- 1Department of Cell Biology and Genetics, Biosciences Center, Federal University of Rio Grande do Norte, Natal, Brazil
- 2Department of Genetics and Evolution, Federal University of São Carlos, São Carlos, Brazil
- 3Department of Fundamental Science, Faculty of Science and Technology, Surindra Rajabhat University, Muang, Thailand
- 4Applied Science Program, Faculty of Interdisciplinary Studies, Khon Kaen University, Nong Khai Campus, Nong Khai, Thailand
- 5Program of Biology, Faculty of Science, Khon Kaen University, Khon Kaen, Thailand
Fishes of the genus Acanthurus (Acanthuridae) are strongly related to reef environments, in a broad biogeographic context worldwide. Although their biological aspects are well known, cytogenetic information related to this genus remains incipient. In this study, Acanthurus species from populations inhabiting coastal regions of the Southwest Atlantic (SWA), South Atlantic oceanic islands (Fernando de Noronha Archipelago and Trindade Island), Greater Caribbean (GC), and Indo-Pacific Ocean (the center of the origin of the group) were analyzed to investigate their evolutionary differentiation. For this purpose, we employed conventional cytogenetic procedures and fluorescence in situ hybridization of 18S rDNA, 5S rDNA, and H3 and H2B-H2A histone sequences. The Atlantic species (A. coeruleus, A. chirurgus, and A. bahianus) did not show variations among them, despite their vast continental and insular distribution. In contrast, A. coeruleus from SWA and GC diverged from each other in the number of 18S rDNA sites, a condition likely associated with the barrier created by the outflows of the Amazonas/Orinoco rivers. The geminate species A. tractus had a cytogenetic profile similar to that of A. bahianus. However, the chromosomal macrostructures and the distribution of rDNA and hisDNA sequences revealed moderate to higher rates of diversification when Acanthurus species from recently colonized areas (Atlantic Ocean) were compared to A. triostegus, a representative species from the Indian Ocean. Our cytogenetic data covered all Acanthurus species from the Western Atlantic, tracked phylogenetic diversification throughout the dispersive process of the genus, and highlighted the probable diversifying role of ocean barriers in this process.
Introduction
Acanthuridae (surgeonfishes, tangs, and unicornfishes) represents a charismatic group of primarily large-bodied herbivorous fish species, which play an important ecological role in benthic communities and the resilience of coral environments in all tropical and subtropical seas (Randall, 2001; Green and Bellwood, 2009; Russ et al., 2018). The common name “surgeonfish” refers to the peculiar scalpel-like modified scales on both sides of the caudal peduncle that is used in inter- and intraspecific aggressive interactions (Randall, 2001). Due to their attractive colors and shapes, surgeonfishes dominate aquarium trade in several areas (Sadovy and Vincent, 2002; Papavlasopoulou et al., 2014).
The family comprises six genera and 85 species (Fricke et al., 2021). Acanthurus is the most diverse clade with 40 species, of which 90% are endemic to the Indo-Pacific, the origin and dispersion center of the group (Randall, 2001). Despite the growing set of genetic data on Acanthuridae, spanning the nuclear (Bernardi et al., 2018) and the mitochondrial (Ludt et al., 2020) genomes, the cytogenetic features of Acanthuridae are still largely incipient (<10% of species) (Arai and Inoue, 1976; Affonso et al., 2014; Fernandes et al., 2015).
Four species of Acanthurus: A. coeruleus (blue tang surgeonfish), A. bahianus (barber surgeonfish), A. chirurgus (doctorfish), and A. tractus (ocean surgeonfish), are found in the Western Atlantic, with an extensive distribution from the Caribbean to southern Brazil, including the island regions of Fernando de Noronha and Trindade and Martim Vaz (Rocha et al., 2002). In addition, the wide distribution of Acanthurus around the world offers a suitable model for investigating chromosomal specificities fixed among populations in the marine environment. Several species of Acanthurus are found in the Pacific and Indo-Pacific oceans, the center of origin of the genus, among which A. triostegus (convict surgeonfish) is one most representative species. Acanthurus triostegus has a remarkable ability to extend its larval stage by slowing metamorphosis (McCormick, 1999), with populations between the Indian and Pacific oceans exhibiting genetic structure (Grulois et al., 2020). Currently, the karyotype of this species is only known in representatives of the Pacific regions (Arai and Inoue, 1976; Ojima and Yamamoto, 1990); comparisons with samples from the Indian Ocean will be useful for estimating evolutionary divergences across the Indo-Pacific region. Concerning the Western Atlantic region, the freshwater outflows from the Amazonas (Brazil) and Orinoco (Venezuela) rivers delimit the Brazilian and the Greater Caribbean (GC) biogeographic provinces (Floeter et al., 2008). Although this barrier interferes with the genetic structure of some Acanthurus species (Rocha et al., 2002; Rocha 2003), it is not yet known whether the barrier contributes to karyotype differentiation between divided populations.
Due to the high evolutionary dynamic of multigene families, such as the ribosomal DNA genes (Gornung, 2013), with a vital role in protein synthesis, and of histones (H1, H2B-H2A, H3, and H4), acting in the structural organization of chromatin and regulation of gene expression in eukaryotes (Chioda et al., 2002), they have been largely used in evolutionary and population approaches (e.g., Motta-Neto et al., 2012; Amorim et al., 2017). The preferential participation of concerted evolution in the ribosomal DNA (Gonzalez and Sylvester, 2001) or by birth-and-death evolution in histone genes (Rooney and Ward, 2005) promotes differential arrays that can be followed in the genome of the species and populations (Lima-Filho et al., 2012; Costa et al., 2016).
Chromosomal variations result in the amplification of adaptive aspects of species (Kess et al., 2020). Additionally, micro or macrostructural cytogenetic patterns can be correlated with the species distribution, and action of biogeographic barriers (Motta-Neto et al., 2019), and thus associated to the diversification of Acanthuridae in the marine environment. Although mapping of repetitive DNA sequences has produced effective cytogenetic markers for detecting cryptic evolutionary diversification among Atlantic reef fishes (Costa et al., 2014; Amorim et al., 2016; Nirchio et al., 2017), little is known about the chromosomal organization in Acanthuridae. In this study, the chromosomal distribution of rDNA and histone genes among five species of Acanthurus from the Southwest Atlantic (SWA), Greater Caribbean (GC), and Indian Ocean (IO) were utilized to track their karyotype evolution and assess population stratifications. The results reveal a panel of increasing karyotype diversification associated with the historical biogeography of Acanthurus and present evidence of intraspecific variability between Atlantic areas.
Materials and Methods
Sampling and Chromosome Preparation
Individuals of A. coeruleus, A. bahianus, A. tractus, and A. chirurgus were collected from different regions of the Western Atlantic, Brazilian Northeast coast (Rio Grande do Norte State), insular Atlantic regions (Fernando de Noronha archipelago and Trindade Island), and Florida Keys, an island archipelago in southern Florida (United States), belonging to GC province. Acanthurus triostegus individuals were obtained from the Andaman Sea in the Indian Ocean (Table 1).
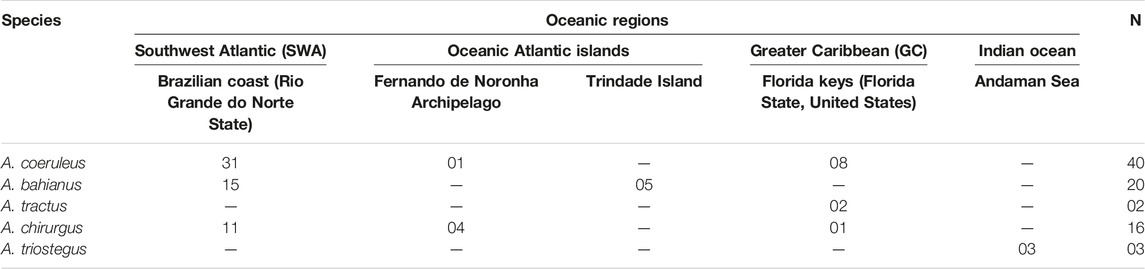
TABLE 1. Data of the species and populations of Acanthurus (Acanthuridae) used in cytogenetic analyses.
All field and laboratory protocols used in this study, including specimen sampling, were approved by the Ethics Committee on the Use of Animals at the Federal University of Rio Grande do Norte (Proc.#044-15). Sample collections were authorized by the Chico Mendes Institute for Biodiversity Conservation (ICMBio), System of Authorization, and Information on Biodiversity (SISBIO-Licenses No. 19135-1, 131360-1 and 27,027-2).
The specimens were subjected to in vivo mitotic stimulation for 24 h using an attenuated antigen complex (Molina et al., 2010). The chromosome preparations were performed in short-term culture according to Gold et al. (1990). Silver-stained nucleolus organizer regions (Ag-NORs) and heterochromatic regions were visualized following the protocols described by Howell and Black (1980) and Sumner (1972), respectively.
Barcoding of Cryptic Species
Because of the cryptic identification of A. bahianus and the newly resurrected A. tractus species (Bernal and Rocha, 2011) and their sympatric occurrence in GC (Castellanos-Gell et al., 2012), genetic analyses using sequences of the cytochrome oxidase I (COI) were performed to confirm their taxonomic status. For this purpose, fragments of fins were removed, preserved in 95% ethanol, and stored at 4 °C. The total DNA of each specimen was extracted (Sambrook et al., 1989) and amplified by polymerase chain reaction (PCR) using primers for the COI gene. The PCR reactions consisted of 1 μL of total DNA, 0.5 U Taq polymerase, 0.4 μL of 50 mM MgCl2, 1 μL of 10 x buffer, 0.5 μL 10 mM dNTP, 0.3 μL of each primer (10 μM) (L1987 and H2609) (Palumbi, 1991), and ultrapure water until the final volume of 25 μL. The amplification reactions were performed with an initial denaturation cycle at 95°C for 5 min; followed by 30 cycles at 94°C for 30 s, 49°C for 30 s, 72°C for 55 s, and a final extension at 72°C for 5 min. The PCR products were purified using the enzyme ExoSAP-IT (Applied Biosystems, Waltham, Massachusetts, EUA) and sequenced by ACTGene Ltd. The COI gene sequences of the individuals were compared to GenBank (www.ncbi.nlm.nih.gov/genbank/) and BOLD (www.boldsystems.org) databases employing BOLD identification tools and Blastn Search Tool, respectively, with those of A. tractus, confirming its taxonomic status.
Probes Preparation
The 5S rDNA (200 bp) and 18S rDNA (1,400 bp) probes were obtained by PCR from the nuclear DNA of A. coeruleus individuals from Northeast Brazilian coast using primers A 5′-TAC GCC CGA TCT CGT CCG ATC-3′ and B 5′ -CAG GCT GGT ATG GCC GTA AGC-3' (Pendás et al., 1994) and NS1 5′-GTA GTC ATA TGC TTG TCT C-3′ and NS8 5′-TCC GCA GGT TCA CCT ACG GA-3′ (White et al., 1990), respectively. The 5S rDNA and 18S rDNA probes were labeled with biotin-14-dATP and digoxigenin-11-dUTP, respectively, using nick translation according to the manufacturer’s recommendations (Roche, Mannheim, Germany). Meanwhile, primers H2BAD 5′-CCC -CCC GAG ATG TGA TGG TAG A-3 ′ and H2BAR 5′-AGT ACA GCC TGG ATG TTT GGT AA-3′ were used to amplify the H2B-H2A sequences and primers H3D 5′-ATG GCT CGT ACC AAG CAG ACV GC-3′ and H3R 5′-ATA TCC TTR GGC ATR ATR GTG AC-3′ to amplify H3 sequences. Both primer sets were designed using the gene sequences of Mytilus edulis (Albig et al., 2003), and the genes were amplified according to Giribert and Distel (2003). Biotin-14-dATP and digoxigenin-11-dUTP were used to label H2B-H2A hisDNA and H3 hisDNA, respectively, using nick translation according to the manufacturer’s recommendations (Roche, Mannheim, Germany).
Fluorescence in situ Hybridization (FISH)
FISH experiments were performed according to the protocols described by Pinkel et al. (1986). Mitotic chromosomes were treated with RNAse (20 μg/ml in 2 x SSC) at 37°C for 1 h and then with pepsin (0.005% in 10 mM HCl) at 37°C for 10 min, fixed with 1% formaldehyde for 10 min, and dehydrated using a series of alcohol solutions (70,85, and 100%) for 5 min. The chromosomal preparations were incubated in 70% formamide/2 × SSC at 72°C for 5 min. The hybridization solution ((50% formamide, 2 x SSC, and 10% dextran sulfate) and the denatured probe (5 ng/μL), with a final volume of 30 μL, were deposited on the slides, and hybridization was performed for 16 h at 37°C. Post-hybridization washes were performed using 15% formamide/0.2 × SSC at 42°C for 20 min, followed by washes using 0.1 × SSC at 60°C for 15 min and 0.5%/4 × SSC Tween 20 for 5 min at 25°C. Hybridization signals were detected using rhodamine-conjugated anti-digoxigenin for 18S rDNA and H3 hisDNA probes and FITC-conjugated streptavidin (Vector, Burlingame, CA, United States) for 5S rDNA and H2B-H2A hisDNA. Chromosomes were counterstained with Vectashield antifade with DAPI (4′,6-diamidine-2-phenylindole dihydrochloride) (1.5 μg/ml; Vector Laboratories Burlingame, CA, United States).
Microscopy and Image Analyses
Chromosomal images were obtained using an Olympus BX51 epifluorescence photomicroscope (Olympus, Tokyo, Japan) coupled to an Olympus DP73 digital capture system using the cellSens software (Olympus, Tokyo, Japan). Chromosomes were classified as metacentric (m), submetacentric (sm), subtelocentric (st), and acrocentric 1) according to their arms ratio (Levan et al., 1964). The fundamental number (FN) was established considering the occurrence of two arms on the meta-, submeta-, and subtelocentric chromosomes, and only one on the acrocentric chromosomes. Karyotypes were organized according to the decreasing order of size of the chromosomes within each of their respective morphological classes. Ideograms representing chromosomes and repetitive DNA class arrays were prepared using the Photoshop CS6 software.
Results
Acanthurus triostegus from the Andaman Sea, analyzed for the first time has 2n = 48 acrocentric chromosomes (FN = 48), with Ag-NOR sites located in the short arm of pair 24. Meanwhile, A. coeruleus (2n = 48; 2 s + 4th + 42a; FN = 54), A. tractus (2n = 36; 12 m + 2 s + 4th + 18a; FN = 54), A. bahianus (2n = 36; 12 m + 2 s + 4th + 18a; FN = 54), and A. chirurgus (2n = 34; 12 m + 2 s + 4th + 16a; FN = 52) presented karyotype patterns similar to those previously reported (Affonso et al., 2014; Fernandes et al., 2015) (Figure 1). Different populations of A. coeruleus, A. chirurgus, and A. bahianus (Table 1) displayed similar karyotype structures when compared to each other.
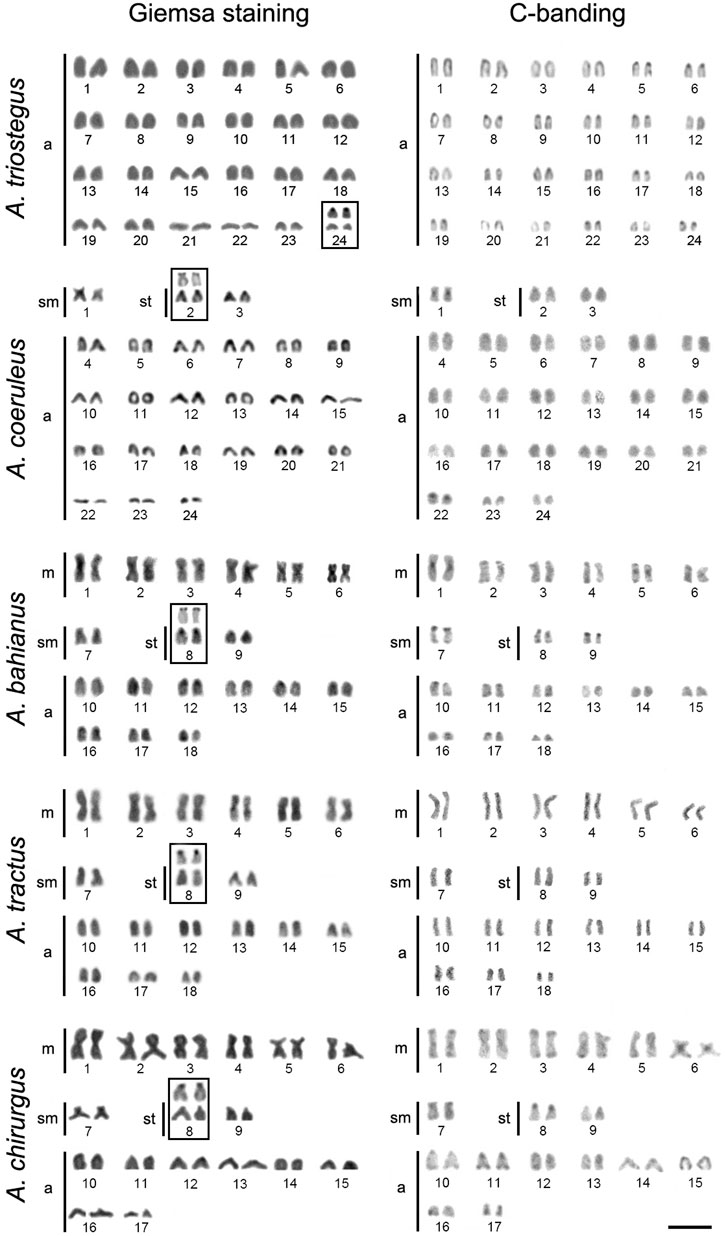
FIGURE 1. Karyotypes of Acanthurus triostegus, Acanthurus coeruleus, Acanthurus tractus, Acanthurus bahianus, and Acanthurus chirurgus after Giemsa-staining and C-banding. Boxes highlight the chromosome pair with Ag-NORs (silver stained NORs). m–metacentric, sm–submetacentric, and a–acrocentric chromosomes. Scale bar = 5 μm.
The 18S rDNA sites occur exclusively in the short arm of pair 24 in A. triostegus, meanwhile, the sites are located in the short arms of the largest subtelocentric pair (pair 8), without inter-population variability, in A. tractus, A. bahianus, and A. chirurgus. In contrast, the number of 18S rDNA sites in A. coeruleus differs between individuals from the Brazilian coast (two loci in the short arms of pairs 2 and 13) –and from the Florida Keys (only one locus in the short arms of pair 2) (Figures 2, 3).
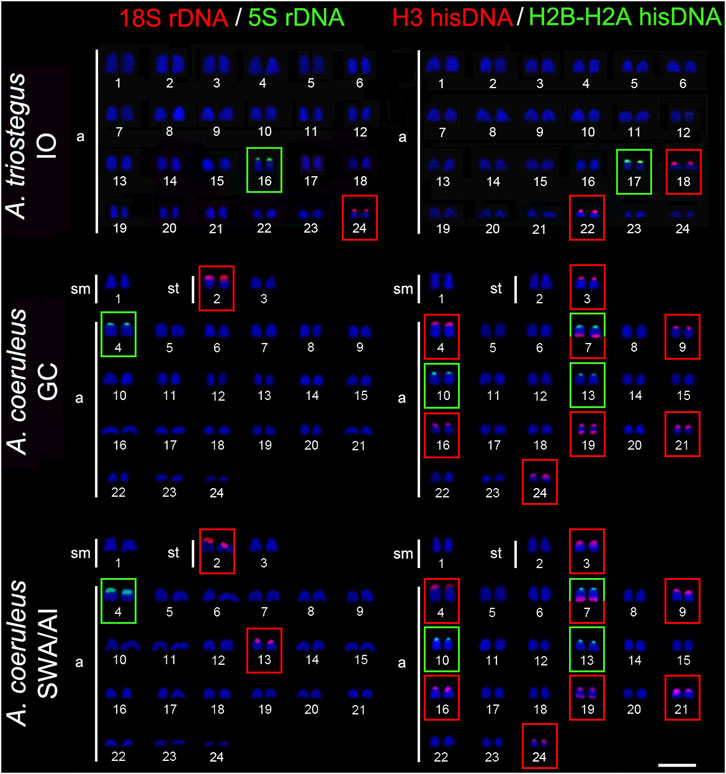
FIGURE 2. Fluorescence in situ hybridization signals indicating the distribution of the 18S rDNA (red signals), 5S rDNA (green signals), H3 hisDNA (red signals) and H2B-H2A hisDNA (green signals) sites in chromosomes of Acanthurus triostegus and Acanthurus coeruleus. Scale bar = 5 μm. IO, Indian Ocean; SWA, Southwest Atlantic; AI, South Atlantic Islands; GC, Greater Caribbean.
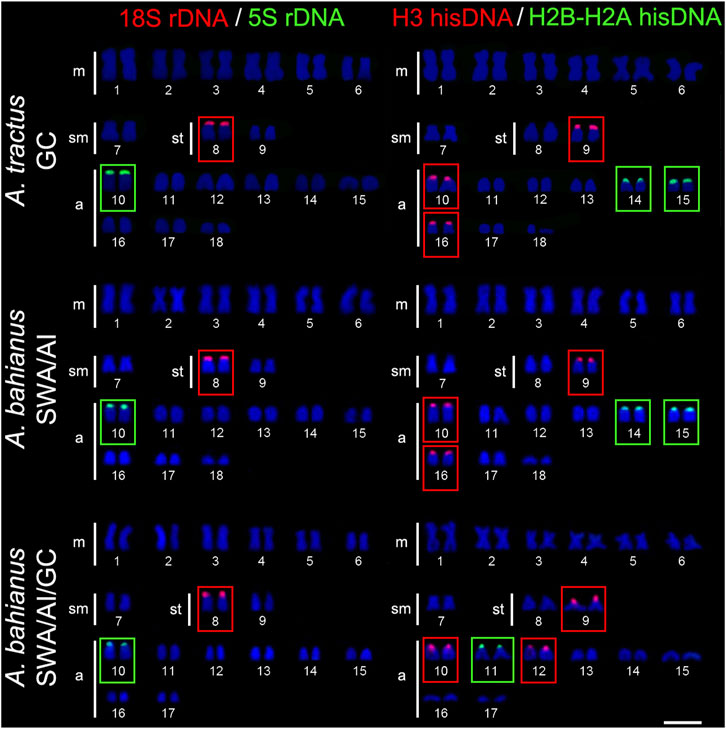
FIGURE 3. Fluorescence in situ hybridization signals showing the distribution of the 18S rDNA (red signals), 5S rDNA (green signals), H3 hisDNA (red signals) and H2B-H2A hisDNA (green signals) sites in chromosomes of Acanthurus tractus, Acanthurus bahianus, and Acanthurus chirurgus. Scale bar = 5 μm. SWA, Southwest Atlantic; AI, South Atlantic Islands; GC, Greater Caribbean.
The 5S rDNA sites are located in the short arms of pair 16 in A. triostegus. In the Atlantic species, A. coeruleus, A. tractus, A. bahianus, and A. chirurgus, the sites occur in the short arms of the largest acrocentric pair: pairs 4, 10, and 10 in individuals from Florida Keys (GC), Brazilian coast (SWA), and Atlantic oceanic islands, respectively (Figures 2, 3).
In all species, the H2B-H2A hisDNA sites are located exclusively in the short arms of the chromosomes but with numerous divergences among them: only one locus in A. triostegus (pair 17), only one locus also in A. chirurgus (pair 11), two loci in A. tractus and A. bahianus (pairs 14 and 15), and three loci in A. coeruleus (pairs 7, 10 and 13), highlighting a syntenic array with the H3 hisDNA in pair 7 (Figures 2, 3). In turn, the H3 hisDNA showed notable variations in the number of loci and distribution among species. In A. triostegus, two loci were located in the short arms of pairs 18 and 22 (Figure 2). In A. coeruleus nine loci, the largest number among species, are distributed in the short arms of pairs 3, 4 (co-located with a 5S rDNA site), 16, 21, and 24, and in the terminal regions of the long arms of pairs 7 and 19, in this latter with a bitelomeric arrangement (Figure 2). Meanwhile, A. tractus and A. bahianus presented three loci: in the short arms of pairs 9, 10 (co-localized with 5S rDNA site), and in the short arms of pair 16. Acanthurus chirurgus also showed three loci: in the short arms of pairs 9, 10 (co-localized with 5S rDNA site) and in pair 12 (Figure 3).
Discussion
The historical biogeography and current geographic context of Acanthurus species offer useful conditions for estimating the putative effects promoted by barriers and large oceanic spaces. In this context, our present data demonstrated cytogenetic variations and large-scale karyoevolutionary changes among populations and species, highlighting varied levels of divergence.
Karyotype Diversification in Western Atlantic Species of Acanthurus
Geographic barriers and ecological limitations can promote genetic structuring and endemism in coastal areas and oceanic islands of the Western Atlantic (Pinheiro et al., 2018). Karyotype divergences have been identified among several reef fish species divided by the Amazonas/Orinoco river plume (Nirchio et al., 2008; Rocha and Molina, 2008; Motta-Neto et al., 2019), probably promoted by divergent evolutionary forces under gene flow limitation.
Acanthurus species currently inhabiting the Western Atlantic are derived from two lineages that reached this oceanic region by different routes of colonization. Inferences based on mitochondrial and nuclear genetic sequences and fossil information suggest that one of them, reaching the region through the Tethys seaway, gave rise to A. bahianus, A. tractus, and A. chirurgus, at around 17.1 Mya (14.5–24.8 95% highest posterior density, HPD). The other one, coming through the Isthmus of Panama, colonized more recently the Western Atlantic, at around 13.1 Mya (7.6–18.8 HPD) (Siqueira et al., 2019). Acanthurus coeruleus (2n = 48), whose Atlantic colonization is derived from the Pacific lineage, has the largest number of acrocentric chromosomes, sharing the greatest karyotype similarity with A. triostegus (2n = 48a), a basal Acanthurus species from the Indo-Pacific Ocean (Sorenson et al., 2013). In general, conspicuous series of sequential rearrangements mainly derived from pericentric inversions, centric and in tandem fusions, promoted the karyotype diversification in Atlantic Acanthurus (Affonso et al., 2014).
Given the current known biogeographic history, the evolutionary origin (homologous or homoplasic) of the set of three pairs of two-armed chromosomes in all the Atlantic Acanthurus species (Figure 4) deserves further investigation, given that reconstructed phylogenetic relationships based in molecular evidences indicate that A. coeruleus is phylogenetically distant from A. bahianus, A. tractus, and A. chirurgus (Sorenson et al., 2013). However, the common origin of this set of chromosomes by pericentric inversions in A. tractus, A. bahianus, and A. chirurgus, as well as of the identical set of six large metacentric pairs by Robertsonian fusions and with a similar distribution of repetitive sequences (Affonso et al., 2014; Fernandes et al., 2015; present work), indicate a synapomorphic condition fixed among these Western Atlantic species.
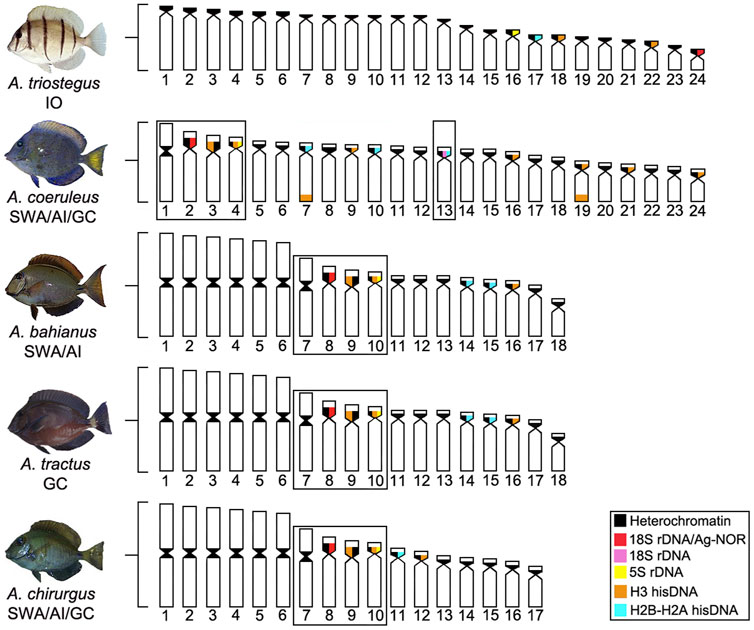
FIGURE 4. Ideogram showing the organization of different classes of repetitive DNAs (rDNA and hisDNA) in five species and populations of Acanthurus. The boxes highlight a set of two-armed chromosomes shared by all Atlantic species. The dotted box highlights a chromosome pair bearing an extra 18S rDNA site in Southwest Atlantic individuals. GC, Greater Caribbean; SWA, Southwest Atlantic; IO, Indian Ocean.
The evolutionary split between A. tractus and A. bahianus was preliminarily estimated at around one Mya. (Castellanos-Gell et al., 2012). Far from the origin of the Amazonas-Orinoco freshwater outflow barrier (around 10 Mya; Lovejoy et al., 1998), this recent event was not enough to fixate differential chromosomal characteristics between these two species. As these geminate species share similar karyotype patterns and have secondary contacts due to punctuated invasions of A. bahianus from the north to the south Atlantic regions in more recent times (Castellanos-Gell et al., 2012), they are very suitable targets for further evolutionary investigations. In fact, the sympatry between these geminate species is a scenario propitious to introgressive hybridization, a recurrent condition in Acanthurus species (Marie et al., 2007; DiBattista et al., 2016) and with evolutionarily consequences to Caribbean populations. On the other hand, the karyotype of A. chirurgus is significantly differentiated by its smaller diploid number (2n = 34) than that of A. bahianus and A. tractus (2n = 36). This autapomorphic condition is likely derived from in tandem fusion between a small acrocentric and the larger submetacentric chromosome pairs (Affonso et al., 2014).
Successive chromosomal differentiation in number and structure has occurred throughout the evolution of the Atlantic Acanthurus karyotypes; however, microstructural changes involving the repetitive fraction of the genome show diverse patterns. Repetitive sequences are important components of genomic differentiation and evolutionary processes (López-Flores and Garrido-Ramos, 2012; Biscotti et al., 2015). Eventually, these redundant sequences are also efficient as population (Lima-Filho et al., 2012) or cytotaxonomic markers (Amorim et al., 2016), even in groups with marked chromosomal conservatism (Motta-Neto et al., 2011a; Calado et al., 2013).
Indeed, while the distribution of the rDNA sequences presents a more regular distribution pattern (Fernandes et al., 2015), our present data show that histone sequences have a more dynamic diversification among species. The histone multigene family is known to play a fundamental role in the structural organization of chromatin in eukaryotes, as well as in the regulation of gene expression (Chioda et al., 2002), showing a considerable level of organization on the chromosomes of various fish groups (Hashimoto et al., 2011; Costa et al., 2016; Borges et al., 2019). Within Acanthurus, the Atlantic species (A. tractus, A. bahianus, A. chirurgus, and mainly A. coeruleus) show a more variable chromosomal distribution for the H2B/H2A and H3 histones than the Indo-Pacific lineage (A. triostegus). In fact, among the Atlantic species, such histone sites are clustered in multiple chromosome pairs, including bitelomeric arrays in some chromosome pairs of A. coeruleus.
Except for a few H3 sites occupying telomeric positions in A. coeruleus, hisDNA sites are mainly present in centromeric heterochromatic regions. Similar patterns in other fish groups reinforce their functional co-localization with complex sets of repetitive DNAs (Hashimoto et al., 2011; Lima-Filho et al., 2012), including transposable elements (Roehrdanz et al., 2010; Costa et al., 2014, 2016). Based on provisional chromosome arrangements, our FISH signals also indicate the overlapping of H2B/H2A and H3 histones sequences with each other and with rDNA sequences, both 18S and 5S rDNA, in some heterochromatic regions. The association of distinct DNA classes highlights multiple evolutionary processes that model the repetitive DNA fraction in Acanthurus species. Additionally, H3 hisDNA showed more abundant sites than H2B-H2A. Despite its uncertain cause, this frequency has also been observed in other marine species, such as Rachycentron canadum (Rachycentridae) (Costa et al., 2014), Ocyurus chrysurus (Lutjanidae) (Costa et al., 2016), and Centropomus species (Centropomidae) (Borges et al., 2019). The number of H2B-H2A and H3 sites declined with respect to the evolutionary divergence of the Atlantic species: A. coeruleus with three and eight sites; A. tractus and A. bahianus with two and three sites; and A. chirurgus with one and three sites, respectively. Meanwhile, A. triostegus presents the lowest frequency: one and 2 sites. The distribution of hisDNA in these species indicates an evolution by a stochastic birth-and-death process (Novozhilov et al., 2006), with the reduction of sites along with their diversification steps.
Karyoevolution of the Genus Acanthurus in the Biogeographic Context
The cytogenetic profiles of Acanthurus species reveal evolutionary steps of chromosomal organization at the macro and microstructural levels, which are supported by historical biogeographic events and phylogenetic relationships. The occurrence of exclusive 2n = 48 acrocentric chromosomes in A. triostegus, a basal species with divergence contemporary to the genus Acanthurus (Sorenson et al., 2013), to the ancient genus Prionurus (P. microlepidotus; Arai and Inoue, 1976), and the paraphyletic Ctenochaetus (Ojima and Yamamoto, 1990), consolidates this karyotype as baseline for Acanthuridae in a most parsimonious manner. In addition, A. triostegus shares other symplesiomorphic traits with several Percomorpha groups, such as unique Ag-NORs/18S rDNA sites and heterochromatin restricted to centromeric regions (Galetti et al., 2000).
In addition to biological factors interfering with the gene flow of marine fish populations (McCormick, 1999; Otwoma et al., 2018; Grulois et al., 2020), stochastic physical events (tectonic processes, glaciations, and opening or closure of oceanic barriers) have modeled the complex biogeography of Acanthuridae (Siqueira et al., 2019) and its karyotype diversification. Cytogenetic comparisons in Atlantic Acanthurus highlight conspicuous synapomorphies regarding the karyotype structure and the organization of repetitive DNA classes, concerning the most basal pattern represented by the A. triostegus lineage.
The dispersive potential of A. triostegus (Fisher and Hogan, 2007; Liggins et al., 2016; Otwoma et al., 2018) favor gene flow in the marine environment (Rocha, 2003). These characteristics are possible explanations for the conservative basal karyotype of this species and the karyotype homogeneity between the Indian and Pacific populations. In contrast, A. coeruleus, A. chirurgus, and A. tractus/A. bahianus, with recent divergence in the Atlantic, and under different evolutionary and ecological changes, such as allopatry, population fragmentation, and niche displacement (Siqueira et al., 2019), show a more evident chromosomal diversification. Indeed, the divergence between the A. coeruleus lineage and the clade composed of A. tractus/A. bahianus and A. chirurgus (19 Mya), and those between the two last clades (10 Mya) (Figure 5), coincides with global changes in ocean dynamics (tectonic events) that affected the levels of richness and endemism of reef fish (Floeter et al., 2008). In the Atlantic Ocean, the isolation of coastal habitats during glaciations, which promoted recurrent variations at sea level, has influenced the patterns of genetic structuring (Souza et al., 2015) and, in more recent times, it may have contributed to a higher rate of chromosome evolution in reef fish.
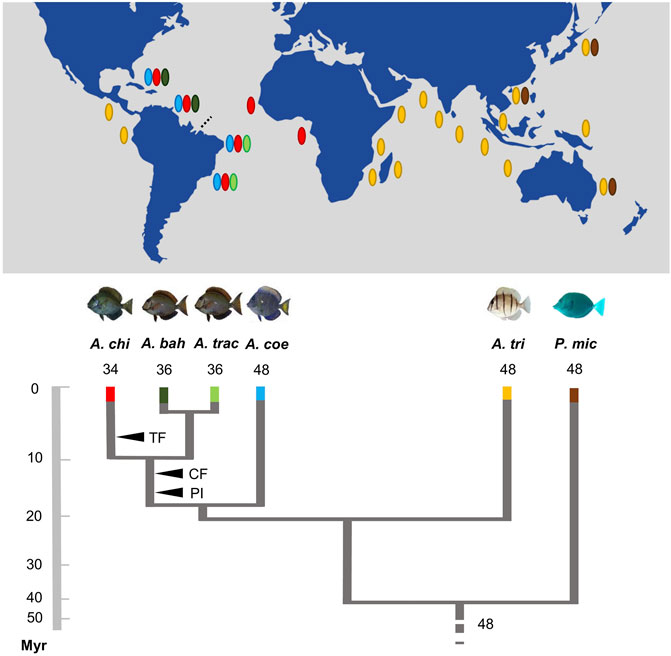
FIGURE 5. Karyotype diversification, phylogenetic relationships, and schematic geographic distribution of the Acanthurus species analyzed. The black arrowheads indicate shared and exclusive chromosomal rearrangements in the Atlantic species (CF: six centric fusions events; PI: three pericentric inversions events; TF: in tandem fusion) regarding their phylogenetic diversification (adapted from Bernal and Rocha, 2011; Sorenson et al., 2013). The dotted line highlights the Amazonas River plume. The names of the species are identified by abbreviations—Acanthurus chirurgus (A. chi); Acanthurus bahianus (A. bah); Acanthurus tractus (A. tra); Acanthurus coeruleus (A. coe); Acanthurus triostegus (A. tri); Prionurus microlepidotus (P. mic). It shows an inverted geographic distribution to the species Acanthurus tractus and Acanthurus bahianus analyzed.
In general, acanthurids have a very low population structure, even at great distances, such as the 3,540 km between the Brazilian region and Santa Helena Island (Rocha et al., 2002). It is believed that the high potential for dispersion (Lessios and Robertson, 2006) and colonization events of Acanthurus (Otwoma et al., 2018) promote genetic homogeneity and limited evolutionary dynamism among species karyotypes. In this context, the karyotype characteristics of Atlantic island populations are only now being clarified. Cytogenetic comparisons between A. chirurgus from the Fernando de Noronha Archipelago (FNA) and A. bahianus from Trindade Island, and among A. coeruleus from FNA, the Brazilian coast, and GC indicated that, despite the great oceanic distances and geographic isolation, there was no noticeable variation in their karyotypes.
In contrast, a certain level of variation occurs between specimens of A. coeruleus from GC and the SWA, in which there are two additional 18S rDNA sites in the latter. These additional sites correspond to non-active NORs (negative Ag-NORs) and a cytogeographic characteristic of this population. Significantly, this rDNA marker complements other phylogeographic pieces of evidence indicating population stratification between these areas (Rocha et al., 2002), as a consequence of the outflow barrier from the Amazon and Orinoco rivers. On the other hand, the geminate species, A. bahianus and A. tractus, resulting from their high sensibility to ecological effects of the Amazonas-Orinoco outows (Rocha et al., 2002), share a common cytogenetic pattern. Similar to some other reef fishes (Getlekha et al., 2018), these commonalities are derived from the recent evolutionary diversification of these groups, which impairs the fixation of chromosomal rearrangements. The sympatric occurrence of A. bahianus and A. tractus in some areas of the GC (Castellanos-Gell et al., 2012) opens a particular condition for investigating reproductive isolation in the absence of conspicuous karyotype diversification.
It is noteworthy that karyotype variability and diversification between populations and species, such as Lutjanidae (Nirchio et al., 2008; Rocha and Molina, 2008; Costa et al., 2016), Haemulidae (Motta-Neto et al., 2011b), and Grammatidae (Molina et al., 2012), have also been observed in Atlantic regions. These data increase the evidence of the primary interference of the Amazonas/Orinoco River plume in the karyotype differentiation of Atlantic reef fishes. Overall, our data highlight the use of integrating chromosomal patterns, including microstructural characters, phylogenetics, and historical biogeography, in elucidating the karyotype evolution of marine fishes, connectivity among biogeographic provinces and on the estimation of chromosomal divergences among marine populations.
Data Availability Statement
The original contributions presented in the study are included in the article/supplementary material, further inquiries can be directed to the corresponding author.
Ethics Statement
The animal study was reviewed and approved by The animal study was reviewed and approved by the Committee of Ethics for the use of Animals of the Federal University of Rio Grande do Norte (Proc. #044/2015).
Author Contributions
MF and WM contributed conception and design of the study; MF, GC, KP, WS and AT performed cytogenetic analyses. ASS performed sequence analyses. C-N, AB, GC, RS performed specimen’s collection. MF and WM wrote the first draft of the manuscript; MC, LB, AT and WM, wrote sections of the manuscript. All authors contributed to manuscript revision, read and approved the submitted version.
Funding
This work received national funds through CNPq Brazilian National Council for Scientific and Technological Development through projects Proc. 442664/2015-0 and Proc. 442626/2019-3. This research was partly supported by INCT “Marine Sciences” (565054/2010-4).
Conflict of Interest
The authors declare that the research was conducted in the absence of any commercial or financial relationships that could be construed as a potential conflict of interest.
Publisher’s Note
All claims expressed in this article are solely those of the authors and do not necessarily represent those of their affiliated organizations, or those of the publisher, the editors and the reviewers. Any product that may be evaluated in this article, or claim that may be made by its manufacturer, is not guaranteed or endorsed by the publisher.
Acknowledgments
The authors thank ICMBio SISBIO for permits to collect specimens (licenses 19135-1, 27027-2 and 131360-1). We are also grateful to Jose Garcia Jr for taxonomic identification of specimens.
References
Affonso, P. R. A. M., Fernandes, M. A., Almeida, J. S., and Molina, W. F. (2014). Sequential Steps of Chromosomal Differentiation in Atlantic Surgeonfishes: Evolutionary Inferences. Scientific World J. 2014, 1–7. doi:10.1155/2014/825703
Albig, W., Warthorst, U., Drabent, B., Prats, E., Cornudella, L., and Doenecke, D. (2003). Mytilus edulis Core Histone Genes Are Organized in Two Clusters Devoid of Linker Histone Genes. J. Mol. Evol. 56, 597–606. doi:10.1007/S00239-002-2428-8
Amorim, K. D. J., Cioffi, M. B., Bertollo, L. A. C., and Soares, R. X. (2017). Interregional Cytogenetic Comparisons in Halichoeres and Thalassoma Wrasses (Labridae) of Coastal and Insular Regions of the Southwestern Atlantic. Genet. Mol. Res. 16 (2). doi:10.4238/gmr16029650
Amorim, K. D. J., Cioffi, M. B., Soares, R. X., and Costa, G. W. W. (2016). Co-located 18S/5S rDNA Arrays: an Ancient and Unusual Chromosomal Trait in Julidini Species (Labridae, Perciformes). Comp. Cytogenet. 10, 555–570. doi:10.3897/CompCytogen.v10i4.10227
Arai, R., and Inoue, M. (1976). Chromosomes of Seven Species of Pomacentridae and Two Species of Acanthuridae from Japan. Bull. Natl. Mus. Nat. Sci. A2, 73–78.
Bernal, M. A., and Rocha, L. (2011). Acanthurus Tractus, Poey, 1860, a Valid Western Atlantic Species of Surgeonfish (Teleostei, Acanthuridae), Distinct from Acanthurus Bahianus Castelnau, 1855. Zootaxa 2905, 63–68. doi:10.11646/zootaxa.2905.1.5
Bernardi, G., Nelson, P., Paddack, M., Rulmal, J., and Crane, N. (2018). Genomic Islands of Divergence in the Yellow Tang and the Brushtail Tang Surgeonfishes. Ecol. Evol. 8, 8676–8685. doi:10.1002/ece3.4417
Biscotti, M. A., Olmo, E., and Heslop-Harrison, J. S. (2015). Repetitive DNA in Eukaryotic Genomes. Chromosome Res. 23, 415–420. doi:10.1007/s10577-015-9499-z
Borges, A. T., Cioffi, M. B., Bertollo, L. A. C., and Soares, R. X. (2019). Paracentric Inversions Differentiate the Conservative Karyotypes in Two Centropomus Species (Teleostei: Centropomidae). Cytogenet. Genome Res. 157, 239–248. doi:10.1159/000499748
Calado, L. L., Bertollo, L. A. C., Costa, G. W. W. F., and Molina, W. F. (2013). Cytogenetic Studies of Atlantic Mojarras (Perciformes: Gerreidae): Chromosomal Mapping of 5S and 18S Ribosomal Genes Using Double FISH. Aquacult. Res. 44, 829–835. doi:10.1111/j.1365-2109.2012.03089.x
Castellanos-Gell, J., Barcia, A. R., Monteagudo, P. C., and Metcalfe, C. (2012). The Surgeonfish, Acanthurus Bahianus, Has Crossed the Amazon–Orinoco Outflow Barrier. Mar. Biol. 159, 1561–1565. doi:10.1007/s00227-012-1942-5
Chioda, M., Eskeland, R., and Thompson, E. M. (2002). Histone Gene Complement, Variant Expression, and mRNA Processing in a Urochordate Oikopleura dioica that Undergoes Extensive Polyploidization. Mol. Biol. Evol. 19, 2247–2260. doi:10.1093/oxfordjournals.molbev.a004048
Costa, G. W. W. F., Cioffi, M. B., Bertollo, L. A. C., and Molina, W. F. (2016). The Evolutionary Dynamics of Ribosomal Genes, Histone H3, and Transposable Rex Elements in the Genome of atlantic Snappers. J. Hered. 107, 173–180. doi:10.1093/jhered/esv136
Costa, G. W. W. F., Cioffi, M. B., Bertollo, L. A. C., and Molina, W. F. (2014). Unusual Dispersion of Histone Repeats on the Whole Chromosomal Complement and Their Colocalization with Ribosomal Genes in Rachycentron canadum (Rachycentridae, Perciformes). Cytogenet. Genome Res. 144, 1–6. doi:10.1159/000366301
DiBattista, J. D., Whitney, J., Craig, M. T., Hobbs, J. A., Rocha, L. A., Feldheim, K. A., et al. (2016). Surgeons and Suture Zones: Hybridization Among Four Surgeonfish Species in the Indo-Pacific with Variable Evolutionary Outcomes. Mol. Phylogenet. Evol. 101, 203–215. doi:10.1016/j.ympev.2016.04.036
Fernandes, M. A., Affonso, P. R. A. M., Costa, G. W. W. F., and Cioffi, M. B. (2015). Atlantic Surgeonfishes bear Only Minor Microstructural Changes in Highly Derived Karyotypes. Zool. Anz. 254, 62–66. doi:10.1016/j.jcz.2014.11.003
Fisher, R., and Hogan, J. D. (2007). Morphological Predictors of Swimming Speed: a Case Study of Pre-settlement Juvenile Coral Reef Fishes. J. Exp. Biol. 210, 2436–2443. doi:10.1242/jeb.004275
Floeter, S. R., Rocha, L. A., Robertson, D. R., Joyeux, J. C., and Smith-Vaniz, W. F. (2008). Atlantic Reef Fish Biogeography and Evolution. J. Biogeogr. 35, 22–47. doi:10.1111/j.1365-2699.2007.01790.x
Fricke, R., Eschmeyer, W. N., and Fong, J. D. (2021). Eschmeyer's Catalog of Fishes: Genera/Species by Family/Subfamily. Available at: http://researcharchive.calacademy.org/research/ichthyology/catalog/SpeciesByFamily.asp). Electronic version (accessed March 16, 2021).
Galetti, P. M., Aguilar, C. T., and Molina, W. F. (2000). An Overview of marine Fish Cytogenetics. Hydrobiologia 420, 55–62. doi:10.1023/A:1003977418900
Getlekha, N., Cioffi, M. B., Maneechot, N., Bertollo, L. A. C., Supiwong, W., Tonomtong, A., et al. (2018). Contrasting Evolutionary Paths Among Indo-Pacific Pomacentrus Species Promoted by Extensive Pericentric Inversions and Genome Organization of Repetitive Sequences. Zebrafish 15, 45–54. doi:10.1089/zeb.2017.1484
Giribert, G., and Distel, D. (2003). “Bivalve Phylogeny and Molecular Data,” in ” in Molecular Systematics and Phylogeography of Molluscks. Editors C. Lydeard, and D. R. Lindberg (Washington: Smithsonian Books), 45–90.
Gold, J. R., Li, Y. C., Shipley, N. S., and Powers, P. K. (1990). Improved Methods for Working with Fish Chromosomes with a Review of Metaphase Chromosome Banding. J. Fish. Biol. 37, 563–575. doi:10.1111/j.1095-8649.1990.tb05889.x
Gonzalez, I. L., and Sylvester, J. E. (2001). Human rDNA: Evolutionary Patterns within the Genes and Tandem Arrays Derived from Multiple Chromosomes. Genomics 736, 255–263. doi:10.1006/geno.2001.6540
Green, A. L., and Bellwood, D. R. (2009). Monitoring Functional Groups of Herbivorous Reef Fishes as Indicators of Coral Reef Resilience: A Practical Guide for Coral Reef Managers in the Asia Pacific Region. Manual. Gland. Switzerland: The International Union for the Conservation of Nature and Natural Resources.
Grulois, D., Hogan, R. I., Paygambar, S., Planes, S., and Fauvelot, C. (2020). New Microsatellite DNA Markers to Resolve Population Structure of the Convict Surgeonfish, Acanthurus triostegus, and Cross-Species Amplifications on Thirteen Other Acanthuridae. Mol. Biol. Rep. 47, 8243–8250. doi:10.1007/s11033-020-05773-0
Hashimoto, D. T., Ferguson-Smith, M. A., Rens, W., Foresti, F., and Porto-Foresti, F. (2011). Chromosome Mapping of H1 Histone and 5S rRNA Gene Clusters in Three Species of Astyanax (Teleostei, Characiformes). Cytogenet. Genome Res. 134, 64–71. doi:10.1159/000323512
Howell, W. M., and Black, D. A. (1980). Controlled Silver-Staining of Nucleolus Organizer Regions with a Protective Colloidal a 1-step Method. Experientia 36, 1014–1015.
Kess, T., Bentzen, P., Lehnert, S. J., Sylvester, E. V. A., Lien, S., Kent, M. P., et al. (2020). Modular Chromosome Rearrangements Reveal Parallel and Nonparallel Adaptation in a marine Fish. Ecol. Evol. 10, 638–653. doi:10.1002/ece3.5828
Lessios, H. A., and Robertson, D. R. (2006). Crossing the Impassable: Genetic Connections in 20 Reef Fishes across the Eastern Pacific Barrier. Proc. R. Soc. Lond. B Biol. Sci. 7, 2201–2208. doi:10.1098/rspb.2006.3543
Levan, A., Fredga, K., and Sandberg, A. A. (1964). Nomenclature for Centromeric Position on Chromosomes. Hereditas 52, 201–220. doi:10.1111/j.1601-5223.1964.tb01953.x
Liggins, L., Treml, E. A., Possingham, H. P., and Riginos, C. (2016). Seascape Features, rather Than Dispersal Traits, Predict Spatial Genetic Patterns in Co-distributed Reef Fishes. J. Biogeogr. 43, 256–267. doi:10.1111/jbi.12647
Lima-Filho, P. A., Cioffi, M. B., Bertollo, L. A. C., and Molina, W. F. (2012). Chromosomal and Morphological Divergences in Atlantic Populations of the Frillfin Goby Bathygobius Soporator (Gobiidae, Perciformes). J. Exp. Mar. Biol. Ecol. 434-435, 63–70. doi:10.1016/J.Jembe.2012.08.004
López-Flores, I., and Garrido-Ramos, M. A. (2012). “The Repetitive DNA Content of Eukaryotic Genomes,” in Genome Dynamics. Editor M. A. Garrido-Ramos (Basel: Karger), 1–28.
Lovejoy, N. R., Bermingham, E., and Martin, A. P. (1998). Marine Incursion into South America. Nature 396, 421–422. doi:10.1038/24757
Ludt, W. B., Rocha, L. A., and Chakrabarty, P. (2020). The First Complete Mitochondrial Genomes of Sawtail Surgeonfishes (Acanthuridae: Prionurus). Mitochondrial DNA B 5, 212–213. doi:10.1080/23802359.2019.1699465
Marie, A. D., van Herwerden, L., and Choat, J. H. (2007). Hybridization of Reef Fishes at the Indo-Pacific Biogeographic Barrier: a Case Study. Coral Reefs 26, 841–850. doi:10.1007/s00338-007-0273-3
McCormick, M. I. (1999). Delayed Metamorphosis of a Tropical Reef Fish (Acanthurus triostegus): a Field experiment. Mar. Ecol. Prog. Ser. 76, 25–38.
Molina, W. F., Alves, D. E. O., Araujo, W. C., Martinez, P. A., Silva, M. F. M., and Costa, G. W. W. F. (2010). Performance of Human Immunostimulating Agents in the Improvement of Fish Cytogenetic Preparations. Genet. Mol. Res. 9, 1807–1814. doi:10.4238/Vol9-3gmr840
Molina, W. F., Costa, G. W. W. F., Cioffi, M. B., and Bertollo, L. A. C. (2012). Chromosomal Differentiation and Speciation in Sister-Species of Grammatidae (Perciformes) from the Western Atlantic. Helgol. Mar. Res. 66, 363–370. doi:10.1007/S10152-011-0276-X
Motta-Neto, C. C., Cioffi, M. B., Bertollo, L. A. C., and Molina, W. F. (2011b). Extensive Chromosomal Homologies and Evidence of Karyotypic Stasis in Atlantic Grunts of the Genus Haemulon (Perciformes). J. Exp. Mar. Biol. Ecol. 401, 75–79. doi:10.1016/J.Jembe.2011.02.044
Motta-Neto, C. C., Cioffi, M. B., Bertollo, L. A. C., and Molina, W. F. (2011a). Molecular Cytogenetic Analysis of Haemulidae Fish Evolutionary Conservation. J. Exp. Mar. Biol. Ecol. 407, 97–100. doi:10.1016/J.Jembe.2011.07.014
Motta-Neto, C. C., Cioffi, M. B., Costa, G. W. W. F., and Amorim, K. D. J. (2019). Overview on Karyotype Stasis in Atlantic Grunts (Eupercaria, Haemulidae) and the Evolutionary Extensions for Other marine Fish Groups. Front. Mar. Sci., 61–12. doi:10.3389/fmars.2019.00628
Motta-Neto, C. C., Lima-Filho, P. A., Araújo, W. C., Bertollo, L. A. C., and Molina, W. F. (2012). Differentiated Evolutionary Pathways in Haemulidae (Perciformes): Karyotype Stasis versus Morphological Differentiation. Rev. Fish. Biol. Fisher. 22, 457–465. doi:10.1007/S11160-011-9236-4
Nirchio, M., Oliveira, C., Siccha Ramirez, Z. R., Sene, V. F., Sola, L., Milana, V., et al. (2017). The Mugil curema Species Complex: a New Karyotype for the Pacific white Mullet Mitochondrial Lineage. Comp. Cytogenet. 11, 225–237. doi:10.3897/CompCytogen.v11i2.11579
Nirchio, M., Rondon, R., Oliveira, C., and Ferreira, I. A. (2008). Cytogenetic Studies in Three Species of Lutjanus (Perciformes: Lutjanidae: Lutjaninae) from the Isla Margarita, Venezuela. Neotrop. Ichthyol. 6, 101–108. doi:10.1590/S1679-62252008000100012
Ojima, Y., and Yamamoto, K. (1990). Cellular DNA Contents of Fishes Determined by Flow Cytometry. La Kromosomo II 57, 1871–1888.
Otwoma, L. M., Diemel, V., Reuter, H., Kochzius, M., and Meyer, A. (2018). Genetic Population Structure of the Convict Surgeonfish Acanthurus triostegus: a Phylogeographic Reassessment across its Range. J. Fish. Biol. 93, 597–608. doi:10.1111/jfb.13686
Palumbi, S. R. (1991). “Nucleic Acids II: the Polymerase Chain Reaction,” in ” in Molecular Systematics. Editors D. M. Hillis, and C. Moritz (Sunderland: Mable Sinauer and Associates Inc.), 205–247.
Papavlasopoulou, I., Vardakas, L., Perdikaris, C., Kommatas, D., and Paschos, I. (2014). Ornamental Fish in Pet Stores in Greece: a Threat to Biodiversity?. Mediterr. Mar. Sci. 15, 126–134. doi:10.12681/mms.484
Pendás, A. M., Moran, P., Freije, J. P., and Garcia-Vazquez, E. (1994). Chromosomal Mapping and Nucleotide Sequence of Two Tandem Repeats of Atlantic salmon 5S rDNA. Cytogenet. Cell Genet. 67, 31–36. doi:10.1159/000133792
Pinheiro, H. T., Rocha, L. A., and Macieira, R. M. (2018). South-western Atlantic Reef Fishes: Zoogeographical Patterns and Ecological Drivers Reveal a Secondary Biodiversity centre in the Atlantic Ocean. Divers. Distrib 24, 951–965. doi:10.1111/ddi.12729
Pinkel, D., Straume, T., and Gray, J. W. (1986). Cytogenetic Analysis Using Quantitative, High-Sensitivity, Fluorescence Hybridization. P. Natl. Acad. Sci. U.S.A. 83, 2934–2938. doi:10.1073/Pnas.83.9.2934
Randall, J. E. (2001). ““Acanthuridae. Surgeonfishes (Tangs, Unicornfishes),” in FAO Species Identification Guide for Fishery Purposes,” in The Living marine Resources of the Western Central Pacific. Editors K. E. Carpenter, and V. Niem (Rome: Bony fishes part 4 Labridae to LatimeriidaeFAO), Vol. 6, 3653–3683.
Rocha, E. C., and Molina, W. F. (2008). Cytogenetic Analysis in Western Atlantic Snappers (Perciformes, Lutjanidae). Genet. Mol. Biol. 31/2, 461–467. doi:10.1590/S1415-47572008000300011
Rocha, L. A., Bass, A. L., Robertson, D. R., and Bowen, B. W. (2002). Adult Habitat Preferences, Larval Dispersal, and the Comparative Phylogeography of Three Atlantic Surgeonfishes (Teleostei: Acanthuridae). Mol. Ecol. 11, 243–252. doi:10.1046/j.0962-1083.2001.01431.x
Rocha, L. A. (2003). Patterns of Distribution and Processes of Speciation in Brazilian Reef Fishes. J. Biogeogr. 30, 1161–1171. doi:10.1046/j.1365-2699.2003.00900.x
Roehrdanz, R., Heilmann, L., Senechal, P., Sears, S., and Evenson, P. (2010). Histone and Ribosomal RNA Repetitive Gene Clusters of the Boll Weevil Are Linked in a Tandem Array. Insect Mol. Biol. 19, 463–471. doi:10.1111/j.1365-2583.2010.01006.x
Rooney, A. P., and Ward, T. J. (2005). Evolution of a Large Ribosomal RNA Multigene Family in Filamentous Fungi: Birth and Death of a Concerted Evolution Paradigm. PNAS 102, 5084–5089. doi:10.1073/pnas.0409689102
Russ, G. R., Payne, C. S., Bergseth, B. J., Rizzari, J. R., Abesamis, R. A., and Alcala, A. C. (2018). Decadal-scale Response of Detritivorous Surgeonfishes (Family Acanthuridae) to No-Take marine reserve protection and Changes in Benthic Habitat. J. Fish. Biol. 93, 887–900. doi:10.1111/jfb.13809
Sadovy, Y. J., and Vincent, A. C. J. (2002). in “Ecological Issues and the Trades in Live Reef Fishes,” in Coral Reef Fishes. Editor P.F. Sale (San Diego, USA: Academic Press), 391–420.
Sambrook, J., Fritschi, E. F., and Maniatis, T. (1989). Molecular Cloning: A Laboratory Manual. New York: Cold Spring Harbor Laboratory Press.
Siqueira, A. C., Bellwood, D. R., and Cowman, P. F. (2019). Historical Biogeography of Herbivorous Coral Reef Fishes: The Formation of an Atlantic Fauna. J. Biogeogr. 46, 1611–1624. doi:10.1111/jbi.13631
Sorenson, L., Santini, F., Carnevale, G., and Alfaro, M. E. (2013). A Multilocus Timetree of Surgeonfishes (Acanthuridae, Percomorpha), with Revised Family Taxonomy. Mol. Phylogenet. Evol. 68, 150–160. doi:10.1016/j.ympev.2013.03.014
Souza, A. S., Dias, E. A., Galetti, P. M., Garcia-Machado, E., Pichorim, M., and Molina, W. F. (2015). Wide-range Genetic Connectivity of Coney, Cephalopholis Fulva (Epinephelidae), through Oceanic Islands and continental Brazilian Coast. Acad. Bras. Ciênc. 87, 121–136. doi:10.1590/0001-3765201520130411
Sumner, A. T. (1972). A Simple Technique for Demonstrating Centromeric Heterochromatin. Exp. Cel Res. 75, 304–306.
White, T. J., Bruns, T., Lee, S., and Taylor, J. (1990). “Amplification and Direct Sequencing of Fungal Ribosomal RNA Genes for Phylogenetics,” in ” in PCR Protocols: A Guide to Methods and Applications. Editors M. A. Innis, D. H. Gelfand, J. J. Shinsky, and T. J. White (New York, NY: Academic Press), 315–322.
Keywords: marine fish, comparative cytogenetic, hisDNA, oceanic barrier, multigenic family
Citation: Fernandes MA, Cioffi MdB, Bertollo LAC, Costa GWWFd, Motta-Neto CCd, Borges AT, Soares RX, Souza ASd, Pinthong K, Supiwong W, Tanomtong A and Molina WF (2021) Evolutionary Tracks of Chromosomal Diversification in Surgeonfishes (Acanthuridae: Acanthurus) Along the World’s Biogeographic Domains. Front. Genet. 12:760244. doi: 10.3389/fgene.2021.760244
Received: 17 August 2021; Accepted: 18 October 2021;
Published: 29 October 2021.
Edited by
:Mariana Härter Remião, Federal University of Pelotas,BrazilReviewed by
:Leandro Argolo, Vale Technological Institute (ITV), BrazilAlessio Iannucci, University of Florence, Italy
Copyright © 2021 Fernandes, Cioffi, Bertollo, Costa, Motta-Neto, Borges, Soares, Souza, Pinthong, Supiwong, Tanomtong and Molina. This is an open-access article distributed under the terms of the Creative Commons Attribution License (CC BY). The use, distribution or reproduction in other forums is permitted, provided the original author(s) and the copyright owner(s) are credited and that the original publication in this journal is cited, in accordance with accepted academic practice. No use, distribution or reproduction is permitted which does not comply with these terms.
*Correspondence: Wagner Franco Molina, bW9saW5hd2ZAeWFob28uY29tLmJy