- 1Department of Biomedical Sciences, University of Minnesota Medical School, Duluth, MN, United States
- 2Department of Neuroscience, University of Minnesota Medical School, Minneapolis, MN, United States
Almost all cellular processes are regulated by the approximately 24 h rhythms that are endogenously driven by the circadian clock. mRNA translation, as the most energy consuming step in gene expression, is temporally controlled by circadian rhythms. Recent research has uncovered key mechanisms of translational control that are orchestrated by circadian rhythmicity and in turn feed back to the clock machinery to maintain robustness and accuracy of circadian timekeeping. Here I review recent progress in our understanding of translation control mechanisms in the circadian clock, focusing on a role for the mammalian/mechanistic target of rapamycin (mTOR) signaling pathway in modulating entrainment, synchronization and autonomous oscillation of circadian clocks. I also discuss the relevance of circadian mTOR functions in disease.
Introduction
As explained by the central dogma of molecular biology, genetic information flows from DNA to RNA to make a functional product, a protein. Protein synthesis accounts for ∼75% of a cell’s total energy consumption and is highly regulated in cells (Lane and Martin, 2010). Translational control (regulation of protein synthesis) plays a significant role in the regulation of gene expression under physiological conditions. Deregulation of translational control is frequently involved in the pathophysiology of human diseases, including cancer, metabolic syndromes, and neurological disorders (Sonenberg and Hinnebusch, 2007; Hershey et al., 2012).
Circadian (∼24 h) rhythmicity is an autonomous biological property that controls a variety of biochemical, physiological, and behavioral processes in all living organisms (Hall and Rosbash, 1993; Young, 1998; Takahashi et al., 2008). The rhythmic processes are driven by autonomous oscillations of “clock genes” in cells. Whereas a significant role for protein synthesis in the circadian clock was found half a century ago (Feldman, 1967, 1968), novel mechanisms of mRNA translation control are being discovered in recent years. Some of these findings have been nicely summarized in three reviews (Lim and Allada, 2013b; Green, 2018; Torres et al., 2018). Here I discuss the latest progress in our understanding of translational control mechanisms in the circadian clock, focusing on a critical role for the mammalian/mechanistic target of rapamycin (mTOR) signaling pathway.
Circadian Rhythms and Circadian Clocks
Circadian rhythms are endogenously driven by proteins called “circadian clocks” that oscillate in either their physical levels or functional states on a daily basis. The fundamental property enables organisms to temporally coordinate their physiology and behavior, according to changes in daily light/darkness cycles, food availability, temperature, moisture, and air pressure in the environment (Rosbash, 2009). Thus, organisms can predict and prepare for upcoming environmental changes to meet their physiological needs (Reppert and Weaver, 2002). Rhythmic physiological and metabolic processes are normally coupled and synchronized to the environmental cycles so that optimal physiological and metabolic efficiencies can be attained at the right time of a day.
The circadian system is hierarchically organized. In Drosophila the central clock cells are located in the large and small lateral ventral neurons (l-LNvs and s-LNvs) of the optic lobe (Dubowy and Sehgal, 2017), which synthesize pigment dispersing factor (PDF) as a circadian neuromodulator among clock neurons. In mammals, the suprachiasmatic nucleus (SCN) of the anterior hypothalamus is the master pacemaker (Moore, 2013). SCN receives photic input from the retina, generates robust circadian rhythms and sends out neural and endocrine signals as rhythmic outputs to various brain regions as well as peripheral organs and systems. In the body, functions of the autonomic nervous system, endocrine and immune systems are all regulated by the SCN (Mohawk et al., 2012; Gamble et al., 2014). Clock genes are ubiquitously expressed in almost all cells and tissues. Almost all types of cells can perform circadian oscillations, with different robustness, accuracy, and period (Liu et al., 2007). Thus, rhythms in various organs and systems need to be orchestrated by the master pacemaker and synchronized to the environmental light/dark cycles (Aton and Herzog, 2005; Golombek and Rosenstein, 2010).
In cells circadian oscillations are driven by autonomous genetic feedback loops. Work over past three decades has identified evolutionarily conserved transcriptional/translational feedback loops (TTFLs) and about a dozen genes that account for cellular circadian oscillations (Hall and Rosbash, 1993; Young, 1998; Takahashi, 2017). In mammals, the heterodimers of transcription factors CLOCK and BMAL1 activate gene transcription of Per and Cryptochrome (Cry). PER and CRY proteins form multiprotein complexes. Once the complexes accumulate to certain levels in the cytosol, they translocate back to the cell nucleus, associate with CLOCK/BMAL1 heterodimers, and repress Per and Cry gene transcription (Takahashi et al., 2008).
Per gene expression functions as a “knob” of the clock and is tightly regulated by intracellular and extracellular signals via complex mechanisms. Firstly, rhythmic Per transcription is activated by the CLOCK: BMAL1 complexes through the E-box enhancers in the promoter region. Secondly, at the post-transcriptional level Per mRNA processing is regulated by methylation (Fustin et al., 2013). Thirdly, as the degradation rate of PER proteins is also a key determinant of the length of a circadian cycle, PER cycling is controlled by sophisticated post-translational modifications such as phosphorylation (Kloss et al., 1998; Lowrey et al., 2000; Lee et al., 2001; Meng et al., 2008; Chiu et al., 2011) and ubiquitination (Busino et al., 2007; Siepka et al., 2007; Hirano et al., 2013; Yoo et al., 2013).
Recent work has started to uncover a key role for translational control in regulating clock gene expression. In Drosophila, the RNA binding proteins Ataxin-2 (Atx2) interacts with Twenty-four (Tyf) to activate Per mRNA translation in pacemaker neurons to sustain robustness of circadian behavioral rhythms (Lim et al., 2011; Lim and Allada, 2013a; Zhang et al., 2013). A targeted RNAi screen revealed knockdown of the atypical translation factor NAT1 lengthens circadian period and reduces PER protein levels in PDF neurons (Bradley et al., 2012). In mice, we show that as the downstream targets of the mitogen-activated protein kinase (MAPK)/extracellular Signal-regulated Kinase (ERK) pathway, MAPK interacting protein kinases (MNKs) phosphorylate the cap-binding protein eIF4E in the SCN. Activities of the MAPK/MNK/eIF4E pathway can be activated upon light exposure at night. Phosphorylation of eIF4E stimulates Per1 and Per2 mRNA translation and functions as a facilitator of photic entrainment of the SCN circadian clock (Cao et al., 2015). Besides these mechanisms, another emerging translational control pathway with more complexity is the mTOR signaling.
mTor Signaling
mTOR is an evolutionarily conserved serine/threonine protein kinase, also known as FK506-binding protein 12-rapamycin-associated protein 1 (FRAP1). mTOR forms two multiprotein complexes in cells, the mTOR complex (mTORC) 1 and mTORC2. mTORC1 and mTORC2 share some protein components, including mTOR, mLST8 (mammalian lethal with sec13 protein 8, also known as GβL), and DEPTOR (the inhibitory DEP domain containing mTOR-interacting protein). mTORC1 also includes Raptor (the regulator-associated protein of the mammalian target of rapamycin) and PRAS40 (proline-rich Akt substrate of 40 kDa). Raptor interacts with the TOS (target of rapamycin signaling) motifs mTOR in a rapamycin-sensitive manner and is essential for mTORC1 activity. mTORC2 consists of Rictor (the rapamycin insensitive companion of mTOR), mSIN1(mammalian stress activated MAP kinase-interacting protein 1), and PROTOR 1 and 2 (proteins observed with rictor 1 and 2). Rictor and mSIN1 are both critical for mTORC2 function (Lipton and Sahin, 2014; González and Hall, 2017; Saxton and Sabatini, 2017).
mTOR signaling refers to an intracellular signaling network centered on mTORC1 and mTORC2. mTOR signaling senses intracellular signals and also responds to extracellular stimuli. It can be activated by upstream signals including growth factors (e.g., insulin and insulin-like growth factor-1), energy status (e.g., oxygen and ATP levels), nutrients (e.g., leucine and arginine), as well as neurotransmitters (e.g., glutamate and neuropeptides). Growth factors and mitogens inhibit the Tuberous Sclerosis Complex (TSC) complex. TSC is a key negative regulator of mTORC1. It is a GTPase activating protein for the small GTPase Rheb, which directly binds and activates mTORC1. Once activated, mTOR signaling controls fundamental biological processes including protein synthesis and turnover, lipid and glucose metabolism, autophagy, cytoskeleton organization, etc. (González and Hall, 2017; Saxton and Sabatini, 2017). mTORC1 has the most defined role in translational control. mTORC1 exhibits protein kinase activity and regulates mRNA translation by regulation of its translation effectors, which include the eukaryotic initiation factor 4E-binding proteins (4E-BPs) and ribosomal protein S6 kinases (S6K1 and S6K2) (Hay and Sonenberg, 2004).
mTor and Translational Control
In general, translational control can be achieved via two mechanisms: (1) impacting on the mRNAs by sequence specific RNA binding proteins or small non-coding RNAs such as microRNAs; (2) impacting on the translational apparatus, which include translation factors, ribosomes and tRNAs. The latter predominantly affects the step of translation initiation (Hershey et al., 2012).
All nuclear transcribed mRNAs are capped at the 5′-ends with the 7-methyl-guanosine. Eukaryotic translation initiation factor 4E (eIF4E) is a cap-binding protein. It recognizes and binds to the mRNA 5′ m7GpppN (where N is any nucleotide) (Hinnebusch et al., 2016). eIF4G is a scaffolding protein that associates with 4E and 4A. eIF4A is a RNA helicase that resolves mRNA secondary structures. eIF4F (including 4E, 4G, and 4A) complex interacts with eIF3 to recruit the small ribosomal subunit and initiates cap-dependent translation initiation. The eIF4E-binding proteins (4E-BPs) control eIF4E binding to the cap structure. 4E-BP binding of eIF4E causes repression of cap-dependent translation initiation and can be relieved by phosphorylation of 4E-BPs through mTORC1 (Gingras et al., 1999). Activated by various extracellular and intracellular cues, mTORC1 phosphorylates 4E-BPs to lead to its dissociation from eIF4E (Brunn et al., 1997; Gingras et al., 1999), which allows cap-dependent mRNA translation to initiate. Thus, mTORC1 regulates cap-dependent translation via 4E-BPs.
As another major branch of mTORC1, S6K1 is activated by phosphorylation on its hydrophobic motif site, Thr389. S6K1 in turn phosphorylates a number of proteins that control mRNA translation. It phosphorylates eukaryotic translation initiation factor 4B (eIF4B) at S422, which is a cofactor of eIF4A and increases its processivity (Holz et al., 2005). S6K1 also phosphorylates and promotes the degradation of PDCD4 (Programmed Cell Death 4). PDCD4 inhibits eIF4B and enhances the translation efficiency of spliced mRNAs via its interaction with SKAR (S6K1 Aly/REF-like target, Dorrello et al., 2006), a component of exon-junction complexes involved in mRNA splicing (Ma et al., 2008). S6K1 inactivates eukaryotic elongation factor-2 kinase (eEF2K) (Wang et al., 2001; Knebel et al., 2002), which is a negative regulator of eukaryotic elongation factor 2 (eEF2), by phosphorylating it at S366, and thus regulates translation elongation.
mTor and the Circadian Clock
Intracellular signal transduction pathways control circadian timing and entrainment by regulating clock gene expression at different levels (Gillette and Mitchell, 2002; Evans, 2016). In general, a signaling pathway that is important for the circadian clock is usually regulated by circadian rhythmicity and therefore exhibits rhythmic activities under constant conditions. Moreover, the signaling pathway is often regulated by the extracellular signals and couples these signals to circadian gene expression. Thus, the feedback loops within the clock is coupled to a feedforward loop involving the environmental cues, the signaling pathway and the clock. The mTOR pathway is a typical signaling example that couples environment cues to the clock cells and its network. In this section, I will discuss the interactions between the mTOR pathway and the circadian clock.
mTOR Regulation of the Circadian Clock
Work over the past decade has started to uncover a multifaceted role of mTOR in the circadian clock. Firstly, mTOR signaling is part of the photic entrainment pathway in the SCN; secondly, mTOR regulates autonomous clock properties in a variety of circadian oscillators; thirdly, mTOR regulates network properties of coupled circadian oscillators, such as the SCN neurons.
Regulation of Photic Entrainment of the SCN Circadian Clock by mTORC1
To adapt to the changing environment, the circadian clock is constantly adjusted by environment signals. Light is the most important cue to regulate the SCN clock. Photic input is received by the retina and relayed to the SCN via the retinohypothalamic tract (RHT). The pathway is distinct from the image-forming visual pathway in that the reception of light is mediated by intrinsically photosensitive retina ganglion cells (ipRGCs), which express the photopigment melanopsin (Peirson and Foster, 2006; Panda, 2007). RHT terminals form synaptic connections with the ventral SCN neurons. The excitatory neurotransmitter glutamate and the neuropeptide pituitary adenylate cyclase-activating peptide (PACAP) are released at the RHT terminals (Hannibal, 2002) upon photic stimulation at night. They in turn bind to their receptors on the SCN neurons and evoke activation of intracellular signaling events that regulate clock gene expression and trigger clock resetting (Golombek and Rosenstein, 2010). At aforementioned, cellular rhythmic clock gene expression is driven by transcription/translational feedback loops. A major negative feedback loop is composed of CLOCK/BMAL1-driven rhythmic Per and Cry expression. Per and Cry levels are high during the day and low at night. Light at night triggers transient upregulation of Per and Cry expression, which will shift the phase of cyclic gene expression and reset the SCN clock. Light at the early night delays the clock whereas light at the late night advances the clock.
In searching for intracellular signaling pathways that mediate photic entrainment of the SCN clock, it is found that light at night activates S6K1 by inducing its phosphorylation at Thr389. In turn, activated S6K1 phosphorylates its downstream translation effectors including the ribosomal protein S6 (S6), a component of the 40S ribosomal subunit. S6K1 activation and S6 phosphorylation often correlate with translation efficiency of a subset of mRNAs which have a 5′-terminal oligopyrimidine (TOP) tract (Meyuhas and Dreazen, 2009), whereas evidence exists that neither S6K1 nor S6 phosphorylation is required for translational response of these mRNAs (Stolovich et al., 2002). In the SCN, it is found that protein products of TOP mRNAs such as eEF1A (eukaryotic elongation factor 1A) and Jun B are light-inducible in a rapamycin-sensitive manner (Cao et al., 2010). Light also increases phosphorylation of 4E-BP1 at Thr37/46 in the SCN (Cao et al., 2008). Phosphorylation of 4E-BP1 triggers its dissociation from eIF4E and activates cap-dependent translation. These activities are mTORC1-dependent, as rapamycin blocks light-induced S6K1 and 4E-BP1 phosphorylation. Light-induced mTORC1 activation appears to be important for photic entrainment of the SCN clock, as rapamycin modulates light-induced phase shifts of wheel-running and body temperature rhythms in mice (Cao et al., 2010). Effects of rapamycin on behavioral phase shift are consistent with its inhibition of light-induced PER1 and PER2 proteins in the SCN. Together, these results demonstrate that the mTORC1 signaling is an integral part of the photic entrainment pathway that regulates light-inducible mRNA translation in the SCN, although precise translational control mechanisms via S6K1 remain to be delineated.
During photic entrainment, eIF4E is a pivotal point where the MAPK and mTORC1 pathways cross to control mRNA translation in the SCN. As aforementioned, MAPK pathway activates MNKs, which in turn phosphorylates eIF4E in the photo-recipient SCN cells and facilitates light-induced Per1 and Per2 mRNA translation. mTORC1 phosphorylates and inhibits the eIF4E repressor protein 4E-BP1. 4E-BP1 represses the eIF4E-dependent translation of Vip. Thus, mTORC1 activation disinhibits Vip mRNA translation and increases the abundance of Vip (also see section “Regulation of Synchronization of SCN Neurons by mTOR”). The light-regulated mTORC1 and MAPK pathways are summarized in Figure 1.
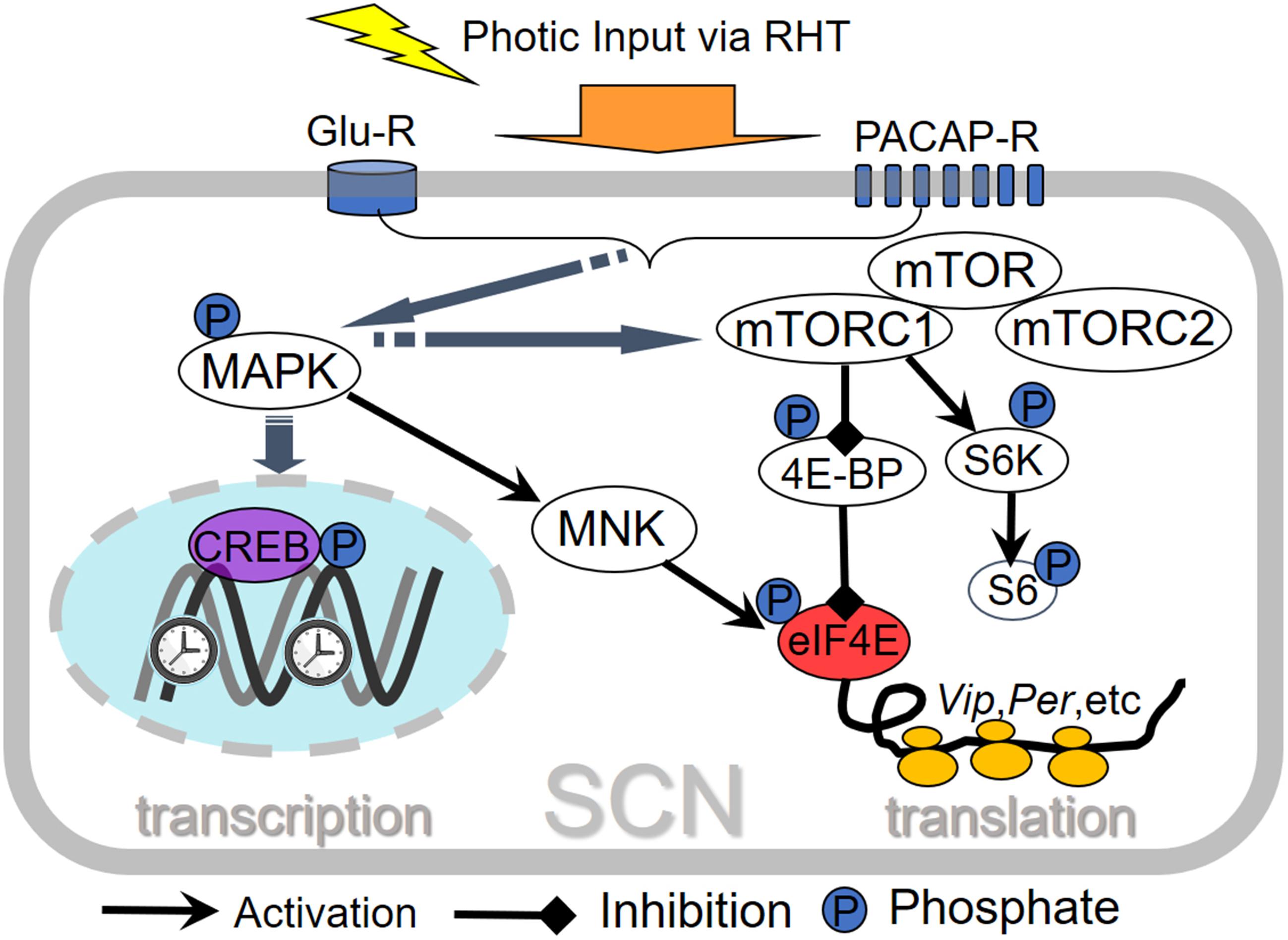
FIGURE 1. Schematic overview of light-regulated translational control pathways in the SCN. Light at night activates mTORC1, which in turn regulates its translational effectors ribosomal protein S6 kinases (S6Ks, including S6K1, and S6K2) and eukaryotic translation initiation factor 4E-binding proteins (4E-BPs). 4E-BP phosphorylation leads to its dissociation from eIF4E and activation of cap-dependent translational initiation. Vip (vasoactive intestinal peptide) mRNA translation is regulated by 4E-BPs. In another pathway, photic ERK/MAPK activation leads to phosphorylation of eIF4E via MNK kinases and promotes mRNA translation of Per1 and Per2 in the SCN. Thus, the MAPK and mTOR pathways converge on eIF4E to regulate mRNA translation in the SCN.
Regulation of Synchronization of SCN Neurons by mTOR
As the master pacemaker in mammals, unique anatomical and physiological features enable the SCN to generate accurate and robust rhythms. One of such features is the unique coupling mechanism among SCN neurons. SCN neurons are heterogenous in their expression of neuropeptides, pacemaking ability, response to light, and periods of their firing rhythms (Welsh et al., 1995, 2010; Herzog et al., 1998; Shirakawa et al., 2000; Aton and Herzog, 2005). In general, the ventral SCN neurons express VIP (vasoactive intestinal peptide), and the dorsal SCN neurons express AVP (arginine vasopressin). Some cells in between express GRP (gastrin releasing peptide). The ventral SCN cells receive photic input from the RHT and are directly entrained by light. In turn, these neurons send out output to the dorsal SCN neurons and reset their rhythms. To produce a coherent daily output, the SCN cells must entrain to each other. SCN intercellular coupling is essential for synchrony among cellular oscillators and robustness against genetic or environmental perturbations (Liu et al., 2007). Studies over the past decade have found that VIP signaling is particularly important for coupling SCN neurons.
Vasoactive intestinal peptide is a peptide of 28 amino acid and a ligand of G protein-coupled receptors (Gozes and Brenneman, 1993). Vip expression is enriched in the SCN cells and is also found in a subset of GABAergic neurons in the neocortex, olfactory bulb, some midbrain and brainstem regions as well as the gut and the pancreas (Liu et al., 2018). Through its receptor VPAC2R (encoded by the Vipr2 gene), VIP signaling is essential for synchrony between ventral and dorsal SCN cells. Loss of VIP or VPAC2R leads to unstable, low amplitude circadian cycling in individual SCN cells and weak rhythms or arrhythmicity in SCN slices and animals (Harmar et al., 2002; Colwell et al., 2003; Cutler et al., 2003; Aton et al., 2005; Maywood et al., 2006). The direct protein product of the Vip gene is prepro-VIP, a 170-amino acid peptide. How Vip mRNA translation was regulated was not known.
As aforementioned, 4E-BPs are translational repressors and their activities are inhibited after phosphorylation by mTORC1. In the post-mitotic adult brain, cell growth and division are limited, and phosphorylation of 4E-BPs is low in a variety of brain regions, presumably because of the relatively moderate demand for protein synthesis. However, it is found that 4E-BPs are highly phosphorylated in the SCN (Cao and Obrietan, 2010), indicating a unique role for 4E-BPs in the SCN circadian clock. Indeed, it is found that 4E-BP1 specifically inhibits mRNA translation of Vip. By phosphorylating 4E-BP1, mTORC1 promotes Vip mRNA translation and increases the abundance of VIP in the SCN (Cao et al., 2013). In 4E-BP1 null mice, levels of prepro-VIP (precursor protein of VIP) and VIP are increased in the SCN. Consequently, these animals re-entrain to a shifted light/dark cycle more quickly and show resistance to the rhythm-disruptive effects of constant light. At the tissue level, the 4E-BP1 null SCN slices exhibit a shorter period and higher amplitude of PER2::LUCIFERASE (PER2::LUC) rhythms, consistent with enhanced coupling among SCN cells (Cao et al., 2013).
Conversely, in Mtor heterozygotes prepro-VIP and VIP level is decreased, and the PER2::LUC rhythms in SCN are damped with a lengthened period (Cao et al., 2013; Ramanathan et al., 2018). These mice show longer period under constant conditions and are more susceptible to the effects of constant light (Cao et al., 2013). To test whether mTOR regulates SCN cell synchrony, the mTORC1 inhibitor PP242 was applied and PER2::LUC bioluminescence imaging was performed on SCN slices. SCN synchrony is indeed disrupted by PP242 (Liu et al., 2018), consistent with its rhythm-damping effects. As mTOR inhibition decreases Vip expression, the effects of PP242 may be ascribed to decreased VIP level in the SCN. To test whether mTOR regulates SCN synchrony through VIP neurons, conditional mTOR knockout mice were created, where mTOR gene was specifically knocked out in VIP cells (Liu et al., 2018). Indeed, these mice exhibit significant circadian defects, including weakened circadian behavioral rhythmicity under constant light, disrupted circadian behavior under a skeleton photoperiod, and decreased synchrony among SCN cells. These phenotypes largely resemble those seen in the Vip or VPAC2 null mice (Harmar et al., 2002; Colwell et al., 2003; Cutler et al., 2003; Aton et al., 2005; Maywood et al., 2006) as well as in rats treated with VIP antagonists (Gozes et al., 1995), suggesting that mTOR regulates circadian synchrony via VIP signaling. However, additional mechanisms cannot be excluded. For example, as mTOR regulates the amplitude of cellular circadian oscillators (Ramanathan et al., 2018, see section “Regulation of Autonomous Properties of the Circadian Clocks by mTOR” ), decreased intercellular coupling could be due to attenuated cellular oscillations. Further studies are needed to identify additional mechanisms whereby the mTOR signaling controls SCN synchrony. Figure 2 recapitulates the current model to explain how intracellular mTOR signaling can regulate intercellular coupling in the SCN via translational control of Vip.
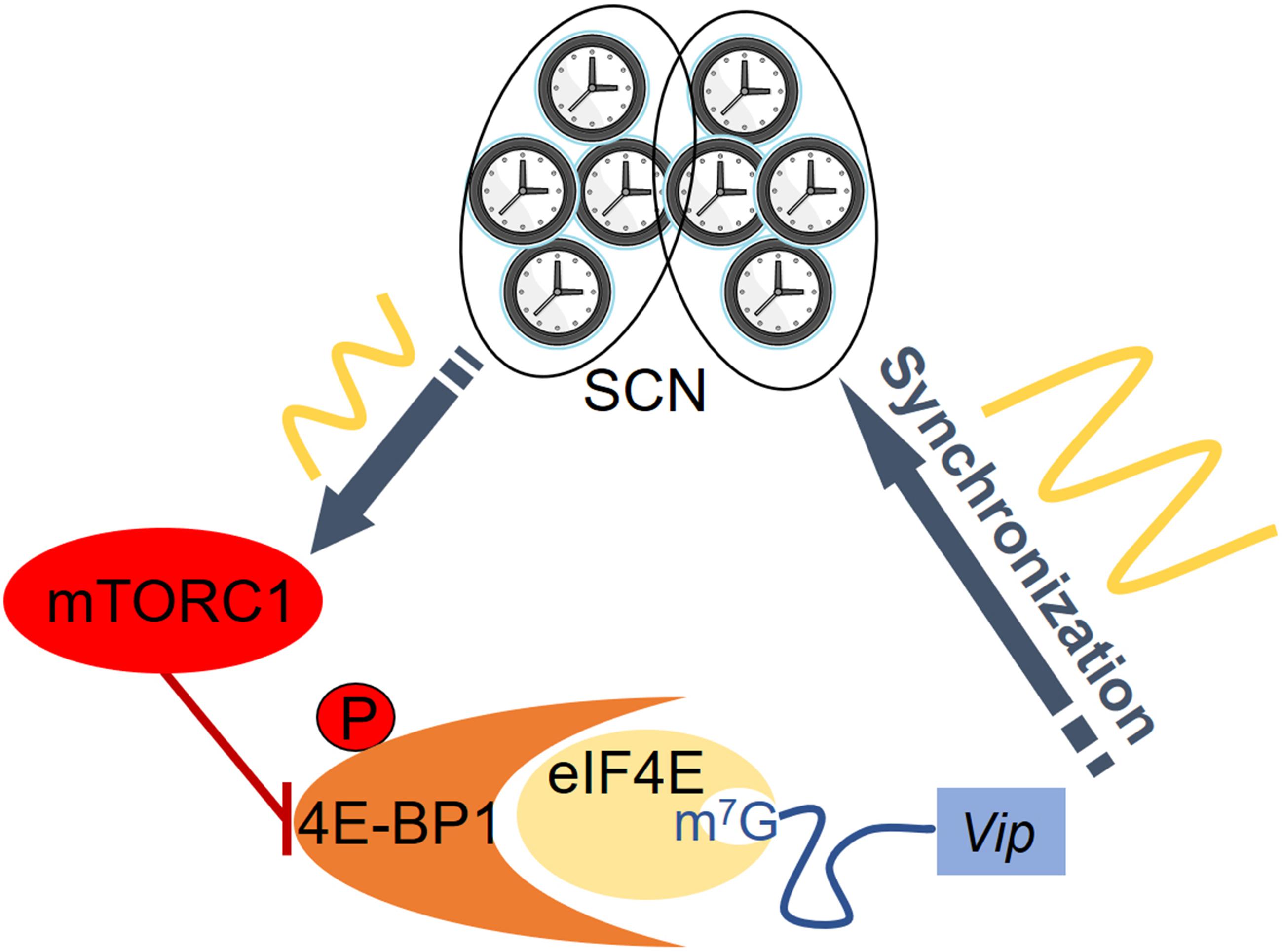
FIGURE 2. The mTORC1/4E-BP1 pathway regulates SCN cell synchrony via translational control of Vip. Rhythmic mTORC1 signaling phosphorylates 4E-BP1 to promote cap-dependent mRNA translation of Vip and increases the level of VIP in SCN. VIP signaling promotes synchrony of SCN cells and increases the robustness of clock gene oscillation. SCN clock synchrony can be enhanced by removing the translational repressor 4E-BP1.
Regulation of Autonomous Properties of the Circadian Clocks by mTOR
mTOR is a major integrator of intracellular signals that senses energy status (e.g., oxygen and ATP levels), and nutrients (e.g., leucine and arginine levels) to regulate cell growth and metabolism. Presumably it can serve as a linker between cellular metabolic states and the circadian timing process. A genome-wide RNAi screen in human cells identified hundreds of genes that regulate cellular clock functions (Zhang et al., 2009). The insulin signaling pathway was identified as the most overrepresented pathway. Downregulation of its multiple components such as PI3K and mTOR alters circadian period. Recently, the effects of mTOR manipulation on autonomous circadian clock properties were studied in various cellular and tissue oscillators (Ramanathan et al., 2018).
mTOR regulates fundamental clock properties (e.g., period and amplitude) in a variety of clock models. mTOR inhibition increases period and reduces amplitude, whereas activation of mTOR shortens period and augments amplitude in fibroblasts, hepatocytes, and adipocytes (Ramanathan et al., 2018). These results are consistent with studies showing dose-dependent lengthening of circadian period and damping of amplitude in human U2OS cells in response to rapamycin and torin1 treatments (Feeney et al., 2016; Lipton et al., 2017). Constitutive activation of mTOR in Tsc2−/− fibroblasts alters the dynamics of clock gene oscillations and elevates levels of core clock proteins, including CRY1, BMAL1, and CLOCK (Lipton et al., 2017; Ramanathan et al., 2018). However, serum stimulation upregulates CRY1 in an mTOR-dependent but Bmal1- and Period-independent manner (Ramanathan et al., 2018). Moreover, mTOR also regulates properties of the ex vivo SCN and liver clocks in a similar way (Ramanathan et al., 2018). In mice, heterozygous mTor knockout mice show lengthened circadian period of locomotor activity rhythms under constant conditions. Consistently, the 4E-BP1 knockout mice, where mTOR activity is increased, show shortened circadian period (Cao et al., 2013). However, TOR modulates circadian period in the opposite direction in Drosophila. Overexpressing S6K in the ventral lateral neurons, the central pacemaker cells, lengthens the circadian period (Zheng and Sehgal, 2010). Consistently, another study reports that knockout of Tor in Per expressing cells decreases circadian period of locomotor rhythms in flies (Kijak and Pyza, 2017). The reasons for this discrepancy between mice and flies are not clear, possibly due to different clock mechanisms in these species.
The circadian functions of mTOR in disease models are more intriguing. One study suggests that circadian rhythms of mTOR activities in cancer cells should be considered in chemotherapy In the study by Okazaki et al. (2014), circadian mTOR activities are found in mouse renal carcinoma. The rhythmic mTOR activities affect the efficacy of everolimus, a rapalog mTOR inhibitor that is clinically applied to treat cancers of the kidney, pancreas, breast, and brain. The drug is more effective in improving survival of tumor-bearing mice if applied at the time of a day when mTOR activities are elevated.
Studies also suggest that aberrant mTOR activities underlie circadian dysfunction under pathological conditions. In one study, mTOR is found to mediate the effects of circadian disruption caused by hypoxia, which is seen in many disease conditions such as cancer. When hypoxic cells are permitted to acidify to recapitulate the tumor microenvironment, the circadian clock is impacted through the transcriptional activities of hypoxia-inducible factors (HIFs) at clock genes. Acidification of cells suppresses mTORC1 signaling and restoring mTORC1 signaling rescues clock oscillation (Walton et al., 2018). In another study, Lipton et al. (2017) investigated circadian rhythms in a mouse model of TSC. In the TSC mice mTOR activities are constitutively elevated. They find that Tsc-deficient mice demonstrate shorter wheel-running period and disrupted core body temperature rhythms in constant darkness. Mechanistically, translation of Bmal1 mRNA is increased and BMAL1 protein degradation is decreased, both of which lead to increased BMAL1 protein level and abnormal clock functions in the TSC tissues. Interestingly, reducing the dose of Bmal1 genetically rescues circadian behavioral phenotypes in the TSC mouse models. The results, together with the findings of mTOR regulation of physiological clock properties, support a significant role for mTOR in circadian timekeeping under normal conditions as well as in mediating circadian dysfunction under disease conditions.
Circadian Regulation of mTOR Activities and mRNA Translation
As is the case with many circadian clock-regulated signaling pathways, mTOR activities are regulated by the circadian clock and in turn the rhythmic mTOR activities reinforce the clock function. Indeed, one of the most prominent features of mTOR signaling is the temporal regulation of its activities by the circadian clock. Since mTOR was first studied in the SCN clock a decade ago, dozens of studies have identified circadian mTOR activities in different cells, tissues and organisms. First of all, mTORC1 activities exhibit robust circadian oscillations in the SCN under constant conditions, as indicated by rhythmic S6 and 4E-BP1 phosphorylation (Cao et al., 2011, 2013). In the mouse brain, mTORC1 activities also exhibit daily oscillations in the arcuate nucleus, hippocampus as well as the frontal cortex (Khapre et al., 2014; Saraf et al., 2014; Albert et al., 2015). These brain regions are important for circadian rhythms, feeding, learning, memory, and emotions. In Drosophila, TOR rhythms are also found in the brain and in particular the ventral lateral neurons (Zheng and Sehgal, 2010; Kijak and Pyza, 2017). In peripheral tissues, mTOR activities are rhythmic in the liver, cardiac and skeletal muscles, adipocytes, and retinal photoreceptors (Huang et al., 2013; Jouffe et al., 2013; Shavlakadze et al., 2013; Khapre et al., 2014; Drägert et al., 2015a,b; Lipton et al., 2015, 2017; Chang et al., 2016). Interestingly, mTOR also shows circadian rhythms in human osteosarcomas, mouse renal carcinomas as well as human breast cancer cells (Zhang et al., 2009, 2018; Okazaki et al., 2014). These circadian mTOR studies are summarized in Table 1. It remains elusive, however, what mechanisms drive rhythmic mTOR activities in different tissues and cells. In the SCN, cellular S6K1 activity levels correlates with cellular Per1 but not Per2 transcription due to unknown mechanisms (Cao et al., 2011).
Several mechanistic studies have highlighted a role for mTOR signaling as an output pathway which links circadian rhythmicity to mRNA translation. Jouffe et al. (2013) investigated circadian coordination of mRNA translation in the mouse liver. They identified rhythmic activation of a number of translational control signaling pathways, including the mTORC1 pathway and the ERK/MAPK pathway. They found that the circadian clock influences the temporal translation of a subset of mRNAs that are mainly involved in ribosome biogenesis. The circadian clock also controls the transcription of ribosomal protein mRNAs and ribosomal RNAs. Together these data demonstrate that the circadian clock exerts its function by temporal translation of a subset of mRNAs that are involved in ribosome biogenesis. In another study by the same group, they found that Bmal1 deletion affects both transcriptional and post-transcriptional levels of rhythmic output. Translation efficiencies of genes with 5′-terminal oligopyrimidine tract (5′-TOP) sequences and genes involved in mitochondrial activity (many of which harbor a Translation Initiator of Short 5′-UTR motif) are differentially regulated during the diurnal cycle (Atger et al., 2015).
Lipton et al. (2015) made a surprising finding that the canonical clock protein BMAL1 also functions as a translation factor by associating with the translational machinery and promoting protein synthesis. Interestingly, translational activity of BMAL1 is regulated by rhythmic phosphorylation at Ser42 by the mTORC1/S6K1 pathway. S6K1-mediated phosphorylation is critical for BMAL1 stimulation of protein synthesis. Thus, these results demonstrate that the mTORC1/S6K1 pathway links circadian timing to rhythmic translation via BMAL1. Thus, the transcriptional feedback loop in the circadian clock is coupled to a translational regulatory loop mediated by the mTOR pathway. As translational control is involved in a number of cellular processes, this mechanism is potentially important to understand circadian regulation of many biological processes.
Summary
mRNA translation is subject to complex regulation mechanisms. Among these, temporal regulation of mRNA translation occurs on a daily basis in various tissues as coordinated by the circadian clock and its output signaling pathways such as the mTOR signaling. In turn, rhythmic mTOR signaling and mRNA translation feedback to the clock machinery and regulate important clock functions, including its timing, response to entrainment cues, as well as the network properties among circadian oscillators. Deregulation of translational control is linked to circadian clock dysfunction, as seen in the TSC and hypoxia mouse models. Knowledge of mTOR and translational control in the circadian clock is not only essential for understanding the basic clockwork mechanisms, but also could provide insights into mechanistic links between circadian dysfunctions and human diseases so that therapeutic strategies can be developed for these disorders.
Author Contributions
The author confirms being the sole contributor of this work and approved it for publication.
Conflict of Interest Statement
The author declares that the research was conducted in the absence of any commercial or financial relationships that could be construed as a potential conflict of interest.
References
Albert, V., Cornu, M., and Hall, M. N. (2015). mTORC1 signaling in Agrp neurons mediates circadian expression of Agrp and NPY but is dispensable for regulation of feeding behavior. Biochem. Biophys. Res. Commun. 464, 480–486. doi: 10.1016/j.bbrc.2015.06.161
Atger, F., Gobet, C., Marquis, J., Martin, E., Wang, J., Weger, B., et al. (2015). Circadian and feeding rhythms differentially affect rhythmic mRNA transcription and translation in mouse liver. Proc. Natl. Acad. Sci. U.S.A. 112, E6579–E6588. doi: 10.1073/pnas.1515308112
Aton, S. J., Colwell, C. S., Harmar, A. J., Waschek, J., and Herzog, E. D. (2005). Vasoactive intestinal polypeptide mediates circadian rhythmicity and synchrony in mammalian clock neurons. Nat. Neurosci. 8, 476–483. doi: 10.1038/nn1419
Aton, S. J., and Herzog, E. D. (2005). Come together, right now: synchronization of rhythms in a mammalian circadian clock. Neuron 48, 531–534. doi: 10.1016/j.neuron.2005.11.001
Bradley, S., Narayanan, S., and Rosbash, M. (2012). NAT1/DAP5/p97 and atypical translational control in the Drosophila circadian oscillator. Genetics 192, 943–957. doi: 10.1534/genetics.112.143248
Brunn, G. J., Hudson, C. C., Sekulic, A., Williams, J. M., Hosoi, H., Houghton, P. J., et al. (1997). Phosphorylation of the translational repressor PHAS-I by the mammalian target of rapamycin. Science 277, 99–101. doi: 10.1126/science.277.5322.99
Busino, L., Bassermann, F., Maiolica, A., Lee, C., Nolan, P. M., Godinho, S. I., et al. (2007). SCFFbxl3 controls the oscillation of the circadian clock by directing the degradation of cryptochrome proteins. Science 316, 900–904. doi: 10.1126/science.1141194
Cao, R., Anderson, F. E., Jung, Y. J., Dziema, H., and Obrietan, K. (2011). Circadian regulation of mammalian target of rapamycin signaling in the mouse suprachiasmatic nucleus. Neuroscience 181, 79–88. doi: 10.1016/j.neuroscience.2011.03.005
Cao, R., Gkogkas, C. G., De Zavalia, N., Blum, I. D., Yanagiya, A., Tsukumo, Y., et al. (2015). Light-regulated translational control of circadian behavior by eIF4E phosphorylation. Nat. Neurosci. 18, 855–862. doi: 10.1038/nn.4010
Cao, R., Lee, B., Cho, H. Y., Saklayen, S., and Obrietan, K. (2008). Photic regulation of the mTOR signaling pathway in the suprachiasmatic circadian clock. Mol. Cell. Neurosci. 38, 312–324. doi: 10.1016/j.mcn.2008.03.005
Cao, R., Li, A., Cho, H. Y., Lee, B., and Obrietan, K. (2010). Mammalian target of rapamycin signaling modulates photic entrainment of the suprachiasmatic circadian clock. J. Neurosci. 30, 6302–6314. doi: 10.1523/JNEUROSCI.5482-09.2010
Cao, R., and Obrietan, K. (2010). mTOR signaling and entrainment of the mammalian circadian clock. Mol. Cell. Pharmacol. 2, 125–130.
Cao, R., Robinson, B., Xu, H., Gkogkas, C., Khoutorsky, A., Alain, T., et al. (2013). Translational control of entrainment and synchrony of the suprachiasmatic circadian clock by mTOR/4E-BP1 signaling. Neuron 79, 712–724. doi: 10.1016/j.neuron.2013.06.026
Chang, S. W., Yoshihara, T., Machida, S., and Naito, H. (2016). Circadian rhythm of intracellular protein synthesis signaling in rat cardiac and skeletal muscles. Biochem. Biophys. Rep. 9, 153–158.
Chiu, J. C., Ko, H. W., and Edery, I. (2011). NEMO/NLK phosphorylates PERIOD to initiate a time-delay phosphorylation circuit that sets circadian clock speed. Cell 145, 357–370. doi: 10.1016/j.cell.2011.04.002
Colwell, C. S., Michel, S., Itri, J., Rodriguez, W., Tam, J., Lelievre, V., et al. (2003). Disrupted circadian rhythms in VIP- and PHI-deficient mice. Am. J. Physiol. Regul. Integr. Comp. Physiol. 285, R939–R949. doi: 10.1152/ajpregu.00200.2003
Cutler, D. J., Haraura, M., Reed, H. E., Shen, S., Sheward, W. J., Morrison, C. F., et al. (2003). The mouse VPAC2 receptor confers suprachiasmatic nuclei cellular rhythmicity and responsiveness to vasoactive intestinal polypeptide in vitro. Eur. J. Neurosci. 17, 197–204. doi: 10.1046/j.1460-9568.2003.02425.x
Dorrello, N. V., Peschiaroli, A., Guardavaccaro, D., Colburn, N. H., Sherman, N. E., and Pagano, M. (2006). S6K1- and betaTRCP-mediated degradation of PDCD4 promotes protein translation and cell growth. Science 314, 467–471. doi: 10.1126/science.1130276
Drägert, K., Bhattacharya, I., Hall, M. N., Humar, R., Battegay, E., and Haas, E. (2015a). Basal mTORC2 activity and expression of its components display diurnal variation in mouse perivascular adipose tissue. Biochem. Biophys. Res. Commun. 473, 317–322. doi: 10.1016/j.bbrc.2016.03.102
Drägert, K., Bhattacharya, I., Pellegrini, G., Seebeck, P., Azzi, A., Brown, S. A., et al. (2015b). Deletion of Rictor in brain and fat alters peripheral clock gene expression and increases blood pressure. Hypertension 66, 332–339. doi: 10.1161/HYPERTENSIONAHA.115.05398
Dubowy, C., and Sehgal, A. (2017). Circadian rhythms and sleep in Drosophila melanogaster. Genetics 205, 1373–1397. doi: 10.1534/genetics.115.185157
Evans, J. A. (2016). Collective timekeeping among cells of the master circadian clock. J. Endocrinol. 230, R27–R49. doi: 10.1530/JOE-16-0054
Feeney, K. A., Hansen, L. L., Putker, M., Olivares-Yanez, C., Day, J., Eades, L. J., et al. (2016). Daily magnesium fluxes regulate cellular timekeeping and energy balance. Nature 532, 375–379. doi: 10.1038/nature17407
Feldman, J. F. (1967). Lengthening the period of a biological clock in Euglena by cycloheximide, an inhibitor of protein synthesis. Proc. Natl. Acad. Sci. U.S.A. 57, 1080–1087. doi: 10.1073/pnas.57.4.1080
Feldman, J. F. (1968). Circadian rhythmicity in amino acid incorporation in Euglena gracilis. Science 160, 1454–1456. doi: 10.1126/science.160.3835.1454
Fustin, J. M., Doi, M., Yamaguchi, Y., Hida, H., Nishimura, S., Yoshida, M., et al. (2013). RNA-methylation-dependent RNA processing controls the speed of the circadian clock. Cell 155, 793–806. doi: 10.1016/j.cell.2013.10.026
Gamble, K. L., Berry, R., Frank, S. J., and Young, M. E. (2014). Circadian clock control of endocrine factors. Nat. Rev. Endocrinol. 10, 466–475. doi: 10.1038/nrendo.2014.78
Gillette, M. U., and Mitchell, J. W. (2002). Signaling in the suprachiasmatic nucleus: selectively responsive and integrative. Cell Tissue Res. 309, 99–107. doi: 10.1007/s00441-002-0576-1
Gingras, A. C., Raught, B., and Sonenberg, N. (1999). eIF4 initiation factors: effectors of mRNA recruitment to ribosomes and regulators of translation. Annu. Rev. Biochem. 68, 913–963. doi: 10.1146/annurev.biochem.68.1.913
Golombek, D. A., and Rosenstein, R. E. (2010). Physiology of circadian entrainment. Physiol. Rev. 90, 1063–1102. doi: 10.1152/physrev.00009.2009
González, A., and Hall, M. N. (2017). Nutrient sensing and TOR signaling in yeast and mammals. EMBO J. 36, 397–408. doi: 10.15252/embj.201696010
Gozes, I., and Brenneman, D. E. (1993). Neuropeptides as growth and differentiation factors in general and VIP in particular. J. Mol. Neurosci. 4, 1–9. doi: 10.1007/BF02736685
Gozes, I., Lilling, G., Glazer, R., Ticher, A., Ashkenazi, I. E., Davidson, A., et al. (1995). Superactive lipophilic peptides discriminate multiple vasoactive intestinal peptide receptors. J. Pharmacol. Exp. Ther. 273, 161–167.
Green, C. B. (2018). Circadian posttranscriptional regulatory mechanisms in mammals. Cold Spring Harb. Perspect. Biol. 10:a030692. doi: 10.1101/cshperspect.a030692
Hall, J. C., and Rosbash, M. (1993). Oscillating molecules and how they move circadian clocks across evolutionary boundaries. Proc. Natl. Acad. Sci. U.S.A. 90, 5382–5383. doi: 10.1073/pnas.90.12.5382
Hannibal, J. (2002). Neurotransmitters of the retino-hypothalamic tract. Cell Tissue Res. 309, 73–88. doi: 10.1007/s00441-002-0574-3
Harmar, A. J., Marston, H. M., Shen, S., Spratt, C., West, K. M., Sheward, W. J., et al. (2002). The VPAC(2) receptor is essential for circadian function in the mouse suprachiasmatic nuclei. Cell 109, 497–508. doi: 10.1016/S0092-8674(02)00736-5
Hay, N., and Sonenberg, N. (2004). Upstream and downstream of mTOR. Genes Dev. 18, 1926–1945. doi: 10.1101/gad.1212704
Hershey, J. W., Sonenberg, N., and Mathews, M. B. (2012). Principles of translational control: an overview. Cold Spring Harb. Perspect. Biol. 4:a011528. doi: 10.1101/cshperspect.a011528
Herzog, E. D., Takahashi, J. S., and Block, G. D. (1998). Clock controls circadian period in isolated suprachiasmatic nucleus neurons. Nat. Neurosci. 1, 708–713. doi: 10.1038/3708
Hinnebusch, A. G., Ivanov, I. P., and Sonenberg, N. (2016). Translational control by 5’-untranslated regions of eukaryotic mRNAs. Science 352, 1413–1416. doi: 10.1126/science.aad9868
Hirano, A., Yumimoto, K., Tsunematsu, R., Matsumoto, M., Oyama, M., Kozuka-Hata, H., et al. (2013). FBXL21 regulates oscillation of the circadian clock through ubiquitination and stabilization of cryptochromes. Cell 152, 1106–1118. doi: 10.1016/j.cell.2013.01.054
Holz, M. K., Ballif, B. A., Gygi, S. P., and Blenis, J. (2005). mTOR and S6K1 mediate assembly of the translation preinitiation complex through dynamic protein interchange and ordered phosphorylation events. Cell 123, 569–580. doi: 10.1016/j.cell.2005.10.024
Huang, C. C., Ko, M. L., and Ko, G. Y. (2013). A new functional role for mechanistic/mammalian target of rapamycin complex 1 (mTORC1) in the circadian regulation of L-type voltage-gated calcium channels in avian cone photoreceptors. PLoS One 8:e73315. doi: 10.1371/journal.pone.0073315
Jouffe, C., Cretenet, G., Symul, L., Martin, E., Atger, F., Naef, F., et al. (2013). The circadian clock coordinates ribosome biogenesis. PLoS Biol. 11:e1001455. doi: 10.1371/journal.pbio.1001455
Khapre, R. V., Kondratova, A. A., Patel, S., Dubrovsky, Y., Wrobel, M., Antoch, M. P., et al. (2014). BMAL1-dependent regulation of the mTOR signaling pathway delays aging. Aging (Albany NY) 6, 48–57. doi: 10.18632/aging.100633
Kijak, E., and Pyza, E. (2017). TOR signaling pathway and autophagy are involved in the regulation of circadian rhythms in behavior and plasticity of L2 interneurons in the brain of Drosophila melanogaster. PLoS One 12:e0171848. doi: 10.1371/journal.pone.0171848
Kloss, B., Price, J. L., Saez, L., Blau, J., Rothenfluh, A., Wesley, C. S., et al. (1998). The Drosophila clock gene double-time encodes a protein closely related to human casein kinase Iepsilon. Cell 94, 97–107. doi: 10.1016/S0092-8674(00)81225-8
Knebel, A., Haydon, C. E., Morrice, N., and Cohen, P. (2002). Stress-induced regulation of eukaryotic elongation factor 2 kinase by SB 203580-sensitive and -insensitive pathways. Biochem. J. 367, 525–532. doi: 10.1042/bj20020916
Lane, N., and Martin, W. (2010). The energetics of genome complexity. Nature 467, 929–934. doi: 10.1038/nature09486
Lee, C., Etchegaray, J. P., Cagampang, F. R., Loudon, A. S., and Reppert, S. M. (2001). Posttranslational mechanisms regulate the mammalian circadian clock. Cell 107, 855–867. doi: 10.1016/S0092-8674(01)00610-9
Lim, C., and Allada, R. (2013a). ATAXIN-2 activates PERIOD translation to sustain circadian rhythms in Drosophila. Science 340, 875–879. doi: 10.1126/science.1234785
Lim, C., and Allada, R. (2013b). Emerging roles for post-transcriptional regulation in circadian clocks. Nat. Neurosci. 16, 1544–1550. doi: 10.1038/nn.3543
Lim, C., Lee, J., Choi, C., Kilman, V. L., Kim, J., Park, S. M., et al. (2011). The novel gene twenty-four defines a critical translational step in the Drosophila clock. Nature 470, 399–403. doi: 10.1038/nature09728
Lipton, J. O., Boyle, L. M., Yuan, E. D., Hochstrasser, K. J., Chifamba, F. F., Nathan, A., et al. (2017). Aberrant proteostasis of BMAL1 UNDERLIES circadian abnormalities in a paradigmatic mTOR-opathy. Cell Rep. 20, 868–880. doi: 10.1016/j.celrep.2017.07.008
Lipton, J. O., and Sahin, M. (2014). The neurology of mTOR. Neuron 84, 275–291. doi: 10.1016/j.neuron.2014.09.034
Lipton, J. O., Yuan, E. D., Boyle, L. M., Ebrahimi-Fakhari, D., Kwiatkowski, E., Nathan, A., et al. (2015). The circadian protein BMAL1 regulates translation in response to S6K1-mediated phosphorylation. Cell 161, 1138–1151. doi: 10.1016/j.cell.2015.04.002
Liu, A. C., Welsh, D. K., Ko, C. H., Tran, H. G., Zhang, E. E., Priest, A. A., et al. (2007). Intercellular coupling confers robustness against mutations in the SCN circadian clock network. Cell 129, 605–616. doi: 10.1016/j.cell.2007.02.047
Liu, D., Stowie, A., De Zavalia, N., Leise, T., Pathak, S. S., Drewes, L. R., et al. (2018). mTOR signaling in VIP neurons regulates circadian clock synchrony and olfaction. Proc. Natl. Acad. Sci. U.S.A. 115, E3296–E3304. doi: 10.1073/pnas.1721578115
Lowrey, P. L., Shimomura, K., Antoch, M. P., Yamazaki, S., Zemenides, P. D., Ralph, M. R., et al. (2000). Positional syntenic cloning and functional characterization of the mammalian circadian mutation tau. Science 288, 483–492. doi: 10.1126/science.288.5465.483
Ma, X. M., Yoon, S. O., Richardson, C. J., Julich, K., and Blenis, J. (2008). SKAR links pre-mRNA splicing to mTOR/S6K1-mediated enhanced translation efficiency of spliced mRNAs. Cell 133, 303–313. doi: 10.1016/j.cell.2008.02.031
Maywood, E. S., Reddy, A. B., Wong, G. K., O’neill, J. S., O’brien, J. A., Mcmahon, D. G., et al. (2006). Synchronization and maintenance of timekeeping in suprachiasmatic circadian clock cells by neuropeptidergic signaling. Curr. Biol. 16, 599–605. doi: 10.1016/j.cub.2006.02.023
Meng, Q. J., Logunova, L., Maywood, E. S., Gallego, M., Lebiecki, J., Brown, T. M., et al. (2008). Setting clock speed in mammals: the CK1 epsilon tau mutation in mice accelerates circadian pacemakers by selectively destabilizing PERIOD proteins. Neuron 58, 78–88. doi: 10.1016/j.neuron.2008.01.019
Meyuhas, O., and Dreazen, A. (2009). Ribosomal protein S6 kinase from TOP mRNAs to cell size. Prog. Mol. Biol. Transl. Sci. 90, 109–153. doi: 10.1016/S1877-1173(09)90003-5
Mohawk, J. A., Green, C. B., and Takahashi, J. S. (2012). Central and peripheral circadian clocks in mammals. Annu. Rev. Neurosci. 35, 445–462. doi: 10.1146/annurev-neuro-060909-153128
Moore, R. Y. (2013). The suprachiasmatic nucleus and the circadian timing system. Prog. Mol. Biol. Transl. Sci. 119, 1–28. doi: 10.1016/B978-0-12-396971-2.00001-4
Okazaki, H., Matsunaga, N., Fujioka, T., Okazaki, F., Akagawa, Y., Tsurudome, Y., et al. (2014). Circadian regulation of mTOR by the ubiquitin pathway in renal cell carcinoma. Cancer Res. 74, 543–551. doi: 10.1158/0008-5472.CAN-12-3241
Panda, S. (2007). Multiple photopigments entrain the Mammalian circadian oscillator. Neuron 53, 619–621. doi: 10.1016/j.neuron.2007.02.017
Peirson, S., and Foster, R. G. (2006). Melanopsin: another way of signaling light. Neuron 49, 331–339. doi: 10.1016/j.neuron.2006.01.006
Ramanathan, C., Kathale, N. D., Liu, D., Lee, C., Freeman, D. A., Hogenesch, J. B., et al. (2018). mTOR signaling regulates central and peripheral circadian clock function. PLoS Genet. 14:e1007369. doi: 10.1371/journal.pgen.1007369
Ratnayake, L., Adhvaryu, K. K., Kafes, E., Motavaze, K., and Lakin-Thomas, P. (2018). A component of the TOR (Target Of Rapamycin) nutrient-sensing pathway plays a role in circadian rhythmicity in Neurospora crassa. PLoS Genet. 14:e1007457. doi: 10.1371/journal.pgen.1007457
Reppert, S. M., and Weaver, D. R. (2002). Coordination of circadian timing in mammals. Nature 418, 935–941. doi: 10.1038/nature00965
Rosbash, M. (2009). The implications of multiple circadian clock origins. PLoS Biol. 7:e62. doi: 10.1371/journal.pbio.1000062
Saraf, A., Luo, J., Morris, D. R., and Storm, D. R. (2014). Phosphorylation of eukaryotic translation initiation factor 4E and eukaryotic translation initiation factor 4E-binding protein (4EBP) and their upstream signaling components undergo diurnal oscillation in the mouse hippocampus: implications for memory persistence. J. Biol. Chem. 289, 20129–20138. doi: 10.1074/jbc.M114.552638
Saxton, R. A., and Sabatini, D. M. (2017). mTOR signaling in growth, metabolism, and disease. Cell 168, 960–976. doi: 10.1016/j.cell.2017.02.004
Shavlakadze, T., Anwari, T., Soffe, Z., Cozens, G., Mark, P. J., Gondro, C., et al. (2013). Impact of fasting on the rhythmic expression of myogenic and metabolic factors in skeletal muscle of adult mice. Am. J. Physiol. Cell Physiol. 305, C26–C35. doi: 10.1152/ajpcell.00027.2013
Shirakawa, T., Honma, S., Katsuno, Y., Oguchi, H., and Honma, K. I. (2000). Synchronization of circadian firing rhythms in cultured rat suprachiasmatic neurons. Eur. J. Neurosci. 12, 2833–2838. doi: 10.1046/j.1460-9568.2000.00170.x
Siepka, S. M., Yoo, S. H., Park, J., Song, W., Kumar, V., Hu, Y., et al. (2007). Circadian mutant overtime reveals F-box protein FBXL3 regulation of cryptochrome and period gene expression. Cell 129, 1011–1023. doi: 10.1016/j.cell.2007.04.030
Sonenberg, N., and Hinnebusch, A. G. (2007). New modes of translational control in development, behavior, and disease. Mol. Cell. 28, 721–729. doi: 10.1016/j.molcel.2007.11.018
Stolovich, M., Tang, H., Hornstein, E., Levy, G., Cohen, R., Bae, S. S., et al. (2002). Transduction of growth or mitogenic signals into translational activation of TOP mRNAs is fully reliant on the phosphatidylinositol 3-kinase-mediated pathway but requires neither S6K1 nor rpS6 phosphorylation. Mol. Cell. Biol. 22, 8101–8113. doi: 10.1128/MCB.22.23.8101-8113.2002
Takahashi, J. S. (2017). Transcriptional architecture of the mammalian circadian clock. Nat. Rev. Genet. 18, 164–179. doi: 10.1038/nrg.2016.150
Takahashi, J. S., Hong, H. K., Ko, C. H., and Mcdearmon, E. L. (2008). The genetics of mammalian circadian order and disorder: implications for physiology and disease. Nat. Rev. Genet. 9, 764–775. doi: 10.1038/nrg2430
Torres, M., Becquet, D., Franc, J. L., and Francois-Bellan, A. M. (2018). Circadian processes in the RNA life cycle. Wiley Interdiscip. Rev. RNA 9:e1467. doi: 10.1002/wrna.1467
Walton, Z. E., Patel, C. H., Brooks, R. C., Yu, Y., Ibrahim-Hashim, A., Riddle, M., et al. (2018). Acid suspends the circadian clock in hypoxia through Inhibition of mTOR. Cell 174:e32. doi: 10.1016/j.cell.2018.05.009
Wang, L., Gout, I., and Proud, C. G. (2001). Cross-talk between the ERK and p70 S6 kinase (S6K) signaling pathways, MEK-dependent activation of S6K2 in cardiomyocytes. J. Biol. Chem. 276, 32670–32677. doi: 10.1074/jbc.M102776200
Welsh, D. K., Logothetis, D. E., Meister, M., and Reppert, S. M. (1995). Individual neurons dissociated from rat suprachiasmatic nucleus express independently phased circadian firing rhythms. Neuron 14, 697–706. doi: 10.1016/0896-6273(95)90214-7
Welsh, D. K., Takahashi, J. S., and Kay, S. A. (2010). Suprachiasmatic nucleus: cell autonomy and network properties. Annu. Rev. Physiol. 72, 551–577. doi: 10.1146/annurev-physiol-021909-135919
Yoo, S. H., Mohawk, J. A., Siepka, S. M., Shan, Y., Huh, S. K., Hong, H. K., et al. (2013). Competing E3 ubiquitin ligases govern circadian periodicity by degradation of CRY in nucleus and cytoplasm. Cell 152, 1091–1105. doi: 10.1016/j.cell.2013.01.055
Young, M. W. (1998). The molecular control of circadian behavioral rhythms and their entrainment in Drosophila. Annu. Rev. Biochem. 67, 135–152. doi: 10.1146/annurev.biochem.67.1.135
Zhang, E. E., Liu, A. C., Hirota, T., Miraglia, L. J., Welch, G., Pongsawakul, P. Y., et al. (2009). A genome-wide RNAi screen for modifiers of the circadian clock in human cells. Cell 139, 199–210. doi: 10.1016/j.cell.2009.08.031
Zhang, Y., Giacchetti, S., Parouchev, A., Hadadi, E., Li, X., Dallmann, R., et al. (2018). Dosing time dependent in vitro pharmacodynamics of Everolimus despite a defective circadian clock. Cell Cycle 17, 33–42. doi: 10.1080/15384101.2017.1387695
Zhang, Y., Ling, J., Yuan, C., Dubruille, R., and Emery, P. (2013). A role for Drosophila ATX2 in activation of PER translation and circadian behavior. Science 340, 879–882. doi: 10.1126/science.1234746
Keywords: mRNA, translational control, circadian clock, mTOR, entrainment, synchronization, oscillation, SCN
Citation: Cao R (2018) mTOR Signaling, Translational Control, and the Circadian Clock. Front. Genet. 9:367. doi: 10.3389/fgene.2018.00367
Received: 25 June 2018; Accepted: 22 August 2018;
Published: 10 September 2018.
Edited by:
Maritza Jaramillo, Institut National de la Recherche Scientifique (INRS), CanadaReviewed by:
Yoshihiro Shimizu, RIKEN, JapanFabrizio Loreni, Università degli Studi di Roma “Tor Vergata”, Italy
Copyright © 2018 Cao. This is an open-access article distributed under the terms of the Creative Commons Attribution License (CC BY). The use, distribution or reproduction in other forums is permitted, provided the original author(s) and the copyright owner(s) are credited and that the original publication in this journal is cited, in accordance with accepted academic practice. No use, distribution or reproduction is permitted which does not comply with these terms.
*Correspondence: Ruifeng Cao, cmNhb0B1bW4uZWR1