- 1International Rice Research Institute, Rice Genetic Design and Validation Unit, Rice Breeding Innovations, Los Baños, Philippines
- 2Department of Biology and Biotechnology “L. Spallanzani”, University of Pavia, Pavia, Italy
Developing nutritious rice with a higher yield is one approach to alleviating the problem of micronutrient deficiency in developing countries, especially human malnutrition involving zinc and iron (Fe) deficiency, and achieving better adoption. The transport of micronutrients such as Fe and Zn is mainly regulated via the nicotianamine synthase (OsNAS) gene family, whereas yield is a complex trait that involves multiple loci. Genome editing via CRISPR (clustered regularly interspaced short palindromic repeat)-Cas9, focusing on the OsNAS2 promoter, particularly the deletion of the cis-regulatory element ARR1AT at position −933, was conducted for an enhanced accumulation of Zn in the grain and per plant. The results showed that our promoter editing increased Zn concentration per plant. Evidence also showed that an improved spikelet number per main panicle led to increased grain per plant. The traits were inherited in “transgene-free” and homozygous plant progenies. Further investigation needs to be conducted to validate trait performance under field conditions and elucidate the cause of the spikelet increase.
1 Introduction
A simple meal can satisfy hunger, but only an enriched, nutritious meal can help overcome “hidden hunger.” Nutrient deficiency is more common in Africa, South Asia, and Latin America, affecting approximately three billion people worldwide (Kumar et al., 2019). Widespread malnutrition deficiencies are iron-deficiency anemia, zinc deficiency, and vitamin A deficiency, which cause severe and long-lasting effects on the human body, such as stunting intellectual and physical growth or diminishing immune system response (Majumder et al., 2019). During pregnancy, a diet with malnutrition increases the risk of giving birth to a low-birth-weight baby, thus affecting fetal growth and even risking the infant’s survival (Kumar et al., 2019).
Micronutrients play various key roles in plant metabolism and homeostasis. One essential micronutrient is zinc (Zn), which is involved in numerous functions or biochemical processes in plants, such as acting as a cofactor, synthesizing nucleotides, and activating enzymes or plant growth hormones (e.g., auxin). Similarly, Zn is involved in plant growth and metabolic processes, such as gene expression and the synthesis of proteins, lipids, and nucleic acids (Zaman et al., 2018). Iron (Fe) is also a vital micronutrient that plays a significant role in plant physiological and biochemical pathways. It is a component of various enzymes, such as cytochromes, and is involved in the maintenance of chloroplast structure and function, as well as chlorophyll synthesis and respiration (Rout and Sahoo, 2015). However, most staple foods, such as rice, maize, and wheat, are inherently low in micronutrient content and cannot meet the daily requirements. Therefore, a growing challenge is to increase the nutritional value of crops, especially rice. Approximately 3.5 billion people consume rice; however, because of the extensive grain processing (polishing, steaming, and parboiling), rice loses a considerable quantity of micronutrients before consumption (Prom-u-thai et al., 2020). The nutritional quality of polished rice needs to be increased for Zn and Fe by 12 ppm and 11 ppm, respectively, from their baselines (2 ppm for Fe and 16 ppm for Zn) to be able to meet the 30% estimated average requirement (EAR) (Van Der Straeten et al., 2020). Because of constraints on the limited natural rice variation with increased Zn and Fe content in conventional breeding, genetic engineering is a helpful tool for generating rice plants with elevated micronutrient content. Transgenic approaches mainly target the key genes for the translocation, storage, and uptake of Fe and Zn. Different strategies, such as overexpression of single or multiple genes or silencing, successfully increased grain Fe and Zn concentrations.
Studies have reported increased micronutrient concentrations by overexpressing the OsNAS2 gene in rice (Johnson et al., 2011) or by combining genes (OsNAS2 and HvNAAT), which revealed an elevated Fe concentration of up to 55 μg/g (Diaz-Benito et al., 2018). Trijatmiko et al. (2016) reported encouraging results with up to 15 μg/g Fe and 45.7 μg/g Zn in polished rice from transgenic plants grown under field conditions. Wu et al. (2019) created transgenic rice lines expressing AtNRAMP3, AtNAS1, and PvFer cassettes that reached 48.18 μg/g Zn and 13.65 μg/g Fe in brown rice. The majority of studies have reported increased Zn/Fe concentrations through the insertion of genetic material from the same or different species into the rice genome to enhance their uptake, translocation, or storage (Ludwig and Slamet-Loedin, 2019). Future strategies for rice biofortification will use the CRISPR system, which has become a standard tool in gene editing. One important aspect is the possibility of fast-generating “transgene-free” plants with the desired trait improvement.
Many agricultural traits are related to yield indirectly, such as plant height, panicle length, seed length, and growth period, or directly, such as spikelet number per plant, panicle number per plant, and 1000-grain weight (Li et al., 2019). Using CRISPR-Cas9, several attempts have been made to improve rice yield. The target genes were Gn1a (grain number 1a), DEP1 (dense and erect panicle 1), GS3 (grain size 3), and IPA1 (ideal plant architecture 1). T2 generation mutation analysis revealed enhanced grain number, dense erect panicles, and larger grain size (Li et al., 2016). Other studies involved OsGS3, OsGW2, and OsGn1a in a multiplex genome-editing approach, resulting in a triplet mutant and 30%–68% more yield per panicle (Zhou et al., 2019). In addition, phytohormones are known to be related to yield, such as abscisic acid (ABA) and cytokinin (CK), which are involved in plant growth and development and stress response. The ABA receptors pyrabactin resistance 1-like (PYL1), PYL4, and PYL6 were targeted using a genome-editing approach, resulting in up to 31% more grain than that in the wild-type counterparts under field conditions (Miao et al., 2018). Edited plants with mutated OsLOG5, targeting the rice cytokinin-activation enzyme-like gene, display seed traits with significantly higher numbers (increased seed setting rate, total grain number, grain number per panicle, and 1000-grain weight) than the control plants (Wang et al., 2020).
OsNAS2 and other Zn transporter and acquisition genes are known to be regulated via the type-B OsRR-mediated signaling pathway (Gao et al., 2019). The possible disruption of the binding site, known as the ARR1AT motif, of OsRR could likely decrease the inhibitory effect on Zn uptake and/or translocation and lead to increased Zn concentration within the crop plant and/or grain. The Zn concentration is correlated with cytokinin concentration (Gao et al., 2019): higher Zn per plant could lead to an increase in cytokinin concentration and, subsequently, an improvement in yield-related traits such as panicle branching and seed development.
This research studied the OsNAS2 promoter sequence modification introduced using the genome-editing tool CRISPR in the elite rice variety IR64. Three IR64-CRISPR lines targeting an ARR1AT motif were analyzed over several generations, and candidate plants were selected based on increased Zn and Fe concentrations in rice grain, the absence of the nuclease and biomarker, and a possible homozygous mutation. Consequently, the T3 “transgene-free” and homozygous plants were analyzed for yield-related traits and micronutrient concentrations.
2 Materials and methods
2.1 Promoter cis-regulatory element analysis
The promoter was analyzed for locating CREs using New PLACE (https://www.dna.affrc.go.jp/PLACE/?action=newplace, Higo et al., 1999). The region 1,000 bp upstream of the translational OsNAS2 start was analyzed, and all ARR1ATs and GTGANTG10 CREs were identified to select the possible on-target site. Subsequently, the edited on-target region was analyzed to identify the missing ARR1AT motif in the candidate plants.
2.2 Designing of single-guide RNA, vector construct, and indica rice transformation
The single-guide RNA (sgRNA) sequences targeting the OsNAS2 promoter were identified using CRISPR-P (http://crispr.hzau.edu.cn/CRISPR/, Version 1.0, Lei et al., 2014). Oryza indica (ASM465v1) was used as the reference genome, and the reference sequence was obtained from the Gramene Oryza sativa indica group (ASM465v1, www.gramene.org). sgRNA was selected based on the on-target score and off-target sites (Table 1). A compact CRISPR vector system was used to generate genetically modified plants. The cloning and Agrobacterium transformation were performed as described in Ludwig and Slamet-Loedin (2020) to develop a CRISPR construct (Supplementary Figure S1), namely, IRS1421 (sgRNA11).
2.3 Plant growth
After being generated in the tissue culture facility, the T0 seedlings were transferred into the CL4 greenhouse bays at the International Rice Research Institute (IRRI). The genome-edited plants were planted singly into pots and grown at 28°C ± 7°C day temperature and 23°C ± 4°C night temperature. For the analysis of T1, T2, and T3 plant generations, the dormancy of 25–50 rice seeds per plant line was broken at 45°C. The seeds were seeded and, after germination, sown on soil-filled trays. After 18 days, the rice seedlings were transplanted Into the wet bed or wet bed and pot (T2 generation) of the transgenic screenhouse at IRRI.
2.4 Nuclease and biomarker screening
In the T0 and following generations, the presence of the Cas9 nuclease and the biomarker hygromycin phosphotransferase (HPH) was determined via a PCR approach. The KAPA3G Plant PCR Kit (Sigma-Aldrich) or the Phire Plant Direct PCR Kit (Thermo Fisher) were used for amplification with specifically designed Cas9 primers, generating a 2,132-bp amplicon in the presence of the nuclease. For the identification of HPH, a 554-bp PCR amplicon was detected. The same primers were used to analyze the T1, T2, and T3 rice plant generations for “transgene-free” plants.
2.5 T7 endonuclease assay
The sgRNA11 on-target site was amplified in a 15 µL PCR mixture using specific primers (Supplementary Table S1), and the PCR products (5 µL) were analyzed by 1% agarose gel electrophoresis. Only plants with strong single-amplicon signals with the expected size were used for the T7E1 assay. A measure of 4–5 µl of the PCR product was mixed with NEBuffer 2 and incubated at 95°C for 10 min, 85°C for 5 min, and 25°C for 5 min and stored at 10°C. Afterward, 0.5 U T7 endonuclease 1 was added, and the mixture was incubated at 37°C for 1 h. Consequently, the reaction products were analyzed via gel electrophoresis. Because of the inability to differenciate between homozygous and wild type plants, all single amplicon samples were reanazlyed as follow to determine the zygosity: the genome-edited PCR amplicon was mixed with the IR64 wild-type PCR amplicon (1:1) and analyzed as described before.
2.6 Seed processing for X-ray fluorescence and/or inductively coupled plasma-optical emission spectrometry
Paddy rice was manually dissected to remove the hull; it was broken open using forceps to extract the brown rice seed. A measure of 3–4 g of brown rice (∼150 seeds) was prepared for X-ray fluorescence (XRF) measurement. Each sample was measured twice.
For inductively coupled plasma-optical emission spectrometry (ICP-OES), the samples were polished for 55 s using a Kett Pearlest Grain Polisher. The rice seeds were then visually examined for uncleanliness and corrected if necessary.
2.7 Amplification of sgRNA on-target site and sequencing
Specific primers (Supplementary Table S1) were designed to amplify the target site of sgRNA11. An 898-bp PCR amplicon containing the target region of genome-edited rice lines was either directly sent for sequencing or cloned into the sequencing vector pGEM-T Easy or TOPO-TA for further analysis.
2.8 T0 plant analysis
The leaf samples of the T0-generated IR64 seedlings were taken, and genomic DNA was extracted using a modified CTAB method. All regenerated plants were screened for the presence of the Cas9 nuclease and biomarker HPH via a PCR approach to detect the existence of T-DNA in the T0 generation. Plants without T-DNA insertion were discarded. The remaining plants were screened via the T7E1 assay to determine mutations within the region of interest. Subsequently, the promoter sites were amplified with specific primers (Supplementary Table S1) and sequenced by Sanger sequencing. The sequencing files were analyzed using Synthego ICE (https://ice.synthego.com/#/, Conant et al., 2022) to evaluate and identify variations in the amplicons. The seeds were harvested and processed. Brown rice seeds were evaluated for micronutrient content via XRF to detect plants with higher Zn and Fe concentrations than those of the control plants (tissue culture control named TC).
2.9 T1/T2/T3 plant analysis
Three candidate events (IR64-IRS1421-001, IR64-IRS1421-026, and IR64-IRS1421-036) were selected from the T0 generation for further analysis. The subsequent generations were analyzed to confirm the germline-editing search for “transgene-free” plants and higher Zn and Fe concentrations. All plants were analyzed via PCR for the presence of the Cas9 nuclease and the biomarker HPH to identify “transgene-free” rice plants. Brown rice (T3 and T4 seeds) and polished rice (T2 seeds) were submitted for XRF and ICP-OES analyses, respectively. Furthermore, based on the screening results and micronutrient concentrations, “transgene-free” or candidate plants (nuclease- or biomarker-free) were evaluated for sequence variation in the sgRNA11 target sites via the T7E1 assay. Consequently, PCR productor Plasmid DNA was sent (T2 and T3 plants) or plasmid DNA (T1 plants) were sent for Sanger sequencing. Through Synthego ICE, the sequencing files were analyzed to identify variations within the target sequence. Agronomic characteristics of the T3 plant generation, such as plant yield, plant height, tiller number, and panicle characteristics, were analyzed compared to wild-type plants with edited “transgene-free” generations.
2.10 Panicle analysis
The main panicle was harvested and then spread on a white background. Pictures were taken using a Nikon D5200 camera (lens: Nikon DX AF-S NIKKOR 18–105 mm 1:3.5-56G ED). The panicle analysis was performed using the widely used open-source image analysis software ImageJ (Schneider et al., 2012). Different panicle traits were analyzed: rachis length, primary branch number (PBN), secondary branch number (SBN), primary branch average length (PBL), secondary branch average length (SBL), secondary branches per primary branch (SBperPB), and spikelet number.
2.11 Off-target analysis
Off-target analysis was carried out using PCR and a T7 endonuclease assay. Specific primers (Supplementary Table S2) were used to amplify the identified 10 off-target sites of sgRNA11 using either the KAPA3G Plant PCR Kit (Sigma-Aldrich) or the Phire Plant Direct PCR Kit (Thermo Fisher). A measure of 5 µl of the PCR product was analyzed by 1% agarose gel electrophoresis. The T7E1 assay was then performed on the samples with the expected band size. A measure of 3 µl of the PCR product was mixed with NEBuffer 2, water, and 3 µL of IR64-wild-type PCR amplicon and incubated at 95°C for 10 min, 85°C for 5 min, and 25°C for 5 min and stored at 10°C. T7 endonuclease 1 (0.5 U) was added, and the mixture was incubated at 37°C. Lastly, digestion products were visualized via 1.2% agarose gel electrophoresis.
2.12 RT-PCR analysis
Leaf samples were harvested in the late-flowering stage, and total RNA was extracted utilizing the RNeasy Mini Kit with the RNase-Free DNase Set (QIAGEN), according to the manufacturer’s instructions. Subsequently, reverse transcription was performed using the Transcriptor First Strand cDNA Synthesis Kit (Roche). Quantitative PCR was then conducted in 20 μL reaction volumes using the SYBR Select Master Mix (Applied Biosystems) on a 7500 Fast Real-Time PCR system (Applied Biosystems). The primer efficiency of each oligonucleotide was calculated using the following dilution series: 1, 1/2, 1/4, 1/8, 1/16, and 1/32. Using the Pfaffl method, the efficiency-corrected relative gene expression was calculated with reference to the housekeeping gene OsTPI. For each edited rice line and wild-type, three and nine biological replicates were analyzed, respectively. Three technical replicates were measured for each sample.
3 Results
3.1 Promoter cis-regulatory element analysis
A 1-kb-upstream OsNAS2 promoter analysis revealed a total of 11 ARR1AT (site #S000454) and 6 GTGANTG10 (site #000378) motifs (Figure 1A), in which the target ARR1AT motif is at position −933 and was deleted through the genome editing approach. At the same time, the CRE GTGANTG10 motif at position −928 was disrupted as well. The ARR1AT motif is an ARR1-binding element identified in Arabidopsis thaliana as a response regulator (Sakai et al., 2000) and is linked to cytokinin regulation (Melton et al., 2019). GTGANTG10, with its GTGA motif, is also found in the tobacco (Nicotiana tabacum) promoter of the late pollen gene g10 and is a homolog of the tomato gene lat56, regulating sperm cell-specific gene expression (Rogers et al., 2001).
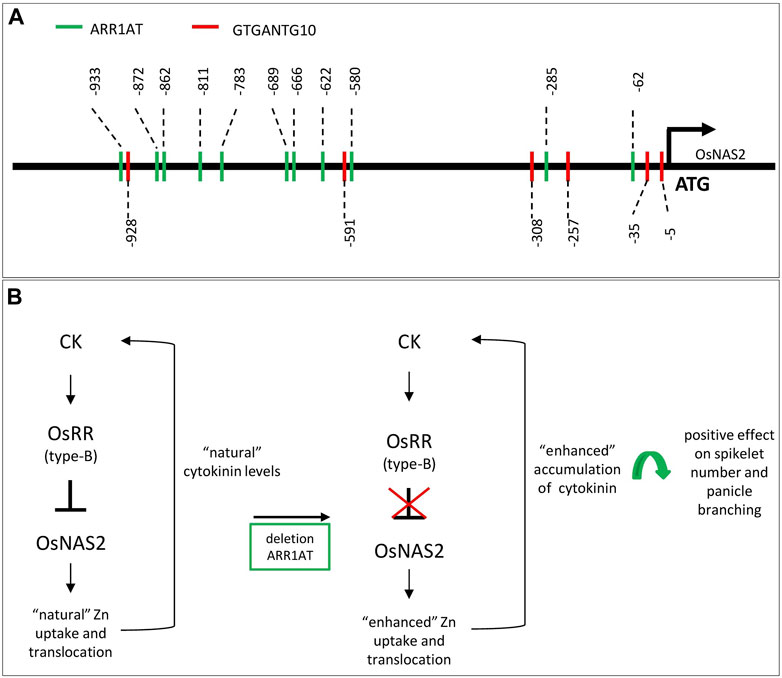
FIGURE 1. Promoter structure of OsNAS2 in elite rice IR64 and the theorized mechanism for enhanced Zn concentration in rice grain: (A) identification of CRE ARR1AT (in green) and GTGANTG10 (in red) within the 1-kb promoter region. (B) Hypothetical network of the positive effect on seed number and panicle branching after disruption of the ARR1AT motif in the OsNAS2 promoter region. CK, cytokinin; OsRR, Oryza sativa spp. response regulators.
3.2 Analysis of elite rice variety IR64 plants edited by CRISPR-Cas9
3.2.1 T0 generation: transformation efficiency evaluation, CRISPR-Cas9-induced sequence variation, and Zn/Fe concentrations in candidate events
A total of 101 IR64-IRS1421 T0 plants were generated via Agrobacterium tumefaciens-mediated transformation. All generated T0 plants were screened for the Cas9 nuclease located close to the right border (RB) and the biomarker HPH located near the left border (LB) by PCR using sequence-specific oligonucleotides (Supplementary Table S1) to confirm the full T-DNA insertion. A transformation efficiency of 89.11% was detected, and overall, 92 plants (91.09% mutation rate) were identified with a sequence modification via the T7E1 assay (Table 2). Taking into consideration the T7E1 screening as well as the Cas9/HPH test, a total of 67 T0 plants were sequenced, and 29 different alleles were identified (Supplementary Figure S2).
Based on different sequencing results, 31 events were selected and evaluated for Zn and Fe concentrations via XRF. Three candidate events (IR64-IRS1421-001, IR64-IRS1421-026, and IR64-IRS1421-036) were selected, displaying diverse characteristics in identified sequence variation (Figure 2) and increased Zn/Fe concentrations in brown rice (Figure 3).
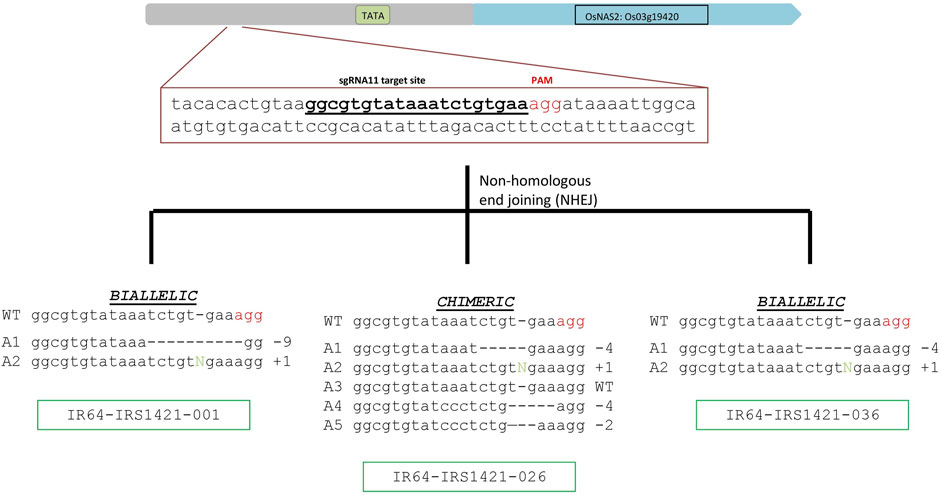
FIGURE 2. sgRNA target site and identified alleles in the selected T0 candidates: sgRNA11 target-site sequence and PAM (indicated in red letters), as well as the different alleles identified in the selected candidates. Two candidates are biallelic (IR64-IRS1421-001 and IR64-IRS1421-036), and IR64-IRS1421-026 is chimeric, with four different allele variations identified.
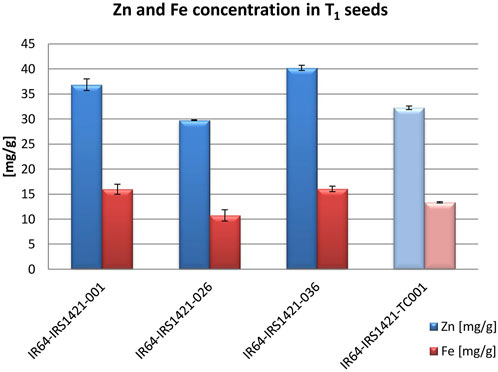
FIGURE 3. T1 seed Zn and Fe concentrations compared to the tissue culture control (TC): 150 brown rice seeds from each candidate and control plant were measured using XRF. Each sample was measured twice. The standard error indicates the difference in the measurements of each sample.
3.2.2 T1/T2 generation: screening and selection process to identify plant lines with increased Fe and Zn concentrations in rice grain
The genome-edited plants of the T1 and T2 generations were grown in the transgenic screenhouse at IRRI, and the leaf samples were taken 2–3 weeks after transplanting them into the wet bed (T1) or wet bed and pot (T2). Genomic DNA was extracted from young leaves and used for screening the presence and absence of the nuclease and biomarkers. IR64-IRS1421-001-T1 plant screening conferred two “transgene-free” plants (no Cas9 and HPH were detected) out of 20 plants. Most T1 plants still had the nuclease and/or biomarker presence (Table 2; Supplementary Figure S3). All 20 tested plants were analyzed via T7E1 and/or sequencing and were either still biallelic or had a wild-type allele. The IR64-IRS1421-036-T1 generation had three “transgene-free” plants, and the IR64-IRS1421-026-T1 generation displayed only one plant out of 19 or 20 plants, respectively. Similarly, for these two candidate lines, most plants thus far presented Cas9 and HPH amplicons (Table 2). Furthermore, the sequencing analysis and T7E1 screening showed biallelic or wild-type plants. Only IR64-IRS1421-026-007 was identified as a homozygote. Unfortunately, the plants still presented the Cas9 and HPH amplicons. After preliminary selection, 14 T2 rice seed samples were sent for ICP analysis (Supplementary Table S3). Considering the genotypic and phenotypic data, three candidate plants (IR64-IRS1421-001-009, IR64-IRS1421-026-007, and IR64-IRS1421-036-006) were chosen, one for each candidate event. The T2 plant generation was screened as described for the T1 plant generation. Only the IR64-IRS1421-001–009-T2 generation displayed four and two plants positive for Cas9 and HPH, respectively. Half or most of the total T2 plant generation still presented the nuclease and biomarker amplicon for the other two candidate lines (Table 2). An increased mutation rate (Table 2) was obtained in each generation by eliminating the heterozygous plants. Selection was carried out to generate “transgene-free” homozygous plants. The T3 seeds of eight candidate lines were sent for XRF analysis to determine the Zn and Fe concentrations in the brown rice (Supplementary Table S4). Selection for the following generation was first based on the Zn/Fe concentrations in the brown rice, followed by the presence and absence of Cas9 and HPH. For example, IR64-IRS1421-026-007 lines are “transgene-free” as opposed to IR64-IRS1421-001-009 and IR64-IRS1421-036-006 lines. Interestingly, the indels manifested within the germline (Figure 4) throughout the generations correspond to the increased Zn/Fe concentrations in the rice grain.
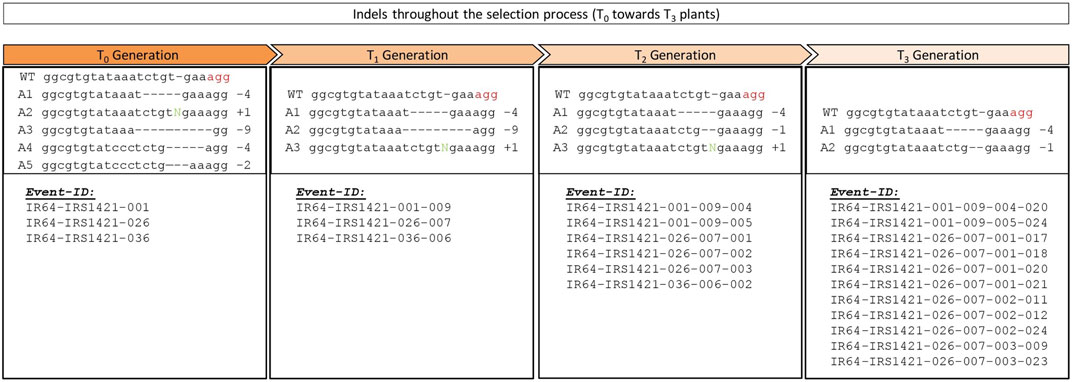
FIGURE 4. Allele variation throughout the selection process from T0 to T3: different alleles identified in each generation and their corresponding Event ID.
3.2.3 T3 generation: identification of “transgene-free” plants with a homozygous mutant in the promoter target region and their respective Zn/Fe concentrations in T4 brown rice
The majority of the plants in the T3 generation are Cas9-free or “transgene-free”, except for IR64-IRS1421-036-006-009, which has only three Cas9-free plants and all still display HPH (Supplementary Figure S3). However, the mutation rate within the T3 plant generation (192 plants in total) is in the range of 80%–100%, which is mainly homozygous (Table 2). The sequencing results of the homozygous plants display either a 4-bp or 1-bp deletion at the sgRNA11 on-target site (Figure 4). Furthermore, the Zn and Fe concentrations of T4 brown rice were detected via XRF. The estimated Zn concentration was calculated with respect to the total yield per plant to obtain an overview of the general Zn distribution per plant (Supplementary Figure S4). Taking these results into account, 11 “transgene-free” and homozygous rice plants were selected for further studies. The 4-bp-deletion plants displayed significantly higher grain number per plant, total grain weight per plant, flag leaf width, panicle length, filled grain number, and plant height but did not differ significantly in other phenotypic traits vis-à-vis the IR64 wild-type control (Table 3; Supplementary Table S5) and had a higher Zn concentration per plant than the wild type (Figure 5). Compared with the wild-type plant, the 1-bp deletion plants displayed significant differences in panicle count, 100-seed weight, and rachis length only (Supplementary Table S5). The types of mutations in these progenies correspond to the mutations in their parental lines (Figure 2).
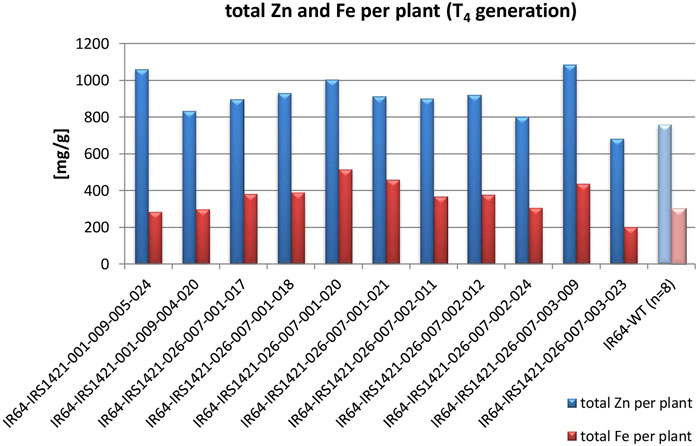
FIGURE 5. Estimated Zn and Fe concentrations per plant of selected T4 candidates compared to the IR64 wild-type control: Zn and Fe concentrations were measured via XRF, and the total Zn and Fe concentration per plant was calculated based on the total plant yield.
3.2.4 Analysis of different panicle traits of the selected 11 T3 candidate plants
Different panicle traits were analyzed: rachis length, primary branch average length (PBL), primary branch number (PBN), secondary branch average length (SBL), secondary branch number (SBN), secondary branches per primary branch (SBperPB), and spikelet number. Seven plants out of 11 displayed a longer rachis length than the wild-type control, and significant differences were observed for the 1-bp-deletion plants compared with the wild type. The majority of edited “transgene-free” plants showed a similar trend, but no significant differences in secondary branch traits (SBN and SBperPB) and spikelet number per main panicle were observed (Figures 6A–D). The remaining panicle characteristics did not differ from those of the wild-type plants (Supplementary Table S5; Supplementary Table S6).
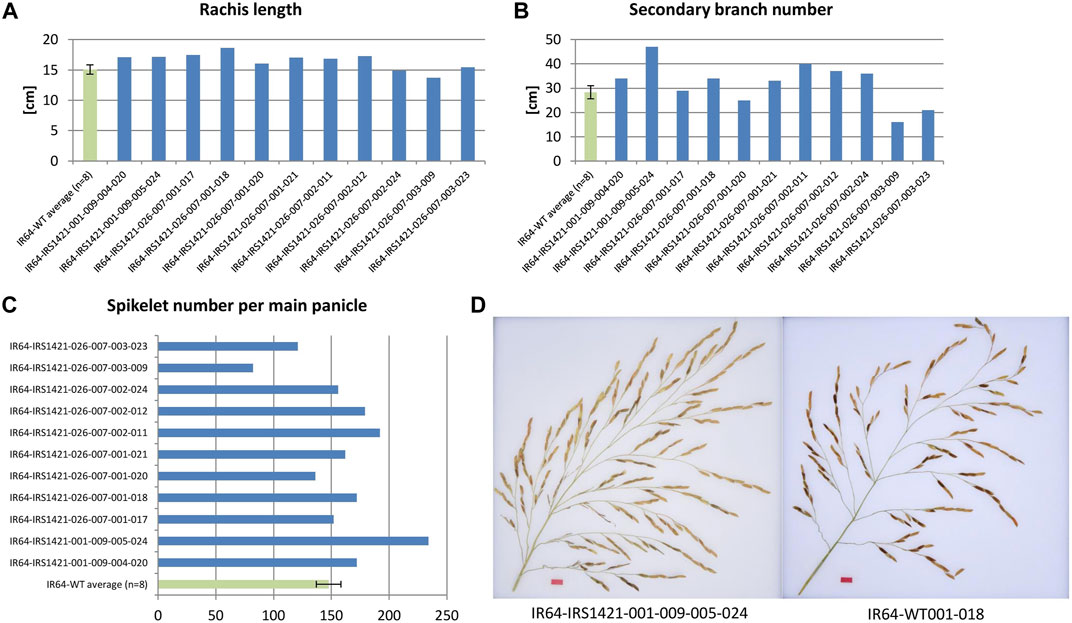
FIGURE 6. Presentation of measured panicle traits of T3 selected candidate plants. (A, B) Rachis length and secondary branch number (SBN) of the edited “transgene-free” plants compared to the IR64 wild-type plant (N = 8), respectively. (C) Spikelet number per main panicle of edited rice plants compared to the control (N = 8). (D) Example image of a main panicle of the edited plant IR64-IRS1421-001-009-005-024 and an IR64 wild-type plant.
3.2.5 Off-target analysis via the T7 endonuclease assay
All 11 plants were tested for off-target cutting via the T7 endonuclease assay. The off-target region was amplified using specifically designed oligonucleotides (Supplementary Table S2). All 11 rice plants in all 10 off-targets tested negative except for off-target 6, for which IR64-IRS1421-001-009-005-024 and IR64-IRS1421-001-009-004-020 displayed an extra band (Table 4; Supplementary Figure S5A). This one off-target cutting site is located in an intergenic region in chromosome 7 and does not affect any nearby genes. Furthermore, all 110 samples were sent for sequencing to confirm the T7E1 assay results (Supplementary Figure S5B).
3.2.6 RT-PCR analysis of T4 homozygous, “transgene-free” plants
Six high-performing edited rice lines were analyzed via RT-PCR, and the leaf samples at the late-flowering stage were used to measure OsNAS2 expression. Significantly higher expression of OsNAS2 compared to the wild-type control was measured for IR64-IRS1421-001-009-005-024 only. The other five selected rice lines displayed a similar trend (Supplementary Figure S7).
4 Discussion
The overall high sgRNA11 efficacy observed in this study suggests a good selection process based on the criteria at that time since the CRISPR-Cas9-based sequence mutation mainly depends on the specific cleavage and effectiveness of sgRNA. The unique nucleotide sequences (8–12 nt) at the 3′-end of sgRNA and the total length and nucleotide composition affect the on- and off-target efficiency (Uniyal et al., 2019). Furthermore, the secondary structure plays a significant role in the efficiency of the CRISPR-Cas9 system: weaker guide sequence structures are more efficient than stronger guide sequence structures, taking into account the CG content range (30%–80%) and base-pairing scores (Jensen et al., 2017; Javaid and Choi, 2021). In addition, a short T-rich sequence (TTTT) at the 3′-end of sgRNA should be avoided; this motif blocks the efficiency of the genome-editing CRISPR-Cas9 system (Graf et al., 2019).
The T7 endonuclease I assay was used to validate the CRISPR-Cas9 edits, which is inexpensive, simple to use, and more sensitive for detecting mutants than the Surveyor assay (Vouillot et al., 2015). Nonetheless, assay performance can be influenced by different aspects such as length and base-pair mismatch identity, flanking sequence, secondary structure of the target, and mutant sequence abundance (Sadowski, 1971; Dickie et al., 1987; Mashal et al., 1995). Unfortunately, the T7E1 assay is not able to detect homozygous mutations. To make it capable of identifying the homozygous plants, the PCR amplicon of the on-target site of wild-type and genome-edited plants was mixed (1:1). Already, earlier studies demonstrated that T7E1 had a maximum efficiency in a DNA pool containing only 5%–30% mutated DNA (Vouillot et al., 2015), which is sufficient for indel analysis.
Engineering higher micronutrient content (such as Zn) is a challenging task in staple crops because of the involvement of diverse processes, from uptake by the plant root to translocation and accumulation in the grain or edible plant parts (Gao et al., 2019). The leading work for biofortification is the overexpression of different genes or gene combinations involved in uptake, translocation, or storage acquired from the same or other species (Ludwig and Slamet-Loedin, 2019). Similar challenges are known for improving yield in crops since grain yield is a complex trait controlled by multiple loci. This study initially focused on developing rice with enhanced micronutrient content; however, editing of a single or a few base pairs in the promoter of a target gene through the CRISPR-Cas9 genome-editing tool shows an increase in Zn concentration of the grain in T4 seeds and also a potential increase in yield-related traits per plant. The OsNAS2 promoter was targeted because of the significant role of the OsNAS2 gene in the uptake and translocation of Zn/Fe in the rice plant. Evidence shows that minor editing happened at the cis-regulatory element ARR1AT. The CRE ARR1AT is known to be the binding element of ARR1 found in A. thaliana (Sakai et al., 2000). Throughout the selection process, rice plants with enhanced Zn and/or Fe concentrations were selected and analyzed for the presence of biomarkers, nucleases, presence (T0)/absence (T1 ongoing), and sequence variations. The selected high-Zn and high-plant-yield-edited plants showed either a 4-bp or 1-bp deletion at the ARR1AT motif at position −933 and a possible correlation with the detected increased micronutrient concentrations. A recent study demonstrated that cytokinin plays a key role in controlling Zn in plants and in involving type-B OsRR as a regulator for OsNAS genes (Figure 1B) (Gao et al., 2019). One single cytokinin response element has been recognized, which is a type-B response regulator linked to the core sequence 5′-GAT (T/C)-3′ in Arabidopsis (Brenner et al., 2012). Analysis of the 1-kb promoter region upstream of the translational start of OsNAS2 displayed 11 core sequence 5′-GAT (T/C)-3′ motifs (Figures A1–A). The Zn transporter and acquisition genes such as OsNAS2 are regulated via the type-B OsRR-mediated signaling pathway (Gao et al., 2019). We hypothesize that by destroying one of the possible binding sites (ARR1AT motif) of the OsRR regulators, the inhibitory effect was minimized or stopped, and a desirable enhanced Zn uptake or translocation could occur (Figures A1–B). Few in planta studies have described loss-of-function mutations, such as mutations within the core sequence of CRE in the ARR6 promoter that strongly decrease the responsiveness to cytokinin in Arabidopsis (Ramireddy, 2009). In the MYB family, 11 type-B ARRs containing this N-terminal CRE are thought to be negatively regulated (Muthamilarasan et al., 2014). Moreover, the motif deletion led to a constitutively active protein Iform in Arabidopsis (Gao et al., 2019). In rice, it was demonstrated that cytokinin activated the promoter of OsNSHB2 (non-symbiotic hemoglobin); deletions or mutations of the predicted ARR1-binding CRE in the promoter confirmed its function as a cytokinin response element. The motif deletion drastically decreased the inducibility of the promoter cytokinin (Ross et al., 2004). Gao et al. (2019) described the inhibitory effect of cytokinin on the expression of Zn acquisition and translocation genes (OsNAS, OsZIP, and OsHMAs) in rice. Moreover, studies have shown that cytokinin-deficient plants exhibit an elevated Zn concentration, and that Zn uptake is regulated by cytokinin in a dose-dependent manner (Gao et al., 2019). Accumulating data demonstrate that cytokinin plays an important role in responding to nutrient status (Sakakibara et al., 2006; Ramireddy et al., 2014; Gao et al., 2019) with its wide repression of nutrient transport (Sakakibara et al., 2006). Furthermore, it is known that cytokinin is involved in diverse physiological and developmental processes of the plant, such as shoot and root development, leaf expansion, vascular differentiation, senescence, and spikelet number per panicle (Deveshwar et al., 2020; Prasad, 2022). In addition, Gao et al. (2019) suggested that cytokinin concentration is correlated with Zn availability and that a possible spatial control of cytokinin metabolism could be exploited for crop nutritional improvement. Furthermore, cytokinin concentration affects SAM size and activity in plants and, subsequently, plant height, tiller number, and panicle branches (Du et al., 2017). The association of cytokinin with agronomic traits, particularly spikelet number, could be observed in the edited “transgene-free” plants.
Although Zn concentration still varies in T4 seeds, indications are given that an increased Zn concentration can be achieved by disrupting the ARR1AT motif. In addition, evidence shows that yield-related traits, such as spikelet number, increased in most selected plants. Much research is required to further analyze the following generation of currently achieved “transgene-free” homozygous plants. Despite that, it has been shown that the same genotype (gene, QTL, etc.) can have a diverse or even opposite effect in a different genetic background (Shen et al., 2018). A feasible next step could be backcrossing to eliminate possible somaclonal mutations to clean up the genetic background.
In this study, we hypothesize that the disruption of the CRE ARR1AT motif at position −933 in the OsNAS promoter can enhance Zn concentration in the rice plant and have a positive effect on yield-related traits such as spikelet number and panicle branching. The estimated total Zn concentration is still diverse, and yield-related traits still vary, but we can see a positive trend toward an increase. This diversity might be due to genetic background variations, which need further analysis. The traits need to be further validated under field conditions to elucidate the cause of the spikelet increase.
Data availability statement
The original contributions presented in the study are included in the article/Supplementary Material; further inquiries can be directed to the corresponding author.
Author contributions
YL: conceptualization, investigation, methodology, project administration, supervision, validation, visualization, writing–original draft, and writing–review and editing. CD: investigation, project administration, supervision, and writing–review and editing. EA: investigation, visualization, and writing–review and editing. RM-C: investigation and writing–review and editing. AK: writing–review and editing. RR: writing–review and editing. IS-L: funding acquisition, investigation, project administration, resources, supervision, validation, and writing–review and editing.
Funding
The author(s) declare that financial support was received for the research, authorship, and/or publication of this article. This research was funded by HarvestPlus: Developing high-iron and high-zinc transgenic rice to alleviate iron deficiency in Bangladesh and Southeast Asia (Agreement No. 2015H5315) and the United States Agency for International Development under the contribution agreement CGIAR (MTO No. 069033) designated to the IRRI OneRice project—Healthier Rice.
Acknowledgments
The authors are grateful to the Daniel Voytas Laboratory (University of Minnesota) for providing the CRISPR vector constructs.
Conflict of interest
The authors declare that the research was conducted in the absence of any commercial or financial relationships that could be construed as a potential conflict of interest.
Publisher’s note
All claims expressed in this article are solely those of the authors and do not necessarily represent those of their affiliated organizations, or those of the publisher, the editors, and the reviewers. Any product that may be evaluated in this article, or claim that may be made by its manufacturer, is not guaranteed or endorsed by the publisher.
Supplementary material
The Supplementary Material for this article can be found online at: https://www.frontiersin.org/articles/10.3389/fgeed.2023.1308228/full#supplementary-material
References
Brenner, W. G., Ramireddy, E., Heyl, A., and Schmülling, T. (2012). Gene regulation by cytokinin in Arabidopsis. Front. Plant Sci. 31 (3), 8. doi:10.3389/fpls.2012.00008
Conant, D., Hsiau, T., Rossi, N., Oki, J., Maures, T., Waite, K., et al. (2022). Inference of CRISPR edits from sanger trace data. CRISPR J. 5, 123–130. doi:10.1089/crispr.2021.0113
Deveshwar, P., Prusty, A., Sharma, S., and Tyagi, A. K. (2020). Phytohormone-mediated molecular mechanisms involving multiple genes and QTL govern grain number in rice. Front. Genet. 11, 586462. doi:10.3389/fgene.2020.586462
Diaz-Benito, P., Banakar, R., Rodriguez-Menendez, S., Capell, T., Pereiro, R., Christou, P., et al. (2018). Iron and zinc in the embryo and endosperm of rice (Oryza sativa L.) seeds in contrasting 2’-deoxymugineic acid/nicotianamine scenarios. Front. Plant Sci. 9, 1190. doi:10.3389/fpls.2018.01190
Dickie, P., McFadden, G., and Morgan, A. R. (1987). The site-specific cleavage of synthetic Holliday junction analogs and related branched DNA structures by bacteriophage T7 endonuclease I. J. Biol. Chem. 262, 14826–14836. doi:10.1016/s0021-9258(18)47870-2
Du, Y., Liu, L., Li, M., Fang, S., Shen, X., Chu, J., et al. (2017). UNBRANCHED3 regulates branching by modulating cytokinin biosynthesis and signaling in maize and rice. New Phytol. 214, 721–733. doi:10.1111/nph.14391
Gao, S., Xiao, Y., Xu, F., Gao, X., Cao, S., Zhang, F., et al. (2019). Cytokinin-dependent regulatory module underlies the maintenance of zinc nutrition in rice. New Phytol. 224, 202–215. doi:10.1111/nph.15962
Graf, R., Li, X., Chu, V. T., and Rajewsky, K. (2019). sgRNA sequence motifs blocking efficient CRISPR/Cas9-Mediated gene editing. Cell Rep. 26 (5), 1098–1103. doi:10.1016/j.celrep.2019.01.024
Higo, K., Ugawa, Y., Iwamoto, M., and Korenaga, T. (1999). Plant cis-acting regulatory DNA elements (PLACE) database: 1999. Nucl. Acids Res. 27 (1), 297–300. doi:10.1093/nar/27.1.297
Javaid, N., and Choi, S. (2021). CRISPR/Cas system and factors affecting its precision and efficiency. Front. Cell Dev. Biol. 24 (9), 761709. doi:10.3389/fcell.2021.761709
Jensen, K. T., Fløe, L., Petersen, T. S., Huang, J., Xu, F., Bolund, L., et al. (2017). Chromatin accessibility and guide sequence secondary structure affect CRISPR-Cas9 gene editing efficiency. FEBS Lett. 591 (13), 1892–1901. doi:10.1002/1873-3468.12707
Johnson, A. A. T., Kyriacou, B., Callahan, D. L., Carruthers, L., Stangoulis, J., Lombi, E., et al. (2011). Constitutive overexpression of the OsNAS gene family reveals single-gene strategies for effective iron- and zinc-biofortification of rice endosperm. PLoS ONE 6, e24476. doi:10.1371/journal.pone.0024476
Kumar, S., Palve, A., Joshi, C., Srivastava, R., and Rukhsar, S. (2019). Crop biofortification for iron (Fe), zinc (Zn), and vitamin A with transgenic approaches. Heliyon 15 (6), e01914. doi:10.1016/j.heliyon.2019.e01914
Lei, Y., Lu, L., Liu, H.-Y., Xing, F., and Chen, L.-L. (2014). CRISPR-P: a web tool for synthetic single-guide RNA design of CRISPR-system in plants. Mol. Plant 7 (9), 1494–1496. doi:10.1093/mp/ssu044
Li, M., Li, X., Zhou, Z., Wu, P., Fang, M., Pan, X., et al. (2016). Reassessment of the four yield-related genes Gn1a, DEP1, GS3, and IPA1 in rice using CRISPR/Cas9 system. Front. Plant Sci. 30, 377. doi:10.3389/fpls.2016.00377
Li, R., Li, M., Ashraf, U., Liu, S., and Zhang, J. (2019). Exploring the relationships between yield and yield-related traits for rice varieties released in China from 1978 to 2017. Front. Plant Sci. 10, 543. doi:10.3389/fpls.2019.00543
Ludwig, Y., and Slamet-Loedin, I. H. (2019). Genetic biofortification to enrich rice and wheat grain iron: from genes to product. Front. Plant Sci. 10, 833. doi:10.3389/fpls.2019.00833
Ludwig, Y., and Slamet-Loedin, I. H. (2020). “Rice gene knockout or downregulation through CRISPR-cas9,” in CRISPR-cas methods. Editors M. T. Islam, P. K. Bhowmik, and K. A. Molla (New York, NY, USA: Springer), 35–50. doi:10.1007/978-1-0716-0616-2_3
Majumder, S., Datta, K., and Datta, S. K. (2019). Rice biofortification: high iron, zinc, and vitamin-A to fight against “hidden hunger”. Agronomy 9, 803. doi:10.3390/agronomy9120803
Mashal, R. D., Koontz, J., and Sklar, J. (1995). Detection of mutations by cleavage of DNA heteroduplexes with bacteriophage resolvases. Nat. Genet. 9, 177–183. doi:10.1038/ng0295-177
Melton, A. E., Zwack, P. J., Rashotte, A. M., and Goertzen, L. R. (2019). Identification and functional characterization of the marshallia (asteraceae) clade III cytokinin response factor (CRF). Plant Signal Behav. 14 (9), e1633886. doi:10.1080/15592324.2019.1633886
Miao, C., Xiao, L., Zou, C., Zhao, Y., Bressan, R. A., Zhu, J.-K., et al. (2018). Mutations in a subfamily of abscisic acid receptor genes promote rice growth and productivity. PNAS 115 (23), 6058–6063. doi:10.1073/pnas.1804774115
Muthamilarasan, M., Khandelwal, R., Yadav, C. B., Bonthala, V. S., Khan, Y., and Prasad, M. (2014). Identification and molecular characterization of MYB Transcription Factor Superfamily in C4 model plant foxtail millet (Setaria italica L.). PLoS One 3 (10), e109920. doi:10.1371/journal.pone.0109920
Prasad, R. (2022). Cytokinin and its key role to enrich the plant nutrients and growth under adverse conditions: an update. Front. Genet. 13, 883924. doi:10.3389/fgene.2022.883924
Prom-u-thai, C., Rashid, A., Ram, H., Zou, C., Guimaraes Guilherme, L. R., Branco Corguinha, A. P., et al. (2020). Simultaneous biofortification of rice with zinc, iodine, iron and selenium through foliar treatment of a micronutrient cocktail in five countries. Front. Plant Sci. 13 (11), 589835. doi:10.3389/fpls.2020.589835
Ramireddy, E. (2009). Functional characterization of B-type response regulators of Arabidopsis thaliana. Dissertation. Germany, Berlin: Freie Universität Berlin.
Ramireddy, E., Chang, L., and Schmülling, T. (2014). Cytokinin as a mediator for regulating root system architecture in response to environmental cues. Plant Signal. Behav. 9, e27771. doi:10.4161/psb.27771
Rogers, H. J., Bate, N., Combe, J., Sullivan, J., Sweetman, J., Swan, C., et al. (2001). Functional analysis of cis-regulatory elements within the promoter of the tobacco late pollen gene g10. Plant Mol. Biol. 45, 577–585. doi:10.1023/a:1010695226241
Ross, E. J. H., Stone, J. M., Elowsky, C. G., Arredondo-Peter, R., Klucas, R. V., and Sarath, G. (2004). Activation of the Oryza sativa non-symbiotic haemoglobin-2 promoter by the cytokinin-regulated transcription factor, ARR1. J. Exp. Bot. 55, 1721–1731. doi:10.1093/jxb/erh211
Rout, G. R., and Sahoo, S. (2015). Role of iron in plant growth and metabolism. Rev. Agric. Sci. 3, 1–24. doi:10.7831/ras.3.1
Sadowski, P. D. (1971). Bacteriophage T7 endonuclease I: properties of the enzyme purified from T7 phage-infected Escherichia coli B. J. Biol. Chem. 246, 209–216. doi:10.1016/s0021-9258(18)62551-7
Sakai, H., Aoyama, T., and Oka, A. (2000). Arabidopsis ARR1 and ARR2 response regulators operate as transcriptional activators. Plant J. 24, 703–711. doi:10.1046/j.1365-313x.2000.00909.x
Sakakibara, H., Takei, K., and Hirose, N. (2006). Interactions between nitrogen and cytokinin in the regulation of metabolism and development. Trends Plant Sci. 11, 440–448. doi:10.1016/j.tplants.2006.07.004
Schneider, C. A., Rasband, W. S., and Eliceiri, K. W. (2012). NIH image to ImageJ: 25 years of image analysis. Nat. Methods 9 (7), 671–675. doi:10.1038/nmeth.2089
Shen, L., Wang, C., Fu, Y., Wang, J., Liu, Q., Zhang, X., et al. (2018). QTL editing confers opposing yield performance in different rice varieties. J. Integr. Plant Biol. 60 (2), 89–93. doi:10.1111/jipb.12501
Trijatmiko, K. R., Dueñas, C., Tsakirpaloglou, N., Torrizo, L., Arines, F. M., Adeva, C., et al. (2016). Biofortified indica rice attains iron and zinc nutrition dietary targets in the field. Sci. Rep. 6, 19792. doi:10.1038/srep19792
Uniyal, A. P., Mansotra, K., Yadav, S. K., and Kumar, V. (2019). An overview of designing and selection of sgRNAs for precise genome editing by the CRISPR-Cas9 system in plants. 3Biotech 9 (6), 223. doi:10.1007/s13205-019-1760-2
Van Der Straeten, D., Bhullar, N. K., De Steur, H., Gruissem, W., MacKenzie, D., Pfeiffer, W., et al. (2020). Multiplying the efficiency and impact of biofortification through metabolic engineering. Nat. Commun. 15, 5203. doi:10.1038/s41467-020-19020-4
Vouillot, L., Thélie, A., and Pollet, N. (2015). Comparison of T7E1 and Surveyor mismatch cleavage assays to detect mutations triggered by engineered nucleases. G3 7 (3), 407–415. doi:10.1534/g3.114.015834
Wang, C., Wang, G., Gao, Y., Lu, G., Habben, J. E., Mao, G., et al. (2020). A cytokinin-activation enzyme-like gene improves grain yield under various field conditions in rice. Plant Mol. Biol. 102 (4-5), 373–388. doi:10.1007/s11103-019-00952-5
Wu, T.-Y., Gruissem, W., and Bhullar, N. K. (2019). Targeting intracellular transport combined with efficient uptake and storage significantly increases grain iron and zinc levels in rice. Plant Biotechnol. J. 17 (1), 9–20. doi:10.1111/pbi.12943
Zaman, Q., Aslam, Z., Yaseen, M., Ihsan, M. Z., Khaliq, A., Fahad, S., et al. (2018). Zinc biofortification in rice: leveraging agriculture to moderate hidden hunger in developing countries. Arch. Agron. Soil Sci. 64, 147–161. doi:10.1080/03650340.2017.1338343
Keywords: biofortification, rice, CRISPR-Cas9, Zn, Fe, OsNAS2 promoter, promoter editing
Citation: Ludwig Y, Dueñas C Jr., Arcillas E, Macalalad-Cabral RJ, Kohli A, Reinke R and Slamet-Loedin IH (2024) CRISPR-mediated promoter editing of a cis-regulatory element of OsNAS2 increases Zn uptake/translocation and plant yield in rice. Front. Genome Ed. 5:1308228. doi: 10.3389/fgeed.2023.1308228
Received: 06 October 2023; Accepted: 27 December 2023;
Published: 23 January 2024.
Edited by:
Sheshu Madhav Maganti, Indian Institute of Rice Research (ICAR), IndiaReviewed by:
Santosh Kumar Gupta, National Institute of Plant Genome Research (NIPGR), IndiaValentine Otang Ntui, International Institute of Tropical Agriculture (IITA), Kenya
Copyright © 2024 Ludwig, Dueñas, Arcillas, Macalalad-Cabral, Kohli, Reinke and Slamet-Loedin. This is an open-access article distributed under the terms of the Creative Commons Attribution License (CC BY). The use, distribution or reproduction in other forums is permitted, provided the original author(s) and the copyright owner(s) are credited and that the original publication in this journal is cited, in accordance with accepted academic practice. No use, distribution or reproduction is permitted which does not comply with these terms.
*Correspondence: Inez H. Slamet-Loedin, aS5zbGFtZXQtbG9lZGluQGlycmkub3Jn