- 1Department of Cardiology and the Center for Circadian Metabolism and Cardiovascular Disease, Southwest Hospital, Third Military Medical University (Army Medical University), Chongqing, China
- 2Medical Center of Hematology, The Xinqiao Hospital of Army Medical University, Chongqing, China
- 3Department of Anesthesiology, The Second Affiliated Hospital, Chongqing Medical University, Chongqing, China
- 4Department of Obstetrics and Gynaecology, The First Affiliated Hospital of Chongqing Medical University, Chongqing, China
- 5Department of Obstetrics and Gynaecology, The Second Affiliated Hospital of Chongqing Medical University, Chongqing, China
- 6Key Lab of Medical Protection for Electromagnetic Radiation, Ministry of Education of China, Institute of Toxicology, College of Preventive Medicine, Army Medical University (Third Military Medical University), Chongqing, China
- 7Research Center for Nutrition and Food Safety, Institute of Military Preventive Medicine, Army Medical University, Chongqing, China
- 8Key Laboratory of Extreme Environmental Medicine, Department of Pathophysiology, College of High Altitude Military Medicine, Ministry of Education of China, Army Medical University (Third Military Medical University), Chongqing, China
- 9Key Laboratory of High Altitude Medicine, PLA, Army Medical University (Third Military Medical University), Chongqing, China
- 10Department of Dermatology, The First Affiliated Hospital of Chongqing Medical University, Chongqing, China
The circadian clock coordinates physiology, metabolism, and behavior with the 24-h cycles of environmental light. Fundamental mechanisms of how the circadian clock regulates organ physiology and metabolism have been elucidated at a rapid speed in the past two decades. Here we review circadian networks in more than six organ systems associated with complex disease, which cluster around metabolic disorders, and seek to propose critical regulatory molecules controlled by the circadian clock (named clock-controlled checkpoints) in the pathogenesis of complex disease. These include clock-controlled checkpoints such as circadian nuclear receptors in liver and muscle tissues, chemokines and adhesion molecules in the vasculature. Although the progress is encouraging, many gaps in the mechanisms remain unaddressed. Future studies should focus on devising time-dependent strategies for drug delivery and engagement in well-characterized organs such as the liver, and elucidating fundamental circadian biology in so far less characterized organ systems, including the heart, blood, peripheral neurons, and reproductive systems.
Introduction
To align with the daily cycling of environmental conditions associated with the Earth’s rotation, terranean and subterranean organisms have evolved an internal circadian (Latin words: circa, about; dies, day) clock system to organize metabolism, physiology, and behavior (Bass and Lazar, 2016; Zhang et al., 2020b; López-Otín and Kroemer, 2021). The circadian clock manifests as internal and resettable (known as entrainable in the field) circadian rhythms at the molecular, physiological, and organismal levels. The circadian clock system in mammals is organized in a hierarchical manner, orchestrated by a master pacemaker in the hypothalamus. The circadian clock in individual cells is genetically similar and composed of transcriptional-(post)translational feedback loops.
In a time when fundamental and preclinical research on circadian rhythms is progressing at an unprecedented speed, the concept of time medicine (or chronomedicine, circadian medicine) is emerging as a new dimension in medicine (Cederroth et al., 2019; Panda, 2019; Allada and Bass, 2021). Here, we focused on research in the role of the circadian clock in major organs/tissues related to the pathogenesis of complex diseases, particularly those with metabolic disorders, and summarized tissue-specific circadian clock-controlled checkpoints as genes, proteins, and biological pathways that would be valuable for time medicine.
The Architecture of the Circadian System in Mammals
The architecture of the mammalian circadian clock system is established by a pacemaker located in the suprachiasmatic nucleus (SCN) of the hypothalamus (Allada and Bass, 2021). Light activates the non-cone non-rod melanopsin 4-expressing retinal ganglion neurons in the retina, transmitting signals to the SCN through a retinohypothalamic tract. The phase of the SCN is readily entrained by light, but seems refractory to other time signals associated with feeding and body temperature (Buhr et al., 2010; Reinke and Asher, 2019). The SCN coordinates clocks in non-SCN cells and tissues via neuronal or humoral connections and metabolic signaling associated with feeding rhythm. Feeding rhythm is crucial in clock synchronization in the body (Reinke and Asher, 2019), yet different peripheral clocks seem to respond with a broad spectrum of kinetics (Xin et al., 2021). Inverted feeding readily reverses circadian rhythms in liver and adipose tissue (Manella et al., 2021; Xin et al., 2021). Recently, a multi-omics study indicated that diurnal rhythms of transcriptomes, metabolomes, and clocks in the heart and kidney are resistant to circadian entrainment by feeding rhythm (Xin et al., 2021). The tissue-specific response to inverted feeding is gated by the temporal signaling from the SCN clock and the liver-clock (Manella et al., 2021; Xin et al., 2021). Thus, the coordinated actions of the SCN-peripheral clock axes orchestrate the harmony of mammalian physiological activities throughout the day (Figure 1), including sleep-wake cycle, endocrine secretion, blood pressure, energy metabolism, and urine production (Bass and Lazar, 2016).
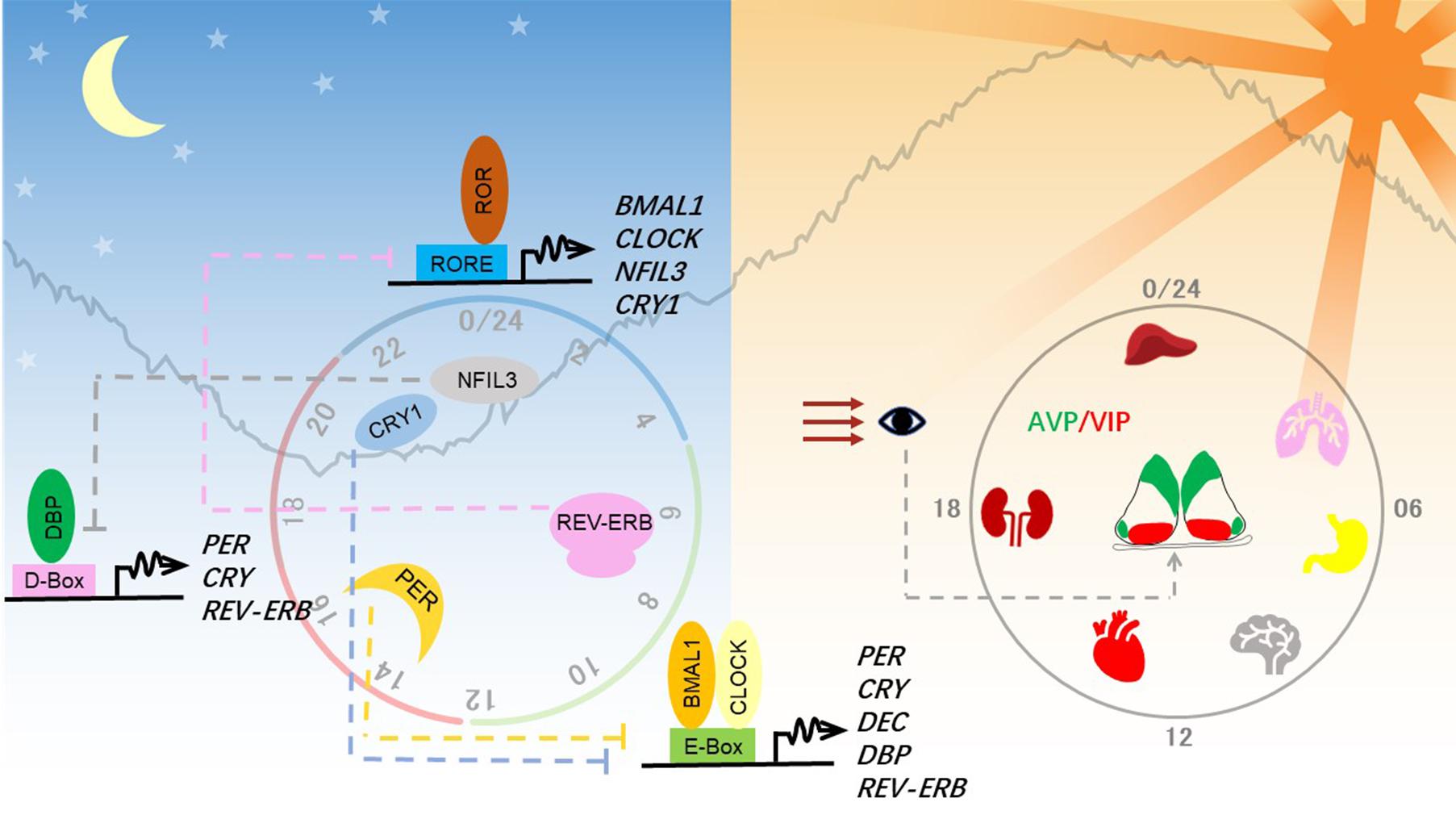
Figure 1. Molecular and anatomical structure of the circadian clock system. Left panel: Molecular mechanisms of the circadian clock mainly consist of three interlocked transcriptional-translational feedback loops. Transcriptional factors activate the transcription of repressor genes via binding to promoter regions containing different combinations of three cis-elements and thereby generating a different phase. These protein products of the repressor genes then enter the nucleus and feed back to repress their own transcription to close the circadian loop. Right panel: Circadian clocks exist in almost all tissues in mammals. The hypothalamic suprachiasmatic nucleus (SCN) in the brain serves as the master clock or the central clock, which is entrained by light through the retinohypothalamic tract pathway. Neuropeptides AVP and VIP maintain the robust cell-autonomous rhythmicity and light entrainment, respectively, in the SCN. The SCN clock synchronizes slave clocks in peripheral tissues through neuronal and humoral pathways throughout the body.
Clocks in individual neurons of the SCN pacemaker are coupled to establish a robust circadian rhythm, which can last for weeks in vitro without dampening (Welsh et al., 2009). This strong intercellular coupling property is crucial for organizing the body’s clock. Both gap junctions and paracrine neurotransmitter signaling contribute to intercellular coupling. The SCN pacemaker is made of a pair of neuronal lobes containing ∼10,000 neurons, which are divided into a ventral core region and a dorsal shell region. The core region sits above the retinohypothalamic tract and receives input signals from environmental light. The shell region receives signals from the core region through neuronal projections and gap junctions. These two regions can be distinguished by the concentration of different neuropeptides, represented by vasoactive intestinal polypeptide (VIP) in the core neurons and arginine vasopressin (AVP) in the shell neurons. Upon light entrainment, VIP is released from the core neurons, depolarizing shell regions, leading to the resetting of the SCN clock. Recently, Shan et al. (2020) devised a color-switch dual-color imaging system in mice and showed that AVP-expressing shell neurons are crucial for maintaining cell-autonomous circadian rhythm in the SCN.
Molecular Mechanisms of the Circadian Clock
In mammals, the circadian clock is mainly composed of three transcriptional-(post)translational feedback loops (Figure 1). Transcription factors BMAL1 and CLOCK heterodimerize and activate transcription of the clock genes Period (PER1, PER2) and Cryptochrome (CRY1, CRY2). As they increasingly accumulate in the cytosol, PER and CRY heterodimerize, translocate into the nucleus, and shut down the expression of their own genes by inhibiting BMAL1-CLOCK. The turnover and activity of these core clock proteins are controlled by post-translational modifications, including phosphorylation, ubiquitination, acetylation, and O-linked β-N-acetylglucosaminylation (O-GlcNAcylation) (Panda, 2016; Takahashi, 2017). Phosphorylation of PER2 serine 662 is a key regulatory node in setting the speed of the clock, initiating the casein kinase 1δ/ε-controlled phosphorylation events and ubiquitin-mediated protein degradation.
BMAL1-CLOCK also activates the expression of REV-ERBα (NR1D1) and REV-ERBβ (NR1D2), which repress expression of BMAL1 and NFIL3 (nuclear factor, interleukin 3-regulated, also known as E4BP4). Retinoic acid-related orphan receptor (ROR) and REV-ERB competitively bind to RORE cis-elements, resulting in the regulation of one stabilizing loop of the circadian clock. The other stabilizing loop is composed of the PAR-bZIP (proline and acidic amino acid-rich basic leucine zipper) factors DBP (D-box binding protein), TEF (thyrotroph embryonic factor), and HLF (hepatic leukemia factor), whose expression is controlled by BMAL1-CLOCK. REV-ERB/ROR-controlled repressor NFIL3 and the PAR-bZIP transcription factors regulate expression of genes containing D-box cis-elements. Together, these three interlocking feedback loops generate robust circadian rhythms of the clock genes and output genes, whose phase and amplitude are determined by combinations of circadian cis-regulatory elements in the promoter and enhancer regions.
Circadian Physiology in Major Organs Associated With Complex Disease
Liver
The liver is a prototypical peripheral organ that is deeply regulated by the circadian clock system. Both transcriptional and organelle-level mechanisms orchestrate diurnal rhythms of liver physiology, ranging from the well-characterized metabolic functions (including metabolism of glucose, glycogen, lipids, and amino acids) to cell growth and xenobiotic responses. For example, the circadian clock drives diurnal expression of glycogen synthase 2 (GYS2) to modulate glycogen synthesis, phosphoenolpyruvate carboxykinase 1 (PEPCK1) and glucose-6-phosphatase, catalytic (G6PC) for gluconeogenesis; and insulin induced gene 2 (INSIG2) for cholesterol and bile acid synthesis (Bass and Lazar, 2016; Panda, 2016; Reinke and Asher, 2019; Zhang et al., 2020b). PER2 and CRY1 bind to nuclear hormone receptors, such as REV-ERBα/β, PPARα, and GR to output the diurnal rhythms to glucose and lipid metabolism (Lamia et al., 2008; Schmutz et al., 2010; Zhang et al., 2010). Emerging evidence suggests a SCN-liver axis in the control of diurnal rhythm in hepatic glucose metabolism. REV-ERBα/β in SCN GABAergic neurons are required for rhythmic insulin-mediated suppression of glucose production in the liver (Ding et al., 2021). In addition to cell-autonomous effects, the hepatocyte clock can modulate the circadian rhythm of local microenvironment. Hepatic REV-ERBα/β is required for the circadian rhythmicity of transcriptomes and metabolomes in local endothelial cells and Kupffer cells in the liver, which is associated with circadian rhythms of glutathione metabolism, glycolysis, and hexosamine pathway (Guan et al., 2020).
It has become apparent that clock proteins can execute temporal order through crosstalk to organelle structure and dynamics. To meet the daily demand of cellular energy and stress adaptation, mitochondrial structure and organization are regulated by the circadian clock. Hepatic BMAL1 is crucial for circadian rhythm of the mitochondrial fission, fusion, and turnover by autophagy, in part through its transcriptional target genes involved in mitochondrial fission and fusion (Jacobi et al., 2015). Nutrient-sensing pathways such as AMPK and Sirtuins drive circadian rhythm of phosphorylation of mitochondrial fission executor, dynamin-related protein 1 (DRP1, peak at CT 12 h). This in turn leads to diurnal oscillations of mitochondrial morphology between fission and fusion and cellular energy (Schmitt et al., 2018). In synchrony with the cycling of mitochondrial structure, mitochondrial fatty acid beta-oxidation (peak at ZT0) and protein abundance of mitochondrial rate-limiting enzymes (CPT1A peak at ZT 0 h and PDHB peak at ZT 3 h) exhibit diurnal rhythms, which are all controlled by PER1/PER2 (Neufeld-Cohen et al., 2016).
Lysosomes exhibit diurnal activities in the liver. Autophagy promotes lysosomal degradation of CRY1, contributing to clock regulation (Toledo et al., 2018). Using p62/SQSTM1 as a marker, Ryzhikov et al. (2019) found that basal autophagosome formation (peak at ZT 8 h) is coupled to proteasomal protein degradation (peak at ZT 10 h) during the resting phase. Interestingly, proinflammatory signals such as lipopolysaccharide (LPS) alter the substrate preference of autophagy from cytosolic proteins to mitochondrial proteins (Ryzhikov et al., 2019). Diurnal expression of autophagic genes is conferred in part through diurnal transcriptional activity of CCAAT enhancer binding protein beta (C/EBPβ) (Ma et al., 2011). Emerging evidence supports the strong circadian regulation of autophagy at the proteomic and post-translational levels. Wang Y. et al. (2018) showed that lysosomal transport signature is enriched in diurnal proteome in liver, however, there is no circadian rhythm of the transcripts encoding these proteins. Autophagic proteins and their regulator, mammalian target of rapamycin complex 1 (mTORC1), which is among the 25% of the hepatic circadian phospho-proteome, exhibit circadian rhythm in liver (Robles et al., 2017). Cytosolic PER2 tethers tuberous sclerosis 1 protein (TSC1) to suppress the activity of mTORC1, contributing to diurnal rhythms of protein synthesis and autophagy in liver (Wu et al., 2019). To date, the majority of evidence suggesting clock-output pathways to lysosomes and autophagosomes is based on biochemical markers. Compelling evidence from microscopy of subcellular structure would help establish the links between lysosome/autophagy and circadian clocks in the liver and other tissues.
Heart and Skeletal Muscle
Heart and the Cardiovascular System
The heart and cardiovascular system exhibit circadian rhythm of genes and functions from the heart tissue to various types of blood vessels (Crnko et al., 2019). Heart tissue is a specialized mechanic pump in the body, which creates a unique organ system where two physiological cycles interact like a Russian doll. Namely, the second-scale cardiac cycle is gauged by the circadian clock. It is estimated that 13% of gene transcripts and 8% of proteins in the mouse heart are diurnal (Martino et al., 2004; Podobed et al., 2014). Under constant darkness, a lower percentage (6–8%) of gene transcripts oscillate in a circadian manner (Storch et al., 2002; Zhang et al., 2014). In the aorta, 4–5% of genes oscillate in a circadian manner (Rudic et al., 2005; Zhang et al., 2014). These circadian pathways span from cellular energy metabolism and sarcolemma calcium signaling, to cellular signaling pathways.
The cardiomyocyte clock is crucial in driving the cell-autonomous oscillation of heart function (Durgan et al., 2005; Bray et al., 2008). Mitochondrial function and dynamics sit at the hub of this regulation (Zhang et al., 2020a). Intervening in CLOCK function by overexpressing a dominant-negative mutant abolishes diurnal variation of mitochondrial oxidative metabolism (peak ZT 18h) and tolerance to ischemic-reperfusion injury (peak ZT 0 h) (Durgan et al., 2005, 2010; Bray et al., 2008). These phenotypes are associated with the abolished oscillation of PDK4, a metabolic gauge between glycolysis and oxidative metabolism. Genetic deletion of the Bmal1 gene in mouse cardiomyocytes results in dilated cardiomyopathy, decreased heart rate and increased arrhythmias (Lefta et al., 2012; Schroder et al., 2013). In human embryonic stem cell-derived cardiomyocytes, loss of BMAL1 inhibits mitochondrial fission and mitophagy, impairs oxidative metabolism, and leads to disorganized sarcolemmal structure, decreased contractility, and dilated cardiomyopathy (Li E. et al., 2020). Mechanistically, BMAL1/CLOCK bipartite TF trans-activates expression of the mitophagy receptor gene BNIP3 (Li E. et al., 2020). Provided that BNIP3 is not a robust diurnal gene in the mouse heart (source: CirGRDB, CircaMetDB), it remains open to post-translational or organelle-level regulation of mitochondria which contributes to diurnal oscillation of the cardiac cycle and metabolism in the heart.
Moreover, clock-controlled transcription factors orchestrate the cardiac cycle and metabolism (Figure 2). Krüppel-like factor 15 (KLF15), a BMAL1-controlled output TF, determines diurnal oscillation of the cardiac cycle (peak ZT 6 h) and susceptibility to ischemic-reperfusion injury to the heart (Jeyaraj et al., 2012; Li L. et al., 2020). The cardiac arrhythmogenesis in KLF15 transgenic animals phenocopies that in an inducible cardiomyocyte-specific Bmal1 knockout model (Schroder et al., 2013). Though initially thought to act through diurnal expression of ion channels, transcriptome profiling demonstrates that KLF15 controls the diurnal oscillation of cellular energy metabolism (peak ZT 14–20 h) and tissue remodeling and repair (peak ZT 0–6 h) (Zhang et al., 2015). Compelling evidence links the activity of oxygen-sensing HIF-1α transcription factor to the heart-clock, which outputs the diurnal control of glycolysis and the hypoxia response (Eckle et al., 2012; Wu et al., 2017). Chemical activation of REV-ERBα protects against heart failure, which is partially linked to transcription repression (Zhang et al., 2017). While it is intriguing to propose TF-specific labor division in organizing various aspects of the cardiac cycle, contractility, and metabolism throughout the day, it remains unsolved how these clock-output TFs forge a network to coordinate diurnal oscillation of heart physiology and metabolism.
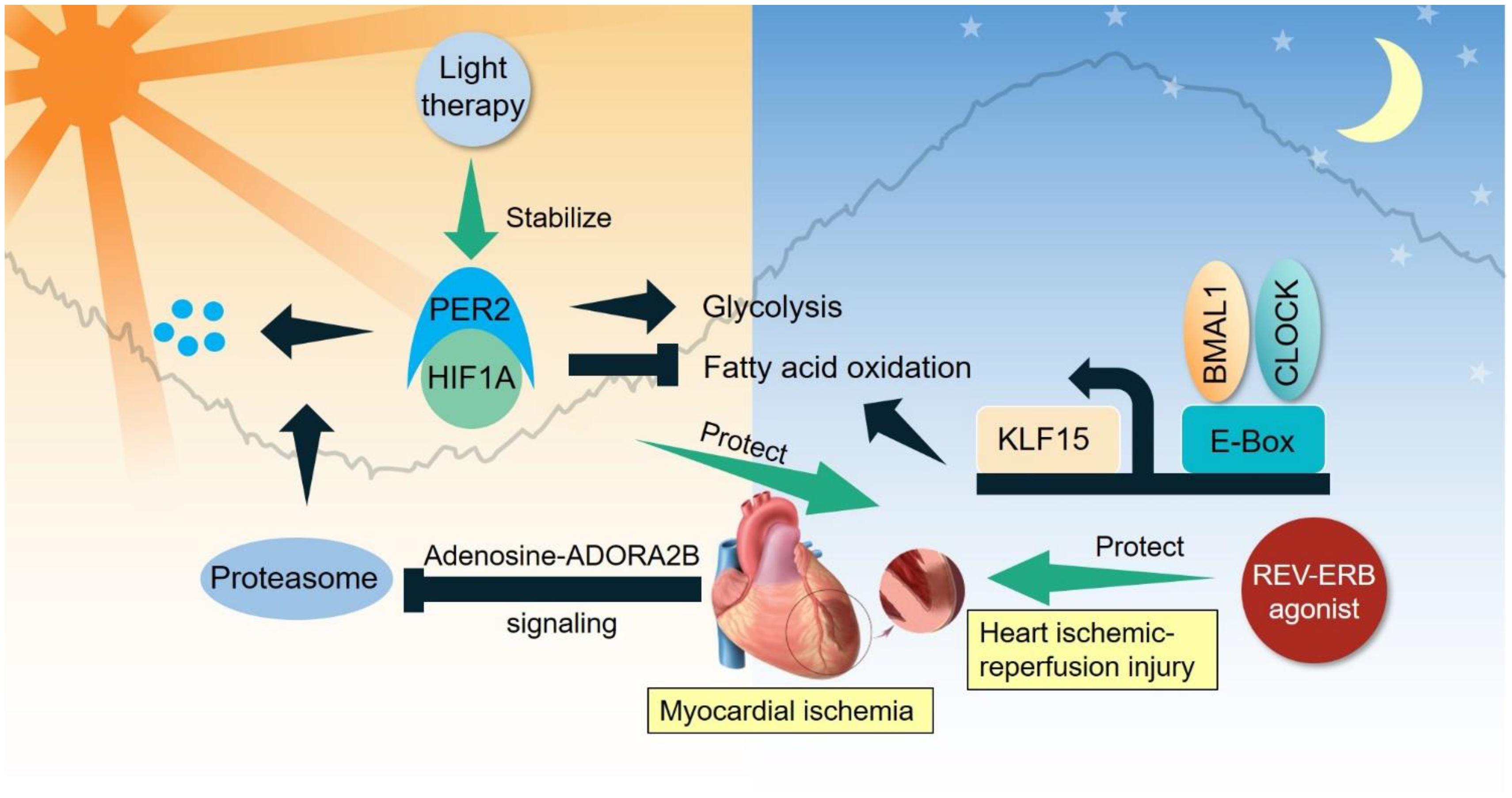
Figure 2. Clock-controlled checkpoints in the heart. Myocardial ischemia stabilizes PER2 to promote glycolysis and suppress fatty acid oxidation. BMAL1/CLOCK bipartite TF modulate the diurnal oscillation of fatty acid oxidation partly through KLF15. Light therapy stabilizes PER2 to benefit ischemic-reperfusion injury, while REV-ERB agonist also protects against ischemic-reperfusion injury.
Skeletal Muscle
Skeletal muscle is the most abundant tissue in mammals, comprising approximately 40% of body mass. Similar to other tissues, skeletal muscle also possesses its own self-sustaining endogenous molecular clock. Approximately 20% of genes (>2300 genes) show 24 h oscillations in skeletal muscle, and these genes participate in a wide range of functions, including myogenesis, transcription and metabolism (Chatterjee and Ma, 2016). Recently, the importance of circadian rhythms for skeletal muscle has been made more evident by the skeletal muscle phenotype observed in models of molecular clock disruption (Figure 3).
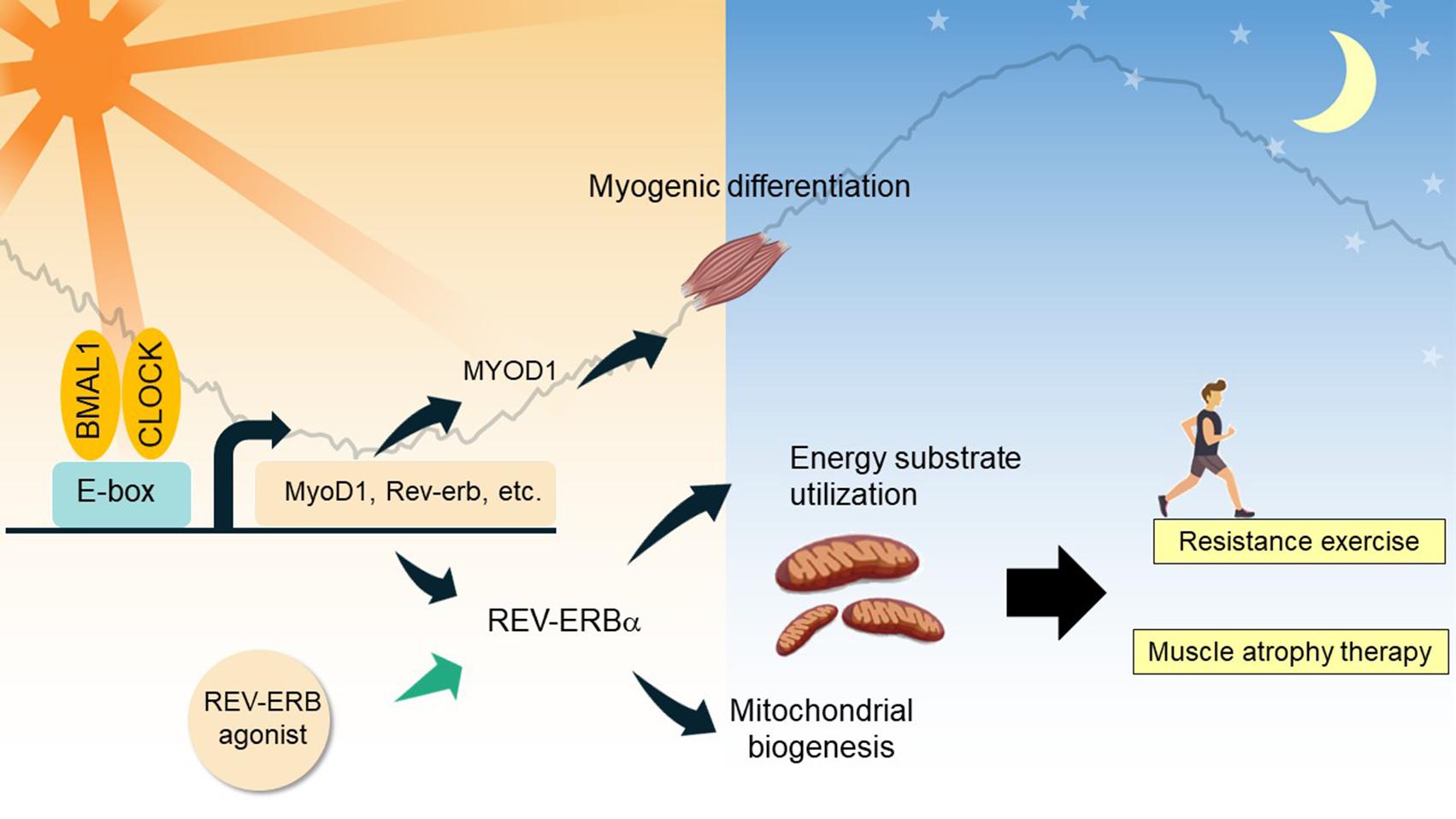
Figure 3. Clock-controlled checkpoints in skeletal muscle. In myocytes, BMAL1 and CLOCK regulate diurnal expression of genes involved in myogenic differentiation and energy substrate utilization. REV-ERBα and its interactome regulate expression of mitochondrial biogenic genes and energy substrate utilization, contributing to myofiber type switch. As demonstrated in chemical biology studies, REV-ERBα agonism holds promise to treat skeletal muscle atrophy, and improve resistance exercise performance.
Firstly, the circadian clock network plays a prominent role in muscle differentiation and maintenance. A study of Bmal1-null mice clearly shows atrophy and tissue damage in skeletal muscle, which could be attributed to the blunted circadian expression of MyoD1 (myogenic differentiation 1) and a defect in the regenerative myogenic process accompanied by lowered repair and an altered satellite cell expansion process (Schiaffino et al., 2016; Vitale et al., 2019). Clock nuclear receptors REV-ERBα/β are highly expressed in proliferating myoblast cells and repress key genes involved in myotube differentiation (Everett and Lazar, 2014; Chatterjee and Ma, 2016). Schroder et al. (2015) investigated a Bmal1 conditional knockout line in adult skeletal muscle, and showed that remarkably, this model mimics the advanced aging phenotype in the musculoskeletal system in Bmal1-null mice, characterized by increased fibrosis, bone calcification, and decreased joint collagen. Skeletal muscle BMAL1 exclusively controls diurnal expression of core clock genes and nuclear receptor genes (Dyar et al., 2014; Schroder et al., 2015). CRY2 regulates the circadian rhythm of myogenic differentiation through stabilization of Cyclin D1 and Tmem176b transcripts (Lowe et al., 2018).
Secondly, the circadian clock participates in the temporal control of energy metabolism in skeletal muscle and contributes to systemic metabolism. Early studies indicate that metabolic genes involved in substrate utilization and energy storage are expressed in a temporally segregated manner over a 24-h period, suggesting a clock-controlled orchestration of distinct catabolic and anabolic metabolic pathways in skeletal muscle (Chatterjee and Ma, 2016; Gabriel and Zierath, 2019). Further, a number of studies have demonstrated the mechanisms underlying the interplay between the molecular clock and fuel preference in cellular energy metabolism (Dyar et al., 2014; Mayeuf-Louchart et al., 2015; Perrin et al., 2018). Muscle-specific Bmal1 knockout resulted in a clear up-regulation of genes involved in lipid metabolism concomitant with the down-regulation of circadian genes involved in glucose utilization, indicating muscle fiber type switches to a slow oxidative fiber-type, together with a substrate shift from carbohydrate to lipid utilization (Schiaffino et al., 2016). BMAL1 also controls HIF-1α activity in myocytes, contributing to the regulation of anaerobic glycolysis (Peek et al., 2017). REV-ERBα is highly expressed in oxidative fiber types, which promotes oxidative metabolism and ultimately exercise performance, in part through the nutrient-sensing LKB-AMPK-SIRT1-PGC1α signaling complex (Woldt et al., 2013). Increased fatty acid utilization, hyperglycemia, and a clear fast-to-slow MyHC in soleus muscle were observed in Rev-Erbα-deficient mice (Woldt et al., 2013), whereas activation of REV-ERBα by synthetic agonists resulted in lipid lowering and anti-obesity effects (Cho et al., 2012).
Thirdly, the circadian clock has strong control of muscle strength and locomotor function. Results from circadian transcriptomics studies indicate that many essential functions and physiological processes in skeletal muscle are influenced by the transcriptional output of the clock (Dyar et al., 2018a; Perrin et al., 2018). As observed in mice, a high proportion of cycling transcripts peak midway through the dark phase, coinciding with the peak period of physical activity and feeding in nocturnal species. Clock genes in the negative limb such as CRY and PER, control the diurnal rhythm of exercise performance through regulation of energy substrate utilization (Jordan et al., 2017; Ezagouri et al., 2019). Histone deacetylase 3 (HDAC3), the interacting partner of clock proteins including REV-ERB, also governs exercise performance (Hong et al., 2017). Meanwhile, a chronotype effect on the circadian expression of many types of physical performance has also been observed (Chatterjee and Ma, 2016; Vitale et al., 2019). For example, optimal human muscle torque, strength and power are generally displayed in the late afternoon but not in the morning, suggesting that locomotor activity may coordinate the phase of the intrinsic rhythmic expression of genes in skeletal muscle.
Besides the above mentioned circadian regulation on skeletal muscle, physical activity could function as a strong clock entrainment signal, particularly for the skeletal muscle clock (Sato et al., 2019). Resistance exercise is capable of shifting the expression of diurnally regulated genes in human skeletal muscle (Zambon et al., 2003). Loss of muscle activity leads to marked muscle atrophy and reduced expression of core clock genes in mouse skeletal muscle (Zambon et al., 2003). Overall, recent findings demonstrate the intimate interplay between the cell-autonomous circadian clock and muscle physiology.
Blood
Many parameters in blood exhibit circadian rhythmicity, including leukocytes, erythrocytes, chemokines (e.g., CCL2, CCL5), cytokines (e.g., TNFα, IL-6), and hormones (Schilperoort et al., 2020). The most apparent oscillation in blood is observed in the number and type of circulating leukocytes, which peak in the resting phase and reach a trough in the activity phase during 24 h in humans and rodents (He et al., 2018). This time-dependent alteration of leukocytes reflects a rhythmic mobilization from hematopoietic organs and the recruitment process to tissue/organs (Méndez-Ferrer et al., 2008; Scheiermann et al., 2012). For example, the mobilization of leukocytes from the bone marrow is regulated by photic cues which are transmitted to the SCN and modulate the microenvironment of the bone marrow through adrenergic signals (Méndez-Ferrer et al., 2008).
Leukocytes exit the blood by a series of interactions with the endothelium, which involves various adhesion molecules, chemokines and chemokine receptors (Vestweber, 2015). Using a screening approach, He et al. (2018) depicted the time-dependent expression profile of the pro-migratory molecules on different endothelial cells and leukocyte subsets. Specific inhibition of the promigratory molecule or depletion of Bmal1 in leukocyte subsets or endothelial cells can diminish the rhythmic recruitment of the leukocyte subset to tissues/organs, indicating that the spatiotemporal emigration of leukocytes is highly dependent on the tissue context and cell-autonomous rhythms (Scheiermann et al., 2012; He et al., 2018). Cell-autonomous clocks also control diurnal migration of neutrophils (Adrover et al., 2019), Ly6C-high inflammatory monocytes (Nguyen et al., 2013) in the blood and leukocyte trafficking in the lymph nodes (Druzd et al., 2017).
Furthermore, the circadian recruitment process of leukocytes was not only found in the steady state but also in some pathologic states, such as natural aging (Adrover et al., 2019), the LPS-induced inflammatory scenario (He et al., 2018), and parasite infections (Hopwood et al., 2018). These findings suggest that leukocyte migration retains a circadian rhythmicity in response to pathogenic insults. Although mammalian erythrocytes lack the genetic oscillator, the peroxiredoxin system in erythrocytes has been shown to follow 24-h redox cycles (O’Neill and Reddy, 2011). Moreover, the membrane conductance and cytoplasmic conductivity of erythrocytes exhibit circadian rhythmicity depending on cellular K++ levels (Henslee et al., 2017). These observations indicate that non-transcriptional oscillators can regulate the circadian rhythms in denucleated cells. In addition to leukocytes and erythrocytes, other parameters in blood like chemokines and cytokines also exhibit a circadian rhythmicity (Schilperoort et al., 2020). Together, emerging evidence shows that the circadian rhythm can be easily found in blood elements which are essential contributors to the maintenance of circadian physiology (Figure 4A).
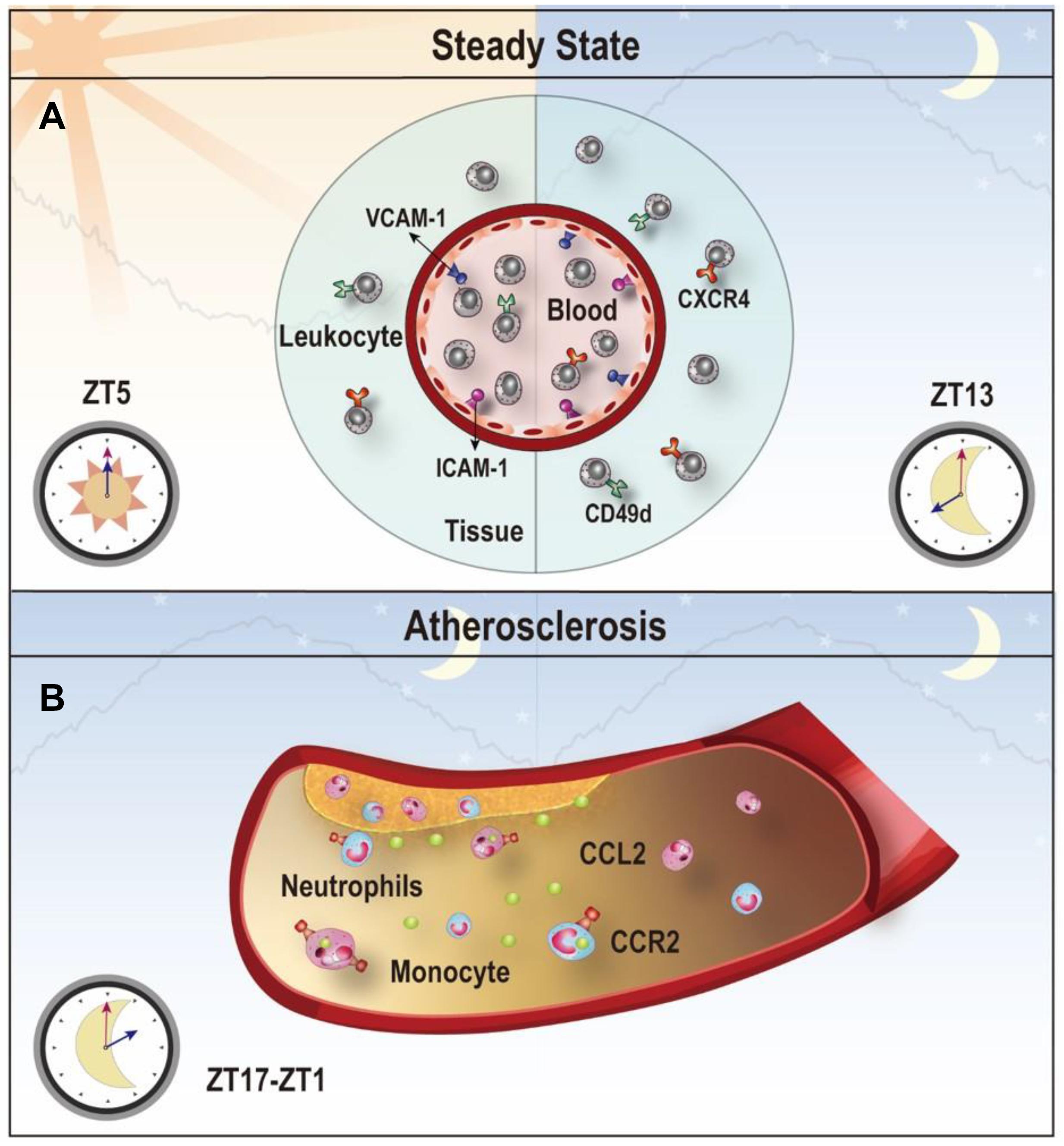
Figure 4. Clock-controlled checkpoints in leukocyte migration. (A) Leukocyte migration is controlled by the circadian clock. Rhythmic expression of promigratory molecules, such as ICAM-1, VCAM-1, CD49d, and CXCR4, promotes migration and retention of leukocytes to tissues, which peaks at ZT5 in the steady state. (B) Chemokine CCL2-CCR2 signaling is a clock-controlled checkpoint in leukocyte migration under atherosclerosis. Myeloid cells adhere to atherosclerotic lesions in a rhythmic manner with a peak between ZT17-ZT1 because of the diurnal expression of the CCL2-CCR2 axis. Targeting the CCL2-CCR2 axis in this time period may reduce inflammation during atherogenesis.
Peripheral and Central Nervous System
Circadian clock directs multiple metabolic and physiological functions in both the peripheral and central nervous system (Figure 5). In the central nervous system, many physiological processes controlled by extra-SCN hypothalamic nuclei display diurnal rhythms, such as those involved in energy and temperature regulation, glucose and lipid metabolism (Paul et al., 2020). Clocks in the forebrain, arcuate nucleus and dorsomedial hypothalamus can integrate external cues including temperature and nutrition cycles. Complete loss of circadian behavior was found in forebrain/SCN-specific Bmal1 knockout mice, and the related circadian rhythms in peripheral tissues was differentially affected by light/dark cycles and feeding (Izumo et al., 2014). Time-restricted feeding in mice has been shown to impair the body temperature homeostasis (Zhang et al., 2020c). Circadian gene expression analysis in the dorsomedial hypothalamus revealed that rhythmically reprogramming of thermoregulation gene expression is involved in the impairment of body temperature regulation (Zhang et al., 2020c). Integrative cistromic and transcriptomic analysis showed that REV-ERB-dependent leptin signaling in the arcuate nucleus plays an important role in the control of diurnal leptin sensitivity and food intake in diet-induced obesity (Adlanmerini et al., 2021). With more and more neuronal circadian oscillators uncovered, circadian rhythms of the circuit-level communication, organization, and physiological functions need to be explored.
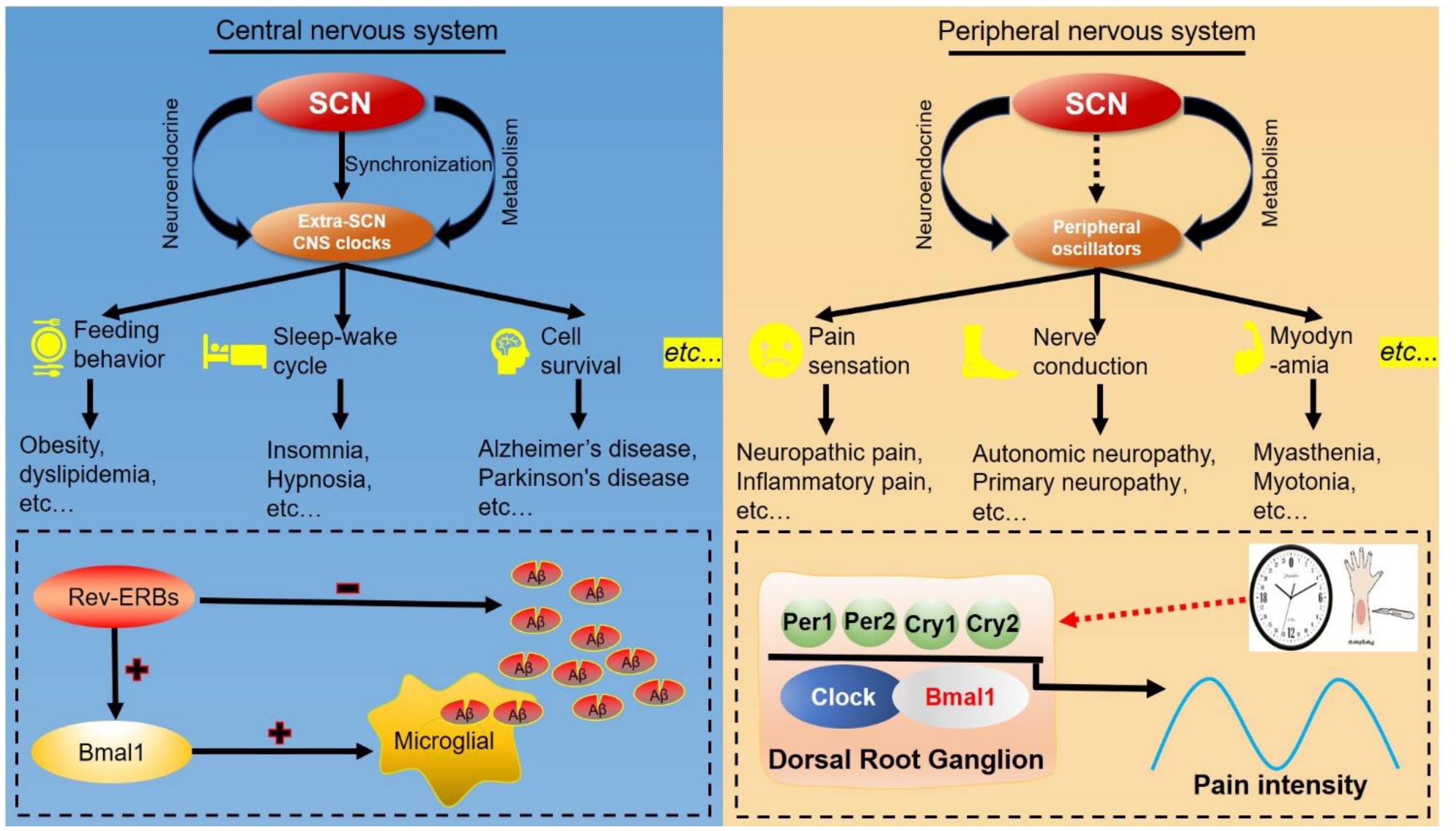
Figure 5. Clock-controlled checkpoints in neurons. The suprachiasmatic nucleus (SCN) synchronizes extra-SCN clocks and peripheral oscillators in the central nervous system (CNS) and peripheral nervous system. Left: The SCN master oscillator and its output extra-SCN CNS clocks control diverse functions in the central nervous system, including feeding behavior, sleep–wake cycles and cell survival. For example, REV-ERB decreases the number of amyloid-beta (Aβ) and increases BMAL1 transcription to accelerate microglial uptake of Aβ. Right: Peripheral oscillators can regulate functions and diseases of the peripheral nervous system, such as pathological pain. Rhythmic expression of BMAL1 and PER2 in dorsal root ganglions modulate the response to noxious stimulation, and establish the circadian rhythm of pathological pain.
The hypothalamus-pituitary-adrenal (HPA) axis is a major neuroendocrine pathway involved in stress response, metabolism, and circadian rhythm. HPA is regulated in a circadian manner, and peaks in the dawn in humans, or in the onset of the dark phase for nocturnal animals (Oster et al., 2017). Rhythmic release of the inhibitory neuropeptide, vasopressin, from the SCN periodically inhibits corticotrophin-releasing hormone (CRH)-neurons in the paraventricular nucleus (PVN) of hypothalamus. Vasopressin may reach the CRH neurons of PVN via either extracellular space/fluid or direct neuronal projection (Vrang et al., 1995; Tousson and Meissl, 2004). Release of CRH into the anterior pituitary promotes the release of adrenocorticotrophic hormone (ACTH) into the blood. Plasma ACTH is circadian, however, ACTH precursor Pomc does not oscillate at the transcript level in the anterior pituitary (Oster et al., 2017). ACTH reaches the adrenal gland and promotes secretion of glucocorticoids to the blood. The adrenal-clock controls circadian and acute secretion of glucocorticoids (Leliavski et al., 2014). Bmal1-deficiency decreases the diurnal rise of plasma glucocorticoids around the onset of the dark phase. Bmal1-decificency abolishes stress-induced rise of plasma glucocorticoids, but does not affect stress-induced rise of plasma ACTH. PER2 governs the diurnal rise in plasma glucocorticoid at the onset of the dark phase (Yang et al., 2009; Russell et al., 2021). PER2 does not regulate glucocorticoid secretion towards major stressors, such as hypoglycemia, ACTH, and physical restraint, neither does PER2 regulate ACTH peptide rhythm in the hypothalamus (Yang et al., 2009). Together, these findings demonstrate that both the SCN-clock and the adrenal-clock control the circadian activity of the HPA axis.
The peripheral nervous system mainly consists of sensory neurons, and their primary function is to transmit the sensory signals such as pain, touch, itch, and temperature sensation (Niesler et al., 2021). Pain sensitivity follows a daily cycle in many clinical conditions, and there is strong evidence to support the rhythmicity in response to nociceptive stimuli (Bruguerolle and Labrecque, 2007). The processing of painful stimulation occurs in the dorsal horn (DH), an area of the spinal cord that receives noxious stimulation signals from peripheral tissues via several types of primary afferent nerve fibers (Crodelle et al., 2019). Pain perception exhibits a 24-h rhythm regardless of whether pain threshold is objectively or subjectively assessed (Burish et al., 2019). Mechanistically, BMAL1, CLOCK, PER1, and REV-ERBα contribute to neuropathic pain at evening and cluster headache at midnight (Burish et al., 2019). Core clock genes are rhythmically expressed in neurons of the dorsal root ganglion (Kim H. K. et al., 2020). A Per2 mutation abolishes the circadian rhythm of the inflammatory pain response (peak ZT4) (Zhang et al., 2012b). Together, these studies provide evidence to support a potential strategy for improvement of pain treatment based on the circadian rhythm. More detailed studies are required to explore this phenomenon and more clinical trials needed to validate these findings.
Male Reproductive System
Diurnal rhythms of sperm count, sperm motility, and sperm chromatin integrity have been found in rodents. There are also several studies which have investigated the diurnal variation of semen parameters in humans (Ni et al., 2019; Sati, 2020). Variations in semen parameters were found across different time points in most of the studies, although a high-resolution sampling study is required to confidently profile the circadian pattern. It is also well known that semen parameters have circannual variation both in men and male animals (Chemineau et al., 2008; Xie et al., 2018). These studies suggest that the circadian clock and its regulatory mechanisms may play an important role in the regulation of the male reproductive system.
Clock genes are expressed in different parts of the male reproductive system, including extra-testicular ducts and accessary organs. However, the presence of a cell-autonomous clock in testes remains controversial (Figure 6; Alvarez et al., 2003; Mazzoccoli et al., 2012). In insects, per mRNA in testes oscillate under light-dark conditions, but the diurnal rhythm is not self-sustaining under constant darkness (Gvakharia et al., 2000). Leydig cells, the primary androgen-secreting cells in testes, express BMAL1 in a circadian manner, however, the mRNA levels of clock genes are not diurnal in testes (Chen et al., 2017). Dexamethasone synchronizes the expression of several circadian clock genes and steroidogenic-related genes in Leydig cells in vitro (Chen et al., 2017). Expression of PER1 protein is strictly isolated to certain stages of spermatogenesis, i.e., spermatogonia and condensing spermatids, while the expression of CLOCK is restricted to round spermatids (Alvarez et al., 2003). In the diurnal transcriptome atlas for major neural and peripheral tissues of the Papio anubis (baboon), 1672 cycling genes were identified in testes (Mure et al., 2018). However, the core clock genes such as CLOCK, BMAL1, PER1-3, CRY1-2, and RORA were not in the list. It is likely that a cell-autonomous clock is restricted to specific cell types such as the Leydig cell. The molecular analysis using bulk tissues masks the rhythmicity since clock genes may be expressed ubiquitously in different types of cells including the clock-less cells. The use of single-cell omics in circadian studies would resolve this issue since these techniques have already been applied in the testes (Guo et al., 2018; Lau et al., 2020; Shami et al., 2020).
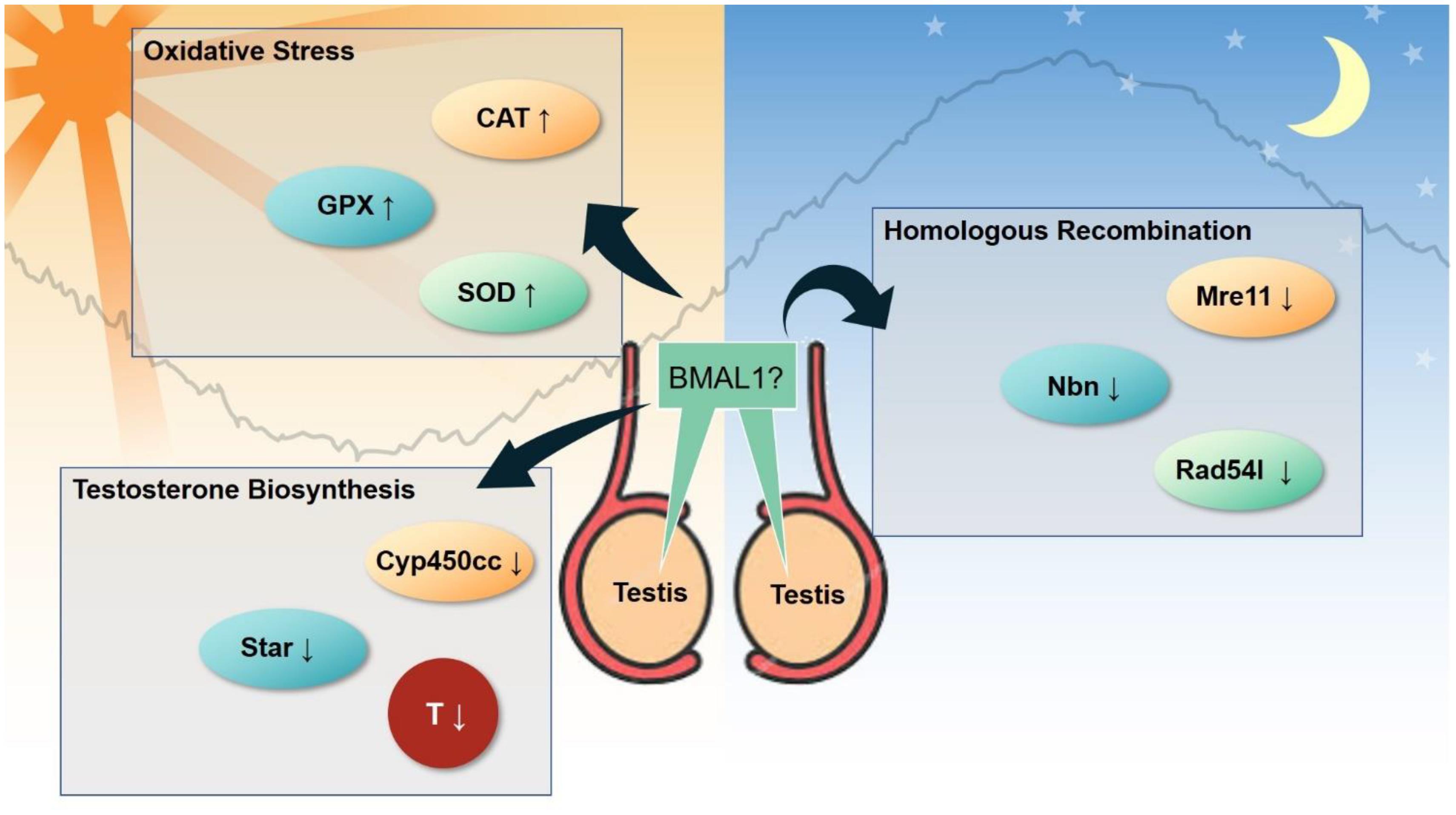
Figure 6. Emerging clock-controlled checkpoints in the testis. Emerging evidence suggests the role of the core clock protein BMAL1 in the regulation of oxidative stress, homologous recombination and testosterone biosynthesis in testis, which may potentially contribute to the pathogenesis of circadian-related disorders. CAT, catalase. GPx, glutathione peroxidase. SOD, superoxide dismutase. Cyp450cc, cytochrome P450 side-chain cleavage. Star, steroidogenic acute regulatory protein. T, testosterone. Mre11, double strand break repair nuclease. Nbn, nibrin. Rad54l, RAD54 like.
Clock genes are essential for the development of the male reproductive system. Knockout of the Bmal1 gene induces infertility and decreases the production of testosterone in mice (Alvarez et al., 2008). Bmal1-deficient male mice seem to have defects in copulatory behaviors (Schoeller et al., 2016). Interestingly, if Bmal1 was conditionally knocked out during adult life, the fertility of the mice was conserved (Yang et al., 2016). Circadian clock becomes functional at around embryonic days 13–18 (Umemura and Yagita, 2020). It has to be determined whether Bmal1 has a non-clock function on the male reproduction or that the testes-clock is required for the male reproduction before sex maturity. If induced deletion of Bmal1 on embryonic days 13–18 still preserved fertility in males, it would clearly support the non-clock function of BMAL1 in male fertility. Knockdown of Clock in mouse testes leads to reproductive damages, such as small litter size, decreased in vitro fertility rate, blastula formation rate, and acrosin activity of the sperm (Liang et al., 2013). Mechanistically, BMAL1 and CLOCK regulate the assembly of chromatoid bodies through interaction with MVH, a key component of the sperm chromatoid body (Peruquetti et al., 2012). A deficiency of Cry1 was found to increase apoptosis of mouse testicular germ cells and decrease sperm count (Li C. et al., 2018). Sertoli cells provide a nurturing and supportive niche for spermatogenesis. The specific knockdown of Rora in Sertoli cells at puberty leads to declined sperm count in rats (Mandal et al., 2018). In C. elegans, NHR-23/NR1F1 (RORA) depletion causes infertility due to the arrest of primary spermatocytes rather than haploid sperm (Ragle et al., 2020).
Disruption of circadian cycles is associated with high activity of the hypothalamic-pituitary-adrenal (HPA) axis. Lassi et al. (2021) showed that inverted feeding (night restricted feeding, of which night refers to the light phase) leads to dampened rhythm and high circulating levels of corticosterone in male founder mice. By carefully controlling the mating and breeding procedure, Lassi et al. (2021) demonstrated that paternal circadian disruption by inverted feeding can be transmitted to male offspring through hyper-corticosteronemia at conception. Hyper-corticosteronemia at conception resulted in a plethora of metabolic abnormalities in the male offspring, such as hyperphagia, hyper-metabolism, hyperglycemia, and hyper-corticosteronemia (Lassi et al., 2021). This trans-generational transmission of paternal circadian disruption does not seem to be pathogenic, as body weight, glucose tolerance, and insulin levels remained normal. Interestingly, wildtype male offspring of Clock mutant males recapitulate the high energy metabolism, indicating that inverted feeding imprints the high HPA activity in male offspring in part through the circadian clock (Lassi et al., 2021). Thus, paternal glucocorticoid signaling at conception may transmit the effects of circadian disruption to the offspring.
In humans, genetic polymorphisms of circadian genes have been linked to fertility and semen quality. Three single nucleotide polymorphisms (SNPs) of the Clock gene (rs11932595, rs6811520, and rs6850524) were associated with the risk of infertility in a case-control study of 961 Slovenian and Serbian Caucasian men (Hodžić et al., 2013). Another two Clock SNPs (rs1801260 and rs3817444) were found to correlate with infertility risk in 672 Chinese men (Shen et al., 2015). Several SNPs were also associated with semen volume, sperm count, sperm motility, as well as the levels of testosterone and follicle-stimulating hormone (Zhang et al., 2012a; Shen et al., 2015). In an observational study of 106 university students, levels of testosterone correlated with scores on the Composite Scale of Morningness, an indicator of chronotype, but not with sleep duration (Randler et al., 2012). However, it is not clear whether there is substantial damage to male fertility in syndromes related to clock gene mutations, such as Familial Advanced Sleep Phase Syndrome.
Female Reproductive System: Placenta
The placenta is an organ that functions to exchange nutrients, gasses, wastes, and hormones between the mother and fetus. Growing evidence suggests the presence of a circadian clock in the placenta (Figure 7). Hypoxia has been shown to induce circadian expression of PER2 and DEC1 genes in human placental cells (Frigato et al., 2009). Transcript levels of clock genes BMAL1, CLOCK, CRY1-2, and PER1-2 oscillate in the murine gravid uterus and placenta during late gestation (Ratajczak et al., 2010). Placental function also expresses a daily rhythm, as observed in the maternal plasma human chorionic gonadotropin (hCG) levels during early pregnancy, at the time of maximum placental hCG expression (Díaz-Cueto et al., 1994). Pérez et al. (2015) revealed the first piece of evidence demonstrating circadian expression of CLOCK, BMAL1, PER2, and CRY1 genes in the human full-term placenta. Clarkson-Townsend et al. (2020) found seasonal rhythmicity of DEC1 in human full-term placentas, adding evidence for the placenta as a peripheral clock. While clock mutant mice exhibit defects in female fertility and growth retardation associated with the placenta, the standard transcriptional/translation feedback loops of the oscillator mechanism in placenta appear less coordinated and robust. Rather, one study showed BMAL1 and PER rhythms are of low amplitude and not anti-phasic, suggesting a weak, if any, functioning of the core clock in the placenta (Wharfe et al., 2011). As of now, it is premature to conclude that a self-sustaining circadian clock is present in the placenta. It is likely that only certain regions of the placenta have a functional clock. Demarez et al. (2021) showed that the trophoblast layer of the labyrinth zone has a functional clock by late gestation, which controls diurnal expression and activity of ABCB1, a xenobiotic efflux pump. A simple bioinformatics survey in the Human Protein Atlas database revealed strikingly high levels of core clock proteins in the placenta, such as PER2, CRY1, BMAL1. Together, these studies provide justification for the need to explore cell type-specific clocks in the placenta and link the circadian clock to the nexus of maternal-fetal communication.
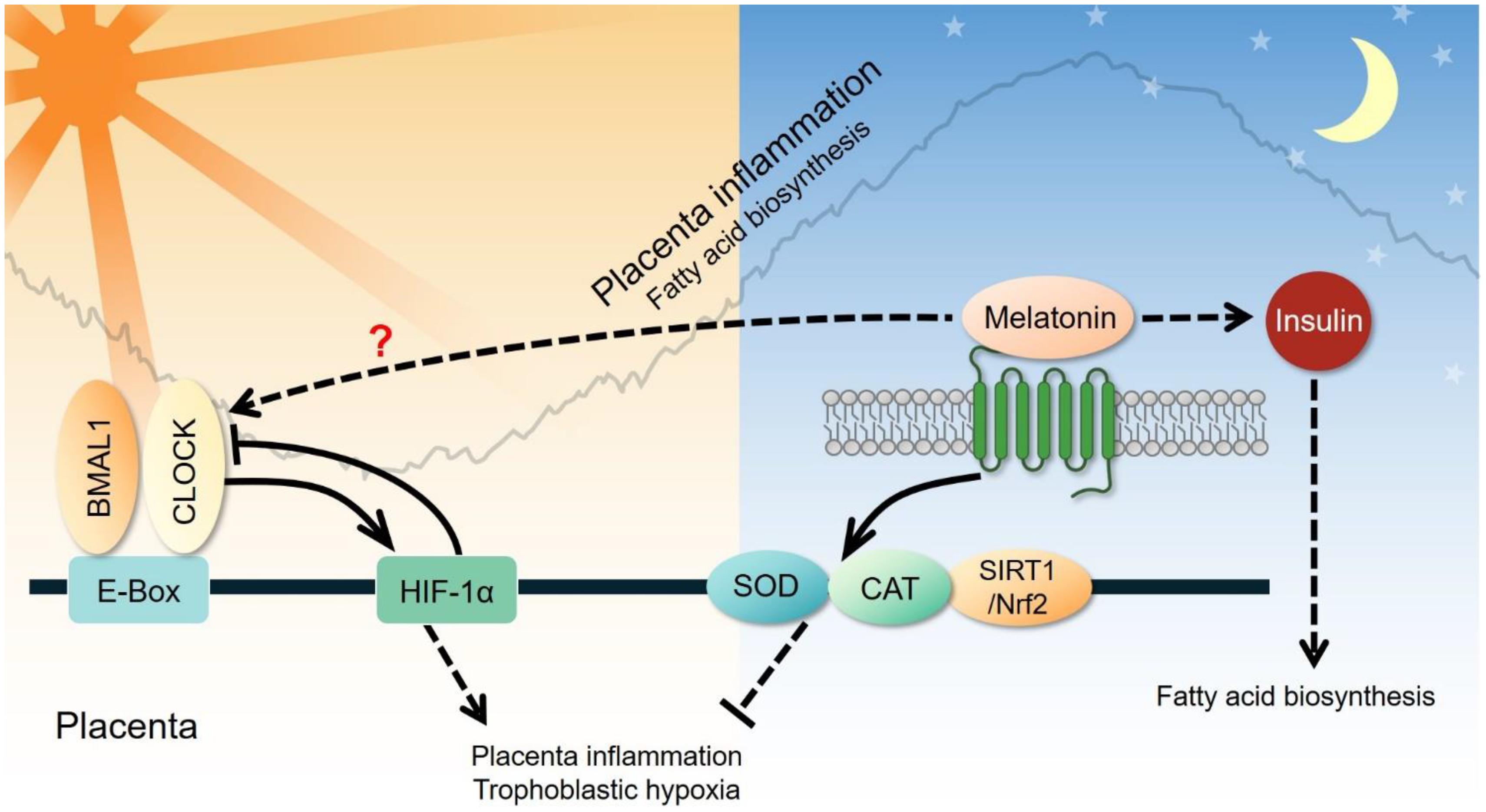
Figure 7. Clock-controlled checkpoints in the placenta. Placental inflammation and trophoblastic hypoxia are promoted by the CLOCK-HIF-1α signaling pathway during the active phase and suppressed through the melatonin-superoxide dismutase (SOD)-catalase (CAT) signaling pathway during the rest phase. However, the exact relationship between circadian genes and melatonin remains unclear. Melatonin can also elevate plasma insulin and subsequently increase fatty acid biosynthesis in the placenta.
Furthermore, melatonin and steroid hormones are known to follow circadian pattern due to the diurnal activity of the pineal gland and the hypothalamus-pituitary axis (Urlep and Rozman, 2013). Melatonin, a pineal gland secreted factor, is secreted mainly during the nighttime of the diurnal cycle. Melatonin plays an essential role in synchronizing the peripheral tissues to the 24-h circadian rhythms and likely provides feedback to the hypothalamus-pituitary-adrenal axis and the hypothalamus-pituitary-gonad axis (Shi L. et al., 2013; Huang et al., 2020). Melatonin can regulate the expression of clock genes in the placenta via its melatonin receptors (Lanoix et al., 2006), and this may contribute to adverse pregnancy outcomes (Lanoix et al., 2012; Olcese et al., 2013; Shimada et al., 2016).
Circadian Pathophysiology in Complex Diseases
Metabolic Associated Fatty Liver Disease
Metabolic associated fatty liver disease (MAFLD), formerly known as non-alcoholic fatty liver disease, is the liver manifestation of metabolic syndrome, and affects 25% of the global population (Bayoumi et al., 2020). MAFLD is the beginning stage of a continuum of chronic liver diseases, which ends with cirrhosis, liver failure, or hepatocellular carcinoma (Friedman et al., 2018). MAFLD is characterized by fat-loaded hepatocytes without signs of liver injury. Recent epidemiological studies have revealed a clear association between circadian rhythm disorder and the incidence of MAFLD (Peng et al., 2017; Wang H. et al., 2018; Hu et al., 2020). Clock mutant mice are prone to liver steatosis when fed a high-fat diet, which was able to be restored to homeostasis by time-restricted feeding (Chaix et al., 2019; Saran et al., 2020; Zhang et al., 2020b). The vast majority of hepatic triglycerides in the human liver come from adipose-released fatty acids (59%), followed by de novo lipogenesis (26%), and the diet (15%) (Francque et al., 2020). Studies of germline, liver-specific, and adipose-specific deletion of core clock genes indicate that core clocks contribute to liver steatosis mainly through extra-hepatic organs, such as the hypothalamus and adipose (Turek et al., 2005; Paschos et al., 2012; Zhang et al., 2020b), and may be dependent on exposure to circadian insult in early life (Yang et al., 2016).
Nuclear receptors and other non-clock transcription factors are emerging as regulators of diurnal lipid metabolism involved in the pathogenesis of MAFLD. Twenty of the 49 nuclear receptor genes exhibit a diurnal rhythm in the liver, providing the first line of transcription factors linking metabolism and clock (Yang et al., 2006). It has been well-established that REV-ERB and the co-repressor HDAC3 orchestrate diurnal rhythms of lipogenesis, and bile acid production (Bass and Lazar, 2016; Panda, 2016). Peroxisome-proliferating activator receptors (PPARα/β/γ) promote remodeling of the diurnal lipid metabolism in liver. A high-fat diet induces de novo oscillation of thousands of transcripts through PPARγ (peak ZT 8 h) in the liver, and contributes to diurnal rhythms of genes involved in lipid storage and gluconeogenesis (Eckel-Mahan et al., 2013). PPARδ is required for the diurnal rhythm of hepatic lipogenesis, and release of a diurnal lipid phosphatidylcholine 18:0/18:1 (peak at ZT 18 h) that promotes muscle fatty acid uptake (Liu et al., 2013). A high-fat diet enhances the daily cycling of enhancer activities associated with two opposing transcription factors, i.e., PPARα for fatty acid oxidation and SREBP1c for de novo lipogenesis (Guan et al., 2018). PPARs promote resolution of inflammation in immune cells through tethering apart the pro-inflammatory signaling complex, such as nuclear factor-kappa B and AP-1 (Li and Yang, 2011). Myeloid cell-specific disruption of Per1/Per2 results in hepatic steatosis, inflammation, and liver injury (Xu et al., 2014). Introducing PPARγ2 transgene back to clock-less macrophages helps to resolve the exacerbation of inflammation (Xu et al., 2014). Currently, dual-, and pan-PPAR agonists are intensively investigated as potential therapeutics for chronic liver diseases (Francque et al., 2020).
Cardiovascular Disease
Heart Disease
Heart diseases, including ischemic heart disease, diabetic cardiomyopathy, and heart failure, have a relationship to circadian clocks throughout their pathogenesis and prognosis (Crnko et al., 2019; Zhang et al., 2020a, b). Experimental models of heart disease, such as cardiac ischemia-reperfusion, pressure overload-induced cardiac hypertrophy, and diabetic cardiomyopathy, show clear disturbance of circadian clock oscillation in the heart (Young et al., 2001, 2002; Kung et al., 2007). Shift work increases the susceptibility to ischemic heart disease (Knutsson et al., 1986; Vetter et al., 2016), as does circadian disruption of behavioral rhythm in mice (Martino et al., 2007).
Ischemic heart disease is initiated by insufficient supply of blood (ischemia) to heart tissue due to obstruction of coronary arteries. Adaptive remodeling of heart metabolism is key to recovery and survival after ischemia (Sedej, 2018). Compelling evidence demonstrates several key clock-controlled checkpoints in heart metabolism that are crucial for treating ischemic heart disease. Myocardial ischemia induces adenosine-ADORA2B signaling that stabilizes PER2 through inhibition of proteasomal degradation (Eckle et al., 2012). PER2 promotes glycolysis and suppresses fatty acid oxidation in a HIF-1α-dependent manner, leading to reduced myocardial infarction. Interestingly, strong light exposure (10,000 lux) in the subjective day time stabilizes PER2 and protects the heart from ischemic-reperfusion injury. As reviewed in a previous section, BMAL1/CLOCK bipartite TF can modulate the diurnal oscillation of fatty acid oxidation, in part through transcriptional activity of a clock-output protein KLF15. REV-ERBα agonism protects against ischemic-reperfusion injury in the heart, though the detailed clock-controlled mechanism is not fully characterized (Stujanna et al., 2017). A transcriptional network including PER2-HIF1α and BMAL1/CLOCK-KLF15 is emerging as a clock-controlled checkpoint that licenses diurnal oscillation of cellular energy metabolism for metabolic reprogramming in ischemic heart disease (Figure 2).
Atherosclerosis
Atherosclerosis is a chronic process of plaque build-up in the vessel wall driven by lipid deposition and leukocyte infiltration to the subendothelial space (Wolf and Ley, 2019; Libby, 2021). The stenosis and restriction of the blood flow caused by the plaque make atherosclerosis the main cause of cardiovascular disease (Swirski and Nahrendorf, 2013). Epidemiological studies have demonstrated a strong connection between the disruption of circadian rhythms and atherogenic risk factors, such as lipid disorder and impaired glucose tolerance (Gooley, 2016; Poggiogalle et al., 2018). Leukocyte recruitment is greatly involved in the development of atherosclerosis (Swirski and Nahrendorf, 2013). In murine models of induced atherosclerosis using ApoE–/– mice on a high-fat diet, neutrophils and monocytes were recruited to the atherosclerotic lesions rhythmically due to a morning peak of the CCL2 rhythm on the endothelium and the CCR2 rhythm on neutrophils and monocytes (Winter et al., 2018). Targeting the CCR2-CCL2 axis at a specific time achieved better attenuation of myeloid cell adhesion (Winter et al., 2018). Disturbing the rhythmicity of the SCN clock may be sufficient to promote atherosclerosis. For example, feeding low-fat diet to ApoE–/– mice generated more atherosclerotic lesions in aortic roots under constant light exposure, compared to feeding the same diet under normal light-dark cycles (Chalfant et al., 2020). Another mouse model using APOE∗3-Leiden mice with alternating light/dark cycles also exhibited more severe atherosclerosis with more macrophages in the lesion due to increased expression of ICAM-1 and CCL2 in the lesions (Schilperoort et al., 2020). The findings from these studies suggest that disturbance of the circadian rhythm can aggravate atherosclerosis. The rhythmic recruitment of myeloid cells to the atherosclerotic lesions driven by the circadian expression of the CCL2-CCR2 axis provides a mechanistic basis for chronotherapeutic treatments (Figure 4B).
Neurodegenerative Diseases and Diabetic Neuropathy
Diabetic neuropathy is associated with a markedly reduced quality of life and poor prognosis (Callaghan et al., 2012). Impairment of cognitive function is increasingly being considered as an inevitable complication of type 2 diabetes mellitus (T2DM), and it has been associated with the risk of Alzheimer’s disease (AD) and vascular dementia. Considerable overlap has also been identified in the pathophysiology of T2DM and AD (Biessels and Despa, 2018). Sundown syndrome or sundowning, a well-known rhythmical neuropsychiatric phenomenon taking place in the late afternoon or early evening, is commonly observed in AD patients (Khachiyants et al., 2011). Another neuropsychiatric phenomenon is that pain intensity is often higher during night compared to day time. In addition, bright light exposure or exogenous melatonin supplementation has been found to improve sundowning symptoms, cognitive deficits, and sleep quality in AD patients (Roccaro and Smirni, 2020).
Emerging evidence supports the role of the circadian clock in the pathogenesis and progression of these neurological diseases. Pharmacological inhibition or genetic knockdown of REV-ERB was found to increase Bmal1 transcription and to accelerate microglial uptake of amyloid-beta (Aβ) (Lee et al., 2020; Liu W. W. et al., 2020). Deletion of Rev-Erbα in an AD model was shown to decrease Aβ number and prevent the increase in AD-associated microglia markers such as TREM2 and CD45 (Lee et al., 2020). Exogenous light can inhibit the synthesis and secretion of melatonin, while the absence of light can promote production and secretion of melatonin. Melatonin can exhibit its effects regarding alleviating circadian disruption through regulation of related clock genes including PER1 and BMAL1 (Yamanaka et al., 2010), which have been confirmed to be associated with the development of neurodegenerative diseases. Deletion of Per1/Per2 has been found to increase Chi3l1 expression in cerebrospinal fluid, which is a widely biomarker that is increased with the development of AD (Lananna et al., 2020). Loss of Bmal1 in astrocytes was demonstrated to promote neuronal death due to aging and neurodegenerative diseases through the regulation of astrogliosis in a synergistic manner by a cell-autonomous mechanism and a lesser non-cell-autonomous signal from neurons (Lananna et al., 2018). These studies further confirmed that circadian clock-controlled checkpoints such as REV-ERBα play an important role in the pathogenesis of neurological diseases (Figure 5).
At present, accumulated β-amyloid (Aβ) in extracellular senile plaques and abnormally hyperphosphorylated tau in intracellular neurofibrillary tangles have been identified as the pathological features of AD (Polanco et al., 2018). Melatonin, produced by the pineal gland and associated with circadian rhythms, has been demonstrated to play an important role in the interaction with Aβ and tau pathology. Melatonin has been found to suppress the reduction of PI3K activity, pSer473 on Akt and pSer9 on GSK-3β, and this can lead to the reduction of Aβ aggregation in AD (Ali and Kim, 2015). Additionally, melatonin can effectively attenuate tau pathology in AD through regulation of kinases including death-associated protein kinase 1 (DAPK1) (Chen et al., 2020). In a post-transcriptional manner melatonin can significantly decrease DAPK1 expression in mouse primary cortical neurons and human neuronal cell lines. Moreover, melatonin directly binds to DAPK1 to increase DAPK1 degradation resulting in decreased tau phosphorylation at multiple sites related to AD (Chen et al., 2020). Based on these studies, it is clear that melatonin signaling is a promising clock-controlled checkpoint in the pathogenesis of AD and further exploration of effective therapeutic targets based on these findings is warranted.
Neuropathic pain is another important symptom in diabetic neuropathy, and this symptom can severely decrease the patients’ quality of life. One previous human study has suggested that patients’ pain intensity of diabetic neuropathy is often exacerbated at night (Gilron et al., 2013), indicating that pain intensity in diabetic neuropathy follows a circadian rhythm. However, the related mechanism has not yet been elucidated. One study attempted to characterize the circadian properties of neuropathic pain hypersensitivity in animal models of type I and type II diabetes (Akamine et al., 2018). Using a diabetic mouse model, they found that accumulation of sorbitol in the sciatic nerve may modulate the circadian properties of diabetes-induced neuropathic pain hypersensitivity. However, as described above, various forms of neuropathic pain share similar circadian properties. The special mechanism underlying the circadian rhythm of diabetes-induced neuropathic pain requires further research.
Male Reproductive Disorder
The 24/7 modern lifestyle and social system has resulted in substantial changes in circadian behaviors of humans. Approximately 20 percent of the population are shift workers (Rajaratnam and Arendt, 2001). The social jetlag between work days and weekends is even more prevalent (Leypunskiy et al., 2018; Zhang et al., 2019). The effect of circadian disruption on the male reproductive system deserves attention. Several studies have analyzed the association between shift work and infertility risk, semen quality and/or reproductive hormones, as summarized by Caetano et al. (2020). Nevertheless, the results of these studies did not reach a consensus. One of the major reasons for this controversy may be that shift work consists of different work schedules, e.g., permanent night work and rotating shift work, which may lead to different health effects. A study of 1346 men in the community showed that rotating shift workers, but not permanent night workers, had lower sperm count compared to day workers (Liu K. et al., 2020). In the same publication, social jetlag between work days and free days was found to be quantitatively associated with decreased sperm count in 796 male university students.
Circadian disorder may contribute to the pathogenesis of male reproductive disorder in rodents. Male Sprague-Dawley rats with prolonged light exposure (20-h light:4 h-dark) were found to have increased sperm count and sperm motility and lower proportion of sperm abnormalities, while the rats with prolonged exposure to darkness (4-h light : 20-h dark) only showed a decreased proportion of sperm abnormalities (Moustafa, 2020). In contrast, another study reported that photoperiod changes only induced alterations in testosterone levels but had no effect on other characteristics such as semen quality and pregnancy rate after mating (Majrashi et al., 2017). Moreover, when pregnant rats were exposed to constant light, their male offspring showed a decrease in numerous indicators, including testosterone levels, number of Sertoli cells, sperm count and normal sperm count (Ogo et al., 2020). To simulate exposure to rotating shift work, a recent study put male mice into rotating light/dark cycles (Liu K. et al., 2020). The authors found that the sperm count of the male mice decreased, while the circadian oscillation of sperm count was not affected. In addition, sleep deprivation or sleep restriction is also hazardous to the male reproductive system (Chen et al., 2016). Despite numerous studies indicate the essential role of circadian disorder on the pathogenesis of male infertility, the underlying mechanism remains to be clarified (Figure 6).
In regards to the clock-controlled checkpoints in male reproductive system, several studies have suggested that reactive oxygen species (ROS) are activated in part through glutathione peroxidase, and superoxide dismutase (Torres et al., 2014; Alvarenga et al., 2015; Moustafa, 2020). Alteration of the testosterone levels were also observed in some circadian misalignment models (Qin et al., 2014; Arrebola and Abecia, 2017; Majrashi et al., 2017; Moustafa, 2020). The current knowledge around specific details of molecular events following the input of circadian misalignment is limited. Liu K. et al. (2020) suggested that the homologous recombination pathway was disrupted following circadian desynchrony in a rodent model. It remains unknown whether any of these molecular events are induced by unique signals. We speculate that BMAL1 may be a master regulator. BMAL1 is well known to govern the synthesis of testosterone (Alvarez et al., 2003), and regulates oxidative stress in other organ systems such as the vasculature and liver (Jacobi et al., 2015; Xie et al., 2020), although no such evidence is available for the testis. It is not clear whether disruption of the homologous recombination can also be induced by BMAL1, but the expression of core clock genes including BMAL1 is altered in the mouse testis (Liu K. et al., 2020). Twelve micro (mi)RNA are altered in the testes of Cry1 mutant mice, all of which are predicted to target the components of the core clock (Li C. et al., 2018). These findings deserve further confirmation under different exposure conditions. In addition, emerging evidence suggests that retinoic acid and its nuclear receptors are controlled by the clock during spermatogenesis (Bittman, 2016).
Diabetic Complications Related to the Female Reproductive System
Gestational Diabetes Mellitus
Gestational diabetes mellitus (GDM) is a complication of pregnancy that has similar characteristics as type 2 diabetes mellitus (T2D), such as glucose intolerance, insulin resistance, and impaired insulin secretion (Catalano et al., 1999). The pathogenesis of GDM is associated with abnormal expression of circadian-related genes. In a cohort of 40 Greek pregnant women with GDM, four with T2D and 20 healthy pregnant women, significant reductions in the peripheral blood BMAL1, PER3, PPARD, and CRY2 transcript levels were found in the GDM group, supporting the view that disorders of clock gene expression may play a pathogenic role in GDM (Ratajczak et al., 2010). Pappa et al. (2013a) studied BMAL1 polymorphisms in GDM women and healthy controls and showed that the rs7950226 (G > A) and rs11022775 (T > C) polymorphisms of the BMAL1 gene, combined with the rs7950226A/rs11022775C haplotypes were able to increase the susceptibility to GDM. In addition, the expression level of BMAL1 mRNA from peripheral blood was significantly decreased in GDM patients compared to healthy controls (Pappa et al., 2013b).
Circadian neuroendocrine factors, such as glucocorticoids and melatonin, are altered in GDM (Pilorz et al., 2009; Sen and Hoffmann, 2020). Fabiś et al. (2002) found that melatonin increases blood insulin levels and decreases the synthesis of free fatty acids in experiments conducted on rats. In pancreatic β-cells, a genome-wide association analysis of 18,236 type 2 diabetic subjects demonstrated that mutations in melatonin receptor 2 (MT2) inhibits their response to melatonin, blocking the release of insulin from pancreatic β-cells (Prokopenko et al., 2009). Tuomi et al. (2016) also demonstrated that melatonin treatment reduced insulin secretion in risk G-allele carriers, which suggests that enhanced melatonin signaling decreases insulin secretion in the pancreas. More recently, genotyping of 1,025 Chinese women with a history of GDM showed that gestational weight gain may alter the effect of circadian-associated melatonin receptor 1B (MTNR1B) gene variants on long-term glucose changes (Nisa et al., 2018). GDM is known to be associated with chronic inflammation and the accumulation of oxidative damage in the placenta without affecting placental anatomy and their vascular structure in the majority of cases. Nevertheless, little has been studied on the distribution and pathophysiology of core clock genes in the GDM placenta.
Preeclampsia
Preeclampsia (PE) is characterized by hypertension and proteinuria after 20 weeks of gestation. The most accepted mechanism leading to the etiology of PE is shallow trophoblast invasion and abolished spiral artery remodeling that leads to placental hypoxia and oxidative stress. The most well-established link between circadian rhythm and PE is melatonin. Nakamura et al. (2001) reported that nocturnal melatonin levels in pregnant women with PE are significantly lower than those in normal pregnant women. Lanoix et al. (2012) also demonstrated reduced blood levels of melatonin in pregnant women with PE, compared to those with normal pregnancies. These results indicate that melatonin may be involved in the pathogenesis of PE. Melatonin is a potent antioxidant and may reduce the oxidative damage caused by ROS in the placenta (Manda et al., 2007; Tan et al., 2007; de la Sierra et al., 2009). Reiter et al. (2014) reported that melatonin protects cells against oxidative stress in the ovary and placenta. Another study has shown that melatonin prevents oxidative stress by inducing the expression and activity of catalase and superoxide dismutase, and inhibiting the expression of vascular endothelial growth factor (Valenzuela et al., 2015). In a mouse model, Lee et al. (2019a) found that melatonin reduces placental oxidative stress associated with intrauterine inflammation, which is capable of causing maternal placental malperfusion. A recent study demonstrated that the CLOCK gene may participate in the pathogenesis of PE via hypoxia. They found that the oscillation of CLOCK mRNA and protein levels are abnormal in the placenta of human patients and in rodent models of PE (Li Y. et al., 2020). The impairment of trophoblast proliferation, migration, and invasion under hypoxic conditions is able to be reversed by silencing the CLOCK gene in trophoblast cells (Li Y. et al., 2020). Therefore, studies on placental clock-controlled checkpoints in oxidative stress and the response to hypoxia may provide mechanistic insights into the pathogenesis of PE (Figure 7).
Preterm Birth
Preterm birth is defined as birth at a gestational age of fewer than 37 weeks and is one of the major causes of neonatal death worldwide (Kumar et al., 2017). Many studies have indicated that maternal shift work is related to preterm birth. In a study of occupationally exposed pregnancy cohorts, McDonald et al. (1988) found that a long work duration and shift changes were correlated with preterm birth. A cohort study of 845 female textile workers in China in 1992 showed that shift work increased the risk of preterm birth (Xu et al., 1994). Findings from a prospective cohort study of 1,908 pregnant women indicated that women who worked night shifts during pregnancy had a 50% increased risk of preterm delivery (Pompeii et al., 2005). Prospective cohort studies in the Nurses’ Health Study have suggested that night shift work is associated with an increased risk of early preterm birth (Whelan et al., 2007). In a study of 673 pregnant women from Singapore, they found that women with night-eating had a higher risk of preterm delivery and speculated that this may be due to the discrepancy between the timing of eating and circadian rhythms (Loy et al., 2020). Interestingly, one study used full-spectrum light at night to suppress maternal melatonin secretion which resulted in lower serum melatonin concentrations and fewer contractions in full-term pregnant women. The authors suggested that light therapy or melatonin therapy may have the ability to delay labor and overcome preterm birth (Olcese et al., 2013). Furthermore, Lee et al. (2019b) indicated that melatonin treatment could alleviate LPS-induced intrauterine/placenta inflammation and reduce preterm birth in mice by activating the silent information regulator transcript-1/nuclear factor-erythroid 2-related factor 2 signaling pathway. It is clear that further investigations should be conducted into the links between clock-dependent maternal inflammation in the placenta and placenta. However, meta-analyses studies showed that there is no association between shift work and preterm birth (Bonzini et al., 2011; van Melick et al., 2014). Shift work can dramatically alter sleep/wake rhythms and meal timing, which may also drive preterm birth. Therefore, studies eliminating these confounding factors should be conducted in order to unravel the underlying dysregulation of circadian oscillation caused by shift work and its implication for preterm birth.
Obesity-Associated Circadian Regulation of Inter-Organ Communication
Obesity is a common comorbid risk factor in the pathogenesis of the complex disease, which is mechanistically linked to ectopic lipid deposition (Roden and Shulman, 2019) and metabolic-associated inflammation (metaflammation) (Hotamisligil, 2017). Mounting evidence supports the close links between circadian dysfunction and obesity, which can be referred to in previous reviews (Bass and Lazar, 2016; Panda, 2016; Reinke and Asher, 2019). Briefly, both irregular behavioral rhythms and clock dysfunction lead to obesity in rodents. Jet lag or constant light exposure contributes to leptin resistance (a hallmark of obesity), increased adiposity and weight gain (Shi S. Q. et al., 2013; Kettner et al., 2015). A high caloric diet increases food intake in the sleep phase, and results in a dampened daily rhythm of food intake (Kohsaka et al., 2007). Similarly, genetic mutation of core clock genes such as Bmal1, Clock, and Per2 leads to a dampened rhythm of food intake, and profound susceptibility to diet-induced obesity (Turek et al., 2005; Yang et al., 2009; Paschos et al., 2012).
Obesity is not merely a problem of overnutrition, but also a dysfunction of inter-organ communication, particularly in the adipocyte-brain axis. Adipocyte BMAL1 controls diurnal rhythms of de novo synthesis of polyunsaturated fatty acids (PUFA) through periodic expression of stearoyl-CoA desaturase 1 (SCD1) and long-chain fatty-acid elongase ELOVL6 (Paschos et al., 2012). The release of PUFA to the circulation may engage the hypothalamic circuit and inhibit food intake. However, the circadian rhythm of adipose SCD1 transcript is not found in major omics studies (source: CircaDB, CircaMetDB, CirGRDB). Instead, the SCD2 transcript oscillates robustly in adipose tissue. Adipocyte O-GlcNAc transferase promotes SCD1/2-controlled fatty acid desaturation and tissue accumulation of anandamide, which activates local cannabinoid receptor signaling and promotes diet-induced hyperphagia and obesity (Li M.-D. et al., 2018). Adipocyte O-GlcNAcylation may promote obesity through its well-established target clock proteins, such as BMAL1, CLOCK and PER2 (Durgan et al., 2011; Kim et al., 2012; Kaasik et al., 2013; Li et al., 2013, 2019; Liu et al., 2021). Adipocyte REV-ERBα limits adipocyte expansion under a high caloric diet (Hunter et al., 2021). Deletion of Rev-Erbα in adipocytes results in profound obesity without adipose inflammation and fibrosis. It appears that REV-ERBα controls SCD1 expression and fatty acid desaturation in adipose tissue (Hunter et al., 2021).
Despite these findings, the receiving end in the brain is not fully characterized. Recently, Cedernaes et al. (2019) have shown that clocks in AgRP neurons (hunger neurons) of hypothalamus govern circadian transcriptional response to leptin, a critical adipose-secreted endocrine factor (Cedernaes et al., 2019). AgRP neurons project to the paraventricular nucleus (PVN) of hypothalamus, which coordinates neuronal inputs to elicit feeding and satiety. Kim E. R. et al. (2020) reported that the PVN-clock determines the diurnal rhythm of energy metabolism through rhythmic sensitivity to GABAergic inputs. Ablation of the PVN-clock results in obesity. Thus, clock-controlled checkpoints involved in the metabolism and signaling of PUFA, anandamide, and leptin may coordinately link adipocytes and hypothalamic neurons, which maintain energy homeostasis through inter-organ communication.
The hypothalamic-pituitary-adrenal (HPA) axis provides another potent means to mediate inter-organ crosstalk during obesity. Cushing syndrome presents extreme obesity when HPA goes wrong. Both hyper-cortisolism and low-amplitude rhythm of plasma glucocorticoids are well-known indicators of natural aging from rodents to nonhuman primates and humans (Thompson et al., 2020). However, it is still unsolved whether and how the circadian rhythm of HPA is involved in the pathogenesis of the complex disease. Adrenal gland-specific manipulation of the core clock genes would provide insights into this question.
In addition, obesity-associated inter-organ communication can take place between peripheral tissues. Metabolite profiling of diet-induced obese mice reveals that obesity decreases the temporal correlation of metabolites among seven organs and serum (Dyar et al., 2018b). Comparing metabolites in alanine metabolism and gluconeogenesis among organs and serum suggests that the glucose-alanine cycle, which transports amino acids from the muscle to liver for glucose production, is highly active in obesity (Dyar et al., 2018b). Though still in the early stage, the application of multi-omics, and single-cell omics would facilitate the elucidation of clock-controlled checkpoints in the obesity-associated inter-organ communication, which holds promise for diagnosis, prevention, and treatment of complex disease.
Time Medicine
As we reviewed above, the circadian clock is widely involved in the pathophysiology of complex diseases, particularly metabolic diseases. Time medicine is emerging as an interdisciplinary field to understand and target clock-controlled checkpoints for the improvement of well-being and health (Cederroth et al., 2019; Ruben et al., 2019; Allada and Bass, 2021; López-Otín and Kroemer, 2021). Time medicine includes two major parts, namely, clinical treatment based on the internal time, and drugging the clock.
Clinical Treatment Based on the Internal Time
For optimizing the time of clinical treatment, the time-of-day effect of both drug treatments and surgeries needs to be extensively determined in future clinical studies. Proof-of-principle examples are bedtime dosing of the anti-dyslipidemia drug statins and afternoon heart surgeries (Cederroth et al., 2019). It is reported that more than 75% of drugs from 106 clinical trials have shown obvious time-of-day effects or toxicity (Ruben et al., 2019), corroborating the idea that circadian time should be taken into consideration at both the pre-clinical and clinical stages (Cederroth et al., 2019). The onsets of complex diseases show a diurnal pattern. For example, cancer pain erupts at 10 a.m., stroke and sudden cardiac death usually occurs between 6 and 12 a.m., and asthma occurs at 4 a.m. Therefore, detailed clinical logs are also required for time-optimization of clinical treatment.
While hormones such as melatonin and glucocorticoids are well-established biomarkers of the circadian rhythm, tissue-specific biomarkers are largely unexplored. The circadian transcriptome is usually the starting point to identify tissue-specific biomarkers for chronotherapy. However, it is impractical to obtain clinical samples (especially for the brain) suitable for circadian studies, which normally require frequent sampling around the clock with decent temporal resolution. To fill the gap, an unsupervised algorithm called cyclic ordering by periodic structure (CYCLOPS) was developed to order clinical samples into circadian structure without time indication (Anafi et al., 2017). CYCLOPS analysis of RNA-seq data in 13 human tissues indicates that nearly half of the protein coding transcriptome is rhythmic in at least one tissue (Ruben et al., 2018). Most excitingly, they found that nearly a thousand of these cycling genes, which are involved in drug delivery and metabolism, or as drug targets, may mediate time-of-day drug efficacy. Empirical validation of these findings and large-scale input of data into CYCLOPS would improve the precision and accuracy of circadian biomarkers.
Prediction of the circadian phase in vivo is another important aspect for optimizing the time of clinical treatment. Due to the dynamic nature, profiling the circadian transcriptome atlas of human tissues is not enough but the phase information by itself is equally important for optimization of the time of clinical treatment. The prediction of the circadian phase of an individual’s drug target tissue(s) is a hot topic. In order to achieve this, several algorithms were invented, including Molecular-Timetable, ZeitZeiger, BIO-CLOCK, PLSR, and Time-Signature (Naef and Talamanca, 2020). The phase prediction process mainly includes four steps: training algorithms with time-indicated RNA seq data to extract biomarkers, building low-dimensional circadian trajectory, cross validation with known time labeled sample, and finally inferring the unknown sample’s phase. Using dim light melatonin onset (DLMO) as an SCN phase indicator, the accuracy of these algorithms was verified through inferring the phase of SCN, with a maximum prediction error of approximately 3 h. Besides SCN, more druggable tissues’ specific biomarkers and clinical feasible phase prediction methods need to be developed in the future.
Drugging the Clock
Dysregulation of the circadian rhythm is a hallmark of complex diseases (López-Otín and Kroemer, 2021). Alteration of the period length results in abnormal sleep-wake patterns (Ashbrook et al., 2020). Circadian amplitude damping always precedes neurodegenerative disorders and accelerates aging related disorders (Abbott et al., 2020). The promising efficacy of time-restricted feeding in preclinical anti-obesity and anti-cardiometabolic disease studies indicates a robust phase misalignment in these complex diseases related to life style (Panda, 2016). The scheduling of light exposure and diet quality represents a handy approach to restore circadian rhythms and health. For example, short-term exposure to bright light shifts the phase of SCN and alleviates jet-lag or circadian related mood disorders (Blume et al., 2019). Time-restricted eating improves the metabolic profile in cardiometabolic diseases (Panda, 2016). Future work targeting clock-controlled checkpoints hold great promise for translating these mechanisms into clinical practice and devising small chemicals for applications in people that have compliance issues with these cues.
Clock-modulating compounds represent a highly valuable approach to reset the clock. Several small molecules have been identified from chemical screening (Ruan et al., 2021). GSK3-β and CKIε/δ inhibitors were discovered from the LOPAC library (Library of Pharmacologically Active Compounds) to shorten or lengthen the circadian period. CRY1 stabilizer KL001 increases the circadian period and inhibits hepatic glucose production in vitro. SR9009/SR9011 and Nobiletin regulate circadian amplitude through REV-ERB/ROR agonism (He et al., 2016; Nohara et al., 2019). Notably, cordycepin shifts circadian phase in both central and peripheral clocks through RUVBL2-mediated circadian chromatin remodeling (Ju et al., 2020). Structure-based virtual screen is an alternative method, which is time and labor saving. Encouragingly, a recent virtual screen based on the crystal structure of melatonin receptor 1 uncovered several bioactive molecules from 150 million ‘lead-like’ molecules, which may have potentially therapeutics significance (Stein et al., 2020). Another study using a molecular-docking approach also found small molecules which have circadian amplitude-modulating activity, binding to CLOCK and disrupting its interaction with BMAL1 (Doruk et al., 2020). In light of these advances, more clock-modulating compounds targeting clock-controlled checkpoints would likely be available in the near future and their clinical efficacy would be tested for better care and treatment of complex diseases.
Conclusion
Over the past two decades, circadian regulation of physiology and metabolism has been the key direction of circadian rhythm research. Substantial progress has been made in elucidating circadian clock-controlled pathways and checkpoints in peripheral tissues and their relevance in complex diseases. Despite these achievements, the majority of the research has been focused on a few prototypical organs, such as the liver, and to a lesser degree, the heart and muscle. We anticipate that the next phase of this research would lead to translational medicine in liver disease, deep mechanistic insights in circadian biology and medicine related to the heart and muscle, and further findings in less characterized tissues and organs relevant to complex diseases.
Author Contributions
M-DL: conceptualization, investigation (intro and liver), and supervision. HX: investigation (liver) and visualization. YY and WH: investigation (blood). XY and GD: investigation (neuron). HL, HZ, and T-LH: investigation (female reproduction). DT, FD and ZZ: investigation (heart). T-LH: investigation (female reproduction). QC: investigation (male reproduction). DJ: investigation (intro and time medicine). KC: investigation (muscle). M-DL, HX, YY, XY, HL, DT, HZ, ZZ, T-LH, QC, GD, DJ, KC, FD, and WH: writing. All authors contributed to the article and approved the submitted version.
Funding
This work was supported by the National Natural Science Foundation of China Grants 92057109 (M-DL), 82073548 (KC), 31971054 (WH), 81871208 (QC), 81871185 (MH), 81873663 (ZZ), 81701096 (GD), and PLA Youth Training Project for Medical Science 20QNPY020 (FD).
Conflict of Interest
The authors declare that the research was conducted in the absence of any commercial or financial relationships that could be construed as a potential conflict of interest.
Publisher’s Note
All claims expressed in this article are solely those of the authors and do not necessarily represent those of their affiliated organizations, or those of the publisher, the editors and the reviewers. Any product that may be evaluated in this article, or claim that may be made by its manufacturer, is not guaranteed or endorsed by the publisher.
Acknowledgments
We thank Yanli Wu for administrative assistance and Jamie de Seymour for language editing.
References
Abbott, S. M., Malkani, R. G., and Zee, P. C. (2020). Circadian disruption and human health: a bidirectional relationship. Eur. J. Neurosci. 51, 567–583. doi: 10.1111/ejn.14298
Adlanmerini, M., Nguyen, H. C. B., Krusen, B. M., Teng, C. W., Geisler, C. E., Peed, L. C., et al. (2021). Hypothalamic REV-ERB nuclear receptors control diurnal food intake and leptin sensitivity in diet-induced obese mice. J. Clin. Invest. 131:e140424. doi: 10.1172/JCI140424
Adrover, J. M., del Fresno, C., Crainiciuc, G., Cuartero, M. I., Casanova-Acebes, M., Weiss, L. A., et al. (2019). A neutrophil timer coordinates immune defense and vascular protection. Immunity 50, 390–402.e10. doi: 10.1016/j.immuni.2019.01.002
Akamine, T., Kusunose, N., Matsunaga, N., Koyanagi, S., and Ohdo, S. (2018). Accumulation of sorbitol in the sciatic nerve modulates circadian properties of diabetes-induced neuropathic pain hypersensitivity in a diabetic mouse model. Biochem. Biophys. Res. Commun. 503, 181–187. doi: 10.1016/j.bbrc.2018.05.209
Ali, T., and Kim, M. O. (2015). Melatonin ameliorates amyloid beta-induced memory deficits, tau hyperphosphorylation and neurodegeneration via PI3/Akt/GSk3β pathway in the mouse hippocampus. J. Pineal Res. 59, 47–59. doi: 10.1111/jpi.12238
Allada, R., and Bass, J. (2021). Circadian mechanisms in medicine. N. Engl. J. Med. 384, 550–561. doi: 10.1056/NEJMra1802337
Alvarenga, T. A., Hirotsu, C., Mazaro-Costa, R., Tufik, S., and Andersen, M. L. (2015). Impairment of male reproductive function after sleep deprivation. Fertil. Steril. 103, 1355–1362.e1. doi: 10.1016/j.fertnstert.2015.02.002
Alvarez, J. D., Chen, D., Storer, E., and Sehgal, A. (2003). Non-cyclic and developmental stage-specific expression of circadian clock proteins during murine Spermatogenesis1. Biol. Reprod. 69, 81–91. doi: 10.1095/biolreprod.102.011833
Alvarez, J. D., Hansen, A., Ord, T., Bebas, P., Chappell, P. E., Giebultowicz, J. M., et al. (2008). The circadian clock protein BMAL1 is necessary for fertility and proper testosterone production in mice. J. Biol. Rhythms 23, 26–36. doi: 10.1177/0748730407311254
Anafi, R. C., Francey, L. J., Hogenesch, J. B., and Kim, J. (2017). CYCLOPS reveals human transcriptional rhythms in health and disease. Proc. Natl. Acad. Sci. U S A. 114, 5312–5317. doi: 10.1073/pnas.1619320114
Arrebola, F., and Abecia, J.-A. (2017). Effects of season and artificial photoperiod on semen and seminal plasma characteristics in bucks of two goat breeds maintained in a semen collection center. Vet. World 10, 521–525. doi: 10.14202/vetworld.2017.521-525
Ashbrook, L. H., Krystal, A. D., Fu, Y. H., and Ptáček, L. J. (2020). Genetics of the human circadian clock and sleep homeostat. Neuropsychopharmacology 45, 45–54. doi: 10.1038/s41386-019-0476-477
Bass, J., and Lazar, M. A. (2016). Circadian time signatures of fitness and disease. Science 354, 994–999. doi: 10.1126/science.aah4965
Bayoumi, A., Grønbæk, H., George, J., and Eslam, M. (2020). The epigenetic drug discovery landscape for metabolic-associated fatty liver disease. Trends Genet. 36, 429–441. doi: 10.1016/j.tig.2020.03.003
Biessels, G. J., and Despa, F. (2018). Cognitive decline and dementia in diabetes mellitus: mechanisms and clinical implications. Nat. Rev. Endocrinol. 14, 591–604. doi: 10.1038/s41574-018-0048-47
Bittman, E. L. (2016). Timing in the testis. J. Biol. Rhythms 31, 12–36. doi: 10.1177/0748730415618297
Blume, C., Garbazza, C., and Spitschan, M. (2019). Effects of light on human circadian rhythms, sleep and mood. Somnologie 23, 147–156. doi: 10.1007/s11818-019-00215-x
Bonzini, M., Palmer, K. T., Coggon, D., Carugno, M., Cromi, A., and Ferrario, M. M. (2011). Shift work and pregnancy outcomes: a systematic review with meta-analysis of currently available epidemiological studies. BJOG Int. J. Obstet. Gynaecol. 118, 1429–1437. doi: 10.1111/j.1471-0528.2011.03066.x
Bray, M. S., Shaw, C. A., Moore, M. W. S., Garcia, R. A. P., Zanquetta, M. M., Durgan, D. J., et al. (2008). Disruption of the circadian clock within the cardiomyocyte influences myocardial contractile function, metabolism, and gene expression. Am. J. Physiol. - Hear. Circ. Physiol. 294, 1036–1047. doi: 10.1152/ajpheart.01291.2007
Bruguerolle, B., and Labrecque, G. (2007). Rhythmic pattern in pain and their chronotherapy. Adv. Drug Deliv. Rev. 59, 883–895. doi: 10.1016/j.addr.2006.06.001
Buhr, E. D., Yoo, S.-H., and Takahashi, J. S. (2010). Temperature as a universal resetting cue for mammalian circadian oscillators. Science 330, 379–385. doi: 10.1126/science.1195262
Burish, M. J., Chen, Z., and Yoo, S. H. (2019). Emerging relevance of circadian rhythms in headaches and neuropathic pain. Acta Physiol. 225:e13161. doi: 10.1111/apha.13161
Caetano, G., Bozinovic, I., Dupont, C., Léger, D., Lévy, R., and Sermondade, N. (2020). Impact of sleep on female and male reproductive functions: a systematic review. Fertil. Steril. 115, 715–731. doi: 10.1016/j.fertnstert.2020.08.1429
Callaghan, B. C., Cheng, H. T., Stables, C. L., Smith, A. L., and Feldman, E. L. (2012). Diabetic neuropathy: clinical manifestations and current treatments. Lancet Neurol. 11, 521–534. doi: 10.1016/S1474-4422(12)70065-70060
Catalano, P. M., Huston, L., Amini, S. B., and Kalhan, S. C. (1999). Longitudinal changes in glucose metabolism during pregnancy in obese women with normal glucose tolerance and gestational diabetes mellitus. Am. J. Obstet. Gynecol. 180, 903–916. doi: 10.1016/s0002-9378(99)70662-70669
Cedernaes, J., Huang, W., Ramsey, K. M., Waldeck, N., Cheng, L., Marcheva, B., et al. (2019). Transcriptional basis for rhythmic control of hunger and metabolism within the AgRP neuron. Cell Metab. 29, 1078–1091.e5. doi: 10.1016/j.cmet.2019.01.023
Cederroth, C. R., Albrecht, U., Bass, J., Brown, S. A., Dyhrfjeld-Johnsen, J., Gachon, F., et al. (2019). Medicine in the fourth dimension. Cell Metab. 30, 238–250. doi: 10.1016/j.cmet.2019.06.019
Chaix, A., Lin, T., Le, H. D., Chang, M. W., and Panda, S. (2019). Time-Restricted feeding prevents obesity and metabolic syndrome in mice lacking a circadian clock. Cell Metab. 29, 303–319.e4. doi: 10.1016/j.cmet.2018.08.004
Chalfant, J. M., Howatt, D. A., Tannock, L. R., Daugherty, A., and Pendergast, J. S. (2020). Circadian disruption with constant light exposure exacerbates atherosclerosis in male apolipoproteine-deficient mice. Sci. Rep. 10:9920. doi: 10.1038/s41598-020-66834-66839
Chatterjee, S., and Ma, K. (2016). Circadian clock regulation of skeletal muscle growth and repair. F1000Research 5:1549. doi: 10.12688/f1000research.9076.1
Chemineau, P., Guillaume, D., Migaud, M., Thiry, J., Pellicer-Rubio, M., and Malpaux, B. (2008). Seasonality of reproduction in mammals: intimate regulatory mechanisms and practical implications. Reprod. Domest. Anim. 43, 40–47. doi: 10.1111/j.1439-0531.2008.01141.x
Chen, D., Mei, Y., Kim, N., Lan, G., Gan, C. L., Fan, F., et al. (2020). Melatonin directly binds and inhibits death-associated protein kinase 1 function in Alzheimer’s disease. J. Pineal Res. 69:e12665. doi: 10.1111/jpi.12665
Chen, H., Gao, L., Xiong, Y., Yang, D., Li, C., Wang, A., et al. (2017). Circadian clock and steroidogenic-related gene expression profiles in mouse Leydig cells following dexamethasone stimulation. Biochem. Biophys. Res. Commun. 483, 294–300. doi: 10.1016/j.bbrc.2016.12.149
Chen, Q., Yang, H., Zhou, N., Sun, L., Bao, H., Tan, L., et al. (2016). Inverse U-shaped association between sleep duration and semen quality: longitudinal observational study (MARHCS) in chongqing. China. Sleep 39, 79–86. doi: 10.5665/sleep.5322
Cho, H., Zhao, X., Hatori, M., Yu, R. T., Barish, G. D., Lam, M. T., et al. (2012). Regulation of circadian behaviour and metabolism by REV-ERB-α and REV-ERB-β. Nature 485, 123–127. doi: 10.1038/nature11048
Clarkson-Townsend, D. A., Kennedy, E., Everson, T. M., Deyssenroth, M. A., Burt, A. A., Hao, K., et al. (2020). Seasonally variant gene expression in full-term human placenta. FASEB J. 34, 10431–10442. doi: 10.1096/fj.202000291R
Crnko, S., Du Pré, B. C., Sluijter, J. P. G. G., and Van Laake, L. W. (2019). Circadian rhythms and the molecular clock in cardiovascular biology and disease. Nat. Rev. Cardiol. 16, 437–447. doi: 10.1038/s41569-019-0167-164
Crodelle, J., Piltz, S. H., Hagenauer, M. H., and Booth, V. (2019). Modeling the daily rhythm of human pain processing in the dorsal horn. PLoS Comput. Biol. 15:e1007106. doi: 10.1371/journal.pcbi.1007106
de la Sierra, A., Redon, J., Banegas, J. R., Segura, J., Parati, G., Gorostidi, M., et al. (2009). Prevalence and factors associated with circadian blood pressure patterns in hypertensive patients. Hypertension 53, 466–472. doi: 10.1161/HYPERTENSIONAHA.108.124008
Demarez, C., De Assis, L. V. M., Krohn, M., Ramella, N., Schwaninger, M., Oster, H., et al. (2021). The trophoblast clock controls transport across placenta in mice. Development 148:dev197673. doi: 10.1242/dev.197673
Díaz-Cueto, L., Méndez, J. P., Barrios-de-Tomasi, J., Lee, J. Y., Wide, L., Veldhuis, J. D., et al. (1994). Amplitude regulation of episodic release, in vitro biological to immunological ratio, and median charge of human chorionic gonadotropin in pregnancy. J. Clin. Endocrinol. Metab. 78, 890–897. doi: 10.1210/jcem.78.4.8157717
Ding, G., Li, X., Hou, X., Zhou, W., Gong, Y., Liu, F., et al. (2021). REV-ERB in GABAergic neurons controls diurnal hepatic insulin sensitivity. Nature 592, 763–767. doi: 10.1038/s41586-021-03358-w
Doruk, Y. U., Yarparvar, D., Akyel, Y. K., Gul, S., Taskin, A. C., Yilmaz, F., et al. (2020). A CLOCK-binding small molecule disrupts the interaction between CLOCK and BMAL1 and enhances circadian rhythm amplitude. J. Biol. Chem. 295, 3518–3531. doi: 10.1074/jbc.RA119.011332
Druzd, D., Matveeva, O., Ince, L., Harrison, U., He, W., Schmal, C., et al. (2017). Lymphocyte circadian clocks control lymph node trafficking and adaptive immune responses. Immunity 46, 120–132. doi: 10.1016/j.immuni.2016.12.011
Durgan, D. J., Hotze, M. A., Tomlin, T. M., Egbejimi, O., Graveleau, C., Abel, E. D., et al. (2005). The intrinsic circadian clock within the cardiomyocyte. Am. J. Physiol. - Hear. Circ. Physiol. 289, 1530–1541. doi: 10.1152/ajpheart.00406.2005
Durgan, D. J., Pat, B. M., Laczy, B., Bradley, J. A., Tsai, J. Y., Grenett, M. H., et al. (2011). O-GlcNAcylation, novel post-translational modification linking myocardial metabolism and cardiomyocyte circadian clock. J. Biol. Chem. 286, 44606–44619. doi: 10.1074/jbc.M111.278903
Durgan, D. J., Pulinilkunnil, T., Villegas-Montoya, C., Garvey, M. E., Frangogiannis, N. G., Michael, L. H., et al. (2010). Ischemia/Reperfusion Tolerance is Time-of-Day–Dependent. Circ. Res. 106, 546–550. doi: 10.1161/CIRCRESAHA.109.209346
Dyar, K. A., Ciciliot, S., Wright, L. E., Biensø, R. S., Tagliazucchi, G. M., Patel, V. R., et al. (2014). Muscle insulin sensitivity and glucose metabolism are controlled by the intrinsic muscle clock. Mol. Metab. 3, 29–41. doi: 10.1016/j.molmet.2013.10.005
Dyar, K. A., Hubert, M. J., Mir, A. A., Ciciliot, S., Lutter, D., Greulich, F., et al. (2018a). Transcriptional programming of lipid and amino acid metabolism by the skeletal muscle circadian clock. PLoS Biol. 16:e2005886. doi: 10.1371/journal.pbio.2005886
Dyar, K. A., Lutter, D., Artati, A., Ceglia, N. J., Liu, Y., Armenta, D., et al. (2018b). Atlas of circadian metabolism reveals system-wide coordination and communication between clocks. Cell 174, 1571–1585. doi: 10.1016/j.cell.2018.08.042
Eckel-Mahan, K. L., Patel, V. R., De Mateo, S., Orozco-Solis, R., Ceglia, N. J., Sahar, S., et al. (2013). Reprogramming of the circadian clock by nutritional challenge. Cell 155, 1464–1478. doi: 10.1016/j.cell.2013.11.034
Eckle, T., Hartmann, K., Bonney, S., Reithel, S., Mittelbronn, M., Walker, L. A., et al. (2012). Adora2b-elicited Per2 stabilization promotes a HIF-dependent metabolic switch crucial for myocardial adaptation to ischemia. Nat. Med. 18, 774–782. doi: 10.1038/nm.2728
Everett, L. J., and Lazar, M. A. (2014). Nuclear receptor Rev-erbα: up, down, and all around. Trends Endocrinol. Metab. 25, 586–592. doi: 10.1016/j.tem.2014.06.011
Ezagouri, S., Zwighaft, Z., Sobel, J., Baillieul, S., Doutreleau, S., Ladeuix, B., et al. (2019). Physiological and molecular dissection of daily variance in exercise capacity. Cell Metab. 30, 78–91.e4. doi: 10.1016/j.cmet.2019.03.012
Fabiś, M., Pruszyńska, E., and Maćkowiak, P. (2002). In vivo and in situ action of melatonin on insulin secretion and some metabolic implications in the rat. Pancreas 25, 166–169. doi: 10.1097/00006676-200208000-00009
Francque, S., Szabo, G., Abdelmalek, M. F., Byrne, C. D., Cusi, K., Dufour, J.-F., et al. (2020). Nonalcoholic steatohepatitis: the role of peroxisome proliferator-activated receptors. Nat. Rev. Gastroenterol. Hepatol. 18, 24–39. doi: 10.1038/s41575-020-00366-365
Friedman, S. L., Neuschwander-Tetri, B. A., Rinella, M., and Sanyal, A. J. (2018). Mechanisms of NAFLD development and therapeutic strategies. Nat. Med. 24, 908–922. doi: 10.1038/s41591-018-0104-9
Frigato, E., Lunghi, L., Ferretti, M. E., Biondi, C., and Bertolucci, C. (2009). Evidence for circadian rhythms in human trophoblast cell line that persist in hypoxia. Biochem. Biophys. Res. Commun. 378, 108–111. doi: 10.1016/j.bbrc.2008.11.006
Gabriel, B. M., and Zierath, J. R. (2019). Circadian rhythms and exercise — re-setting the clock in metabolic disease. Nat. Rev. Endocrinol. 15, 197–206. doi: 10.1038/s41574-018-0150-x
Gilron, I., Bailey, J. M., and Vandenkerkhof, E. G. (2013). Chronobiological characteristics of neuropathic pain: clinical predictors of diurnal pain rhythmicity. Clin. J. Pain 29, 755–759. doi: 10.1097/AJP.0b013e318275f287
Gooley, J. J. (2016). Circadian regulation of lipid metabolism. Proc. Nutr. Soc. 75, 440–450. doi: 10.1017/S0029665116000288
Guan, D., Xiong, Y., Borck, P. C., Jang, C., Doulias, P.-T. T., Papazyan, R., et al. (2018). Diet-Induced circadian enhancer remodeling synchronizes opposing hepatic lipid metabolic processes. Cell 174, 831–842.e12. doi: 10.1016/j.cell.2018.06.031
Guan, D., Xiong, Y., Trinh, T. M., Xiao, Y., Hu, W., Jiang, C., et al. (2020). The hepatocyte clock and feeding control chronophysiology of multiple liver cell types. Science 369, 1388–1394. doi: 10.1126/science.aba8984
Guo, J., Grow, E. J., Mlcochova, H., Maher, G. J., Lindskog, C., Nie, X., et al. (2018). The adult human testis transcriptional cell atlas. Cell Res. 28, 1141–1157. doi: 10.1038/s41422-018-0099-92
Gvakharia, B. O., Kilgore, J. A., Bebas, P., and Giebultowicz, J. M. (2000). Temporal and spatial expression of the period gene in the reproductive system of the codling moth. J. Biol. Rhythms 15, 4–12. doi: 10.1177/074873040001500102
He, B., Nohara, K., Park, N., Park, Y. S., Guillory, B., Zhao, Z., et al. (2016). The small molecule nobiletin targets the molecular oscillator to enhance circadian rhythms and protect against metabolic syndrome. Cell Metab. 23, 610–621. doi: 10.1016/j.cmet.2016.03.007
He, W., Holtkamp, S., Hergenhan, S. M., Kraus, K., de Juan, A., Weber, J., et al. (2018). Circadian expression of migratory factors establishes lineage-specific signatures that guide the homing of leukocyte subsets to tissues. Immunity 49, 1175–1190.e7. doi: 10.1016/j.immuni.2018.10.007
Henslee, E. A., Crosby, P., Kitcatt, S. J., Parry, J. S. W., Bernardini, A., Abdallat, R. G., et al. (2017). Rhythmic potassium transport regulates the circadian clock in human red blood cells. Nat. Commun. 8:1978. doi: 10.1038/s41467-017-02161-2164
Hodžić, A., Ristanović, M., Zorn, B., Tulić, C., Maver, A., Novaković, I., et al. (2013). Genetic variation in circadian rhythm genes CLOCK and ARNTL as risk factor for male infertility. PLoS One 8:e59220. doi: 10.1371/journal.pone.0059220
Hong, S., Zhou, W., Fang, B., Lu, W., Loro, E., Damle, M., et al. (2017). Dissociation of muscle insulin sensitivity from exercise endurance in mice by HDAC3 depletion. Nat. Med. 23, 223–234. doi: 10.1038/nm.4245
Hopwood, T. W., Hall, S., Begley, N., Forman, R., Brown, S., Vonslow, R., et al. (2018). The circadian regulator BMAL1 programmes responses to parasitic worm infection via a dendritic cell clock. Sci. Rep. 8:3782. doi: 10.1038/s41598-018-22021-22025
Hotamisligil, G. S. (2017). Inflammation, metaflammation and immunometabolic disorders. Nature 542, 177–185. doi: 10.1038/nature21363
Hu, C., Zhang, Y., Wang, S., Lin, L., Peng, K., Du, R., et al. (2020). Association of bedtime with the risk of non-alcoholic fatty liver disease among middle-aged and elderly Chinese adults with pre-diabetes and diabetes. Diabetes. Metab. Res. Rev. 36:e3322. doi: 10.1002/dmrr.3322
Huang, Y., Xu, C., He, M., Huang, W., and Wu, K. (2020). Saliva cortisol, melatonin levels and circadian rhythm alterations in Chinese primary school children with dyslexia. Medicine (Baltimore) 99:e19098. doi: 10.1097/MD.0000000000019098
Hunter, A. L., Pelekanou, C. E., Barron, N. J., Northeast, R. C., Grudzien, M., Adamson, A. D., et al. (2021). Adipocyte NR1D1 dictates adipose tissue expansion during obesity. eLife 10:e63324. doi: 10.7554/eLife.63324
Izumo, M., Pejchal, M., Schook, A. C., Lange, R. P., Walisser, J. A., Sato, T. R., et al. (2014). Differential effects of light and feeding on circadian organization of peripheral clocks in a forebrain Bmal1 mutant. eLife 3:e04617. doi: 10.7554/eLife.04617
Jacobi, D., Liu, S., Burkewitz, K., Kory, N., Knudsen, N. H., Alexander, R. K., et al. (2015). Hepatic Bmal1 regulates rhythmic mitochondrial dynamics and promotes metabolic fitness. Cell Metab. 22, 709–720. doi: 10.1016/j.cmet.2015.08.006
Jeyaraj, D., Haldar, S. M., Wan, X., McCauley, M. D., Ripperger, J. A., Hu, K., et al. (2012). Circadian rhythms govern cardiac repolarization and arrhythmogenesis. Nature 483, 96–101. doi: 10.1038/nature10852
Jordan, S. D., Kriebs, A., Vaughan, M., Duglan, D., Fan, W., Henriksson, E., et al. (2017). CRY1/2 selectively repress PPARδ and limit exercise capacity. Cell Metab. 26, 243–255.e6. doi: 10.1016/j.cmet.2017.06.002
Ju, D., Zhang, W., Yan, J., Zhao, H., Li, W., Wang, J., et al. (2020). Chemical perturbations reveal that RUVBL2 regulates the circadian phase in mammals. Sci. Transl. Med. 12:eaba0769. doi: 10.1126/scitranslmed.aba0769
Kaasik, K., Kivimäe, S., Allen, J. J., Chalkley, R. J., Huang, Y., Baer, K., et al. (2013). Glucose sensor O-GlcNAcylation coordinates with phosphorylation to regulate circadian clock. Cell Metab. 17, 291–302. doi: 10.1016/j.cmet.2012.12.017
Kettner, N. M., Mayo, S. A., Hua, J., Lee, C., Moore, D. D., and Fu, L. (2015). Circadian dysfunction induces leptin resistance in mice. Cell Metab. 22, 448–459. doi: 10.1016/j.cmet.2015.06.005
Khachiyants, N., Trinkle, D., Son, S. J., and Kim, K. Y. (2011). Sundown syndrome in persons with dementia: an update. Psychiatry Investig. 8, 275–287. doi: 10.4306/pi.2011.8.4.275
Kim, E. Y., Jeong, E. H., Park, S., Jeong, H. J., Edery, I., and Cho, J. W. (2012). A role for O-GlcNAcylation in setting circadian clock speed. Genes Dev. 26, 490–502. doi: 10.1101/gad.182378.111
Kim, E. R., Xu, Y., Cassidy, R. M., Lu, Y., Yang, Y., Tian, J., et al. (2020). Paraventricular hypothalamus mediates diurnal rhythm of metabolism. Nat. Commun. 11:3794. doi: 10.1038/s41467-020-17578-7
Kim, H. K., Lee, S. Y., Koike, N., Kim, E., Wirianto, M., Burish, M. J., et al. (2020). Circadian regulation of chemotherapy-induced peripheral neuropathic pain and the underlying transcriptomic landscape. Sci. Rep. 10:13844. doi: 10.1038/s41598-020-70757-w
Knutsson, A., Jonsson, B. G., Akerstedt, T., and Orth-Gomer, K. (1986). Increased risk of ischaemic heart disease in shift workers. Lancet 328, 89–92. doi: 10.1016/S0140-6736(86)91619-91613
Kohsaka, A., Laposky, A. D., Ramsey, K. M., Estrada, C., Joshu, C., Kobayashi, Y., et al. (2007). High-Fat diet disrupts behavioral and molecular circadian rhythms in mice. Cell Metab. 6, 414–421. doi: 10.1016/j.cmet.2007.09.006
Kumar, S., Sharma, S., and Thaker, R. (2017). Occupational, environmental, and lifestyle factors and their contribution to preterm birth – an overview. Indian J. Occup. Environ. Med. 21:9. doi: 10.4103/ijoem.IJOEM_155_16
Kung, T. A., Egbejimi, O., Cui, J., Ha, N. P., Durgan, D. J., Essop, M. F., et al. (2007). Rapid attenuation of circadian clock gene oscillations in the rat heart following ischemia-reperfusion. J. Mol. Cell. Cardiol. 43, 744–753. doi: 10.1016/j.yjmcc.2007.08.018
Lamia, K. A., Storch, K. F., and Weitz, C. J. (2008). Physiological significance of a peripheral tissue circadian clock. Proc. Natl. Acad. Sci. U S A. 105, 15172–15177. doi: 10.1073/pnas.0806717105
Lananna, B. V., Nadarajah, C. J., Izumo, M., Cedeño, M. R., Xiong, D. D., Dimitry, J., et al. (2018). Cell-Autonomous regulation of astrocyte activation by the circadian clock protein BMAL1. Cell Rep. 25, 1–9.e5. doi: 10.1016/j.celrep.2018.09.015
Lananna, B. V., McKee, C. A., King, M. W., Del-Aguila, J. L., Dimitry, J. M., Farias, F. H. G., et al. (2020). Chi3l1/YKL-40 is controlled by the astrocyte circadian clock and regulates neuroinflammation and Alzheimer’s disease pathogenesis. Sci. Transl. Med. 12:eaax3519. doi: 10.1126/scitranslmed.aax3519
Lanoix, D., Guérin, P., and Vaillancourt, C. (2012). Placental melatonin production and melatonin receptor expression are altered in preeclampsia: new insights into the role of this hormone in pregnancy. J. Pineal Res. 53, 417–425. doi: 10.1111/j.1600-079X.2012.01012.x
Lanoix, D., Ouellette, R., and Vaillancourt, C. (2006). Expression of melatoninergic receptors in human placental choriocarcinoma cell lines. Hum. Reprod. 21, 1981–1989. doi: 10.1093/humrep/del120
Lassi, M., Tomar, A., Comas-Armangué, G., Vogtmann, R., Dijkstra, D. J., Corujo, D., et al. (2021). Disruption of paternal circadian rhythm affects metabolic health in male offspring via nongerm cell factors. Sci. Adv. 7, 25–31. doi: 10.1126/sciadv.abg6424
Lau, X., Munusamy, P., Ng, M. J., and Sangrithi, M. (2020). Single-Cell RNA sequencing of the cynomolgus macaque testis reveals conserved transcriptional profiles during mammalian spermatogenesis. Dev. Cell 54, 548–566.e7. doi: 10.1016/j.devcel.2020.07.018
Lee, J., Kim, D. E., Griffin, P., Sheehan, P. W., Kim, D. H., Musiek, E. S., et al. (2020). Inhibition of REV-ERBs stimulates microglial amyloid-beta clearance and reduces amyloid plaque deposition in the 5XFAD mouse model of Alzheimer’s disease. Aging Cell 19:e13078. doi: 10.1111/acel.13078
Lee, J. Y., Li, S., Shin, N. E., Na, Q., Dong, J., Jia, B., et al. (2019a). Melatonin for prevention of placental malperfusion and fetal compromise associated with intrauterine inflammation-induced oxidative stress in a mouse model. J. Pineal Res. 67:e12591. doi: 10.1111/jpi.12591
Lee, J. Y., Song, H., Dash, O., Park, M., Shin, N. E., McLane, M. W., et al. (2019b). Administration of melatonin for prevention of preterm birth and fetal brain injury associated with premature birth in a mouse model. Am. J. Reprod. Immunol. 82:e13151. doi: 10.1111/aji.13151
Lefta, M., Campbell, K. S., Feng, H. Z., Jin, J. P., and Esser, K. A. (2012). Development of dilated cardiomyopathy in Bmal1-deficient mice. Am. J. Physiol. Hear. Circ. Physiol. 303, H475–H485. doi: 10.1152/ajpheart.00238.2012
Leliavski, A., Shostak, A., Husse, J., and Oster, H. (2014). Impaired glucocorticoid production and response to stress in arntl-deficient male mice. Endocrinology 155, 133–142. doi: 10.1210/en.2013-1531
Leypunskiy, E., Kıcıman, E., Shah, M., Walch, O. J., Rzhetsky, A., Dinner, A. R., et al. (2018). Geographically resolved rhythms in twitter use reveal social pressures on daily activity patterns. Curr. Biol. 28, 3763–3775.e5. doi: 10.1016/j.cub.2018.10.016
Li, C., Xiao, S., Hao, J., Liao, X., and Li, G. (2018). Cry1 deficiency leads to testicular dysfunction and altered expression of genes involved in cell communication, chromatin reorganization, spermatogenesis, and immune response in mouse testis. Mol. Reprod. Dev. 85, 325–335. doi: 10.1002/mrd.22968
Li, M.-D., Vera, N. B., Yang, Y., Zhang, B., Ni, W., Ziso-Qejvanaj, E., et al. (2018). Adipocyte OGT governs diet-induced hyperphagia and obesity. Nat. Commun. 9:5103. doi: 10.1038/s41467-018-07461-x
Li, E., Li, X., Huang, J., Xu, C., Liang, Q., Ren, K., et al. (2020). BMAL1 regulates mitochondrial fission and mitophagy through mitochondrial protein BNIP3 and is critical in the development of dilated cardiomyopathy. Protein Cell 11, 661–679. doi: 10.1007/s13238-020-00713-x
Li, L., Li, H., Tien, C.-L., Jain, M. K., and Zhang, L. (2020). Kruppel-Like factor 15 regulates the circadian susceptibility to ischemia reperfusion injury in the heart. Circulation 141, 1427–1429. doi: 10.1161/CIRCULATIONAHA.119.041664
Li, M.-D., Ruan, H.-B., Hughes, M. E., Lee, J.-S., Singh, J. P., Jones, S. P., et al. (2013). O-GlcNAc signaling entrains the circadian clock by inhibiting BMAL1/CLOCK ubiquitination. Cell Metab. 17, 303–310. doi: 10.1016/j.cmet.2012.12.015
Li, M.-D., and Yang, X. (2011). A retrospective on nuclear receptor regulation of inflammation: lessons from GR and PPARs. PPAR Res. 2011:742785. doi: 10.1155/2011/742785
Li, Y. H., Liu, X., Vanselow, J. T., Zheng, H., Schlosser, A., and Chiu, J. C. (2019). O-GlcNAcylation of PERIOD regulates its interaction with CLOCK and timing of circadian transcriptional repression. PLoS Genet. 15:e1007953. doi: 10.1371/journal.pgen.1007953
Li, Y., Li, J., Hou, Y., Huang, L., Bian, Y., Song, G., et al. (2020). Circadian clock gene Clock is involved in the pathogenesis of preeclampsia through hypoxia. Life Sci. 247:117441. doi: 10.1016/j.lfs.2020.117441
Liang, X., Cheng, S., Jiang, X., He, X., Wang, Y., Jiang, Z., et al. (2013). The noncircadian function of the circadian clock gene in the regulation of male fertility. J. Biol. Rhythms 28, 208–217. doi: 10.1177/0748730413486873
Libby, P. (2021). The changing landscape of atherosclerosis. Nature 592, 524–533. doi: 10.1038/s41586-021-03392-3398
Liu, K., Hou, G., Wang, X., Chen, H., Shi, F., Liu, C., et al. (2020). Adverse effects of circadian desynchrony on the male reproductive system: an epidemiological and experimental study. Hum. Reprod. 35, 1515–1528. doi: 10.1093/humrep/deaa101
Liu, W. W., Wei, S. Z., Huang, G. D., Liu, L. B., Gu, C., Shen, Y., et al. (2020). BMAL1 regulation of microglia-mediated neuroinflammation in MPTP-induced Parkinson’s disease mouse model. FASEB J. 34, 6570–6581. doi: 10.1096/fj.201901565RR
Liu, S., Brown, J. D., Stanya, K. J., Homan, E., Leidl, M., Inouye, K., et al. (2013). A diurnal serum lipid integrates hepatic lipogenesis and peripheral fatty acid use. Nature 502, 550–554. doi: 10.1038/nature12710
Liu, X., Blaženović, I., Contreras, A. J., Pham, T. M., Tabuloc, C. A., Li, Y. H., et al. (2021). Hexosamine biosynthetic pathway and O-GlcNAc-processing enzymes regulate daily rhythms in protein O-GlcNAcylation. Nat. Commun. 12:4173. doi: 10.1038/s41467-021-24301-24307
López-Otín, C., and Kroemer, G. (2021). Hallmarks of health. Cell 184, 33–63. doi: 10.1016/j.cell.2020.11.034
Lowe, M., Lage, J., Paatela, E., Munson, D., Hostager, R., Yuan, C., et al. (2018). Cry2 is critical for circadian regulation of myogenic differentiation by Bclaf1-Mediated mRNA stabilization of cyclin D1 and Tmem176b. Cell Rep. 22, 2118–2132. doi: 10.1016/j.celrep.2018.01.077
Loy, S. L., Cheung, Y. B., Cai, S., Colega, M. T., Godfrey, K. M., Chong, Y.-S., et al. (2020). Maternal night-time eating and sleep duration in relation to length of gestation and preterm birth. Clin. Nutr. 39, 1935–1942. doi: 10.1016/j.clnu.2019.08.018
Ma, D., Panda, S., and Lin, J. D. (2011). Temporal orchestration of circadian autophagy rhythm by C/EBPβ. EMBO J. 30, 4642–4651. doi: 10.1038/emboj.2011.322
Majrashi, K. A., Barakat, I. A., Al-Himaidi, A. R., and Adham, K. G. (2017). Effect of exogenous melatonin treatment on the reproductive characteristics and progeny of male rats exposed to different periods from light and darkness. Physiol. Res. 66, 507–518. doi: 10.33549/physiolres.933556
Manda, K., Ueno, M., and Anzai, K. (2007). AFMK, a melatonin metabolite, attenuates X-ray-induced oxidative damage to DNA, proteins and lipids in mice. J. Pineal Res. 42, 386–393. doi: 10.1111/j.1600-079X.2007.00432.x
Mandal, K., Sarkar, R. K., Sen Sharma, S., Jain, A., and Majumdar, S. S. (2018). Sertoli cell specific knockdown of RAR-related orphan receptor (ROR) alpha at puberty reduces sperm count in rats. Gene 641, 18–24. doi: 10.1016/j.gene.2017.10.032
Manella, G., Sabath, E., Aviram, R., Dandavate, V., Ezagouri, S., Golik, M., et al. (2021). The liver-clock coordinates rhythmicity of peripheral tissues in response to feeding. Nat. Metab. 3, 829–842. doi: 10.1038/s42255-021-00395-397
Martino, T. A., Tata, N., Belsham, D. D., Chalmers, J., Straume, M., Lee, P., et al. (2007). Disturbed diurnal rhythm alters gene expression and exacerbates cardiovascular disease with rescue by resynchronization. Hypertension 49, 1104–1113. doi: 10.1161/HYPERTENSIONAHA.106.083568
Martino, T., Arab, S., Straume, M., Belsham, D. D., Tata, N., Cai, F., et al. (2004). Day/night rhythms in gene expression of the normal murine heart. J. Mol. Med. 82, 256–264. doi: 10.1007/s00109-003-0520-1
Mayeuf-Louchart, A., Staels, B., and Duez, H. (2015). Skeletal muscle functions around the clock. Diab. Obes. Metab. 17, 39–46. doi: 10.1111/dom.12517
Mazzoccoli, G., Francavilla, M., Giuliani, F., Aucella, F., Vinciguerra, M., Pazienza, V., et al. (2012). Clock gene expression in mouse kidney and testis: analysis of periodical and dynamical patterns. J. Biol. Regul. Homeost. Agents 26, 303–311.
McDonald, A. D., McDonald, J. C., Armstrong, B., Cherry, N. M., Nolin, A. D., and Robert, D. (1988). Prematurity and work in pregnancy. Occup. Environ. Med. 45, 56–62. doi: 10.1136/oem.45.1.56
Méndez-Ferrer, S., Lucas, D., Battista, M., and Frenette, P. S. (2008). Haematopoietic stem cell release is regulated by circadian oscillations. Nature 452, 442–447. doi: 10.1038/nature06685
Moustafa, A. (2020). Effect of light-dark cycle misalignment on the hypothalamic-pituitary-gonadal axis, testicular oxidative stress, and expression of clock genes in adult male rats. Int. J. Endocrinol. 2020:1426846. doi: 10.1155/2020/1426846
Mure, L. S., Le, H. D., Benegiamo, G., Chang, M. W., Rios, L., Jillani, N., et al. (2018). Diurnal transcriptome atlas of a primate across major neural and peripheral tissues. Science 359:eaao0318. doi: 10.1126/science.aao0318
Naef, F., and Talamanca, L. (2020). How to tell time: advances in decoding circadian phase from omics snapshots. F1000Research 9:1150. doi: 10.12688/f1000research.26759.1
Nakamura, Y., Tamura, H., Kashida, S., Takayama, H., Yamagata, Y., Karube, A., et al. (2001). Changes of serum melatonin level and its relationship to feto-placental unit during pregnancy. J. Pineal Res. 30, 29–33. doi: 10.1034/j.1600-079x.2001.300104.x
Neufeld-Cohen, A., Robles, M. S., Aviram, R., Manella, G., Adamovich, Y., Ladeuix, B., et al. (2016). Circadian control of oscillations in mitochondrial rate-limiting enzymes and nutrient utilization by PERIOD proteins. Proc. Natl. Acad. Sci. U S A. 113, E1673–E1682. doi: 10.1073/pnas.1519650113
Nguyen, K. D., Fentress, S. J., Qiu, Y., Yun, K., Cox, J. S., and Chawla, A. (2013). Circadian gene bmal1 regulates diurnal oscillations of Ly6Chi inflammatory monocytes. Science 341, 1483–1488. doi: 10.1126/science.1240636
Ni, W., Liu, K., Hou, G., Pan, C., Wu, S., Zheng, J., et al. (2019). Diurnal variation in sperm DNA fragmentation: analysis of 11,382 semen samples from two populations and in vivo animal experiments. Chronobiol. Int. 36, 1455–1463. doi: 10.1080/07420528.2019.1649275
Niesler, B., Kuerten, S., Demir, I. E., and Schäfer, K.-H. (2021). Disorders of the enteric nervous system — a holistic view. Nat. Rev. Gastroenterol. Hepatol. 18, 393–410. doi: 10.1038/s41575-020-00385-382
Nisa, H., Qi, K. H. T., Leng, J., Zhou, T., Liu, H., Li, W., et al. (2018). The circadian Rhythm–Related MTNR1B genotype, gestational weight gain, and postpartum glycemic changes. J. Clin. Endocrinol. Metab. 103, 2284–2290. doi: 10.1210/jc.2018-2071
Nohara, K., Mallampalli, V., Nemkov, T., Wirianto, M., Yang, J., Ye, Y., et al. (2019). Nobiletin fortifies mitochondrial respiration in skeletal muscle to promote healthy aging against metabolic challenge. Nat. Commun. 10:3923. doi: 10.1038/s41467-019-11926-y
O’Neill, J. S., and Reddy, A. B. (2011). Circadian clocks in human red blood cells. Nature 469, 498–503. doi: 10.1038/nature09702
Ogo, F. M., Siervo, G. E. M. L., de Moraes, A. M. P., Machado, K. G., de, B., Scarton, S. R., et al. (2020). Extended light period in the maternal circadian cycle impairs the reproductive system of the rat male offspring. J. Dev. Orig. Health Dis. 12, 595–602. doi: 10.1017/S2040174420000975
Olcese, J., Lozier, S., and Paradise, C. (2013). Melatonin and the circadian timing of human parturition. Reprod. Sci. 20, 168–174. doi: 10.1177/1933719112442244
Oster, H., Challet, E., Ott, V., Arvat, E., de Kloet, E. R., Dijk, D. J., et al. (2017). The functional and clinical significance of the 24-hour rhythm of circulating glucocorticoids. Endocr. Rev. 38, 3–45. doi: 10.1210/er.2015-1080
Panda, S. (2016). Circadian physiology of metabolism. Science 354, 1008–1015. doi: 10.1126/science.aah4967
Panda, S. (2019). The arrival of circadian medicine. Nat. Rev. Endocrinol. 15, 67–69. doi: 10.1038/s41574-018-0142-x
Pappa, K. I., Gazouli, M., Anastasiou, E., Iliodromiti, Z., Antsaklis, A., and Anagnou, N. P. (2013a). Circadian clock gene expression is impaired in gestational diabetes mellitus. Gynecol. Endocrinol. 29, 331–335. doi: 10.3109/09513590.2012.743018
Pappa, K. I., Gazouli, M., Anastasiou, E., Iliodromiti, Z., Antsaklis, A., and Anagnou, N. P. (2013b). The major circadian pacemaker ARNT-like protein-1 (BMAL1) is associated with susceptibility to gestational diabetes mellitus. Diabetes Res. Clin. Pract. 99, 151–157. doi: 10.1016/j.diabres.2012.10.015
Paschos, G. K., Ibrahim, S., Song, W. L., Kunieda, T., Grant, G., Reyes, T. M., et al. (2012). Obesity in mice with adipocyte-specific deletion of clock component Arntl. Nat. Med. 18, 1768–1777. doi: 10.1038/nm.2979
Paul, J. R., Davis, J. A., Goode, L. K., Becker, B. K., Fusilier, A., Meador-Woodruff, A., et al. (2020). Circadian regulation of membrane physiology in neural oscillators throughout the brain. Eur. J. Neurosci. 51, 109–138. doi: 10.1111/ejn.14343
Peek, C. B., Levine, D. C., Cedernaes, J., Taguchi, A., Kobayashi, Y., Tsai, S. J., et al. (2017). Circadian clock interaction with HIF1α mediates oxygenic metabolism and anaerobic glycolysis in skeletal muscle. Cell Metab. 25, 86–92. doi: 10.1016/j.cmet.2016.09.010
Peng, K., Lin, L., Wang, Z., Ding, L., Huang, Y., Wang, P., et al. (2017). Short sleep duration and longer daytime napping are associated with non-alcoholic fatty liver disease in Chinese adults. J. Diab. 9, 827–836. doi: 10.1111/1753-0407.12489
Pérez, S., Murias, L., Fernández-Plaza, C., Díaz, I., González, C., Otero, J., et al. (2015). Evidence for clock genes circadian rhythms in human full-term placenta. Syst. Biol. Reprod. Med. 61, 360–366. doi: 10.3109/19396368.2015.1069420
Perrin, L., Loizides-Mangold, U., Chanon, S., Gobet, C., Hulo, N., Isenegger, L., et al. (2018). Transcriptomic analyses reveal rhythmic and CLOCK-driven pathways in human skeletal muscle. eLife 7:e34114. doi: 10.7554/eLife.34114
Peruquetti, R. L., de Mateo, S., and Sassone-Corsi, P. (2012). Circadian proteins CLOCK and BMAL1 in the chromatoid body, a RNA processing granule of male germ cells. PLoS One 7:e42695. doi: 10.1371/journal.pone.0042695
Pilorz, V., Steinlechner, S., and Oster, H. (2009). Age and oestrus cycle-related changes in glucocorticoid excretion and wheel-running activity in female mice carrying mutations in the circadian clock genes Per1 and Per2. Physiol. Behav. 96, 57–63. doi: 10.1016/j.physbeh.2008.08.010
Podobed, P., Glen Pyle, W., Ackloo, S., Alibhai, F. J., Tsimakouridze, E. V., Ratcliffe, W. F., et al. (2014). The day/night proteome in the murine heart. Am. J. Physiol. - Regul. Integr. Comp. Physiol. 307, 121–137. doi: 10.1152/ajpregu.00011.2014
Poggiogalle, E., Jamshed, H., and Peterson, C. M. (2018). Circadian regulation of glucose, lipid, and energy metabolism in humans. Metabolism 84, 11–27. doi: 10.1016/j.metabol.2017.11.017
Polanco, J. C., Li, C., Bodea, L. G., Martinez-Marmol, R., Meunier, F. A., and Götz, J. (2018). Amyloid-β and tau complexity - towards improved biomarkers and targeted therapies. Nat. Rev. Neurol. 14, 22–40. doi: 10.1038/nrneurol.2017.162
Pompeii, L. A., Savitz, D. A., Evenson, K. R., Rogers, B., and McMahon, M. (2005). Physical exertion at work and the risk of preterm delivery and small-for-gestational- age birth. Obstet. Gynecol. 106, 1279–1288. doi: 10.1097/01.AOG.0000189080.76998.f8
Prokopenko, I., Langenberg, C., Florez, J. C., Saxena, R., Soranzo, N., Thorleifsson, G., et al. (2009). Variants in MTNR1B influence fasting glucose levels. Nat. Genet. 41, 77–81. doi: 10.1038/ng.290
Qin, F., Zhang, J., Cao, H., Guo, W., Chen, L., Shen, O., et al. (2014). Circadian alterations of reproductive functional markers in male rats exposed to 1800 MHz radiofrequency field. Chronobiol. Int. 31, 123–133. doi: 10.3109/07420528.2013.830622
Ragle, J. M., Aita, A. L., Morrison, K. N., Martinez-Mendez, R., Saeger, H. N., Ashley, G. A., et al. (2020). The conserved molting/circadian rhythm regulator NHR-23/NR1F1 serves as an essential co-regulator of C. elegans spermatogenesis. Development 147:dev193862. doi: 10.1242/dev.193862
Rajaratnam, S. M., and Arendt, J. (2001). Health in a 24-h society. Lancet 358, 999–1005. doi: 10.1016/S0140-6736(01)06108-6106
Randler, C., Ebenhöh, N., Fischer, A., Höchel, S., Schroff, C., Stoll, J. C., et al. (2012). Chronotype but not sleep length is related to salivary testosterone in young adult men. Psychoneuroendocrinology 37, 1740–1744. doi: 10.1016/j.psyneuen.2012.02.008
Ratajczak, C. K., Herzog, E. D., and Muglia, L. J. (2010). Clock gene expression in gravid uterus and extra-embryonic tissues during late gestation in the mouse. Reprod. Fertil. Dev. 22, 743–750. doi: 10.1071/RD09243
Reinke, H., and Asher, G. (2019). Crosstalk between metabolism and circadian clocks. Nat. Rev. Mol. Cell Biol. 20, 227–241. doi: 10.1038/s41580-018-0096-99
Reiter, R. J., Tan, D. X., Korkmaz, A., and Rosales-Corral, S. A. (2014). Melatonin and stable circadian rhythms optimize maternal, placental and fetal physiology. Hum. Reprod. Update 20, 293–307. doi: 10.1093/humupd/dmt054
Robles, M. S., Humphrey, S. J., and Mann, M. (2017). Phosphorylation is a central mechanism for circadian control of metabolism and physiology. Cell Metab. 25, 118–127. doi: 10.1016/j.cmet.2016.10.004
Roccaro, I., and Smirni, D. (2020). Fiat lux: the light became therapy. an overview on the bright light therapy in Alzheimer’s disease sleep disorders. J. Alzheimer’s Dis. 77, 113–125. doi: 10.3233/JAD-200478
Roden, M., and Shulman, G. I. (2019). The integrative biology of type 2 diabetes. Nature 576, 51–60. doi: 10.1038/s41586-019-1797-1798
Ruan, W., Yuan, X., and Eltzschig, H. K. (2021). Circadian rhythm as a therapeutic target. Nat. Rev. Drug Discov. 20, 287–307. doi: 10.1038/s41573-020-00109-w
Ruben, M. D., Smith, D. F., FitzGerald, G. A., and Hogenesch, J. B. (2019). Dosing time matters. Science 365, 547–549. doi: 10.1126/science.aax7621
Ruben, M. D., Wu, G., Smith, D. F., Schmidt, R. E., Francey, L. J., Lee, Y. Y., et al. (2018). A database of tissue-specific rhythmically expressed human genes has potential applications in circadian medicine. Sci. Transl. Med. 10:eaat8806. doi: 10.1126/scitranslmed.aat8806
Rudic, R. D., McNamara, P., Reilly, D., Grosser, T., Curtis, A. M., Price, T. S., et al. (2005). Bioinformatic analysis of circadian gene oscillation in mouse aorta. Circulation 112, 2716–2724. doi: 10.1161/CIRCULATIONAHA.105.568626
Russell, A. L., Miller, L., Yi, H., Keil, R., Handa, R. J., and Wu, T. J. (2021). Knockout of the circadian gene. Per2, disrupts corticosterone secretion and results in depressive-like behaviors and deficits in startle responses. BMC Neurosci. 22:5. doi: 10.1186/s12868-020-00607-y
Ryzhikov, M., Ehlers, A., Steinberg, D., Xie, W., Oberlander, E., Brown, S., et al. (2019). Diurnal rhythms spatially and temporally organize autophagy. Cell Rep. 26, 1880–1892.e6. doi: 10.1016/j.celrep.2019.01.072
Saran, A. R., Dave, S., and Zarrinpar, A. (2020). Circadian rhythms in the pathogenesis and treatment of fatty liver disease. Gastroenterology 158, 1948–1966.e1. doi: 10.1053/j.gastro.2020.01.050
Sati, L. (2020). Chronodisruption: effects on reproduction, transgenerational health of offspring and epigenome. Reproduction 160, R79–R94. doi: 10.1530/REP-20-0298
Sato, S., Basse, A. L., Schönke, M., Chen, S., Samad, M., Altıntas̨, A., et al. (2019). Time of exercise specifies the impact on muscle metabolic pathways and systemic energy homeostasis. Cell Metab. 30, 92–110.e4. doi: 10.1016/j.cmet.2019.03.013
Scheiermann, C., Kunisaki, Y., Lucas, D., Chow, A., Jang, J. E., Zhang, D., et al. (2012). Adrenergic nerves govern circadian leukocyte recruitment to tissues. Immunity 37, 290–301. doi: 10.1016/j.immuni.2012.05.021
Schiaffino, S., Blaauw, B., and Dyar, K. A. (2016). The functional significance of the skeletal muscle clock: lessons from Bmal1 knockout models. Skelet. Muscle 6, 1–9. doi: 10.1186/s13395-016-0107-105
Schilperoort, M., van den Berg, R., Bosmans, L. A., van Os, B. W., Dollé, M. E. T., Smits, N. A. M., et al. (2020). Disruption of circadian rhythm by alternating light-dark cycles aggravates atherosclerosis development in APOE∗3-Leiden.CETP mice. J. Pineal Res. 68:e12614. doi: 10.1111/jpi.12614
Schmitt, K., Grimm, A., Dallmann, R., Oettinghaus, B., Restelli, L. M., Witzig, M., et al. (2018). Circadian control of DRP1 activity regulates mitochondrial dynamics and bioenergetics. Cell Metab. 27, 657–666.e5. doi: 10.1016/j.cmet.2018.01.011
Schmutz, I., Ripperger, J. A., Baeriswyl-Aebischer, S., and Albrecht, U. (2010). The mammalian clock component PERIOD2 coordinates circadian output by interaction with nuclear receptors. Genes Dev. 24, 345–357. doi: 10.1101/gad.564110
Schoeller, E. L., Clark, D. D., Dey, S., Cao, N. V., Semaan, S. J., Chao, L. W., et al. (2016). Bmal1 is required for normal reproductive behaviors in male mice. Endocrinology 157, 4914–4929. doi: 10.1210/en.2016-1620
Schroder, E. A., Harfmann, B. D., Zhang, X., Srikuea, R., England, J. H., Hodge, B. A., et al. (2015). Intrinsic muscle clock is necessary for musculoskeletal health. J. Physiol. 593, 5387–5404. doi: 10.1113/JP271436
Schroder, E. A., Lefta, M., Zhang, X., Bartos, D. C., Feng, H.-Z., Zhao, Y., et al. (2013). The cardiomyocyte molecular clock, regulation of Scn5a, and arrhythmia susceptibility. Am. J. Physiol. Physiol. 304, C954–C965. doi: 10.1152/ajpcell.00383.2012
Sedej, S. (2018). Ketone bodies to the rescue for an aging heart? Cardiovasc. Res. 114, e1–e2. doi: 10.1093/cvr/cvx218
Sen, A., and Hoffmann, H. M. (2020). Role of core circadian clock genes in hormone release and target tissue sensitivity in the reproductive axis. Mol. Cell. Endocrinol. 501:110655. doi: 10.1016/j.mce.2019.110655
Shami, A. N., Zheng, X., Munyoki, S. K., Ma, Q., Manske, G. L., Green, C. D., et al. (2020). Single-Cell RNA sequencing of human, macaque, and mouse testes uncovers conserved and divergent features of mammalian spermatogenesis. Dev. Cell 54, 529–547.e12. doi: 10.1016/j.devcel.2020.05.010
Shan, Y., Abel, J. H., Li, Y., Izumo, M., Cox, K. H., Jeong, B., et al. (2020). Dual-Color single-cell imaging of the suprachiasmatic nucleus reveals a circadian role in network synchrony. Neuron 108, 164–179.e7. doi: 10.1016/j.neuron.2020.07.012
Shen, O., Ding, X., Nie, J., Xia, Y., Wang, X., Tong, J., et al. (2015). Variants of the CLOCK gene affect the risk of idiopathic male infertility in the Han-Chinese population. Chronobiol. Int. 32, 959–965. doi: 10.3109/07420528.2015.1056305
Shi, L., Li, N., Bo, L., and Xu, Z. (2013). Melatonin and hypothalamic-pituitary-gonadal axis. Curr. Med. Chem. 20, 2017–2031. doi: 10.2174/09298673113209990114
Shi, S. Q., Ansari, T. S., McGuinness, O. P., Wasserman, D. H., and Johnson, C. H. (2013). Circadian disruption leads to insulin resistance and obesity. Curr. Biol. 23, 372–381. doi: 10.1016/j.cub.2013.01.048
Shimada, M., Seki, H., Samejima, M., Hayase, M., and Shirai, F. (2016). Salivary melatonin levels and sleep-wake rhythms in pregnant women with hypertensive and glucose metabolic disorders: a prospective analysis. Biosci. Trends 10, 34–41. doi: 10.5582/bst.2015.01123
Stein, R. M., Kang, H. J., McCorvy, J. D., Glatfelter, G. C., Jones, A. J., Che, T., et al. (2020). Virtual discovery of melatonin receptor ligands to modulate circadian rhythms. Nature 579, 609–614. doi: 10.1038/s41586-020-2027-2020
Storch, K. F., Lipan, O., Leykin, I., Viswanathan, N., Davis, F. C., Wong, W. H., et al. (2002). Extensive and divergent circadian gene expression in liver and heart. Nature 417, 78–83. doi: 10.1038/nature744
Stujanna, E. N., Murakoshi, N., Tajiri, K., Xu, D., Kimura, T., Qin, R., et al. (2017). Rev-erb agonist improves adverse cardiac remodeling and survival in myocardial infarction through an anti-inflammatory mechanism. PLoS One 12:e0189330. doi: 10.1371/journal.pone.0189330
Swirski, F. K., and Nahrendorf, M. (2013). Leukocyte behavior in atherosclerosis, myocardial infarction, and heart failure. Science 339, 161–166. doi: 10.1126/science.1230719
Takahashi, J. S. (2017). Transcriptional architecture of the mammalian circadian clock. Nat. Rev. Genet. 18, 164–179. doi: 10.1038/nrg.2016.150
Tan, D.-X. X., Manchester, L. C., Terron, M. P., Flores, L. J., and Reiter, R. J. (2007). One molecule, many derivatives: a never-ending interaction of melatonin with reactive oxygen and nitrogen species? J. Pineal Res. 42, 28–42. doi: 10.1111/j.1600-079X.2006.00407.x
Thompson, M. E., Fox, S. A., Berghänel, A., Sabbi, K. H., Phillips-Garcia, S., Enigk, D. K., et al. (2020). Wild chimpanzees exhibit humanlike aging of glucocorticoid regulation. Proc. Natl. Acad. Sci. U S A. 117, 8424–8430. doi: 10.1073/pnas.1920593117
Toledo, M., Batista-Gonzalez, A., Merheb, E., Aoun, M. L., Tarabra, E., Feng, D., et al. (2018). Autophagy regulates the liver clock and glucose metabolism by degrading CRY1. Cell Metab. 28, 268–281.e4. doi: 10.1016/j.cmet.2018.05.023
Torres, M., Laguna-Barraza, R., Dalmases, M., Calle, A., Pericuesta, E., Montserrat, J. M., et al. (2014). Male fertility is reduced by chronic intermittent hypoxia mimicking sleep apnea in mice. Sleep 37, 1757–1765. doi: 10.5665/sleep.4166
Tousson, E., and Meissl, H. (2004). Suprachiasmatic nuclei grafts restore the circadian rhythm in the paraventricular nucleus of the hypothalamus. J. Neurosci. 24, 2983–2988. doi: 10.1523/JNEUROSCI.5044-03.2004
Tuomi, T., Nagorny, C. L. F., Singh, P., Bennet, H., Yu, Q., Alenkvist, I., et al. (2016). Increased melatonin signaling is a risk factor for type 2 diabetes. Cell Metab. 23, 1067–1077. doi: 10.1016/j.cmet.2016.04.009
Turek, F. W., Joshu, C., Kohsaka, A., Lin, E., Ivanova, G., McDearmon, E., et al. (2005). Obesity and metabolic syndrome in circadian clock mutant mice. Science 308, 1043–1045. doi: 10.1126/science.1108750
Umemura, Y., and Yagita, K. (2020). Development of the circadian core machinery in mammals. J. Mol. Biol. 432, 3611–3617. doi: 10.1016/j.jmb.2019.11.026
Urlep, Z., and Rozman, D. (2013). The interplay between circadian system, cholesterol synthesis, and steroidogenesis affects various aspects of female reproduction. Front. Endocrinol. (Lausanne) 4:111. doi: 10.3389/fendo.2013.00111
Valenzuela, F. J., Vera, J., Venegas, C., Pino, F., and Lagunas, C. (2015). Circadian system and melatonin hormone: risk factors for complications during pregnancy. Obstet. Gynecol. Int. 2015:825802. doi: 10.1155/2015/825802
van Melick, M. J. G. J., van Beukering, M. D. M., Mol, B. W., Frings-Dresen, M. H. W., and Hulshof, C. T. J. (2014). Shift work, long working hours and preterm birth: a systematic review and meta-analysis. Int. Arch. Occup. Environ. Health. 87, 835–849. doi: 10.1007/s00420-014-0934-939
Vestweber, D. (2015). How leukocytes cross the vascular endothelium. Nat. Rev. Immunol. 15, 692–704. doi: 10.1038/nri3908
Vetter, C., Devore, E. E., Wegrzyn, L. R., Massa, J., Speizer, F. E., Kawachi, I., et al. (2016). Association between rotating night shiftwork and risk of coronary heart disease among women. JAMA - J. Am. Med. Assoc. 315, 1726–1734. doi: 10.1001/jama.2016.4454
Vitale, J. A., Bonato, M., La Torre, A., and Banfi, G. (2019). The role of the molecular clock in promoting skeletal muscle growth and protecting against sarcopenia. Int. J. Mol. Sci. 20:4318. doi: 10.3390/ijms20174318
Vrang, N., Larsen, P. J., and Mikkelsen, J. D. (1995). Direct projection from the suprachiasmatic nucleus to hypophysiotrophic corticotropin-releasing factor immunoreactive cells in the paraventricular nucleus of the hypothalamus demonstrated by means ofPhaseolus vulgaris-leucoagglutinin tract tracing. Brain Res. 684, 61–69. doi: 10.1016/0006-8993(95)00425-P
Wang, H., Gu, Y., Zheng, L., Liu, L., Meng, G., Wu, H., et al. (2018). Association between bedtime and the prevalence of newly diagnosed non-alcoholic fatty liver disease in adults. Liver Int. 38, 2277–2286. doi: 10.1111/liv.13896
Wang, Y., Song, L., Liu, M., Ge, R., Zhou, Q., Liu, W., et al. (2018). A proteomics landscape of circadian clock in mouse liver. Nat. Commun. 9:1553. doi: 10.1038/s41467-018-03898-3892
Welsh, D. K., Takahashi, J. S., and Kay, S. A. (2009). Suprachiasmatic nucleus: cell autonomy and network properties. Annu. Rev. Physiol. 72, 551–577. doi: 10.1146/annurev-physiol-021909-135919
Wharfe, M. D., Mark, P. J., and Waddell, B. J. (2011). Circadian variation in placental and hepatic clock genes in rat pregnancy. Endocrinology 152, 3552–3560. doi: 10.1210/en.2011-2081
Whelan, E. A., Lawson, C. C., Grajewski, B., Hibert, E. N., Spiegelman, D., and Rich-Edwards, J. W. (2007). Work schedule during pregnancy and spontaneous abortion. Epidemiology 18, 350–355. doi: 10.1097/01.ede.0000259988.77314.a4
Winter, C., Silvestre-Roig, C., Ortega-Gomez, A., Lemnitzer, P., Poelman, H., Schumski, A., et al. (2018). Chrono-pharmacological targeting of the CCL2-CCR2 axis ameliorates atherosclerosis. Cell Metab. 28, 175–182.e5. doi: 10.1016/j.cmet.2018.05.002
Woldt, E., Sebti, Y., Solt, L. A., Duhem, C., Lancel, S., Eeckhoute, J., et al. (2013). Rev-erb-α modulates skeletal muscle oxidative capacity by regulating mitochondrial biogenesis and autophagy. Nat. Med. 19, 1039–1046. doi: 10.1038/nm.3213
Wolf, D., and Ley, K. (2019). Immunity and inflammation in atherosclerosis. Circ. Res. 124, 315–327. doi: 10.1161/CIRCRESAHA.118.313591
Wu, R., Dang, F., Li, P., Wang, P., Xu, Q., Liu, Z., et al. (2019). The circadian protein Period2 suppresses mTORC1 activity via recruiting Tsc1 to mTORC1 complex. Cell Metab. 29, 653–667.e6. doi: 10.1016/j.cmet.2018.11.006
Wu, Y., Tang, D., Liu, N., Xiong, W., Huang, H., Li, Y., et al. (2017). Reciprocal regulation between the circadian clock and hypoxia signaling at the genome level in mammals. Cell Metab. 25, 73–85. doi: 10.1016/j.cmet.2016.09.009
Xie, M., Tang, Q., Nie, J., Zhang, C., Zhou, X., Yu, S., et al. (2020). BMAL1-Downregulation aggravates porphyromonas gingivalis -induced atherosclerosis by encouraging oxidative stress. Circ. Res. 126, E15–E29. doi: 10.1161/CIRCRESAHA.119.315502
Xie, M., Utzinger, K. S., Blickenstorfer, K., and Leeners, B. (2018). Diurnal and seasonal changes in semen quality of men in subfertile partnerships. Chronobiol. Int. 35, 1375–1384. doi: 10.1080/07420528.2018.1483942
Xin, H., Deng, F., Zhou, M., Huang, R., Ma, X., Tian, H., et al. (2021). A multi-tissue multi-omics analysis reveals distinct kinetics in entrainment of diurnal transcriptomes by inverted feeding. iScience 24:102335. doi: 10.1016/j.isci.2021.102335
Xu, H., Li, H., Woo, S. L., Kim, S. M., Shende, V. R., Neuendorff, N., et al. (2014). Myeloid cell-specific disruption of Period1 and period2 exacerbates diet-induced inflammation and insulin resistance. J. Biol. Chem. 289, 16374–16388. doi: 10.1074/jbc.M113.539601
Xu, X., Ding, M., Li, B., and Christiani, D. C. (1994). Association of rotating shiftwork with preterm births and low birth weight among never smoking women textile workers in China. Occup. Environ. Med. 51, 470–474. doi: 10.1136/oem.51.7.470
Yamanaka, Y., Suzuki, Y., Todo, T., Honma, K. I., and Honma, S. (2010). Loss of circadian rhythm and light-induced suppression of pineal melatonin levels in Cry1 and Cry2 double-deficient mice. Genes Cells 15, 1063–1071. doi: 10.1111/j.1365-2443.2010.01443.x
Yang, G., Chen, L., Grant, G. R., Paschos, G., Song, W.-L., Musiek, E. S., et al. (2016). Timing of expression of the core clock gene Bmal1 influences its effects on aging and survival. Sci. Transl. Med. 8:324ra16. doi: 10.1126/scitranslmed.aad3305
Yang, S., Liu, A., Weidenhammer, A., Cooksey, R. C., McClain, D., Kim, M. K., et al. (2009). The role of mPer2 clock gene in glucocorticoid and feeding rhythms. Endocrinology 150, 2153–2160. doi: 10.1210/en.2008-2705
Yang, X., Downes, M., Yu, R. T., Bookout, A. L., He, W., Straume, M., et al. (2006). Nuclear receptor expression links the circadian clock to metabolism. Cell 126, 801–810. doi: 10.1016/j.cell.2006.06.050
Young, M. E., Razeghi, P., and Taegtmeyer, H. (2001). Clock genes in the heart: characterization and attenuation with hypertrophy. Circ. Res. 88, 1142–1150. doi: 10.1161/hh1101.091190
Young, M. E., Wilson, C. R., Razeghi, P., Guthrie, P. H., and Taegtmeyer, H. (2002). Alterations of the circadian clock in the heart by streptozotocin-induced diabetes. J. Mol. Cell. Cardiol. 34, 223–231. doi: 10.1006/jmcc.2001.1504
Zambon, A. C., McDearmon, E. L., Salomonis, N., Vranizan, K. M., Johansen, K. L., Adey, D., et al. (2003). Time- and exercise-dependent gene regulation in human skeletal muscle. Genome Biol. 4:R61. doi: 10.1186/gb-2003-4-10-r61
Zhang, E. E., Liu, Y., Dentin, R., Pongsawakul, P. Y., Liu, A. C., Hirota, T., et al. (2010). Cryptochrome mediates circadian regulation of cAMP signaling and hepatic gluconeogenesis. Nat. Med. 16, 1152–1156. doi: 10.1038/nm.2214
Zhang, J., Ding, X., Li, Y., Xia, Y., Nie, J., Yi, C., et al. (2012a). Association of CLOCK gene variants with semen quality in idiopathic infertile Han-Chinese males. Reprod. Biomed. Online 25, 536–542. doi: 10.1016/j.rbmo.2012.07.018
Zhang, J., Li, H., Teng, H., Zhang, T., Luo, Y., Zhao, M., et al. (2012b). Regulation of peripheral clock to oscillation of substance p contributes to circadian inflammatory pain. Anesthesiology 117, 149–160. doi: 10.1097/ALN.0b013e31825b4fc1
Zhang, L., Prosdocimo, D. A., Bai, X., Fu, C., Zhang, R., Campbell, F., et al. (2015). KLF15 establishes the landscape of diurnal expression in the heart. Cell Rep. 13, 2368–2375. doi: 10.1016/j.celrep.2015.11.038
Zhang, L., Zhang, R., Tien, C.-L., Chan, R. E., Sugi, K., Fu, C., et al. (2017). REV-ERBα ameliorates heart failure through transcription repression. JCI Insight 2:e95177. doi: 10.1172/jci.insight.95177
Zhang, R., Lahens, N. F., Ballance, H. I., Hughes, M. E., and Hogenesch, J. B. (2014). A circadian gene expression atlas in mammals: implications for biology and medicine. Proc. Natl. Acad. Sci. U S A. 111, 16219–16224. doi: 10.1073/pnas.1408886111
Zhang, Z., Cajochen, C., and Khatami, R. (2019). Social jetlag and chronotypes in the chinese population: analysis of data recorded by wearable devices. J. Med. Internet Res. 21:e13482. doi: 10.2196/13482
Zhang, J., Chatham, J. C., and Young, M. E. (2020a). Circadian regulation of cardiac physiology: rhythms that keep the heart beating. Annu. Rev. Physiol. 82, 79–101. doi: 10.1146/annurev-physiol-020518-114349
Zhang, Z., Xin, H., and Li, M.-D. (2020b). Circadian rhythm of lipid metabolism in health and disease. Small Methods 4:1900601. doi: 10.1002/smtd.201900601
Keywords: circadian clock, complex disease, checkpoint, Nr1d1/Rev-erbα, melatonin, circadian rhythm, diabetes complications, systems biology
Citation: Li M-D, Xin H, Yuan Y, Yang X, Li H, Tian D, Zhang H, Zhang Z, Han T-L, Chen Q, Duan G, Ju D, Chen K, Deng F, He W and Biological Rhythm Academic Consortium in Chongqing (2021) (2021) Circadian Clock-Controlled Checkpoints in the Pathogenesis of Complex Disease. Front. Genet. 12:721231. doi: 10.3389/fgene.2021.721231
Received: 06 June 2021; Accepted: 16 August 2021;
Published: 07 September 2021.
Edited by:
Guang-Zhong Wang, Shanghai Institute of Nutrition and Health, Shanghai Institutes for Biological Sciences (CAS), ChinaReviewed by:
Qingchun Tong, University of Texas Health Science Center at Houston, United StatesLuoying Zhang, Huazhong University of Science and Technology, China
Copyright © 2021 Li, Xin, Yuan, Yang, Li, Tian, Zhang, Zhang, Han, Chen, Duan, Ju, Chen, Deng, He and Biological Rhythm Academic Consortium in Chongqing (BRACQ). This is an open-access article distributed under the terms of the Creative Commons Attribution License (CC BY). The use, distribution or reproduction in other forums is permitted, provided the original author(s) and the copyright owner(s) are credited and that the original publication in this journal is cited, in accordance with accepted academic practice. No use, distribution or reproduction is permitted which does not comply with these terms.
*Correspondence: Min-Dian Li, bWluZGlhbmxpQHRtbXUuZWR1LmNu; Zhihui Zhang, eHl6cGpAdG1tdS5lZHUuY24=; Ting-Li Han, dGluZ2xpaGFuQGNxbXUuZWR1LmNu; Qing Chen, Y2hlbnFpbmdmb3J3YXJkQGdtYWlsLmNvbQ==; Guangyou Duan, ZGd5MTk4NmFuZXN0aGVzaWFAMTI2LmNvbQ==; Dapeng Ju, anVkYXBlbmcxOTg1MTAwOEBnbWFpbC5jb20=; Ka Chen, Y2hlbmthQHRtbXUuZWR1LmNu; Fang Deng, Y2VsbGRmQHRtbXUuZWR1LmNu; Wenyan He, d2VueWFuX2hlQGNxbXUuZWR1LmNu
†These authors share first authorship