- 1College of Veterinary Medicine, Northwest A&F University, Xianyang, China
- 2China Animal Health and Epidemiology Center, Qingdao, China
Clustered regularly interspaced short palindromic repeats (CRISPR)-associated protein 9 (Cas9) is a precise genome manipulating technology that can be programmed to induce double-strand break (DSB) in the genome wherever needed. After nuclease cleavage, DSBs can be repaired by non-homologous end joining (NHEJ) or homology-directed repair (HDR) pathway. For producing targeted gene knock-in or other specific mutations, DSBs should be repaired by the HDR pathway. While NHEJ can cause various length insertions/deletion mutations (indels), which can lead the targeted gene to lose its function by shifting the open reading frame (ORF). Furthermore, HDR has low efficiency compared with the NHEJ pathway. In order to modify the gene precisely, numerous methods arose by inhibiting NHEJ or enhancing HDR, such as chemical modulation, synchronized expression, and overlapping homology arm. Here we focus on the efficiency and other considerations of these methodologies.
Overview of CRISPR-Cas9 System
Clustered regularly interspaced short palindromic repeats (CRISPR) represents a family of DNA sequences in bacteria and archaea (Barrangou, 2015). This family is characterized by direct palindromic repeats, where sequences are the same in both directions, varying in size from 21 to 37 bp (Barrangou and Marraffini, 2014), interspaced by spacers, which have fragments gathered from viruses or phages that previously tried to infect the cell (Horvath and Barrangou, 2010; Morange, 2015). To appreciate this characteristic array, it is termed CRISPR (Jansen et al., 2002; Mojica and Rodriguez-Valera, 2016). CRISPR-associated (cas) genes are invariably located adjacent to a CRISPR locus (Jansen et al., 2002). The CRISPR-Cas system can be grouped into three types: type I, type II, and type III. In addition, there are 12 subtypes of the CRISPR-Cas system, which are based on their exclusive genetic content and structural differences (Makarova et al., 2015). Cas1 and cas2 are universal across types and subtypes, whereas cas3, cas9, cas10 are signature genes for type I, type II, and type III, respectively (Makarova et al., 2011). Here in this review we only focus on type II. The CRISPR-Cas system functions as a defense system in bacteria and archaea against bacteriophage infection, conjugation, and natural transformation by degrading foreign nucleic acid that enters the cell (Marraffini, 2015). The CRISPR-Cas system involves three distinct mechanistic stages: adaptation, biogenesis, and interference (Marraffini and Sontheimer, 2010). The adaptation stage involves the integration of fragments of foreign DNA (termed “protospacers,” captured, excised, and inserted by Cas proteins) into the CRISPR array as new spacers. New spacers are usually added at the beginning of the CRISPR locus next to the leader sequence, creating a chronological record of viral infections (Sorek et al., 2013) and protecting the cell from further infection. During the biogenesis stage, the CRISPR array is transcribed as a single long transcript (termed “pre-crRNA”) containing much of the CRISPR array (Marraffini and Sontheimer, 2010) and is then processed and matured to produce CRISPR RNAs (crRNAs) with only one spacer sequence. As for the interference stage, the spacers in these crRNAs guide cas proteins to foreign DNAs and cleave them (van der Oost et al., 2009; Wiedenheft et al., 2012; Barrangou, 2013). The type II CRISPR-Cas system needs only cas9 to execute immunity in the presence of an existing targeting spacer sequence (Sapranauskas et al., 2011). It requires two small RNAs: the crRNA and the trans-encoded crRNA(tracrRNA) (Deltcheva et al., 2011). TracrRNA forms a secondary structure that interacts with cas9 protein and it has a complementary region that enables itself to bind to pre-crRNA (Anders et al., 2014; Jinek et al., 2014; Nishimasu et al., 2014). The dsRNA formed between pre-crRNA and tracrRNA is then handled by RNase III to form mature crRNA guides that are used in genome editing. When crRNA and tracrRNA are combined together, they are collectively termed as guide RNA (gRNA) (Jinek et al., 2012). Another important short (3–5 bp) DNA termed protospacer adjacent motif (PAM) is required for targeting. PAM is a component of the invading virus or plasmid, but it is not a component of the bacterial CRISPR locus. Cas9 will not successfully bind to or cleave the target DNA sequence if it is not followed by the PAM sequence (Mojica et al., 2009). The first step in target recognition is the transient binding of Cas9 to PAM sequences within the target DNA, which promotes the unwinding of the two DNA strands immediately upstream of the PAM (Sternberg et al., 2014), the spacer sequence of the crRNA binds with the unwinded DNA (6–8 bp in length), then forms an RNA-DNA heteroduplex and triggers cleavage at the targeted site (Sternberg et al., 2014; Szczelkun et al., 2014). After recognition, the CRISPR-Cas9 system introduces a crRNA-specific DSB in the target sequence, which is further resolved either by homology-directed repair (HDR) or non-homologous end joining (NHEJ).
NHEJ Pathway
Typically, cells employ two main mechanisms to repair DSBs: classical NHEJ and HDR (Symington and Gautier, 2011). There are also many alternative error-prone DSB repair pathways: single-strand annealing (SSA) and breakage-induced replication (BIR) (Pardo et al., 2009; Jasin and Rothstein, 2013). SSA does not require a homologous template, and rejoining DNA ends with direct sequence repeats (Symington, 2014). BIR repairs one-ended DSBs, a process that is caused by the collapse of a replication fork (Mayle et al., 2015). When DSBs occur in cells, the first reaction is usually carried out in an NHEJ manner. Compared to other DNA repair and DNA recombination pathways, the NHEJ pathway is a robust, error-prone but predominant and fast pathway with high flexibility. It can recognize diverse end structures at DSBs and accomplish diverse repair results (Aravind and Koonin, 2001; Gu and Lieber, 2008; Salsman and Dellaire, 2017). NHEJ can be classified into two types: canonical NHEJ (c-NHEJ) and alternative NHEJ (alt-NHEJ), also called microhomology-mediated end-joining (MMEJ) (Bae et al., 2014). c-NHEJ is active throughout the cell cycle and stabilizes the DSB from translocations (Roth et al., 1995; Soutoglou et al., 2007). Based on different DNA ends, NHEJ is capable of employing different strategies. The whole process deals with assembling the core complex, which recognizes broken ends and keeps them together so that the following processing factors can act (Waters et al., 2014). The core complex is considered to include the Ku heterodimer (Ku80/70), the DNA-dependent protein kinase catalytic subunit (DNA-PKcs), DNA ligase IV, the X-ray repair cross-complementing protein 4 (XRCC4), the XRCC4-like factor (XLF, or Cernunnos). Ku is a heterodimer, composed of two subunits (70 and 83 kD), that recognizes and binds to blunt DSBs first (Walker et al., 2001). In c-NHEJ, Ku recruits DNA-PKcs to the DSB site and forms a very stable complex that remains bound to the end (Weterings et al., 2003). Their assembly activates the kinase activity of DNA-PK and orchestrates c-NHEJ (Davis et al., 2014; Radhakrishnan et al., 2014). DNA-PK phosphorylates a host of DNA damage response proteins and thus regulates c-NHEJ and DSB processing and recruits Artemis nuclease (Moshous et al., 2001). However, DNA-PK mostly phosphorylates itself, which is crucial in DSB processing (Neal et al., 2014). Artemis has 5′ to 3′ single-stranded DNA exonuclease activity and DNA-PKcs-dependent 5′ and 3′ endonuclease activity on hairpins and single-stranded overhangs (Moshous et al., 2001). Ku and DNA-PKcs alone can also promote multiple DNA end-processing activities at the break site. The X family of DNA polymerases (pol mu and pol lambda) adds missing nucleotides at the DSB ends (Daley et al., 2005; Paull, 2005). Next, the DSBs will be ligated by Ligase IV/XRCC4/XLF, which is regulated by DNA-PK. Ligase IV/XRCC4/XLF forms an extended filament that wraps and stabilizes DNA and stimulates ligation (Tsai et al., 2007; Andres et al., 2012). Recent research also showed that a newly identified PAXX (a paralog of XRCC4 and XLF), a member of the XRCC4 superfamily, is another important mediator of c-NHEJ, which interacts directly with Ku. In most cases DNA is repaired via the c-NHEJ pathway and its efficiency can approach nearly 90% (Yang et al., 2013; Dow et al., 2015), which constitutes the basis of CRISPR/Cas9 technology (Vartak and Raghavan, 2015). The NHEJ process is illustrated in Figure 1.
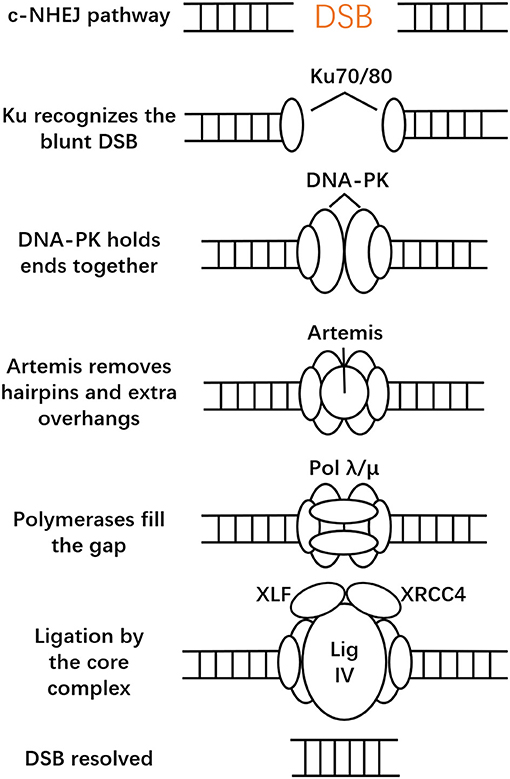
Figure 1. Canonical non-homologous end joining (c-NHEJ). After CRISPR-Cas9 introduced a DSB, NHEJ is initiated by the binding of the Ku heterodimeric complex. This then forms the core complex, which is considered to recognize broken ends and keeps them together. The ends will then be ligated by various ligases.
HDR Pathway
HDR is a faithful repair pathway. It comes into action mainly in the S- or G2-phase of the cell cycle and requires homologous DNA sequences. Homologous recombination is the desired mechanism for precise genome editing, which only happens in the presence of a homologous duplex template to repair the broken site. When DSB occurs, pathway choice depends on end resection (Symington and Gautier, 2011). The MRE11-RAD50-NBS1 (MRN, MRX in yeast) complex recognizes dsDNA and first creates a nick 15–20 bp from the 5′-ends of the DSB (Symington, 2014). Exonucleases such as SGS1-DNA2 and EXO1 complete the resection step (Kim and Mirkin, 2018). It then moves into flanking dsDNA regions and recruits ataxia telangiectasia mutated (ATM) kinase, the key upstream kinase of DSB signaling (Falck et al., 2005), and interacts with CtIP (Makharashvili and Paull, 2015). MRN also tethers DNA ends, which increases its local concentration and thus facilitates ATM activation (Dupre et al., 2006).
The MRN complex consists of three subunits. MRE11 is a Mn2+ dependent nuclease involved in homologous recombination, telomere maintenance, and DNA DSB repair (Paull, 2015). SAE2 activates MRE11 for its dsDNA-specific endonuclease activity (Cannavo and Cejka, 2014) and regulates the resection step during appropriate stages of the cell cycle (Mathiasen and Lisby, 2014). RAD50 belongs to the structural maintenance of chromosomes (SMC) family, and contains ATPase activity (de Jager et al., 2001). RAD50 becomes dimerized and its DNA-binding activity is activated after ATP binding. Two MRE11 genes will then bind to the ATPase heads of the RAD50 homodimer, enabling itself to interact with RAD50 (Williams et al., 2008). RAD50 forms the core of MRN and uses its extended coiled-coil domain to tether DSB ends during HR (Williams et al., 2010; Hohl et al., 2011). NBS1 contains a Fork-Head associated (FHA) domain and BRCA1 C-terminal (BRCT) domain, binds MRE11 and recruits ATM, linking the core MRN activities to DNA damage response (DDR) proteins domains at its N terminus (Glover et al., 2004; Williams et al., 2009).
Histone variant H2AX is phosphorylated by ATM, which becomes γH2AX throughout the area surrounding the breakage within seconds after damage occurs (Rogakou et al., 1998). This sets off elaborate ubiquitylation and SUMOylation cascades to promote recruitment of BRCA1 (Morris et al., 2009) and 53BP1 (Stewart, 2009) but it is not crucial for the activation of ATM substrates such as CHK2 and p53 (Kang et al., 2005). Then MDC1, a large nuclear factor, directly binds to γH2AX and functions as a molecular scaffold that interacts with ATM and NBS1, promoting further MRN accumulation. In addition, MDC1 also helps ATM spread on DSB-flanking chromatin and furthers H2AX activation (Spycher et al., 2008). It also mediates the accumulation of many DDR factors, including 53BP1 and BRCA1 (Wang et al., 2002; Stucki and Jackson, 2006; Kim et al., 2007). ATM leads to phosphorylation of DDR cascades such as BRCA1, Chk2, p53, etc., (Shiloh, 2003; Lavin, 2008). DSBs also activate ataxia telangiectasia and RAD3-related protein (ATR). Full ATR activation requires not only itself and DNA damage sensors but also proteins that function as signal transducers and effectors, such as RPA, RAD17, TopBP1, Claspin, and Chk1. ssDNA overhangs will be coated by replication protein A (RPA) rapidly, and the ssDNA-RPA complex acts as a scaffold to attract ATR/ATR-interacting protein (ATRIP) (Zou and Elledge, 2003) and other DNA damage checkpoint kinases to trigger DDR (Chen and Wold, 2014). It will then be replaced with adenosine triphosphate (ATP)-dependent recombinase RAD51 (San Filippo et al., 2008) with the help of BRCA1 and BRCA2 as described above (Prakash et al., 2015). ssDNA can be generated by nuclease resection, such as the MRN-C-terminal binding protein interacting protein (CtIP) for short resection, and EXO1/BLM for long resection (Mladenov et al., 2016). In mammals, resection depends on CtIP and needs to be phosphorylated by CDK first (Huertas and Jackson, 2009). BRCA1 contributes to HR by colocalizing with MRN after DNA damage occurs and interacts directly with the resection factor CtIP (Sartori et al., 2007). BRCA1 assists RAD51 binding to ssDNA by evicting RPA (Zelensky et al., 2014) and promotes the recruitment of BRCA2 to DSBs through the bridging protein PALB2 (Sy et al., 2009). BRCA1 also appears to inhibit the resection suppressor 53BP1 (Bunting et al., 2010). RAD51 is a DNA strand-exchange protein that exists in mammalian cells and forms a filament referred to as the presynaptic complex (van der Heijden et al., 2007; Hilario et al., 2009). The assembly of a RAD51 nucleoprotein filament promotes homologous search by locating and pairing the 3′-overhang with a homologous duplex DNA and catalyzing strand invasion (termed single-end invasion, SEI) (Morrical, 2015; Ma et al., 2017). The two ends of the DSB are identical, but one end serves as the “first end,” which searches for the homologous sequence and forms a displacement loops (D-loops) structure while the other end waits for the latter process (Kim and Mirkin, 2018). Besides RAD51, DNA strand exchange also requires RAD54 and RDH54/TID1, which performs this step by stabilizing RAD51-ssDNA presynaptic filaments (Mazin et al., 2003).
Resolution of the exchanged DNA strands includes the Holliday Junction (HJ) pathway and the synthesis-dependent strand annealing (SDSA) pathway. Dissolution is the primary pathway for HJ resolution, which involves the BLM helicase-Topoisomerase IIIα-RMI1-RMI2 (BTR) complex. The BTR complex promotes branch migration of Holliday junctions (Karow et al., 2000) and also acts to suppress crossing over during homologous recombination (Wu and Hickson, 2003). Thus, this dissolution pathway gives rise exclusively to non-crossovers. The other pathways use structure-selective resolvases (SLX-MUS complex and GEN1) to process the exchange intermediates and can produce both crossover and non-crossover products (West et al., 2015). The SLX1 and MUS81-EME1 nucleases bind in close proximity on the SLX4 scaffold and process HJs (Castor et al., 2013). SLX1 catalyzes the initial incision and MUS81 introduces the second cut on the opposing strand (Wyatt and West, 2014). GEN1 is a member of the RAD2/XPG family and can only access and cleave recombination intermediates when the nuclear membrane breaks down (Rass et al., 2010). GEN1 first forms a dimeric complex that contains the two active sites and then performs a dual symmetric incision at HJs, generating nicked duplex products that can be ligated.
The SDSA pathway also begins with the generation of a D-loop structure like the HJ pathway but also includes DNA synthesis in the 3′-direction, which extends the heteroduplex (Daley et al., 2014). The translocating D-loop then collapses, and the other resected DSB end will anneal to this extended DSB end. Both ends will go through replicative extension and ligation, which generates non-crossover products.
When it comes to single-stranded template repair (SSTR), the repair mechanism is quite different from the dsDNA repair template scenario. Richardson et al. found that human Cas9-induced SSTR requires the Fanconi anemia (FA) pathway, which was previously implicated in responses to interstrand crosslinks rather than nuclease-induced breaks (Richardson et al., 2018). They confirmed that SSTR is RAD51-independent while dsDNA donor HDR is RAD51-dependent. After FA pathway knockdown, the efficiency of SSTR decreased while simultaneously the levels of NHEJ increased, and the total editing stayed relatively constant. This means that the FA pathway can drive the repair events from NHEJ to SSTR. Additionally, FA pathway knockdown specifically inhibits SSTR and has no effect on NHEJ. RAD51C and XRCC3 are required for SSTR, but RAD51B and XRCC2 are not. They also found that FANCD2, a central player in the FA pathway, enriched even in the absence of an exogenous homology donor. In short, SSTR is much more efficient than HDR from a dsDNA donor but still needs future investigations. The HDR pathway is demonstrated in Figure 2.
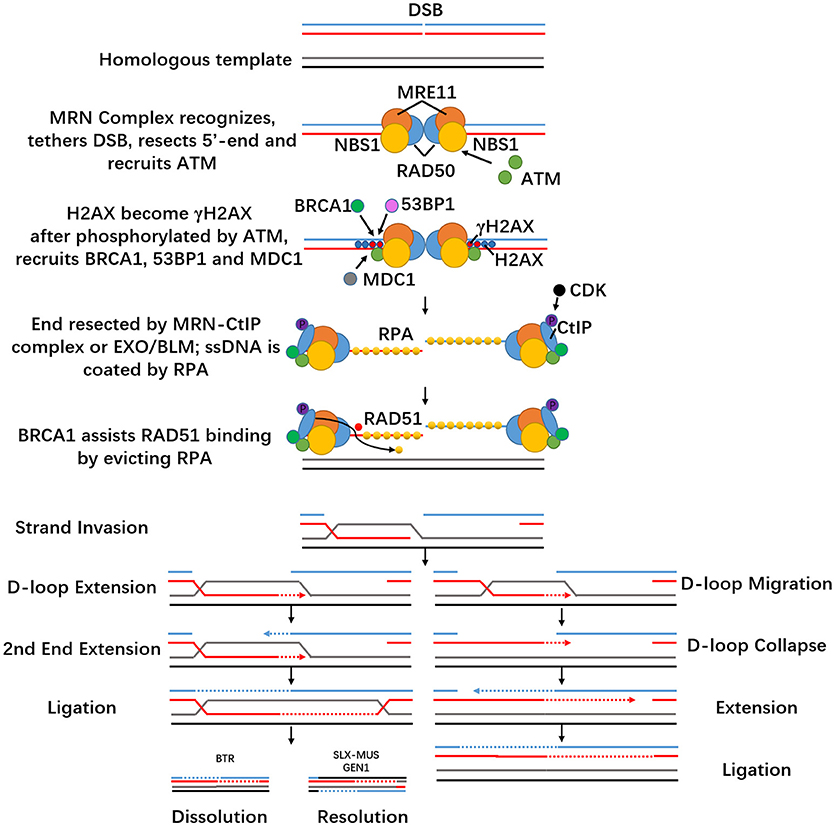
Figure 2. Homology-directed repair HDR. When DSB happens in the S- or G2-phase of the cell cycle and homologous sequence exists near the DSB, DSB can be handled through the HDR pathway if the ends of the DSB are resected. Ends will be coated with various proteins and then invade homologous duplex DNA to form an exchange intermediate: the D-loop structure. Most D-loop structures will be extended by DNA synthesis (dashed arrow). The second end pairs to the D-loop and starts extension. This pathway is called the double Holliday junction pathway. Ligation generates the characteristic double Holliday junction, which may be cleaved by HJ resolvases into either crossover or non-crossover products. The synthesis-dependent strand annealing pathway is illustrated on the right. After D-loop formation, replication and branch migration take place which can lead to D-loop translocation. The translocating D-loop is unstable and collapses easily. After collapse, the extended first end may anneal to complementary ssDNA in the resected second end. Replicative extension of both ends and ligation generates non-crossover products.
Favoring the HDR Pathway Using Chemical and Genetic Modulation
DSBs caused by Cas9 can go through both the NHEJ and HDR pathways, but in most cases they are handled by NHEJ (Frit et al., 2014), so it seems reasonable to inhibit key enzymes (e.g., DNA ligase IV) of the NHEJ pathway. Maruyama et al. (2015) investigated the effect of SCR7, a putative inhibitor of ligase IV, which targets the DNA binding domain of ligase IV, impeding its ability to bind to DSBs (Srivastava et al., 2012) in human epithelial (A549) and melanoma (MelJuSo) cell lines. Results showed that SCR7 promotes a 21 bp precise insertion (ssDNA donor with two 100 bp homology arms) in A549 cells 3-fold at 0.01 μM and 19-fold for MelJuSo at 1 μM. They also assessed the effect of SCR7 on insertion of a ~800 bp fragment (ssDNA donor with ~80 bp homology flanking sequence on both sides of the DSB) into a murine bone marrow-derived dendritic cell line (DC2.4 cells). After treating the DC2.4 cells with 1 μM SCR7, the efficiency of insertion increased by ~13-fold. It is worth mentioning that SCR7 affects lymphocyte development and can induce apoptosis. As additional approaches to DNA ligase IV inhibition, Chu et al. used the adenovirus 4 (Ad4) E1B55K and E4orf6 proteins to suppress NHEJ. These two proteins can mediate the ubiquitination and proteasomal degradation of DNA ligase IV (Forrester et al., 2011). Results showed that HDR efficiency was enhanced up to 7-fold (5 to 36%) by the Ad4 protein in human HEK293 cells. And in a mouse Burkitt lymphoma cell line, the addition of Ad4 proteins reduced transfection efficiency from 40 to 27%, but promoted HDR by 5-fold (Chu et al., 2015). Yu et al. identified two small molecules (L755507 and Brefeldin A) that could improve HDR efficiency. L755507, a β3-adrenergic receptor agonist, can increase HDR by 3-fold at 5 μM in mouse ESCs. Brefeldin A, an inhibitor of intracellular protein transport from the ER to the Golgi apparatus, promotes HDR by 2-fold at 0.1 μM in mouse ESCs (Yu et al., 2015).
Pinder et al. identified that RS-1 can enhance HDR between 3- and 6-fold, varying with the locus and transfection factor in HEK-293A human embryonic kidney and U2OS osteosarcoma cell lines (Pinder et al., 2015). RS-1 is a compound that stabilizes the association between Rad51 and DNA. They also found that an optimized ratio for Cas9/gRNA to homology donor plasmid doubled the HDR efficiency. Upon BRCA1 over-expression, HDR is increased by 2- to 3-fold.
Besides these inhibiting molecules, HDR can also be promoted using small interfering RNA (siRNA) to inhibit the expression of Ku protein, which is the pioneer protein in the NHEJ pathway. Li et al. assessed this method on pig fetal fibroblasts (Li et al., 2018). The result showed that by inhibiting Ku70 or Ku80 expression, HR can be promoted by 1.6- to 3-fold, as well as SSA and ssODN-mediated repairs. Yu et al. constructed a Rad51 and a Rad50 co-expression vector to evaluate its performance (Yu et al., 2011). It was determined that HR efficiency increased 110–245%. Chu et al. used short hairpin RNA (shRNA) in HEK293 cells to suppress key NHEJ pathway proteins such as KU70, KU80, and DNA ligase IV. Results showed that HDR efficiency was enhanced from 5 to 8–14% when transfected with single shRNAs against KU70, KU80, or DNA ligase IV. Furthermore, they found that the expression of the target gene diminished in the cells undergoing NHEJ blockade, which may be caused by local chromatin remodeling through extended DNA damage (Chu et al., 2015).
Timed Delivery of the CRISPR-Cas9 System
While HDR is typically restricted to the S and G2 phases of the cell cycle, its efficiency can be increased by synchronizing and capturing cells at the S and G2 phases or using timed delivery. Lin et al. combined cell cycle synchronization techniques, using chemical inhibitors to arrest the cells at specific phases of the cell cycle with direct nucleofection of pre-assembled Cas9 ribonucleoprotein (RNP) in HEK293T cells (Lin et al., 2014). Results showed that using lower Cas9/RNP concentrations and cell cycle arrest can improve HDR efficiency to 31% (3.4-fold) at maximum. Consistent with this strategy, Yang et al. successfully enhanced HDR by three- to six-fold using the microtubule polymerization inhibitor nocodazole or ABT-751 (Yang et al., 2016). As for non-proliferating cells, BRCA1 is inhibited by the 53BP1 (Escribano-Diaz et al., 2013) and KEAP1-CUL3 complexes (Orthwein et al., 2015), and RIF1 thus will not bind to DSB. In addition, CtIP can function normally after being phosphorylated by CDK, but CDK is absent when cells stay in the G0/G1 phase (Escribano-Diaz et al., 2013). In order to overcome this inhibition, Orthwein et al. overexpressed mutated activated CtIP. This depleted the 53BP1 and KEAP1-CUL3 complexes simultaneously, which successfully activated the HDR pathway in G1 cells (Orthwein et al., 2015).
Enhancing HDR by Using Overlapping Sequences
Several types of donor DNA have been used, such as plasmid DNA and synthetic oligonucleotides (Carroll and Beumer, 2014). Rational design of homology repair templates strongly enhances HDR efficiency (Renaud et al., 2016). By using a linear repair template with homologous flanks in zebrafish, HDR can increase by almost 10-fold (Irion et al., 2014). Chu et al. assessed the influence of the lengths of homology regions of the repair template on HDR efficiency (Chu et al., 2015). A homologous template is the most important component of HDR-mediated genome editing. It usually contains intended mutations or insertions flanked by homologous regions. Templates can be plasmids (up to kilobases modification) or single-stranded oligodeoxynucleotides (ssODN) (50–100 nt modification). It is suggested that sequencing around the interested region should be carried out because cell-specific mutations and single nucleotide polymorphisms (SNPs) can influence gRNA targeting up to 6-fold, merely one mismatch in 100 bases (Tham et al., 2016). This problem can be overcome by amplifying the homology arm from the genomic DNA extracted from target cells or by synthesize consulting sequence analysis (Salsman and Dellaire, 2017). It is important to remember that DSB should always be as close as possible to the region of homology, within 10 nt up and to a maximum of 100 nt (Elliott et al., 1998). Furthermore, having each homology arm about 50–100% the size of the payload that can promote HDR (Li et al., 2014).
Enhancing HDR by Using Modified Cas9
As mentioned above, DSB ends must be resected so that they can enter the HDR pathway. CtIP, a key protein in early steps of DSB resection, is essential for HDR initiation. In order to ensure the presence of functional activated CtIP, Charpentier et al. fused a minimal N-terminal fragment of CtIP to the Cas9 protein (Charpentier et al., 2018). Forcing CtIP to the DNA cleavage site, through fusion to either catalytically dead Cas9 (dCas9) or Cas9 together with 800 bp homology arms, obtained a 2-fold increase in the efficiency of HDR in human fibroblast RG37DR cells, iPS cells, and rat zygotes. However, the expression of dCas9-CtIP is not sufficient by itself to stimulate integration. In addition, the patterns of indels induced by modified Cas9 were different from Cas9. This may be because the modified Cas9 induced a different balance of the end-joining pathway.
As for point mutation, current approaches to target base correction are inefficient and typically induce an abundance of random insertions and deletions at the target locus. Alexis et al. reported a powerful approach called a “base-editing (BE) system” to introduce specific point mutations without introducing DSB or a donor template by linking deaminases and dCas9 together (Komor et al., 2016). dCas9 helps deaminase to locate and deaminases modify cytidine to uridine. This conversion will then be repaired through various pathways (Hess et al., 2017). BE2 (optimized BE system) results from the addition of the uracil DNA glycosylase inhibitor, which increases the base-editing efficiency of the C>T substitution 3-fold (Hess et al., 2016). Additionally, the BE3 system was realized by changing the dCas9 to Cas9 D10A. This improvement achieved a 6-fold increase in efficiency over BE2 but exhibited a slightly increased indel frequency as nicks can lead to NHEJ at a low rate (Certo et al., 2011). Target-activation-induced cytidine deaminase (AID), a similar system, uses nickase Cas9 D10A to recruit the cytidine deaminase PmCDA1 to the target, achieving a mutation frequency of 35%. Adding UGI can obtain 2- to 3-fold increase in efficiency and reduction in deletions (Nishida et al., 2016). In addition, targeted AID-mediated mutagenesis (TAM) (Ma et al., 2016) and CRISPR-X (Hess et al., 2016) can generate transitions and transversions. These systems can both achieve the precision editing of single C>T bases with a low rate of indels as well as sequence diversification. By further Cas9 engineering, the inhibition of HR during the G1 phase will be overcome.
The CRISPR-Cas system has been fully studied and adapted for various applications over the decades, which gives us the ability to manipulate the genome as we desire. It has certain limitations, such as off-target effects (which can be overcame by rational sgRNA design; Doench et al., 2016) and low efficiency, which can be improved by utilizing the methodologies as described above. These promising strategies have proven their enhancement in the HDR pathway more than once with results varying from 2- to 30-fold. Combining various approaches can be a potential method of maximizing the rates of HDR. Here we only reviewed NHEJ inhibition by using inhibitors or hindering certain gene expression with siRNA or shRNA, CRISPR-Cas delivery in the G2/S phase, adding homologous arms in donor templets and using modified Cas9. These tactics surely make the CRISPR-Cas system more efficient. There is no doubt that more and more ways to boost CRISPR-Cas are imminent.
Author Contributions
ML, WM, and DC designed the structure of this review. ML wrote the first version of the manuscript. SR, XT, KG, and QF helped to revise the manuscript. All authors have reviewed the final version of the manuscript.
Funding
This research was supported by the Qinghai Province Major R&D and Transformation Project (2018-NK-125), Xianyang Science and Technology Major Project (2017K01-34), Key Industrial Innovation Chains of Shaanxi Province (2018ZDCXL-NY-01-06), and the PhD research startup fund of the Northwest Agriculture and Forestry University (00500/Z109021716).
Conflict of Interest Statement
The authors declare that the research was conducted in the absence of any commercial or financial relationships that could be construed as a potential conflict of interest.
References
Anders, C., Niewoehner, O., Duerst, A., and Jinek, M. (2014). Structural basis of PAM-dependent target DNA recognition by the Cas9 endonuclease. Nature 513, 569–573. doi: 10.1038/nature13579
Andres, S. N., Vergnes, A., Ristic, D., Wyman, C., Modesti, M., and Junop, M. (2012). A human XRCC4-XLF complex bridges DNA. Nucleic Acids Res. 40, 1868–1878. doi: 10.1093/nar/gks022
Aravind, L., and Koonin, E. V. (2001). Prokaryotic homologs of the eukaryotic DNA-end-binding protein Ku, novel domains in the Ku protein and prediction of a prokaryotic double-strand break repair system. Genome Res. 11, 1365–1374. doi: 10.1101/gr.181001
Bae, S., Kweon, J., Kim, H. S., and Kim, J. S. (2014). Microhomology-based choice of Cas9 nuclease target sites. Nat. Methods 11, 705–706. doi: 10.1038/nmeth.3015
Barrangou, R. (2013). CRISPR-Cas systems and RNA-guided interference. Wiley Interdiscip. Rev. RNA 4, 267–278. doi: 10.1002/wrna.1159
Barrangou, R. (2015). The roles of CRISPR-Cas systems in adaptive immunity and beyond. Curr. Opin. Immunol. 32, 36–41. doi: 10.1016/j.coi.2014.12.008
Barrangou, R., and Marraffini, L. A. (2014). CRISPR-Cas systems: prokaryotes upgrade to adaptive immunity. Mol. Cell 54, 234–244. doi: 10.1016/j.molcel.2014.03.011
Bunting, S. F., Callen, E., Wong, N., Chen, H. T., Polato, F., Gunn, A., et al. (2010). 53BP1 inhibits homologous recombination in Brca1-deficient cells by blocking resection of DNA breaks. Cell 141, 243–254. doi: 10.1016/j.cell.2010.03.012
Cannavo, E., and Cejka, P. (2014). Sae2 promotes dsDNA endonuclease activity within Mre11-Rad50-Xrs2 to resect DNA breaks. Nature 514, 122–125. doi: 10.1038/nature13771
Carroll, D., and Beumer, K. J. (2014). Genome engineering with TALENs and ZFNs: repair pathways and donor design. Methods 69, 137–141. doi: 10.1016/j.ymeth.2014.03.026
Castor, D., Nair, N., Declais, A. C., Lachaud, C., Toth, R., Macartney, T. J., et al. (2013). Cooperative control of holliday junction resolution and DNA repair by the SLX1 and MUS81-EME1 nucleases. Mol. Cell 52, 221–233. doi: 10.1016/j.molcel.2013.08.036
Certo, M. T., Ryu, B. Y., Annis, J. E., Garibov, M., Jarjour, J., Rawlings, D. J., et al. (2011). Tracking genome engineering outcome at individual DNA breakpoints. Nat. Methods 8, 671–676. doi: 10.1038/nmeth.1648
Charpentier, M., Khedher, A. H. Y., Menoret, S., Brion, A., Lamribet, K., Dardillac, E., et al. (2018). CtIP fusion to Cas9 enhances transgene integration by homology-dependent repair. Nat.Commun. 9:1133. doi: 10.1038/s41467-018-03475-7
Chen, R., and Wold, M. S. (2014). Replication protein a: single-stranded DNA's first responder: dynamic DNA-interactions allow replication protein A to direct single-strand DNA intermediates into different pathways for synthesis or repair. Bioessays 36, 1156–1161. doi: 10.1002/bies.201400107
Chu, V. T., Weber, T., Wefers, B., Wurst, W., Sander, S., Rajewsky, K., et al. (2015). Increasing the efficiency of homology-directed repair for CRISPR-Cas9-induced precise gene editing in mammalian cells. Nat. Biotechnol. 33, 543–548. doi: 10.1038/nbt.3198
Daley, J. M., Gaines, W. A., Kwon, Y., and Sung, P. (2014). Regulation of DNA pairing in homologous recombination. Cold Spring Harb. Perspect. Biol. 6:a017954. doi: 10.1101/cshperspect.a017954
Daley, J. M., Laan, R. L., Suresh, A., and Wilson, T. E. (2005). DNA joint dependence of pol X family polymerase action in nonhomologous end joining. J. Biol. Chem. 280, 29030–29037. doi: 10.1074/jbc.M505277200
Davis, A. J., Chen, B. P., and Chen, D. J. (2014). DNA-PK: a dynamic enzyme in a versatile DSB repair pathway. DNA Repair 7, 21–29. doi: 10.1016/j.dnarep.2014.02.020
de Jager, M., van Noort, J., van Gent, D. C., Dekker, C., Kanaar, R., and Wyman, C. (2001). Human Rad50/Mre11 is a flexible complex that can tether DNA ends. Mol. Cell 8, 1129–1135. doi: 10.1016/S1097-2765(01)00381-1
Deltcheva, E., Chylinski, K., Sharma, C. M., Gonzales, K., Chao, Y., Pirzada, Z. A., et al. (2011). CRISPR RNA maturation by trans-encoded small RNA and host factor RNase III. Nature 471, 602–607. doi: 10.1038/nature09886
Doench, J. G., Fusi, N., Sullender, M., Hegde, M., Vaimberg, E. W., Donovan, K. F., et al. (2016). Optimized sgRNA design to maximize activity and minimize off-target effects of CRISPR-Cas9. Nat. Biotechnol. 34, 184–191. doi: 10.1038/nbt.3437
Dow, L. E., Fisher, J., O'Rourke, K. P., Muley, A., Kastenhuber, E. R., Livshits, G., et al. (2015). Inducible in vivo genome editing with CRISPR-Cas9. Nat. Biotechnol. 33, 390–394. doi: 10.1038/nbt.3155
Dupre, A., Boyer-Chatenet, L., and Gautier, J. (2006). Two-step activation of ATM by DNA and the Mre11-Rad50-Nbs1 complex. Nat. Struct. Mol. Biol. 13, 451–457. doi: 10.1038/nsmb1090
Elliott, B., Richardson, C., Winderbaum, J., Nickoloff, J. A., and Jasin, M. (1998). Gene conversion tracts from double-strand break repair in mammalian cells. Mol. Cell Biol. 18, 93–101.
Escribano-Diaz, C., Orthwein, A., Fradet-Turcotte, A., Xing, M., Young, J. T., Tkac, J., et al. (2013). A cell cycle-dependent regulatory circuit composed of 53BP1-RIF1 and BRCA1-CtIP controls DNA repair pathway choice. Mol. Cell 49, 872–883. doi: 10.1016/j.molcel.2013.01.001
Falck, J., Coates, J., and Jackson, S. P. (2005). Conserved modes of recruitment of ATM, ATR and DNA-PKcs to sites of DNA damage. Nature 434, 605–611. doi: 10.1038/nature03442
Forrester, N. A., Sedgwick, G. G., Thomas, A., Blackford, A. N., Speiseder, T., Dobner, T., et al. (2011). Serotype-specific inactivation of the cellular DNA damage response during adenovirus infection. J. Virol. 85, 2201–2211. doi: 10.1128/JVI.01748-10
Frit, P., Barboule, N., Yuan, Y., Gomez, D., and Calsou, P. (2014). Alternative end-joining pathway(s): bricolage at DNA breaks. DNA Repair 17, 81–97. doi: 10.1016/j.dnarep.2014.02.007
Glover, J. N., Williams, R. S., and Lee, M. S. (2004). Interactions between BRCT repeats and phosphoproteins: tangled up in two. Trends Biochem. Sci. 29, 579–585. doi: 10.1016/j.tibs.2004.09.010
Gu, J., and Lieber, M. R. (2008). Mechanistic flexibility as a conserved theme across 3 billion years of nonhomologous DNA end-joining. Genes Dev. 22, 411–415. doi: 10.1101/gad.1646608
Hess, G. T., Fresard, L., Han, K., Lee, C. H., Li, A., Cimprich, K. A., et al. (2016). Directed evolution using dCas9-targeted somatic hypermutation in mammalian cells. Nat. Methods 13, 1036–1042. doi: 10.1038/nmeth.4038
Hess, G. T., Tycko, J., Yao, D., and Bassik, M. C. (2017). Methods and applications of CRISPR-mediated base editing in eukaryotic genomes. Mol. Cell 68, 26–43. doi: 10.1016/j.molcel.2017.09.029
Hilario, J., Amitani, I., Baskin, R. J., and Kowalczykowski, S. C. (2009). Direct imaging of human Rad51 nucleoprotein dynamics on individual DNA molecules. Proc. Natl. Acad. Sci. U.S.A. 106, 361–368. doi: 10.1073/pnas.0811965106
Hohl, M., Kwon, Y., Galvan, S. M., Xue, X., Tous, C., Aguilera, A., et al. (2011). The Rad50 coiled-coil domain is indispensable for Mre11 complex functions. Nat. Struct. Mol. Biol. 18, 1124–1131. doi: 10.1038/nsmb.2116
Horvath, P., and Barrangou, R. (2010). CRISPR/Cas, the immune system of bacteria and archaea. Science 327, 167–170. doi: 10.1126/science.1179555
Huertas, P., and Jackson, S. P. (2009). Human CtIP mediates cell cycle control of DNA end resection and double strand break repair. J. Biol. Chem. 284, 9558–9565. doi: 10.1074/jbc.M808906200
Irion, U., Krauss, J., and Nusslein-Volhard, C. (2014). Precise and efficient genome editing in zebrafish using the CRISPR/Cas9 system. Development 141, 4827–4830. doi: 10.1242/dev.115584
Jansen, R., Embden, J. D., Gaastra, W., and Schouls, L. M. (2002). Identification of genes that are associated with DNA repeats in prokaryotes. Mol. Microbiol. 43, 1565–1575. doi: 10.1046/j.1365-2958.2002.02839.x
Jasin, M., and Rothstein, R. (2013). Repair of strand breaks by homologous recombination. Cold Spring Harb. Perspect. Biol. 5:a012740. doi: 10.1101/cshperspect.a012740
Jinek, M., Chylinski, K., Fonfara, I., Hauer, M., Doudna, J. A., and Charpentier, E. (2012). A programmable dual-RNA-guided DNA endonuclease in adaptive bacterial immunity. Science 337, 816–821. doi: 10.1126/science.1225829
Jinek, M., Jiang, F., Taylor, D. W., Sternberg, S. H., Kaya, E., Ma, E., et al. (2014). Structures of Cas9 endonucleases reveal RNA-mediated conformational activation. Science 343:1247997. doi: 10.1126/science.1247997
Kang, J., Ferguson, D., Song, H., Bassing, C., Eckersdorff, M., Alt, F. W., et al. (2005). Functional interaction of H2AX, NBS1, and p53 in ATM-dependent DNA damage responses and tumor suppression. Mol. Cell Biol. 25, 661–670. doi: 10.1128/MCB.25.2.661-670.2005
Karow, J. K., Constantinou, A., Li, J. L., West, S. C., and Hickson, I. D. (2000). The Bloom's syndrome gene product promotes branch migration of holliday junctions. Proc. Natl. Acad. Sci. U.S.A. 97, 6504–6508. doi: 10.1073/pnas.100448097
Kim, H., Chen, J., and Yu, X. (2007). Ubiquitin-binding protein RAP80 mediates BRCA1-dependent DNA damage response. Science 316, 1202–1205. doi: 10.1126/science.1139621
Kim, K. P., and Mirkin, E. V. (2018). So similar yet so different: the two ends of a double strand break. Mutat. Res. 809, 70–80. doi: 10.1016/j.mrfmmm.2017.06.007
Komor, A. C., Kim, Y. B., Packer, M. S., Zuris, J. A., and Liu, D. R. (2016). Programmable editing of a target base in genomic DNA without double-stranded DNA cleavage. Nature 533, 420–424. doi: 10.1038/nature17946
Lavin, M. F. (2008). Ataxia-telangiectasia: from a rare disorder to a paradigm for cell signalling and cancer. Nat. Rev. Mol. Cell Biol. 9, 759–769. doi: 10.1038/nrm2514
Li, G., Liu, D., Zhang, X., Quan, R., Zhong, C., Mo, J., et al. (2018). Suppressing Ku70/Ku80 expression elevates homology-directed repair efficiency in primary fibroblasts. Int. J. Biochem. Cell Biol. 99, 154–160. doi: 10.1016/j.biocel.2018.04.011
Li, K., Wang, G., Andersen, T., Zhou, P., and Pu, W. T. (2014). Optimization of genome engineering approaches with the CRISPR/Cas9 system. PLoS ONE 9:e105779. doi: 10.1371/journal.pone.0105779
Lin, S., Staahl, B. T., Alla, R. K., and Doudna, J. A. (2014). Enhanced homology-directed human genome engineering by controlled timing of CRISPR/Cas9 delivery. Elife 3:e04766. doi: 10.7554/eLife.04766
Ma, C. J., Gibb, B., Kwon, Y., Sung, P., and Greene, E. C. (2017). Protein dynamics of human RPA and RAD51 on ssDNA during assembly and disassembly of the RAD51 filament. Nucleic Acids Res. 45, 749–761. doi: 10.1093/nar/gkw1125
Ma, Y., Zhang, J., Yin, W., Zhang, Z., Song, Y., and Chang, X. (2016). Targeted AID-mediated mutagenesis (TAM) enables efficient genomic diversification in mammalian cells. Nat. Methods 13, 1029–1035. doi: 10.1038/nmeth.4027
Makarova, K. S., Haft, D. H., Barrangou, R., Brouns, S. J., Charpentier, E., Horvath, P., et al. (2011). Evolution and classification of the CRISPR-Cas systems. Nat. Rev. Microbiol. 9, 467–477. doi: 10.1038/nrmicro2577
Makarova, K. S., Wolf, Y. I., Alkhnbashi, O. S., Costa, F., Shah, S. A., Saunders, S. J., et al. (2015). An updated evolutionary classification of CRISPR-Cas systems. Nat. Rev. Microbiol. 13, 722–736. doi: 10.1038/nrmicro3569
Makharashvili, N., and Paull, T. T. (2015). CtIP: A DNA damage response protein at the intersection of DNA metabolism. DNA Repair 32, 75–81. doi: 10.1016/j.dnarep.2015.04.016
Marraffini, L. A. (2015). CRISPR-Cas immunity in prokaryotes. Nature 526, 55–61. doi: 10.1038/nature15386
Marraffini, L. A., and Sontheimer, E. J. (2010). CRISPR interference: RNA-directed adaptive immunity in bacteria and archaea. Nat. Rev. Genet. 11, 181–190. doi: 10.1038/nrg2749
Maruyama, T., Dougan, S. K., Truttmann, M. C., Bilate, A. M., Ingram, J. R., and Ploegh, H. L. (2015). Increasing the efficiency of precise genome editing with CRISPR-Cas9 by inhibition of nonhomologous end joining. Nat. Biotechnol. 33, 538–542. doi: 10.1038/nbt.3190
Mathiasen, D. P., and Lisby, M. (2014). Cell cycle regulation of homologous recombination in Saccharomyces cerevisiae. FEMS Microbiol. Rev. 38, 172–184. doi: 10.1111/1574-6976.12066
Mayle, R., Campbell, I. M., Beck, C. R., Yu, Y., Wilson, M., Shaw, C. A., et al. (2015). DNA REPAIR. Mus81 and converging forks limit the mutagenicity of replication fork breakage. Science 349, 742–747. doi: 10.1126/science.aaa8391
Mazin, A. V., Alexeev, A. A., and Kowalczykowski, S. C. (2003). A novel function of Rad54 protein. stabilization of the Rad51 nucleoprotein filament. J. Biol. Chem. 278, 14029–14036. doi: 10.1074/jbc.M212779200
Mladenov, E., Magin, S., Soni, A., and Iliakis, G. (2016). DNA double-strand-break repair in higher eukaryotes and its role in genomic instability and cancer: cell cycle and proliferation-dependent regulation. Semin. Cancer Biol. 37–38, 51–64. doi: 10.1016/j.semcancer.2016.03.003
Mojica, F. J., Diez-Villasenor, C., Garcia-Martinez, J., and Almendros, C. (2009). Short motif sequences determine the targets of the prokaryotic CRISPR defence system. Microbiology 155, 733–740. doi: 10.1099/mic.0.023960-0
Mojica, F. J., and Rodriguez-Valera, F. (2016). The discovery of CRISPR in archaea and bacteria. FEBS J. 283, 3162–3169. doi: 10.1111/febs.13766
Morange, M. (2015). What history tells us XXXVII. CRISPR-Cas: the discovery of an immune system in prokaryotes. J. Biosci. 40, 221–223. doi: 10.1007/s12038-015-9532-6
Morrical, S. W. (2015). DNA-pairing and annealing processes in homologous recombination and homology-directed repair. Cold Spring Harb. Perspect. Biol. 7:a016444. doi: 10.1101/cshperspect.a016444
Morris, J. R., Boutell, C., Keppler, M., Densham, R., Weekes, D., Alamshah, A., et al. (2009). The SUMO modification pathway is involved in the BRCA1 response to genotoxic stress. Nature 462, 886–890. doi: 10.1038/nature08593
Moshous, D., Callebaut, I., de Chasseval, R., Corneo, B., Cavazzana-Calvo, M., Le Deist, F., et al. (2001). Artemis, a novel DNA double-strand break repair/V(D)J recombination protein, is mutated in human severe combined immune deficiency. Cell 105, 177–186. doi: 10.1016/S0092-8674(01)00309-9
Neal, J. A., Sugiman-Marangos, S., VanderVere-Carozza, P., Wagner, M., Turchi, J., Lees-Miller, S. P., et al. (2014). Unraveling the complexities of DNA-dependent protein kinase autophosphorylation. Mol. Cell Biol. 34, 2162–2175. doi: 10.1128/MCB.01554-13
Nishida, K., Arazoe, T., Yachie, N., Banno, S., Kakimoto, M., Tabata, M., et al. (2016). Targeted nucleotide editing using hybrid prokaryotic and vertebrate adaptive immune systems. Science 353:aaf8729. doi: 10.1126/science.aaf8729
Nishimasu, H., Ran, F. A., Hsu, P. D., Konermann, S., Shehata, S. I., Dohmae, N., et al. (2014). Crystal structure of Cas9 in complex with guide RNA and target DNA. Cell 156, 935–949. doi: 10.1016/j.cell.2014.02.001
Orthwein, A., Noordermeer, S. M., Wilson, M. D., Landry, S., Enchev, R. I., Sherker, A., et al. (2015). A mechanism for the suppression of homologous recombination in G1 cells. Nature 528, 422–426. doi: 10.1038/nature16142
Pardo, B., Gomez-Gonzalez, B., and Aguilera, A. (2009). DNA repair in mammalian cells: DNA double-strand break repair: how to fix a broken relationship. Cell Mol. Life Sci. 66, 1039–1056. doi: 10.1007/s00018-009-8740-3
Paull, T. T. (2005). Saving the ends for last: the role of pol mu in DNA end joining. Mol. Cell 19, 294–296. doi: 10.1016/j.molcel.2005.07.008
Paull, T. T. (2015). Mechanisms of ATM Activation. Annu. Rev. Biochem. 84, 711–738. doi: 10.1146/annurev-biochem-060614-034335
Pinder, J., Salsman, J., and Dellaire, G. (2015). Nuclear domain 'knock-in' screen for the evaluation and identification of small molecule enhancers of CRISPR-based genome editing. Nucleic Acids Res. 43, 9379–9392. doi: 10.1093/nar/gkv993
Prakash, R., Zhang, Y., Feng, W., and Jasin, M. (2015). Homologous recombination and human health: the roles of BRCA1, BRCA2, and associated proteins. Cold Spring Harb. Perspect. Biol. 7:a016600. doi: 10.1101/cshperspect.a016600
Radhakrishnan, S. K., Jette, N., and Lees-Miller, S. P. (2014). Non-homologous end joining: emerging themes and unanswered questions. DNA Repair 17, 2–8. doi: 10.1016/j.dnarep.2014.01.009
Rass, U., Compton, S. A., Matos, J., Singleton, M. R., Ip, S. C., Blanco, M. G., et al. (2010). Mechanism of Holliday junction resolution by the human GEN1 protein. Genes Dev. 24, 1559–1569. doi: 10.1101/gad.585310
Renaud, J. B., Boix, C., Charpentier, M., De Cian, A., Cochennec, J., Duvernois-Berthet, E., et al. (2016). Improved genome editing efficiency and flexibility using modified oligonucleotides with TALEN and CRISPR-Cas9 Nucleases. Cell Rep. 14, 2263–2272. doi: 10.1016/j.celrep.2016.02.018.
Richardson, C. D., Kazane, K. R., Feng, S. J., Zelin, E., Bray, N. L., Schafer, A. J., et al. (2018). CRISPR-Cas9 genome editing in human cells occurs via the Fanconi anemia pathway. Nat. Genet. 50, 1132–1139. doi: 10.1038/s41588-018-0174-0
Rogakou, E. P., Pilch, D. R., Orr, A. H., Ivanova, V. S., and Bonner, W. M. (1998). DNA double-stranded breaks induce histone H2AX phosphorylation on serine 139. J. Biol. Chem. 273, 5858–5868.
Roth, D. B., Lindahl, T., and Gellert, M. (1995). Repair and recombination. how to make ends meet. Curr. Biol. 5, 496–499.
Salsman, J., and Dellaire, G. (2017). Precision genome editing in the CRISPR era. Biochem. Cell Biol. 95, 187–201. doi: 10.1139/bcb-2016-0137
San Filippo, J., Sung, P., and Klein, H. (2008). Mechanism of eukaryotic homologous recombination. Annu. Rev. Biochem. 77, 229–257. doi: 10.1146/annurev.biochem.77.061306.125255
Sapranauskas, R., Gasiunas, G., Fremaux, C., Barrangou, R., Horvath, P., and Siksnys, V. (2011). The Streptococcus thermophilus CRISPR/Cas system provides immunity in Escherichia coli. Nucleic Acids Res. 39, 9275–9282. doi: 10.1093/nar/gkr606
Sartori, A. A., Lukas, C., Coates, J., Mistrik, M., Fu, S., Bartek, J., et al. (2007). Human CtIP promotes DNA end resection. Nature 450, 509–514. doi: 10.1038/nature06337
Shiloh, Y. (2003). ATM and related protein kinases: safeguarding genome integrity. Nat. Rev. Cancer 3, 155–168. doi: 10.1038/nrc1011
Sorek, R., Lawrence, C. M., and Wiedenheft, B. (2013). CRISPR-mediated adaptive immune systems in bacteria and archaea. Annu. Rev. Biochem. 82, 237–266. doi: 10.1146/annurev-biochem-072911-172315
Soutoglou, E., Dorn, J. F., Sengupta, K., Jasin, M., Nussenzweig, A., Ried, T., et al. (2007). Positional stability of single double-strand breaks in mammalian cells. Nat. Cell Biol. 9, 675–682. doi: 10.1038/ncb1591
Spycher, C., Miller, E. S., Townsend, K., Pavic, L., Morrice, N. A., Janscak, P., et al. (2008). Constitutive phosphorylation of MDC1 physically links the MRE11-RAD50-NBS1 complex to damaged chromatin. J. Cell Biol. 181, 227–240. doi: 10.1083/jcb.200709008
Srivastava, M., Nambiar, M., Sharma, S., Karki, S. S., Goldsmith, G., Hegde, M., et al. (2012). An inhibitor of nonhomologous end-joining abrogates double-strand break repair and impedes cancer progression. Cell 151, 1474–1487. doi: 10.1016/j.cell.2012.11.054
Sternberg, S. H., Redding, S., Jinek, M., Greene, E. C., and Doudna, J. A. (2014). DNA interrogation by the CRISPR RNA-guided endonuclease Cas9. Nature 507, 62–67. doi: 10.1038/nature13011
Stewart, G. S. (2009). Solving the RIDDLE of 53BP1 recruitment to sites of damage. Cell Cycle 8, 1532–1538. doi: 10.4161/cc.8.10.8351
Stucki, M., and Jackson, S. P. (2006). gammaH2AX and MDC1: anchoring the DNA-damage-response machinery to broken chromosomes. DNA Repair 5, 534–543. doi: 10.1016/j.dnarep.2006.01.012
Sy, S. M., Huen, M. S., and Chen, J. (2009). PALB2 is an integral component of the BRCA complex required for homologous recombination repair. Proc. Natl. Acad. Sci. U.S.A. 106, 7155–7160. doi: 10.1073/pnas.0811159106
Symington, L. S. (2014). End resection at double-strand breaks: mechanism and regulation. Cold Spring Harb. Perspect. Biol. 6:a016436. doi: 10.1101/cshperspect.a016436
Symington, L. S., and Gautier, J. (2011). Double-strand break end resection and repair pathway choice. Annu. Rev. Genet. 45, 247–271. doi: 10.1146/annurev-genet-110410-132435
Szczelkun, M. D., Tikhomirova, M. S., Sinkunas, T., Gasiunas, G., Karvelis, T., Pschera, P., et al. (2014). Direct observation of R-loop formation by single RNA-guided Cas9 and Cascade effector complexes. Proc. Natl. Acad. Sci. U.S.A. 111, 9798–9803. doi: 10.1073/pnas.1402597111
Tham, K. C., Kanaar, R., and Lebbink, J. H. (2016). Mismatch repair and homeologous recombination. DNA Repair 38, 75–83. doi: 10.1016/j.dnarep.2015.11.010
Tsai, C. J., Kim, S. A., and Chu, G. (2007). Cernunnos/XLF promotes the ligation of mismatched and noncohesive DNA ends. Proc. Natl. Acad. Sci. U.S.A. 104, 7851–7856. doi: 10.1073/pnas.0702620104
van der Heijden, T., Seidel, R., Modesti, M., Kanaar, R., Wyman, C., and Dekker, C. (2007). Real-time assembly and disassembly of human RAD51 filaments on individual DNA molecules. Nucleic Acids Res. 35, 5646–5657. doi: 10.1093/nar/gkm629
van der Oost, J., Jore, M. M., Westra, E. R., Lundgren, M., and Brouns, S. J. (2009). CRISPR-based adaptive and heritable immunity in prokaryotes. Trends Biochem. Sci. 34, 401–407. doi: 10.1016/j.tibs.2009.05.002
Vartak, S. V., and Raghavan, S. C. (2015). Inhibition of nonhomologous end joining to increase the specificity of CRISPR/Cas9 genome editing. FEBS J. 282, 4289–4294. doi: 10.1111/febs.13416
Walker, J. R., Corpina, R. A., and Goldberg, J. (2001). Structure of the Ku heterodimer bound to DNA and its implications for double-strand break repair. Nature 412, 607–614. doi: 10.1038/35088000
Wang, B., Matsuoka, S., Carpenter, P. B., and Elledge, S. J. (2002). 53BP1, a mediator of the DNA damage checkpoint. Science 298, 1435–1438. doi: 10.1126/science.1076182
Waters, C. A., Strande, N. T., Wyatt, D. W., Pryor, J. M., and Ramsden, D. A. (2014). Nonhomologous end joining: a good solution for bad ends. DNA Repair 17, 39–51. doi: 10.1016/j.dnarep.2014.02.008
West, S. C., Blanco, M. G., Chan, Y. W., Matos, J., Sarbajna, S., and Wyatt, H. D. (2015). Resolution of recombination intermediates: mechanisms and regulation. Cold Spring Harb. Symp. Q. Biol. 80, 103–109. doi: 10.1101/sqb.2015.80.027649
Weterings, E., Verkaik, N. S., Bruggenwirth, H. T., Hoeijmakers, J. H., and van Gent, D. C. (2003). The role of DNA dependent protein kinase in synapsis of DNA ends. Nucleic Acids Res. 31, 7238–7246. doi: 10.1093/nar/gkg889
Wiedenheft, B., Sternberg, S. H., and Doudna, J. A. (2012). RNA-guided genetic silencing systems in bacteria and archaea. Nature 482, 331–338. doi: 10.1038/nature10886
Williams, G. J., Lees-Miller, S. P., and Tainer, J. A. (2010). Mre11-Rad50-Nbs1 conformations and the control of sensing, signaling, and effector responses at DNA double-strand breaks. DNA Repair 9, 1299–1306. doi: 10.1016/j.dnarep.2010.10.001
Williams, R. S., Dodson, G. E., Limbo, O., Yamada, Y., Williams, J. S., Guenther, G., et al. (2009). Nbs1 flexibly tethers Ctp1 and Mre11-Rad50 to coordinate DNA double-strand break processing and repair. Cell 139, 87–99. doi: 10.1016/j.cell.2009.07.033
Williams, R. S., Moncalian, G., Williams, J. S., Yamada, Y., Limbo, O., Shin, D. S., et al. (2008). Mre11 dimers coordinate DNA end bridging and nuclease processing in double-strand-break repair. Cell 135, 97–109. doi: 10.1016/j.cell.2008.08.017
Wu, L., and Hickson, I. D. (2003). The Bloom's syndrome helicase suppresses crossing over during homologous recombination. Nature 426, 870–874. doi: 10.1038/nature02253
Wyatt, H. D., and West, S. C. (2014). Holliday junction resolvases. Cold Spring Harb. Perspect. Biol. 6:a023192. doi: 10.1101/cshperspect.a023192
Yang, D., Scavuzzo, M. A., Chmielowiec, J., Sharp, R., Bajic, A., and Borowiak, M. (2016). Enrichment of G2/M cell cycle phase in human pluripotent stem cells enhances HDR-mediated gene repair with customizable endonucleases. Sci. Rep. 6:21264. doi: 10.1038/srep21264
Yang, H., Wang, H., Shivalila, C. S., Cheng, A. W., Shi, L., and Jaenisch, R. (2013). One-step generation of mice carrying reporter and conditional alleles by CRISPR/Cas-mediated genome engineering. Cell 154, 1370–1379. doi: 10.1016/j.cell.2013.08.022
Yu, C., Liu, Y., Ma, T., Liu, K., Xu, S., Zhang, Y., et al. (2015). Small molecules enhance CRISPR genome editing in pluripotent stem cells. Cell Stem Cell 16, 142–147. doi: 10.1016/j.stem.2015.01.003
Yu, S., Song, Z., Luo, J., Dai, Y., and Li, N. (2011). Over-expression of RAD51 or RAD54 but not RAD51/4 enhances extra-chromosomal homologous recombination in the human sarcoma (HT-1080) cell line. J. Biotechnol. 154, 21–24. doi: 10.1016/j.jbiotec.2011.03.023
Zelensky, A., Kanaar, R., and Wyman, C. (2014). Mediators of homologous DNA pairing. Cold Spring Harb. Perspect. Biol. 6:a016451. doi: 10.1101/cshperspect.a016451
Keywords: CRISPR-Cas9, HDR, NHEJ, HDR enhancement, DSB, cell arrest, NHEJ inhibitors
Citation: Liu M, Rehman S, Tang X, Gu K, Fan Q, Chen D and Ma W (2019) Methodologies for Improving HDR Efficiency. Front. Genet. 9:691. doi: 10.3389/fgene.2018.00691
Received: 31 August 2018; Accepted: 11 December 2018;
Published: 07 January 2019.
Edited by:
David Jay Segal, University of California, Davis, United StatesReviewed by:
James Carney, Sandia National Laboratories (SNL), United StatesAlex Michael Ward, Sangamo BioSciences, United States
Copyright © 2019 Liu, Rehman, Tang, Gu, Fan, Chen and Ma. This is an open-access article distributed under the terms of the Creative Commons Attribution License (CC BY). The use, distribution or reproduction in other forums is permitted, provided the original author(s) and the copyright owner(s) are credited and that the original publication in this journal is cited, in accordance with accepted academic practice. No use, distribution or reproduction is permitted which does not comply with these terms.
*Correspondence: Wentao Ma, bWF3ZW50YW9AbndzdWFmLmVkdS5jbg==
Dekun Chen, Y2RrQG53c3VhZi5lZHUuY24=