CRISPR-Based Approaches for Gene Regulation in Non-Model Bacteria
- 1Department of Chemical Engineering, University of Massachusetts Amherst, Amherst, MA, United States
- 2Biotechnology Training Program, University of Massachusetts Amherst, Amherst, MA, United States
- 3Molecular and Cellular Biology Graduate Program, University of Massachusetts Amherst, Amherst, MA, United States
CRISPR interference (CRISPRi) and CRISPR activation (CRISPRa) have become ubiquitous approaches to control gene expression in bacteria due to their simple design and effectiveness. By regulating transcription of a target gene(s), CRISPRi/a can dynamically engineer cellular metabolism, implement transcriptional regulation circuitry, or elucidate genotype-phenotype relationships from smaller targeted libraries up to whole genome-wide libraries. While CRISPRi/a has been primarily established in the model bacteria Escherichia coli and Bacillus subtilis, a growing numbering of studies have demonstrated the extension of these tools to other species of bacteria (here broadly referred to as non-model bacteria). In this mini-review, we discuss the challenges that contribute to the slower creation of CRISPRi/a tools in diverse, non-model bacteria and summarize the current state of these approaches across bacterial phyla. We find that despite the potential difficulties in establishing novel CRISPRi/a in non-model microbes, over 190 recent examples across eight bacterial phyla have been reported in the literature. Most studies have focused on tool development or used these CRISPRi/a approaches to interrogate gene function, with fewer examples applying CRISPRi/a gene regulation for metabolic engineering or high-throughput screens and selections. To date, most CRISPRi/a reports have been developed for common strains of non-model bacterial species, suggesting barriers remain to establish these genetic tools in undomesticated bacteria. More efficient and generalizable methods will help realize the immense potential of programmable CRISPR-based transcriptional control in diverse bacteria.
Introduction
Since the development of CRISPR interference (CRISPRi) (Qi et al., 2013) and CRISPR activation (CRISPRa) (Bikard et al., 2013) in 2013, they have become efficient and prevalent tools for transcriptional regulation in bacteria. CRISPR-Cas originates as a form of prokaryotic immunity, with systems comprising one or more CRISPR-associated (Cas) proteins and a short guide RNA (gRNA) that complex together to target and cleave foreign DNA or RNA molecules, such as viruses (Nussenzweig and Marraffini, 2020). The gRNA leads the complex to target sequence via complementarity between the protospacer sequence of the gRNA and the target site on the DNA/RNA molecule. Various mechanisms exist to prevent cleavage of chromosomal DNA, which most often involves a protospacer adjacent motif (PAM) or equivalent next to the target site that is not present in the CRISPR arrays on the chromosome (Jackson et al., 2017).
Researchers developed CRISPRi technology by deactivating the nuclease activity of select Cas enzymes to create mutant dCas proteins that bind, but do not cleave, the DNA target (Qi et al., 2013). Most CRISPRi systems repress a gene’s expression through steric inhibition of RNA polymerase binding or extension (Qi et al., 2013), although some repress gene expression through RNA cleavage (Zhang K. et al., 2020; Rahman et al., 2021). Gene repression over 100-fold has been reported for several diverse CRISPRi tools and can approach near knockout levels of gene expression (Qi et al., 2013; Miao et al., 2019). Targeting a different sequence is easily achieved by changing the short protospacer sequence on the gRNA to bind a location within the promoter, untranslated region, or coding sequence of the target gene based on simple design rules (Qi et al., 2013; Zetsche et al., 2015; Zhang et al., 2017). Additionally, multiplexed gene repression can be achieved by simply expressing multiple gRNA within a cell (Qi et al., 2013; Zhang et al., 2017).
Shortly after the development of CRISPRi, researchers developed CRISPRa for bacterial transcriptional activation by combining the dCas protein with a transcriptional activator that recruits transcription machinery to the target gene’s promoter to increase gene expression (Bikard et al., 2013). The specific mechanism for transcriptional activation depends on the activator, which can be incorporated by directly fusing a transcriptional activator domain to the dCas protein (Bikard et al., 2013; Ho et al., 2020; Schilling et al., 2020), incorporating RNA scaffolds into the gRNA sequence to recruit activator domains to the dCas complex (Dong et al., 2018; Liu Y. et al., 2019; Fontana et al., 2020a), or using non-covalent protein-protein interaction domains to complex the transcriptional activator and dCas protein (Villegas Kcam et al., 2021; Villegas Kcam et al., 2022). Unlike CRISPRi, however, CRISPRa has complex design rules that often strongly depend on the CRISPRa technology (i.e., type of activation domain and approach to couple the activation domain and dCas complex) as wells as several other factors (Liu Y. et al., 2019; Fontana et al., 2020a; Ho et al., 2020; Villegas Kcam et al., 2021). These other considerations include the basal expression of the target gene and the location of the binding site for the CRISPRa complex, where activation is typically achieved in a narrow range upstream of the target gene’s promoter and the activation strength fluctuates sharply as the nucleotide position shifts. Combined with the PAM requirement for DNA binding, these requirements greatly restrict the available DNA target sites for effective gene activation, especially for endogenous genes. Due to these relatively stringent design rules for gene activation and often low (<10-fold) activation levels compared to CRISPRi repression (Bikard et al., 2013; Liu W. et al., 2019; Fontana et al., 2020a; Villegas Kcam et al., 2021), CRISPRa development has been slower in bacteria than in eukaryotes (Kampmann, 2018; Fontana et al., 2020b). Despite the current limitations of CRISPRa, however, the simplicity and inherent properties of CRISPRi/a gene regulation can provide strong transcriptional control of multiple genes simultaneously, making these approaches often easier and faster than traditional methods and allowing for dynamic transcriptional control.
CRISPR systems are classified into a variety of classes, types, subtypes, and variants, each with unique genes and properties (Koonin et al., 2017; Makarova et al., 2020). Many systems have been engineered to create effective CRISPRi/a tools. The first and most common tool is derived from the Type II Cas9 system, which comprises a single deactivated Cas9 (dCas9) protein and two small RNAs that create the gRNA (Qi et al., 2013). These two RNAs can be combined into a synthetic single guide RNA (sgRNA) for easier synthesis, but each sgRNA requires an independent promoter for expression (Jiang et al., 2015). Although many different dCas9 variants exist, the Streptococcus pyogenes dCas9 (Sp dCas9) system is the most common due to its short PAM sequence and strong transcription regulation abilities. Recently, tools derived from the Type V Cas12a (formerly Cpf1) system have been developed, which uses a single deactivated Cas12a (dCas12a) protein and one gRNA (Zetsche et al., 2015; Kim et al., 2017; Zhang et al., 2017; Miao et al., 2019). Unlike dCas9, dCas12a can process its gRNA from CRISPR arrays, providing easier multiplexed regulation (Fonfara et al., 2016; Zhang et al., 2017). Additionally, several studies have suggested that dCas12a variants are less toxic than dCas9 variants across different bacterial phyla (Liu W. et al., 2019; Knoot et al., 2020; Kuo et al., 2020), making them an attractive alternative to dCas9. The most common dCas12a variants used in bacteria are derived from Francisella tularensis subsp. novicida (Fn dCas12a) and Acidaminococcus sp. BV3L6 (As dCas12a). Several Type I CRISPRi/a tools have been designed, but due to the large number of genes in these systems, most tools are implemented by reprogramming the host species’ endogenous CRISPR system for gene repression (Luo et al., 2015; Xu et al., 2021; Villegas Kcam et al., 2022). Only a handful of CRISPRi tools from other systems have been reported for transcriptional regulation in bacteria, likely due to the novelty of the system (Rahman et al., 2021) or high cellular toxicity observed upon expression (Zhang K. et al., 2020).
Despite the unique traits and relevance of a vast diversity of bacteria, CRISPRi/a tools have been primarily developed in the model bacteria Escherichia coli and Bacillus subtilis. Yet, non-model bacteria (a broad definition of non-model, excluding E. coli and B. subtilis, is used here) offer great promise in research and industry spanning a wide range of medical, environmental, and biomanufacturing applications. For example, Streptomyces, Sorangium, and Photorhabdus spp. naturally produce bioactive secondary metabolites, such as antibiotics, and contain silent biosynthetic gene clusters with unknown and potentially useful products (Ye et al., 2019; Tian et al., 2020; Ke et al., 2021). Additionally, Rhodococcus and Corynebacterium spp. can produce valuable chemicals from cheap and simple feedstock and are tolerant to harsh conditions, making them ideal cell factories (Cleto et al., 2016; DeLorenzo et al., 2018). However, several conditions must be reached to successfully establish efficient CRISPRi/a tools in a non-model bacterium. In this mini-review, we detail these criteria, emphasizing the importance of characterized genetic parts to tightly control the expression of CRISPRi/a systems to limit potential toxicity while providing sufficient expression for effective transcriptional control. We demonstrate that despite the potential difficulties in creating these tools in non-model bacteria, they have been established across eight different bacterial phyla and have been used for a variety of applications, including high-throughput genome-wide selections. Finally, we highlight the current challenges to developing CRISPRi/a tools in non-model bacteria and novel species, which suggest directions for future progress.
Requirements and Challenges to Establish CRISPRi/a in Non-Model Bacteria
Several criteria must be met to successfully establish an effective CRISPRi/a tool in a non-model species or strain. First, the conditions for culturing, maintaining, and genetically manipulating the strain (often referred to as strain “domestication”) must be determined. For a phylogenetically similar strain to a previously established model bacteria, such as many Bacillus species (Zhan et al., 2020) and Enterobacteriaceae (Ho et al., 2020), suitable culture conditions may be similar to those previously determined. For novel or fastidious species, however, trial and error and patience may be required to determine appropriate culture conditions for growth and genetic manipulation, such as the obligate intracellular pathogen Chlamydia trachomatis (Ouellette, 2018). Additionally, introducing foreign DNA is often challenging for a non-model bacterium, as many are genetically recalcitrant, especially pathogens (Fernandes et al., 2021b) and novel strains (Zhao et al., 2020; Jin et al., 2022), and establishing a sufficient genetic transformation method can require significant effort. Additionally, care must be taken when introducing synthetic DNA to circumvent the bacterial host’s native immunity that may degrade foreign DNA, including restriction-modification and CRISPR systems (Marraffini and Sontheimer, 2008; Jin et al., 2022), such as by mimicking the recipient strain’s methylation patterns (Monk et al., 2015; Zhao et al., 2020). More discussion on the isolation and domestication of non-model bacteria can be found in other reviews (Vartoukian et al., 2010; Lewis et al., 2021; Riley and Guss, 2021).
Next, reliable genetic parts for the non-model bacterium are required to be able to express and tightly control the CRISPRi/a tool, including promoters, ribosome binding sites, terminators, and expression or integration vectors. For many non-model bacteria, especially novel species, these genetic part libraries are unavailable, and so, the necessary genetic parts must be created and characterized. In some cases, established genetic parts may be transferable from a model bacterium to a related species, such as promoters between Gram-positive bacteria (Liew et al., 2010). However, genetic parts often do not function equivalently between bacterial species or even strains (Tong et al., 2015; Leonard et al., 2018). Each CRISPRi/a component should be expressed using unique genetic parts to prevent repeated DNA sequences. Since dCas protein expression can elicit cytotoxicity, high strength promoters used for overexpression may not be optimal. If existing genetic parts are insufficient for a new bacterial species, identifying genetic regulatory elements from the endogenous genome provides an alternative to synthetic DNA design strategies (Fernandes et al., 2019). Libraries of genetic parts and inducible promoters are excellent tools to tune the expression of CRISPRi/a systems, and several studies have established such toolboxes in non-model bacteria to facilitate the development of genetic tools such as CRISPRi/a (Mimee et al., 2015; Leonard et al., 2018; Shin et al., 2019; Teh et al., 2019; Liow et al., 2020). These libraries and tunable parts are especially important to control the expression of the CRISPRi/a tool to minimize potential cellular toxicity and to precisely control transcriptional regulation (Qu et al., 2019; Bosch et al., 2021; Shabestary et al., 2021).
In the design of a synthetic CRISPRi/a system for a bacterium, consideration should be given to prevent interference with endogenous CRISPR systems and/or anti-CRISPR genes harbored on the strain’s genome. If the foreign and native CRISPR-Cas types are too similar, the introduction of the synthetic gRNA may induce cleavage of the host bacterium’s genome (via the catalytically active endogenous Cas enzyme) and can cause cell death in a DNA repair-deficient strain or undesired mutations if the strain has appropriate DNA repair pathways. This can be avoided by choosing a CRISPRi/a tool that does not share significant homology to any endogenous CRISPR-Cas. Native CRISPR-Cas systems can be predicted from the sequenced genome or proteome using computer software (Couvin et al., 2018; Chai et al., 2019), aiding in CRISPRi/a tool selection for novel strains. Alternatively, the native system can be engineered to create a CRISPRi/a tool via genetic manipulation, such as the deletion of the native cas2/3 or cas3 gene responsible for cleavage in Type I-F systems (Zheng et al., 2019; Qin et al., 2021; Xu et al., 2021) or mutating the native cas9 sequence for Type II systems (Shields et al., 2020; Dammann et al., 2021). Anti-CRISPR proteins, which inhibit CRISPR systems through a variety of mechanisms (Pawluk et al., 2018), may require deletion or disruption before a heterologous CRISPRi/a tool can be expressed (Xu et al., 2021). Online tools and databases are available to predict and describe anti-CRISPR proteins from protein sequences to help select an appropriate CRISPRi/a system (Wang et al., 2020; Wang et al., 2021a).
Finally, the CRISPRi/a components should be expressed at a level that provides adequate transcriptional repression or activation for the given application without significant cellular toxicity. Many studies have reported CRISPRi toxicity for diverse bacteria, while little is known about CRISPRa toxicity due to limited reports in the literature. These observed forms of toxicity include changes in cell morphology (Cho et al., 2018; Ouellette et al., 2021) and slower growth or complete growth inhibition (Rock et al., 2017; Yu et al., 2018; Wurihan et al., 2019; Zhang K. et al., 2020; Brito et al., 2020). To prevent toxicity, one can use a less toxic CRISPRi/a system for the host species (Rock et al., 2017; Zhao et al., 2019), or reduce the expression of the components by substituting genetic parts (Qu et al., 2019). Expansive libraries of genetic parts, including inducible and constitutive promoters, ribosome binding sites, and protein degradation tags, can be used to tune gene expression and characteristics of the CRISPRi/a tool (Depardieu and Bikard, 2020; Fleck and Grundner, 2021; Ouellette et al., 2021). However, the components cannot be expressed so low that it cannot effectively repress or activate the target gene(s), especially during multiplexed gene regulation that relies on a shared dCas protein pool for multiple gRNAs (Zhang and Voigt, 2018; Zhao et al., 2018). A careful balance is required to express the CRISPRi/a components.
Current CRISPRi/a Tools for Non-Model Bacteria
Different CRISPRi/a tools have been established in a range of bacteria that span many phyla and have been used for a variety of applications, as summarized here (Table 1). Overwhelmingly, these studies have utilized the Sp dCas9 CRISPRi system. More detailed information for each study can be found in Supplementary Table S1 (Supplementary Data Sheet 1).
Actinomycetota
CRISPRi has been well established in a wide range of Actinobacteria, including Mycobacteria, Streptomyces, and Corynebacterium, and has been used for metabolic engineering and the elucidation of gene functions in both small studies and genome-wide screens (Table 1). Additionally, several CRISPRi tools are commonly used in Mycobacteria (Choudhary et al., 2015; Rock et al., 2017; Agarwal, 2020; Fleck and Grundner, 2021) and Streptomycetes (Tong et al., 2015; Li et al., 2018; Zhao et al., 2018). CRISPRa has also been recently establish in Corynebacterium (Liu W. et al., 2019) and Streptomycetes (Ameruoso et al., 2021).
Cyanobacteria
CRISPRi/a is especially useful in cyanobacteria due to their polyploidal genomes (Kirtania et al., 2019). CRISPRi is relatively well-established in a wide range of cyanobacterial species, including those of research and industrial significance, and has been used for metabolic engineering, transcriptional regulatory networks, and the study of gene functions in small studies and a genome-wide screen/selection (Table 1). Many CRISPRi tools are available in cyanobacteria, each with their own characteristics (Gordon et al., 2016; Yao et al., 2016; Liu D. et al., 2020; Choi and Woo, 2020). CRISPRa has not yet been reported in cyanobacteria.
Firmicutes
CRISPRi is well-established in a wide range of Firmicutes, including Bacilli, Clostridia, Staphylococci, and Streptococci (Table 1). CRISPRi tools have been developed and used for metabolic engineering, elucidation of gene functions, and genome-wide screens and selections. CRISPRa has been reported in Bacillus amyloliquefaciens and Paenibacillus polymyxa in tool development work and some metabolic engineering applications (Schilling et al., 2020; Zhao et al., 2020).
Proteobacteria
CRISPRi and CRISPRa are well established in a wide variety of Proteobacteria, including Klebsiella, Salmonella, Pseudomonas, and Vibrio (Table 1). These tools have been developed and used for metabolic engineering, synthetic transcriptional regulatory networks, and mapping gene function using small gRNA sets and genome-wide screens and selections. Reports of CRISPRi are far more common than CRISPRa.
Other Bacterial Phyla
CRISPRi has also been reported in the phyla Chlamydiae, Tenericutes, Spirochaetes, and Bacteroidetes (Table 1). Although these reports have primarily been for tool development, some have used CRISPRi to investigate gene function (Fernandes et al., 2021b; Brockett et al., 2021) or create synthetic genetic circuits (Mimee et al., 2015; Taketani et al., 2020).
Applications of CRISPRi/a in Non-Model Bacteria
CRISPRi/a tools can be used for a variety of applications in non-model bacteria (Figure 1). The most common application is mapping a gene’s function by altering its gene expression and assaying cellular phenotypic change under some applied selective condition (Figure 1A). CRISPRi is particularly useful for investigating essential genes because its repression can be titrated to prevent full knockdown and cell death (Knoot et al., 2020; Bosch et al., 2021). Additionally, epistatic effects of multiple genes can easily be investigation by simply expressing multiple gRNA within the same cell (Ellis et al., 2021; McNeil et al., 2022). Although not as common as CRISPRi due to stricter design rules (Fontana et al., 2020a), CRISPRa can be used to induce expression of silent genes to investigate their functions and products, including entire silent biosynthetic gene clusters (Ke et al., 2021). Combined, these are the most common use of CRISPRi/a tools in non-model bacteria, with 80 reports across six phyla (Table 1) (Behler et al., 2018; Stamsås et al., 2018; Ke et al., 2021). The recent development of Mobile-CRISPRi (Peters et al., 2019), CRAGE-CRISPR (Ke et al., 2021), and a workflow for introducing genetic manipulation tools into non-model gut bacteria (Jin et al., 2022) will facilitate the expansion of CRISPRi/a tools into new species and strains, including recalcitrate pathogens and novel species without sequenced genomes.
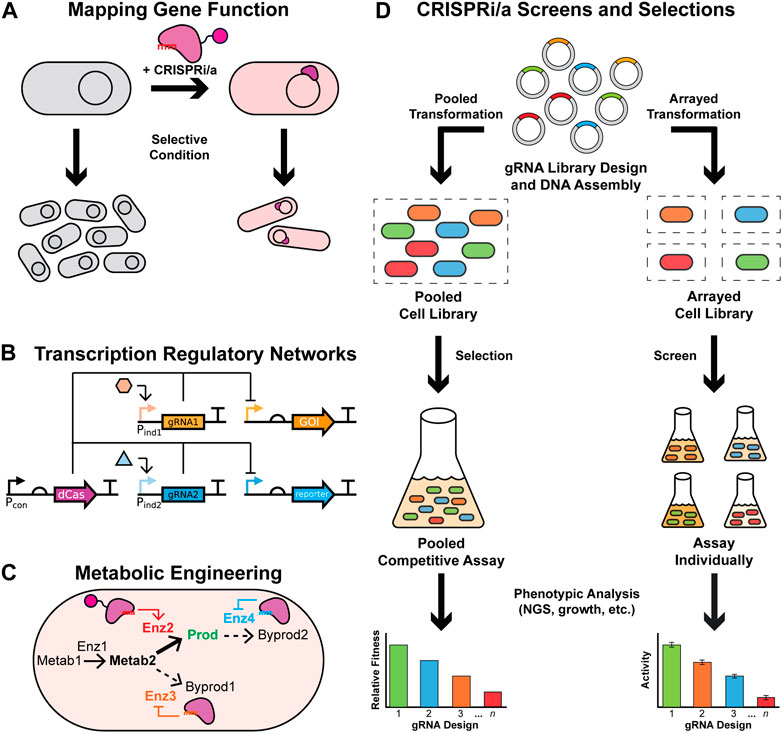
FIGURE 1. Applications of CRISPRi/a tools in bacteria. (A) CRISPRi/a tools can be used to study gene function by targeting a gene for repression or activation and assaying phenotypic differences under a given condition. (B) CRISPRi/a can control transcription regulatory networks through the expression of multiple guide RNAs and a single deactivated Cas (dCas) protein. By incorporating inducible promoters for the guide RNAs (gRNAs) and/or dCas protein, dynamic genetic circuits can be created that respond to multiple input stimuli to alter the expression of output genes, such as a gene of interest (GOI) and a reporter gene. (C) CRISPRi/a can be used for dynamic metabolic engineering by regulating gene expression to redirect carbon flux towards production of a desired biochemical, such as using CRISPRi to repress genes encoding competing enzymes (dashed arrows) while using CRISPRa to activate genes for the desired biochemical production (bold arrow). (D) CRISPRi/a has been used for high-throughput, large-scale screens and selections ranging from targeted sets of genes to genome-wide libraries. After designing and assembling the gRNA library for the given application, the library is transformed into bacteria that express the cognate dCas protein in either a mixed pooled (i.e., cells containing different gRNA(s) are mixed together) or an individual array format (i.e., cells containing different gRNA(s) are cultured separately). The resulting pooled cell library can be used in competitive selections for functional genomics analyses by applying selective conditions and measuring the relative gRNA fitness. An arrayed cell library can be assayed one-by-one to determine the relationship between strain activity (e.g. production or phenotype) and gene repression/activation.
Additionally, CRISPRi/a can be used to control transcription regulatory networks, such as genetic circuits, by designing and expressing gRNA to regulate the output promoter for each logic gate or node (Figure 1B). CRISPRi/a is especially effective for controlling complex synthetic transcription regulatory networks as the gRNA can be designed to target nearly any arbitrary sequence with an appropriate PAM (or equivalent) sequence (Taketani et al., 2020; Ellis et al., 2021). CRISPRi/a circuits can be fully synthetic and auxiliary to the native genetic regulatory networks, such as a heterologous sensor or multi-input circuit that senses and responds to external inputs in complex environments (Mimee et al., 2015; Taketani et al., 2020). Alternatively, CRISPRi/a can be interfaced with native gene regulatory systems to control the host’s metabolism in response to external stimuli, such as cell density, through either heterologous (Liu Y. et al., 2020) or even indigenous sensor systems (Tian et al., 2020). However, caution must be taken to prevent the expression of too many gRNA at once since they compete over the limited dCas protein resource and, thus, can decrease the repression of target genes (Del Vecchio et al., 2008; Li et al., 2018; Zhang and Voigt, 2018). Synthetic CRISPRi/a regulatory networks are rare in non-model bacteria, having been reported in only seven studies across four phyla, and primarily incorporate CRISPRi (Table 1). However, a single CRISPRa genetic circuit in Salmonella has been reported (Bhokisham et al., 2020).
CRISPRi/a tools have also been used to redirect carbon and energy flow for metabolic engineering in non-model bacteria (Figure 1C). CRISPRi is often used to repress a native gene(s), including essential genes, to redirect carbon flux towards a desired product (Wang et al., 2017; Shabestary et al., 2018) or bioactive molecule (Yu et al., 2018; Liu et al., 2021b). CRISPRa can be used to activate the desired metabolic pathway to increase biosynthesis of the desired product, such as an anti-cancer drug in a weakly-expressed biosynthetic gene cluster (Peng et al., 2018; Ye et al., 2019). In most examples, the CRISPRi/a components are constitutively expressed, yet some studies employ dynamic metabolic engineering strategies by utilizing inducible systems and/or genetically encoded biosensors to switch between cell growth and product biosynthesis states to improve production (Liu Y. et al., 2020; Tian et al., 2020; Shabestary et al., 2021). These tools can be used to tune endogenous metabolism and/or heterologous metabolic pathways (Peng et al., 2018; Banerjee et al., 2020). CRISPRi/a tools are most often combined with other metabolic engineering techniques, such as the deletion, overexpression, or mutation of select genes and optimization of medium, to further increase titers of the desired product (Park et al., 2019; Dietsch et al., 2021; Kozaeva et al., 2021).
Large-scale CRISPRi screens and selections have been developed to investigate genotype-phenotype relationships through gRNA fitness (Figure 1D). These assays can use small, targeted libraries, such as essential genes or genes in a metabolic pathway (Shields et al., 2020; Göttl et al., 2021), or large genome-wide libraries targeting nearly all genes in the bacterial genome (Lee et al., 2019; Jiang et al., 2020). Additionally, CRISPRi libraries can be constructed in two major forms—pooled libraries, where cells containing different gRNA are mixed during library construction (Bosch et al., 2021; Rahman et al., 2021), a strategy known as multiplexing, or arrayed libraries where different gRNA designs are constructed individually in different clonal populations, typically arrayed in microtiter plates (Liu et al., 2017; Göttl et al., 2021). Pooled competitive selections are more common due to the ease of DNA construction and analysis of large, genome-scale gRNA libraries with >10,000 designs by next-generation sequencing (Lee et al., 2019; Bosch et al., 2021). However, because all cells directly compete in pooled competitive growth assays, “cheaters” may arise that take advantage of different strain interactions, so the results of any individual gRNA design should be verified in isolation (Yao et al., 2020; Liu X. et al., 2021). Additionally, the results from these pooled CRISPRi screens or selections are specific to the gRNA design and not the target gene since confounding effects (i.e., off-target effects) could produce false positives or negatives, so careful design of gRNA libraries is vital (Cui et al., 2018; Wang T. et al., 2018). Genome-wide CRISPRi screens or selections are relatively uncommon (Table 1). While not demonstrated to date, genome-wide bacterial CRISPRa is theoretically possible, provided the design rules for activation are met (Fontana et al., 2020a).
Conclusion and Perspectives
CRISPRi has been established in non-model bacteria across eight phyla and applied from small, single gene functional studies to large genome-wide screens. The creation of new tools and protocols for introducing CRISPRi/a into non-model bacteria will facilitate the continuation of this rapid expansion. Several novel and exciting CRISPRa tools with greater activation and unique characteristics have been developed recently in both model and non-model bacteria, yet there remains a need for stronger and more versatile bacterial CRISPRa tools, especially for the activation of native genes. These bacterial CRISPRa tools have lagged behind the development of both eukaryotic CRISPRa tools and bacterial CRISPRi tools. However, the recent development of several new CRISPRa systems with less stringent design rules and higher levels of activation (>10-fold) shows great promise for effective, tailored gene activation in bacteria (Liu Y. et al., 2019; Fontana et al., 2020a; Ho et al., 2020; Villegas Kcam et al., 2021). These CRISPRa technologies were created using directed evolution and thorough tool design. Further improvements could be achieved by creating CRISPRa tools from CRISPR systems with more relaxed PAM requirements, directed evolution of CRISPRa components (activator domain, gRNA scaffold(s), and dCas protein) for greater activation, and high-throughput screening of gRNAs and promoters to uncover additional nuanced design rules for a given tool. CRISPRa has the potential to become a more effective and widely used tool for programmable gene activation in both model and non-model bacteria for a variety of industrial and research applications, such as metabolic engineering and elucidation of gene function. While many CRISPRi/a approaches in non-model bacteria have been established using genetic parts that are not well-defined or characterized, the creation of comprehensive genetic part toolboxes for these strains, which are vital for the rational design and precise control of CRISPRi/a tools, will accelerate further development and optimization of the tools. Finally, CRISPRi/a approaches have primarily been developed for more genetically tractable strains of non-model bacteria. There is a need for efficient workflows to domesticate and introduce CRISPRi/a tools to novel bacterial species and strains. Despite these current challenges, CRISPRi/a technology remains a versatile approach for programmable transcriptional regulation in non-model bacteria.
Author Contributions
SC and LA conceived of the review and wrote the manuscript. All authors read and approved the manuscript.
Funding
This material is based upon work supported by the National Science Foundation under Grant No. DMR-1904901 to LA. Additional funding for this work was provided by startup funds to LA from the University of Massachusetts Amherst and a seed grant award from the UMass ADVANCE program funded by the National Science Foundation (awards #1824090 and #2136150). This work is also supported by the National Science Foundation Graduate Research Fellowship under Grant No. DGE- 1451512 to SC.
Conflict of Interest
The authors declare that the research was conducted in the absence of any commercial or financial relationships that could be construed as a potential conflict of interest.
Publisher’s Note
All claims expressed in this article are solely those of the authors and do not necessarily represent those of their affiliated organizations, or those of the publisher, the editors and the reviewers. Any product that may be evaluated in this article, or claim that may be made by its manufacturer, is not guaranteed or endorsed by the publisher.
Acknowledgments
The authors would like to thank members of the Andrews research group for their discussions contributing to the manuscript.
Supplementary Material
The Supplementary Material for this article can be found online at: https://www.frontiersin.org/articles/10.3389/fgeed.2022.892304/full#supplementary-material
References
Afonina, I., Ong, J., Chua, J., Lu, T., and Kline, K. A. (2020). Multiplex CRISPRi System Enables the Study of Stage-specific Biofilm Genetic Requirements in Enterococcus faecalis. mBio 11. doi:10.1128/mBio.01101-20
Agarwal, N. (2020). Construction of a Novel CRISPRi-Based Tool for Silencing of Multiple Genes in Mycobacterium tuberculosis. Plasmid 110, 102515. doi:10.1016/j.plasmid.2020.102515
Ameruoso, A., Villegas Kcam, M. C., Cohen, K. P., and Chappell, J. (2021). Activating Natural Product Synthesis Using CRISPR Interference and Activation Systems in Streptomyces. bioRxiv. [Preprint]. doi:10.1101/2021.10.28.466254
Bai, J., Dai, Y., Farinha, A., Tang, A. Y., Syal, S., Vargas-Cuebas, G., et al. (2021). Essential Gene Analysis in Acinetobacter Baumannii by High-Density Transposon Mutagenesis and CRISPR Interference. J. Bacteriol. 203, 1. doi:10.1128/JB.00565-20
Banerjee, D., Eng, T., Lau, A. K., Sasaki, Y., Wang, B., Chen, Y., et al. (2020). Genome-scale Metabolic Rewiring Improves Titers Rates and Yields of the Non-native Product Indigoidine at Scale. Nat. Commun. 11, 5385. doi:10.1038/s41467-020-19171-4
Banta, A. B., Enright, A. L., Siletti, C., and Peters, J. M. (2020). A High-Efficacy CRISPR Interference System for Gene Function Discovery in Zymomonas Mobilis. Appl. Environ. Microbiol. 86, 1. doi:10.1128/AEM.01621-20
Baranowski, C., Welsh, M. A., Sham, L.-T., Eskandarian, H. A., Lim, H. C., Kieser, K. J., et al. (2018). Maturing Mycobacterium Smegmatis Peptidoglycan Requires Non-canonical Crosslinks to Maintain Shape. eLife 7, e37516. doi:10.7554/eLife.37516
Batianis, C., Kozaeva, E., Damalas, S. G., Martín‐Pascual, M., Volke, D. C., Nikel, P. I., et al. (2020). An Expanded CRISPRi Toolbox for Tunable Control of Gene Expression inPseudomonas Putida. Microb. Biotechnol. 13, 368–385. doi:10.1111/1751-7915.13533
Behle, A., Dietsch, M., Goldschmidt, L., Murugathas, W., Brandt, D., Busche, T., et al. (2021). Uncoupling of the Diurnal Growth Program by Artificial Genome Relaxation in Synechocystis Sp. PCC 6803. bioRxiv. [Preprint], 453758. doi:10.1101/2021.07.26.453758
Behler, J., Sharma, K., Reimann, V., Wilde, A., Urlaub, H., and Hess, W. R. (2018). The Host-Encoded RNase E Endonuclease as the crRNA Maturation Enzyme in a CRISPR-Cas Subtype III-Bv System. Nat. Microbiol. 3, 367–377. doi:10.1038/s41564-017-0103-5
Berlec, A., Škrlec, K., Kocjan, J., Olenic, M., and Štrukelj, B. (2018). Single Plasmid Systems for Inducible Dual Protein Expression and for CRISPR-Cas9/CRISPRi Gene Regulation in Lactic Acid Bacterium Lactococcus Lactis. Sci. Rep. 8, 1009. doi:10.1038/s41598-018-19402-1
Bhokisham, N., VanArsdale, E., Stephens, K. T., Hauk, P., Payne, G. F., and Bentley, W. E. (2020). A Redox-Based Electrogenetic CRISPR System to Connect with and Control Biological Information Networks. Nat. Commun. 11, 2427. doi:10.1038/s41467-020-16249-x
Bikard, D., Jiang, W., Samai, P., Hochschild, A., Zhang, F., and Marraffini, L. A. (2013). Programmable Repression and Activation of Bacterial Gene Expression Using an Engineered CRISPR-Cas System. Nucleic Acids Res. 41, 7429–7437. doi:10.1093/nar/gkt520
Bosch, B., DeJesus, M. A., Poulton, N. C., Zhang, W., Engelhart, C. A., Zaveri, A., et al. (2021). Genome-wide Gene Expression Tuning Reveals Diverse Vulnerabilities of M. tuberculosis. Cell 184, 4579–4592. e24. doi:10.1016/j.cell.2021.06.033
Brito, L. F., Schultenkämper, K., Passaglia, L. M. P., and Wendisch, V. F. (2020). CRISPR Interference-Based Gene Repression in the Plant Growth Promoter Paenibacillus Sonchi Genomovar Riograndensis SBR5. Appl. Microbiol. Biotechnol. 104, 5095–5106. doi:10.1007/s00253-020-10571-6
Brockett, M. R., Lee, J., Cox, J. V., Liechti, G. W., and Ouellette, S. P. (2021). A Dynamic, Ring-Forming Bactofilin Critical for Maintaining Cell Size in the Obligate Intracellular Bacterium Chlamydia trachomatis. Infect. Immun. 89, 1. doi:10.1128/IAI.00203-21
Bruder, M. R., Pyne, M. E., Moo-Young, M., Chung, D. A., and Chou, C. P. (2016). Extending CRISPR-Cas9 Technology from Genome Editing to Transcriptional Engineering in the Genus Clostridium. Appl. Environ. Microbiol. 82, 6109–6119. doi:10.1128/AEM.02128-16
Brzostek, A., Płociński, P., Minias, A., Ciszewska, A., Gąsior, F., Pawełczyk, J., et al. (2021). Dissecting the RecA-(In)dependent Response to Mitomycin C in Mycobacterium tuberculosis Using Transcriptional Profiling and Proteomics Analyses. Cells 10, 1168. doi:10.3390/cells10051168
Cao, Y., Li, X., Li, F., and Song, H. (2017). CRISPRi-sRNA: Transcriptional-Translational Regulation of Extracellular Electron Transfer in Shewanella Oneidensis. ACS Synth. Biol. 6, 1679–1690. doi:10.1021/acssynbio.6b00374
Caro, F., Place, N. M., and Mekalanos, J. J. (2019). Analysis of Lipoprotein Transport Depletion in Vibrio cholerae Using CRISPRi. Proc. Natl. Acad. Sci. U.S.A. 116, 17013–17022. doi:10.1073/pnas.1906158116
Chai, G., Yu, M., Jiang, L., Duan, Y., and Huang, J. (2019). HMMCAS: A Web Tool for the Identification and Domain Annotations of CAS Proteins. IEEE/ACM Trans. Comput. Biol. Bioinf. 16, 1313–1315. doi:10.1109/TCBB.2017.2665542
Chen, W., Zhang, Y., Yeo, W.-S., Bae, T., and Ji, Q. (2017). Rapid and Efficient Genome Editing in Staphylococcus aureus by Using an Engineered CRISPR/Cas9 System. J. Am. Chem. Soc. 139, 3790–3795. doi:10.1021/jacs.6b13317
Cheung, C.-Y., McNeil, M. B., and Cook, G. M. (2021). Utilization of CRISPR Interference to Investigate the Contribution of Genes to Pathogenesis in a Macrophage Model of Mycobacterium tuberculosis Infection. J. Antimicrob. Chemother. 77, 615–619. doi:10.1093/jac/dkab437
Cho, S., Choe, D., Lee, E., Kim, S. C., Palsson, B., and Cho, B.-K. (2018). High-Level dCas9 Expression Induces Abnormal Cell Morphology in Escherichia coli. ACS Synth. Biol. 7, 1085–1094. doi:10.1021/acssynbio.7b00462
Choi, S. Y., and Woo, H. M. (2020). CRISPRi-dCas12a: A dCas12a-Mediated CRISPR Interference for Repression of Multiple Genes and Metabolic Engineering in Cyanobacteria. ACS Synth. Biol. 9, 2351–2361. doi:10.1021/acssynbio.0c00091
Choudhary, E., Sharma, R., Kumar, Y., and Agarwal, N. (2019). Conditional Silencing by CRISPRi Reveals the Role of DNA Gyrase in Formation of Drug-Tolerant Persister Population in Mycobacterium tuberculosis. Front. Cell Infect. Microbiol. 9, 70. doi:10.3389/fcimb.2019.00070
Choudhary, E., Thakur, P., Pareek, M., and Agarwal, N. (2015). Gene Silencing by CRISPR Interference in Mycobacteria. Nat. Commun. 6, 6267. doi:10.1038/ncomms7267
Cleto, S., Jensen, J. V., Wendisch, V. F., and Lu, T. K. (2016). Corynebacterium Glutamicum Metabolic Engineering with CRISPR Interference (CRISPRi). ACS Synth. Biol. 5, 375–385. doi:10.1021/acssynbio.5b00216
Colquhoun, J. M., Farokhyfar, M., Hutcheson, A. R., Anderson, A., Bethel, C. R., Bonomo, R. A., et al. (2021). OXA-23 β-Lactamase Overexpression in Acinetobacter Baumannii Drives Physiological Changes Resulting in New Genetic Vulnerabilities. mBio 12. doi:10.1128/mBio.03137-21
Couvin, D., Bernheim, A., Toffano-Nioche, C., Touchon, M., Michalik, J., Néron, B., et al. (2018). CRISPRCasFinder, an Update of CRISRFinder, Includes a Portable Version, Enhanced Performance and Integrates Search for Cas Proteins. Nucleic Acids Res. 46, W246–W251. doi:10.1093/nar/gky425
Cui, L., Vigouroux, A., Rousset, F., Varet, H., Khanna, V., and Bikard, D. (2018). A CRISPRi Screen in E. coli Reveals Sequence-specific Toxicity of dCas9. Nat. Commun. 9, 1912. doi:10.1038/s41467-018-04209-5
Czajka, J. J., Banerjee, D., Eng, T., Menasalvas, J., Yan, C., Munoz, N. M., et al. (2021). Optimizing a High Performing Multiplex-CRISPRi P. Putida Strain with Integrated Metabolomics and 13C-Metabolic Flux Analyses. bioRxiv. [Preprint], 473729. doi:10.1101/2021.12.23.473729
Dai, Y., Pinedo, V., Tang, A. Y., Cava, F., and Geisinger, E. (2021). A New Class of Cell Wall-Recycling L , D -Carboxypeptidase Determines β-Lactam Susceptibility and Morphogenesis in Acinetobacter Baumannii. mBio 12. doi:10.1128/mBio.02786-21
Dammann, A. N., Chamby, A. B., Catomeris, A. J., Davidson, K. M., Tettelin, H., van Pijkeren, J.-P., et al. (2021). Genome-Wide Fitness Analysis of Group B Streptococcus in Human Amniotic Fluid Reveals a Transcription Factor that Controls Multiple Virulence Traits. PLoS Pathog. 17, e1009116. doi:10.1371/journal.ppat.1009116
de Bakker, V., Liu, X., Bravo, A. M., and Veening, J.-W. (2022). CRISPRi-seq for Genome-wide Fitness Quantification in Bacteria. Nat. Protoc. 17, 252–281. doi:10.1038/s41596-021-00639-6
de Wet, T. J., Winkler, K. R., Mhlanga, M., Mizrahi, V., and Warner, D. F. (2020). Arrayed CRISPRi and Quantitative Imaging Describe the Morphotypic Landscape of Essential Mycobacterial Genes. eLife 9, e60083. doi:10.7554/eLife.60083
Del Vecchio, D., Ninfa, A. J., and Sontag, E. D. (2008). Modular Cell Biology: Retroactivity and Insulation. Mol. Syst. Biol. 4, 161. doi:10.1038/msb4100204
DeLorenzo, D. M., Diao, J., Carr, R., Hu, Y., and Moon, T. S. (2021). An Improved CRISPR Interference Tool to Engineer Rhodococcus Opacus. ACS Synth. Biol. 10, 786–798. doi:10.1021/acssynbio.0c00591
DeLorenzo, D. M., Rottinghaus, A. G., Henson, W. R., and Moon, T. S. (2018). Molecular Toolkit for Gene Expression Control and Genome Modification in Rhodococcus Opacus PD630. ACS Synth. Biol. 7, 727–738. doi:10.1021/acssynbio.7b00416
Depardieu, F., and Bikard, D. (2020). Gene Silencing with CRISPRi in Bacteria and Optimization of dCas9 Expression Levels. Methods 172, 61–75. doi:10.1016/j.ymeth.2019.07.024
Dewachter, L., Liu, X., Dénéréaz, J., de Bakker, V., Costa, C., Baldry, M., et al. (2021). Amoxicillin-resistant Streptococcus Pneumoniae Can Be Resensitized by Targeting the Mevalonate Pathway as Indicated by sCRilecs-Seq. bioRxiv. [Preprint], 460059. doi:10.1101/2021.09.13.460059
Dietsch, M., Behle, A., Westhoff, P., and Axmann, I. M. (2021). Metabolic Engineering of Synechocystis Sp. PCC 6803 for the Photoproduction of the Sesquiterpene Valencene. Metab. Eng. Commun. 13, e00178. doi:10.1016/j.mec.2021.e00178
Domenech, A., Slager, J., and Veening, J.-W. (2018). Antibiotic-Induced Cell Chaining Triggers Pneumococcal Competence by Reshaping Quorum Sensing to Autocrine-like Signaling. Cell Rep. 25, 2390–2400. e3. doi:10.1016/j.celrep.2018.11.007
Dong, C., Fontana, J., Patel, A., Carothers, J. M., and Zalatan, J. G. (2018). Synthetic CRISPR-Cas Gene Activators for Transcriptional Reprogramming in Bacteria. Nat. Commun. 9, 2489. doi:10.1038/s41467-018-04901-6
Dong, X., Jin, Y., Ming, D., Li, B., Dong, H., Wang, L., et al. (2017). CRISPR/dCas9-mediated Inhibition of Gene Expression in Staphylococcus aureus. J. Microbiol. Methods 139, 79–86. doi:10.1016/j.mimet.2017.05.008
Dutta, A. K., Choudhary, E., Wang, X., Záhorszka, M., Forbak, M., Lohner, P., et al. (2019). Trehalose Conjugation Enhances Toxicity of Photosensitizers against Mycobacteria. ACS Cent. Sci. 5, 644–650. doi:10.1021/acscentsci.8b00962
Ellis, N. A., Kim, B., Tung, J., and Machner, M. P. (2021). A Multiplex CRISPR Interference Tool for Virulence Gene Interrogation in Legionella pneumophila. Commun. Biol. 4, 1–13. doi:10.1038/s42003-021-01672-7
Fackler, N., Heffernan, J., Juminaga, A., Doser, D., Nagaraju, S., Gonzalez-Garcia, R. A., et al. (2021). Transcriptional Control of Clostridium Autoethanogenum Using CRISPRi. Synth. Biol. 6, ysab008. doi:10.1093/synbio/ysab008
Faulkner, V., Cox, A. A., Goh, S., van Bohemen, A., Gibson, A. J., Liebster, O., et al. (2020). Re-sensitization of Mycobacterium Smegmatis to Rifampicin Using CRISPR Interference Demonstrates its Utility for the Study of Non-essential Drug Resistance Traits. Front. Microbiol. 11, 619427. doi:10.3389/fmicb.2020.619427
Fernandes, L. G. V., Guaman, L. P., Vasconcellos, S. A., Heinemann, M. B., Picardeau, M., and Nascimento, A. L. T. O. (2019). Gene Silencing Based on RNA-Guided Catalytically Inactive Cas9 (dCas9): a New Tool for Genetic Engineering in Leptospira. Sci. Rep. 9, 1839. doi:10.1038/s41598-018-37949-x
Fernandes, L. G. V., Hornsby, R. L., Nascimento, A. L. T. O., and Nally, J. E. (2021a). Application of CRISPR Interference (CRISPRi) for Gene Silencing in Pathogenic Species of Leptospira. JoVE 174, e62631. doi:10.3791/62631
Fernandes, L. G. V., Hornsby, R. L., Nascimento, A. L. T. O., and Nally, J. E. (2021b). Genetic Manipulation of Pathogenic Leptospira: CRISPR Interference (CRISPRi)-Mediated Gene Silencing and Rapid Mutant Recovery at 37 °C. Sci. Rep. 11, 1768. doi:10.1038/s41598-021-81400-7
Fleck, N., and Grundner, C. (2021). A Cas12a-Based CRISPR Interference System for Multigene Regulation in Mycobacteria. J. Biol. Chem. 297, 100990. doi:10.1016/j.jbc.2021.100990
Fonfara, I., Richter, H., Bratovič, M., Le Rhun, A., and Charpentier, E. (2016). The CRISPR-Associated DNA-Cleaving Enzyme Cpf1 Also Processes Precursor CRISPR RNA. Nature 532, 517–521. doi:10.1038/nature17945
Fontana, J., Dong, C., Kiattisewee, C., Chavali, V. P., Tickman, B. I., Carothers, J. M., et al. (2020a). Effective CRISPRa-Mediated Control of Gene Expression in Bacteria Must Overcome Strict Target Site Requirements. Nat. Commun. 11, 1618. doi:10.1038/s41467-020-15454-y
Fontana, J., Sparkman-Yager, D., Zalatan, J. G., and Carothers, J. M. (2020b). Challenges and Opportunities with CRISPR Activation in Bacteria for Data-Driven Metabolic Engineering. Curr. Opin. Biotechnol. 64, 190–198. doi:10.1016/j.copbio.2020.04.005
Gallay, C., Sanselicio, S., Anderson, M. E., Soh, Y. M., Liu, X., Stamsås, G. A., et al. (2021). CcrZ Is a Pneumococcal Spatiotemporal Cell Cycle Regulator that Interacts with FtsZ and Controls DNA Replication by Modulating the Activity of DnaA. Nat. Microbiol. 6, 1175–1187. doi:10.1038/s41564-021-00949-1
Ganguly, J., Martin‐Pascual, M., and Kranenburg, R. (2020). CRISPR Interference (CRISPRi) as Transcriptional Repression Tool for Hungateiclostridium Thermocellum DSM 1313. Microb. Biotechnol. 13, 339–349. doi:10.1111/1751-7915.13516
Gani, Z., Boradia, V. M., Kumar, A., Patidar, A., Talukdar, S., Choudhary, E., et al. (2021). Mycobacterium tuberculosis Glyceraldehyde‐3‐phosphate Dehydrogenase Plays a Dual Role-As an Adhesin and as a Receptor for Plasmin(ogen). Cell. Microbiol. 23, e13311. doi:10.1111/cmi.13311
Gauttam, R., Mukhopadhyay, A., Simmons, B. A., and Singer, S. W. (2021). Development of Dual‐inducible Duet‐expression Vectors for Tunable Gene Expression Control and CRISPR Interference‐based Gene Repression in Pseudomonas Putida KT2440. Microb. Biotechnol. 14, 2659–2678. doi:10.1111/1751-7915.13832
Gauttam, R., Seibold, G. M., Mueller, P., Weil, T., Weiß, T., Handrick, R., et al. (2019). A Simple Dual-Inducible CRISPR Interference System for Multiple Gene Targeting in Corynebacterium Glutamicum. Plasmid 103, 25–35. doi:10.1016/j.plasmid.2019.04.001
Gelin, M., Paoletti, J., Nahori, M.-A., Huteau, V., Leseigneur, C., Jouvion, G., et al. (2020). From Substrate to Fragments to Inhibitor Active In Vivo against Staphylococcus aureus. ACS Infect. Dis. 6, 422–435. doi:10.1021/acsinfecdis.9b00368
Geng, P., Leonard, S. P., Mishler, D. M., and Barrick, J. E. (2019). Synthetic Genome Defenses against Selfish DNA Elements Stabilize Engineered Bacteria against Evolutionary Failure. ACS Synth. Biol. 8, 521–531. doi:10.1021/acssynbio.8b00426
Gibson, A. J., Passmore, I. J., Faulkner, V., Xia, D., Nobeli, I., Stiens, J., et al. (2021). Probing Differences in Gene Essentiality between the Human and Animal Adapted Lineages of the Mycobacterium tuberculosis Complex Using TnSeq. Front. Vet. Sci. 8, 760717. doi:10.3389/fvets.2021.760717
Gordon, G. C., Korosh, T. C., Cameron, J. C., Markley, A. L., Begemann, M. B., and Pfleger, B. F. (2016). CRISPR Interference as a Titratable, Trans-acting Regulatory Tool for Metabolic Engineering in the Cyanobacterium Synechococcus Sp. Strain PCC 7002. Metab. Eng. 38, 170–179. doi:10.1016/j.ymben.2016.07.007
Göttl, V. L., Schmitt, I., Braun, K., Peters-Wendisch, P., Wendisch, V. F., and Henke, N. A. (2021). CRISPRi-Library-Guided Target Identification for Engineering Carotenoid Production by Corynebacterium Glutamicum. Microorganisms 9, 670. doi:10.3390/microorganisms9040670
Guzzo, M., Castro, L. K., Reisch, C. R., Guo, M. S., and Laub, M. T. (2020). A CRISPR Interference System for Efficient and Rapid Gene Knockdown in Caulobacter crescentus. mBio 11. doi:10.1128/mBio.02415-19
Higo, A., and Ehira, S. (2019). Spatiotemporal Gene Repression System in the Heterocyst-Forming Multicellular Cyanobacterium Anabaena Sp. PCC 7120. ACS Synth. Biol. 8, 641–646. doi:10.1021/acssynbio.8b00496
Higo, A., Isu, A., Fukaya, Y., Ehira, S., and Hisabori, T. (2018). Application of CRISPR Interference for Metabolic Engineering of the Heterocyst-Forming Multicellular Cyanobacterium Anabaena Sp. PCC 7120. Plant Cell Physiology 59, 119–127. doi:10.1093/pcp/pcx166
Higo, A., Nishiyama, E., Nakamura, K., Hihara, Y., and Ehira, S. (2019). cyAbrB Transcriptional Regulators as Safety Devices to Inhibit Heterocyst Differentiation in Anabaena Sp. Strain PCC 7120. J. Bacteriol. 201, 1. doi:10.1128/JB.00244-19
Ho, H. I., Fang, J. R., Cheung, J., and Wang, H. H. (2020). Programmable CRISPR‐Cas Transcriptional Activation in Bacteria. Mol. Syst. Biol. 16, e9427. doi:10.15252/msb.20199427
Hogan, A. M., Rahman, A. S. M. Z., Lightly, T. J., and Cardona, S. T. (2019). A Broad-Host-Range CRISPRi Toolkit for Silencing Gene Expression in Burkholderia. ACS Synth. Biol. 8, 2372–2384. doi:10.1021/acssynbio.9b00232
Huang, C.-H., Shen, C. R., Li, H., Sung, L.-Y., Wu, M.-Y., and Hu, Y.-C. (2016). CRISPR Interference (CRISPRi) for Gene Regulation and Succinate Production in Cyanobacterium S. Elongatus PCC 7942. Microb. Cell Fact. 15, 196. doi:10.1186/s12934-016-0595-3
Huang, J., Chen, J., Wang, Y., Shi, T., Ni, X., Pu, W., et al. (2021). Development of a Hyperosmotic Stress Inducible Gene Expression System by Engineering the MtrA/MtrB-dependent NCgl1418 Promoter in Corynebacterium Glutamicum. Front. Microbiol. 12. doi:10.3389/fmicb.2021.718511
Huang, L. H., Liu, Q. J., Sun, X. W., Li, X. J., Liu, M., Jia, S. R., et al. (2020). Tailoring Bacterial Cellulose Structure through CRISPR Interference‐mediated Downregulation of galU in Komagataeibacter Xylinus CGMCC 2955. Biotechnol. Bioeng. 117, 2165–2176. doi:10.1002/bit.27351
Irnov, I., Wang, Z., Jannetty, N. D., Bustamante, J. A., Rhee, K. Y., and Jacobs-Wagner, C. (2017). Crosstalk between the Tricarboxylic Acid Cycle and Peptidoglycan Synthesis in Caulobacter crescentus through the Homeostatic Control of α-ketoglutarate. PLoS Genet. 13, e1006978. doi:10.1371/journal.pgen.1006978
Jackson, S. A., McKenzie, R. E., Fagerlund, R. D., Kieper, S. N., Fineran, P. C., and Brouns, S. J. J. (2017). CRISPR-cas: Adapting to Change. Science 356, eaal5056. doi:10.1126/science.aal5056
Jiang, F., Zhou, K., Ma, L., Gressel, S., and Doudna, J. A. (2015). A Cas9-Guide RNA Complex Preorganized for Target DNA Recognition. Science 348, 1477–1481. doi:10.1126/science.aab1452
Jiang, W., Oikonomou, P., and Tavazoie, S. (2020). Comprehensive Genome-wide Perturbations via CRISPR Adaptation Reveal Complex Genetics of Antibiotic Sensitivity. Cell 180, 1002–1017. e31. doi:10.1016/j.cell.2020.02.007
Jin, W.-B., Li, T.-T., Huo, D., Qu, S., Li, X. V., Arifuzzaman, M., et al. (2022). Genetic Manipulation of Gut Microbes Enables Single-Gene Interrogation in a Complex Microbiome. Cell 185, 547–562. e22. doi:10.1016/j.cell.2021.12.035
Judd, J. A., Canestrari, J., Clark, R., Joseph, A., Lapierre, P., Lasek-Nesselquist, E., et al. (2021). A Mycobacterial Systems Resource for the Research Community. mBio 12. doi:10.1128/mBio.02401-20
Kaczmarzyk, D., Cengic, I., Yao, L., and Hudson, E. P. (2018). Diversion of the Long-Chain Acyl-ACP Pool in Synechocystis to Fatty Alcohols through CRISPRi Repression of the Essential Phosphate Acyltransferase PlsX. Metab. Eng. 45, 59–66. doi:10.1016/j.ymben.2017.11.014
Kampmann, M. (2018). CRISPRi and CRISPRa Screens in Mammalian Cells for Precision Biology and Medicine. ACS Chem. Biol. 13, 406–416. doi:10.1021/acschembio.7b00657
Ke, J., Robinson, D., Wu, Z.-Y., Kuftin, A., Louie, K., Kosina, S., et al. (2022). CRAGE-CRISPR Facilitates Rapid Activation of Secondary Metabolite Biosynthetic Gene Clusters in Bacteria. Cell Chem. Biol. 29, 696–710. doi:10.1016/j.chembiol.2021.08.009
Kiattisewee, C., Dong, C., Fontana, J., Sugianto, W., Peralta-Yahya, P., Carothers, J. M., et al. (2021). Portable Bacterial CRISPR Transcriptional Activation Enables Metabolic Engineering in Pseudomonas Putida. Metab. Eng. 66, 283–295. doi:10.1016/j.ymben.2021.04.002
Kim, S. K., Kim, H., Ahn, W.-C., Park, K.-H., Woo, E.-J., Lee, D.-H., et al. (2017). Efficient Transcriptional Gene Repression by Type V-A CRISPR-Cpf1 from Eubacterium Eligens. ACS Synth. Biol. 6, 1273–1282. doi:10.1021/acssynbio.6b00368
Kim, S. K., Yoon, P. K., Kim, S. J., Woo, S. G., Rha, E., Lee, H., et al. (2020). CRISPR Interference‐mediated Gene Regulation in Pseudomonas Putida KT 2440. Microb. Biotechnol. 13, 210–221. doi:10.1111/1751-7915.13382
Kirtania, P., Hódi, B., Mallick, I., Vass, I. Z., Fehér, T., Vass, I., et al. (2019). A Single Plasmid Based CRISPR Interference in Synechocystis 6803 - A Proof of Concept. PLOS ONE 14, e0225375. doi:10.1371/journal.pone.0225375
Knoops, A., Vande Capelle, F., Fontaine, L., Verhaegen, M., Mignolet, J., Goffin, P., et al. (2022). The CovRS Environmental Sensor Directly Controls the ComRS Signaling System to Orchestrate Competence Bimodality in Salivarius Streptococci. mBio 13. doi:10.1128/mbio.03125-21
Knoot, C. J., Biswas, S., and Pakrasi, H. B. (2020). Tunable Repression of Key Photosynthetic Processes Using Cas12a CRISPR Interference in the Fast-Growing Cyanobacterium Synechococcus Sp. UTEX 2973. ACS Synth. Biol. 9, 132–143. doi:10.1021/acssynbio.9b00417
Koonin, E. V., Makarova, K. S., and Zhang, F. (2017). Diversity, Classification and Evolution of CRISPR-Cas Systems. Curr. Opin. Microbiol. 37, 67–78. doi:10.1016/j.mib.2017.05.008
Kozaeva, E., Volkova, S., Matos, M. R. A., Mezzina, M. P., Wulff, T., Volke, D. C., et al. (2021). Model-guided Dynamic Control of Essential Metabolic Nodes Boosts Acetyl-Coenzyme A-dependent Bioproduction in Rewired Pseudomonas Putida. Metab. Eng. 67, 373–386. doi:10.1016/j.ymben.2021.07.014
Kuo, J., Yuan, R., Sánchez, C., Paulsson, J., and Silver, P. A. (2020). Toward a Translationally Independent RNA-Based Synthetic Oscillator Using Deactivated CRISPR-Cas. Nucleic Acids Res. 48, 8165–8177. doi:10.1093/nar/gkaa557
Landeta, C., McPartland, L., Tran, N. Q., Meehan, B. M., Zhang, Y., Tanweer, Z., et al. (2019). Inhibition ofPseudomonas aeruginosaandMycobacterium Tuberculosisdisulfide Bond Forming Enzymes. Mol. Microbiol. 111, 918–937. doi:10.1111/mmi.14185
Lee, H. H., Ostrov, N., Wong, B. G., Gold, M. A., Khalil, A. S., and Church, G. M. (2019). Functional Genomics of the Rapidly Replicating Bacterium Vibrio Natriegens by CRISPRi. Nat. Microbiol. 4, 1105–1113. doi:10.1038/s41564-019-0423-8
Lee, M., and Woo, H. M. (2020). A Logic NAND Gate for Controlling Gene Expression in a Circadian Rhythm in Cyanobacteria. ACS Synth. Biol. 9, 3210–3216. doi:10.1021/acssynbio.0c00455
Lee, S. S., Shin, H., Jo, S., Lee, S.-M., Um, Y., and Woo, H. M. (2018). Rapid Identification of Unknown Carboxyl Esterase Activity in Corynebacterium Glutamicum Using RNA-Guided CRISPR Interference. Enzyme Microb. Technol. 114, 63–68. doi:10.1016/j.enzmictec.2018.04.004
Leonard, S. P., Perutka, J., Powell, J. E., Geng, P., Richhart, D. D., Byrom, M., et al. (2018). Genetic Engineering of Bee Gut Microbiome Bacteria with a Toolkit for Modular Assembly of Broad-Host-Range Plasmids. ACS Synth. Biol. 7, 1279–1290. doi:10.1021/acssynbio.7b00399
Lewis, W. H., Tahon, G., Geesink, P., Sousa, D. Z., and Ettema, T. J. G. (2021). Innovations to Culturing the Uncultured Microbial Majority. Nat. Rev. Microbiol. 19, 225–240. doi:10.1038/s41579-020-00458-8
Li, J., Tang, Q., Li, Y., Fan, Y.-Y., Li, F.-H., Wu, J.-H., et al. (2020a). Rediverting Electron Flux with an Engineered CRISPR-ddAsCpf1 System to Enhance the Pollutant Degradation Capacity of Shewanella Oneidensis. Environ. Sci. Technol. 54, 3599–3608. doi:10.1021/acs.est.9b06378
Li, J., and Ye, B.-C. (2021). Metabolic Engineering of Pseudomonas Putida KT2440 for High-Yield Production of Protocatechuic Acid. Bioresour. Technol. 319, 124239. doi:10.1016/j.biortech.2020.124239
Li, L., Wei, K., Zheng, G., Liu, X., Chen, S., Jiang, W., et al. (2018). CRISPR-Cpf1-Assisted Multiplex Genome Editing and Transcriptional Repression in Streptomyces. Appl. Environ. Microbiol. 84, 1. doi:10.1128/AEM.00827-18
Li, M., Chen, J., Wang, Y., Liu, J., Huang, J., Chen, N., et al. (2020b). Efficient Multiplex Gene Repression by CRISPR-dCpf1 in Corynebacterium Glutamicum. Front. Bioeng. Biotechnol. 8, 357. doi:10.3389/fbioe.2020.00357
Li, Q., Chen, J., Minton, N. P., Zhang, Y., Wen, Z., Liu, J., et al. (2016). CRISPR-based Genome Editing and Expression Control Systems inClostridium acetobutylicumandClostridium Beijerinckii. Biotechnol. J. 11, 961–972. doi:10.1002/biot.201600053
Li, Z., and Liu, J. Z. (2017). Transcriptomic Changes in Response to Putrescine Production in Metabolically Engineered Corynebacterium Glutamicum. Front. Microbiol. 8, 1987. doi:10.3389/fmicb.2017.01987
Liew, A. T. F., Theis, T., Jensen, S. O., Garcia-Lara, J., Foster, S. J., Firth, N., et al. (2011). A Simple Plasmid-Based System that Allows Rapid Generation of Tightly Controlled Gene Expression in Staphylococcus aureus. Microbiology 157, 666–676. doi:10.1099/mic.0.045146-0
Liow, L. T., Go, M. K., Chang, M. W., and Yew, W. S. (2020). Toolkit Development for Cyanogenic and Gold Biorecovery Chassis Chromobacterium Violaceum. ACS Synth. Biol. 9, 953–961. doi:10.1021/acssynbio.0c00064
Liu, D., Johnson, V. M., and Pakrasi, H. B. (2020a). A Reversibly Induced CRISPRi System Targeting Photosystem II in the Cyanobacterium Synechocystis Sp. PCC 6803. ACS Synth. Biol. 9, 1441–1449. doi:10.1021/acssynbio.0c00106
Liu, W., Tang, D., Wang, H., Lian, J., Huang, L., and Xu, Z. (2019a). Combined Genome Editing and Transcriptional Repression for Metabolic Pathway Engineering in Corynebacterium Glutamicum Using a Catalytically Active Cas12a. Appl. Microbiol. Biotechnol. 103, 8911–8922. doi:10.1007/s00253-019-10118-4
Liu, X., Gallay, C., Kjos, M., Domenech, A., Slager, J., Kessel, S. P., et al. (2017). High‐throughput CRISPRi Phenotyping Identifies New Essential Genes in Streptococcus Pneumoniae. Mol. Syst. Biol. 13, 931. doi:10.15252/msb.20167449
Liu, X., Kimmey, J. M., Matarazzo, L., de Bakker, V., Van Maele, L., Sirard, J.-C., et al. (2021a). Exploration of Bacterial Bottlenecks and Streptococcus Pneumoniae Pathogenesis by CRISPRi-Seq. Cell Host Microbe 29, 107–120. e6. doi:10.1016/j.chom.2020.10.001
Liu, Y., Chen, J., Crisante, D., Jaramillo Lopez, J. M., and Mahadevan, R. (2020b). Dynamic Cell Programming with Quorum Sensing-Controlled CRISPRi Circuit. ACS Synth. Biol. 9, 1284–1291. doi:10.1021/acssynbio.0c00148
Liu, Y., Khan, S., Wu, P., Li, B., Liu, L., Ni, J., et al. (2021b). Uncovering and Engineering a Mini-Regulatory Network of the TetR-Family Regulator SACE_0303 for Yield Improvement of Erythromycin in Saccharopolyspora Erythraea. Front. Bioeng. Biotechnol. 9. doi:10.3389/fbioe.2021.692901
Liu, Y., Wan, X., and Wang, B. (2019b). Engineered CRISPRa Enables Programmable Eukaryote-like Gene Activation in Bacteria. Nat. Commun. 10, 3693. doi:10.1038/s41467-019-11479-0
Liu, Y., Wang, H., Li, S., Zhang, Y., Cheng, X., Xiang, W., et al. (2021c). Engineering of Primary Metabolic Pathways for Titer Improvement of Milbemycins in Streptomyces Bingchenggensis. Appl. Microbiol. Biotechnol. 105, 1875–1887. doi:10.1007/s00253-021-11164-7
Lunge, A., Gupta, R., Choudhary, E., and Agarwal, N. (2020). The Unfoldase ClpC1 of Mycobacterium tuberculosis Regulates the Expression of a Distinct Subset of Proteins Having Intrinsically Disordered Termini. J. Biol. Chem. 295, 9455–9473. doi:10.1074/jbc.RA120.013456
Luo, M. L., Mullis, A. S., Leenay, R. T., and Beisel, C. L. (2015). Repurposing Endogenous Type I CRISPR-Cas Systems for Programmable Gene Repression. Nucleic Acids Res. 43, 674–681. doi:10.1093/nar/gku971
Mai, J., Rao, C., Watt, J., Sun, X., Lin, C., Zhang, L., et al. (2019). Mycobacterium tuberculosis 6C sRNA Binds Multiple mRNA Targets via C-Rich Loops Independent of RNA Chaperones. Nucleic Acids Res. 47, 4292–4307. doi:10.1093/nar/gkz149
Makarova, K. S., Wolf, Y. I., Iranzo, J., Shmakov, S. A., Alkhnbashi, O. S., Brouns, S. J. J., et al. (2020). Evolutionary Classification of CRISPR-Cas Systems: a Burst of Class 2 and Derived Variants. Nat. Rev. Microbiol. 18, 67–83. doi:10.1038/s41579-019-0299-x
Mariscal, A. M., Kakizawa, S., Hsu, J. Y., Tanaka, K., González-González, L., Broto, A., et al. (2018). Tuning Gene Activity by Inducible and Targeted Regulation of Gene Expression in Minimal Bacterial Cells. ACS Synth. Biol. 7, 1538–1552. doi:10.1021/acssynbio.8b00028
Mårli, M. T. (2020). Using CRISPR Interference to Study Novel Biofilm-Associated Genes in Staphylococcus aureus. Available at: https://nmbu.brage.unit.no/nmbu-xmlui/handle/11250/2682085 (Accessed January 28, 2022).
Marraffini, L. A., and Sontheimer, E. J. (2008). CRISPR Interference Limits Horizontal Gene Transfer in Staphylococci by Targeting DNA. Science 322, 1843–1845. doi:10.1126/science.1165771
Marreddy, R. K. R., Wu, X., Sapkota, M., Prior, A. M., Jones, J. A., Sun, D., et al. (2019). The Fatty Acid Synthesis Protein Enoyl-ACP Reductase II (FabK) Is a Target for Narrow-Spectrum Antibacterials for Clostridium difficile Infection. ACS Infect. Dis. 5, 208–217. doi:10.1021/acsinfecdis.8b00205
McNeil, M. B., and Cook, G. M. (2019). Utilization of CRISPR Interference to Validate MmpL3 as a Drug Target in Mycobacterium tuberculosis. Antimicrob. Agents Chemother. 63, 1. doi:10.1128/AAC.00629-19
McNeil, M. B., Keighley, L. M., Cook, J. R., Cheung, C. Y., and Cook, G. M. (2021). CRISPR Interference Identifies Vulnerable Cellular Pathways with Bactericidal Phenotypes in Mycobacterium tuberculosis. Mol. Microbiol. 116, 1033–1043. doi:10.1111/mmi.14790
McNeil, M. B., Ryburn, H. W. K., Harold, L. K., Tirados, J. F., and Cook, G. M. (2020). Transcriptional Inhibition of the F 1 F 0 -Type ATP Synthase Has Bactericidal Consequences on the Viability of Mycobacteria. Antimicrob. Agents Chemother. 64, 1. doi:10.1128/AAC.00492-20
McNeil, M. B., Ryburn, H. W., Tirados, J., Cheung, C.-Y., and Cook, G. M. (2022). Multiplexed Transcriptional Repression Identifies a Network of Bactericidal Interactions between Mycobacterial Respiratory Complexes. iScience 25, 103573. doi:10.1016/j.isci.2021.103573
Miao, C., Zhao, H., Qian, L., and Lou, C. (2019). Systematically Investigating the Key Features of the DNase Deactivated Cpf1 for Tunable Transcription Regulation in Prokaryotic Cells. Synthetic Syst. Biotechnol. 4, 1–9. doi:10.1016/j.synbio.2018.11.002
Mimee, M., Tucker, A. C., Voigt, C. A., and Lu, T. K. (2015). Programming a Human Commensal Bacterium, Bacteroides Thetaiotaomicron, to Sense and Respond to Stimuli in the Murine Gut Microbiota. Cell Syst. 1, 62–71. doi:10.1016/j.cels.2015.06.001
Mo, X.-H., Zhang, H., Wang, T.-M., Zhang, C., Zhang, C., Xing, X.-H., et al. (2020). Establishment of CRISPR Interference in Methylorubrum Extorquens and Application of Rapidly Mining a New Phytoene Desaturase Involved in Carotenoid Biosynthesis. Appl. Microbiol. Biotechnol. 104, 4515–4532. doi:10.1007/s00253-020-10543-w
Monk, I. R., Tree, J. J., Howden, B. P., Stinear, T. P., and Foster, T. J. (2015). Complete Bypass of Restriction Systems for Major Staphylococcus aureus Lineages. mBio 6. doi:10.1128/mBio.00308-15
Mougiakos, I., Mohanraju, P., Bosma, E. F., Vrouwe, V., Finger Bou, M., Naduthodi, M. I. S., et al. (2017). Characterizing a Thermostable Cas9 for Bacterial Genome Editing and Silencing. Nat. Commun. 8, 1647. doi:10.1038/s41467-017-01591-4
Müh, U., Pannullo, A. G., Weiss, D. S., and Ellermeier, C. D. (2019). A Xylose-Inducible Expression System and a CRISPR Interference Plasmid for Targeted Knockdown of Gene Expression in Clostridioides Difficile. J. Bacteriol. 201, 1. doi:10.1128/JB.00711-18
Myrbråten, I. S., Stamsås, G. A., Chan, H., Angeles, D. M., Knutsen, T. M., Salehian, Z., et al. (2021). SmdA Is a Novel Cell Morphology Determinant in Staphylococcus aureus. bioRxiv. [Preprint], 469651. doi:10.1101/2021.11.23.469651
Myrbråten, I. S., Wiull, K., Salehian, Z., Håvarstein, L. S., Straume, D., Mathiesen, G., et al. (2019). CRISPR Interference for Rapid Knockdown of Essential Cell Cycle Genes in Lactobacillus Plantarum. mSphere 4. doi:10.1128/mSphere.00007-19
Nadolinskaia, N. I., Zamakhaev, M. V., Shumkov, M. S., Armianinova, D. K., Karpov, D. S., and Goncharenko, A. V. (2021). CRISPR Interference of Adenylate Cyclases from Mycobacterium tuberculosis. Appl. Biochem. Microbiol. 57, 421–425. doi:10.1134/S0003683821040128
Noirot-Gros, M.-F., Forrester, S., Malato, G., Larsen, P. E., and Noirot, P. (2019). CRISPR Interference to Interrogate Genes that Control Biofilm Formation in Pseudomonas Fluorescens. Sci. Rep. 9, 15954. doi:10.1038/s41598-019-52400-5
Nussenzweig, P. M., and Marraffini, L. A. (2020). Molecular Mechanisms of CRISPR-Cas Immunity in Bacteria. Annu. Rev. Genet. 54, 93–120. doi:10.1146/annurev-genet-022120-112523
Ouellette, S. P. (2018). Feasibility of a Conditional Knockout System for Chlamydia Based on CRISPR Interference. Front. Cell Infect. Microbiol. 8, 59. doi:10.3389/fcimb.2018.00059
Ouellette, S. P., Blay, E. A., Hatch, N. D., and Fisher-Marvin, L. A. (2021). CRISPR Interference to Inducibly Repress Gene Expression in Chlamydia trachomatis. Infect. Immun. 89, 1. doi:10.1128/IAI.00108-21
Park, J., Shin, H., Lee, S.-M., Um, Y., and Woo, H. M. (2018). RNA-guided Single/double Gene Repressions in Corynebacterium Glutamicum Using an Efficient CRISPR Interference and its Application to Industrial Strain. Microb. Cell Fact. 17, 4. doi:10.1186/s12934-017-0843-1
Park, J., Yu, B. J., Choi, J.-i., and Woo, H. M. (2019). Heterologous Production of Squalene from Glucose in Engineered Corynebacterium Glutamicum Using Multiplex CRISPR Interference and High-Throughput Fermentation. J. Agric. Food Chem. 67, 308–319. doi:10.1021/acs.jafc.8b05818
Pawluk, A., Davidson, A. R., and Maxwell, K. L. (2018). Anti-CRISPR: Discovery, Mechanism and Function. Nat. Rev. Microbiol. 16, 12–17. doi:10.1038/nrmicro.2017.120
Peng, R., Wang, Y., Feng, W.-w., Yue, X.-j., Chen, J.-h., Hu, X.-z., et al. (2018). CRISPR/dCas9-mediated Transcriptional Improvement of the Biosynthetic Gene Cluster for the Epothilone Production in Myxococcus Xanthus. Microb. Cell Fact. 17, 15. doi:10.1186/s12934-018-0867-1
Peters, J. M., Koo, B.-M., Patino, R., Heussler, G. E., Hearne, C. C., Qu, J., et al. (2019). Enabling Genetic Analysis of Diverse Bacteria with Mobile-CRISPRi. Nat. Microbiol. 4, 244–250. doi:10.1038/s41564-018-0327-z
Qi, L. S., Larson, M. H., Gilbert, L. A., Doudna, J. A., Weissman, J. S., Arkin, A. P., et al. (2013). Repurposing CRISPR as an RNA-Guided Platform for Sequence-specific Control of Gene Expression. Cell 152, 1173–1183. doi:10.1016/j.cell.2013.02.022
Qin, Z., Yang, Y., Yu, S., Liu, L., Chen, Y., Chen, J., et al. (2021). Repurposing the Endogenous Type I-E CRISPR/Cas System for Gene Repression in Gluconobacter Oxydans WSH-003. ACS Synth. Biol. 10, 84–93. doi:10.1021/acssynbio.0c00456
Qu, J., Prasad, N. K., Yu, M. A., Chen, S., Lyden, A., Herrera, N., et al. (2019). Modulating Pathogenesis with Mobile-CRISPRi. J. Bacteriol. 201. doi:10.1128/JB.00304-19
Quiñones-Garcia, S., Gilman, R. H., Sheen, P., and Zimic, M. (2021). Silencing of an Efflux Pump Coding Gene Decreases the Efflux Rate of Pyrazinoic Acid in Mycobacterium Smegmatis. bioRxiv. [Preprint], 466536. doi:10.1101/2021.10.29.466536
Rahman, K., Jamal, M., Chen, X., Zhou, W., Yang, B., Zou, Y., et al. (2021). Reprogramming Mycobacterium tuberculosis CRISPR System for Gene Editing and Genome-wide RNA Interference Screening. Genomics, Proteomics Bioinforma. 2021, 1. doi:10.1016/j.gpb.2021.01.008
Randall, S. E., Martini, M. C., Zhou, Y., Joubran, S. R., and Shell, S. S. (2020). MamA Essentiality in Mycobacterium Smegmatis Is Explained by the Presence of an Apparent Cognate Restriction Endonuclease. BMC Res. Notes 13, 462. doi:10.1186/s13104-020-05302-z
Rath, D., Amlinger, L., Hoekzema, M., Devulapally, P. R., and Lundgren, M. (2015). Efficient Programmable Gene Silencing by Cascade. Nucleic Acids Res. 43, 237–246. doi:10.1093/nar/gku1257
Riley, L. A., and Guss, A. M. (2021). Approaches to Genetic Tool Development for Rapid Domestication of Non-model Microorganisms. Biotechnol. Biofuels 14, 30. doi:10.1186/s13068-020-01872-z
Rock, J. M., Hopkins, F. F., Chavez, A., Diallo, M., Chase, M. R., Gerrick, E. R., et al. (2017). Programmable Transcriptional Repression in Mycobacteria Using an Orthogonal CRISPR Interference Platform. Nat. Microbiol. 2, 1–9. doi:10.1038/nmicrobiol.2016.274
Santos, M., Pacheco, C. C., Yao, L., Hudson, E. P., and Tamagnini, P. (2021). CRISPRi as a Tool to Repress Multiple Copies of Extracellular Polymeric Substances (EPS)-Related Genes in the Cyanobacterium Synechocystis Sp. PCC 6803. Life 11, 1198. doi:10.3390/life11111198
Sato’o, Y., Hisatsune, J., Yu, L., Sakuma, T., Yamamoto, T., and Sugai, M. (2018). Tailor-made Gene Silencing of Staphylococcus aureus Clinical Isolates by CRISPR Interference. PLOS ONE 13, e0185987. doi:10.1371/journal.pone.0185987
Savková, K., Huszár, S., Baráth, P., Pakanová, Z., Kozmon, S., Vancová, M., et al. (2021). An ABC Transporter Wzm-Wzt Catalyzes Translocation of Lipid-Linked Galactan across the Plasma Membrane in Mycobacteria. Proc. Natl. Acad. Sci. U.S.A. 118. doi:10.1073/pnas.2023663118
Schilling, C., Koffas, M. A. G., Sieber, V., and Schmid, J. (2020). Novel Prokaryotic CRISPR-Cas12a-Based Tool for Programmable Transcriptional Activation and Repression. ACS Synth. Biol. 9, 3353–3363. doi:10.1021/acssynbio.0c00424
Schultenkämper, K., Brito, L. F., López, M. G., Brautaset, T., and Wendisch, V. F. (2019). Establishment and Application of CRISPR Interference to Affect Sporulation, Hydrogen Peroxide Detoxification, and Mannitol Catabolism in the Methylotrophic Thermophile Bacillus Methanolicus. Appl. Microbiol. Biotechnol. 103, 5879–5889. doi:10.1007/s00253-019-09907-8
Schultenkämper, K., Gütle, D. D., López, M. G., Keller, L. B., Zhang, L., Einsle, O., et al. (2021). Interrogating the Role of the Two Distinct Fructose-Bisphosphate Aldolases of Bacillus Methanolicus by Site-Directed Mutagenesis of Key Amino Acids and Gene Repression by CRISPR Interference. Front. Microbiol. 12.
Sha, Y., Qiu, Y., Zhu, Y., Sun, T., Luo, Z., Gao, J., et al. (2020). CRISPRi-Based Dynamic Regulation of Hydrolase for the Synthesis of Poly-γ-Glutamic Acid with Variable Molecular Weights. ACS Synth. Biol. 9, 2450–2459. doi:10.1021/acssynbio.0c00207
Shabestary, K., Anfelt, J., Ljungqvist, E., Jahn, M., Yao, L., and Hudson, E. P. (2018). Targeted Repression of Essential Genes to Arrest Growth and Increase Carbon Partitioning and Biofuel Titers in Cyanobacteria. ACS Synth. Biol. 7, 1669–1675. doi:10.1021/acssynbio.8b00056
Shabestary, K., Hernández, H. P., Miao, R., Ljungqvist, E., Hallman, O., Sporre, E., et al. (2021). Cycling between Growth and Production Phases Increases Cyanobacteria Bioproduction of Lactate. Metab. Eng. 68, 131–141. doi:10.1016/j.ymben.2021.09.010
Shields, R. C., Walker, A. R., Maricic, N., Chakraborty, B., Underhill, S. A. M., and Burne, R. A. (2020). Repurposing the Streptococcus Mutans CRISPR-Cas9 System to Understand Essential Gene Function. PLoS Pathog. 16, e1008344. doi:10.1371/journal.ppat.1008344
Shin, J., Kang, S., Song, Y., Jin, S., Lee, J. S., Lee, J.-K., et al. (2019). Genome Engineering of Eubacterium Limosum Using Expanded Genetic Tools and the CRISPR-Cas9 System. ACS Synth. Biol. 8, 2059–2068. doi:10.1021/acssynbio.9b00150
Singh, A. K., Carette, X., Potluri, L.-P., Sharp, J. D., Xu, R., Prisic, S., et al. (2016). Investigating Essential Gene Function inMycobacterium Tuberculosisusing an Efficient CRISPR Interference System. Nucleic Acids Res. 44, e143. doi:10.1093/nar/gkw625
Singh, K. H., Jha, B., Dwivedy, A., Choudhary, E., N, A. G., Ashraf, A., et al. (2017). Characterization of a Secretory Hydrolase from Mycobacterium tuberculosis Sheds Critical Insight into Host Lipid Utilization by M. tuberculosis. J. Biol. Chem. 292, 11326–11335. doi:10.1074/jbc.M117.794297
Son, J., Jang, S. H., Cha, J. W., and Jeong, K. J. (2020). Development of CRISPR Interference (CRISPRi) Platform for Metabolic Engineering of Leuconostoc Citreum and its Application for Engineering Riboflavin Biosynthesis. Ijms 21, 5614. doi:10.3390/ijms21165614
Spoto, M., Guan, C., Fleming, E., and Oh, J. (2020). A Universal, Genomewide GuideFinder for CRISPR/Cas9 Targeting in Microbial Genomes. mSphere 5. doi:10.1128/mSphere.00086-20
Spoto, M., Riera Puma, J. P., Fleming, E., Guan, C., Nzutchi, Y. O., Kim, D., et al. (2021). Large-scale CRISPRi and Transcriptomics of Staphylococcus Epidermidis Identify Genetic Factors Implicated in Commensal-Pathogen Lifestyle Versatility. bioRxiv. [Preprint]. doi:10.1101/2021.04.29.442003
Stamsås, G. A., Myrbråten, I. S., Straume, D., Salehian, Z., Veening, J. W., Håvarstein, L. S., et al. (2018). CozEa and CozEb Play Overlapping and Essential Roles in Controlling Cell Division in Staphylococcus aureus. Mol. Microbiol. 109, 615–632. doi:10.1111/mmi.13999
Stolle, A.-S., Meader, B. T., Toska, J., and Mekalanos, J. J. (2021). Endogenous Membrane Stress Induces T6SS Activity in Pseudomonas aeruginosa. Proc. Natl. Acad. Sci. U.S.A. 118. doi:10.1073/pnas.2018365118
Sun, J., Wang, Q., Jiang, Y., Wen, Z., Yang, L., Wu, J., et al. (2018). Genome Editing and Transcriptional Repression in Pseudomonas Putida KT2440 via the Type II CRISPR System. Microb. Cell Fact. 17, 41. doi:10.1186/s12934-018-0887-x
Takacs, C. N., Scott, M., Chang, Y., Kloos, Z. A., Irnov, I., Rosa, P. A., et al. (2021). A CRISPR Interference Platform for Selective Downregulation of Gene Expression in Borrelia Burgdorferi. Appl. Environ. Microbiol. 87, 1. doi:10.1128/AEM.02519-20
Taketani, M., Zhang, J., Zhang, S., Triassi, A. J., Huang, Y.-J., Griffith, L. G., et al. (2020). Genetic Circuit Design Automation for the Gut Resident Species Bacteroides Thetaiotaomicron. Nat. Biotechnol. 38, 962–969. doi:10.1038/s41587-020-0468-5
Tan, S. Z., Reisch, C. R., and Prather, K. L. J. (2018). A Robust CRISPR Interference Gene Repression System in Pseudomonas. J. Bacteriol. 200, 1. doi:10.1128/JB.00575-17
Tao, W., Lv, L., and Chen, G.-Q. (2017). Engineering Halomonas Species TD01 for Enhanced Polyhydroxyalkanoates Synthesis via CRISPRi. Microb. Cell Fact. 16, 48. doi:10.1186/s12934-017-0655-3
Teh, M. Y., Ooi, K. H., Danny Teo, S. X., Bin Mansoor, M. E., Shaun Lim, W. Z., and Tan, M. H. (2019). An Expanded Synthetic Biology Toolkit for Gene Expression Control in Acetobacteraceae. ACS Synth. Biol. 8, 708–723. doi:10.1021/acssynbio.8b00168
Thakur, P., Gantasala, N. P., Choudhary, E., Singh, N., Abdin, M. Z., and Agarwal, N. (2016). The Preprotein Translocase YidC Controls Respiratory Metabolism in Mycobacterium tuberculosis. Sci. Rep. 6, 24998. doi:10.1038/srep24998
Tian, J., Yang, G., Gu, Y., Sun, X., Lu, Y., and Jiang, W. (2020). Developing an Endogenous Quorum-sensing Based CRISPRi Circuit for Autonomous and Tunable Dynamic Regulation of Multiple Targets in Streptomyces. Nucleic Acids Res. 48, 8188–8202. doi:10.1093/nar/gkaa602
Tong, Y., Charusanti, P., Zhang, L., Weber, T., and Lee, S. Y. (2015). CRISPR-Cas9 Based Engineering of Actinomycetal Genomes. ACS Synth. Biol. 4, 1020–1029. doi:10.1021/acssynbio.5b00038
Tong, Y., Whitford, C. M., Blin, K., Jørgensen, T. S., Weber, T., and Lee, S. Y. (2020). CRISPR-Cas9, CRISPRi and CRISPR-BEST-Mediated Genetic Manipulation in Streptomycetes. Nat. Protoc. 15, 2470–2502. doi:10.1038/s41596-020-0339-z
Ultee, E., van der Aart, L. T., Zhang, L., van Dissel, D., Diebolder, C. A., van Wezel, G. P., et al. (2020). Teichoic Acids Anchor Distinct Cell Wall Lamellae in an Apically Growing Bacterium. Commun. Biol. 3, 1–9. doi:10.1038/s42003-020-1038-6
Vartoukian, S. R., Palmer, R. M., and Wade, W. G. (2010). Strategies for Culture of 'unculturable' Bacteria. FEMS Microbiol. Lett. 309, no. doi:10.1111/j.1574-6968.2010.02000.x
Villegas Kcam, M. C., Tsong, A. J., and Chappell, J. (2022). Uncovering the Distinct Properties of a Bacterial Type I-E CRISPR Activation System. ACS Synth. Biol. 11, 1000–1003. doi:10.1021/acssynbio.1c00496
Villegas Kcam, M. C., Tsong, A. J., and Chappell, J. (2021). Rational Engineering of a Modular Bacterial CRISPR-Cas Activation Platform with Expanded Target Range. Nucleic Acids Res. 49, 4793–4802. doi:10.1093/nar/gkab211
Wang, J., Dai, W., Li, J., Li, Q., Xie, R., Zhang, Y., et al. (2021a). AcrHub: an Integrative Hub for Investigating, Predicting and Mapping Anti-CRISPR Proteins. Nucleic Acids Res. 49, D630–D638. doi:10.1093/nar/gkaa951
Wang, J., Dai, W., Li, J., Xie, R., Dunstan, R. A., Stubenrauch, C., et al. (2020). PaCRISPR: a Server for Predicting and Visualizing Anti-CRISPR Proteins. Nucleic Acids Res. 48, W348–W357. doi:10.1093/nar/gkaa432
Wang, J., Zhao, P., Li, Y., Xu, L., and Tian, P. (2018a). Engineering CRISPR Interference System in Klebsiella pneumoniae for Attenuating Lactic Acid Synthesis. Microb. Cell Fact. 17, 56. doi:10.1186/s12934-018-0903-1
Wang, K., and Nicholaou, M. (2017). Suppression of Antimicrobial Resistance in MRSA Using CRISPR-dCas9. Clin. Lab. Sci. 30, 207–213. doi:10.29074/ascls.30.4.207
Wang, M., Liu, L., Fan, L., and Tan, T. (2017). CRISPRi Based System for Enhancing 1-butanol Production in Engineered Klebsiella pneumoniae. Process Biochem. 56, 139–146. doi:10.1016/j.procbio.2017.02.013
Wang, T., Guan, C., Guo, J., Liu, B., Wu, Y., Xie, Z., et al. (2018b). Pooled CRISPR Interference Screening Enables Genome-Scale Functional Genomics Study in Bacteria with Superior Performance. Nat. Commun. 9, 2475. doi:10.1038/s41467-018-04899-x
Wang, T., Wang, M., Zhang, Q., Cao, S., Li, X., Qi, Z., et al. (2019). Reversible Gene Expression Control in Yersinia pestis by Using an Optimized CRISPR Interference System. Appl. Environ. Microbiol. 85, 1. doi:10.1128/AEM.00097-19
Wang, W., and Sun, B. (2021). VraCP Regulates Cell Wall Metabolism and Antibiotic Resistance in Vancomycin-Intermediate Staphylococcus aureus Strain Mu50. J. Antimicrob. Chemother. 76, 1712–1723. doi:10.1093/jac/dkab113
Wang, X., Fu, Y., Wang, M., and Niu, G. (2021b). Synthetic Cellobiose-Inducible Regulatory Systems Allow Tight and Dynamic Controls of Gene Expression in Streptomyces. ACS Synth. Biol. 10, 1956–1965. doi:10.1021/acssynbio.1c00152
Wang, Y., Yue, X., Yuan, S., Hong, Y., Hu, W., and Li, Y. (2021c). Internal Promoters and Their Effects on the Transcription of Operon Genes for Epothilone Production in Myxococcus Xanthus. Front. Bioeng. Biotechnol. 9. doi:10.3389/fbioe.2021.758561
Wang, Y., Zhang, Z.-T., Seo, S.-O., Lynn, P., Lu, T., Jin, Y.-S., et al. (2016). Gene Transcription Repression inClostridium Beijerinckiiusing CRISPR-dCas9. Biotechnol. Bioeng. 113, 2739–2743. doi:10.1002/bit.26020
Wen, Z., Minton, N. P., Zhang, Y., Li, Q., Liu, J., Jiang, Y., et al. (2017). Enhanced Solvent Production by Metabolic Engineering of a Twin-Clostridial Consortium. Metab. Eng. 39, 38–48. doi:10.1016/j.ymben.2016.10.013
Werner, J. N., Shi, H., Hsin, J., Huang, K. C., Gitai, Z., and Klein, E. A. (2020). AimB Is a Small Protein Regulator of Cell Size and MreB Assembly. Biophysical J. 119, 593–604. doi:10.1016/j.bpj.2020.04.029
Wiles, T. J., Schlomann, B. H., Wall, E. S., Betancourt, R., Parthasarathy, R., and Guillemin, K. (2020). Swimming Motility of a Gut Bacterial Symbiont Promotes Resistance to Intestinal Expulsion and Enhances Inflammation. PLoS Biol. 18, e3000661. doi:10.1371/journal.pbio.3000661
Williams McMackin, E. A., Marsden, A. E., and Yahr, T. L. (2019). H-NS Family Members MvaT and MvaU Regulate the Pseudomonas aeruginosa Type III Secretion System. J. Bacteriol. 201, 1. doi:10.1128/JB.00054-19
Woolston, B. M., Emerson, D. F., Currie, D. H., and Stephanopoulos, G. (2018). Rediverting Carbon Flux in Clostridium Ljungdahlii Using CRISPR Interference (CRISPRi). Metab. Eng. 48, 243–253. doi:10.1016/j.ymben.2018.06.006
Wu, J., Cheng, Z.-H., Min, D., Cheng, L., He, R.-L., Liu, D.-F., et al. (2020). CRISPRi System as an Efficient, Simple Platform for Rapid Identification of Genes Involved in Pollutant Transformation by Aeromonas Hydrophila. Environ. Sci. Technol. 54, 3306–3315. doi:10.1021/acs.est.9b07191
Wu, X., Zha, J., Koffas, M. A. G., and Dordick, J. S. (2019). ReducingStaphylococcus Aureusresistance to Lysostaphin Using CRISPR‐dCas9. Biotechnol. Bioeng. 116, 3149–3159. doi:10.1002/bit.27143
Wurihan, W., Huang, Y., Weber, A. M., Wu, X., and Fan, H. (2019). Nonspecific Toxicities of Streptococcus Pyogenes and Staphylococcus aureus dCas9 in Chlamydia trachomatis. Pathogens Dis. 77, ftaa005. doi:10.1093/femspd/ftaa005
Xiang, L., Qi, F., Jiang, L., Tan, J., Deng, C., Wei, Z., et al. (2020). CRISPR‐dCas9‐mediated Knockdown of prtR , an Essential Gene in Pseudomonas aeruginosa. Lett. Appl. Microbiol. 71, 386–393. doi:10.1111/lam.13337
Xiao, J., Jia, H., Pan, L., Li, Z., Lv, L., Du, B., et al. (2019). Application of the CRISPRi System to Repress sepF Expression in Mycobacterium Smegmatis. Infect. Genet. Evol. 72, 183–190. doi:10.1016/j.meegid.2018.06.033
Xiong, Z.-Q., Wei, Y.-Y., Kong, L.-H., Song, X., Yi, H.-X., and Ai, L.-Z. (2020). Short Communication: An Inducible CRISPR/dCas9 Gene Repression System in Lactococcus Lactis. J. Dairy Sci. 103, 161–165. doi:10.3168/jds.2019-17346
Xu, Z., Li, Y., Cao, H., Si, M., Zhang, G., Woo, P. C. Y., et al. (2021). A Transferrable and Integrative Type I-F Cascade for Heterologous Genome Editing and Transcription Modulation. Nucleic Acids Res. 49, e94. doi:10.1093/nar/gkab521
Yamada, S., Suzuki, Y., Kouzuma, A., and Watanabe, K. (2022). Development of a CRISPR Interference System for Selective Gene Knockdown in Acidithiobacillus Ferrooxidans. J. Biosci. Bioeng. 133, 105–109. doi:10.1016/j.jbiosc.2021.10.012
Yan, Y.-S., Yang, Y.-Q., Zou, L.-S., Zhang, L., and Xia, H.-Y. (2022). MilR3, a Unique SARP Family Pleiotropic Regulator in Streptomyces Bingchenggensis. Europepmc. [Preprint]. doi:10.21203/rs.3.rs-1248187/v1
Yao, L., Cengic, I., Anfelt, J., and Hudson, E. P. (2016). Multiple Gene Repression in Cyanobacteria Using CRISPRi. ACS Synth. Biol. 5, 207–212. doi:10.1021/acssynbio.5b00264
Yao, L., Shabestary, K., Björk, S. M., Asplund-Samuelsson, J., Joensson, H. N., Jahn, M., et al. (2020). Pooled CRISPRi Screening of the Cyanobacterium Synechocystis Sp PCC 6803 for Enhanced Industrial Phenotypes. Nat. Commun. 11, 1666. doi:10.1038/s41467-020-15491-7
Ye, W., Liu, T., Zhu, M., Zhang, W., Huang, Z., Li, S., et al. (2019). An Easy and Efficient Strategy for the Enhancement of Epothilone Production Mediated by TALE-TF and CRISPR/dcas9 Systems in Sorangium Cellulosum. Front. Bioeng. Biotechnol. 7, 334. doi:10.3389/fbioe.2019.00334
Yi, Y.-C., and Ng, I.-S. (2021). Redirection of Metabolic Flux in Shewanella Oneidensis MR-1 by CRISPRi and Modular Design for 5-aminolevulinic Acid Production. Bioresour. Bioprocess. 8, 13. doi:10.1186/s40643-021-00366-6
Yoon, J., and Woo, H. M. (2018). CRISPR Interference-Mediated Metabolic Engineering ofCorynebacterium Glutamicumfor Homo-Butyrate Production. Biotechnol. Bioeng. 115, 2067–2074. doi:10.1002/bit.26720
Yu, L., Su, W., Fey, P. D., Liu, F., and Du, L. (2018). Yield Improvement of the Anti-MRSA Antibiotics WAP-8294A by CRISPR/dCas9 Combined with Refactoring Self-Protection Genes inLysobacter enzymogenesOH11. ACS Synth. Biol. 7, 258–266. doi:10.1021/acssynbio.7b00293
Yunus, I. S., Anfelt, J., Sporre, E., Miao, R., Hudson, E. P., and Jones, P. R. (2022). Synthetic Metabolic Pathways for Conversion of CO2 into Secreted Short-To Medium-Chain Hydrocarbons Using Cyanobacteria. Metab. Eng. 72, 14–23. doi:10.1016/j.ymben.2022.01.017
Zetsche, B., Gootenberg, J. S., Abudayyeh, O. O., Slaymaker, I. M., Makarova, K. S., Essletzbichler, P., et al. (2015). Cpf1 Is a Single RNA-Guided Endonuclease of a Class 2 CRISPR-Cas System. Cell 163, 759–771. doi:10.1016/j.cell.2015.09.038
Zhan, Y., Xu, Y., Zheng, P., He, M., Sun, S., Wang, D., et al. (2020). Establishment and Application of Multiplexed CRISPR Interference System in Bacillus Licheniformis. Appl. Microbiol. Biotechnol. 104, 391–403. doi:10.1007/s00253-019-10230-5
Zhang, B., Liu, Z.-Q., Liu, C., and Zheng, Y.-G. (2016). Application of CRISPRi in Corynebacterium Glutamicum for Shikimic Acid Production. Biotechnol. Lett. 38, 2153–2161. doi:10.1007/s10529-016-2207-z
Zhang, K., Zhang, Z., Kang, J., Chen, J., Liu, J., Gao, N., et al. (2020a). CRISPR/Cas13d-Mediated Microbial RNA Knockdown. Front. Bioeng. Biotechnol. 8, 856. doi:10.3389/fbioe.2020.00856
Zhang, L., Ramijan, K., Carrión, V. J., van der Aart, L. T., Willemse, J., van Wezel, G. P., et al. (2021). An Alternative and Conserved Cell Wall Enzyme that Can Substitute for the Lipid II Synthase MurG. mBio 12. doi:10.1128/mBio.03381-20
Zhang, L., Willemse, J., Yagüe, P., de Waal, E., Claessen, D., and van Wezel, G. P. (2020b). Branching of Sporogenic Aerial Hyphae in sflA and sflB Mutants of Streptomyces Coelicolor Correlates to Ectopic Localization of DivIVA and FtsZ in Time and Space. bioRxiv. [Preprint], 424426. doi:10.1101/2020.12.26.424426
Zhang, S., and Voigt, C. A. (2018). Engineered dCas9 with Reduced Toxicity in Bacteria: Implications for Genetic Circuit Design. Nucleic Acids Res. 46, 11115–11125. doi:10.1093/nar/gky884
Zhang, X., Wang, J., Cheng, Q., Zheng, X., Zhao, G., and Wang, J. (2017). Multiplex Gene Regulation by CRISPR-ddCpf1. Cell Discov. 3, 1–9. doi:10.1038/celldisc.2017.18
Zhang, Y., and Yuan, J. (2021). CRISPR/Cas12a‐mediated Genome Engineering in the Photosynthetic Bacterium Rhodobacter Capsulatus. Microb. Biotechnol. 14, 2700–2710. doi:10.1111/1751-7915.13805
Zhao, C., Shu, X., and Sun, B. (2017). Construction of a Gene Knockdown System Based on Catalytically Inactive (“Dead”) Cas9 (dCas9) in Staphylococcus aureus. Appl. Environ. Microbiol. 83, 1. doi:10.1128/AEM.00291-17
Zhao, R., Liu, Y., Zhang, H., Chai, C., Wang, J., Jiang, W., et al. (2019). CRISPR-Cas12a-Mediated Gene Deletion and Regulation in Clostridium Ljungdahlii and its Application in Carbon Flux Redirection in Synthesis Gas Fermentation. ACS Synth. Biol. 8, 2270–2279. doi:10.1021/acssynbio.9b00033
Zhao, X., Zheng, H., Zhen, J., Shu, W., Yang, S., Xu, J., et al. (2020). Multiplex Genetic Engineering Improves Endogenous Expression of Mesophilic α-amylase Gene in a Wild Strain Bacillus Amyloliquefaciens 205. Int. J. Biol. Macromol. 165, 609–618. doi:10.1016/j.ijbiomac.2020.09.210
Zhao, Y., Li, L., Zheng, G., Jiang, W., Deng, Z., Wang, Z., et al. (2018). CRISPR/dCas9-Mediated Multiplex Gene Repression inStreptomyces. Biotechnol. J. 13, 1800121. doi:10.1002/biot.201800121
Keywords: bacterial gene regulation, CRISPR interference (CRISPRi), CRISPR activation (CRISPRa), transcriptional interference, transcriptional activation, non-model bacteria, genome-wide library
Citation: Call SN and Andrews LB (2022) CRISPR-Based Approaches for Gene Regulation in Non-Model Bacteria. Front. Genome Ed. 4:892304. doi: 10.3389/fgeed.2022.892304
Received: 08 March 2022; Accepted: 11 April 2022;
Published: 23 June 2022.
Edited by:
Yunzi Luo, Tianjin University, ChinaReviewed by:
Jin Wang, Shenzhen Second People’s Hospital, ChinaCopyright © 2022 Call and Andrews. This is an open-access article distributed under the terms of the Creative Commons Attribution License (CC BY). The use, distribution or reproduction in other forums is permitted, provided the original author(s) and the copyright owner(s) are credited and that the original publication in this journal is cited, in accordance with accepted academic practice. No use, distribution or reproduction is permitted which does not comply with these terms.
*Correspondence: Lauren B. Andrews, lbandrews@umass.edu