- Laboratoire de Chimie Bactérienne UMR 7283, Centre National de la Recherche Scientifique (CNRS), Aix-Marseille University, Marseille, France
Microbial Molecular hydrogen (H2) cycling plays an important role in several ecological niches. Hydrogenases (H2ases), enzymes involved in H2 metabolism, are of great interest for investigating microbial communities, and producing BioH2. To obtain an overall picture of the genetic ability of Cyanobacteria to produce H2ases, we conducted a phylum wide analysis of the distribution of the genes encoding these enzymes in 130 cyanobacterial genomes. The concomitant presence of the H2ase and genes involved in the maturation process, and that of well-conserved catalytic sites in the enzymes were the three minimal criteria used to classify a strain as being able to produce a functional H2ase. The [NiFe] H2ases were found to be the only enzymes present in this phylum. Fifty-five strains were found to be potentially able produce the bidirectional Hox enzyme and 33 to produce the uptake (Hup) enzyme. H2 metabolism in Cyanobacteria has a broad ecological distribution, since only the genomes of strains collected from the open ocean do not possess hox genes. In addition, the presence of H2ase was found to increase in the late branching clades of the phylogenetic tree of the species. Surprisingly, five cyanobacterial genomes were found to possess homologs of oxygen tolerant H2ases belonging to groups 1, 3b, and 3d. Overall, these data show that H2ases are widely distributed, and are therefore probably of great functional importance in Cyanobacteria. The present finding that homologs to oxygen-tolerant H2ases are present in this phylum opens new perspectives for applying the process of photosynthesis in the field of H2 production.
Introduction
Microbial hydrogen (H2) metabolism is a process that occurs in many different environments. In addition to being a key metabolic factor in several biological communities, H2 has attracted considerable interest as a candidate environmentally friendly energy carrier. The use of photosynthetic organisms such as microalgae and cyanobacteria has been tested worldwide for this purpose. In cyanobacteria, the main enzymes involved in H2 metabolism are nitrogenases and hydrogenases (H2ases) (Reviewed in Bothe et al., 2010). Nitrogenases fix molecular nitrogen (N2) and produce H2 as a byproduct (D'Eustachio and Hardy, 1964). H2ases are metalloprotein enzymes which catalyze in several microorganisms the reversible reaction:
(for a recent Review see Peters et al., 2015).
They are usually classified into three phylogenetically independent classes: [Fe] H2ases, [FeFe] H2ases, and [NiFe] H2ases (Vignais and Billoud, 2007). Since [Fe] H2ases are light-sensitive enzymes (Chen et al., 2002), they can be considered as for limited interest in the context of H2 photoproduction. The [FeFe] H2ases present in anaerobic bacteria and some phototrophic eukaryotes preferentially catalyze the evolution of H2 at high frequencies; these enzymes are also characterized by their high sensitivity to oxygen (O2) (Melis et al., 2000; Florin et al., 2001; Winkler et al., 2002; Peters et al., 2015). The [NiFe] H2ases, which have been found to exist in Archaea and in several aerobic and anaerobic bacterial phyla, are mainly involved in H2 oxidation but can also catalyze the reduction of protons to H2 (Vignais and Billoud, 2007). They consist of a large subunit containing the bimetallic center [NiFe] and a small subunit containing [FeS] clusters (Volbeda et al., 1995, 1996; Peters et al., 2015). Based on a phylogenetic analysis of the large subunit, and more specifically, on two highly conserved regions located in this subunit near the [NiFe] center (the L1 and L2 regions), the [NiFe] H2ases have been classified into the eight different groups presented in Table 1 (Vignais et al., 2001; Vignais and Billoud, 2007). The maturation of [NiFe] H2ases involves six proteins (HypFCDEAB), which synthesize the non-protein ligands (CO and CN) and assemble the active site (Dernedde et al., 1996; Hansel et al., 2001; Hoffmann et al., 2006). In the last step in the process of biosynthesis, the C terminal part of the large subunit is cleaved by a specific peptidase (Thiemermann et al., 1996; Devine et al., 2009).
Although the activity of most of the [NiFe] H2ases tends to be inhibited by O2, some members of this class remain active in the presence of O2 and have therefore been called O2-tolerant. The O2-tolerant H2ases described for the first time in the anoxigenic bacterium Rubrivivax gelatinosus (Maness et al., 2002) occur in the Group 1 membrane-bound H2ases (MBH), the H2-signaling group (RH, Group 2b) (Buhrke et al., 2005; Duché et al., 2005), the tetrameric bifunctional H2ases (group 3b) (Jenney and Adams, 2008; Kwan et al., 2015), the bidirectional H2ases (group 3d) (Horch et al., 2013; Karstens et al., 2015) and the recently identified Group 5 Actinobacterial-H2ases (Table 2) (Constant et al., 2010; Lubitz et al., 2014). In the case of the MBH enzymes, the main difference between the standard and tolerant members focuses on the [FeS] cluster located near the [NiFe] site. Instead of the canonical [4Fe4S] present in the standard enzymes, a [4Fe3S] cluster coordinated by six cysteine residues occurs in the tolerant enzymes (Pandelia et al., 2011; Shomura et al., 2011). This proximal [4Fe3S] is the most striking feature thought to be linked to O2-tolerance (Goris et al., 2011; Lukey et al., 2011). The O2-insensitivity of the RH-H2ases of Ralstonia eutropha H16 depends on the size and shape of the intramolecular hydrophobic cavity giving access to the active [NiFe] site (Buhrke et al., 2005). The molecular mechanism underlying the O2-tolerance of the Group 3 SH enzymes and that of the actinobacterial H2ases still remains to be elucidated.
Cyanobacteria, the only prokaryotes capable of oxygenic photosynthesis, form a large and morphologically diverse bacterial group consisting of five morphological subsections. The unicellular organisms that undergo binary fission belong to subsection I (Chroococcales). The unicellular strains that divide through multiple fission processes form subsection II (Pleurocapsales), and subsection III consists of filamentous strains which are unable to perform cell differentiation (Oscillatoriales). The strains in subsections IV and V are filamentous and able to differentiate specific cells called heterocysts, which are dedicated to N2 fixation (Rippka et al., 1979). Cyanobacteria are widely distributed in various environments (from oceans to desert crusts), where they contribute importantly to primary production and N2 fixation processes (Garcia-Pichel et al., 2003). N2-fixation in these organisms is mainly achieved by a molybdenum-iron ([MoFe]) nitrogenase which consists of two subunits, a Fe-protein encoded by nifH, and a Mo-Fe protein encoded by nifDK genes (Smith and Eady, 1992). The maturation process requires three essential (nifBEN) and three no essential genes (nifUSV) (Reviewed in: Rubio and Ludden, 2008). The reduction of N2 is accompanied by the formation of H2 (Berman-Frank et al., 2003). Cyanobacteria contain two different [NiFe] H2ases: the bidirectional [NiFe] H2ase (Hox, Group 3d) and the uptake H2ase (Hup, Group 2a) (Tamagnini et al., 2007). The Hup H2ase is a heterodimeric enzyme encoded by the hupSL genes, which consumes the H2 produced by the nitrogenase (Houchins and Burris, 1981; Lindblad and Sellstedt, 1990). The bidirectional Hox H2ase, which can oxidize H2 and reduce H+, can exist in both diazotrophic and non-diazotrophic strains, and is thought to be a heteropentameric enzyme encoded by hoxEFUYH genes (Schmitz et al., 1995). In the unicellular cyanobacterium Synechocystis PCC 6803, the bidirectional H2ase has been shown to be essential under mixotrophic and nitrate limiting conditions, which suggests that this enzyme functions as electron sink for reduced flavodoxin/ferredoxin (Gutekunst et al., 2014). The ability of the Hox enzymes to be quickly reactivated after being inhibited by O2 has made them the most frequently used H2ase in studies on H2 production in cyanobacteria (Serebryakova et al., 1996; Germer et al., 2009; McIntosh et al., 2011). The main limitations of using the cyanobacterial Hox enzymes in large scale H2 production processes are the low levels of H2 produced and the fast reversal of the enzymatic reaction into oxidation (Tamagnini et al., 2007; Rögner, 2013). During the last decade, genetic engineering approaches were used in several studies in order to overcome these technological barriers with a relative success (Masukawa et al., 2002; McNeely et al., 2010; Baebprasert et al., 2011; Ortega-Ramos et al., 2014; Nyberg et al., 2015). Cyanobacterial strains and/or genomes have also been widely explored in order to unravel the complex picture of H2ases (Ludwig et al., 2006; Barz et al., 2010; Kothari et al., 2012, 2013). These studies have opened new perspectives, since they have shed light on the H2 production potential of strains other than those previously used as laboratory models. Since the publication of these studies, larger numbers of cyanobacterial genomes have been sequenced, which has greatly improved the genomic coverage of all the phylum (Shih et al., 2013). In order to investigate cyanobacterial H2 metabolism more closely, we performed a large-scale analysis of H2ases genes distribution in cyanobacteria, which consisted in searching for the genes encoding H2ases and the proteins required for their maturation in 130 cyanobacterial genomes. The distribution of H2ases in the cyanobacterial phylum inhabiting various environments is discussed.
Results
Distribution of H2ase Encoding Genes and of Genes Involved in Their Maturation Process
Our genomic search for genes encoding H2ase and the proteins involved in their maturation helped to complete the picture of which strains may possibly synthesize functional H2ase.
A phylum-wide analysis of the genomic distribution of H2ase genes among the cyanobacterial genomes in the CyanoGEBA dataset (Shih et al., 2013) showed that only [NiFe] H2ases are present in these organisms. No obvious homologs of [FeFe] or [Fe] H2ases were identified. We assumed that only genomes possessing all the hox and hup genes carry a complete set of H2ase-encoding genes. A complete set of H2ase-encoding genes was deciphered in 52% of the genomes studied (Figure 1A). Among the 130 genomes analyzed, 49 did not show any H2ase-encoding genes (Figure 1A), and 13 genomes did not present the complete set of genes required to encode a functional H2ase (Supplementary Table 1). The lack of H2ase genes may be attributable to the bacterial habitat, since the proportions of H2ases-free genomes differ from one ecological niche to another: the highest proportion of H2ase-free strains was detected in the open ocean (89%), and the remaining 11% carried only hup genes, which suggests that the cyanobacterial contribution to H2 production in the open ocean is negligible (Figure 1B). The distribution of H2ase genes and of genes required for their maturation was found to vary in the cyanobacterial phylum, but all the organisms belonging to subsections IV and V have a complete set of genes encoding H2ases. H2 oxidation and H+ reduction activities seem to be generally conserved in these species (Figure 1C). Since the uptake H2ase is involved in functional nitrogenase processes, the co-occurrence of H2ases, and nitrogenase in various environments was investigated by studying the distribution of [FeMo] nitrogenase structural genes (nifH and nifDK), the nifBEN, and the nifUSV genes involved in the synthesis of the [FeMo]-cofactor synthesis. The nifH, nifDK, and nifBEN genes were found in all the cyanobacteria genomes (Supplementary Table 2). The nifBEN genes were found in co-occurrence with nifUSV genes except is six genomes (Supplementary Table 2). Since the nifSU genes have been reported to be dispensable in Anabaena variabilis (Lyons and Thiel, 1995), one might conclude that their absence does not necessarily mean that the strain is not able to fix nitrogen. It is therefore concluded that all the strains listed in Supplementary Table 2, and whose genomes contain nifH, nifDK, and nifBEN genes are potentially nitrogen-fixing.
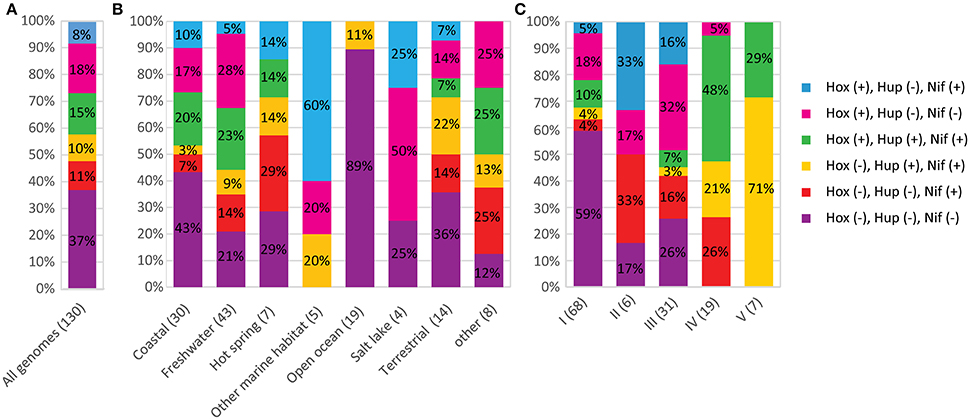
Figure 1. Co-occurrence of H2ases and nitrogenase in cyanobacteria. The number of genomes analyzed in each graph is shown in parentheses. The various combinations of hox, hup, nif genes are presented in different colors. The symbols (+) and (–) indicate that the genes are present or absent respectively. (A) Co-occurrence of H2ase and nitrogenase encoding genes in the 130 genomes analyzed in this study. (B) Distribution of H2ase and nitrogenase-encoding genes depending on the habitat of the strains. (C) Distribution of H2ase and nitrogenase-encoding genes depending on the morphological classification of the strains.
The data obtained here, indicate that nif genes are present in genomes harboring hup (10%) or hox (8%), or both (15%). Eleven percent of the genomes possess nifH and nifDK without harboring the hox and hup genes (Figure 1A). The co-occurrence of nif and hup genes seems to be significantly more frequent in the genomes of strains belonging to subsection V (Figure 1C).
To further assess the distribution of genes encoding H2ases among the cyanobacterial phylum, a phylogenetic tree was constructed using 21 concatenated sequences corresponding to the 130 cyanobacterial proteins listed in Supplementary Table 3 (see Methods Section). The hox and hup genes were found to occur more frequently in the late branches of the tree, although the distribution of hox is patchier (Figures 2, 3). The presence of hyp genes was always associated with that of at least one of the Hox or Hup sequences, and these genes therefore occur less frequently in the early branching clades, which is clearly illustrated in the case of clade g (Figures 2, 3). The distribution of the nitrogenase-encoding genes (nifH and nifDK) is in agreement with the phylogenetic tree previuously presented (Bandyopadhyay et al., 2010). These genes are present in four genomes in the early branches of cyanobacterial evolution: clade a [Synechococcus sp. JA-2-3B′a(2–13), Synechococcus sp. JA-3-3Ab], clade b (Pseudanabaena sp. PCC 6802), clade c (Cyanothece sp. PCC 7425), and six genomes of clade d. No hup genes were ever detected in these early clades, which suggests that the nitrogenase may function naturally in the absence of uptake H2ase. This was previously found to occur in Synechococcus sp. and Cyanothece PCC 7425, which fix N2 under anaerobic conditions (Bandyopadhyay et al., 2011). In the genomes of the strains Nostocales and Stignematales (subsections V and VI), which belong to clade h, the nif and hup genes were always found to co-occur (Figures 2, 3). It is also worth noting that the co-occurrence of hox, hup and nif genes was observed only in the late branches of the tree.
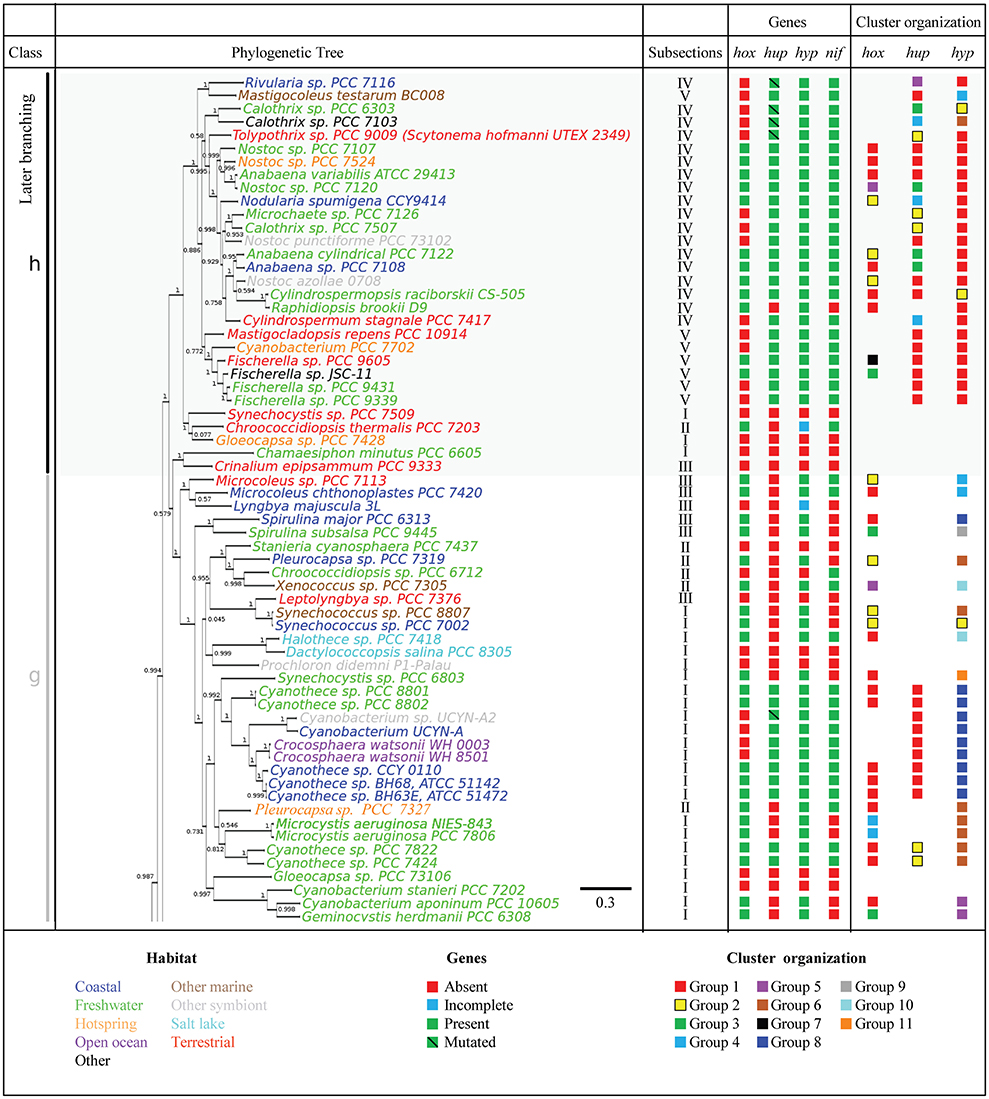
Figure 2. Phylogenetic distribution of H2ases, and nitrogenase in Cyanobacteria. The species tree used in this study is shown in the left panel. The tree was rooted using the sequences of four outgroup organisms (See Section Methods). The genomes are shown in different colors depending on the habitat of the strains. The presence or absence of selected genes is indicated by green and red squares, respectively. The blue square indicates genomes where the set of hyp, hup, or hox genes is incomplete (See Supplementary Table 1 for details). The green barred square indicates genetic polymorphism in catalytic residues. The cluster arrangement of hup, hox, and hyp genes shown in Figure 4 is summarized in the right panel of this picture.
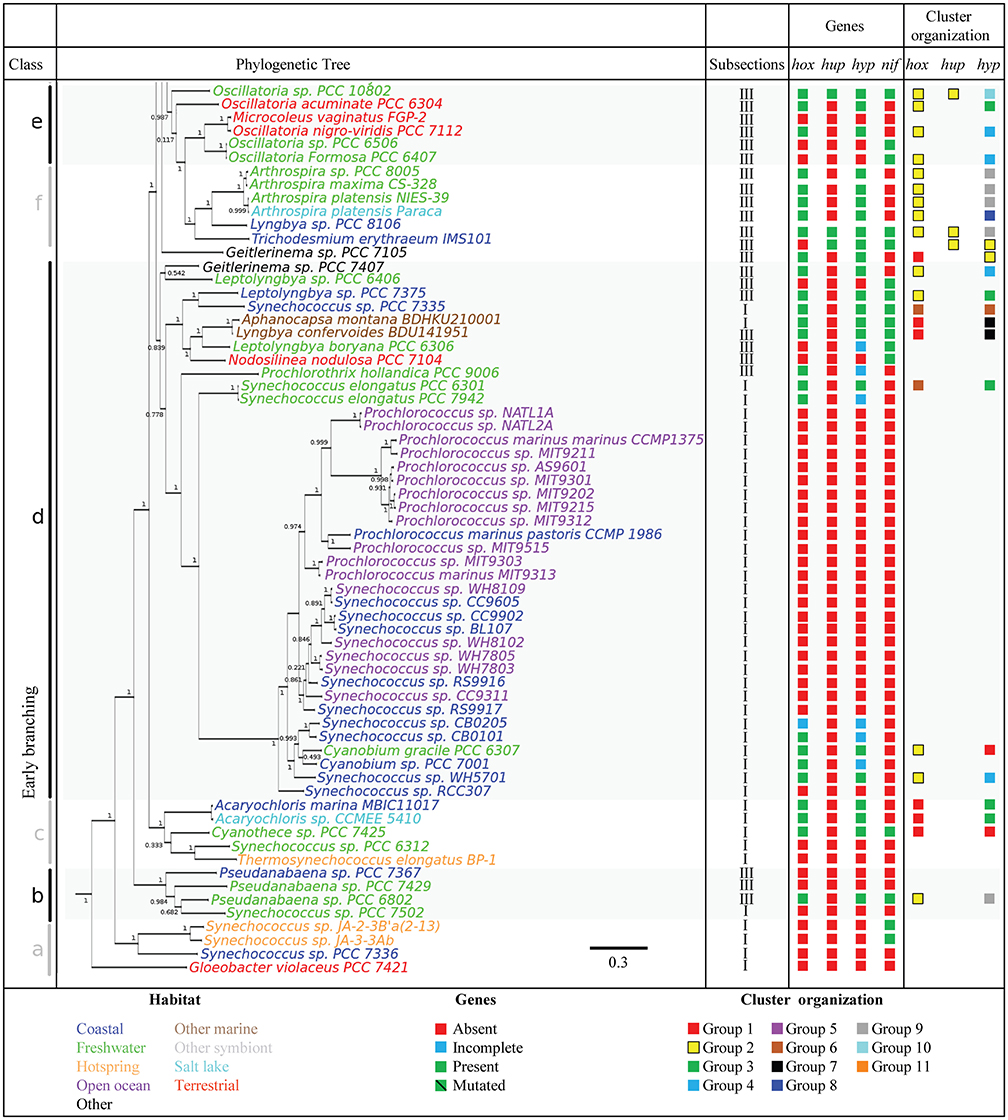
Figure 3. Phylogenetic distribution of H2ases, and nitrogenase in Cyanobacteria. The species tree used in this study is shown in the left panel. The tree was rooted using the sequences of four outgroup organisms (See Section Methods). The genomes are shown in different colors depending on the habitat of the strains. The presence or absence of selected genes is indicated by green and red squares, respectively. The blue square indicates genomes where the set of hyp, hup, or hox genes is incomplete (See Supplementary Table 1 for details). The green barred square indicates genetic polymorphism in catalytic residues. The cluster arrangement of hup, hox, and hyp genes shown in Figure 4 is summarized in the right panel of this picture.
Distribution, Conservation, and Physical Organization of the hox Genes
The genes encoding the bidirectional H2ases (hoxY, hoxH) and the hoxU, hoxE, and hoxF genes encoding the diaphorase part are widely distributed among the cyanobacterial phylum and are particularly abundant in the genomes of organisms belonging to subsections II, III, and IV (Figures 1B,C and Supplementary Table 4). All the hox genes listed in Supplementary Table 4 potentially encode soluble H2ases belonging to subgroup 3d (Vignais et al., 2001). In the large subunit (HoxH), the sequences of the L1 and L2 motifs typical of each [NiFe] group show a high level of conservation. Only a few amino-acid substitutions were observed in the L1 motif in seven genomes of strains from various habitats (terrestrial, coastal, and freshwater strains) (Supplementary Table 5). The Cysteine residues involved in the coordination of metal ions are strictly conserved in all the HoxH and HoxY sequences. The three subunits in the diaphorase of the bidirectional H2ase part (HoxE, HoxF, and HoxU) also contain the conserved cysteine residues potentially required for the coordination of [2Fe2S] and [4Fe4S] clusters. These cysteine residues are largely conserved, since the only few exceptions observed were HoxF and HoxU proteins in Synechococcus sp. CB0205, P. hollandica PCC 9006, and Cyanobium sp. PCC 7001 (Supplementary Table 1). These genomes also lack some of the genes involved in the maturation process (Supplementary Table 1). The bidirectional H2ase in these strains may therefore not be active. The last step in the maturation of the bidirectional H2ases involves the HoxW endopeptidase. The co-occurrence of the hoxW gene and the hox structural genes (HYUEF) was observed in all the genomes analyzed (Supplementary Table 4). Based on the difference between the patterns of expression of the structural hox genes and hoxW, it has been suggested that the endopeptidase HoxW might have multiple functions in cyanobacteria (Wünschiers et al., 2003). The results of the present study confirm this assumption, since hoxW homologs were found to exist in four genomes containing no hoxYHUEF genes (Supplementary Table 4). In addition, the presence of multicopies of the hoxW gene observed in three genomes provides a further argument supporting this hypothesis (Supplementary Table 4).
Seven different patterns of organization were observed among the structural hox genes (Figure 4A, Supplementary Figure 1). In Group 1, the hoxE, hoxF, hoxU, hoxY, and hoxH genes are clustered together and show the same orientation, whereas the hoxW gene occupies another position in the genome. Group 1 includes 26 genomes belonging to all the subsections except subsection V. Group 2 includes 20 genomes belonging to subsections I, II and IV, and all the structural hox genes (EFUYHW) are clustered together in the same orientation (Figure 4A). Group 3 contains two genomes belonging to subsections I and V: the hoxE, hoxF, hoxU, hoxY are clustered together and in the same orientation, whereas the hoxW and hoxH are located in another part of the genomes. The hox genes are more widely scattered in Groups 4-6: hoxE and hoxF are clustered together and the other hox genes are either clustered or scattered in various combinations. All the hox genes hoxF, hoxU, hoxY, hoxH, and hox W are clustered together in Fiscarella sp. PCC 9605 (Group 7), whereas hoxE is located in another part of the genome. The organization of the hox genes is generally not conserved throughout the tree of species, where the seven groups are randomly distributed among the eight clades (Figures 2, 3).
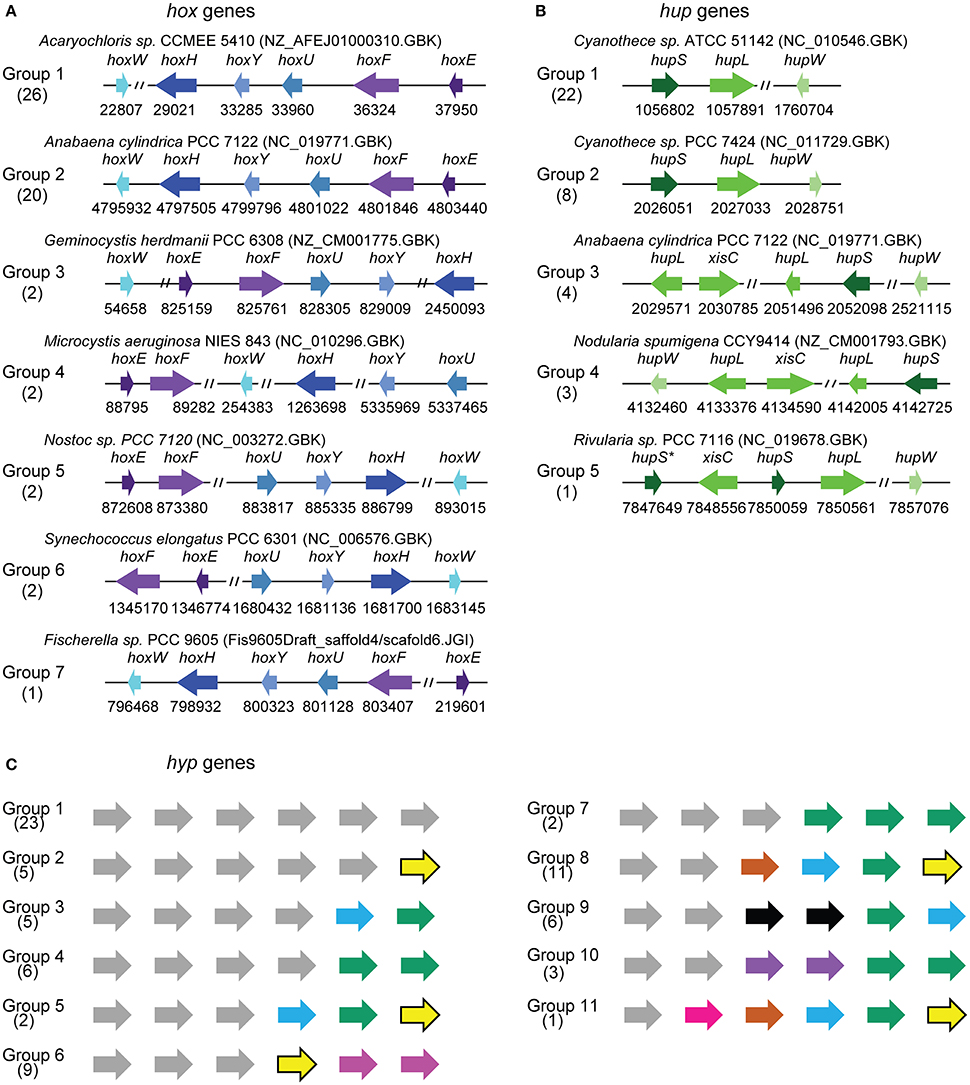
Figure 4. Representative cluster arrangements of hox, hup and hyp genes in cyanobacterial genomes. (A) Physical organization of hoxHYDEFWgenes. (B) Physical organization of hupSLW genes. (C) Physical organization of the hypABCDEF genes. The genes located in the same region are indicated in the same color. The complete physical data on all the genomes studied are presented in Supplementary Figure 1.
Distribution, Conservation, and Physical Organization of the hup Genes
HupL and HupS homologs encoding the large and small subunits of the uptake H2ase, respectively, were identified only in genomes of diazotrophic strains belonging to subsections I, III, IV, and V (Figure 1C and Supplementary Table 6). The strains carrying uptake H2ase genes are widely distributed in various habitats. They are absent only in the genomes of strains collected from salt lakes (Figure 1B). The amino acid sequences of HupS and HupL show a high degree of conservation: the L1 and L2 motifs typical of H2ases belonging to group 2a (Vignais and Billoud, 2007) were found to be conserved in all the Hup sequences analyzed. These motifs include the cysteine residues involved in the coordination of the [NiFe] in the case of HupL and [FeS] in that of HupS (Vignais and Billoud, 2007). In the genomes of Calothrix sp. PCC 7103, Tolypothrix sp. PCC 9009, Rivularia sp. PCC 7116, Cyanobacterium sp. UCYN A2, and Calothrix sp. PCC 6303, since the HupS sequence shows deletions and substitutions of the residues involved in the binding of [FeS] cluster, these enzymes may be inactive or might have different enzymatic characteristics (Supplementary Figure 2). The specific peptidase HupW was identified in all the genomes carrying hupSL genes (Supplementary Table 6). The HupW sequences consistently showed well-conserved residues thought to contribute importantly to the specific interactions between the peptidase and its cognate H2ase subunit (Devine et al., 2009).
In all the genomes analyzed, the hupS and hupL genes form clusters. The organization of the five groups of hup genes depends on the location of the hupW gene and the disruption (or otherwise) of hupS or hupL genes by the xisC gene (Figure 4B). The distribution of these clustering groups varies in the tree of species (Figures 2, 3). Groups 1 or 2 are mostly present throughout the late branches of the tree (clades f, e, g, and h), whereas groups 3, 4, and 5 occur only in clade h (Figure 4B).
Distribution, Conservation, and Physical Organization of the hyp Genes
Almost all the cyanobacterial genomes harboring structural H2ase genes (hox, hup, or both) also harbor the hypABCDEF genes known to encode proteins involved in the maturation of the H2ase (Supplementary Table 7), apart from the genomes of Chroococcidiopsis thermalis PCC 7203, Synechococcus elongatus PCC 7942, Synechococcus sp. CB0101, and Synechococcus sp. PCC 7336, from which some hyp genes are missing. (Supplementary Table 1). Whether the maturation of the H2ase in these strains involves different mechanisms, or whether the maturation process is not efficient in these case is still an open question.
Since little is known about the process of H2ase maturation in cyanobacteria, we analyzed the amino acid composition of the Hyp proteins in the light of the data available in the literature on other organisms. All the information based on the resolution of the crystal structure of the HypF protein of Caldanaerobacter subterraneus (Shomura and Higuchi, 2012), that of the HypECDA of Thermococcus kodakarensis (Watanabe et al., 2009, 2012; Tominaga et al., 2013) and that of the HypB of Archaeoglobus fulgidus, Bradyrhizobium japonicum, and Escherichia coli (Olson and Maier, 2000; Chan et al., 2012; Douglas et al., 2013) are summarized in Supplementary Table 7 and Supplementary Figures 3–8. The fact that the cyanobacterial Hyp sequences showed highly conserved residues reported to contribute importantly to the Hyp features (Supplementary Table 7 and Supplementary Figures 3–8) suggests that the process of maturation of the H2ase enzymes in cyanobacteria might be similar to that described in other organisms (Hansel et al., 2001; Shomura and Higuchi, 2012; Watanabe et al., 2012; Douglas et al., 2013; Tominaga et al., 2013). The hyp genes are either clustered together in various combinations or scattered throughout the genome without any correlations being detected with the diazotrophic ability of the strains or their habitat or their classification (Figure 4C, Supplementary Figure 1). The hyp genes can be classified into 11 main classes depending on their patterns of organization. The genomes in class 1 carry all the hyp genes in a single cluster, while those in class 2 carry five clustered hyp genes and one gene located in another part of the genome, for example. Many rearrangements of the hyp clusters have occurred during the evolution of cyanobacteria, and the number of clusters increases in the late branches of the tree. In clade h, the genes are all clustered together and show a similar pattern of organization (Figures 2, 3, Supplementary Figure 1).
O2-Tolerant H2ases
A search for homologs of O2-tolerant H2ases encoding genes in all the cyanobacterial genomes available in the NCBI database yielded positive findings in five genomes (Table 2, Supplementary Table 8). A blast analysis using the MBH H2ase Hyd1 from E.coli (Group 1, accession number: 3UQY PDB) showed a match with a protein from Lyngbya confervoides BDU141951 (Chandrababunaidu et al., 2015). Multiple sequence alignments indicated that the six cysteine residues (C17, C19, C20, C115, C120, and C149 in E. coli HydI) involved in the coordination of the proximal [4Fe3S] as well as the proline residue (residue 242 in HydI), both of which are typical of this class of O2-tolerant enzymes, are conserved in the protein of L. confervoides BDU141951 (Figure 5).
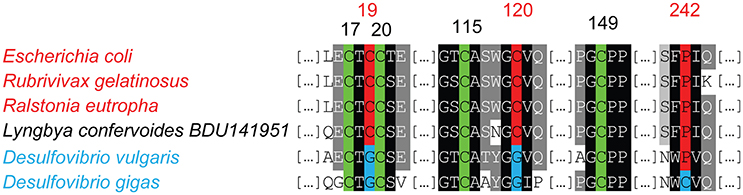
Figure 5. Amino acid Alignment of the proximal cluster sequences in the small subunit of the membrane bound H2ases (MBH). The genomes harboring O2-tolerant MBH encoding genes, and the residues contributing importantly to O2-tolerance are marked in red. The genomes harboring O2-sensitive MBH encoding genes are indicated in blue. The cysteine residues conserved in both enzymes are shown in green.
The Hox enzyme of Aphanocapsa montana BDHKU210001 (Bhattacharyya et al., 2015) showed similarities with the SH H2ase of R. eutropha (Group 3d, accession numer: AAP85843.1). The HoxH, HoxY, and HoxU proteins showed 51, 50, and 45% identity, respectively, with their respective homologs in the R. eutropha enzyme. Homologs of the Pyrococcus furiosus H2ase SH (Group 3b) were identified in Leptolyngbya boryana PCC 6306, Cyanothece sp. PCC 7425 and Mastigocoleus testarum BC008. The sequences encoding the four subunits α (pf0894), β (pf0894), γ (pf0892), and δ (pf0893) showed an average rate of identity of 33% with those of P. furiosus. In the small subunit, the four cysteine residues serving as ligands in the coordination of the [4Fe-4S] cluster in the small subunit are conserved. In conclusion, three of the four O2-tolerant enzymes described so far are present in the cyanobacterial phylum. Three of the strains potentially producing these enzymes are marine (Aphanocapsa montana BDHKU210001, Lyngbya confervoides BDU141951 and Mastigocoleus testarum BC008), and the other two originate from freshwater environments (Cyanothece sp. PCC 7425, and Leptolyngbya boryana PCC 6306).
The maturation process of the MBH-O2 tolerant H2ase of Ralstonia eutropha has been shown to involve some hox specific genes in addition to the hyp genes (Bernhard et al., 1996; Schubert et al., 2007; Ludwig et al., 2009; Fritsch et al., 2011a). The peptidase specific for this enzyme is encoded by the hoxM gene. hoxO and hoxQ genes encode for specific chaperones and hoxZ for a b-type cytochrome (Bernhard et al., 1996; Schubert et al., 2007). The maturation process of the MBH-O2 tolerant H2ase of R. eutropha has been shown to also involve the Hox LRTV proteins (Fritsch et al., 2011b). Homologs of the hoxZMLOQRTV genes were searched in the genome of the cyanobacterium Lyngbya confervoides BDU14195, and as a control in genomes of other organisms known to harbor the MBH-O2 tolerant enzyme (E. coli (Evans et al., 2013), Alteromonas macleodii (Vargas et al., 2011), Hydrogenovibrio marinus DSM 11271 (Shomura et al., 2011), Rubrivivax gelatinosus (Maness et al., 2002), and Salmonella enterica (Bowman et al., 2014). The result of this analysis showed that while the hoxZ, hoxM, hoxL, hoxO, and hoxQ were conserved in all non-cyanobacterial genomes analyzed, only the hoxZ, and hoxM genes were identified in Lyngbya confervoides BDU14195 (Supplementary Table 9). The ability of this cyanobacterium to produce an active MBH-O2 tolerant enzyme is therefore questionable. Since the maturation process of the other O2-tolerant H2ases found in cyanobacteria has not been reported to require any specific proteins other than the Hyp, it is possible that Aphanocapsa montana BDHKU210001, Cyanothece sp. PCC 7425 and Mastigocoleus testarum BC008 might produce active O2-tolerant H2ases. The genome of Leptolyngbya boryana PCC 6306 was found to contain only the hypAB genes, this strains can therefore regarded as inable to build an active O2-tolerant H2ase (Supplementary Table 1).
Discussion
The present analyses of the distribution of genes encoding H2ases in cyanobacterial genomes suggest that H2 metabolism is widely distributed among the various ecological niches that have been colonized by these organisms. H2ase genes and the genes encoding proteins necessary to the maturation process feature prominently in the late branching clades of the cyanobacterial tree of species, which suggests that the need for H2 production and/or uptake has followed the phylogenic evolution of this phylum. The fact that all the structural genes in these enzymes and their maturation process genes have been largely conserved in many cyanobacterial genomes indicates, if these genes are really expressed, that they might play an important physiological role in the bacterial strains inhabiting various environments. Considerable rates of H2 production by cyanobacteria have been reported to occur in microbial mats (Marshall et al., 2012), and Microcoleus spp has been found to be a predominant H2 producer in the microbial mats formed in the Elkhorn Slough estuary, Monterey Bay (Burow et al., 2012). These data further indicate that functional studies on H2ases in environmental strains in addition to laboratory models would greatly improve our understanding of H2 metabolism in this bacterial phylum. No bidirectional H2ase genes were detected in the genomes of open ocean strains (Prochlorococcus and Synechococcus in particular), in agreement with previous results (Barz et al., 2010). The latter study also showed that heterotrophic bacteria inhabiting this environment also lacked bidirectional H2ase encoding genes. The O2 concentration of open ocean waters measured during a period of several months was found to be above 200 μM (Emerson et al., 2002) which may not favor the contribution of the Hox enzyme to the process of H2 metabolism under anaerobic conditions (Khanna and Lindblad, 2015). The distribution of hup, hox and nif genes is highly variable in freshwater, hot spring and terrestrial environments (Figure 1), possibly because of the various conditions that organisms may encounter in these ecological niches.
Nineteen genomes of strains belonging to subsections I, II, III and IV contain nif genes but no hup genes (Figures 1–3 and Supplementary Table 2). In this background, one might expect the H2 production rate of nitrogenase to play an important role in the absence of uptake H2ase. The deletion of the hupL gene in the filamentous diazotrophic strains Nostoc PCC 7120 and Nostoc PCC 7422 has indeed been found to improve the H2 production (Masukawa et al., 2002; Yoshino et al., 2007). In the unicellular cyanobacterium Cyanothece PCC 7822, which fixes nitrogen under aerobiosis, HupL has been shown to be essential to activity of the nitrogenase in the presence of O2. The authors concluded that the main function of the HupSL complex in this bacterium is the protection of the nitrogenase from O2 (Zhang et al., 2014). The present data show that most of the strains possessing nif genes and lacking the uptake H2ase are unicellular [Aphanocapsa montana BDHKU210001, Chroococcidiopsis sp. PCC 6712, Nodosilinea nodulosa PCC 7104, Synechococcus sp. JA-2-3B'a(2–13), Synechococcus sp. JA-3-3Ab]. All these strains are known to undergo N2 fixation under anaerobic conditions (Suplementary Table 2). In future studies, it would be interesting to investigate whether the absence of the uptake H2ase in these strains results in high H2 production.
The finding that genes potentially encoding O2-tolerant H2ases are present in five cyanobacterial genomes is of great interest. Since Lyngbya confervoides BDU141951 genome does not contain all the accessories hox genes important for the maturation process of the MBH-O2 tolerant enzyme, and since the genome of Leptolyngbya boryana PCC 6306 contains only the hypAB genes, it is likely that these two strains are not able to produce an active O2-tolerant enzyme. Whether the other three cyanobacterial strains found here to possess genes encoding for O2-tolerant enzyme actually produce these enzymes needs to be analyzed. The possible input of theses enzymes to the physiology of these organisms in both marine and freshwater environments is an intriguing question. These enzymes are probably involved in the oxidation of H2, like most of their homologs in other organisms. However, in the aerobic soil bacterium Mycobacterium smegmatis, an O2-tolerant H2ase has been found to produce H2, thus enabling this organism to cope with the hypoxia occurring in its ecological niche (Berney et al., 2014). The possibility that O2-tolerant H2ase may play a similar role in cyanobacteria is a tempting hypothesis. Whether the cyanobacterial strains found to possess genes encoding for O2-tolerant H2ases could be for interest in the context of photosynthetic H2 production is a perspective worth exploring in the future.
Methods
Datasets
The genome set analyzed in this study includes 126 cyanobacterial genomes of the CyanoGeba dataset (Shih et al., 2013; Calteau et al., 2014), and genomes of Aphanocapsa montana BDHKU210001, Cyanobacterium sp. UCYN-A2, Lyngbya confervoides BDU141951, Mastigocoleus testarum BC008 which are present in the JGI database (https://img.jgi.doe.gov/cgi-bin/mer/main.cgi). In the case of H2ases not generally found to occur in cyanobacteria (the [FeFe] H2ases, and [NiFe] H2ases other than Hox and Hup), the analysis also included cyanobacterial genomes present in the NCBI database (https://blast.ncbi.nlm.nih.gov/Blast.cgi). The complete list of the genomes analyzed and their accession numbers is given in Supplementary Table 3.
Database Search and Sequences Analysis
The cyanobacterial genomes present in the databases cited above were analyzed using the sequences listed in Supplementary Table 10 as queries. The e-values were adapted to the legnt of the sequences analyzed. A BLASTp (Altschul et al., 1990) analysis was conducted with a specific threshold e-value for each protein, in order to limit the number of paralogs found and therefore to avoid false positives (Supplementary Table 8). Best Reciprocal Blast Hits method and context genomic analysis were used to discriminate false positive and to choose the best the e-value threshold. Sequence alignments were carried out with Clustal-W and displayed with GeneDoc (Thompson et al., 1994; Nicholas et al., 1997). Phylogenetic analysis was performed using the Neighbor-Joining (NJ) method (Saitou and Nei, 1987) implemented in Clustalw to identify eventual false positive.
Phylogenetic Analysis
The species tree was generated by concatenating 21 conserved proteins selected from the phylogenetic markers proposed for use with bacterial genome trees (Wu and Eisen, 2008). The 21 selected proteins are: DnaG, Pgk, PyrG, RplB, RplC, RplD, RplE, RplF, RplL, RplM, RplN, RplP, RplT, RpoB, RpsC, RpsE, RpsI, RpsK, RpsS, SmpB, and Tsf. The sequences of these proteins from Anabaena variabilis ATCC 29413 were used as queries in BlastP analyses. The genomes of Chloroflexus auranticus J-10, Rhodobacter sphaeroides 2.4.1, Heliobacterium modesticaldum Ice1, and Chlorobium tepidum TLS were used as outgroups to root the tree as previously used (Calteau et al., 2014). Multiple sequence alignments of the proteins were performed using MUSCLE 3.8.31 (Edgar, 2004). The alignments were concatenated and the phylogenetic tree was generated with PhyML 3.3.2 (BioNJalgorithm/default parameters) (Guindon et al., 2009).
Author Contributions
AL designed the study and wrote the paper, VP conducted the work, and ST participated in the analysis.
Conflict of Interest Statement
The authors declare that the research was conducted in the absence of any commercial or financial relationships that could be construed as a potential conflict of interest.
Acknowledgments
This research was supported by the A*MIDEX project (n° ANR-11-IDEX-0001-02), and the Agence Nationale de la Recherche (ANR). The authors thank Luisana Avilan for her helpful comments and Jessica Blanc for revising the English manuscript.
Supplementary Material
The Supplementary Material for this article can be found online at: http://journal.frontiersin.org/article/10.3389/fgene.2016.00223/full#supplementary-material
References
Altschul, S. F., Gish, W., Miller, W., Myers, E. W., and Lipman, D. J. (1990). Basic local alignment search tool. J. Mol. Biol. 215, 403–410. doi: 10.1016/S0022-2836(05)80360-2
Baebprasert, W., Jantaro, S., Khetkorn, W., Lindblad, P., and Incharoensakdi, A. (2011). Increased H2 production in the cyanobacterium Synechocystis sp. strain PCC 6803 by redirecting the electron supply via genetic engineering of the nitrate assimilation pathway. Metab. Eng. 13, 610–616. doi: 10.1016/j.ymben.2011.07.004
Bagramyan, K., Mnatsakanyan, N., Poladian, A., Vassilian, A., and Trchounian, A. (2002). The roles of hydrogenases 3 and 4, and the F0F1-ATPase, in H2 production by Escherichia coli at alkaline and acidic pH. FEBS Lett. 516, 172–178. doi: 10.1016/S0014-5793(02)02555-3
Bandyopadhyay, A., Elvitigala, T., Welsh, E., Stöckel, J., Liberton, M., Min, H., et al. (2011). Novel metabolic attributes of the genus Cyanothece, comprising a group of unicellular nitrogen-fixing cyanobacteria. MBio 2:e00214-11. doi: 10.1128/mBio.00214-11
Bandyopadhyay, A., Stöckel, J., Min, H., Sherman, L. A., and Pakrasi, H. B. (2010). High rates of photobiological H2 production by a cyanobacterium under aerobic conditions. Nat. Commun. 1:139. doi: 10.1038/ncomms1139
Barz, M., Beimgraben, C., Staller, T., Germer, F., Opitz, F., Marquardt, C., et al. (2010). Distribution analysis of hydrogenases in surface waters of marine and freshwater environments. PLoS ONE 5:e13846. doi: 10.1371/journal.pone.0013846
Berman-Frank, I., Lundgren, P., and Falkowski, P. (2003). Nitrogen fixation and photosynthetic oxygen evolution in cyanobacteria. Res. Microbiol. 154, 157–164. doi: 10.1016/S0923-2508(03)00029-9
Berney, M., Greening, C., Conrad, R., Jacobs, W. R. Jr., and Cook, G. M. (2014). An obligately aerobic soil bacterium activates fermentative hydrogen production to survive reductive stress during hypoxia. Proc. Natl. Acad. Sci. U.S.A. 111, 11479–11484. doi: 10.1073/pnas.1407034111
Bernhard, M., Schwartz, E., Rietdorf, J., and Friedrich, B. (1996). The Alcaligenes eutrophus membrane-bound hydrogenase gene locus encodes functions involved in maturation and electron transport coupling. J. Bacteriol. 178, 4522–4529. doi: 10.1128/jb.178.15.4522-4529.1996
Bhattacharyya, S., Chandrababunaidu, M. M., Sen, D., Panda, A., Ghorai, A., Bhan, S., et al. (2015). Draft genome sequence of exopolysaccharide-producing cyanobacterium Aphanocapsa montana BDHKU 210001. Genome Announc. 3, e00057–15. doi: 10.1128/genomeA.00057-15
Bothe, H., Schmitz, O., Yates, M. G., and Newton, W. E. (2010). Nitrogen fixation and hydrogen metabolism in cyanobacteria. Microbiol. Mol. Biol. Rev. 74, 529–551. doi: 10.1128/MMBR.00033-10
Bowman, L., Flanagan, L., Fyfe, P. K., Parkin, A., Hunter, W. N., and Sargent, F. (2014). How the structure of the large subunit controls function in an oxygen-tolerant [NiFe]-hydrogenase. Biochem. J. 458, 449–458. doi: 10.1042/BJ20131520
Bryant, F. O., and Adams, M. W. (1989). Characterization of hydrogenase from the hyperthermophilic archaebacterium, Pyrococcus furiosus. J. Biol. Chem. 264, 5070–5079.
Buhrke, T., Lenz, O., Krauss, N., and Friedrich, B. (2005). Oxygen tolerance of the H2-sensing [NiFe] hydrogenase from Ralstonia eutropha H16 is based on limited access of oxygen to the active site. J. Biol. Chem. 280, 23791–23796. doi: 10.1074/jbc.M503260200
Buhrke, T., Lenz, O., Porthun, A., and Friedrich, B. (2004). The H2-sensing complex of Ralstonia eutropha: interaction between a regulatory [NiFe] hydrogenase and a histidine protein kinase. Mol. Microbiol. 51, 1677–1689. doi: 10.1111/j.1365-2958.2003.03933.x
Burow, L. C., Woebken, D., Bebout, B. M., McMurdie, P. J., Singer, S. W., Pett-Ridge, J., et al. (2012). Hydrogen production in photosynthetic microbial mats in the Elkhorn Slough estuary, Monterey Bay. ISME J. 6, 863–874. doi: 10.1038/ismej.2011.142
Calteau, A., Fewer, D. P., Latifi, A., Coursin, T., Laurent, T., Jokela, J., et al. (2014). Phylum-wide comparative genomics unravel the diversity of secondary metabolism in Cyanobacteria. BMC Genomics 15:977. doi: 10.1186/1471-2164-15-977
Chan, K. H., Lee, K. M., and Wong, K. B. (2012). Interaction between hydrogenase maturation factors HypA and HypB is required for [NiFe]-hydrogenase maturation. PLoS ONE 7:e32592. doi: 10.1371/journal.pone.0032592
Chandrababunaidu, M. M., Diya, S., and Tripathy, S. (2015). Draft genome sequence of filamentous marine cyanobacterium Lyngbya confervoides strain BDU141951. Genome Announc. 3, e00066–15. doi: 10.1128/genomeA.00066-15
Chen, Z., Lemon, B. J., Huang, S., Swartz, D. J., Peters, J. W., and Bagley, K. A. (2002). Infrared studies of the CO-inhibited form of the Fe-only hydrogenase from Clostridium pasteurianum I: examination of its light sensitivity at cryogenic temperatures. Biochemistry 41, 2036–2043. doi: 10.1021/bi011510o
Constant, P., Chowdhury, S. P., Pratscher, J., and Conrad, R. (2010). Streptomycetes contributing to atmospheric molecular hydrogen soil uptake are widespread and encode a putative high-affinity [NiFe]-hydrogenase. Environ. Microbiol. 12, 821–829. doi: 10.1111/j.1462-2920.2009.02130.x
Dementin, S., Burlat, B., Fourmond, V., Leroux, F., Liebgott, P. P., Abou Hamdan, A., et al. (2011). Rates of intra- and intermolecular electron transfers in hydrogenase deduced from steady-state activity measurements. J. Am. Chem. Soc. 133, 10211–10221. doi: 10.1021/ja202615a
Dernedde, J., Eitinger, T., Patenge, N., and Friedrich, B. (1996). hyp gene products in Alcaligenes eutrophus are part of a hydrogenase-maturation system. Eur. J. Biochem. 235, 351–358. doi: 10.1111/j.1432-1033.1996.00351.x
D'Eustachio, A. J., and Hardy, R. W. F. (1964). Reductants and electron transport in nitrogen fixation. Biochem. Biophys. Res. Commun. 15, 319–323. doi: 10.1016/0006-291X(64)90167-6
Devine, E., Holmqvist, M., Stensjö, K., and Lindblad, P. (2009). Diversity and transcription of proteases involved in the maturation of hydrogenases in Nostoc punctiforme ATCC 29133 and Nostoc sp. strain PCC 7120. BMC Microbiol. 9:53. doi: 10.1186/1471-2180-9-53
Douglas, C. D., Ngu, T. T., Kaluarachchi, H., and Zamble, D. B. (2013). Metal transfer within the Escherichia coli HypB-HypA complex of hydrogenase accessory proteins. Biochemistry 52, 6030–6039. doi: 10.1021/bi400812r
Duché, O., Elsen, S., Cournac, L., and Colbeau, A. (2005). Enlarging the gas access channel to the active site renders the regulatory hydrogenase HupUV of Rhodobacter capsulatus O2 sensitive without affecting its transductory activity. FEBS J. 272, 3899–3908. doi: 10.1111/j.1742-4658.2005.04806.x
Edgar, R. C. (2004). MUSCLE: a multiple sequence alignment method with reduced time and space complexity. BMC Bioinformatics 5:113. doi: 10.1186/1471-2105-5-113
Emerson, S., Stump, C., Johnson, B., and Karl, D. M. (2002). In situ determination of oxygen and nitrogen dynamics in the upper ocean. Deep Sea Res. Part I Oceanogr. Res. Pap. 49, 941–952. doi: 10.1016/S0967-0637(02)00004-3
Evans, R. M., Parkin, A., Roessler, M. M., Murphy, B. J., Adamson, H., Lukey, M. J., et al. (2013). Principles of sustained enzymatic hydrogen oxidation in the presence of oxygen -the crucial influence of high potential Fe-S clusters in the electron relay of [NiFe]-hydrogenases. J. Am. Chem. Soc. 135, 2694–2707. doi: 10.1021/ja311055d
Florin, L., Tsokoglou, A., and Happe, T. (2001). A novel type of iron hydrogenase in the green alga Scenedesmus obliquus is linked to the photosynthetic electron transport chain. J. Biol. Chem. 276, 6125–6132. doi: 10.1074/jbc.M008470200
Fritsch, J., Lenz, O., and Friedrich, B. (2011a). The maturation factors HoxR and HoxT contribute to oxygen tolerance of membrane-bound [NiFe] hydrogenase in Ralstonia eutropha H16. J. Bacteriol. 193, 2487–2497. doi: 10.1128/JB.01427-10
Fritsch, J., Scheerer, P., Frielingsdorf, S., Kroschinsky, S., Friedrich, B., Lenz, O., et al. (2011b). The crystal structure of an oxygen-tolerant hydrogenase uncovers a novel iron-sulphur centre. Nature 479, 249–252. doi: 10.1038/nature10505
Garcia-Pichel, F., Belnap, J., Neuer, S., and Schanz, F. (2003). Estimates of global cyanobacterial biomass and its distribution. Arch. Hydrobiol. Suppl. Algol. Stud. 109, 213–227. doi: 10.1127/1864-1318/2003/0109-0213
Germer, F., Zebger, I., Saggu, M., Lendzian, F., Schulz, R., and Appel, J. (2009). Overexpression, isolation, and spectroscopic characterization of the bidirectional [NiFe] hydrogenase from Synechocystis sp. PCC 6803. J. Biol. Chem. 284, 36462–36472. doi: 10.1074/jbc.M109.028795
Goris, T., Wait, A. F., Saggu, M., Fritsch, J., Heidary, N., Stein, M., et al. (2011). A unique iron-sulfur cluster is crucial for oxygen tolerance of a [NiFe]-hydrogenase. Nat. Chem. Biol. 7, 310–318. doi: 10.1038/nchembio.555
Guindon, S., Delsuc, F., Dufayard, J. F., and Gascuel, O. (2009). Estimating maximum likelihood phylogenies with PhyML. Methods Mol. Biol. 537, 113–137. doi: 10.1007/978-1-59745-251-9_6
Gutekunst, K., Chen, X., Schreiber, K., Kaspar, U., Makam, S., and Appel, J. (2014). The bidirectional NiFe-hydrogenase in Synechocystis sp. PCC 6803 is reduced by flavodoxin and ferredoxin and is essential under mixotrophic, nitrate-limiting conditions. J. Biol. Chem. 289, 1930–1937. doi: 10.1074/jbc.M113.526376
Hansel, A., Axelsson, R., Lindberg, P., Troshina, O. Y., Wünschiers, R., and Lindblad, P. (2001). Cloning and characterisation of a hyp gene cluster in the filamentous cyanobacterium Nostoc sp. strain PCC 73102. FEMS Microbiol. Lett. 201, 59–64. doi: 10.1111/j.1574-6968.2001.tb10733.x
Hendrickson, E. L., and Leigh, J. A. (2008). Roles of coenzyme F420-reducing hydrogenases and hydrogen- and F420-dependent methylenetetrahydromethanopterin dehydrogenases in reduction of F420 and production of hydrogen during methanogenesis. J. Bacteriol. 190, 4818–4821. doi: 10.1128/JB.00255-08
Higuchi, Y., Ogata, H., Miki, K., Yasuoka, N., and Yagi, T. (1999). Removal of the bridging ligand atom at the Ni-Fe active site of [NiFe] hydrogenase upon reduction with H2, as revealed by X-ray structure analysis at 1.4 Å resolution. Structure 7, 549–556. doi: 10.1016/S0969-2126(99)80071-9
Hoffmann, D., Gutekunst, K., Klissenbauer, M., Schulz-Friedrich, R., and Appel, J. (2006). Mutagenesis of hydrogenase accessory genes of Synechocystis sp. PCC 6803: additional homologues of hypA and hypB are not active in hydrogenase maturation. FEBS J. 273, 4516–4527. doi: 10.1111/j.1742-4658.2006.05460.x
Horch, M., Rippers, Y., Mroginski, M. A., Hildebrandt, P., and Zebger, I. (2013). Combining spectroscopy and theory to evaluate structural models of metalloenzymes: a case study on the soluble [NiFe] hydrogenase from Ralstonia eutropha. Chemphyschem 14, 185–191. doi: 10.1002/cphc.201200853
Houchins, J. P., and Burris, R. H. (1981). Comparative characterization of two distinct hydrogenases from Anabaena sp. strain 7120. J. Bacteriol. 146, 215–221.
Jenney, F. E. Jr., and Adams, M. W. W. (2008). Hydrogenases of the model hyperthermophiles. Ann. N.Y. Acad. Sci. 252–266. doi: 10.1196/annals.1419.013
Karstens, K., Wahlefeld, S., Horch, M., Grunzel, M., Lauterbach, L., Lendzian, F., et al. (2015). Impact of the iron-sulfur cluster proximal to the active site on the catalytic function of an O2-tolerant NAD+-reducing [NiFe]-hydrogenase. Biochemistry 54, 389–403. doi: 10.1021/bi501347u
Kaster, A. K., Moll, J., Parey, K., and Thauer, R. K. (2011). Coupling of ferredoxin and heterodisulfide reduction via electron bifurcation in hydrogenotrophic methanogenic archaea. Proc. Natl. Acad. Sci. U.S.A. 108, 2981–2986. doi: 10.1073/pnas.1016761108
Khanna, N., and Lindblad, P. (2015). Cyanobacterial hydrogenases and hydrogen metabolism revisited: recent progress and future prospects. Int. J. Mol. Sci. 16, 10537–10561. doi: 10.3390/ijms160510537
Kothari, A., Potrafka, R., and Garcia-Pichel, F. (2012). Diversity in hydrogen evolution from bidirectional hydrogenases in cyanobacteria from terrestrial, freshwater and marine intertidal environments. J. Biotechnol. 162, 105–114. doi: 10.1016/j.jbiotec.2012.04.017
Kothari, A., Vaughn, M., and Garcia-Pichel, F. (2013). Comparative genomic analyses of the cyanobacterium, Lyngbya aestuarii BL J, a powerful hydrogen producer. Front. Microbiol. 4:363. doi: 10.3389/fmicb.2013.00363
Kwan, P., McIntosh, C. L., Jennings, D. P., Hopkins, R. C., Chandrayan, S. K., Wu, C. H., et al. (2015). The [NiFe]-hydrogenase of Pyrococcus furiosus exhibits a new type of oxygen tolerance. J. Am. Chem. Soc. 137, 13556–13565. doi: 10.1021/jacs.5b07680
Lauterbach, L., and Lenz, O. (2013). Catalytic production of hydrogen peroxide and water by oxygen-tolerant [NiFe]-hydrogenase during H2 cycling in the presence of O2. J. Am. Chem. Soc. 135, 17897–17905. doi: 10.1021/ja408420d
Lindblad, P., and Sellstedt, A. (1990). Occurrence and localization of an uptake hydrogenase in the filamentous heterocystous cyanobacterium Nostoc PCC 73102. Protoplasma 159, 9–15. doi: 10.1007/BF01326630
Lubitz, W., Ogata, H., Rüdiger, O., and Reijerse, E. (2014). Hydrogenases. Chem. Rev. 114, 4081−4148. doi: 10.1021/cr4005814
Ludwig, M., Schubert, T., Zebger, I., Wisitruangsakul, N., Saggu, M., Strack, A., et al. (2009). Concerted action of two novel auxiliary proteins in assembly of the active site in a membrane-bound [NiFe] hydrogenase. J. Biol. Chem. 284, 2159–2168. doi: 10.1074/jbc.M808488200
Ludwig, M., Schulz-Friedrich, R., and Appel, J. (2006). Occurrence of hydrogenases in cyanobacteria and anoxygenic photosynthetic bacteria: implications for the phylogenetic origin of cyanobacterial and algal hydrogenases. J. Mol. Evol. 63, 758–768. doi: 10.1007/s00239-006-0001-6
Lukey, M. J., Roessler, M. M., Parkin, A., Evans, R. M., Davies, R. A., Lenz, O., et al. (2011). Oxygen-tolerant [NiFe]-hydrogenases: the individual and collective importance of supernumerary cysteines at the proximal Fe-S cluster. J. Am. Chem. Soc. 133, 16881–16892. doi: 10.1021/ja205393w
Lyons, E. M., and Thiel, T. (1995). Characterization of nifB, nifS, and nifU genes in the cyanobacterium Anabaena variabilis: NifB is required for the vanadium-dependent nitrogenase. J. Bacteriol. 177, 1570–1575. doi: 10.1128/jb.177.6.1570-1575.1995
Maness, P. C., Smolinski, S., Dillon, A. C., Heben, M. J., and Weaver, P. F. (2002). Characterization of the oxygen tolerance of a hydrogenase linked to a carbon monoxide oxidation pathway in Rubrivivax gelatinosus. Appl. Environ. Microbiol. 68, 2633–2636. doi: 10.1128/AEM.68.6.2633-2636.2002
Marques, M. C., Coelho, R., De Lacey, A. L., Pereira, I. A. C., and Matias, P. M. (2010). The three-dimensional structure of [nifese] hydrogenase from Desulfovibrio vulgaris hildenborough: a hydrogenase without a bridging ligand in the active site in its oxidised, “as-isolated” state. J. Mol. Biol. 396, 893–907. doi: 10.1016/j.jmb.2009.12.013
Marshall, C. W., Ross, D. E., Fichot, E. B., Norman, R. S., and May, H. D. (2012). Electrosynthesis of commodity chemicals by an autotrophic microbial community. Appl. Environ. Microbiol. 78, 8412–8420. doi: 10.1128/AEM.02401-12
Masukawa, H., Mochimaru, M., and Sakurai, H. (2002). Disruption of the uptake hydrogenase gene, but not of the bidirectional hydrogenase gene, leads to enhanced photobiological hydrogen production by the nitrogen-fixing cyanobacterium Anabaena sp. PCC 7120. Appl. Microbiol. Biotechnol. 58, 618–624. doi: 10.1007/s00253-002-0934-7
McDowall, J. S., Murphy, B. J., Haumann, M., Palmer, T., Armstrong, F. A., and Sargent, F. (2014). Bacterial formate hydrogenlyase complex. Proc. Natl. Acad. Sci. U.S.A. 111, E3948–E3956. doi: 10.1073/pnas.1407927111
McIntosh, C. L., Germer, F., Schulz, R., Appel, J., and Jones, A. K. (2011). The [NiFe]-hydrogenase of the cyanobacterium Synechocystis sp. PCC 6803 works bidirectionally with a bias to H2 production. J. Am. Chem. Soc. 133, 11308–11319. doi: 10.1021/ja203376y
McNeely, K., Xu, Y., Bennette, N., Bryant, D. A., and Dismukes, G. C. (2010). Redirecting reductant flux into hydrogen production via metabolic engineering of fermentative carbon metabolism in a cyanobacterium. Appl. Environ. Microbiol. 76, 5032–5038. doi: 10.1128/AEM.00862-10
Melis, A., Zhang, L., Forestier, M., Ghirardi, M. L., and Seibert, M. (2000). Sustained photobiological hydrogen gas production upon reversible inactivation of oxygen evolution in the green alga Chlamydomonas reinhardtii. Plant Physiol. 122, 127–136. doi: 10.1104/pp.122.1.127
Nicholas, K. B., Nicholas, J. H. B., and Deerfield, D. W. (1997). GeneDoc: analysis and visualization of genetic variation. Embnew. News 4.
Nyberg, M., Heidorn, T., and Lindblad, P. (2015). Hydrogen production by the engineered cyanobacterial strain Nostoc PCC 7120 ΔhupW examined in a flat panel photobioreactor system. J. Biotechnol. 215, 35–43. doi: 10.1016/j.jbiotec.2015.08.028
Olson, J. W., and Maier, R. J. (2000). Dual roles of Bradyrhizobium japonicum nickelin protein in nickel storage and GTP-dependent Ni mobilization. J. Bacteriol. 182, 1702–1705. doi: 10.1128/JB.182.6.1702-1705.2000
Ortega-Ramos, M., Jittawuttipoka, T., Saenkham, P., Czarnecka-Kwasiborski, A., Bottin, H., Cassier-Chauvat, C., et al. (2014). Engineering synechocystis PCC6803 for hydrogen production: influence on the tolerance to oxidative and sugar stresses. PLoS ONE 9:e89372. doi: 10.1371/journal.pone.0089372
Oxelfelt, F., Tamagnini, P., and Lindblad, P. (1998). Hydrogen uptake in Nostoc sp. strain PCC 73102. Cloning and characterization of a hupSL homologue. Arch. Microbiol. 169, 267–274. doi: 10.1007/s002030050571
Pandelia, M. E., Nitschke, W., Infossi, P., Giudici-Orticoni, M. T., Bill, E., and Lubitz, W. (2011). Characterization of a unique [FeS] cluster in the electron transfer chain of the oxygen tolerant [NiFe] hydrogenase from Aquifex aeolicus. Proc. Natl. Acad. Sci. U.S.A. 108, 6097–6102. doi: 10.1073/pnas.1100610108
Peters, J. W., Schut, G. J., Boyd, E. S., Mulder, D. W., Shepard, E. M., Broderick, J. B., et al. (2015). [FeFe]- and [NiFe]-hydrogenase diversity, mechanism, and maturation. Biochim. Biophys. Acta Mol. Cell Res. 1853, 1350–1369. doi: 10.1016/j.bbamcr.2014.11.021
Rippka, R., Deruelles, J., Waterbury, J. B., Herdman, M., and Stanier, R. Y. (1979). Generic assignments, strain histories and properties of pure cultures of cyanobacteria. J. Gen. Microbiol. 111, 1–61. doi: 10.1099/00221287-111-1-1
Rögner, M. (2013). Metabolic engineering of cyanobacteria for the production of hydrogen from water. Biochem. Soc. Trans. 41, 1254–1259. doi: 10.1042/BST20130122
Roncaroli, F., Bill, E., Friedrich, B., Lenz, O., Lubitz, W., and Pandelia, M. E. (2015). Cofactor composition and function of a H2 -sensing regulatory hydrogenase as revealed by Mössbauer and EPR spectroscopy. Chem. Sci. 6, 4495–4507. doi: 10.1039/C5SC01560J
Rubio, L. M., and Ludden, P. W. (2008). Biosynthesis of the iron-molybdenum cofactor of nitrogenase. Annu. Rev. Microbiol. 62, 93–111. doi: 10.1146/annurev.micro.62.081307.162737
Saitou, N., and Nei, M. (1987). The neighbor-joining method: a new method for reconstructing phylogenetic trees. Mol. Biol. Evol. 4, 406–425.
Schäfer, C., Friedrich, B., and Lenz, O. (2013). Novel, oxygen-insensitive group 5 [NiFe]-hydrogenase in Ralstonia eutropha. Appl. Environ. Microbiol. 79, 5137–5145. doi: 10.1128/AEM.01576-13
Schmitz, O., Boison, G., Hilscher, R., Hundeshagen, B., Zimmer, W., Lottspeich, F., et al. (1995). Molecular biological analysis of a bidirectional hydrogenase from cyanobacteria. Eur. J. Biochem. 233, 266–276. doi: 10.1111/j.1432-1033.1995.266_1.x
Schubert, T., Lenz, O., Krause, E., Volkmer, R., and Friedrich, B. (2007). Chaperones specific for the membrane-bound [NiFe]-hydrogenase interact with the Tat signal peptide of the small subunit precursor in Ralstonia eutropha H16. Mol. Microbiol. 66, 453–467. doi: 10.1111/j.1365-2958.2007.05933.x
Serebryakova, L. T., Medina, M., Zorin, N. A., Gogotov, I. N., and Cammack, R. (1996). Reversible hydrogenase of Anabaena variabilis ATCC 29413: catalytic properties and characterization of redox centres. FEBS Lett. 383, 79–82. doi: 10.1016/0014-5793(96)00228-1
Shih, P. M., Wu, D., Latifi, A., Axen, S. D., Fewer, D. P., Talla, E., et al. (2013). Improving the coverage of the cyanobacterial phylum using diversity-driven genome sequencing. Proc. Natl. Acad. Sci. U.S.A. 110, 1053–1058. doi: 10.1073/pnas.1217107110
Shomura, Y., and Higuchi, Y. (2012). Structural basis for the reaction mechanism of S-carbamoylation of HypE by HypF in the maturation of [NiFe]-hydrogenases. J. Biol. Chem. 287, 28409–28419. doi: 10.1074/jbc.M112.387134
Shomura, Y., Yoon, K. S., Nishihara, H., and Higuchi, Y. (2011). Structural basis for a [4Fe-3S] cluster in the oxygen-tolerant membrane-bound [NiFe]-hydrogenase. Nature 479, 253–256. doi: 10.1038/nature10504
Smith, B. E., and Eady, R. R. (1992). Metalloclusters of the nitrogenases. Eur. J. Biochem. 205, 1–15. doi: 10.1111/j.1432-1033.1992.tb16746.x
Tamagnini, P., Leitão, E., Oliveira, P., Ferreira, D., Pinto, F., Harris, D. J., et al. (2007). Cyanobacterial hydrogenases: diversity, regulation and applications. FEMS Microbiol. Rev. 31, 692–720. doi: 10.1111/j.1574-6976.2007.00085.x
Thiemermann, S., Dernedde, J., Bernhard, M., Schroeder, W., Massanz, C., and Friedrich, B. (1996). Carboxyl-terminal processing of the cytoplasmic NAD-reducing hydrogenase of Alcaligenes eutrophus requires the hoxW gene product. J. Bacteriol. 178, 2368–2374. doi: 10.1128/jb.178.8.2368-2374.1996
Thompson, J. D., Higgins, D. G., and Gibson, T. J. (1994). CLUSTAL W: improving the sensitivity of progressive multiple sequence alignment through sequence weighting, position-specific gap penalties and weight matrix choice. Nucleic Acids Res. 22, 4673–4680. doi: 10.1093/nar/22.22.4673
Tominaga, T., Watanabe, S., Matsumi, R., Atomi, H., Imanaka, T., and Miki, K. (2013). Crystal structures of the carbamoylated and cyanated forms of HypE for [NiFe] hydrogenase maturation. Proc. Natl. Acad. Sci. U.S.A. 110, 20485–20490. doi: 10.1073/pnas.1313620110
Vargas, W. A., Weyman, P. D., Tong, Y., Smith, H. O., and Xu, Q. (2011). [NiFe] Hydrogenase from Alteromonas macleodii with unusual stability in the presence of oxygen and high temperature. Appl. Environ. Microbiol. 77, 1990–1998. doi: 10.1128/AEM.01559-10
Vignais, P. M., and Billoud, B. (2007). Occurrence, classification, and biological function of hydrogenases: an overview. Chem. Rev. 107, 4206–4272. doi: 10.1021/cr050196r
Vignais, P. M., Billoud, B., and Meyer, J. (2001). Classification and phylogeny of hydrogenases. FEMS Microbiol. Rev. 25, 455–501. doi: 10.1111/j.1574-6976.2001.tb00587.x
Vitt, S., Ma, K., Warkentin, E., Moll, J., Pierik, A. J., Shima, S., et al. (2014). The F420-reducing [NiFe]-Hydrogenase complex from Methanothermobacter marburgensis, the first X-ray structure of a group 3 family member. J. Mol. Biol. 426, 2813–2826. doi: 10.1016/j.jmb.2014.05.024
Volbeda, A., Charon, M. H., Piras, C., Hatchikian, E. C., Frey, M., and Fontecilla-Camps, J. C. (1995). Crystal structure of the nickel–iron hydrogenase from Desulfovibrio gigas. Nature 373, 580–587. doi: 10.1038/373580a0
Volbeda, A., Garcin, E., Piras, C., De Lacey, A. L., Fernandez, V. M., Hatchikian, E. C., et al. (1996). Structure of the [NiFe] hydrogenase active site: evidence for biologically uncommon Fe ligands. J. Am. Chem. Soc. 118, 12989–12996. doi: 10.1021/ja962270g
Watanabe, S., Arai, T., Matsumi, R., Atomi, H., Imanaka, T., and Miki, K. (2009). Crystal structure of HypA, a nickel-binding metallochaperone for [NiFe] hydrogenase maturation. J. Mol. Biol. 394, 448–459. doi: 10.1016/j.jmb.2009.09.030
Watanabe, S., Matsumi, R., Atomi, H., Imanaka, T., and Miki, K. (2012). Crystal structures of the HypCD complex and the HypCDE ternary complex: transient intermediate complexes during [NiFe] hydrogenase maturation. Structure 20, 2124–2137. doi: 10.1016/j.str.2012.09.018
Winkler, M., Heil, B., Heil, B., and Happe, T. (2002). Isolation and molecular characterization of the [Fe]-hydrogenase from the unicellular green alga Chlorella fusca. Biochim. Biophys. Acta 1576, 330–334. doi: 10.1016/S0167-4781(02)00239-7
Wu, M., and Eisen, J. (2008). A simple, fast, and accurate method of phylogenomic inference. Genome Biol. 9:R151. doi: 10.1186/gb-2008-9-10-r151
Wünschiers, R., Batur, M., and Lindblad, P. (2003). Presence and expression of hydrogenase specific C-terminal endopeptidases in cyanobacteria. BMC Microbiol. 3:8. doi: 10.1186/1471-2180-3-8
Yoshino, F., Ikeda, H., Masukawa, H., and Sakurai, H. (2007). High photobiological hydrogen production activity of a Nostoc sp. PCC 7422 uptake hydrogenase-deficient mutant with high nitrogenase activity. Mar. Biotechnol. 9, 101–112. doi: 10.1007/s10126-006-6035-3
Keywords: cyanobacteria, genomes, hydrogenase, oxygen tolerance
Citation: Puggioni V, Tempel S and Latifi A (2016) Distribution of Hydrogenases in Cyanobacteria: A Phylum-Wide Genomic Survey. Front. Genet. 7:223. doi: 10.3389/fgene.2016.00223
Received: 25 September 2016; Accepted: 13 December 2016;
Published: 27 December 2016.
Edited by:
Martin G. Klotz, City University of New York (CUNY), USAReviewed by:
Josselin Noirel, Conservatoire National des Arts et Métiers (CNAM), FrancePaulo Oliveira, University of Porto, Portugal
Copyright © 2016 Puggioni, Tempel and Latifi. This is an open-access article distributed under the terms of the Creative Commons Attribution License (CC BY). The use, distribution or reproduction in other forums is permitted, provided the original author(s) or licensor are credited and that the original publication in this journal is cited, in accordance with accepted academic practice. No use, distribution or reproduction is permitted which does not comply with these terms.
*Correspondence: Amel Latifi, bGF0aWZpQGltbS5jbnJzLmZy