- 1Centre for Soybean Research of the State Key Laboratory of Agrobiotechnology and School of Life Sciences, The Chinese University of Hong Kong, Shatin, China
- 2Department of Biotechnology, Chonnam National University, Yeosu, South Korea
Legumes are rich in secondary metabolites, such as polyphenols, alkaloids, and saponins, which are important defense compounds to protect the plant against herbivores and pathogens, and act as signaling molecules between the plant and its biotic environment. Legume-sourced secondary metabolites are well known for their potential benefits to human health as pharmaceuticals and nutraceuticals. During domestication, the color, smell, and taste of crop plants have been the focus of artificial selection by breeders. Since these agronomic traits are regulated by secondary metabolites, the basis behind the genomic evolution was the selection of the secondary metabolite composition. In this review, we will discuss the classification, occurrence, and health benefits of secondary metabolites in legumes. The differences in their profiles between wild legumes and their cultivated counterparts will be investigated to trace the possible effects of domestication on secondary metabolite compositions, and the advantages and drawbacks of such modifications. The changes in secondary metabolite contents will also be discussed at the genetic level to examine the genes responsible for determining the secondary metabolite composition that might have been lost due to domestication. Understanding these genes would enable breeding programs and metabolic engineering to produce legume varieties with favorable secondary metabolite profiles for facilitating adaptations to a changing climate, promoting beneficial interactions with biotic factors, and enhancing health-beneficial secondary metabolite contents for human consumption.
Introduction
Climate change, farmland deterioration, and the resulting food insecurity are major challenges facing the world. An increase in food supply is required to feed the expanding human population. The cultivation of high-yield crops has been used as a strategy to improve food supply. Grain legumes have been suggested as the potential solution to maintaining food and protein security (Considine et al., 2017). Legumes are also beneficial for sustainable agriculture due to the reduced release of greenhouse gases compared to other crops (Stagnari et al., 2017). Besides the beneficial effects on the improvement of soil fertility, legumes could enhance the resistance of soil to ecosystem disturbance, possibly due to the enhanced soil food web complexity (Gao et al., 2020). In agriculture, legumes are common candidates for crop rotation for promoting the growth of other crops such as cereals (Bagayoko et al., 2000; Uzoh et al., 2019). In addition, legumes produce unique secondary metabolites such as isoflavones, which are beneficial to human health (Gepts et al., 2005; Ku et al., 2020). Legumes are known to protect humans from chronic diseases, including cardiovascular diseases, diabetes, obesity, osteoporosis, or even cancer (Kushi et al., 1999; Al-Anazi et al., 2011). Based on the mode of consumption, legumes can be classified into four groups: oil seeds, pulses, vegetable crops, and feed crops (McCrory et al., 2010). Examples of oil seeds are soybean and peanut (McCrory et al., 2010). Pulses are legumes, which are exclusively harvested as dry seeds, such as chickpea, lentils, and peas. Green bean and garden pea are examples of vegetable crops while clover and alfalfa are examples of feed crops (McCrory et al., 2010). Human selection of legumes during domestication has resulted in the alteration, and even loss of diversity, of secondary metabolite contents in these crops, directly and indirectly through the selection pressure on the genes that control the production of secondary metabolites. Understanding the differences in secondary metabolites, and the underlying genetic differences, between the domesticated legume cultivars and their wild progenitors would promote the preservation of legume accessions, which possess the genes for the biosynthesis of beneficial secondary metabolites. This knowledge will facilitate breeding programs and metabolite engineering to produce legume crops with favorable traits for adapting to the changing climate and for human pharmaceutical/nutraceutical use.
Several Domestication-Related Traits Altered the Secondary Metabolite Contents
Domestication traits refer to morphological, biochemical, developmental, or physiological traits that are different between domesticated plants and their immediate wild progenitors (Abbo et al., 2014). A key part of domestication is the improvement of crop yield and harvestability compared to the wild progenitors (Dehaan et al., 2016). Several crop traits, including pod shattering, peduncle length, floral color, days to flowering, 100-seed weight, pod length, leaf length, leaf width, and seed number per pod, have been regarded as domestication-related traits (Lo et al., 2018).
Besides yield and harvestability related traits, other agronomic traits, such as seed size, appearance, and taste, are also subject to selection by breeders. These traits could be regarded as improvements due to post-domestication selection (Abbo et al., 2014). It has been suggested that the selection for larger seeds is related to facilitating single-seed planting (Kaplan, 1981). Breeders have also selected seeds of light colors. The ease of sowing and religious reasons have been proposed to be behind such conscious selections (Heiser, 1988). Therefore, seeds of modern legumes tend to have larger sizes and lighter colors compared to their wild counterparts. Moreover, the bitter taste of seeds has been intentionally eliminated through breeding (Muzquiz et al., 1994). Behind the loss of bitter taste is the loss of the corresponding bitter-tasting secondary metabolites such as alkaloids (Muzquiz et al., 1994).
During domestication, secondary metabolite compositions which facilitate cultivation and improve the appearance and taste of food grains were intentionally selected for by breeders. In some cases, the secondary metabolite composition may be unintentionally selected due to the close proximity of the genes or quantitative trait loci (QTLs) for secondary metabolite biosynthesis to those regulating other traits such as major nutrients and yield. The selection of favorable cultivation areas and the protection by breeders during crop growth limit natural selection pressures due to abiotic and biotic stresses. Domestication brings forth better yield, better taste, and better appearance but also reduces the availability of secondary metabolites in legumes. As a result, domesticated legumes are usually less resistant to biotic stresses compared to their wild counterparts (Muzquiz et al., 1994; Pavan et al., 2016; Bazghaleh et al., 2018; Abraham et al., 2019). The reduced availability of health-beneficial secondary metabolites (Muzquiz et al., 1994; Wang et al., 2010; Fernández-marín et al., 2014; Kaur et al., 2019) also limits the potential of legumes as sources of bioactive compounds for pharmaceutical use. For the growth of the legume plants, the loss of the secondary metabolites in modern cultivars possibly renders the plants more susceptible to abiotic stress and biotic stress. The importance of the secondary metabolites to combating these stresses will be introduced in section “The Roles of Secondary Metabolites in Combating Abiotic and Biotic Interactions.”
Introduction to Secondary Metabolites in Legumes
Definition of Plant Secondary Metabolites
Secondary metabolites are organic compounds derived from primary metabolism that serves key roles in defense and signaling in plants. They contribute to adaptive traits and ecological fitness, including defense mechanisms, tolerance to abiotic/biotic stresses, and interactions with insect pollinators, root-associated microbes, and herbivores. In contrast, primary metabolites are essential for cellular functions, such as growth, development, and reproduction. For example, secondary metabolites can attract insects for pollination or symbiotic rhizobia for nitrogen-fixing nodule formation. They can also be part of the defense mechanisms against herbivores, disease-causing bacteria, fungi, viruses, and parasites. There are also a wide range of secondary metabolites with pharmaceutical, nutraceutical, and toxicological values for humans (Wink, 2013). The contents of secondary metabolites vary among different plant species (Böttger et al., 2018). Legumes are rich in secondary metabolites, such as polyphenols, alkaloids, and saponins (Gupta, 1987).
The Health Benefits of Secondary Metabolites From Legumes
In the recent past, many secondary metabolites in legumes were considered non-nutritive. For example, tannins, glycosides, alkaloids, and saponins affect the digestibility of beans (Gupta, 1987). However, more and more evidence suggests there are health benefits from the secondary metabolites of legumes (Dixon and Sumner, 2003). The health benefits of carotenoids, polyphenols, alkaloids, and saponins, all abundant in legumes, are discussed below and summarized in Table 1.
Carotenoids
Carotenoids are a type of tetraterpenoids, ranging from bright yellow and orange to red, found in algae, photosynthetic bacteria, and plants, including carrot, pumpkin, tomato, sweet potato, and papaya. They can be classified into two groups, carotenes and xanthophylls (Roberts et al., 2009). Xanthophylls differ from carotenes by having oxygenated substituents in their molecules (Roberts et al., 2009). Carotenoids with unsubstituted β-rings, including α-carotene, β-carotene, and β-cryptoxanthin, act as provitamin A (Roberts et al., 2009). The carotenoid compositions in various legume seeds have been previously summarized (Tee et al., 1995). Lutein and zeaxanthin constitute the macular pigments in the retina of the mammalian eye. The oxygenated nature of the lutein and zeaxanthin molecules provides antioxidative protection for the eye from damage by free radicals (Roberts et al., 2009). The prevention of age-related macular degeneration by the consumption of carotenoid-rich foods has been recommended (Bernstein et al., 2016).
Polyphenols
Polyphenols are the major determinants of tissue colors, and generally possess antioxidative activities (Abbas et al., 2017). Polyphenols in plants can be classified into two groups: phenolic acids and flavonoids (Abbas et al., 2017). The occurrence and health benefits of phenolic acids in grain legumes have been previously summarized (Singh et al., 2017). Flavonoids are classified into several sub-classes: flavones, flavonols, flavanones, flavanonols, anthocyanins, flavanols, and isoflavones (Ku et al., 2020). Among flavonoids, isoflavones are only found in legumes. Flavonoids have multiple functions in plants, for example, mediating the responses to biotic and abiotic stresses, controlling the transport of auxins, acting as UV radiation-absorbing pigments to protect the plant against UV damage, attracting pollinating insects, interacting with rhizobia to initiate nodulation for symbiotic nitrogen fixation, and regulating defense against pathogens and herbivores through phytoalexin activities (Kumar and Pandey, 2013). For human health, it has been reported that flavonoids can act as protectants against cellular oxidation, inflammation, viral infections, and cancer (Kleemann et al., 2011). The molecular mechanisms of the health benefits of flavonoids have been recently reviewed (Ku et al., 2020).
Alkaloids
Alkaloids are nitrogen-containing organic heterocyclic compounds that are biologically active. Many alkaloids have pharmaceutical properties. For example, some alkaloids were found to have anti-malarial activities (Onguéné et al., 2013), anticancer activities (Gupta et al., 2015), and abilities to facilitate blood circulation in the brain and to prevent stroke (Kumar and Khanum, 2012). Moreover, several studies reported that alkaloids have potential therapeutic effects on neurodegenerative diseases, such as Alzheimer’s disease, Parkinson’s disease, and Huntington disease (Amirkia and Heinrich, 2014).
Saponins
Saponins are a group of terpenoids found in plants, including onion, ginger, garlic, ginseng, fenugreek, and legumes (Oakenfull, 1981; Sauvaire et al., 1996). These crops are important sources of saponins in the human diet (Oakenfull, 1981; Sauvaire et al., 1996). Chickpea, soybean, lentils, peanut, garden pea, broad bean, and alfalfa are rich in saponins (Oakenfull, 1981). The antibacterial and foaming properties of saponins led to the use of saponins as vaccine adjuvants (Marciani, 2018). In the human body, saponins can bind to bile salts to reduce cholesterol absorption (Marrelli et al., 2016). Moreover, in rats, it was shown that a saponin-rich diet resulted in the reduction of body weight, total cholesterol, triglycerides, very-low-density lipoproteins (VLDL), and low-density lipoproteins (LDL) in serum (Latha et al., 2011; Reddy et al., 2012). Alfalfa saponin extract (ASE) was found to have cholesterol-lowering effects (Wang et al., 2011; Marrelli et al., 2016). The treatment of rats with ASE led to the enhanced expression of cholesterol 7-alpha-hydroxylase (Cyp7a1), an enzyme involved in the bile acid biosynthetic pathway in the livers of hyperlipidemic rats (Marrelli et al., 2016). Besides, ASE treatment also enhanced the expression of low-density lipoprotein receptor (Ldlr), which promotes the uptake and clearance of LDL cholesterol in plasma (Marrelli et al., 2016). Moreover, saponins also have anti-microbial and antioxidant properties, and exhibit cancer-related immunomodulatory effects (Avato et al., 2006).
The Roles of Secondary Metabolites in Combating Abiotic and Biotic Interactions
Polyphenols
Plant roots communicate actively with the soil microbes for mutualistic cycles. Flavonoids are important signaling molecules for the legume-microbe interactions. The ability to form nitrogen fixing nodules with rhizobia is a unique characteristic of legumes (Hirsch et al., 2001). Such mutualism between legume and rhizobium is initiated by flavonoids. Flavonoids released from roots attract rhizobia to migrate toward the roots and stimulate the nod genes, which are essential genes to synthesize Nod factors for infecting the plants (Spaink, 1995). Flavonoids in the root exudates of various legumes for attracting rhizobia have been summarized in a previous review (Haldar and Sengupta, 2015). Moreover, flavonoids stimulate the germination of mycorrhizal fungus spores and enhance hyphal growth (Abdel-lateif et al., 2012). Mycorrhizal fungi form hyphae which penetrate plant roots for the transport of nutrients in rhizosphere to the host plant (Harrison, 2005).
The importance of polyphenols to combating abiotic stress has been discussed in recent reviews (Di Ferdinando et al., 2014; Isah, 2019; Sharma et al., 2019). The antioxidating characteristics of polyphenols help alleviate the oxidative stress brought forth by abiotic stress (Di Ferdinando et al., 2014; Isah, 2019; Sharma et al., 2019). A recent method for screening legume crops for abiotic stress tolerance suggested the accumulation of anthocyanin, which is also an osmolyte, as one of the indicators of abiotic stress tolerance of legume crops (Sinha et al., 2020).
Strigolactones
Based on the molecular structure, strigolactones belong to a group of lactone, which is derived from carotenoid (Jia et al., 2018). Functionally, strigolactones are plant hormones that are released by roots to attract symbiotic arbuscular mycorrhizal fungi and induce the germination of parasitic weed seeds (Jia et al., 2018). Strigolactones have been identified from a broad range of legumes, including Arachis hypogaea, Astragalus sinicus, Cicer arietinum, Glycine max, Lupinus albus, Medicago sativa, Phaseolus vulgaris, Pisum sativum, Psophocarpus tetragonolobus, Trifolium incarnatum, Vicia faba, and Vigna angularis (Yoneyama et al., 2008).
A study showed that the expression of several secretory proteins of Rhizophagus irregularis, an arbuscular mycorrhizal fungus, was induced by strigolactone treatment (Tsuzuki et al., 2016). Among these proteins, Strigolactone-Induced Putative Secreted Protein 1 (SIS1) showed the highest induction fold by both strigolactone treatment and Medicago truncatula root symbiosis. SIS1 is important for colonization and the formation of stunted arbuscules (Tsuzuki et al., 2016). Therefore, the strigolactone-induced is an essential protein for the symbiosis (Tsuzuki et al., 2016).
Broomrapes, especially Orobanche crenata, are believed to be the major parasitic weeds of legumes. The effects of the parasitic weeds on legumes include local damage of the plants and yield loss (Rubiales and Fernández-Aparicio, 2012). The germination of Orobanche seeds is induced by strigolatones (Yoneyama et al., 2008).
Alkaloids and Saponins
Alkaloids and saponins are known for contributing to the bitter taste of plants (Drewnowski and Gomez-Carneros, 2000). The toxicity of alkaloids has been reported (Wink, 2013). Several studies report that alkaloids and saponins are related to the resistance to herbivores. For example, yellow lupin cultivar with higher level of alkaloids in the leaves is more resistant to aphid than the cultivar with lower level of alkaloids (Adhikari et al., 2012). The removal of the bitter taste from modern lupin cultivars has enabled them to be a protein source in animal feed to reduce the dependence on soybean (Abraham et al., 2019). However, “sweet” lupins are more susceptible to predators (Muzquiz et al., 1994). Saponins have been thought to be responsible for the resistance to insect attacks, as the saponin preparation garden pea (Pisum sativum L.) resistant to Azuki bean beetle (Callosobruchus chinensis L.) inhibited the development of the beetle (Applebaum et al., 1969).
Alterations of Secondary Metabolite Profiles in Legumes During Domestication
Polyphenols and Carotenoids Determine the Colors of Seeds and Flowers
The seed coat color is mainly determined by polyphenols such as tannins (Heiser, 1988; Espinosa-Alonso et al., 2006). It is common for the pigmentation patterns of domesticated crops to be altered compared to their wild relatives. The loss of pigment in the seed coat of cultivated P. vulgaris is an obvious example of the effects of domestication (McClean et al., 2018). In a survey of 18 Lablab purpureus (L. purpureus) germplasms, including wild, semi-domesticated, and cultivated accessions, it was found that all the wild accessions have gray-brown and mottled seed coat (Maass, 2006). However, cultivated accessions display a spectrum of seed coat colors, including cream-white, cream, tan, and black (Maass, 2006). Unlike the wild accessions, some cultivated accessions do not have mottled seed coats (Maass, 2006). Among 11 landraces and two cultivated accessions of peanut (Arachis hypogaea L.), it was found that all the cultivated accessions have a single seed coat color: tan (Husain and Mallikarjuna, 2012), while the landraces are either red or tan (Husain and Mallikarjuna, 2012). Some landraces even have variegated seed coats (Husain and Mallikarjuna, 2012). In another study, it was shown that cultivated peanut (A. hypogaea) could have purple, brown, red, or white seed coats and some have variegated seed coats (Bertioli et al., 2011). In a survey of a soybean population consisting of 1,957 domesticated and 1,079 wild accessions, it was found that almost all wild accessions have purple flowers and black seed coats (Jeong et al., 2019), whereas the domesticated soybean accessions have more diverse seed coat colors, including colorless (yellow or green seeds), brown, or black, and more diverse floral colors, including white or purple (Jeong et al., 2019). In another study on 110 cultivated, 130 landrace, and 62 wild soybean accessions, it was reported that all cultivated accessions have yellow seeds, and landrace accessions have yellow, green, brown, or black seeds, while all the wild accessions have black seeds (Wang et al., 2018). Similarly, the modern cultivated pea cv. Cameor (P. sativum) has transparent seed coat while the wild accession (P. sativum subsp. elatius JI64) has pigmented seed coat (Smýkal et al., 2014). In another study on cultivated (Lens culinaris ssp. Culinaris) and wild lentils (Lens culinaris ssp. orientalis, L. culinaris ssp. odemensis, L. culinaris ssp. tomentosus, Lens nigricans, and Lens ervoides, Lens lamottei), although wild accessions do not necessarily have darker seed coats, wild accessions have more complexed patterns on the seed coats (Singh et al., 2014). The seed coats of the cultivated accessions have either no or dotted patterns (Singh et al., 2014). However, many of the wild accessions have marbled pattern on seed coats (Singh et al., 2014). For chickpea (C. arietinum), the light color of the cultivated seeds is thought to be non-existing in wild accessions (Penmetsa et al., 2016). The seed coat color is related to the defense against herbivore. It has been suggested that a black seed coat protects the seed from night-time foragers (Porter, 2013).
Polyphenols also give rise to the colors of flowers (Wiesner et al., 2017). In cowpea, cultivated accessions have a wide range of floral colors while most of the wild accessions have only purple flowers (Lo et al., 2018). Similarly, cultivated soybean accessions have purple, white, or other colors of flowers (Sundaramoorthy et al., 2015; Jeong et al., 2019), whereas most of the wild soybean accessions have only purple flowers (Sundaramoorthy et al., 2015). On the contrary, in common bean, most of the cultivated accessions have only white flowers while the wild accessions have white, pink, or purple flowers (García et al., 1997). Cultivated lentils (L. culinaris ssp. Culinaris) have white or purple flowers but some wild lentils, L. culinaris ssp. odemensis, L. culinaris ssp. tomentosus and Lentil ervoides, have only purple flowers (Singh et al., 2014). The white flowers of cultivated chickpea (C. arietinum) is thought to be non-existing in wild accessions (Penmetsa et al., 2016). For pea, cultivated peas (P. sativum) usually have white flowers while purple flowers are found in wild peas (Hellens et al., 2010). The contrasting flower colors contributed to the establishment of the Mendel’s Laws.
The Co-Evolution of Seed and Floral Colors With Foragers and Pollinators
As discussed above, cultivated legumes usually have lighter seed coat colors compared to the wild counterparts. During domestication, light seed coat colors have been preferred by farmers. The loss of color is associated with the loss of secondary metabolites, such as tannins (Heiser, 1988). As mentioned before, a dark seed coat may protect the seeds from night-time foragers in the wild (Porter, 2013). However, the potential increase in loss of sown seeds to wild animals may not be significant as farmers usually have measures to keep foragers away from crops. Another example is the loss of bitter compounds, such as alkaloids and saponins in domesticated legumes. The loss of such compounds would have enhanced the loss of seeds due to foraging by animals and is usually not advantageous for the survival of the crops without the protection provided by farmers. Therefore, the loss of bitter compounds in domesticated legumes is also known as a conscious selection by breeders during domestication. In soybean, most of the elite cultivated soybean seeds are yellow. It was found that the stay-green G gene is associated with green seeds and it controls seed dormancy, but is lost in elite cultivated soybean seeds (Wang et al., 2018). In the survey of 110 cultivated, 130 landrace, and 62 wild soybean accessions, it was found that the G genotype is present in only 4% of the cultivated accessions, 21% of the landraces while it is found in 100% of the wild accessions (Wang et al., 2018).
It has been suggested that floral color has co-evolved with pollinators such as birds and bees. Bees tend to be attracted to yellow flowers while birds tend to prefer red flowers due to their different visual sensitivities (Toon et al., 2014). Bee-pollinated plants usually have yellow, white, or blue flowers while bird-pollinated plants usually have red flowers (Toon et al., 2014). The transition from bee-pollination to bird-pollination of Australian egg-and-bacon pea is related to the number of bird species in the geographical region where the plants grow (Toon et al., 2014). The yellow color of the Lotus flower, together with the orientation, size, petal morphology, sucrose-dominant nectar composition, and scent of the flower, was reported as a factor contributing to the transition to pollination by birds (Cronk and Ojeda, 2008).
Carotenoid Level Is Related to Seed Dispersal by Animals
Besides polyphenols, carotenoids also play a role in determining tissue colors. During domestication, the profitability of seeds is a major concern for farmers. Therefore, genotypes with reduced seed dispersal, including through pod shattering and seed dispersal by animals, were actively selected for by breeders and farmers. In a study on the seeds of 10 legume genera: Arachis (peanut), Cicer (chickpea), Glycine (soybean), Lathyrus (vetch), Lens (lentil), Lupinus (lupin), Phaseolus (bean), Pisum (pea), Vicia (fava bean), and Vigna (cowpea), drastic changes in the levels and compositions of carotenoids in seeds were found in domesticated cultivars compared to their wild counterparts (Fernández-marín et al., 2014). An average of 48% reduction in carotenoids was found in the seeds of these 10 legumes. Besides, the compositions of carotenoids were more complex in the wild species of Cicer, Glycine, Lathyrus, Lens, Lupinus, and Vigna. In the study, neoxanthin, violaxanthin, lutein epoxide, and antheraxanthin were only found in the wild species but not the domesticated varieties. It was suggested that seeds with lower carotenoid levels are less attractive to seed dispersers (Fernández-marín et al., 2014). In contrast, attracting seed dispersers has been suggested to be an adaptation of wild legumes (Brǿnnvik and von Wettberg, 2019). It was suggested that seed dispersal by birds is an important factor contributing to the widespread of P. vulgaris from Mexico to South America (Brǿnnvik and von Wettberg, 2019).
Isoflavones Are Unique to Legumes
Isoflavones are a sub-class of flavonoid uniquely found in legumes. Soybean is a rich and common source of isoflavones for human consumption (Ku et al., 2020). In a study of seed isoflavone contents using 209 wild, 580 landrace, and 106 cultivated soybean accessions, it was found that landraces had the highest average level of total seed isoflavone, followed by wild accessions and then cultivated accessions (Wang et al., 2010). The higher average total seed isoflavone content in landraces compared to cultivated accessions was also reported in another study using 927 landraces and 241 cultivars (Azam et al., 2020). For individual isoflavone contents, it was suggested that high genistin and glycitin contents, with low daidzin levels, were artificially selected for. The significantly lower daidzin contents lead to the lower average total seed isoflavone levels in cultivated accessions compared to wild accessions (Wang et al., 2010). There are debates over the reasons behind the artificial selection of such seed isoflavone traits in domesticated legumes. Regarding seed nutrient content, a negative correlation between the total isoflavone level and the protein level has been reported in seeds (Primomo et al., 2005; Morrison et al., 2008; Liang et al., 2010; Smallwood et al., 2014), and a positive correlation between total seed isoflavone level and seed oil level has also been reported (Morrison et al., 2008; Liang et al., 2010). However, there has also been a report on the negative correlation between total seed isoflavone level and seed oil level (Smallwood et al., 2014). The total seed isoflavone has also been correlated to yield (Primomo et al., 2005; Smallwood et al., 2014; Zhang et al., 2014), as well as the resistance against pathogens (Carter et al., 2018). When two soybean cultivars, RCAT1004 and DH4202, which are resistant and sensitive to cyst nematodes respectively, were grown in a cyst nematode-infested environment, the resistant cultivar had a higher seed isoflavone level (Carter et al., 2018). A putative QTL related to cyst nematode susceptibility was found close to that related to total seed isoflavone content (Carter et al., 2018). During domestication, besides the deliberate selection for reduced seed isoflavone level to reduce the bitterness of the seed, the isoflavone level may also be unintentionally selected together with other desirable traits, such as nutrient composition, yield, and resistance to biotic stress.
Alkaloid and Saponin Contents Are Related to Taste-Focused Breeding
The bitter taste of legume seeds tends to be eliminated during domestication. For example, domesticated lupin cultivars are less bitter than the wild relatives, which have significantly higher levels of alkaloids in their seeds. Modern lupin cultivars are referred to as “sweet” lupins. In a survey of 20 sweet lupins and 29 bitter lupins, the bitter taste of lupins was found to be positively correlated to the seed alkaloid content, with lupanine being the main alkaloid (Muzquiz et al., 1994). Although seed saponin level has been correlated to the bitterness of seeds in general (Mohan et al., 2016), it may not be related to the bitterness of lupin seeds. In a survey of the seed saponin contents in sweet vs. bitter lupins, the level of saponin was undetectable in the seeds of both sweet and bitter varieties of L. albus (Shim et al., 2003).
Saponin is also a contributing factor to the bitterness of seeds (Okubo et al., 1992). It was found that many of the wild ancestors of Vigna spp. are more resistant than their cultivated counterparts to Callosobruchus chinensis or Callosobruchus maculatus (Tomooka et al., 2000). It is possible that the drop in saponin contents when legumes became domesticated is related to the loss of insect resistance capability in cultivated species. Several wild chickpea accessions have higher seed saponin levels than cultivated chickpea accessions (Kaur et al., 2019). Several wild pigeonpea (Cajanus scarabaeoides) accessions have higher seed saponin contents than the cultivated pigeonpea accessions (Cajanus cajan; Sekhon et al., 2017). However, cultivated pigeonpea accessions do not necessarily have lower seed saponin contents than the wild accessions (Sekhon et al., 2017). Seed saponin content is not the sole factor leading to the insect resistance of legumes.
Besides total seed saponin content, individual saponin components in legumes are also studied. Saponins can be classified into four groups: group A saponins, group B saponins, group E saponins, and 2,3-dihydro-2,5-dihydroxy-6-methyl-4H-pyran-4-one (DDMP) saponins (Sawai and Saito, 2011; Krishnamurthy et al., 2013). The aglycone form of group A saponins is named soyasapogenol A, while that of DDMP saponins is named soyasapogenol B. The basic structure of soyasapogenol A and B is β-amyrin. Soyasapogenol A is a β-amyrin with a hydroxyl group at C-21, C-22, and C-24, while soyasapogenol B has a hydroxyl group at C-22 and C-24 only (Sawai and Saito, 2011). DDMP saponins are relatively unstable and are often degraded into group B and group E saponins during food processing (Sundaramoorthy et al., 2019). Among the various groups of saponins, group A saponins, which have an acetylated oligosaccharide chain attached to C-22 of soyasapogenol A, are thought to be mostly responsible for the undesirable taste of soybean seeds (Shiraiwa et al., 1991).
In a survey of saponin compositions among 800 cultivated soybean accessions and 329 wild soybean accessions, it was found that the saponin type Aa was predominant in cultivated soybean accessions, while the saponin type AaBc was predominant in wild soybean accessions (Tsukamoto et al., 1993). In another survey of the total seed saponin levels in 17 wild and one cultivated legumes, it was found that the total saponin level was highest in Glycine soja (G. soja; wild soybean; Shim et al., 2003). In a study of seed saponin composition of 3,025 G. soja accessions, diverse compositions of seed saponins were found among the accessions (Krishnamurthy et al., 2013). Moreover, naturally occurring wild soybean mutants that lack group A saponins were found (Krishnamurthy et al., 2013; Takahashi et al., 2016; Rehman et al., 2018). Wild legumes do not necessarily have higher seed saponin contents. Instead, the diverse genetic backgrounds among wild legumes allow the discovery of novel allelic forms for desirable seed saponin compositions.
Polyphenols and Strigolactones Are Related to Biotic Interactions
Flavonoids are signaling molecules for legume-microbe interaction (Abdel-lateif et al., 2012). In a test of nodulating capability of Rhizobium japonicum (R. japonicum), it was found that all the strains of R. japonicum in the test could nodulate cowpea, sirato, and wild soybean (Heront and Pueppket, 1984). However, nine out of the 11 strains could not form infection threads with two of the three commercial soybean cultivars in the test (Heront and Pueppket, 1984). In another study, after inoculating 36 G. soja (wild soybean) accessions with R. japonicum, 20 formed normal nodules while 16 could not form nodules or formed abnormal nodule-like structures (Pueppke et al., 1998). It was hypothesized that the different nodulating phenotypes were due to the different flavonoid profiles in the root exudates (Pueppke et al., 1998). However, the flavonoid profiles of root exudates are similar between the nodulating group and the non-nodulating group (Pueppke et al., 1998). The flavonoid profiles of root exudates were also compared between wild soybean accessions and the cultivated soybean Peking (Pueppke et al., 1998). Although many of the wild soybeans showed a more complexed root exudate profile, a strong correlation between the different root exudates and the nodulating phenotypes was not found (Pueppke et al., 1998). The effects of domestication on the flavonoid profiles in legume root exudates remain unclear. On the other hand, the root polyphenol compositions of wild lentil (Lens ervoides) and cultivated lentil (Lens culinaris) were compared after the infection of Aphanomyces euteiches, which is a legume pathogen (Bazghaleh et al., 2018). The wild lentil was more tolerant to A. euteiches than the cultivated lentil pathogen (Bazghaleh et al., 2018). The wild lentil generally had higher levels of polyphenols compared to the cultivated lentil (Bazghaleh et al., 2018). Although the amount of legume species and accessions is not enough to conclude the effect of domestication on the root polyphenol compositions after pathogen infection, genotypic difference exists between wild and cultivated legumes and is associated with polyphenol accumulation in roots under biotic stress.
Strigolactones are stimulants of seed germination (Brun et al., 2018). The yield of faba bean (V. faba) is also limited by parasitic weeds. Faba bean (V. faba) germplasms resistant to parasitic weeds, broomrape (Orobanche and Phelipanche spp.) were found (Fernández-Aparicio et al., 2014). The resistant germplasms have low or undetectable levels of strigolactones in the root exudates at all plant ages (Fernández-Aparicio et al., 2014). It was suggested that the screening of germplasms with low strigalactone levels in root exudates is a strategy to breed for weed resistant germplasms. Like faba bean (V. faba), most of the commercial pea (Pisum sativum L.) cultivars are susceptible to the attack by crenate broompape (O. crenata Forsk.), which is a parasitic weed of legumes (Pavan et al., 2016). In a screen of O. crenata resistant pea germplasms, a landrace pea germplasm was selected. Repeated self-pollination of the landrace germplasm resulted in the O. crenata resistant line ROR12 (Pavan et al., 2016), which exhibited several unique characters: (1) compared to a O. crenata susceptible cultivar, the root exudates of ROR12, which had a lower strigolactone level, had a lower capability to stimulate the germination of O. crenata seeds; (2) in the field, the number of O. crenata shoots per host plant of ROR12 was lower; and (3) the emergence of O. crenata on ROR12 was delayed. It was proposed that the resistance to O. crenata was related to the reduced strigolactone level in the root exudates (Pavan et al., 2016).
Strigolactones are also involved in legume-microbe interaction. The treatment of synthetic strigolactone (GR24) to pea (Pisum sativum L.) roots enhanced the nodule number on the roots due to Rhizobium leguminosarum bv. viciae (RLV248) inoculation (Foo and Davies, 2011). Mutant rms1 of pea (Pisum sativum L.) had undetectable levels of orobanchol and orobanchyl acetate and a low level of fabacyl acetate in the root exudates (Foo and Davies, 2011). Compared to the wild type, rms1 mutant had less nodules on the roots after being inoculated with R. leguminosarum bv. viciae (RLV248; Foo and Davies, 2011). Commercial legume germplasms usually have lower levels of strigolactones in the root exudate (Fernández-Aparicio et al., 2014; Pavan et al., 2016).
The low levels of strigolactones may result in the reduced number of nodules on the roots. However, the nodulating phenotype may not be of consideration during domestication as the application of nitrogen fertilizer is a common practice during domestication.
Genes That Regulate the Different Secondary Metabolite-Related Traits
Several methods are currently being employed to identify the genes and mutations underlying legume domestication phenotypes (Olsen and Wendel, 2013). In general, secondary metabolites are present at higher levels in wild progenitors than in the domesticated counterparts as a result of artificial selection (Nagl et al., 1997; Lindig-Cisneros et al., 2002; Gepts, 2014). The selection of cultivars based on ease of farming and other commercial attributes may have occurred at the expense of potentially beneficial secondary metabolites. The reduction in genetic diversity is one of the main impacts of domestication. However, the genetic richness of wild populations can be used to improve cultivated legumes. Traditional plant breeding is a millenary process for the improvement and development of new crop varieties. According to breeding objectives, new legume varieties are produced by crossing parents with desired traits and selecting among segregating progenies those individuals with both high yield and the target trait. In this way, pest-resistant varieties have been developed with genetic resistance to pathogens (Lavaud et al., 2015). Traits related to pigmentation and defense against pathogens or herbivores are characteristically domestication-related traits governed by secondary metabolites. Besides biosynthesis-related genes, transport-related genes are also important. The roles of transporters, including ATP-binding cassette (ABC) transporters and multidrug and toxic compound extrusion (MATE) transporters in secondary metabolite secretion and accumulation have been summarized in previous reviews (Yazaki, 2005; Ku et al., 2020). In this section, examples of genes and loci controlling secondary metabolite biosynthesis and transport in legumes will be discussed.
Biosynthesis-Related Genes
Pigmentation-Related Traits
Polyphenols are the major determinants of tissue colors, including the colors of seed coats of legumes, both by their presence and their quantities (Espinosa-Alonso et al., 2006). The major polyphenols responsible for seed coat color in legumes are flavonoids, such as anthocyanins, flavonol glycosides, and proanthocyanidins (condensed tannins). Flavonoid quantities vary according to the seed developmental stages, genotypes, and species. The biosynthetic pathway leading to the biosynthesis of flavonoids has been elucidated and is conserved among seed-producing plants. Flavonoids and isoflavonoids are derived from the phenylpropanoid pathway (Dastmalchi and Dhaubhadel, 2014). Many genes in this pathway, including enzymes, transporters, and regulatory factors, have been characterized. The first committed step is the formation of a bicyclic tetrahydroxy chalcone (naringenin chalcone) catalyzed by a chalcone synthase (CHS). Legumes produce an additional trihydroxy chalcone (THC), isoliquiritigenin chalcone (Dastmalchi and Dhaubhadel, 2014). This THC is the end product of the coupled activities of CHS and the legume-specific chalcone reductase (CHR). Compounds such as daidzein, medicarpin, and glyceollin are derived from isoliquiritigenin. Flavonoid production follows the conversion of naringenin chalcone to (2S)-naringenin by chalcone isomerase (CHI). Flavone 3-hydroxylase (F3H) catalyzes the hydroxylation of (2S)-naringenin, eryodictyol, and pentahydroxyl flavanones to yield (2R,3R)-dihydrokaempferol, dihydroquercetin, and dihydromyricetin, respectively (Tanaka et al., 2008). Flavonoid 3'-hydroxylase (F3'H) and flavonoid 3',5'-hydroxylase (F3'5'H) catalyze the hydroxylation of flavanones, flavanols, and flavones, and determine the structures of flavonoids and anthocyanins (Tanaka, 2006). Other enzymes in the pathway include dihydroflavonol 4-reductase (DFR) and anthocyanidin synthase (ANS). The biosynthesis pathway of flavonoids is illustrated in Figure 1.
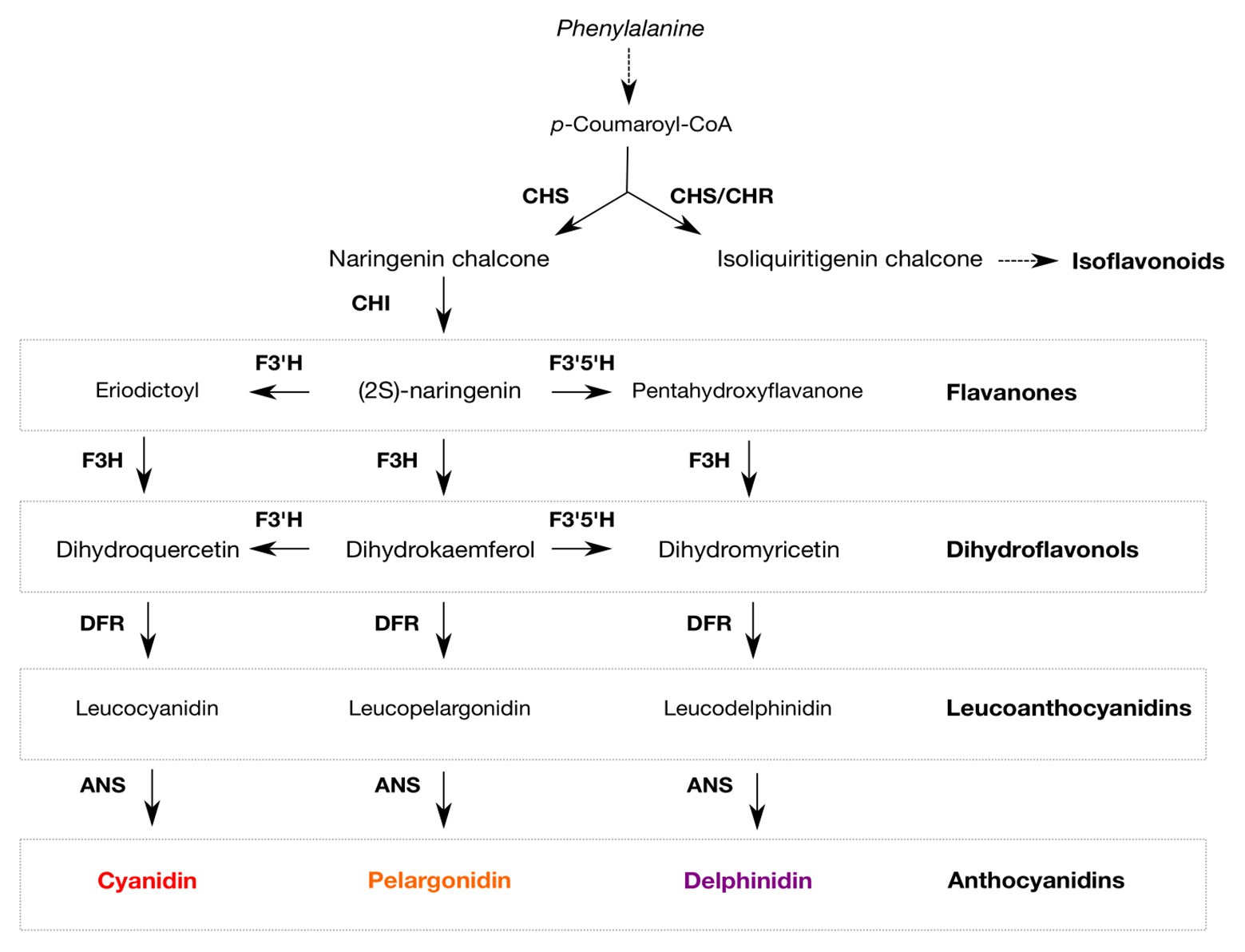
Figure 1. Schematic representation of the flavonoid biosynthetic pathway. Enzymes involved in the pathway are indicated in bold: chalcone synthase (CHS), chalcone reductase (CHR), flavone 3-hydroxylase (F3H), flavonoid 3'-hydroxylase (F3'H), flavonoid 3',5'-hydroxylase (F3'5'H), dihydroflavonol 4-reductase (DFR), and anthocyanidin synthase (ANS).
Pigmentation mechanisms have been studied in different legumes. A transcriptomic analysis was performed to identify the genes associated with seed coat color in peanut (A. hypogaea; Wan et al., 2016). Lower proanthocyanidin and anthocyanin contents were detected in a peanut mutant with a brown cracking seed coat (pscb). Transcriptomic analyses revealed that the structural genes of the phenylpropanoid biosynthetic pathway were downregulated in the pscb mutant, while the genes related to melanin production were upregulated at the late developmental stages. This expression pattern was consistent with the higher melanin content in the pscb mutant compared to the wild type. Differential expression analyses of RNA-seq data between the wild type and pscb mutant revealed three candidate genes (c36498_g1, c40902_g2, and c33560_g1) as being responsible for the seed coat color trait. C33560_g1 encodes a R2R3-MYB transcription factor. Its homologs in Arabidopsis and apple are associated with the regulation of the phenylpropanoid biosynthesis pathway (Rowan et al., 2009; Vimolmangkang et al., 2013). C36498_g1 and c40902_g2 encode a caffeoyl-CoA O-methyltransferase and a kinesin-4-like protein, respectively. Putative functions of the encoded proteins were associated with cell wall organization.
Soybeans cultivated for the commercial market are either completely yellow or have pigmentation restricted to the hilum (Palmer et al., 2004). Wild soybeans accumulate flavonoids and anthocyanins within the entire epidermal layer of the seed coat, giving them a black or brown color (Todd and Vodkin, 1993; Song et al., 2016). Quantitative trait loci (QTL) governing seed coat color in soybean have been identified using genetic and genomic analyses to elucidate the genetic changes that resulted in this domestication trait (Todd and Vodkin, 1996; Tuteja et al., 2004, 2009; Song et al., 2016). The I, R, and T loci were found to be involved in the flavonoid biosynthesis pathway (Palmer et al., 2004; Yang et al., 2010). The I locus on chromosome eight inhibits pigmentation of the seed coat. There are four alleles (I, ii, ik, and i) at the I locus where I and ii are the two dominant forms (Song et al., 2016). The presence of the I allele results in the absence of pigmentation and a yellow seed coat at maturity. This allele contains an inverted repeat of the CHS gene cluster. This structure triggers posttranscriptional gene silencing (PTGS), which inhibits the expression of CHS gene family members and their functions in the flavonoid biosynthesis pathway (Tuteja et al., 2004). The ii allele inhibits pigmentation, resulting in a yellow seed coat with a pigmented hilum (Palmer et al., 2004). Meanwhile, the recessive ik and i alleles allow pigment production, with the ik allele restringing pigments to the saddle and hilum regions of the seed coat (Palmer et al., 2004). The R and T loci determine the type and accumulation of pigments in the seed coat (Buzzetl et al., 1987; Todd and Vodkin, 1993). Higher flavonoid and anthocyanin contents of seeds are currently of great interest due to the antioxidant properties and flavors of these compounds. Recently, the wild soybean reference genome of G. soja W05 was used to identify additional alleles of the causal structural gene variation that controls soybean seed coat pigmentation (Xie et al., 2019). The analysis of a seed coat color QTL that overlaps with the known I locus showed that the W05 reference genome possesses the same inverted repeat of the CHS gene cluster as the domesticated soybean reference genome, G. max (Williams 82). This indicates that additional factors also played a role in causing the seed color changes during domestication. A comparative genomic analysis of W05 against two domesticated soybeans (Wm82 and ZH13) revealed the generation of a small interfering RNA (siRNA) from a large structural rearrangement next to the CHS gene cluster in Wm82 and ZH13. Through experimental validation, a subtilisin promoter was shown to drive the expression of a chimeric transcript that reads through a subtilisin gene fragment and an anti-CHS1 gene region, resulting in PTGS and inhibits the expression of CHS genes.
Flavonoids also contribute to floral pigmentation (Tanaka, 2006; Tanaka et al., 2008). Domesticated cowpea (Vigna unguiculata L. Walp) shows phenotypic variation compared to its wild relatives. Among the domestication traits, a wide range of floral and seed coat colors can be found in the cultivated cowpea. The wild variety shows purple flowers and dark seed pigmentation. Purple flowers are the results of diacylated delphinidin-based anthocyanins (Tanaka et al., 2008). A QTL analysis of the determinants of floral color in cowpea was performed in a biparental mapping population (wild × cultivated crosses; Lo et al., 2018). A single major QTL for floral color, CFcol7, was mapped in a 64-cM region on chromosome Vu07 containing 254 annotated genes, among which a transcription factor, Vigun07g110700, was identified as a homolog of Arabidopsis AT4G09820.1 and Medicago truncatula (Mt) TT8, involved in the regulation of flavonoid biosynthesis (Nesi et al., 2000; Li et al., 2016). In soybean, one QTL for floral pigmentation was identified on linkage group G (Josie et al., 2007).
Defense-Related Traits
Toxic secondary metabolites in legumes confer resistance against pathogens and herbivores. However, the accumulation of these compounds in legume crops is not desirable for human consumption or as animal feed since they impart a bitter taste and could present acute toxicity if ingested in sufficient quantities (Daverio et al., 2014). Alkaloids are some of the main secondary metabolites produced and stored by legumes with characteristic toxicity (Wink, 2013). Examples are quinolizidine alkaloids (QAs) produced by the genera Lupinus, Baptisia, Thermopsis, Genista, Cytisus, Echinosophora, and Sophora (Ohmiya et al., 1995). The breeding of low-alkaloid (sweet) varieties changed the agronomic roles of lupins from green manure and forage crops to grain legumes with high protein and fiber contents and health benefits (Sweetingham and Kingwell, 2008; Arnoldi et al., 2015). Four species within the genus Lupinus have been domesticated and are important legume crops: Lupinus angustifolius (narrow-leafed lupin or blue lupin), L. albus (white lupin), Lupinus luteus (yellow lupin), and Lupinus mutabilis (Andean lupin) (Gustafsson and Gadd, 1965; Reinhard et al., 2006). QAs produced by lupins include lupanine, angustifoline, lupinine, sparteine, multiflorine, and aphylline (Frick et al., 2017). The use of lupins for food purposes depends on their QA levels and each species has a characteristic alkaloid composition. Domestication has reduced the amount of alkaloids in lupins, but it has also increased their susceptibility to several aphid species (Philippi et al., 2015). QAs are derived from the decarboxylation of L-lysine (Lys) by a lysine decarboxylase (LDC, EC 4.1.1.18) to form cadaverine (Bunsupa et al., 2012a,b), which is then converted to 5-aminopentanal via oxidative deamination by a copper amine oxidase (CuAO, EC 1.4.3.22). 5-aminopentanal is spontaneously cyclized to a Δ1 piperideine Schiff base formation (Leistner and Spenser, 1973; Gupta et al., 1979; Golebiewski and Spenser, 1988; Bunsupa et al., 2012b), which then undergoes further modifications (e.g., oxygenation, dehydrogenation, hydroxylation, or esterification) to produce a range of Lys-derived alkaloids, including lupinine, sparteine, lupanine, and multiflorane (Ohmiya et al., 1995; Bunsupa et al., 2012b; Frick et al., 2017). QAs are stored in the form of QA esters. In lupins, QA esters are converted from lupinine/epilupinine and 13α-tigloyloxymultiflorine/13α-tigloyloxylupanine by two types of acetyltransferases (ATs): (+)-epilupinine/(−)-lupinine O-coumaroyl/feruloyltransferase (ECT/EFT-LCT/LFT) and (−)-13α-hydroxymultiflorine/(+)-13α-hydroxylupanine O-tigloyltransferase (HMT/HLT; EC 2.3.1.93), respectively (Saito et al., 1992; Okada et al., 2005; Bunsupa et al., 2012a). The biosynthesis pathway of QAs is illustrated in Figure 2. An HMT/HLT-type acetyltransferase was isolated and characterized at the molecular level in L. albus (Okada et al., 2005) while an acyltransferase-like gene, Lupinus angustifolius acyltransferase (LaAT), has been proposed to be involved in the QA biosynthetic pathway (Bunsupa et al., 2011).
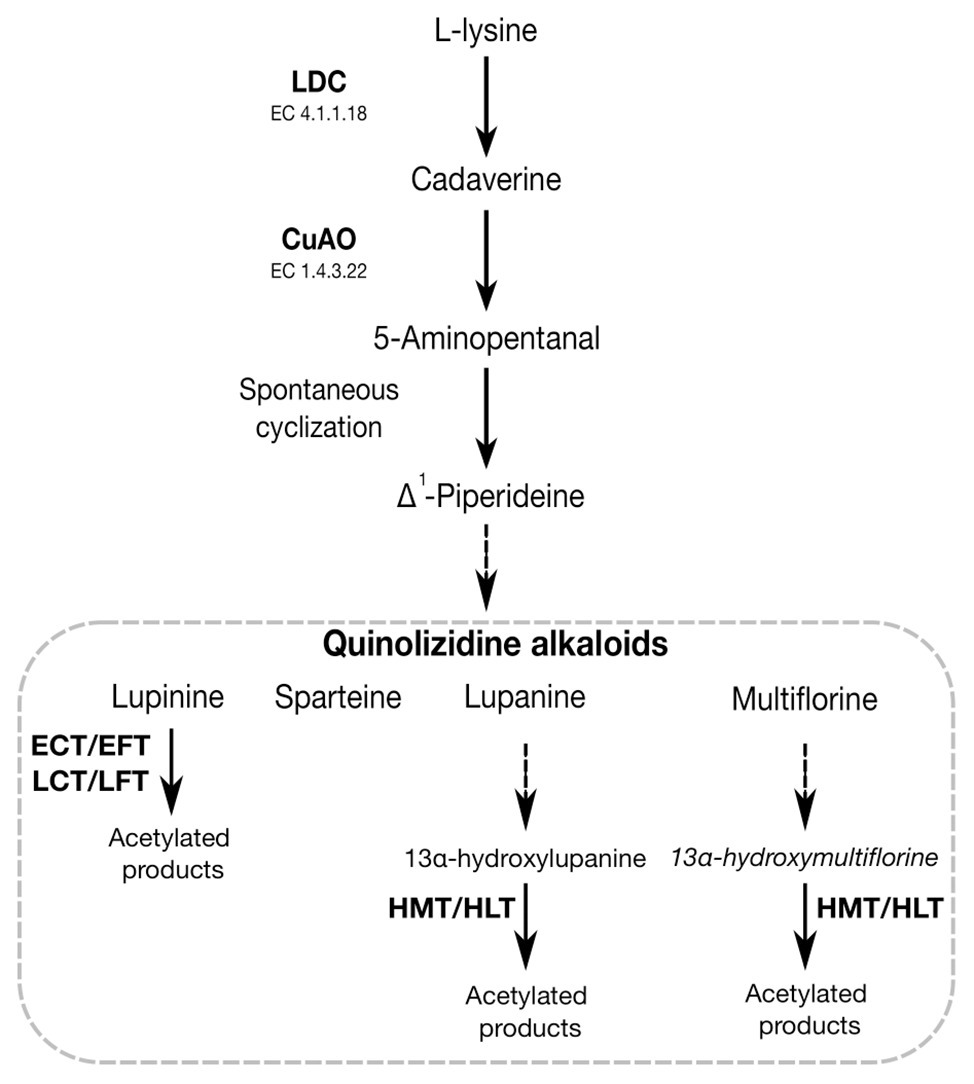
Figure 2. Schematic representation of the pathway leading to the synthesis of quinolizidine alkaloid compounds. Enzymes involved in the pathway are indicated in bold: lysine decarboxylase (LDC), copper amine oxidase (CuAO), (+)-epilupinine O-coumaroyltransferase (ECT), (+)-epilupinine feruloyltransferase (EFT), (−)-lupinine O-coumaroyltransferase (LCT), (−)-lupinine feruloyltransferase (LFT), (−)-13α-hydroxymultiflorine transferase (HMT), and (+)-13α-hydroxylupanine O-tigloyltransferas (HLT).
Domestication-related genetic modifications resulting in low-alkaloid phenotypes are generally results of naturally occurring (spontaneous) mutations (Gustafsson and Gadd, 1965). The domestication of lupins led to the active selection by farmers/breeders for sweet varieties which were low in alkaloids. In the late 1920s, the first low-alkaloid lines were obtained from wild germplasms of L. luteus and L. angustifolius (von Sengbusch, 1942). Subsequently, sweet types were also obtained for L. albus and L. mutabilis in the 1930s (Taylor et al., 2020). Several recessive low-alkaloid mutations have been discovered in L. angustifolius: iucundus (iuc), esculentus (es), depressus (depr), and tantalus (Swiecicki and Swiecicki, 1995; Kurlovich, 2002; Taylor et al., 2020), among which, the iucundus locus is the most prevalent allele in cultivars (Taylor et al., 2020). Molecular mapping efforts have allowed researchers to map the iucundus locus to a 746-kb region on chromosome NLL-07 (Nelson et al., 2006, 2010; Hane et al., 2017). The reference L. angustifolius genome also facilitated the identification of markers linked to iucundus that are suitable for marker-assisted selection (MAS). Specifically, an allele marker, IucLi, has been identified for the iucundus locus, and could be used for MAS in wild × domesticated crosses in lupin breeding programs (Li et al., 2011). Recently, 12 candidate genes for the alkaloid locus iucundus and the major QTLs associated with total QA contents were identified using a transcriptomic approach (Kroc et al., 2019b). The most promising candidate, RAP2-7, encodes an ethylene-responsive transcription factor (ERF) that co-segregated with the iucundus locus and is likely to be involved in the regulation of QA biosynthesis in L. angustifolius (Kroc et al., 2019a). Other candidate genes include a 4-hydroxy-tetrahydrodipicolinate synthase (DHDPS) involved in Lys biosynthesis as well as genes involved in plant secondary metabolism (Kroc et al., 2019b).
Recessive low-alkaloid mutations in L. albus have also been identified: pauper, mitis, reductus, exiguus, and nutricius (Hackbarth, 1957; Troll, 1958; Porsche, 1964). As in the case of L. angustifolius, one locus, pauper, is the most studied in L. albus (Rychel and Książkiewicz, 2019). The Kiev mutant × P27174 recombinant inbred lines (RILs) population was used for the first genetic map of L. albus where the pauper locus was located on linkage group 11 (Phan et al., 2007). Recently, a high-resolution map was developed to provide a high-resolution QTL assay of the agronomic traits of L. albus (Michał et al., 2017). The pauper locus was localized in the linkage group ALB18 (Michał et al., 2017). The Lup021586 gene was identified in the region and showed 100% nucleotide identity to LaAT, the acyltransferase gene previously identified in L. angustifolius (Bunsupa et al., 2011). LAGI01_35805, an L. albus homolog of LaAT that is highly similar to L. angustifolius Lup021586 gene, has been proposed as a molecular marker for the pauper locus (Rychel and Książkiewicz, 2019). Meanwhile, four low-alkaloid alleles have been identified in L. luteus, including dulcis, amoenus, liber, and v (von Sengbusch, 1942; Gustafsson and Gadd, 1965). However, there is limited information on the genetic basis for the low-alkaloid trait in this species. Efforts to improve the genomic resources of L. luteus are underway. The first genetic map for L. luteus has been recently released (Iqbal et al., 2019). A high-quality reference genome will help to implement MAS and identify loci responsible for the low-alkaloid content in L. luteus.
On the other hand, phytoalexins are a class of secondary metabolites with antimicrobial activities that are synthesized de novo after biotic and abiotic stresses (Walton, 1997). Phytoalexin biosynthesis can be induced by pathogens or a type of stress-mimicking compounds called elicitors (Angelova et al., 2006), and are produced by a range of crops including those in the Fabaceae family (Ahuja et al., 2012). Phytoalexins produced by the family Leguminosae comprise a variety of chemical compounds, including flavonoid phytoalexins derived from the shikimic acid pathway. In species such as soybean, prenylated pterocarpans, i.e., glyceollins, are synthesized in response to fungal pathogens such as Phytophthora sojae and Macrophomina phaseolina (Lygin et al., 2013). Soybean produces six forms of the isoflavonoid phytoalexin, glyceollin, where glyceollin I, glyceollin II, and glyceollin III are the predominant isomers (Banks and Dewick, 1983), derived from the addition of a dimethylallyl chain to (6aS,11aS)-3,9,6a-trihydroxypterocarpan (glycinol) at either C-4 or C-2 by prenyltransferases (PTs). Two isoflavonoid PTs have been identified in soybean: 4-dimethylallyltransferase (G4DT) and glycinol 2-dimethylallyltransferase (G2DT; Akashi et al., 2009; Yoneyama et al., 2016). Molecular characterization of PT genes revealed that G4DT and G2DT are paralogs resulting from a whole-genome duplication (Yoneyama et al., 2016). A genome-wide analysis of PT genes in G. max Wm82 identified 77 PT-encoding genes with 11 putative isoflavonoid-specific PTs (Sukumaran et al., 2018). One of the candidate genes, GmPT01 (G2DT-2) was induced by P. sojae infection and AgNO3, which mimics pathogen attack and lies in the QTL linked to P. sojae resistance. It was suggested that GmPT01 is one of the genes involved in the partial resistance and could be used in breeding for increased fungal resistance. Other genes related to P. sojae resistance include a CHS gene, GmCHR2A, located near a QTL linked to P. sojae resistance (Sepiol et al., 2017). Additionally, studies have shown that resistant and susceptible genotypes differ in their timing of activating glyceollin biosynthesis (Yoshikawa et al., 1978; Hahn et al., 1985). A rapid activation of the biosynthetic pathway allows a high level of accumulation of these low-molecular weight compounds and confers resistance to pathogens. Soybean genotypes encoding the P. sojae resistance gene, Rps1k, have shown a rapid activation of glyceollin biosynthesis and higher resistance to the pathogen (Yoshikawa et al., 1978; Hahn et al., 1985). Recently, a member of the NAC (NAM/ATAF1/2/CUC2)-family of transcription factor (TF) genes, GmNAC42-1, was identified using comparative transcriptomics (Jahan et al., 2020). GmNAC42-1 binds the promoter of G4DT and plays a role in the accumulation of glyceollin I. However, additional TFs are expected to participate in the regulation of glyceollin biosynthesis.
The phytoalexins in pea (P. sativum) are pisatin and maackiain (Ahuja et al., 2012). Pisatin, a 6α-hydroxyl-pterocarpan phytoalexin, is the major phytoalexin in pea produced in response to fungal infections by Nectria haematococca, Botrytis cinerea, and Mycosphaerella pinodes (Van den Heuvel and Glazener, 1975; Shiraishi et al., 1978; Etebu and Osborn, 2010). Its biosynthetic pathway has been partially characterized (Paiva et al., 1994; DiCenzo and Vanetten, 2006; Kaimoyo and VanEtten, 2008; Celoy and VanEtten, 2014). Pisatin and maackiain are synthetized via two chiral intermediates, (−)-7,2'-dihydroxy-4',5'-methylenedioxyisoflavanone [(−)-sophorol] and (−)-7,2'-dihydroxy-4',5'-methylenedioxyisoflavanol [(−)-DMDI; Preisig et al., 1989; Akashi et al., 2006; DiCenzo and Vanetten, 2006]. Sophorol reductase (SOR) is responsible for the production of sophorol, and it can be inactivated by RNA-mediated genetic interference (RNAi) which inhibits the production of pisatin in transgenic pea hairy roots (Kaimoyo and VanEtten, 2008). The pathway diverges for pisatin production after (−)-DMDI formation. In the last step of the pathway, the methylation of (+)-6α-hydroxymaackiain (6α-HMK) at the C-3 position by 6α-hydroxymaackiain methyltransferase (HMM2) results in the formation of (+)-pisatin. (−)-DMDI is converted to (−)-maackiain by hydroxisoflavanol dehydratase (HILD). The biosynthesis pathway of (+)-pisatin and (−)-maackiain is illustrated in Figure 3. M. pinodes causes ascochyta blight, the most important foliar disease of field pea, which responds by accumulating pisatin (Shiraishi et al., 1978). Efforts have been made to elucidate the QTLs associated with the disease resistance and to facilitate the introgression of resistance into pea cultivars (Wroth, 1999; Timmerman-Vaughan et al., 2004; Prioul-Gervais et al., 2007; Fondevilla et al., 2011). However, only moderate resistance has been reported with such efforts in pea cultivars (Kraft et al., 1998). Wild relatives present a high phytoalexin diversity (Lindig-Cisneros et al., 2002). The genetic controls for the resistance to M. pinodes were studied in a wild P. sativum subsp. syriacum accession P665 (Fondevilla et al., 2008). Six QTLs associated with M. pinodes resistance were detected in linkage groups II, III, IV, and V (Timmerman-Vaughan et al., 2004; Prioul-Gervais et al., 2007). Quantitative trait loci MpV.1 and MpII.1 were specific for seedlings under growth chamber conditions and MpIII.3 and MpIV.1 for adult plant resistance in field conditions (Timmerman-Vaughan et al., 2004; Prioul-Gervais et al., 2007). In contrast, QTLs MpIII.1 and MpIII.2 were detected both for seedling and field resistance (Timmerman-Vaughan et al., 2004; Prioul-Gervais et al., 2007). MpIII.2 overlaps with a QTL previously reported to be related to the resistance against ascochyta blight complex Asc3.1 (Timmerman-Vaughan et al., 2004; Prioul-Gervais et al., 2007). A resistance-gene analog (RGA1.1) was identified in the vicinity of this QTL using P. sativum populations (Timmerman-Vaughan et al., 2004; Prioul-Gervais et al., 2007). QTLs associated with partial resistance to the root rot-causing A. euteiches have also been identified in pea, and would be useful for improving and facilitating the existing recurrent selection-based breeding program (Kraft, 1988; Lewis and Gritton, 1992). Pilet-Nayel et al. (2002) crossed susceptible lines with partially resistant ones in order to map the QTLs associated with resistance against A. euteiches. The genetic map revealed seven such genomic regions and Aph1 located on the linkage group IVb was the most promising. Other minor QTLs were also identified in the 13 linkage groups obtained in the genetic mapping (Pilet-Nayel et al., 2002). Meta-analyses of four RILs of pea revealed 27 meta-Aphanomyces resistance QTLs, including 11 with high consistency across populations, locations, years, and isolates (Hamon et al., 2013). Seven highly consistent genomic regions were identified with the potential for use in MAS for pea improvement. Resistance QTLs located in these seven regions were further validated (Lavaud et al., 2015). Backcross-assisted selection programs were used to generate near-isogenic lines (NILs) carrying the resistance alleles of individual or combined resistance QTLs. The effects of two major QTLs, Ae-Ps4.5, and Ae-Ps7.6, were validated. The NILs carrying the resistance alleles of these two QTLs showed the highest resistance to A. euteiches strains. Several minor-effect QTLs were also validated, including Ae-Ps2.2 and Ae-Ps5.1. Genome-wide analyses further validated most of these resistance QTLs and detected additional novel resistance loci (Desgroux et al., 2016). Putative candidate genes in these loci were related to biotic stress responses.
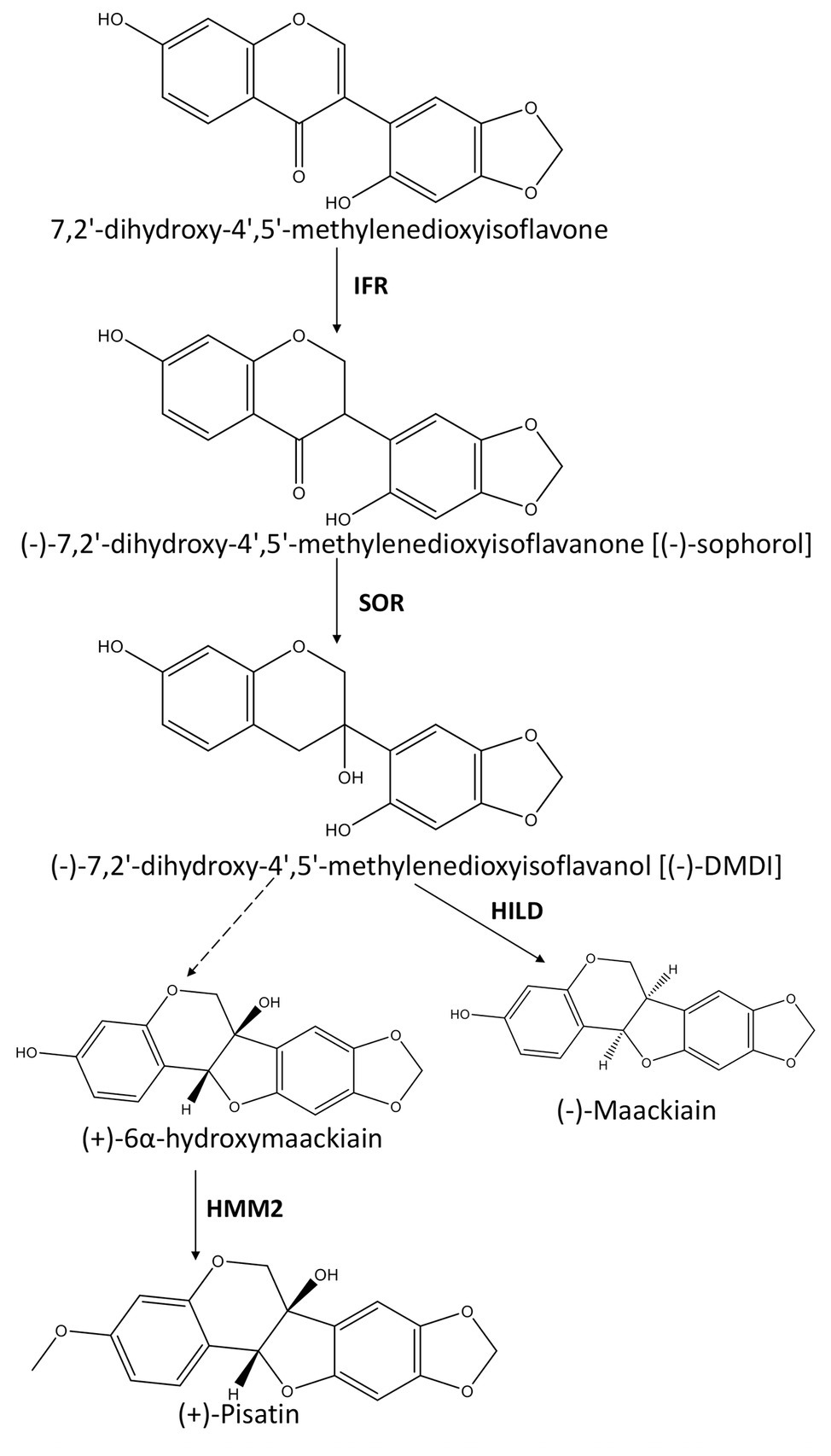
Figure 3. Schematic representation of the pathway leading to the synthesis of (+)-pisatin and (−)-maackiain. Enzymes involved in the pathway are indicated in bold: isoflavone reductase (IFR), sophorol reductase (SOR), (+)-6α-hydroxymaackiain 3-O-methyltransferase (HMM2), and hydroxisoflavanol dehydratase (HILD). The steps to convert (−)-7,2'-dihydroxy-4',5'-methylenedioxyisoflavanol (DMDI) to (+)-6α-hydroxymaackiain are unknown (dotted arrow).
Transporters
ATP-binding cassette transporters and multidrug and toxic compound extrusion transporters play important roles in the secretion and accumulation of secondary metabolites (Yazaki, 2005; Ku et al., 2020). These transporters are associated with microbe interaction and nutrient accumulation of legumes (Sugiyama et al., 2007; Zhang et al., 2010; Fondevilla et al., 2011; Li et al., 2016).
ABC Transporter
In soybean (G. max), an ABC transporter was reported to be involved in the root secretion of genistein, which is an important signaling molecule for mediating the symbiosis with rhizobia (Sugiyama et al., 2007). In M. truncatula, two half-ABC transporters, STR and STR2, are essential for arbuscule development in arbuscular mycorrhizal symbiosis (Zhang et al., 2010). The expression of the STR and STR2 genes was induced in cortical cells containing arbuscules (Zhang et al., 2010). STR and STR2 dimerize to form a transporter, which is located in the peri-arbuscular membrane and is important for the arbuscule development and therefore the symbiosis (Zhang et al., 2010). The str mutant and STR2-silenced transgenic roots exhibited stunted arbuscules after inoculating with Glomus versiforme (Zhang et al., 2010). In pea, using microarray technology, an ABC transporter was found to have a higher expression in P. sativum ssp. syriacum accession P665, which is resistant to Mycosphaerella pinodes, than the sensitive accession Messire (Fondevilla et al., 2011).
MATE Transporter
Seeds of wild soybeans (G. soja) generally have higher antioxidant contents than cultivated soybeans (G. max; Li et al., 2016). Statistical analysis showed the high correlation among the levels of seed total antioxidants, phenolics, and flavonoids (Li et al., 2016). Using RILs resulted from the cross between the wild soybean W05 (G. soja) and the cultivated soybean C08 (G. max), QTLs regulating the contents of antioxidants, phenolics, and flavonoids in soybean seeds were identified, which share a common genomic region (Li et al., 2016). In the target genomic region, three genes, GmMATE1, GmMATE2, and GmMATE4, were predicted to encode MATE transporters (Li et al., 2016). These MATE genes are possible candidates for investigating the basis behind the different seed antioxidant contents between wild soybeans (G. soja) and cultivated soybeans (G. max).
Molecular Breeding and Secondary Metabolite Content
As covered in this review, legumes produce a diverse array of secondary metabolites including a large subset of compounds with biopharmaceutical/nutraceutical properties. The production of these phytochemicals can be increase through crop improvement using classical breeding to genetic approaches (Jacob et al., 2016). Legumes with increased health-beneficial secondary metabolites are potential raw materials for producing pharmaceutical products.
The genetic variability of legume species is fundamental to identify parental lines to be used in breeding programs and exploit legume secondary metabolites. Modern targeted breeding programs use tools, such as quantitative trait loci, marked-assisted selection, and genomics applications (Collard and Mackill, 2008; Jacob et al., 2016). DNA-based molecular markers are used to characterize genomic regions (insertions, deletions, mutations) controlling a particular trait or gene to differentiate individuals for germplasm identification and characterization (Nadeem et al., 2018). Molecular markers provide breeders with a valuable resource to accelerate selection programs and mark complex traits, which are influenced by environmental factors or not observable at early stages of plant development. Flavonoids have pharmacological effects, such as antioxidants for human nutrition or anti-inflammatory effects among others. Also, nutritional value of legumes can be enhanced by increasing flavonoid content though breeding selection (D’Amelia et al., 2018). In this case, molecular markers have been used to study genetic variability in legumes to obtain varieties with high total flavonoid content. Genetic heritability of flavonoids is high and germplasms with different flavonoid content can lead to the identification of potential markers to use in breeding (Caseys et al., 2015). Flavonoid content was determined in 57 peanut accessions to evaluate the association between molecular markers and flavonoid content (Hou et al., 2017). Four expressed sequence tag-simple sequence repeat (EST-SSRs) markers were identified related to high flavonoid content in Chinese peanut germplasm. Functions of these markers were analyzed and related to outer membrane protein porin, heat-shock transcription factor, and lectins (Hou et al., 2017). Further studies are required to confirm the functions of these ESTs in flavonoid synthesis in peanuts. In soybean, three novel alleles were identified associated to flavonoid hydroxylase genes, F3'H and F3'5'H, related to pigmentation traits (Guo and Qiu, 2013). These molecular markers were identified using a set of gene-tagged markers based on the sequence variation of GmF3'H and GmF3'5'H in different soybean accessions, including cultivars, landraces, and wild soybeans (Guo and Qiu, 2013). Domestication process does not appear to erode diversity since four GmF3'H alleles were identified among cultivated soybeans, while G. soja contained only the GmF3'H allele. In the case of GmF3'5'H, 92.2% of wild soybean contained the GmF3'5'H-a allele, while three GmF3'5'H alleles occurred among cultivated soybeans (Guo and Qiu, 2013). In white clover (Trifolium repens), diversity array technology (DArT) and microsatellite markers were used to discover marker-trait associations for flavonoid accumulation and biomass (Ballizany et al., 2016). Significant associations to concentrations of flavonols quercetin, kaempferol, and Quercetin:Kaempferol ratio were found to markers on linkage group 1–2. Additionally, the study revealed deleterious alleles in an elite cultivar indicating that genetic variability from wild germplasm could be used for white clover improvement (Ballizany et al., 2016).
Engineering Secondary Metabolite Contents in Legumes
In addition to breeding programs to improve domesticated varieties and broaden the gene pool of cultivars, secondary metabolite contents can also be modified through plant metabolic engineering (DellaPenna, 2001). The identification of genes involved in the biosynthesis pathways of diverse secondary metabolites has helped to drive strategies to optimize the production of target compounds. Increased production of target metabolites can be achieved by altering the primary or secondary metabolism of an organism, for example, through the overexpression of genes in biosynthetic pathways or by knocking out gene expressions and hence the enzymatic activities of competing pathways. In soybean, the manipulation of the (iso)flavonoid pathway and its effect on the resistance to P. sojae has been studied (Cheng et al., 2015; Chen et al., 2018; Zhou et al., 2018). GmIFR, encoding an isoflavone reductase (IFR), was identified and overexpressed in soybean (Cheng et al., 2015). IFR catalyzes an intermediate step in the biosynthesis of glyceollins (Graham et al., 1990) and its constitutive expression in transgenic soybean plants enhances the resistance to P. sojae, along with higher glyceollin contents. Similar effects on pathogen resistance were obtained by the overexpression of coenzyme A ligase (GmPI4L) in transgenic soybean plants (Chen et al., 2018). Further attempts to elucidate enzymes/genes responsible for the resistance to pathogens include the overexpression of a chalcone isomerase, GmCHI1A, in soybean hairy roots, which enhanced daidzein accumulation and resistance to P. sojae strain P6497R compared to the control (Zhou et al., 2018). In alfalfa, nutritional value was increased by engineering genistein glucoside production (Deavours and Dixon, 2005). A transgenic alfalfa was developed by constitutively expressing an isoflavone synthase, MtIFS1, from M. truncatula. However, in the MtIFS1-expressing transgenic alfalfa, isoflavonoid production and accumulation was tissue-specific and affected by environmental factors such as UV-B and the disease-causing pathogen, Phoma medicaginis (Deavours and Dixon, 2005). RNAi-mediated gene silencing of isoflavone reductase, SOR, and hydroxymaackiain-3-O-methyltransferase in pea (P. sativum) allowed the identification of DMDI, an intermediary in the production of pisatin and maackiain (Kaimoyo and VanEtten, 2008). Other viable strategies of engineering secondary metabolite pathways include biosynthesis in microorganisms and modulation of gene expressions through manipulating the expressions of transcription factors (Du et al., 2010). Recently, genome-scale models have been used to represent the metabolic capabilities of legumes, including alfalfa and soybean (Pfau et al., 2018; Moreira et al., 2019). This approach allows the integration of different kinds of omics data to get new insights into plant-microbe interactions (diCenzo et al., 2016; Pfau et al., 2018; Contador et al., 2020). Models of plant metabolic pathways could also be used in the design of optimal-use biosynthesis pathways of secondary metabolites.
Conclusion
Domestication generally results in the reduction in secondary metabolites, which are often related to the bitter taste of seeds and the resistance of plants to biotic stresses. The phenomenon is consistent with the reported decrease in crop biodiversity due to domestication (Food and Agricultural Organization of the United Nations, 2010). Having numerous health-beneficial secondary metabolites, legumes have the great potential to be employed as the sources of bioactive compounds for pharmaceutical use. On the other hand, besides abiotic stresses, the changing climate may also bring forth unpredictable biotic stresses such as insect infestations. From these perspectives, it is important to retain the biodiversity of legumes in order to maintain a healthy gene pool to produce new cultivars that can respond to future changes in their environments. Understanding the genes that govern the beneficial secondary metabolite compositions in legumes will facilitate the use of wild legumes in breeding programs or metabolic engineering to promote crop diversity, as well as to produce legumes with favorable secondary metabolite profiles.
Author Contributions
H-ML planned and coordinated the writing. Y-SK put together the first complete draft and prepared the table. CC drew Figures 1, 2. M-SN prepared the table and drew Figure 3. All authors contributed to the search of literature and writings. All authors contributed to the article and approved the submitted version.
Funding
This work was supported by grants from the Hong Kong Research Grants Council General Research Fund (14143916) and Area of Excellence Scheme (AoE/M-403/16).
Disclaimer
Any opinions, findings, conclusions or recommendations expressed in this publication do not reflect the views of the Government of the Hong Kong Special Administrative Region or the Innovation and Technology Commission.
Conflict of Interest
The authors declare that the research was conducted in the absence of any commercial or financial relationships that could be construed as a potential conflict of interest.
Acknowledgments
J. Chu copy-edited this manuscript.
References
Abbas, M., Saeed, F., Anjum, F. M., Afzaal, M., Tufail, T., Bashir, M. S., et al. (2017). Natural polyphenols: an overview. Int. J. Food Prop. 20, 1689–1699. doi: 10.1080/10942912.2016.1220393
Abbo, S., van-Oss, P. R., Gopher, A., Saranga, Y., Ofner, I., and Peleg, Z. (2014). Plant domestication versus crop evolution: a conceptual framework for cereals and grain legumes. Trends Plant Sci. 19, 351–360. doi: 10.1016/j.tplants.2013.12.002
Abdel-lateif, K., Bogusz, D., and Hocher, V. (2012). The role of flavonoids in the establishment of plant roots endosymbioses with arbuscular mycorrhiza fungi, rhizobia and Frankia bacteria. Plant Signal. Behav. 7, 636–641. doi: 10.4161/psb.20039
Abraham, E. M., Ganopoulos, I., Madesis, P., Mavromatis, A., Mylona, P., Nianiou-Obeidat, I., et al. (2019). The use of lupin as a source of protein in animal feeding: genomic tools and breeding approaches. Int. J. Mol. Sci. 20:851. doi: 10.3390/ijms20040851
Acquaviva, R., Russo, A., Galvano, F., Galvano, G., Barcellona, M. L., Volti, G. L., et al. (2003). Cyanidin and cyanidin 3-O-β-D-glucoside as DNA cleavage protectors and antioxidants. Cell Biol. Toxicol. 19, 243–252. doi: 10.1023/b:cbto.0000003974.27349.4e
Adhikari, K. N., Edwards, O. R., Wang, S., Ridsdill-Smith, T. J., and Buirchell, B. (2012). The role of alkaloids in conferring aphid resistance in yellow lupin (Lupinus luteus L.). Crop Pasture Sci. 63, 444–451. doi: 10.1071/CP12189
Ahuja, I., Kissen, R., and Bones, A. M. (2012). Phytoalexins in defense against pathogens. Trends Plant Sci. 17, 73–90. doi: 10.1016/j.tplants.2011.11.002
Akashi, T., Sasaki, R., Aoki, T., Ayabe, S., and Yazaki, K. (2009). Molecular cloning and characterization of a cDNA for pterocarpan 4-dimethylallyltransferase catalyzing the key prenylation step in the biosynthesis of glyceollin, a soybean phytoalexin. Plant Physiol. 149, 683–693. doi: 10.1104/pp.108.123679
Akashi, T., VanEtten, H. D., Sawada, Y., Wasmann, C. C., Uchiyama, H., and Ayabe, S. (2006). Catalytic specificity of pea O-methyltransferases suggests gene duplication for (+)-pisatin biosynthesis. Phytochemistry 67, 2525–2530. doi: 10.1016/j.phytochem.2006.09.010
Al-Anazi, A. F., Qureshi, V. F., Javaid, K., and Qureshi, S. (2011). Preventive effects of phytoestrogens against postmenopausal osteoporosis as compared to the available therapeutic choices: an overview. J. Nat. Sci. Biol. Med. 2, 154–163. doi: 10.4103/0976-9668.92322
Amirkia, V., and Heinrich, M. (2014). Alkaloids as drug leads – a predictive structural and biodiversity-based analysis. Phytochem. Lett. 10, xlviii–liii. doi: 10.1016/j.phytol.2014.06.015
Angelova, Z., Georgiev, S., and Roos, W. (2006). Elicitation of plants. Biotechnol. Biotechnol. Equip. 20, 72–83. doi: 10.1080/13102818.2006.10817345
Applebaum, S. W., Marco, S., and Birk, Y. (1969). Saponins as possible factors of resistance of legume seeds to the attack of insects. J. Agric. Food Chem. 17, 618–622. doi: 10.1021/jf60163a020
Arnoldi, A., Boschin, G., Zanoni, C., and Lammi, C. (2015). The health benefits of sweet lupin seed flours and isolated proteins. J. Funct. Foods 18, 550–563. doi: 10.1016/j.jff.2015.08.012
Arts, I. C. W., van de Putte, B., and Hollman, P. C. H. (2000). Catechin contents of foods commonly consumed in the Netherlands. 1. Fruits, vegetables, staple foods, and processed foods. J. Agric. Food Chem. 48, 1746–1751. doi: 10.1021/jf000025h
Avato, P., Bucci, R., Tava, A., Vitali, C., Rosato, A., Bialy, Z., et al. (2006). Antimicrobial activity of saponins from Medicago sp.: structure-activity relationship. Phytother. Res. 20, 454–457. doi: 10.1002/ptr.1876
Azam, M., Zhang, S., Abdelghany, A. M., Shaibu, A. S., Feng, Y., Li, Y., et al. (2020). Seed isoflavone profiling of 1168 soybean accessions from major growing ecoregions in China. Food Res. Int. 130:108957. doi: 10.1016/j.foodres.2019.108957
Bagayoko, M., Buerkert, A., Lung, G., Bationo, A., and Römheld, V. (2000). Cereal/legume rotation effects on cereal growth in Sudano-Sahelian West Africa: soil mineral nitrogen, mycorrhizae and nematodes. Plant Soil 218, 103–116. doi: 10.1023/A:1014957605852
Ballizany, W. L., Griffiths, A. G., Franzmayr, B. K., Jahufer, M. Z. Z., Hofmann, R. W., and Barrett, B. A. (2016). “Marker-trait associations for flavonoids and biomass in white clover (Trifolium repens L.)” in Breeding in a world of scarcity. eds. I. Roldán-Ruiz, J. Baert, and D. Reheul (Cham: Springer International Publishing), 225–229.
Banks, S. W., and Dewick, P. M. (1983). Biosysnthesis of glyceollins I, II and III in soybean. Phytochemistry 22, 2729–2733. doi: 10.1016/S0031-9422(00)97682-9
Bazghaleh, N., Prashar, P., Purves, R. W., and Vandenberg, A. (2018). Polyphenolic composition of lentil roots in response to infection by Aphanomyces euteiches. Front. Plant Sci. 9:1131. doi: 10.3389/fpls.2018.01131
Bernstein, P. S., Li, B., Vachali, P. P., Gorusupudi, A., Shyma, R., Henriksen, B. S., et al. (2016). Lutein, zeaxanthin, and meso-zeaxanthin: the basic and clinical science underlying carotenoid-based nutritional interventions against ocular disease. Prog. Retin. Eye Res. 50, 34–66. doi: 10.1016/j.preteyeres.2015.10.003
Bertioli, D. J., Seijo, G., Freitas, F. O., Valls, J. F. M., Leal-Bertioli, S. C. M., and Moretzsohn, M. C. (2011). An overview of peanut and its wild relatives. Plant Genet. Resour. 9, 134–149. doi: 10.1017/S1479262110000444
Böttger, A., Vothknecht, U., Bolle, C., and Wolf, A. (eds.) (2018). “Plant secondary metabolites and their general function in plants” in Lessons on caffeine, cannabis & co: Plant derived drugs and their interaction with human receptors (Switzerland: Springer Nature), 3–17.
Brǿnnvik, H., and von Wettberg, E. J. (2019). Bird dispersal as a pre-adaptation for domestication in legumes: insights for neo-domestication. Front. Plant Sci. 10:1293. doi: 10.3389/fpls.2019.01293
Brun, G., Braem, L., Thoiron, S., Gevaert, K., Goormachtig, S., and Delavault, P. (2018). Seed germination in parasitic plants what insights can we expect from strigolactone research? J. Exp. Bot. 69, 2265–2280. doi: 10.1093/jxb/erx472
Bunsupa, S., Katayama, K., Ikeura, E., Oikawa, A., Toyooka, K., Saito, K., et al. (2012a). Lysine decarboxylase catalyzes the first step of quinolizidine alkaloid biosynthesis and coevolved with alkaloid production in leguminosae. Plant Cell 24, 1202–1216. doi: 10.1105/tpc.112.095885
Bunsupa, S., Okada, T., Saito, K., and Yamazaki, M. (2011). An acyltransferase-like gene obtained by differential gene expression profiles of quinolizidine alkaloid-producing and nonproducing cultivars of Lupinus angustifolius. Plant Biotechnol. J. 28, 89–94. doi: 10.5511/plantbiotechnology.10.1109b
Bunsupa, S., Yamazaki, M., and Saito, K. (2012b). Quinolizidine alkaloid biosynthesis: recent advances and future prospects. Front. Plant Sci. 3:239. doi: 10.3389/fpls.2012.00239
Buzzetl, R. I., Buttery, B. R., and MacTavish, D. C. (1987). Biochemical genetics of black pigmentation of soybean seed. J. Hered. 78, 53–54. doi: 10.1093/oxfordjournals.jhered.a110309
Carter, A., Tenuta, A., Rajcan, I., Welacky, T., Woodrow, L., and Eskandari, M. (2018). Identification of quantitative trait loci for seed isoflavone concentration in soybean (Glycine max) against soybean cyst nematode stress. Plant Breed. 137, 721–729. doi: 10.1111/pbr.12627
Caseys, C., Stritt, C., Glauser, G., Blanchard, T., and Lexer, C. (2015). Effects of hybridization and evolutionary constraints on secondary metabolites: the genetic architecture of phenylpropanoids in European Populus species. PLoS One 10:e0128200. doi: 10.1371/journal.pone.0128200
Celoy, R. M., and VanEtten, H. D. (2014). (+)-Pisatin biosynthesis: from (−) enantiomeric intermediates via an achiral 7,2'dihydroxy-4',5'-methyllenedioxyisoflav-3-ene. Phytochemistry 98, 120–127. doi: 10.1016/j.phytochem.2013.10.017
Chen, X., Fang, X., Zhang, Y., Wang, X., Zhang, C., Yan, X., et al. (2018). Overexpression of a soybean 4-coumaric acid: coenzyme a ligase (GmPI4L) enhances resistance to Phytophthora sojae in soybean. Funct. Plant Biol. 46, 304–313. doi: 10.1071/FP18111
Cheng, Q., Li, N., Dong, L., Zhang, D., Fan, S., Jiang, L., et al. (2015). Overexpression of soybean isoflavone reductase (GmIFR) enhances resistance to Phytophthora sojae in soybean. Front. Plant Sci. 6:1024. doi: 10.3389/fpls.2015.01024
Collard, B. C. Y., and Mackill, D. J. (2008). Marker-assisted selection: an approach for precision plant breeding in the twenty-first century. Philos. Trans. R. Soc. Lond. Ser. B Biol. Sci. 363, 557–572. doi: 10.1098/rstb.2007.2170
Considine, M. J., Siddique, K. H. M., and Foyer, C. H. (2017). Nature’ s pulse power: legumes, food security and climate change. J. Exp. Bot. 68, 1815–1818. doi: 10.1093/jxb/erx099
Contador, C. A., Lo, S. -K., Chan, S. H. J., and Lam, H. -M. (2020). Metabolic analyses of nitrogen fixation in the soybean microsymbiont Sinorhizobium fredii using constraint-based modeling. mSystems 5, e00516–e00519. doi: 10.1128/mSystems.00516-19
Cronk, Q., and Ojeda, I. (2008). Bird-pollinated flowers in an evolutionary and molecular context. J. Exp. Bot. 59, 715–727. doi: 10.1093/jxb/ern009
D’Amelia, V., Aversano, R., Chiaiese, P., and Carputo, D. (2018). The antioxidant properties of plant flavonoids: their exploitation by molecular plant breeding. Phytochem. Rev. 17, 611–625. doi: 10.1007/s11101-018-9568-y
Dastmalchi, M., and Dhaubhadel, S. (2014). “Soybean seed isoflavonoids: biosynthesis and regulation” in Phytochemicals – Biosynthesis, function and application. ed. R. Jetter (Cham, Switzerland: Springer), 1–21.
Daverio, M., Cavicchiolo, M. E., Grotto, P., Lonati, D., Cananzi, M., and Da Dalt, L. (2014). Bitter lupine beans ingestion in a child: a disregarded cause of acute anticholinergic toxicity. Eur. J. Pediatr. 173, 1549–1551. doi: 10.1007/s00431-013-2088-2
Deavours, B. E., and Dixon, R. A. (2005). Metabolic engineering of isoflavonoid biosynthesis in alfalfa. Plant Physiol. 138, 2245–2259. doi: 10.1104/pp.105.062539
Dehaan, L. R., Van Tassel, D. L., Anderson, J. A., Asselin, S. R., Barnes, R., Baute, G. J., et al. (2016). A pipeline strategy for grain crop domestication. Crop Sci. 56, 917–930. doi: 10.2135/cropsci2015.06.0356
DellaPenna, D. (2001). Plant metabolic engineering. Plant Physiol. 125, 160–163. doi: 10.1104/pp.125.1.160
Desgroux, A., Anthoëne, V. L., Roux-Duparque, M., Rivière, J. -P., Aubert, G., Tayeh, N., et al. (2016). Genome-wide association mapping of partial resistance to Aphanomyces euteiches in pea. BMC Genomics 17:124. doi: 10.1186/s12864-016-2429-4
Di Ferdinando, M., Brunetti, C., Fini, A., and Tattini, M. (2014). “Flavonoids as antioxidants in plants under abiotic stresses” in Abiotic stress responses in plants: Metabolism, productivity and sustainability. eds. P. Ahmad and M. N. V. Prasad (New York, NY: Springer), 159–179.
Dias, T. R., Alves, M. G., Casal, S., Silva, B. M., and Oliveira, P. F. (2016). The single and synergistic effects of the major tea components caffeine, epigallocatechin-3-gallate and L-theanine on rat sperm viability. Food Funct. 7, 1301–1305. doi: 10.1039/c5fo01611h
diCenzo, G. C., Checcucci, A., Bazzicalupo, M., Mengoni, A., Viti, C., Dziewit, L., et al. (2016). Metabolic modelling reveals the specialization of secondary replicons for niche adaptation in Sinorhizobium meliloti. Nat. Commun. 7:12219. doi: 10.1038/ncomms12219
DiCenzo, G. L., and Vanetten, H. D. (2006). Studies on the late steps of (+) pisatin biosynthesis: evidence for (−) enantiomeric intermediates. Phytochemistry 67, 675–683. doi: 10.1016/j.phytochem.2005.12.027
Dixon, R. A., and Sumner, L. W. (2003). Legume natural products: understanding and manipulating complex pathways for human and animal health. Plant Physiol. 131, 878–885. doi: 10.1104/pp.102.017319
Drewnowski, A., and Gomez-Carneros, C. (2000). Bitter taste, phytonutrients, and the consumer: a review. Am. J. Clin. Nutr. 72, 1424–1435. doi: 10.1093/ajcn/72.6.1424
Du, H., Huang, Y., and Tang, Y. (2010). Genetic and metabolic engineering of isoflavonoid biosynthesis. Appl. Microbiol. Biotechnol. 86, 1293–1312. doi: 10.1007/s00253-010-2512-8
Espinosa-Alonso, L. G., Lygin, A., Widholm, J. M., Valverde, M. E., and Paredes-Lopez, O. (2006). Polyphenols in wild and weedy Mexican common beans (Phaseolus vulgaris L.). J. Agric. Food Chem. 54, 4436–4444. doi: 10.1021/jf060185e
Etebu, E., and Osborn, A. M. (2010). Molecular quantification of the pea footrot disease pathogen (Nectria haematococca) in agricultural soils. Phytoparasitica 38, 447–454. doi: 10.1007/s12600-010-0122-8
Fernández-Aparicio, M., Kisugi, T., Xie, X., Rubiales, D., and Yoneyama, K. (2014). Low strigolactone root exudation: a novel mechanism of broomrape (Orobanche and Phelipanche spp.) resistance available for faba bean breeding. J. Agric. Food Chem. 62, 7063–7071. doi: 10.1021/jf5027235
Fernández-marín, B., Milla, R., Martín-robles, N., Arc, E., Kranner, I., Becerril, J. M., et al. (2014). Side-effects of domestication: cultivated legume seeds contain similar tocopherols and fatty acids but less carotenoids than their wild counterparts. BMC Plant Biol. 14:1599. doi: 10.1186/s12870-014-0385-1
Fernández-tomé, S., and Hernández-ledesma, B. (2019). Current state of art after twenty years of the discovery of bioactive peptide lunasin. Food Res. Int. 116, 71–78. doi: 10.1016/j.foodres.2018.12.029
Fondevilla, S., Küster, H., Krajinski, F., Cubero, J. I., and Rubiales, D. (2011). Identification of genes differentially expressed in a resistant reaction to Mycosphaerella pinodes in pea using microarray technology. BMC Genomics 12:28. doi: 10.1186/1471-2164-12-28
Fondevilla, S., Satovic, Z., Rubiales, D., Moreno, M. T., and Torres, A. M. (2008). Mapping of quantitative trait loci for resistance to Mycosphaerella pinodes in Pisum sativum subsp. syriacum. Mol. Breed. 21, 439–454. doi: 10.1007/s11032-007-9144-4
Foo, E., and Davies, N. W. (2011). Strigolactones promote nodulation in pea. Planta 234, 1073–1081. doi: 10.1007/s00425-011-1516-7
Food and Agricultural Organization of the United Nations (2010). Crop biodiversity: use it or lose it. Available at: http://www.fao.org/news/story/en/item/46803/icode/ [Accessed July 2, 2020].
Frick, K. M., Kamphuis, L. G., Kadambot, S. H. M., Singh, K. B., and Foley, R. C. (2017). Quinolizidine alkaloid biosynthesis in lupins and prospects for grain quality improvement. Front. Plant Sci. 8:87. doi: 10.3389/fpls.2017.00087
Gao, D., Wang, X., Fu, S., and Zhao, J. (2020). Legume plants enhance the resistance of soil to ecosystem disturbance site description and experimental. Front. Plant Sci. 8:1295. doi: 10.3389/fpls.2017.01295
García, E. H., Peña-valdivia, C. B., Aguirre, J. R. R., and Muruaga, J. S. M. (1997). Morphological and agronomic traits of a wild population and an improved cultivar of common bean (Phaseolus vulgaris L.). Ann. Bot. 79, 207–213. doi: 10.1006/anbo.1996.0329
Gepts, P. (2014). “Domestication of plants” in Encyclopedia of agriculture and food systems. ed. N. K. Van Alfen (Cambridge, Massachusetts: Academic Press), 474–486.
Gepts, P., Beavis, W. D., Brummer, E. C., Shoemaker, R. C., Stalker, H. T., Weeden, N. F., et al. (2005). Legumes as a model plant family. Genomics for food and feed report of the cross-legume advances through genomics conference. Plant Physiol. 137, 1228–1235. doi: 10.1104/pp.105.060871.1228
Golebiewski, W. M., and Spenser, I. D. (1988). Biosynthesis of the lupine alkaloids. II. Sparteine and lupanine. Can. J. Chem. 66, 1734–1748. doi: 10.1139/v88-280
Graham, T. L., Kim, J. E., and Graham, M. Y. (1990). Role of constitutive isoflavone conjugates in the accumulation of glyceollin in soybean infected with Phytophthora megasperma. Mol. Plant-Microbe Interact. 3, 157–166. doi: 10.1094/MPMI-3-157
Guo, Y., and Qiu, L. -J. (2013). Allele-specific marker development and selection efficiencies for both flavonoid 3'-hydroxylase and flavonoid 3',5'-hydroxylase genes in soybean subgenus soja. Theor. Appl. Genet. 126, 1445–1455. doi: 10.1007/s00122-013-2063-3
Gupta, Y. P. (1987). Anti-nutritional and toxic factors in food legumes: a review. Plant Foods Hum. Nutr. 37, 201–228. doi: 10.1007/BF01091786
Gupta, R. N., Horsewood, P., Koo, S. H., and Spenser, I. D. (1979). The biosynthesis of the Lythraceae alkaloids. I. The lysine-derived fragment. Can. J. Chem. 57, 1606–1614. doi: 10.1139/v79-260
Gupta, A. P., Pandotra, P., Kushwaha, M., Khan, S., Sharma, R., and Gupta, S. (2015). “Alkaloids: a source of anticancer agents from nature” in Studies in natural products chemistry. ed. Atta-ur-Rahman (Amsterdam, Netherlands: Elsevier B.V.), 341–445.
Gustafsson, A., and Gadd, I. (1965). Mutations and crop improvement. II. The genus Lupinus (Leguminosae). Hereditas 53, 15–39. doi: 10.1111/j.1601-5223.1965.tb01977.x
Hackbarth, J. (1957). Die Gene der Lupinenarten. III Weiße Lupine (Lupinus albus). Zeitschrift für Pflanzenzüchtung 37, 185–191.
Hahn, M. G., Bonhoff, A., and Grisebach, H. (1985). Quantitative locaglyceollin I in relation to fungal hyphae in soybean roots infected with Phytophthora megasperma f. sp. glycinea. Plant Physiol. 77, 591–601. doi: 10.1104/pp.77.3.591
Haldar, S., and Sengupta, S. (2015). Plant-microbe cross-talk in the rhizosphere: insight and biotechnological potential. Open Microbiol. J. 9, 1–7. doi: 10.2174/1874285801509010001
Hamon, C., Coyne, C. J., MacGee, R. J., Lesné, A., Esnault, R., Mangin, P., et al. (2013). QTL meta-analysis provides a comprehensive view of loci controlling partial resistance to Aphanomyces euteichesin four sources of resistance in pea. BMC Plant Biol. 13:45. doi: 10.1186/1471-2229-13-45
Hane, J. K., Ming, Y., Kamphuis, L. G., Nelson, M. N., Garg, G., Atkins, C. A., et al. (2017). A comprehensive draft genome sequence for lupin (Lupinus angustifolius), an emerging health food: insights into plant–microbe interactions and legume evolution. Plant Biotechnol. J. 15, 318–330. doi: 10.1111/pbi.12615
Harrison, M. J. (2005). Signaling in the arbuscular mycorrhizal symbiosis. Annu. Rev. Microbiol. 59, 19–42. doi: 10.1146/annurev.micro.58.030603.123749
Hassan, S. M., Byrd, J. A., Cartwright, A. L., and Bailey, C. A. (2010). Hemolytic and antimicrobial activities differ among saponin-rich extracts from guar, quillaja, yucca, and soybean. Appl. Biochem. Biotechnol. 162, 1008–1017. doi: 10.1007/s12010-009-8838-y
Heiser, C. B. (1988). Aspects of unconscious selection and the evolution of domesticated plants. Euphytica 37, 77–81. doi: 10.1007/BF00037227
Hellens, R. P., Moreau, C., Lin-Wang, K., Schwinn, K. E., Thomson, S. J., Fiers, M. W. E. J., et al. (2010). Identification of Mendel’s white flower character. PLoS One 5:e13230. doi: 10.1371/journal.pone.0013230
Heront, D. S., and Pueppket, S. G. (1984). Mode of infection, nodulation specificity, and indigenous plasmids of 11 fast-growing Rhizobium japonicum strains. J. Bacteriol. 160, 1061–1066. doi: 10.1128/JB.160.3.1061-1066.1984
Hirsch, A. M., Lum, M. R., and Downie, J. A. (2001). What makes the rhizobia-legume symbiosis so special? Plant Physiol. 127, 1484–1492. doi: 10.1104/pp.010866.1484
Hollman, P. C. H., and Arts, I. C. W. (2000). Flavonols, flavones and flavanols – nature, occurrence and dietary burden. J. Sci. Food Agric. 80, 1081–1093. doi: 10.1002/(SICI)1097-0010(20000515)80:7<1081::AID-JSFA566>3.0.CO;2-G
Hou, M., Mu, G., Zhang, Y., Cui, S., Yang, X., and Liu, L. (2017). Evaluation of total flavonoid content and analysis of related EST-SSR in Chinese peanut germplasm. Crop Breed. Appl. Biotechnol. 17, 221–227. doi: 10.1590/1984-70332017v17n3a34
Hsieh, C., Martínez-Villaluenga, C., De Lumen, B. O., and Hernández-Ledesma, B. (2017). Updating the research on the chemopreventive and therapeutic role of the peptide lunasin. J. Sci. Food Agric. 98, 2070–2079. doi: 10.1002/jsfa.8719
Husain, F., and Mallikarjuna, N. (2012). Genetic diversity in Bolivian landrace lines of groundnut (Arachis hypogaea L.). Indian J. Genet. Plant Breed. 72, 384–389.
Iqbal, M. M., Huynh, M., Udall, J. A., Kilian, A., Adhikari, K. N., Berger, J. D., et al. (2019). The first genetic map for yellow lupin enables genetic dissection of adaptation traits in an orphan grain legume crop. BMC Genet. 20:68. doi: 10.1186/s12863-019-0767-3
Isah, T. (2019). Stress and defense responses in plant secondary metabolites production. Biol. Res. 52:39. doi: 10.1186/s40659-019-0246-3
Jacob, C., Carrasco, B., and Schwember, A. R. (2016). Advances in breeding and biotechnology of legume crops. Plant Cell Tissue Organ Cult. 127, 561–584. doi: 10.1007/s11240-016-1106-2
Jahan, M. A., Harris, B., Lowery, M., Infante, A. M., Percifield, R. J., and Kovinich, N. (2020). Glyceollin transcription factor GmMYB29A2 regulates soybean resistance to Phytophthora sojae. Plant Physiol. 183, 530–546. doi: 10.1104/pp.19.01293
Jeong, H. J., Jeong, J. B., Kin, D. S., and De Lumen, B. O. (2007). Inhibition of core histone acetylation by the cancer preventive peptide lunasin. J. Agric. Food Chem. 55, 632–637. doi: 10.1021/jf062405u
Jeong, H. J., Lam, Y., and De Lumen, B. O. (2002). Barley lunasin suppresses ras-induced colony formation and inhibits core histone acetylation in mammalian cells. J. Agric. Food Chem. 50, 5903–5908. doi: 10.1021/jf0256945
Jeong, H. J., Lee, J. R., Jeong, J. B., Park, J. H., and De Lumen, B. O. (2009). The cancer preventive seed peptide lunasin from rye is bioavailable and bioactive. Nutr. Cancer 61, 680–686. doi: 10.1080/01635580902850082
Jeong, S. C., Moon, J. -K., Park, S. -K., Kim, M. -S., Lee, K., Lee, S. R., et al. (2019). Genetic diversity patterns and domestication origin of soybean. Theor. Appl. Genet. 132, 1179–1193. doi: 10.1007/s00122-018-3271-7
Jeong, H. J., Park, J. H., Lam, Y., and De Lumen, B. O. (2003). Characterization of lunasin isolated from soybean. J. Agric. Food Chem. 51, 7901–7906. doi: 10.1021/jf034460y
Jia, K., Baz, L., and Al-babili, S. (2018). From carotenoids to strigolactones. J. Exp. Bot. 69, 2189–2204. doi: 10.1093/jxb/erx476
Josie, J., Alcivar, A., Rainho, J., and Kassem, M. A. (2007). Genomic regions containing QTL for plant height, internodes length, and fower color in soybean [Glycine max (L.) Merr.]. BIOS 78, 119–126. doi: 10.1893/0005-3155(2007)78[119:RAGRCQ]2.0.CO;2
Kaimoyo, E., and VanEtten, H. D. (2008). Inactivation of pea genes by RNAi supports the involvement of two similar O-methyltransferases in the biosynthesis of (+)-pisatin and of chiral intermediates with a configuration opposite that found in (+)-pisatin. Phytochemistry 69, 76–87. doi: 10.1016/j.phytochem.2007.06.013
Kaplan, L. (1981). What is the origin of the common bean? Econ. Bot. 35, 240–254. doi: 10.1007/BF02858692
Kaur, K., Grewal, S. K., Gill, P. S., and Singh, S. (2019). Comparison of cultivated and wild chickpea genotypes for nutritional quality and antioxidant potential. J. Food Sci. Technol. 56, 1864–1876. doi: 10.1007/s13197-019-03646-4
Kleemann, R., Verschuren, L., Morrison, M., Zadelaar, S., van Erk, M. J., Wielinga, P. Y., et al. (2011). Anti-inflammatory, anti-proliferative and anti-atherosclerotic effects of quercetin in human in vitro and in vivo models. Atherosclerosis 218, 44–52. doi: 10.1016/j.atherosclerosis.2011.04.023
Knekt, P., Kumpulainen, J., Järvinen, R., Rissanen, H., Heliövaara, M., Reunanen, A., et al. (2002). Flavonoid intake and risk of chronic diseases. Am. J. Clin. Nutr. 76, 560–568. doi: 10.1093/ajcn/76.3.560
Kraft, J. M., Dunne, B., Goulden, D., and Armstrong, S. (1998). A search for resistance in peas to Mycosphaerella pinodes. Plant Dis. 82, 251–253. doi: 10.1094/PDIS.1998.82.2.251
Krishnamurthy, P., Chung, G., and Singh, R. J. (2013). Saponin polymorphism in the Korean wild soybean (Glycine soja Sieb. And Zucc.). Plant Breed. 132, 121–126. doi: 10.1111/pbr.12016
Křížová, L., Dadáková, K., Kašparovská, J., and Kašparovský, T. (2019). Isoflavones. Molecules 24:1076. doi: 10.3390/molecules24061076
Kroc, M., Czepiel, K., Wilczura, P., Mokrzycka, M., and Swięcicki, W. (2019a). Development and validation of a gene-targeted dCAPS marker for marker-assisted selection of low-alkaloid content in seeds of narrow-leafed lupin (Lupinus angustifolius L.). Genes 10:428. doi: 10.3390/genes10060428
Kroc, M., Koczyk, G., Kamel, K. A., Czepiel, K., Fedorowicz-Strońska, O., Paweł, K., et al. (2019b). Transcriptome-derived investigation of biosynthesis of quinolizidine alkaloids in narrow-leafed lupin (Lupinus angustifolius L.) highlights candidate genes linked to iucundus locus. Sci. Rep. 9:2231. doi: 10.1038/s41598-018-37701-5
Ku, Y. -S., Ng, M. -S., Cheng, S. -S., Lo, A. W. -Y., Xiao, Z., Shin, T. -S., et al. (2020). Understanding the composition, biosynthesis, accumulation and transport of flavonoids in crops for the promotion of crops as healthy sources of flavonoids for human consumption. Nutrients 12:1717. doi: 10.3390/nu12061717
Kumar, G. P., and Khanum, F. (2012). Neuroprotective potential of phytochemicals. Pharmacogn. Rev. 6, 81–90. doi: 10.4103/0973-7847.99898
Kumar, S., and Pandey, A. K. (2013). Chemistry and biological activities of flavonoids: an overview. Sci. World J. 2013:162750. doi: 10.1155/2013/162750
Kumar, A., Singh, A., and Ekavali, (2015). A review on Alzheimer’s disease pathophysiology and its management: an update. Pharmacol. Rep. 67, 195–203. doi: 10.1016/j.pharep.2014.09.004
Kurlovich, B. S. (2002). “Genetics of lupins” in Lupins: Geography, classification, genetic resources and breeding. ed. B. S. Kurlovich (St Petersburg, Russia; Pellosniemi, Finland: OY International North Express), 313–350.
Kushi, L. H., Meyer, K. A., and Jacobs, D. R. Jr. (1999). Cereals, legumes, and chronic disease risk reduction: evidence from epidemiologic studies. Am. J. Clin. Nutr. 70(3 suppl), 451S–458S. doi: 10.1093/ajcn/70.3.451s
Latha, B. P., Reddy, I. R. M., Vijaya, T., Rao, S. D., Ismail, S. M., and Girish, B. P. (2011). Effect of saponin rich extract of Achyranthes aspera on high fat diet fed male wistar rats. J. Pharm. Res. 4, 3190–3193.
Lavaud, C., Lesné, A., Piriou, C., Le Roy, G., Boutet, G., Moussart, A., et al. (2015). Validation of QTL for resistance to Aphanomyces euteiches in different pea genetic backgrounds using near-isogenic lines. Theor. Appl. Genet. 128, 2273–2288. doi: 10.1007/s00122-015-2583-0
Leistner, E., and Spenser, I. D. (1973). Biosynthesis of the piperidine nucleus. Incorporation of chirally labeled [1-3H]cadaverine. J. Am. Chem. Soc. 95, 4715–4725. doi: 10.1021/ja00795a041
Lewis, M. E., and Gritton, E. T. (1992). Use of one cycle of recurrent selection per year for increasing resistance to Aphanomyces root rot in peas. J. Am. Soc. Hortic. Sci. 117, 638–642. doi: 10.21273/JASHS.117.4.638
Li, M. W., Muñoz, N. B., Wong, C. F., Wong, F. L., Wong, K., Wong, J. W. H., et al. (2016). QTLs regulating the contents of antioxidants, phenolics, and flavonoids in soybean seeds share a common genomic region. Front. Plant Sci. 7:854. doi: 10.3389/fpls.2016.00854
Li, X., Yang, H., Buirchell, B., and Yan, G. (2011). Development of a DNA marker tightly linked to low-alkaloid gene iucundus in narrow-leafed lupin (Lupinus angustifolius L.) for marker-assisted selection. Crop Pasture Sci. 62, 218–224. doi: 10.1071/CP10352
Liang, H., Yu, Y., Wang, S., Lian, Y., Wang, T., Wei, Y., et al. (2010). QTL mapping of isoflavone, oil and protein contents in soybean (Glycine max L. Merr.). Agric. Sci. China 9, 1108–1116. doi: 10.1016/S1671-2927(09)60197-8
Lindig-Cisneros, R., Dirzo, R., and Espinosa-Garcia, F. J. (2002). Effects of domestication and agronomic selection on phytoalexin antifungal defense in Phaseolus beans. Ecol. Res. 17, 315–321. doi: 10.1046/j.1440-1703.2002.00491.x
Lo, S., Muñoz-amatriaín, M., Boukar, O., He, I., Cisse, N., Guo, Y., et al. (2018). Identification of QTL controlling domestication-related traits in cowpea (Vigna unguiculata L. Walp). Sci. Rep. 8:6261. doi: 10.1038/s41598-018-24349-4
López-cortez, M. D. S., Rosales-martínez, P., Arellano-cárdenas, S., and Cornejo-Mazón, M. (2016). “Antioxidants properties and effect of processing methods on bioactive compounds of legumes” in Grain legumes. ed. A. K. Goyal (Rijeka: IntechOpen).
Lygin, A. V., Zernova, O. V., Hill, C. B., Kholina, N. A., Widholm, J. M., Hartman, G. L., et al. (2013). Glyceollin is an important component of soybean plant defense against Phytophthora sojae and Macrophomina phaseolina. Phytopathology 103, 984–994. doi: 10.1094/PHYTO-12-12-0328-R
Maass, B. L. (2006). Changes in seed morphology, dormancy and germination from wild to cultivated hyacinth bean germplasm (Lablab purpureus: Papilionoideae). Genet. Resour. Crop. Evol. 53, 1127–1135. doi: 10.1007/s10722-005-2782-7
Man, S., Gao, W., Zhang, Y., Huang, L., and Liu, C. (2010). Chemical study and medical application of saponins as anti-cancer agents. Fitoterapia 81, 703–714. doi: 10.1016/j.fitote.2010.06.004
Marciani, D. J. (2018). Elucidating the mechanisms of action of saponin-derived adjuvants. Trends Pharmacol. Sci. 39, 573–585. doi: 10.1016/j.tips.2018.03.005
Marrelli, M., Conforti, F., Araniti, F., and Statti, G. A. (2016). Effects of saponins on lipid metabolism: a review of potential health benefits in the treatment of obesity. Molecules 21:1404. doi: 10.3390/molecules21101404
McClean, P. E., Bett, K. E., Stonehouse, R., Lee, R., Pflieger, S., Moghaddam, S. M., et al. (2018). White seed color in common bean (Phaseolus vulgaris) results from convergent evolution in the P (pigment) gene. New Phytol. 219, 1112–1123. doi: 10.1111/nph.15259
McCrory, M. A., Hamaker, B. R., Lovejoy, J. C., and Eichelsdoerfer, P. E. (2010). Pulse consumption, satiety, and weight management. Adv. Nutr. 1, 17–30. doi: 10.3945/an.110.1006
Menéndez, A. B., Calzadila, P. I., Sansberro, P. A., Espasandin, F. D., Gazquez, A., Bordenave, C. D., et al. (2019). Polyamines and legumes: joint stories of stress, nitrogen fixation and environment. Front. Plant Sci. 10:1415. doi: 10.3389/fpls.2019.01415
Michał, K., Nazzicari, N., Yang, H., Nelson, M. N., Renshaw, D., Rychel, S., et al. (2017). A high-density consensus linkage map of white lupin highlights synteny with narrow-leafed lupin and provides markers tagging key agronomic traits. Sci. Rep. 7:15335. doi: 10.1038/s41598-017-15625-w
Mohan, V. R., Tresina, P. S., and Daffodil, E. D. (2016). Antinutritional factors in legume seeds: Characteristics and determination. 1st Edn. Cambridge, Massachusetts: Academic Press.
Moreira, T. B., Shaw, R., Luo, X., Ganguly, O., Kim, H. -S., Ferreira Coelho, L. G., et al. (2019). A genome-scale metabolic model of soybean (Glycine max) highlights metabolic fluxes in seedlings. Plant Physiol. 180, 1912–1929. doi: 10.1104/pp.19.00122
Morrison, M. J., Cober, E. R., Saleem, M. F., McLaughlin, N. B., Frégeau-Reid, J., Ma, B. L., et al. (2008). Changes in isoflavone concentration with 58 years of genetic improvement of short-season soybean cultivars in Canada. Crop Sci. 48, 2201–2208. doi: 10.2135/cropsci2008.01.0023
Muñoz-Esparza, N., Latorre-Moratalla, M. L., Comas-Basté, O., Toro-Funes, N., Veciana-Nogués, M. T., and Vidal-Carou, C. V. (2019). Polyamines in food. Front. Nutr. 6:108. doi: 10.3389/fnut.2019.00108
Muzquiz, M., Cuadrado, C., Ayet, G., De Cuadra, C., Burbano, C., and Osagie, A. (1994). Variation of alkaloid components of lupin seeds in 49 genotypes of Lupinus albus L. from different countries and locations. J. Agric. Food Chem. 42, 1447–1450. doi: 10.1021/jf00043a011
Nadeem, M. A., Nawaz, M. A., Shahid, M. Q., Doğan, Y., Comertpay, G., Yıldız, M., et al. (2018). DNA molecular markers in plant breeding: current status and recent advancements in genomic selection and genome editing. Biotechnol. Biotechnol. Equip. 32, 261–285. doi: 10.1080/13102818.2017.1400401
Nagl, W., Ignacimuthu, S., and Becker, J. (1997). Genetic engineering and regeneration of Phaseolus and Vigna. State of the art and new attempts. J. Plant Physiol. 150, 625–644. doi: 10.1016/S0176-1617(97)80277-5
Nelson, M. N., Moolhuijzen, P. M., Boersma, J. G., Chudy, M., Lesniewska, K., Bellgard, M., et al. (2010). Aligning a new reference genetic map of Lupinus angustifolius with the genome sequence of the model legume Lotus japonicus. DNA Res. 17, 73–83. doi: 10.1093/dnares/dsq001
Nelson, M. N., Phan, H. T. T., Ellwood, S. R., Moolhuijzen, P. M., Hane, J., Williams, A., et al. (2006). The first gene-based map of Lupinus angustifolius L.-location of domestication genes and conserved synteny with Medicago truncatula. Theor. Appl. Genet. 113, 225–238. doi: 10.1007/s00122-006-0288-0
Nesi, N., Debeaujon, I., Jond, C., Pelletier, G., Caboche, M., and Lepiniec, L. (2000). The TT8 gene encodes a basic helix-loop-helix domain protein required for expression of DFR and BAN genes in Arabidopsis siliques. Plant Cell 12, 1863–1878. doi: 10.1105/tpc.12.10.1863
Oakenfull, D. (1981). Saponins in food-a review. Food Chem. 7, 19–40. doi: 10.1016/0308-8146(81)90019-4
Ohmiya, S., Saito, K., and Murakoshi, I. (1995). “Lupine alkaloids” in The alkaloids: Chemistry and pharmacology. ed. G. A. Cordell (San Diego, CA: Academic Press), 1–114.
Ojwang, L. O., Yang, L., Dykes, L., and Awika, J. (2013). Proanthocyanidin profile of cowpea (Vigna unguiculata) reveals catechin-O-glucoside as the dominant compound. Food Chem. 139, 35–43. doi: 10.1016/j.foodchem.2013.01.117
Okada, T., Hirai, M. Y., Suzuki, H., Yamazaki, M., and Saito, K. (2005). Molecular characterization of a novel quinolizidine akaloid O-tigloyltransferase: cDNA cloning, catalytic activity of recombinant protein and expression analysis in Lupinus plants. Plant Cell Physiol. 46, 233–244. doi: 10.1093/pcp/pci021
Okubo, K., Iijima, M., Kobayashi, Y., Yoshikoshi, M., Uchida, T., and Kudou, S. (1992). Components responsible for the undesirable taste of soybean seeds. Biosci. Biotechnol. Biochem. 56, 99–103. doi: 10.1271/bbb.56.99
Olsen, K. M., and Wendel, J. F. (2013). A bountiful harvest: genomic insights into crop domestication phenotypes. Annu. Rev. Plant Biol. 64, 47–70. doi: 10.1146/annurev-arplant-050312-120048
Onguéné, P. A., Ntie-kang, F., Lifongo, L. L., Ndom, J. C., Sippl, W., and Mbaze, L. M. (2013). The potential of anti-malarial compounds derived from African medicinal plants. Part I: a pharmacological evaluation of alkaloids and terpenoids. Malar. J. 12:449. doi: 10.1186/1475-2875-13-81
Paiva, N. L., Sun, Y., Dixon, R. A., VanEtten, H. D., and Hrazdina, G. (1994). Molecular cloning of isoflavone reductase from pea (Pisum sativum L): evidence for a 3R-isoflavanone intermediate in (+)-pisatin biosynthesis. Arch. Biochem. Biophys. 312, 501–510. doi: 10.1006/abbi.1994.1338
Palmer, R. G., Pfeiffer, T. W., Buss, G. R., and Kilen, T. C. (2004). “Qualitative genetics” in Soybeans: Improvement, production, and uses. eds. R. M. Shibles, J. E. Harper, R. F. Wilson, and R. C. Shoemaker (Madison, WI: American Society of Agronomy, Crop Science Society of America, Soil Science Society of America), 137–233.
Pavan, S., Schiavulli, A., Marcotrigiano, A. R., Bardaro, N., Bracuto, V., Ricciardi, F., et al. (2016). Characterization of low-strigolactone germplasm in pea (Pisum sativum L.) resistant to crenate broomrape (Orobanche crenata Forsk.). Mol. Plant-Microbe Interact. 29, 743–749. doi: 10.1094/MPMI-07-16-0134-R
Penmetsa, R. V., Carrasquilla-Garcia, N., Bergmann, E. M., Vance, L., Castro, B., Kassa, M. T., et al. (2016). Multiple post-domestication origins of kabuli chickpea through allelic variation in a diversification-associated transcription factor. New Phytol. 211, 1440–1451. doi: 10.1111/nph.14010
Pfau, T., Christian, N., Masakapalli, S. K., Sweetlove, L. J., Poolman, M. G., and Ebenhöh, O. (2018). The intertwined metabolism during symbiotic nitrogen fixation elucidated by metabolic modelling. Sci. Rep. 8:12504. doi: 10.1038/s41598-018-30884-x
Phan, H. T. T., Ellwood, S. R., Adhikari, K. N., Nelson, M. N., and Oliver, R. P. (2007). The first genetic and comparative map of white lupin (Lupinus albus L.): identification of QTLs for anthracnose resistance and flowering time, and a locus for alkaloid content. DNA Res. 14, 59–70. doi: 10.1093/dnares/dsm009
Philippi, J., Schliephake, E., Jürgens, E., Jansen, H. U., and Ordon, F. (2015). Feeding behavior of aphids on narrow-leafed lupin (Lupinus angustifolius) genotypes varying in the content of quinolizidine alkaloids. Entomol. Exp. Appl. 156, 37–51. doi: 10.1111/eea.12313
Pietta, P., Minoggio, M., and Bramati, L. (2003). Plant polyphenols: structure, occurrence and bioactivity. Stud. Nat. Prod. Chem. 28, 257–312. doi: 10.1016/S1572-5995(03)80143-6
Pilet-Nayel, M. -L., Muehlbauer, F. J., Mcgee, R. J., Kraft, J. M., Baranger, A., and Coyne, C. J. (2002). Quantitative trait loci for partial resistance to Aphanomyces root rot in pea. Theor. Appl. Genet. 106, 28–39. doi: 10.1007/s00122-002-0985-2
Porsche, W. (1964). Untersuchungen über die Vererbung der Alkaloidarmut und die Variabilität des Restalkaloidgehaltes Bei lupinus albus L. Der Züchter 34, 251–256. doi: 10.1007/BF00705826
Porter, S. S. (2013). Adaptive divergence in seed color camouflage in contrasting soil environments. New Phytol. 197, 1311–1320. doi: 10.1111/nph.12110
Preisig, C. L., Matthews, D. E., and VanEtten, H. D. (1989). Purification and characterization of S-Adenosyl-L-methionine:6a-hydroxymaackiain 3-O-methyltransferase from Pisum sativum. Plant Physiol. 91, 559–566. doi: 10.1104/pp.91.2.559
Primomo, V. S., Poysa, V., Ablett, G. R., Jackson, C. -J., Gijzen, M., and Rajcan, I. (2005). Mapping QTL for individual and total isoflavone content in soybean seeds. Crop Sci. 45, 2454–2464. doi: 10.2135/cropsci2004.0672
Prioul-Gervais, S., Deniot, G., Receveur, E. -M., Frankewitz, A., Fourmann, M., Rameau, C., et al. (2007). Candidate genes for quantitative resistance to Mycosphaerella pinodes in pea (Pisum sativum L.). Theor. Appl. Genet. 114, 971–984. doi: 10.1007/s00122-006-0492-y
Pueppke, S. G., Bolaños-Vásquez, M. C., Werner, D., Bec-Ferté, M. -P., Promé, J. -C., and Krishnan, H. B. (1998). Release of flavonoids by the soybean cultivars McCall and Peking and their perception as signals by the nitrogen-fixing symbiont Sinorhizobium fredii. Plant Physiol. 117, 599–608. doi: 10.1104/pp.117.2.599
Quintero-soto, M. F., Saracho-peña, A. G., Chavez-ontiveros, J., and Garzon-tiznado, J. A. (2018). Phenolic profiles and their contribution to the antioxidant activity of selected chickpea genotypes from Mexico and ICRISAT collections. Plant Foods Hum. Nutr. 73, 122–129. doi: 10.1007/s11130-018-0661-6
Reddy, M. R. I., Latha, P. B., Vijaya, T., and Rao, D. S. (2012). The saponin-rich fraction of a Gymnema sylvestre R. Br. Aqueous leaf extract reduces cafeteria and high-fat diet-induced obesity. Z. Naturforsch. C Biosci. 67, 39–46. doi: 10.1515/znc-2012-1-206
Rehman, H. M., Nawas, M. A., Shah, Z. H., Yang, S. H., and Chung, G. (2018). Characterization of naturally occurring wild soybean mutant (sg-5) lacking astringent saponins using whole genome sequencing approach. Plant Sci. 267, 148–156. doi: 10.1016/j.plantsci.2017.11.014
Reinhard, H., Rupp, H., Sager, F., Streule, M., and Zoller, O. (2006). Quinolizidine alkaloids and phomopsins in lupin seeds and lupin containing food. J. Chromatogr. A 1112, 353–360. doi: 10.1016/j.chroma.2005.11.079
Roberts, R. L., Green, J., and Lewis, B. (2009). Lutein and zeaxanthin in eye and skin health. Clin. Dermatol. 27, 195–201. doi: 10.1016/j.clindermatol.2008.01.011
Romeo, F. V., Fabroni, S., Ballistreri, G., Muccilli, S., Spina, A., and Rapisarda, P. (2018). Characterization and antimicrobial activity of alkaloid extracts from seeds of different genotypes of Lupinus spp. Sustainability 10:788. doi: 10.3390/su10030788
Rossi, A., Serraino, I., Dugo, P., Di Paola, R., Mondello, L., Genovese, T., et al. (2012). Protective effects of anthocyanins from blackberry in a rat model of acute lung inflammation. Free Radic. Res. 37, 891–900. doi: 10.1080/1071576031000112690
Rowan, D. D., Cao, M., Lin-Wang, K., Cooney, J. M., Jensen, D. J., Austin, P. T., et al. (2009). Environmental regulation of leaf colour in red 35S:PAP1 Arabidopsis thaliana. New Phytol. 182, 102–115. doi: 10.1111/j.1469-8137.2008.02737.x
Rubiales, D., and Fernández-Aparicio, M. (2012). Innovations in parasitic weeds management in legume crops. A review. Agron. Sustain. Dev. 32, 433–449. doi: 10.1007/s13593-011-0045-x
Rychel, S., and Książkiewicz, M. (2019). Development of gene-based molecular markers tagging low alkaloid pauper locus in white lupin (Lupinus albus L.). J. Appl. Genet. 60, 269–281. doi: 10.1007/s13353-019-00508-9
Saito, K., Suzuki, H., Takamatsu, S., and Murakoshi, I. (1992). Acyltransferases for lupin alkaloids in Lupinus hirsutus. Phytochemistry 32, 87–91. doi: 10.1016/0031-9422(92)80112-R
Sauvaire, Y., Baissac, Y., Leconte, O., Petit, P., and Ribes, G. (1996). “Steroid saponins from fenugreek and some of their biological properties” in Saponins used in food and agriculture. Advances in experimental medicine and biology. eds. G. R. Waller and K. Yamasaki (Boston, MA: Springer).
Sawai, S., and Saito, K. (2011). Triterpenoid biosynthesis and engineering in plants. Front. Plant Sci. 2:25. doi: 10.3389/fpls.2011.00025
Sekhon, J., Grewal, S. K., Singh, I., and Kaur, J. (2017). Evaluation of nutritional quality and antioxidant potential of pigeonpea genotypes. J. Food Sci. Technol. 54, 3598–3611. doi: 10.1007/s13197-017-2818-y
Sepiol, C. J., Yu, J., and Dhaubhadel, S. (2017). Genome-wide identification of Chalcone Reductase gene family in soybean: insight into root-specific GmCHRs and Phytophthora sojae resistance. Front. Plant Sci. 8:2073. doi: 10.3389/fpls.2017.02073
Sharma, A., Shahzad, B., Rehman, A., Bhardwaj, R., Landi, M., and Zheng, B. (2019). Response of phenylpropanoid pathway and the role of polyphenols in plants under abiotic stress. Molecules 24:2452. doi: 10.3390/molecules24132452
Shi, J., Arunasalam, K., Yeung, D., Kakuda, Y., Mittal, G., and Jiang, Y. (2004). Saponins from edible legumes: chemistry, processing, and health benefits. J. Med. Food 7, 67–78. doi: 10.1089/109662004322984734
Shi, Y., Guo, R., Wang, X., Yuan, D., Zhang, S., Wang, J., et al. (2014). The regulation of alfalfa saponin extract on key genes involved in hepatic cholesterol metabolism in hyperlipidemic rats. PLoS One 9:e88282. doi: 10.1371/journal.pone.0088282
Shim, S. I., Jun, W. J., and Kang, B. H. (2003). Evaluation of nutritional and antinutritional components in Korean wild legumes. Plant Foods Hum. Nutr. 58, 1–11. doi: 10.1023/B:QUAL.0000041166.10069.6f
Shiraishi, T., Oku, H., Tsuji, Y., and Ouchi, S. (1978). Inhibitory effect of pisatin on infection process of Mycosphaerella pinodes on pea. Ann. Phytopath. Soc. Japan 44, 641–645.
Shiraiwa, M., Kudo, S., Shimoyamada, M., Harada, K., and Okubo, K. (1991). Composition and structure of “group a saponin” in soybean seed. Agric. Biol. Chem. 55, 315–322. doi: 10.1080/00021369.1991.10870574
Singh, M., Bisht, I. S., Kumar, S., Dutta, M., Bansal, K. C., Karale, M., et al. (2014). Global wild annual Lens collection: a potential resource for lentil genetic base broadening and yield enhancement. PLoS One 9:e107781. doi: 10.1371/journal.pone.0107781
Singh, B., Jatinder, S. P., Kaur, A., and Singh, N. (2017). Phenolic composition and antioxidant potential of grain legume seeds: a review. Food Res. Int. 101, 1–16. doi: 10.1016/j.foodres.2017.09.026
Sinha, R., Bala, M., Kumar, M., Sharma, T. R., and Singh, A. K. (2020). “Methods for screening legume crops for abiotic stress tolerance through physiological and biochemical approaches” in Legume genomics: Methods and protocols, methods in molecular biology. eds. M. Jain and R. Garg (New York: Springer US).
Smallwood, C. J., Nyinyi, C. N., Kopsell, D. A., Sams, C. E., West, D. R., Chen, P., et al. (2014). Detection and confirmation of quantitative trait loci for soybean seed isoflavones. Crop Sci. 54, 595–606. doi: 10.2135/cropsci2013.05.0340
Smýkal, P., Vernoud, V., Blair, M. W., Soukup, A., and Thompson, R. D. (2014). The role of the testa during development and in establishment of dormancy of the legume seed. Front. Plant Sci. 5:351. doi: 10.3389/fpls.2014.00351
Soda, K. (2010). Polyamine intake, dietary pattern, and cardiovascular disease. Med. Hypotheses 75, 299–301. doi: 10.1016/j.mehy.2010.03.008
Song, J., Liu, Z., Hong, H., Ma, Y., Tian, L., Li, X., et al. (2016). Identification and validation of loci governing seed coat color by combining association mapping and bulk segregation analysis in soybean. PLoS One 11:e0159064. doi: 10.1371/journal.pone.0159064
Spaink, H. P. (1995). The molecular basis of infection and nodulation by rhizobia: the ins and outs of sympathogenesis. Annu. Rev. Phytopathol. 33, 345–368. doi: 10.1146/annurev.py.33.090195.002021
Stagnari, F., Maggio, A., Galieni, A., and Pisante, M. (2017). Multiple benefits of legumes for agriculture sustainability: an overview. Chem. Biol. Technol. Agric. 4:2. doi: 10.1186/s40538-016-0085-1
Sugiyama, A., Shitan, N., and Yazaki, K. (2007). Involvement of a soybean ATP-binding cassette-type transporter in the secretion of genistein, a signal flavonoid in legume-Rhizobium symbiosis. Plant Physiol. 144, 2000–2008. doi: 10.1104/pp.107.096727
Sukumaran, A., Mcdowell, T., Chen, L., Renaud, J., and Dhaubhadel, S. (2018). Isoflavonoid-specific prenyltransferase gene family in soybean: GmPT01, a pterocarpan 2-dimethylallyltransferase involved in glyceollin biosynthesis. Plant J. 96, 966–981. doi: 10.1111/tpj.14083
Sundaramoorthy, J., Palaniswamy, S., Park, G. T., Son, H. R., Tsukamoto, C., Lee, J. -D., et al. (2019). Characterization of a new sg-5 variant with reduced biosynthesis of group a saponins in soybean (Glycine max (L.) Merr.). Mol. Breed. 39:144. doi: 10.1007/s11032-019-1066-4
Sundaramoorthy, J., Park, G. T., Lee, J. -D., Kim, J. H., Seo, H. S., and Song, J. T. (2015). Genetic and molecular regulation of flower pigmentation in soybean. J. Korean Soc. Appl. Biol. Chem. 58, 555–562. doi: 10.1007/s13765-015-0077-z
Sweetingham, M., and Kingwell, R. (2008). “Lupins– reflections and future possibilities.” in Proceedings 12th International Lupin Conference; September 14–18, 2008; Geraldton: International Lupin Association, 514–524.
Swiecicki, W., and Swiecicki, W. K. (1995). Domestication and breeding improvement of narrow-leafed lupin (L. angustifolius L.). J. Appl. Genet. 36, 155–167.
Takahashi, Y., Li, X. -H., Tsukamoto, C., and Wang, K. -J. (2016). Identification of a novel variant lacking group a soyasaponin in a Chinese wild soybean (Glycine soja Sieb. & Zucc.): implications for breeding significance. Plant Breed. 135, 607–613. doi: 10.1111/pbr.12403
Tanaka, Y. (2006). Flower colour and cytochromes P450. Phytochem. Rev. 5, 283–291. doi: 10.1007/s11101-006-9003-7
Tanaka, Y., Sasaki, N., and Ohmiya, A. (2008). Biosynthesis of plant pigments: anthocyanins, betalains and carotenoids. Plant J. 54, 733–749. doi: 10.1111/j.1365-313X.2008.03447.x
Taylor, J. L., De Angelis, G., and Nelson, M. N. (2020). “How have narrow-leafed lupin genomic resources enhanced our understanding of lupin domestication” in The lupin genome. eds. K. B. Singh, L. G. Kamphuis, and M. N. Nelson (Cham, Switzerland: Springer International Publishing), 95–108.
Tee, E. S., Goh, A. H., and Khor, S. C. (1995). Carotenoid composition and content of legumes, tubers and starchy roots by HPLC. Malays. J. Nutr. 1, 63–74.
Timmerman-Vaughan, G. M., Frew, T. J., Butler, R., Murray, S., Gilpin, M., Fallon, K., et al. (2004). Validation of quantitative trait loci for Ascochyta blight resistance in pea (Pisum sativum L.), using populations from two crosses. Theor. Appl. Genet. 109, 1620–1631. doi: 10.1007/s00122-004-1779-5
Todd, J. J., and Vodkin, L. O. (1993). Pigmented soybean (Glycine max) seed coats accumulate proanthocyanidins during development. Plant Physiol. 102, 663–670. doi: 10.1104/pp.102.2.663
Todd, J. J., and Vodkin, L. O. (1996). Duplications that suppress and deletions that restore expression from a chalcone synthase multigene family. Plant Cell 8, 687–699. doi: 10.1105/tpc.8.4.687
Tomooka, N., Kashiwaba, K., Vaughan, D. A., Ishimoto, M., and Egawa, Y. (2000). The effectiveness of evaluating wild species: searching for sources of resistance to bruchid beetles in the genus Vigna subgenus Ceratotropis. Euphytica 115, 27–41. doi: 10.1023/A:1003906715119
Toon, A., Cook, L. G., and Crisp, M. D. (2014). Evolutionary consequences of shifts to bird-pollination in the Australian pea-flowered legumes (Mirbelieae and Bossiaeeae). BMC Evol. Biol. 14:43. doi: 10.1186/1471-2148-14-43
Troll, H. J. (1958). Erbgänge des Alkaloidgehaltes und Beobachtungen über Heterosiswirkung bei Lupinus albus. Zeitschrift für Pflanzenzüchtung 39, 35–46.
Tsukamoto, C., Kikuchi, A., Harada, K., Kitamura, K., and Okubo, K. (1993). Genetic and chemical polymorphisms of saponins in soybean seed. Phytochemistry 34, 1351–1356. doi: 10.1016/0031-9422(91)80028-y
Tsuzuki, S., Handa, Y., Takeda, N., and Kawaguchi, M. (2016). Strigolactone-induced putative secreted protein 1 is required for the establishment of symbiosis by the arbuscular mycorrhizal fungus Rhizophagus irregularis. Mol. Plant-Microbe Interact. 29, 277–286. doi: 10.1094/MPMI-10-15-0234-R
Tuteja, J. H., Clough, S. J., Chan, W., and Vodkin, L. O. (2004). Tissue-specific gene silencing mediated by a naturally occurring chalcone synthase gene cluster in Glycine max. Plant Cell 16, 819–835. doi: 10.1105/tpc.021352.1
Tuteja, J. H., Zabala, G., Varala, K., Hudson, M., and Vodkin, L. O. (2009). Endogenous, tissue-specific short interfering RNAs silence the chalcone synthase gene family in Glycine max seed coats. Plant Cell 21, 3063–3077. doi: 10.1105/tpc.109.069856
Uzoh, I. M., Igwe, C. A., Okebalama, C. B., and Babalola, O. O. (2019). Legume-maize rotation effect on maize productivity and soil fertility parameters under selected agronomic practices in a sandy loam soil. Sci. Rep. 9:8539. doi: 10.1038/s41598-019-43679-5
Van den Heuvel, J., and Glazener, J. A. (1975). Comparative abilities of fungi pathogenic and nonpathogenic to bean (Phaseolus vulgaris) to metabolize phaseollin. Neth. J. Plant Pathol. 81, 125–137. doi: 10.1007/BF01976803
Vimolmangkang, S., Han, Y., Wei, G., and Korban, S. S. (2013). An apple MYB transcription factor, MdMYB3, is involved in regulation of anthocyanin biosynthesis and flower development. BMC Plant Biol. 13:176. doi: 10.1186/1471-2229-13-176
von Sengbusch, R. (1942). Sweet lupins and oil lupins. The history of the origin of some new crop plants. Landwirtsch. Jahrb. 91, 719–880.
Voutilainen, S., Nurmi, T., Mursu, J., and Rissanen, T. H. (2006). Carotenoids and cardiovascular health. Am. J. Clin. Nutr. 83, 1265–1271. doi: 10.1093/ajcn/83.6.1265
Walton, J. D. (1997). “Biochemical plant pathology” in Plant biochemistry. eds. P. M. Dey and J. B. Harborne (London, UK: Academic Press), 487–502.
Wan, L., Li, B., Pandey, M. K., Wu, Y., Lei, Y., Yan, L., et al. (2016). Transcriptome analysis of a new peanut seed coat mutant for the physiological regulatory mechanism involved in seed coat cracking and pigmentation. Front. Plant Sci. 7:1491. doi: 10.3389/fpls.2016.01491
Wang, M., Li, W., Fang, C., Xu, F., Liu, Y., Wang, Z., et al. (2018). Parallel selection on a dormancy gene during domestication of crops from multiple families. Nat. Genet. 50, 1435–1441. doi: 10.1038/s41588-018-0229-2
Wang, C., Wang, Y., Shi, Y., Yan, X., He, Y., and Fan, W. (2011). Effects of alfalfa saponins on the lipid metabolism, antioxidation and immunity of weaned piglets. Acta Pratacul. Sin. 20, 210–218.
Wang, C., Zhao, T., and Gai, J. (2010). Genetic variability and evolutionary peculiarity of isoflavone content and its components in soybean germplasm from China. Sci. Agric. Sin. 43, 3919–3929.
Wiesner, M., Hanschen, F. S., Maul, R., Neugart, S., Schreiner, M., and Baldermann, S. (2017). “Nutritional quality of plants for food and fodder” in Encyclopedia of applied plant sciences. eds. B. Thomas and D. J. Murphy (Cambridge, Massachusetts: Academic Press), 285–291.
Wink, M. (2013). Evolution of secondary metabolites in legumes (Fabaceae). S. Afr. J. Bot. 89, 164–175. doi: 10.1016/j.sajb.2013.06.006
Wroth, J. M. (1999). Evidence suggests that Mycosphaerella pinodes infection of Pisum sativum is inherited as a quantitative trait. Euphytica 107, 193–204. doi: 10.1023/A:1003688430893
Xie, M., Chung, C. Y., Li, M., Wong, F., Wang, X., Liu, A., et al. (2019). A reference-grade wild soybean genome. Nat. Commun. 10:1216. doi: 10.1038/s41467-019-09142-9
Yang, K., Jeong, N., Moon, J. K., Lee, Y. H., Lee, S. H., Kim, H. M., et al. (2010). Genetic analysis of genes controlling natural variation of seed coat and flower colors in soybean. J. Hered. 101, 757–768. doi: 10.1093/jhered/esq078
Yazaki, K. (2005). Transporters of secondary metabolites. Curr. Opin. Plant Biol. 8, 301–307. doi: 10.1016/j.pbi.2005.03.011
Yoneyama, K., Akashi, T., and Aoki, T. (2016). Molecular characterization of soybean pterocarpan 2-dimethylallyltransferase in glyceollin biosynthesis: local gene and whole-genome duplications of prenyltransferase genes led to the structural diversity of soybean prenylated isoflavonoids. Plant Cell Physiol. 57, 2497–2509. doi: 10.1093/pcp/pcw178
Yoneyama, K., Xie, X., Sekimoto, H., Takeuchi, Y., Ogasawara, S., Akiyama, K., et al. (2008). Strigolactones, host recognition signals for root parasitic plants and arbuscular mycorrhizal fungi, from Fabaceae plants. New Phytol. 179, 484–494. doi: 10.1111/j.1469-8137.2008.02462.x
Yoshikawa, M., Yamauchi, K., and Masago, H. (1978). Glyceollin: its role in restricting fungal growth in resistant soybean hypocotyls infected with Phytophthora megasperma var. sojae. Physiol. Plant Pathol. 12, 73–82. doi: 10.1016/0048-4059(78)90020-6
Zhang, Q., Blaylock, L. A., and Harrison, M. J. (2010). Two Medicago truncatula half-ABC transporters are essential for arbuscule development in arbuscular mycorrhizal symbiosis. Plant Cell 22, 1483–1497. doi: 10.1105/tpc.110.074955
Zhang, J., Ge, Y., Han, F., Li, B., Yan, S., Sun, J., et al. (2014). Isoflavone content of soybean cultivars from maturity group 0 to VI grown in northern and Southern China. J. Am. Oil Chem. Soc. 91, 1019–1028. doi: 10.1007/s11746-014-2440-3
Zhang, Y., Pechan, T., and Chang, S. K. C. (2018). Antioxidant and angiotensin-I converting enzyme inhibitory activities of phenolic extracts and fractions derived from three phenolic-rich legume varieties. J. Funct. Foods 42, 289–297. doi: 10.1016/j.jff.2017.12.060
Zhou, Y., Huang, J., Zhang, X., Zhu, L., Wang, X., Guo, N., et al. (2018). Overexpression of chalcone isomerase (CHI) increases resistance against Phytophthora sojae in soybean. J. Plant Biol. 61, 309–319. doi: 10.1007/s12374-018-0017-7
Keywords: legume, domestication, secondary metabolite, defense, health benefit
Citation: Ku Y-S, Contador CA, Ng M-S, Yu J, Chung G and Lam H-M (2020) The Effects of Domestication on Secondary Metabolite Composition in Legumes. Front. Genet. 11:581357. doi: 10.3389/fgene.2020.581357
Edited by:
Jiayin Pang, University of Western Australia, AustraliaReviewed by:
Nicolas Rispail, Spanish National Research Council, SpainEric Von Wettberg, University of Vermont, United States
Copyright © 2020 Ku, Contador, Ng, Yu, Chung and Lam. This is an open-access article distributed under the terms of the Creative Commons Attribution License (CC BY). The use, distribution or reproduction in other forums is permitted, provided the original author(s) and the copyright owner(s) are credited and that the original publication in this journal is cited, in accordance with accepted academic practice. No use, distribution or reproduction is permitted which does not comply with these terms.
*Correspondence: Gyuhwa Chung, Y2h1bmdAY2hvbm5hbS5hYy5rcg==; Hon-Ming Lam, aG9ubWluZ0BjdWhrLmVkdS5oaw==