- 1Department of Plastic and Cosmetic Surgery, Nanfang Hospital, Southern Medical University, Guangzhou, Guangdong, China
- 2The Second School of Clinical Medicine, Southern Medical University, Guangzhou, Guangdong, China
Obesity is a chronic disease that affects the energy balance of the whole body. In addition to increasing fat mass, tissue fibrosis occurred in white adipose tissue in obese condition. Fibrosis is the over-activation of fibroblasts leading to excessive accumulation of extracellular matrix, which could be caused by various factors, including the status of adipocytes. The morphology of adipocytes responds rapidly and dynamically to nutrient fluctuations. Adaptive hypertrophy of normal adipocytes protects peripheral organs from damage from lipotoxicity. However, the biological behavior of hypertrophic adipocytes in chronic obesity is abnormally altered. Adipocytes lead to fibrotic remodeling of the extracellular matrix by inducing unresolved chronic inflammation, persistent hypoxia, and increasing myofibroblast numbers. Moreover, adipocyte-induced fibrosis not only restricts the flexible expansion and contraction of adipose tissue but also initiates the development of various diseases through cellular autonomic and paracrine effects. Regarding anti-fibrotic therapy, dysregulated intracellular signaling and epigenetic changes represent potential candidate targets. Thus, modulation of adipocytes may provide potential therapeutic avenues for reversing pathological fibrosis in adipose tissue and achieving the anti-obesity purpose.
1 Introduction
In recent years, the growing prevalence of obesity has become a major public health problem worldwide. As the body’s largest energy store, adipose tissue plays an important role in controlling energy balance throughout the body. Benefiting from the characteristic loose ECM structure, mature adipocytes can support fatty acid release or storage by resizing from small cells with a diameter of 20–70 μm to large cells with a diameter of 300 μm (1). At the same time, adipocytes can secrete many lipid and protein factors through endocrine action, which have a profound impact on the metabolism of other tissues. Recent studies have shown that adipocytes exhibit remarkable plasticity during periods of caloric excess, driving the development of extracellular matrix remodeling. In the early stages of obesity, adipocytes in subcutaneous adipose tissue adapt to increased energy supply by increasing intracellular lipid accumulation and hypertrophy. However, the over-expanded adipocytes in chronic obesity are characterized by up-regulation of inflammatory activity, secretion dysfunction, and abnormal differentiation, inducing a microenvironment conducive to fibrosis (2). Fibrosis is a chronic process of ECM excessive accumulation characterized by hyperactivation of myofibroblasts. The highly rigid ECM in fibrotic adipose tissue induces apoptosis and lipid leakage in normal adipocytes through shear stress, thereby triggering common metabolic syndromes such as dyslipidemia and insulin resistance (3). In addition, elevated concentrations of pro-fibrotic adipokines in circulation have been shown to exert a negative influence on other tissues and organs. The persistent fibrotic response detected in chronic diseases such as cirrhosis, systemic scleroderma, and heart failure is closely related to uncontrolled adipocytes. The medical burden caused by fibrotic diseases is enormous. It is estimated that up to 45% of deaths in developed countries can be attributed to organ failure due to fibrosis-related diseases (4, 5).
The synergistic involvement of myofibroblasts in tissue fibrosis with different types of cells, such as inflammatory immune cells, has been extensively explored. However, little attention has been paid to the contribution of adipocytes in extracellular matrix remodeling. Here, we update our current understanding of adipose tissue fibrotic remodeling in the obese state, focusing on the role of adipocytes. A better understanding of the autocrine, paracrine and endocrine communication mechanisms of adipocytes may provide further insights into the pathobiology of obesity-related fibrotic diseases. Finally, we delve into the challenges and prospects encountered in this field, aiming to stimulate significant research in related domains and provide novel insights for the prevention and treatment strategies of fibrosis-related diseases.
2 Adipocytes regulate adipose tissue fibrosis
2.1 Adipocytes promote fibrosis by releasing free fatty acids
In obesity, the inherent ability of adipose tissue to store and sense nutrients is compromised, resulting in free fatty acids (FFA) spilling into the periphery and circulation (Figure 1). Miller et al. explored the effect of free fatty acids on adipose tissue function by developing a unique dietary regimen and found out that the peroxidized n-3-enriched diet led to lipotoxicity of white adipose tissue, as evidenced by increased fibrosis, lipofuscin, and reduced anti-inflammatory markers (6). Mechanisms of lipotoxicity involve various cellular processes, including mitochondrial damage, and activation of intracellular inflammation-related signaling pathways (7). Furthermore, signaling pathways related to fatty acid-mediated adipose tissue fibrosis are also particularly enriched. Targeted stimulation of ERK signaling in hypertrophic adipocytes increases free fatty acid production and release from lipolysis, which further upregulates inflammatory pathways in adipocytes (8). Obese adults with low fatty acid prevalence have less adipose tissue fibrosis compared with high fatty acid prevalence, which is associated with lower activation of the SAPK/JNK pathway (9). In addition, the bile acid-activated nuclear receptor, FXR has been implicated in the control of and may be a key determinant of adipocyte size and adipose tissue function under metabolic stress. Sustained FXR expression in adipose tissue limits its storage capacity, leading to elevated plasma-free fatty acids, ultimately promoting adipose tissue fibrosis (10). Besides, lipotoxic cell damage also mediates fibrotic responses in various organs such as the heart and kidneys (11, 12).
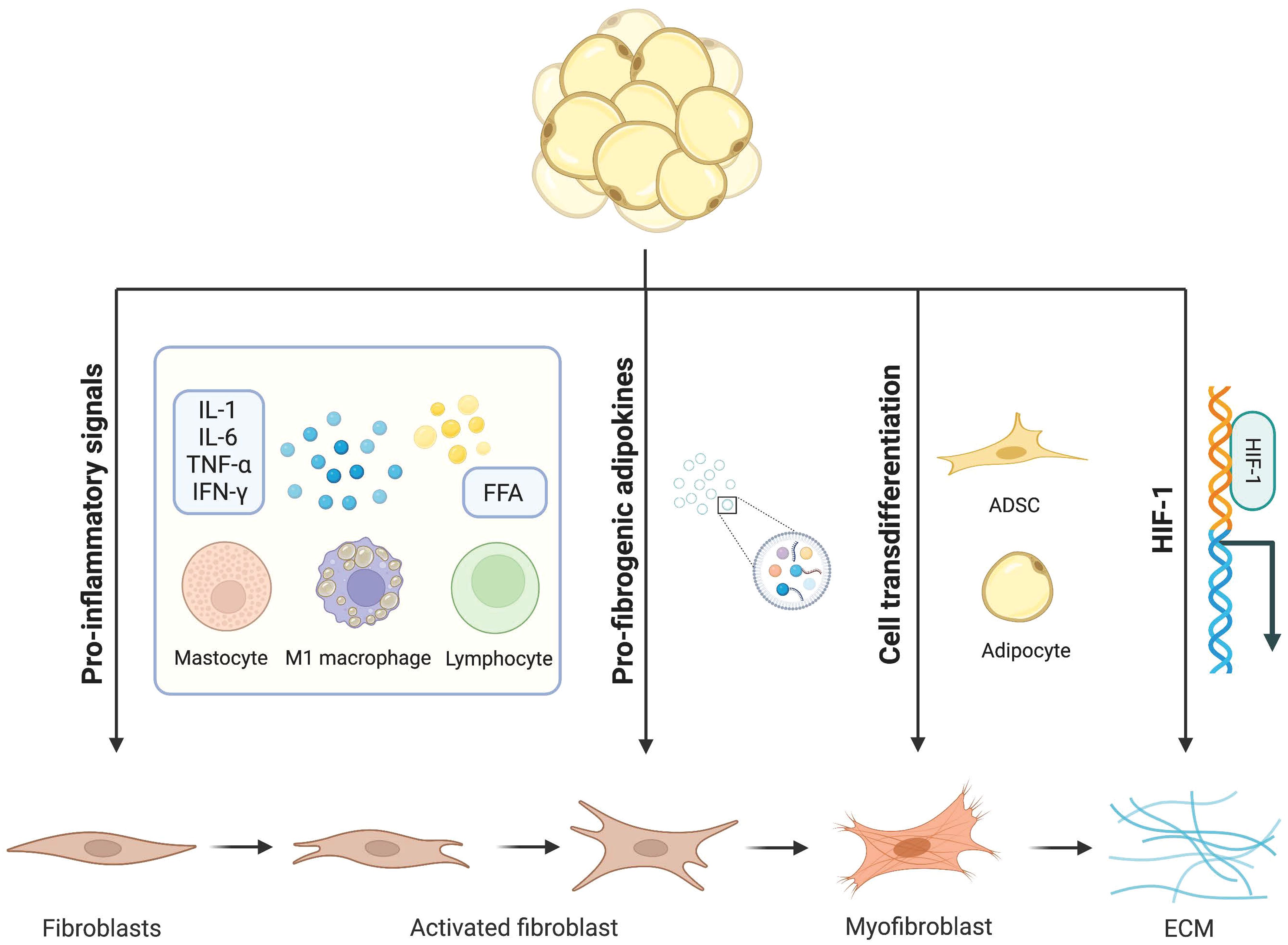
Figure 1 Mechanisms of adipocytes in adipose tissue fibrosis. Dysfunctional adipocytes secrete proinflammatory cytokines and free fatty acids, which activate immune inflammatory cells and amplify the inflammatory cascade. In addition, imbalanced adipokines and hypoxia-inducible factors further promote the development of adipose tissue fibrosis. In this condition, mature adipocytes and adipose stem cells transdifferentiate into myofibroblasts. Activated myofibroblasts aggregate and a large amount of extracellular matrix is deposited in adipose tissue.
2.2 Adipocytes contribute to fibrosis via dysregulated secretion of adipokines
Apart from its crucial involvement in lipid metabolism, adipose tissue serves as an essential endocrine organ by releasing a range of specific cytokines and hormones referred to as adiponectins (13). Adipokines interact with receptors on target cells, triggering intracellular signaling pathways and inducing various effects. In cases of obesity-induced disrupted fibrotic adipose tissue, there is an overproduction of dysregulated adipokines that often display proinflammatory and profibrotic characteristics.
2.2.1 Adiponectin
Adiponectin, one of the most abundant circulating adipokines, is a protective protein highly expressed in adipocytes (14, 15). Activation of adiponectin receptors has been shown to exert potent anti-inflammatory and anti-fibrotic effects in nonalcoholic steatohepatitis (NASH) models (16). In vitro studies have demonstrated that adiponectin can inhibit fibroblast activation induced by TGF-β, LPS, and Wnt signaling pathways, as well as downregulate collagen and α-SMA gene expression (17, 18). Therefore, adiponectin can be identified as a negative regulator of tissue fibrosis. Unfortunately, obesity significantly reduces the secretion of adiponectin (19). In humans, plasma concentration of adiponectin is negatively correlated with body weight and BMI (20). Furthermore, exercise-induced mitigation of high-fat diet-induced hypertrophy in adipocytes and collagen deposition leads to a significant increase in adiponectin levels within the adipose tissue (21).
2.2.2 Proinflammatory cytokines
Low-grade chronic inflammation caused by adipocytes in obesity is thought to be a key factor in adipose tissue fibrosis. Although increased numbers of adipocytes are well tolerated for obesity, hypertrophy of their size is considered a deleterious process (22). When a certain threshold is reached, anabolic stress causes widespread molecular changes in fat cells. Dysfunctional adipocytes release more pro-inflammatory factors such as tumor necrosis factor-α (TNF-α), interleukin 6 (IL-6) and interleukin 1 (IL-1) (23). Among them, TNF-α and IL-6 are potent agonists of collagen synthesis. Overexpression of TNF-α induced macrophage infiltration and subsequent fibrosis in adipose tissues under the HFD regimen (24). Furthermore, TNF-α promoted the differentiation of mesenchymal stem cells into fibroblasts by activating NF-κB signaling (25). In a model of renal fibrosis, specific blockade of IL-6 signaling reduced the number of phosphorylated signal transducers and activators of transcription (p-STAT3)-activated fibroblasts and the deposition of extracellular matrix proteins (26). As for changes in inflammatory fatty acid metabolism, they indirectly modulate fibrosis by increasing lipotoxicity or altering cell fate (27).
2.2.3 Hypoxia-inducible factor
WAT is one of the most vascularized tissues in the body. Angiogenesis is the physiological process of forming new blood vessels based on existing blood vessels, which is essential for the maintenance of normal tissue physiological function and tissue remodeling. With further lipid accumulation, the density and function of intrinsic capillaries cannot support the massive expansion of adipose tissue and hypoxia ensues (28). On the one hand, hypoxia indirectly promotes fibrosis by activating the inflammatory response. The necrosis of fat cells caused by hypoxia is conducive to the infiltration of pro-inflammatory leukocytes. Studies have also shown that hypoxia can alter the adipose-derived mesenchymal stem cells (ADSCs) secretion profile of (METS) patients to release more pro-inflammatory factors and trigger a specific inflammatory state (29). On the other hand, both the stability and transcriptional activity of the hypoxia-inducible factor(HIF-1) were increased in adipocytes and further activated the pro-fibrotic transcriptional program under hypoxia (30). AT of transgenic ob/ob mice expressing HIF-1 exhibit increased fibrosis and upregulation of several ECM genes (31). HIF-1 promoted the accumulation of type I collagen in response to transforming growth factor-β (TGF-β) signaling by forming a transcriptional complex with small mothers against decapentaplegic 3 (Smad3) (32). At the same time, studies have also shown that activation of HIF-1 signaling can promote epithelial-mesenchymal transition (EMT), thereby exacerbating fibrotic damage (33). Moreover, selective inhibition of HIF-1 reduces adipose tissue inflammatory infiltration and tissue fibrosis in high-fat diet mice (34).
2.2.4 Visfatin
Visfatin is highly enriched in visceral adipose tissue, and its expression level in plasma increases with the development of obesity (35). Treatment of 3T3-L1 preadipocytes with visfatin resulted in up-regulation of adipose tissue fibrosis markers such as collagen type VI (Col6) (36). In addition, visfatin accelerated the development of liver fibrosis by increasing the expression of α-SMA, fibronectin, vimentin, and CTGF as well as inflammatory chemokines (37).
2.2.5 HMGB1
The novel adipokine high mobility group box 1 (HMGB1) is a 30 kDa DNA-binding protein, which is an important regulator of extracellular matrix remodeling. HMGB1 was present in a variety of cells but mainly secreted by adipocytes in visceral adipose tissue in obese patients (38). As an autocrine medium, HMGB1 in the extracellular environment has a certain pro-inflammatory effect on human adipocytes, which increased the expression of toll-like receptor 4 (TLR4) and toll-like receptor 2 (TLR2) as well as promoted the infiltration of M1-type macrophages in adipose tissue through the NF-κB signaling pathway (39). In addition, HMGB1 increased the release of active TGF-β1 in macrophages and fibroblasts to accelerate the transformation of fibroblasts into myofibroblasts (40). However, no direct link between HMGB1 and adipose tissue fibrosis has been reported, and further research is needed in the future.
2.2.6 Leptin
Leptin is a small peptide derived from adipose tissue. During normal physiology, leptin crossed the blood-brain barrier and inhibited the secretion of neuropeptide Y and agoutin to reduce hunger and increase energy expenditure. Leptin also bonds directly to leptin receptors and inhibited lipid synthesis in adipocytes (41). Obesity-related hyperleptinemia may be another cause of fibrosis. Leptin has been shown to induce proadipogenic and proinflammatory Signaling in adipocytes and ASCs by activating the mTOR pathway (42). The propelling role of leptin in adipose tissue fibrosis has been further confirmed. Leptin activated TGF-β and CTGF through the phosphatidylinositol 3 kinase (PI3K)- protein kinase B (AKT) signaling pathway to induce collagen deposition and promote fibrosis (43, 44). In addition, leptin-mediated aldosterone production may be a new mechanism for obesity-related fibrosis (45).
2.2.7 DPT
Dermatopontin (DPT), also known as tyrosine-rich acidic mechanism protein (TRAMP), can promote collagen accumulation to some extent. However, the secretion of DPT from visceral adipose tissue was greatly increased in the obese state, which exacerbated the remodeling of the extracellular matrix and the occurrence of chronic inflammation in adipose tissue (46).
2.2.8 Endotrophin
A novel adipocyte-derived factor endotrophic is the C-terminal cleavage product involving this C5 domain of recombinant collagen type-VIα3 (COL6α3). The expression of endotrophic is mainly derived from fully differentiated adipocytes and is hardly in ADSCs. Its levels are upregulated in obese individuals (47). Endorphins can act as powerful co-stimulators of existing pathological processes in “unhealthy” adipose tissue, triggering further enhancement of fibrosis and inflammation when challenged with a high-fat diet (HFD). Profibrotic and proinflammatory genes were significantly upregulated in the fat pad of Endotrophin-overexpressing transgenic mice. Furthermore, in a diet-induced obesity (DIO) model, transgenic mice efficiently induced a fibrotic microenvironment in adipose tissue by upregulating collagens, collagen cross-linking enzymes lipoxygenase (LOX), and additional ECM constituents. Besides, some of these fibrotic actions may be exerted through upregulation of the TGF-β pathway (48).
2.3 Adipocytes promote fibrosis by extracellular vesicle delivery
Extracellular vesicles (EVs) have been identified as a novel mode of communication between different cells and tissues. Intracellular signaling depends on the functional molecular composition of EVs, reflecting the physiological state of producing cells and tissues (49). Mature adipocytes secrete more EVs under the challenge of metabolic stress, elevating the level of circulating EVs (50, 51). Recently, an emerging role of adipocyte-derived EVs in obesity-related fibrotic comorbidities has been recognized. Obesity altered the secretion profile of functional miRNAs in adipocyte-derived EVs. MiRNAs involved in pro-inflammatory signaling and programmed cell death were up-regulated, and those involved in anti-fibrotic and angiogenic pathways were excluded (52, 53). Not only that, EVs released from adipocytes derived from obese patients are enriched in different proteins involved in ECM remodeling to promote adipose tissue fibrosis during obesity, including collagens, metalloproteinases, and ECM receptors (54).
2.4 Adipose cells promote fibrosis by activating immune cells
Adipose tissue is recognized as a natural reservoir of inflammatory cells, including macrophages, natural killer cells, mast cells, eosinophils, and lymphocytes (55). Inflammatory cells are mainly responsible for maintaining immune homeostasis under physiological conditions. However, in the obese adipose tissue microenvironment, adipocytes secrete inflammatory factors to recruit and activate surrounding inflammatory cells. Crosstalk between adipocytes and inflammatory cells further promotes the process of fibrosis. For example, the levels of Adipocyte-secreted exosomal microRNA-34a (miR-34a) were elevated in obesity compared to normal, which favored the infiltration of M1 macrophages (56). A unique structure called a crown-like structure (CLS) is formed by macrophages to clear necrotic fat cells. Macrophage-inducible C-type lectin (Mincle) is localized in CLS and promotes the expression of fibrosis-related genes, thereby leading to myofibroblast formation possibly through intercellular communication between macrophages and fibroblasts (57). Furthermore, Adipocytes induced with macrophage-derived medium from obese adipose tissue significantly reduced the expression of adipogenic genes such as peroxisome proliferator-activated receptor-γ (PPAR-γ), while overexpression of inflammatory and extracellular matrix synthesis genes (58). Similarly, white adipose tissue (WAT) from obese humans and mice contains more mast cells than from lean individuals (59). Mast cells adhere and activate fibroblasts in a PAI1-dependent manner, culminating in a cascade of events leading to fibrogenesis (60). At the same time, it has also been shown that the amount of Group 1 innate lymphoid cells (ILC1s) is increased in obese T2D patients and induces adipose fibrosis by releasing IFN-γ (61).
2.5 Adipocytes promote fibrosis by downregulating adipogenic differentiation of adipose precursor cells
In addition to differentiated mature adipocytes, adipose tissue also contains various other cell types. Among them, the most extensively discussed are the adipose precursor cells located in the stromal vascular fractions (SVFs) of adipose tissue. Adipose precursor cells from different reservoirs exhibit significant phylogenetic differences and heterogeneity, as well as varying differentiation potential (62). Under normal physiological conditions, these adipose precursor cells maintain a high degree of self-renewal and proliferation to support the homeostasis and expansion of adipose tissue (63). However, obesity impairs the immunoregulation and survival efficacy of ADSCs. ADSCs derived from obese individuals demonstrate impaired anti-inflammatory phenotypes (64). When exposed to an excessive accumulation of inflammatory cytokines in their microenvironment, stem cell transformation and proliferation abilities become compromised, leading to premature cellular decline (65). Additionally, aging ADSCs not only exhibit down-regulated ability for adipogenic differentiation but also show significant enrichment in genes related to collagen production and inflammatory function (66). Studies have further revealed that with increasing BMI levels, there is upregulation of IL-1R-like 1 expression in mature adipocytes which can inhibit the differentiation of adipose precursor cells thereby reducing adaptive expansion capacity within the adipose tissue (67).
2.6 Adipocytes promote fibrosis by transdifferentiation into myofibroblasts
Fibroblasts and myofibroblasts are the major producers of adipose tissue fibrosis (Figure 2). Myofibroblasts possess both the ECM synthesis capacity of fibroblasts and the cytoskeletal contractility of smooth muscle cells. This enables myofibroblasts to exert pressure on the ECM, thereby activating and releasing latent TGF-β within it, exacerbating the tissue fibrosis process (68). The traditional view of cell differentiation holds that cells follow a defined differentiation trajectory during development, starting with stem cells and ending in a terminally differentiated state. However, in addition to classical fibroblasts and myofibroblasts be able to be derived from adipocytes with high plasticity. This process of interconversion of adipocyte and myofibroblast fates has been described as adipocyte mesenchymal transition (AMT), marked by the downregulation of adipogenic markers and the acquisition of a mesenchymal phenotype (69). AMT is involved in various pathophysiological processes such as wound healing, scleroderma, and cancer (70, 71). Moreover, the ability of adipocytes to transdifferentiate into a fibroblast-like phenotype was greatly enhanced under the challenge of HFD. The presence of AMT in the pathological microenvironment of adipose tissue in obesity exacerbates fiber remodeling in the extracellular matrix.
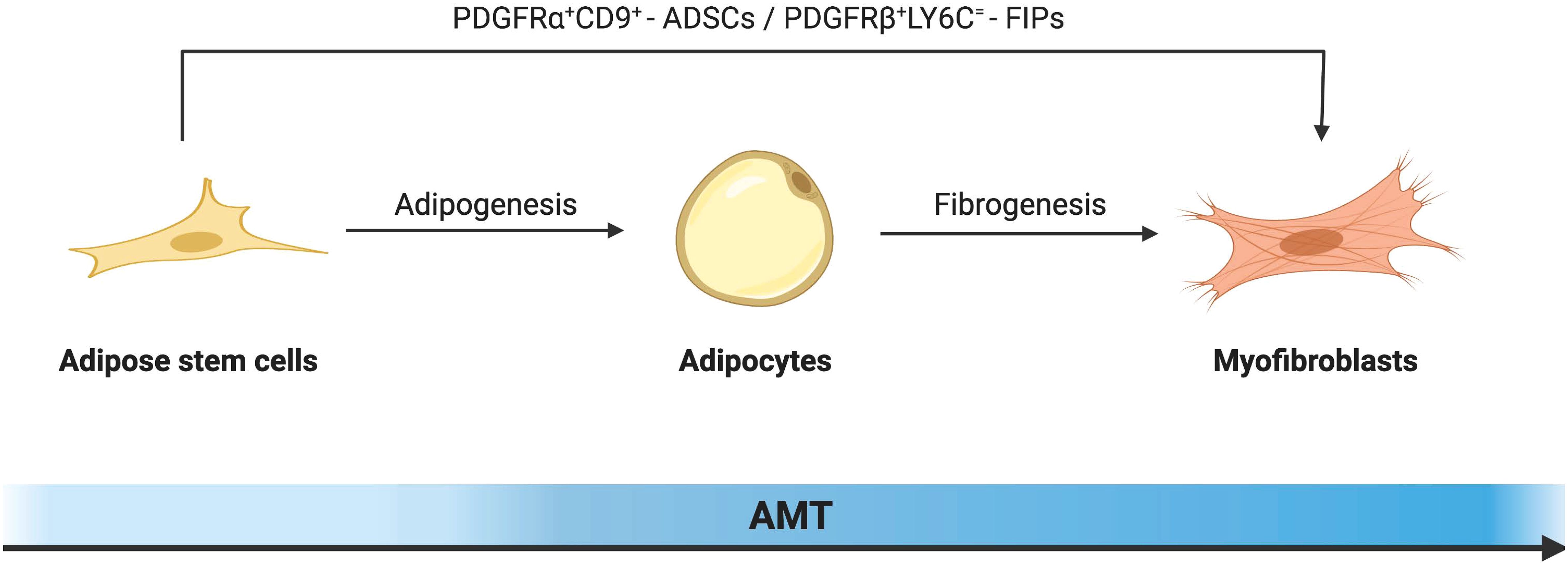
Figure 2 Schematic diagram illustrating the cellular mechanism of adipocyte-mesenchymal transition (AMT) in adipose tissue remodeling under the challenge of obesity. In response to a high-fat diet, alongside the classical fibroblast activation pathway, highly plastic adipocytes can also undergo transdifferentiation into myofibroblasts. By downregulating key adipogenic gene markers including PPAR-γ, both mature adipocytes and adipose stem cells initiate the fibrosis pathway as the predominant mechanism, leading to morphological alterations and gene reprogramming that drive their differentiation towards a myofibroblast phenotype.
2.6.1 ADSCs to myofibroblasts
ADSCs can be transformed into myofibroblasts under the regulation of specific cytokines. ADSCs mainly exist in the stromal vascular part of adipose tissue, and their surface expressions are CD34+, CD45-, CD31- and Scal+, which have the potential for multidirectional differentiation similar to bone marrow mesenchymal stem cells (72). Its biological behavior is largely determined by the surrounding microenvironment (73). Under the challenge of the high-fat diet, the expression of cell proliferation marker cyclin D1 in ADSCs was up-regulated, which greatly enhanced their proliferation ability and promoted many aggregations in its fibrous region (74). At the same time, it has been shown that ADSCs isolated from obese mice are significantly enhanced in their ability to secrete extracellular matrix components and acquire a myofibroblast-like phenotype (75). Even, fibroblasts derived from ADSCs produced more extracellular matrix and migrated faster than primary skin fibroblasts (76). This may be due to the abundant TGF-β in the adipose tissue of obese mice. TGF-β is generally considered to be a master regulator of tissue fibrosis. By binding to type I and type II receptors, it promotes phosphorylation of Smad2/3 and enhances ECM deposition (77). Increased TGF-β in adipose tissue promotes the expression of recombinant integrin alpha 5 (ITGA5) and myocardin-related transcription factor (MRTFA). Among them, MRTFA is an important pathogenic factor of AMT transformation (78).
The platelet-derived growth factor receptor alpha (PDGFRα) gene in ADSCs is a major regulator of AMT transformation in obesity. Marcelin et al. defined two sub-populations under the cell population based on the level of the cluster of differentiation 9 (CD9) expression. Among them, the low expression of PDGFRα+CD9 cells was rich in adipogenesis and lipid metabolism-related genes, like the well-known ADSCs. In PDGFRα+CD9 high cells, the expression of transcription factors promoting adipogenesis was low, while the expression of TGF-β signaling mediating pro-fibrosis and genes involved in ECM synthesis were highly expressed. In the mediation of PDGF signaling, the two cell subpopulations were out of balance. PDGFRα+CD9 low cells transformed into PDGFRα+CD9 high fibrophilic phenotype, and the expression of fibrosis markers increased hundreds of times. In addition, there was an increased frequency of CD9-high relative to low-expressing CD9 progenitors and more severe omental adipose tissue fibrosis in severely obese subjects (79–81). This evidence suggests that PDGFRα is an important regulator of cell differentiation direction in the early fibroblast-adipocyte lineage, promoting myofibroblast proliferation and differentiation at the expense of adipocyte production.
Other genes related to AMT in ADSCs have also been reported. LY6C+ PDGFRβ+ ADSCs, also known as fibro-inflammatory progenitors (FIPs), are potential contributors to fibrosis. mRNAs for proinflammatory cytokines and extracellular matrix components were more abundant in FIPs compared to normal ADSCs. In addition, FIPs exhibit some anti-lipogenic ability. It is not only insensitive to adipogenic stimulation itself but also can release secreted factors to inhibit the differentiation of adipocytes from ASDCs (82). Further studies showed that fine-tuning mitochondrial function was a key regulator of progenitor fate and function in white adipose tissue (83). This reminds us that ADSCs are heterogeneous cell populations with different adipogenic potentials, and it is expected that more characteristic molecular markers can be identified in the future to predict potential differentiation trajectories.
2.6.2 Adipocytes to myofibroblasts
At first, mature adipocytes were thought to be terminally differentiated cells that could not proliferate. In 1986, When Sugihara et al. cultured adipocytes using the ceiling culture method, they found that adipocytes took on a fibroblast-like appearance and named this cell morphology dedifferentiated adipocytes (84). With further studies of adipocyte plasticity, it was found that dedifferentiated adipocytes (DFAT) not only resembled fibroblasts morphologically but also had altered gene expression during dedifferentiation (85). Genes related to adipogenesis and mitochondrial activity were downregulated while lipid droplets were rapidly secreted. Dedifferentiated adipocytes have up-regulated expression of genes associated with cell renewal and reprogramming, exhibiting stem cell-like properties such as certain proliferative capacity and the ability to re-differentiate into different cell lineages. In vitro differentiation experiments show that adipocytes were driven by transforming growth factor β to preferentially undergo fibrogenic differentiation (86). Through pulse-chase lineage tracing of mouse mature adipocytes. Zhang et al. confirmed in vivo that dedifferentiated mature adipocytes possess certain proliferation and redifferentiation potential and can transdifferentiate in response to bleomycin stimulation for myofibroblasts. The transdifferentiation of dermal adipocytes into myofibroblasts is essential for the repair of skin wounds. Furthermore, using single-cell RNA sequencing (scRNA-seq), they found that adipocytes first dedifferentiate into PDGFRα+ preadipocytes. Thus, the transdifferentiation of mature adipocytes into myofibroblasts may occur through a two-step process of dedifferentiation back to ADSCs and then differentiation into myofibroblasts (69, 70). However, there is evidence that the transformation between fibroblasts and adipocytes is reciprocal (87–89). In other words, AMT is a double-edged sword in the process of tissue fibrosis. It is not only an additional pathogenic source of myofibroblasts but also a key part of reversing fibrosis.
3 The influence of adipose tissue fibrosis on other diseases
Adipose tissue is widely distributed throughout the human body and exhibits close interactions with various tissues and organs, rendering it a crucial biosensor for metabolic health (Figure 3). Different adipose tissue reservoirs possess distinct morphologies and functions, enabling them to perceive and respond to external signals from the systemic circulation and local microenvironment of other organs. In cases of obesity, adipocytes release fibrophilic adipokines, proinflammatory factors, and undergo transdifferentiation into myofibroblasts within the fat pad itself, significantly impeding the adaptability necessary for adipocyte growth and proliferation. Consequently, damaged fibrofatty tissue can directly infiltrate neighboring tissues such as the heart, bones, and joints leading to detrimental effects. Moreover, it can also transmit information to metabolically active organs through paracrine effects, interference in intercellular communication pathways, as well as abnormal accumulation of lipid components thereby elevating the risk of fibrosis development. Extensive literature has documented lipotoxicity observed in diverse human diseases along with experimental animal models. Lipotoxicity is the abnormal accumulation of toxic lipids in cells primarily caused by FFA. The mechanisms of lipotoxicity involve various cellular processes, including organelle damage and activation of intracellular signaling pathways. Damaged cells are prone to apoptosis or necrotic cell death, which releases many inflammatory cytokines and fibrotic mediators to further aggravate tissue fibrosis (90). In addition, in scleroderma and cancer, the loss of large amounts of intradermal fat is often associated with an adverse outcome. Therefore, fibrotic adipose tissue is not just localized damage to adipose tissue. It can also participate in the occurrence and development of a variety of diseases through strong cell-autonomous and paracrine effects, and ultimately produce systemic effects.
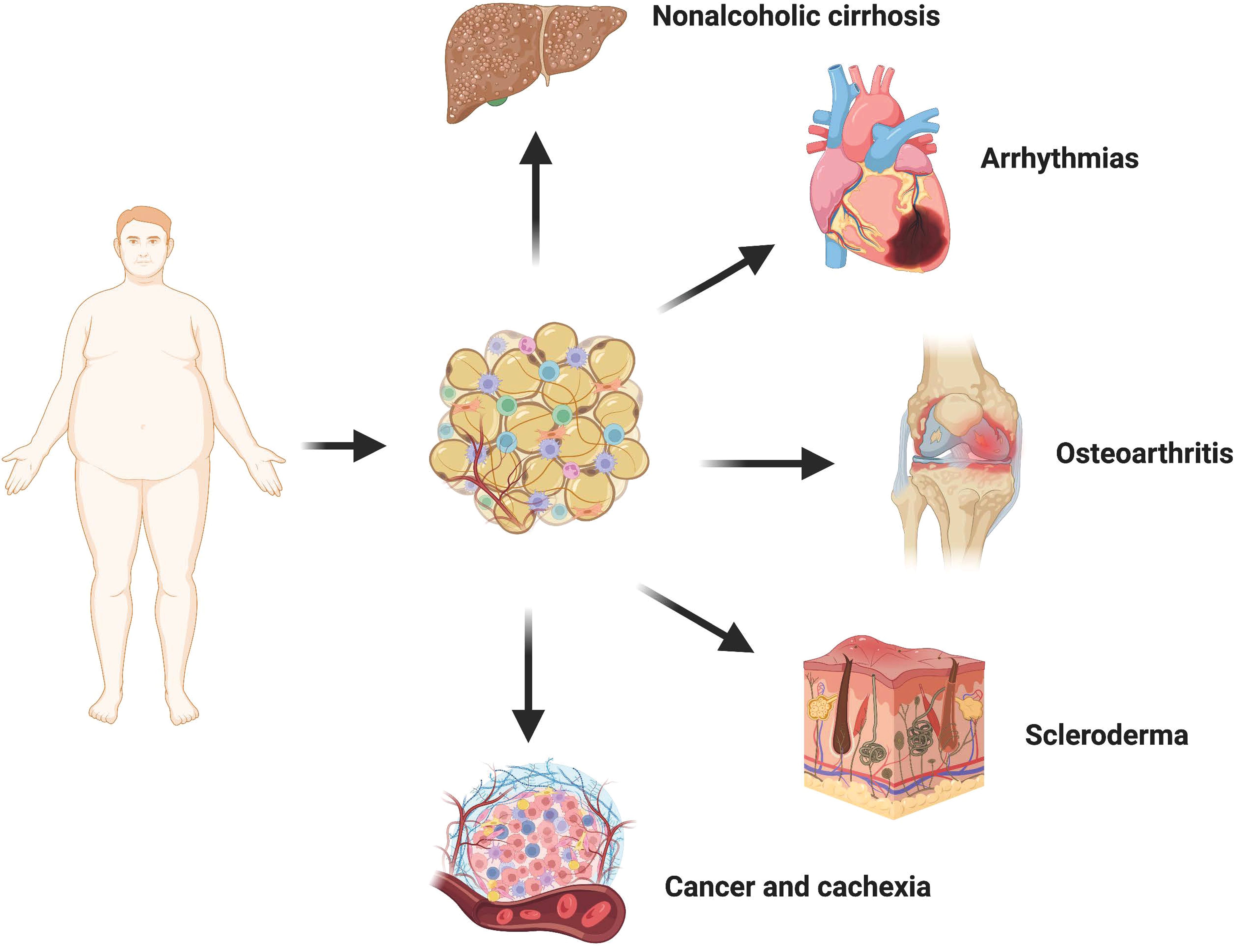
Figure 3 Crosstalk between adipose tissue and other organs. In the state of obesity, adipocytes undergo transdifferentiation into myofibroblasts via the release of various fibrophilic cytokines, thereby promoting local fibrosis of the adipose tissue. The presence of fibrotic adipose tissue not only significantly restricts the hypertrophy and proliferative adaptability of adipocytes themselves but also exerts a detrimental influence on neighboring tissues and organs through direct infiltration. Consequently, there is an increased risk and severity associated with non-alcoholic liver cirrhosis, cardiovascular disease, osteoarthritis, cancer, and scleroderma.
3.1 Osteoarthritis
Osteoarthritis (OA) is a complex disease with multiple contributing factors, characterized by pain, joint dysfunction, and chronic disability. The pathogenesis of this condition involves the interplay between increased biomechanical joint load due to obesity or systemic inflammation and metabolic dysfunction (91, 92). Notably, obesity induces fibrosis in the subchondral fat pad, significantly elevating the susceptibility to osteoarthritis (93). Moreover, fibrosis of the fatty pad beneath the patella seems to be a common feature of most osteoarthritis. During total knee replacement, Harasymowicz et al. biopsied the synovial and subpatellar fat pad of the knee in patients with end-stage osteoarthritis: adiponectin expression in synovial adipose tissue was significantly reduced in obese patients, showing marked fibrosis and macrophage infiltration, which may affect the nutrient supply to the articular cartilage (94). In addition, the fat pad under the patella, as an endocrine organ, in combination with other pathogenic factors such as mechanical loading, releases inflammatory mediators and adipokines such as IL-1, interleukin-13 (IL-13) and leptin into the knee joint, exacerbating the pathological damage of osteoarthritis (95–97). In contrast, transgenic mice with lipodystrophy exhibited diminished spontaneous knee injury and pain-related behaviors, along with resistance to high-fat diet-induced proinflammatory tendencies. Conversely, their susceptibility to osteoarthritis could be reinstated through adipose tissue transplantation (92).
3.2 Nonalcoholic cirrhosis
Non-alcoholic cirrhosis (NAFLD) is the most common chronic liver disease worldwide, mainly affecting people with obesity and type 2 diabetes. The reduction of central obesity in patients, particularly the longitudinal decrease in subcutaneous adipose tissue (SAT) and VAT volume, contributes to the histological amelioration of NAFLD (98). The study of Leven et al. showed that collagen deposition in visceral white adipose tissue (vWAT) of NAFLD patients was significantly increased (99). This indicates that there is a certain relationship between the fibrosis of adipose tissue and the occurrence and development of NAFLD, which may be related to the increase of lipid components. During obesity, the release of adipose tissue into circulation surpasses the liver’s inherent capacity to buffer lipids. Furthermore, obesity diminishes the production of Neuregulin 4, an adipokine that enhances hepatic lipid metabolism (100). Many types of lipid components have been shown to cause liver damage, including FFA, triglyceride (TG), free cholesterol (FC), etc. Injured hepatocytes released numerous inflammatory cytokines and fibrotic mediators, further aggravating liver pathology (101). Recently, Yu et al. showed that lipid accumulation-induced hepatocyte senescence activates hepatic stellate cells through the nuclear factor erythroid 2-related factor 2 (Nrf2)-antioxidant response element pathway. Under FFA-treated conditions, hepatocytes significantly increased the activation of co-cultured primary hepatic stellate cells (HSCs) and the expression of pro-fibrotic molecules while senescent (102). Dysregulation of adipokines further exacerbates liver injury in obesity. Cysteine-like protein 1 secreted by white adipose tissue (WAT) aggravates liver injury and inflammation in a mouse model of nonalcoholic steatohepatitis (NASH) under high-fat diet conditions by activating Toll-like receptor 4 (103). Moreover, hypolipinemia associated with obesity also contributes to the development of hepatic steatosis, fibrosis, and hepatocellular carcinoma (104).
3.3 Cancer and cachexia
Epidemiological evidence strongly indicates a significant correlation between excess weight or obesity and the development of numerous types of cancer (105). Gene expression associated with cancer progression is profoundly affected by obesity, and adipocytes from obese individuals create more favorable conditions for tumor formation. Data suggest that breast cancer incidence and metastatic risk are significantly higher in populations with higher BMI (106). It may be that factors secreted by adipose tissue, particularly in obese individuals, alter the transcriptome profile of breast cancer cells and promote reprogramming of cancer cell metabolism to produce a more aggressive phenotype (107, 108). Obesity induces upregulation of aromatase expression and downregulation of sex hormone binding globulin levels in adipose tissue, resulting in elevated free estrogen content. Consequently, this stimulates endometrial hyperplasia and augments the risk of cancer (109, 110). Leptin facilitates the proliferation and functional activation of endometrial cancer cells by modulating JAK2/STAT3, MAPK/ERK, and PI3K/AKT signaling pathways (111). Furthermore, obesity-generated fibrotic fat microenvironment also contributes to increased overall tumor or cancer fibrosis levels. Incio et al. found that pancreatic ductal adenocarcinoma (PDAC) in obese patients presented with hypertrophic adipocytes and more pronounced ECM deposition (112). Dense connective tissue hyperplasia compromises blood perfusion and poses a huge obstacle to the delivery and efficacy of chemotherapeutic drugs, leading to poorer treatment outcomes.
Obesity is not only a risk factor for cancer development but is also directly associated with poor prognosis in multiple tumor types. About 80% of cancer patients in advanced stages develop cachexia, characterized by continued uncontrolled weight loss, which directly leads to death in 22-40% of patients with end-stage cancer (113). Reduction in fat cell size, rupture of the capsule, and excessive deposition of extracellular matrix were observed in both patients and mouse models of cachexia. Even more interesting, Myofibroblasts in fibrotic regions often surround fat cells (114). Compared with normal fibroblasts, CAF has heterogeneity and high plasticity (115). ADSCs are an important cell source of CAF, and this process is closely related to the activation of the Wnt signaling pathway (116). In addition, it was noted that overweight or obese patients had higher levels of ADSCs circulating in their blood compared to cancer patients with lower body weight (117). Taken together, it is reasonable to assume that increased stromal stiffness in adipose tissue is an important mediator of cancer onset and progression. And inhibiting the excessive deposition of stroma by blocking the transformation of ADSCs to CAF may be a very promising treatment for fibrosis-related cancers.
3.4 Arrhythmias and heart failure
The smooth transmission of cardiomyocyte potential depends on the special electrophysiological and structural characteristics of heart tissue, abnormal cardiac excitation will lead to arrhythmia. Atrial fibrillation is the most common type in clinical practice and has a high risk of death due to the risk of hemodynamic abnormalities and thromboembolism (118). Over the years, a variety of risk factors for arrhythmias have been identified, among which an increase in the number of non-excitable cells due to abnormalities in cardiac tissue remodeling has been extensively studied. Obesity generally leads to more frequent and persistent atrial fibrillation, which may indicate that fiber remodeling of extra-cardiac adipose tissue may play a role in promoting myocardial fibrosis (119, 120).
Extracardial adipose tissue (EAT) is in direct contact with the atria and shares a common blood supply with the myocardium. To mitigate lipotoxicity, epicardial adipose tissue (EAT) demonstrates enhanced rates of FFA uptake compared to subcutaneous adipose tissue (SAT), and exhibits heightened sensitivity towards variations in dietary lipid content. While the heart predominantly relies on lipids as metabolic substrates, excessive lipid accumulation can give rise to detrimental consequences. Excessive deposition of lipids may lead to severe arrhythmogenic complications (121). Simultaneously, recent experiments have shown that there is a close relationship between fibrofatty infiltration and arrhythmia caused by myocardial fibrosis (122, 123). The infiltration of fat cells itself interferes with the normal conduction of cardiomyocyte potential (124). Extracardiac adipose tissue has been proven to secrete fibrophilic mediators, such as connective tissue growth factor CTGF, TGF-β, and Activin A to promote myocardial fibrosis (125, 126). Moreover, epicardial adipose tissue serves as a localized indicator of systemic inflammation in individuals with obesity and has the capability to secrete diverse inflammatory mediators, including IL-6 and TNFα (127). Under the combined drive of these factors, the pericardial mesenchymal stem cells can migrate to the ventricular muscle and transform into fibroblasts, promoting the fibrotic remodeling of the myocardium (128). Infiltrated adipocytes, fibroblasts, and myofibroblasts can form gap junctions with adjacent cardiomyocytes through connexin, affecting normal electrophysiological conduction of cardiomyocytes.
At the same time, it has been thought that the effect of obesity on heart failure is achieved by increasing the load of the heart through hemodynamics. However, most obese patients have only a slight increase in heart volume, most of which is ejection fraction reserved heart failure (HFpEF), which is characterized by fibrotic failure of the cardiac microvascular supply and limited expansion of the heart (129, 130). Most significantly, bariatric surgery effectively mitigates ventricular repolarization heterogeneity in obese patients (131).
3.5 Scleroderma
Scleroderma (SSc) is marked by excessive deposition of ECM proteins caused by abnormal activation of myofibroblasts, which mainly affects the skin and blood vessel walls, not only causing inconvenience to patients’ daily life but also potentially causing fatal organ dysfunction (132). Dysfunctions of adipocytes and abnormal secretion of adipokines are key events in the progression of scleroderma fibrosis. First, skin fibrosis in SSc is often accompanied by significant loss of intradermal fat. Moreover, myofibroblasts in scleroderma have been shown to originate at least in part from subdermal fat cells (133). More importantly, in chronological terms, atrophy of intradermal adipose tissue usually precedes myofibroblast accumulation and subsequent skin thickening (86). Further studies showed that ADSCs produced AMT in bleomycin-induced fibrotic skin, exhibiting the characteristics of myofibroblasts (134). This may be caused by the significantly up-regulated nuclear receptor corepressor (NcoR) signal in SSc. NCoR is a negative regulator of gene expression. It recruits histone deacetylation (transcriptional inhibition) enzymes to the DNA promoter region, and its main function in adipose tissue is to inhibit PPAR-γ transcriptional activity (135). Injection of normal ADSCs or fat transplantation into scleroderma patients has achieved good results in softening skin and alleviating fibrosis in animal models and clinics (136, 137). This evidence conveys a valuable message that the loss of adipose tissue in scleroderma is not merely a pathological phenomenon, but a direct contribution to skin fibrosis.
4 Anti-fibrotic strategies targeting adipose tissue fibrosis
Imbalance in energy homeostasis causes adipose tissue fibrosis, which exacerbates the progression of obesity-related fibrotic disease. Therefore, focusing on maintaining the healthy expansion of adipocytes and preventing excessive deposition of extracellular matrix components is an attractive proposition against pathological fibrotic remodeling. Current major strategies focus on altering the function of white adipose tissue, targeted therapy, and dietary therapy.
4.1 Dietary therapy
Factors that predispose to obesity in modern lifestyles include longer periods of food intake and shorter periods of fasting. However, it is unrealistic to expect overweight patients to strictly adhere to daily calorie restrictions (DR). Comparatively, intermittent eating (IF) is widely adopted as a more operational dietary regimen involving repeated and regular energy restriction, resulting in multiple health benefits. Meanwhile, it was found that IF mediates adaptive tissue remodeling of WAT to prevent HFD-induced adipose tissue inflammation and fibrosis (138). In addition to reducing energy intake, supplementing some nutrients with special functions is also an effective means to resist WAT fibrosis. Berberine, present in a variety of Chinese herbal plants, has been shown to have therapeutic potential in the treatment of diabetes and dyslipidemia. As a natural anti-inflammatory compound, berberine reverses signaling and controls HFD-induced macrophage infiltration and polarization. Beyond that, berberine alleviates adipose tissue fibrosis by inducing AMP-activated kinase signaling in high-fat diet-induced obese mice (139). Recently, in a randomized controlled trial, Lontchi-Yimagou et al. found that increasing vitamin D supplementation within the normal range had a beneficial effect on suppressing adipose tissue inflammation and fibrosis in obese subjects. This phenomenon was also confirmed in adipocyte-specific vitamin D receptor knockout (Ad-VDR KO) mice (140). Similarly, oral administration of the antioxidant vitamin E has also been reported to increase oxidative stress and reduce collagen deposition in vWAT in obese mice, thereby increasing the lipid storage capacity of adipocytes and reducing obesity-related lipotoxicity (141). Unfortunately, it is unclear how long the effects of oral nutritional supplementation treatments can be sustained.
4.2 Medical treatment
4.2.1 Promotes WAT browning
Unlike WAT, which is primarily used for energy storage, BAT converts energy into heat by decoupling-protein uncoupling protein 1 (UCP-1) (142). Meanwhile, WAT deposits contain polyocular cells that express UCP-1, called beige cells, which are stimulated by the browning process when exposed to cold or other stimuli. In recent years, more and more studies have shown that the browning and fibrotic remodeling of adipose tissue are two opposing processes. MRTFA-deficient mice are resistant to HFD-induced adipocyte overexpansion and have increased numbers of beige adipocytes in their WAT (143). Further studies revealed that positive regulatory domain 16 (PRDM16) that controls BAT development could promote β-hydroxybutyrate (BHB) secretion by driving fatty acid oxidative metabolism, which rescued adipose expressing HIF1α or TGFβ-treated PDGFRα+ ADSCs cell differentiation potential (144). Interestingly, the anti-fibrotic capacity of BAT may also act in a UCP-1-independent manner. Activation of the PRDM16 transcriptional complex effectively suppressed adipose tissue fibrosis through direct interaction with GTF2IRD1 (145). And it is worth noting that many browning-inducing drugs have been shown to have potent anti-fibrotic effects at the same time (Table 1). Therefore, induction of browning of white fat is a potential therapeutic strategy against HFD-induced adipose tissue fibrosis and related dysfunction.
4.2.2 Enhance lipid metabolism
Dysfunction of adipocytes begins with excessive lipid accumulation, so accelerating the metabolic function of adipose tissue and improving its lipid buffering capacity can prevent the occurrence of obesity-related fibrotic comorbidities. As a key site of oxidative phosphorylation, mitochondria play a complex and important role in maintaining the metabolic homeostasis of adipocytes. Serine/thionine kinase 25 (Skt25) protects against diet-induced adipose tissue fibrosis by regulating mitochondrial activity in adipose tissue (160). At the same time, there is evidence that the impaired metabolic function of adipocytes in the obese state is closely related to the down-regulation of miR-30a. Overexpression of miR-30a in adipose tissue allows smooth expansion of adipose tissue in a manner that preserves insulin sensitivity (161). Furthermore, proteomic analysis revealed that miR-30a restores WAT homeostasis by targeting plasminogen activator inhibitor 1 (PAI-1) to limit the pro-fibrotic program.
4.3 Targeted therapy
CD248 (endothelin/tumor endothelial marker 1) is a type I transmembrane glycoprotein that is most abundantly expressed in human mature white adipocytes and significantly correlated with body mass index (BMI). Petrus et al. suggest that CD248 acts as an adipocyte sensor in the microenvironment and may mediate the abnormal biological behavior of adipocytes. Transcriptome analysis of human WAT revealed that CD248 expression was positively correlated with inflammation, hypoxia, and ECM remodeling. CD248 deficiency protects against high-fat diet-induced WAT dysfunction. Besides, adipocyte-specific knockout exhibited a greater therapeutic effect than systemic knockout. Notably, these improvements were also achieved in mice following the onset of obesity. Therefore, the rescue of WAT fibrosis by selectively reducing CD248 expression in adipocytes is an attractive target for future drug development (162). However, the specific molecular mechanism by which CD248 acts has not been fully described. Therefore, a better understanding of the signaling pathways downstream of CD248 is critical for adipocyte-specific targeted therapy.
5 Conclusion
In general, in the state of obesity, fat cells are dysfunctional and interact with immune cells to produce a fibrogenic cellular microenvironment, which affects the plasticity of white adipose tissue and the normal function of surrounding organs and promotes the occurrence and development of a variety of fibrosis-related diseases. Specifically, adipocytes promote fibrosis by secreting inflammatory mediators, and adipokines and changing their fate to become myofibroblasts. This suggests that when studying many systemic diseases associated with fibrosis, it is time to focus on the nearest reservoir of adipose tissue. At present, the use of adipose tissue to regulate fibrosis has great untapped potential, but due to the complexity of the dialogue between adipose cells and fibroblasts, there is still a lot of work to be done to figure out the specific mechanism of adipose cells involved in regulating the process of tissue fibrosis.
First, most studies of adipocyte secretory factors promoting fibrosis have been limited to animals or in vitro. The specific signaling pathway that promotes fibrosis is still unknown. Secondly, further studies of the AMT are needed to provide more solid evidence to confirm whether myofibroblasts produced by fat cells are a one-step or multi-step process. Besides, it is expected to decipher the key aggregation points downstream of various signaling pathways in the adipocyte-myofibroblast transition process, to realize the controllability of transformation. Finally, inducing differentiation of myofibroblasts into brown adipocytes is a feasible strategy for antagonizing fibrosis. However, it should be noted that most of the anti-fibrosis drugs mentioned in this paper have a wide range of targets, so the side effects of drugs should not be underestimated. Therefore, it is urgent to find effective brown inducers or inducers for specific adipose tissue. At the same time, another great challenge is how to effectively complete the transformation of myofibroblasts into brown fat cells under pathological conditions. Because in an obese state, cells are much less sensitive to browning agonists. Moreover, there is a lack of drugs that can effectively increase the expression of brown adipose tissue in humans. We believe that if these mechanisms can be elucidated through subsequent experimental studies and brown fat cells are indeed effective in antagonizing fibrosis, regulating the biological behavior of fat cells will be a new option for treating many fibrosis-related diseases.
Author contributions
QZ: Writing – original draft. CL: Writing – review & editing, Data curation, Methodology. FL: Methodology, Writing – review & editing. YL: Writing – review & editing. JC: Methodology, Writing – review & editing. JG: Funding acquisition, Methodology, Writing – original draft, Writing – review & editing.
Funding
The author(s) declare financial support was received for the research, authorship, and/or publication of this article. We appreciate the financial support provided by the National Nature Science Foundation of China (81772101, 81871573, 81901976, 81971852).
Conflict of interest
The authors declare that the research was conducted in the absence of any commercial or financial relationships that could be construed as a potential conflict of interest.
Publisher’s note
All claims expressed in this article are solely those of the authors and do not necessarily represent those of their affiliated organizations, or those of the publisher, the editors and the reviewers. Any product that may be evaluated in this article, or claim that may be made by its manufacturer, is not guaranteed or endorsed by the publisher.
Glossary
References
1. Stenkula KG, Erlanson-Albertsson C. Adipose cell size: importance in health and disease. Am J Physiol Regul Integr Comp Physiol. (2018) 315(2):R284–r95. doi: 10.1152/ajpregu.00257.2017
2. Liu F, He J, Wang H, Zhu D, Bi Y. Adipose morphology: a critical factor in regulation of human metabolic diseases and adipose tissue dysfunction. Obes surgery. (2020) 30:5086–100. doi: 10.1007/s11695-020-04983-6
3. Klein S, Gastaldelli A, Yki-Järvinen H, Scherer PE. Why does obesity cause diabetes? Cell Metab. (2022) 34:11–20. doi: 10.1016/j.cmet.2021.12.012
4. Weiskirchen R, Weiskirchen S, Tacke F. Organ and tissue fibrosis: Molecular signals, cellular mechanisms and translational implications. Mol aspects Med. (2019) 65:2–15. doi: 10.1016/j.mam.2018.06.003
5. Distler JHW, Györfi A-H, Ramanujam M, Whitfield ML, Königshoff M, Lafyatis R. Shared and distinct mechanisms of fibrosis. Nat Rev Rheumatol. (2019) 15:705–30. doi: 10.1038/s41584-019-0322-7
6. Miller JL, Blaszkiewicz M, Beaton C, Johnson CP, Waible S 2nd, Dubois AL, et al. A peroxidized omega-3-enriched polyunsaturated diet leads to adipose and metabolic dysfunction. J Nutr Biochem. (2019) 64:50–60. doi: 10.1016/j.jnutbio.2018.10.010
7. Mao Y, Luo W, Zhang L, Wu W, Yuan L, Xu H, et al. STING-IRF3 triggers endothelial inflammation in response to free fatty acid-induced mitochondrial damage in diet-induced obesity. Arteriosclerosis thrombosis Vasc Biol. (2017) 37:920–9. doi: 10.1161/ATVBAHA.117.309017
8. Ozaki K-i, Awazu M, Tamiya M, Iwasaki Y, Harada A, Kugisaki S, et al. Targeting the ERK signaling pathway as a potential treatment for insulin resistance and type 2 diabetes. Am J Physiol Endocrinol Metab. (2016) 310(8):E643–E51. doi: 10.1152/ajpendo.00445.2015
9. Van Pelt DW, Guth LM, Wang AY, Horowitz JF. Factors regulating subcutaneous adipose tissue storage, fibrosis, and inflammation may underlie low fatty acid mobilization in insulin-sensitive obese adults. Am J Physiology-Endocrinology Metab. (2017) 313:E429–E39. doi: 10.1152/ajpendo.00084.2017
10. van Zutphen T, Stroeve JHM, Yang J, Bloks VW, Jurdzinski A, Roelofsen H, et al. FXR overexpression alters adipose tissue architecture in mice and limits its storage capacity leading to metabolic derangements. J Lipid Res. (2019) 60:1547–61. doi: 10.1194/jlr.M094508
11. D'Agati VD, Chagnac A, de Vries AP, Levi M, Porrini E, Herman-Edelstein M, et al. Obesity-related glomerulopathy: clinical and pathologic characteristics and pathogenesis. Nat Rev Nephrology. (2016) 12:453–71. doi: 10.1038/nrneph.2016.75
12. Chumakova G, Gritsenko O, Gruzdeva O, Dyleva Y. Analysis of probable lipotoxic damage and myocardial fibrosis in epicardial obesity. Aging. (2021) 13:14806–15. doi: 10.18632/aging.203148
13. Kita S, Maeda N, Shimomura I. Interorgan communication by exosomes, adipose tissue, and adiponectin in metabolic syndrome. J Clin Invest. (2019) 129:4041–9. doi: 10.1172/JCI129193
14. Holland WL, Scherer PE. Cell Biology. Ronning after the adiponectin receptors. Sci (New York NY). (2013) 342:1460–1. doi: 10.1126/science.1249077
15. Straub LG, Scherer PE. Metabolic messengers: adiponectin. Nat Metab. (2019) 1:334–9. doi: 10.1038/s42255-019-0041-z
16. Asahara N, Okada-Iwabu M, Iwabu M, Wada K, Oka K, Yamauchi T, et al. A monoclonal antibody activating AdipoR for type 2 diabetes and nonalcoholic steatohepatitis. Sci advances. (2023) 9:eadg4216. doi: 10.1126/sciadv.adg4216
17. Fang F, Liu L, Yang Y, Tamaki Z, Wei J, Marangoni RG, et al. The adipokine adiponectin has potent anti-fibrotic effects mediated via adenosine monophosphate-activated protein kinase: novel target for fibrosis therapy. Arthritis Res Ther. (2012) 14:R229. doi: 10.1186/ar4070
18. Reinke L, Lam AP, Flozak AS, Varga J, Gottardi CJ. Adiponectin inhibits Wnt co-receptor, Lrp6, phosphorylation and β-catenin signaling. Biochem Biophys Res Commun. (2016) 470:606–12. doi: 10.1016/j.bbrc.2016.01.097
19. Kim AY, Park YJ, Pan X, Shin KC, Kwak SH, Bassas AF, et al. Obesity-induced DNA hypermethylation of the adiponectin gene mediates insulin resistance. Nat Commun. (2015) 6:7585. doi: 10.1038/ncomms8585
20. Kistorp C, Faber J, Galatius S, Gustafsson F, Frystyk J, Flyvbjerg A, et al. Plasma adiponectin, body mass index, and mortality in patients with chronic heart failure. Circulation. (2005) 112:1756–62. doi: 10.1161/CIRCULATIONAHA.104.530972
21. de Sousa Neto IV, Prestes J, Pereira GB, Almeida JA, Ramos GV, de Souza FHV, et al. Protective role of intergenerational paternal resistance training on fibrosis, inflammatory profile, and redox status in the adipose tissue of rat offspring fed with a high-fat diet. Life Sci. (2022) 295:120377. doi: 10.1016/j.lfs.2022.120377
22. Li Q, Spalding KL. Profiling hypertrophic adipocytes in humans, from transcriptomics to diagnostics. EBioMedicine. (2022) 81:104105. doi: 10.1016/j.ebiom.2022.104105
23. Reilly SM, Saltiel AR. Adapting to obesity with adipose tissue inflammation. Nat Rev Endocrinology. (2017) 13:633–43. doi: 10.1038/nrendo.2017.90
24. Matsui Y, Tomaru U, Miyoshi A, Ito T, Fukaya S, Miyoshi H, et al. Overexpression of TNF-α converting enzyme promotes adipose tissue inflammation and fibrosis induced by high fat diet. Exp Mol pathology. (2014) 97:354–8. doi: 10.1016/j.yexmp.2014.09.017
25. Hou J, Ma T, Cao H, Chen Y, Wang C, Chen X, et al. TNF-α-induced NF-κB activation promotes myofibroblast differentiation of LR-MSCs and exacerbates bleomycin-induced pulmonary fibrosis. J Cell Physiol. (2018) 233:2409–19. doi: 10.1002/jcp.26112
26. Chen W, Yuan H, Cao W, Wang T, Chen W, Yu H, et al. Blocking interleukin-6 trans-signaling protects against renal fibrosis by suppressing STAT3 activation. Theranostics. (2019) 9:3980–91. doi: 10.7150/thno.32352
27. Hwang S, Chung KW. Targeting fatty acid metabolism for fibrotic disorders. Arch pharmacal Res. (2021) 44:839–56. doi: 10.1007/s12272-021-01352-4
28. Corvera S, Solivan-Rivera J, Yang Loureiro Z. Angiogenesis in adipose tissue and obesity. Angiogenesis. (2022) 25:439–53. doi: 10.1007/s10456-022-09848-3
29. Oliva-Olivera W, Castellano-Castillo D, von Meyenn F, Cardona F, Lönnberg T, Tinahones FJ. Human adipose tissue-derived stem cell paracrine networks vary according metabolic risk and after TNFα-induced death: An analysis at the single-cell level. Metabolism: Clin experimental. (2021) 116:154466. doi: 10.1016/j.metabol.2020.154466
30. Ali MM, Phillips SA, Mahmoud AM. HIF1α/TET1 pathway mediates hypoxia-induced adipocytokine promoter hypomethylation in human adipocytes. Cells. (2020) 9. doi: 10.3390/cells9010134
31. Halberg N, Khan T, Trujillo ME, Wernstedt-Asterholm I, Attie AD, Sherwani S, et al. Hypoxia-inducible factor 1alpha induces fibrosis and insulin resistance in white adipose tissue. Mol Cell Biol. (2009) 29:4467–83. doi: 10.1128/MCB.00192-09
32. Baumann B, Hayashida T, Liang X, Schnaper HW. Hypoxia-inducible factor-1α promotes glomerulosclerosis and regulates COL1A2 expression through interactions with Smad3. Kidney Int. (2016) 90:797–808. doi: 10.1016/j.kint.2016.05.026
33. Higgins DF, Kimura K, Bernhardt WM, Shrimanker N, Akai Y, Hohenstein B, et al. Hypoxia promotes fibrogenesis in vivo via HIF-1 stimulation of epithelial-to-mesenchymal transition. J Clin Invest. (2007) 117:3810–20. doi: 10.1172/JCI30487
34. Sun K, Halberg N, Khan M, Magalang UJ, Scherer PE. Selective inhibition of hypoxia-inducible factor 1α ameliorates adipose tissue dysfunction. Mol Cell Biol. (2013) 33:904–17. doi: 10.1128/MCB.00951-12
35. Fukuhara A, Matsuda M, Nishizawa M, Segawa K, Tanaka M, Kishimoto K, et al. Visfatin: a protein secreted by visceral fat that mimics the effects of insulin. Sci (New York NY). (2005) 307:426–30. doi: 10.1126/science.1097243
36. Ezzati-Mobaser S, Malekpour-Dehkordi Z, Nourbakhsh M, Tavakoli-Yaraki M, Ahmadpour F, Golpour P, et al. The up-regulation of markers of adipose tissue fibrosis by visfatin in pre-adipocytes as well as obese children and adolescents. Cytokine. (2020) 134:155193. doi: 10.1016/j.cyto.2020.155193
37. Heo YJ, Choi SE, Lee N, Jeon JY, Han SJ, Kim DJ, et al. Visfatin exacerbates hepatic inflammation and fibrosis in a methionine-choline-deficient diet mouse model. J Gastroenterol hepatology. (2021) 36:2592–600. doi: 10.1111/jgh.15465
38. Zhang J, Zhang L, Zhang S, Yu Q, Xiong F, Huang K, et al. HMGB1, an innate alarmin, plays a critical role in chronic inflammation of adipose tissue in obesity. Mol Cell endocrinology. (2017) 454:103–11. doi: 10.1016/j.mce.2017.06.012
39. Ghosh AR, Bhattacharya R, Bhattacharya S, Nargis T, Rahaman O, Duttagupta P, et al. Adipose recruitment and activation of plasmacytoid dendritic cells fuel metaflammation. Diabetes. (2016) 65:3440–52. doi: 10.2337/db16-0331
40. Zou H, Ming B, Li J, Xiao Y, Lai L, Gao M, et al. Extracellular HMGB1 contributes to the chronic cardiac allograft vasculopathy/fibrosis by modulating TGF-β1 signaling. Front Immunol. (2021) 12:641973. doi: 10.3389/fimmu.2021.641973
41. Liu Y, Li Y, Liang J, Sun Z, Wu Q, Liu Y, et al. The mechanism of leptin on inhibiting fibrosis and promoting browning of white fat by reducing ITGA5 in mice. Int J Mol Sci. (2021) 22. doi: 10.3390/ijms222212353
42. Palhinha L, Liechocki S, Hottz ED, Pereira J, de Almeida CJ, Moraes-Vieira PMM, et al. Leptin induces proadipogenic and proinflammatory signaling in adipocytes. Front endocrinology. (2019) 10:841. doi: 10.3389/fendo.2019.00841
43. Rahmouni K. Leptin-induced sympathetic nerve activation: signaling mechanisms and cardiovascular consequences in obesity. Curr hypertension Rev. (2010) 6:104–209. doi: 10.2174/157340210791170994
44. Martínez-Martínez E, Jurado-López R, Valero-Muñoz M, Bartolomé MV, Ballesteros S, Luaces M, et al. Leptin induces cardiac fibrosis through galectin-3, mTOR and oxidative stress: potential role in obesity. J hypertension. (2014) 32:1104–14. doi: 10.1097/HJH.0000000000000149
45. Huby AC, Antonova G, Groenendyk J, Gomez-Sanchez CE, Bollag WB, Filosa JA, et al. Adipocyte-derived hormone leptin is a direct regulator of aldosterone secretion, which promotes endothelial dysfunction and cardiac fibrosis. Circulation. (2015) 132:2134–45. doi: 10.1161/CIRCULATIONAHA.115.018226
46. Unamuno X, Gómez-Ambrosi J, Ramírez B, Rodríguez A, Becerril S, Valentí V, et al. Dermatopontin, A novel adipokine promoting adipose tissue extracellular matrix remodelling and inflammation in obesity. J Clin Med. (2020) 9. doi: 10.3390/jcm9041069
47. Park J, Scherer PE. Adipocyte-derived endotrophin promotes Malignant tumor progression. J Clin Invest. (2012) 122:4243–56. doi: 10.1172/JCI63930
48. Sun K, Park J, Gupta OT, Holland WL, Auerbach P, Zhang N, et al. Endotrophin triggers adipose tissue fibrosis and metabolic dysfunction. Nat Commun. (2014) 5:3485. doi: 10.1038/ncomms4485
49. Stremersch S, De Smedt SC, Raemdonck K. Therapeutic and diagnostic applications of extracellular vesicles. J Controlled release Off J Controlled Release Society. (2016) 244:167–83. doi: 10.1016/j.jconrel.2016.07.054
50. Durcin M, Fleury A, Taillebois E, Hilairet G, Krupova Z, Henry C, et al. Characterisation of adipocyte-derived extracellular vesicle subtypes identifies distinct protein and lipid signatures for large and small extracellular vesicles. J extracellular vesicles. (2017) 6:1305677. doi: 10.1080/20013078.2017.1305677
51. Pardo F, Villalobos-Labra R, Sobrevia B, Toledo F, Sobrevia L. Extracellular vesicles in obesity and diabetes mellitus. Mol aspects Med. (2018) 60:81–91. doi: 10.1016/j.mam.2017.11.010
52. Eirin A, Meng Y, Zhu XY, Li Y, Saadiq IM, Jordan KL, et al. The micro-RNA cargo of extracellular vesicles released by human adipose tissue-derived mesenchymal stem cells is modified by obesity. Front Cell Dev Biol. (2021) 9:660851. doi: 10.3389/fcell.2021.660851
53. Wolfson B, Zhang Y, Gernapudi R, Duru N, Yao Y, Lo PK, et al. A high-fat diet promotes mammary gland myofibroblast differentiation through microRNA 140 downregulation. Mol Cell Biol. (2017) 37. doi: 10.1128/MCB.00461-16
54. Camino T, Lago-Baameiro N, Bravo SB, Molares-Vila A, Sueiro A, Couto I, et al. Human obese white adipose tissue sheds depot-specific extracellular vesicles and reveals candidate biomarkers for monitoring obesity and its comorbidities. Trans Res J Lab Clin Med. (2022) 239:85–102. doi: 10.1016/j.trsl.2021.01.006
55. Trim WV, Lynch L. Immune and non-immune functions of adipose tissue leukocytes. Nat Rev Immunol. (2022) 22:371–86. doi: 10.1038/s41577-021-00635-7
56. Pan Y, Hui X, Hoo RLC, Ye D, Chan CYC, Feng T, et al. Adipocyte-secreted exosomal microRNA-34a inhibits M2 macrophage polarization to promote obesity-induced adipose inflammation. J Clin Invest. (2019) 129:834–49. doi: 10.1172/JCI123069
57. Tanaka M, Ikeda K, Suganami T, Komiya C, Ochi K, Shirakawa I, et al. Macrophage-inducible C-type lectin underlies obesity-induced adipose tissue fibrosis. Nat Commun. (2014) 5:4982. doi: 10.1038/ncomms5982
58. Lacasa D, Keophiphath M, Miranville A, Clement K. Macrophage-secreted factors impair human adipogenesis: involvement of proinflammatory state in preadipocytes. Endocrinology. (2007) 148:868–77. doi: 10.1210/en.2006-0687
59. Liu J, Divoux A, Sun J, Zhang J, Clément K, Glickman JN, et al. Genetic deficiency and pharmacological stabilization of mast cells reduce diet-induced obesity and diabetes in mice. Nat Med. (2009) 15:940–5. doi: 10.1038/nm.1994
60. Pincha N, Hajam EY, Badarinath K, Batta SPR, Masudi T, Dey R, et al. PAI1 mediates fibroblast-mast cell interactions in skin fibrosis. J Clin Invest. (2018) 128:1807–19. doi: 10.1172/JCI99088
61. Wang H, Shen L, Sun X, Liu F, Feng W, Jiang C, et al. Adipose group 1 innate lymphoid cells promote adipose tissue fibrosis and diabetes in obesity. Nat Commun. (2019) 10:3254. doi: 10.1038/s41467-019-11270-1
62. Corvera S. Cellular heterogeneity in adipose tissues. Annu Rev Physiol. (2021) 83:257–78. doi: 10.1146/annurev-physiol-031620-095446
63. Jeffery E, Church CD, Holtrup B, Colman L, Rodeheffer MS. Rapid depot-specific activation of adipocyte precursor cells at the onset of obesity. Nat Cell Biol. (2015) 17:376–85. doi: 10.1038/ncb3122
64. Pham DV, Nguyen TK, Nguyen BL, Kim JO, Jeong JH, Choi I, et al. Adiponectin restores the obesity-induced impaired immunomodulatory function of mesenchymal stromal cells via glycolytic reprogramming. Acta Pharm Sin B. (2024) 14:273–91. doi: 10.1016/j.apsb.2023.10.019
65. Gustafson B, Nerstedt A, Smith U. Reduced subcutaneous adipogenesis in human hypertrophic obesity is linked to senescent precursor cells. Nat Commun. (2019) 10:2757. doi: 10.1038/s41467-019-10688-x
66. Kar A, Alvarez M, Garske KM, Huang H, Lee SHT, Deal M, et al. Age-dependent genes in adipose stem and precursor cells affect regulation of fat cell differentiation and link aging to obesity via cellular and genetic interactions. Genome Med. (2024) 16:19. doi: 10.1186/s13073-024-01291-x
67. Challa TD, Straub LG, Balaz M, Kiehlmann E, Donze O, Rudofsky G, et al. Regulation of de novo adipocyte differentiation through cross talk between adipocytes and preadipocytes. Diabetes. (2015) 64:4075–87. doi: 10.2337/db14-1932
68. Wipff PJ, Rifkin DB, Meister JJ, Hinz B. Myofibroblast contraction activates latent TGF-beta1 from the extracellular matrix. J Cell Biol. (2007) 179:1311–23. doi: 10.1083/jcb.200704042
69. Zhang Z, Shao M, Hepler C, Zi Z, Zhao S, An YA, et al. Dermal adipose tissue has high plasticity and undergoes reversible dedifferentiation in mice. J Clin Invest. (2019) 129:5327–42. doi: 10.1172/JCI130239
70. Shook BA, Wasko RR, Mano O, Rutenberg-Schoenberg M, Rudolph MC, Zirak B, et al. Dermal adipocyte lipolysis and myofibroblast conversion are required for efficient skin repair. Cell Stem Cell. (2020) 26:880–95.e6. doi: 10.1016/j.stem.2020.03.013
71. Strong AL, Pei DT, Hurst CG, Gimble JM, Burow ME, Bunnell BA. Obesity enhances the conversion of adipose-derived stromal/stem cells into carcinoma-associated fibroblast leading to cancer cell proliferation and progression to an invasive phenotype. Stem Cells Int. (2017) 2017:9216502. doi: 10.1155/2017/9216502
72. Bunnell BA. Adipose tissue-derived mesenchymal stem cells. Cells. (2021) 10. doi: 10.3390/cells10123433
73. Baer PC. Adipose-derived mesenchymal stromal/stem cells: An update on their phenotype in vivo and in vitro. World J Stem Cells. (2014) 6:256–65. doi: 10.4252/wjsc.v6.i3.256
74. Keophiphath M, Achard V, Henegar C, Rouault C, Clément K, Lacasa D. Macrophage-secreted factors promote a profibrotic phenotype in human preadipocytes. Mol Endocrinol (Baltimore Md). (2009) 23:11–24. doi: 10.1210/me.2008-0183
75. Seo BR, Bhardwaj P, Choi S, Gonzalez J, Andresen Eguiluz RC, Wang K, et al. Obesity-dependent changes in interstitial ECM mechanics promote breast tumorigenesis. Sci Trans Med. (2015) 7:301ra130. doi: 10.1126/scitranslmed.3010467
76. Gersch RP, Raum JC, Calvert C, Percec I. Fibroblasts derived from human adipose stem cells produce more effective extracellular matrix and migrate faster compared to primary dermal fibroblasts. Aesthetic Surg J. (2020) 40:108–17. doi: 10.1093/asj/sjz071
77. Meng XM, Nikolic-Paterson DJ, Lan HY. TGF-β: the master regulator of fibrosis. Nat Rev Nephrology. (2016) 12:325–38. doi: 10.1038/nrneph.2016.48
78. Lin JZ, Rabhi N, Farmer SR. Myocardin-related transcription factor A promotes recruitment of ITGA5+ Profibrotic progenitors during obesity-induced adipose tissue fibrosis. Cell Rep. (2018) 23:1977–87. doi: 10.1016/j.celrep.2018.04.057
79. Iwayama T, Steele C, Yao L, Dozmorov MG, Karamichos D, Wren JD, et al. PDGFRα signaling drives adipose tissue fibrosis by targeting progenitor cell plasticity. Genes Dev. (2015) 29:1106–19. doi: 10.1101/gad.260554.115
80. Marcelin G, Ferreira A, Liu Y, Atlan M, Aron-Wisnewsky J, Pelloux V, et al. A PDGFRα-mediated switch toward CD9(high) adipocyte progenitors controls obesity-induced adipose tissue fibrosis. Cell Metab. (2017) 25:673–85. doi: 10.1016/j.cmet.2017.01.010
81. Holley RJ, Tai G, Williamson AJ, Taylor S, Cain SA, Richardson SM, et al. Comparative quantification of the surfaceome of human multipotent mesenchymal progenitor cells. Stem Cell Rep. (2015) 4:473–88. doi: 10.1016/j.stemcr.2015.01.007
82. Hepler C, Shan B, Zhang Q, Henry GH, Shao M, Vishvanath L, et al. Identification of functionally distinct fibro-inflammatory and adipogenic stromal subpopulations in visceral adipose tissue of adult mice. eLife. (2018) 7. doi: 10.7554/eLife.39636
83. Joffin N, Paschoal VA, Gliniak CM, Crewe C, Elnwasany A, Szweda LI, et al. Mitochondrial metabolism is a key regulator of the fibro-inflammatory and adipogenic stromal subpopulations in white adipose tissue. Cell Stem Cell. (2021) 28:702–17.e8. doi: 10.1016/j.stem.2021.01.002
84. Sugihara H, Yonemitsu N, Miyabara S, Yun K. Primary cultures of unilocular fat cells: characteristics of growth in vitro and changes in differentiation properties. Differentiation; Res Biol diversity. (1986) 31:42–9. doi: 10.1111/j.1432-0436.1986.tb00381.x
85. Wang QA, Song A, Chen W, Schwalie PC, Zhang F, Vishvanath L, et al. Reversible De-differentiation of Mature White Adipocytes into Preadipocyte-like Precursors during Lactation. Cell Metab. (2018) 28:282–8.e3. doi: 10.1016/j.cmet.2018.05.022
86. Marangoni RG, Korman BD, Wei J, Wood TA, Graham LV, Whitfield ML, et al. Myofibroblasts in murine cutaneous fibrosis originate from adiponectin-positive intradermal progenitors. Arthritis Rheumatol (Hoboken NJ). (2015) 67:1062–73. doi: 10.1002/art.38990
87. Cattaneo P, Mukherjee D, Spinozzi S, Zhang L, Larcher V, Stallcup WB, et al. Parallel lineage-tracing studies establish fibroblasts as the prevailing in vivo adipocyte progenitor. Cell Rep. (2020) 30:571–82.e2. doi: 10.1016/j.celrep.2019.12.046
88. Sowa Y, Kishida T, Louis F, Sawai S, Seki M, Numajiri T, et al. Direct conversion of human fibroblasts into adipocytes using a novel small molecular compound: implications for regenerative therapy for adipose tissue defects. Cells. (2021) 10. doi: 10.3390/cells10030605
89. Plikus MV, Guerrero-Juarez CF, Ito M, Li YR, Dedhia PH, Zheng Y, et al. Regeneration of fat cells from myofibroblasts during wound healing. Sci (New York NY). (2017) 355:748–52. doi: 10.1126/science.aai8792
90. Hwang S, Chung KW. Targeting fatty acid metabolism for fibrotic disorders. Arch Pharmacal Res. (2021) 44:839–56. doi: 10.1007/s12272-021-01352-4
91. Berenbaum F, Griffin TM, Liu-Bryan R. Review: metabolic regulation of inflammation in osteoarthritis. Arthritis Rheumatol (Hoboken NJ). (2017) 69:9–21. doi: 10.1002/art.39842
92. Collins KH, Lenz KL, Pollitt EN, Ferguson D, Hutson I, Springer LE, et al. Adipose tissue is a critical regulator of osteoarthritis. Proc Natl Acad Sci United States America. (2021) 118. doi: 10.1073/pnas.2021096118
93. Yusuf E, Nelissen RG, Ioan-Facsinay A, Stojanovic-Susulic V, DeGroot J, van Osch G, et al. Association between weight or body mass index and hand osteoarthritis: a systematic review. Ann rheumatic diseases. (2010) 69:761–5. doi: 10.1136/ard.2008.106930
94. Harasymowicz NS, Clement ND, Azfer A, Burnett R, Salter DM, Simpson A. Regional differences between perisynovial and infrapatellar adipose tissue depots and their response to class II and class III obesity in patients with osteoarthritis. Arthritis Rheumatol (Hoboken NJ). (2017) 69:1396–406. doi: 10.1002/art.40102
95. Warmink K, Kozijn AE, Bobeldijk I, Stoop R, Weinans H, Korthagen NM. High-fat feeding primes the mouse knee joint to develop osteoarthritis and pathologic infrapatellar fat pad changes after surgically induced injury. Osteoarthritis cartilage. (2020) 28:593–602. doi: 10.1016/j.joca.2020.03.008
96. Griffin TM, Fermor B, Huebner JL, Kraus VB, Rodriguiz RM, Wetsel WC, et al. Diet-induced obesity differentially regulates behavioral, biomechanical, and molecular risk factors for osteoarthritis in mice. Arthritis Res Ther. (2010) 12:R130. doi: 10.1186/ar3068
97. Wirth W. Joint-adjacent subcutaneous adipose tissue - An obesity-related imaging biomarker associated with structural osteoarthritis progression? Osteoarthritis cartilage. (2023) 31:1420–2. doi: 10.1016/j.joca.2023.08.002
98. Shen W, Middleton MS, Cunha GM, Delgado TI, Wolfson T, Gamst A, et al. Changes in abdominal adipose tissue depots assessed by MRI correlate with hepatic histologic improvement in non-alcoholic steatohepatitis. J hepatology. (2023) 78:238–46. doi: 10.1016/j.jhep.2022.10.027
99. Leven AS, Gieseler RK, Schlattjan M, Schreiter T, Niedergethmann M, Baars T, et al. Association of cell death mechanisms and fibrosis in visceral white adipose tissue with pathological alterations in the liver of morbidly obese patients with NAFLD. Adipocyte. (2021) 10:558–73. doi: 10.1080/21623945.2021.1982164
100. Wang GX, Zhao XY, Meng ZX, Kern M, Dietrich A, Chen Z, et al. The brown fat-enriched secreted factor Nrg4 preserves metabolic homeostasis through attenuation of hepatic lipogenesis. Nat Med. (2014) 20:1436–43. doi: 10.1038/nm.3713
101. Mendez-Sanchez N, Cruz-Ramon VC, Ramirez-Perez OL, Hwang JP, Barranco-Fragoso B, Cordova-Gallardo J. New aspects of lipotoxicity in nonalcoholic steatohepatitis. Int J Mol Sci. (2018) 19. doi: 10.3390/ijms19072034
102. Yu H, Jiang X, Dong F, Zhang F, Ji X, Xue M, et al. Lipid accumulation-induced hepatocyte senescence regulates the activation of hepatic stellate cells through the Nrf2-antioxidant response element pathway. Exp Cell Res. (2021) 405:112689. doi: 10.1016/j.yexcr.2021.112689
103. Liu B, Xiang L, Ji J, Liu W, Chen Y, Xia M, et al. Sparcl1 promotes nonalcoholic steatohepatitis progression in mice through upregulation of CCL2. J Clin Invest. (2021) 131. doi: 10.1172/JCI144801
104. Kamada Y, Matsumoto H, Tamura S, Fukushima J, Kiso S, Fukui K, et al. Hypoadiponectinemia accelerates hepatic tumor formation in a nonalcoholic steatohepatitis mouse model. J hepatology. (2007) 47:556–64. doi: 10.1016/j.jhep.2007.03.020
105. Lauby-Secretan B, Scoccianti C, Loomis D, Grosse Y, Bianchini F, Straif K. Body fatness and cancer–viewpoint of the IARC working group. New Engl J Med. (2016) 375:794–8. doi: 10.1056/NEJMsr1606602
106. Chen DC, Chung YF, Yeh YT, Chaung HC, Kuo FC, Fu OY, et al. Serum adiponectin and leptin levels in Taiwanese breast cancer patients. Cancer letters. (2006) 237:109–14. doi: 10.1016/j.canlet.2005.05.047
107. Blücher C, Iberl S, Schwagarus N, Müller S, Liebisch G, Höring M, et al. Secreted factors from adipose tissue reprogram tumor lipid metabolism and induce motility by modulating PPARα/ANGPTL4 and FAK. Mol Cancer Res MCR. (2020) 18:1849–62. doi: 10.1158/1541-7786.MCR-19-1223
108. Parrales A, Ranjan A, Iwakuma T. Unsaturated fatty acids regulate stemness of ovarian cancer cells through NF-κB. Stem Cell Invest. (2017) 4:49. doi: 10.21037/sci.2017.05.07
109. Dottino JA, Zhang Q, Loose DS, Fellman B, Melendez BD, Borthwick MS, et al. Endometrial biomarkers in premenopausal women with obesity: an at-risk cohort. Am J obstetrics gynecology. (2021) 224:278.e1–.e14. doi: 10.1016/j.ajog.2020.08.053
110. Nelson LR, Bulun SE. Estrogen production and action. J Am Acad Dermatol. (2001) 45:S116–24. doi: 10.1067/mjd.2001.117432
111. Gao J, Tian J, Lv Y, Shi F, Kong F, Shi H, et al. Leptin induces functional activation of cyclooxygenase-2 through JAK2/STAT3, MAPK/ERK, and PI3K/AKT pathways in human endometrial cancer cells. Cancer science. (2009) 100:389–95. doi: 10.1111/j.1349-7006.2008.01053.x
112. Incio J, Liu H, Suboj P, Chin SM, Chen IX, Pinter M, et al. Obesity-induced inflammation and desmoplasia promote pancreatic cancer progression and resistance to chemotherapy. Cancer discovery. (2016) 6:852–69. doi: 10.1158/2159-8290.CD-15-1177
113. Fearon K, Strasser F, Anker SD, Bosaeus I, Bruera E, Fainsinger RL, et al. Definition and classification of cancer cachexia: an international consensus. Lancet Oncol. (2011) 12:489–95. doi: 10.1016/S1470-2045(10)70218-7
114. Alves MJ, Figuerêdo RG, Azevedo FF, Cavallaro DA, Neto NI, Lima JD, et al. Adipose tissue fibrosis in human cancer cachexia: the role of TGFβ pathway. BMC cancer. (2017) 17:190. doi: 10.1186/s12885-017-3178-8
115. Kobayashi H, Enomoto A, Woods SL, Burt AD, Takahashi M, Worthley DL. Cancer-associated fibroblasts in gastrointestinal cancer. Nat Rev Gastroenterol hepatology. (2019) 16:282–95. doi: 10.1038/s41575-019-0115-0
116. Okumura T, Ohuchida K, Kibe S, Iwamoto C, Ando Y, Takesue S, et al. Adipose tissue-derived stromal cells are sources of cancer-associated fibroblasts and enhance tumor progression by dense collagen matrix. Int J cancer. (2019) 144:1401–13. doi: 10.1002/ijc.31775
117. Deng T, Lyon CJ, Bergin S, Caligiuri MA, Hsueh WA. Obesity, inflammation, and cancer. Annu Rev pathology. (2016) 11:421–49. doi: 10.1146/annurev-pathol-012615-044359
118. January CT, Wann LS, Calkins H, Chen LY, Cigarroa JE, Cleveland JC Jr., et al. 2019 AHA/ACC/HRS focused update of the 2014 AHA/ACC/HRS guideline for the management of patients with atrial fibrillation: A report of the american college of cardiology/american heart association task force on clinical practice guidelines and the heart rhythm society in collaboration with the society of thoracic surgeons. Circulation. (2019) 140:e125–e51. doi: 10.1161/CIR.0000000000000665
119. Krishnan A, Chilton E, Raman J, Saxena P, McFarlane C, Trollope AF, et al. Are interactions between epicardial adipose tissue, cardiac fibroblasts and cardiac myocytes instrumental in atrial fibrosis and atrial fibrillation? Cells. (2021) 10. doi: 10.3390/cells10092501
120. Ishii Y, Abe I, Kira S, Harada T, Takano M, Oniki T, et al. Detection of fibrotic remodeling of epicardial adipose tissue in patients with atrial fibrillation: Imaging approach based on histological observation. Heart Rhythm O2. (2021) 2:311–23. doi: 10.1016/j.hroo.2021.05.006
121. Coronel R. The pro- or antiarrhythmic actions of polyunsaturated fatty acids and of cholesterol. Pharmacol Ther. (2017) 176:40–7. doi: 10.1016/j.pharmthera.2017.02.004
122. Nalliah CJ, Bell JR, Raaijmakers AJA, Waddell HM, Wells SP, Bernasochi GB, et al. Epicardial adipose tissue accumulation confers atrial conduction abnormality. J Am Coll Cardiol. (2020) 76:1197–211. doi: 10.1016/j.jacc.2020.07.017
123. Patni N, Vuitch F, Garg A. Postmortem findings in a young man with congenital generalized lipodystrophy, type 4 due to CAVIN1 mutations. J Clin Endocrinol Metab. (2019) 104:957–60. doi: 10.1210/jc.2018-01331
124. Abe I, Teshima Y, Kondo H, Kaku H, Kira S, Ikebe Y, et al. Association of fibrotic remodeling and cytokines/chemokines content in epicardial adipose tissue with atrial myocardial fibrosis in patients with atrial fibrillation. Heart rhythm. (2018) 15:1717–27. doi: 10.1016/j.hrthm.2018.06.025
125. Venteclef N, Guglielmi V, Balse E, Gaborit B, Cotillard A, Atassi F, et al. Human epicardial adipose tissue induces fibrosis of the atrial myocardium through the secretion of adipo-fibrokines. Eur Heart J. (2015) 36:795–805a. doi: 10.1093/eurheartj/eht099
126. Zeller J, Krüger C, Lamounier-Zepter V, Sag S, Strack C, Mohr M, et al. The adipo-fibrokine activin A is associated with metabolic abnormalities and left ventricular diastolic dysfunction in obese patients. ESC Heart failure. (2019) 6:362–70. doi: 10.1002/ehf2.12409
127. Packer M. Epicardial adipose tissue may mediate deleterious effects of obesity and inflammation on the myocardium. J Am Coll Cardiol. (2018) 71:2360–72. doi: 10.1016/j.jacc.2018.03.509
128. Naftali-Shani N, Itzhaki-Alfia A, Landa-Rouben N, Kain D, Holbova R, Adutler-Lieber S, et al. The origin of human mesenchymal stromal cells dictates their reparative properties. J Am Heart Assoc. (2013) 2:e000253. doi: 10.1161/JAHA.113.000253
129. Mohammed SF, Hussain S, Mirzoyev SA, Edwards WD, Maleszewski JJ, Redfield MM. Coronary microvascular rarefaction and myocardial fibrosis in heart failure with preserved ejection fraction. Circulation. (2015) 131:550–9. doi: 10.1161/CIRCULATIONAHA.114.009625
130. Obokata M, Reddy YNV, Pislaru SV, Melenovsky V, Borlaug BA. Evidence supporting the existence of a distinct obese phenotype of heart failure with preserved ejection fraction. Circulation. (2017) 136:6–19. doi: 10.1161/CIRCULATIONAHA.116.026807
131. Patel K, Bajaj N, Statton B, Li X, Herath NS, Nyamakope K, et al. Bariatric surgery reverses ventricular repolarisation heterogeneity in obesity: mechanistic insights into fat-related arrhythmic risk. Eur Heart J. (2022) 43:ehac544.658. doi: 10.1093/eurheartj/ehac544.658
132. Rosa I, Romano E, Fioretto BS, Manetti M. The contribution of mesenchymal transitions to the pathogenesis of systemic sclerosis. Eur J Rheumatol. (2020) 7:S157–s64. doi: 10.5152/eurjrheum.2019.19081
133. Marangoni RG, Lu TT. The roles of dermal white adipose tissue loss in scleroderma skin fibrosis. Curr Opin Rheumatol. (2017) 29:585–90. doi: 10.1097/BOR.0000000000000437
134. Ohgo S, Hasegawa S, Hasebe Y, Mizutani H, Nakata S, Akamatsu H. Bleomycin inhibits adipogenesis and accelerates fibrosis in the subcutaneous adipose layer through TGF-β1. Exp Dermatol. (2013) 22:769–71. doi: 10.1111/exd.12256
135. Korman B, Marangoni RG, Lord G, Olefsky J, Tourtellotte W, Varga J. Adipocyte-specific repression of PPAR-gamma by NCoR contributes to scleroderma skin fibrosis. Arthritis Res Ther. (2018) 20:145. doi: 10.1186/s13075-018-1630-z
136. Rosa I, Romano E, Fioretto BS, Matucci-Cerinic M, Manetti M. Adipose-derived stem cells: Pathophysiologic implications vs therapeutic potential in systemic sclerosis. World J Stem Cells. (2021) 13:30–48. doi: 10.4252/wjsc.v13.i1.30
137. Strong AL, Rubin JP, Kozlow JH, Cederna PS. Fat grafting for the treatment of scleroderma. Plast reconstructive surgery. (2019) 144:1498–507. doi: 10.1097/PRS.0000000000006291
138. Liu B, Page AJ, Hatzinikolas G, Chen M, Wittert GA, Heilbronn LK. Intermittent fasting improves glucose tolerance and promotes adipose tissue remodeling in male mice fed a high-fat diet. Endocrinology. (2019) 160:169–80. doi: 10.1210/en.2018-00701
139. Wang L, Ye X, Hua Y, Song Y. Berberine alleviates adipose tissue fibrosis by inducing AMP-activated kinase signaling in high-fat diet-induced obese mice. Biomedicine pharmacotherapy = Biomedecine pharmacotherapie. (2018) 105:121–9. doi: 10.1016/j.biopha.2018.05.110
140. Lontchi-Yimagou E, Kang S, Goyal A, Zhang K, You JY, Carey M, et al. Insulin-sensitizing effects of vitamin D repletion mediated by adipocyte vitamin D receptor: Studies in humans and mice. Mol Metab. (2020) 42:101095. doi: 10.1016/j.molmet.2020.101095
141. Alcalá M, Sánchez-Vera I, Sevillano J, Herrero L, Serra D, Ramos MP, et al. Vitamin E reduces adipose tissue fibrosis, inflammation, and oxidative stress and improves metabolic profile in obesity. Obes (Silver Spring Md). (2015) 23:1598–606. doi: 10.1002/oby.21135
142. Ikeda K, Kang Q, Yoneshiro T, Camporez JP, Maki H, Homma M, et al. UCP1-independent signaling involving SERCA2b-mediated calcium cycling regulates beige fat thermogenesis and systemic glucose homeostasis. Nat Med. (2017) 23:1454–65. doi: 10.1038/nm.4429
143. McDonald ME, Li C, Bian H, Smith BD, Layne MD, Farmer SR. Myocardin-related transcription factor A regulates conversion of progenitors to beige adipocytes. Cell. (2015) 160:105–18. doi: 10.1016/j.cell.2014.12.005
144. Wang W, Ishibashi J, Trefely S, Shao M, Cowan AJ, Sakers A, et al. A PRDM16-driven metabolic signal from adipocytes regulates precursor cell fate. Cell Metab. (2019) 30:174–89.e5. doi: 10.1016/j.cmet.2019.05.005
145. Hasegawa Y, Ikeda K, Chen Y, Alba DL, Stifler D, Shinoda K, et al. Repression of adipose tissue fibrosis through a PRDM16-GTF2IRD1 complex improves systemic glucose homeostasis. Cell Metab. (2018) 27:180–94.e6. doi: 10.1016/j.cmet.2017.12.005
146. Zhao B, Hu M, Wu H, Ren C, Chen J, Zhang X, et al. Peroxisome proliferator-activated receptor-γ and its related pathway in bone marrow mesenchymal stem cell differentiation co-cultured with mechanically stretched ligament fibroblasts. Int J Mol Med. (2018) 42:219–27. doi: 10.3892/ijmm.2018.3578
147. Pan JA, Lin H, Yu JY, Zhang HL, Zhang JF, Wang CQ, et al. MiR-21-3p inhibits adipose browning by targeting FGFR1 and aggravates atrial fibrosis in diabetes. Oxid Med Cell longevity. (2021) 2021:9987219. doi: 10.1155/2021/9987219
148. Ruzehaji N, Frantz C, Ponsoye M, Avouac J, Pezet S, Guilbert T, et al. Pan PPAR agonist IVA337 is effective in prevention and treatment of experimental skin fibrosis. Ann rheumatic diseases. (2016) 75:2175–83. doi: 10.1136/annrheumdis-2015-208029
149. Guo F, Seldin M, Péterfy M, Charugundla S, Zhou Z, Lee SD, et al. NOTUM promotes thermogenic capacity and protects against diet-induced obesity in male mice. Sci Rep. (2021) 11:16409. doi: 10.1038/s41598-021-95720-1
150. Li B, Po SS, Zhang B, Bai F, Li J, Qin F, et al. Metformin regulates adiponectin signalling in epicardial adipose tissue and reduces atrial fibrillation vulnerability. J Cell Mol Med. (2020) 24:7751–66. doi: 10.1111/jcmm.15407
151. Guzmán-Ruiz R, Tercero-Alcázar C, López-Alcalá J, Sánchez-Ceinos J, Malagón MM, Gordon A. The potential role of the adipokine HMGB1 in obesity and insulin resistance. Novel effects adipose Tissue Biol Mol Cell endocrinology. (2021) 536:111417. doi: 10.1016/j.mce.2021.111417
152. Kulkarni AS, Brutsaert EF, Anghel V, Zhang K, Bloomgarden N, Pollak M, et al. Metformin regulates metabolic and nonmetabolic pathways in skeletal muscle and subcutaneous adipose tissues of older adults. Aging Cell. (2018) 17. doi: 10.1111/acel.12723
153. Biondo LA, Batatinha HA, Souza CO, Teixeira AAS, Silveira LS, Alonso-Vale MI, et al. Metformin mitigates fibrosis and glucose intolerance induced by doxorubicin in subcutaneous adipose tissue. Front Pharmacol. (2018) 9:452. doi: 10.3389/fphar.2018.00452
154. Bril F, Kalavalapalli S, Clark VC, LoMonaco R, Soldevila-Pico C, Liu IC, et al. Response to pioglitazone in patients with nonalcoholic steatohepatitis with vs without type 2 diabetes. Clin Gastroenterol Hepatol Off Clin Pract J Am Gastroenterological Assoc. (2018) 16:558–66.e2. doi: 10.1016/j.cgh.2017.12.001
155. Wu M, Melichian DS, Chang E, Warner-Blankenship M, Ghosh AK, Varga J. Rosiglitazone abrogates bleomycin-induced scleroderma and blocks profibrotic responses through peroxisome proliferator-activated receptor-gamma. Am J pathology. (2009) 174:519–33. doi: 10.2353/ajpath.2009.080574
156. Ren Y, Du C, Shi Y, Wei J, Wu H, Cui H. The Sirt1 activator, SRT1720, attenuates renal fibrosis by inhibiting CTGF and oxidative stress. Int J Mol Med. (2017) 39:1317–24. doi: 10.3892/ijmm.2017.2931
157. Han W, Wang C, Yang Z, Mu L, Wu M, Chen N, et al. SRT1720 retards renal fibrosis via inhibition of HIF1α /GLUT1 in diabetic nephropathy. J endocrinology. (2019) 241(1):85–98. doi: 10.1530/JOE-18-0536
158. Wang S, Zhou Y, Zhang Y, He X, Zhao X, Zhao H, et al. Roscovitine attenuates renal interstitial fibrosis in diabetic mice through the TGF-β1/p38 MAPK pathway. Biomedicine pharmacotherapy = Biomedecine pharmacotherapie. (2019) 115:108895. doi: 10.1016/j.biopha.2019.108895
159. Albright V, Xu M, Palanisamy A, Cheng J, Stack M, Zhang B, et al. Micelle-coated, hierarchically structured nanofibers with dual-release capability for accelerated wound healing and infection control. Advanced healthcare materials. (2018) 7:e1800132. doi: 10.1002/adhm.201800132
160. Nielsen KN, Peics J, Ma T, Karavaeva I, Dall M, Chubanava S, et al. NAMPT-mediated NAD(+) biosynthesis is indispensable for adipose tissue plasticity and development of obesity. Mol Metab. (2018) 11:178–88. doi: 10.1016/j.molmet.2018.02.014
161. Koh EH, Chernis N, Saha PK, Xiao L, Bader DA, Zhu B, et al. miR-30a remodels subcutaneous adipose tissue inflammation to improve insulin sensitivity in obesity. Diabetes. (2018) 67:2541–53. doi: 10.2337/db17-1378
Keywords: obesity, fibrosis, adipocytes, fibroblasts, AMT
Citation: Zhang Q, Lu C, Lu F, Liao Y, Cai J and Gao J (2024) Challenges and opportunities in obesity: the role of adipocytes during tissue fibrosis. Front. Endocrinol. 15:1365156. doi: 10.3389/fendo.2024.1365156
Received: 03 January 2024; Accepted: 01 April 2024;
Published: 15 April 2024.
Edited by:
Julianne Hall, Quinnipiac University, United StatesReviewed by:
Suchandan Sikder, James Cook University, AustraliaJunfei Zhao, Guangdong Academy of Medical Sciences, China
Copyright © 2024 Zhang, Lu, Lu, Liao, Cai and Gao. This is an open-access article distributed under the terms of the Creative Commons Attribution License (CC BY). The use, distribution or reproduction in other forums is permitted, provided the original author(s) and the copyright owner(s) are credited and that the original publication in this journal is cited, in accordance with accepted academic practice. No use, distribution or reproduction is permitted which does not comply with these terms.
*Correspondence: Junrong Cai, drjunrongcai@outlook.com; Jianhua Gao, doctorgaojianhua@outlook.com
†ORCID: Qian Zhang, orcid.org/0000-0002-8994-8281
Feng Lu, orcid.org/0000-0002-4150-4366
Yunjun Liao, orcid.org/0000-0001-5264-3962
Junrong Cai, orcid.org/0000-0002-5314-0264
Jianhua Gao, orcid.org/0000-0001-9453-1993