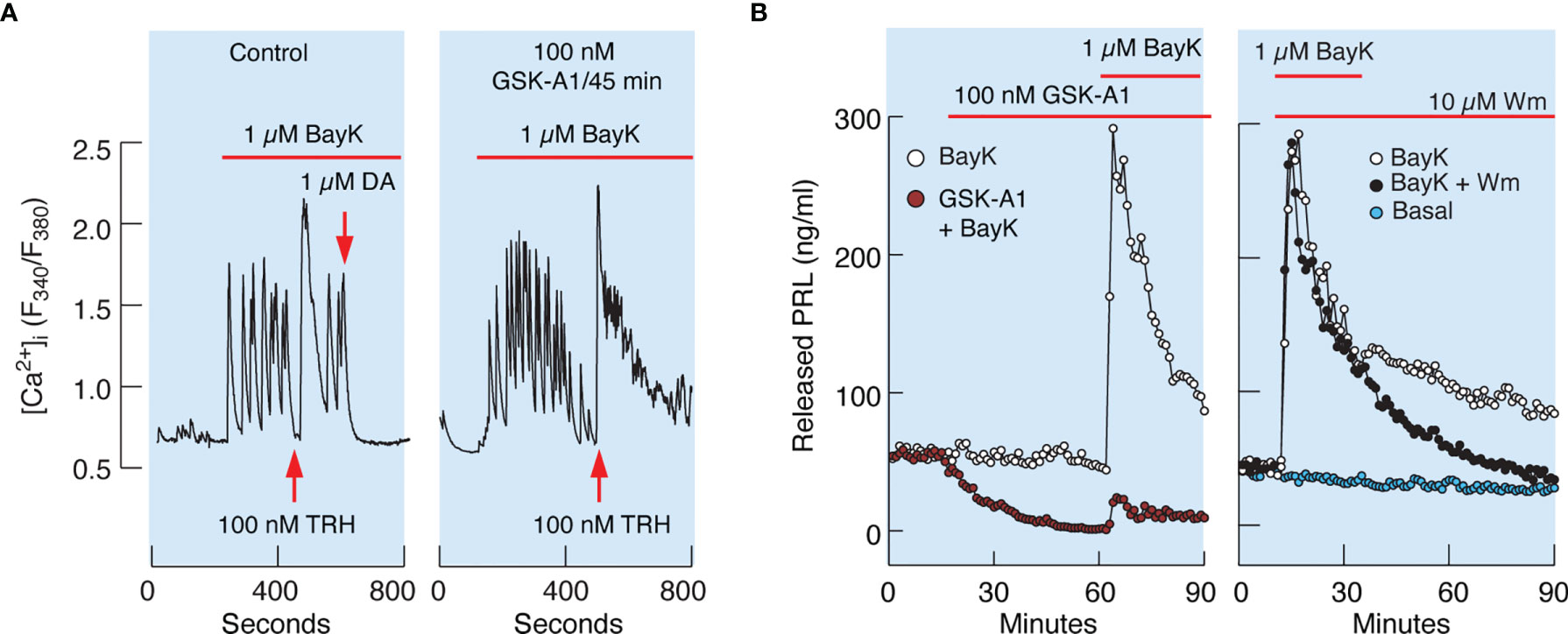
Figure 8 Inhibition of PI4KA by GSK-A1 causes a decrease in BayK-induced PRL release downstream of facilitated voltage-gated calcium influx. (A) BayK-stimulated voltage-gated calcium influx (left) and lack of effect of GSK-A1 on the BayK-induced rise in [Ca2+]i (right). Time of addition of TRH and Dopamine (DA) is indicated by the arrows. Traces shown are representative from three independent experiments. (B, left) In cells treated with GSK-A1, basal PRL release was progressively reduced and BayK was unable to fully restore calcium–secretion coupling. Mean ± SEM values (ng/ml) during 20 min application: BayK 186 ± 12 vs. GSK-A1+BayK = 14 ± 1; P < 0.0001. (B, right), When Wm and Bay K were applied together, the initial increase in PRL secretion was comparable to that of BayK-induced PRL release; mean ± SEM values (ng/ml) during 20 min application were 182 ± 11 (w/o Wm) vs. 176 ± 10 (with Wm). However, in Wm-treated cells, BayK-stimulated PRL release gradually declined, with a rate comparable to that of the inhibition of basal PRL release shown in the left panel, in contrast to control cells.
It has been well established that TRH-stimulated calcium mobilization from the intracellular pool increases the release of PRL in vitro (18). As mentioned earlier, TRH-stimulated calcium mobilization was not affected by prolonged (45 min) treatment with 10 µM Wm and 100 nM GSK-A1 (Figures 7 and 8). In general, TRH-induced calcium signaling consist of an early spike response, which reflects calcium mobilization from intracellular pools, and sustained calcium transients, reflecting extracellular calcium influx (18, 39). Both calcium signaling phases were unaffected in cells treated with GSK-A1 for 45 min (Figures 9A, B). Dopamine abolished sustained intracellular calcium transients, confirming that they were driven by voltage-gated calcium channels and that the selected cells were lactotrophs (Figures 9A, B). Additional administration of TRH in the presence of dopamine shows the independence of the spike phase from calcium influx in the controls (Figure 9A) and that the blockade of PI4KA does not affect the repeated mobilization of calcium from intracellular stores (Figure 9B).
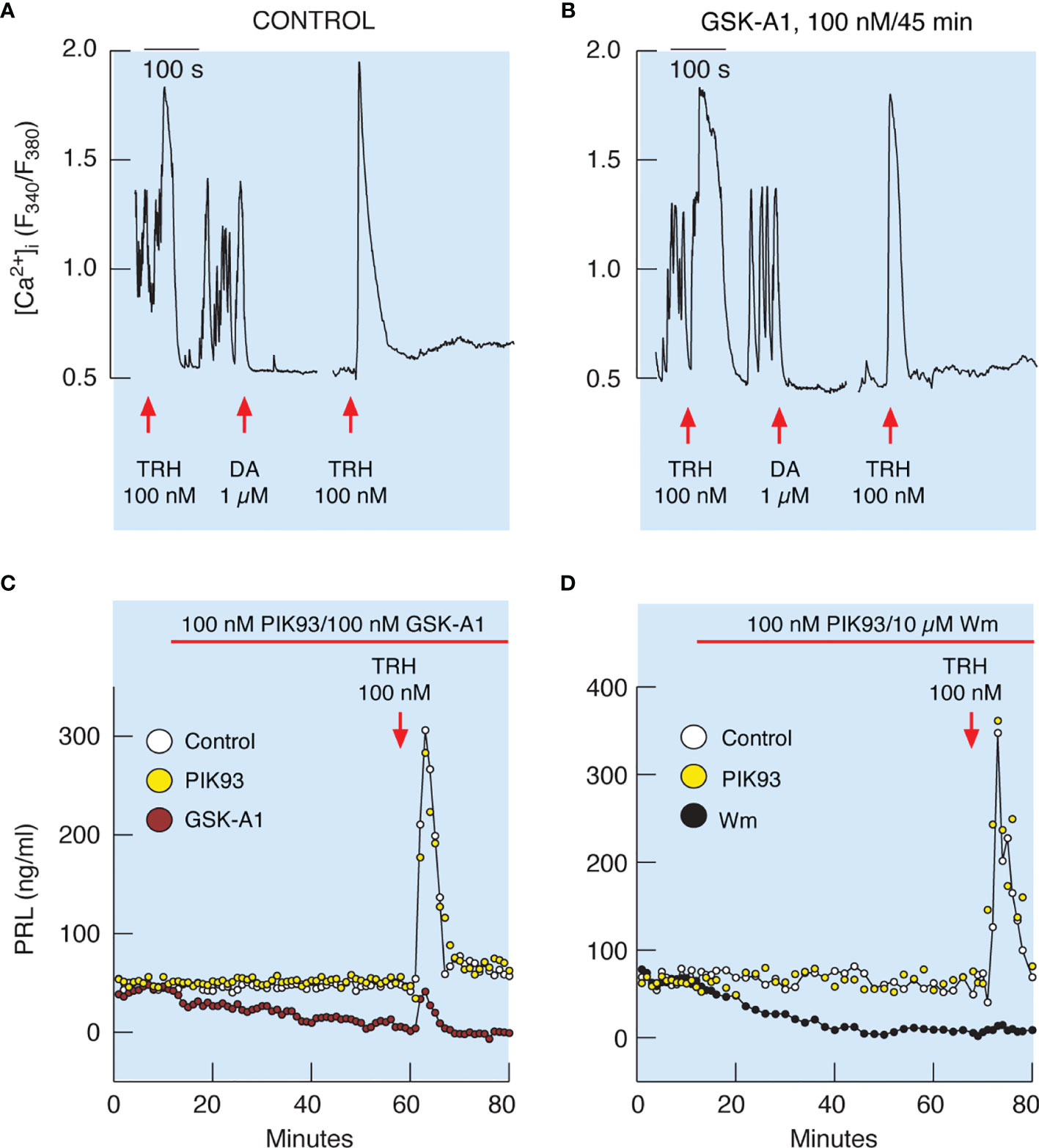
Figure 9 Inhibition of PI4KA blocks TRH-stimulated PRL release downstream of TRH-induced calcium mobilization from intracellular pools. (A, B) To identify single lactotrophs, control (A) and GSK-A1-pretreated (B) cells were treated with TRH and dopamine (DA), washed for 15 min, and restimulated with TRH. GSK-A1 was added 45 min before recording, during TRH and DA treatments, and during the rinsing periods. DA blocks voltage-gated calcium influx, which is also a useful pharmacological tool for demonstrating extracellular calcium independence of spike response and extracellular calcium dependence of sustained calcium transients, as shown by another application of TRH in the presence of dopamine. Traces shown are representative from three independent experiments. (C, D) TRH-induced PRL release was dramatically reduced in GSK-A1- and Wm-treated cells, but not in PIK93-treated cells. Mean ± SEM values (ng/ml) during 20 min TRH application were: (C) TRH 107 ± 14 ng/ml, GSK-A1+ TRH = 14 ± 3 (P < 0.0001), and PIK+TRH 107 ± 15; (D) TRH 172 ± 31 ng/ml, Wm + TRH = 10 ± 1 (P < 0.0001), and PIK93+TRH 205 ± 30.
Figure 9C shows that the profile of TRH-induced PRL release in perfused control cells is biphasic, composed of an early spike phase and a sustained plateau phase, and is therefore comparable to the TRH-stimulated calcium signaling profile. In contrast to calcium signaling, however, TRH-induced PRL release was dramatically reduced in GSK-A1 (Figure 9C) and Wm (Figure 9D) treated cells. Administration of 1 µM PIK93, a PI4KB inhibitor, was ineffective (Figures 9C, D). Therefore, functional PI4KA is also critical for the release of PRL induced by calcium mobilization.
Discussion
In this study, we examined the expression of the PIK genes that control production of phosphoinositides, including PI4P, PI (4,5)P2, and PI (3,4,5)P3, and the possible role of these signaling molecules in hormone secretion in pituitary cells. The focus of this study was on PRL-secreting lactotrophs of the anterior pituitary gland. The data indicate quantitative rather than qualitative differences in PIK gene expression among pituitary cell types. Lactotrophs and all other cell types expressed all four PI4K genes and three PIP5K1 genes, as well as Pikfyve and Pip4k2c, indicating that the pituitary gland is well equipped for PI4P and PI (4,5)P2 synthesis. Among the four members of class I PI3KC genes, Pik3ca expression was detected at the single cell level as well as Pik3r1, which encodes a regulatory subunit of this enzyme. However, the presence of the PI3K regulatory subunit genes Pik3r2 and Pik3r3 in pituitary cells is indicative that other forms of PI3KC could also be expressed in pituitary cells but below the threshold of detection by scRNAseq. The expression of these genes was also observed in scRNAseq analysis of adult male pituitary cells (33); the NCBI Gene Expression Omnibus, GSE:132224.
We performed a pharmacological assessment of the role of these enzymes in hormone secretion initially using Wm, which effectively blocks all PI3Ks (19) and PI4KA/PI4KB (42). These experiments showed that Wm blocks basal PRL release in cultured pituitary cells from females in a time- and concentration-dependent manner, without affecting basal LH and GH secretion. We also observed inhibitory effect of Wm on PRL release in pituitary cells from adult male rats. Therefore, although lactotrophs, somatotrophs, and gonadotrophs all express the Wm-sensitive PIK gene products at comparable levels, only PRL secretion is affected. To elucidate the mechanism by which Wm inhibits PRL release, but not LH and GH release, and which kinases account for inhibitory action, we pursued several leads in this study.
In general, progressive inhibition of PRL release by Wm during continuous administration could reflect inhibition of de novo PRL synthesis, leading to a gradual depletion of the secretory pool and a decrease in PRL release. However, three lines of evidence challenge this hypothesis. First, the τ50 of Wm-induced inhibition of PRL release was shorter than that induced by cycloheximide, a protein synthesis blocker. Second, Wm did not affect the expression of the PRL gene. Third, Wm blockade of PRL release was not accompanied by a decrease in the intracellular PRL pool, but rather by its significant increase. Therefore, Wm does not affect prolactin synthesis, but inhibits the release of stored hormone in secretory vesicles of pituitary lactotrophs.
It has been well established that in lactotroph cells, spontaneous plateau-bursting type APs, accompanied by [Ca2+]i transients, are of sufficient amplitude to trigger calcium-dependent hormone release that contributes to what is called basal secretion (16). It has also been well established that blocking spontaneous electrical activity by removal of bath calcium or adding dopamine abolishes firing of APs, calcium signaling, and basal PRL release (16, 17). Conversely, high potassium and Bay K8644 stimulation of PRL release, also reported by others (43–45), confirms the critical role of voltage-gated calcium influx in the hormone release process. Therefore, it was reasonable to hypothesize that Wm inhibits PRL release by blocking the spontaneous and/or the facilitated voltage-gated calcium influx. However, we found that Wm treatment did not affect voltage-gated calcium influx. Furthermore, we showed that Wm inhibited TRH-stimulated PRL release without affecting IP3-dependent calcium mobilization. Together, these observations indicate that Wm inhibits basal as well as stimulated PRL secretion downstream of the calcium generating molecular events.
Wm is not a specific inhibitor of PIK enzymes, as it inhibits several lipid (and protein) kinases with different potencies. Class I PI3Ks are inhibited by Wm with an IC50 of 1-5 nM (29); class II PI3K with an IC50 of 1.2 nM (7); and class III PI3Ks, with an IC50 of 50-300 nM (46). Wm also inhibits the PI3K-related kinases with different potencies (low to high IC50s): PRKDC, 16 nM; SMG1, 60 nM; ATM, 100-150 nM; mTOR, 200 nM; and ATR, 1.8 µM (47–50). The polo-like kinase is also inhibited by Wm with an IC50 of 24-48 nM (51) as well as smooth muscle myosin light chain kinase with an IC50 of 0.17 µM (52), mitogen-activated protein kinases, with an IC50 of 0.2-0.3 µM (53), and PI4Ks, with an IC50 of 0.3 µM (20). Our scRNAseq data indicate that Atr, Plk1, and Mylk are not expressed in pituitary cells, but the other genes in this list are present.
Due to the documented role of PI3Ks in fish pituitary hormone secretion (14, 54, 55) and their inhibition by Wm, we initially hypothesized that Wm blockade of PI3KCs in lactotrophs caused inhibition of PRL release. We tested this hypothesis in two ways. Our concentration-dependence study with pituitary cells in static incubations and perfused cells showed no effects of Wm in the nanomolar range of concentration, which are sufficient to block PI3KCs. Administration of LY294002, another established PI3KC inhibitor (21), also did not affect basal PRL release. Therefore, inhibition of PI3KCs by these compounds does not account for inhibition of spontaneous and stimulated PRL release during 3 h of treatment.
Because ATR and smooth muscle myosin light chain kinase are not detectable in lactotrophs, and high concentrations of Wm also inhibit type-III PI4Ks, we focused on the latter enzymes. The inhibitory action of Wm on PRL release was mimicked by GSK-A1, a highly potent and specific PI4KA inhibitor, with an IC50 of ~10 nM (25). The time-courses of PRL release inhibition induced by Wm and GSK-A1 were also identical. Furthermore, GSK-A1-induced inhibition of PRL release was accompanied by an increase in cellular PRL content. Finally, GSK-A1 inhibited PRL release without affecting Bay K8644-, high potassium-, and TRH-induced calcium signaling. In contrast, PIK93, which inhibits PI4KB in vitro with an IC50 of 14 nM (56), did not inhibit basal and TRH-stimulated PRL release when administrated in up to 1 µM concentration. These observations strongly support a role of PI4KA in calcium dependent PRL release.
Since PI4P is a substrate for PI(4, 5)P2 production, there was a possibility that PI(4, 5)P2 changes account for the inhibition of PRL release by blocking PI4KA. In general, the role of PI(4, 5)P2 in the control of ion channels is well established, including voltage-gated potassium channels (10, 57), inwardly rectifying potassium channels (58, 59), calcium-activated potassium channels (60, 61), and voltage-gated calcium channels (62). PI(4, 5)P2 also enhances calcium-secretion coupling downstream of calcium signaling (11, 12). Although the genes for these channels are also expressed in pituitary lactotrophs (33), the lack of effect of Wm and GSK-A1 on voltage-gated and TRH-stimulated calcium signaling suggests that PI(4, 5)P2 production/metabolism is not significantly affected by PI4KA inhibition on the timescale of the current studies.
As stated above, PI(4, 5)P2 can be synthesized via two enzymatic pathways: the phosphorylation of PI4P at position 5 of the inositol head group by PIP5Ks or phosphorylation of PI5P at position 4 by PIP4Ks (9). However, the bulk of the PI(4, 5)P2 in animal cells is synthesized by the PI4K-PIP5K pathway (63). In experiments using the PIP5K1A and PIP5K1C inhibitors, ISA2011B and UNC3230, respectively, no inhibition of PRL release was observed. Assuming that these inhibitors indeed inhibited PI(4, 5)P2 synthesis, these data suggested that a decrease in basal PI(4, 5)P2 is not responsible for the Wm-inhibition of PRL release. This was in agreement with previous studies showing that Wm or GSK-A1 treatment does not decrease PI(4, 5)P2 levels in spite of their strong effects on PI4P levels (20, 25, 64). It is therefore reasonable to conclude that plasma membrane PI4P is critical for basal and stimulated PRL release independently of PI(4, 5)P2.
In conclusion, we present here pharmacological evidence for the critical role of PI4KA in the release of PRL in mammalian lactotrophs, highlighting the contribution of plasma membrane PI4P in supporting calcium secretion coupling downstream of calcium signaling. Others also observed that the addition of PI4P potentiates calcium-induced insulin exocytosis (65). The role of PI4P in vesicle exit from the Golgi is well established, but PI4KB plays a major role in this process (66). PI4P was also reported to regulate exocytosis in S. cerevisiae by promoting vesicle docking (67). Additional studies are needed to elucidate the exact molecular steps controlled by PI4P in the exocytotic pathway in mammalian lactotrophs downstream of calcium signaling. The existence of conditional knockout PI4KA mice (25, 64), also provides an opportunity to test this hypothesis more directly by silencing this gene in different hormone-producing cells.
Data Availability Statement
The raw data supporting the conclusions of this article will be made available by the authors, without undue reservation. Single cell RNA sequencing data are accessible at NCBI GEO database, accession GSE184319.
Ethics Statement
The animal study was reviewed and approved by The National Institute of Child Health and Human Development, Animal Care and Use Committee (Animal Protocol 19-041).
Author Contributions
Conceptualization: SS and TB. Experimental Work: MK, AG-I, MT, RP, KS and SJS. Writing – original draft: SS. Writing – review and editing: all authors. Data analysis and figure preparation: SS and PF. Supervision and funding acquisition: SS, AS, and TB. All authors contributed to the article and approved the submitted version.
Funding
This work was supported by National Institutes of Health grants from the Intramural Research Program of the Eunice Kennedy Shriver NICHD and NIDDK.
Conflict of Interest
The authors declare that the research was conducted in the absence of any commercial or financial relationships that could be construed as a potential conflict of interest.
Publisher’s Note
All claims expressed in this article are solely those of the authors and do not necessarily represent those of their affiliated organizations, or those of the publisher, the editors and the reviewers. Any product that may be evaluated in this article, or claim that may be made by its manufacturer, is not guaranteed or endorsed by the publisher.
Acknowledgments
Confocal imaging was performed at the NICHD Microscopy and Imaging Core of NICHD, NIH, with the kind assistance of Drs. Vincent Schram and Lynne Holtzclaw. scRNAseq was performed by Molecular Genomics Core of NICHD, NIH with the kind assistance of Dr. Steven Coon.
References
1. Burgoyne RD, Morgan A. Secretory Granule Exocytosis. Physiol Rev (2003) 83:581–632. doi: 10.1152/physrev.00031.2002
2. Martin TF. Tuning Exocytosis for Speed: Fast and Slow Modes. Biochim Biophys Acta (2003) 1641:157–65. doi: 10.1016/S0167-4889(03)00093-4
3. Gustavsson N, Wu B, Han W. Calcium Sensing in Exocytosis. Adv Exp Med Biol (2012) 740:731–57. doi: 10.1007/978-94-007-2888-2_32
4. Salaun C, James DJ, Chamberlain LH. Lipid Rafts and the Regulation of Exocytosis. Traffic (2004) 5:255–64. doi: 10.1111/j.1600-0854.2004.0162.x
5. van Meer G, Sprong H. Membrane Lipids and Vesicular Traffic. Curr Opin Cell Biol (2004) 16:373–8. doi: 10.1016/j.ceb.2004.06.004
6. Boura E, Nencka R. Phosphatidylinositol 4-Kinases: Function, Structure, and Inhibition. Exp Cell Res (2015) 337:136–45. doi: 10.1016/j.yexcr.2015.03.028
7. Falasca M, Maffucci T. Role of Class II Phosphoinositide 3-Kinase in Cell Signalling. Biochem Soc Trans (2007) 35:211–4. doi: 10.1042/BST0350211
8. Vicinanza M, Korolchuk VI, Ashkenazi A, Puri C, Menzies FM, Clarke JH, et al. Pi(5)P Regulates Autophagosome Biogenesis. Mol Cell (2015) 57:219–34. doi: 10.1016/j.molcel.2014.12.007
9. Mathre S, Reddy KB, Ramya V, Krishnan H, Ghosh A, Raghu P. Functional Analysis of the Biochemical Activity of Mammalian Phosphatidylinositol 5 Phosphate 4-Kinase Enzymes. Biosci Rep (2019) 39(2):BSR20182210. doi: 10.1042/BSR20182210
10. Hille B, Dickson EJ, Kruse M, Vivas O, Suh BC. Phosphoinositides Regulate Ion Channels. Biochim Biophys Acta (2015) 1851:844–56. doi: 10.1016/j.bbalip.2014.09.010
11. Milosevic I, Sorensen JB, Lang T, Krauss M, Nagy G, Haucke V, et al. Plasmalemmal Phosphatidylinositol-4,5-Bisphosphate Level Regulates the Releasable Vesicle Pool Size in Chromaffin Cells. J Neurosci (2005) 25:2557–65. doi: 10.1523/JNEUROSCI.3761-04.2005
12. Martin TF. Role of PI(4,5)P(2) in Vesicle Exocytosis and Membrane Fusion. Subcell Biochem (2012) 59:111–30. doi: 10.1007/978-94-007-3015-1_4
13. Balla T. Phosphoinositides: Tiny Lipids With Giant Impact on Cell Regulation. Physiol Rev (2013) 93:1019–137. doi: 10.1152/physrev.00028.2012
14. Pemberton JG, Chang JP. Ligand-Biased Regulation of PtdIns(3,4,5)P3-Dependent Signal Transduction in GPCR Control of Pituitary Hormone Release. Endocrinology (2017) 158:378–401. doi: 10.1210/en.2016-1552
15. Stojilkovic SS, Tabak J, Bertram R. Ion Channels and Signaling in the Pituitary Gland. Endocr Rev (2010) 31:845–915. doi: 10.1210/er.2010-0005
16. Van Goor F, Zivadinovic D, Martinez-Fuentes AJ, Stojilkovic SS. Dependence of Pituitary Hormone Secretion on the Pattern of Spontaneous Voltage-Gated Calcium Influx. Cell Type-Specific Action Potential Secretion Coupling. J Biol Chem (2001) 276:33840–6. doi: 10.1074/jbc.M105386200
17. Gonzalez-Iglesias AE, Murano T, Li S, Tomic M, Stojilkovic SS. Dopamine Inhibits Basal Prolactin Release in Pituitary Lactotrophs Through Pertussis Toxin-Sensitive and -Insensitive Signaling Pathways. Endocrinology (2008) 149:1470–9. doi: 10.1210/en.2007-0980
18. Lachowicz A, Van Goor F, Katzur AC, Bonhomme G, Stojilkovic SS. Uncoupling of Calcium Mobilization and Entry Pathways in Endothelin-Stimulated Pituitary Lactotrophs. J Biol Chem (1997) 272:28308–14. doi: 10.1074/jbc.272.45.28308
19. Shpetner H, Joly M, Hartley D, Corvera S. Potential Sites of PI-3 Kinase Function in the Endocytic Pathway Revealed by the PI-3 Kinase Inhibitor, Wortmannin. J Cell Biol (1996) 132:595–605. doi: 10.1083/jcb.132.4.595
20. Nakanishi S, Catt KJ, Balla T. A Wortmannin-Sensitive Phosphatidylinositol 4-Kinase That Regulates Hormone-Sensitive Pools of Inositolphospholipids. Proc Natl Acad Sci USA (1995) 92:5317–21. doi: 10.1073/pnas.92.12.5317
21. Vlahos CJ, Matter WF, Hui KY, Brown RF. A Specific Inhibitor of Phosphatidylinositol 3-Kinase, 2-(4-Morpholinyl)-8-Phenyl-4H-1-Benzopyran-4-One (LY294002). J Biol Chem (1994) 269:5241–8. doi: 10.1016/S0021-9258(17)37680-9
22. Gharbi SI, Zvelebil MJ, Shuttleworth SJ, Hancox T, Saghir N, Timms JF, et al. Exploring the Specificity of the PI3K Family Inhibitor LY294002. Biochem J (2007) 404:15–21. doi: 10.1042/BJ20061489
23. Knight ZA, Gonzalez B, Feldman ME, Zunder ER, Goldenberg DD, Williams O, et al. A Pharmacological Map of the PI3-K Family Defines a Role for P110alpha in Insulin Signaling. Cell (2006) 125:733–47. doi: 10.1016/j.cell.2006.03.035
24. Leivers AL, Tallant M, Shotwell JB, Dickerson S, Leivers MR, McDonald OB, et al. Discovery of Selective Small Molecule Type III Phosphatidylinositol 4-Kinase Alpha (PI4KIIIalpha) Inhibitors as Anti Hepatitis C (HCV) Agents. J Med Chem (2014) 57:2091–106. doi: 10.1021/jm400781h
25. Bojjireddy N, Botyanszki J, Hammond G, Creech D, Peterson R, Kemp DC, et al. Pharmacological and Genetic Targeting of the PI4KA Enzyme Reveals Its Important Role in Maintaining Plasma Membrane Phosphatidylinositol 4-Phosphate and Phosphatidylinositol 4,5-Bisphosphate Levels. J Biol Chem (2014) 289:6120–32. doi: 10.1074/jbc.M113.531426
26. Semenas J, Hedblom A, Miftakhova RR, Sarwar M, Larsson R, Shcherbina L, et al. The Role of PI3K/AKT-Related PIP5K1alpha and the Discovery of Its Selective Inhibitor for Treatment of Advanced Prostate Cancer. Proc Natl Acad Sci USA (2014) 111:E3689–98. doi: 10.1073/pnas.1405801111
27. Wright BD, Loo L, Street SE, Ma A, Taylor-Blake B, Stashko MA, et al. The Lipid Kinase PIP5K1C Regulates Pain Signaling and Sensitization. Neuron (2014) 82:836–47. doi: 10.1016/j.neuron.2014.04.006
28. Previde RM, Wang K, Smiljanic K, Janjic MM, Nunes MT, Stojilkovic SS. Expression and Role of Thyrotropin Receptors in Proopiomelanocortin-Producing Pituitary Cells. Thyroid (2021) 31:850–8. doi: 10.1089/thy.2020.0222
29. Arcaro A, Wymann MP. Wortmannin Is a Potent Phosphatidylinositol 3-Kinase Inhibitor: The Role of Phosphatidylinositol 3,4,5-Trisphosphate in Neutrophil Responses. Biochem J (1993) 296( Pt 2):297–301. doi: 10.1042/bj2960297
30. Yano H, Agatsuma T, Nakanishi S, Saitoh Y, Fukui Y, Nonomura Y, et al. Biochemical and Pharmacological Studies With KT7692 and LY294002 on the Role of Phosphatidylinositol 3-Kinase in Fc Epsilon RI-Mediated Signal Transduction. Biochem J (1995) 312( Pt 1):145–50. doi: 10.1038/nmeth.2019
31. Burke JE, Inglis AJ, Perisic O, Masson GR, McLaughlin SH, Rutaganira F, et al. Structures of PI4KIIIbeta Complexes Show Simultaneous Recruitment of Rab11 and Its Effectors. Science (2014) 344:1035–8. doi: 10.1126/science.1253397
32. Fletcher PA, Prévide RM, Smiljanic K, Sherman A, Coon SL, Stojilkovic SS, et al. Transcriptomic Heterogeneity of Sox2-Expressing Pituitary Cells. BioRxiv (2021) 12.10.472137. doi: 10.1101/2021.12.10.472137
33. Fletcher PA, Smiljanic K, Maso Previde R, Iben JR, Li T, Rokic MB, et al. Cell Type- and Sex-Dependent Transcriptome Profiles of Rat Anterior Pituitary Cells. Front Endocrinol (Lausanne) (2019) 10:623. doi: 10.3389/fendo.2019.00623
34. Mcinnes L, Healy J, Saul N, Groberger L. Uniform Manifold Approximation and Projection. J Open Source Softw (2018) 3(29). doi: 10.21105/joss.00861
35. Schindelin J, Arganda-Carreras I, Frise E, Kaynig V, Longair M, Pietzsch T, et al. Fiji: An Open-Source Platform for Biological-Image Analysis. Nat Methods (2012) 9:676–82. doi: 10.1038/nmeth.2019
36. Janjic MM, Previde RM, Fletcher PA, Sherman A, Smiljanic K, Abebe D, et al. Divergent Expression Patterns of Pituitary Gonadotropin Subunit and GnRH Receptor Genes to Continuous GnRH In Vitro and In Vivo. Sci Rep (2019) 9:20098. doi: 10.1038/s41598-019-56480-1
37. Gonzalez-Iglesias AE, Jiang Y, Tomic M, Kretschmannova K, Andric SA, Zemkova H, et al. Dependence of Electrical Activity and Calcium Influx-Controlled Prolactin Release on Adenylyl Cyclase Signaling Pathway in Pituitary Lactotrophs. Mol Endocrinol (2006) 20:2231–46. doi: 10.1210/me.2005-0363
38. Fruman DA, Cantley LC, Carpenter CL. Structural Organization and Alternative Splicing of the Murine Phosphoinositide 3-Kinase P85 Alpha Gene. Genomics (1996) 37:113–21. doi: 10.1006/geno.1996.0527
39. Hinkle PM, Nelson EJ, Ashworth R. Characterization of the Calcium Response to Thyrotropin-Releasing Hormone in Lactotrophs and GH Cells. Trends Endocrinol Metab (1996) 7:370–4. doi: 10.1016/S1043-2760(96)00188-9
40. Szentpetery Z, Szakacs G, Bojjireddy N, Tai AW, Balla T. Genetic and Functional Studies of Phosphatidyl-Inositol 4-Kinase Type IIIalpha. Biochim Biophys Acta (2011) 1811:476–83. doi: 10.1016/j.bbalip.2011.04.013
41. Sohn M, Ivanova P, Brown HA, Toth DJ, Varnai P, Kim YJ, et al. Lenz-Majewski Mutations in PTDSS1 Affect Phosphatidylinositol 4-Phosphate Metabolism at ER-PM and ER-Golgi Junctions. Proc Natl Acad Sci USA (2016) 113:4314–9. doi: 10.1677/joe.0.1050183
42. Balla T, Downing GJ, Jaffe H, Kim S, Zolyomi A, Catt KJ. Isolation and Molecular Cloning of Wortmannin-Sensitive Bovine Type III Phosphatidylinositol 4-Kinases. J Biol Chem (1997) 272:18358–66. doi: 10.1074/jbc.272.29.18358
43. Drouva SV, Rerat E, Bihoreau C, Laplante E, Rasolonjanahary R, Clauser H, et al. Dihydropyridine-Sensitive Calcium Channel Activity Related to Prolactin, Growth Hormone, and Luteinizing Hormone Release From Anterior Pituitary Cells in Culture: Interactions With Somatostatin, Dopamine, and Estrogens. Endocrinology (1988) 123:2762–73. doi: 10.1210/endo-123-6-2762
44. Hall TR, Harvey S, Chadwick A. Mechanisms of Release of Prolactin From Fowl Anterior Pituitary Glands Incubated In Vitro: Effects of Calcium and Cyclic Adenosine Monophosphate. J Endocrinol (1985) 105:183–8. doi: 10.1677/joe.0.1050183
45. Merritt JE, Tomlinson S, Brown BL. Flunarizine, a Calcium Influx Blocker, Inhibits TRH-But Not Potassium-Stimulated Prolactin Secretion. Acta Endocrinol (Copenh) (1984) 107:31–5. doi: 10.1530/acta.0.1070031
46. Volinia S, Hiles I, Ormondroyd E, Nizetic D, Antonacci R, Rocchi M, et al. Molecular Cloning, cDNA Sequence, and Chromosomal Localization of the Human Phosphatidylinositol 3-Kinase P110 Alpha (PIK3CA) Gene. Genomics (1994) 24:472–7. doi: 10.1006/geno.1994.1655
47. Brunn GJ, Williams J, Sabers C, Wiederrecht G, Lawrence JC Jr., Abraham RT. Direct Inhibition of the Signaling Functions of the Mammalian Target of Rapamycin by the Phosphoinositide 3-Kinase Inhibitors, Wortmannin and LY294002. EMBO J (1996) 15:5256–67. doi: 10.1002/j.1460-2075.1996.tb00911.x
48. Chan DW, Son SC, Block W, Ye R, Khanna KK, Wold MS, et al. Purification and Characterization of ATM From Human Placenta. A Manganese-Dependent, Wortmannin-Sensitive Serine/Threonine Protein Kinase. J Biol Chem (2000) 275:7803–10. doi: 10.1074/jbc.275.11.7803
49. Sarkaria JN, Tibbetts RS, Busby EC, Kennedy AP, Hill DE, Abraham RT. Inhibition of Phosphoinositide 3-Kinase Related Kinases by the Radiosensitizing Agent Wortmannin. Cancer Res (1998) 58:4375–82. doi: 10.1016/j.chembiol.2004.11.009
50. Yamashita A, Ohnishi T, Kashima I, Taya Y, Ohno S. Human SMG-1, A Novel Phosphatidylinositol 3-Kinase-Related Protein Kinase, Associates With Components of the mRNA Surveillance Complex and Is Involved in the Regulation of Nonsense-Mediated mRNA Decay. Genes Dev (2001) 15:2215–28. doi: 10.1101/gad.913001
51. Liu Y, Shreder KR, Gai W, Corral S, Ferris DK, Rosenblum JS. Wortmannin, a Widely Used Phosphoinositide 3-Kinase Inhibitor, Also Potently Inhibits Mammalian Polo-Like Kinase. Chem Biol (2005) 12:99–107. doi: 10.1016/j.chembiol.2004.11.009
52. Nakanishi S, Kakita S, Takahashi I, Kawahara K, Tsukuda E, Sano T, et al. Wortmannin, a Microbial Product Inhibitor of Myosin Light Chain Kinase. J Biol Chem (1992) 267:2157–63. doi: 10.1016/S0021-9258(18)45857-7
53. Ferby IM, Waga I, Sakanaka C, Kume K, Shimizu T. Wortmannin Inhibits Mitogen-Activated Protein Kinase Activation Induced by Platelet-Activating Factor in Guinea Pig Neutrophils. J Biol Chem (1994) 269:30485–8. doi: 10.1016/S0021-9258(18)43839-2
54. Pemberton JG, Stafford JL, Chang JP. Ligand-Selective Signal Transduction by Two Endogenous GnRH Isoforms Involves Biased Activation of the Class I PI3K Catalytic Subunits P110beta, P110gamma, and P110delta in Pituitary Gonadotropes and Somatotropes. Endocrinology (2015) 156:218–30. doi: 10.1210/en.2014-1640
55. Pemberton JG, Orr ME, Stafford JL, Chang JP. PI3K Signalling in GnRH Actions on Dispersed Goldfish Pituitary Cells: Relationship With PKC-Mediated LH and GH Release and Regulation of Long-Term Effects on Secretion and Total Cellular Hormone Availability. Gen Comp Endocrinol (2014) 205:268–78. doi: 10.1016/j.ygcen.2014.03.011
56. Borawski J, Troke P, Puyang X, Gibaja V, Zhao S, Mickanin C, et al. Class III Phosphatidylinositol 4-Kinase Alpha and Beta Are Novel Host Factor Regulators of Hepatitis C Virus Replication. J Virol (2009) 83:10058–74. doi: 10.1128/JVI.02418-08
57. Zhang H, Craciun LC, Mirshahi T, Rohacs T, Lopes CM, Jin T, et al. PIP(2) Activates KCNQ Channels, and Its Hydrolysis Underlies Receptor-Mediated Inhibition of M Currents. Neuron (2003) 37:963–75. doi: 10.1016/S0896-6273(03)00125-9
58. Huang CL, Feng S, Hilgemann DW. Direct Activation of Inward Rectifier Potassium Channels by PIP2 and Its Stabilization by Gbetagamma. Nature (1998) 391:803–6. doi: 10.1038/35882
59. Zhang H, He C, Yan X, Mirshahi T, Logothetis DE. Activation of Inwardly Rectifying K+ Channels by Distinct PtdIns(4,5)P2 Interactions. Nat Cell Biol (1999) 1:183–8. doi: 10.1038/11103
60. Lu M, Hebert SC, Giebisch G. Hydrolyzable ATP and PIP(2) Modulate the Small-Conductance K+ Channel in Apical Membranes of Rat Cortical-Collecting Duct (CCD). J Gen Physiol (2002) 120:603–15. doi: 10.1085/jgp.20028677
61. Vaithianathan T, Bukiya A, Liu J, Liu P, Asuncion-Chin M, Fan Z, et al. Direct Regulation of BK Channels by Phosphatidylinositol 4,5-Bisphosphate as a Novel Signaling Pathway. J Gen Physiol (2008) 132:13–28. doi: 10.1085/jgp.200709913
62. Suh BC, Leal K, Hille B. Modulation of High-Voltage Activated Ca(2+) Channels by Membrane Phosphatidylinositol 4,5-Bisphosphate. Neuron (2010) 67:224–38. doi: 10.1016/j.neuron.2010.07.001
63. Stephens LR, Hughes KT, Irvine RF. Pathway of Phosphatidylinositol(3,4,5)-Trisphosphate Synthesis in Activated Neutrophils. Nature (1991) 351:33–9. doi: 10.1038/351033a0
64. Alvarez-Prats A, Bjelobaba I, Aldworth Z, Baba T, Abebe D, Kim YJ, et al. Schwann-Cell-Specific Deletion of Phosphatidylinositol 4-Kinase Alpha Causes Aberrant Myelination. Cell Rep (2018) 23:2881–90. doi: 10.1016/j.celrep.2018.05.019
65. Waselle L, Gerona RR, Vitale N, Martin TF, Bader MF, Regazzi R. Role of Phosphoinositide Signaling in the Control of Insulin Exocytosis. Mol Endocrinol (2005) 19:3097–106. doi: 10.1210/me.2004-0530
66. Waugh MG. The Great Escape: How Phosphatidylinositol 4-Kinases and PI4P Promote Vesicle Exit From the Golgi (and Drive Cancer). Biochem J (2019) 476:2321–46. doi: 10.1042/BCJ20180622
Keywords: pituitary, lactotrophs, prolactin, regulated exocytosis, PI4-kinases, phosphoinositides, intracellular calcium
Citation: Kučka M, Gonzalez-Iglesias AE, Tomić M, Prévide RM, Smiljanic K, Sokanovic SJ, Fletcher PA, Sherman A, Balla T and Stojilkovic SS (2021) Calcium-Prolactin Secretion Coupling in Rat Pituitary Lactotrophs Is Controlled by PI4-Kinase Alpha. Front. Endocrinol. 12:790441. doi: 10.3389/fendo.2021.790441
Received: 06 October 2021; Accepted: 02 December 2021;
Published: 30 December 2021.
Edited by:
Damian G. Romero, University of Mississippi Medical Center, United StatesReviewed by:
Yves Combarnous, Centre National de la Recherche Scientifique (CNRS), FranceAndre Souza Mecawi, Federal University of São Paulo, Brazil
Copyright © 2021 Kučka, Gonzalez-Iglesias, Tomić, Prévide, Smiljanic, Sokanovic, Fletcher, Sherman, Balla and Stojilkovic. This is an open-access article distributed under the terms of the Creative Commons Attribution License (CC BY). The use, distribution or reproduction in other forums is permitted, provided the original author(s) and the copyright owner(s) are credited and that the original publication in this journal is cited, in accordance with accepted academic practice. No use, distribution or reproduction is permitted which does not comply with these terms.
*Correspondence: Stanko S. Stojilkovic, stojilks@mail.nih.gov
†Present address: Marek Kučka, Friedrich Miescher Laboratory of the Max Planck Society, Tübingen, Germany
Arturo E. Gonzalez-Iglesias, Epidemiology and Disease Control Program, Florida Department of Health, Tallahassee, FL, United States
‡Deceased