- 1Discipline of Genetics, School of Life Sciences, University of KwaZulu-Natal, Westville, South Africa
- 2Office of Malaria Research, South African Medical Research Council, Cape Town, South Africa
Malaria is a great concern for global health and accounts for a large amount of morbidity and mortality, particularly in Africa, with sub-Saharan Africa carrying the greatest burden of the disease. Malaria control tools such as insecticide-treated bed nets, indoor residual spraying, and antimalarial drugs have been relatively successful in reducing the burden of malaria; however, sub-Saharan African countries encounter great challenges, the greatest being antimalarial drug resistance. Chloroquine (CQ) was the first-line drug in the 20th century until it was replaced by sulfadoxine–pyrimethamine (SP) as a consequence of resistance. The extensive use of these antimalarials intensified the spread of resistance throughout sub-Saharan Africa, thus resulting in a loss of efficacy for the treatment of malaria. SP was replaced by artemisinin-based combination therapy (ACT) after the emergence of resistance toward SP; however, the use of ACTs is now threatened by the emergence of resistant parasites. The decreased selective pressure on CQ and SP allowed for the reintroduction of sensitivity toward those antimalarials in regions of sub-Saharan Africa where they were not the primary drug for treatment. Therefore, the emergence and spread of antimalarial drug resistance should be tracked to prevent further spread of the resistant parasites, and the re-emergence of sensitivity should be monitored to detect the possible reappearance of sensitivity in sub-Saharan Africa.
Introduction
Malaria is a global health concern regarding morbidity and mortality, with approximately 228 million worldwide cases and an estimated 405,000 deaths in 2018 (World Health Organisation (WHO), 2019). Underprivileged, rural populations consisting of young children and pregnant women are disproportionately affected by malaria. The cornerstone of malaria control efforts for the past decade has been to address the inequalities, including the availability of commodities (Taylor et al., 2017). Sub-Saharan Africa experiences the greatest burden of the malaria disease, accounting for approximately 90% of the world’s Plasmodium falciparum infections and deaths with almost all malaria caused by P. falciparum in this area (Yeka et al., 2012; Maitland, 2016). P. falciparum is the most virulent of the malaria parasites that infect humans (Recker et al., 2018). Therefore, the utilization of effective tools for malaria control has increased to lessen the burden of malaria (Greenwood et al., 2005). In spite of increased efforts, many countries in Africa are still confronted with challenges with malaria control, partially as a consequence of the identified limitations in public health structures as well as the infrastructure of primary health care (Xia et al., 2014). The development of resistance toward antimalarial drugs is one of the main challenges when dealing with malaria control and elimination, as such antimalarial drug resistance in a setting where access to health care is limited has severe consequences (Takala-Harrison and Laufer, 2015). Drug pressure, which refers to the extensive and/or misuse of antimalarial drugs, is an identified factor associated with the emergence of resistance; additionally, the misuse or sole use of a drug can encourage selection of resistant strains (Hanboonkunupakarn and White, 2016).
In Africa, malaria control programs rest majorly on vector control and the use of antimalarial drugs (Conrad and Rosenthal, 2019). There are different types of malaria, namely, asymptomatic, uncomplicated, and severe malaria. Asymptomatic malaria refers to malaria parasites being present in the blood, providing a reservoir for transmission, without the individual displaying symptoms (Chourasia et al., 2017). Uncomplicated malaria refers to malaria symptoms presented by a patient together with a positive parasitological test (Grobusch and Kremsner, 2005). Severe malaria is defined by positive parasitological test (microscopy or rapid diagnostic test) detecting P. falciparum and at least one condition for severe disease such as severe anemia or respiratory distress (Conroy et al., 2019).
Sub-Saharan African countries used chloroquine (CQ) as the first-line drug for malaria up to the start of this millennium. However, it was replaced with sulfadoxine–pyrimethamine (SP) after CQ was declared ineffective as a result of resistant parasites (Lusingu and Von Seidlein, 2008) but soon lost its efficacy when parasites began to develop resistance toward it. Mutations in the P. falciparum CQ-resistant transporter (pfcrt) gene and the P. falciparum multidrug-resistance gene 1 (pfmdr-1) have been implicated in CQ resistance (CQR) (Bin Dajem and Al-Qahtani, 2010). CQR was observed on the Thai-Cambodian border and concurrently in South America in the late 1950s (Young and Moore, 1961; Harinasuta et al., 1965). In Southeast Asia, specifically Thailand and Myanmar, drug resistance in local parasite populations is a serious concern, with P. falciparum in the region tending to develop resistance (Parker et al., 2015). Key factors associated with drug resistance in these regions is drug pressure, which results in selection of resistant parasites and their propagation by local transmission and reservoir migration (Wernsdorfer, 1994). In 1978, resistance spread to Africa, with confirmed treatment failures in both Kenya and Tanzania, later reaching West Africa in 1980. CQ continued to be the first-line treatment for uncomplicated P. falciparum malaria in the most sub-Saharan countries until after 2,000 despite its declining use (Flegg et al., 2013). However, some countries, including South Africa, Zambia, Tanzania, and Kenya (D’Alessandro and Buttiëns, 2001; Mwendera et al., 2016), were only forced to switch to SP as the new first-line treatment for malaria, as levels of CQR increased (Gatton et al., 2004). Shortly after its introduction as the new first-line treatment for malaria, polymorphisms in the parasite’s genes—pfdhps [P. falciparum dihydropteroate synthase (DHFR)] and pfdhfr [P. falciparum dihydrofolate reductase (DHPS)]—became widespread throughout Africa (Winstanley et al., 2004). The association of the mutations with SP treatment failure in children made the antimalarial unsuitable for therapy (Desai et al., 2015). In Africa, the use of SP for clinical malaria was stopped as a consequence of its extensive resistance. However, in malaria endemic areas, it is still used for intermittent preventative treatment during pregnancy (Zhao et al., 2020). By 2007, 90% of sub-Saharan Africa had implemented policies of artemisinin-based combination therapy (ACT) for the treatment of uncomplicated malaria as a consequence of widespread CQ and SP resistance (Frosch et al., 2011). The efficacy of ACTs leads to substantial declines in morbidity and mortality in areas with high malaria endemicity. But the success of such therapies is threatened by the appearance of artemisinin-resistant strains of P. falciparum from Thai-Cambodian and Thai-Myanmar borders (Ajayi and Ukwaja, 2013). There have been isolated reports of artemisinin-resistant parasites in sub-Saharan Africa, but they have not become established on the continent yet (Lu et al., 2017a). The emergence and spread of artemisinin-resistant parasites would have an immense impact on Africa’s control efforts (Raman et al., 2019), especially as the world is anticipating a global elimination strategy (World Health Organisation (WHO), 2020). P. falciparum contains Kelch13 (K13), which is needed in the asexual erythrocytic development stage. Although details of its function are not fully known (Siddiqui et al., 2020), they are highly conserved, and single-point mutations are associated with artemisinin resistance (Birnbaum et al., 2020). According to Meshnick (1998), the mechanism of action of artemisinin is in two steps: first, an initial activation that catalyzes the cleavage of endoperoxide produces free radicals. In the second step, these free radicals are responsible for killing the parasites. However, a single-nucleotide polymorphism (SNP) in the gene associated with up-regulated pathway antagonizes the artemisinin oxidation activity (Fairhurst and Dondorp, 2016). A recent study revealed that parasites with inactivated K13 or its mutated form displayed reduced hemoglobin endocytosis (Birnbaum et al., 2020). K13 is important in the uptake and degradation of hemoglobin, which is vital for parasite survival. The mutations and mislocalization of K13 induce artemisinin resistance (Xie et al., 2020); therefore, a close observation of ACT resistance is necessary, as they are the current first-line treatment for P. falciparum malaria (Siddiqui et al., 2020).
A key challenge for malaria control is the emergence and spread of antimalarial drug resistance, particularly in the malaria endemic areas of sub-Saharan Africa where disastrous consequences are observed as a result of the spread of CQ- and SP-resistant P. falciparum strains (von Seidlein and Dondorp, 2015). Furthermore, the development of ACT resistance threatens the control efforts in malaria elimination. This review aims to track the spread of CQ and SP resistance in sub-Saharan Africa. It identifies malaria control strategies employed and ways to combat the challenges being faced to further prevent the emergence and spread of resistant parasites.
Malaria Control in Sub-Saharan Africa
A global public health concern of this century includes controlling vector-borne diseases such as malaria; therefore, much effort has focused on the development of vector control approaches (Tizifa et al., 2018). These approaches encompass methods directed toward the malaria vector by restricting its ability to transmit malaria by shielding areas that are recognized as receptive areas for transmission. Receptivity of the disease is dependent on the vectorial capacity of local vector populations, besides the presence of the vector; this includes the vector population size, biting habits, and the longevity of the sporogony period. These parameters are greatly affected by local ecology, climate, and human and vector behaviors (Smith Gueye et al., 2016). Examples of vector control strategies include insecticide-treated nets (ITNs) and indoor residual spraying (IRS).
In Africa, ITNs are the most extensively used intervention for malaria control, signifying the main tool for vector control in almost all malaria endemic African countries (World Health Organisation (WHO), 2017). ITNs act as a direct barrier to mosquito biting, hence proving effective in the reduction of malaria-related morbidity and mortality. Additionally, they reduce vector density and the average life span by providing community-wide protection through the killing of mosquitoes (Scates et al., 2020). They exploit the indoor feeding and resting behavioral patterns displayed by some Anopheles mosquitoes (Steinhardt et al., 2017). The universal coverage of ITN distribution, with mass distribution campaigns conducted in intervals, is implemented by most national malaria control programs (World Health Organisation (WHO), 2017). More than 800 million ITNs have been distributed in sub-Saharan Africa between 2011 and 2016, to ultimately achieve universal coverage (Olapeju et al., 2018). Through this initiative, a greater proportion (30% in 2010 to 54% in 2016) of Africans in malaria endemic areas slept under ITNs (Olapeju et al., 2018). In sub-Saharan Africa, 67–73% of the total 663 million prevented malaria cases in the past 15 years have been credited to the widespread distribution and use of ITNs (World Health Organisation (WHO), 2017). Another main method used for malaria control on a large scale includes the spraying of houses with insecticides, referred to as IRS. This method has aided the elimination of malaria from large parts of Latin America, Europe, Russia, and Asia. There are also successful IRS programs implemented in parts of Africa (Pluess et al., 2010). It is believed to function by repelling mosquitoes from entering houses as well as through killing female mosquitoes that rest inside houses after taking up a blood meal, thus suggesting that IRS is largely effective against endophilic mosquitoes (those species resting indoors). Additionally, this method is reliant on the vectorial mass effect, which refers to the reduction in transmission as a result of increased mortality of adult vectors typically after feeding (Najera and Zaim, 2001). Considering the slow development and implementation of alternative interventions, ITNs and IRS continue to be the foundation of the malaria control agenda. Consequently, a great challenge is the optimization of the continued use and sustained success of existing ITNs and IRS, while new vector control tools are being studied (Okumu and Moore, 2011).
Antimalarial drugs are used as a malaria control strategy to essentially reduce transmission. During the 20th century, CQ, a safe and inexpensive antimalarial, was a pillar of malaria control and eradication; however, it has lost its effectiveness. Of late, SP was the only alternative extensively available, but the increase and spread of drug resistance have compromised its effectiveness. Resistance to CQ and SP appeared in Southeast Asia, thereafter, spread to Africa (Naidoo and Roper, 2010). Therefore, the World Health Organization (WHO) recommended the implementation of policies approving ACTs as the primary treatment strategy for uncomplicated malaria in the majority of malaria endemic countries (Frosch et al., 2011). In spite of this, according to the 2008 World Malaria Report, ACTs were used to treat only 3% of children with suspected malaria (World Health Organisation (WHO), 2008), implying that CQ and SP were still used to treat malaria in children. Although ACTs are the recommended first-line treatment for malaria in both Asia and Africa, artemisinin-resistant P. falciparum strains have appeared and spread within Southeast Asia, subsequently leading to a reduction in treatment efficacy (Dondorp and Ringwald, 2013). Since Africa holds the greatest burden of malaria, there is a concern regarding the impact of artemisinin resistance on malaria morbidity and mortality (Slater et al., 2016).
The Mechanisms and Contributing Factors Associated With Antimalarial Drug Resistance in Plasmodium falciparum
Drug resistance is a growing problem in the fight to control malaria, as pathogens regularly develop mechanisms that allow them to survive the use of drugs. These mechanisms are generally the result of mutations that affect the drug’s target site, thereby hindering or completely preventing binding between the drug and its target (Table 1). Another way that drug resistance may occur is by the increased levels of the target—this means that more drug is needed to reach inhibition of the parasite.
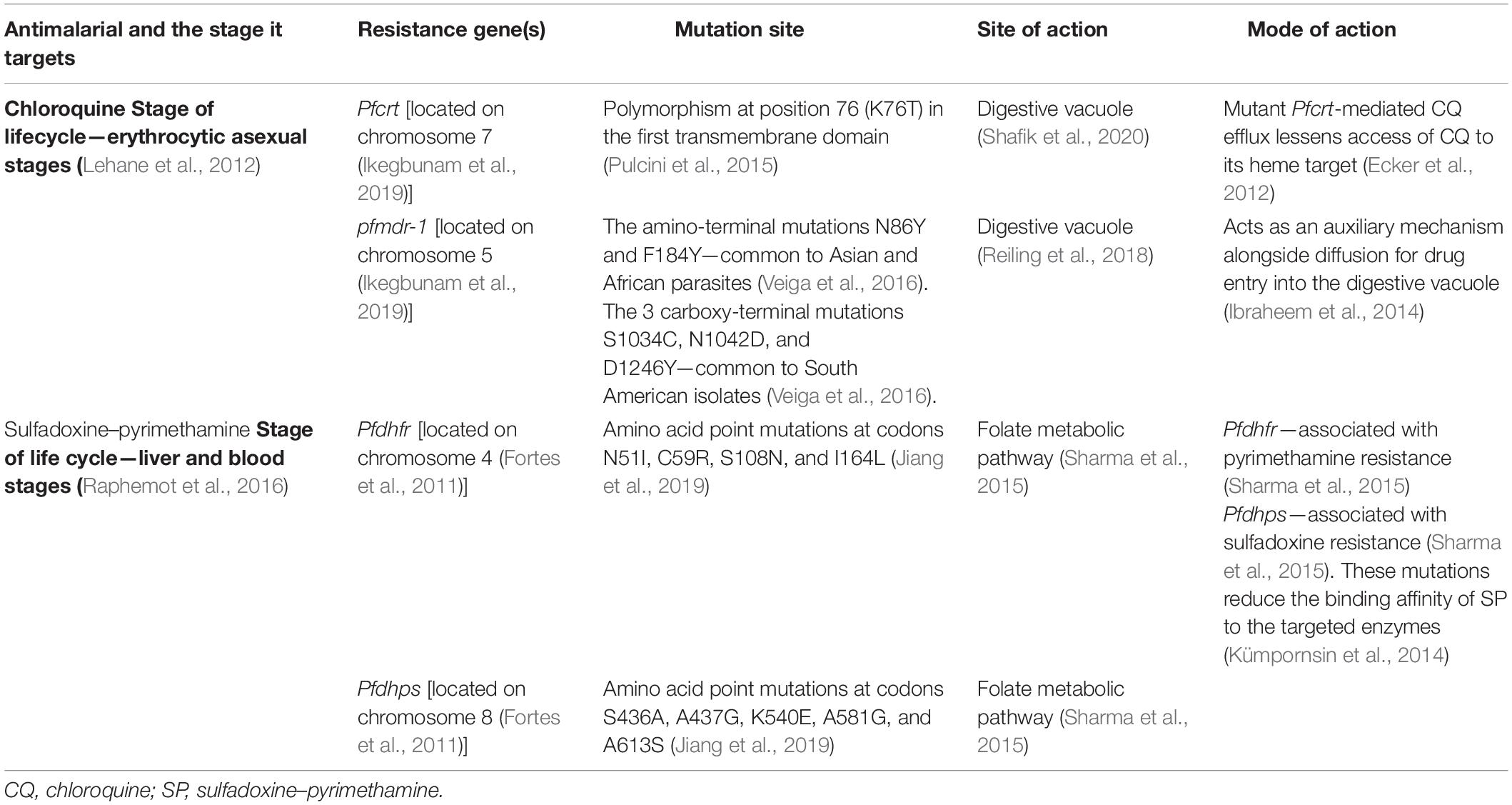
Table 1. Summary of chloroquine- and sulfadoxine–pyrimethamine-resistant genes, their mutation sites, site, and mode of action.
Chloroquine functions by accumulating in the parasite’s acidic food vacuole (Lehane et al., 2012; Lawrenson et al., 2018). The xenobiotic inhibits heme catabolism (i.e., the mechanism by which the malaria parasite “feeds”). Upon infection, host hemoglobin is degraded by the parasite; this releases heme and leads to the ultimate development of an acidic lysosome-like digestive vacuole (Coban, 2020). Downstream, there is accumulation of heme–CQ complexes, which negatively impact parasite survival (Loria et al., 1999; Lehane et al., 2012).
CQ is able to move through biological membranes and accumulate in the acidic digestive vacuole (Ehlgen et al., 2012). This digestive vacuole functions to conduct proteolysis of hemoglobin, which produces dipeptides and ferriprotoporphyrin IX (a substance that is toxic to the parasite at high concentrations). The parasite biocrystallizes ferriprotoporphyrin IX to hemozoin—this is inhibited by use of CQ (Reiling et al., 2018). CQR arises when CQ cannot accumulate at this active site to break the parasite’s hemoglobin degradation cycle that Plasmodium needs (Loria et al., 1999).
CQR is often associated with genes pfcrt and/or pfmdr-1. The P. pfcrt gene is 424-amino acid long and is made up of 10 predicted transmembrane domains (Griffin et al., 2012). It is expressed at all infected erythrocyte stages with maximal expression at the trophozoite stage (Roepe, 2011). In its natural state, CQ is able to permeate the membrane of the food vacuole but becomes protonated in the vacuole and cannot pass the membrane to exit the vacuole (Homewood et al., 1972; Chinappi et al., 2010). As a result, there is a collection of CQ in the vacuole that binds to heme.
However, mutation on the gene increases export of CQ molecules. It has been reported that mutations in pfcrt lead to CQ and hydrogen ions being transported out of the food vacuole, which is where CQ exerts its effects from (Lehane and Kirk, 2008; Ecker et al., 2012). As a result, CQ is not activated and does not perform as it should. This is seen phenotypically as CQR.
Gene pfmdr-1 has also been implicated in CQR. It mediates the production of P-glycoprotein homolog 1. The protein is localized to the food vacuole membrane (Cowman et al., 1991; Njokah et al., 2016). It is a member of a family of proteins that couple ATP hydrolysis to translocation of solutes across cell membranes (Cowman et al., 1991). Structural modeling of pfmdr-1 assesses biophysical mechanisms of this gene and its protein in conferring resistance with respect to aminoquinoline (Ferreira et al., 2011). It has been established that pfmdr-1 is involved in transporting xenobiotics (such as CQ) to the food vacuole. To do this, it is located along the food vacuole membrane and pushes CQ away off the cytosol (Dorsey et al., 2001; Ibraheem et al., 2014). The binding domain is on the cytosol-facing side of the food vacuole, allowing it to first encounter antimalarials—this side is where most resistance-causing mutations occur (Ibraheem et al., 2014). Mutations in pfmdr-1 prevent movement of antimalarials from cytosol into the food vacuole—this reduces potency of some drugs such as CQ, which act in the food vacuole, but drugs that inhibit targets outside the food vacuole can become more potent (Wicht et al., 2020).
SP is an antifolate that is routinely used to treat uncomplicated malaria (Terlouw et al., 2003). Sulfadoxine (SDX) inhibits the activity of DHFR, and pyrimethamine (PYR) inhibits DHPS; these constituents are active against asexual erythrocytic stages of P. falciparum (Sandefur et al., 2007). Both DHFR and DHPS are central in parasite metabolism. Specifically, these enzymes are in the folate metabolic pathway. At the end of the pathway, reduced folate cofactors are produced, which are needed for DNA synthesis and metabolism of particular amino acids (Hyde, 2009).
Dihydrofolate reductase is the third enzyme involved in the folate-synthesis pathway in which it combines pteridine with para-amino benzoic acid to form dihydropteroate (Heinberg and Kirkman, 2015). SDX is a structural analog of para-amino benzoic acid, allowing it to inhibit the DHPS enzyme through competitive inhibition (Nzila, 2006). PYR is a competitive inhibitor of DHFR (Sandefur et al., 2007); thus, in its presence, the folate-metabolism pathway is halted or made less effective. Both SDX and PYR function through competitive inhibition of different targets. In combination, two different enzymes of the same pathway are disrupted and present as resistance to SP.
The Spread of Chloroquine Resistance in Sub-Saharan Africa
Introduced in the mid-1940s, CQ became the most extensively utilized therapeutic antimalarial drug by 1950. Quinine was essentially swapped out for CQ in areas such as tropical Africa, which lacked systematic malaria eradication programs, therefore making it a pillar of presumptive and mass treatment in eradication campaigns (Nuwaha, 2001). CQ was used as the main malaria treatment therapy up to 1990, owing to its safety, efficacy, and low cost. However, CQR emerged in various parts of the world and quickly spread to West Africa in the 1980s and 1990s (Dagnogo et al., 2018) (Figure 1). Treatment failure, particularly in children who are too young to have acquired immunity, in malaria endemic regions is attributed to the presence of CQR (Trape et al., 1998). CQR is primarily related to an SNP in pfcrt, thus leading to an amino acid mutation from threonine to lysine at codon 76 (wild-type K to the mutant T-K76T) (Fidock et al., 2000); additionally, SNPs in exons 2, 3, 4, 6, 9, 10, and 11 of the pfcrt gene display possible association with CQR (Awasthi and Das, 2013). Three main haplotypes occur in codons 72–76 of pfcrt, thus resulting in the wild-type CVMNK and CQR haplotypes CVIET and SVMNT. In Africa, the most dominant mutant haplotype is CVIET (Thomsen et al., 2013). Another mutation conferring resistance to CQ is the N86Y allele of pfmdr-1 (Ebel et al., 2020). The role of pfmdr-1 mutations (N86Y, Y184F, S1034C, and D1246Y) in facilitating in vitro and in vivo CQR has received a lot of interest in research (Oladipo et al., 2015). CQR advances in three particular ways in each newly affected country, including its increasing spread over a number of locations and regions, the increased prevalence of resistant strains in each area, and the increase in the intensity of resistance (Trape, 2001).
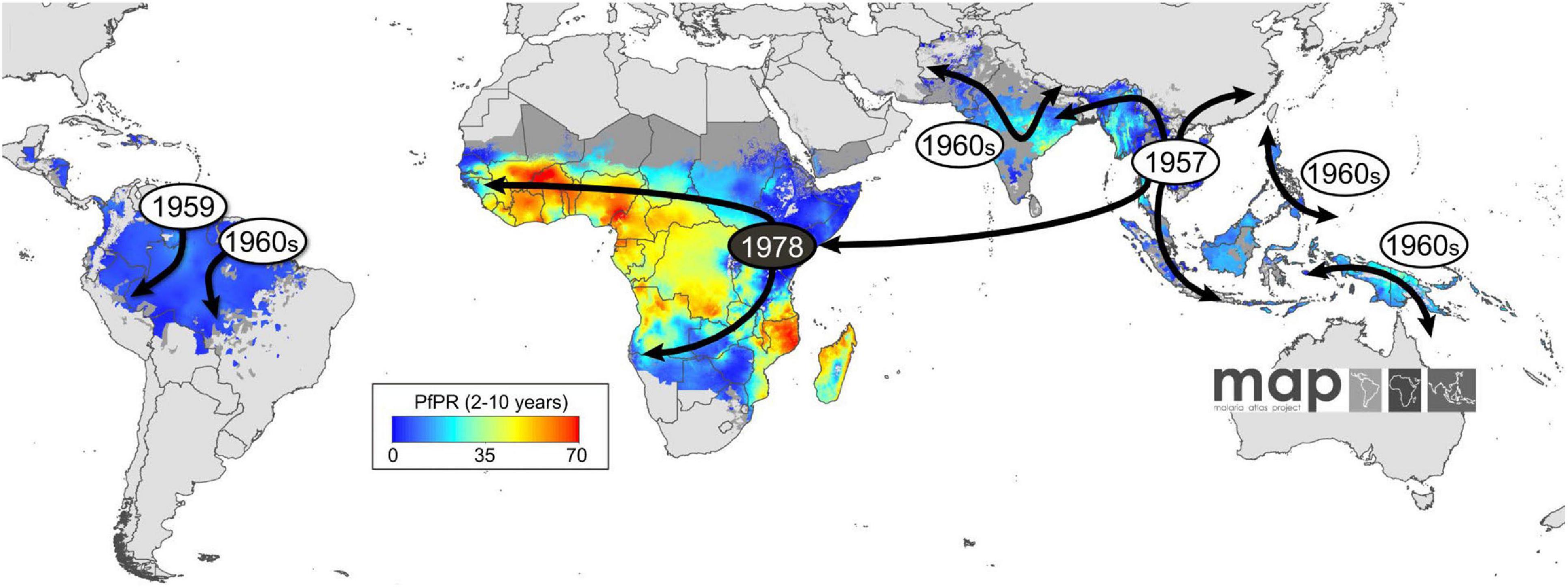
Figure 1. The appearance and global spread of chloroquine resistance (CQR) in P. falciparum. Resistance is thought to have arisen in at least six independent origin (gray circles) and moved progressively as a CQ-driven selective sweep, including from Asia to Africa, where it established itself on the East coast in the late 1970s (black circle). The geographic spread of CQR is overlaid onto a current map of P. falciparum endemicity modeled for 2010. This map was derived from P. falciparum parasite rate (PfPR) surveys, age standardized to the 2- to 10-year age range, using model-based geostatistics (Ecker et al., 2012).
The first reported cases of CQ-resistant infections were confirmed in non-immune tourists in Kenya and Tanzania in 1978, followed by semi−immune Kenyans in 1982 (Shretta et al., 2000). According to Annual Report: Diagnosis, Treatment and Prevention of Malaria: Nairobi, Kenyan Ministry of Health, 61–80% of isolated parasites were resistant to CQ, and 30% of cases treated with CQ experienced clinical treatment failure (Shretta et al., 2000). According to a study, 92% (22 of 24) of isolates assessed for CQR carried the point mutation, Asn to Tyr in pfmdr-1 codon 86, which was the most frequently reported sequence variation related to CQR in Africa. Furthermore, 83% (20 of 24) had an Asp to Tyr mutation, and both mutations were observed in 82% (18 of 24) of the isolates. Both alleles have reported association with CQR. In addition, 75% (18 of 24) of samples each had polymorphisms on both cg2 and pfmdr-l (Omar et al., 2001). Polymorphisms in the cg2 gene, located in the 36-kb region on chromosome 7, are also related to CQR. This gene is characterized by 12 point mutations and three length polymorphisms, namely, kappa, gamma, and omega, and are associated with clones encompassing CQR phenotypes (Viana et al., 2006). Durand et al. (1999) used DNA sequencing to confirm the association of cg2 with CQR. It was found that the cg2 genotype including identical κ14 repeats and particular ω16 repeats displayed a strong association with CQR in all P. falciparum isolates from the African countries included in the study. CQR was heightened during the 1980s but continued to be the first-line treatment for uncomplicated malaria. In 1998, revised guideline for malaria treatment officially replaced CQ with a combination of SDX and PYR (Shretta et al., 2000).
In Tanzania, CQR was first demonstrated in semi-immune citizens in 1982 and in 1983 resistance spread and was reported at 34% among a Zanzibar school population. Furthermore, studies between 1982 and 1985 in various Tanzanian revealed a 20% average in vivo-resistant rate in schoolchildren (Trape, 2001). Consequently, CQ was the official first-line antimalarial drug for uncomplicated malaria until the end of July 2001. The parliament of Tanzania was notified by the Minister of Health, during the 2002–2003 budgetary session, that a decision was made to suspend CQ as the first-line antimalarial drug. The grounds for that decision were based on researched evidence of high cure-rate failure observed for CQ, of approximately 60% (Mubyazi and Gonzalez-Block, 2005).
By 1983, CQR had been reported in several sub-Saharan countries such as Madagascar, Burundi, Sudan, Uganda, Zambia, Comoros, Burkina Faso, Malawi, and Mozambique (Nuwaha, 2001).
The prevalence of CQR in Malawi by 1992 was an estimated 85% (Kublin et al., 2003), therefore prompting Malawi to be the first country in sub-Saharan Africa to terminate the routine use of CQ in 1993. This was supported by the increased failure of CQ treatment and its inability to produce sufficient clinical and hematological recovery. In 1990, more than 80% of Malawian children treated with CQ presented high-level parasitological resistance (Bloland et al., 1993). The removal for CQ in Malawi was supplemented by reduced prevalence of the CQR molecular marker, pfcrt T76, from 85% to 13% between 1992 and 2000 (Kublin et al., 2003). Widespread sensitivity to CQ was observed in Malawi after the removal of CQ as the first-line drug for malaria treatment. In 2005, a clinical trial estimated CQ efficacy at 99%, 12 years after removal. Furthermore, CQ susceptibility was maintained when used for recurring malaria episodes (Takala-Harrison and Laufer, 2015).
In 1999, a 90% prevalence of the pfcrt K76T mutation was identified in infected Mozambican children. A trial in southern Mozambique estimated an average of 47% for CQ’s clinical efficacy between 2001 and 2002. Furthermore, another study revealed more than 90% occurrence of the mutant CVIET haplotype in the same area (Thomsen et al., 2013). This led to the abandonment of CQ treatment for malaria in 2003 (Galatas et al., 2017).
By 1984, CQR was observed in countries including Gabon, Angola, Namibia, Senegal, Zimbabwe, and South Africa (Nuwaha, 2001). A study in Senegal examined the prevalence of pfcrt to determine the molecular level of CQR. A high prevalence of single pfcrt CVMNK wild-type haplotype was observed in the study, and the data suggested that increased prevalence of pfcrt wild types is occurring country-wide (Ndiaye et al., 2012). The use of CQ was abandoned by health authorities in 2003 (Trape et al., 1998). In Zimbabwe, 50% of the population reside in malaria-risk areas, and malaria transmission occurs in 51 out of 59 administrative regions (Mlambo et al., 2007). Until 1983, there were no cases of CQR in Zimbabwe; however, in 1984, the first seven cases were reported in Zambezi Valley. CQR was initially confined to the Zambezi Valley, but by 1990, it was clear that the problem had spread throughout the country (Makono and Sibanda, 1999). According to a study facilitated in the Njelele area of Gokwe following a malaria outbreak, 83% of infections were CQ resistant, with only 3% of cases responding to CQ treatment (Mharakurwa and Mugochi, 1994). The establishment of maintainable national surveillance approaches was necessary to counteract the increasing CQR and monitor its spread throughout the country (Makono and Sibanda, 1999). The clinical failure of CQ was exceptionally high and, thus, only remained the drug of choice until 2000 (Mlambo et al., 2007).
Since 1932, South Africa had faced sporadic malaria epidemics, with KwaZulu Natal, Mpumalanga, and Limpopo bearing the greatest burden within the country (Knight et al., 2009). In 1985, KwaZulu Natal had its first report of in vitro CQR, which spread under persistent CQ pressure, thereby leading to an escalation in cases and treatment failures. In spite of being treated with CQ four times, 3% of malaria-treated patients remained positive (Ukpe et al., 2013). CQ was replaced and no longer used as the first-line drug for malaria treatment by February 1988 (Knight et al., 2009). Malaria parasites stayed susceptible to CQ in Mpumalanga and Limpopo until the mid-to-late 1990s. The number of CQ-resistant parasites escalated, thus prompting the replacement of CQ as the drug of choice in Mpumalanga and Limpopo in 1997 and 1998, respectively (Maharaj et al., 2013).
The Spread of Sulfadoxine–Pyrimethamine Resistance in Sub-Saharan Africa
Africa, most predominantly, sub-Saharan Africa, still remains a home to malaria, bearing high morbidity, mortality, and risk of transmission, in spite of significant reduction in malaria incidence in the region, with children and pregnant women being the most vulnerable (Zareen et al., 2016). Malaria in pregnancy is estimated to cause hundreds of thousand infant deaths every year (Kajubi et al., 2019). Therefore, in 2012, WHO recommended SP as an intermittent preventive treatment (IPT) for fetal malaria prevention in pregnant women across malarious regions (Capan et al., 2010). However, efficacy of chemoprophylaxis with SP was halted by poor compliance of patients, which has led to emergence of drug-resistant strains of P. falciparum (Oyibo and Agomo, 2011).
The SP is a combination comprising SDX, which inhibits DHPS, a bifunctional protein that interacts with hydroxymethylpterin pyrophosphokinase, and PYR, which inhibits DHFR bifunctional protein (Nzila et al., 2000); it, therefore, synergistically inhibits the folate biosynthesis pathway of P. falciparum (Ahmed et al., 2006).
The molecular basis of Plasmodium resistance to SP is shown to be point mutations (Gatton et al., 2004) at seven sites in the DHPS gene (dhfr) that confer resistance to PYR and five sites in the DHFR (dhps) gene that confer resistance to SDX. Different combinations of mutations in each gene lead to varied resistance levels of SP (Pearce et al., 2003). For pfdhps, several point mutations—Ser → Ala at codon 436, Ala → Gly at codon 437, Lys → Glu at codon 540, Ala → Gly at codon 581, and Ser → Phe at codon 436—coupled with either Ala → Thr or Ala → Ser at codon 613 confer resistance to SDX. For pfdhfr, a point mutation of Ser → Asn changes at position 108 (S108N); resistance mutations Asn → Ile at codon 51 and/or Cys → Arg at codon 59 (C59R) and a Ser → Thr mutation at position 108 (S108N) with an Ala → Val change at position 16, synergistically confering PYR resistance in Plasmodium species (Kublin et al., 2003). The DHPS domain of the bifunctional 7,8-dihydro-6-hydroxymethylpterin pyrophosphokinase (PPPK)-dhps enzyme for SDX and the DHFR domain of the bifunctional DHFR-thymidylate synthase (DHFRTS) enzyme for PYR are key targets of SP drug, as these enzymes are responsible for the folic acid biosynthetic pathway (Basco et al., 1998). After approximately 50 years of SP’s introduction, kinetics in children is still poorly understood; therefore, dosing is predominantly based on age for practical reasons, which may result in the administration of inaccurate quantities (De Kock et al., 2018). Increasing resistance to SP is linked to the stepwise acquisition of specific point mutations at specific codons in the dhfrdhps genes, which alter the drug-binding sites of SP (Oyibo and Agomo, 2011). However, the prophylaxis treatment of malaria was selected for double and triple mutations in dhps and dhfr genotypes, respectively, which are implicated in Plasmodium resistance to SP across sub-Saharan Africa (Capan et al., 2010; Sridaran et al., 2010). Accumulation of mutations within the genes (N51I, C59R, and S108N) in pfdhfr and mutations in codons A437G, K540E, and A581G within pfdhps, which confer resistance of Plasmodium to SP (Takala-Harrison and Laufer, 2015), has spread across sub-Saharan Africa (Figure 2).
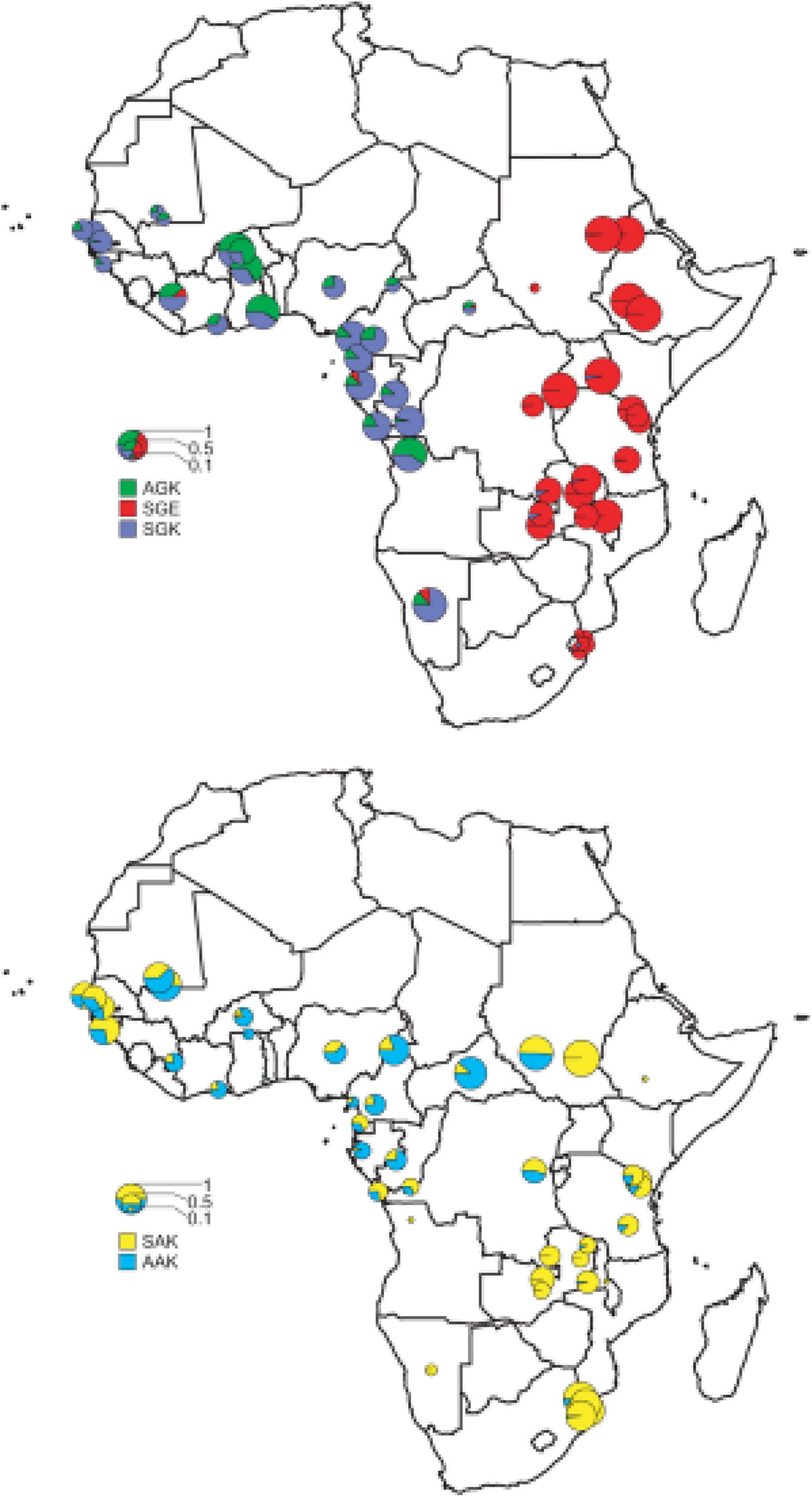
Figure 2. The distribution of the major pfdhps alleles across sub-Saharan Africa. Resistant alleles; the upper map shows the relative proportions of the three major resistance alleles, SGK, AGK, and SGE. Wild-type alleles; the lower map shows the ratio of SAK and AAK alleles among wild-type pfdhps alleles (Pearce et al., 2009).
In Benin, pfdhps, which confers resistance to SDX, contained a mixture of wild-type and mutant alleles (A437 and G437). High mutated alleles of pfdhfr were reported for codons N51I, C59R, and codon S108N. The pfdhfr (N51I, C59R, and S108N) proportion of single, triple, and quadruple mutations was very high in the study population (Ogouyèmi-Hounto et al., 2013). In Nigeria, parasite clearance was observed in pregnant women using SP (Kalanda et al., 2006), with mutations, N51I, C59R, S108N, and the triple mutation conferring a high level of Plasmodium resistance to SP (Iriemenam et al., 2012). A study conducted in Mali between August 2004 and January 2006 and in Ghana in 2003 supported the use of SP + AS (SP and amodiaquine) to treat young children with uncomplicated P. falciparum malaria and IPT in pregnant women (Tagbor et al., 2006; Tekete et al., 2011; Maiga et al., 2015). Additionally, in Mali, prevalence of each of the three pfdhfr mutations at codons N51I, C59R, and S108N was observed in 2007. Notably, pfdhps mutations at codons A437G and K540E as well as pfdhfr triple and pfdhps A437G quadruple mutations were present (Dicko et al., 2010). SP resistance was still quite low (Figueiredo et al., 2008; Dicko et al., 2012) in 2008 but rapidly increased in Mali in 2010 and Burkina Faso in 2012 (Coulibaly et al., 2014). The triple mutant pfdhfr in Africa evolved from the southeast Asian lineage (Coulibaly et al., 2014), while the N51I + S108N double-mutant pfdhfr alleles are a local origin. Both the pfdhps double mutants (Gly-437 and Glu-540) and the pfdhfr triple mutants were individually associated with SP treatment failure in children aged less than 5 years, with pfdhps and pfdhfr quintuple mutations being highly associated with SP treatment failure in Ibadan, Nigeria (Happi et al., 2005). The pfdhfr N51I, C59R, S108N triple mutation had a prevalence close to 100% in Cameroon, western Africa. The most frequent pfdhps mutation there was A437G, with a prevalence of 76.5% and had a higher significant prevalence in pregnant women with SP uptake (Chauvin et al., 2015).
Ghana, a western African country, in 2003 also recorded the quadruple mutation in pfdhps A437G and pfdhfr—N51I, C59R, and S108N (Marks et al., 2005)—but SP was still effective in 2001 for treating uncomplicated P. falciparum malaria whether as monotherapy or combined therapy in Cameroon (Basco et al., 2002). Meanwhile, emergence of SP combination resistance was sustained for many years before introduction of ACTs as a frontline antimalarial in Africa (Marks et al., 2005). There is a strong relationship between protective efficacy and the frequency of resistance mutations, as negative correlation exists between SP prophylaxis efficacy and parasite mutations, indicating that as resistance increases, protective efficacy decreases. This decreased efficacy of SP on IPTi was reported across Ghana and Gabon where decreased efficacy was linked to pfdhfr triple mutation (Griffin et al., 2010). A study in Niger showed a high proportion of pfdhfr N51I, C59R, and S108N haplotypes associated with resistance to PYR and pfdhps S436AFH and A437G mutations associated with reduced susceptibility to SDX (Grais et al., 2018). In 2004, a molecular genotyping study conducted in Laine, Guinea, found three pfdhfr mutations in 85.6% patients and quintuple pfdhfr/pfdhps mutations in 9.6% showing a progressive increase in resistance to SP (Bonnet et al., 2007). No pfdhfr I164L or pfdhps A581G mutations were found, but pfdhps K540E mutation was found in Mali, with a very low prevalence in Mali and Burkina-Faso (Coulibaly et al., 2014).
In Central Africa, between 2002 and 2003, there was adequate clearance of parasitemia in children treated with SP with low presence of pfdhfr S108N, pfdhfr triple, and pfdhfr/pfdhps quadruple mutation genotypes. This shows that studies conducted a decade ago still showed some SP efficacy despite accompanying resistance genotypes. The parasite population of Uige, Angola, revealed high frequency mutations in pfcrt, pfdhps, and pfdhfr, conferring resistance to CQ and SP (Coulibaly et al., 2014).
The frequency of the double A437G, K540E mutant pfdhps allele (conferring SDX resistance) increased from 200% to 300%, and the triple mutant pfdhfr (N51I, C59R, and S108N) allele (conferring PYR resistance) increased by 37–63% in Tanzanian population when SP was used as a first-line antimalarial (Malisa et al., 2010). A study conducted in 2002 in Tanzania confirmed the presence of pfdhfr/pfdhps mutations even when SP was efficient (Mbugi et al., 2006). SP resistance was reported across regions of Tanzania where the triple mutations (N51I, C59R, and S108N) were constantly predominant across all the regions, implying a high level of SP resistance (Schönfeld et al., 2007; Bertin et al., 2011; Baraka et al., 2015), which was corroborated by same result in Benin, a western African country (Bertin et al., 2011). No relationship exists between the number of mutations and the degree of parasitological resistance (Eriksen et al., 2004). Additionally, Tanzania not only reported SP failure, but its prophylaxis was of no significant effect in northern Tanzania in 2006, as triple mutations previously reported in other parts of Africa were also detected (Gesase et al., 2009). The highest mutations of pfdhfr and pfdhps genes were predominantly distributed across eastern and southern Africa where SP use has been the highest (Enosse et al., 2008; Griffin et al., 2010). In 2005, northwest Ethiopia displayed a gradual reduction of individual pfdhps/pfdhfr mutations; triple, quadruple, and quintuple mutations were observed after 5 years of SP withdrawal as a frontline antimalarial (Hailemeskel et al., 2013), but quintupled in western Kenya between 2008 and 2009 (Iriemenam et al., 2012). Moreover, by 2000, the East African Network for Monitoring Antimalarial Treatment (EANMAT) reported SP failure across East Africa, with Rwanda and Burundi exceeding the critical 25% value SP failure rates (Rapuoda et al., 2003); this could be due to a low uptake of SP by pregnant women due to weak health system and sub-optimal implementation policy, among other factors (Martin et al., 2020). The pfdhps A581G and pfdhfr I164L mutations, which confer SP resistance, have been found to be unevenly distributed across East Africa, suggesting a high level of SP resistance, as indicated by the prevalence of the K540E mutation, found across East Africa (Naidoo and Roper, 2011).
Zimbabwe reported high prevalence of pfcrt (K76T) to be 64%, 82%, and 92% in Chiredzi, Kariba, and Bindura, respectively. On the pfdhfr locus, the presence of triple mutations at codons N51I, C59R, and S108N was approximately 50% across the three locations, therefore indicative of widespread prevalence of molecular markers associated with CQ and PYR resistance in Zimbabwe (Schleicher et al., 2018). KwaZulu Natal in South Africa had reported SP resistance in 2000 and thus changed its malaria policy to the use of ACTs for malaria treatment (Knight et al., 2009).
In 2010, the quadruple mutation (A437G, N51I, C59R, and S108N) haplotypes were widespread throughout sub-Saharan Africa, particularly in Uganda, Mali, Kenya, and Malawi (Takechi et al., 2001; Osman et al., 2007), but the quintuple and sextuple mutants were found only in specific regions of Africa (Walker et al., 2017). The quintuple mutants, which plateaued in East and Southern Africa, were relatively rare in west Africa and parts of central Africa. Estimates of the prevalence of the pfdhps-A581G mutation suggest that the sextuple haplotype had become established in Rwanda, Burundi, D R Congo, southwestern Uganda, northwestern Tanzania, southeastern Kenyan, and northeastern Tanzanian borders.
Genotyping of pfdhfr responsible for PYR resistance showed no mutation in codons A16V, C59R, or I164L, while there was 84% mutation at codons N51I and S108N (Osman et al., 2007). Additionally, linkage exists between CQ mutation, pfcrt K76T, SDX mutation, pfdhps K540E, and PYR mutation pfdhfr (N51I and S108N), thus conferring parasite resistance against both CQ and SP. The C59R mutation observed in Mali, Kenya, and Malawi was, however, absent in Sudan in spite of 80% prevalence of pfdhps K540E. Pfdhfr N51I and S108N occurred in more than 80% (Osman et al., 2007). The A437G mutation at pfdhps and the triple mutation (N51I, C59R, and S108N) at pfdhfr associated with SP resistance is concurrent with those found even outside sub-Saharan Africa (Checchi et al., 2002). In six countries of Eastern and Southern Africa, more than 90% prevalence of pfdhps-K540E was implicated in SP resistance (Iriemenam et al., 2012). However, countries like Cameroon, Ghana, Zambia, Mozambique, Mali, and Zimbabwe had three doses of SP in their policy for all pregnant women, which seemed efficacious with resistant genotypes concurrently found in these countries (Mayor et al., 2008; Maiga et al., 2011; Kayentao et al., 2013). The quadruple mutation was uncommon compared with triple mutation, which confers a major resistance to SP in Africa (Peters et al., 2007). Conclusively, a systematic analysis conducted in African countries between 1996 and 2006 reported delayed clearance alluding to SP treatment failure (ter Kuile et al., 2015).
Combating Chloroquine and Sulfadoxine–Pyrimethamine Resistance
Several publications confirm the widespread resistance of CQ and SP, with substantial occurrence in Asian and African countries. The reasons for this hinged on various factors, which include, but is not limited to, the overuse of these antimalarial medications, underdosage of the medications in the treatment of active malaria infection, and high susceptibility of the parasite to adapt at the genetic metabolic levels rapidly (Hyde, 2007). General preventive as well as some of the specific measures have been considered as ways to combat further spread of antimalarial resistance.
Vector Control
Vector control makes use of ITNs/long-lasting insecticidal nets (LLINs) and IRS, which are the mainstay of malaria control. These two strategies have proven useful in the reduction of local malaria transmission by protecting susceptible individuals against infective mosquito bites, thereby leading to a reduction in the level of malaria intensity. When sleeping under the net, the net serves as a means of preventing mosquito contact or bite. Reducing the vector population through this means can indirectly serve as a measure to mitigate the spread of CQ- and SP-resistant Plasmodium. In fact, since 2007, the WHO has recommended universal coverage with ITN (Macintyre et al., 2011; World Health Organisation (WHO), 2011). IRS has also been reportedly effective in reducing the challenge of transmission. It serves as a means to terminating these vectors, thereby reducing the possible malaria morbidity and mortality (World Health Organisation (WHO), 2011). When vector control measures are well applied in combination, it is evident that the outcome of malaria transmission (CQ/SP resistant in this case) will be mitigated (World Health Organisation (WHO), 2010; Thu et al., 2017).
The Introduction of Artemisinin-Based Combination Therapies
An important strategy continues to be the use of effective antimalarials. However, this is threatened by the extensive resistance observed in the parasite toward the most affordable classes of drugs, thus highlighting the need for novel antimalarials (Winstanley et al., 2004) and ultimately a vaccine. The widespread resistance of the known frontline antimalarial drugs—CQ and SP—in April 2002 led to the introduction of ACTs as the first-line treatment for malaria by the WHO. Notably in the treatment of P. falciparum, it is recommended that two or more drugs must be combined. The combined drugs must have different modes of action. Since artemisinin-based compounds (dihydroartemisinin, artemether, and artesunate) are fast acting, they are usually combined with other classes of antimalarial with long half-life such as mefloquine, amodiaquine, lumefantrine, and piperaquine (Bell and Winstanley, 2005). The ACTs, which come in various combinations, is used to replace CQ and SP in order to prevent further increasing morbidity and mortality resulting from the observed resistance in the initial use of first-line medications (World Health Organisation (WHO), 2003; Dalrymple, 2009). The high efficacy and ability to interrupt the development of resistance supported the use of ACTs as the core of malaria treatment (Laxminarayan et al., 2006). The artemisinin component of ACTs has the ability to reduce parasitemia; thus, the progression from uncomplicated malaria to a severe form of the disease can be prevented by early treatment with ACTs, consequently reducing the amount of severe malaria cases and the rate of malaria mortality (Ndeffo Mbah et al., 2015). By June 2008, most malaria endemic nations have adopted the ACTs as its front-line antimalarial medication (World Health Organisation (WHO), 2008). Part of the measures put in place to avoid similar medication resistance as seen in CQ and SP is the disallowance of artemisinin monotherapy medications. This was part of the World Health Assembly resolution of 2007 (World Health Organisation (WHO), 2008).
Medication Adherence and Avoiding Self-Medication
Among humans, there are a variety of beliefs that can impact our behavior significantly. These beliefs are scarcely changed and can therefore affect adherence to antimalarials such as ACTs, thus highlighting the need for patients’ beliefs to be considered when care is offered (Amponsah et al., 2015). Numerous interventions have been developed and employed in malaria endemic areas to promote adherence to medications. Clear guidelines to better adherence can be achieved through deeper understanding of the relative efficacy of each intervention (Fuangchan et al., 2014). The understanding of malaria treatment could be improved by community education, such as training of dispensers and promoting public awareness, thus improving adherence to antimalarials (Denis, 1998). To ensure malaria treatment approaches continue to be effective and improve the possibility of a positive outcome, medication adherence should be evaluated regularly, thereafter used as tools to encourage appropriate action, if necessary (Gerstl et al., 2015).
The CQ/SP resistance developed partly due to widespread presumptuous use of these medications without prior objective confirmation of the diagnosis. Due to the poverty and economic setting of many malaria endemic countries especially in sub-Saharan Africa, most people self-medicate and thereby inappropriately use CQ/SP (Idowu et al., 2006). To this end, the WHO essentially recommended and introduced affordable point-of-care rapid diagnostic tests for definitive malaria testing and diagnosing before the usage of an antimalarial even in under-resourced settings. This is expected to be accompanied by correct and complete usage of the antimalarial especially in this era of ACTs (White et al., 2009; Thu et al., 2017).
Combating the Counterfeit Medication Market
Generally, counterfeit medication presents a great challenge globally, thus contributing to the burden presented by the development of antimalarial drug resistance. Studies done in Eastern DR Congo showed that majority of the CQ has its active ingredient to be significantly underdosed while the SP was overdosed in terms of the active ingredients (Atemnkeng et al., 2007). A similar study in Nigeria corroborated the fact that the menace of counterfeit medication is huge, with 50% of CQ dispensed by street vendors underdosed of the active ingredients (Idowu et al., 2006). In light of this, the development of CQ and SP resistance is substantiated. However, going forward, this can be combatted by action at the policy level. Enacting the laws guiding counterfeit medications and strengthening of the institutions responsible will go a long way to reduce, if not totally remove, counterfeit medications in the society. This can mitigate the CQ/SP resistance in the long run (Yadav and Rawal, 2016). Another way to combat the problem of counterfeit medication is the provision of novel, effective, and affordable antimalarial drugs. This issue is addressed by the Medicines for Malaria Venture, whose aim consists of the discovery, development, and the facilitation of those antimalarials (Medicines for Malaria Venture [MMV], 2020). MMV is a non-profit Swiss foundation, officially introduced in 1999. It was one of the first public–private partnerships created to address the drug innovation gap of malaria (Bathurst and Hentschel, 2006). Developing medicines for children addresses the population most at risk of dying from malaria, thus serving as motivation for MMV to develop child-friendly formulations of current antimalarials and to develop next-generation medicines (Medicines for Malaria Venture [MMV], 2020). In 2009, Novartis and MMV developed an artemether–lumefantrine formulation, called Coartem® Dispersible, adapted to cater to the needs of children affected by P. falciparum malaria and was the first successfully co-developed product launched by the MMV, in which 400 million products were distributed (Premji, 2009; Medicines for Malaria Venture [MMV], 2020). MMV continues to develop and improve access to affordable medicines to lessen the global burden of malaria.
Reintroduction of Chloroquine in the Chloroquine-Sensitive Environment
Various studies have shown that with replacement of CQ/SP as the first line of treatment and subsequent replacement with ACTs, there was notable decrease in the prevalence of pfcrt K76T present in such communities. This was first demonstrated in Malawi in 2004, with the prevalence of pfcrt K76T reaching an undetectable level (Plowe, 2014).
Similar studies have validated this point in China and Zambia, with the outcome of both studies showing a resurgence of CQ sensitivity (Mwanza et al., 2016; Lu et al., 2017b). Considering the role of CQ/SP safety profile and affordability, this looks promising, and reintroduction of CQ/SP may be the way to go in the near future.
Conclusion
In sub-Saharan Africa, where the burden of malaria infection is great, one of the core challenges for malaria control is the emergence and spread of antimalarial drug resistance. Although other strategies are employed for malaria control such as ITNs and IRS, which are the foundation of the malaria control agenda, antimalarial drugs are essential to reduce transmission. However, because of their accessibility and affordability, CQ and SP—both first-line drugs in the past—were used excessively, which resulted in the establishment of resistance, which in turn hindered malaria control in sub-Saharan Africa. This prompted the introduction of ACTs, which decreased the pressure on CQ and SP in some regions of sub-Saharan Africa, thus encouraging their reintroduction in those regions. Ongoing antimalarial drug resistance should be closely monitored in sub-Saharan Africa to prevent the establishment and spread of resistance and to detect the return of sensitivity, which could result in the possible reintroduction of specific antimalarials.
Author Contributions
AR: writing, editing, and collation. LM, OO, and OA: writing and editing. RM: supervision and editing. MA and MO: conceptualization, supervision, editing, and funding.
Funding
This work was supported by the National Research Foundation, (NRF) South Africa (Grant No. 120368).
Conflict of Interest
The authors declare that the research was conducted in the absence of any commercial or financial relationships that could be construed as a potential conflict of interest.
References
Ahmed, A., Lumb, V., Das, M. K., Dev Wajihullah, V., and Sharma, Y. D. (2006). Prevalence of mutations associated with higher levels of sulfadoxine-pyrimethamine resistance in plasmodium falciparum isolates from car nicobar Island and Assam, India. Antimicrob. Agents Chemother. 50, 3934–3938. doi: 10.1128/aac.00732-06
Ajayi, N. A., and Ukwaja, K. N. (2013). Possible artemisinin-based combination therapy-resistant malaria in Nigeria: a report of three cases. Rev. Soc. Bras. Med. Trop. 46, 525–527. doi: 10.1590/0037-8682-0098-2013
Amponsah, A. O., Vosper, H., and Marfo, A. F. A. (2015). Patient related factors affecting adherence to antimalarial medication in an urban estate in ghana. Malar. Res. Treat. 2015, 452539–452539.
Atemnkeng, M. A., Chimanuka, B., and Plaizier-Vercammen, J. (2007). Quality evaluation of chloroquine, quinine, sulfadoxine–pyrimethamine and proguanil formulations sold on the market in East Congo DR. J. Clin. Pharm. Ther. 32, 123–132. doi: 10.1111/j.1365-2710.2007.00797.x
Awasthi, G., and Das, A. (2013). Genetics of chloroquine-resistant malaria: a haplotypic view. Memor. Instit. Oswal. Cruz 108, 947–961. doi: 10.1590/0074-0276130274
Baraka, V., Ishengoma, D. S., Fransis, F., Minja, D. T. R., Madebe, R. A., Ngatunga, D., et al. (2015). High-level Plasmodium falciparum sulfadoxine-pyrimethamine resistance with the concomitant occurrence of septuple haplotype in Tanzania. Mal. J. 14:439.
Basco, L. K., Tahar, R., and Ringwald, P. (1998). Molecular basis of in vivo resistance to sulfadoxine-pyrimethamine in African adult patients infected with Plasmodium falciparum malaria parasites. Antimicr. Agents Chemother. 42, 1811–1814. doi: 10.1128/aac.42.7.1811
Basco, L., Same-Ekobo, A., Ngane, V., Ndounga, M., Metoh, T., Ringwald, P., et al. (2002). Therapeutic efficacy of sulfadoxine-pyrimethamine, amodiaquine and the sulfadoxine-pyrimethamine-amodiaquine combination against uncomplicated Plasmodium falciparum malaria in young children in Cameroon. Bull. World Health Organiz. 80, 538–545.
Bathurst, I., and Hentschel, C. (2006). Medicines for malaria venture: sustaining antimalarial drug development. Trends Parasitol. 22, 301–307. doi: 10.1016/j.pt.2006.05.011
Bell, D., and Winstanley, P. (2005). Current issues in the treatment of uncomplicated malaria in Africa. Br. Med. Bull. 71, 29–43. doi: 10.1093/bmb/ldh031
Bertin, G., Briand, V., Bonaventure, D., Carrieu, A., Massougbodji, A., Cot, M., et al. (2011). Molecular markers of resistance to sulphadoxine-pyrimethamine during intermittent preventive treatment of pregnant women in Benin. Mal. J. 10:196. doi: 10.1186/1475-2875-10-196
Bin Dajem, S. M., and Al-Qahtani, A. (2010). Analysis of gene mutations involved in chloroquine resistance in Plasmodium falciparum parasites isolated from patients in the southwest of Saudi Arabia. Ann. Saudi Med. 30, 187–192. doi: 10.4103/0256-4947.62826
Birnbaum, J., Scharf, S., Schmidt, S., Jonscher, E., Hoeijmakers, W. A. M., Flemming, S., et al. (2020). A Kelch13-defined endocytosis pathway mediates artemisinin resistance in malaria parasites. Science 367:51. doi: 10.1126/science.aax4735
Bloland, P. B., Lackritz, E. M., Kazembe, P. N., Were, J. B., Steketee, R., and Campbell, C. C. (1993). Beyond chloroquine: implications of drug resistance for evaluating malaria therapy efficacy and treatment policy in Africa. J. Infect. Dis. 167, 932–937. doi: 10.1093/infdis/167.4.932
Bonnet, M., Roper, C., Félix, M., Coulibaly, L., Kankolongo, G. M., and Guthmann, J. P. (2007). Efficacy of antimalarial treatment in Guinea: in vivo study of two artemisinin combination therapies in Dabola and molecular markers of resistance to sulphadoxine-pyrimethamine in N’Zérékoré. Malar. J. 6:54.
Capan, M., Mombo-Ngoma, G., Makristathis, A., and Ramharter, M. (2010). Anti-bacterial activity of intermittent preventive treatment of malaria in pregnancy: comparative in vitro study of sulphadoxine-pyrimethamine, mefloquine, and azithromycin. Mal J. 9:303. doi: 10.1186/1475-2875-9-303
Chauvin, P., Menard, S., Iriart, X., Nsango, S. E., Tchioffo, M. T., Abate, L., et al. (2015). Prevalence of Plasmodium falciparum parasites resistant to sulfadoxine/pyrimethamine in pregnant women in Yaoundé, Cameroon: emergence of highly resistant pfdhfr/pfdhps alleles. J. Antimicrob. Chemother. 70, 2566–2571. doi: 10.1093/jac/dkv160
Checchi, F., Durand, R., Balkan, S., Vonhm, B. T., Kollie, J. Z., Biberson, P., et al. (2002). High Plasmodium falciparum resistance to chloroquine and sulfadoxine-pyrimethamine in Harper, Liberia: results in vivo and analysis of point mutations. Transact. R. Soc. Trop. Med. Hygiene 96, 664–669. doi: 10.1016/s0035-9203(02)90346-9
Chinappi, M., Via, A., Marcatili, P., and Tramontano, A. (2010). On the mechanism of chloroquine resistance in Plasmodium falciparum. PLoS One 5:e14064.
Chourasia, M. K., Raghavendra, K., Bhatt, R. M., Swain, D. K., Valecha, N., and Kleinschmidt, I. (2017). Burden of asymptomatic malaria among a tribal population in a forested village of central India: a hidden challenge for malaria control in India. Public Health 147, 92–97. doi: 10.1016/j.puhe.2017.02.010
Coban, C. (2020). The host targeting effect of chloroquine in malaria. Curr. Opin. Immunol. 66, 98–107. doi: 10.1016/j.coi.2020.07.005
Conrad, M. D., and Rosenthal, P. J. (2019). Antimalarial drug resistance in Africa: the calm before the storm? Lancet Infect. Dis. 19, e338–e351.
Conroy, A. L., Datta, D., and John, C. C. (2019). What causes severe malaria and its complications in children? Lessons learned over the past 15 years. BMC Med. 17:52.
Coulibaly, S. O., Kayentao, K., Taylor, S., Guirou, E. A., Khairallah, C., Guindo, N., et al. (2014). Parasite clearance following treatment with sulphadoxine-pyrimethamine for intermittent preventive treatment in Burkina-Faso and Mali: 42-day in vivo follow-up study. Mal. J. 13:41. doi: 10.1186/1475-2875-13-41
Cowman, A. F., Karcz, S., Galatis, D., and Culvenor, J. G. (1991). A P-glycoprotein homologue of Plasmodium falciparum is localized on the digestive vacuole. J. Cell Biol. 113, 1033–1042. doi: 10.1083/jcb.113.5.1033
Dagnogo, O., Ako, A. B., Ouattara, L., Dago, N. D., Coulibaly, D. N. g, Touré, A. O., et al. (2018). Towards a re-emergence of chloroquine sensitivity in Côte d’Ivoire? Mal. J. 17, 413–413.
D’Alessandro, U., and Buttiëns, H. (2001). History and importance of antimalarial drug resistance. Trop Med. Int. Health 6, 845–848. doi: 10.1046/j.1365-3156.2001.00819.x
Dalrymple, D. G. (2009). Artemisia Annua, Artemisinin, ACTs and Malaria Control in Africa: The Interplay of Tradition, Science and Public Policy, Working Paper. Geneva: Medicines for Malaria Venture.
De Kock, M., Tarning, J., Workman, L., Allen, E. N., Tekete, M. M., Djimde, A. A., et al. (2018). Population pharmacokinetic properties of sulfadoxine and pyrimethamine: a pooled analysis to inform optimal dosing in african children with uncomplicated Malaria. Antimicr. Agents Chemother. 62:e01370-17.
Denis, M. B. (1998). Improving compliance with quinine + tetracycline for treatment of malaria: evaluation of health education interventions in Cambodian villages. Bull. World Health Organ. 76(Suppl. 1), 43–49.
Desai, M., Gutman, J., Taylor, S. M., Wiegand, R. E., Khairallah, C., Kayentao, K., et al. (2015). Impact of sulfadoxine-pyrimethamine resistance on effectiveness of intermittent preventive therapy for malaria in pregnancy at clearing infections and preventing low birth weight. Clin. Infect. Dis. 62, 323–333. doi: 10.1093/cid/civ881
Dicko, A., Konare, M., Traore, D., Testa, J., Salamon, R., Doumbo, O., et al. (2012). The implementation of malaria intermittent preventive trialtreatment with sulphadoxine-pyrimethamine in infants reduced all-cause mortality in the district of Kolokani, Mali: results from a cluster randomized control. Mal. J. 11:73.
Dicko, A., Sagara, I., Djimdé, A. A., Touré, S. O., Traore, M., Dama, S., et al. (2010). Molecular markers of resistance to sulphadoxine-pyrimethamine one year after implementation of intermittent preventive treatment of malaria in infants in Mali. Mal. J. 9:9.
Dondorp, A. M., and Ringwald, P. (2013). Artemisinin resistance is a clear and present danger. Trends Parasitol 29, 359–360. doi: 10.1016/j.pt.2013.05.005
Dorsey, G., Kamya, M. R., Singh, A., and Rosenthal, P. J. (2001). Polymorphisms in the Plasmodium falciparum pfcrt and pfmdr-1 genes and clinical response to chloroquine in Kampala, Uganda. J. Infect Dis. 183, 1417–1420.
Durand, R., Gabbett, E., Di Piazza, J-P., Delabre, J-F., and Le Bras, J. (1999). Analysis of κ and ω repeats of the cg2 gene and chloroquine susceptibility in isolates of Plasmodium falciparum from sub-Saharan Africa. Mol. Biochem. Parasitol. 101, 185–197. doi: 10.1016/s0166-6851(99)00073-0
Ebel, E. R., Reis, F., Petrov, D. A., and Beleza, S. (2020). Shifts in antimalarial drug policy since 2006 have rapidly selected P. falciparum resistance alleles in Angola. doi: 10.1101/2020.09.29.310706
Ecker, A., Lehane, A. M., Clain, J., and Fidock, D. A. (2012). pfcrt and its role in antimalarial drug resistance. Trends Parasitol. 28, 504–514. doi: 10.1016/j.pt.2012.08.002
Ehlgen, F., Pham, J. S., de Koning-Ward, T., Cowman, A. F., and Ralph, S. A. (2012). Investigation of the Plasmodium falciparum food vacuole through inducible expression of the chloroquine resistance transporter (PfCRT). PLoS One 7:e38781. doi: 10.1371/journal.pone.0038781
Enosse, S., Magnussen, P., Abacassamo, F., ómez-Olivé, X. G., Rønn, A. M., Thompson, R., et al. (2008). Rapid increase of Plasmodium falciparum dhfr/dhps resistant haplotypes, after the adoption of sulphadoxine-pyrimethamine as first line treatment in 2002, in southern Mozambique. Mal. J. 7:115.
Eriksen, J., Mwankusye, S., Mduma, S., Kitua, A., Swedberg, G., Tomson, G., et al. (2004). Patterns of resistance and DHFR/DHPS genotypes of Plasmodium falciparum in rural Tanzania prior to the adoption of sulfadoxine—pyrimethamine as first-line treatment. Transact. R. Soc. Trop. Med. Hyg. 98, 347–353. doi: 10.1016/j.trstmh.2003.10.010
Fairhurst, R. M., and Dondorp, A. M. (2016). Artemisinin-resistant Plasmodium falciparum malaria. Microbiol. Spect. 4:13. doi: 10.1128/microbiolspec.EI1110-0013-2016
Ferreira, P. E., Holmgren, G., Veiga, M. I., Uhlén, P., Kaneko, A., and Gil, J. P. (2011). Pfmdr1: Mechanisms of Transport Modulation by Functional Polymorphisms. PLoS One 6:e23875. doi: 10.1371/journal.pone.0023875
Fidock, D. A., Nomura, T., Talley, A. K., Cooper, R. A., Dzekunov, S. M., Ferdig, M. T., et al. (2000). Mutations in the P. falciparum digestive vacuole transmembrane protein PfCRT and evidence for their role in chloroquine resistance. Mol. Cell 6, 861–871. doi: 10.1016/s1097-2765(05)00077-8
Figueiredo, P., Benchimol, C., Lopes, D., Bernardino, L., do Rosário, V. E., Varandas, L., et al. (2008). Prevalence of pfmdr1, pfcrt, pfdhfr and pfdhps mutations associated with drug resistance, in Luanda, Angola. Mal. J. 7:236.
Flegg, J. A., Metcalf, C. J. E., Gharbi, M., Venkatesan, M., Shewchuk, T., Hopkins Sibley, C., et al. (2013). Trends in antimalarial drug use in Africa. Am. J. Trop. Med. Hyg. 89, 857–865.
Fortes, F., Dimbu, R., Figueiredo, P., Neto, Z., do Rosário, V. E., and Lopes, D. (2011). Evaluation of prevalence’s of pfdhfr and pfdhps mutations in Angola. Malaria J. 10:22. doi: 10.1186/1475-2875-10-22
Frosch, A. E. P., Venkatesan, M., and Laufer, M. K. (2011). Patterns of chloroquine use and resistance in sub-Saharan Africa: a systematic review of household survey and molecular data. Mal. J. 10:116. doi: 10.1186/1475-2875-10-116
Fuangchan, A., Dhippayom, T., and Kongkaew, C. (2014). Intervention to promote patients’ adherence to antimalarial medication: a systematic review. Am. J. Trop. Med. Hyg. 90, 11–19. doi: 10.4269/ajtmh.12-0598
Galatas, B., Nhamussua, L., Candrinho, B., Mabote, L., Cisteró, P., Gupta, H., et al. (2017). In-Vivo efficacy of chloroquine to clear asymptomatic infections in mozambican adults: a randomized, placebo-controlled trial with implications for elimination strategies. Sci. Rep. 7, 1356–1356.
Gatton, M. L., Martin, L. B., and Cheng, Q. (2004). Evolution of resistance to sulfadoxine-pyrimethamine in Plasmodium falciparum. Antimicrob Agents Chemother. 48, 2116–2123. doi: 10.1128/aac.48.6.2116-2123.2004
Gerstl, S., Namagana, A., Palacios, L., Mweshi, F., Aprile, S., and Lima, A. (2015). High adherence to malaria treatment: promising results of an adherence study in South Kivu, Democratic Republic of the Congo. Mal. J. 14:414.
Gesase, S., Gosling, R. D., Hashim, R., Ord, R., Naidoo, I., Madebe, R., et al. (2009). High resistance of Plasmodium falciparum to sulphadoxine/pyrimethamine in northern Tanzania and the emergence of dhps resistance mutation at Codon 581. PLoS One 4:e4569. doi: 10.1371/journal.pone.0004569
Grais, R. F. I., Laminou, M., Woi-Messe, L., Makarimi, R., Bouriema, S. H., Langendorf, C., et al. (2018). Molecular markers of resistance to amodiaquine plus sulfadoxine–pyrimethamine in an area with seasonal malaria chemoprevention in south central Niger. Mal. J. 17:98.
Greenwood, B. M., Bojang, K., Whitty, C. J., and Targett, G. A. (2005). Malaria. Lancet 365, 1487–1498.
Griffin, C. E., Hoke, J. M., Samarakoon, U., Duan, J., Mu, J., Ferdig, M. T., et al. (2012). Mutation in the Plasmodium falciparum CRT protein determines the stereospecific activity of antimalarial cinchona alkaloids. Antimicrob Agents Chemother. 56, 5356–5364. doi: 10.1128/aac.05667-11
Griffin, J. T., Cairns, M., Ghani, A. C., Roper, C., Schellenberg, D., Carneiro, I., et al. (2010). Protective efficacy of intermittent preventive treatment of malaria in infants (IPTi) using sulfadoxine-pyrimethamine and parasite resistance. PLoS One 5:e12618. doi: 10.1371/journal.pone.0012618
Grobusch, M. P., and Kremsner, P. G. (2005). Uncomplicated malaria. Curr. Top. Microbiol. Immunol. 295, 83–104.
Hailemeskel, E., Kassa, M., Taddesse, G., Mohammed, H., Woyessa, A., Tasew, G., et al. (2013). Prevalence of sulfadoxine-pyrimethamine resistance-associated mutations in dhfr and dhps genes of Plasmodium falciparum three years after SP withdrawal in Bahir Dar, Northwest Ethiopia. Acta Trop. 128, 636–641. doi: 10.1016/j.actatropica.2013.09.010
Hanboonkunupakarn, B., and White, N. J. (2016). The threat of antimalarial drug resistance. Trop. Dis. Travel Med. Vacc. 2:10.
Happi, C. T., Gbotosho, G. O., Folarin, O. A., Akinboye, D. O., Yusuf, B. O., Ebong, O. O., et al. (2005). Polymorphisms in Plasmodium falciparum dhfr and dhps genes and age related in vivo sulfadoxine–pyrimethamine resistance in malaria-infected patients from Nigeria. Acta Trop. 95, 183–193. doi: 10.1016/j.actatropica.2005.06.015
Harinasuta, T., Suntharasamai, P., and Viravan, C. (1965). Chloroquine-resistant falciparum malaria in Thailand. Lancet 2, 657–660. doi: 10.1016/s0140-6736(65)90395-8
Heinberg, A., and Kirkman, L. (2015). The molecular basis of antifolate resistance in Plasmodium falciparum: looking beyond point mutations. Annal. N. Y. Acad. Sci. 1342, 10–18. doi: 10.1111/nyas.12662
Homewood, C. A., Warhurst, D. C., Peters, W., and Baggaley, V. C. (1972). Lysosomes, pH and the Anti-malarial action of chloroquine. Nature 235, 50–52. doi: 10.1038/235050a0
Hyde, J. E. (2009). Exploring the folate pathway in Plasmodium falciparum. Acta Trop. 94, 191–206. doi: 10.1016/j.actatropica.2005.04.002
Hyde, J. E. (2007). Drug-resistant malaria - an insight. FEBS J. 274, 4688–4698. doi: 10.1111/j.1742-4658.2007.05999.x
Ibraheem, Z. O., Abd Majid, R., Noor, S. M., Sedik, H. M., and Basir, R. (2014). Role of different pfcrt and pfmdr-1 mutations in conferring resistance to antimalaria drugs in Plasmodium falciparum. Mal. Res. Treat. 2014:950424.
Idowu, O. A., Apalara, S. B., and Lasisi, A. A. (2006). Assessment of quality of chloroquine tablets sold by drug vendors in Abeokuta, Nigeria. Tanzan Health Res. Bull. 8, 45–46.
Ikegbunam, M. N., Nkonganyi, C. N., Thomas, B. N., Esimone, C. O., Velavan, T. P., and Ojurongbe, O. (2019). Analysis of Plasmodium falciparumpfcrt and pfmdr1 genes in parasite isolates from asymptomatic individuals in Southeast Nigeria 11 years after withdrawal of chloroquine. Malaria J. 18:343. doi: 10.1186/s12936-019-2977-6
Iriemenam, N. C., Shah, M., Gatei, W., van Eijk, A. M., Ayisi, J., Kariuki, S., et al. (2012). Temporal trends of sulphadoxine-pyrimethamine (SP) drug-resistance molecular markers in Plasmodium falciparum parasites from pregnant women in western Kenya. Mal. J. 11:134. doi: 10.1186/1475-2875-11-134
Jiang, T., Chen, J., Fu, H., Wu, K., Yao, Y., Eyi, J. U. M., et al. (2019). High prevalence of pfdhfr-pfdhps quadruple mutations associated with sulfadoxine-pyrimethamine resistance in Plasmodium falciparum isolates from Bioko Island, Equatorial Guinea. Malaria J. 18:101. doi: 10.1186/s12936-019-2734-x
Kajubi, R., Ochieng, T., Kakuru, A., Jagannathan, P., Nakalembe, M., Ruel, T., et al. (2019). Monthly sulfadoxine-pyrimethamine versus dihydroartemisinin-piperaquine for intermittent preventive treatment of malaria in pregnancy: a double-blind, randomised, controlled, superiority trial. Lancet 393, 1428–1439. doi: 10.1016/s0140-6736(18)32224-4
Kalanda, G. C., Hill, J., Verhoeff, F. H., and Brabin, B. J. (2006). Comparative efficacy of chloroquine and sulphadoxine–pyrimethamine in pregnant women and children: a meta-analysis. Trop Med. Int. Health 11, 569–577. doi: 10.1111/j.1365-3156.2006.01608.x
Kayentao, K., Garner, P., Maria van Eijk, A., Naidoo, I., Roper, C., Mulokozi, A., et al. (2013). Intermittent preventive therapy for malaria during pregnancy using 2 vs 3 or more doses of sulfadoxine-pyrimethamine and risk of low birth weight in africa: systematic review and meta-analysis. JAMA 309, 594–604. doi: 10.1001/jama.2012.216231
Knight, S. E., Anyachebelu, E. J., Geddes, R., and Maharaj, R. (2009). Impact of delayed introduction of sulfadoxine–pyrimethamine and arthemeter–lumefantrine on malaria epidemiology in KwaZulu-Natal, South Africa. Trop Med. Int. Health 14, 1086–1092. doi: 10.1111/j.1365-3156.2009.02333.x
Kublin, J. G., Cortese, J. F., Njunju, E. M., Mukadam, R. A., Wirima, J. J., Kazembe, P. N., et al. (2003). Reemergence of chloroquine-sensitive Plasmodium falciparum malaria after cessation of chloroquine use in Malawi. J. Infect. Dis. 187, 1870–1875. doi: 10.1086/375419
Kümpornsin, K., Modchang, C., Heinberg, A., Ekland, E. H., Jirawatcharadech, P., Chobson, P., et al. (2014). Origin of robustness in generating drug-resistant malaria parasites. Mol. Biol. Evol. 31, 1649–1660. doi: 10.1093/molbev/msu140
Lawrenson, A., Cooper, D., O’Neill, P., and Berry, N. (2018). Study of the antimalarial activity of 4-aminoquinoline compounds against chloroquine-sensitive and chloroquine-resistant parasite strains. J. Mol. Model. 24:237. doi: 10.1007/s00894-018-3755-z
Laxminarayan, R., Over, M., and Smith, D. L. (2006). Will a global subsidy of new antimalarials delay the emergence of resistance and save lives? Health Aff. (Millwood) 25, 325–336. doi: 10.1377/hlthaff.25.2.325
Lehane, A. M., and Kirk, K. (2008). Chloroquine resistance-conferring mutations in pfcrt give rise to a chloroquine-associated H+ leak from the malaria parasite’s digestive vacuole. Antimicrob Agents Chemothe.r 52, 4374–4380. doi: 10.1128/aac.00666-08
Lehane, A. M., McDevitt, C. A., Kirk, K., and Fidock, D. A. (2012). Degrees of chloroquine resistance in Plasmodium - is the redox system involved? Int. J. Parasitol. Drugs Drug Resist. 2, 47–57. doi: 10.1016/j.ijpddr.2011.11.001
Loria, P., Miller, S., Foley, M., and Tilley, L. (1999). Inhibition of the peroxidative degradation of haem as the basis of action of chloroquine and other quinoline antimalarials. Biochem. J. 339(Pt 2), 363–370. doi: 10.1042/0264-6021:3390363
Lu, F., Culleton, R., Zhang, M., Ramaprasad, A., von Seidlein, L., Zhou, H., et al. (2017b). Emergence of indigenous artemisinin-resistant Plasmodium falciparum in Africa. N. Engl. J. Med. 376, 991–993.
Lu, F., Zhang, M., Culleton, R. L., Xu, S., Tang, J., Zhou, H., et al. (2017a). Return of chloroquine sensitivity to Africa? Surveillance of African Plasmodium falciparum chloroquine resistance through malaria imported to China. Paras. Vect. 10, 355.
Lusingu, J. P., and Von Seidlein, L. (2008). Challenges in malaria control in sub-Saharan Africa: the vaccine perspective. Tanzan J. Health Res. 10, 253–266.
Macintyre, K., Littrell, M., Keating, J., Hamainza, B., Miller, J., and Eisele, T. P. (2011). Determinants of hanging and use of ITNs in the context of near universal coverage in Zambia. Health Policy Plann. 27, 316–325. doi: 10.1093/heapol/czr042
Maharaj, R., Raman, J., Morris, N., Moonasar, D., Durrheim, D. N., Seocharan, I., et al. (2013). Epidemiology of malaria in South Africa: From control to elimination. SAMJ South Afr. Med. J. 103, 779–783. doi: 10.7196/samj.7441
Maiga, H., Djimde, A. A., Beavogui, A. H., Toure, O., Tekete, M., Sangare, C. P. O., et al. (2015). Efficacy of sulphadoxine-pyrimethamine + artesunate, sulphadoxine-pyrimethamine + amodiaquine, and sulphadoxine-pyrimethamine alone in uncomplicated falciparum malaria in Mali. Mal. J. 14:64. doi: 10.1186/s12936-015-0557-y
Maiga, O. M., Kayentao, K., Traoré, B. T., Djimde, A., Traoré, B., Diallo, M., et al. (2011). Superiority of 3 Over 2 doses of intermittent preventive treatment with sulfadoxine-pyrimethamine for the prevention of malaria during pregnancy in mali: a randomized controlled trial. Clin. Infect. Dis. 53, 215–223. doi: 10.1093/cid/cir374
Maitland, K. (2016). Severe malaria in african children – the need for continuing investment. N. Engl. J. Med. 375, 2416–2417. doi: 10.1056/nejmp1613528
Makono, R., and Sibanda, S. (1999). Review of the prevalence of malaria in Zimbabwe with specific reference to parasite drug resistance (1984-96). Trans. R. Soc. Trop. Med. Hyg. 93, 449–452. doi: 10.1016/s0035-9203(99)90331-0
Malisa, A. L., Pearce, R. J., Abdulla, S., Mshinda, H., Kachur, P. S., Bloland, P., et al. (2010). Drug coverage in treatment of malaria and the consequences for resistance evolution – evidence from the use of sulphadoxine/pyrimethamine. Mal. J. 9:190.
Marks, F., Evans, J., Meyer, C. G., Browne, E. N., Flessner, C., von Kalckreuth, V., et al. (2005). High prevalence of markers for sulfadoxine and pyrimethamine resistance in Plasmodium falciparum in the absence of drug pressure in the Ashanti region of Ghana. Antimicrob Agents Chemother. 49, 1101–1105. doi: 10.1128/aac.49.3.1101-1105.2005
Martin, M. K., Venantius, K. B., Patricia, N., Bernard, K., Keith, B., Allen, K., et al. (2020). Correlates of uptake of optimal doses of sulfadoxine-pyrimethamine for prevention of malaria during pregnancy in East-Central Uganda. Mal. J. 19:153.
Mayor, A., Serra-Casas, E., Sanz, S., Aponte, J. J., Macete, E., Mandomando, I., et al. (2008). Molecular Markers of resistance to sulfadoxine-pyrimethamine during intermittent preventive treatment for malaria in mozambican infants. J. Infect. Dis. 197, 1737–1742. doi: 10.1086/588144
Mbugi, E. V., Mutayoba, B. M., Malisa, A. L., Balthazary, S. T., Nyambo, T. B., and Mshinda, H. (2006). Drug resistance to sulphadoxine-pyrimethamine in Plasmodium falciparum malaria in Mlimba, Tanzania. Mal. J. 5:94.
Medicines for Malaria Venture (MMV) (2020). Available online at: www.mmv.org (accessed October 27, 2020).
Meshnick, S. R. (1998). Artemisinin antimalarials: mechanisms of action and resistance. Med. Trop. (Mars) 58(Suppl. 3), 13–17.
Mharakurwa, S., and Mugochi, T. (1994). Chloroquine-resistant falciparum malaria in an area of rising endemicity in Zimbabwe. J. Trop. Med. Hyg. 97, 39–45.
Mlambo, G., Sullivan, D., Mutambu, S. L., Soko, W., Mbedzi, J., Chivenga, J., et al. (2007). High prevalence of molecular markers for resistance to chloroquine and pyrimethamine in Plasmodium falciparum from Zimbabwe. Parasitol. Res. 101, 1147–1151. doi: 10.1007/s00436-007-0597-5
Mubyazi, G. M., and Gonzalez-Block, M. A. (2005). Research influence on antimalarial drug policy change in Tanzania: case study of replacing chloroquine with sulfadoxine-pyrimethamine as the first-line drug. Mal. J. 4, 51–51.
Mwanza, S., Joshi, S., Nambozi, M., Chileshe, J., Malunga, P., Kabuya, J-B., et al. (2016). The return of chloroquine-susceptible Plasmodium falciparum malaria in Zambia. Malaria J. 15, 584–584. doi: 10.1186/s12936-016-1637-3
Mwendera, C., de Jager, C., Longwe, H., Phiri, K., Hongoro, C., and Mutero, C. M. (2016). Malaria research and its influence on anti-malarial drug policy in Malawi: a case study. Health Res. Policy Syst. 14:41. doi: 10.1186/s12961-016-0108-1
Naidoo, I., and Roper, C. (2010). Following the path of most resistance: dhps K540E dispersal in African Plasmodium falciparum. Trends Parasitol. 26, 447–456. doi: 10.1016/j.pt.2010.05.001
Naidoo, I., and Roper, C. (2011). Drug resistance maps to guide intermittent preventive treatment of malaria in African infants. Parasitology 138, 1469–1479. doi: 10.1017/s0031182011000746
Najera, J. A., and Zaim, M. (2001). Malaria Vector Control – Insecticides for Indoor Residual Spraying. Geneva: World Health Organization Communicable Disease Control, Prevention and Eradication.
Ndeffo Mbah, M. L., Parikh, S., and Galvani, A. P. (2015). Comparing the impact of artemisinin-based combination therapies on malaria transmission in sub-Saharan Africa. Am. J. Trop. Med. Hyg. 92, 555–560. doi: 10.4269/ajtmh.14-0490
Ndiaye, M., Faye, B., Tine, R., Ndiaye, J. L., Lo, A., Abiola, A., et al. (2012). Assessment of the molecular marker of Plasmodium falciparum chloroquine resistance (Pfcrt) in Senegal after several years of chloroquine withdrawal. Am. J. Trop. Med. Hyg. 87, 640–645. doi: 10.4269/ajtmh.2012.11-0709
Njokah, M. J., Kang’ethe, J. N., Kinyua, J., Kariuki, D., and Kimani, F. T. (2016). In vitro selection of Plasmodium falciparum pfcrt and pfmdr1 variants by artemisinin. Malar. J. 15:381.
Nuwaha, F. (2001). The challenge of chloroquine-resistant malaria in sub-Saharan Africa. Health Policy Plann. 16, 1–12. doi: 10.1093/heapol/16.1.1
Nzila, A. (2006). The past, present and future of antifolates in the treatment of Plasmodium falciparum infection. J. Antimicrob. Chemother. 57, 1043–1054. doi: 10.1093/jac/dkl104
Nzila, A. M., Mberu, E. K., Sulo, J., Dayo, H., Winstanley, P. A., Sibley, C. H., et al. (2000). Towards an understanding of the mechanism of pyrimethamine-sulfadoxine resistance in Plasmodium falciparum: genotyping of dihydrofolate reductase and dihydropteroate synthase of Kenyan parasites. Antimicrob. Agents Chemother. 44, 991–996. doi: 10.1128/aac.44.4.991-996.2000
Ogouyèmi-Hounto, A., Ndam, N. T., Kinde Gazard, D., d’Almeida, S., Koussihoude, L., Ollo, E., et al. (2013). Prevalence of the molecular marker of Plasmodium falciparum resistance to chloroquine and sulphadoxine/pyrimethamine in Benin seven years after the change of malaria treatment policy. Mal. J. 12:147.
Okumu, F. O., and Moore, S. J. (2011). Combining indoor residual spraying and insecticide-treated nets for malaria control in Africa: a review of possible outcomes and an outline of suggestions for the future. Malar. J. 10:208.
Oladipo, O. O., Wellington, O. A., and Sutherland, C. J. (2015). Persistence of chloroquine-resistant haplotypes of Plasmodium falciparum in children with uncomplicated Malaria in Lagos, Nigeria, four years after change of chloroquine as first-line antimalarial medicine. Diagn. Pathol. 10:41. doi: 10.1186/s13000-015-0276-2
Olapeju, B., Choiriyyah, I., Lynch, M., Acosta, A., Blaufuss, S., Filemyr, E., et al. (2018). Age and gender trends in insecticide-treated net use in sub-Saharan Africa: a multi-country analysis. Mal. J. 17:423.
Omar, S. A. I, Adagu, S., Gump, D. W., Ndaru, N. P., and Warhurst, D. C. (2001). Plasmodium falciparum in Kenya: high prevalence of drug-resistance-associated polymorphisms in hospital admissions with severe malaria in an epidemic area. Annal. Trop. Med. Parasitol. 95, 661–669. doi: 10.1080/00034980120103234
Osman, M. E., Mockenhaupt, F. P., Bienzle, U., Elbashir, M. I., and Giha, H. A. (2007). Field-based evidence for linkage of mutations associated with chloroquine (pfcrt/pfmdr1) and sulfadoxine-pyrimethamine (pfdhfr/pfdhps) resistance and for the fitness cost of multiple mutations in P. falciparum. Infect. Genet. Evol. 7, 52–59. doi: 10.1016/j.meegid.2006.03.008
Oyibo, W. A., and Agomo, C. O. (2011). Scaling up of intermittent preventive treatment of malaria in pregnancy using sulphadoxine-pyrimethamine: prospects and challenges. Mat. Child Health J. 15, 542–552. doi: 10.1007/s10995-010-0608-5
Parker, D., Carrara, V., Pukrittayakamee, S., McGready, R., and Nosten, F. (2015). Malaria ecology along the Thailand– Myanmar border. Mal. J. 14:921.
Pearce, R. J., Drakeley, C., Chandramohan, D., Mosha, F., and Roper, C. (2003). Molecular determination of point mutation haplotypes in the dihydrofolate reductase and dihydropteroate synthase of Plasmodium falciparum in three districts of northern Tanzania. Antimicrob Agents Chemother. 47, 1347–1354. doi: 10.1128/aac.47.4.1347-1354.2003
Pearce, R. J., Pota, H., Evehe, M. S., El-Hadj, B., Mombo-Ngoma, G., Malisa, A. L., et al. (2009). Multiple origins and regional dispersal of resistant dhps in African Plasmodium falciparum malaria. PLoS Med. 6:e1000055. doi: 10.1371/journal.pmed.1000055
Peters, P. J., Thigpen, M. C., Parise, M. E., and Newman, R. D. (2007). Safety and toxicity of sulfadoxine/pyrimethamine: implications for malaria prevention in pregnancy using intermittent preventive treatment. Drug Saf. 30, 481–501. doi: 10.2165/00002018-200730060-00003
Pluess, B., Tanser, F. C., Lengeler, C., and Sharp, B. L. (2010). Indoor residual spraying for preventing malaria. Cochr. Database Syst. Rev. 2010:CD006657.
Pulcini, S., Staines, H. M., Lee, A. H., Shafik, S. H., Bouyer, G., Moore, C. M., et al. (2015). Mutations in the Plasmodium falciparum chloroquine resistance transporter, PfCRT, enlarge the parasite’s food vacuole and alter drug sensitivities. Sci. Rep. 5:14552. doi: 10.1038/srep14552
Raman, J., Kagoro, F. M., Mabuza, A., Malatje, G., Reid, A., Frean, J., and Barnes, K. I. (2019). Absence of kelch13 artemisinin resistance markers but strong selection for lumefantrine-tolerance molecular markers following 18 years of artemisinin-based combination therapy use in Mpumalanga Province, South Africa (2001–2018). Malaria J. 18:280. doi: 10.1186/s12936-019-2911-y
Raphemot, R., Posfai, D., and Derbyshire, E. R. (2016). Current therapies and future possibilities for drug development against liver-stage malaria. J. Clin. Investigat. 126, 2013–2020. doi: 10.1172/jci82981
Rapuoda, B., Ali, A., Bakyaita, N., Mutabingwa, T., Mwita, A., Rwagacondo, C., et al. (2003). The efficacy of antimalarial monotherapies, sulfadoxine-pyrimethamine and amodiaquine in East Africa: implication for sub-regional policy. The East African Network for Monitoring Antimalarial Treatment (EANMAT). Trop. Med. Int. Health 8, 860–867. doi: 10.1046/j.1360-2276.2003.01114.x
Recker, M., Bull, P. C., and Buckee, C. O. (2018). Recent advances in the molecular epidemiology of clinical malaria. F1000Research 7, F1000FacultyRev-1159.
Reiling, S. J., Krohne, G., Friedrich, O., Geary, T. G., and Rohrbach, P. (2018). Chloroquine exposure triggers distinct cellular responses in sensitive versus resistant Plasmodium falciparum parasites. Sci. Rep. 8:11137.
Roepe, P. D. (2011). PfCRT-mediated drug transport in malarial parasites. Biochemistry 50, 163–171. doi: 10.1021/bi101638n
Sandefur, C. I., Wooden, J. M. I, Quaye, K., Sirawaraporn, W., and Sibley, C. H. (2007). Pyrimethamine-resistant dihydrofolate reductase enzymes of Plasmodium falciparum are not enzymatically compromised in vitro. Mol. Biochem. Parasitol. 154, 1–5. doi: 10.1016/j.molbiopara.2007.03.009
Scates, S. S., Finn, T. P., Wisniewski, J., Dadi, D., Mandike, R., Khamis, M., et al. (2020). Costs of insecticide-treated bed net distribution systems in sub-Saharan Africa. Mal. J. 19, 105–105.
Schleicher, T. R., Yang, J., Freudzon, M., Rembisz, A., Craft, S., Hamilton, M., et al. (2018). A mosquito salivary gland protein partially inhibits Plasmodium sporozoite cell traversal and transmission. Nat. Commun. 9:2908.
Schönfeld, M., Barreto Miranda, I., Schunk, M., Maduhu, I., Maboko, L., Hoelscher, M., et al. (2007). Molecular surveillance of drug-resistance associated mutations of Plasmodium falciparum in south-west Tanzania. Mal. J. 6:2.
Shafik, S. H., Cobbold, S. A., Barkat, K., Richards, S. N., Lancaster, N. S., Llinás, M., et al. (2020). The natural function of the malaria parasite’s chloroquine resistance transporter. Nat. Commun. 11:3922. doi: 10.1038/s41467-020-17781-6
Sharma, J., Khan, S., Dutta, P., and Mahanta, J. (2015). Molecular determination of antifolate resistance associated point mutations in Plasmodium falciparum dihydrofolate reductase (dhfr) and dihydropteroate synthetase (dhps) genes among the field samples in Arunachal Pradesh. J. Vector Borne Dis. 52, 116–121.
Shretta, R., Omumbo, J., Rapuoda, B., and Snow, R. W. (2000). Using evidence to change antimalarial drug policy in Kenya. Trop. Med. Int. Health 5, 755–764. doi: 10.1046/j.1365-3156.2000.00643.x
Siddiqui, F. A., Boonhok, R., Cabrera, M., Mbenda, H. G. N., Wang, M., Min, H., et al. (2020). Role of Plasmodium falciparum Kelch 13 protein mutations in P. falciparum populations from northeastern myanmar in mediating artemisinin resistance. mBio 11:e001134-19.
Slater, H. C., Griffin, J. T., Ghani, A. C., and Okell, L. C. (2016). Assessing the potential impact of artemisinin and partner drug resistance in sub-Saharan Africa. Mal. J. 15, 10–10.
Smith Gueye, C., Newby, G., Gosling, R. D., Whittaker, M. A., Chandramohan, D., Slutsker, L., et al. (2016). Strategies and approaches to vector control in nine malaria-eliminating countries: a cross-case study analysis. Mal. J. 15:2.
Sridaran, S., McClintock, S. K., Syphard, L. M., Herman, K. M., Barnwell, J. W., and Udhayakumar, V. (2010). Anti-folate drug resistance in Africa: meta-analysis of reported dihydrofolate reductase (dhfr) and dihydropteroate synthase (dhps) mutant genotype frequencies in African Plasmodium falciparum parasite populations. Mal. J. 9:247. doi: 10.1186/1475-2875-9-247
Steinhardt, L. C., Jean, Y. S., Impoinvil, D., Mace, K. E., Wiegand, R., Huber, C. S., et al. (2017). Effectiveness of insecticide-treated bednets in malaria prevention in Haiti: a case-control study. Lancet Global Health 5, e96–e103.
Tagbor, H., Bruce, J., Browne, E., Randal, A., Greenwood, B., and Chandramohan, D. (2006). Efficacy, safety, and tolerability of amodiaquine plus sulphadoxine-pyrimethamine used alone or in combination for malaria treatment in pregnancy: a randomised trial. Lancet 368, 1349–1356. doi: 10.1016/s0140-6736(06)69559-7
Takala-Harrison, S., and Laufer, M. K. (2015). Antimalarial drug resistance in Africa: key lessons for the future. Annal. N. Y. Acad. Sci. 1342, 62–67. doi: 10.1111/nyas.12766
Takechi, M., Matsuo, M., Ziba, C., Macheso, A., Butao, D. I, Zungu, L., et al. (2001). Therapeutic efficacy of sulphadoxine/pyrimethamine and susceptibility in vitro of P. falciparum isolates to sulphadoxine-pyremethamine and other antimalarial drugs in Malawian children. Trop. Med. Int. Health 6, 429–434. doi: 10.1046/j.1365-3156.2001.00735.x
Taylor, C., Florey, L., and Ye, Y. (2017). Equity trends in ownership of insecticide-treated nets in 19 sub-Saharan African countries. Bull. World Health Organ. 95, 322–332. doi: 10.2471/blt.16.172924
Tekete, M. M., Toure, S., Fredericks, A., Beavogui, A. H., Sangare, C. P. O., Evans, A., et al. (2011). Effects of amodiaquine and artesunate on sulphadoxine-pyrimethamine pharmacokinetic parameters in children under five in Mali. Mal. J. 10:275. doi: 10.1186/1475-2875-10-275
ter Kuile, F. O., van Eijk, A. M., and Filler, S. J. (2015). Effect of sulfadoxine-pyrimethamine resistance on the efficacy of intermittent preventive therapy for malaria control during pregnancy: a systematic review. JAMA 297, 2603–2616. doi: 10.1001/jama.297.23.2603
Terlouw, D. J., Nahlen, B. L., Courval, J. M., Kariuki, S. K., Rosenberg, O. S., Oloo, A. J., et al. (2003). Sulfadoxine-pyrimethamine in treatment of malaria in Western Kenya: increasing resistance and underdosing. Antimicrob Agents Chemother. 47, 2929–2932. doi: 10.1128/aac.47.9.2929-2932.2003
Thomsen, T. T., Madsen, L. B., Hansson, H. H., Tomás, E. V., Charlwood, D. I, Bygbjerg, C., et al. (2013). Rapid selection of Plasmodium falciparum chloroquine resistance transporter gene and multidrug resistance gene-1 haplotypes associated with past chloroquine and present artemether-lumefantrine use in Inhambane District, southern Mozambique. Am. J. Trop. Med. Hyg. 88, 536–541. doi: 10.4269/ajtmh.12-0525
Thu, A. M., Phyo, A. P., Landier, J., Parker, D. M., and Nosten, F. H. (2017). Combating multidrug-resistant Plasmodium falciparum malaria. FEBS J. 284, 2569–2578.
Tizifa, T. A., Kabaghe, A. N., McCann, R. S., van den Berg, H., Van Vugt, M., and Phiri, K. S. (2018). Prevention efforts for Malaria. Curr. Trop. Med. Rep. 5, 41–50.
Trape, J. F. (2001). The public health impact of chloroquine resistance in Africa. Am. J. Trop. Med. Hyg. 64, 12–17. doi: 10.4269/ajtmh.2001.64.12
Trape, J. F., Pison, G., Preziosi, M. P., Enel, C., Desgrées du Loû, A., Delaunay, V., et al. (1998). Impact of chloroquine resistance on malaria mortality. C R Acad. Sci. III 321, 689–697.
Ukpe, I. S., Moonasar, D., Raman, J., Barnes, K. I., Baker, L., and Blumberg, L. (2013). Case management of malaria: Treatment and chemoprophylaxis. S. Afr. Med. J. 103(10 Pt 2), 793–798. doi: 10.7196/samj.7443
Veiga, M. I., Dhingra, S. K., Henrich, P. P., Straimer, J., Gnädig, N., Uhlemann, A-C., et al. (2016). Globally prevalent pfmdr1 mutations modulate Plasmodium falciparum susceptibility to artemisinin-based combination therapies. Nat. Commun. 7:11553. doi: 10.1038/ncomms11553
Viana, G. M. R., Machado, R. L. D., Calvosa, V. S. P., and Póvoa, M. M. (2006). Mutations in the pfmdr1, cg2, and pfcrt genes in Plasmodium falciparum samples from endemic malaria areas in Rondonia and Pará State, Brazilian Amazon Region. Cad Saude Publica 22, 2703–2711. doi: 10.1590/s0102-311x2006001200019
von Seidlein, L., and Dondorp, A. (2015). Fighting fire with fire: mass antimalarial drug administrations in an era of antimalarial resistance. Exp. Rev. Anti. Infect. Ther. 13, 715–730. doi: 10.1586/14787210.2015.1031744
Walker, P. G. T., Floyd, J., ter Kuile, F., and Cairns, M. (2017). Estimated impact on birth weight of scaling up intermittent preventive treatment of malaria in pregnancy given sulphadoxine-pyrimethamine resistance in Africa: a mathematical model. PLoS Med. 14:e1002243. doi: 10.1371/journal.pmed.1002243
Wernsdorfer, W. H. (1994). Epidemiology of drug resistance in malaria. Acta Tropica 56, 143–156. doi: 10.1016/0001-706x(94)90060-4
White, N. J., Pongtavornpinyo, W., Maude, R. J., Saralamba, S., Aguas, R., Stepniewska, K., et al. (2009). Hyperparasitaemia and low dosing are an important source of anti-malarial drug resistance. Mal. J. 8:253.
Wicht, K. J., Mok, S., and Fidock, D. A. (2020). Molecular Mechanisms of drug resistance in Plasmodium falciparum malaria. Annu. Rev. Microbiol. 74, 431–454. doi: 10.1146/annurev-micro-020518-115546
Winstanley, P., Ward, S., Snow, R., and Breckenridge, A. (2004). Therapy of falciparum malaria in sub-saharan Africa: from molecule to policy. Clin. Microbiol. Rev. 17, 612–637. doi: 10.1128/cmr.17.3.612-637.2004
World Health Organisation (WHO) (2008). World Malaria Report 2007. Geneva: World Health Organization.
World Health Organisation (WHO) (2003). World Malaria Report 2002. Geneva: World Health Organization.
World Health Organisation (WHO) (2010). WHO Technical Report Series 957. Geneva: World Health Organization.
World Health Organisation (WHO) (2011). World Malaria Report 2010. Geneva: World Health Organization.
World Health Organisation (WHO) (2017). World Malaria Report 2016. Geneva: World Health Organization.
World Health Organisation (WHO) (2019). World Malaria Report 2018. Geneva: World Health Organization.
World Health Organisation (WHO) (2020). World Malaria Report 2018. Geneva: World Health Organization.
Xia, Z.-G., Wang, R.-B., Wang, D.-Q., Feng, J., Zheng, Q., Deng, C.-S., et al. (2014). China-Africa cooperation initiatives in malaria control and elimination. Adv. Parasitol. 86, 319–337. doi: 10.1016/b978-0-12-800869-0.00012-3
Xie, S. C., Ralph, S. A., and Tilley, L. (2020). K13, the cytostome, and artemisinin resistance. Trends Parasitol. 36, 533–544. doi: 10.1016/j.pt.2020.03.006
Yadav, S., and Rawal, G. (2016). The menace due to fake antimalarial drugs. Int. J. Pharmaceut. Chem. Anal. 3, 53–55. doi: 10.5958/2394-2797.2016.00007.1
Yeka, A., Gasasira, A., Mpimbaza, A., Achan, J., Nankabirwa, J., Nsobya, S., et al. (2012). Malaria in Uganda: challenges to control on the long road to elimination: I. Epidemiology and current control efforts. Acta Trop. 121, 184–195. doi: 10.1016/j.actatropica.2011.03.004
Young, M. D., and Moore, D. V. (1961). Chloroquine resistance in Plasmodium falciparum. Am. J. Trop. Med. Hyg. 10, 317–320.
Zareen, S., Ur Rehman, H., Gul, N., Ur Rehman, M., Bibi, S., Bakht, A., et al. (2016). Malaria is still a life-threatening disease review. J. Entomol. Zool. Stud. JEZS 105, 105–112.
Keywords: antimalarial drug resistance, chloroquine, malaria, malaria control, sulfadoxine–pyrimethamine
Citation: Roux AT, Maharaj L, Oyegoke O, Akoniyon OP, Adeleke MA, Maharaj R and Okpeku M (2021) Chloroquine and Sulfadoxine–Pyrimethamine Resistance in Sub-Saharan Africa—A Review. Front. Genet. 12:668574. doi: 10.3389/fgene.2021.668574
Received: 16 February 2021; Accepted: 20 April 2021;
Published: 25 June 2021.
Edited by:
Apichai Tuanyok, University of Florida, United StatesReviewed by:
Rajeev Kumar Mehlotra, Case Western Reserve University, United StatesChenqi Wang, University of South Florida, United States
Copyright © 2021 Roux, Maharaj, Oyegoke, Akoniyon, Adeleke, Maharaj and Okpeku. This is an open-access article distributed under the terms of the Creative Commons Attribution License (CC BY). The use, distribution or reproduction in other forums is permitted, provided the original author(s) and the copyright owner(s) are credited and that the original publication in this journal is cited, in accordance with accepted academic practice. No use, distribution or reproduction is permitted which does not comply with these terms.
*Correspondence: Moses Okpeku, b2twZWt1bUB1a3puLmFjLnph