- 1Institut für Populationsgenetik, Veterinärmedizinische Universität Wien, Wien, Austria
- 2Vienna Graduate School of Population Genetics, Vienna, Austria
Climate change is a major evolutionary force triggering thermal adaptation in a broad range of species. While the consequences of global warming are being studied for an increasing number of species, limited attention has been given to the evolutionary dynamics of endosymbionts in response to climate change. Here, we address this question by studying the dynamics of Wolbachia, a well-studied endosymbiont of Drosophila melanogaster. D. melanogaster populations infected with 13 different Wolbachia strains were exposed to novel hot and cold laboratory environments for up to 180 generations. The short-term dynamics suggested a temperature-related fitness difference resulting in the increase of clade V strains in the cold environment only. Our long-term analysis now uncovers that clade V dominates in all replicates after generation 60 irrespective of temperature treatment. We propose that adaptation of the Drosophila host to either temperature or Drosophila C virus (DCV) infection are the cause of the replicated, temporally non-concordant Wolbachia dynamics. Our study provides an interesting case demonstrating that even simple, well-controlled experiments can result in complex, but repeatable evolutionary dynamics, thus providing a cautionary note on too simple interpretations on the impact of climate change.
Introduction
The global change in climate imposes strong pressure on many species to deal with increasing temperatures (Thuiller et al., 2005; Cheung et al., 2009; Klausmeyer and Shaw, 2009)—using either mitigation strategies (e.g., shifts in range or activity periods; Davis, 2001; Menzel et al., 2006; Chen et al., 2011), or through genetic changes (e.g., thermal adaptation; Calosi et al., 2008; Marshall et al., 2010; Somero, 2010; Hoffmann and Sgrò, 2011). In the presence of endosymbiotic bacteria, adaptation to temperature could occur by genetic changes either in the host or bacteria—even co-evolutionary processes between both of them could contribute to thermal adaptation.
Wolbachia are intracellular α-Proteobacteria found in many insect and other arthropod species (Baldo et al., 2006; Mateos et al., 2006; Werren et al., 2008), infecting about two thirds of all insects (Hilgenboecker et al., 2008; Miller, 2013). They are predominantly transmitted through the female germline and often confer fitness advantages; e.g., virus protection (Hedges et al., 2008; Chrostek et al., 2013; Faria et al., 2018), learning ability (Bi et al., 2018), increased fecundity (Fry et al., 2004), resistance to heat stress (Gruntenko et al., 2017), and influence longevity (Maistrenko et al., 2016). On the other hand, Wolbachia frequently also imposes considerable costs on its host through the reduction in effective population size by male-killing (Hurst et al., 1999), feminization of genetic males (Rigaut, 1997) and cytoplasmic incompatibility (Bourtzis et al., 1996; Hoffman and Turelli, 1997). In addition to fitness effects of Wolbachia on its host, the fitness of the infected host and the probability of vertical transmission also affect the fitness of Wolbachia. Among the factors contributing to these fitness components are temperature (Jia et al., 2009; Bordenstein and Bordenstein, 2011), bacterial density in the host (Breeuwer and Werren, 1993; Bourtzis et al., 1996; Noda et al., 2001), and the genetic background of the host (Olsen et al., 2001; Reynolds and Hoffmann, 2002; Fry et al., 2004).
Multiple strains—sometimes several supergroups—of Wolbachia may compete within a host population (Dean et al., 2003; Mouton et al., 2003). While coinfection and thus competition within single hosts has been described (Fleury et al., 2000; Hiroki et al., 2004; Ant and Sinkins, 2018), competition mainly occurs between hosts. The relative fitness of multiple Wolbachia strains can be measured by the spread of the fitter strain(s) in sexual populations. A particularly interesting question is how the fitness of different Wolbachia strains is affected by the environment.
A pioneering study used experimental evolution to study temperature adaptation by exposing a replicated polymorphic Drosophila melanogaster population infected by multiple Wolbachia strains to two different temperature regimes (Versace et al., 2014). The dynamics of Wolbachia infection were monitored by clade-specific SNPs in Pool-Seq data (Schlötterer et al., 2014) from up to four replicates at multiple time points in hot and cold temperature regimes. The striking result was that in the cold temperature regime Wolbachia from a single clade (V) very rapidly predominated. Even in hot-evolved replicates that were shifted to the cold temperature regime the same Wolbachia clade V dominated. This consistent association of clade V with cold temperatures was considered strong support for environmentally triggered fitness differences between Wolbachia strains. Here, we extend the previous work by characterizing the Wolbachia dynamics on the level of individual strains rather than clades and our analyses cover substantially more generations in more replicates.
Materials and Methods
Drosophila melanogaster Population and Culture Conditions
We reanalyze an evolve-and-resequence experiment (Turner et al., 2011) for which allele frequency changes in D. melanogaster (Orozco-terWengel et al., 2012; Tobler et al., 2014; Franssen et al., 2015), and Wolbachia strain turnover during the first 50 generations were reported (Versace et al., 2014); detailed descriptions of the experimental setup can be found there. Briefly, 10 replicate populations each with approximately 1000 individuals were created from 113 D. melanogaster isofemale lines collected in Portugal and were subsequently kept in two different temperature regimes: five replicates in a hot environment fluctuating between 18 and 28°C, and five replicates in a cold environment fluctuating between 10 and 20°C. Of the 113 isofemale lines, 47 were known to carry Wolbachia. The 10 replicates at generation 0 are considered as the base population.
The previous datasets included only time points from the early phase of the experimental evolution cages. Here, we extend the analyses to advanced phases of the experiment by including additional time points and replicates for both the hot and the cold evolved populations: while Orozco-terWengel et al. (2012) and Versace et al. (2014) analyzed up to three replicates in the hot evolved populations until generation F37, we now include data for up to five replicates at multiple earlier and later time points until generation F180 in the hot environment. For the cold environment, Versace et al. (2014) analyzed four replicates in generation F15. Here, we add the fifth replicate and multiple time points up to generation F100 (Supplementary Datasheet S2).
Sequencing and Postprocessing
Single females of each of the 47 isofemale lines infected with Wolbachia were sequenced individually (2 × 100 bp; ∼10–30× autosome coverage). The infection status of the isofemale lines had been previously determined using the protocol described below (section “Confirmation of Wolbachia Infection Status”). Pools of flies (Kofler et al., 2011; Schlötterer et al., 2014) were sequenced at different time points over the course of the experiment, including three replicates of the base population (∼500 flies per generation and replicate; paired-end; ∼30× autosome coverage; various read lengths, library preparation protocols, providers, and sequencing platforms following the development of Illumina sequencing over a decade; Supplementary Datasheet S2).
Reads were trimmed with ReadTools v1.2.1 (Gómez-Sánchez and Schlötterer, 2017; parameters: –disable5pTrim –mottQualityThreshold 20 –minReadLength 34); mapped with novoalign v3.08 (Novocraft, 2018; parameters: -r RANDOM) and bwa v0.7.17 (Li, 2013; parameters: mem) using our standard DistMap pipeline (Pandey and Schlötterer, 2013) against the combined reference genome of D. melanogaster v6.03 (Thurmond et al., 2019), wMel (AE017196.1), and common gut bacteria (Petkau et al., 2016; Acetobacter pasteurianus, AP011170.1; Lactobacillus brevis, CP000416.1; Lactobacillus plantarum, CP013753.1); filtered for quality and overlap with the wMel genome or the mtDNA genome with samtools v1.9 (Li et al., 2009; parameters: -f0x02 -q 5); and had duplicates removed with picard v2.12.1-SNAPSHOT (The Broad Institute, 2018; parameters: MarkDuplicates REMOVE_DUPLICATES = true).
Variant Calling and Marker Sites
Variants in the 47 sequenced individuals were called using freebayes v1.2.0 (Garrison and Marth, 2012; parameters: -p2 –pooled-discrete) using the alignments of novoalign and bwa jointly to account for the mapper-specific influence of insert size differences on SNP calling (Kofler et al., 2016). Among the resulting variants, we selected SNPs that only occurred in a true subset of the 47 samples, i.e., allow to discern among strains, and met minimum coverage and quality criteria using bcftools v1.9 [Li, 2011; parameters: -i ‘TYPE = “SNP” & INFO/DP < 3∗mean(DP) & NS = 47 & NUMALT = 1 & QUAL > 40 & MQM > 50 & MQM/MQMR > 4/5 & MQM/MQMR < 5/4 & RPL/RPR > 1/3 & RPL/RPR < 3 & SAF/SAR > 1/3 & SAF/SAR < 3 & SRF/SRR > 1/3 & SRF/SRR < 3’ -e ‘FORMAT/GT! = “hom,”’ where “mean(DP)” in the first condition is the mean depth of all 47 samples over all sites calculated beforehand, and separately for Wolbachia and mtDNA contigs], leaving us with 197 high-quality marker SNPs to discern among the Wolbachia strains present in the 47 infected founder lines (Supplementary Datasheet S3: markers_wmel.vcf.gz) and 29 marker SNPs to discern among the mitochondrial clades (Supplementary Datasheet S3: markers_mtDNA.vcf.gz). Not all SNPs are equally informative, being shared by two or more strains.
Strain and Clade Identification
Based on the polymorphic sites (markers), we distinguished 13 Wolbachia strains (Figure 1), 10 of which have private SNPs. Comparing the markers to the strain-specific SNPs identified previously (Versace et al., 2014), we identify the same overall clade structure with some additional, previously unresolved, fine structure. Accordingly, we continue using the same naming convention. This assignment is fully consistent for Wolbachia and mitochondria strains to clades based on the SNPs provided in Richardson et al. (2012), where the clade structure was originally established.
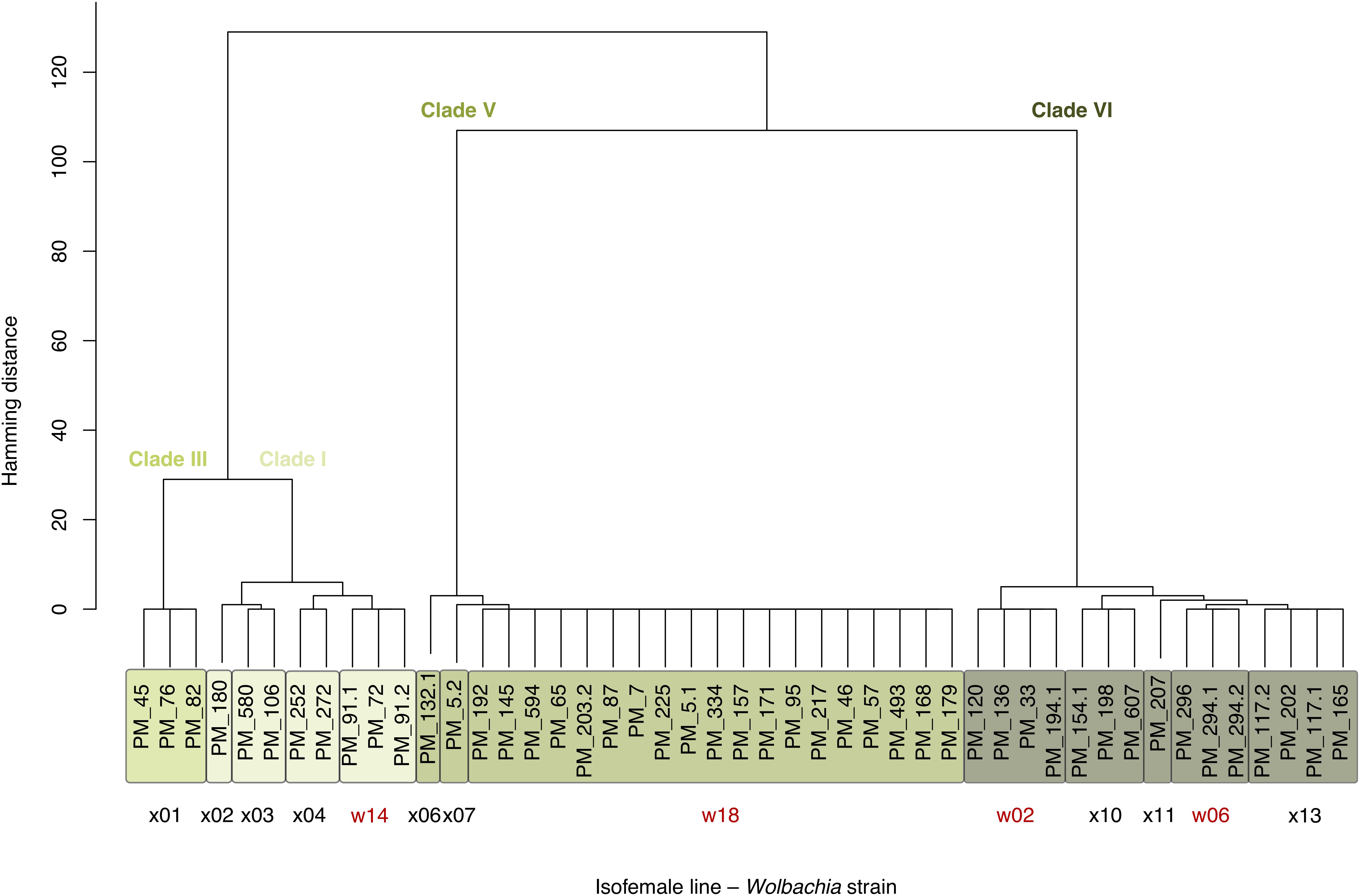
Figure 1. 47 Isofemale lines of the D. melanogaster base population carry 13 Wolbachia strains representing four clades. The tree shows the isofemale lines clustered by Hamming distance h over 197 high-quality marker SNPs on the wMel genome. The three clusters at h = 29 correspond to the Wolbachia superclades discussed in Versace et al. (2014), where also strains w02, w06, w14, and w18 (red) were introduced. The four clusters at h = 6 (color labels) correspond to the Wolbachia clades as defined in Richardson et al. (2012). Clade VI strains resemble the variant also known as wMelCS.
Estimation of Strain Frequencies
SNP frequencies in all Pool-Seq samples at the previously detected marker sites were called with freebayes (parameters: -F 0.01 -C 2 –pooled-continuous). Given markers for n strains and a Pool-Seq sample with reference allele frequencies b at m marker sites, we estimate the n-vector of corresponding strain frequencies x by minimizing w|Ax-b| subject to constraints 0 < xj < 1 and ∑xj = 1 with the conjugate-gradient method, in which A is an m × n-matrix with columns containing 1 where the corresponding strain is marked by the reference allele and 0 otherwise, b an m-vector of called reference allele frequencies, and w an m-vector containing the coverage depth at the marker sites, serving as weights to the minimization procedure.
Ideally, only SNPs private to a strain (i.e., that have a multiplicity of 1) should be used to estimate the frequency of a given strain as the median over the frequencies of the strain-specific SNPs. However, private SNPs are not available for all strains. We thus use the most informative subset of marker sites large enough to differentiate among all strains, obtained by iteratively removing the marker sites with the highest multiplicity until the Shannon entropy (Shannon, 1948) per matrix row is maximized. This retains 75 of the 197 SNPs that differentiate Wolbachia strains, 120 of the 180 SNPs that differentiate clades, and all 155 SNPs that differentiate superclades, as well as all 21 mtDNA SNPs that differentiate mtDNA superclades. Since the median is the central point that minimizes the mean absolute deviation, our procedure is equivalent to median estimation when used with only private SNPs and equal weights (Stepniak, 2016). Code is provided in Supplementary Datasheet S4.
Confirmation of Wolbachia Infection Status
While Versace et al. (2014) demonstrated that all flies were infected with Wolbachia within less than 37 generations in either temperature regime, the infection could be lost at later generations. We therefore confirmed the infection status at the final generation (F100 in the cold, F180 in the hot environment) via PCR of at least 30 individual male flies per replicate. We extracted DNA using a salting-out procedure (Miller et al., 1988). To determine the Wolbachia infection status, we performed PCR using primers wsp81F (5′-TGGTCCAATAAGTGATGAAGAAAC-3′) and wsp691R (5′-AAAAATTAAACGCTACTCCA-3′) (Braig et al., 1998) resulting in a 630 bp fragment of the Wolbachia wsp gene. To rule out that the absence of a wsp PCR fragment was due to low quality DNA or suboptimal PCR conditions, we chose primers LV125-F (5′-GAGTCGGTTTCCCACAAAG-3′) and LV125-R (5′-GAGCACATCTACGAGTTTCC-3′) to amplify in parallel a 349 bp fragment of D. melanogaster DNA in the same PCR reaction. PCR amplifications were performed in 20 μl reaction volumes using 2.5 mM MgCl2, 0.2 mM dNTPs, 10 pmol of each primer, 0.4 U FIREPol Taq Polymerase in buffer B (Solis Biodyne, Tartu, Estonia), and ca. 10 ng genomic DNA. PCRs were run under the following conditions: 3 min at 94°C for initial denaturation followed by 32 cycles of 94°C for 30 s, 55°C for 30 s, 72°C for 50 s, and a final extension step of 72°C for 7 min. In a few samples with weak or absent amplification of the wsp PCR fragment, an additional PCR with Wolbachia-specific primers (wMel-clades_fw: 5′-CACTTTTCTGCTGCTGTTATAC-3′, wMel- clades_rv: 5′-AGAGGGTATTTATGGTAGCAAG-3′) was used with the same conditions to verify the presence or absence of Wolbachia.
Copy Number Estimation
We estimated Wolbachia and mitochondrial copy numbers from the coverage depth of the Wolbachia, or mitochondria, genome relative to the coverage depth of the Drosophila autosomes to account for read depth heterogeneity among libraries. Since the low GC content results in a systematic underestimation of read coverage, we corrected for GC bias by GC matching: all positions in the reference genome are assigned an effective GC content, defined as the average GC content of a DNA fragment that covers this position, and calculated as a weighted count of GC bases around the focal position, with weights constructed from the estimated read length and insert size distributions. Positions are then binned by GC content. The copy number is obtained as a weighted mean over GC bins of the relative coverage depths on the target contigs (wMel, mtDNA) and normalization contigs (all Drosophila autosomes), with weights mn/(m+n) accounting for the number of positions m on the target contig and n on the normalization contigs within each GC bin (Supplementary Datasheet S4). Copy numbers are given as copies per host cell.
Results
Based on 197 informative polymorphisms, we distinguished 13 distinct Wolbachia strains from 47 Wolbachia-infected isofemale lines. These 13 strains cluster into three major groups and can be assigned to clades I, III, V, and VI as defined by Richardson et al. (2012). No strain belonging to clade II or clade IV was identified (Figure 1).
Previous studies reported variation in copy number between different Wolbachia strains (Min and Benzer, 1997; Ijichi et al., 2002; Salzberg et al., 2005; Chrostek et al., 2013). Grouping the 47 samples into four clades confirmed pronounced differences. The highest copy number was seen in clade VI and the lowest in clade III. The copy numbers of clade V and VI, in particular, are clearly differentiated (Figure 2).
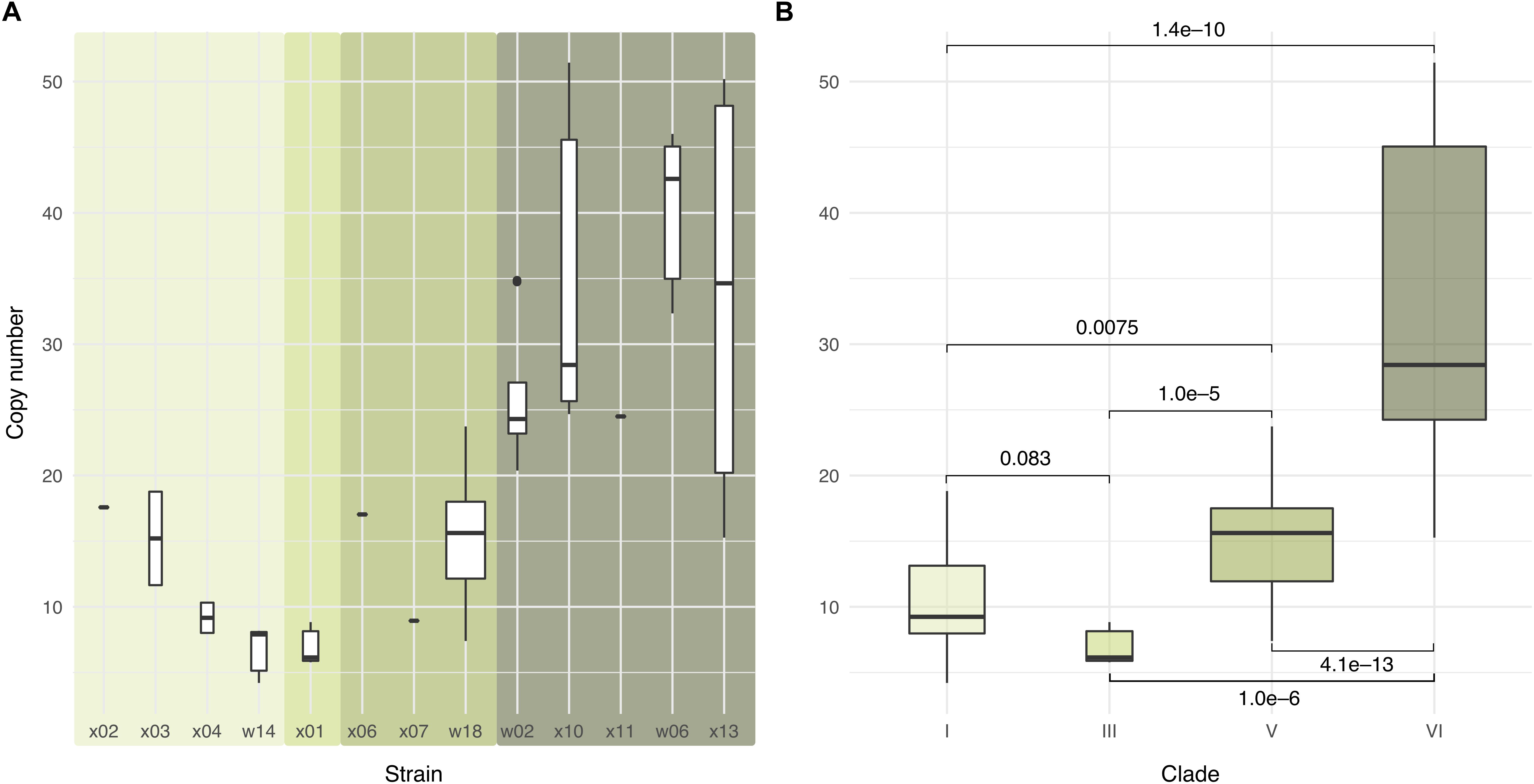
Figure 2. Wolbachia copy numbers differ substantially among strains. Box plots in the upper row show Wolbachia copy numbers in representative individuals from the 47 isofemale lines (A) by strain and (B) by clade, with boxes indicating the two middle quartiles of the distribution and whiskers extending to the largest or smallest value no further than 1.5 times the inter-quartile range from the hinge. Background shading in (A) indicates the clades depicted in (B). Brackets labels in (B) show the p-values from a two-sided Wilcox test for the null hypothesis of the same mean copy number (Supplementary Table S1, also for strains).
The relative frequency of the Wolbachia strains is obtained from Pool-Seq data by taking advantage of the strain/clade specific SNPs. Nevertheless, this method is not informative about the fraction of flies being infected. Versace et al. (2014) tested individual flies and found that at generation 37, all flies tested were infected. We confirm the infection status after long-term evolution (generations 100 in the cold and 180 in the hot environment). With at least 30 sampled flies from each replicate, we conclude that the infection status did not change between generation 37 and generations 100 or 180 (Supplementary Table S2).
Consistent with the results of Versace et al. (2014) we find that in the cold environment, clade V very quickly replaces the other Wolbachia clades (Figure 3, upper row). In the hot environment, a different pattern is observed. In all five replicates, clade VI is predominant during the first generations, as already noted in Versace et al. (2014). Starting around generation 70, however, the same clade V that dominates in the cold environment replaces the other Wolbachia genotypes (Figure 3, lower row, Supplementary Figure S1, and Supplementary Table S3). Owing to the delayed response, the anti-correlation between clade V and clade VI is a bit weaker in the hot environment (Supplementary Figure S2). This long-term behavior differs from expectations based on the results of Versace et al. (2014), who only studied the dynamics in the hot environment until generation 37.
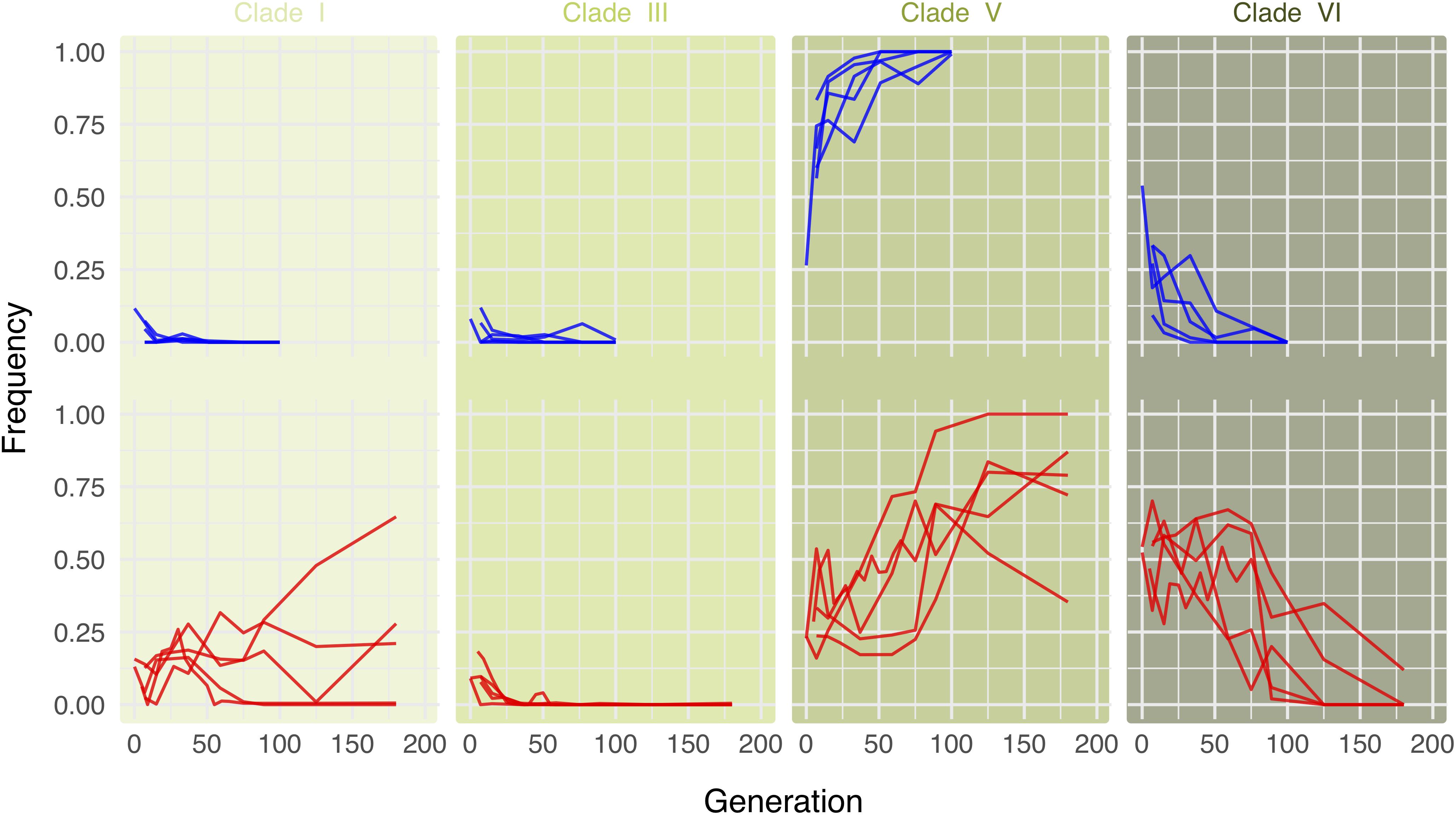
Figure 3. Long-term dynamics reveal that the same Wolbachia clades are successful in both hot and cold environments. Lines show clade frequencies over time for five replicates each in either the cold (upper row; blue) or the hot (lower row; red) environment, based on the most informative 120 of the 180 marker SNPs differentiating among clades. Clade V is consistently more successful in the long term in either environment.
The dynamics of Wolbachia strain turnover are also reflected in the mean Wolbachia coverage at the different time points. In the hot environment, we first observe an increase in coverage, which reflects mainly the increasing infection frequency. After generation 37, the copy number drops, reflecting the taking over of low-copy-number strains (Figure 4 and Supplementary Figure S3). In the cold, the infection frequency also increases, but unlike the hot environment, this does not result in a higher coverage, because the low-copy number strains predominate already at the early generations. Rather, we notice first a drop in coverage, followed by a recovery of the coverage as the entire population becomes infected with Wolbachia until generation 33 (Versace et al., 2014).
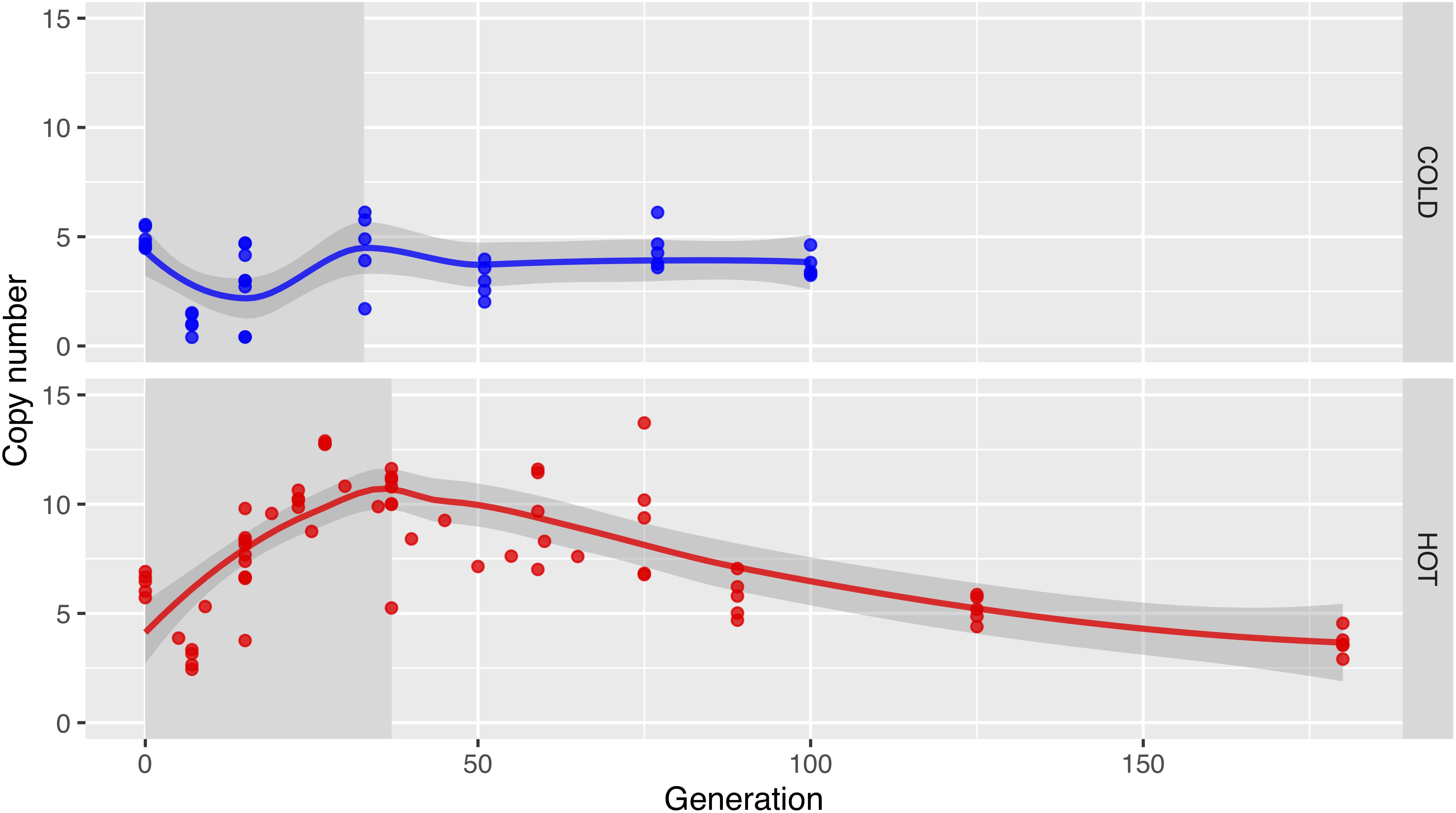
Figure 4. In the long term, Wolbachia copy numbers decrease in the hot environment. Dots show the Wolbachia copy numbers in replicates estimated with GC matching from the relative coverage depths in cold (upper row; blue) and hot (lower row; red) environments, the lines visualize the main trend obtained by Loess-smoothing. Only a fraction of the population was infected before generation 33 in the cold environment or generation 37 in the hot environment (gray background shading).
Given the high consistency of the phylogenetic relationship of mtDNA and Wolbachia seen in 290 melanogaster lines (Richardson et al., 2012), we expected that the Wolbachia dynamics are mirrored by the mtDNA dynamics. A direct comparison is, however, complicated by the lower number of SNPs in the mtDNA, resulting in a lower resolution and more noise. Therefore, we compared the dynamics on the level of super clades, as defined by Versace et al. (2014), which combines clade I, II, and III. Consistent with our expectation, we find an excellent overall correlation between Wolbachia and mitochondria (Supplementary Figure S4). We attribute the minor deviations to difficulties with an unambiguous clade assignment, rather than biological differences (Figure 5).
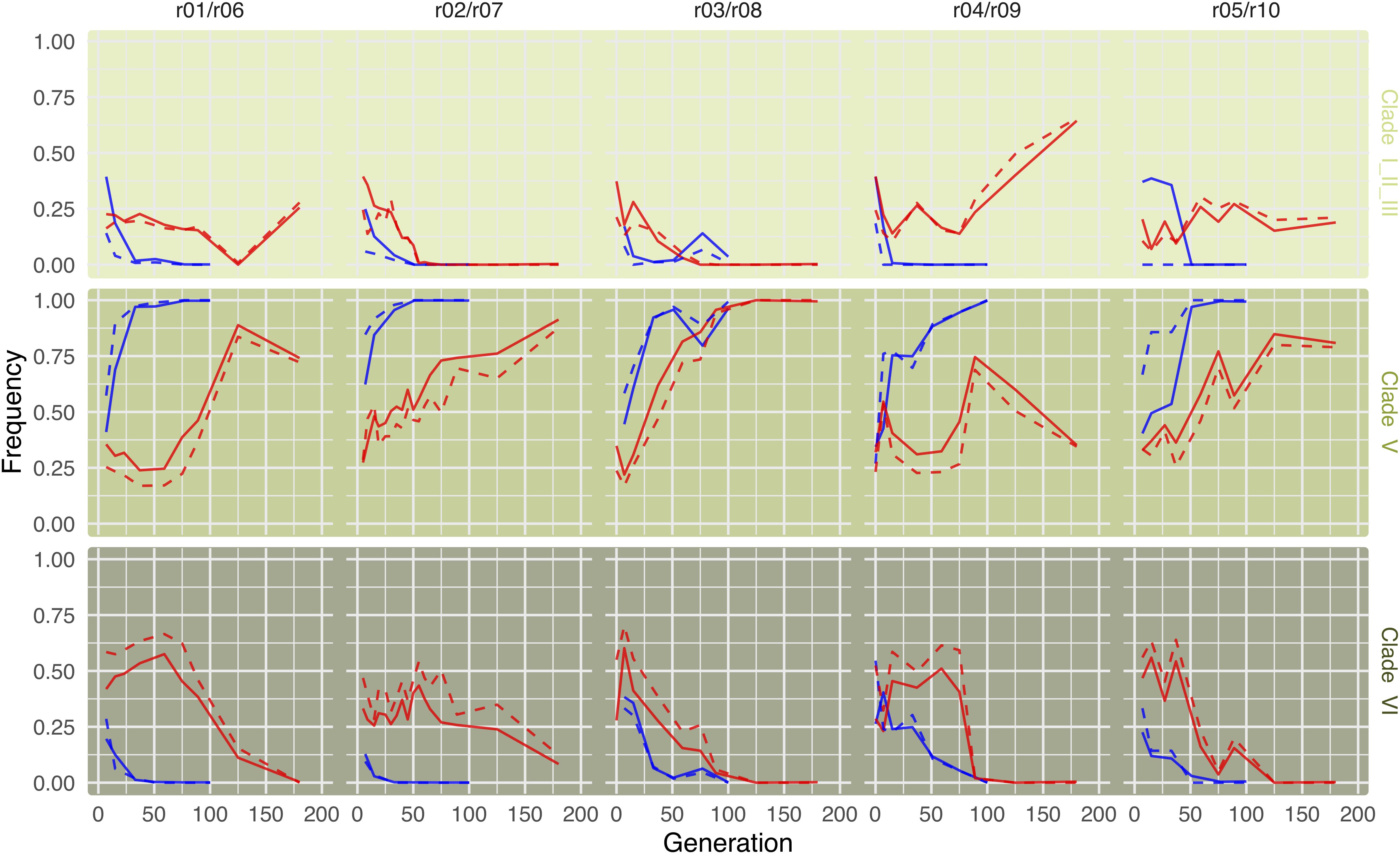
Figure 5. Mitochondrial dynamics on the superclade level are consistent with Wolbachia dynamics. Lines indicated superclade (as defined by Versace et al., 2014) frequencies for both mitochondria (solid lines) and Wolbachia (dashed lines) in cold (blue) and hot (red) environments. While frequencies are based on 155 marker SNPs differentiating among the three superclades, mitochondria frequencies are based on only 21 differentiating marker SNPs (owing to their smaller genome). The grouping of two replicates from different temperature treatments in a panel is random. All replicates evolved independently.
Like Wolbachia copy number, we also evaluated whether mtDNA copy numbers change during the experiment. Unlike Wolbachia, the mtDNA copy number is very stable. This observation is fully consistent with the very similar copy numbers in all strains analyzed (Figure 6).
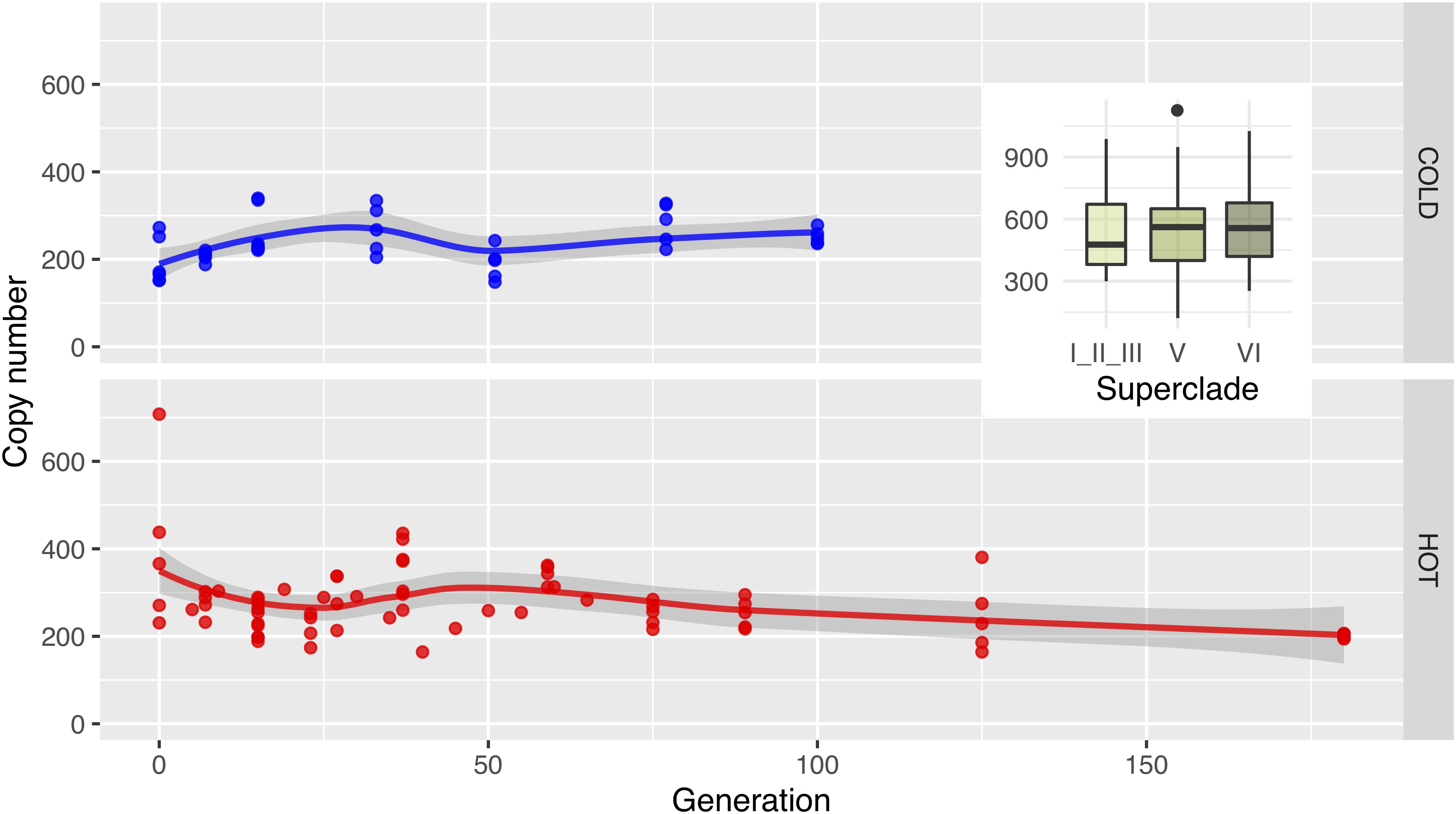
Figure 6. Mitochondrial copy numbers remain constant. Dots show the mitochondrial copy number for all replicates in cold (upper row; blue) and hot (lower row; red) environments, while the lines visualize the main trend via Loess-smoothing. Copy numbers were estimated with GC matching from the relative coverage depths. Boxplots in the insert show the copy numbers of mitochondria in the isofemale lines on superclade level (compare Figure 2; p-values from a Wilcox test in Supplementary Table S1).
Discussion
Compared to Versace et al. (2014), this study covers three advancements. First, we increased the number of replicates and show that the results of Versace were robust. Second, we provide a full SNP catalog of all Wolbachia strains in the experiment. This analysis showed that multiple different strains contribute to the clade specific dynamics previously described. Hence, Wolbachia strains belonging to the same clade are behaving similarly in their evolutionary response. Third, we increase the number of generations by more than fourfold. While the long-term dynamics in the cold environment do not change, we notice an interesting difference in the hot environment. While during the first generations the turnover of Wolbachia genotypes is rather modest, at later generations the same clade V that predominates in the cold cage outcompetes all other ones in the hot cage.
The temporal inconsistency of the evolutionary response cannot be explained by stochastic changes, as it is observed in all five replicates—albeit with different dynamics. The dynamics of the Wolbachia strains is not consistent with temperature being the only factor determining the frequency of the Wolbachia strains in the evolving replicates. Because the temperature regime is not changing over time, a consistent trend would have been expected. We observe, however, that the early phase differs from later time points. In the following, we will discuss some scenarios, which may explain the repeatable pattern of temporal heterogeneous Wolbachia dynamics.
The first hypothesis is that some uncontrolled environmental variables have changed during the experiment. This may include slight modifications in the food, due to different suppliers or modification in the maintenance protocol. As evolved flies are more fecund than ancestral ones from the base population (e.g., Barghi et al., 2019), egg laying time was reduced and larval density may also have changed.
The second hypothesis assumes that adaptation of the host affected the dynamics of the different Wolbachia strains, as has been shown for other stocks maintained in the laboratory (Correa and Ballard, 2014). Given that our experiment was designed to study the impact of temperature, adaptation of Drosophila to the new temperature regime may explain the dynamics. In a similar experiment, Drosophila simulans has been shown to have phenotypically converged at generation 60 (Barghi et al., 2019). Thus, it may be possible that different Wolbachia strains may be favored before and after the flies reaching trait optimum.
The third hypothesis is motivated by the observation that the evolved populations sometimes showed symptoms that are typical hallmarks of Drosophila C virus (DCV) infection (black, elongated, dying larvae and pupae; Ashburner and Roote, 2007). We propose that the dynamics may relate to the impact of Wolbachia copy number on host fitness in the presence of the DCV. A high Wolbachia copy number has been shown to be favorable in DCV infected flies when clade VI was compared to clades I, II, and III (Chrostek et al., 2013). Consistent with this, in a DCV-challenged population infected with clades I, II, III, and V, Wolbachia of the clade with the higher copy number (V) increased relative to clade I/III, but in the control population, no change was observed (Faria et al., 2016). A particularly interesting feature of our evolving populations is that, for the first time, two high-copy clades, V and VI, can be directly compared against each other. Because in our experiment clade VI has the highest copy number (Figure 2), this Wolbachia strain should provide the highest protection against DCV, but it is outcompeted by clade V. We attribute this apparent discrepancy to the fitness costs caused by high Wolbachia copy numbers (Fleury et al., 2000; Fry et al., 2004; Zhukova and Kiseleva, 2012; Chrostek and Teixeira, 2015; Martinez et al., 2015).
Another explanation for the increase of clade V is that if the Drosophila host responds to this DCV challenge by developing resistance, the advantage of the high copy Wolbachia may be diminished and another Wolbachia strain with lower copy number may take over. We addressed this hypothesis and analyzed the dynamics of two sequence variants, which confer DCV resistance in Drosophila (Martins et al., 2014) as an indicator of the resistance level of the Drosophila host. The resistance allele of the pastrel locus occurs at very low frequencies only and does not respond during the experiment (Supplementary Figure S5). The second resistance allele increases in some replicates, but not in all. While it is possible that other DCV resistance loci contribute, we do not have strong evidence for the Drosophila host developing DCV resistance during the experiment. This implies that if the evolving populations were challenged by DCV and developed strategies against DCV, this has been mainly achieved by changing the Wolbachia strain composition—a hypothesis that could be experimentally tested in future studies.
Finally, as a fourth hypothesis, Wolbachia may have adapted to their new environment by the acquisition of new mutations. We consider this highly unlikely because in all five replicates the same three Wolbachia strains increased in frequency in the hot environment at the later generations. This would require that all three, highly similar, strains independently acquired new mutations providing a fitness advantage. Furthermore, one would need to find additional explanations, such as epistasis, for the observation that only a single clade increases in frequency at the later generations. Finally, we did not detect new mutations in these strains that could explain the increase in fitness (data not shown).
Independent of the actual cause for the changes in Wolbachia dynamics, our study demonstrated that long-term experimental evolution may uncover evolutionary dynamics that remain unnoticed in short-term experiments. Particularly interesting would be further work to illuminate the influence of the host genotype on the observed Wolbachia dynamics.
As the hot environment was found to have short- and long-term dynamics, our experiments also highlight the difficulty in making predictions about the impact of temperature changes, thus providing a cautionary note on too simple interpretations on the impact of climate change.
Data Availability Statement
All short-read data used in this study are available from the European Nucleotide Archive (PRJEB37761), but have in parts also been made available earlier (see Supplementary Datasheet S2 for details). Variant data are included as Supplementary Datasheet S3.
Author Contributions
CS designed the study. VN conducted the experiments. TV performed a subset of the PCR measurements. RM performed the analysis. RM and CS wrote the manuscript. All authors contributed to manuscript revision and approved the submitted version.
Funding
This work was supported by the Austrian Science Funds (FWF, P27630 and W1225) and the European Research Council (ERC, ArchAdapt). Open-access funding was provided by the Austrian Science Fund.
Conflict of Interest
The authors declare that the research was conducted in the absence of any commercial or financial relationships that could be construed as a potential conflict of interest.
Acknowledgments
Illumina sequencing for a subset of the data was performed at the VBCF NGS Unit (www.viennabiocenter.org/facilities).
Supplementary Material
The Supplementary Material for this article can be found online at: https://www.frontiersin.org/articles/10.3389/fgene.2020.00482/full#supplementary-material
References
Ant, T. H., and Sinkins, S. P. (2018). A Wolbachia triple-strain infection generates self-incompatibility in Aedes albopictus and transmission instability in Aedes aegypti. Parasit. Vectors 11:295. doi: 10.1186/s13071-018-2870-0
Ashburner, M., and Roote, J. (2007). Maintenance of a Drosophila laboratory: general procedures. Cold Spring Harb. Protoc. 2007:pdb.ip35. doi: 10.1101/pdb.ip35
Baldo, L., Dunning Hotopp, J. C., Jolley, K. A., Bordenstein, S. R., Biber, S. A., Choudhury, R. R., et al. (2006). Multilocus sequence typing system for the endosymbiont Wolbachia pipientis. Appl. Environ. Microbiol. 72, 7098–7110. doi: 10.1128/AEM.00731-06
Barghi, N., Tobler, R., Nolte, V., Jakšiæ, A. M., Mallard, F., Otte, K. A., et al. (2019). Genetic redundancy fuels polygenic adaptation in Drosophila. PLoS Biol. 17:e3000128. doi: 10.1371/journal.pbio.3000128
Bi, J., Zheng, Y., Wang, R.-F., Ai, H., Haynes, P. R., Brownlie, J. C., et al. (2018). Wolbachia infection may improve learning and memory capacity of Drosophila by altering host gene expression through microRNA. Insect Biochem. Mol. Biol. 106, 47–54. doi: 10.1016/j.ibmb.2018.11.007
Bordenstein, S. R., and Bordenstein, S. R. (2011). Temperature affects the tripartite interactions between bacteriophage WO, Wolbachia, and cytoplasmic incompatibility. PLoS One 6:e29106. doi: 10.1371/journal.pone.0029106
Bourtzis, K., Nirgianaki, A., Markakis, G., and Savakis, C. (1996). Wolbachia infection and cytoplasmic incompatibility in Drosophila species. Genetics 144, 1063–1073.
Braig, H. R., Zhou, W., Dobson, S. L., and O’Neill, S. L. (1998). Cloning and characterization of a gene encoding the major surface protein of the bacterial endosymbiont Wolbachia pipientis. J. Bacteriol. 180, 2373–2378.
Breeuwer, J. A. J., and Werren, J. H. (1993). Cytoplasmic incompatibility and bacterial density in Nasonia vitripennis. Genetics 135, 565–574.
Calosi, P., Bilton, D. T., and Spicer, J. I. (2008). Thermal tolerance, acclimatory capacity and vulnerability to global climate change. Biol. Lett. 4, 99–102. doi: 10.1098/rsbl.2007.0408
Chen, I.-C., Hill, J. K., Ohlemuller, R., Roy, D. B., and Thomas, C. D. (2011). Rapid range shifts of species associated with high levels of climate warming. Science 333, 1024–1026. doi: 10.1126/science.1206432
Cheung, W. W. L., Lam, V. W. Y., Sarmiento, J. L., Kearney, K., Watson, R., and Pauly, D. (2009). Projecting global marine biodiversity impacts under climate change scenarios. Fish Fish. 10, 235–251. doi: 10.1111/j.1467-2979.2008.00315.x
Chrostek, E., Marialva, M. S. P., Esteves, S. S., Weinert, L. A., Martinez, J., Jiggins, F. M., et al. (2013). Wolbachia variants induce differential protection to viruses in Drosophila melanogaster: a phenotypic and phylogenomic analysis. PLoS Genet. 9:e1003896. doi: 10.1371/journal.pgen.1003896
Chrostek, E., and Teixeira, L. (2015). Mutualism breakdown by amplification of Wolbachia genes. PLoS Biol. 13:e1002065. doi: 10.1371/journal.pbio.1002065
Correa, C. C., and Ballard, J. W. O. (2014). What can symbiont titres tell us about co-evolution of Wolbachia and their host? J. Invertebr. Pathol. 118, 20–27. doi: 10.1016/j.jip.2014.02.009
Davis, M. B. (2001). Range shifts and adaptive responses to quaternary climate change. Science 292, 673–679. doi: 10.1126/science.292.5517.673
Dean, M. D., Ballard, K. J., Glass, A., and Ballard, J. W. O. (2003). Influence of two Wolbachia strains on population structure of East African Drosophila simulans. Genetics 165, 1959–1969.
Faria, V. G., Martins, N. E., Magalhães, S., Paulo, T. F., Nolte, V., Schlötterer, C., et al. (2016). Drosophila adaptation to viral infection through defensive symbiont evolution. PLoS Genet. 12:e1006297. doi: 10.1371/journal.pgen.1006297
Faria, V. G., Martins, N. E., Schlötterer, C., and Sucena, É (2018). Readapting to DCV infection without Wolbachia: frequency changes of Drosophila antiviral alleles can replace endosymbiont protection. Genome Biol. Evol. 10, 1783–1791. doi: 10.1093/gbe/evy137
Fleury, F., Vavre, F., Ris, N., Fouillet, P., and Boulétreau, M. (2000). Physiological cost induced by the maternally-transmitted endosymbiont Wolbachia in the Drosophila parasitoid Leptopilina heterotoma. Parasitology 121, 493–500. doi: 10.1017/S0031182099006599
Franssen, S. U., Nolte, V., Tobler, R., and Schlötterer, C. (2015). Patterns of linkage disequilibrium and long range hitchhiking in evolving experimental Drosophila melanogaster populations. Mol. Biol. Evol. 32, 495–509. doi: 10.1093/molbev/msu320
Fry, A. J., Palmer, M. R., and Rand, D. M. (2004). Variable fitness effects of Wolbachia infection in Drosophila melanogaster. Heredity 93, 379–389. doi: 10.1038/sj.hdy.6800514
Garrison, E., and Marth, G. (2012). Haplotype-based variant detection from short-read sequencing. ArXiv [Preprint]. arXiv:1207.3907.
Gómez-Sánchez, D., and Schlötterer, C. (2017). ReadTools: a universal toolkit for handling sequence data from different sequencing platforms. Mol. Ecol. Resour. 18, 676–680. doi: 10.1111/1755-0998.12741
Gruntenko, N. Å, Ilinsky, Y. Y., Adonyeva, N. V., Burdina, E. V., Bykov, R. A., Menshanov, P. N., et al. (2017). Various Wolbachia genotypes differently influence host Drosophila dopamine metabolism and survival under heat stress conditions. BMC Evol. Biol. 17:252. doi: 10.1186/s12862-017-1104-y
Hedges, L. M., Brownlie, J. C., O’neill, S. L., and Johnson, K. N. (2008). Wolbachia and virus protection in insects. Science 322, 702–702.
Hilgenboecker, K., Hammerstein, P., Schlattmann, P., Telschow, A., and Werren, J. H. (2008). How many species are infected with Wolbachia? – a statistical analysis of current data: Wolbachia infection rates. FEMS Microbiol. Lett. 281, 215–220. doi: 10.1111/j.1574-6968.2008.01110.x
Hiroki, M., Tagami, Y., Miura, K., and Kato, Y. (2004). Multiple infection with Wolbachia inducing different reproductive manipulations in the butterfly Eurema hecabe. Proc. R. Soc. Lond. B Biol. Sci. 271, 1751–1755. doi: 10.1098/rspb.2004.2769
Hoffman, A. A., and Turelli, M. (1997). “Cytoplasmic incompatibility in insects,” in Influential Passengers: Inherited Microorganisms and Arthropod Reproduction, eds S. L. O’Neill, A. A. Hoffmann, and J. H. Werren (Oxford: Oxford University Press).
Hoffmann, A. A., and Sgrò, C. M. (2011). Climate change and evolutionary adaptation. Nature 470, 479–485. doi: 10.1038/nature09670
Hurst, G. D. D., Jiggins, F. M., Schulenburg, J. H. G., von der, Bertrand, D., West, S. A., Goriacheva, I. I., et al. (1999). Male-killing Wolbachia in two species of insect. Proc. R. Soc. Lond. B Biol. Sci. 266, 735–740. doi: 10.1098/rspb.1999.0698
Ijichi, N., Kondo, N., Matsumoto, R., Shimada, M., Ishikawa, H., and Fukatsu, T. (2002). Internal spatiotemporal population dynamics of infection with three Wolbachia strains in the Adzuki bean beetle, Callosobruchus chinensis (Coleoptera: Bruchidae). Appl. Environ. Microbiol. 68, 4074–4080. doi: 10.1128/AEM.68.8.4074-4080.2002
Jia, F.-X., Yang, M.-S., Yang, W.-J., and Wang, J.-J. (2009). Influence of continuous high temperature conditions on Wolbachia infection frequency and the fitness of Liposcelis tricolor (Psocoptera: Liposcelididae). Environ. Entomol. 38, 1365–1372. doi: 10.1603/022.038.0503
Klausmeyer, K. R., and Shaw, M. R. (2009). Climate change, habitat loss, protected areas and the climate adaptation potential of species in mediterranean ecosystems worldwide. PLoS One 4:e6392. doi: 10.1371/journal.pone.0006392
Kofler, R., Langmüller, A. M., Nouhaud, P., Otte, K. A., and Schlötterer, C. (2016). Suitability of different mapping algorithms for genome-wide polymorphism scans with pool-seq data. G3 (Bethesda) 6, 3507–3515. doi: 10.1534/g3.116.034488
Kofler, R., Orozco-terWengel, P., De Maio, N., Pandey, R. V., Nolte, V., Futschik, A., et al. (2011). PoPoolation: a toolbox for population genetic analysis of next generation sequencing data from pooled individuals. PLoS One 6:e15925. doi: 10.1371/journal.pone.0015925
Li, H. (2011). A statistical framework for SNP calling, mutation discovery, association mapping and population genetical parameter estimation from sequencing data. Bioinformatics 27, 2987–2993. doi: 10.1093/bioinformatics/btr509
Li, H. (2013). Aligning sequence reads, clone sequences and assembly contigs with BWA-MEM. ArXiv [Preprint]. arXiv:1303.3997.
Li, H., Handsaker, B., Wysoker, A., Fennell, T., Ruan, J., Homer, N., et al. (2009). The sequence alignment/map format and SAMtools. Bioinformatics 25, 2078–2079. doi: 10.1093/bioinformatics/btp352
Maistrenko, O. M., Serga, S. V., Vaiserman, A. M., and Kozeretska, I. A. (2016). Longevity-modulating effects of symbiosis: insights from Drosophila–Wolbachia interaction. Biogerontology 17, 785–803. doi: 10.1007/s10522-016-9653-9
Marshall, D. J., McQuaid, C. D., and Williams, G. A. (2010). Non-climatic thermal adaptation: implications for species’ responses to climate warming. Biol. Lett. 6, 669–673. doi: 10.1098/rsbl.2010.0233
Martinez, J., Ok, S., Smith, S., Snoeck, K., Day, J. P., and Jiggins, F. M. (2015). Should symbionts be nice or selfish? Antiviral effects of Wolbachia are costly but reproductive parasitism is not. PLoS Pathog. 11:e1005021. doi: 10.1371/journal.ppat.1005021
Martins, N. E., Faria, V. G., Nolte, V., Schlotterer, C., Teixeira, L., Sucena, E., et al. (2014). Host adaptation to viruses relies on few genes with different cross-resistance properties. Proc. Natl. Acad. Sci. U.S.A. 111, 5938–5943. doi: 10.1073/pnas.1400378111
Mateos, M., Castrezana, S. J., Nankivell, B. J., Estes, A. M., Markow, T. A., and Moran, N. A. (2006). Heritable endosymbionts of Drosophila. Genetics 174, 363–376. doi: 10.1534/genetics.106.058818
Menzel, A., Sparks, T. H., Estrella, N., Koch, E., Aasa, A., Ahas, R., et al. (2006). European phenological response to climate change matches the warming pattern. Glob. Change Biol. 12, 1969–1976. doi: 10.1111/j.1365-2486.2006.01193.x
Miller, S. A., Dykes, D. D., and Polesky, H. F. (1988). A simple salting out procedure for extracting DNA from human nucleated cells. Nucleic Acids Res. 16, 1215–1215. doi: 10.1093/nar/16.3.1215
Miller, W. J. (2013). Bugs in transition: the dynamic world of Wolbachia in insects. PLoS Genet. 9:e1004069. doi: 10.1371/journal.pgen.1004069
Min, K.-T., and Benzer, S. (1997). Wolbachia, normally a symbiont of Drosophila, can be virulent, causing degeneration and early death. Proc. Natl. Acad. Sci. U.S.A. 94, 10792–10796. doi: 10.1073/pnas.94.20.10792
Mouton, L., Henri, H., Bouletreau, M., and Vavre, F. (2003). Strain-specific regulation of intracellular Wolbachia density in multiply infected insects. Mol. Ecol. 12, 3459–3465. doi: 10.1046/j.1365-294X.2003.02015.x
Noda, H., Koizumi, Y., Zhang, Q., and Deng, K. (2001). Infection density of Wolbachia and incompatibility level in two planthopper species, Laodelphax striatellus and Sogatella furcifera. Insect Biochem. Mol. Biol. 31, 727–737. doi: 10.1016/S0965-1748(00)00180-6
Novocraft (2018). Novoalign. Powerful Tool Designed for Mapping of Short Reads Onto a Reference Genome. Available online at: http://www.novocraft.com/products/novoalign/ (accessed June 17, 2019).
Olsen, K., Reynolds, K. T., and Hoffmann, A. A. (2001). A field cage test of the effects of the endosymbiont Wolbachia on Drosophila melanogaster. Heredity 86, 731–737.
Orozco-terWengel, P., Kapun, M., Nolte, V., Kofler, R., Flatt, T., and Schlötterer, C. (2012). Adaptation of Drosophila to a novel laboratory environment reveals temporally heterogeneous trajectories of selected alleles. Mol. Ecol. 21, 4931–4941. doi: 10.1111/j.1365-294X.2012.05673.x
Pandey, R. V., and Schlötterer, C. (2013). DistMap: a toolkit for distributed short read mapping on a hadoop cluster. PLoS One 8:e72614. doi: 10.1371/journal.pone.0072614
Petkau, K., Fast, D., Duggal, A., and Foley, E. (2016). Comparative evaluation of the genomes of three common Drosophila -associated bacteria. Biol. Open 5, 1305–1316. doi: 10.1242/bio.017673
Reynolds, K. T., and Hoffmann, A. A. (2002). Male age, host effects and the weak expression or non-expression of cytoplasmic incompatibility in Drosophila strains infected by maternally transmitted Wolbachia. Genet. Res. 80, 79–87. doi: 10.1017/S0016672302005827
Richardson, M. F., Weinert, L. A., Welch, J. J., Linheiro, R. S., Magwire, M. M., Jiggins, F. M., et al. (2012). Population genomics of the Wolbachia endosymbiont in Drosophila melanogaster. PLoS Genet. 8:e1003129. doi: 10.1371/journal.pgen.1003129
Rigaut, T. (1997). “Inherited microorganisms and sex determination of arthropod hosts,” in Influential Passengers: Inherited Microorganisms and Arthropod Reproduction, eds S. L. O’Neill, A. A. Hoffmann, and J. H. Werren (Oxford: Oxford University Press), 81–108.
Salzberg, S. L., Hotopp, J. C. D., Delcher, A. L., Pop, M., Smith, D. R., Eisen, M. B., et al. (2005). Serendipitous discovery of Wolbachia genomes in multiple Drosophila species. Genome Biol. 6:R23.
Schlötterer, C., Tobler, R., Kofler, R., and Nolte, V. (2014). Sequencing pools of individuals — mining genome-wide polymorphism data without big funding. Nat. Rev. Genet. 15, 749–763. doi: 10.1038/nrg3803
Shannon, C. E. (1948). A mathematical theory of communication. Bell Syst. Tech. J. 27, 379–423. doi: 10.1002/j.1538-7305.1948.tb01338.x
Somero, G. N. (2010). The physiology of climate change: how potentials for acclimatization and genetic adaptation will determine “winners” and “losers.” J. Exp. Biol. 213, 912–920. doi: 10.1242/jeb.037473
Stepniak, C. (2016). Interesting properties of variation characteristics. Aust. J. Stat. 39:341. doi: 10.17713/ajs.v39i4.254
The Broad Institute (2018). Picard. Picard. Available online at: http://broadinstitute.github.io/picard/ (accessed June 17, 2019).
Thuiller, W., Lavorel, S., Araujo, M. B., Sykes, M. T., and Prentice, I. C. (2005). Climate change threats to plant diversity in Europe. Proc. Natl. Acad. Sci. U.S.A. 102, 8245–8250. doi: 10.1073/pnas.0409902102
Thurmond, J., Goodman, J. L., Strelets, V. B., Attrill, H., Gramates, L. S., Marygold, S. J., et al. (2019). FlyBase 2.0: the next generation. Nucleic Acids Res. 47, D759–D765. doi: 10.1093/nar/gky1003
Tobler, R., Franssen, S. U., Kofler, R., Orozco-terWengel, P., Nolte, V., Hermisson, J., et al. (2014). Massive habitat-specific genomic response in D. melanogaster populations during experimental evolution in hot and cold environments. Mol. Biol. Evol. 31, 364–375. doi: 10.1093/molbev/mst205
Turner, T. L., Stewart, A. D., Fields, A. T., Rice, W. R., and Tarone, A. M. (2011). Population-based resequencing of experimentally evolved populations reveals the genetic basis of body size variation in Drosophila melanogaster. PLoS Genet. 7:e1001336. doi: 10.1371/journal.pgen.1001336
Versace, E., Nolte, V., Pandey, R. V., Tobler, R., and Schlötterer, C. (2014). Experimental evolution reveals habitat-specific fitness dynamics among Wolbachia clades in Drosophila melanogaster. Mol. Ecol. 23, 802–814. doi: 10.1111/mec.12643
Werren, J. H., Baldo, L., and Clark, M. E. (2008). Wolbachia: master manipulators of invertebrate biology. Nat. Rev. Microbiol. 6, 741–751. doi: 10.1038/nrmicro1969
Keywords: experimental evolution, evolve-and-resequence, strain frequency, copy number, microecology, microbe–host interaction, DCV resistance
Citation: Mazzucco R, Nolte V, Vijayan T and Schlötterer C (2020) Long-Term Dynamics Among Wolbachia Strains During Thermal Adaptation of Their Drosophila melanogaster Hosts. Front. Genet. 11:482. doi: 10.3389/fgene.2020.00482
Received: 15 November 2019; Accepted: 17 April 2020;
Published: 14 May 2020.
Edited by:
Margarida Matos, University of Lisbon, PortugalReviewed by:
Perran Ross, The University of Melbourne, AustraliaPieter Arnold, The Australian National University, Australia
Copyright © 2020 Mazzucco, Nolte, Vijayan and Schlötterer. This is an open-access article distributed under the terms of the Creative Commons Attribution License (CC BY). The use, distribution or reproduction in other forums is permitted, provided the original author(s) and the copyright owner(s) are credited and that the original publication in this journal is cited, in accordance with accepted academic practice. No use, distribution or reproduction is permitted which does not comply with these terms.
*Correspondence: Christian Schlötterer, Q2hyaXN0aWFuLlNjaGxvZXR0ZXJlckB2ZXRtZWR1bmkuYWMuYXQ=