- 1UMR 6290, Centre National de la Recherche Scientifique, Rennes, France
- 2Institute of Genetics and Development of Rennes (IGDR), Université de Rennes 1, Rennes, France
- 3Efevre Tech Ltd., Larnaca, Cyprus
- 4Department of Biological Sciences, University of Cyprus, Nicosia, Cyprus
Histone modifications are key epigenetic regulators that control chromatin structure and gene transcription, thereby impacting on various important cellular phenotypes. Over the past decade, a growing number of studies have indicated that changes in various histone modifications have a significant influence on the aging process. Furthermore, it has been revealed that the abundance and localization of histone modifications are responsive to various environmental stimuli, such as diet, which can also affect gene expression and lifespan. This supports the notion that histone modifications can serve as a main cellular platform for signal integration. Hence, in this review we focus on the role of histone modifications during aging, report the data indicating that diet affects histone modification levels and explore the idea that histone modifications may function as an intersection through which diet regulates lifespan. A greater understanding of the epigenetic mechanisms that link environmental signals to longevity may provide new strategies for therapeutic intervention in age-related diseases and for promoting healthy aging.
Introduction
Biological aging or senescence is a multidimensional process that is manifested by degradation of biological function over time, which in the meantime confers susceptibility to different diseases (Cătană et al., 2018). A plethora of factors, both environmental and intrinsic, can affect aging. It is well accepted that a healthy diet and lifestyle exerts a positive effect on aging (Liang et al., 2018), while exposure to multiple stresses can have the opposite outcome (Amdam, 2011). Dietary choices during every stage of life, from embryo to old age, can significantly impact our growth and health (Upadhyaya et al., 2017). Many nutrients of food and derivatives produced during food digestion and metabolism have been well described for their roles in building and sustaining healthy organisms. On the contrary, severe food deprivation in developing countries or excess fat intake in the developed westernized world are known to negatively affect health (Nowacka-Woszuk et al., 2018; Uchiyama et al., 2018). Those diets lead to disorders such as cardiovascular diseases and diabetes, which dramatically affect people’s lifespan and well-being. The effect of diet on the human body was traditionally observed by changes in physiology at the organ level (e.g., fatty liver, atheromatic plaques, heart failures) or at the organismal level (obesity). However, for a long time it was unclear how diet affects the cells at the molecular level and, in turn, how these changes impact on lifespan.
The molecular mechanisms underlying the aging process have been the focus of various studies in the last few decades. Recently, nine tentative genetic and biochemical hallmarks of aging have been established: genomic instability, telomere attrition, altered intercellular communication, loss of proteostasis, deregulated nutrient sensing, mitochondrial dysfunction, cellular senescence, stem cell exhaustion, and epigenetic alterations (López-Otín et al., 2013). Among those, the epigenetic alteration hallmark has been attracting much interest over the last years for two main reasons: (1) there is increasing evidence linking epigenetic changes to age-related disorders, and (2) the potential reversibility of epigenetic marks makes them promising therapeutic targets to alleviate age-related pathologies (Milagro et al., 2013).
Cells respond to different nutrients through various signaling pathways, which eventually converge onto chromatin in order to regulate the expression of multiple genes involved in the specific cellular response. During aging, changes in gene expression have been consistently reported (de Magalhães et al., 2009; Hu et al., 2014; Rangaraju et al., 2015), and these changes can be deleterious or protective depending on the targeted gene and how its expression is regulated. However, although the signaling pathways that allow the cells to respond to specific environmental inputs are well-studied, substantial knowledge regarding the integration of the different signals at the chromatin level is still lacking. The basic unit of chromatin is the nucleosome, a dynamic structure consisting of 147 base pairs of DNA wrapped around an octamer of histone proteins. This octamer comprises two copies of each of the core histones: H2A, H2B, H3 and H4. Histones can be decorated with various post-translational modifications (PTMs), including acetylation, methylation, phosphorylation, and ubiquitination, and these PTMs are deposited and removed by specialized histone modifying enzymes (Bannister and Kouzarides, 2011). Due to this characteristic, epigenetic modifications are reversible and thus able to dynamically modulate chromatin structure in order to activate or silence gene expression. Furthermore, histones usually possess multiple PTMs, which can communicate among themselves to influence the presence of each other. These communications are known as cross-talks, and happen in a context-dependent manner, orchestrating a more complex transcriptional output than each PTM in isolation (Murr, 2010; Molina-Serrano et al., 2013).
Several studies in various organisms demonstrated that environmental inputs could influence histone PTM deposition, thereby modulating chromatin structure and gene expression. For this reason, histone modifications are an excellent interface through which extracellular signals, like dietary manipulations or stress, may affect cellular phenotypes (Badeaux and Shi, 2013; Gut and Verdin, 2013). The effect of epigenetic changes on the aging phenotype has been studied extensively in the last decades. Hence, in this review, we summarize the knowledge acquired regarding the role of histone PTMs in regulating aging, and present the evidence linking dietary interventions to PTMs, some of which are then directly affecting lifespan (Figure 1).
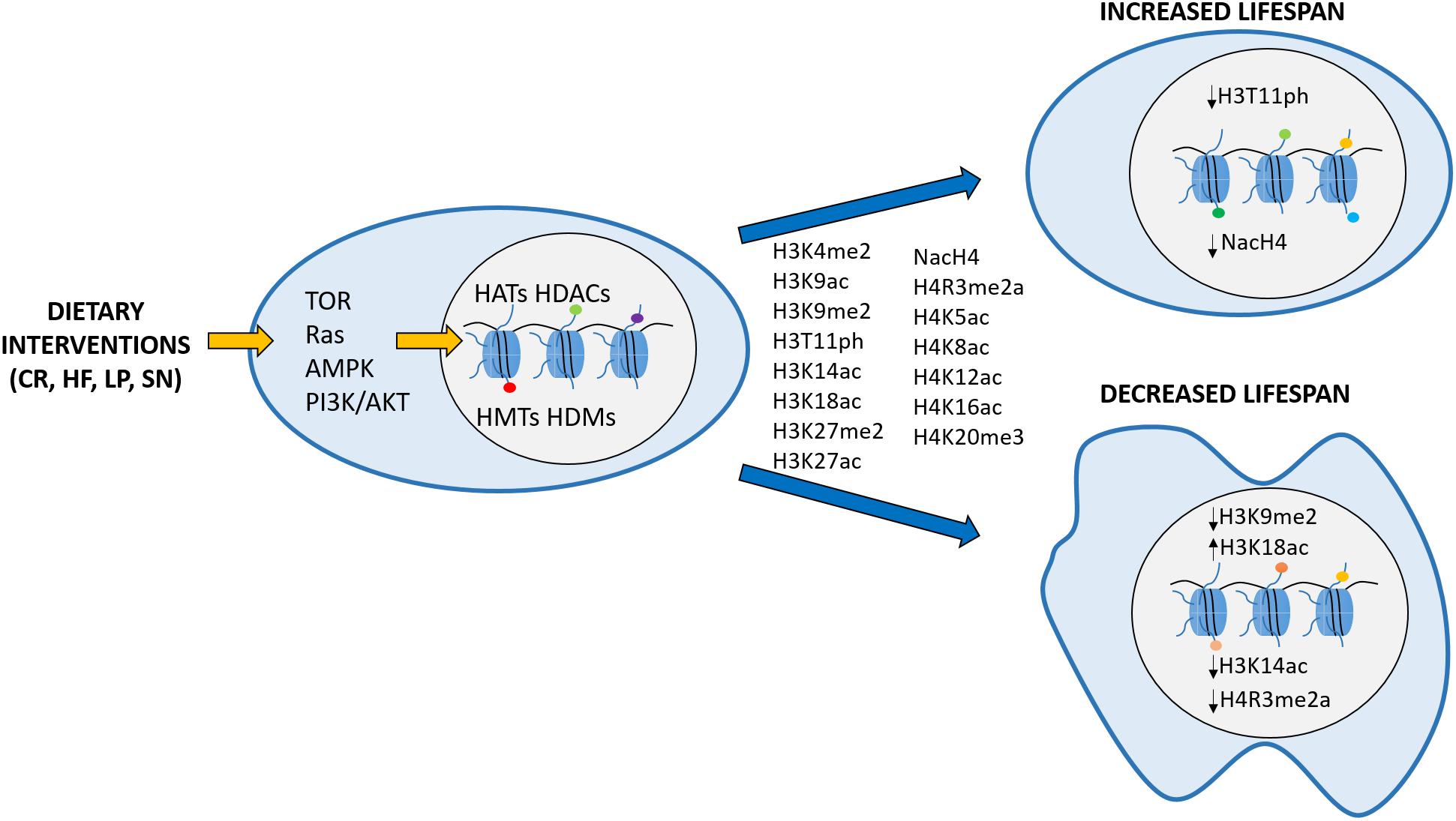
Figure 1. Schematic representation depicting the hypothesis that histone modifications act as an intermediate between diet and longevity. Calorie restriction (CR), high fat (HF), low protein (LP), single nutrient (SN) conditions are sensed by the cell through signaling pathways like TOR, Ras, AMPK or PI3K/AKT, promoting changes on the epigenome. Examples of histone modifications that are affected by dietary interventions are indicated between the two blue arrows. Specific changes in some of those modifications (H3K9me2, H3K18ac, NacH4, H3T11ph, H3K14ac, and H4R3me2a) have been consistently linked to a particular lifespan effect and are shown within the respective cell nucleus. Arrows next to each modification point to increase (↑) or decrease (↓) in the corresponding modification levels.
Histone Levels and PTMs are Linked to Lifespan Regulation
Histones and many of their PTMs undergo changes in their levels during aging, affecting different genomic regions in various tissues. This often complicates the interpretation on their specific effects on lifespan. There are three possible scenarios on how histones and their PTMs could affect lifespan: they can cause global changes in the transcriptome, alter the expression of key longevity genes or utilize both of these mechanisms (Cheung et al., 2010; Han et al., 2012; Sun et al., 2014; Ashapkin et al., 2017). Global histone loss during aging and senescence has been repeatedly described from yeast to humans (Feser et al., 2010; Tsurumi and Li, 2012; Hu et al., 2014). The implication of histone loss in aging was initially supported by the observation that in yeast, histone overexpression extends lifespan (Feser et al., 2010). Together with the drop of nucleosome occupancy (up to 50% in Saccharomyces cerevisiae) derived from the histone loss during aging, there is a marked redistribution of the remaining nucleosomes (Hu et al., 2014). This generates a general upregulation of many genes and an increase in genome instability that are both possible causes for cellular aging. Consistently, studies in Drosophila melanogaster have shown that decreased heterochromatin levels and thus reduction of nucleosomes are directly related to aging, whilst an increase in heterochromatin promotes longevity. This phenotype is associated with nucleosomal changes at the ribosomal DNA locus, thus affecting rRNA synthesis (Larson et al., 2012). Despite the important role of histone levels in aging, a large body of work in aging research has focused on histone PTMs and the enzymes that mediate them, as it was observed that manipulation of their levels can trigger substantial changes in lifespan. During the last decade, an increasing number of histone residues and PTMs have been linked to aging in various eukaryotic organisms (Table 1). However, this task has shown to be challenging due to the difficulty to isolate lifespan phenotypes from the plethora of cellular mechanisms affected by the experimental dietary interventions. For this reason, the baker’s yeast S. cerevisiae has served as an excellent model to test the role of histone residues in lifespan, as it allows for easy genetic manipulation, therefore obtaining causal links between histone point mutations and longevity. Following this concept, systematic, high-throughput lifespan studies in yeast using the H3/H4 histone mutant library showed that many histone residues, which can also bear modifications, could potentially affect lifespan, although still more in-depth studies are needed to better understand the aging effects of the associated PTMs (Sen et al., 2015). The recent establishment of a similar H2A/H2B histone mutant library could expand the number of histone residues that are associated with lifespan regulation, as these histones remain currently less studied (Jiang et al., 2017).
Histone H3 PTMs Related to Lifespan
H3K4
Lysine 4 on histone H3 can be found in one of three possible methylated forms: mono, di or trimethylated (H3K4me1, -2, and -3).The trimethylated form usually localizes at the promoter of actively transcribed genes (Santos-Rosa et al., 2002), and has been shown to have a strong implication with aging in several model organisms. In S. cerevisiae, deletion of different components or catalytic inactivation of the H3K4 methyltransferase complex COMPASS reduces significantly replicative lifespan (Smith et al., 2008; Ryu et al., 2014; Cruz et al., 2018). This effect is due to the impaired expression of several genes that are normally induced during aging. In Caenorhabditis elegans however, RNAi knockdown of several components of the H3K4me3 methyltransferase ASH-2 complex extend lifespan (Greer et al., 2010). This lifespan extension is dependent on the presence of H3K4me3 demethylase RBR-2, as deletion of RBR-2 abrogates the lifespan extension effect generated by the ASH-2 knockdown. Greer et al. (2010) also found that overexpression of RBR-2 extends lifespan, strongly suggesting that these two opposing chromatin modifiers must operate in the same lifespan-related pathway. However, results on the effect of RBR-2 are contradictory, as different reports have shown that mutation or RNAi-mediated knockdown of this enzyme both increases (Lee, 2003; Ni et al., 2012; Alvares et al., 2014) and decreases C. elegans lifespan (Greer et al., 2010; Maures et al., 2011). In D. melanogaster, RNAi depletion or mutation of Lid, the RBR-2 ortholog, causes an increase in the H3K4me3 levels and a reduction in male fly lifespan. Interestingly, under the same manipulation lifespan of female flies remains unperturbed (Li et al., 2010). Another H3K4 demethylase, LSD-1, has also been associated with lifespan. LSD-1 targets mono and dimethylated (H3K4me1 and H3K4me2, respectively) residues, and its depletion in C. elegans has been reported to extend lifespan compared to WT (McColl et al., 2008; Maures et al., 2011).
H3K9
Histone H3 lysine 9 can be acetylated (H3K9ac) and methylated (H3K9me), and both modifications have been connected to aging. While acetylation of this residue is usually found in active chromatin (Karmodiya et al., 2012), di and trimethylation (H3K9me2 and H3K9me3, respectively) usually mark constitutive heterochromatin and inactive euchromatin (Peters et al., 2001; Rougeulle et al., 2004). H3K9ac has been shown to increase during aging in D. melanogaster (Peleg et al., 2016), but decrease with age in rat liver cells (Kawakami et al., 2009). In mice this mark is deacetylated by SIRT6, a sirtuin that controls the expression of multiple glycolytic genes and triggers age-associated degenerative processes when is depleted (Mostoslavsky et al., 2006; Kawahara et al., 2009; Zhong et al., 2010). In line with this evidence, SIRT6 overexpression increased male longevity in transgenic mice, regulating specific genes in a similar way as when mice follow a CR diet (Kanfi et al., 2012). On the other hand, H3K9me3 and H3K9me2 have been shown to decrease during aging in fibroblast cells, C. elegans and D. melanogaster (Scaffidi and Misteli, 2006; Larson et al., 2012; Ni et al., 2012). Another report in D. melanogaster showed a general increase in H3K9me3 due to a more widespread distribution of this mark in the genome, including euchromatic regions (Wood et al., 2010). More recently, Djeghloul et al. (2016) have shown that the ability of hematopoietic stem cells to generate B lymphocytes is dependent on the H3K9 methyltransferase SUV39H1. The levels of this enzyme and H3K9me3 decrease with age in these cells, contributing to the aging-related decrease in immune function and this effect has been associated with changes in heterochromatin regulation. Accordingly, overexpressing SUV39H1 in aging cells improves the generation of B lymphocytes (Djeghloul et al., 2016).However, in progeroid mouse cells depletion of SUV39H1 reduces H3K9me3 levels and this delays aging due to restored DNA repair capacity. Remarkably, when SUV39H1 is deleted in combination with the metalloprotease ZMPSTE24, those mice have a 60% increase in lifespan (Liu et al., 2013).
H3T11
Histone H3 threonine 11 can be phosphorylated (H3T11ph) by different protein kinases and plays a role in several cellular functions, including meiosis (Govin et al., 2010), DNA replication checkpoint (Shimada et al., 2008), and nutritional stress (Oh et al., 2018). The levels of this modification are regulated by the Sch9 signaling pathway in response to glucose levels in food. H3T11 then regulates the expression of several genes implicated in the nutritional response. Interestingly, phosphorylation of this residue is implicated in S. cerevisiae aging, as mutating H3T11 to alanine clearly extends yeast chronological lifespan (Oh et al., 2018). Importantly, deletion of CKA1, the catalytic unit of the CK2 Serine/Threonine kinase complex that targets H3T11 shows the same effect. H3T11A and cka1Δ phenotypes seem to be caused ultimately by an increased tolerance to media acidification. In normal conditions, glucose depletion increases the levels of acetic acid, promoting phosphorylation of H3T11. This mechanism is obviously not functional in the mutants, changing the transcriptional output of the cell in a way that causes cellular longevity. The mechanism by which this histone phosphorylation affects transcription is, however, still elusive(Oh et al., 2018).
H3K14
In S. cerevisiae, H3K14 acetylation has been described to have a role in rDNA silencing and replication-dependent nucleosome assembly (Xu et al., 2016). Mutation of this residue markedly reduced yeast replicative lifespan, a phenotype enhanced when other residues in the histone H3 tail, such as K9, K18, and K23, that are acetylated are also mutated in combination with H3K14 (Xu et al., 2016).
H3K18
In mammals, acetylation at H3K18 (H3K18ac), a mark enriched at gene promoters and associated with active sites (Wang et al., 2008), is also involved in aging. H3K18ac is frequently found at pericentric chromatin, where is removed by SIRT6 (Kanfi et al., 2012). H3K18ac removal allows silencing of pericentric heterochromatin preventing mitotic errors and cellular aging. On the contrary, absence of SIRT6 increases H3K18ac thus, stimulating aberrant accumulation of pericentric transcripts, triggering acute aging (Tasselli et al., 2016).
H3K27
H3K27 can be found in one of three possible methylated forms: mono, di or trimethylated (H3K27me1, 2 and 3), and its trimethylated form is strongly associated with heterochromatin (Pan et al., 2018). In C. elegans, H3K27me3 levels have been observed to decrease with aging (Jin et al., 2011; Ni et al., 2012), correlating with the increasing levels of the H3K27me3 demethylase UTX-1 (Jin et al., 2011). Accordingly, knockdown of UTX-1 extends worm lifespan (Maures et al., 2011). A similar effect has been observed in mammalian cells, where UTX-1 levels increase with aging in parallel to a decrease in H3K27me3 (Jin et al., 2011). Other reports using human TIG3 cell cultures showed a decrease in H3K27me3 and its associated methyltransferase PRC2 complex in senescent cells(Bracken et al., 2007). In mouse brain H3K27me3 also decreases with age (Gong et al., 2015). However, another article also reported both upregulation and downregulation of H3K27me3 at the promoter of different genes in aged murine hematopoietic stem cells (Sun et al., 2014). In flies and killifish, however, H3K27me3 seems to affect lifespan differently from mammals and C. elegans. In killifish, PRC2 and H3K27me3 levels increase during aging (Baumgart et al., 2014), whereas heterozygous mutations in the catalytic subunit of the PRC2 complex reduced H3K27me3 levels and increased lifespan in D. melanogaster (Siebold et al., 2010). Consistent with this evidence, mutations in the Polycomb-antagonist complex TRX suppressed the lifespan extension of the PRC2 mutant while increasing H3K27me3 levels (Siebold et al., 2010). TRX is a complex that promotes H3K27 acetylation (H3K27ac), a modification that counteracts H3K27 methylation, further supporting the implication of this histone residue in the regulation of lifespan. Why H3K27 methylation states have a different effect on aging in the various organisms studied remains quite puzzling. This discrepancy could be a consequence of the regulation of distinct sets of longevity genes in the studied organisms, a difference in the conditions used during each of these studies or a combination of the two reasons as well as other yet unknown factors.
H3S28
Histone H3 serine 28 can be found in a phosphorylated form (H3S28ph), and this PTM cross-talks with H3K27 PTMs to respond to stress signaling by displacing the polycomb complex from its target genes (Gehani et al., 2010). Interestingly, Joos et al. (2018) have shown in a recent report that flies expressing ectopically a H3S28A mutant have increased longevity and improved stress resistance through the regulation of well-established longevity genes.
H3K36
H3K36 can also be found in three possible methylated forms but only its trimethylated state has been strongly associated with aging. H3K36me3 localizes at gene bodies, and it is associated with active transcription (Bannister et al., 2005). In S. cerevisiae H3K36me3 levels decrease during aging, triggering cryptic transcription (Sen et al., 2015); a pattern also observed in D. melanogaster and C. elegans (Wood et al., 2010; Pu et al., 2015). H3 lysine 36 substitutions performed in S. cerevisiae showed decreased lifespan and this effect was consistently observed in the absence of the H3K36 methyltransferase Set2 (Ryu et al., 2014; Sen et al., 2015). Interestingly, deletion of the H3K36 demethylase Rph1 had the opposite effect (Sen et al., 2015). Set2 is required for proper function of the TOR pathway and thus implicated in regulating the transcriptional response to nutrient stress (McDaniel et al., 2017). In agreement with this, depletion or inactivation of the Set2-ortholog MET-1 in C. elegans shortened lifespan (Pu et al., 2015), while depletion of the demethylase JMJD-2 enhanced longevity (Ni et al., 2012). More recently, deletion of the H3K36 dimethyltransferase SET-18 in C. elegans has been shown to extend lifespan through changes in the expression of DAF-16, a forkhead transcription factor that mediates the insulin/IGF conserved pathway(Su et al., 2018). Intriguingly, expression of SET-18 is upregulated in muscle cells of old worms, showing concomitant decrease in daf-16 expression.
H3K56
H3K56 is a residue involved in nucleosome assembly, genomic stability and transcription, and can be found both in acetylated and methylated forms (Das et al., 2009; Yuan et al., 2009; Jack et al., 2013). In S. cerevisiae H3K56 acetylation levels decrease with age, and mutating the residue greatly reduces lifespan (Dang et al., 2009; Feser et al., 2010; Sen et al., 2015). Furthermore, deletion of Rtt109, the main acetyltransferase targeting this residue, shortens yeast lifespan, an effect also observed when deleting the H3K56-associated deacetylases Hst3 and Hst4 (Feser et al., 2010). The fact that both loss and increase of this acetylation has the same effect on lifespan possibly reflects the implication of this residue and of its modification in various molecular mechanisms.
H3K79
H3K79, just like other lysine residues, can exist in one of three possible methylated forms: mono, di or trimethylated, which are involved in many cellular pathways, including transcription regulation, telomeric silencing, cell-cycle checkpoint, DNA repair, and cellular development (Farooq et al., 2016). While H3K79me3 has been shown to increase with age in yeast (Rhie et al., 2013), mutating the lysine residue into glutamic acid (H3K79E) has an evident reduction in replicative lifespan (Sen et al., 2015). Deletion of Dot1, the H3K79 methyltransferase also reduces lifespan (Ryu et al., 2014). In contrary to what has been observed in yeast, in aged mouse brain this modification is reduced (Gong et al., 2015).
Histone H4 PTMs Associated With Lifespan
NacH4
N-terminal acetylation is present in all core histones, but is more abundant on histones H4 and H2A, catalyzed by the N-terminal acetyltransferase Nat4 (aka NatD, Naa40). This histone acetyltransferase has high substrate selectivity and its enzymatic activity is conserved from yeast to human (Ree et al., 2018). This modification crosstalks with the adjacent arginine 3 asymmetric dimethylation mark (H4R3me2a) to regulate ribosomal RNA expression in response to glucose levels suggesting that Nat4 and NacH4 act as a sensor for cell growth (Schiza et al., 2013). Mutating H4R3 into lysine (H4R3K) severely reduces yeast replicative lifespan, while loss of NacH4 by H4S1 mutation or Nat4 deletion extends yeast replicative lifespan in a similar manner to calorie restriction (CR). Constitutive expression of Nat4 counteracts the longevity effect induced by CR through a mechanism that involves the induction of specific stress-response genes and nicotinamidase Pnc1, a major regulator of Sir2 activity (Molina-Serrano et al., 2016).
H4K12
H4K12 can be found both in acetylated and methylated forms. In its acetylated form this residue is associated with active transcription (Wang et al., 2008). Interestingly, Peleg and colleagues found that old mice have deregulated H4K12ac levels in certain key genes, impairing their ability to learn (Peleg et al., 2010). Following this observation, they also show that recovering physiological levels of this modification by treatment with the histone deacetylase inhibitor SAHA restores the expression of learning-induced genes and the cognitive abilities of old mice. In another report, Peleg and colleagues also found that impairing Chameau, the main H4K12 acetyltransferase in D. melanogaster, promotes longevity and reduces age-related phenotypes(Peleg et al., 2016).
H4K16
H4K16 is usually found in an acetylated form and it is implicated in nucleosome–nucleosome interactions as well as higher order chromatin structure. This modification is present in active chromatin (Kimura et al., 2002; Suka et al., 2002) and its levels increase during aging (Dang et al., 2009). H4K16ac is removed by Sir2/SIRT1, a histone deacetylase with well-documented implications in aging (Houtkooper et al., 2012). Yeast mutant strains in which lysine 16 on H4 has been substituted by arginine (H4K16R) or glutamine (H4K16Q) show a decrease in replicative lifespan (Dang et al., 2009; Kozak et al., 2010). Consistently, deletion of the H4K16 deacetylase Sir2 has been extensively reported to shorten replicative lifespan in yeast (Kaeberlein et al., 1999; Dang et al., 2009), and deletion of Sas2, the major H4K16 acetyltransferase, has the opposite effect (Dang et al., 2009; Kozak et al., 2010). Along the same lines, evidence shows that Sir2 overexpression increases lifespan in yeast, worms and flies (Kaeberlein et al., 1999; Tissenbaum and Guarente, 2001; Rogina and Helfand, 2004), although this effect seems to be dependent on the genetic background (Kaeberlein et al., 2004; Burnett et al., 2011). In the brain of aged mice, SIRT1-associated genes are found to be deregulated, and overexpression of this enzyme can suppress this age-dependent deregulation (Oberdoerffer et al., 2008). Moreover, mice mildly overexpressing this enzyme have a better overall health, with lower levels of DNA damage and fewer spontaneous carcinomas (Herranz et al., 2010). Interestingly, deletion of Rpd3, another H4K16 deacetylase has the opposite effect to Sir2 deletion, increasing lifespan in yeast and D. melanogaster (Kim et al., 1999; Jiang et al., 2002; Rogina et al., 2002). However, while heterozygous flies have extended longevity, the complete removal of Rpd3 in D. melanogaster is lethal (Rogina et al., 2002). Whether the contradicting effects observed between Rpd3 and Sir2 are a consequence of targeting histones located on different genes is open to discussion. In mice, homozygotic mutants of the enzyme ZMPSTE24 exhibit reduced H4K16 acetylation and premature aging features. Notably, supplementing the drinking water of these mice with the histone deacetylase inhibitor sodium butyrate delays premature cellular aging in these mutants and increases lifespan (Krishnan et al., 2011).
Histone H2B PTMs Associated With Lifespan
Histone H2B monoubiquitination at lysine 123 in yeast and, correspondingly, lysine 120 in vertebrates is required for the trimethylation of both H3K4 and H3K79 (Nakanishi et al., 2009). Interestingly, the levels of this H2B modification increase in replicative aged cells (Rhie et al., 2013). Yeast cells expressing a H2BK123R mutant are short-lived, a result reproduced by deleting the components of the H2B ubiquitinase complex Rad6/Bre1 and the deubiquitinase Ubp10 (Rhie et al., 2013). Moreover, strains carrying a deletion of some components of the SAGA/SLIK histone deubiquitinase complex, including Sgf73, Ubp8 and Sgf11 are long lived. This effect is specific to these three complex subunits, as deletion of other components of SAGA/SLIK have normal or shortened replicative lifespan (McCormick et al., 2014). Interestingly, the lifespan extension observed by deletion of each of these three components is dependent on Sir2 and linked to H4K16 acetylation as well as methylation at H3K4 and H3K79 residues (Rhie et al., 2013).
Dietary Influences on Histone PTMs
Environmental factors such as exercise (Denham et al., 2014), circadian rhythms (Orozco-Solis and Sassone-Corsi, 2014) and even sexual stimuli (Promislow and Kaeberlein, 2014) are shown to influence gene expression and longevity in different organisms. However, nutrient availability and diet is to date the most thoroughly studied environmental factor to affect longevity. Additionally, diet is known to significantly affect the epigenome. Specifically, Wolff et al. (1998) showed for the first time that pregnant female mice fed with a methyl donor rich diet produced higher percentage of offspring with wild-type color coat compared to the ones that fed on a control diet. Since then, the scientific community working within this field was interested to determine whether diet can affect chromatin structure and gene transcription through epigenetic changes. In fact, histone PTMs are excellent intermediaries to connect changes between the environmental input (nutrient availability) and the biological output (transcriptional regulation) (Badeaux and Shi, 2013). Different dietary interventions like high-fat (HF) diet, low protein (LP) diet, and CR showed that extreme dietary conditions affect multiple nutrient sensing pathways and can cause global histone modification changes (Donohoe and Bultman, 2012; Ribarič, 2012) (Table 2). However, even though dietary interventions, such as CR, have been shown to impact on lifespan, the causal evidence that establishes histone modifications as the molecular bridge between diet and longevity is still underdeveloped. Nevertheless, below we briefly introduce nutrient-signaling pathways that feed into histone modifications and then summarize the evidence which demonstrate that dietary interventions influence the occurrence of lifespan-associated histone modifications described above.
Nutrient-Sensing Signaling Pathways Involving Histone Modifications
Sirtuins are probably the best-studied family of enzymes implicated in changing the epigenome as a response to environmental signals. Sirtuins are histone deacetylases (HDACs) that mediate lysine deacetylation through NAD hydrolysis, yielding O-acetyl-ADP-ribose nicotinamide. As NAD-dependent deacetylases, sirtuins are a perfect candidate to mediate lifespan response to nutrients, having a dual role as NAD-sensors and transcriptional regulators trough the deposition of PTMs in histones and other target proteins (Houtkooper et al., 2012). Sir2/SIRT1 is the most studied sirtuin in aging, and it has been extensively reported that raising its activity by altering NAD+/NADH levels, genetic manipulation or chemical stimulation robustly extends lifespan in yeast, C. elegans, D. melanogaster and mammals (Guarente, 2013). This effect is not exclusive of Sir2/SIRT1, as other members of this family have also been consistently implicated in lifespan regulation (Grabowska et al., 2017). NAD+ levels increase during fasting or exercising, while HF diet reduces the NAD+/NADH ratio (Cantó et al., 2010; Kim et al., 2011). The NAD+ levels would therefore influence the deacetylation of H3K9, H3K56 and H4K16, among other sirtuin targets with strong effect on lifespan regulation.
Target of Rapamycin (TOR) is a well-preserved nutrient sensing pathway that has been extensively linked to CR and lifespan regulation, and its inhibition through rapamycin increases lifespan in several organisms (Johnson et al., 2013). Many yeast proteins (and their orthologs in higher eukaryotes) acting downstream the Tor pathway, like Sch9 and Rim15 kinases, the stress-response transcription factors Msn2/4 and the H3K36 HDMs Gis1 and Rph1 have been linked to lifespan regulation (Pedruzzi et al., 2000; Fabrizio et al., 2001; Kaeberlein et al., 2005; Wei et al., 2008; Sen et al., 2015). The H3K36 methyltransferase, Set2, is also required for TOR signaling and regulates the transcriptional response to nutrient stress (McDaniel et al., 2017). TOR shares many downstream components with the glucose-sensing Ras/PKA, another pathway linked to lifespan regulation. The histone deacetylase Sir2 is phosphorylated by this pathway, which then regulates its ability to mediate lifespan under CR conditions (Kang et al., 2015). In mammals, the cAMP/CREB signaling pathway, which is activated during short term fasting (Altarejos and Montminy, 2011), relies on the PKA kinase to promote CREB association with CBP/p300, a coactivator family with the ability to acetylate histones (Bannister and Kouzarides, 1996; Ogryzko et al., 1996). Interestingly, the cAMP/CREB pathway has been the focus of studies regarding the cognitive deficits that appear with aging (Hansen and Zhang, 2013).
AMPK/Snf1 is another energy sensing pathway induced by ATP depletion that regulates transcription through many epigenetic functions. The AMPK/Snf1 kinase complexes can be translocated from the cytoplasm into the nuclei upon activation, where they can regulate the activity of several transcription factors (Salminen et al., 2016). AMPK/Snf1 is a histone kinase itself that phosphorylates yeast H3S10 and mammalian H2BS36 residues (Lo et al., 2005; Bungard et al., 2010). AMPK/Snf1 also regulates the activity of several histone acetyltransferases and deacetylases through phosphorylation of these enzymes (Salminen et al., 2016). This pathway also affects histone acetylation and deacetylation by controlling acetyl CoA and NAD+ levels.
The insulin signaling pathway, conserved from C. elegans to mammals, has been extensively associated with aging in different organisms. Mutations of its different components regulate genes that are involved in lifespan (Kenyon, 2011), and genetic interventions that decrease growth hormone and insulin-like growth factor signaling robustly extend longevity in mammals (Brown-Borg, 2015). The PI3K/AKT pathway is central to insulin signaling and can phosphorylate several chromatin-modifying enzymes such as the histone acetyltransferase p300, the H3K27 methyltransferase EZH2 and BMI1, a core component of the polycomb repressive complex 1 associated with H2AK119 ubiquitination (Cha et al., 2005; Huang and Chen, 2005; Nacerddine et al., 2012).
Effects of Dietary Interventions on Lifespan-Associated Histone Modifications
Caloric Restriction
Among the various dietary interventions, CR is the best characterized, and it can increase lifespan in yeast, worm, flies, rodents, and primates. In primates, CR can delay the onset of long-lasting age-related diseases such as cardiovascular disease, type 2 diabetes, degenerative diseases and cancer (Ribarič, 2012). CR is shown to extend lifespan even under alternate day fasting (ADF), where animals were fed with normal diet 1 day and caloric restricted diet the next day (Ribarič, 2012). The beneficial effects of CR arise from multiple mechanisms, including reduction of oxidative stress, modulation of metabolic pathways and, interestingly, chromatin remodeling (Jiménez-Chillarón et al., 2012). Here we provide an extensive overview of the links to histone modifications (Table 2).
In yeast, CR has been shown to regulate the expression of stress response genes through changes in the modification of key histone residues. N-terminal acetylation on histone H4 (NacH4) and the phosphorylation of H3T11 are two histone residues that have been linked to CR-mediated pathways. Both PTMs regulate lifespan through the transcriptional regulation of different stress-response genes. Specifically, CR decreases the acetylation levels at the N-terminus of histone H4. This, in turn, upregulates several stress-response genes, including the one encoding for nicotinamidase Pnc1, a main regulator of Sir2 activity and a well-characterized gene involved in replicative lifespan (Molina-Serrano et al., 2016). CR also affects H3T11 phosphorylation, in this case enhancing the modification. This mechanism regulates the expression of several stress-response genes, which eventually affect chronological lifespan by influencing cell tolerance to media acidification (Oh et al., 2018). These are two of the best examples of histone modifications through which a dietary input (CR) affects the expression of key regulators that eventually impact on cellular longevity.
CR stimulates several epigenetic effects in mammals. Rats fed with a diet containing an indigestible starch showed a reduction of the key transcription factor ChREBP (carbohydrate response element binding protein) and THRSP (thyroid hormone-responsive spot 14 protein) levels. The promoter/enhancer and transcribed regions of the Thrsp gene fed with indigestible starch showed a decrease in acetylation in the H3K9, H3K14, H4K5, H4K8, H4K12, and H4K16 residues compared with rats fed with digestible starch (Shimada et al., 2011). Another effect of CR is the increase in histone H4 acetylation on the Glut4 (glucose transporter 4) gene promoter in adipose tissue of obese mice. GLUT4 is responsible for regulating glucose metabolism through uptake into adipocytes, and the increased acetylation enhances its expression. Moreover, 50% CR during gestation in rats reduced GLUT4 in offspring muscles. These reduction is concomitant with a decrease in H3K14ac and an increase in H3K9me2 (Jiménez-Chillarón et al., 2012). A 50% CR diet in rats during gestation also showed epigenetic changes in the offspring liver. Those changes included an increase in H3K4me3 and a decrease in the H3K4me2 levels at the Igf1 locus, a gene essential for the insulin signaling pathway (Jiménez-Chillarón et al., 2012). In human cell cultures glucose restriction reduces the expression of the p16(INK4A) gene. This is accompanied by a decrease in H3ac and H3K4me2 and an increase in H3K9me3 promoter levels (Li et al., 2011). Additionally, glucose restriction increased H3ac, H4ac and H3K4me2 levels on the Tert promoter and increased its expression in human fetal lung fibroblasts (Daniel and Tollefsbol, 2015). Free-feeding (ad libitum) long-lived rats have the same gene expression profile as the ones fed with CR. The overlapping expression profile contained genes that encode for proteins involved in histone acetylation and deacetylation such as NUR77 (NR4A1) and mortality-like factor 1 (MORF4L1), as well as other additional chromatin regulators like BAG4, MEF2C, HDAC5, BCOR11, H3F3B, BRD3, KAT6A, HIST1HD AND CHMP1A. These proteins may be implicated in the dietary memory effect (Wood et al., 2015).
CR seems to work through several pathways, including sirtuin activation (Herranz and Serrano, 2010; Li et al., 2011), inhibition of lipogenesis, regulation of the mitochondrial function and glucose homeostasis. SIRT1 acts as a metabolic sensor of CR and can delay aging by providing increased stress resistance through regulation of the tumor-suppressor protein p53 and the fork head box O gene FOXO3a. SIRT1 deacetylates histones as well as non-histone proteins, such as the transcription factor NF-kB. Interestingly, many CR mimetic phytochemicals work as sirtuin activators (vel Szic et al., 2015). For example, resveratrol (RSV), a compound obtained from the skins of red grapes, is a caloric restriction mimetic which activates Sir2/SIRT1 in a dose dependent manner (Price et al., 2012). RSV increases lifespan in yeast, worms, flies, and fish (Price et al., 2012). In mammalian cells, RSV also activates the AMPK pathway and directly phosphorylates PGC-1α, the key regulator of energy metabolism. In human peritoneal mesothelial cells, RSV delays replicative aging by mobilization of antioxidant and DNA repair mechanisms (Ribarič, 2012). Other compounds like 2-deoxyglucose, a glucose analog that blocks glucose metabolism, can also induce SIRT1 in mammals (Davis and Ross, 2007).
High-Fat Diet (HF)
High-fat diet is another well characterized dietary intervention. Mice fed in these conditions may develop obesity, together with impaired glucose tolerance and enhanced pyruvate tolerance. Rats fed with HF diet that become obese (obesity prone – OP)show hepatic cellular aging. However, some mice develop resistance (obesity resistant – OR) and thus do not show the OP phenotype (Zhang et al., 2012). Interestingly, the liver cells of OP mice have increased p16(Ink4a) and p21(Cip1) mRNA levels compared to OR mice. These two genes are regulators of cellular aging, and their upregulation is associated with changes in PTM levels. The p16(Ink4a) gene showed higher H4ac and lower H3K27me levels at the promoter and/or the coding region in the liver of OP compared to OR mice (Zhang et al., 2012). Similar results have been observed in p16(Ink4a) expression when comparing OP to control mice (Zhang et al., 2018). This is one of the earliest examples of how diet affects histone modifications, which in turn impact on specific genes that regulate cellular lifespan (Zhang et al., 2012). However, the p21(Cip1) gene results are contradictory. While the p21(Cip1) gene showed an increase in H3ac, H4ac and H3K4me2 and decreased H3K27me3 levels at the promoter and/or the coding region in OP mice compared to OR mice (Zhang et al., 2012), the same author in a later report found that p21(Cip1) is decreased in HF-treated mice compared to control, with no significant PTM changes (Zhang et al., 2018). In recent reports, HF feeding in mice has been associated with reduced H3K18ac and H3K23ac levels in white adipose tissue and in the pancreas (Carrer et al., 2017), while hepatic gene transcription regulation is associated with changes in H3K27ac levels (Siersbæk et al., 2017). Interestingly, the effects of HF diet can be reversed in mice, as H3K27ac and global hepatic gene transcription levels return to similar levels to control after weight loss (Siersbæk et al., 2017). In another study using diet-induce obese mice (DIO) as prediabetic model, the authors analyzed the liver of these mice and found 10 significantly downregulated and 5 significantly upregulated PTMs compared to control (Nie et al., 2017). Particularly, H3K36me2 was increased 80% in DIO mice, suggesting a possible involvement in prediabetes development. Interestingly, H3K36me2 increase was reversed by metformin, a widely used drug for treating Type 2 Diabetes (Nie et al., 2017). HF diet has also been shown to affect considerably the expression of neuropeptides in the brain (hypothalamus and brain stem), which are responsible for controlling feeding behavior, energy metabolism and body-weight homeostasis (Milagro et al., 2013). Particularly, hypothalamic arcuate nucleus (ARH) neurons, which control feeding behavior in adult mice, show increased levels of histone deacetylases HDAC5 and HDAC8 when the mice are fed with long-term (4 weeks) HF diet (Funato et al., 2011).
HF diet effects are transmitted from embryonic stages into adulthood, regardless of the dietary choices later in life (Howie et al., 2009; Milagro et al., 2013). Maternal HF diet during gestation and lactation is responsible for a plethora of epigenetic changes in the offspring, and some of the HF diet hereditary epigenetic changes can last for up to two generations (Dunn and Bale, 2009). For example, timed-pregnant rats fed with a HF diet throughout gestation and lactation showed reduced p16(Ink4a) levels in the mammary gland. These changes were associated with reduced H4ac levels and increased HDAC3 recruitment within the p16(Ink4a) promoter region (Zheng et al., 2012a). The offspring of HF fed mother rats also have altered PTMs in their livers compared to the offspring from mothers fed with the control diet. Additionally, Pck1, the main control point for the regulation of gluconeogenesis, showed decreased H3ac, H3K4me2, H3K9me3 and H3K27me3 levels in HF conditions (Strakovsky et al., 2011). Adiponectin (regulator of glucose and fatty acid metabolism) expression in the adipose tissue was also reduced in the offspring of mice fed with HF diet during pregnancy, due to a decrease in H3K9ac and concomitant increase in H3K9me levels at the gene promoter (Masuyama and Hiramatsu, 2012). In the same report, the authors observed an increase in leptin levels (hormone, regulator of energy balance and inhibitor of hunger) in the adipose tissue, combined with increased H4K20me at the promoter of its gene (Masuyama and Hiramatsu, 2012). Other studies using pregnant young adult female Japanese macaques fed with a HF diet during pregnancy showed enrichment of the transcriptional coactivators MED1 (mediator complex subunit 1) and NCOA1 (nuclear receptor coactivator 1) on the THRβ (Thyroid hormone receptor B) gene in fetal liver. The THRβ gene promoter was also enriched by histone H3K9ac and H3K14ac, and the expression of THRβ was elevated compared to the offspring from mothers with control diet. Deregulation of thyroid hormones in the offspring of mothers fed with HF diet can lead to obesity (Suter et al., 2012). Interestingly, maternal HF diet can affect the offspring in a different manner based on their genders. Specifically, HF diet during pregnancy in mice gave birth to male offspring with high triglycerides and increased the expression of the antioxidant genes Pon1 and Pon3 compare to controls. On the contrary, in female offspring the changes in Pon1 and Pon3 mRNA levels were not statistically significant. The hepatic Pon1 promoter had significantly more histone H4 acetylation and H3K4me2 in both genders. However, H3K9me3 was only associated with antioxidant genes in females (Strakovsky et al., 2014). Paternal HF diet changed the epigenome in spermatozoa and offspring liver. Specifically, under HF diet, H3K4me was enriched in paternal sperm around the transcriptional start site (TSS) of genes involved in development regulatory processes such as Hoxd11, Hoxd13, Bai3, Foxp2, and Foxa2. In contrast, in the offspring liver, H3K4me was enriched in genes controlling lipid biosynthesis, fatty acid synthesis and the oxidation-reduction process (Terashima et al., 2015).
Low-Protein (LP) Diet
Maternal malnutrition may lead to several epigenetic alterations in the offspring. For example, gestational protein restriction in mice decreased H3K4me3 and H4K20me3 while it increased H3K9me3 and H3K27me3 levels on the Igf2 (Insulin-like growth factor-II) locus, resulting in its repression in fetal liver (Strakovsky et al., 2011). IGF2 deficiency in humans and mice leads to hepatic lipid homeostasis and growth restriction of the embryo (Strakovsky et al., 2011). LP diet in pregnant rats also led to hyper-cholesterolemia in postnatal rat offspring (d21), which persisted into adulthood (d130). Specifically, LP diet reduced the acetylation and increased the trimethylation of H3K9 at the Cyp7a1 (Cholesterol 7 alpha-hydroxylase) gene promoter, causing its transcriptional repression in the rat offspring. The increase in H3K9me3 deposition was preceded by reduction of its demethylase Jmjd2 during fetal life. In embryonic stage (D19), H3K9me3 levels were not increased and Cyp7a1 expression was modestly reduced (Sohi et al., 2011). Maternal diet during pregnancy can affect certain metabolic processes during the offspring’s mammary gland development and may predispose the offspring to breast cancer later in life. Accordingly, decrease of p21(Cip1) mRNA and protein levels was observed in the offspring mammary gland of Sprague–Dawley rats fed with LP diet during gestation. p21(Cip1) down-regulation was associated with reduced acetylation of H3 and H3K4me2 in its promoter (Zheng et al., 2012b).
Female pregnant pigs fed with LP diet gave birth to male piglets with hepatic activation of G6PC gene. These observations were combined with reduced H3K9me3 and increased histone H3 acetylation and H3K4me3 levels on the G6PC promoter compared with the offspring males from control diet fed mothers. Increase of G6PC gene expression in male piglets could possibly contribute to adult-onset hyperglycemia (Jia et al., 2012). LP diet in pigs during pregnancy and lactation caused upregulation of myostatin (MSTN) mRNA and protein levels in the muscle of male piglets 28 weeks of age. The MSTN increased levels were associated with enrichment of H3K9ac and H3K4me3 marks and increase of FOXO3 (forkhead box class O family member protein 3) and GR (glucocorticoid receptor) levels on the gene promoter of piglets from LP fed sows compared to the piglets from sows fed standard protein (Jia et al., 2016).
Maternal LP diet during preimplantation also affected epigenetically the embryos. Particularly, mice fed with LP diet prior to embryonic implantation caused reduction of expression of GATA6 transcription factor in the primitive endoderm of their embryos. The GATA6 expression change was linked to reduction of H3 and H4 acetylation and reduced RNA polymerase II levels on its promoter. Consistently, they also observed upregulation of the Hdac1 gene (Sun et al., 2015).
Effects of Specific Nutrient Components
Several natural compounds from widely accessible food products can act as regulators of the epigenome. Sulforaphane, an isothiocyanate from broccoli and cabbage, and allylcompounds from garlic (diallyl disulfide and S-allyl mercaptocysteine) can have HDAC inhibitory effect. Phenethyl isothiocyanate (PEITC), present in cruciferous vegetables; and epigallocatechin-3-gallate (EGCG) from green tea are found to have HDAC inhibitory activity too. Another HDAC inhibitor, sodium butyrate, is found in cheese and butter and can regulate histone acetylation levels at the p21(Cip1) promoter (Davie, 2003). Also luteolin, found in parsley, thyme, peppermint, basil herb, celery and artichoke; and curcumin from turmeric can block HDAC activity. Additionally, curcumin has HAT inhibitory activity, since it binds the HAT p300 and causes conformational change in the enzyme, decreasing its affinity to histones H3 and H4 and acetyl CoA (Stefanska et al., 2012). Diindolylmethane, a digestion byproduct of indole-3-carbinol generated in the stomach from different vegetables (including broccoli, cabbage, cauliflower, mustard and radish) causes proteasomal degradation of the histone deacetylases HDAC1, HDAC2 and HDAC3. Chromatin immunoprecipitation (ChIP) experiments showed a reduction in the localization of these enzymes at the p21(Cip1) and p27(Kip1) gene promoters (Shankar et al., 2013). Dietary polyphenols such as RSV, quercetin and catechins have also an effect activating the HDAC Sir2/SIRT1. RSV binds to SIRT1 to cause a conformational change that allows tighter fluorophore holding and stronger peptide substrate binding (Borra et al., 2005). RSV also activates the HAT p300, which participates in the formation of an active chromatin structure (Narayanan et al., 2003). Despite the clear correlation between specific nutrients and activity or localization of particular histone modifiers, it is still not clear which substrates are affected in all these cases. More studies are required to determine whether molecular mechanisms are directed through histone modifications or through other non-histone substrates of the nutrient-regulated HDACs and HATs.
The epigenetic effects of particular nutrients are extensively studied in cancer cells. Curcumin downregulates p300 expression in B-cell non-Hodgkin lymphoma cell (Chen et al., 2007) and promotes the proteasome-dependent degradation of p300 and CBP (Shankar et al., 2013). Curcumin also inhibits the expression of HDAC1 and 2 in breast cancer or cervical cancer lines (Cătană et al., 2018), and of HDAC1, 3 and 8 in Raji cells while increasing H4 acetylation (Liu et al., 2005). Curcumin also decreases acetylation levels of H3 and H4 in glioblastoma cancer cells and adult neural-derived stem cells (Park et al., 2012). PEITC has been found to increase global levels of acetylated histone H3 and H3K4 methylation while decreasing the level of H3K9me3 in prostate cancer cells (Wang et al., 2007). EGCG decreases HDAC activity and blocks HDAC1-3 expression while raising H3K9ac, H3K18ac and H4ac levels in prostate cancer cells (Pandey et al., 2010). Other reports have shown that EGCG increases the expression of p16(INK4A) p21(CIP1) in skin cancer cells by downregulation of HDAC activity and increasing the H3K9ac, H3K14ac, H4K5ac, H4K12ac, and H4K16ac levels (Nandakumar et al., 2011). Diallyl disulfide inhibitory effects on HDACs are correlated to tumor suppression through p21(CIP1) induction in human colon cancer cells (Myzak and Dashwood, 2006; Nian et al., 2009; Stefanska et al., 2012). Sulforaphane mediates histone acetylation changes locally on p21(Cip1) and Bax gene promoters in mice and human colon and prostate cancer cells, thus being considered an important chemopreventive factor (Myzak et al., 2006; Dashwood and Ho, 2007). Particularly, single dose of sulforaphane can significantly inhibit HDAC activity and increase histone H3 and H4 acetylation levels globally and locally on those genes (Myzak et al., 2006). In addition, sulforaphane was also shown to inhibit HDAC activity in peripheral blood mononuclear cells (PBMCs) in humans (Myzak et al., 2007). The HDAC inhibitory action of luteolin is associated with the inhibition of cancer cell growth, survival and invasion in human epithelioid lung cancer cells (Attoub et al., 2011). Genistein, found in soy products, was found to increase H3ac, H4ac and H3K4me2 at the p21(CIP1) and p16(INK4A) transcription start sites, causing their reactivation in human prostate cancer cell lines (Majid et al., 2008). Finally hydroxycitrate (HCA), found in tropical plants, is a competitive inhibitor of the ATP citrate lyase. This enzyme has a role in histone acetylation and is involved in tumorigenesis and cancer cell metabolism through activation of the Akt pathway (Cătană et al., 2018).
Concluding Remarks
Accumulating data demonstrate that diet affects the epigenome in a significant degree. Moreover, it is well established that histone modifications can regulate lifespan. However, only a handful of scientific reports showed a direct mechanistic link between diet and longevity through changes in histone PTMs. This demonstrates the difficulty in finding causal evidence that link dietary interventions to histone modification-associated mechanisms which subsequently alter lifespan, especially in mammals. One of the reasons for this obstacle is the lack of experimental models that allow for direct interrogation of the effects of histone mutants on the lifespan phenotype. It is not surprising that S. cerevisiae, with its advantage in genetic amenability and well-established protocols has helped the most in studying the direct role of PTMs in aging. Interestingly, various dietary interventions seem to affect modifications on histone residues that have already been linked to lifespan regulation in yeast such as H3K4me, H3K9ac, H3K9me, H3K14ac, H3K27me, and H4K16ac among others. This is perhaps a good indicator that histone PTMs serve as a crossroad between diet and longevity.
To date, CR is the best-described dietary intervention affecting longevity, mainly through changes in global histone acetylation levels and the regulation of multiple genes. Systematic, high-throughput studies showed that many histone residues could potentially regulate lifespan (Sen et al., 2015). Together with the fact that H2A and H2B histone modifications are substantially less studied, it is easy to hypothesize that other unidentified histone PTMs mediating the dietary effects on cell lifespan remain to be discovered. The detection of novel changes in histone modifications altered under different dietary interventions could be achieved through systematic mass spectrometry studies.
Intriguingly, one of the key features of epigenetic marks is their dynamic nature, opening the possibility to reprogram the epigenome with the aim to enhance our lifespan. Some conceivable ways to accomplish this could involve interventions in our diet and daily habits. Diet can regulate aspects such as lipid intake and NAD+ levels, promoting a lipid-specific gene expression profile (McDonnell et al., 2016), affecting histone acetylation by regulating sirtuin activity, and possibly changing the expression of longevity genes (Cantó et al., 2010). Another possibility would entail natural compounds with an effect on lifespan that could be supplemented at the right dose in our diet. For example, while high fat diet shows cellular aging in the liver of rodents (Zhang et al., 2012), RSV intake can correct this effect and extend the lifespan of these mice through AMPK/SIRT1 activation and improved insulin sensitivity (Donohoe and Bultman, 2012). The circadian clock, which has strong correlation with epigenetic mechanisms and nutrient sensing, could also contribute to enhance health span and healthy aging with simple changes in the sleep/wake cycles (Orozco-Solis and Sassone-Corsi, 2014). Another approach to lengthen our lifespan and improve our health would involve altering pharmacologically the epigenome of individuals to delay the aging process. Drugs like metformin and rapamycin can mimic some of the CR effects and showed very promising lifespan extension results in mice (Harrison et al., 2009; Martin-Montalvo et al., 2013). Accordingly, multiple therapeutic approaches have been explored with the aim to reprogram histone marks in order to avoid the appearance or progression of aging. Perhaps the most explored route is the usage of HDAC inhibitors to treat diseases like neurodegeneration, cancer, metabolic disorders and others (Tang et al., 2013). HMT inhibitors are also promising, and had shown beneficial effects in the memory of aging mice by targeting H3K9me3 (Snigdha et al., 2016). The results obtained with these drugs are quite promising, and thus many other inhibitors that target different histone modifying enzymes are nowadays under development (Helin and Dhanak, 2013).
The long-term goal of many studies is to decipher the right balance between exercise and personalized nutrition leading to beneficial epigenetic changes that will increase our health span. An advantage of epigenetic regulation, in addition to being reversible, is based on the fact that it can be inherited by the progeny helping to perpetuate the acquired longevity effects during several generations (Greer et al., 2011). However, this is a double-edged sword, as the opposite scenario is also possible, where the epigenetic consequences of choosing a bad diet could also be passed to our descendants (Huypens et al., 2016). The epigenetic component of the aging process is undeniably complex, but future studies could unravel the precise underlying mechanisms that could illuminate preventive or therapeutic dietary interventions in order to promotehealth and longevity.
Author Contributions
All authors wrote the manuscript. DK and DM-S contributed equally to this work.
Funding
This work was co-funded by the European Regional Development Fund and the Republic of Cyprus through the Research Promotion Foundation (Project: EXCELLENCE/1216/0215).
Conflict of Interest Statement
DK was employed by company Efevre Tech Ltd.
The remaining authors declare that the research was conducted in the absence of any commercial or financial relationships that could be construed as a potential conflict of interest.
Acknowledgments
We apologize to all authors whose work could not be cited because of space limitations.
References
Altarejos, J. Y., and Montminy, M. (2011). CREB and the CRTC co-activators: sensors for hormonal and metabolic signals. Nat. Rev. Mol. Cell Biol. 12, 141–151. doi: 10.1038/nrm3072
Alvares, S. M., Mayberry, G. A., Joyner, E. Y., Lakowski, B., and Ahmed, S. (2014). H3K4 demethylase activities repress proliferative and postmitotic aging. Aging Cell 13, 245–253. doi: 10.1111/acel.12166
Amdam, G. V. (2011). Social context, stress, and plasticity of aging. Aging Cell 10, 18–27. doi: 10.1111/j.1474-9726.2010.00647.x
Ashapkin, V. V., Kutueva, L. I., and Vanyushin, B. F. (2017). Aging as an epigenetic phenomenon. Curr. Genomics 18, 385–407. doi: 10.2174/1389202918666170412112130
Attoub, S., Hassan, A. H., Vanhoecke, B., Iratni, R., Takahashi, T., Gaben, A.-M., et al. (2011). Inhibition of cell survival, invasion, tumor growth and histone deacetylase activity by the dietary flavonoid luteolin in human epithelioid cancer cells. Eur. J. Pharmacol. 651, 18–25. doi: 10.1016/j.ejphar.2010.10.063
Badeaux, A. I., and Shi, Y. (2013). Emerging roles for chromatin as a signal integration and storage platform. Nat. Rev. Mol. Cell Biol. 14, 211–224. doi: 10.1038/nrm3545
Bannister, A. J., and Kouzarides, T. (1996). The CBP co-activator is a histone acetyltransferase. Nature 384, 641–643. doi: 10.1038/384641a0
Bannister, A. J., and Kouzarides, T. (2011). Regulation of chromatin by histone modifications. Cell Res. 21, 381–395. doi: 10.1038/cr.2011.22
Bannister, A. J., Schneider, R., Myers, F. A., Thorne, A. W., Crane-Robinson, C., and Kouzarides, T. (2005). Spatial distribution of Di- and Tri-methyl lysine 36 of histone H3 at active genes. J. Biol. Chem. 280, 17732–17736. doi: 10.1074/jbc.M500796200
Baumgart, M., Groth, M., Priebe, S., Savino, A., Testa, G., Dix, A., et al. (2014). RNA-seq of the aging brain in the short-lived fish N. furzeri - conserved pathways and novel genes associated with neurogenesis. Aging Cell 13, 965–974. doi: 10.1111/acel.12257
Borra, M. T., Smith, B. C., and Denu, J. M. (2005). Mechanism of human SIRT1 activation by resveratrol. J. Biol. Chem. 280, 17187–17195. doi: 10.1074/jbc.M501250200
Bracken, A. P., Kleine-Kohlbrecher, D., Dietrich, N., Pasini, D., Gargiulo, G., Beekman, C., et al. (2007). The Polycomb group proteins bind throughout the INK4A-ARF locus and are disassociated in senescent cells. Genes Dev. 21, 525–530. doi: 10.1101/gad.415507
Brown-Borg, H. M. (2015). The somatotropic axis and longevity in mice. Am. J. Physiol. Endocrinol. Metab. 309, E503–E510. doi: 10.1152/ajpendo.00262.2015
Bungard, D., Fuerth, B. J., Zeng, P.-Y., Faubert, B., Maas, N. L., Viollet, B., et al. (2010). Signaling kinase AMPK activates stress-promoted transcription via histone H2B phosphorylation. Science 329, 1201–1205. doi: 10.1126/science.1191241
Burnett, C., Valentini, S., Cabreiro, F., Goss, M., Somogyvári, M., Piper, M. D., et al. (2011). Absence of effects of Sir2 overexpression on lifespan in C. elegans and Drosophila. Nature 477, 482–485. doi: 10.1038/nature10296
Cantó, C., Jiang, L. Q., Deshmukh, A. S., Mataki, C., Coste, A., Lagouge, M., et al. (2010). Interdependence of AMPK and SIRT1 for metabolic adaptation to fasting and exercise in skeletal muscle. Cell Metab. 11, 213–219. doi: 10.1016/j.cmet.2010.02.006
Carrer, A., Parris, J. L. D., Trefely, S., Henry, R. A., Montgomery, D. C., Torres, A., et al. (2017). Impact of a high-fat diet on tissue Acyl-CoA and histone acetylation levels. J. Biol. Chem. 292, 3312–3322. doi: 10.1074/jbc.M116.750620
Cătană, C.-S., Atanasov, A. G., and Berindan-Neagoe, I. (2018). Natural products with anti-aging potential: affected targets and molecular mechanisms. Biotechnol. Adv. 36, 1649–1656. doi: 10.1016/j.biotechadv.2018.03.012
Cha, T.-L., Zhou, B. P., Xia, W., Wu, Y., Yang, C.-C., Chen, C.-T., et al. (2005). Akt-mediated phosphorylation of EZH2 suppresses methylation of lysine 27 in histone H3. Science 310, 306–310. doi: 10.1126/science.1118947
Chen, Y., Shu, W., Chen, W., Wu, Q., Liu, H., and Cui, G. (2007). Curcumin, both histone deacetylase and p300/CBP-specific inhibitor, represses the activity of nuclear factor kappa B and Notch 1 in Raji cells. Basic Clin. Pharmacol. Toxicol. 101, 427–433. doi: 10.1111/j.1742-7843.2007.00142.x
Cheung, I., Shulha, H. P., Jiang, Y., Matevossian, A., Wang, J., Weng, Z., et al. (2010). Developmental regulation and individual differences of neuronal H3K4me3 epigenomes in the prefrontal cortex. Proc. Natl. Acad. Sci. U.S.A. 107, 8824–8829. doi: 10.1073/pnas.1001702107
Cruz, C., Della Rosa, M., Krueger, C., Gao, Q., Horkai, D., King, M., et al. (2018). Tri-methylation of histone H3 lysine 4 facilitates gene expression in ageing cells. eLife 7:e34081. doi: 10.7554/eLife.34081
Dang, W., Steffen, K. K., Perry, R., Dorsey, J. A., Johnson, F. B., Shilatifard, A., et al. (2009). Histone H4 lysine 16 acetylation regulates cellular lifespan. Nature 459, 802–807. doi: 10.1038/nature08085
Daniel, M., and Tollefsbol, T. O. (2015). Epigenetic linkage of aging, cancer and nutrition. J. Exp. Biol. 218, 59–70. doi: 10.1242/jeb.107110
Das, C., Lucia, M. S., Hansen, K. C., and Tyler, J. K. (2009). CBP / p300-mediated acetylation of histone H3 on lysine 56. Nature 459, 113–117. doi: 10.1038/nature07861
Dashwood, R. H., and Ho, E. (2007). Dietary histone deacetylase inhibitors: from cells to mice to man. Semin. Cancer Biol. 17, 363–369. doi: 10.1016/j.semcancer.2007.04.001
Davie, J. R. (2003). Inhibition of histone deacetylase activity by butyrate. J. Nutr. 133, 2485S–2493S. doi: 10.1093/jn/133.7.2485S
Davis, C. D., and Ross, S. A. (2007). Dietary components impact histone modifications and cancer risk. Nutr. Rev. 65, 88–94. doi: 10.1111/j.1753-4887.2007.tb00285.x
de Magalhães, J. P., Curado, J., and Church, G. M. (2009). Meta-analysis of age-related gene expression profiles identifies common signatures of aging. Bioinformatics 25, 875–881. doi: 10.1093/bioinformatics/btp073
Denham, J., Marques, F. Z., O’Brien, B. J., and Charchar, F. J. (2014). Exercise: putting action into our epigenome. Sports Med. 44, 189–209. doi: 10.1007/s40279-013-0114-1
Djeghloul, D., Kuranda, K., Kuzniak, I., Barbieri, D., Naguibneva, I., Choisy, C., et al. (2016). Age-associated decrease of the histone methyltransferase SUV39H1 in HSC perturbs heterochromatin and B lymphoid differentiation. Stem Cell Rep. 6, 970–984. doi: 10.1016/j.stemcr.2016.05.007
Donohoe, D. R., and Bultman, S. J. (2012). Metaboloepigenetics: interrelationships between energy metabolism and epigenetic control of gene expression. J. Cell. Physiol. 227, 3169–3177. doi: 10.1002/jcp.24054
Dunn, G. A., and Bale, T. L. (2009). Maternal high-fat diet promotes body length increases and insulin insensitivity in second-generation mice. Endocrinology 150, 4999–5009. doi: 10.1210/en.2009-0500
Fabrizio, P., Pozza, F., Pletcher, S. D., Gendron, C. M., and Longo, V. D. (2001). Regulation of longevity and stress resistance by Sch9 in yeast. Science 292, 288–290. doi: 10.1126/science.1059497
Farooq, Z., Banday, S., Pandita, T. K., and Altaf, M. (2016). The many faces of histone H3K79 methylation. Mutat. Res. Rev. Mutat. Res. 768, 46–52. doi: 10.1016/j.mrrev.2016.03.005
Feser, J., Truong, D., Das, C., Carson, J. J., Kieft, J., Harkness, T., et al. (2010). Elevated histone expression promotes life span extension. Mol. Cell 39, 724–735. doi: 10.1016/j.molcel.2010.08.015
Funato, H., Oda, S., Yokofujita, J., Igarashi, H., and Kuroda, M. (2011). Fasting and high-fat diet alter histone deacetylase expression in the medial hypothalamus. PLoS One 6:e18950. doi: 10.1371/journal.pone.0018950
Gehani, S. S., Agrawal-Singh, S., Dietrich, N., Christophersen, N. S., Helin, K., and Hansen, K. (2010). Polycomb group protein displacement and gene activation through MSK-dependent H3K27me3S28 phosphorylation. Mol. Cell 39,886–900. doi: 10.1016/j.molcel.2010.08.020
Gong, H., Qian, H., Ertl, R., Astle, C. M., Wang, G. G., Harrison, D. E., et al. (2015). Histone modifications change with age, dietary restriction and rapamycin treatment in mouse brain. Oncotarget 6, 15882–15890. doi: 10.18632/oncotarget.4137
Govin, J., Dorsey, J., Gaucher, J., Rousseaux, S., Khochbin, S., and Berger, S. L. (2010). Systematic screen reveals new functional dynamics of histones H3 and H4 during gametogenesis. Genes Dev. 24, 1772–1786. doi: 10.1101/gad.1954910
Grabowska, W., Sikora, E., and Bielak-Zmijewska, A. (2017). Sirtuins, a promising target in slowing down the ageing process. Biogerontology 18, 447–476. doi: 10.1007/s10522-017-9685-9
Greer, E. L., Maures, T. J., Hauswirth, A. G., Green, E. M., Leeman, D. S., Maro, G. S., et al. (2010). Members of the H3K4 trimethylation complex regulate lifespan in a germline-dependent manner in C. elegans. Nature 466, 383–387. doi: 10.1038/nature09195
Greer, E. L., Maures, T. J., Ucar, D., Hauswirth, A. G., Mancini, E., Lim, J. P., et al. (2011). Transgenerational epigenetic inheritance of longevity in Caenorhabditis elegans. Nature 479, 365–371. doi: 10.1038/nature10572
Guarente, L. (2013). Calorie restriction and sirtuins revisited. Genes Dev. 27, 2072–2085. doi: 10.1101/gad.227439.113
Gut, P., and Verdin, E. (2013). The nexus of chromatin regulation and intermediary metabolism. Nature 502, 489–498. doi: 10.1038/nature12752
Han, Y., Han, D., Yan, Z., Boyd-Kirkup, J. D., Green, C. D., Khaitovich, P., et al. (2012). Stress-associated H3K4 methylation accumulates during postnatal development and aging of rhesus macaque brain. Aging Cell 11, 1055–1064. doi: 10.1111/acel.12007
Hansen, R. T., and Zhang, H.-T. (2013). Senescent-induced dysregulation of cAMP/CREB signaling and correlations with cognitive decline. Brain Res. 1516, 93–109. doi: 10.1016/j.brainres.2013.04.033
Harrison, D. E., Strong, R., Sharp, Z. D., Nelson, J. F., Astle, C. M., Flurkey, K., et al. (2009). Rapamycin fed late in life extends lifespan in genetically heterogeneous mice. Nature 460, 392–395. doi: 10.1038/nature08221
Helin, K., and Dhanak, D. (2013). Chromatin proteins and modifications as drug targets. Nature 502, 480–488. doi: 10.1038/nature12751
Herranz, D., Muñoz-Martin, M., Cañamero, M., Mulero, F., Martinez-Pastor, B., Fernandez-Capetillo, O., et al. (2010). Sirt1 improves healthy ageing and protects from metabolic syndrome-associated cancer. Nat. Commun. 1:3. doi: 10.1038/ncomms1001
Herranz, D., and Serrano, M. (2010). SIRT1: recent lessons from mouse models. Nat. Rev. Cancer 10, 819–823. doi: 10.1038/nrc2962
Houtkooper, R. H., Pirinen, E., and Auwerx, J. (2012). Sirtuins as regulators of metabolism and healthspan. Nat. Rev. Mol. Cell Biol. 13, 225–238. doi: 10.1038/nrm3293
Howie, G. J., Sloboda, D. M., Kamal, T., and Vickers, M. H. (2009). Maternal nutritional history predicts obesity in adult offspring independent of postnatal diet. J. Physiol. 587, 905–915. doi: 10.1113/jphysiol.2008.163477
Hu, Z., Chen, K., Xia, Z., Chavez, M., Pal, S., Seol, J.-H., et al. (2014). Nucleosome loss leads to global transcriptional up-regulation and genomic instability during yeast aging. Genes Dev. 28, 396–408. doi: 10.1101/gad.233221.113
Huang, W.-C., and Chen, C.-C. (2005). Akt phosphorylation of p300 at Ser-1834 is essential for its histone acetyltransferase and transcriptional activity. Mol. Cell. Biol. 25, 6592–6602. doi: 10.1128/MCB.25.15.6592-6602.2005
Huypens, P., Sass, S., Wu, M., Dyckhoff, D., Tschöp, M., Theis, F., et al. (2016). Epigenetic germline inheritance of diet-induced obesity and insulin resistance. Nat. Genet. 48, 497–499. doi: 10.1038/ng.3527
Jack, A. P., Bussemer, S., Hahn, M., Pünzeler, S., Snyder, M., Wells, M., et al. (2013). H3K56me3 is a novel, conserved heterochromatic mark that largely but not completely overlaps with H3K9me3 in both regulation and localization. PLoS One 8:e51765. doi: 10.1371/journal.pone.0051765
Jia, Y., Cong, R., Li, R., Yang, X., Sun, Q., Parvizi, N., et al. (2012). Maternal low-protein diet induces gender-dependent changes in epigenetic regulation of the glucose-6-phosphatase gene in newborn piglet liver. J. Nutr. 142, 1659–1665. doi: 10.3945/jn.112.160341
Jia, Y., Gao, G., Song, H., Cai, D., Yang, X., and Zhao, R. (2016). Low-protein diet fed to crossbred sows during pregnancy and lactation enhances myostatin gene expression through epigenetic regulation in skeletal muscle of weaning piglets. Eur. J. Nutr. 55, 1307–1314. doi: 10.1007/s00394-015-0949-3
Jiang, J. C., Wawryn, J., Kumara, H., and Jazwinski, S. M. (2002). Distinct roles of processes modulated by histone deacetylases Rpd3p, Hda1p, and Sir2p in life extension by caloric restriction in yeast. Exp. Gerontol. 37, 1023–1030. doi: 10.1016/S0531-5565(02)00064-5
Jiang, S., Liu, Y., Xu, C., Wang, Y., Gong, J., Shen, Y., et al. (2017). Dissecting nucleosome function with a comprehensive histone H2A and H2B mutant library. G3 7, 3857–3866. doi: 10.1534/g3.117.300252
Jiménez-Chillarón, J. C., Díaz, R., Martínez, D., Pentinat, T., Ramón-Krauel, M., Ribó, S., et al. (2012). The role of nutrition on epigenetic modifications and their implications on health. Biochimie 94, 2242–2263. doi: 10.1016/j.biochi.2012.06.012
Jin, C., Li, J., Green, C. D., Yu, X., Tang, X., Han, D., et al. (2011). Histone demethylase UTX-1 regulates C. elegans life span by targeting the insulin/IGF-1 signaling pathway. Cell Metab. 14, 161–172. doi: 10.1016/j.cmet.2011.07.001
Johnson, S. C., Rabinovitch, P. S., and Kaeberlein, M. (2013). mTOR is a key modulator of ageing and age-related disease. Nature 493, 338–345. doi: 10.1038/nature11861
Joos, J. P., Saadatmand, A. R., Schnabel, C., Viktorinová, I., Brand, T., Kramer, M., et al. (2018). Ectopic expression of S28A-mutated Histone H3 modulates longevity, stress resistance and cardiac function in Drosophila. Sci. Rep. 8:2940. doi: 10.1038/s41598-018-21372-3
Kaeberlein, M., Kirkland, K. T., Fields, S., and Kennedy, B. K. (2004). Sir2-independent life span extension by calorie restriction in yeast. PLoS Biol. 2:e296. doi: 10.1371/journal.pbio.0020296
Kaeberlein, M., McVey, M., and Guarente, L. (1999). The SIR2/3/4 complex and SIR2 alone promote longevity in Saccharomyces cerevisiae by two different mechanisms. Genes Dev. 13, 2570–2580. doi: 10.1101/gad.13.19.2570
Kaeberlein, M., Powers, R. W., Steffen, K. K., Westman, E. A., Hu, D., Dang, N., et al. (2005). Regulation of yeast replicative life span by TOR and Sch9 in response to nutrients. Science 310, 1193–1196. doi: 10.1126/science.1115535
Kanfi, Y., Naiman, S., Amir, G., Peshti, V., Zinman, G., Nahum, L., et al. (2012). The sirtuin SIRT6 regulates lifespan in male mice. Nature 483, 218–221. doi: 10.1038/nature10815
Kang, W. K., Kim, Y. H., Kang, H. A., Kwon, K.-S., and Kim, J.-Y. (2015). Sir2 phosphorylation through cAMP-PKA and CK2 signaling inhibits the lifespan extension activity of Sir2 in yeast. eLife 4:e09709. doi: 10.7554/eLife.09709
Karmodiya, K., Krebs, A. R., Oulad-Abdelghani, M., Kimura, H., and Tora, L. (2012). H3K9 and H3K14 acetylation co-occur at many gene regulatory elements, while H3K14ac marks a subset of inactive inducible promoters in mouse embryonic stem cells. BMC Genomics 13:424. doi: 10.1186/1471-2164-13-424
Kawahara, T. L., Michishita, E., Adler, A. S., Damian, M., Berber, E., Lin, M., et al. (2009). SIRT6 links histone H3 lysine 9 deacetylation to NF-kappaB-dependent gene expression and organismal life span. Cell 136, 62–74. doi: 10.1016/j.cell.2008.10.052
Kawakami, K., Nakamura, A., Ishigami, A., Goto, S., and Takahashi, R. (2009). Age-related difference of site-specific histone modifications in rat liver. Biogerontology 10, 415–421. doi: 10.1007/s10522-008-9176-0
Kenyon, C. (2011). The first long-lived mutants: discovery of the insulin/IGF-1 pathway for ageing. Philos. Trans. R. Soc. B Biol. Sci. 366, 9–16. doi: 10.1098/rstb.2010.0276
Kim, H.-J., Kim, J. H., Noh, S., Hur, H. J., Sung, M. J., Hwang, J.-T., et al. (2011). Metabolomic analysis of livers and serum from high-fat diet induced obese mice. J. Proteome Res. 10, 722–731. doi: 10.1021/pr100892r
Kim, S., Benguria, A., Lai, C. Y., and Jazwinski, S. M. (1999). Modulation of life-span by histone deacetylase genes in Saccharomyces cerevisiae. Mol. Biol. Cell 10, 3125–3136. doi: 10.1091/mbc.10.10.3125
Kimura, A., Umehara, T., and Horikoshi, M. (2002). Chromosomal gradient of histone acetylation established by Sas2p and Sir2p functions as a shield against gene silencing. Nat. Genet. 32, 370–377. doi: 10.1038/ng993
Kozak, M. L., Chavez, A., Dang, W., Berger, S. L., Ashok, A., Guo, X., et al. (2010). Inactivation of the Sas2 histone acetyltransferase delays senescence driven by telomere dysfunction. EMBO J. 29, 158–170. doi: 10.1038/emboj.2009.314
Krishnan, V., Chow, M. Z. Y., Wang, Z., Zhang, L., Liu, B., Liu, X., et al. (2011). Histone H4 lysine 16 hypoacetylation is associated with defective DNA repair and premature senescence in Zmpste24-deficient mice. Proc. Natl. Acad. Sci. U.S.A. 108, 12325–12330. doi: 10.1073/pnas.1102789108
Larson, K., Yan, S.-J., Tsurumi, A., Liu, J., Zhou, J., Gaur, K., et al. (2012). Heterochromatin formation promotes longevity and represses ribosomal RNA synthesis. PLoS Genet. 8:e1002473. doi: 10.1371/journal.pgen.1002473
Lee, S. S. (2003). DAF-16 target genes that control C. elegans life-span and metabolism. Science 300, 644–647. doi: 10.1126/science.1083614
Li, L., Greer, C., Eisenman, R. N., and Secombe, J. (2010). Essential Functions of the histone demethylase lid. PLoS Genet. 6:e1001221. doi: 10.1371/journal.pgen.1001221
Li, Y., Daniel, M., and Tollefsbol, T. O. (2011). Epigenetic regulation of caloric restriction in aging. BMC Med. 9:98. doi: 10.1186/1741-7015-9-98
Liang, Y., Liu, C., Lu, M., Dong, Q., Wang, Z., Wang, Z., et al. (2018). Calorie restriction is the most reasonable anti-ageing intervention: a meta-analysis of survival curves. Sci. Rep. 8:5779. doi: 10.1038/s41598-018-24146-z
Liu, B., Wang, Z., Zhang, L., Ghosh, S., Zheng, H., and Zhou, Z. (2013). Depleting the methyltransferase Suv39h1 improves DNA repair and extends lifespan in a progeria mouse model. Nat. Commun. 4:1868. doi: 10.1038/ncomms2885
Liu, H., Chen, Y., Cui, G., and Zhou, J. (2005). Curcumin, a potent anti-tumor reagent, is a novel histone deacetylase inhibitor regulating B-NHL cell line Raji proliferation. Acta Pharmacol. Sin. 26, 603–609. doi: 10.1111/j.1745-7254.2005.00081.x
Lo, W.-S., Gamache, E. R., Henry, K. W., Yang, D., Pillus, L., and Berger, S. L. (2005). Histone H3 phosphorylation can promote TBP recruitment through distinct promoter-specific mechanisms. EMBO J. 24, 997–1008. doi: 10.1038/sj.emboj.7600577
López-Otín, C., Blasco, M. A., Partridge, L., Serrano, M., and Kroemer, G. (2013). The hallmarks of aging. Cell 153, 1194–1217. doi: 10.1016/j.cell.2013.05.039
Majid, S., Kikuno, N., Nelles, J., Noonan, E., Tanaka, Y., Kawamoto, K., et al. (2008). Genistein induces the p21WAF1/CIP1 and p16ink4a tumor suppressor genes in prostate cancer cells by epigenetic mechanisms involving active chromatin modification. Cancer Res. 68, 2736–2744. doi: 10.1158/0008-5472.CAN-07-2290
Martin-Montalvo, A., Mercken, E. M., Mitchell, S. J., Palacios, H. H., Mote, P. L., Scheibye-Knudsen, M., et al. (2013). Metformin improves healthspan and lifespan in mice. Nat. Commun. 4:2192. doi: 10.1038/ncomms3192
Masuyama, H., and Hiramatsu, Y. (2012). Effects of a high-fat diet exposure in Utero on the metabolic syndrome-like phenomenon in mouse offspring through epigenetic changes in adipocytokine gene expression. Endocrinology 153, 2823–2830. doi: 10.1210/en.2011-2161
Maures, T. J., Greer, E. L., Hauswirth, A. G., and Brunet, A. (2011). The H3K27 demethylase UTX-1 regulates C. elegans lifespan in a germline-independent, insulin-dependent manner. Aging Cell 10, 980–990. doi: 10.1111/j.1474-9726.2011.00738.x
McColl, G., Killilea, D. W., Hubbard, A. E., Vantipalli, M. C., Melov, S., and Lithgow, G. J. (2008). Pharmacogenetic analysis of lithium-induced delayed aging in Caenorhabditis elegans. J. Biol. Chem. 283, 350–357. doi: 10.1074/jbc.M705028200
McCormick, M. A., Mason, A. G., Guyenet, S. J., Dang, W., Garza, R. M., Ting, M. K., et al. (2014). The SAGA histone deubiquitinase module controls yeast replicative lifespan via Sir2 interaction. Cell Rep. 8, 477–486. doi: 10.1016/j.celrep.2014.06.037
McDaniel, S. L., Hepperla, A. J., Huang, J., Dronamraju, R., Adams, A. T., Kulkarni, V. G., et al. (2017). H3K36 methylation regulates nutrient stress response in Saccharomyces cerevisiae by enforcing transcriptional fidelity. Cell Rep. 19, 2371–2382. doi: 10.1016/j.celrep.2017.05.057
McDonnell, E., Crown, S. B., Fox, D. B., Kitir, B., Ilkayeva, O. R., Olsen, C. A., et al. (2016). Lipids reprogram metabolism to become a major carbon source for histone acetylation. Cell Rep. 17, 1463–1472. doi: 10.1016/j.celrep.2016.10.012
Milagro, F. I., Mansego, M. L., De Miguel, C., and Martínez, J. A. (2013). Dietary factors, epigenetic modifications and obesity outcomes: progresses and perspectives. Mol. Aspects Med. 34, 782–812. doi: 10.1016/j.mam.2012.06.010
Molina-Serrano, D., Schiza, V., Demosthenous, C., Stavrou, E., Oppelt, J., Kyriakou, D., et al. (2016). Loss of Nat4 and its associated histone H4 N-terminal acetylation mediates calorie restriction-induced longevity. EMBO Rep. 17, 1829–1843. doi: 10.15252/embr.201642540
Molina-Serrano, D., Schiza, V., and Kirmizis, A. (2013). Cross-talk among epigenetic modifications: lessons from histone arginine methylation. Biochem. Soc. Trans. 41, 751–759. doi: 10.1042/BST20130003
Mostoslavsky, R., Chua, K. F., Lombard, D. B., Pang, W. W., Fischer, M. R., Gellon, L., et al. (2006). Genomic instability and aging-like phenotype in the absence of mammalian SIRT6. Cell 124, 315–329. doi: 10.1016/j.cell.2005.11.044
Murr, R. (2010). Interplay between different epigenetic modifications and mechanisms. Adv. Genet. 70, 101–141. doi: 10.1016/B978-0-12-380866-0.60005-8
Myzak, M. C., and Dashwood, R. H. (2006). Histone deacetylases as targets for dietary cancer preventive agents: lessons learned with butyrate, diallyl disulfide, and sulforaphane. Curr. Drug Targets 7, 443–452. doi: 10.2174/138945006776359467
Myzak, M. C., Dashwood, W. M., Orner, G. A., Ho, E., and Dashwood, R. H. (2006). Sulforaphane inhibits histone deacetylase in vivo and suppresses tumorigenesis in Apcminus mice. FASEB J. 20, 506–508. doi: 10.1096/fj.05-4785fje
Myzak, M. C., Tong, P., Dashwood, W.-M., Dashwood, R. H., and Ho, E. (2007). Sulforaphane retards the growth of human PC-3 xenografts and inhibits HDAC activity in human subjects. Exp. Biol. Med. 232, 227–234.
Nacerddine, K., Beaudry, J.-B., Ginjala, V., Westerman, B., Mattiroli, F., Song, J.-Y., et al. (2012). Akt-mediated phosphorylation of Bmi1 modulates its oncogenic potential, E3 ligase activity, and DNA damage repair activity in mouse prostate cancer. J. Clin. Invest. 122, 1920–1932. doi: 10.1172/JCI57477
Nakanishi, S., Lee, J. S., Gardner, K. E., Gardner, J. M., Takahashi, Y., Chandrasekharan, M. B., et al. (2009). Histone H2BK123 monoubiquitination is the critical determinant for H3K4 and H3K79 trimethylation by COMPASS and Dot1. J. Cell Biol. 186, 371–377. doi: 10.1083/jcb.200906005
Nandakumar, V., Vaid, M., and Katiyar, S. K. (2011). (-)-Epigallocatechin-3-gallate reactivates silenced tumor suppressor genes, Cip1/p21 and p 16 INK4a, by reducing DNA methylation and increasing histones acetylation in human skin cancer cells. Carcinogenesis 32, 537–544. doi: 10.1093/carcin/bgq285
Narayanan, B. A., Narayanan, N. K., Re, G. G., and Nixon, D. W. (2003). Differential expression of genes induced by resveratrol in LNCaP cells: P53-mediated molecular targets. Int. J. Cancer 104, 204–212. doi: 10.1002/ijc.10932
Ni, Z., Ebata, A., Alipanahiramandi, E., and Lee, S. S. (2012). Two SET domain containing genes link epigenetic changes and aging in Caenorhabditis elegans. Aging Cell 11, 315–325. doi: 10.1111/j.1474-9726.2011.00785.x
Nian, H., Delage, B., Ho, E., and Dashwood, R. H. (2009). Modulation of histone deacetylase activity by dietary isothiocyanates and allyl sulfides: studies with sulforaphane and garlic organosulfur compounds. Environ. Mol. Mutagen. 50, 213–221. doi: 10.1002/em.20454
Nie, L., Shuai, L., Zhu, M., Liu, P., Xie, Z.-F., Jiang, S., et al. (2017). The landscape of histone modifications in a high-fat diet-induced obese (DIO) mouse model. Mol. Cell. Proteomics 16, 1324–1334. doi: 10.1074/mcp.M117.067553
Nowacka-Woszuk, J., Szczerbal, I., Malinowska, A. M., and Chmurzynska, A. (2018). Transgenerational effects of prenatal restricted diet on gene expression and histone modifications in the rat. PLoS One 13:e0193464. doi: 10.1371/journal.pone.0193464
Oberdoerffer, P., Michan, S., McVay, M., Mostoslavsky, R., Vann, J., Park, S.-K., et al. (2008). SIRT1 redistribution on chromatin promotes genomic stability but alters gene expression during aging. Cell 135, 907–918. doi: 10.1016/j.cell.2008.10.025
Ogryzko, V. V., Schiltz, R. L., Russanova, V., Howard, B. H., and Nakatani, Y. (1996). The transcriptional coactivators p300 and CBP are histone acetyltransferases. Cell 87, 953–959. doi: 10.1016/S0092-8674(00)82001-2
Oh, S., Suganuma, T., Gogol, M. M., and Workman, J. L. (2018). Histone H3 threonine 11 phosphorylation by Sch9 and CK2 regulates chronological lifespan by controlling the nutritional stress response. eLife 7:e36157. doi: 10.7554/eLife.36157
Orozco-Solis, R., and Sassone-Corsi, P. (2014). Circadian clock: linking epigenetics to aging. Curr. Opin. Genet. Dev. 26, 66–72. doi: 10.1016/j.gde.2014.06.003
Pan, M.-R., Hsu, M.-C., Chen, L.-T., and Hung, W.-C. (2018). Orchestration of H3K27 methylation: mechanisms and therapeutic implication. Cell. Mol. Life Sci. 75, 209–223. doi: 10.1007/s00018-017-2596-8
Pandey, M., Shukla, S., and Gupta, S. (2010). Promoter demethylation and chromatin remodeling by green tea polyphenols leads to re-expression of GSTP1 in human prostate cancer cells. Int. J. Cancer 126, 2520–2533. doi: 10.1002/ijc.24988
Park, L. K., Friso, S., and Choi, S.-W. (2012). Nutritional influences on epigenetics and age-related disease. Proc. Nutr. Soc. 71, 75–83. doi: 10.1017/S0029665111003302
Pedruzzi, I., Bürckert, N., Egger, P., and De Virgilio, C. (2000). Saccharomyces cerevisiae Ras/cAMP pathway controls post-diauxic shift element-dependent transcription through the zinc finger protein Gis1. EMBO J. 19, 2569–2579. doi: 10.1093/emboj/19.11.2569
Peleg, S., Feller, C., Forne, I., Schiller, E., Sévin, D. C., Schauer, T., et al. (2016). Life span extension by targeting a link between metabolism and histone acetylation in Drosophila. EMBO Rep. 17, 455–469. doi: 10.15252/embr.201541132
Peleg, S., Sananbenesi, F., Zovoilis, A., Burkhardt, S., Bahari-Javan, S., Agis-Balboa, R. C., et al. (2010). Altered histone acetylation is associated with age-dependent memory impairment in mice. Science 328, 753–756. doi: 10.1126/science.1186088
Peters, A. H., O’Carroll, D., Scherthan, H., Mechtler, K., Sauer, S., Schöfer, C., et al. (2001). Loss of the Suv39h histone methyltransferases impairs mammalian heterochromatin and genome stability. Cell 107, 323–337. doi: 10.1016/S0092-8674(01)00542-6
Price, N. L., Gomes, A. P., Ling, A. J. Y., Duarte, F. V., Martin-Montalvo, A., North, B. J., et al. (2012). SIRT1 is required for AMPK activation and the beneficial effects of resveratrol on mitochondrial function. Cell Metab. 15, 675–690. doi: 10.1016/j.cmet.2012.04.003
Promislow, D. E. L., and Kaeberlein, M. (2014). Chemical warfare in the battle of the sexes. Science 343, 491–492. doi: 10.1126/science.1250174
Pu, M., Ni, Z., Wang, M., Wang, X., Wood, J. G., Helfand, S. L., et al. (2015). Trimethylation of Lys36 on H3 restricts gene expression change during aging and impacts life span. Genes Dev. 29, 718–731. doi: 10.1101/gad.254144.114
Rangaraju, S., Solis, G. M., Thompson, R. C., Gomez-Amaro, R. L., Kurian, L., Encalada, S. E., et al. (2015). Suppression of transcriptional drift extends C. elegans lifespan by postponing the onset of mortality. eLife 4:e08833. doi: 10.7554/eLife.08833
Ree, R., Varland, S., and Arnesen, T. (2018). Spotlight on protein N-terminal acetylation. Exp. Mol. Med. 50:90. doi: 10.1038/s12276-018-0116-z
Rhie, B.-H., Song, Y.-H., Ryu, H.-Y., and Ahn, S. H. (2013). Cellular aging is associated with increased ubiquitylation of histone H2B in yeast telomeric heterochromatin. Biochem. Biophys. Res. Commun. 439, 570–575. doi: 10.1016/j.bbrc.2013.09.017
Rogina, B., and Helfand, S. L. (2004). Sir2 mediates longevity in the fly through a pathway related to calorie restriction. Proc. Natl. Acad. Sci. U.S.A. 101, 15998–16003. doi: 10.1073/pnas.0404184101
Rogina, B., Helfand, S. L., and Frankel, S. (2002). Longevity regulation by Drosophila Rpd3 deacetylase and caloric restriction. Science 298, 1745–1745. doi: 10.1126/science.1078986
Rougeulle, C., Chaumeil, J., Sarma, K., Allis, C. D., Reinberg, D., Avner, P., et al. (2004). Differential histone H3 Lys-9 and Lys-27 methylation profiles on the X chromosome. Mol. Cell. Biol. 24, 5475–5484. doi: 10.1128/MCB.24.12.5475-5484.2004
Ryu, H.-Y., Rhie, B.-H., and Ahn, S. H. (2014). Loss of the Set2 histone methyltransferase increases cellular lifespan in yeast cells. Biochem. Biophys. Res. Commun. 446, 113–118. doi: 10.1016/j.bbrc.2014.02.061
Salminen, A., Kauppinen, A., and Kaarniranta, K. (2016). AMPK/Snf1 signaling regulates histone acetylation: impact on gene expression and epigenetic functions. Cell. Signal. 28, 887–895. doi: 10.1016/j.cellsig.2016.03.009
Santos-Rosa, H., Schneider, R., Bannister, A. J., Sherriff, J., Bernstein, B. E., Emre, N. C. T., et al. (2002). Active genes are tri-methylated at K4 of histone H3. Nature 419, 407–411. doi: 10.1038/nature01080
Scaffidi, P., and Misteli, T. (2006). Lamin A-dependent nuclear defects in human aging. Science 312, 1059–1063. doi: 10.1126/science.1127168
Schiza, V., Molina-Serrano, D., Kyriakou, D., Hadjiantoniou, A., and Kirmizis, A. (2013). N-alpha-terminal acetylation of histone H4 regulates arginine methylation and ribosomal DNA silencing. PLoS Genet. 9:e1003805. doi: 10.1371/journal.pgen.1003805
Sen, P., Dang, W., Donahue, G., Dai, J., Dorsey, J., Cao, X., et al. (2015). H3K36 methylation promotes longevity by enhancing transcriptional fidelity. Genes Dev. 29, 1362–1376. doi: 10.1101/gad.263707.115
Shankar, S., Kumar, D., and Srivastava, R. K. (2013). Epigenetic modifications by dietary phytochemicals: implications for personalized nutrition. Pharmacol. Ther. 138, 1–17. doi: 10.1016/j.pharmthera.2012.11.002
Shimada, M., Mochizuki, K., and Goda, T. (2011). Feeding rats dietary resistant starch reduces both the binding of ChREBP and the acetylation of histones on the Thrsp gene in the jejunum. J. Agric. Food Chem. 59, 1464–1469. doi: 10.1021/jf103111u
Shimada, M., Niida, H., Zineldeen, D. H., Tagami, H., Tanaka, M., Saito, H., et al. (2008). Chk1 is a histone H3 threonine 11 kinase that regulates DNA damage-induced transcriptional repression. Cell 132, 221–232. doi: 10.1016/j.cell.2007.12.013
Siebold, A. P., Banerjee, R., Tie, F., Kiss, D. L., Moskowitz, J., and Harte, P. J. (2010). Polycomb repressive complex 2 and trithorax modulate Drosophila longevity and stress resistance. Proc. Natl. Acad. Sci. U.S.A. 107, 169–174. doi: 10.1073/pnas.0907739107
Siersbæk, M., Varticovski, L., Yang, S., Baek, S., Nielsen, R., Mandrup, S., et al. (2017). High fat diet-induced changes of mouse hepatic transcription and enhancer activity can be reversed by subsequent weight loss. Sci. Rep. 7:40220. doi: 10.1038/srep40220
Smith, E. D., Tsuchiya, M., Fox, L. A., Dang, N., Hu, D., Kerr, E. O., et al. (2008). Quantitative evidence for conserved longevity pathways between divergent eukaryotic species. Genome Res. 18, 564–570. doi: 10.1101/gr.074724.107
Snigdha, S., Prieto, G. A., Petrosyan, A., Loertscher, B. M., Dieskau, A. P., Overman, L. E., et al. (2016). H3K9me3 inhibition improves memory, promotes spine formation, and increases BDNF levels in the aged hippocampus. J. Neurosci. 36, 3611–3622. doi: 10.1523/JNEUROSCI.2693-15.2016
Sohi, G., Marchand, K., Revesz, A., Arany, E., and Hardy, D. B. (2011). Maternal protein restriction elevates cholesterol in adult rat offspring due to repressive changes in histone modifications at the Cholesterol 7 α- Hydroxylase promoter. Mol. Endocrinol. 25, 785–798. doi: 10.1210/me.2010-0395
Stefanska, B., Karlic, H., Varga, F., Fabianowska-Majewska, K., and Haslberger, A. (2012). Epigenetic mechanisms in anti-cancer actions of bioactive food components–the implications in cancer prevention. Br. J. Pharmacol. 167, 279–297. doi: 10.1111/j.1476-5381.2012.02002.x
Strakovsky, R. S., Zhang, X., Zhou, D., and Pan, Y.-X. (2011). Gestational high fat diet programs hepatic phosphoenolpyruvate carboxykinase gene expression and histone modification in neonatal offspring rats: programming gluconeogenesis by gestational high fat diet. J. Physiol. 589, 2707–2717. doi: 10.1113/jphysiol.2010.203950
Strakovsky, R. S., Zhang, X., Zhou, D., and Pan, Y.-X. (2014). The regulation of hepatic Pon1 by a maternal high-fat diet is gender specific and may occur through promoter histone modifications in neonatal rats. J. Nutr. Biochem. 25, 170–176. doi: 10.1016/j.jnutbio.2013.09.016
Su, L., Li, H., Huang, C., Zhao, T., Zhang, Y., Ba, X., et al. (2018). Muscle-specific histone H3K36 dimethyltransferase SET-18 shortens lifespan of Caenorhabditis elegans by repressing daf-16a expression. Cell Rep. 22, 2716–2729. doi: 10.1016/j.celrep.2018.02.029
Suka, N., Luo, K., and Grunstein, M. (2002). Sir2p and Sas2p opposingly regulate acetylation of yeast histone H4 lysine16 and spreading of heterochromatin. Nat. Genet. 32, 378–383. doi: 10.1038/ng1017
Sun, C., Denisenko, O., Sheth, B., Cox, A., Lucas, E. S., Smyth, N. R., et al. (2015). Epigenetic regulation of histone modifications and Gata6 gene expression induced by maternal diet in mouse embryoid bodies in a model of developmental programming. BMC Dev. Biol. 15:3. doi: 10.1186/s12861-015-0053-1
Sun, D., Luo, M., Jeong, M., Rodriguez, B., Xia, Z., Hannah, R., et al. (2014). Epigenomic profiling of young and aged HSCs reveals concerted changes during aging that reinforce self-renewal. Cell Stem Cell 14, 673–688. doi: 10.1016/j.stem.2014.03.002
Suter, M. A., Sangi-Haghpeykar, H., Showalter, L., Shope, C., Hu, M., Brown, K., et al. (2012). Maternal high-fat diet modulates the fetal thyroid axis and thyroid gene expression in a nonhuman primate model. Mol. Endocrinol. 26, 2071–2080. doi: 10.1210/me.2012-1214
Tang, J., Yan, H., and Zhuang, S. (2013). Histone deacetylases as targets for treatment of multiple diseases. Clin. Sci. Lond. Engl. 124, 651–662. doi: 10.1042/CS20120504
Tasselli, L., Xi, Y., Zheng, W., Tennen, R. I., Odrowaz, Z., Simeoni, F., et al. (2016). SIRT6 deacetylates H3K18ac at pericentric chromatin to prevent mitotic errors and cellular senescence. Nat. Struct. Mol. Biol. 23, 434–440. doi: 10.1038/nsmb.3202
Terashima, M., Barbour, S., Ren, J., Yu, W., Han, Y., and Muegge, K. (2015). Effect of high fat diet on paternal sperm histone distribution and male offspring liver gene expression. Epigenetics 10, 861–871. doi: 10.1080/15592294.2015.1075691
Tissenbaum, H. A., and Guarente, L. (2001). Increased dosage of a sir-2 gene extends lifespan in Caenorhabditis elegans. Nature 410, 227–230. doi: 10.1038/35065638
Tsurumi, A., and Li, W. X. (2012). Global heterochromatin loss: a unifying theory of aging? Epigenetics 7, 680–688. doi: 10.4161/epi.20540
Uchiyama, R., Kupkova, K., Shetty, S. J., Linford, A. S., Pray-Grant, M. G., Wagar, L. E., et al. (2018). Histone H3 lysine 4 methylation signature associated with human undernutrition. Proc. Natl. Acad. Sci. U.S.A. 115, E11264–E11273. doi: 10.1073/pnas.1722125115
Upadhyaya, B., Larsen, T., Barwari, S., Louwagie, E., Baack, M., and Dey, M. (2017). Prenatal exposure to a maternal high-fat diet affects histone modification of cardiometabolic genes in newborn rats. Nutrients 9:407. doi: 10.3390/nu9040407
vel Szic, K. S., Declerck, K., Vidakoviæ, M., and Vanden Berghe, W. (2015). From inflammaging to healthy aging by dietary lifestyle choices: is epigenetics the key to personalized nutrition? Clin. Epigenetics 7:33. doi: 10.1186/s13148-015-0068-2
Wang, L. G., Beklemisheva, A., Liu, X. M., Ferrari, A. C., Feng, J., and Chiao, J. W. (2007). Dual action on promoter demethylation and chromatin by an isothiocyanate restored GSTP1 silenced in prostate cancer. Mol. Carcinog. 46, 24–31. doi: 10.1002/mc.20258
Wang, Z., Zang, C., Rosenfeld, J. A., Schones, D. E., Barski, A., Cuddapah, S., et al. (2008). Combinatorial patterns of histone acetylations and methylations in the human genome. Nat. Genet. 40, 897–903. doi: 10.1038/ng.154
Wei, M., Fabrizio, P., Hu, J., Ge, H., Cheng, C., Li, L., et al. (2008). Life span extension by calorie restriction depends on rim15 and transcription factors downstream of Ras/PKA. Tor, and Sch9. PLoS Genet. 4:e13. doi: 10.1371/journal.pgen.0040013
Wheatley, K. E., Nogueira, L. M., Perkins, S. N., and Hursting, S. D. (2011). Differential effects of calorie restriction and exercise on the adipose transcriptome in diet-induced obese mice. J. Obes. 2011, 1–13. doi: 10.1155/2011/265417
Wolff, G. L., Kodell, R. L., Moore, S. R., and Cooney, C. A. (1998). Maternal epigenetics and methyl supplements affect agouti gene expression in Avy/a mice. FASEB J. 12, 949–957. doi: 10.1096/fasebj.12.11.949
Wood, J. G., Hillenmeyer, S., Lawrence, C., Chang, C., Hosier, S., Lightfoot, W., et al. (2010). Chromatin remodeling in the aging genome of Drosophila. Aging Cell 9, 971–978. doi: 10.1111/j.1474-9726.2010.00624.x
Wood, S. H., van Dam, S., Craig, T., Tacutu, R., O’Toole, A., Merry, B. J., et al. (2015). Transcriptome analysis in calorie-restricted rats implicates epigenetic and post-translational mechanisms in neuroprotection and aging. Genome Biol. 16:285. doi: 10.1186/s13059-015-0847-2
Xu, H., Su, T., and Xue, Y. (2016). Histone H3 N-terminal acetylation sites especially K14 are important for rDNA silencing and aging. Sci. Rep. 6:21900. doi: 10.1038/srep21900
Yuan, J., Pu, M., Zhang, Z., and Lou, Z. (2009). Histone H3-K56 acetylation is important for genomic stability in mammals. Cell Cycle 8, 1747–1753. doi: 10.4161/cc.8.11.8620
Zhang, X., Xu, G. B., Zhou, D., and Pan, Y.-X. (2018). High-fat diet modifies expression of hepatic cellular senescence gene p16(INK4a) through chromatin modifications in adult male rats. Genes Nutr. 13:6. doi: 10.1186/s12263-018-0595-5
Zhang, X., Zhou, D., Strakovsky, R., Zhang, Y., and Pan, Y.-X. (2012). Hepatic cellular senescence pathway genes are induced through histone modifications in a diet-induced obese rat model. Am. J. Physiol. Gastrointest. Liver Physiol. 302, G558–G564. doi: 10.1152/ajpgi.00032.2011
Zheng, S., Li, Q., Zhang, Y., Balluff, Z., and Pan, Y.-X. (2012a). Histone deacetylase 3 (HDAC3) participates in the transcriptional repression of the p16 INK4a gene in mammary gland of the female rat offspring exposed to an early-life high-fat diet. Epigenetics 7, 183–190. doi: 10.4161/epi.7.2.18972
Zheng, S., Rollet, M., Yang, K., and Pan, Y.-X. (2012b). A gestational low-protein diet represses p21WAF1/Cip1 expression in the mammary gland of offspring rats through promoter histone modifications. Br. J. Nutr. 108, 998–1007. doi: 10.1017/S0007114511006222
Keywords: diet, histone modification, lifespan, aging, longevity, high-fat diet, low-protein diet, caloric restriction
Citation: Molina-Serrano D, Kyriakou D and Kirmizis A (2019) Histone Modifications as an Intersection Between Diet and Longevity. Front. Genet. 10:192. doi: 10.3389/fgene.2019.00192
Received: 10 December 2018; Accepted: 22 February 2019;
Published: 12 March 2019.
Edited by:
Yonghan He, Chinese Academy of Sciences, ChinaReviewed by:
George A. Garinis, Foundation for Research and Technology – Hellas, GreeceKaoru Tominaga, Jichi Medical University, Japan
Gil Atzmon, University of Haifa, Israel
Copyright © 2019 Molina-Serrano, Kyriakou and Kirmizis. This is an open-access article distributed under the terms of the Creative Commons Attribution License (CC BY). The use, distribution or reproduction in other forums is permitted, provided the original author(s) and the copyright owner(s) are credited and that the original publication in this journal is cited, in accordance with accepted academic practice. No use, distribution or reproduction is permitted which does not comply with these terms.
*Correspondence: Antonis Kirmizis, kirmizis@ucy.ac.cy
†These authors have contributed equally to this work