- 1Laboratory of Neurobiology, Faculty of Veterinary Medicine and Animal Science, Poznań University of Life Sciences, Poznań, Poland
- 2Molecular and Cell Biology Unit, Poznań University of Medical Sciences, Poznań, Poland
- 3Department of Paediatric Diabetes and Obesity, Poznań University of Medical Sciences, Poznań, Poland
The prenatal period, during which a fully formed newborn capable of surviving outside its mother’s body is built from a single cell, is critical for human development. It is also the time when the foetus is particularly vulnerable to environmental factors, which may modulate the course of its development. Both epidemiological and animal studies have shown that foetal programming of physiological systems may alter the growth and function of organs and lead to pathology in adulthood. Nutrition is a particularly important environmental factor for the pregnant mother as it affects the condition of offspring. Numerous studies have shown that an unbalanced maternal metabolic status (under- or overnutrition) may cause long-lasting physiological and behavioural alterations, resulting in metabolic disorders, such as obesity and type 2 diabetes (T2DM). Various diets are used in laboratory settings in order to induce maternal obesity and metabolic disorders, and to alter the offspring development. The most popular models are: high-fat, high-sugar, high-fat-high-sugar, and cafeteria diets. Maternal undernutrition models are also used, which results in metabolic problems in offspring. Similarly to animal data, human studies have shown the influence of mothers’ diets on the development of children. There is a strong link between the maternal diet and the birth weight, metabolic state, changes in the cardiovascular and central nervous system of the offspring. The mechanisms linking impaired foetal development and adult diseases remain under discussion. Epigenetic mechanisms are believed to play a major role in prenatal programming. Additionally, sexually dimorphic effects on offspring are observed. Therefore, further research on both sexes is necessary.
Introduction: We Are What Our Mothers Eat
Over the years numerous epidemiological findings and data from animal studies revealed the effects of mother’s nutrition on the development of offspring. Researchers proposed the concept of foetal/early programming, according to which early environmental factors can permanently organise or imprint physiological and behavioural systems. Programming is defined as the process in which environmental factor(s), acting during the sensitive period, affect the structure and functions of tissues and organs, leading to lifelong effects (1, 2). Accordingly, researchers suggested redirecting investigations towards the intrauterine environment rather than the environment in later childhood and indicated that the womb may be more important than home (1).
Although hunger is still common nowadays, especially in Africa, paradoxically, all over the world more people are dying of the health problems caused by overeating rather than malnutrition. This subject is particularly interesting at the time when obesity is a worldwide problem, and it is even greater due to the COVID-19 pandemic. As pandemic-related lockdowns reduced people’s physical activity and increased overeating (the factors contributing to the development of obesity), the rates of these metabolic problems are expected to increase rapidly in our society in the future, also among mothers-to-be and their offspring. It is a well-known fact that the Western pattern diet (WPD), which is common in the US and other developed countries, may chronically activate the innate immune system and inhibit the adaptive immune system. Thus, the WPD impairs adaptive immunity while ramping up innate immunity. This leads to chronic inflammation and severely impairs the host’s defence against viral pathogens, including COVID-19 (3). A report on 4,103 patients with COVID-19 disease in New York City showed that the most important clinical features leading to hospital admission were age >65 years and obesity (4). The author of another study on critically ill adults with COVID-19 admitted to two hospitals in New York City reported that the majority were men aged over 60 years and nearly half of them were obese (5). These findings were also confirmed by the authors of European studies on 165 adult patients with a mean BMI 26, who found that severe forms of COVID-19 were associated with high visceral adiposity (6).
According to the findings of studies on animals and humans, both maternal under and overnutrition/obesity affects the cognitive function and the development of neurological and psychiatric disorders in offspring.
The foetal programming concept suggests that maternal nutritional imbalance (both under- and overnutrition) have a long-term effect on the health of offspring and on the risk of diseases such as diabetes (7). The mechanisms responsible for the effects of maternal undernutrition and obesity on the increased risk of future metabolic disease have not been thoroughly investigated. They include changes in the foetal supply of nutrients, genetic and epigenetic factors. Moreover, as results from animal studies on perinatal high-fat diet (HFD) programming, the potential mechanisms of neural pathways include circulating factors, such as hormones (leptin, insulin), nutrients (fatty acids, triglycerides (TG) and glucose), and inflammatory cytokines.
The aim of this paper was to review the data on maternal undernutrition, obesity, and diabetes provided by the authors of research on humans and animals, and to discuss the potential mechanisms underlying the foetal metabolic programming. The main focus of this review is the foetal metabolic system and central nervous system (CNS), because they seem to be the most vulnerable to the harmful effects of programming (8). The mechanism(s) contributing to the sexual dimorphism of metabolic diseases were also investigated. The detailed analysis of data concerning sexual differences observed in the offspring of malnourished and overnourished mothers is shown in the Table 1. The understanding of these mechanisms could provide useful tools for the prevention and treatment of these diseases in offspring.
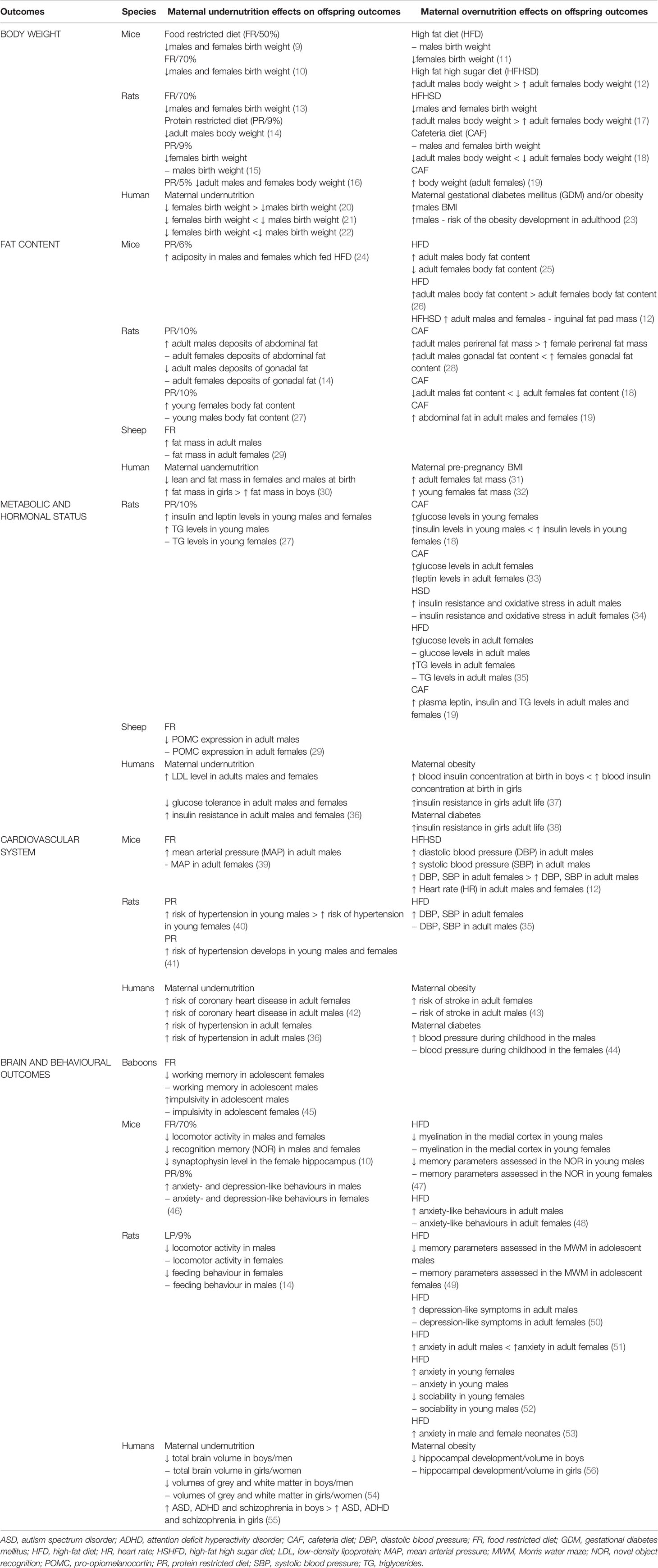
Table 1 Maternal under- and overnutrition induce sex-specific differences in body weight, fat content, metabolic and hormonal status, cardiovascular system and brain and behavioural outcomes in offspring; ↑ - increase; ↓ decrease; - no change.
Animal Models of Obesity With Special Emphasis on Diet-Induced Obesity
Studies on different animal species, mostly mice, rats, and sheep, allow us to understand the mechanisms responsible for the development of obesity and diabetes, and search for intervention strategies. Obesity can be induced in animals chemically (with drugs), surgically, genetically, or through diet (57–61). Obesity has multifactorial aetiology and in the light of the increasing occurrence of unhealthy nutrition (e.g. the consumption of fast food), the rising prevalence of obesity suggests that it is caused by environmental factors. The authors of this review focused mostly on diet-induced obesity in rodents and made references to numerous excellent comprehensive reviews of other models of animal obesity (59, 60).
The most popular models of diet-induced obesity are: high-fat (HFD), high-sugar (HSD), high-fat-high-sugar (HFHSD), and cafeteria (CAF) diets. High-fat diets caused metabolic imbalance, decreased energy expenditure and, as a result, increased the body weight of laboratory animals (62, 63). However, there are differences between species and even strains of rodents. Both Wistar and Sprague-Dawley rats can be used as models of HFD-induced obesity (64), although the metabolic effects caused by this type of dietary regimen are more pronounced in Wistar rats. In recent decades the HFD has been most widely applied in experiments on rodents, but there were significant differences in the composition and fat content of the diets provided to animals to induce obesity. The fat in these diets came from multiple sources, including animals (lard, tallow), plants (olive oil, sunflower, corn, coconut), and fish. The fat composition seems to have a major role in obesity because saturated fats cause more deleterious effects than unsaturated fats (63, 65). Another important factor is the concentration of fat in the diet, which usually ranges from 30% to 60%. The diet-feeding period also differed between studies – it usually ranged from 4 to 16 weeks (62, 63).
Similarly to the situation observed in highly developed countries, a high-sugar diet also contributed to the development of obesity in laboratory animals. In experimental models, sucrose was fed separately from the standard feed, as a superadditive or mixed with drinking water (61). The HSD negatively influenced the glycaemic control in rodents. These effects were similar to those observed after feeding an HFD. However, the body weight gain and adiposity in HSD-fed animals were lesser than after feeding an HFD (59).
A combination of HFD and HSD better imitates human characteristics. The HFHSD has widely been used in studies on rodents as a Western pattern diet. However, Omar et al. showed that the HFHSD was not as effective as the HFD in C57BL/6J mice (66).
The CAF diet is employed in laboratory settings, where animals receive a mix of high-fat and high-sugar food products, which are commonly consumed by people (e.g. cake, biscuits, crisps, processed meat, peanut butter, chocolate, cheese, dried fruit) (60, 61, 65). The components of the CAF diet have a high energy value and are highly palatable, which increases animals’ tendency to overconsumption (60, 61, 65). This model mimics the occurrence of obesity in humans as a consequence of a tasty but unbalanced diet. However, this diet varies in the number and type of products used and its energy value [for more details see (67)].
Experimental paradigms may also vary in terms of exposure to these diets, i.e. the length of food consumption before and/or during pregnancy and/or lactation, which may also influence the results. Among the environmental factors influencing the programming of the foetal phenotype, particular attention is paid to disturbed maternal nutrition. Animal and epidemiological studies have indicated that foetal nutritional deprivation is a strong programming stimulus. On the other hand experimental evidence suggests that maternal overnutrition can result in a phenotype of the offspring characteristic of metabolic syndrome. Moreover, both in humans and animal studies, it has been confirmed that prenatal, perinatal and postnatal factors that are associated with disturbed maternal and offspring nutrition are additive (28, 68–71). In consequence, they lead to unfavourable changes in metabolism in adulthood, and induce diabetes. In utero, epigenetic changes exacerbate the negative effects associated with the influence of environmental factors throughout life (68–71).
Animal Models of Diabetes
There are two major types of diabetes, i.e. type 1 (T1DM) and type 2 (T2DM). The latter is the most common, as it represents more than 90% of all cases. T1DM is caused by an autoimmune destruction of the insulin-producing β-cells in the pancreas (72). Due to the pathophysiology of T1DM, insulin therapy is implemented at the onset of this disease. On the other hand, T2DM can be associated with elevated, normal, or low insulin levels, depending on the stage at which the levels of this hormone are measured. This is a progressive disorder, which is manifested by diminishing pancreatic function over time. The authors of this review mostly focused on T2DM occurring during gestation, as it is often associated with obesity and more prevalent than T1DM. Gestational diabetes mellitus (GDM) is an issue of particular interest in this review. This is a heterogeneous entity and affects mostly insulin-resistant overweight and obese women. It is also a strong female risk factor for the progression of T2DM (73).
There are multiple animal models used for the induction of diabetes – mostly rodents (mice and rats) as well as sheep, dogs, cats, and other animals. Diabetes can be induced surgically, chemically or genetically. The surgical method requires pancreatectomy, i.e. removal of most of the pancreatic tissue. Diabetes can be induced non-surgically through damage to the pancreatic cells. This effect can be obtained through the administration of drugs such as alloxan and streptozotocin (STZ, toxins destroying β-cells of the pancreas), which cause insulin deficiency and hyperglycaemia in animals. Alloxan diabetogenicity occurs through the rapid uptake of the drug by insulin-secreting cells, the formation of reactive oxygen species, and disturbances in intracellular calcium homeostasis. It is also necessary to remember that the range of the diabetogenic dose of alloxan is quite narrow and even a small overdose may be generally toxic and kill animals due to kidney failure (74).
Like alloxan, the dose range of STZ is narrow. To date researchers have applied single or multiple injections in experimental paradigms with different animal species and strains (75–78). STZ is taken up by pancreatic β-cells via the glucose transporter GLUT2, which changes the DNA in these cells and provokes its fragmentation. For more detailed information on the mechanisms of alloxan and STZ action see Szkudelski (74).
A combination of HFD and STZ is often employed in laboratory settings. These two stressors mimic the pathology of T2DM, though on a shorter timescale than the one observed in humans. The use of HFD causes insulin resistance and/or glucose intolerance, while the administration of STZ reduces functional β‐cell mass (79). Another advantage of this model is that it mimics the slow pathogenesis of T2DM occurring in most humans, which progresses from the slow development of an adult-onset diet-induced obesity to glucose intolerance, insulin resistance (and the resulting compensatory insulin release) and, finally, STZ-induced partial β-cell death. Despite its limitations, e.g. the use of two stressors – HFD and STZ, the HFD/STZ is a reasonable animal model of T2DM and represents the late stage of the disease (80).
Sex-dependent differences in the induction of T2DM by STZ are also of particular interest in this review because, according to scientific reports, males are more prone to develop diabetes (81, 82). There are also sex-specific differences in the development and complications of diabetes in humans. For example, diabetes is considered a stronger risk factor for cardiovascular diseases in women than men (81). Women tend to store the fat tissue, whereas men tend to mobilise adipose tissue burning (83). Women exhibit greater insulin sensitivity than men. There are sex-specific differences in body fat distribution, which point to the crucial role of sex hormones (84).
There are also various genetic models of diabetes, but they will not be discussed here because they do not fall within the scope of this review [e.g. see (85)].
Animal models of T2DM provide not only an opportunity to investigate the pathophysiology underlying this disorder, but also enable the assessment of potential strategies for the treatment and prevention of the disease and related complications.
Animal Models of Undernutrition
To date research on undernutrition has been successfully conducted on various species of animals, such as laboratory rodents (rats, mice), sheep, pigs, and primates. Due to the fact that rodents and sheep are the most commonly used species in this research area, they will be henceforth discussed in this review. For more information on the advantages and disadvantages of other species see the review by Swanson (86).
Perinatal undernutrition can be induced in rodents through either maternal underfeeding (general food restriction or protein content restriction) during gestation or the modification of the offspring’s energy intake during the suckling period. Rodents have been used in numerous studies to examine different degrees of dietary restriction during gestation – from mild (30%), through moderate (50%), up to severe (70%) protein restriction (PR) or food restriction (FR) [for reviews see (87, 88)]. Postnatal manipulations usually include various forms of maternal milk restriction, e.g. maternal deprivation (which promotes perinatal stress) (89–91), early weaning induced with pharmacological compounds such as bromocriptine a drug inhibiting lactation (92)], non-pharmacological early weaning (in the last three days of the suckling period, when nipple suction can be interrupted with a physical barrier, e.g. by wrapping the breast area with a bandage (93–95) or rearing pups in large litters (96, 97). Maternal undernutrition in sheep is usually induced through FR [30-50% of the control feed allowance (98)]. However, there are some changes in the time of nutritional insult.
In conclusion, researchers interested in studying the effects of the maternal diet on offspring in laboratory settings have a variety of options, but due caution is necessary to ensure proper dietary controls and provide a detailed description of the diets used during the experiment, including the time of exposure and considering possible sex differences. The choice of a suitable animal model is a key point affecting the translational potential of the results.
Effects of Maternal Overnutrition on Body Weight and Fat Content: Animal Studies
Maternal overnutrition and/or obesity affect the weight of rodent offspring in a time-dependent manner (Figure 1). White et al. conducted a study on rats in which they investigated the relationship between the effects of maternal obesity and an HFD of the offspring on their body weight. They observed that these effects were independent and additive (99). Maternal obesity induced by an HFD caused a significantly lower birth weight of rats (17, 100, 101) and mice (102, 103). Similarly, the offspring of the female rats receiving a CAF diet before and during pregnancy were lighter (28, 104–106). However, other studies showed that the birth weights of the mice delivered by dams exposed to an HFD were significantly greater than those of the control offspring (25, 107). Similarly, the maternal consumption of a CAF diet resulted in a greater body weight of neonatal rats (19). Additionally, several experiments showed that the administration of a CAF diet (70) and an HFD (108, 109) before and during pregnancy did not influence the birth weight of rat and mice pups (110). The differences in the results of various studies may have been caused by the use of different sources of fat and the duration of feeding the diets to the dams and/or offspring (before pregnancy, and/or during pregnancy, and/or lactation), as well as the different effect of obese pregnancies on placental functions. Obesity may increase the placental growth and cause foetal overgrowth or reduce the placental blood flow and limit the foetal growth (111).
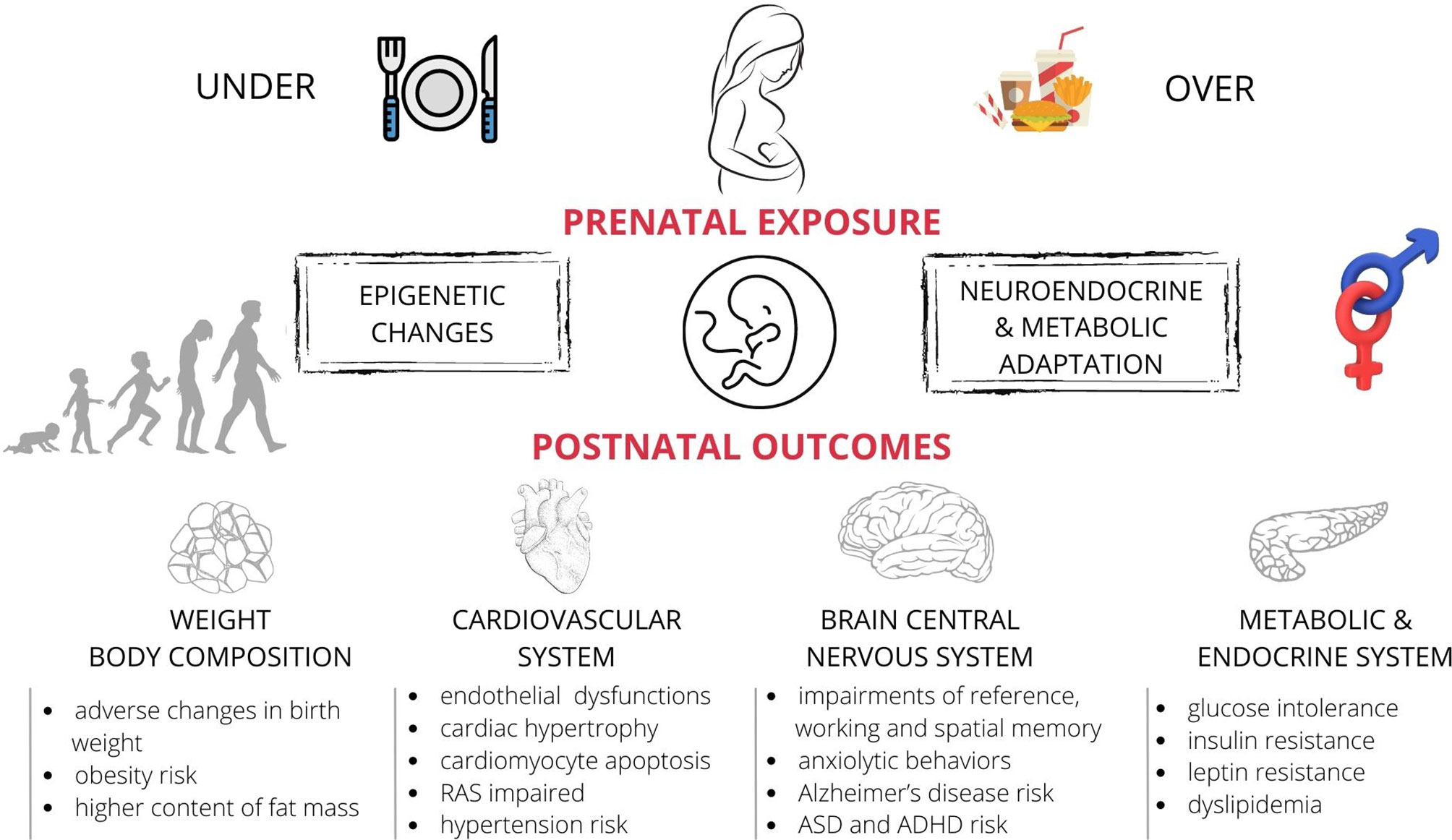
Figure 1 The effects of maternal nutritional imbalance (under- and overnutrition) on offspring during foetal development. Short and long-term negative outcomes (observed both in humans and laboratory animals) include the adverse effects of unbalanced diet on offspring’s metabolism, hormonal state, changes in the body weight and fat content, and abnormal function of the nervous and cardiovascular systems. These effects appear to be sex-specific. ASD, autism spectrum disorder; ADHD, attention deficit hyperactivity disorder; RAS, the renin-angiotensin system.
Thus, pre-gestational and/or gestational overnutrition of female rodents resulted in a heavier weight of adult offspring, which led to obesity. This effect was observed when: i) the birth weight was significantly lower [rats/HFHSD (17)], ii) the birth weight was not affected (mice/male/HFHCD) (11), and iii) the birth weight was significantly greater [mice/HFD (25, 107)]. Sasson et al. observed that pre-gestational exposure of mice to an HFD restricted the growth of newborn pups, but there was no effect on the weight of adult animals (103). Importantly, studies also revealed a catch-up in the body weight of offspring. The birth weight of the offspring of the female rats fed a CAF diet was either smaller or greater than the weight of the animals in the control group. However, the differences in the body weight disappeared during further development (19, 106).
There is not much data on the sex-specific differences in the effects of maternal diet on the weight of offspring. King et al. found that the maternal HFD did not affect the birth weight of male mice offspring, but reduced the birth weight of females (11). Nivoit et al. found that the offspring of obese rat dams had lower birth weights than the offspring of the animals in the control group. However, when the rats matured, the adult offspring of the HFHSD females became heavier than those in the control group, and these differences were less pronounced in the females (17). Similarly, Samuelsson et al. have found that in the offspring of HFHSD mothers, adult male mice were heavier than females (12). Matuszewska et al. (18) observed that on postnatal day (PND) 25 both male and female offspring of the dams fed a CAF diet had lower body weights than the animals in the control group. However, there was no difference in this parameter on PND 3. Moreover, on PND 25 the CAF females were lighter than the CAF males. On the contrary, in a study conducted by Jacobs et al., feeding mothers the CAF diet resulted in an increase in body weight of adult female rat offspring (19).
As numerous studies on rodents showed, changes in the body weight of the offspring of obese dams seem to be caused by an increase in the body fat content. Increased proportions of the body fat content (112) were described in the rat offspring of females fed HFD (99, 112), HFHSD (17), and CAF diets (18, 28, 70). The authors of the aforementioned studies with the CAF diet observed an increase in the fat content, but there was no change (70) or a decrease in the body weight of the offspring receiving the other two protocols of dietary regime (18, 28). The authors of studies on mice also observed that the fat content in the offspring was influenced by the HFD provided to their mothers (25, 103, 113).
Researchers also observed sex-specific changes in the fat content in offspring. Dahlhoff et al. found that the male offspring of HFD mice had more fat than the animals in the control group, but the female offspring had a lower body fat content than the animals in the control group (25). Masuyama and Hiramatsu (26) observed that maternal obesity induced by an HFD caused an increased fat mass gain in the male and female offspring at 14 weeks of age, but this effect was less pronounced in the females. However, in a mouse model of HFHSD, both adult male and female offspring had increased inguinal fat pad mass (12). Similarly, male and female offspring of rats exposed to CAF diet had higher abdominal fat content (19). Moreover, among the four-week offspring of CAF mothers the males had a higher perirenal fat mass than the females, whereas the females had a greater gonadal fat content than the males (28). Matuszewska et al. (18) observed that both the male and female offspring of mothers receiving a CAF diet for four weeks before pregnancy, and then during pregnancy and lactation, had a lower fat content on PND 25, but these changes were more pronounced in the female CAF offspring.
Effects of Maternal Overnutrition on Body Weight and Fat Content: Human Studies
Maternal overweight is a risk factor for foetal macrosomia and it increases the risk of development of obesity throughout childhood and adolescence (114–117). High maternal weight during all three trimesters of pregnancy increases the risk of elevated birth weight of offspring (118, 119). The meta-analysis of clinical studies showed that excessive gestational weight gain increased the risk of childhood obesity by 33% (120). The proposed mechanisms underlying these associations include abnormal placental transfer, epigenetic mechanisms, and altered peripheral and central metabolic profile in offspring (114, 115).
A higher pre-pregnancy BMI also increases the total body fat mass during childhood, and abdominal subcutaneous and preperitoneal fat mass (121, 122). Eshrigui et al. (31) indicated a direct linkage between the maternal pre-pregnancy BMI and the fat mass index, the percentage of body fat, the visceral adipose tissue, and the android-to-gynoid fat ratio of offspring.
Cohort clinical studies showed that metabolic disorders resulting from the obesity of offspring were sex-specific (23). The male but not female offspring exposed to gestational diabetes mellitus (GDM) and/or obesity had a higher BMI and were more likely to develop obesity from late childhood to early adulthood (123). Maternal glycaemia appears to be a major factor initiating adiposity in male infants, whereas the maternal BMI is the main predictor in female infants (23). Moreover, Chaparo et al. and Eshriqui et al. found that the maternal pre-pregnancy BMI was directly associated with a greater fat mass in daughters, but not sons (31, 32).
Effects of Maternal Undernutrition on Body Weight and Fat Content: Animal Studies
Numerous studies assessing the effects of malnutrition on a number of animal models have shown that either food restriction (FR) or protein restriction (PR) can programme offspring to altered adiposity and body weight at different stages of gestation and postnatal life.
In rats both paradigms of maternal malnutrition (regardless of the timeframe of undernutrition, whether energy restriction lasted throughout gestation or only part of this period) often resulted in a lower birth weight (up to ~40%) (124–127). However, most of these studies showed that despite the lower birth weight observed in the offspring, these animals were often characterised by increased adiposity, gain of the adipose tissue or adipocyte size at later stages of life (124, 128, 129). Few studies showed that a lower body weight could persist for several days, weeks (126, 127, 130) or even for up to ten months, especially when the dam’s age was taken into consideration, as Ware et al. (131) observed. In their experiment dams were mated at different ages, i.e. at 2 and 4 months of age (the young maternal group), and at 6 and 9 months of age (the old maternal group). The animals in both age groups were fed a low protein (LP) diet (50% restriction) or a control diet throughout gestation. After weaning both the male and female offspring of these dams were fed a control diet until 9 months of age. After this period the offspring were fed either the control diet or an HFD (40% of fat) for 9 weeks. In general, at 10 months of age the male offspring of the animals fed the LP diet were smaller than the control counterparts. However, the male offspring of the older LP dams had over a 50% lower weight gain than the male offspring of the young LP mothers. The male offspring of the LP females also had less fat and a smaller adipocyte diameter than the animals in the control group, regardless of the dams’ age. On the other hand, although the female offspring exhibited similar changes in adiposity, the maternal diet had no effect on their body weight or weight gain at 10 months of age (131). The offspring of PR mothers also seemed to be more sensitive to the deleterious effects of the HFD as they exhibited apparent catch-up growth to match the body weight of the control counterparts fed the hypercaloric diet (125).
Available data also suggest that the detrimental in utero effects of maternal malnutrition can be transmitted transgenerationally, with altered glucose homeostasis (132), number of larger adipocytes (subcutaneous white adipose tissue on the abdomen) in generation F2 (males), as well as an increased area of GFAP-immunoreactive astrocytes, which is a marker of neuroinflammation (124). Importantly, these effects seem to be transmitted mainly through the maternal rather than paternal line (132).
It is also noteworthy that the majority of experiments on maternal malnutrition focused on male offspring. There is also some evidence [e.g. (14, 15, 131, 133)] that exposure to maternal undernutrition programmes the adipose tissue in a sex-dependent manner, as it seems to be the case in humans. Recently Christians et al. published a systematic review and meta-analysis, in which they analysed the effects of PR and FR on the physiological traits of offspring (e.g. body weight, body fat percentage, fat pad weight, concentration of blood lipids), and checked if any of these traits were more severely affected in either sex. In general, the meta-analysis showed that the birth weight of offspring was consistently reduced in both sexes, regardless of the stage of gestation when the PR or FR occurred. The authors also concluded that less than a half of the studies they analysed tested the interaction between the sex of the offspring and the maternal diet, which may have resulted in a higher incidence of false-positive sex-specific effects (87). However, a maternal FR diet in murine (9, 10): and rat (13) models resulted in a decrease in birth weight in both male and female offspring. Zambardo et al. have found that in the rat offspring of PR mothers, females after birth were lighter than males (15). Weight loss has also been reported in the adult rat offspring of PR mothers of both sexes (16), or in males only (14). Bellinger et al. have described that abdominal fat deposits increased only in rat males, but when it comes to gonadal fat – fat content increased only in females (14). Furthermore, Vega et al. have found that body fat content increased in juvenile rat females only (27), while Begum et al. showed that fat content increased in adult sheep males (29).
Effects of Maternal Undernutrition on Body Weight and Fat Content: Human Studies
World human conflicts and socio-geographical conditions resulted in times of famine and provided unique opportunities to study the influence of undernourishment during pregnancy on the health of offspring in childhood and adulthood (134, 135). Maternal malnutrition during pregnancy results in a small size of offspring at birth, and the low birth weight is interpreted as an indicator of foetal malnutrition (134–136). However, Roseboom et al., who analysed the children of the mothers who were pregnant during the Dutch famine, observed that the babies whose mothers were caught by famine in the first trimester of gestation, were heavier at birth than the population average before and after the period of starvation. On the other hand, the birth weights of the babies affected by the famine in late gestation were usually lower than the birth weights of the babies who were born before or conceived after the famine (137). Additionally, the exposure to the Dutch famine during gestation affected the sex ratio of liveborn babies. The percentage of boys born alive was lower, especially after the exposure to famine during late gestation (137). Interestingly, the offspring of prenatally undernourished fathers, but not mothers during the Dutch famine were heavier and more obese than the offspring of the fathers and mothers who had not been undernourished prenatally (138).
Researchers also observed that malnutrition during the first and second trimesters of pregnancy was associated with an increased prevalence of obesity (regardless of the birth weight) (137), whereas malnutrition in the third trimester correlated with a lower to normal weight in adult life (139, 140). Hence, exposure to famine in early gestation was associated with greater prevalence of obesity in adult life.
The aforementioned human studies showed that maternal overnutrition was associated with macrosomia and increased fat content in progeny, which predisposed them to metabolic imbalance later in life. There were sex-specific differences in these results. On the other hand, studies on humans showed that although the children of malnourished mothers had lower birth weights, they tended to be overweight and obese later in life. This relationship could be explained by the so-called ‘thrifty phenotype’ hypothesis, which proposes epidemiological associations between poor foetal and infant growth, and the subsequent development of T2DM and the metabolic syndrome. This stems from the effects of poor nutrition in early life, which leads to permanent changes in glucose-insulin metabolism (2, 141–143).
The sex-specific birth weight changes in human offspring of children of malnourished mothers have been also reported. Ntenda et al. (21) and Thurstans et al. (22) have found that boys developed greater decrease in body weight than girls after birth, while Sakisaka et al. (20) described the opposite effect where the lower birth weight was more pronounced in girls than in boys compared to the control groups. Andersen et al. have described that along with the decrease in weight in newborns, fat mass decreased in both sexes, however, later in life, fat mass increased and this effect was more pronounced in girls (30).
Effects of Maternal Overnutrition on Metabolic and Hormonal Status of Offspring: Animal Studies
Maternal overnutrition also affects the metabolic status of animals. The murine and rat offspring of HFD dams had higher blood glucose, TG and/or cholesterol levels (33, 144–147). Moreover, the offspring of obese rat dams fed an HFD, CAF or HSD had higher insulin, leptin, and adiposity levels (145, 146, 148, 149). Research showed that a CAF diet induced obesity more effectively and resulted in a more pronounced increase in the plasma leptin, TG, and cholesterol levels than the HFD protocol (150, 151). Moreover, the maternal HFD, HSD, and CAF diets resulted in a low-grade inflammatory phenotype in the rat offspring. They had higher levels of pro-inflammatory cytokines such as interleukins: IL-1β, IL-6, and tumour necrosis factor α (TNF-α), which mimics the situation observed in obese patients (152–154).
Overnutrition and/or obesity lead to a combination of peripheral and central nervous system alterations and affect the brain circuitry controlling energy homeostasis and adverse programming of central appetite regulators (149). Central leptin and insulin resistance is commonly observed in the offspring of obese HFD-fed rat females (149). Researchers observed changes in the brain appetite regulators, such as downregulated hypothalamic neuropeptide Y (NPY) and upregulated hypothalamic proopiomelanocortin POMC receptor expression in the offspring of HFD-fed rat females (149). Moreover, epigenetic markers at POMC are influenced by both maternal food excess and restriction [for a review see (155)].
Recently researchers have paid more attention to sex-specific differences in the offspring’s response to mother’s overnutrition. For example, there were sex-specific differences in the blood glucose levels of 25-day-old female offspring of dams fed a CAF diet. These animals had higher glucose levels than those in the control group (18). The concentration of insulin was elevated both in the male and female CAF offspring on postnatal day 25 (PND 25), but this rise in insulin levels was more pronounced in the females (18). Similarly, Bayol et al. observed that feeding rats a CAF diet during pregnancy, lactation, and post-weaning period resulted in sex-specific differences in the glucose and insulin levels in the offspring (33). The concentration of glucose in the ten-week-old female offspring of the rats fed a CAF diet was higher than in the control animals. However, in the studies conducted by Bayol and Matuszewska there was no difference in the glucose levels between the male offspring of the CAF rats and the animals in the control group. Khan et al. have shown that glucose and TG levels were raised only in female rat offspring of HFD mothers (35). Another study revealed sex-specific differences in insulin secretion and leptin transcription – the males had a higher circulating insulin level, whereas the females had an elevated leptin transcript level (33). Rodriguez et al. also observed maternal HSD-induced insulin resistance and oxidative stress in the male but not in the female rat offspring (34). Chowen et al. (156) also noted differences between the males and females in their response to obesogenic diets/environments and the possible implication of hypothalamic astrocytes.
Sanchez-Garrido et al. observed that obesity was transmitted to offspring in a sex-specific manner by the paternal line. The male, but not female offspring of rat fathers with HFD-induced obesity had higher body weights and leptin levels. However, these animals did not exhibit glucose intolerance. Moreover, the authors noted a decrease in the luteinising hormone (LH) levels and an exacerbated drop in the testosterone levels in the male offspring. On the other hand, the female offspring exhibited reduced LH response to kisspeptin-10 (157).
Effects of Maternal Overnutrition on Metabolic and Hormonal Status of Offspring: Human Studies
The children of obese mothers (BMI > 30 kg/m2) had higher percentage of body fat, systolic blood pressure, TG, and leptin levels, and developed insulin resistance at the age of 8 (121). An interim analysis of 898 obese and normal weight mothers and their offspring from the Programming of Enhanced Adiposity Risk in Childhood-Early Screening (PEACHES) (158) cohort study showed that the children of obese, GDM-negative mothers with late-pregnancy dysglycaemia had a worse outcome with higher weight gain (150–154) and a higher BMI in early childhood than the children of obese mothers treated for GDM (158). Furthermore, the Helsinki birth cohort study showed not only a positive correlation between the BMI of the mothers and offspring, but also a higher body fat percentage in the children of the mothers with a higher BMI (159). The study also showed that the higher maternal BMI increased the risk of the offspring’s death, cancer, stroke, coronary heart disease, and T2DM, with the latter two being the most strongly correlated. Apart from that, the risk of developing TDM2 was higher in women than men, while the incidence of coronary heart disease was greater in men (43).
Moreover, exposure to endocrine disruptors (EDs), which becomes increasingly widespread in the environment, influences weight regulation and has obesogenic effects on humans (160–163). EDs act as hormones at low but persistent doses and mostly mimic oestrogen properties. They activate or inactivate cellular receptors, cell responses, and other targets, and lead to higher insulin resistance and hyperinsulinaemia. EDs also change the adipokine level in a sex-specific way. For example, in the Canadian Maternal-Infant Research on Environmental Chemicals Study, newborns exhibited sex-specific differences in the leptin and adiponectin levels, caused by the maternal exposure to bisphenol A (BPA) in utero. The female offspring had higher leptin levels than males. By contrast, adiponectin did not differ between the sexes, but was inversely related to the BPA level in the males (164). The topic of environmental chemicals in the context of parental/early exposure to them and programming of obesity and diabetes type 2 is of great importance, but beyond the scope of this review. For reviews on this subject, see e.g. (165–167). Moreover, the concept of transgenerational inheritance – an endocrine of an adverse outcome after a chemical exposure was proposed in 2005 and discussed later by other researchers [e.g. (165, 168, 169)].
The risk factors leading to the development of T2DM also include sugar-sweetened beverages (SSBs). The meta-analysis of prospective cohort studies showed that the men and women drinking SSBs in the highest quantile had a 26% excess risk of developing T2DM than those in the lowest quantile (170).
Male and female offspring seem to adapt differently to the milieu created by maternal obesity and diabetes during pregnancy (171), which may be connected to the sex hormone regulation of several genes involved in both the physiological control of metabolism and the pathophysiological background of cardiometabolic diseases (172, 173). Shields et al. (37) and Krishnaveni et al. (38, 174) noted that girls not only had a higher cord blood insulin concentration at birth than their male peers, but when exposed to GDM in utero, they were more likely to develop insulin resistance later in life. Studies also suggest that androgen excess during pregnancy may play a crucial role in insulin resistance programming in female offspring (175). The teenage daughters of mothers with polycystic ovarian syndrome (PCOS) or congenital adrenal hyperplasia (CAH) had hyperinsulinaemia (176, 177).
Effects of Maternal Undernutrition on Metabolic and Hormonal Status of Offspring: Animal Studies
The offspring of undernourished rat dams tend to exhibit numerous metabolic and hormonal changes in the postnatal period. There are relatively frequent alterations in insulin signalling and glucose tolerance, transcriptional and protein changes in the adipose tissue, liver, and pancreas, as well as inflammatory markers. Changes in insulin signalling and glucose tolerance involve hyperglycaemia (either after fasting or in non-fasted animals), lower insulin sensitivity, as well as higher insulin increment during glucose tolerance test (GTT) (126, 128, 178). This metabolic imbalance can be accompanied by increased blood concentrations of TG and low-density lipoproteins (LDL), as well as decreased concentration of high-density lipoproteins (HDL) (128, 130). Moreover, there was a strong positive correlation between the plasma TG concentration and the body weight of the offspring of the dams fed a diet with 70% FR throughout the gestation (130).
According to data, the livers of the offspring of malnourished mothers are also highly affected, but the results vary across studies. On the one hand, the male offspring of rat dams subjected to severe PR in a diet (80% restriction in the diet protein content) during the second half of gestation exhibited hepatic steatosis at the age of 90 days (128), whereas Lecoutre et al. observed that 70% FR during gestation and lactation did not affect the liver mass or the content of liver lipids (also in male offspring, aged 4 months) (179). This observation is consistent with the results of the study conducted by Morris et al. on the male offspring (aged 110 days) of FR dams (70% FR throughout gestation), whose liver weight did not change, either. Interestingly, a lower liver weight was observed in a group of younger rats (aged 55 days). Transcriptional liver profiling in the group of younger animals revealed that although these individuals did not yet exhibit the characteristics of the metabolic syndrome phenotype that was observed in older rats, the results suggest that these animals might exhibit metabolic abnormalities in advance of the full metabolic syndrome phenotype observed later in life (i.e. at 110 days of age) (180). The effects of maternal malnutrition during pregnancy on the liver may also be transgenerational and transmitted mainly through the maternal line, as described by Hanafi et al. The F2 foetuses of F1 females fed an LP diet for 2 months after weaning (60% PR) exhibited an overexpression of GLUT2 and glucokinase (GK) in the liver. Moreover, these genes as well as UCP2 were also overexpressed in the pancreas, which suggests that these F2 offspring might be predisposed to diabetes later in life. Indeed, higher postnatal levels of fasting blood glucose, insulin, and HOMA-IR later in life (at the age of 20 and 30 weeks) indicated that maternal undernutrition significantly altered glucose tolerance in generation F2 (132).
Research has shown that maternal malnutrition highly affects the adipose tissue function. Guan et al. observed changes in 650 genes in the visceral adipose tissue in the male offspring of dams subjected to 60% PR throughout pregnancy and lactation. The analysis of the results revealed a global upregulation of the genes involved in carbohydrate, lipid, and protein metabolism, as well as adipocyte differentiation and angiogenesis. This suggests that maternal PR during gestation and lactation programmes the susceptibility to visceral adiposity later in life (181). Importantly, research findings also indicate that the restriction of the mother’s calorie intake (20% restriction, gestational days 1-12) significantly affects the function of the brown adipose tissue (BAT) in the offspring of both sexes (aged 25 days) (182). Maternal malnutrition may also induce epigenetic changes in the adipose tissue of the offspring, e.g. in the means of programming changes in the miRNA or CpG site methylation [for a review see (179)]. Sex-specific alterations (e.g. in the POMC expression) were observed in the offspring of food-restricted sheep. Begum et al. noted a lower POMC expression (accompanied by increased fat mass in adulthood) in the male adult sheep which had been undernourished during early pregnancy, but there was no difference in the females (29). In rats, offspring of PR mothers (both juvenile males and females) had elevated insulin and leptin levels, but TG levels were elevated only in males.
Effects of Maternal Undernutrition on Metabolic and Hormonal Status of Offspring: Human Studies
The spectrum of maternal malnutrition spans undernutrition with caloric and/or macro- and micronutrient restrictions during pregnancy. Research has shown that the timing of nutritional restriction seems to play an important role on the health of offspring later in life. Studies on the offspring of the mothers who were pregnant during the Dutch famine (183), Biafran (184), and Chinese (185) provided data on the consequences of maternal caloric restriction in offspring, who developed glucose intolerance, microalbuminuria, an atherogenic lipid profile with a higher LDL/HDL ratio, and hypercholesterolaemia (186). Roseboom et al. indicated that the exposure to the famine at an early gestational age was correlated with an increased risk of coronary heart disease, atherogenic lipid profiles, and higher adiposity (187). Painter et al. (188)observed that the exposure to the famine in the middle of gestation was related to microalbuminuria and impaired renal function. The children whose mothers were malnourished during pregnancy had lower insulin levels and insulin-like growth factor 1 (IGF-1) concentrations, but higher proteolytic activity of the insulin-like growth factor binding protein (the protein binding and regulating the IGF activity) immediately after birth. This may have affected the growth of the foetus (189–191). The proper nutrition of children after birth causes a rapid increase in the insulin and IGF-1 levels, which may result in a rapid weight gain, insulin resistance, and T2DM in adulthood (referred to a as the catch-up growth hypothesis) (189, 191).
Researchers found a correlation between the low birth weight and increased risk of developing overweight and obesity, altered body composition, T2DM, hypertension, and cardiovascular disease (24). This evidence is in line with the data from the aforementioned epidemiological studies on individuals exposed to the Dutch famine in utero. There was a correlation between the exposure to famine in utero and the development of diabetes (192), high blood pressure (193), and impaired body composition of the offspring later in life (24, 139).
Similar data were presented by Finer et al., who researched the metabolic outcomes of exposure to famine during developmental life in rural Bangladesh. Their research additionally showed that gestational and postnatal windows of exposure had variable effects on the offspring’s phenotype (194).
These data could be explained by the thrifty phenotype hypothesis, first described by Hales and Baker. According to the hypothesis, suboptimal nutrition in utero may result not only in impaired growth of the foetus and children being born small for gestational age/with intrauterine growth retardation (SGA/IUGR), but also in the reprogramming of metabolic pathways to ensure foetal survival in the challenging in utero milieu. However, when these individuals experience the postnatal environment with excessive or normal nutrition, they are more prone to develop impaired glucose tolerance (2, 141–143).
In summary, similarly to the animal data, the studies on humans showed marked alterations in the metabolic and hormonal profiles of the offspring of obese, diabetic, and undernourished mothers. There are emerging reports suggesting that female offspring (animal studies on overnutrition) and girls (human studies; exposure to GDM in utero) may be more vulnerable to insulin resistance later in life (36). However, further research on sex-specific differences needs to be conducted, especially to reveal the underlying mechanisms. Studies also have shown that the timing of nutritional restriction seems to play an important role on the health of offspring later in life.
Effects of Maternal Overnutrition on Offspring Cardiovascular System: Animal Studies
Animal studies, mainly on rodents (rats and mice) (12, 195), but also on sheep (196–198) and non-human primates showed that maternal overnutrition affects the cardiovascular system (199) (Figure 1). The maternal HFD caused cardiac hypertrophy and reduced the vascular density of the cardiac muscle in rodents (200, 201) and primates (199). Moreover, lactational overnutrition (resulting from the reduced litter size) altered the cardiac gene expression in rodents. This may impair cardiac anatomy/metabolism and increase the susceptibility to myocardial injury after an ischaemic insult (202). The potential mechanisms related to myocardial dysfunction include impaired cardiac insulin signalling, metabolic status, increased oxidative stress, inflammation, and mitochondrial dysfunction (200, 201). In the sheep model of maternal obesity, in which the offspring’s entire heart function was evaluated in the Langendorff model (an in vitro system), the contractile function of the heart was impaired (198). Moreover, the phosphorylation of AMP-activated protein kinase (a cardioprotective signalling pathway) was reduced in the hearts of the foetuses of obese mothers, but the stress-signalling pathway p38 MAPK was upregulated. This indicated impaired cardiac insulin signalling, as compared with the animals in the control group (198). In animal models (especially mice and rats) maternal obesity altered DNA methylation, which was responsible for the abnormal fat metabolism (203, 204), and histone acetylation in the promoter regions of adiponectin and leptin (107). These changes had the atherogenic effect because they increased the adhesion of particles circulating in the blood vessels. In consequence of these epigenetic changes the levels of LDL and free fatty acids increased and altered the nitric oxide synthase (NOS) function, which is a well-known factor in the development of hypertension (205). Maternal overnutrition may also alter specific gene expression and result in cardiomyocyte hypertrophy and abnormal heart development (206, 207).
The exposure of developing mouse and rat offspring to maternal obesity led to their hypertension in adulthood, which deteriorated with age (12, 208, 209). Maternal obesity also programmes the vascular system in offspring. Endothelial dysfunction was observed in the small mesenteric arteries of all offspring whose mothers consumed a fat-rich diet. This showed that this disorder commonly occurs with elevated blood pressure. Endothelial dysfunction may be a consequence of insulin resistance and could reflect the activation of inflammatory pathways as a result of increased adiposity (210).
Sex-specific differences were observed in the offspring of obese mice and rats. Both male and female offspring had elevated blood pressure, but it was more pronounced in the females (12, 35). These studies suggested that female offspring exhibited greater sensitivity to overnutritional insults during foetal life and the cardiovascular development. The following factors influence the development of hypertension in offspring: increased sympathetic activity, increased renal norepinephrine concentration and renin expression, as well as vascular conditions (12, 209, 211).
Effects of Maternal Overnutrition on Offspring Cardiovascular System: Human Studies
Multiple observational studies on humans revealed a correlation between maternal obesity before and during pregnancy and an increased risk of cardiovascular anomalies (114, 115, 212, 213). The exposure of the foetus to maternal obesity increased its blood pressure, altered the vascular system (endothelial function), and myocardial function (199, 214). Researchers observed a positive correlation between maternal obesity and/or gestational weight gain and higher systolic blood pressure in the offspring (114, 215–217). Moreover, a cohort study showed that a higher maternal BMI increased the risk of hospital admissions of adult offspring due to cardiovascular events (43, 213). Additionally, the vascular markers of endothelial dysfunction were increased in the children exposed to maternal diabetes and obesity during foetal life (218). Atherosclerosis may also be influenced by the maternal diet and/or maternal hypercholesterolaemia, which may cause the development of this disease in offspring (219). The inherent risk of hypertension and vascular dysfunction resulting from maternal obesity, cardiac hypertrophy, and contractile dysfunction is also observed in the offspring (200). Epidemiological studies on humans showed that epigenetic mechanisms were involved in the cardiological consequences of maternal obesity and/or diabetes (206). Moreover, the epigenetic mechanisms of altered metabolic control in the offspring of obese mothers may cause abnormal placental function, altered cholesterol circulation, and higher blood pressure in humans (206, 220, 221).
Only a few studies described the influence of sex on the programming of cardiovascular risk, following a pregnancy complicated by obesity and/or diabetes. Ericsson et al. noted a correlation between maternal obesity and stroke only in the female offspring (43). Aceti et al. conducted a systematic review and observed a strong correlation between maternal diabetes and increased blood pressure in the offspring during childhood, with a stronger correlation in the male rather than female offspring (44).
Effects of Maternal Undernutrition on Offspring Cardiovascular System: Animal Studies
Hypertension (40, 196, 222, 223), coronary heart disease/heart hypertrophy (40, 224), and vascular dysfunction (225) are among the disorders observed in the offspring of undernourished females in experimental animal models. A global restriction of maternal food intake (30-70% food restriction) during pregnancy resulted in arterial hypertension both in murine (222) and rat offspring (40, 226). There were similar observations made on sheep (196) and cows (223). Studies on pregnant rats (227, 228), mice (229), and sheep (230) showed that a reduced maternal dietary protein intake programmed hypertension in offspring. The elevated arterial blood pressure observed in these animals may have been caused by the increased cardiovascular sympathetic tone (227), suppressed activity of the hypothalamic-pituitary adrenal (HPA) axis, impaired function of the renin-angiotensin system (RAS) (231), and/or altered structure and function of the vessels and the cardiac muscle (40, 224). Torrens et al. and Ozaki et al. observed that the dietary protein restriction or global undernutrition in rats compromised the maternal cardiovascular adaptations to pregnancy and led to the endothelial and peripheral artery dysfunction in the offspring (40, 224). The sheep offspring exposed to a maternal LP diet throughout the foetal period developed right and left ventricular hypertrophy (232). Cardiac enlargement was also observed in the offspring of the rats fed an LP diet (233). In addition, they had a lower heart weight, which was associated with an increased rate of cardiomyocyte apoptosis and a lower total number of cardiomyocytes per heart at birth (234). Maternal undernutrition also affects the epigenetic mechanisms that control and change the transcription of genes involved in the cardiovascular homeostasis. A study on rats showed that the offspring of the females which received a LP diet had incorrect histone modifications of a specific enzyme, and these changes were associated with an increase in the blood cholesterol level (235). A study on mice showed that the offspring of the females which received a restricted diet had cardiovascular diseases in adulthood (236). Studies on animals showed that a restrictive diet of rat mothers during pregnancy resulted in significant miRNA dysregulation in the offspring’s heart (237). Specific miRNA molecules responsible for maintaining the elasticity of blood vessels were inhibited (238), which reduced vascular contractility. Altered global DNA methylation was observed in the offspring of rodent females receiving a restrictive diet. It caused the dysregulation of the renin-angiotensin system in rats (239) and endothelium-dependent artery vasodilation in mice (225). Few of these epigenetic-dependent changes can induce the development of arterial hypertension in offspring. Barros et al. hypothesised that epigenetic mechanisms were involved in the overactivation of the sympathetic nervous system in the offspring of mothers fed an LP diet (227).
The authors of studies on the functioning of the circulatory system in the offspring of malnourished mothers did not usually describe sex-specific differences. However, Ozaki et al. found that hypertension developed more rapidly and severely in the male rather than female rat offspring exposed to a maternal LP diet during pregnancy (40). Additionally, male, but not the female mice offspring of malnourished mothers had elevated mean arterial pressure (MAP) (39). Elmes et al. observed that the effects of the mother’s LP diet on the increased blood pressure of rat offspring were not sex-specific (41).
Effects of Maternal Undernutrition on Offspring Cardiovascular System: Human Studies
For ethical reasons there is not much data on the influence of maternal malnutrition on cardiac disorders in their children. However, scientists try to analyse the effects of famine in different areas of the world. The analysis of data coming from the offspring of the mothers who starved during pregnancy (e.g. during the Dutch Hunger Winter and during the Second World War) indicate that exposure to famine during foetal life increases the risk of cardiovascular diseases (219, 240). Research has also shown that the people who were exposed to malnutrition in utero more often develop coronary heart disease and hypertension (241–243). According to the results of Le Clair et al. (42) and Barker (36), the risk of developing hypertension and coronary heart disease increases in both sexes. Maternal FR causes changes in miRNA, which alters the level of vascular endothelium-derived growth factor involved in the proper maturation and differentiation of the endothelium and its receptors (244).
To sum up, the data presented in the aforementioned animal studies on rodents, sheep, and non-human primates as well as the observations conducted on humans showing the effects of diet on the cardiovascular system clearly indicate that the maternal diet has influence on the cardiovascular system of offspring. Various mechanism responsible for these alterations have been proposed, with a special focus on epigenetic mechanisms. So far the sparse data from animal studies have suggested that female offspring exhibit greater sensitivity to overnutritional insults affecting the development of the cardiovascular system during foetal life. However, it is necessary to conduct further research, especially on animal models of undernutrition as well as the children of undernourished mothers, to analyse sex-specific differences.
Effects of Maternal Overnutrition on Brain and Behavioural Outcomes in Offspring: Animal Studies
Animal models of maternal overnutrition have provided evidence of persistent changes in offspring’s cognition and behaviour, and complemented human epidemiological data with potential insights into the mechanism linking maternal excessive food intake to adverse neurodevelopmental and psychiatric outcomes in offspring (Figure 1). Studies across different animal species indicated effects of maternal overnutrition on memory impairment e.g. working and spatial memory (mice 240, and rats 91, 241, 242).
The study by Robb et al. (49) confirmed a sexually dimorphic effect in the Morris water maze performance, where males performed worse than females. Graf et al. observed that the mice pups whose mothers consumed an HFD exhibited altered myelination and neurobehavioural deficits, and these effects were sex-dependent (47). The disturbed myelination in the medial cortex was observed in the male but not female offspring of HFD-fed dams. These structural changes were correlated with changes in the males’ behaviour only (assigned in the novel object recognition test).
Research has also shown the relationship between maternal obesity and depression. Young adult male rodents exposed to an HFD either in utero (48) or during lactation (50) exhibited higher rates of depression-like symptoms than the offspring of control group. The rodent offspring of the dams fed an HFD before mating and during gestation and lactation exhibited more anxiolytic behaviours than the control offspring (245, 246). The authors of some studies on animals also suggested that maternal obesity may increase the risk of neurodegenerative diseases such as Alzheimer’s disease in offspring (246, 247).
The following mechanisms might be responsible for behavioural alterations in the offspring of dams fed a CAF diet and HFD: reduced expression of neurotrophins, lower concentrations of synaptophysin and BDNF, which are involved in the development of memory (248, 249). Studies on rodents showed that maternal overnutrition hindered the neuronal growth and maturation by altering differentiation, neurogenesis, and disruption in apoptotic processes in such areas of the brain as the hippocampus, hypothalamus, and the cerebral cortex (102, 250, 251). Apart from the impaired neuronal anatomy and function, the offspring of HFD-fed dams exhibit altered neurotransmission (252). Research on rodents showed that the serotonin axon density and embryonic neuronal survival in the brain regions critical for behavioural regulation were reduced (250, 253). The offspring of female mice and rats fed an HFD had impaired mesolimbic dopaminergic signalling, which was associated with impaired reward response to food (254, 255). The levels of expression of the N-methyl-D-aspartate receptor (NMDA) receptor subunit NR2B in the hippocampus of rat offspring were significantly reduced in response to maternal overnutrition (249). The adult offspring of mice exposed to maternal obesity and an HFD during gestation and lactation were also characterised by increased hippocampal expression of GABAA receptor (256). Increased anxiety in the rat offspring resulted in higher glutamatergic activity in the prefrontal cortex. It was associated with reduced inhibitory input into the glutamate synapses through the cannabinoid/GABA receptors, whose expression decreased. These changes were sex-dependent, and they were more intense in the males than females (51). On the other hand, Kang et al. found that maternal HFD increased anxiety and decreased the sociability of the female offspring only (52). The study on non-human primates (Japanese macaques) showed that the maternal HFD caused behavioural changes in the female offspring, which exhibited higher anxiety. Instead, male exhibited increased aggression (257). On the other hand, the observed increase in the anxiety-like behaviour was not sex-specific in the murine (256) and rat (53) offspring of mothers fed an HFD.
Researchers have suggested multiple mechanisms underlying the effects of maternal HFD on the offspring’s brain, e.g. increased hippocampal lipid peroxidation, microglial activation in pups, and increased peripheral and central proinflammatory cytokine expression in the post-weaning and adult life (245). Other suggested mechanisms include: dysregulation of insulin, glucose, and leptin signalling in the developing brain (notably in the regions involved in behavioural regulation such as cortex, amygdala, thalamus, hippocampus and hypothalamus) (250, 258). Animal studies have also corroborated associations between maternal obesity, placental inflammation, foetal brain inflammation, and abnormal neurodevelopment in offspring (259). Moreover, these multidirectional changes associated with long-term effects of poor maternal diet are often accompanied by epigenetic changes [for details see the review by Moody et al. (252)]. It is important to note that there have been few studies on sex-specific differences in early programming of behaviour and brain function (as compared with data on males alone) and further research is necessary.
Effects of Maternal Overnutrition on Brain and Behavioural Outcomes in Offspring: Human Studies
According to the findings of recent studies on humans, maternal overnutrition/obesity affects the cognitive function and the development of neurological and psychiatric disorders in offspring (55). Research has provided evidence that there is a correlation between maternal overnutrition and/or obesity and the poorer cognitive performance of offspring, including lower IQ, poorer motor, spatial, and verbal skills (246, 260, 261). Maternal overweight is a predictor of children’s poorer psychosocial development, as evidenced by lower social competence and increased risk of depression and anxiety (262–264). Brain imaging techniques are very valuable in this respect, but they are still relatively underrepresented. An MRI study revealed a significant correlation between prenatal exposure to maternal obesity and a smaller hippocampal volume in boys but not girls (56). Moreover, sex-dependent differences were observed across hippocampal subfields (CA1, CA2/3, CA4, dentate gyrus, and subiculum) (56). Thus, the study suggested that boys may be more vulnerable than girls to the negative consequences of exposure to maternal obesity, as manifested by hippocampal development. There are also sex-dependent differences in susceptibility to neurodevelopmental disorders.
Exposure to maternal obesity also induces alterations in the HPA axis of offspring. Prenatal maternal overnutrition exposes offspring to maternal metabolism abnormalities (e.g. high levels of glucose, TG, and total cholesterol), which are associated with increased activity of the HPA axis, for example, in the means of changes in the children’s cortisol reactivity (262, 263). This is another possible mechanism through which maternal overnutrition may increase the risk of cognitive decline and anxiety-related disorders in offspring (265).
The authors of studies also suggest that there might be a correlation between maternal obesity and increased symptoms of ADHD (266) and autism spectrum disorder in children (267). The analysis of long-term human studies also showed a correlation between maternal obesity and the increased risk of offspring developing anorexia and/or bulimia later in life (268, 269).
Effects of Maternal Undernutrition on Brain and Behavioural Outcomes in Offspring: Animal Studies
Global caloric and/or protein restriction during gestation is correlated with deficits in exploration, social behaviour, emotionality, avoidance conditioning, learning, and memory in adult life (270–275). Batista et al. observed that maternal protein malnutrition caused autism spectrum disorder in rat offspring (276). Changes in the behaviour of the offspring of malnourished mothers were accompanied by neurochemical and neuroanatomical abnormalities such as: abnormal proliferation, apoptosis, astrogenesis, neuronal differentiation, dendritic arborisation and cerebral astrogliosis in the cortex and hippocampus (277, 278), and lower thickness of the visual cortex, parietal neocortex, dentate gyrus, CA3 region of the hippocampus and cerebellum (276, 279, 280). Experiments on rats also showed that FR during gestation reduced the essential factors enhancing neuronal proliferation, growth, and maintenance, e.g. BDNF and insulin-like growth factor (IGF) (252, 275). Additionally, the offspring of malnourished rats exhibited changes in their central neurochemical profiles, such as altered NMDA transmission and receptor composition, a decrease in the synaptic NO in the hippocampus (252, 281), enhanced GABA-ergic inputs to the hippocampus, and unfavourable changes in the noradrenergic, serotonergic, and dopaminergic transmission (252, 271, 282, 283). Consequently, nutrition-induced deficits in the synaptic transmission impaired memory formation and the LTP (long-term potentiation) (252). Barra et al. suggested that prenatal malnutrition-induced brain disturbances depend on two mechanisms: i) the direct effect of foetal programming on the brain, including negative epigenetic changes in foetal progenitor cells and developing neurons, which will form the cerebral cortex, hippocampus, and other brain regions involved in neuroplasticity, and ii) the indirect effect on the brain mediated by the postnatal development of obesity/metabolic syndrome (271).
Rodriguez et al. conducted research on baboons, which have a similar genetic constitution and developmental trajectory to humans. The researchers observed that moderate global nutrient restriction during pregnancy and lactation affected the development of the offspring brain in a sex-specific manner (45). The baboon offspring exposed to moderate maternal nutrient restriction were less motivated and their working memory was impaired. The female offspring learnt better than the males, whose learning was impaired and they were more impulsive (45).
Natt et al. have described the enhancement of anxiety and depressive behaviour in male, but not female mice offspring from PR mothers (46). However, Akitake et al. have described a decrease in motor activity and recognition memory in male and female mice, while hippocampal synaptophysin level was decreased only in females (10). Additionally, Bellinger et al. in their study on rat offspring of LP mothers, have found that locomotor activity was impaired in males but feeding behaviour was impaired in females (14).
Effects of Maternal Undernutrition on Brain and Behavioural Outcomes in Offspring: Human Studies
Two meta-analyses described the effects of maternal malnutrition on the learning, memory, and behaviour of the schoolchildren who were small for their gestational age. These children exhibited cognitive impairments, lower verbal and performance IQ, and more behavioural disorders than the children in the control group (271, 284, 285).
Researchers also observed that prenatal exposure to famine (during the Dutch famine) permanently affected the size of the brain, and these changes were sex-dependent. A structural magnetic resonance imaging was conducted on the Dutch famine birth cohort members when they were about 67 years old (54). The MRI showed that the total brain volume as well as the volumes of grey and white matter were smaller only in the early exposed males. The intracranial and total brain volumes of the prenatally exposed males were smaller than the volumes of the control subjects. Such changes were not observed in the females (54). Smith and Reyes described that sons of malnourished mothers are more likely to develop ASD and ADHD than daughters (55).
To sum up, the results discussed above showed that multiple animal models of over- and undernutrition as well as the data obtained in human studies provide convincing insight supporting the influence of an unhealthy maternal diet on the development of the brain and behavioural outcomes in offspring. The authors of these studies also suggested sex-specific responses to maternal diets and proposed various mechanisms underlying such divergences, among which the epigenetic ones have been the most explored recently. Imaging techniques, such as MRI, are invaluable for further analysis of subtle differences in the brain structures of the offspring to obese mothers, with emphasis on possible sex-specific differences.
Conclusions and Future Directions – From Laboratory to Clinical Settings
This review provided the evidence based on epidemiological data and animal models that both maternal over- and undernutrition changes the metabolic profiles, alters the body weight and fat content, and influences the offspring’s brain development and behaviour. Moreover, the effects observed in offspring are long-lasting. There is a relatively wide range of scientific publications discussing changes in the peripheral nervous system (i.e. hormones and metabolites), but there is not much data on changes in the central nervous system (i.e. in the brain). Non-invasive imaging techniques such as MRI could help to further and more precisely investigate these changes not only within different brain structures but also in the peripheral organs (e.g. changes in the fat distribution). These techniques could be used to monitor the development of diseases and during treatments.
As results from the studies conducted on animals and humans, there are sex-specific differences in the effects of the maternal diet on numerous physiological and anatomical traits. Therefore, further research should be conducted, especially on the epigenetic mechanisms through which both maternal over- and undernutrition affects offspring. These epigenetic processes, including DNA methylation, histone modification, chromatin remodelling, and RNA-based mechanisms may affect gene expression and change the foetal neuroendocrine system through modification of the brain circuitry (286). Epigenetic testing could be used in clinical settings as a biomarker for early diagnosis of the risk of obesity and as a predictor of response to obesity interventions. However, in epigenetic studies on humans it is a challenge to select relevant tissues accessible to examination such as peripheral blood cells (PBC), buccal epithelial cells (BEC), or hair follicles. As both maternal and paternal history and experiences exert influence through the epigenomic information which is not contained in the DNA sequence, including variations in sperm and oocyte cytosine methylation and chromatin patterning, the influence of non-coding RNAs and mitochondria of both maternal and paternal lines should be considered (287, 288). Currently, very little is known about the effects of the paternal metabolic status on the outcomes of offspring. Moreover, the mechanisms involved in the intergenerational transmission of undernutrition, obesity, and diabetes are difficult to study in humans because of the complex relationships between the maternal and foetal conditions, and the postnatal environmental factors.
Therefore, it is also necessary to continue using relevant animal models of metabolic diseases. As the consumption of SSBs is a high risk factor for the development of T2DM, and due to the fact that endocrine disruptors lead to the development of metabolic diseases, animal models with these variables should be developed and employed. Although animal models will never be able to fully mimic the human situation, they make it possible to study the mechanisms through which the mother’s diet programmes the organs and systems of the offspring. Moreover, thanks to animal models of obesity, diabetes, and undernutrition it is possible to search for prevention and treatment strategies, and to increase the awareness of sex-specific risk factors. It is necessary to conduct further research on the sex-dependent pathophysiological mechanisms of undernutrition, obesity, and T2DM, because it could contribute to more personalised care in the future.
The subject of this review seems to be particularly relevant at the time of the pandemic and the post-COVID period, when lockdowns reduce people’s physical activity and increase the consumption of unhealthy food products, which results in higher incidence of obesity. Therefore, it is necessary to develop and implement local, national, and international prevention and treatment strategies to slow down the rate of incidence of obesity. These insights compel us to revise generally held notions to accommodate the prospect that biological parenting, including proper diet, commences well before birth, even prior to conception. As the womb may be more important than home, it is also necessary to urgently implement these strategies long before conception as well as during the crucial and vulnerable times of pregnancy and lactation.
Author Contributions
EG wrote the draft of the manuscript and especially the following sections: Animal models of obesity with special emphasis on the diet-induced obesity, Effects of maternal overnutrition on body weight and fat content: animal and human studies, Effects of maternal undernutrition on body weight and fat content: human studies, Effects of maternal overnutrition on metabolic and hormonal status of offspring. animal studies, Effects of maternal overnutrition and undermatron on the cardiovascular system in offspring: animal and human studies, Effects of maternal overnutrition and undernutrition on the brain and behavioral outcomes in offspring: animal and human studies. Prepared the figure. JM–collected and formatted literature database. KZ wrote the following sections: Animal models of undernutrition, Effects of maternal undernutrition on body weight and fat content, and metabolic and hormonal status of offspring: animal studies, edited the whole text. AG-K, IK- P, and BS wrote the following sections: Effects of maternal overnutrition on metabolic and hormonal status of offspring: human studies, Effects of maternal undernutrition on metabolic and hormonal status of offspring: human studies and contributed to the Conclusion section; JHS-wrote the following sections: Introduction, Animal models of diabetes, conclusions, overviewed the process of writing the paper. All authors contributed to the article and approved the submitted version.
Funding
The authors acknowledge the National Science Centre PRELUDIUM grant No. 2018/31/N/NZ4/00518 awarded to KZ, grant No. 2019/35/N/NZ9/00663 awarded to JM and the statutory funding (No. 506-511-09-00) from the Faculty of Veterinary Medicine and Animal Science, Poznań University of Life Sciences, Poland.
Conflict of Interest
The authors declare that the research was conducted in the absence of any commercial or financial relationships that could be construed as a potential conflict of interest.
Publisher’s Note
All claims expressed in this article are solely those of the authors and do not necessarily represent those of their affiliated organizations, or those of the publisher, the editors and the reviewers. Any product that may be evaluated in this article, or claim that may be made by its manufacturer, is not guaranteed or endorsed by the publisher.
Acknowledgments
We would like to thank all the researchers who contributed to this review. Due to the large number of scientific publications on the subject, especially those discussing animals models, we were not able to cite all available studies.
References
1. Barker DJ. The Fetal and Infant Origins of Adult Disease. BMJ (1990) 301(6761):1111. doi: 10.1136/bmj.301.6761.1111
2. Barker DJ, Hales CN, Fall CH, Osmond C, Phipps K, Clark PM. Type 2 (Non-Insulin-Dependent) Diabetes Mellitus, Hypertension and Hyperlipidaemia (Syndrome X): Relation to Reduced Fetal Growth. Diabetologia (1993) 36(1):62–7. doi: 10.1007/BF00399095
3. Butler MJ, Barrientos RM. The Impact of Nutrition on COVID-19 Susceptibility and Long-Term Consequences. Brain Behav Immun (2020) 87:53–4. doi: 10.1016/j.bbi.2020.04.040
4. Petrilli CM, Jones SA, Jeam Y. Factors Associated With Hospitalization and Critical Illness Among 4,103 Patients With COVID-19 Disease in New York. BMJ (2020) 369:m1966. doi: 10.1101/2020.04.08.20057794
5. Cummings MJ, Baldwin MR, Abrams D, Jacobson SD, Meyer BJ, Balough EM, et al. Epidemiology, Clinical Course, and Outcomes of Critically Ill Adults With COVID-19 in New York City: A Prospective Cohort Study. Lancet (2020) 395(10239):1763–70. doi: 10.1016/S0140-6736(20)31189-2
6. Favre G, Legueult K, Pradier C, Raffaelli C, Ichai C, Iannelli A, et al. Visceral Fat Is Associated to the Severity of COVID-19. Metabolism (2021) 115:154440. doi: 10.1016/j.metabol.2020.154440
7. Padmanabhan V, Cardoso RC, Puttabyatappa M. Developmental Programming, a Pathway to Disease. Endocrinology (2016) 157(4):1328–40. doi: 10.1210/en.2016-1003
8. Sullivan EL, Smith MS, Grove KL. Perinatal Exposure to High-Fat Diet Programs Energy Balance, Metabolism and Behavior in Adulthood. Neuroendocrinology (2011) 93(1):1–8. doi: 10.1159/000322038
9. Jimenez-Chillaron JC, Isganaitis E, Charalambous M, Gesta S, Pentinat-Pelegrin T, Faucette RR, et al. Intergenerational Transmission of Glucose Intolerance and Obesity by In Utero Undernutrition in Mice. Diabetes (2009) 58(2):460–8. doi: 10.2337/db08-0490
10. Akitake Y, Katsuragi S, Hosokawa M, Mishima K, Ikeda T, Miyazato M, et al. Moderate Maternal Food Restriction in Mice Impairs Physical Growth, Behavior, and Neurodevelopment of Offspring. Nutr Res (2015) 35(1):76–87. doi: 10.1016/j.nutres.2014.10.014
11. King V, Dakin RS, Liu L, Hadoke PW, Walker BR, Seckl JR, et al. Maternal Obesity has Little Effect on the Immediate Offspring But Impacts on the Next Generation. Endocrinology (2013) 154(7):2514–24. doi: 10.1210/en.2013-1013
12. Samuelsson AM, Matthews PA, Argenton M, Christie MR, McConnell JM, Jansen EH, et al. Diet-Induced Obesity in Female Mice Leads to Offspring Hyperphagia, Adiposity, Hypertension, and Insulin Resistance: A Novel Murine Model of Developmental Programming. Hypertension (2008) 51(2):383–92. doi: 10.1161/HYPERTENSIONAHA.107.101477
13. Sánchez-Garrido MA, Castellano JM, Ruiz-Pino F, Garcia-Galiano D, Manfredi-Lozano M, Leon S, et al. Metabolic Programming of Puberty: Sexually Dimorphic Responses to Early Nutritional Challenges. Endocrinology (2013) 154(9):3387–400. doi: 10.1210/en.2012-2157
14. Bellinger L, Sculley DV, Langley-Evans SC. Exposure to Undernutrition in Fetal Life Determines Fat Distribution, Locomotor Activity and Food Intake in Ageing Rats. Int J Obes (Lond) (2006) 30(5):729–38. doi: 10.1038/sj.ijo.0803205
15. Zambrano E, Bautista CJ, Deas M, Martinez-Samayoa PM, Gonzalez-Zamorano M, Ledesma H, et al. A Low Maternal Protein Diet During Pregnancy and Lactation has Sex- and Window of Exposure-Specific Effects on Offspring Growth and Food Intake, Glucose Metabolism and Serum Leptin in the Rat. J Physiol (2006) 571(Pt 1):221–30. doi: 10.1113/jphysiol.2005.100313
16. Woods LL, Weeks DA, Rasch R. Programming of Adult Blood Pressure by Maternal Protein Restriction: Role of Nephrogenesis. Kidney Int (2004) 65(4):1339–48. doi: 10.1111/j.1523-1755.2004.00511.x
17. Nivoit P, Morens C, Van Assche FA, Jansen E, Poston L, Remacle C, et al. Established Diet-Induced Obesity in Female Rats Leads to Offspring Hyperphagia, Adiposity and Insulin Resistance. Diabetologia (2009) 52(6):1133–42. doi: 10.1007/s00125-009-1316-9
18. Matuszewska J, Zalewski T, Klimaszyk A, Ziarniak K, Jurga S, Chmurzynska A, et al. Mothers’ Cafeteria Diet Induced Sex-Specific Changes in Fat Content, Metabolic Profiles, and Inflammation Outcomes in Rat Offspring. Sci Rep (2021) 11(1):18573. doi: 10.1038/s41598-021-97487-x
19. Jacobs S, Teixeira DS, Guilherme C, da Rocha CF, Aranda BC, Reis AR, et al. The Impact of Maternal Consumption of Cafeteria Diet on Reproductive Function in the Offspring. Physiol Behav (2014) 129:280–6. doi: 10.1016/j.physbeh.2014.03.003
20. Sakisaka K, Wakai S, Kuroiwa C, Cuadra Flores L, Kai I, Mercedes Aragón M, et al. Nutritional Status and Associated Factors in Children Aged 0-23 Months in Granada, Nicaragua. Public Health (2006) 120(5):400–11. doi: 10.1016/j.puhe.2005.10.018
21. Ntenda PAM, Chuang YC. Analysis of Individual-Level and Community-Level Effects on Childhood Undernutrition in Malawi. Pediatr Neonatol (2018) 59(4):380–9. doi: 10.1016/j.pedneo.2017.11.019
22. Thurstans S, Opondo C, Seal A, Wells J, Khara T, Dolan C, et al. Boys Are More Likely to be Undernourished Than Girls: A Systematic Review and Meta-Analysis of Sex Differences in Undernutrition. BMJ Glob Health (2020) 5(12):e004030. doi: 10.1136/bmjgh-2020-004030
23. Lingwood BE, Henry AM, d’Emden MC, Fullerton AM, Mortimer RH, Colditz PB, et al. Determinants of Body Fat in Infants of Women With Gestational Diabetes Mellitus Differ With Fetal Sex. Diabetes Care (2011) 34(12):2581–5. doi: 10.2337/dc11-0728
24. Sutton EF, Gilmore LA, Dunger DB, Heijmans BT, Hivert MF, Ling C, et al. Developmental Programming: State-of-the-Science and Future Directions-Summary From a Pennington Biomedical Symposium. Obes (Silver Spring) (2016) 24(5):1018–26. doi: 10.1002/oby.21487
25. Dahlhoff M, Pfister S, Blutke A, Rozman J, Klingenspor M, Deutsch MJ, et al. Peri-Conceptional Obesogenic Exposure Induces Sex-Specific Programming of Disease Susceptibilities in Adult Mouse Offspring. Biochim Biophys Acta (2014) 1842(2):304–17. doi: 10.1016/j.bbadis.2013.11.021
26. Masuyama H, Hiramatsu Y. Additive Effects of Maternal High Fat Diet During Lactation on Mouse Offspring. PloS One (2014) 9(3):e92805. doi: 10.1371/journal.pone.0092805
27. Vega CC, Reyes-Castro LA, Rodríguez-González GL, Bautista CJ, Vázquez-Martínez M, Larrea F, et al. Resveratrol Partially Prevents Oxidative Stress and Metabolic Dysfunction in Pregnant Rats Fed a Low Protein Diet and Their Offspring. J Physiol (2016) 594(5):1483–99. doi: 10.1113/JP271543
28. George G, Draycott SAV, Muir R, Clifford B, Elmes MJ, Langley-Evans SC. Exposure to Maternal Obesity During Suckling Outweighs In Utero Exposure in Programming for Post-Weaning Adiposity and Insulin Resistance in Rats. Sci Rep (2019) 9(1):10134. doi: 10.1038/s41598-019-46518-9
29. Begum G, Davies A, Stevens A, Oliver M, Jaquiery A, Challis J, et al. Maternal Undernutrition Programs Tissue-Specific Epigenetic Changes in the Glucocorticoid Receptor in Adult Offspring. Endocrinology (2013) 154(12):4560–9. doi: 10.1210/en.2013-1693
30. Andersen GS, Girma T, Wells JC, Kæstel P, Leventi M, Hother AL, et al. Body Composition From Birth to 6 Mo of Age in Ethiopian Infants: Reference Data Obtained by Air-Displacement Plethysmography. Am J Clin Nutr (2013) 98(4):885–94. doi: 10.3945/ajcn.113.063032
31. Eshriqui I, Valente AMM, Folchetti LD, de Almeida-Pititto B, Ferreira SRG. Pre-Pregnancy BMI Is Associated With Offspring Body Composition in Adulthood Before Adiposity-Related Disorders: A Retrospective Cohort. Public Health Nutr (2021) 24(6):1296–303. doi: 10.1017/S1368980020005285
32. Chaparro MP KI, Byberg L. Maternal Prepregnancy BMI and Offspring Body Composition in Young Adulthood: The Modifying Role of Offspring Sex and Birth Order. Public Health Nutr (2018) 20:3084–9. doi: 10.1017/S1368980017002191
33. Bayol SA, Simbi BH, Bertrand JA, Stickland NC. Offspring From Mothers Fed a ‘Junk Food’ Diet in Pregnancy and Lactation Exhibit Exacerbated Adiposity That Is More Pronounced in Females. J Physiol (2008) 586(13):3219–30. doi: 10.1113/jphysiol.2008.153817
34. Rodriguez L, Otero P, Panadero MI, Rodrigo S, Alvarez-Millan JJ, Bocos C. Maternal Fructose Intake Induces Insulin Resistance and Oxidative Stress in Male, But Not Female, Offspring. J Nutr Metab (2015) 2015:158091. doi: 10.1155/2015/158091
35. Khan IY, Taylor PD, Dekou V, Seed PT, Lakasing L, Graham D, et al. Gender-Linked Hypertension in Offspring of Lard-Fed Pregnant Rats. Hypertension (2003) 41(1):168–75. doi: 10.1161/01.HYP.0000047511.97879.FC
36. Barker DJ. Fetal Origins of Coronary Heart Disease. BMJ (1995) 311(6998):171–4. doi: 10.1136/bmj.311.6998.171
37. Shields BM, Knight B, Hopper H, Hill A, Powell RJ, Hattersley AT, et al. Measurement of Cord Insulin and Insulin-Related Peptides Suggests That Girls Are More Insulin Resistant Than Boys at Birth. Diabetes Care (2007) 30(10):2661–6. doi: 10.2337/dc06-1501
38. Krishnaveni GV, Veena SR, Hill JC, Kehoe S, Karat SC, Fall CH. Intrauterine Exposure to Maternal Diabetes Is Associated With Higher Adiposity and Insulin Resistance and Clustering of Cardiovascular Risk Markers in Indian Children. Diabetes Care (2010) 33(2):402–4. doi: 10.2337/dc09-1393
39. Roghair RD, Segar JL, Volk KA, Chapleau MW, Dallas LM, Sorenson AR, et al. Vascular Nitric Oxide and Superoxide Anion Contribute to Sex-Specific Programmed Cardiovascular Physiology in Mice. Am J Physiol Regul Integr Comp Physiol (2009) 296(3):R651–62. doi: 10.1152/ajpregu.90756.2008
40. Ozaki T, Nishina H, Hanson MA, Poston L. Dietary Restriction in Pregnant Rats Causes Gender-Related Hypertension and Vascular Dysfunction in Offspring. J Physiol (2001) 530(Pt 1):141–52. doi: 10.1111/j.1469-7793.2001.0141m.x
41. Elmes MJ, Gardner DS, Langley-Evans SC. Fetal Exposure to a Maternal Low-Protein Diet Is Associated With Altered Left Ventricular Pressure Response to Ischaemia-Reperfusion Injury. Br J Nutr (2007) 98(1):93–100. doi: 10.1017/S000711450769182X
42. Le Clair C, Abbi T, Sandhu H, Tappia PS. Impact of Maternal Undernutrition on Diabetes and Cardiovascular Disease Risk in Adult Offspring. Can J Physiol Pharmacol (2009) 87(3):161–79. doi: 10.1139/Y09-006
43. Eriksson JG, Sandboge S, Salonen MK, Kajantie E, Osmond C. Long-Term Consequences of Maternal Overweight in Pregnancy on Offspring Later Health: Findings From the Helsinki Birth Cohort Study. Ann Med (2014) 46(6):434–8. doi: 10.3109/07853890.2014.919728
44. Aceti A, Santhakumaran S, Logan KM, Philipps LH, Prior E, Gale C, et al. The Diabetic Pregnancy and Offspring Blood Pressure in Childhood: A Systematic Review and Meta-Analysis. Diabetologia (2012) 55(11):3114–27. doi: 10.1007/s00125-012-2689-8
45. Rodriguez JS, Bartlett TQ, Keenan KE, Nathanielsz PW, Nijland MJ. Sex-Dependent Cognitive Performance in Baboon Offspring Following Maternal Caloric Restriction in Pregnancy and Lactation. Reprod Sci (2012) 19(5):493–504. doi: 10.1177/1933719111424439
46. Nätt D, Barchiesi R, Murad J, Feng J, Nestler EJ, Champagne FA, et al. Perinatal Malnutrition Leads to Sexually Dimorphic Behavioral Responses With Associated Epigenetic Changes in the Mouse Brain. Sci Rep (2017) 7(1):11082. doi: 10.1038/s41598-017-10803-2
47. Graf AE LS, Waidyaratne G, Thompson MD, Tipple TE, Hester ME, Trask AJ, et al. Maternal High Fat Diet Exposure Is Associated With Increased Hepcidin Levels, Decreased Myelination, and Neurobehavioral Changes in Male Offspring. Brain Behav Immun (2016) 58:369–78. doi: 10.1016/j.bbi.2016.08.005
48. Balsevich G, Baumann V, Uribe A, Chen A, Schmidt MV. Prenatal Exposure to Maternal Obesity Alters Anxiety and Stress Coping Behaviors in Aged Mice. Neuroendocrinology (2016) 103(3-4):354–68. doi: 10.1159/000439087
49. Robb JL, Messa I, Lui E, Yeung D, Thacker J, Satvat E, et al. A Maternal Diet High in Saturated Fat Impairs Offspring Hippocampal Function in a Sex-Specific Manner. Behav Brain Res (2017) 326:187–99. doi: 10.1016/j.bbr.2017.02.049
50. Giriko CA, Andreoli CA, Mennitti LV, Hosoume LF, Souto Tdos S, Silva AV, et al. Delayed Physical and Neurobehavioral Development and Increased Aggressive and Depression-Like Behaviors in the Rat Offspring of Dams Fed a High-Fat Diet. Int J Dev Neurosci (2013) 31(8):731–9. doi: 10.1016/j.ijdevneu.2013.09.001
51. Rivera P, Tovar R, Ramirez-Lopez MT, Navarro JA, Vargas A, Suarez J, et al. Sex-Specific Anxiety and Prefrontal Cortex Glutamatergic Dysregulation Are Long-Term Consequences of Pre-and Postnatal Exposure to Hypercaloric Diet in a Rat Model. Nutrients (2020) 12(6). doi: 10.3390/nu12061829
52. Kang SS, Kurti A, Fair DA, Fryer JD. Dietary Intervention Rescues Maternal Obesity Induced Behavior Deficits and Neuroinflammation in Offspring. J Neuroinflamm (2014) 11:156. doi: 10.1186/s12974-014-0156-9
53. Abuaish S, Spinieli RL, McGowan PO. Perinatal High Fat Diet Induces Early Activation of Endocrine Stress Responsivity and Anxiety-Like Behavior in Neonates. Psychoneuroendocrinology (2018) 98:11–21. doi: 10.1016/j.psyneuen.2018.08.003
54. de Rooij SR, Caan MW, Swaab DF, Nederveen AJ, Majoie CB, Schwab M, et al. Prenatal Famine Exposure has Sex-Specific Effects on Brain Size. Brain (2016) 139(Pt 8):2136–42. doi: 10.1093/brain/aww132
55. Smith BL, Reyes TM. Offspring Neuroimmune Consequences of Maternal Malnutrition: Potential Mechanism for Behavioral Impairments That Underlie Metabolic and Neurodevelopmental Disorders. Front Neuroendocrinol (2017) 47:109–22. doi: 10.1016/j.yfrne.2017.07.007
56. Alves JM, Luo S, Chow T, Herting M, Xiang AH, Page KA. Sex Differences in the Association Between Prenatal Exposure to Maternal Obesity and Hippocampal Volume in Children. Brain Behav (2020) 10(2):e01522. doi: 10.1002/brb3.1522
57. Barrett P, Mercer JG, Morgan PJ. Preclinical Models for Obesity Research. Dis Model Mech (2016) 9(11):1245–55. doi: 10.1242/dmm.026443
58. Bray GA, York DA. Hypothalamic and Genetic Obesity in Experimental Animals: An Autonomic and Endocrine Hypothesis. Physiol Rev (1979) 59(3):719–809. doi: 10.1152/physrev.1979.59.3.719
59. Kleinert M, Clemmensen C, Hofmann SM, Moore MC, Renner S, Woods SC, et al. Animal Models of Obesity and Diabetes Mellitus. Nat Rev Endocrinol (2018) 14(3):140–62. doi: 10.1038/nrendo.2017.161
60. Lutz TA, Woods SC. Overview of Animal Models of Obesity. Curr Protoc Pharmacol (2012) Chapter 5:Unit5 61.
61. Pinheiro-Castro N, Silva L, Novaes GM, Ong TP. Hypercaloric Diet-Induced Obesity and Obesity-Related Metabolic Disorders in Experimental Models. Adv Exp Med Biol (2019) 1134:149–61. doi: 10.1007/978-3-030-12668-1_8
62. de Moura EDM, Dos Reis SA, da Conceicao LL, Sediyama C, Pereira SS, de Oliveira LL, et al. Diet-Induced Obesity in Animal Models: Points to Consider and Influence on Metabolic Markers. Diabetol Metab Syndr (2021) 13(1):32. doi: 10.1186/s13098-021-00647-2
63. Hariri N, Thibault L. High-Fat Diet-Induced Obesity in Animal Models. Nutr Res Rev (2010) 23(2):270–99. doi: 10.1017/S0954422410000168
64. Marques C, Meireles M, Norberto S, Leite J, Freitas J, Pestana D, et al. High-Fat Diet-Induced Obesity Rat Model: A Comparison Between Wistar and Sprague-Dawley Rat. Adipocyte (2016) 5(1):11–21. doi: 10.1080/21623945.2015.1061723
65. Small L, Brandon AE, Turner N, Cooney GJ. Modeling Insulin Resistance in Rodents by Alterations in Diet: What Have High-Fat and High-Calorie Diets Revealed? Am J Physiol Endocrinol Metab (2018) 314(3):E251–E65. doi: 10.1152/ajpendo.00337.2017
66. Omar B, Pacini G, Ahren B. Differential Development of Glucose Intolerance and Pancreatic Islet Adaptation in Multiple Diet Induced Obesity Models. Nutrients (2012) 4(10):1367–81. doi: 10.3390/nu4101367
67. Lalanza JF, Snoeren EMS. The Cafeteria Diet: A Standardized Protocol and Its Effects on Behavior. Neurosci Biobehav Rev (2021) 122:92–119. doi: 10.1016/j.neubiorev.2020.11.003
68. Dyer JS, Rosenfeld CR. Metabolic Imprinting by Prenatal, Perinatal, and Postnatal Overnutrition: A Review. Semin Reprod Med (2011) 29(3):266–76. doi: 10.1055/s-0031-1275521
69. Jiang X, Ma H, Wang Y, Liu Y. Early Life Factors and Type 2 Diabetes Mellitus. J Diabetes Res (2013) 2013:485082. doi: 10.1155/2013/485082
70. Mucellini AB, Goularte JF, de Araujo da Cunha AC, Caceres RC, Noschang C, da Silva Benetti C, et al. Effects of Exposure to a Cafeteria Diet During Gestation and After Weaning on the Metabolism and Body Weight of Adult Male Offspring in Rats. Br J Nutr (2014) 111(8):1499–506. doi: 10.1017/S0007114513003838
71. Tamashiro KL, Moran TH. Perinatal Environment and Its Influences on Metabolic Programming of Offspring. Physiol Behav (2010) 100(5):560–6. doi: 10.1016/j.physbeh.2010.04.008
72. Mathis D VL, Benoist C. Beta-Cell Death During Progression to Diabetes. Nature (2001) 414:792–8. doi: 10.1038/414792a
73. Bellamy L CJ, Hingorani AD, Williams D. Type 2 Diabetes Mellitus After Gestational Diabetes: A Systematic Review and Meta-Analysis. Lancet (2009) 373:1773–9. doi: 10.1016/S0140-6736(09)60731-5
74. Szkudelski T. The Mechanism of Alloxan and Streptozotocin Action in B Cells of the Rat Pancreas. Physiol Res (2001) 50(6):537–46.
75. Dickinson JE, Meyer BA, Chmielowiec S, Palmer SM. Streptozocin-Induced Diabetes Mellitus in the Pregnant Ewe. Am J Obstet Gynecol (1991) 165(6 Pt 1):1673–7. doi: 10.1016/0002-9378(91)90013-H
76. Junod A, Lambert AE, Stauffacher W, Renold AE. Diabetogenic Action of Streptozotocin: Relationship of Dose to Metabolic Response. J Clin Invest (1969) 48(11):2129–39. doi: 10.1172/JCI106180
77. Ramsay TG, Wolverton CK, Steele NC. Alteration in IGF-I Mrna Content of Fetal Swine Tissues in Response to Maternal Diabetes. Am J Physiol (1994) 267(5 Pt 2):R1391–6. doi: 10.1152/ajpregu.1994.267.5.R1391
78. Tsai MY, Schallinger LE, Josephson MW, Brown DM. Disturbance of Pulmonary Prostaglandin Metabolism in Fetuses of Alloxan-Diabetic Rabbits. Biochim Biophys Acta (1982) 712(2):395–9. doi: 10.1016/0005-2760(82)90358-7
79. Skovso S. Modeling Type 2 Diabetes in Rats Using High Fat Diet and Streptozotocin. J Diabetes Investig (2014) 5(4):349–58. doi: 10.1111/jdi.12235
80. Fang J-Y, Lin C-H, Huang T-H, Chuang S-Y. In Vivo Rodent Models of Type 2 Diabetes and Their Usefulness for Evaluating Flavonoid Bioactivity. Nutrients (2019) 11(530):1–23. doi: 10.3390/nu11030530
81. Peters SAE, Woodward M. Sex Differences in the Burden and Complications of Diabetes. Curr Diabetes Rep (2018) 18(6):33. doi: 10.1007/s11892-018-1005-5
82. Rossini AA, Williams RM, Appel MC, Like AA. Sex Differences in the Multiple-Dose Streptozotocin Model of Diabetes. Endocrinology (1978) 103(4):1518–20. doi: 10.1210/endo-103-4-1518
83. Link JC, Reue K. Genetic Basis for Sex Differences in Obesity and Lipid Metabolism. Annu Rev Nutr (2017) 37:225–45. doi: 10.1146/annurev-nutr-071816-064827
84. Lovejoy JC, Sainsbury A, Stock Conference Working G. Sex Differences in Obesity and the Regulation of Energy Homeostasis. Obes Rev (2009) 10(2):154–67. doi: 10.1111/j.1467-789X.2008.00529.x
85. Jawerbaum A, White V. Animal Models in Diabetes and Pregnancy. Endocr Rev (2010) 31(5):680–701. doi: 10.1210/er.2009-0038
86. Swanson AM, David AL. Animal Models of Fetal Growth Restriction: Considerations for Translational Medicine. Placenta (2015) 36(6):623–30. doi: 10.1016/j.placenta.2015.03.003
87. Christians JK, Shergill HK, Albert AYK. Sex-Dependent Effects of Prenatal Food and Protein Restriction on Offspring Physiology in Rats and Mice: Systematic Review and Meta-Analyses. Biol Sex Differ (2021) 12(1):21. doi: 10.1186/s13293-021-00365-4
88. Lopes GA, Ribeiro VL, Barbisan LF, Marchesan Rodrigues MA. Fetal Developmental Programing: Insights From Human Studies and Experimental Models. J Matern Fetal Neonatal Med (2017) 30(6):722–8. doi: 10.1080/14767058.2016.1183635
89. dos Santos Oliveira L, de Lima DP, da Silva AA, da Silva MC, de Souza SL, Manhaes-de-Castro R. Early Weaning Programs Rats to Have a Dietary Preference for Fat and Palatable Foods in Adulthood. Behav Processes (2011) 86(1):75–80. doi: 10.1016/j.beproc.2010.09.005
90. Ghizoni H, Figueiredo PM, Moisan MP, Ogias D, Osaki LH, Gama P. Regulation of Corticosterone Function During Early Weaning and Effects on Gastric Cell Proliferation. Nutrition (2014) 30(3):343–9. doi: 10.1016/j.nut.2013.09.003
91. Kikusui T, Nakamura K, Kakuma Y, Mori Y. Early Weaning Augments Neuroendocrine Stress Responses in Mice. Behav Brain Res (2006) 175(1):96–103. doi: 10.1016/j.bbr.2006.08.007
92. de Moura EG, Bonomo IT, Nogueira-Neto JF, de Oliveira E, Trevenzoli IH, Reis AM, et al. Maternal Prolactin Inhibition During Lactation Programs for Metabolic Syndrome in Adult Progeny. J Physiol (2009) 587(Pt 20):4919–29. doi: 10.1113/jphysiol.2009.176289
93. Fraga MC, de Moura EG, da Silva Lima N, Lisboa PC, de Oliveira E, Silva JO, et al. Anxiety-Like, Novelty-Seeking and Memory/Learning Behavioral Traits in Male Wistar Rats Submitted to Early Weaning. Physiol Behav (2014) 124:100–6. doi: 10.1016/j.physbeh.2013.11.001
94. Lima NS, Moura EG, Franco JG, Pinheiro CR, Pazos-Moura CC, Cabanelas A, et al. Developmental Plasticity of Endocrine Disorders in Obesity Model Primed by Early Weaning in Dams. Horm Metab Res (2013) 45(1):22–30. doi: 10.1055/s-0032-1323703
95. Souza LL, de Moura EG, Lisboa PC. Does Early Weaning Shape Future Endocrine and Metabolic Disorders? Lessons From Animal Models. J Dev Orig Health Dis (2020) 11(5):441–51. doi: 10.1017/S2040174420000410
96. Glavas MM, Hui Q, Tuduri E, Erener S, Kasteel NL, Johnson JD, et al. Early Overnutrition Reduces Pdx1 Expression and Induces Beta Cell Failure in Swiss Webster Mice. Sci Rep (2019) 9(1):3619. doi: 10.1038/s41598-019-39177-3
97. Patterson CM, Bouret SG, Park S, Irani BG, Dunn-Meynell AA, Levin BE. Large Litter Rearing Enhances Leptin Sensitivity and Protects Selectively Bred Diet-Induced Obese Rats From Becoming Obese. Endocrinology (2010) 151(9):4270–9. doi: 10.1210/en.2010-0401
98. Khanal P, Nielsen MO. Impacts of Prenatal Nutrition on Animal Production and Performance: A Focus on Growth and Metabolic and Endocrine Function in Sheep. J Anim Sci Biotechnol (2017) 8:75. doi: 10.1186/s40104-017-0205-1
99. White CL, Pistell PJ, Purpera MN, Gupta S, Fernandez-Kim SO, Hise TL, et al. Effects of High Fat Diet on Morris Maze Performance, Oxidative Stress, and Inflammation in Rats: Contributions of Maternal Diet. Neurobiol Dis (2009) 35(1):3–13. doi: 10.1016/j.nbd.2009.04.002
100. Christante CM, Taboga SR, Pinto-Fochi ME, Goes RM. Maternal Obesity Disturbs the Postnatal Development of Gonocytes in the Rat Without Impairment of Testis Structure at Prepubertal Age. Reproduction (2013) 146(6):549–58. doi: 10.1530/REP-13-0037
101. Reynolds CM, Vickers MH, Harrison CJ, Segovia SA, Gray C. High Fat and/or High Salt Intake During Pregnancy Alters Maternal Meta-Inflammation and Offspring Growth and Metabolic Profiles. Physiol Rep (2014) 2(8):e12110. doi: 10.14814/phy2.12110
102. Niculescu MD, Lupu DS. High Fat Diet-Induced Maternal Obesity Alters Fetal Hippocampal Development. Int J Dev Neurosci (2009) 27(7):627–33. doi: 10.1016/j.ijdevneu.2009.08.005
103. Sasson IE, Vitins AP, Mainigi MA, Moley KH, Simmons RA. Pre-Gestational vs Gestational Exposure to Maternal Obesity Differentially Programs the Offspring in Mice. Diabetologia (2015) 58(3):615–24. doi: 10.1007/s00125-014-3466-7
104. Akyol A, Langley-Evans SC, McMullen S. Obesity Induced by Cafeteria Feeding and Pregnancy Outcome in the Rat. Br J Nutr (2009) 102(11):1601–10. doi: 10.1017/S0007114509990961
105. Crew RC, Waddell BJ, Mark PJ. Maternal Obesity Induced by a ‘Cafeteria’ Diet in the Rat Does Not Increase Inflammation in Maternal, Placental or Fetal Tissues in Late Gestation. Placenta (2016) 39:33–40. doi: 10.1016/j.placenta.2016.01.002
106. Sanchez-Blanco C, Amusquivar E, Bispo K, Herrera E. Influence of Cafeteria Diet and Fish Oil in Pregnancy and Lactation on Pups’ Body Weight and Fatty Acid Profiles in Rats. Eur J Nutr (2016) 55(4):1741–53. doi: 10.1007/s00394-015-0992-0
107. Masuyama H, Hiramatsu Y. Effects of a High-Fat Diet Exposure In Utero on the Metabolic Syndrome-Like Phenomenon in Mouse Offspring Through Epigenetic Changes in Adipocytokine Gene Expression. Endocrinology (2012) 153(6):2823–30. doi: 10.1210/en.2011-2161
108. Gupta A, Srinivasan M, Thamadilok S, Patel MS. Hypothalamic Alterations in Fetuses of High Fat Diet-Fed Obese Female Rats. J Endocrinol (2009) 200(3):293–300. doi: 10.1677/JOE-08-0429
109. Srinivasan M, Katewa SD, Palaniyappan A, Pandya JD, Patel MS. Maternal High-Fat Diet Consumption Results in Fetal Malprogramming Predisposing to the Onset of Metabolic Syndrome-Like Phenotype in Adulthood. Am J Physiol Endocrinol Metab (2006) 291(4):E792–9. doi: 10.1152/ajpendo.00078.2006
110. Murabayashi N, Sugiyama T, Zhang L, Kamimoto Y, Umekawa T, Ma N, et al. Maternal High-Fat Diets Cause Insulin Resistance Through Inflammatory Changes in Fetal Adipose Tissue. Eur J Obstet Gynecol Reprod Biol (2013) 169(1):39–44. doi: 10.1016/j.ejogrb.2013.02.003
111. Howell KR, Powell TL. Effects of Maternal Obesity on Placental Function and Fetal Development. Reproduction (2017) 153(3):R97–R108. doi: 10.1530/REP-16-0495
112. Buckley AJ, Keseru B, Briody J, Thompson M, Ozanne SE, Thompson CH. Altered Body Composition and Metabolism in the Male Offspring of High Fat-Fed Rats. Metabolism (2005) 54(4):500–7. doi: 10.1016/j.metabol.2004.11.003
113. Fante T, Simino LA, Reginato A, Payolla TB, Vitoreli DC, Souza M, et al. Diet-Induced Maternal Obesity Alters Insulin Signalling in Male Mice Offspring Rechallenged With a High-Fat Diet in Adulthood. PloS One (2016) 11(8):e0160184. doi: 10.1371/journal.pone.0160184
114. Brunton N DB, Dart A, Azad MB, McGavoc JM. Maternal Obesity Before Pregnancy Predicts Offspring Blood Pressure at 18 Years of Age: A Causal Mediation Analysis. medRxiv (2020). doi: 10.1101/2020.11.22.20236398
115. Gaillard R. Maternal Obesity During Pregnancy and Cardiovascular Development and Disease in the Offspring. Eur J Epidemiol (2015) 30(11):1141–52. doi: 10.1007/s10654-015-0085-7
116. Oken E, Gillman MW. Fetal Origins of Obesity. Obes Res (2003) 11(4):496–506. doi: 10.1038/oby.2003.69
117. Oken E, Rifas-Shiman SL, Field AE, Frazier AL, Gillman MW. Maternal Gestational Weight Gain and Offspring Weight in Adolescence. Obstet Gynecol (2008) 112(5):999–1006. doi: 10.1097/AOG.0b013e31818a5d50
118. Gaillard R, Durmus B, Hofman A, Mackenbach JP, Steegers EA, Jaddoe VW. Risk Factors and Outcomes of Maternal Obesity and Excessive Weight Gain During Pregnancy. Obes (Silver Spring) (2013) 21(5):1046–55. doi: 10.1002/oby.20088
119. Whitaker RC. Predicting Preschooler Obesity at Birth: The Role of Maternal Obesity in Early Pregnancy. Pediatrics (2004) 114(1):e29–36. doi: 10.1542/peds.114.1.e29
120. Tie HT, Xia YY, Zeng YS, Zhang Y, Dai CL, Guo JJ, et al. Risk of Childhood Overweight or Obesity Associated With Excessive Weight Gain During Pregnancy: A Meta-Analysis. Arch Gynecol Obstet (2014) 289(2):247–57. doi: 10.1007/s00404-013-3053-z
121. Catalano PM, Farrell K, Thomas A, Huston-Presley L, Mencin P, de Mouzon SH, et al. Perinatal Risk Factors for Childhood Obesity and Metabolic Dysregulation. Am J Clin Nutr (2009) 90(5):1303–13. doi: 10.3945/ajcn.2008.27416
122. Gaillard R, Steegers EA, Duijts L, Felix JF, Hofman A, Franco OH, et al. Childhood Cardiometabolic Outcomes of Maternal Obesity During Pregnancy: The Generation R Study. Hypertension (2014) 63(4):683–91. doi: 10.1161/HYPERTENSIONAHA.113.02671
123. Li S, Zhu Y, Yeung E, Chavarro JE, Yuan C, Field AE, et al. Offspring Risk of Obesity in Childhood, Adolescence and Adulthood in Relation to Gestational Diabetes Mellitus: A Sex-Specific Association. Int J Epidemiol (2017) 46(6):2104. doi: 10.1093/ije/dyx211
124. Joaquim AO, Coelho CP, Motta PD, Felicio LF, Bondan EF, Teodorov E, et al. Maternal Food Restriction in Rats of the F0 Generation Increases Retroperitoneal Fat, the Number and Size of Adipocytes and Induces Periventricular Astrogliosis in Female F1 and Male F2 Generations. Reprod Fertil Dev (2017) 29(7):1340–8. doi: 10.1071/RD15309
125. Lukaszewski MA, Mayeur S, Fajardy I, Delahaye F, Dutriez-Casteloot I, Montel V, et al. Maternal Prenatal Undernutrition Programs Adipose Tissue Gene Expression in Adult Male Rat Offspring Under High-Fat Diet. Am J Physiol Endocrinol Metab (2011) 301(3):E548–59. doi: 10.1152/ajpendo.00011.2011
126. Munoz-Valverde D, Rodriguez-Rodriguez P, Gutierrez-Arzapalo PY, Lopez de Pablo AL, Carmen Gonzalez M, Lopez-Gimenez R, et al. Effect of Fetal Undernutrition and Postnatal Overfeeding on Rat Adipose Tissue and Organ Growth at Early Stages of Postnatal Development. Physiol Res (2015) 64(4):547–59. doi: 10.33549/physiolres.932811
127. Zhu W, Gui W, Lin X, Yin X, Liang L, Li H. Maternal Undernutrition Modulates Hepatic Micrornas Expression in the Early Life of Offspring. Exp Cell Res (2021) 400(2):112450. doi: 10.1016/j.yexcr.2020.112450
128. de Oliveira JC, Gomes RM, Miranda RA, Barella LF, Malta A, Martins IP, et al. Protein Restriction During the Last Third of Pregnancy Malprograms the Neuroendocrine Axes to Induce Metabolic Syndrome in Adult Male Rat Offspring. Endocrinology (2016) 157(5):1799–812. doi: 10.1210/en.2015-1883
129. Xie L, Zhang K, Rasmussen D, Wang J, Wu D, Roemmich JN, et al. Effects of Prenatal Low Protein and Postnatal High Fat Diets on Visceral Adipose Tissue Macrophage Phenotypes and IL-6 Expression in Sprague Dawley Rat Offspring. PloS One (2017) 12(1):e0169581. doi: 10.1371/journal.pone.0169581
130. Thompson N, Huber K, Bedurftig M, Hansen K, Miles-Chan J, Breier BH. Metabolic Programming of Adipose Tissue Structure and Function in Male Rat Offspring by Prenatal Undernutrition. Nutr Metab (Lond) (2014) 11(1):50. doi: 10.1186/1743-7075-11-50
131. Ware S, Voigt JP, Langley-Evans SC. Body Composition and Behaviour in Adult Rats Are Influenced by Maternal Diet, Maternal Age and High-Fat Feeding. J Nutr Sci (2015) 4:e3. doi: 10.1017/jns.2014.64
132. Hanafi MY, Saleh MM, Saad MI, Abdelkhalek TM, Kamel MA. Transgenerational Effects of Obesity and Malnourishment on Diabetes Risk in F2 Generation. Mol Cell Biochem (2016) 412(1-2):269–80. doi: 10.1007/s11010-015-2633-6
133. Matveyenko AV, Singh I, Shin BC, Georgia S, Devaskar SU. Differential Effects of Prenatal and Postnatal Nutritional Environment on Ss-Cell Mass Development and Turnover in Male and Female Rats. Endocrinology (2010) 151(12):5647–56. doi: 10.1210/en.2010-0978
134. Fall CH. Fetal Malnutrition and Long-Term Outcomes. Nestle Nutr Inst Workshop Ser (2013) 74:11–25. doi: 10.1159/000348384
135. Langley-Evans SC, Sherman RC, Welham SJ, Nwagwu MO, Gardner DS, Jackson AA. Intrauterine Programming of Hypertension: The Role of the Renin-Angiotensin System. Biochem Soc Trans (1999) 27(2):88–93. doi: 10.1042/bst0270088
136. de Gusmao Correia ML, Volpato AM, Aguila MB, Mandarim-de-Lacerda CA. Developmental Origins of Health and Disease: Experimental and Human Evidence of Fetal Programming for Metabolic Syndrome. J Hum Hypertens (2012) 26(7):405–19. doi: 10.1038/jhh.2011.61
137. Roseboom TJ, van der Meulen JH, Ravelli AC, Osmond C, Barker DJ, Bleker OP. Effects of Prenatal Exposure to the Dutch Famine on Adult Disease in Later Life: An Overview. Twin Res (2001) 4(5):293–8. doi: 10.1375/twin.4.5.293
138. Veenendaal MV, Painter RC, de Rooij SR, Bossuyt PM, van der Post JA, Gluckman PD, et al. Transgenerational Effects of Prenatal Exposure to the 1944-45 Dutch Famine. BJOG (2013) 120(5):548–53. doi: 10.1111/1471-0528.12136
139. Ravelli AC, van der Meulen JH, Osmond C, Barker DJ, Bleker OP. Obesity at the Age of 50 Y in Men and Women Exposed to Famine Prenatally. Am J Clin Nutr (1999) 70(5):811–6. doi: 10.1093/ajcn/70.5.811
140. Ravelli GP, Stein ZA, Susser MW. Obesity in Young Men After Famine Exposure In Utero and Early Infancy. N Engl J Med (1976) 295(7):349–53. doi: 10.1056/NEJM197608122950701
141. Hales CN, Barker DJ. Type 2 (Non-Insulin-Dependent) Diabetes Mellitus: The Thrifty Phenotype Hypothesis. Diabetologia (1992) 35(7):595–601. doi: 10.1007/BF00400248
142. Hales CN, Barker DJ, Clark PM, Cox LJ, Fall C, Osmond C, et al. Fetal and Infant Growth and Impaired Glucose Tolerance at Age 64. BMJ (1991) 303(6809):1019–22. doi: 10.1136/bmj.303.6809.1019
143. Ong TP, Guest PC. Nutritional Programming Effects on Development of Metabolic Disorders in Later Life. Methods Mol Biol (2018) 1735:3–17. doi: 10.1007/978-1-4939-7614-0_1
144. Guo F, Jen KL. High-Fat Feeding During Pregnancy and Lactation Affects Offspring Metabolism in Rats. Physiol Behav (1995) 57(4):681–6. doi: 10.1016/0031-9384(94)00342-4
145. Rodriguez-Gonzalez GL, Reyes-Castro LA, Bautista CJ, Beltran AA, Ibanez CA, Vega CC, et al. Maternal Obesity Accelerates Rat Offspring Metabolic Ageing in a Sex-Dependent Manner. J Physiol (2019) 597(23):5549–63. doi: 10.1113/JP278232
146. Williams L, Seki Y, Vuguin PM, Charron MJ. Animal Models of In Utero Exposure to a High Fat Diet: A Review. Biochim Biophys Acta (2014) 1842(3):507–19. doi: 10.1016/j.bbadis.2013.07.006
147. Zhang Q, Xiao X, Zheng J, Li M, Yu M, Ping F, et al. A Maternal High-Fat Diet Induces DNA Methylation Changes That Contribute to Glucose Intolerance in Offspring. Front Endocrinol (Lausanne) (2019) 10:871. doi: 10.3389/fendo.2019.00871
148. Cardenas-Perez RE, Fuentes-Mera L, de la Garza AL, Torre-Villalvazo I, Reyes-Castro LA, Rodriguez-Rocha H, et al. Maternal Overnutrition by Hypercaloric Diets Programs Hypothalamic Mitochondrial Fusion and Metabolic Dysfunction in Rat Male Offspring. Nutr Metab (Lond) (2018) 15:38. doi: 10.1186/s12986-018-0279-6
149. Chen H, Simar D, Lambert K, Mercier J, Morris MJ. Maternal and Postnatal Overnutrition Differentially Impact Appetite Regulators and Fuel Metabolism. Endocrinology (2008) 149(11):5348–56. doi: 10.1210/en.2008-0582
150. Bortolin RC, Vargas AR, Gasparotto J, Chaves PR, Schnorr CE, Martinello KB, et al. A New Animal Diet Based on Human Western Diet Is a Robust Diet-Induced Obesity Model: Comparison to High-Fat and Cafeteria Diets in Term of Metabolic and Gut Microbiota Disruption. Int J Obes (Lond) (2018) 42(3):525–34. doi: 10.1038/ijo.2017.225
151. Buyukdere Y, Gulec A, Akyol A. Cafeteria Diet Increased Adiposity in Comparison to High Fat Diet in Young Male Rats. PeerJ (2019) 7:e6656. doi: 10.7717/peerj.6656
152. Navarro ME, Santos KC, Nascimento AF, Francisqueti FV, Minatel IO, Pierine DT, et al. Renal Inflammatory and Oxidative and Metabolic Changes After 6 Weeks of Cafeteria Diet in Rats. J Bras Nefrol (2016) 38(1):9–14. doi: 10.5935/0101-2800.20160003
153. Reichelt AC, Stoeckel LE, Reagan LP, Winstanley CA, Page KA. Dietary Influences on Cognition. Physiol Behav (2018) 192:118–26. doi: 10.1016/j.physbeh.2018.02.052
154. Sampey BP, Vanhoose AM, Winfield HM, Freemerman AJ, Muehlbauer MJ, Fueger PT, et al. Cafeteria Diet Is a Robust Model of Human Metabolic Syndrome With Liver and Adipose Inflammation: Comparison to High-Fat Diet. Obes (Silver Spring) (2011) 19(6):1109–17. doi: 10.1038/oby.2011.18
155. Candler T, Kuhnen P, Prentice AM, Silver M. Epigenetic Regulation of POMC; Implications for Nutritional Programming, Obesity and Metabolic Disease. Front Neuroendocrinol (2019) 54:100773. doi: 10.1016/j.yfrne.2019.100773
156. Chowen JA, Argente-Arizon P, Freire-Regatillo A, Argente J. Sex Differences in the Neuroendocrine Control of Metabolism and the Implication of Astrocytes. Front Neuroendocrinol (2018) 48:3–12. doi: 10.1016/j.yfrne.2017.05.003
157. Sanchez-Garrido MA, Ruiz-Pino F, Velasco I, Barroso A, Fernandois D, Heras V, et al. Intergenerational Influence of Paternal Obesity on Metabolic and Reproductive Health Parameters of the Offspring: Male-Preferential Impact and Involvement of Kiss1-Mediated Pathways. Endocrinology (2018) 159(2):1005–18. doi: 10.1210/en.2017-00705
158. Gomes D, von Kries R, Delius M, Mansmann U, Nast M, Stubert M, et al. Late-Pregnancy Dysglycemia in Obese Pregnancies After Negative Testing for Gestational Diabetes and Risk of Future Childhood Overweight: An Interim Analysis From a Longitudinal Mother-Child Cohort Study. PloS Med (2018) 15(10):e1002681. doi: 10.1371/journal.pmed.1002681
159. Eriksson JG, Sandboge S, Salonen M, Kajantie E, Osmond C. Maternal Weight in Pregnancy and Offspring Body Composition in Late Adulthood: Findings From the Helsinki Birth Cohort Study (HBCS). Ann Med (2015) 47(2):94–9. doi: 10.3109/07853890.2015.1004360
160. Bhandari R, Xiao J, Shankar A. Urinary Bisphenol a and Obesity in U.S. children. Am J Epidemiol (2013) 177(11):1263–70. doi: 10.1093/aje/kws391
161. Lee DH, Porta M, Jacobs DR Jr, Vandenberg LN. Chlorinated Persistent Organic Pollutants, Obesity, and Type 2 Diabetes. Endocr Rev (2014) 35(4):557–601. doi: 10.1210/er.2013-1084
162. Lobstein T, Brownell KD. Endocrine-Disrupting Chemicals and Obesity Risk: A Review of Recommendations for Obesity Prevention Policies. Obes Rev (2021) 22(11):e13332. doi: 10.1111/obr.13332
163. Wu W, Li M, Liu A, Wu C, Li D, Deng Q, et al. Bisphenol a and the Risk of Obesity a Systematic Review With Meta-Analysis of the Epidemiological Evidence. Dose-Response (2020) 18(2):155932582091949. doi: 10.1177/1559325820916949
164. Ashley-Martin J, Dodds L, Arbuckle TE, Ettinger AS, Shapiro GD, Fisher M, et al. A Birth Cohort Study to Investigate the Association Between Prenatal Phthalate and Bisphenol a Exposures and Fetal Markers of Metabolic Dysfunction. Environ Health (2014) 13:84. doi: 10.1186/1476-069X-13-84
165. Heindel JJ. The Developmental Basis of Disease: Update on Environmental Exposures and Animal Models. Basic Clin Pharmacol Toxicol (2019) 125(Suppl 3):5–13. doi: 10.1111/bcpt.13118
166. Rodgers A, Sferruzzi-Perri AN. Developmental Programming of Offspring Adipose Tissue Biology and Obesity Risk. Int J Obes (Lond) (2021) 45(6):1170–92. doi: 10.1038/s41366-021-00790-w
167. Rolfo A, Nuzzo AM, De Amicis R, Moretti L, Bertoli S, Leone A. Fetal-Maternal Exposure to Endocrine Disruptors: Correlation With Diet Intake and Pregnancy Outcomes. Nutrients (2020) 12(6):1744. doi: 10.3390/nu12061744
168. Anway MD, Cupp AS, Uzumcu M, Skinner MK. Epigenetic Transgenerational Actions of Endocrine Disruptors and Male Fertility. Science (2005) 308(5727):1466–9. doi: 10.1126/science.1108190
169. Jirtle RL, Skinner MK. Environmental Epigenomics and Disease Susceptibility. Nat Rev Genet (2007) 8(4):253–62. doi: 10.1038/nrg2045
170. Malik VS, Popkin BM, Bray GA, Despres JP, Willett WC, Hu FB. Sugar-Sweetened Beverages and Risk of Metabolic Syndrome and Type 2 Diabetes: A Meta-Analysis. Diabetes Care (2010) 33(11):2477–83. doi: 10.2337/dc10-1079
171. Talbot CPJ, Dolinsky VW. Sex Differences in the Developmental Origins of Cardiometabolic Disease Following Exposure to Maternal Obesity and Gestational Diabetes (1). Appl Physiol Nutr Metab (2019) 44(7):687–95. doi: 10.1139/apnm-2018-0667
172. Arnold AP, Lusis AJ. Understanding the Sexome: Measuring and Reporting Sex Differences in Gene Systems. Endocrinology (2012) 153(6):2551–5. doi: 10.1210/en.2011-2134
173. Arnold AP, van Nas A, Lusis AJ. Systems Biology Asks New Questions About Sex Differences. Trends Endocrinol Metab (2009) 20(10):471–6. doi: 10.1016/j.tem.2009.06.007
174. Krishnaveni GV, Hill JC, Leary SD, Veena SR, Saperia J, Saroja A, et al. Anthropometry, Glucose Tolerance, and Insulin Concentrations in Indian Children: Relationships to Maternal Glucose and Insulin Concentrations During Pregnancy. Diabetes Care (2005) 28(12):2919–25. doi: 10.2337/diacare.28.12.2919
175. Puttabyatappa M, Sargis RM, Padmanabhan V. Developmental Programming of Insulin Resistance: Are Androgens the Culprits? J Endocrinol (2020) 245(3):R23–48. doi: 10.1530/JOE-20-0044
176. Sir-Petermann T, Codner E, Perez V, Echiburu B, Maliqueo M, Ladron de Guevara A, et al. Metabolic and Reproductive Features Before and During Puberty in Daughters of Women With Polycystic Ovary Syndrome. J Clin Endocrinol Metab (2009) 94(6):1923–30. doi: 10.1210/jc.2008-2836
177. Tamhane S, Rodriguez-Gutierrez R, Iqbal AM, Prokop LJ, Bancos I, Speiser PW, et al. Cardiovascular and Metabolic Outcomes in Congenital Adrenal Hyperplasia: A Systematic Review and Meta-Analysis. J Clin Endocrinol Metab (2018) 103(11):4097–103. doi: 10.1210/jc.2018-01862
178. Sosa-Larios TC, AM-G A, Reyes-Castro LA, Morimoto S, Jaramillo-Flores ME. Alterations in Lipid Metabolism Due to a Protein-Restricted Diet in Rats During Gestation and/or Lactation. Food Funct (2017). doi: 10.1039/C7FO01513E
179. Lecoutre S, Breton C. Maternal Nutritional Manipulations Program Adipose Tissue Dysfunction in Offspring. Front Physiol (2015) 6:158. doi: 10.3389/fphys.2015.00158
180. Morris TJ, Vickers M, Gluckman P, Gilmour S, Affara N. Transcriptional Profiling of Rats Subjected to Gestational Undernourishment: Implications for the Developmental Variations in Metabolic Traits. PloS One (2009) 4(9):e7271. doi: 10.1371/journal.pone.0007271
181. Guan H, Arany E, van Beek JP, Chamson-Reig A, Thyssen S, Hill DJ, et al. Adipose Tissue Gene Expression Profiling Reveals Distinct Molecular Pathways That Define Visceral Adiposity in Offspring of Maternal Protein-Restricted Rats. Am J Physiol Endocrinol Metab (2005) 288(4):E663–73. doi: 10.1152/ajpendo.00461.2004
182. Palou M, Priego T, Romero M, Szostaczuk N, Konieczna J, Cabrer C, et al. Moderate Calorie Restriction During Gestation Programs Offspring for Lower BAT Thermogenic Capacity Driven by Thyroid and Sympathetic Signaling. Int J Obes (Lond) (2015) 39(2):339–45. doi: 10.1038/ijo.2014.56
183. Lussana F, Painter RC, Ocke MC, Buller HR, Bossuyt PM, Roseboom TJ. Prenatal Exposure to the Dutch Famine Is Associated With a Preference for Fatty Foods and a More Atherogenic Lipid Profile. Am J Clin Nutr (2008) 88(6):1648–52. doi: 10.3945/ajcn.2008.26140
184. Hult M, Tornhammar P, Ueda P, Chima C, Bonamy AK, Ozumba B, et al. Hypertension, Diabetes and Overweight: Looming Legacies of the Biafran Famine. PloS One (2010) 5(10):e13582. doi: 10.1371/journal.pone.0013582
185. Li Y, He Y, Qi L, Jaddoe VW, Feskens EJ, Yang X, et al. Exposure to the Chinese Famine in Early Life and the Risk of Hyperglycemia and Type 2 Diabetes in Adulthood. Diabetes (2010) 59(10):2400–6. doi: 10.2337/db10-0385
186. Lakshmy R. Metabolic Syndrome: Role of Maternal Undernutrition and Fetal Programming. Rev Endocr Metab Disord (2013) 14(3):229–40. doi: 10.1007/s11154-013-9266-4
187. Roseboom T, de Rooij S, Painter R. The Dutch Famine and Its Long-Term Consequences for Adult Health. Early Hum Dev (2006) 82(8):485–91. doi: 10.1016/j.earlhumdev.2006.07.001
188. Painter RC, Roseboom TJ, van Montfrans GA, Bossuyt PM, Krediet RT, Osmond C, et al. Microalbuminuria in Adults After Prenatal Exposure to the Dutch Famine. J Am Soc Nephrol (2005) 16(1):189–94. doi: 10.1681/ASN.2004060474
189. Cianfarani S, Germani D, Branca F. Low Birthweight and Adult Insulin Resistance: The “Catch-Up Growth” Hypothesis. Arch Dis Child Fetal Neonatal Ed (1999) 81(1):F71–3. doi: 10.1136/fn.81.1.F71
190. Jaquet D, Gaboriau A, Czernichow P, Levy-Marchal C. Insulin Resistance Early in Adulthood in Subjects Born With Intrauterine Growth Retardation. J Clin Endocrinol Metab (2000) 85(4):1401–6. doi: 10.1210/jc.85.4.1401
191. Marciniak A, Patro-Malysza J, Kimber-Trojnar Z, Marciniak B, Oleszczuk J, Leszczynska-Gorzelak B. Fetal Programming of the Metabolic Syndrome. Taiwan J Obstet Gynecol (2017) 56(2):133–8. doi: 10.1016/j.tjog.2017.01.001
192. Ravelli AC, van der Meulen JH, Michels RP, Osmond C, Barker DJ, Hales CN, et al. Glucose Tolerance in Adults After Prenatal Exposure to Famine. Lancet (1998) 351(9097):173–7. doi: 10.1016/S0140-6736(97)07244-9
193. Roseboom TJ, van der Meulen JH, Ravelli AC, van Montfrans GA, Osmond C, Barker DJ, et al. Blood Pressure in Adults After Prenatal Exposure to Famine. J Hypertens (1999) 17(3):325–30. doi: 10.1097/00004872-199917030-00004
194. Finer S, Iqbal MS, Lowe R, Ogunkolade BW, Pervin S, Mathews C, et al. Is Famine Exposure During Developmental Life in Rural Bangladesh Associated With a Metabolic and Epigenetic Signature in Young Adulthood? A Historical Cohort Study. BMJ Open (2016) 6(11):e011768. doi: 10.1136/bmjopen-2016-011768
195. Armitage JA, Lakasing L, Taylor PD, Balachandran AA, Jensen RI, Dekou V, et al. Developmental Programming of Aortic and Renal Structure in Offspring of Rats Fed Fat-Rich Diets in Pregnancy. J Physiol (2005) 565(Pt 1):171–84. doi: 10.1113/jphysiol.2005.084947
196. Gopalakrishnan GS, Gardner DS, Dandrea J, Langley-Evans SC, Pearce S, Kurlak LO, et al. Influence of Maternal Pre-Pregnancy Body Composition and Diet During Early-Mid Pregnancy on Cardiovascular Function and Nephron Number in Juvenile Sheep. Br J Nutr (2005) 94(6):938–47. doi: 10.1079/BJN20051559
197. Morrison JL, Botting KJ, Dyer JL, Williams SJ, Thornburg KL, McMillen IC. Restriction of Placental Function Alters Heart Development in the Sheep Fetus. Am J Physiol Regul Integr Comp Physiol (2007) 293(1):R306–13. doi: 10.1152/ajpregu.00798.2006
198. Wang J, Ma H, Tong C, Zhang H, Lawlis GB, Li Y, et al. Overnutrition and Maternal Obesity in Sheep Pregnancy Alter the JNK-IRS-1 Signaling Cascades and Cardiac Function in the Fetal Heart. FASEB J (2010) 24(6):2066–76. doi: 10.1096/fj.09-142315
199. Fan L, Lindsley SR, Comstock SM, Takahashi DL, Evans AE, He GW, et al. Maternal High-Fat Diet Impacts Endothelial Function in Nonhuman Primate Offspring. Int J Obes (Lond) (2013) 37(2):254–62. doi: 10.1038/ijo.2012.42
200. Cerf ME. High Fat Programming and Cardiovascular Disease. Medicina (Kaunas) (2018) 54(5):86. doi: 10.3390/medicina54050086
201. Moreira AS, Teixeira Teixeira M, da Silveira Osso F, Pereira RO, de Oliveira Silva-Junior G, Garcia de Souza EP, et al. Left Ventricular Hypertrophy Induced by Overnutrition Early in Life. Nutr Metab Cardiovasc Dis (2009) 19(11):805–10. doi: 10.1016/j.numecd.2009.01.008
202. Habbout A, Guenancia C, Lorin J, Rigal E, Fassot C, Rochette L, et al. Postnatal Overfeeding Causes Early Shifts in Gene Expression in the Heart and Long-Term Alterations in Cardiometabolic and Oxidative Parameters. PloS One (2013) 8(2):e56981. doi: 10.1371/journal.pone.0056981
203. Borengasser SJ, Zhong Y, Kang P, Lindsey F, Ronis MJ, Badger TM, et al. Maternal Obesity Enhances White Adipose Tissue Differentiation and Alters Genome-Scale DNA Methylation in Male Rat Offspring. Endocrinology (2013) 154(11):4113–25. doi: 10.1210/en.2012-2255
204. Liang X, Yang Q, Fu X, Rogers CJ, Wang B, Pan H, et al. Maternal Obesity Epigenetically Alters Visceral Fat Progenitor Cell Properties in Male Offspring Mice. J Physiol (2016) 594(15):4453–66. doi: 10.1113/JP272123
205. Van De Maele K, Devlieger R, Gies I. In Utero Programming and Early Detection of Cardiovascular Disease in the Offspring of Mothers With Obesity. Atherosclerosis (2018) 275:182–95. doi: 10.1016/j.atherosclerosis.2018.06.016
206. Agarwal P, Morriseau TS, Kereliuk SM, Doucette CA, Wicklow BA, Dolinsky VW. Maternal Obesity, Diabetes During Pregnancy and Epigenetic Mechanisms That Influence the Developmental Origins of Cardiometabolic Disease in the Offspring. Crit Rev Clin Lab Sci (2018) 55(2):71–101. doi: 10.1080/10408363.2017.1422109
207. Neri C EA. Effects of Maternal Obesity on Fetal Programming: Molecular Approaches. Cold Spring Harb Perspect Med (2016) 6(2):a026591. doi: 10.1101/cshperspect.a026591
208. Elahi MM, Cagampang FR, Mukhtar D, Anthony FW, Ohri SK, Hanson MA. Long-Term Maternal High-Fat Feeding From Weaning Through Pregnancy and Lactation Predisposes Offspring to Hypertension, Raised Plasma Lipids and Fatty Liver in Mice. Br J Nutr (2009) 102(4):514–9. doi: 10.1017/S000711450820749X
209. Samuelsson AM, Morris A, Igosheva N, Kirk SL, Pombo JM, Coen CW, et al. Evidence for Sympathetic Origins of Hypertension in Juvenile Offspring of Obese Rats. Hypertension (2010) 55(1):76–82. doi: 10.1161/HYPERTENSIONAHA.109.139402
210. Khan IY, Dekou V, Douglas G, Jensen R, Hanson MA, Poston L, et al. A High-Fat Diet During Rat Pregnancy or Suckling Induces Cardiovascular Dysfunction in Adult Offspring. Am J Physiol Regul Integr Comp Physiol (2005) 288(1):R127–33. doi: 10.1152/ajpregu.00354.2004
211. Drake AJ, Reynolds RM. Impact of Maternal Obesity on Offspring Obesity and Cardiometabolic Disease Risk. Reproduction (2010) 140(3):387–98. doi: 10.1530/REP-10-0077
212. Godfrey KM, Reynolds RM, Prescott SL, Nyirenda M, Jaddoe VW, Eriksson JG, et al. Influence of Maternal Obesity on the Long-Term Health of Offspring. Lancet Diabetes Endocrinol (2017) 5(1):53–64. doi: 10.1016/S2213-8587(16)30107-3
213. Reynolds RM, Allan KM, Raja EA, Bhattacharya S, McNeill G, Hannaford PC, et al. Maternal Obesity During Pregnancy and Premature Mortality From Cardiovascular Event in Adult Offspring: Follow-Up of 1 323 275 Person Years. BMJ (2013) 347:f4539. doi: 10.1136/bmj.f4539
214. McMillen IC, Robinson JS. Developmental Origins of the Metabolic Syndrome: Prediction, Plasticity, and Programming. Physiol Rev (2005) 85(2):571–633. doi: 10.1152/physrev.00053.2003
215. Mamun AA, O’Callaghan M, Callaway L, Williams G, Najman J, Lawlor DA. Associations of Gestational Weight Gain With Offspring Body Mass Index and Blood Pressure at 21 Years of Age: Evidence From a Birth Cohort Study. Circulation (2009) 119(13):1720–7. doi: 10.1161/CIRCULATIONAHA.108.813436
216. Oken E, Taveras EM, Kleinman KP, Rich-Edwards JW, Gillman MW. Gestational Weight Gain and Child Adiposity at Age 3 Years. Am J Obstet Gynecol (2007) 196(4):322 e1–8. doi: 10.1016/j.ajog.2006.11.027
217. Tam CHT, Ma RCW, Yuen LY, Ozaki R, Li AM, Hou Y, et al. The Impact of Maternal Gestational Weight Gain on Cardiometabolic Risk Factors in Children. Diabetologia (2018) 61(12):2539–48. doi: 10.1007/s00125-018-4724-x
218. West NA, Crume TL, Maligie MA, Dabelea D. Cardiovascular Risk Factors in Children Exposed to Maternal Diabetes In Utero. Diabetologia (2011) 54(3):504–7. doi: 10.1007/s00125-010-2008-1
219. Salter A TE, Langley-Evans S. Influence of Maternal Nutrition on the Metabolic Syndrome and Cardiovascular Risk in the Offspring. Clin Lipidol (2009) 4(2):145–58. doi: 10.2217/clp.09.4
220. Chen P, Piaggi P, Traurig M, Bogardus C, Knowler WC, Baier LJ, et al. Differential Methylation of Genes in Individuals Exposed to Maternal Diabetes In Utero. Diabetologia (2017) 60(4):645–55. doi: 10.1007/s00125-016-4203-1
221. Ruchat SM, Houde AA, Voisin G, St-Pierre J, Perron P, Baillargeon JP, et al. Gestational Diabetes Mellitus Epigenetically Affects Genes Predominantly Involved in Metabolic Diseases. Epigenetics (2013) 8(9):935–43. doi: 10.4161/epi.25578
222. Kawamura M, Itoh H, Yura S, Mogami H, Fujii T, Makino H, et al. Isocaloric High-Protein Diet Ameliorates Systolic Blood Pressure Increase and Cardiac Remodeling Caused by Maternal Caloric Restriction in Adult Mouse Offspring. Endocr J (2009) 56(5):679–89. doi: 10.1507/endocrj.K08E-286
223. Mossa F, Carter F, Walsh SW, Kenny DA, Smith GW, Ireland JL, et al. Maternal Undernutrition in Cows Impairs Ovarian and Cardiovascular Systems in Their Offspring. Biol Reprod (2013) 88(4):92. doi: 10.1095/biolreprod.112.107235
224. Torrens C, Brawley L, Anthony FW, Dance CS, Dunn R, Jackson AA, et al. Folate Supplementation During Pregnancy Improves Offspring Cardiovascular Dysfunction Induced by Protein Restriction. Hypertension (2006) 47(5):982–7. doi: 10.1161/01.HYP.0000215580.43711.d1
225. Rexhaj E, Bloch J, Jayet PY, Rimoldi SF, Dessen P, Mathieu C, et al. Fetal Programming of Pulmonary Vascular Dysfunction in Mice: Role of Epigenetic Mechanisms. Am J Physiol Heart Circ Physiol (2011) 301(1):H247–52. doi: 10.1152/ajpheart.01309.2010
226. Vickers MH, Breier BH, McCarthy D, Gluckman PD. Sedentary Behavior During Postnatal Life Is Determined by the Prenatal Environment and Exacerbated by Postnatal Hypercaloric Nutrition. Am J Physiol Regul Integr Comp Physiol (2003) 285(1):R271–3. doi: 10.1152/ajpregu.00051.2003
227. Barros MA, De Brito Alves JL, Nogueira VO, Wanderley AG, Costa-Silva JH. Maternal Low-Protein Diet Induces Changes in the Cardiovascular Autonomic Modulation in Male Rat Offspring. Nutr Metab Cardiovasc Dis (2015) 25(1):123–30. doi: 10.1016/j.numecd.2014.07.011
228. Manning J, Vehaskari VM. Low Birth Weight-Associated Adult Hypertension in the Rat. Pediatr Nephrol (2001) 16(5):417–22. doi: 10.1007/s004670000560
229. Goyal R, Longo LD. Maternal Protein Deprivation: Sexually Dimorphic Programming of Hypertension in the Mouse. Hypertens Res (2013) 36(1):29–35. doi: 10.1038/hr.2012.129
230. Gilbert JS, Lang AL, Grant AR, Nijland MJ. Maternal Nutrient Restriction in Sheep: Hypertension and Decreased Nephron Number in Offspring at 9 Months of Age. J Physiol (2005) 565(Pt 1):137–47. doi: 10.1113/jphysiol.2005.084202
231. Tappia PS, Gabriel CA. Role of Nutrition in the Development of the Fetal Cardiovascular System. Expert Rev Cardiovasc Ther (2006) 4(2):211–25. doi: 10.1586/14779072.4.2.211
232. Gilbert JS, Lang AL, Nijland MJ. Maternal Nutrient Restriction and the Fetal Left Ventricle: Decreased Angiotensin Receptor Expression. Reprod Biol Endocrinol (2005) 3:27. doi: 10.1186/1477-7827-3-27
233. Cheema KK, Dent MR, Saini HK, Aroutiounova N, Tappia PS. Prenatal Exposure to Maternal Undernutrition Induces Adult Cardiac Dysfunction. Br J Nutr (2005) 93(4):471–7. doi: 10.1079/BJN20041392
234. Corstius HB, Zimanyi MA, Maka N, Herath T, Thomas W, van der Laarse A, et al. Effect of Intrauterine Growth Restriction on the Number of Cardiomyocytes in Rat Hearts. Pediatr Res (2005) 57(6):796–800. doi: 10.1203/01.PDR.0000157726.65492.CD
235. Jelinek DF, Andersson S, Slaughter CA, Russell DW. Cloning and Regulation of Cholesterol 7 Alpha-Hydroxylase, the Rate-Limiting Enzyme in Bile Acid Biosynthesis. J Biol Chem (1990) 265(14):8190–7. doi: 10.1016/S0021-9258(19)39056-8
236. Sun C, Denisenko O, Sheth B, Cox A, Lucas ES, Smyth NR, et al. Epigenetic Regulation of Histone Modifications and Gata6 Gene Expression Induced by Maternal Diet in Mouse Embryoid Bodies in a Model of Developmental Programming. BMC Dev Biol (2015) 15:3. doi: 10.1186/s12861-015-0053-1
237. Wang J, Wu Z, Li D, Li N, Dindot SV, Satterfield MC, et al. Nutrition, Epigenetics, and Metabolic Syndrome. Antioxid Redox Signal (2012) 17(2):282–301. doi: 10.1089/ars.2011.4381
238. Khorram O, Chuang TD, Pearce WJ. Long-Term Effects of Maternal Undernutrition on Offspring Carotid Artery Remodeling: Role of Mir-29c. J Dev Orig Health Dis (2015) 6(4):342–9. doi: 10.1017/S2040174415001208
239. Bogdarina I, Welham S, King PJ, Burns SP, Clark AJ. Epigenetic Modification of the Renin-Angiotensin System in the Fetal Programming of Hypertension. Circ Res (2007) 100(4):520–6. doi: 10.1161/01.RES.0000258855.60637.58
240. Alexander BT, Dasinger JH, Intapad S. Fetal Programming and Cardiovascular Pathology. Compr Physiol (2015) 5(2):997–1025. doi: 10.1002/cphy.c140036
241. Alabduljabbar S, Zaidan SA, Lakshmanan AP, Terranegra A. Personalized Nutrition Approach in Pregnancy and Early Life to Tackle Childhood and Adult Non-Communicable Diseases. Life (Basel) (2021) 11(6):476. doi: 10.3390/life11060467
242. Li Y, Jaddoe VW, Qi L, He Y, Wang D, Lai J, et al. Exposure to the Chinese Famine in Early Life and the Risk of Metabolic Syndrome in Adulthood. Diabetes Care (2011) 34(4):1014–8. doi: 10.2337/dc10-2039
243. Roseboom TJ, van der Meulen JH, Osmond C, Barker DJ, Ravelli AC, Schroeder-Tanka JM, et al. Coronary Heart Disease After Prenatal Exposure to the Dutch Famine, 1944-45. Heart (2000) 84(6):595–8. doi: 10.1136/heart.84.6.595
244. Pearce WJ, Khorram O. Maturation and Differentiation of the Fetal Vasculature. Clin Obstet Gynecol (2013) 56(3):537–48. doi: 10.1097/GRF.0b013e31829e5bc9
245. Bilbo SD, Tsang V. Enduring Consequences of Maternal Obesity for Brain Inflammation and Behavior of Offspring. FASEB J (2010) 24(6):2104–15. doi: 10.1096/fj.09-144014
246. Contu L, Hawkes CA. A Review of the Impact of Maternal Obesity on the Cognitive Function and Mental Health of the Offspring. Int J Mol Sci (2017) 18(5):1093. doi: 10.3390/ijms18051093
247. Nizari S, Carare RO, Hawkes CA. Increased Abeta Pathology in Aged Tg2576 Mice Born to Mothers Fed a High Fat Diet. Sci Rep (2016) 6:21981. doi: 10.1038/srep21981
248. Mucellini AB, Laureano DP, Silveira PP, Sanvitto GL. Maternal and Post-Natal Obesity Alters Long-Term Memory and Hippocampal Molecular Signaling of Male Rat. Brain Res (2019) 1708:138–45. doi: 10.1016/j.brainres.2018.12.021
249. Page KC, Jones EK, Anday EK. Maternal and Postweaning High-Fat Diets Disturb Hippocampal Gene Expression, Learning, and Memory Function. Am J Physiol Regul Integr Comp Physiol (2014) 306(8):R527–37. doi: 10.1152/ajpregu.00319.2013
250. Edlow AG. Maternal Obesity and Neurodevelopmental and Psychiatric Disorders in Offspring. Prenat Diagn (2017) 37(1):95–110. doi: 10.1002/pd.4932
251. Tozuka Y, Wada E, Wada K. Diet-Induced Obesity in Female Mice Leads to Peroxidized Lipid Accumulations and Impairment of Hippocampal Neurogenesis During the Early Life of Their Offspring. FASEB J (2009) 23(6):1920–34. doi: 10.1096/fj.08-124784
252. Moody L, Chen H, Pan YX. Early-Life Nutritional Programming of Cognition-the Fundamental Role of Epigenetic Mechanisms in Mediating the Relation Between Early-Life Environment and Learning and Memory Process. Adv Nutr (2017) 8(2):337–50. doi: 10.3945/an.116.014209
253. Ishikawa J, Ishikawa A, Nakamura S. Interferon-Alpha Reduces the Density of Monoaminergic Axons in the Rat Brain. Neuroreport (2007) 18(2):137–40. doi: 10.1097/WNR.0b013e328010231a
254. Naef L, Moquin L, Dal Bo G, Giros B, Gratton A, Walker CD. Maternal High-Fat Intake Alters Presynaptic Regulation of Dopamine in the Nucleus Accumbens and Increases Motivation for Fat Rewards in the Offspring. Neuroscience (2011) 176:225–36. doi: 10.1016/j.neuroscience.2010.12.037
255. Vucetic Z, Kimmel J, Totoki K, Hollenbeck E, Reyes TM. Maternal High-Fat Diet Alters Methylation and Gene Expression of Dopamine and Opioid-Related Genes. Endocrinology (2010) 151(10):4756–64. doi: 10.1210/en.2010-0505
256. Peleg-Raibstein D, Luca E, Wolfrum C. Maternal High-Fat Diet in Mice Programs Emotional Behavior in Adulthood. Behav Brain Res (2012) 233(2):398–404. doi: 10.1016/j.bbr.2012.05.027
257. Sullivan EL, Grayson B, Takahashi D, Robertson N, Maier A, Bethea CL, et al. Chronic Consumption of a High-Fat Diet During Pregnancy Causes Perturbations in the Serotonergic System and Increased Anxiety-Like Behavior in Nonhuman Primate Offspring. J Neurosci (2010) 30(10):3826–30. doi: 10.1523/JNEUROSCI.5560-09.2010
258. Cordner ZA, Tamashiro KL. Effects of High-Fat Diet Exposure on Learning & Memory. Physiol Behav (2015) 152(Pt B):363–71. doi: 10.1016/j.physbeh.2015.06.008
259. Mehta SH KJ, Sokol RJ, Keating DP, Paneth N. The Association Between Maternal Obesity and Neurodevelopmental Outcomes of Offspring. J Pediatr (2014) 165(5):891–6. doi: 10.1016/j.jpeds.2014.07.003
260. Basatemur E, Gardiner J, Williams C, Melhuish E, Barnes J, Sutcliffe A. Maternal Prepregnancy BMI and Child Cognition: A Longitudinal Cohort Study. Pediatrics (2013) 131(1):56–63. doi: 10.1542/peds.2012-0788
261. Tanda R, Salsberry PJ, Reagan PB, Fang MZ. The Impact of Prepregnancy Obesity on Children’s Cognitive Test Scores. Matern Child Health J (2013) 17(2):222–9. doi: 10.1007/s10995-012-0964-4
262. Mina TH, Lahti M, Drake AJ, Denison FC, Raikkonen K, Norman JE, et al. Prenatal Exposure to Maternal Very Severe Obesity Is Associated With Impaired Neurodevelopment and Executive Functioning in Children. Pediatr Res (2017) 82(1):47–54. doi: 10.1038/pr.2017.43
263. Mina TH, Lahti M, Drake AJ, Forbes S, Denison FC, Raikkonen K, et al. Maternal Lipids in Pregnancy Are Associated With Increased Offspring Cortisol Reactivity in Childhood. Psychoneuroendocrinology (2017) 83:79–83. doi: 10.1016/j.psyneuen.2017.04.018
264. Rodriguez A. Maternal Pre-Pregnancy Obesity and Risk for Inattention and Negative Emotionality in Children. J Child Psychol Psychiatry (2010) 51(2):134–43. doi: 10.1111/j.1469-7610.2009.02133.x
265. Sullivan EL, Riper KM, Lockard R, Valleau JC. Maternal High-Fat Diet Programming of the Neuroendocrine System and Behavior. Horm Behav (2015) 76:153–61. doi: 10.1016/j.yhbeh.2015.04.008
266. Rodriguez A, Miettunen J, Henriksen TB, Olsen J, Obel C, Taanila A, et al. Maternal Adiposity Prior to Pregnancy Is Associated With ADHD Symptoms in Offspring: Evidence From Three Prospective Pregnancy Cohorts. Int J Obes (Lond) (2008) 32(3):550–7. doi: 10.1038/sj.ijo.0803741
267. Li M, Fallin MD, Riley A, Landa R, Walker SO, Silverstein M, et al. The Association of Maternal Obesity and Diabetes With Autism and Other Developmental Disabilities. Pediatrics (2016) 137(2):e20152206. doi: 10.1542/peds.2015-2206
268. Lamerz A, Kuepper-Nybelen J, Bruning N, Wehle C, Trost-Brinkhues G, Brenner H, et al. Prevalence of Obesity, Binge Eating, and Night Eating in a Cross-Sectional Field Survey of 6-Year-Old Children and Their Parents in a German Urban Population. J Child Psychol Psychiatry (2005) 46(4):385–93. doi: 10.1111/j.1469-7610.2004.00363.x
269. Stice E, Agras WS, Hammer LD. Risk Factors for the Emergence of Childhood Eating Disturbances: A Five-Year Prospective Study. Int J Eat Disord (1999) 25(4):375–87. doi: 10.1002/(SICI)1098-108X(199905)25:4<375::AID-EAT2>3.0.CO;2-K
270. Almeida SS, Tonkiss J, Galler JR. Prenatal Protein Malnutrition Affects Exploratory Behavior of Female Rats in the Elevated Plus-Maze Test. Physiol Behav (1996) 60(2):675–80. doi: 10.1016/S0031-9384(96)80047-3
271. Barra R, Morgan C, Saez-Briones P, Reyes-Parada M, Burgos H, Morales B, et al. Facts and Hypotheses About the Programming of Neuroplastic Deficits by Prenatal Malnutrition. Nutr Rev (2019) 77(2):65–80. doi: 10.1093/nutrit/nuy047
272. Belluscio LM, Berardino BG, Ferroni NM, Ceruti JM, Canepa ET. Early Protein Malnutrition Negatively Impacts Physical Growth and Neurological Reflexes and Evokes Anxiety and Depressive-Like Behaviors. Physiol Behav (2014) 129:237–54. doi: 10.1016/j.physbeh.2014.02.051
273. Francolin-Silva AL, da Silva Hernandes A, Fukuda MT, Valadares CT, Almeida SS. Anxiolytic-Like Effects of Short-Term Postnatal Protein Malnutrition in the Elevated Plus-Maze Test. Behav Brain Res (2006) 173(2):310–4. doi: 10.1016/j.bbr.2006.06.042
274. Reyes-Castro LA, Rodriguez JS, Charco R, Bautista CJ, Larrea F, Nathanielsz PW, et al. Maternal Protein Restriction in the Rat During Pregnancy and/or Lactation Alters Cognitive and Anxiety Behaviors of Female Offspring. Int J Dev Neurosci (2012) 30(1):39–45. doi: 10.1016/j.ijdevneu.2011.10.002
275. Wang L, Xu RJ. The Effects of Perinatal Protein Malnutrition on Spatial Learning and Memory Behaviour and Brain-Derived Neurotrophic Factor Concentration in the Brain Tissue in Young Rats. Asia Pac J Clin Nutr (2007) 16(Suppl 1):467–72.
276. Batista TH, Giusti-Paiva A, Vilela FC. Maternal Protein Malnutrition Induces Autism-Like Symptoms in Rat Offspring. Nutr Neurosci (2019) 22(9):655–63. doi: 10.1080/1028415X.2018.1427660
277. Matos RJ, Orozco-Solis R, Lopes de Souza S, Manhaes-de-Castro R, Bolanos-Jimenez F. Nutrient Restriction During Early Life Reduces Cell Proliferation in the Hippocampus at Adulthood But Does Not Impair the Neuronal Differentiation Process of the New Generated Cells. Neuroscience (2011) 196:16–24. doi: 10.1016/j.neuroscience.2011.08.071
278. Zhang Y, Wei J, Yang Z. Perinatal Undernutrition Attenuates Field Excitatory Postsynaptic Potentials and Influences Dendritic Spine Density and Morphology in Hippocampus of Male Rat Offspring. Neuroscience (2013) 244:31–41. doi: 10.1016/j.neuroscience.2013.03.061
279. Chertoff M. Protein Malnutrition and Brain Development. Brain Disord Ther (2015) 4(3):171. doi: 10.4172/2168-975X.1000171
280. Feoli AM, Leite MC, Tramontina AC, Tramontina F, Posser T, Rodrigues L, et al. Developmental Changes in Content of Glial Marker Proteins in Rats Exposed to Protein Malnutrition. Brain Res (2008) 1187:33–41. doi: 10.1016/j.brainres.2007.10.035
281. Zhang Y, Li N, Yang Z. Perinatal Food Restriction Impaired Spatial Learning and Memory Behavior and Decreased the Density of Nitric Oxide Synthase Neurons in the Hippocampus of Adult Male Rat Offspring. Toxicol Lett (2010) 193(2):167–72. doi: 10.1016/j.toxlet.2010.01.002
282. Chang YM, Galler JR, Luebke JI. Prenatal Protein Malnutrition Results in Increased Frequency of Miniature Inhibitory Postsynaptic Currents in Rat CA3 Interneurons. Nutr Neurosci (2003) 6(4):263–7. doi: 10.1080/1028415031000151549
283. Soto-Moyano R, Fernandez V, Sanhueza M, Belmar J, Kusch C, Perez H, et al. Effects of Mild Protein Prenatal Malnutrition and Subsequent Postnatal Nutritional Rehabilitation on Noradrenaline Release and Neuronal Density in the Rat Occipital Cortex. Brain Res Dev Brain Res (1999) 116(1):51–8. doi: 10.1016/S0165-3806(99)00074-7
284. Chen J, Chen P, Bo T, Luo K. Cognitive and Behavioral Outcomes of Intrauterine Growth Restriction School-Age Children. Pediatrics (2016) 137(4). doi: 10.1542/peds.2015-3868
285. Murray E, Fernandes M, Fazel M, Kennedy SH, Villar J, Stein A. Differential Effect of Intrauterine Growth Restriction on Childhood Neurodevelopment: A Systematic Review. BJOG (2015) 122(8):1062–72. doi: 10.1111/1471-0528.13435
286. Gibney ER, Nolan CM. Epigenetics and Gene Expression. Heredity (Edinb) (2010) 105(1):4–13. doi: 10.1038/hdy.2010.54
287. Duffy KA, Bale TL, Epperson CN. Germ Cell Drivers: Transmission of Preconception Stress Across Generations. Front Hum Neurosci (2021) 15:642762. doi: 10.3389/fnhum.2021.642762
Keywords: obesity, diabetes, brain, sex differences, behaviour, cardiovascular system, prenatal programming
Citation: Grzęda E, Matuszewska J, Ziarniak K, Gertig-Kolasa A, Krzyśko- Pieczka I, Skowrońska B and Sliwowska JH (2022) Animal Foetal Models of Obesity and Diabetes – From Laboratory to Clinical Settings. Front. Endocrinol. 13:785674. doi: 10.3389/fendo.2022.785674
Received: 29 September 2021; Accepted: 11 January 2022;
Published: 07 February 2022.
Edited by:
Claire Joanne Stocker, Aston University, United KingdomReviewed by:
Kevin Pearson, University of Kentucky, United StatesEmilyn Alejandro, University of Minnesota Twin Cities, United States
Copyright © 2022 Grzęda, Matuszewska, Ziarniak, Gertig-Kolasa, Krzyśko- Pieczka, Skowrońska and Sliwowska. This is an open-access article distributed under the terms of the Creative Commons Attribution License (CC BY). The use, distribution or reproduction in other forums is permitted, provided the original author(s) and the copyright owner(s) are credited and that the original publication in this journal is cited, in accordance with accepted academic practice. No use, distribution or reproduction is permitted which does not comply with these terms.
*Correspondence: Joanna H. Sliwowska, joanna.sliwowska@up.poznan.pl