- 1Department of Gastrocolorectal Surgery, General Surgery Center, The First Hospital of Jilin University, Changchun, Jilin, China
- 2Section of Endocrinology, Department of Internal Medicine, School of Medicine, Yale University, New Haven, CT, United States
- 3National Clinical Research Center for Metabolic Diseases, Key Laboratory of Diabetes Immunology, Central South University, Ministry of Education, Changsha, Hunan, China
- 4Department of Metabolism and Endocrinology, The Second Xiangya Hospital, Central South University, Changsha, Hunan, China
- 5Division of Infection and Immunity, Cardiff University School of Medicine, Cardiff, United Kingdom
There has been a major increase in Type 2 diabetes and obesity in many countries, and this will lead to a global public health crisis, which not only impacts on the quality of life of individuals well but also places a substantial burden on healthcare systems and economies. Obesity is linked to not only to type 2 diabetes but also cardiovascular diseases, musculoskeletal disorders, and certain cancers, also resulting in increased medical costs and diminished quality of life. A number of studies have linked changes in gut in obesity development. Dysbiosis, a deleterious change in gut microbiota composition, leads to altered intestinal permeability, associated with obesity and Type 2 diabetes. Many factors affect the homeostasis of gut microbiota, including diet, genetics, circadian rhythms, medication, probiotics, and antibiotics. In addition, bariatric surgery induces changes in gut microbiota that contributes to the metabolic benefits observed post-surgery. Current obesity management strategies encompass dietary interventions, exercise, pharmacotherapy, and bariatric surgery, with emerging treatments including microbiota-altering approaches showing promising efficacy. While pharmacotherapy has demonstrated significant advancements in recent years, bariatric surgery remains one of the most effective treatments for sustainable weight loss. However, access to this is generally limited to those living with severe obesity. This underscores the need for non-surgical interventions, particularly for adolescents and mildly obese patients. In this comprehensive review, we assess longitudinal alterations in gut microbiota composition and functionality resulting from the two currently most effective anti-obesity treatments: pharmacotherapy and bariatric surgery. Additionally, we highlight the functions of gut microbiota, focusing on specific bacteria, their metabolites, and strategies for modulating gut microbiota to prevent and treat obesity. This review aims to provide insights into the evolving landscape of obesity management and the potential of microbiota-based approaches in addressing this pressing global health challenge.
1 Introduction
In the 10th edition of the IDF Diabetes Atlas, an estimated 483 million adults aged 20–79 years worldwide have type 2 diabetes. By 2030, 579 million, and by 2045, 705 million adults aged 20–79 years are projected to be living with type 2 diabetes (1). There is a strong link between type 2 diabetes and overweight and obesity. The WHO defines obesity as a BMI greater than or equal to 30 for adults. Obesity can lead to type 2 diabetes, cardiovascular diseases, musculoskeletal disorders and some cancers, thus increasing medical costs and seriously compromising quality of life in individuals living with obesity (2). It should be noted that BMI definitions of obesity are dependent on ethnicity, which should be taken into account, for example when considering risk of type 2 diabetes and different ranges of weight and BMI relating to obesity and health have been established for different ethnic communities (3, 4). Other measurements such as waist circumference may be a better measure of obesity (5, 6). Obesity is closely related to insulin resistance, glucose intolerance, and changes in various metabolic factors (7). Obesity is also associated with social disadvantage and reduced socio-economic productivity, thus increasingly creating an economic burden (8).
Gut microbiota play an indispensable role in human health (9). Obesity has been associated with perturbation of the intestinal microbiota and changes in intestinal permeability (10). Specifically, diversity and composition of gut microbiota or the presence and abundance of particular bacteria contribute to obesity development (11–14). Gut microbiota are modulated by several factors including diet, genetics, circadian rhythms, anti-obesity medications, probiotics as well as antibiotics (15). Moreover, gut microbiota are also altered by bariatric surgery, leading to microbial changes that may contribute to the observed metabolic benefits after surgery (16, 17). Gut microbiota play a major role in both causing and alleviating obesity; thus, these bacteria should be taken into account when considering effective treatment.
Current treatments of obesity include diet, exercise, pharmacotherapy and bariatric surgery (7, 18). Over the years many different diets have been suggested. One of the healthiest of these in terms of nutritional composition is the Mediterranean diet to tackle obesity and prevent type 2 diabetes (19). Probiotic therapy, brown adipose tissue transplantation and fecal microbiota transplantation also have potential clinical application (20–22). Although there has been significant progress in pharmacotherapy, in recent years (22, 23), with agents that effectively reduce weight and improve metabolism, bariatric surgery is still considered to be the most effective means of sustainable weight loss (24). Currently, most of the anti-obesity medications are contraindicated for use in pregnant women and adolescents (25), and the application of metabolic and bariatric surgery (MBS) in the pediatric population provides evidence-based effective treatment of severe obesity and related comorbid diseases (26, 27). However, bariatric surgery is also associated with significant surgical risks and complications, and thus it is only suitable for the patients with severe obesity with comorbities. Therefore, adolescents and mildly obese patients would benefit from non-surgical treatments to improve their metabolic disorders.
In this review, we provide an overview of the studies that have evaluated longitudinal changes in gut microbiota composition and functionality associated with the two currently very effective anti-obesity treatments, pharmacotherapy, and bariatric surgery (7, 23, 28). We also summarize the function of the gut microbiota or defined bacteria, their metabolites, and strategies for modulating gut microbiota for the prevention/treatment of obesity (Figure 1).
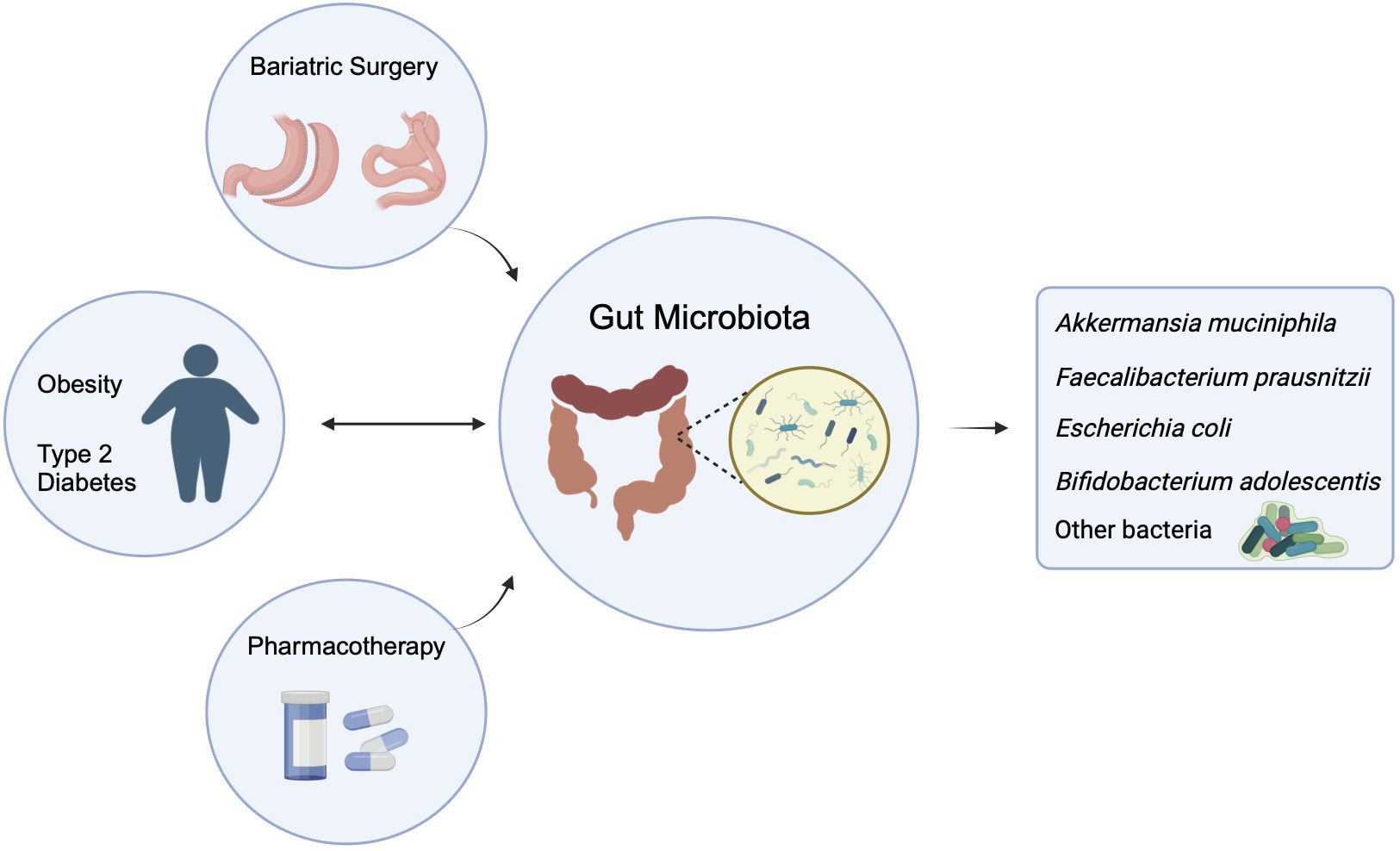
Figure 1 Two-way influence of gut microbiota on obesity and/or type 2 diabetes, and the effect of pharmacotherapy and Bariatric Surgery on the altered gut microbiota. (The figure created by biorender).
2 The role of intestinal microflora in obesity
In humans there are a vast number of microorganisms, which inhabit the skin, mucous membranes and other surfaces as well as different cavities of the body such as the oral, genitourinary, gastrointestinal, and respiratory tracts. It is estimated that the human microbiota number as many as the number of human cells present in our bodies (29). Intestinal microbiota contain genetic information about 150-fold more than the human gene complement (30). Due to the differences in the tissue environment of different parts of the digestive tract, the microbiota are affected by gastric acid, bile acid and secretions from pancreas, and the distribution of intestinal flora is uneven (31). Gastric flora are relatively sparse with about 102 colony-forming units (CFU)/ml, while the total colonic flora can reach 1010-12CFU/ml; moreover, there are obvious differences in the diversity of flora in different segments of digestive tract (32, 33). The intestinal flora of mammals belong mostly to two phyla: Firmicutes and Bacteroidetes, with the other identified phyla including Proteobacteria, Actinobacteria, Fusobacteria and Verrucomicrobia making up a much smaller proportion (34). Previous studies have shown that the gut microbiota play an important role in weight regulation, which can affect fat and energy storage through both energy regulation and gene expression (16). Studies also found that the diversity and abundance of intestinal flora were significantly reduced in people with severe obesity and obesity-related complications, whereas bariatric surgery, carried out as a surgical intervention for morbid obesity, resulted in an increase in microbiota diversity (16). Resveratrol, a natural polyphenol with anti-obesity effect, requires gut microbiota to mediate the effect (35, 36). Those studies suggested that the individuals who are overweight and/or obese may have a specific composition of gut microbiota, which plays an important role in food digestion and energy generation (11, 12, 37).
It is estimated that 10% of the total metabolites in mammalian blood are derived from the gut flora (38), among which short chain fatty acids (SCFAs), lipopolysaccharide (LPS), branched chain amino acids (BCAAs) and bile acids play important roles in metabolic regulation. SCFAs are a product of fermentation of plant polysaccharides produced by different bacteria, which can be absorbed efficiently by the human body (39); The most abundant intestinal SCFAs discovered to date include acetate, propionate, and butyrate, which exhibit anti-inflammatory properties involved in almost every phase of the intestinal mucosal immune response (40). SCFAs not only participate in the regulation of energy metabolism in the body, but also regulate the expression of host genes related to fat metabolism (41). LPS is associated with chronic inflammation, especially in dysbiosis of the intestinal flora with an increase in pathogenic bacteria. These bacteria increase the release of endotoxin LPS, impair the intestinal mucosal barrier and increase intestinal permeability, resulting in low-grade chronic systemic inflammation in the body, which ultimately affects host metabolism, obesity and insulin resistance (42). In addition to LPS, circulating levels of BCAAs tend to be increased in individuals with obesity and are associated with insulin resistance or type 2 diabetes mellitus (43). BCAAS are closely related to the mammalian target of rapamycin signaling pathway (mTOR, mammalian target of rapamycin) in mammals (44), whereas abnormal metabolism of BCAAs may also be associated with accumulation of toxic BCAA metabolites that in turn trigger mitochondrial dysfunction and stress signaling associated with insulin resistance and T2DM (43). Bile acids are metabolites produced by the host and these are biochemically modified by gut bacteria. About 95% of the primary bile acids synthesized in the liver are reabsorbed at the end of the ileum, with half of the remaining 5% bile acids reabsorbed in the colon, and the rest are excreted in feces (45). Bacteria in the small intestine and colon can convert primary bile acids to secondary bile acids, and as a result, the gut microbiota play an important regulatory role in the host’s bile acid cycle. In turn, bile acids influence the composition and overall number of gut microbiota (16, 46). Moreover, reduction in intestinal bile acids contributes to the metabolic benefits of bariatric surgery and a bile acid receptor is required to mediate the effects (47, 48). Other factors are also involved in the interaction between gut microbiota and metabolism, such as Indole derivatives, polyamines, Taurine, ATP, Polysaccharide, hydrogen and methane (40, 49, 50).
3 The effects of pharmacotherapy on gut microbiota
Gut microbial composition is highly diverse and continuously modified by endogenous and exogenous factors (51). Besides the factors that affect obesity and diabetes including diet, exercise and lifestyle, various medications, antibiotics being the largest and strongest contributes, can also add to the changes in gut microbiota (52–54). The effects of even short antibiotic exposure can persist and have long term impacts on human intestinal microbiota (55). Other prominent drugs include chronically administered drugs that include anti-diabetic medications, such as metformin, which alter the gut microbiota (56). The U.S. Food and Drug Administration (FDA) has currently approved medications for the treatment of obesity include short-term phentermine, a combination of phentermine/topiramate, orlistat, a combination of naltrexone and bupropion, liraglutide, and semaglutide (22). Phentermine, combined phentermine and topiramate as well as combined naltrexone and bupropion act on the nervous system, but not much research has been done relating to their effects on gut microbiota. Other FDA approved medications for the treatment of type 2 diabetes, among which biguanides, alpha-glucosidase inhibitors (α-GIs), incretin-based drugs, GLP-1 receptor agonists and DPP-4 inhibitors also affect the gut microbiota (56). Table 1 summaries the effect of pharmacotherapy on gut microbiota (Table 1).
3.1 Orlistat
Orlistat is a reversible inhibitor of pancreatic and gastric lipase, which has beneficial effects on weight loss and metabolism. Orlistat blocks hydrolysis of triglycerides and reduces absorption of ingested fat by about 30% (79). A study showed that high-fat-diet (HFD) fed mice had enriched intestinal bacteria genera including Pseudomonas, Rhodococcus, Roseburia, and Acetivibrio after treatment with orlistat (57). A study in humans showed that the levels of Lactobacillus genus and Lactobacillus gasseri were significantly higher after 8 weeks of Orlistat treatment (58). Orlistat decreased the relative abundance of Alistipes, and Desulfovibrio in the fecal microbiota of HFD-fed mice (60). However, other studies did not demonstrate changes in the microbial diversity, predominant bacteria, enterotypes, and fecal SCFAs were not significantly altered by orlistat (59, 80).
3.2 GLP-1 receptor agonists
GLP-1 is an incretin hormone secreted by gut L cells, a group of intestinal endocrine cells, in response to food ingestion. GLP-1 has many functions including alterations of gut motility and slowing gastric emptying, inhibiting gastric acid and glucagon secretion but increasing insulin secretion (81). GLP-1 receptor agonists (GLP-1RAs) increased intestinal Bacteroidetes to Firmicutes ratio, decreased obesity-related but increased lean-related microbiota phenotypes (61, 82, 83). Studies in mice treated with liraglutide showed a decrease in Erysipelotrichaceae Incertae Sedis, Marvinbryantia, Roseburia, Candidatus, Arthromitus, and Proteobacteria, which were obesity-related phylotypes and an increase in Akkermansia, Blautia, Lactobacillus Parabacteroides and Coprococcus, which were lean-related phylotypes (61, 62), with Akkermansia muciniphila contributing to intestinal health and glucose homeostasis (84). In studies in humans, the genera Collinsella, Akkermansia and Clostridium were enriched in the post-liraglutide-treatment group whereas Alistipes was decreased (63, 64). Little is known about the effect of semaglutide, another GLP-1 RA, on the composition of gut microbiota, although it is highly efficacious in the treatment of obesity (22).
3.3 Metformin
Among all the anti-diabetic medications, metformin, the first-line of medication for T2D treatment, has been most studied in relation to interaction with the gut microbiota. The therapeutic mechanism of metformin, at least in part, requires the involvement of intestinal microbiota (85). Metformin increases a number of different SCFA-producing bacteria, including Blautia, Butyrivibrio, Bifidobacterium bifidum, Megasphaera, and Prevotella (68, 86). Metformin is also associated with an increase in Akkermansia muciniphila, a mucin degrading microbiota (68). In studies in humans, the genera Akkermansia, Ruminococcus, Escherichia and Lactobacillus were enriched whereas Bifidiobacterium, Paraprevotella, Blautia and Faecalibacterium decreased in the post-Metformin-treatment group (65–67). However, these are not the only associated bacteria, and another study in humans suggested that the improvement of metabolism through metformin was mediated by a Bacteroides fragilis (72). The increase of Akkermansia post Metformin treatment is prominent and has been found in both human and mouse model studies (69–71).
3.4 Alpha glucosidase inhibitors
Acarbose, an alpha glucosidase inhibitor used to treat T2DM, is derived from bacterial fermentation; thus, it is logical that acarbose is likely to affect the gut microbiota (87). It is less used with the advent of newer medications as individuals find it difficult to take because of flatulence. Acarbose increases the relative abundances of Lactobacillus and Bifidobacterium in the gut microbiota and depletes Bacteroides in patients with type 2 diabetes (73–75).
3.5 Other anti-diabetic drugs
Sitagliptin, a dipeptidyl peptidase (DPP|0-4) inhibitor, increases the relative abundance of Bacteroidetes and Proteobacteria but decreases Firmicutes (88). In an animal study, the authors found that sitagliptin affects the abundance of SCFA-producing bacteria, Blautia, Roseburia and Clostridium, as well as probiotics Lactobacillus, Bifidobacterium (76). Also in animal studies, dapagliflozin and canagliflozin, SGLT-2 inhibitors, were found to increase the ratio of Bacteroidetes to Firmicutes. Moreover, dapagliflozin increased the abundance of Akkermansia muciniphila and decreased Oscillospira whereas canagliflozin increased the abundance of Olsenella, Alistipes and Alloprevotella, but decreased the abundance of Helicobacter and Mucispirillum (77, 78).
4 The effects of bariatric surgery on gut microbiota
Although diet, exercise and pharmacotherapy can effectively reduce an individual’s body weight and improve their metabolism, metabolic/bariatric surgery is still considered to be the most effective mean of sustainable weight loss (24, 89). The American Society for Metabolic and Bariatric Surgery (ASMBS) endorsed common procedures including Adjustable Gastric Band (AGB), Sleeve Gastrectomy, Roux-en-Y Gastric Bypass (RYGB), Biliopancreatic Diversion with Duodenal Switch (BPD/DS), and Single Anastomosis Duodeno-Ileal Bypass with Sleeve Gastrectomy (SADI-S), although AGB is less successful as a treatment for type 2 diabetes (90). Bariatric surgery is generally reserved for patients with severe obesity (BMI > 40 kg/m2) and moderate obesity (BMI > 35 kg/m2) with at least one additional serious complication such as hypertension, diabetes, or sleep apnea syndrome (91). If other interventions for treating obesity and obesity-related co-morbidities have not been effective, bariatric surgery should be considered for the individuals with class I obesity (BMI 30–34.9 kg/m2). It is noteworthy that BMI risk zones should be adjusted to define obesity with a BMI threshold of 25–27.5 kg/m2 in Asian population (89). The American Society for Metabolic and Bariatric Surgery Pediatric Committee and the American Academy of Pediatrics recommend that children/adolescents with class II obesity (BMI=35 to 39.9) and a co-morbidity, or with class III obesity (BMI=40 and greater) should be considered for bariatric surgery (92, 93). Bariatric surgery improves blood glucose, increases insulin sensitivity, promotes insulin secretion, and changes the levels of incretin (94). In addition, researchers believe that the efficacy of bariatric surgery is also closely related to changes in both the type and abundance of gut microbiota. The surgery effect can be sustained for ten years, allowing patients to achieve sustained weight loss (95).
Previous studies have found that the impact of bariatric surgery on gut microbiota were reflected in the following aspects. Firstly, bariatric surgery alters the number of gut microbiota. Zhang and colleagues were pioneers who found changes of intestinal flora in bariatric surgery patients in 2009 and reported that Firmicutes were significantly decreased in post-gastric-bypass individuals (96). In the following year, Furet and co-authors observed that RYGB rapidly induced gut microbiota adaptation, through which the F. prausnitzii species was directly linked to reduction in the low-grade inflammatory state in obesity and diabetes that was independent of calorie intake (97). Secondly, bariatric surgery affects the gut microbiota by changing the patient’s eating habits. Individuals who underwent gastric bypass surgery had less interest in high-fat and high-sugar foods after surgery (98, 99), and these changes in dietary composition and amount of the food intake also altered the levels of Firmicutes and Bacteroides in the intestinal flora (100). Thirdly, bariatric surgery also changes the pH of the digestive tract as the surgery changes the structure of the gastrointestinal tract, which affects the secretion of gastric acid and the pH of the gastrointestinal tract, resulting in changes in the intestinal luminal environment and concomitantly, the intestinal flora (101, 102). Moreover, changes in colonic pH can affect the composition of colonic microbial communities as well as the proportion of SCFA-producing bacteria (103). Fourthly, bariatric surgery changes enterohepatic cycle diversion that affects gut microbiota. Gastric bypass surgery shortens the time for food emptying and can lead to changes in both primary and secondary bile acid levels, both of which have antimicrobial properties (104, 105). The administration of secondary bile acids altered the composition of the gut microbiota with increased Firmicutes and decreased Bacteroidetes levels (106). Fifthly, the use of antibiotics for bowel preparation for surgery. Bariatric surgery requires the use of prophylactic antibiotics, and different types of antibiotics have different effects on the growth of intestinal bacteria and the overall diversity of bacteria (107). Claesson et al. found that the use of antibiotics increased the abundance of Bacteroides and decreased the proportion of Firmicutes (108). Lastly, gastric bypass surgery can lead to changes in neurohormones which include increased secretion of GLP-1 (109), known to signal satiety, reduce food intake, decrease stomach motility and increase β-cell insulin production (16). All these factors may have an impact on the intestinal microbiota. In addition, GLP-1 directly regulates the composition and function of the intestinal flora (110).
Overall, bariatric surgery changes the internal environment of the intestine and the secretion of gastrointestinal hormones by altering the structure of the gastrointestinal tract that causes the amount and the type of food intake, thereby improving glycolipid metabolism and insulin resistance. All these factors assist in achieving the sustained weight loss that is closely related to the changes in intestinal flora after surgery. Studies using modern technologies, which include large-scale high-throughput sequencing, metagenomic sequencing and metabolomics will provide us with better and broader understanding of the changes in the composition and function of intestinal flora after surgery. This knowledge will help us to identify specific bacteria strains and/or related metabolites for potentially more effective treatment of obesity and associated complications. Table 2 summarizes the alterations in gut microbiota post-surgery (Table 2); further details are reviewed below.
4.1 Adjustable gastric band
AGB surgery is a gastric restrictive procedure in which a prosthetic band is placed around the upper stomach to compartmentalize it into a small pouch and a large remnant, and the adjustable band allows changes in the stoma size. Although AGB surgery has been reported to increase gut microbial gene abundance (123) and one study showed that Actinobacteria increased post AGB surgery (111), more in depth effects of the AGB procedure on gut microbiota have not been investigated as much as in bariatric surgery (124). This is partly because AGB does not make radical changes in gastrointestinal anatomy (125).
4.2 Sleeve gastrectomy
Sleeve gastrectomy is another gastric restrictive procedure that involves permanently removing approximately 80% of the stomach. The remaining stomach retains the pylorus with a shape like a banana. Sleeve gastrectomy significantly alters gut microbiota. Bacteroides Thetaiotaomicron, an obesity-associated gut microbial species, was markedly decreased in obese individuals after sleeve gastrectomy and this procedure also alleviated diet-induced body-weight gain and adiposity in mice (11). In addition, Roseburia, which are highly correlated with T2DM, were also affected by sleeve gastrectomy (126, 127). In a mouse model study, Roseburia were found to be negatively correlated with AUC in the glucose tolerance test after sleeve gastrectomy (128). Multivariate analysis demonstrated differential abundance of Bulleidia increased after surgery (112, 113). SG resulted in increased Bacteroidetes phyla, and an increase in Roseburia species was observed among those achieving diabetes remission (115). Another study showed that, except for a postoperative increase in Faecalibacterium prausnitzii, laparoscopic sleeve gastrectomy (LSG) resulted in the postoperative reduction in Firmicutes including Coprococcus, a butyrate-producing bacterium (114).
4.3 Roux-en-Y gastric bypass
RYGB is both a restrictive and malabsorptive procedure in which a small gastric pouch (∼30 ml) is created and directly linked to the distal jejunum by the Roux limb. The proximal part of the jejunum is subsequently anastomosed 1.5 m below the gastrojejunal anastomosis. It is one of the most common and highly effective operations for treating morbid obesity with or without T2DM. Interestingly, the gut microbiota richness was increased after RYGB (119). Firmicutes were significantly decreased whereas Bacteroidetes were significantly increased in the individuals post-RYGB. Moreover, Akkermansia became prominent in post-gastric-bypass individuals, comparable to the individuals with normal body weight (96, 117, 118). The abundance of F. prausnitzii, which was directly correlated to fasting blood glucose, was lower in individuals with morbid obesity with or without diabetes before RYGB, but increased three months post-RYGB and remained stable until the study end point - six months post-RYGB (97, 120). It was demonstrated that the altered human microbiota due to the surgery reduced fat deposition in the germ-free recipient mice colonized with bacteria from stool samples from the human donors (95).
4.4 Biliopancreatic diversion with duodenal switch
The BPD-DS probably is the most effective approved metabolic operation for the treatment of obesity with T2DM. However, BPD is rarely performed today due to the metabolic disturbances and nutritional deficiencies that occur after the procedure (129). There are two steps of the procedure – the first is to create a sleeve-like stomach, after which the distal jejunum is connected to the outlet of the newly-created stomach. Germ-free mice colonized with microbiota from patients post-BPD-DS, had lower villus height/width and crypt depth in the distal jejunum, as well as lower intestinal glucose absorption (116). The colonized mice also had higher Parabacteroides and lower Blautia, which coincided with improved blood glucose (116). Studies in Wistar Rats showed that the rats had a higher abundance of Bifidobacterium and lower abundance of the Clostridiales in the common and alimentary limbs, similar to that in fecal samples, taken after BPD-DS. Moreover, both the body weight gain and fat mass were positively correlated with the microbial abundances of Clostridiales, contributing to the positive metabolic outcomes of BPD-DS (121, 122).
4.5 Single anastomosis duodeno-ileal bypass with sleeve gastrectomy
SADI-S is the latest procedure endorsed by ASMBS. SADI-S is similar to the BPD-DS but simpler than BPD-DS and RYGB, as there is only one anastomosis. It is an excellent option for patients who have already had a sleeve gastrectomy and are seeking further weight loss. The study using Wistar rats showed that the altered gut microbiota post-SADI-S shared similar signatures and changes in metabolism as seen in the other two types of restrictive and malabsorptive procedures, RYGB and BPD/DS (122).
5 Bacteria closely related to obesity and diabetes
How can we define a causal role for a bacterium in diabetes or obesity? The first criterion of Koch’s postulates is to demonstrate a putative agent causes an infectious disease (130). Evans proposed a modified version as a unified concept for establishing causation by a putative cause of disease. In the modified postulates, three lines of evidence are required: a) an association between the disease phenotypes and the presence of the cells or genetic material of the putative cause; b) the reproduction or reduction of the disease phenotypes by experimental addition or removal of the putative cause in humans or animals; c) and the occurrence of host molecular responses that mechanistically connect the presence of the putative cause to the occurrence of the disease (9, 130). Here, we will use the modified version of Koch’s postulates as a conceptual framework for organizing evidence to illustrate the causative role of gut microbiota in obesity and diabetes, as summarized in Table 3 (Table 3).
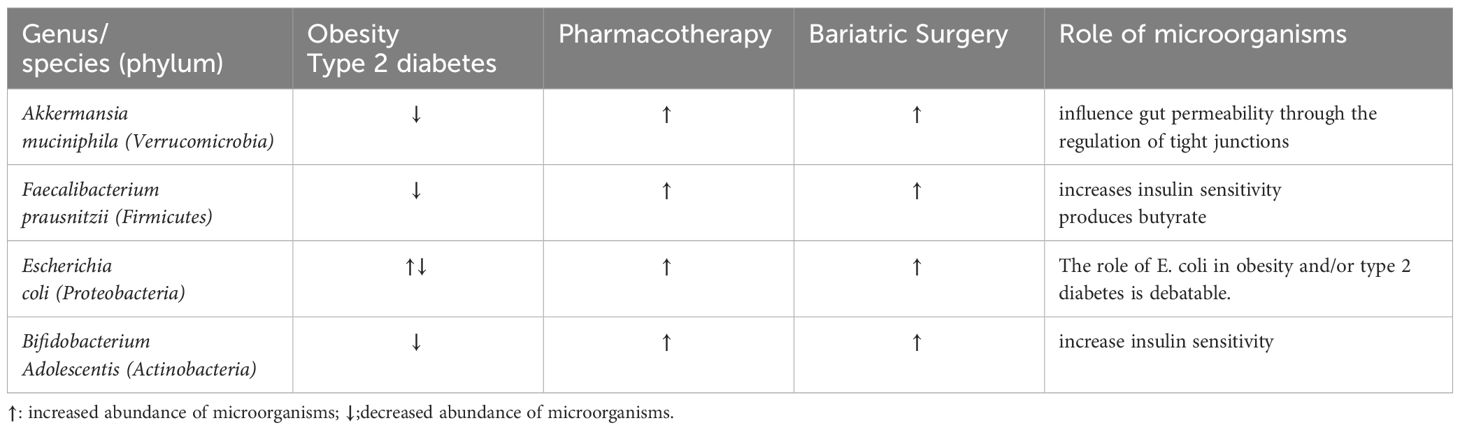
Table 3 Summary of the altered gut microbiota in obesity and T2D and the outcome after medical intervention.
5.1 Akkermansia muciniphila
Akkermansia muciniphila, of the phylum Verrucomicrobia, is extensively present in the intestinal mucosa of humans and other mammals (131), and they account for 1 to 3 percent of the total gut microbiota in healthy adults (132). A. muciniphila has a number of beneficial features in respect of metabolism and is inversely correlated with fasting glucose, waist-to-hip ratio and subcutaneous adipocyte diameter, and importantly, negatively correlated with diabetes, obesity, and metabolic syndromes (133, 134). Oral administration of A. muciniphila at a dose of 2 × 108 bacterial cells per day reversed HFD-induced obesity in mice, and improved several metabolic parameters in both mice and humans (69, 135, 136). A. muciniphila has also been shown to be important in changes associated with therapy used for diabetes. HFD-fed mice treated with metformin showed a higher abundance of Akkermansia than HFD-fed mice without metformin treatment (69). In people living with type 2 diabetes, increased abundance of A. muciniphila was seen in those treated GLP1-agonist therapy (137). Surgical treatment with RYGB also led to increased relative abundance of A. muciniphila (118). With respect to physiological changes associated with A. muciniphila, the bacteria strongly adhere to human colonic cell lines and improve enterocyte monolayer integrity (138). A. muciniphila also induces interleukin 8 (IL-8) production by enterocytes (138), the role of which is not clear. A. muciniphila-derived extracellular vesicles influence gut permeability through the regulation of tight junctions (139). A study also showed that Pili-like proteins of A. muciniphila modulate host immune responses and gut barrier function (140). Thus, according to the modified version of Koch’s postulates, the above examples are evidence for the role of A. muciniphila with a reduction in the bacteria occurring in obesity and diabetes.
5.2 Faecalibacterium prausnitzii
Faecalibacterium prausnitzii (F. prausnitzii) is one of the most abundant bacterial species in the colon of healthy human adults, representing more than 5% of the total intestinal bacterial population (141). This is another bacterial species that is associated with improved glucose metabolism and reduced inflammation. People living with obesity have lower levels of F. prausnitzii in their gut microbiota (142, 143). F. prausnitzii transplantation has been shown to be an effective therapeutic approach for diabetes and related complications. The procedure prevents low-grade inflammation due to altered gut microbiota, protects the pancreas from autoimmunity and increases insulin sensitivity (144, 145). The abundance of F. prausnitzii was increased in people living with type 2 diabetics who had GLP1-agonist therapy (137). The level of F. prausnitzii, which was lower in the individuals living with Type 2 diabetes before RYGB, increased at 3-months post-surgery and remained stable at 6-months post-surgery (97). F. prausnitzii produces butyrate, which beneficially modulates the intestinal immune system, oxidative stress and colonocyte metabolism (146, 147). Taken together, the above studies demonstrated the pathogenic role of F. prausnitzii reduction in obesity and diabetes, based on the modified version of the Koch’s postulates.
5.3 Escherichia coli
Escherichia coli (E. coli) is the most abundant commensal aero-anaerobic Gram-negative bacillus of the vertebrate gut, and most E. coli strains are harmless (148). However, E. coli have been linked to increased weight in both humans and in mice. E. coli was found to be predictive of the development of insulin resistance in postmenopausal obese Caucasian females in Sweden (149). In a HFD-fed mouse model of obesity, E. coli significantly increased body weight and adiposity, and induced impaired glucose tolerance (150). Therapeutically, in a human randomized trial, paradoxically, the investigators found that metformin treatment significantly increased amplicon sequence of E. coli variants (65). The increase of the relative abundance in E. coli, was also observed in patients after VGB or RYGB (95, 97). However, colonization of E. coli in germ-free mice led to increased inflammation in liver, adipose tissue and intestinal tissue under a HFD regimen (150). The role of E. coli in obesity and/or type 2 diabetes is debatable as there are contradictory findings; thus, we need to further clarify the mechanism of action of E. coli in obesity and diabetes in future research.
5.4 Bifidobacterium adolescentis
Bifidobacteria are gram-positive and one of the most important bacteria in the human and animal intestinal environment. The gut microflora in people living with T2D had a lower abundance of Bifidobacterium adolescents (151) whereas metformin promoted the growth of Bifidobacterium adolescentis (152). In an obese rat study, Bifidobacterium adolescentis was increased significantly after RYGB (153). Moreover, Bifidobacterium adolescentis supplementation ameliorated visceral fat accumulation and insulin sensitivity of HFD-induced obesity in rats (154). B. adolescentis has a higher number of core genes including unique environmental tolerance, carbon and nitrogen utilization genes, and a blood sugar regulation gene which was likely the reason why B. adolescentis may reduce T2D, shown in a mouse study (155). Thus, according to the concept of modified version of Koch’s postulates, a reduction in B. adolescentis could play a causative role in obesity and diabetes.
6 Conclusions and perspectives
There has been substantial evidence supporting the role of gut microbiota in the development of obesity and related diseases. It is clear that the composition of the gut microbiota evolves after drug treatment or bariatric surgery, but these observations remain to be confirmed in larger and longer-term cohorts (especially in the patients who regained weight). Although the ratio of Firmicutes/Bacteroidetes was reduced by dietary and surgical interventions, in our review of the literature, we have highlighted an increase of Firmicutes abundance at genus/species levels in almost all types of interventions. Moreover, there are more genera associated with Lactobacillus and fewer with Ruminococcus, both of which are Firmicutes phyla. Interestingly, Akkermansia muciniphila, phylum Verrucomicrobia, was also increased after the interventions and it is worth mentioning that Akkermansia muciniphila has been reported to be useful in a new generation of probiotics. It would be helpful in the clinical setting if some individual bacterial genera/species/strains could serve as biomarkers for evaluation of diabetes and the efficacy of treatment rather than the Firmicutes/Bacteroidetes ratio or microbial diversity index. It is clear that in studies of gut microbiota associated with metabolic disorders, a deeper investigation of bacterial differences at the strain level will be required, as well as understanding changes in other microorganisms including enteroviruses (156) and intestinal fungi (157). It is important that there are more adequately designed studies using next-generation sequencing and ‘omics’ technologies to identify the bona fide causal microorganisms, which could lead to more specific interventions. However, that does not exclude research based on Koch’s hypothesis to identify specific gut bacteria that may play a pathogenic role in obesity and diabetes, or microbes which may be decreased in these conditions, which may lead to novel opportunities for treatment of obesity and diabetes prevention and treatment. Interventions that reduce species associated with uncontrolled diabetes but increase species associated with a healthy gut of individuals without diabetes would be ideal for treating diabetes.
There is no doubt that bariatric surgery affects the gut microbiota, altering their composition and function. Bariatric surgery changes the anatomy of digestive tract, affects gut hormonal homeostasis, influences the amount and choice of food ingestion, all of which contribute to the alteration of the microbiota composition. This complexity is challenging for clinical research. Next-generation sequencing and ‘omics’ technologies will provide powerful tools for better understanding of how the gut microbiota and host metabolism interact and influence the outcome of bariatric surgery. There are still some inconsistent results from studies investigating whether altered gut microbiota cause weight loss after bariatric surgery for the treatment of obesity and diabetes. Thus, whether microbiota evolution is a cause or a consequence of weight loss and improvements in obesity-related diseases is still inconclusive. Much research is needed in investigating the role of the gut microbiota in metabolic outcomes after bariatric surgery and the underlying mechanisms that mediate this vital relationship, in which Koch’s hypotheses may aid our understanding. However, healthy gut microbiota will help better therapeutic outcomes of the surgery and the gut microbiota could be a biomarker for predicting the efficacy of the bariatric surgery and potentially a useful tool for personalized medicine.
We have little knowledge about the majority of gut bacteria, although high throughput sequence technology has helped us identify some of these. Moreover, most gut bacteria cannot be cultured due to inflexible nutrient requirements and/or the need to co-exist with other bacteria (158). However, the ultimate proof of the causality of gut bacteria in obesity will be their ability to induce obesity in a non-obese host, such as germ-free mice (50) as well as the proof of ameliorating obesity when removing them. Although neither humans nor animals live in a germ-free environment, the gnotobiotic animal model provides unique opportunities to better understand the function of the gut bacteria and the molecular interactions between obesity-inducing bacteria and the host. In addition to the known host responses to endotoxins, transcriptomic and proteomic analyses are powerful assets to help us better understand the genome-wide responses of different host tissues to the colonized “pathogens”. With improved knowledge, we will be able to identify potential new targets and design more effective pharmacological interventions. With the increased need for organoid research, highly functional intestinal organ culture systems based on microfluidics have been developed (159). The authors found that observations made previously in vivo could be reliably replicated in vitro with the organoid system, while being able to extract compelling new insights due to the tightly controlled nature of the organoid system (159). New systems like this will be critical for elucidation of the more complex connections between host and microbiome.
The gut microbiota plays an integral role in human health and studies in this area have opened new areas of research in basic human biology and clinical medicine. Koch’s hypothesis can be applied and there is some evidence that specific gut bacteria may play a causative role in non-communicable diseases such as obesity. The study of the gut microbiota has led and will in the future further advance the development of novel means to combat various health issues including the epidemic of obesity and associated devastating complications, that increasingly challenge human society.
Author contributions
LZ: Writing – original draft. PW: Writing – original draft. JH: Writing – original draft. YX: Writing – original draft. FW: Writing – review & editing. JS: Supervision, Writing – review & editing. LW: Supervision, Writing – review & editing.
Funding
The author(s) declare that financial support was received for the research, authorship, and/or publication of this article. This work was supported by funding support from Jilin Province Department of Finance, (grant number JLSWSRCZX2020-067 to LZ), a Medical Research Council grant (MR/K021141/1 to FW) and the National Institutes of Health (HD 097808, DK 126809, DK 130318, Diabetes Action Research and Education Foundation to LW).
Conflict of interest
The authors declare that the research was conducted in the absence of any commercial or financial relationships that could be construed as a potential conflict of interest.
Publisher’s note
All claims expressed in this article are solely those of the authors and do not necessarily represent those of their affiliated organizations, or those of the publisher, the editors and the reviewers. Any product that may be evaluated in this article, or claim that may be made by its manufacturer, is not guaranteed or endorsed by the publisher.
References
2. Organization WH. Fact sheet on obesity and overweight. (2021). Available online at: https://www.who.int/news-room/fact-sheets/detail/obesity-and-overweight#cms.
3. Heymsfield SB, Peterson CM, Thomas DM, Heo M, Schuna JM Jr. Why are there race/ethnic differences in adult body mass index-adiposity relationships? A quantitative critical review. Obes Rev. (2016) 17:262–75. doi: 10.1111/obr.12358
4. Caleyachetty R, Barber TM, Mohammed NI, Cappuccio FP, Hardy R, Mathur R, et al. Ethnicity-specific BMI cutoffs for obesity based on type 2 diabetes risk in England: a population-based cohort study. Lancet Diabetes Endocrinol. (2021) 9:419–26. doi: 10.1016/S2213-8587(21)00088-7
5. Yuan Y, Liu K, Zheng M, Chen S, Wang H, Jiang Q, et al. Analysis of changes in weight, waist circumference, or both, and all-cause mortality in Chinese adults. JAMA Netw Open. (2022) 5:e2225876. doi: 10.1001/jamanetworkopen.2022.25876
6. Ross R, Neeland IJ, Yamashita S, Shai I, Seidell J, Magni P, et al. Waist circumference as a vital sign in clinical practice: a Consensus Statement from the IAS and ICCR Working Group on Visceral Obesity. Nat Rev Endocrinol. (2020) 16:177–89. doi: 10.1038/s41574-019-0310-7
7. Mun EC, Blackburn GL, Matthews JB. Current status of medical and surgical therapy for obesity. Gastroenterology. (2001) 120:669–81. doi: 10.1053/gast.2001.22430
8. Bluher M. Obesity: global epidemiology and pathogenesis. Nat Rev Endocrinol. (2019) 15:288–98. doi: 10.1038/s41574-019-0176-8
9. Zhao L. The gut microbiota and obesity: from correlation to causality. Nat Rev Microbiol. (2013) 11:639–47. doi: 10.1038/nrmicro3089
10. Cox AJ, West NP, Cripps AW. Obesity, inflammation, and the gut microbiota. Lancet Diabetes Endocrinol. (2015) 3:207–15. doi: 10.1016/S2213-8587(14)70134-2
11. Liu R, Hong J, Xu X, Feng Q, Zhang D, Gu Y, et al. Gut microbiome and serum metabolome alterations in obesity and after weight-loss intervention. Nat Med. (2017) 23:859–68. doi: 10.1038/nm.4358
12. Fei N, Zhao L. An opportunistic pathogen isolated from the gut of an obese human causes obesity in germfree mice. ISME J. (2013) 7:880–4. doi: 10.1038/ismej.2012.153
13. Cotillard A, Kennedy SP, Kong LC, Prifti E, Pons N, Le Chatelier E, et al. Dietary intervention impact on gut microbial gene richness. Nature. (2013) 500:585–8. doi: 10.1038/nature12480
14. Crovesy L, Masterson D, Rosado EL. Profile of the gut microbiota of adults with obesity: a systematic review. Eur J Clin Nutr. (2020) 74:1251–62. doi: 10.1038/s41430-020-0607-6
15. Wen L, Duffy A. Factors influencing the gut microbiota, inflammation, and type 2 diabetes. J Nutr. (2017) 147:1468S–75S. doi: 10.3945/jn.116.240754
16. Aron-Wisnewsky J, Dore J, Clement K. The importance of the gut microbiota after bariatric surgery. Nat Rev Gastroenterol Hepatol. (2012) 9:590–8. doi: 10.1038/nrgastro.2012.161
17. Gasmi A, Bjorklund G, Mujawdiya PK, Semenova Y, Dosa A, Piscopo S, et al. Gut microbiota in bariatric surgery. Crit Rev Food Sci Nutr. (2022) 63(28):9299–314. doi: 10.1080/10408398.2022.2067116
18. Vetter ML, Faulconbridge LF, Webb VL, Wadden TA. Behavioral and pharmacologic therapies for obesity. Nat Rev Endocrinol. (2010) 6:578–88. doi: 10.1038/nrendo.2010.121
19. Muscogiuri G, Verde L, Sulu C, Katsiki N, Hassapidou M, Frias-Toral E, et al. Mediterranean diet and obesity-related disorders: what is the evidence? Curr Obes Rep. (2022) 11:287–304. doi: 10.1007/s13679-022-00481-1
20. Zhang Z, Mocanu V, Cai C, Dang J, Slater L, Deehan EC, et al. Impact of fecal microbiota transplantation on obesity and metabolic syndrome-A systematic review. Nutrients. (2019) 11(10):2291. doi: 10.3390/nu11102291
21. White JD, Dewal RS, Stanford KI. The beneficial effects of brown adipose tissue transplantation. Mol Aspects Med. (2019) 68:74–81. doi: 10.1016/j.mam.2019.06.004
22. Angelidi AM, Belanger MJ, Kokkinos A, Koliaki CC, Mantzoros CS. Novel noninvasive approaches to the treatment of obesity: from pharmacotherapy to gene therapy. Endocr Rev. (2022) 43:507–57. doi: 10.1210/endrev/bnab034
23. Williams DM, Nawaz A, Evans M. Drug therapy in obesity: A review of current and emerging treatments. Diabetes Ther. (2020) 11:1199–216. doi: 10.1007/s13300-020-00816-y
24. Lingvay I, Sumithran P, Cohen RV, le Roux CW. Obesity management as a primary treatment goal for type 2 diabetes: time to reframe the conversation. Lancet. (2022) 399:394–405. doi: 10.1016/S0140-6736(21)01919-X
25. Son JW, Kim S. Comprehensive review of current and upcoming anti-obesity drugs. Diabetes Metab J. (2020) 44:802–18. doi: 10.4093/dmj.2020.0258
26. Bolling CF, Armstrong SC, Reichard KW, Michalsky MP, Section On Obesity SOS. Metabolic and bariatric surgery for pediatric patients with severe obesity. Pediatrics. (2019) 144(6):e20193224. doi: 10.1542/peds.2019-3224
27. Griggs CL, Perez NP Jr., Goldstone RN, Kelleher CM, Chang DC, Stanford FC, et al. National trends in the use of metabolic and bariatric surgery among pediatric patients with severe obesity. JAMA Pediatr. (2018) 172:1191–2. doi: 10.1001/jamapediatrics.2018.3030
28. Gloy VL, Briel M, Bhatt DL, Kashyap SR, Schauer PR, Mingrone G, et al. Bariatric surgery versus non-surgical treatment for obesity: a systematic review and meta-analysis of randomised controlled trials. BMJ. (2013) 347:f5934. doi: 10.1136/bmj.f5934
29. Sender R, Fuchs S, Milo R. Are we really vastly outnumbered? Revisiting the ratio of bacterial to host cells in humans. Cell. (2016) 164:337–40. doi: 10.1016/j.cell.2016.01.013
30. Qin J, Li R, Raes J, Arumugam M, Burgdorf KS, Manichanh C, et al. A human gut microbial gene catalogue established by metagenomic sequencing. Nature. (2010) 464:59–65. doi: 10.1038/nature08821
31. O'Hara AM, Shanahan F. The gut flora as a forgotten organ. EMBO Rep. (2006) 7:688–93. doi: 10.1038/sj.embor.7400731
32. Walker MM, Talley NJ. Review article: bacteria and pathogenesis of disease in the upper gastrointestinal tract–beyond the era of Helicobacter pylori. Aliment Pharmacol Ther. (2014) 39:767–79. doi: 10.1111/apt.12666
33. Lin CS, Chang CJ, Lu CC, Martel J, Ojcius DM, Ko YF, et al. Impact of the gut microbiota, prebiotics, and probiotics on human health and disease. BioMed J. (2014) 37:259–68. doi: 10.4103/2319-4170.138314
34. Eckburg PB, Bik EM, Bernstein CN, Purdom E, Dethlefsen L, Sargent M, et al. Diversity of the human intestinal microbial flora. Science. (2005) 308:1635–8. doi: 10.1126/science.1110591
35. Zhang E, Agua A, Huang W. A gut feeling for drugs that have metabolic benefits. Nat Commun. (2023) 14:4464. doi: 10.1038/s41467-023-40167-3
36. Pang J, Raka F, Heirali AA, Shao W, Liu D, Gu J, et al. Resveratrol intervention attenuates chylomicron secretion via repressing intestinal FXR-induced expression of scavenger receptor SR-B1. Nat Commun. (2023) 14:2656. doi: 10.1038/s41467-023-38259-1
37. Lopez-Siles M, Duncan SH, Garcia-Gil LJ, Martinez-Medina M. Faecalibacterium prausnitzii: from microbiology to diagnostics and prognostics. ISME J. (2017) 11:841–52. doi: 10.1038/ismej.2016.176
38. Wikoff WR, Anfora AT, Liu J, Schultz PG, Lesley SA, Peters EC, et al. Metabolomics analysis reveals large effects of gut microflora on mammalian blood metabolites. Proc Natl Acad Sci U S A. (2009) 106:3698–703. doi: 10.1073/pnas.0812874106
39. Canfora EE, Jocken JW, Blaak EE. Short-chain fatty acids in control of body weight and insulin sensitivity. Nat Rev Endocrinol. (2015) 11:577–91. doi: 10.1038/nrendo.2015.128
40. Postler TS, Ghosh S. Understanding the holobiont: how microbial metabolites affect human health and shape the immune system. Cell Metab. (2017) 26:110–30. doi: 10.1016/j.cmet.2017.05.008
41. Backhed F, Ding H, Wang T, Hooper LV, Koh GY, Nagy A, et al. The gut microbiota as an environmental factor that regulates fat storage. Proc Natl Acad Sci U S A. (2004) 101:15718–23. doi: 10.1073/pnas.0407076101
42. Cani PD, Amar J, Iglesias MA, Poggi M, Knauf C, Bastelica D, et al. Metabolic endotoxemia initiates obesity and insulin resistance. Diabetes. (2007) 56:1761–72. doi: 10.2337/db06-1491
43. Lynch CJ, Adams SH. Branched-chain amino acids in metabolic signalling and insulin resistance. Nat Rev Endocrinol. (2014) 10:723–36. doi: 10.1038/nrendo.2014.171
44. Newgard CB, An J, Bain JR, Muehlbauer MJ, Stevens RD, Lien LF, et al. A branched-chain amino acid-related metabolic signature that differentiates obese and lean humans and contributes to insulin resistance. Cell Metab. (2009) 9:311–26. doi: 10.1016/j.cmet.2009.02.002
45. Martinez-Augustin O, Sanchez de Medina F. Intestinal bile acid physiology and pathophysiology. World J Gastroenterol. (2008) 14:5630–40. doi: 10.3748/wjg.14.5630
46. Wahlstrom A, Sayin SI, Marschall HU, Backhed F. Intestinal crosstalk between bile acids and microbiota and its impact on host metabolism. Cell Metab. (2016) 24:41–50. doi: 10.1016/j.cmet.2016.05.005
47. Ding L, Zhang E, Yang Q, Jin L, Sousa KM, Dong B, et al. Vertical sleeve gastrectomy confers metabolic improvements by reducing intestinal bile acids and lipid absorption in mice. Proc Natl Acad Sci U.S.A. (2021) 118(6):e2019388118. doi: 10.1073/pnas.2019388118
48. Ding L, Sousa KM, Jin L, Dong B, Kim BW, Ramirez R, et al. Vertical sleeve gastrectomy activates GPBAR-1/TGR5 to sustain weight loss, improve fatty liver, and remit insulin resistance in mice. Hepatology. (2016) 64:760–73. doi: 10.1002/hep.28689
49. Valdes AM, Walter J, Segal E, Spector TD. Role of the gut microbiota in nutrition and health. BMJ. (2018) 361:k2179. doi: 10.1136/bmj.k2179
50. Samuel BS, Gordon JI. A humanized gnotobiotic mouse model of host-archaeal-bacterial mutualism. Proc Natl Acad Sci U S A. (2006) 103:10011–6. doi: 10.1073/pnas.0602187103
51. Meijnikman AS, Gerdes VE, Nieuwdorp M, Herrema H. Evaluating causality of gut microbiota in obesity and diabetes in humans. Endocr Rev. (2018) 39:133–53. doi: 10.1210/er.2017-00192
52. Saad MJ, Santos A, Prada PO. Linking gut microbiota and inflammation to obesity and insulin resistance. Physiol (Bethesda). (2016) 31:283–93. doi: 10.1152/physiol.00041.2015
53. Falony G, Joossens M, Vieira-Silva S, Wang J, Darzi Y, Faust K, et al. Population-level analysis of gut microbiome variation. Science. (2016) 352:560–4. doi: 10.1126/science.aad3503
54. Murphy EF, Cotter PD, Healy S, Marques TM, O'Sullivan O, Fouhy F, et al. Composition and energy harvesting capacity of the gut microbiota: relationship to diet, obesity and time in mouse models. Gut. (2010) 59:1635–42. doi: 10.1136/gut.2010.215665
55. Jernberg C, Lofmark S, Edlund C, Jansson JK. Long-term ecological impacts of antibiotic administration on the human intestinal microbiota. ISME J. (2007) 1:56–66. doi: 10.1038/ismej.2007.3
56. Montandon SA, Jornayvaz FR. Effects of antidiabetic drugs on gut microbiota composition. Genes (Basel). (2017) 8(10):250. doi: 10.3390/genes8100250
57. Ke J, An Y, Cao B, Lang J, Wu N, Zhao D. Orlistat-induced gut microbiota modification in obese mice. Evid Based Complement Alternat Med. (2020) 2020:9818349. doi: 10.1155/2020/9818349
58. Uehira Y, Ueno H, Miyamoto J, Kimura I, Ishizawa Y, Iijima H, et al. Impact of the lipase inhibitor orlistat on the human gut microbiota. Obes Res Clin Pract. (2023) 17:411–20. doi: 10.1016/j.orcp.2023.08.005
59. Jin J, Cheng R, Ren Y, Shen X, Wang J, Xue Y, et al. Distinctive gut microbiota in patients with overweight and obesity with dyslipidemia and its responses to long-term orlistat and ezetimibe intervention: A randomized controlled open-label trial. Front Pharmacol. (2021) 12:732541. doi: 10.3389/fphar.2021.732541
60. Jin J, Wang J, Cheng R, Ren Y, Miao Z, Luo Y, et al. Orlistat and ezetimibe could differently alleviate the high-fat diet-induced obesity phenotype by modulating the gut microbiota. Front Microbiol. (2022) 13:908327. doi: 10.3389/fmicb.2022.908327
61. Wang L, Li P, Tang Z, Yan X, Feng B. Structural modulation of the gut microbiota and the relationship with body weight: compared evaluation of liraglutide and saxagliptin treatment. Sci Rep. (2016) 6:33251. doi: 10.1038/srep33251
62. Zhao L, Qiu Y, Zhang P, Wu X, Zhao Z, Deng X, et al. Gut microbiota mediates positive effects of liraglutide on dyslipidemia in mice fed a high-fat diet. Front Nutr. (2022) 9:1048693. doi: 10.3389/fnut.2022.1048693
63. Shang J, Liu F, Zhang B, Dong K, Lu M, Jiang R, et al. Liraglutide-induced structural modulation of the gut microbiota in patients with type 2 diabetes mellitus. PeerJ. (2021) 9:e11128. doi: 10.7717/peerj.11128
64. Tsai CY, Lu HC, Chou YH, Liu PY, Chen HY, Huang MC, et al. Gut microbial signatures for glycemic responses of GLP-1 receptor agonists in type 2 diabetic patients: A pilot study. Front Endocrinol (Lausanne). (2021) 12:814770. doi: 10.3389/fendo.2021.814770
65. Mueller NT, Differding MK, Zhang M, Maruthur NM, Juraschek SP, Miller ER 3rd, et al. Metformin affects gut microbiome composition and function and circulating short-chain fatty acids: A randomized trial. Diabetes Care. (2021) 44:1462–71. doi: 10.2337/dc20-2257
66. Forslund K, Hildebrand F, Nielsen T, Falony G, Le Chatelier E, Sunagawa S, et al. Corrigendum: Disentangling type 2 diabetes and metformin treatment signatures in the human gut microbiota. Nature. (2017) 545:116. doi: 10.1038/nature22318
67. Niu X, Lu P, Huang L, Sun Y, Jin M, Liu J, et al. The effect of metformin combined with liraglutide on gut microbiota of Chinese patients with type 2 diabetes. Int Microbiol. (2024) 27:265–76. doi: 10.1007/s10123-023-00380-y
68. de la Cuesta-Zuluaga J, Mueller NT, Corrales-Agudelo V, Velasquez-Mejia EP, Carmona JA, Abad JM, et al. Metformin is associated with higher relative abundance of mucin-degrading akkermansia muciniphila and several short-chain fatty acid-producing microbiota in the gut. Diabetes Care. (2017) 40:54–62. doi: 10.2337/dc16-1324
69. Shin NR, Lee JC, Lee HY, Kim MS, Whon TW, Lee MS, et al. An increase in the Akkermansia spp. population induced by metformin treatment improves glucose homeostasis in diet-induced obese mice. Gut. (2014) 63:727–35. doi: 10.1136/gutjnl-2012-303839
70. Gopinath B, Harris IA, Nicholas M, Casey P, Blyth F, Maher CG, et al. A comparison of health outcomes in older versus younger adults following a road traffic crash injury: a cohort study. PloS One. (2015) 10:e0122732. doi: 10.1371/journal.pone.0122732
71. Lee H, Ko G. Effect of metformin on metabolic improvement and gut microbiota. Appl Environ Microbiol. (2014) 80:5935–43. doi: 10.1128/AEM.01357-14
72. Sun L, Xie C, Wang G, Wu Y, Wu Q, Wang X, et al. Gut microbiota and intestinal FXR mediate the clinical benefits of metformin. Nat Med. (2018) 24:1919–29. doi: 10.1038/s41591-018-0222-4
73. Su B, Liu H, Li J, Sunli Y, Liu B, Liu D, et al. Acarbose treatment affects the serum levels of inflammatory cytokines and the gut content of bifidobacteria in Chinese patients with type 2 diabetes mellitus. J Diabetes. (2015) 7:729–39. doi: 10.1111/1753-0407.12232
74. Gu Y, Wang X, Li J, Zhang Y, Zhong H, Liu R, et al. Analyses of gut microbiota and plasma bile acids enable stratification of patients for antidiabetic treatment. Nat Commun. (2017) 8:1785. doi: 10.1038/s41467-017-01682-2
75. Takewaki F, Nakajima H, Takewaki D, Hashimoto Y, Majima S, Okada H, et al. Habitual dietary intake affects the altered pattern of gut microbiome by acarbose in patients with type 2 diabetes. Nutrients. (2021) 13(6):2107. doi: 10.3390/nu13062107
76. Yan X, Feng B, Li P, Tang Z, Wang L. Microflora disturbance during progression of glucose intolerance and effect of sitagliptin: an animal study. J Diabetes Res. (2016) 2016:2093171. doi: 10.1155/2016/2093171
77. Lee DM, Battson ML, Jarrell DK, Hou S, Ecton KE, Weir TL, et al. SGLT2 inhibition via dapagliflozin improves generalized vascular dysfunction and alters the gut microbiota in type 2 diabetic mice. Cardiovasc Diabetol. (2018) 17:62. doi: 10.1186/s12933-018-0708-x
78. Wang X, Wang Z, Liu D, Jiang H, Cai C, Li G, et al. IDDF2021-ABS-0198 Canagliflozin alleviates diabetic cardiovascular disease via lipid lowering, mitochondrial homeostasis, and gut microbiota regulation. Gut. (2021) 70:A58–A9. doi: 10.1136/gutjnl-2021-IDDF.56
79. Sjostrom L, Rissanen A, Andersen T, Boldrin M, Golay A, Koppeschaar HP, et al. Randomised placebo-controlled trial of orlistat for weight loss and prevention of weight regain in obese patients. Eur Multicentre Orlistat Study Group Lancet. (1998) 352:167–72. doi: 10.1016/S0140-6736(97)11509-4
80. Morales P, Fujio S, Navarrete P, Ugalde JA, Magne F, Carrasco-Pozo C, et al. Impact of dietary lipids on colonic function and microbiota: an experimental approach involving orlistat-induced fat malabsorption in human volunteers. Clin Transl Gastroenterol. (2016) 7:e161. doi: 10.1038/ctg.2016.20
81. MacDonald PE, El-Kholy W, Riedel MJ, Salapatek AM, Light PE, Wheeler MB. The multiple actions of GLP-1 on the process of glucose-stimulated insulin secretion. Diabetes. (2002) 51 Suppl 3:S434–42. doi: 10.2337/diabetes.51.2007.S434
82. Zhao L, Chen Y, Xia F, Abudukerimu B, Zhang W, Guo Y, et al. A glucagon-like peptide-1 receptor agonist lowers weight by modulating the structure of gut microbiota. Front Endocrinol (Lausanne). (2018) 9:233. doi: 10.3389/fendo.2018.00233
83. Zhang Q, Xiao X, Zheng J, Li M, Yu M, Ping F, et al. Featured article: Structure moderation of gut microbiota in liraglutide-treated diabetic male rats. Exp Biol Med (Maywood). (2018) 243:34–44. doi: 10.1177/1535370217743765
84. Moreira GV, Azevedo FF, Ribeiro LM, Santos A, Guadagnini D, Gama P, et al. Liraglutide modulates gut microbiota and reduces NAFLD in obese mice. J Nutr Biochem. (2018) 62:143–54. doi: 10.1016/j.jnutbio.2018.07.009
85. Zhang E, Jin L, Wang Y, Tu J, Zheng R, Ding L, et al. Intestinal AMPK modulation of microbiota mediates crosstalk with brown fat to control thermogenesis. Nat Commun. (2022) 13:1135. doi: 10.1038/s41467-022-28743-5
86. Wu H, Esteve E, Tremaroli V, Khan MT, Caesar R, Manneras-Holm L, et al. Metformin alters the gut microbiome of individuals with treatment-naive type 2 diabetes, contributing to the therapeutic effects of the drug. Nat Med. (2017) 23:850–8. doi: 10.1038/nm.4345
87. Wehmeier UF, Piepersberg W. Biotechnology and molecular biology of the alpha-glucosidase inhibitor acarbose. Appl Microbiol Biotechnol. (2004) 63:613–25. doi: 10.1007/s00253-003-1477-2
88. Kyriachenko Y, Falalyeyeva T, Korotkyi O, Molochek N, Kobyliak N. Crosstalk between gut microbiota and antidiabetic drug action. World J Diabetes. (2019) 10:154–68. doi: 10.4239/wjd.v10.i3.154
89. Eisenberg D, Shikora SA, Aarts E, Aminian A, Angrisani L, Cohen RV, et al. 2022 American society of metabolic and bariatric surgery (ASMBS) and international federation for the surgery of obesity and metabolic disorders (IFSO) indications for metabolic and bariatric surgery. Obes Surg. (2022). 18(12):1345–56. doi: 10.1016/j.soard.2022.08.013
90. Surgery ASfMaB. Bariatric Surgery Procedures (2022). Available online at: https://asmbs.org/patients/bariatric-surgery-procedures.
91. Surgery ASfMaB. Who is a Candidate for Bariatric Surgery? (2022). Available online at: https://asmbs.org/patients/who-is-a-candidate-for-bariatric-surgery.
92. Armstrong SC, Bolling CF, Michalsky MP, Reichard KW, Section On Obesity SOS. Pediatric metabolic and bariatric surgery: evidence, barriers, and best practices. Pediatrics. (2019) 144(6):e20193223. doi: 10.1542/peds.2019-3223
93. Pratt JSA, Browne A, Browne NT, Bruzoni M, Cohen M, Desai A, et al. ASMBS pediatric metabolic and bariatric surgery guidelines, 2018. Surg Obes Relat Dis. (2018) 14:882–901. doi: 10.1016/j.soard.2018.03.019
94. Quercia I, Dutia R, Kotler DP, Belsley S, Laferrere B. Gastrointestinal changes after bariatric surgery. Diabetes Metab. (2014) 40:87–94. doi: 10.1016/j.diabet.2013.11.003
95. Tremaroli V, Karlsson F, Werling M, Stahlman M, Kovatcheva-Datchary P, Olbers T, et al. Roux-en-Y gastric bypass and vertical banded gastroplasty induce long-term changes on the human gut microbiome contributing to fat mass regulation. Cell Metab. (2015) 22:228–38. doi: 10.1016/j.cmet.2015.07.009
96. Zhang H, DiBaise JK, Zuccolo A, Kudrna D, Braidotti M, Yu Y, et al. Human gut microbiota in obesity and after gastric bypass. Proc Natl Acad Sci U S A. (2009) 106:2365–70. doi: 10.1073/pnas.0812600106
97. Furet JP, Kong LC, Tap J, Poitou C, Basdevant A, Bouillot JL, et al. Differential adaptation of human gut microbiota to bariatric surgery-induced weight loss: links with metabolic and low-grade inflammation markers. Diabetes. (2010) 59:3049–57. doi: 10.2337/db10-0253
98. le Roux CW, Bueter M, Theis N, Werling M, Ashrafian H, Lowenstein C, et al. Gastric bypass reduces fat intake and preference. Am J Physiol Regul Integr Comp Physiol. (2011) 301:R1057–66. doi: 10.1152/ajpregu.00139.2011
99. Miras AD, le Roux CW. Bariatric surgery and taste: novel mechanisms of weight loss. Curr Opin Gastroenterol. (2010) 26:140–5. doi: 10.1097/MOG.0b013e328333e94a
100. Ley RE, Turnbaugh PJ, Klein S, Gordon JI. Microbial ecology: human gut microbes associated with obesity. Nature. (2006) 444:1022–3. doi: 10.1038/4441022a
101. Smith CD, Herkes SB, Behrns KE, Fairbanks VF, Kelly KA, Sarr MG. Gastric acid secretion and vitamin B12 absorption after vertical Roux-en-Y gastric bypass for morbid obesity. Ann Surg. (1993) 218:91–6. doi: 10.1097/00000658-199307000-00014
102. Ishida RK, Faintuch J, Paula AM, Risttori CA, Silva SN, Gomes ES, et al. Microbial flora of the stomach after gastric bypass for morbid obesity. Obes Surg. (2007) 17:752–8. doi: 10.1007/s11695-007-9139-6
103. Walker AW, Duncan SH, McWilliam Leitch EC, Child MW, Flint HJ. pH and peptide supply can radically alter bacterial populations and short-chain fatty acid ratios within microbial communities from the human colon. Appl Environ Microbiol. (2005) 71:3692–700. doi: 10.1128/AEM.71.7.3692-3700.2005
104. El Oufir L, Flourie B, Bruley des Varannes S, Barry JL, Cloarec D, Bornet F, et al. Relations between transit time, fermentation products, and hydrogen consuming flora in healthy humans. Gut. (1996) 38:870–7. doi: 10.1136/gut.38.6.870
105. Swann JR, Want EJ, Geier FM, Spagou K, Wilson ID, Sidaway JE, et al. Systemic gut microbial modulation of bile acid metabolism in host tissue compartments. Proc Natl Acad Sci U S A. (2011) 108 Suppl 1:4523–30. doi: 10.1073/pnas.1006734107
106. Islam KB, Fukiya S, Hagio M, Fujii N, Ishizuka S, Ooka T, et al. Bile acid is a host factor that regulates the composition of the cecal microbiota in rats. Gastroenterology. (2011) 141:1773–81. doi: 10.1053/j.gastro.2011.07.046
107. Antonopoulos DA, Huse SM, Morrison HG, Schmidt TM, Sogin ML, Young VB. Reproducible community dynamics of the gastrointestinal microbiota following antibiotic perturbation. Infect Immun. (2009) 77:2367–75. doi: 10.1128/IAI.01520-08
108. Claesson MJ, Cusack S, O'Sullivan O, Greene-Diniz R, de Weerd H, Flannery E, et al. Composition, variability, and temporal stability of the intestinal microbiota of the elderly. Proc Natl Acad Sci U S A. (2011) 108 Suppl 1:4586–91. doi: 10.1073/pnas.1000097107
109. Laferrere B, Heshka S, Wang K, Khan Y, McGinty J, Teixeira J, et al. Incretin levels and effect are markedly enhanced 1 month after Roux-en-Y gastric bypass surgery in obese patients with type 2 diabetes. Diabetes Care. (2007) 30:1709–16. doi: 10.2337/dc06-1549
110. le Roux CW, Welbourn R, Werling M, Osborne A, Kokkinos A, Laurenius A, et al. Gut hormones as mediators of appetite and weight loss after Roux-en-Y gastric bypass. Ann Surg. (2007) 246:780–5. doi: 10.1097/SLA.0b013e3180caa3e3
111. Lee CJ, Florea L, Sears CL, Maruthur N, Potter JJ, Schweitzer M, et al. Changes in gut microbiome after bariatric surgery versus medical weight loss in a pilot randomized trial. Obes Surg. (2019) 29:3239–45. doi: 10.1007/s11695-019-03976-4
112. Sanmiguel CP, Jacobs J, Gupta A, Ju T, Stains J, Coveleskie K, et al. Surgically induced changes in gut microbiome and hedonic eating as related to weight loss: preliminary findings in obese women undergoing bariatric surgery. Psychosom Med. (2017) 79:880–7. doi: 10.1097/PSY.0000000000000494
113. Medina DA, Pedreros JP, Turiel D, Quezada N, Pimentel F, Escalona A, et al. Distinct patterns in the gut microbiota after surgical or medical therapy in obese patients. PeerJ. (2017) 5:e3443. doi: 10.7717/peerj.3443
114. Damms-MaChado A, Mitra S, Schollenberger AE, Kramer KM, Meile T, Konigsrainer A, et al. Effects of surgical and dietary weight loss therapy for obesity on gut microbiota composition and nutrient absorption. BioMed Res Int. (2015) 2015:806248. doi: 10.1155/2015/806248
115. Murphy R, Tsai P, Jullig M, Liu A, Plank L, Booth M. Differential changes in gut microbiota after gastric bypass and sleeve gastrectomy bariatric surgery vary according to diabetes remission. Obes Surg. (2017) 27:917–25. doi: 10.1007/s11695-016-2399-2
116. Anhe FF, Zlitni S, Zhang SY, Choi BS, Chen CY, Foley KP, et al. Human gut microbiota after bariatric surgery alters intestinal morphology and glucose absorption in mice independently of obesity. Gut. (2023) 72:460–71. doi: 10.1136/gutjnl-2022-328185
117. Yadav J, Liang T, Qin T, Nathan N, Schwenger KJP, Pickel L, et al. Gut microbiome modified by bariatric surgery improves insulin sensitivity and correlates with increased brown fat activity and energy expenditure. Cell Rep Med. (2023) 4:101051. doi: 10.1016/j.xcrm.2023.101051
118. Palleja A, Kashani A, Allin KH, Nielsen T, Zhang C, Li Y, et al. Roux-en-Y gastric bypass surgery of morbidly obese patients induces swift and persistent changes of the individual gut microbiota. Genome Med. (2016) 8:67. doi: 10.1186/s13073-016-0312-1
119. Kong LC, Tap J, Aron-Wisnewsky J, Pelloux V, Basdevant A, Bouillot JL, et al. Gut microbiota after gastric bypass in human obesity: increased richness and associations of bacterial genera with adipose tissue genes. Am J Clin Nutr. (2013) 98:16–24. doi: 10.3945/ajcn.113.058743
120. Graessler J, Qin Y, Zhong H, Zhang J, Licinio J, Wong ML, et al. Metagenomic sequencing of the human gut microbiome before and after bariatric surgery in obese patients with type 2 diabetes: correlation with inflammatory and metabolic parameters. Pharmacogenomics J. (2013) 13:514–22. doi: 10.1038/tpj.2012.43
121. Mukorako P, Lopez C, Baraboi ED, Roy MC, Plamondon J, Lemoine N, et al. Alterations of gut microbiota after biliopancreatic diversion with duodenal switch in wistar rats. Obes Surg. (2019) 29:2831–42. doi: 10.1007/s11695-019-03911-7
122. Mukorako P, Lemoine N, Biertho L, Lebel S, Roy MC, Plamondon J, et al. Consistent gut bacterial and short-chain fatty acid signatures in hypoabsorptive bariatric surgeries correlate with metabolic benefits in rats. Int J Obes (Lond). (2022) 46:297–306. doi: 10.1038/s41366-021-00973-5
123. Aron-Wisnewsky J, Prifti E, Belda E, Ichou F, Kayser BD, Dao MC, et al. Major microbiota dysbiosis in severe obesity: fate after bariatric surgery. Gut. (2019) 68:70–82. doi: 10.1136/gutjnl-2018-316103
124. Wang Y, Guo X, Lu X, Mattar S, Kassab G. Mechanisms of weight loss after sleeve gastrectomy and adjustable gastric banding: far more than just restriction. Obes (Silver Spring). (2019) 27:1776–83. doi: 10.1002/oby.22623
125. Ilhan ZE, DiBaise JK, Isern NG, Hoyt DW, Marcus AK, Kang DW, et al. Distinctive microbiomes and metabolites linked with weight loss after gastric bypass, but not gastric banding. ISME J. (2017) 11:2047–58. doi: 10.1038/ismej.2017.71
126. Qin J, Li Y, Cai Z, Li S, Zhu J, Zhang F, et al. A metagenome-wide association study of gut microbiota in type 2 diabetes. Nature. (2012) 490:55–60. doi: 10.1038/nature11450
127. Karlsson FH, Tremaroli V, Nookaew I, Bergstrom G, Behre CJ, Fagerberg B, et al. Gut metagenome in European women with normal, impaired and diabetic glucose control. Nature. (2013) 498:99–103. doi: 10.1038/nature12198
128. Ryan KK, Tremaroli V, Clemmensen C, Kovatcheva-Datchary P, Myronovych A, Karns R, et al. FXR is a molecular target for the effects of vertical sleeve gastrectomy. Nature. (2014) 509:183–8. doi: 10.1038/nature13135
129. Steenackers N, Brouwers E, Mertens A, Van Cleynenbreugel S, Lannoo M, Flamaing J, et al. Late complications of biliopancreatic diversion in an older patient: a case report. BMC Geriatr. (2021) 21:631. doi: 10.1186/s12877-021-02578-z
130. Evans AS. Causation and disease: the Henle-Koch postulates revisited. Yale J Biol Med. (1976) 49:175–95.
131. Belzer C, de Vos WM. Microbes inside–from diversity to function: the case of Akkermansia. ISME J. (2012) 6:1449–58. doi: 10.1038/ismej.2012.6
132. Derrien M, Collado MC, Ben-Amor K, Salminen S, de Vos WM. The Mucin degrader Akkermansia muciniphila is an abundant resident of the human intestinal tract. Appl Environ Microbiol. (2008) 74:1646–8. doi: 10.1128/AEM.01226-07
133. Dao MC, Everard A, Aron-Wisnewsky J, Sokolovska N, Prifti E, Verger EO, et al. Akkermansia muciniphila and improved metabolic health during a dietary intervention in obesity: relationship with gut microbiome richness and ecology. Gut. (2016) 65:426–36. doi: 10.1136/gutjnl-2014-308778
134. Anhe FF, Roy D, Pilon G, Dudonne S, Matamoros S, Varin TV, et al. A polyphenol-rich cranberry extract protects from diet-induced obesity, insulin resistance and intestinal inflammation in association with increased Akkermansia spp. population in the gut microbiota of mice. Gut. (2015) 64:872–83. doi: 10.1136/gutjnl-2014-307142
135. Depommier C, Everard A, Druart C, Plovier H, Van Hul M, Vieira-Silva S, et al. Supplementation with Akkermansia muciniphila in overweight and obese human volunteers: a proof-of-concept exploratory study. Nat Med. (2019) 25:1096–103. doi: 10.1038/s41591-019-0495-2
136. Everard A, Belzer C, Geurts L, Ouwerkerk JP, Druart C, Bindels LB, et al. Cross-talk between Akkermansia muciniphila and intestinal epithelium controls diet-induced obesity. Proc Natl Acad Sci U S A. (2013) 110:9066–71. doi: 10.1073/pnas.1219451110
137. Remely M, Hippe B, Zanner J, Aumueller E, Brath H, Haslberger AG. Gut Microbiota of Obese, Type 2 Diabetic Individuals is Enriched in Faecalibacterium prausnitzii, Akkermansia muciniphila and Peptostreptococcus anaerobius after Weight Loss. Endocr Metab Immune Disord Drug Targets. (2016) 16:99–106. doi: 10.2174/1871530316666160831093813
138. Reunanen J, Kainulainen V, Huuskonen L, Ottman N, Belzer C, Huhtinen H, et al. Akkermansia muciniphila adheres to enterocytes and strengthens the integrity of the epithelial cell layer. Appl Environ Microbiol. (2015) 81:3655–62. doi: 10.1128/AEM.04050-14
139. Chelakkot C, Choi Y, Kim DK, Park HT, Ghim J, Kwon Y, et al. Akkermansia muciniphila-derived extracellular vesicles influence gut permeability through the regulation of tight junctions. Exp Mol Med. (2018) 50:e450. doi: 10.1038/emm.2017.282
140. Ottman N, Reunanen J, Meijerink M, Pietila TE, Kainulainen V, Klievink J, et al. Pili-like proteins of Akkermansia muciniphila modulate host immune responses and gut barrier function. PloS One. (2017) 12:e0173004. doi: 10.1371/journal.pone.0173004
141. Arumugam M, Raes J, Pelletier E, Le Paslier D, Yamada T, Mende DR, et al. Enterotypes of the human gut microbiome. Nature. (2011) 473:174–80. doi: 10.1038/nature09944
142. Andoh A, Nishida A, Takahashi K, Inatomi O, Imaeda H, Bamba S, et al. Comparison of the gut microbial community between obese and lean peoples using 16S gene sequencing in a Japanese population. J Clin Biochem Nutr. (2016) 59:65–70. doi: 10.3164/jcbn.15-152
143. Zhang X, Shen D, Fang Z, Jie Z, Qiu X, Zhang C, et al. Human gut microbiota changes reveal the progression of glucose intolerance. PloS One. (2013) 8:e71108. doi: 10.1371/journal.pone.0071108
144. Ganesan K, Chung SK, Vanamala J, Xu B. Causal relationship between diet-induced gut microbiota changes and diabetes: A novel strategy to transplant faecalibacterium prausnitzii in preventing diabetes. Int J Mol Sci. (2018) 19(12):3720. doi: 10.3390/ijms19123720
145. Munukka E, Rintala A, Toivonen R, Nylund M, Yang B, Takanen A, et al. Faecalibacterium prausnitzii treatment improves hepatic health and reduces adipose tissue inflammation in high-fat fed mice. ISME J. (2017) 11:1667–79. doi: 10.1038/ismej.2017.24
146. Hamer HM, Jonkers D, Venema K, Vanhoutvin S, Troost FJ, Brummer RJ. Review article: the role of butyrate on colonic function. Aliment Pharmacol Ther. (2008) 27:104–19. doi: 10.1111/j.1365-2036.2007.03562.x
147. Hamer HM, Jonkers DM, Bast A, Vanhoutvin SA, Fischer MA, Kodde A, et al. Butyrate modulates oxidative stress in the colonic mucosa of healthy humans. Clin Nutr. (2009) 28:88–93. doi: 10.1016/j.clnu.2008.11.002
148. Tenaillon O, Skurnik D, Picard B, Denamur E. The population genetics of commensal Escherichia coli. Nat Rev Microbiol. (2010) 8:207–17. doi: 10.1038/nrmicro2298
149. Hartstra AV, Bouter KE, Backhed F, Nieuwdorp M. Insights into the role of the microbiome in obesity and type 2 diabetes. Diabetes Care. (2015) 38:159–65. doi: 10.2337/dc14-0769
150. Ju T, Bourrie BCT, Forgie AJ, Pepin DM, Tollenaar S, Sergi CM, et al. The gut commensal escherichia coli aggravates high-fat-diet-induced obesity and insulin resistance in mice. Appl Environ Microbiol. (2023) 89:e0162822. doi: 10.1128/aem.01628-22
151. Le KA, Li Y, Xu X, Yang W, Liu T, Zhao X, et al. Alterations in fecal Lactobacillus and Bifidobacterium species in type 2 diabetic patients in Southern China population. Front Physiol. (2012) 3:496.
152. Bradley CA. Gut microbiota: Trust your gut - metformin and diabetes. Nat Rev Endocrinol. (2017) 13:440. doi: 10.1038/nrendo.2017.72
153. Wu J, Zhang PB, Ren ZQ, Zhou F, Hu HH, Zhang H, et al. Changes of serum lipopolysaccharide, inflammatory factors, and cecal microbiota in obese rats with type 2 diabetes induced by Roux-en-Y gastric bypass. Nutrition. (2019) 67-68:110565. doi: 10.1016/j.nut.2019.110565
154. Chen J, Wang R, Li XF, Wang RL. Bifidobacterium adolescentis supplementation ameliorates visceral fat accumulation and insulin sensitivity in an experimental model of the metabolic syndrome. Br J Nutr. (2012) 107:1429–34. doi: 10.1017/S0007114511004491
155. Qian X, Si Q, Lin G, Zhu M, Lu J, Zhang H, et al. Bifidobacterium adolescentis is effective in relieving type 2 diabetes and may be related to its dominant core genome and gut microbiota modulation capacity. Nutrients. (2022) 14(12):2479. doi: 10.3390/nu14122479
156. Pfeiffer JK, Virgin HW. Viral immunity. Transkingdom control of viral infection and immunity in the mammalian intestine. Science. (2016) 351(6270):10.1126. doi: 10.1126/science.aad5872
157. Zhang F, Aschenbrenner D, Yoo JY, Zuo T. The gut mycobiome in health, disease, and clinical applications in association with the gut bacterial microbiome assembly. Lancet Microbe. (2022) 3:e969–e83. doi: 10.1016/S2666-5247(22)00203-8
158. Sieber JR, McInerney MJ, Gunsalus RP. Genomic insights into syntrophy: the paradigm for anaerobic metabolic cooperation. Annu Rev Microbiol. (2012) 66:429–52. doi: 10.1146/annurev-micro-090110-102844
Keywords: obesity, type 2 diabetes, gut microbiota, bariatric surgery, pharmacotherapy
Citation: Zhang L, Wang P, Huang J, Xing Y, Wong FS, Suo J and Wen L (2024) Gut microbiota and therapy for obesity and type 2 diabetes. Front. Endocrinol. 15:1333778. doi: 10.3389/fendo.2024.1333778
Received: 06 November 2023; Accepted: 06 March 2024;
Published: 26 March 2024.
Edited by:
Maria Gazouli, National and Kapodistrian University of Athens, GreeceReviewed by:
P. Trayhurn, University of Liverpool, United KingdomWendong Huang, City of Hope, United States
Ludovica Verde, University of Naples Federico II, Italy
Copyright © 2024 Zhang, Wang, Huang, Xing, Wong, Suo and Wen. This is an open-access article distributed under the terms of the Creative Commons Attribution License (CC BY). The use, distribution or reproduction in other forums is permitted, provided the original author(s) and the copyright owner(s) are credited and that the original publication in this journal is cited, in accordance with accepted academic practice. No use, distribution or reproduction is permitted which does not comply with these terms.
*Correspondence: Li Wen, li.wen@yale.edu; Jian Suo, suojian@jlu.edu.cn
†These authors have contributed equally to this work and share first authorship