- 1ICAR-National Bureau of Plant Genetic Resources, New Delhi, India
- 2Department of Bioscience and Biotechnology, Banasthali Vidyapith University, Tonk Rajasthan, India
- 3Division of Plant Physiology, ICAR-Indian Agricultural Research Institute, New Delhi, India
- 4Shivaji College, University of Delhi, New Delhi, India
- 5Division of Crop Improvement, ICAR-Indian Grassland and Research Institute, Jhansi, India
Mungbean (Vigna radiata L. Wilczek) is an important food legume crop which contributes significantly to nutritional and food security of South and Southeast Asia. The crop thrives in hot and humid weather conditions, with an optimal temperature range of 28°–35°C, and is mainly cultivated under rainfed environments. However, the rising global temperature has posed a serious threat to mungbean cultivation. Optimal temperature is a vital factor in cellular processes, and every crop species has evolved with its specific temperature tolerance ability. Moreover, variation within a crop species is inevitable, given the diverse environmental conditions under which it has evolved. For instance, various mungbean germplasm can grow and produce seeds in extreme ambient temperatures as low as 20°C or as high as 45°C. This range of variation in mungbean germplasm for heat tolerance plays a crucial role in developing heat tolerant and high yielding mungbean cultivars. However, heat tolerance is a complex mechanism which is extensively discussed in this manuscript; and at the same time individual genotypes have evolved with various ways of heat stress tolerance. Therefore, to enhance understanding towards such variability in mungbean germplasm, we studied morphological, anatomical, physiological, and biochemical traits which are responsive to heat stress in plants with more relevance to mungbean. Understanding heat stress tolerance attributing traits will help in identification of corresponding regulatory networks and associated genes, which will further help in devising suitable strategies to enhance heat tolerance in mungbean. The major pathways responsible for heat stress tolerance in plants are also discussed.
1 Introduction
Mungbean (Vigna radiata L. Wilczek), also commonly called as greengram, is a leguminous crop. It is an annual grain legume crop, cultivated in different soil types of the South-East Asia and South East Africa, Australia, and South America (Parihar et al., 2017). The crop requires warm-humid climatic conditions, with temperature ranging between 25°C and 35°C and a well distributed rainfall of 400–550 mm during growing season. Mungbean has a high range of storage protein (22%–27%) with sugar, minerals, and soluble dietary fibers (Alom et al., 2014). Recently, the crop area and production has increased demand of plant-based protein with affordable market price, as a result, mungbean is now being commercially cultivated in large scale (Keatinge et al., 2011). Global production of mungbean is around 6.0 million tones which comes from a cultivated area of about 7.3 million hectares (Gayacharan et al., 2023). India alone produces mungbean up to 41% of the global production which makes it the largest producer of mungbean followed by Myanmar, Bangladesh and Pakistan (Schreinemachers et al., 2019). Loam to sandy loam soils with good drainage are the best suited for mungbean cultivation. Because of its short life span, nitrogen-fixing ability, low water requirement, great biomass, and high yield mungbean is considered as one of the most important crops in agriculture (Alom et al., 2014). However, the high variability in climatic conditions including rising temperature and unpredictable water deficit environments during its cropping season cause drastic reduction in mungbean productivity (Singh et al., 2016). Several abiotic stresses such as heat, salinity, water-logging, and drought highly affects the growth and development in Mungbean (Dreesen et al., 2012; Bita and Gerats, 2013; Kaur and Nayyar, 2015; Landi et al., 2017; Zandalinas et al., 2017).
Among various factors, global temperature rise is the major challenge in legume crop production. The erratic and low rainfall, soil desertification, evolving new races of pest and pathogens are some other problems associated with the global temperature rise, which are adversely impacting crop production across the globe. Amid climate change, cultivation of legume crops has become more challenging, as these are obligatory adapted to low input environments and are majorly cultivated in rainfed conditions. Legumes are highly impacted by insect, pest primarily attributed to their protein rich nature and narrow genetic base. The regional report of Middle East and North Africa (MENA) region, climate change adversely impacts pulses more than any other crop group (Njuki et al., 2022). The regional report on MENA region indicated yield reduction due to climate change in pulses by 17.2%, followed by oil seeds (6.86%), cereals (4.18%) and fruits and vegetables (1.78%) (Njuki et al., 2022). Similar impact of climate change is observed across the globe with varying severity. However, among all food sources, pulses are the only food for which increased consumption demand is predicted by 2050 (Njuki et al., 2022).
Legumes including mungbean, which are grown in warm-humid climatic conditions are more affected by high temperature. In India, mungbean cultivation during summer season faces severe heat waves, and sometime temperature rises to 45°C, at which most of the cellular processes stop functioning. Various studies have demonstrated that under heat stress, significant yield losses occur in mungbean at reproductive stage of plant (Hamada, 2001; Hall, 2010; Kumar et al., 2013; Kaur and Nayyar, 2015; Sharma et al., 2016; Priya et al., 2020). Studies also described that mungbean under heat stress at reproductive stage is more adversely impacted as compared to vegetative stage under heat stress (Hall, 2010; Priya et al., 2020). Moreover, the male reproductive parts are more at risk to heat stress in comparison to the female reproductive parts in mungbean (Dickson and Boettger, 1984; Monterroso and Wien, 1990). Further, it is also found that physiological processes in reproductive tissues of mungbean are more susceptible to heat stress (Asseng et al., 2011; Kaur et al., 2015).
Heat stress triggers numerous physiological and biochemical processes in mungbean to counter the heat stress impact, but the crop yield is drastically reduced when severity of the heat stress is extreme (HanumanthaRao et al., 2016; Sita et al., 2017). However, very less research has been done to understand the impact of heat stress on mungbean for yield attributing traits and the reproductive parts. Therefore, to increase the productivity of mungbean under heat stress environment, it is important to find out the physio-biochemical and molecular variations for high temperature stress tolerance in the mungbean germplasm, and probe the mechanisms leading to heat sensitivity in mungbean crop. In this review, we provide recent understanding of heat stress effects and tolerance mechanisms in mungbean, focusing on its morphological, and physio-biochemical responses under high temperature stress.
2 Impacts of heat stress in mungbean
Mungbean is a summer season crop that may be cultivated in all dry and semi-arid parts of the world. However, recent global average temperature rise has posed a threat for the mungbean crop production (Abd El Lateff et al., 2018). The constant higher atmospheric temperature for longer duration is highly detrimental for the growth and physiological functions of various food crops (Cao et al., 2011). Severity of the crop damage varies with the timing, duration and magnitude of the elevated temperature, as well as the genotype specific defense response. In mungbean, during the summer season where temperature rise above 40°C causes terminal heat stress during reproductive stage of the plants which is a major concern in mungbean productivity because it results in impaired anthesis, loss of pollen viability, reduced flower fertilization, increased flower drop and shortened period for grain filling (HanumanthaRao et al., 2016; Basu et al., 2019) (Figure 1; Figure 2). Even an increase in temperature by a few degrees changes crop cycle and accelerates flower drop and embryo abortion, and poor grain filling (Kaur and Nayyar, 2015; Kaushal et al., 2016). Also, in kharif season mungbean temperature >40°C occur during early growth stages of the crop, causing similar problem particularly in northern parts of India (HanumanthaRao et al., 2016).
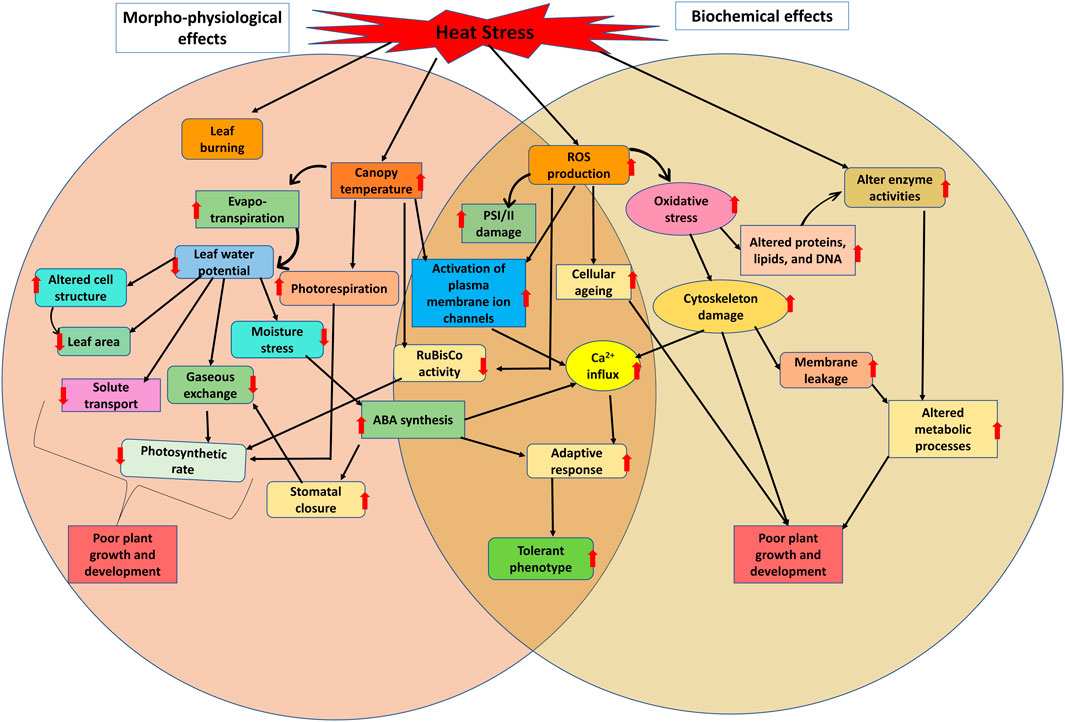
FIGURE 1. General overview of impact of heat stress on physiological and metabolic pathways including morphological changes in plants under heat stress condition.
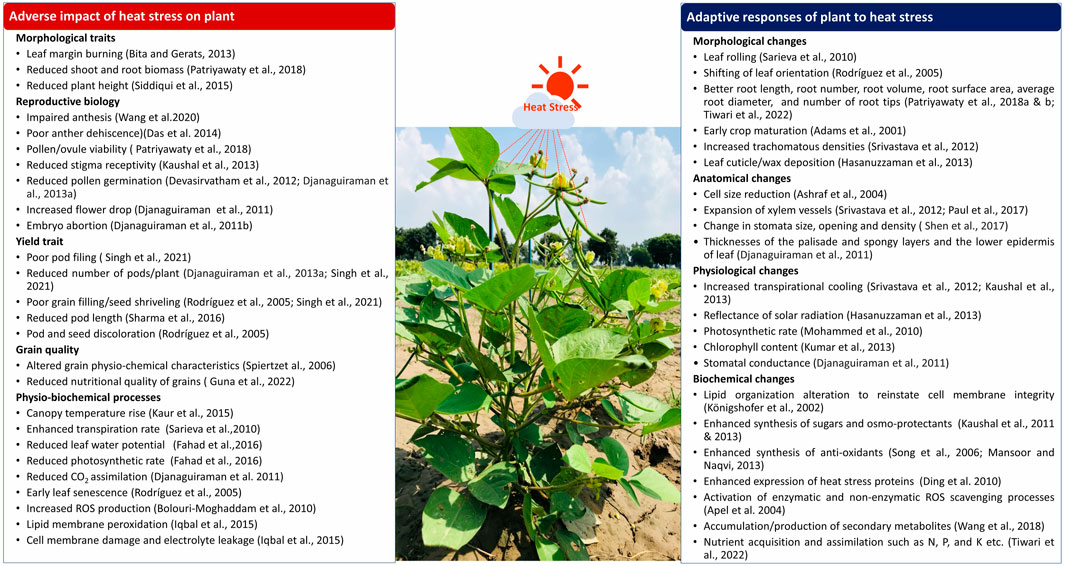
FIGURE 2. Impact of heat stress on morpho-anatomical features, reproductive biology and physio-biochemical properties of plants under heat stress. The corresponding adaptive responses of the plant under stress are also highlighted.
Reproductive organs are highly sensitive to high temperature stress. In mungbean, high temperature cause flower shedding as high as 79% (Kumari and Varma, 1983). However, the genotypic variability in mungbean germplasm is observed attributing to specific or combination of heat stress tolerance mechanisms (Kaur et al., 2015; Baroowa et al., 2016; Sharma et al., 2016). The effect of heat stress in mungbean is not thoroughly investigated yet, and it needs more in-depth research (Kaur et al., 2015).
2.1 Morphological and anatomical changes in mungbean in response to heat stress
Heat stress can cause a range of modifications at morphological and anatomical levels in plants such as scorching of leaves and stems, loss of leaves, inhibition in shoots and roots growth, shrinking of seeds, and damage to fruits. As a result of these alterations, it consequently lead to reduced crop productivity (Vollenweider and Günthardt-Goerg, 2005). In mungbean, heat stress causes many structural changes at morpho-anatomical level (Figures 3A–H). The different phases of reproductive stage such as pollen germination, loss of pollen viability, less pollen load on stigma, poor anther dehiscence, pollen sterility, and poor ovule viability lowers the crop yield (Kaushal et al., 2013). In a study by Kaur et al. (2015) on two mungbean genotypes (SML 832 and SML 668), similar results were observed in response to heat stress treatment. They also observed decrease in plant biomass (16%–19%), total number of pod set, seed yield (35%–40%), number of filled pods (32%–38%), and seed number (43%–47%), due to high temperature exposure.
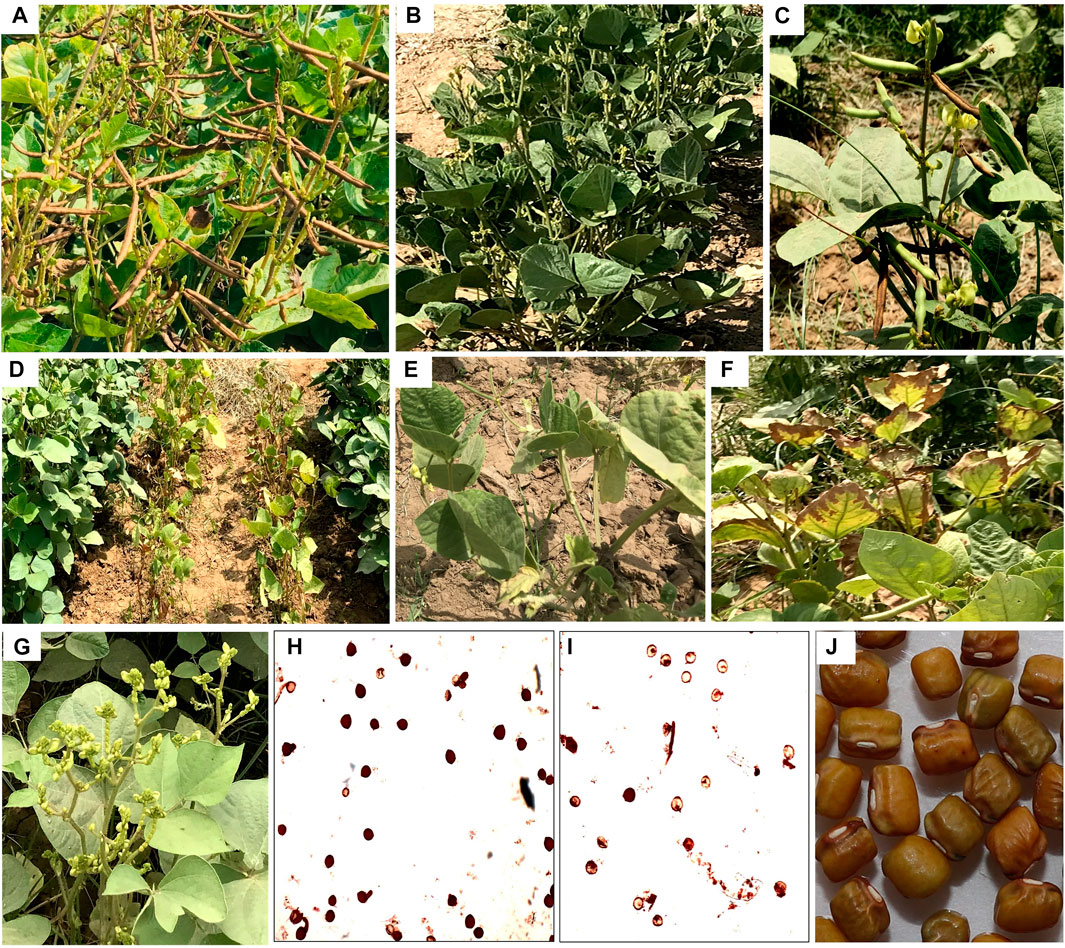
FIGURE 3. Typical morphological symptoms in mungbean plants in response to heat stress. Each genotype has a specific response to heat stress, e.g., flower drop, poor grain filling, pod discoloration, and leaf margin burning (A), upward curvature of leaves to protect plant from heat from sunlight (B), poor pod filling and reduced pod length (C), early onset of maturity (D), leaf abscission due to early onset of senescence to mobilize nutrients towards reproductive parts (E), leaf margin burning (F), almost entire flower drops due to high temperature during reproductive phase (G), heat tolerant genotype with better pollen viability (H) than the heat susceptible one (I), and seed shriveling due to heat stress during grain filling stage (J).
Failure of ovule fertilization is often associated with plant productivity factors such as loss of pollen viability, loss of stigma receptivity and reduced pollen tube growth (Hurkman et al., 2009; Patriyawaty et al., 2018). Pollen becomes often non-viable before fertilizing the flower (Figures 3I,J). It is also reported that the higher ambient temperature reduces the stigma receptivity to pollen causing embryo abortion and poor seed set (Kaushal et al., 2013). Reductions in seed set per pod are reported to be correlated with the poor pollen tube growth and tropism defects (Zinn et al., 2010). Moreover, heat stress can directly damage cell membranes, leading to changes in their permeability. The heat stress can alter the microtubules organization which not only affect the elongation, differentiation, and expansion of cells but also negatively impacts the cytoskeleton structure (Rasheed, 2009; Bita and Gerats, 2013). In addition, heat stress also causes decrease in pod length, seed quality, seed size, and number of seeds per pod which ultimately reduces grain yield and quality in mungbean. Similarly, Sharma et al. (2016) reported that heat stress-induced oxidative stress caused chlorosis, leaf rolling, and leaf blistering in mungbean plants.
Several studies have confirmed the susceptibility of mungbean to rising temperatures (Jha et al., 2017). High-temperature stress can have drastic impacts on plant growth and development, as well as on different physiological activities (HanumanthaRao et al., 2016). For instance, mungbean may lose vigor due to extended exposure to high temperatures results in limiting seedling growth and development (Kumar et al., 2011; Devasirvatham et al., 2012). Heat stress can also result in negative impacts on vegetative growth, including leaf senescence, chlorosis, necrosis, burning, and abscission, reduced internode elongation, and suppression of root and shoot development (Kaushal et al., 2016; Sharma et al., 2016). Other effects of heat stress on mungbean include leaf curling, plant wilting, yellowing and blackening of leaves, reduction in plant height, reduced number of branches, and biomass (Kaur et al., 2015). Figure 2 and Table 1 illustrate the impact of heat stress on mungbean’s morphological and anatomical traits.
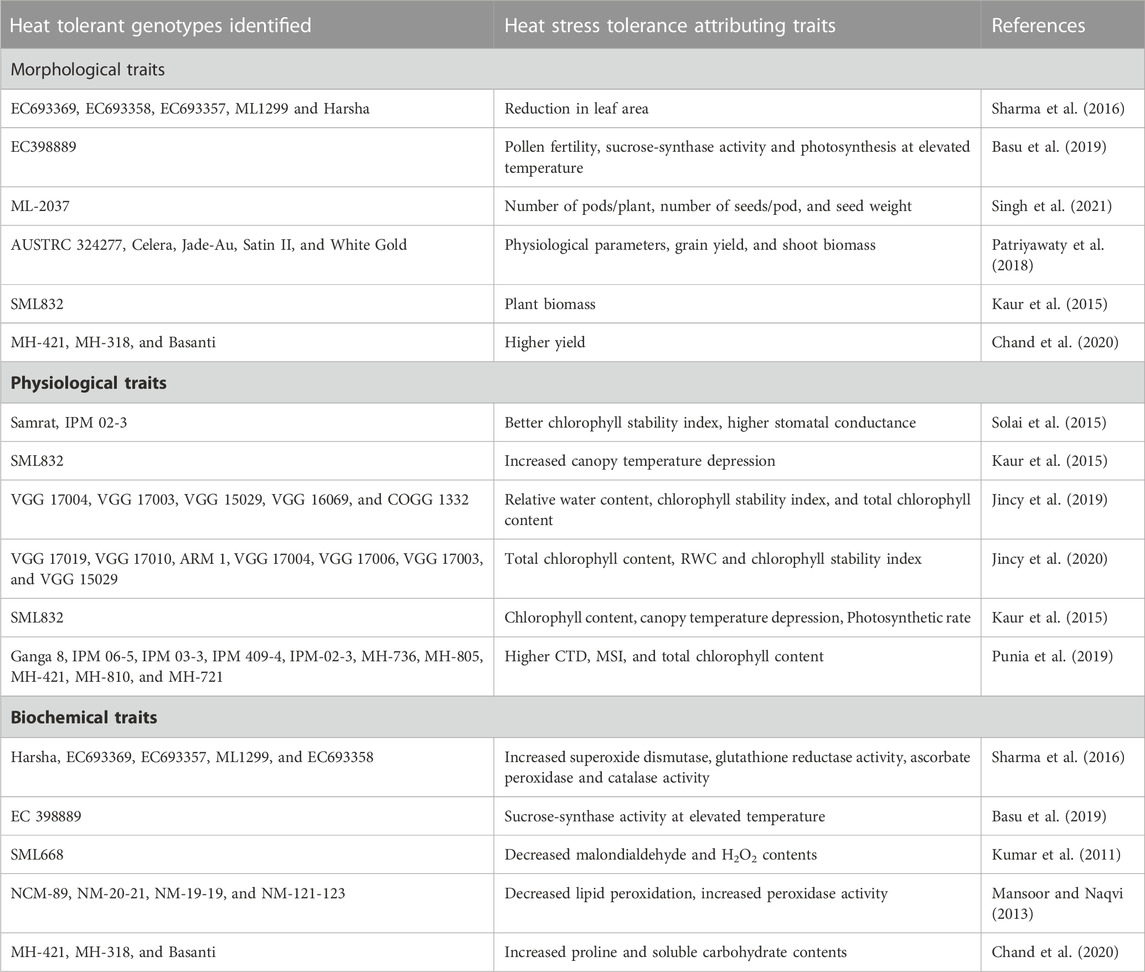
TABLE 1. Sources of heat stress tolerance identified in mungbean for various agro-morphological, physiological and biochemical traits.
2.2 Physiological changes in mungbean under heat stress
Heat stress can have several negative impacts on plant physiology, such as chlorophyll reduction, decreased photosynthesis, decreased transpiration, increased canopy temperature, and increased stomatal aperture (Schoffl et al., 1998; Kaushal et al., 2013). These effects can ultimately lead to reduced plant productivity. Additionally, heat stress can cause membrane damage, protein degradation, and altered metabolism in plant cells (Schoffl et al., 1998; Kaushal et al., 2013). Heat stress can also increase electrolyte leakage in plants due to altered membrane permeability by direct injuries, which affects the differentiation, elongation, and expansion of cells (Rasheed, 2009; Bita and Gerats, 2013). Further, structural alterations in chloroplast protein complexes and reduction in enzyme activity occur due to the initial impacts of thermal stress (Ahmad et al., 2010). Although a modest rise in ambient temperature generally promotes plant growth and development, it shortens the plant’s life span and results in a significant reduction in light uptake during the plant’s growth phase (Kalaji et al., 2016). Additionally, plant water status is a critical component of plant survival under heat stress. Plants try to normalize their canopy temperature through increased transpiration rate, and therefore, heat stress in combination with soil moisture stress proves to be most detrimental to the plant (Simões-Araújo et al., 2003). Unfortunately, most of the legume crops, including mungbean, are cultivated under rainfed conditions in tropical and sub-tropical regions. As a result, increasing global warming and adverse climatic conditions disrupt the monsoon seasons, resulting in uneven/less rainfall, which affects plant growth and development (Simões-Araújo et al., 2003). Heat stress can rapidly reduce tissue water content despite ample availability of soil moisture, similar to drought stress conditions, leading to a disruption in nutrient uptake from roots (Wahid et al., 2007). The drastic water loss due to high transpiration, particularly during the daytime, affects important physiological processes, ultimately resulting in reduced plant growth and development (Fahad et al., 2017). Heat stress can also reduce seed viability and lower plant and yield quality. Moreover, plants exhibit programmed cell death in some specific cells and tissues under heat stress (Anderson and Padhye, 2004).
In mungbean, the ideal temperature for growth and development is 28°C–30°C, and further every degree increase in temperature may reduce crop production by 35%–40% (Tzudir et al., 2014; HanumanthaRao et al., 2016; Sharma et al., 2016). Mungbean plant can thrive well up to 40°C of temperature, after which flower shedding begins (Zinn et al., 2010; Sita et al., 2017). Heat stress reduces leaf area and stomata openings which cause dramatic reduction in the carbon dioxide assimilation rate and photosynthesis during the vegetative stage of the mungbean (HanumanthaRao et al., 2016). The increase in carbon dioxide (CO2) content also causes stomatal closure, which hinders photosynthesis in the mungbean. High CO2 concentration along with high ambient temperature, proves more detrimental for mungbean growth and development (Reardon and Qaderi, 2017). In a study, CO2 assimilation in mungbean was significantly reduced at 40°C, which had a direct impact on photosynthetic efficiency (Karim et al., 2003). High temperatures also reported to reduce the chlorophyll and carotenoid levels, as well as the chlorophyll stability index (Chand et al., 2018). A slightly higher temperature (36°C) temperature treatment on mungbean genotypes indicated that the exposure of plants to high temperature has greater adverse impact on leaf conductance at the pre-flowering stage than the blooming and grain filling stages, although high-temperature treatments had no effect on transpiration rate at any stage, but photosynthetic activity decreased at all three stages (Islam, 2015). Similarly, another study on three prominent mungbean varieties viz., MH 421, MH 318, and Basanti indicated reduction in chlorophyll and carotenoid content, as well as a decrease in chlorophyll stability index in response to high temperature (Chand et al., 2018). Under heat stress, sensitive genotypes (MH 318 and Basanti) showed higher losses in the physiological attributes stated above, whereas tolerant genotypes (MH 421) maintained high yield and physiological functioning (Chand et al., 2018). Evidenced from numerous studies it is found that Photosynthesis is highly sensitive to high temperatures (Sinsawat et al., 2004). Heat stress affects photosynthetic functions in mungbean by disrupting photosynthetic machinery, causing structural aberrations (particularly the thylakoid membrane) and alterations of chloroplast enzymes. Based on mungbean germplasm screening for heat responsive traits various important promising mungbean donors are identified (Table 1). Also, impact of heat stress on physiological processes in various crops is listed in Table 1 and Figure 1, Figure 2.
2.3 Biochemical changes in mungbean under heat stress
High temperatures have a significant impact on the metabolism and biochemistry of mungbean plants. Under heat stress, the formation of reactive oxygen species (ROS), such as hydroxyl radical, singlet oxygen, superoxide radical and hydrogen peroxide, increases, causing protein degradation, membrane damage, and enzyme inactivation, and hence increases oxidative stress (Liu and Huang, 2000). Long-term exposure to relatively high temperature stress can result in severe cellular injury or death, while at extremely high temperatures, this can occur in just minutes (Wahid et al., 2007). These injuries, coupled with a lack of water content, can cause a reduction in ion flow and plant growth, as well as an increase in the generation of toxic compounds and reactive oxygen species (Howarth, 2005). Some of the metabolic effects of heat stress on mungbean plants are briefly described in Figure 1 and Table 2.
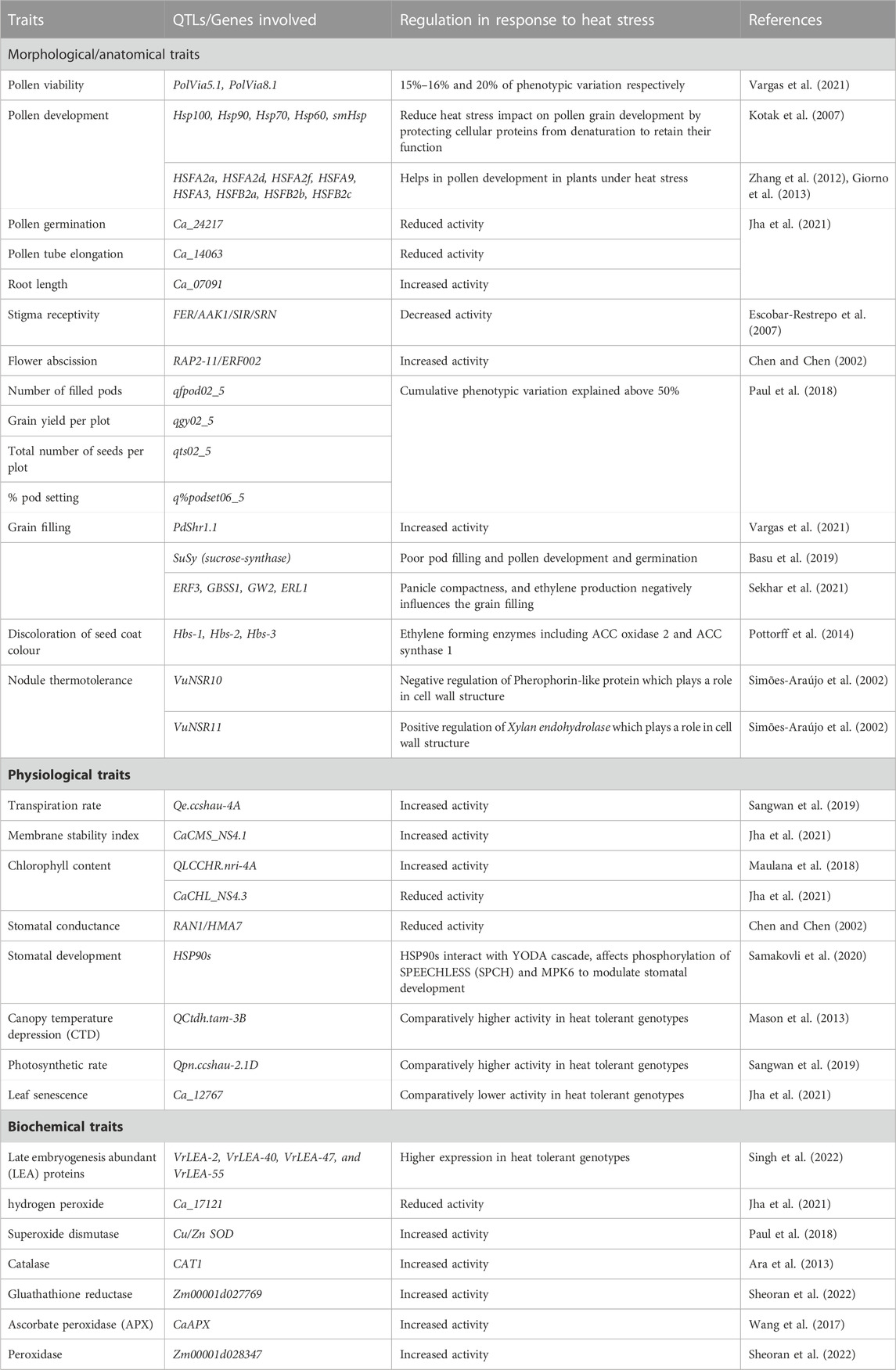
TABLE 2. Candidate genes identified under heat stress tolerance in plants for various agro-morphological, physiological and biochemical traits
In mungbean, high temperature especially >40°/30°C (max/min) reduces leaf water potential and increases oxidative stress resulting in growth suppression and chlorosis which is linked to reduced crop yield (Kumar and Wigge, 2010). High temperature stress can cause a considerable increase in hydrogen peroxide (H2O2) concentration in mungbean as well as in other plants, which could be due to a reduction in catalase activity by blocking catalase production which lowers the enzyme’s steady-state level because of high turnover rate (Scandalios et al., 1997). When plants are exposed to high levels of heat, they experience an oxidative burst (N, 1997) that can cause an increase in H2O2 (Levine et al., 1994; Baker and Orlandi, 1995). Antioxidant enzymes such as SOD and CAT become less active during heat shock, compromising the plant’s defenses and leading to increased levels of oxidant species (Willekens et al., 1995; Foyer et al., 1997; Polle, 1997). This can have a negative impact on cellular metabolism, resulting in high levels of harmful compounds like malondialdehyde and H2O2 that affect plant productivity (Bita and Gerats, 2013). Lipid peroxidation under aerobic conditions, is a natural metabolic process that can be affected by ROS, causing damage to the cell membrane and impairing its function (Blokhina et al., 2003). (Heath and Packer, 1968). The presence of malondialdehyde (MDA) is an indication of oxidative damage, and it is created by the lipid peroxidation of the cell membrane (Mandhania et al., 2006). It has been reported that high temperature stress on four mungbean genotypes (NCM 89, NM 20-21, NM 121-123, and NM 19-19) at seedling stage observed an increase in the levels of lipid peroxidation as there is a considerable increase in MDA concentration (Mansoor and Naqvi, 2013). Studies in seedlings of four mungbean genotypes have shown that an increase in temperature can cause an increase in lipid peroxidation, leading to decreased net photosynthesis, water use efficiency, stomatal conductance, total chlorophyll, and nutrient partitioning in sensitive genotypes. Additionally, heat stress can negatively impact assimilate partitioning and apoplastic to symplastic phloem transport, resulting in a decrease in carbohydrate buildup and viability of pollen grains in mungbean (Kaur et al., 2015; Taiz et al., 2015; Hanif and Wahid, 2018).
Seed shriveling in legumes is primarily linked to reduced synthesis of carbohydrates and storage proteins due to elevated temperature. The four enzymes viz., Adenosine Diphosphate Glucose Pyrophosphorylase, Starch Branching Enzyme, Starch Synthase, and Sucrose Synthase are crucial for the grain filling process (Taiz et al., 2015). In general Sucrose synthase plays in important role in grain filling (Basu et al., 2019), and temperature beyond a certain period affects the enzyme activities and lead to poor grain filling. Similarly, the functioning of Nitrate reductase is also diminished during heat stress, which is a most important enzyme of nitrogen (N) metabolism in plants. It catalyzes the reduction of inorganic N in the form of nitrate to organic form in translational process of proteins. Heat stress is known to adversely affect the nitrate reductase enzyme activity, which is more detrimental for leguminous crops affecting protein biosynthesis during grain filling and reproductive stages (Klimenko et al., 2006; Farooq et al., 2017). Heat stress also impairs the nitrogen-fixing activity of mungbean by restricting the production of root hair and infection thread (Bansal et al., 2014). Furthermore, heat stress reduces seed viability, plant and seed quality (Anderson and Padhye, 2004). The nutritional value of seed is impaired mainly due to adverse impact of heat stress on synthesis and accumulation of protein and carbohydrates (Taiz and Zeiger, 2010).
3 Adaptation strategies in mungbean to develop heat tolerant genotypes
Mungbean plant deploy a number of adaptation mechanisms such as changes in plant morphological and anatomical features, secondary metabolite production and their accumulation in target tissues/cells, production of anti-oxidants, stress hormones and proteins, and alteration in myriad of physiological activities (Figure 2). The adaptation mechanisms that result in thermal tolerance in plants include secondary metabolites, heat-shock proteins (HSPs), ROS scavenging systems, and accumulation of suitable solutes (Nakamoto and Hiyama, 1999; Wahid et al., 2007; Mittler et al., 2012; Hanif and Wahid, 2018). During heat stress, ROS produced by aerobic metabolism have a deleterious impact on cellular catabolism, causing lipid membrane peroxidation as well as damage to proteins and nucleic acids (Bita and Gerats, 2013). To tolerate high temperatures, plants need to have sufficient levels of antioxidants (Awasthi et al., 2015). When subjected to heat stress, plants generate and obtain new stress proteins, such as HSPs. These HSPs are molecular chaperones that play a crucial role in protein folding, translocation, proper aggregation, and degradation under both normal and stressful conditions (Vierling, 1991). HSP100, HSP90, HSP70, HSP60, and the small heat-stress protein (sHSP) family (Wang et al., 2004) are five heat-stress protein/chaperone families that play an important role in mitigating heat-stress, including protecting native proteins against denaturation. Isoprenoids, flavonoids, and carotenoids are secondary metabolites that inhibit peroxidase activity and promote high-temperature stress tolerance (Havaux, 1998; Loreto et al., 1998; Rivero et al., 2004). When plants are exposed to heat stress, they undergo various interconnected morpho-physiological, biochemical, and molecular changes unique to their tolerance and adaptive nature to their surrounding environment, ultimately enabling them to restore redox homoeostasis (Waseem et al., 2011) (Figure 4). This indicates the necessity in developing heat stress tolerance in mungbean cultivars. Mungbean plants have adapted various types of defense mechanism to survive the heat stress, which are discussed in the following sections. Sources of tolerance identified by various workers are highlighted in Table 1.
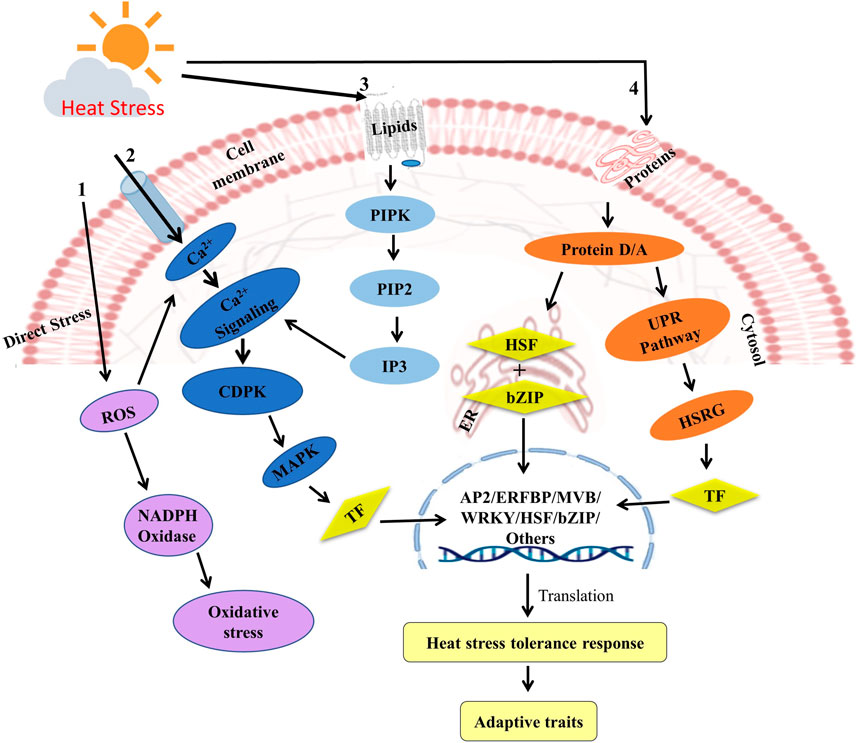
FIGURE 4. Different signaling and defense pathways in response to heat stress in plants. High temperature stress effects the plasma membrane to activate the calcium channels, which induces Ca2+ influx, thus the MAPK cascade regulates and activates the various transcriptional factors which ultimately leads the accumulation of the stress responsive genes, the antioxidants and ROS.
3.1 Morphological and anatomical adaptations involved in mungbean response to heat stress
Increase in atmospheric temperature beyond a certain optimum point drastically reduces the plant growth and development as cellular processes require a specific temperature range. Plants have evolved with a great variability in their morphological adaptation as a part of survival mechanisms, such as early maturation, leaf rolling, and changing leaf orientation to cope up with the certain level of temperature deviations (Srivastava et al., 2012). However, these adaptation mechanisms are often associated with yield losses under high temperature in many crop plants (Adams et al., 2001; Rodriguez et al., 2008). Further rise in atmospheric temperature causes development of symptoms such as scorching of leaves and twigs, shoot and root growth inhibition, early onset of leaf senescence and abscission, and pod discoloration as an adaptation for plant survival. In response to heat stress, plant height is reduced in mungbean, which is specifically attributed to the disruption in cell elongation. This demonstrates that the mungbean plants exhibit the adaptation mechanism to respond heat stimuli. Certain important differences can be detected in the parameters like 100 seed weight, seeds/plant, pods/plant, seed yield, total biomass/plant, branches/plant, and plant height, etc. In mungbean as adaptation traits (Sharma et al., 2016) (Table 1). The reproductive phase of plant growth and development is highly sensitive to heat stress. To overcome certain level of higher temperature, plant deploy two very crucial mechanisms of plant defense viz. Dehydration of pollen grains and embryos, resulting in stress tolerance in the pollen grains, and prolonged dormancy in embryos under stressful conditions (Zinn et al., 2010). As a result, pollen and embryos remain viable under higher temperature, however, the magnitude of tolerance varies among genotypes. Therefore, these traits are considered as important for development of heat-tolerant mungbean varieties (Rainey and Griffiths, 2005).
In a study effects of heat stress on 41 mungbean genotypes for their vegetative and reproductive activities under controlled growing conditions was examined and a few tolerant lines for stress were identified (Sharma et al., 2016). A field study in New Delhi in the Kharif seasons of 2014 and 2015 was conducted to assess the impact of high temperatures on seven mungbean genotypes in a rain-fed environment, and various heat stress responsive morphological traits were identified (Chikukura et al., 2017). Night temperature rise is considered as more crucial for flower drop, as it is observed that under the higher temperature stress, the flower abscission is higher during the night as compared to the daytime (Solai et al., 2015). Under high temperature stress, plants maintain their metabolites level required for flower and pollen and anther development. According to Banon et al. (2004), the reduction of cell size and the closure of stomata can help to decrease excessive water loss and lead to increased stomatal density and enlarged xylem vessels in plants under heat stress. However, Shen et al. (2017) have noted that the anatomical changes in response to heat stress can vary among different species. Some of the important morphological, physiological, biochemical and anatomical responses of mungbean genotypes to heat stress tolerance are highlighted in Figure 1, Figure 2 and Table 2.
3.2 Physiological adaptation in response to heat stress
There are few studies on how high temperature stress affects stage-specific functional physiology in legumes from post-flowering to blooming. The reproductive stage is destined to be more impacted by and prone to temperature vagaries due to its delicate organelle constitution, even if heat stress sensitivity in plants changes with plant growth. Depending on the species and genotype, there are significant inter- and intra-specific variations that affect the response under heat stress (Sakata and Higashitani, 2008; Bita and Gerats, 2013).
The persistence of photosynthesis in plants when they are exposed to stress conditions is supported by the stay-green (SGR) trait, which is also known as delayed leaf senescence. The maintenance of photosynthetic activity in plants under stressful conditions is facilitated by the stay-green (SGR) trait, also referred to as delayed leaf senescence (de Souza Luche et al., 2015). Understanding the role of SGR in plants could lead to improved plant production and productivity (Thomas and Ougham, 2014). During grain filling, the SGR characteristic allows plants to continue photosynthesizing by creating a senescence pattern that increases the amount of sugar produced by photosynthesis (Pinto et al., 2016). According to recent research, SGR wheat genotypes demonstrated improved tolerance to high temperatures due to enhanced structural stability of the photosynthetic apparatus and lower accumulation of harmful reactive oxygen species (Tian et al., 2012). The cultivar “Mairaj-2008” has also demonstrated superior ability to grow under heat stress compared to cultivars that lack the SGR trait and are more susceptible to heat damage (Nawaz et al., 2013). Canopy temperature depression (CTD) could serve as a useful tool for selecting heat-tolerant genotypes based on observable differences in their traits (Mason and Singh, 2014). Studies have shown that lower canopy temperatures promote better yield potential in wheat exposed to elevated temperatures, and CTD is effective in mitigating heat stress in wheat (Amani et al., 1996; Fischer et al., 1998; Mason et al., 2013). Examination of stomatal behavior under stress conditions can be performed using a leaf porometer, which measures fluctuations in gaseous exchange rate that trigger stomatal opening (Chandra et al., 2017). High-yielding cultivars with fully opened stomata have increased transpiration rates, improved CO2 and water vapor diffusion, and enhanced photosynthetic efficiency (Condon et al., 2007).
Chlorophyll fluorescence (ChlF) is a non-invasive marker for photosystem II (PSII) quantum efficiency and can be used to assess early stress in plants, making it a useful tool for investigating plant heat stress tolerance (Sharma et al., 2014; Kalaji et al., 2016). Studies have found that genotypes with high ChlF values, such as the heat-tolerant line RRR46 of common bean, outperform other lines when exposed to heat stress, indicating their potential for future breeding programs (Stefanov et al., 2011). Membrane stability is another important physiological trait that can affect heat tolerance in plants, and higher membrane stability during grain filling has been shown to increase heat tolerance in wheat (Gupta et al., 2013; Ramani et al., 2017).
Flag leaf photosynthetic efficiency has also been found to play a role in heat stress tolerance in crops. For example, the wheat cultivar “Jimai22”showed increased yield under heat stress and demonstrated PSII stability and significant carboxylation activity (Feng et al., 2014). Heat-tolerant mungbean and lentil genotypes have also been found to exhibit better photosynthetic efficiencies under heat stress than heat-sensitive genotypes (Sharma et al., 2016; IG3263, respectively). Conversely, decreased cellular thermostability in rice has been linked to potential reductions in crop output (Maavimani and Saraswathi, 2014). Overall, physiological traits such as ChlF, membrane stability, and photosynthetic efficiency can provide important insights into plant heat stress tolerance and can be used to identify promising genotypes for breeding programs. A comprehensive list of mungbean genotypes based on physiological traits under heat stress tolerance is provided in Table 1.
The traditional theme of research on legume reproduction has tremendously focused on vegetative stage of the plants rather than their physiological and molecular mechanisms underlying legume crop reproductive heat tolerance (Lin et al., 1984; Valliyodan and Nguyen, 2006; Kumar, 2012; Sita et al., 2017). However, recent studies have shown a growing interest in understanding these mechanisms (Devasirvatham et al., 2012; Kaushal et al., 2013; Sita et al., 2017; Patriyawaty et al., 2018). Despite this, there is still a lack of knowledge regarding the specific physiological and molecular mechanisms that allow legume crops to be resilient to heat stress during the reproductive stage (Devasirvatham et al., 2012; Kaushal et al., 2013; Sita et al., 2017; Patriyawaty et al., 2018). One possible explanation for this lack of understanding is that many legume cultivars are difficult to transform genetically, which limits the availability of transgenic evidence (Young and Udvardi, 2009; Song et al., 2013). However, on the basis of current research on other crop groups, particularly cereals, it is possible to suggest physiological and molecular explanations for legumes’ ability to withstand heat stress (Devasirvatham et al., 2012; Kaushal et al., 2013; Sita et al., 2017; Patriyawaty et al., 2018).
3.3 Biochemical adaptation mechanisms involved in mungbean against heat stress
Plants have evolved with a number of biochemical adaptation mechanisms that allow them to tolerate high temperature stress, for example, secondary metabolites, ROS scavenging system and heat-shock proteins (HSPs) (Nakamoto and Hiyama, 1999; Sakamoto and Murata, 2002; Wahid et al., 2007; Mittler et al., 2012). Plants use both non-enzymatic and enzymatic ROS scavenging defense mechanisms to combat ROS production. Non-enzymatic antioxidants such as glutathione (GSH) and ascorbic acid (ASC) collaborate with enzymatic antioxidants such as ascorbate peroxidase (APX), Catalase (CAT), superoxide dismutase (SOD), peroxidase (POX), and glutathione reductase (GR) to maintain high antioxidant levels required for plant heat tolerance. (Suzuki et al., 2012; Awasthi et al., 2015). Isoprenoids, flavonoids, and carotenoids are secondary metabolites that inhibit peroxidase activity and promote high-temperature stress tolerance (Havaux, 1998; Loreto et al., 1998; Rivero et al., 2004). Chickpea grown under high-temperature stress at 35/25 and 45/35°C (day/night, 12 h/12 h) conditions exhibited increased levels of antioxidants such as proline and glutathione (Kumar et al., 2011). The non-enzymatic and enzymatic antioxidant pools are the most efficient and prominent defense mechanisms used by plants. Antioxidants with low molecular weight decrease oxidants without causing significant pro-oxidant activity.
The enormous generation of ROS occurs during heat stress which destroy proteins, lipids, nucleic acids, and carbohydrates (Bita and Gerats, 2013). Mungbean plants can protect themselves from ROS by activating various types of non-enzymatic and enzymatic defensive mechanisms in different cells in plants (Bita and Gerats, 2013). In an experiment under field conditions for heat stress, out of forty-one mungbean genotypes, only a few were found to be heat-tolerant viz., EC693357, ML1299, EC693369, Harsha, and EC693358 which suffered less oxidative damage than heat sensitive mungbean genotypes (Sharma et al., 2016) and when compared to susceptible genotypes, there was increased APX activity in heat resistant genotypes. However, the activity of CAT was increased in both heat-resistant and heat-tolerant genotypes (Sharma et al., 2016). As a result, mungbean genotypes that can withstand under heat stress during reproductive stages could be chosen for screening growth and productivity analysis. Some supplements, such as Ca, K, Mg, and N have been shown to reduce the harmfulness of ROS in plant cells by increasing anti-oxidants such as POD, SOD and CAT (Waraich et al., 2012). Mungbean genotypes can be chosen based on the quantity of enzyme expression, with stress tolerant genotypes having more prominent activities than sensitive genotypes for breeding stress tolerant mungbean cultivars (Kumar et al., 2013).
Aside from the anti-oxidative system, accumulation of various appropriate protective osmolytes such as sugar and their derivatives (polyols), ammonium-based products, proline, and some compounds of sulphonium-based derivatives have been shown in the protection and/or repair of molecules and structures damaged by ROS, as well as in ROS sequestration (Sairam and Tyagi, 2004; Miller et al., 2007). As a result, osmotic adjustment is considered as one of the most promising mechanisms for drought and heat which can be accomplished by accumulating appropriate solutes (e.g., glycine betaine and proline) in protoplasm (Chaves et al., 2003; Bartels and Sunkar, 2005). Proline is one of the prominent osmo-protectant, which also play a crucial role in cellular homeostasis. It also acts as a signaling molecule in triggering specific gene expression, in cell proliferation or cell death, and to alter mitochondrial functions. These are crucial processes for plants’ recovery after stress. Certain natural combinations of osmolytes are formed under abiotic stress conditions such as salt, drought, and heat (Hare et al., 1998; Sakamoto and Murata, 2002). Plants may protect themselves by the accumulation of these solutes in order to increase the tolerance up to certain circumstances. These osmolytes help in increasing the stability of membrane bilayer and proteins. Defensive molecules such as flavonoids, anthocyanin, and plant steroids have been recognized as secondary metabolites that contribute to heat stress tolerance in plants. In response to heat stress, plants also increase their levels of several major phytohormones including ethylene (ET), salicylic acid (SA), and abscisic acid (ABA) to enhance their tolerance. Evaluation of these plant hormones in response to heat stress involves measuring various biochemical and physiological parameters, as well as assessing growth regulating parameters such as photosynthesis and biomass. Additionally, other hormones like auxin, gibberellins (GAs), cytokinins, abscisic acid, brassinosteroids (BRs), and jasmonic acid (JA) have been identified as contributing to heat stress tolerance in plants, as noted by various studies (Peleg and Blumwald, 2011; 2011; Mittler et al., 2012; Zhou et al., 2014; Dobrá et al., 2015; Xia et al., 2015).
3.4 Production of heat shock and regulatory protein in response to heat stress
Plants possess an adaptive mechanism to manage heat stress via the production of stress proteins like heat shock proteins (HSPs). These HSPs act as molecular chaperones and assist in activities like protein folding, translocation, aggregation, and degradation in both regular and stressful environments (Vierling, 1991). When subjected to sudden or constant temperature variations, plants increase their HSP production (Schoffl et al., 1998; Nakamoto and Hiyama, 1999), highlighting the critical nature of HSPs when it comes to temperature stress across all species (Vierling, 1991). Plants in dry and semi-arid regions can synthesize and store HSPs in large quantities. HSPs are only present during specific developmental stages like pollen formation, germination, fruit maturation, and embryogenesis. These proteins protect other proteins from denaturation that could be caused by high temperatures. In sensitive organs and tissues where HSPs accumulate quickly, they can safeguard the metabolic system of the cells, which makes them crucial in a plant’s stress response and overall survival. Numerous research studies have established a close relationship between the development of heat tolerance and the synthesis and buildup of HSPs. An instance of this is the elevated expression of HSP68, which is typically expressed constitutively in mitochondria, when potato, maize, tomato, soybean, and barley cells were placed under heat stress (Neumann et al., 1993). HSP70, extensively studied, is believed to partake in several functions, including but not limited to the folding or assisting of proteins, translocation, protein translation, proteolysis, inhibition of aggregation, as well as the restoration of denatured proteins (Zhang et al., 2005). Many plant species necessitate the induction of HSP70 for heat tolerance of cells and tissues post heat stress. Additionally, HSP101 has been deemed vital (Schoffl et al., 1998). Furthermore, different tissues within the same plant manifest diverse abilities to produce specific proteins at 40°C and the magnitude and duration of the synthesis also differ. Five different types of heat-stress protein/chaperone families are reported; these are HSP100, HSP90, HSP70, HSP60 and the small heat-stress proteins (sHSPs) family (Wang et al., 2004), that play an important role in heat-stress mitigation, including protecting native proteins against denaturation.
3.5 Mitigating heat stress through agricultural practices
Along with development of tolerant mungbean genotypes integration of heat stress mitigation strategies through appropriate agricultural practices will have greater impact on sustaining the mungbean production amid rising global temperature. Agricultural practices such as timely sowing of crop, selection of early short duration genotypes to escape peak heat stress period, seed priming to enhance the seed vigour and initiate heat tolerance defense mechanisms, irrigation management to enhance water use efficiency and maintain plant water potential, mulching and enhancing organic material to enhance soil water retention and reduce soil temperature. Mungbean sown during summer/spring season, particularly in northern part of India, face more threat of heat stress during later stage of crop growth (HanumanthaRao et al., 2016). To overcome the terminal heat stress, early maturing mungbean cultivars with early seedling vigour are very crucial for sustaining mungbean production. Majority of mungbean cultivars matures in 60–70 days, however, the evaluation of mungbean collections reveals availability of mungbean germplasm which matures within 50 days of sowing (Gayacharan et al., 2020). Seed priming is another promising tool for induction of artificial stress memory response in terms of accumulation of secondary metabolites, antioxidants, improved water plant water potential, etc. (Ahmad et al., 2021; Chakraborty and Dwivedi, 2021; Tamindžić et al., 2023). Utilization of heat tolerant mungbean cultivars may further help in mitigating impact of heat stress in mungbean cultivation. Mungbean cultivars such as Pusa Vishal, SML 668, IPM 99-125, and few more listed in Table 1 are helping in sustaining mungbean cultivation during the summer/spring season in northern parts of India.
4 Signaling pathways/factors involved in heat stress tolerance in plants
Plants can detect even a slight deviation in temperature because of the sensing mechanism present on cell membrane through change in fluidity of membrane bilayer. This leads to the conformational changes and post-translation modifications such as phosphorylation/dephosphorylation processes (Kaushal et al., 2016; Sehgal et al., 2016). In response to heat stress plants activate various signaling pathways that lead to the induction of heat stress tolerance genes. There are four major prominent signaling pathways viz. ABA, MAPK, ROS and Ca2+. Under high temperatures, ABA levels increase in plant tissues, leading to the activation of stress specific ABA signaling pathway. The ABA signaling pathway operates through ABA binding to receptors, which subsequently engage phosphatases and kinases to regulate downstream gene expression. In plants, this pathway triggers the activation of heat stress tolerance genes, such as HSP70, HSP90, and HSP101 (Batcho et al., 2020; Priya et al., 2020). Similarly, the MAPK signaling cascade plays a critical role in activating MAPKs, which then phosphorylate transcription factors and trigger the induction of stress-responsive genes such as HSP17, HSP26, and HSP70 under heat stress in mung beans (Wang et al., 2021). Additionally, the ROS signaling pathway has been linked to HSP70 and HSP90 induction under heat stress conditions, and it also interacts with other pathways like ABA and MAPK to regulate gene expression (Banti et al., 2010).
Calcium (Ca2+) is a ubiquitous secondary messenger that plays a critical role in plant stress responses, including heat stress. Under high temperatures, the heat shock reaction (HSR) is initially triggered by the detection of temperature increase by plasma membrane which then activates ion channels like Ca2+ channels and induces an inward flux of Ca2+ into cells (Bokszczanin et al., 2013). According to several mechanisms reported, blockage of calcium channel or chelators causes inward flux of Ca2+ ions which is a significant signal of heat stress. Ca2+ interact with downstream targets, such as calmodulin and calcium-dependent protein kinases (CDPKs), leading to the activation of heat shock transcription factors (HSFs) and several other transcriptional factors such as WRKY39 and stress-responsive genes (Li et al., 2011; 2010). In addition, this Ca2+ influx induces the activation of another signaling cascade system including calcium-dependent protein kinases (CDPKs), mitogen-activated protein kinases (MAPKs), and NADPH oxidase, all of which cause the generation of ROS (Sangwan et al., 2002; Suzuki et al., 2011) (Figure 4). These different signaling and defensive pathways are evolutionary conserved processes in legumes and work similarly in almost all of the legume crops. In plants, the Ca2+ signaling pathway has been shown to be involved in the induction of heat stress tolerance genes, such as HSP70 and HSP90 (Zheng et al., 2020).
Heat stress activates various other signaling molecules such as PIPK (phosphatidylinositol-4-phosphate-5-kinase), PLD (phospholipase-D), phosphatidic acid, IP3 (D-myo-inositol-1,4,5-triphosphate), and PIP2 (phosphatidylinositol-4,5-bisphosphate). In plants, the activation of two UPR (Unfolded protein response) signaling pathways is triggered by heat stress, one in ER and the other in cytoplasm (Sugio et al., 2009; Pincus et al., 2010; Deng et al., 2011). This leads to proteolytic cleavage in the membrane of the endoplasmic reticulum and activates different bZIP transcription factors (Tfr). (Che et al., 2010; Deng et al., 2011). The accumulation of chaperones in ER, along with calcium signaling, activates brassinosteroid signaling, which in turn activates the transcription of heat-tolerant genes (Che et al., 2010). In cytosol, the unfolded proteins activate the cytosolic UPR pathway for HSF and HSFA2 transcription factors to induce downstream heat stress responsive genes (Sugio et al., 2009).
Plants also use phytohormones, such as ABA and brassinosteroids, as well as signaling molecules like nitric oxide to help them tolerate heat stress (Hasanuzzaman et al., 2011; Asthir, 2015). Studies have shown that applying exogenous ABA to Phragmites communis led to a decrease in MDA and H2O2 content and an increase in POX, APX, CAT, and SOD levels, which suggests less oxidative damage in treated plants compared to non-treated plants (Ding et al., 2010). Similarly, spraying BRs on Phaseolus vulgaris increased yield and quality of pods, total phenolic acids in pods, and vegetative growth through the BRs signaling pathway (El-Bassiony et al., 2012). Salicylic acid (SA) is also an important signaling molecule that influences plant growth and development under stress conditions and can act as a protectant under heat stress (Yuan et al., 2008; Hasanuzzaman et al., 2013). Applying SA can increase enzyme activity, carotenoid and chlorophyll levels, photosynthetic rates, ion uptake, flower induction, plant growth, and thermogenesis, and affect the ethylene biosynthesis pathway (Bajguz and Hayat, 2009).
Under heat stress tolerance, nitric oxide (NO) is considered as an another important signaling molecule that regulates various morpho-physiological and biochemical processes in a systematic concentration-dependent manner and acts as a redox-related signaling molecule in legumes (Hasanuzzaman et al., 2013; 2012; 2011; Waraich et al., 2012; Fancy et al., 2017). Exogenous treatment of NO on heat-stressed plants increases the stability and shelf life of chlorophyll molecules, decreases H2O2 content, and increases antioxidant enzyme activities by enhancing sodium nitropruside levels in plants (Yang et al., 2006).
5 Molecular markers and candidate genes associated with heat stress tolerance in plants
The traditional breeding programs are crucial for the identification of stable genetic and genomic resources for their introduction into elite cultivars in addition to advances in biotechnological and molecular approaches (Cabello et al., 2014). Recent efforts on developing new breeding methods have not been enough to cultivate heat tolerant varieties (Grover et al., 2013). Therefore, development of heat tolerance variety is limited through breeding approach. In order to fill this gap, a unique approach has been adapted by breeders for cultivation of stress tolerance variety which is beneficial for developing stress tolerant genotypes with high productivity by interpreting the genomic regions on chromosomes responsible for tolerance (Driedonks et al., 2016). Mungbean is one of the legumes which has rarely received the application of new breeding tools (NBTs) and technologies for understanding the basic tolerance mechanism. However, recent advancement in genome sequencing technologies and their implication in crop research programs has also helped in generating genomic resources in mungbean. Currently there are two genome assemblies (Vradiata_ver6, ASM158444v1) with full genome representation and one assembly (ASM18089v1) with partial genome representation (Tangphatsornruang et al., 2009; Kang et al., 2014; Liu et al., 2016). The genome sequence information is helping in understanding the underlying molecular mechanisms involved in trait expressions.
The utilization of genome sequencing data and advanced genome annotation tools has facilitated the identification of key candidate genes that play essential roles in heat stress tolerance. The genes HSP60, HSP70, HSP90, HSP100, and smHSP are well-known molecular chaperones that are induced by heat stress and are critical in safeguarding plants against heat damage (Kotak et al., 2007; Mishra et al., 2018). Additionally, CBF/DREB1 protein family members and LEA proteins have also been identified as stress-responsive proteins that protect plants from abiotic stresses, including heat stress (Li et al., 2020). Another group of stress-responsive protein that is involved in protecting plants from heat stresses is LEA (Late embryogenesis abundant). In mungbean, some LEA genes viz. VrLEA-55, VrLEA-47, VrLEA-40, and VrLEA-2 were identified by Singh et al. (2022), and reported their upregulation under heat stress conditions. Furthermore, genes encoding antioxidant enzymes such as APX, CAT, and SOD have been identified as significant candidates that aid in scavenging reactive oxygen species under stress conditions. A comprehensive list of identified candidate genes responsible for heat stress tolerance in various plant species is presented in Table 2.
Plant breeding has become more efficient with the aid of Marker aided selection (MAS), however, simple sequence repeats (SSRs) and single nucleotide polymorphisms (SNPs) are commonly used for quantitative trait loci (QTL) analysis in breeding programs (Das and Rao, 2015). Recent advances report that novel breeding approaches utilizing QTL mapping have been effective in developing heat stress tolerance in plants (Jha et al., 2014; Shamsudin et al., 2016). Several QTLs for various traits associated with heat stress tolerance have been identified in different crops. For example, in cowpea, foundation genomic areas linked to heat stress tolerance were identified using SNP markers in a RIL population (CB27 9 IT82E-18), while QTLs associated with browning of seed coats were also discovered (Lucas et al., 2013; Pottorff et al., 2014). In lentil, two QTLs (qHt-ps and qHt-ss) were characterized, which were linked to heat stress tolerance in pod set and seedling survival (Singh et al., 2016). Similarly, in chickpeas, eight QTLs were identified, four of which were located on the CaLG05 genomic region, for pod set, pod filling, seed number, and grain yield with a combined phenotypic variation of up to 50% under heat stress tolerance, while as the remaining QTLs were located on the CaLG06 genomic regions. (Paul et al., 2018). Jha et al. (2021) identified 37 major and 40 minor QTLs for heat stress tolerance using an inbred population in chickpea. They also identified 32 potential candidate genes in the QTL regions that encode HSPs and HSFs and are involved in the regulation of flowering time and pollen development.
Similarly, in non-legume crops such as wheat plants, QTLs associated with grain filling and leaf senescence were reported on chromosome number 5A and 1B (Yang et al., 2002; Mason et al., 2010). Nine QTLs for tillering and grain filling, and three QTLs for green color were also identified in wheat (Kumar and Wigge, 2010). In maize, six QTLs for pollen tube growth and five QTLs for pollen germination, as well as six QTLs for cellular membrane stability, were detected using RFLP mapping technique (Ottaviano et al., 1991; Frova and Sari-Gorla, 1994). These QTLs and genes are evolutionarily conserved and exhibit mostly similar function in all legumes and other crops. A brief summary of different QTLs/genes associated with heat stress tolerance in legumes/crops is mentioned in Table 2. Currently, the identification and characterization of marker genes associated with heat stress tolerance studies have gained more interest and require better attention and understanding in this field.
6 Conclusion and future perspective
Mungbean is a short-duration crop that thrives in a variety of soils and climates. However, its cultivation is increasingly being influenced by heat stress all over the world. To overcome drastic rise in global temperature amid climate change, a comprehensive and holistic approach is required to sustain crop production. The existing variability in ex situ collections conserved in seed genebanks or in situ on-farm should be the first target to find sources of heat tolerance. Further, utilization of such germplasm to incorporate various climate-smart features into mungbean through new breeding tools would enable mungbean cultivars to perform well in a variety of locations and adapt to diverse agro-climatic regions. In addition to recent advances in new breeding tools, the latest developments in genomics, transcriptomics and metabolomics fields could make a great impact on trait identification and may help to accelerate in developing desired mungbean cultivars. Regions of the genome linked to advantageous characteristics, heat tolerance, water-use efficiency, and the photosynthetic pathways, can also be targeted by combining the genome sequence and phenotyping data. Additionally, a thorough examination of the mungbean pan-genome variability should be performed to understand pan-genome variability at species level. Researchers are now well equipped to explore and exploit underlying molecular processes, which may pave the way for developing multi-stress tolerant mungbean that is best suited to adverse growing environments. Modern scientific tools such as mutational breeding and genome editing may prove very useful in creation of desired novel variability and development of heat tolerant genotypes. Integration of suitable agricultural practices to mitigate heat stress may further provide additional protection to crop cultivation amid rising global temperature.
Author contributions
Author RB surveyed the literature and made initial draft of the manuscript. G outlined the manuscript’s content, improved its content and coordinated among the co-authors. SM and SK helped in improving initial draft. JL made significant changes and corrections in text and figures. RP, NM, and DR contributed in further improvement of the figures and scientific contents of physiological aspects. All authors contributed to the article and approved the submitted version.
Acknowledgments
Authors duly acknowledge ICAR-National Bureau of Plant Genetic Resources, New Delhi, ICAR-Indian Agricultural Research institute, New Delhi, Shivaji College, University of Delhi, New Delhi, ICAR-Indian Grassland and Research Institute, Jhansi, and Banasthali Vidyapith University, Tonk Rajasthan for providing necessary support for the study.
Conflict of interest
The authors declare that the research was conducted in the absence of any commercial or financial relationships that could be construed as a potential conflict of interest.
Publisher’s note
All claims expressed in this article are solely those of the authors and do not necessarily represent those of their affiliated organizations, or those of the publisher, the editors and the reviewers. Any product that may be evaluated in this article, or claim that may be made by its manufacturer, is not guaranteed or endorsed by the publisher.
References
Abd El Lateff, E., Abd El-Salam, M., Selim, M., Tawfik, M., Mohamad, E.-K., and Farrag, A. (2018). Effect of climate change on mungbean growth and productivity under Egyptian conditions. Indian J. Agric. Sci. 2, 16–23.
Adams, S. R., Cockshull, K. E., and Cave, C. R. J. (2001). Effect of temperature on the growth and development of tomato fruits. Ann. Bot. 88, 869–877. doi:10.1006/anbo.2001.1524
Ahmad, P., Jaleel, C. A., Salem, M. A., Nabi, G., and Sharma, S. (2010). Roles of enzymatic and nonenzymatic antioxidants in plants during abiotic stress. Crit. Rev. Biotechnol. 30, 161–175. doi:10.3109/07388550903524243
Ahmad, M., Waraich, E. A., Hussain, S., Ayyub, C. M., Ahmad, Z., and Zulfiqar, U. (2021). Improving heat stress tolerance in Camelina sativa and Brassica napus through thiourea seed priming. J. Plant Growth Regul. 41, 2886–2902. doi:10.1007/s00344-021-10482-4
Alom, K. M., Rashid, M. H., and Biswas, M. (2014). Genetic variability, correlation and path analysis in mungbean (Vigna radiata L). J. Environ. Sci. Nat. Resour. 7, 131–138. doi:10.3329/jesnr.v7i1.22161
Amani, I., Fischer, R. A., and Reynolds, M. P. (1996). Canopy temperature depression association with yield of irrigated spring wheat cultivars in a hot climate. J. Agron. Crop Sci. 176, 119–129. doi:10.1111/j.1439-037x.1996.tb00454.x
Anderson, J. A., and Padhye, S. R. (2004). Protein aggregation, radical scavenging capacity, and stability of hydrogen peroxide defense systems in heat-stressed vinca and sweet pea leaves. J. Am. Soc. Hortic. 129, 54–59. doi:10.21273/jashs.129.1.0054
Apel, K., and Hirt, H. (2004). Reactive oxygen species: Metabolism, oxidative stress, and signal transduction. Annu. Rev. Plant Biol. 55, 373–399. doi:10.1146/annurev.arplant.55.031903.141701
Ara, N., Nakkanong, K., Lv, W., Yang, J., Hu, Z., and Zhang, M. (2013). Antioxidant enzymatic activities and gene expression associated with heat tolerance in the stems and roots of two cucurbit species (“Cucurbita maxima” and “Cucurbita moschata”) and their interspecific inbred line “maxchata”. Int. J. Mol. Sci. 14, 24008–24028. doi:10.3390/ijms141224008
Ashraf, M., and Hafeez, M. (2004). Thermotolerance of pearl millet and maize at early growth stages: Growth and nutrient relations. Biol. Plant. 48, 81–86. doi:10.1023/B:BIOP.0000024279.44013.61
Asseng, S., Foster, I. A. N., and Turner, N. C. (2011). The impact of temperature variability on wheat yields. Glob. Chang. Biol. 17, 997–1012. doi:10.1111/j.1365-2486.2010.02262.x
Asthir, B. (2015). Protective mechanisms of heat tolerance in crop plants. J. Plant Interact. 10, 202–210. doi:10.1080/17429145.2015.1067726
Awasthi, R., Bhandari, K., and Nayyar, H. (2015). Temperature stress and redox homeostasis in agricultural crops. Front. Environ. Sci. 3, 11. doi:10.3389/fenvs.2015.00011
Bajguz, A., and Hayat, S. (2009). Effects of brassinosteroids on the plant responses to environmental stresses. Plant Physiol. biochem. 47, 1–8. doi:10.1016/j.plaphy.2008.10.002
Baker, C. J., and Orlandi, E. W. (1995). Active oxygen in plant pathogenesis. Annu. Rev. Phytopathol. 33, 299–321. doi:10.1146/annurev.py.33.090195.001503
Bañon, S., Fernandez, J. A., Franco, J. A., Torrecillas, A., Alarcón, J. J., and Sánchez-Blanco, M. J. (2004). Effects of water stress and night temperature preconditioning on water relations and morphological and anatomical changes of Lotus creticus plants. Sci. Hortic. 101, 333–342. doi:10.1016/j.scienta.2003.11.007
Bansal, M., Kukreja, K., Sunita, S., and Dudeja, S. S. (2014). Symbiotic effectivity of high temperature tolerant mungbean (Vigna radiata) rhizobia under different temperature conditions. Int. J. Curr. Microbiol. Appl. Sci. 3, 807–821.
Banti, V., Mafessoni, F., Loreti, E., Alpi, A., and Perata, P. (2010). The heat-inducible transcription factor HsfA2 enhances anoxia tolerance in Arabidopsis. Plant Physiol. 152, 1471–1483. doi:10.1104/pp.109.149815
Baroowa, B., Gogoi, N., and Farooq, M. (2016). Changes in physiological, biochemical and antioxidant enzyme activities of green gram (Vigna radiata L) genotypes under drought. Acta Physiol. Plant 38, 219. doi:10.1007/s11738-016-2230-7
Bartels, D., and Sunkar, R. (2005). Drought and salt tolerance in plants. Crit. Rev. Plant Sci. 24, 23–58. doi:10.1080/07352680590910410
Basu, P. S., Pratap, A., Gupta, S., Sharma, K., Tomar, R., and Singh, N. P. (2019). Physiological traits for shortening crop duration and improving productivity of greengram (Vigna radiata L. Wilczek) under high temperature. Front. Plant Sci. 10, 1508. doi:10.3389/fpls.2019.01508
Batcho, A. A., Sarwar, M. B., Tariq, L., Rashid, B., Hassan, S., and Husnain, T. (2020). Identification and characterisation of heat shock protein gene (HSP70) family and its expression in Agave sisalana under heat stress. J. Hortic. Sci. Biotechnol. 95, 470–482. doi:10.1080/14620316.2019.1685412
Bita, C. E., and Gerats, T. (2013). Plant tolerance to high temperature in a changing environment: Scientific fundamentals and production of heat stress-tolerant crops. Front. Plant Sci. 4, 273. doi:10.3389/fpls.2013.00273
Blokhina, O., Virolainen, E., and Fagerstedt, K. V. (2003). Antioxidants, oxidative damage and oxygen deprivation stress: A review. Ann. Bot. 91, 179–194. doi:10.1093/aob/mcf118
Bokszczanin, K. L., Consortium, S., and Fragkostefanakis, S. (2013). Perspectives on deciphering mechanisms underlying plant heat stress response and thermotolerance. Front. Plant Sci. 4, 315. doi:10.3389/fpls.2013.00315
Bolouri-Moghaddam, M. R., Le Roy, K., Xiang, L., Rolland, F., and Van den Ende, W. (2010). Sugar signalling and antioxidant network connections in plant cells. FEBS J. 277, 2022–2037. doi:10.1111/j.1742-4658.2010.07633.x
Cabello, J. V., Lodeyro, A. F., and Zurbriggen, M. D. (2014). Novel perspectives for the engineering of abiotic stress tolerance in plants. Curr. Opin. Biotechnol. 26, 62–70. doi:10.1016/j.copbio.2013.09.011
Cao, D., Li, H., Yi, J., Zhang, J., Che, H., Cao, J., et al. (2011). Antioxidant properties of the mung bean flavonoids on alleviating heat stress. PloS one 6, e21071. doi:10.1371/journal.pone.0021071
Chakraborty, P., and Dwivedi, P. (2021). Seed priming and its role in mitigating heat stress responses in crop plants. Curr. Opin. Biotechnol. 21 (2), 1718–1734. doi:10.1007/s42729-021-00474-4
Chand, G., Nandwal, A. S., Kumar, N., Devi, S., and Khajuria, S. (2018). Yield and physiological responses of mungbean Vigna radita (L) Wilczek genotypes to high temperature at reproductive stage. Legume Research-An Int. J. 41, 557–562. doi:10.18805/lr-3795
Chand, G., Nandwal, A. S., Dogra, S., and Sharma, M. (2020). Biochemical response of mungbean [Vigna radiata (L.) wilczek] genotypes under terminal heat stress at reproductive stage. Int. J. Curr. Microbiol. App. Sci. 9, 2975–2986. doi:10.20546/ijcmas.2020.907.351
Chandra, K., Prasad, R., Thakur, P., Madhukar, K., and Prasad, L. C. (2017). Heat tolerance in wheat-A key strategy to combat climate change through molecular markers. Int. J. Curr. Microbiol. Appl. Sci. 6, 662–675. doi:10.20546/ijcmas.2017.603.077
Chaves, M. M., Maroco, J. P., and Pereira, J. S. (2003). Understanding plant responses to drought—From genes to the whole plant. Funct. Plant Biol. 30, 239–264. doi:10.1071/FP02076
Che, P., Bussell, J. D., Zhou, W., Estavillo, G. M., Pogson, B. J., and Smith, S. M. (2010). Signaling from the endoplasmic reticulum activates brassinosteroid signaling and promotes acclimation to stress in arabidopsis. Sci. Signal. 28 (3), ra69. doi:10.1126/scisignal.2001140
Chen, C., and Chen, Z. (2002). Potentiation of developmentally regulated plant defense response by AtWRKY18, a pathogen-induced Arabidopsis transcription factor. Plant Physiol. 129, 706–716. doi:10.1104/pp.001057
Chikukura, L., Bandyopadhyay, S. K., Pathak, H., and Chakrabarti, B. (2017). Effect of elevated temperature stress on growth, yield and yield attributes of mungbean (Vigna radiata) in semi-arid north-west India. Curr. Adv. Agric. Sci. Int. J. 9, 18–22. doi:10.5958/2394-4471.2017.00003.X
Condon, A. G., Reynolds, M. P., Rebetzke, G. J., Ginkel, M. van, Richards, R. A., and Farquhar, G. D. (2007). “Using stomatal aperture-related traits to select for high yield potential in bread wheat,” in Wheat Production in Stressed Environments: Proceedings of the 7th International Wheat Conference, Mar del Plata, Argentina, November–December 2005 (Springer), 617–624.
Das, G., and Rao, G. J. N. (2015). Molecular marker assisted gene stacking for biotic and abiotic stress resistance genes in an elite rice cultivar. Front. Plant Sci. 6, 698. doi:10.3389/fpls.2015.00698
Das, S., Krishnan, P., Nayak, M., and Ramakrishnan, B. (2014). High temperature stress effects on pollens of rice (Oryza sativa L) genotypes. Environ. Exp. Bot. 101, 36–46. doi:10.1016/j.envexpbot.2014.01.004
de Souza Luche, H., da Silva, J. A. G., Nornberg, R., Zimmer, C. M., Arenhardt, E. G., da Rosa Caetano, V., et al. (2015). Stay-green effects on adaptability and stability in wheat. Afr. J. Agric. Res. 10, 1142–1149.
Deng, Y., Humbert, S., Liu, J.-X., Srivastava, R., Rothstein, S. J., and Howell, S. H. (2011). Heat induces the splicing by IRE1 of a mRNA encoding a transcription factor involved in the unfolded protein response in Arabidopsis. Proc. Natl. Acad. Sci. U.S.A. 108, 7247–7252. doi:10.1073/pnas.1102117108
Devasirvatham, V., Gaur, P. M., Mallikarjuna, N., Tokachichu, R. N., Trethowan, R. M., and Tan, D. K. (2012). Effect of high temperature on the reproductive development of chickpea genotypes under controlled environments. Funct. Plant Biol. 39, 1009–1018. doi:10.1071/FP12033
Dickson, M. H., and Boettger, M. A. (1984). Effect of high and low temperatures on pollen germination and seed set in snap beans. J. Am. Soc. Hortic. Sci. 109, 372–374. doi:10.21273/JASHS.109.3.372
Ding, W., Song, L., Wang, X., and Bi, Y. (2010). Effect of abscisic acid on heat stress tolerance in the calli from two ecotypes of Phragmites communis. Biol. Plant. 54, 607–613. doi:10.1007/s10535-010-0110-3
Djanaguiraman, M., Prasad, P. V. V., and Al-Khatib, K. (2011). Ethylene perception inhibitor 1-MCP decreases oxidative damage of leaves through enhanced antioxidant defense mechanisms in soybean plants grown under high temperature stress. Environ. Exp. Bot. 71, 215–223. doi:10.1016/j.envexpbot.2010.12.006
Djanaguiraman, M., Prasad, P. V. V., Boyle, D. L., and Schapaugh, W. T. (2013). Soybean pollen anatomy, viability and pod set under high temperature stress. J. Agron. Crop Sci. 199, 171–177. doi:10.1111/jac.12005
Dobrá, J., Černý, M., Štorchová, H., Dobrev, P., Skalák, J., Jedelský, P. L., et al. (2015). The impact of heat stress targeting on the hormonal and transcriptomic response in Arabidopsis. Plant Sci. 231, 52–61. doi:10.1016/j.plantsci.2014.11.005
Doke, N. (1997). “The oxidative burst: Roles in signal transduction and plant stress,” in Oxidative stress and the molecular biology of antioxidant defenses. Editors J. G. Scandalios New York: Cold Spring Harbor Press), 785–813.
Dreesen, F. E., De Boeck, H. J., Janssens, I. A., and Nijs, I. (2012). Summer heat and drought extremes trigger unexpected changes in productivity of a temperate annual/biannual plant community. Environ. Exp. Bot. 79, 21–30. doi:10.1016/j.envexpbot.2012.01.005
Driedonks, N., Rieu, I., and Vriezen, W. H. (2016). Breeding for plant heat tolerance at vegetative and reproductive stages. Plant Reprod. 29, 67–79. doi:10.1007/s00497-016-0275-9
El-Bassiony, A. M., Ghoname, A. A., El-Awadi, M. E., Fawzy, Z. F., and Gruda, N. (2012). Ameliorative effects of brassinosteroids on growth and productivity of snap beans grown under high temperature. Gesunde Pflanz. 64, 175–182. doi:10.1007/s10343-012-0286-x
Escobar-Restrepo, J.-M., Huck, N., Kessler, S., Gagliardini, V., Gheyselinck, J., Yang, W.-C., et al. (2007). The FERONIA receptor-like kinase mediates male-female interactions during pollen tube reception. Sci 317, 656–660. doi:10.1126/science.1143562
Fahad, S., Hussain, S., Saud, S., Hassan, S., Ihsan, Z., Shah, A. N., et al. (2016). Exogenously applied plant growth regulators enhance the morpho-physiological growth and yield of rice under high temperature. Front. Plant Sci. 7, 1250. doi:10.3389/fpls.2016.01250
Fahad, S., Bajwa, A. A., Nazir, U., Anjum, S. A., Farooq, A., Zohaib, A., et al. (2017). Crop production under drought and heat stress: Plant responses and management options. Front. Plant Sci. 8, 1147. doi:10.3389/fpls.2017.01147
Fancy, N. N., Bahlmann, A.-K., and Loake, G. J. (2017). Nitric oxide function in plant abiotic stress. Plant Cell. Environ. 40, 462–472. doi:10.1111/pce.12707
Farooq, M., Nadeem, F., Gogoi, N., Ullah, A., Alghamdi, S. S., Nayyar, H., et al. (2017). Heat stress in grain legumes during reproductive and grain-filling phases. Crop. Pasture. Sci. 68, 985–1005. doi:10.1071/cp17012
Feng, B., Liu, P., Li, G., Dong, S. T., Wang, F. H., Kong, L. A., et al. (2014). Effect of heat stress on the photosynthetic characteristics in flag leaves at the grain-filling stage of different heat-resistant winter wheat varieties. J. Agron. Crop Sci. 200, 143–155. doi:10.1111/jac.12045
Fischer, R. A., Rees, D., Sayre, K. D., Lu, Z.-M., Condon, A. G., and Saavedra, A. L. (1998). Wheat yield progress associated with higher stomatal conductance and photosynthetic rate, and cooler canopies. Crop Sci. 38, 1467–1475. doi:10.2135/cropsci1998.0011183x003800060011x
Foyer, C. H., Lopez-Delgado, H., Dat, J. F., and Scott, I. M. (1997). Hydrogen peroxide- and glutathione-associated mechanisms of acclimatory stress tolerance and signalling. Physiol. Plant. 100, 241–254. doi:10.1034/j.1399-3054.1997.1000205.x
Frova, C., and Sari-Gorla, M. (1994). Quantitative trait loci (QTLs) for pollen thermotolerance detected in maize. Mol. Genet. Genom. MGG 245, 424–430. doi:10.1007/BF00302254
Gayacharan, , , Tripathi, K., Meena, S. K., Panwar, B. S., Lal, H., Rana, J. C., et al. (2020). Understanding genetic variability in the mungbean (Vigna radiata L) genepool. Ann. Appl. Biol. 177 (3), 346–357. doi:10.1111/aab.12624
Gayacharan, , , Parida, S. K., Mondal, N., Yadav, R., Vishwakarma, H., and Rana, J. C. (2023). Mining legume germplasm for genetic gains: An Indian perspective. Front. Genet. 14, 996828. doi:10.3389/fgene.2023.996828
Giorno, F., Wolters-Arts, M., Mariani, C., and Rieu, I. (2013). Ensuring reproduction at high temperatures: The heat stress response during anther and pollen development. Plants 2, 489–506. doi:10.3390/plants2030489
Grover, A., Mittal, D., Negi, M., and Lavania, D. (2013). Generating high temperature tolerant transgenic plants: Achievements and challenges. Plant Sci. 205, 38–47. doi:10.1016/j.plantsci.2013.01.005
Guna, M., Ramanathan, S., Geethalakshmi, V., Chandrakumar, K., Kokilavani, S., and Djanaguiraman, M. (2022). Effect of high night temperature and CO2 on yield and seed quality of summer green gram (Vigna radiata) under soil plant atmospheric research (SPAR). J. Agric. Meteorol. 24, 229–234. doi:10.54386/jam.v24i3.1685
Gupta, N. K., Agarwal, S., Agarwal, V. P., Nathawat, N. S., Gupta, S., and Singh, G. (2013). Effect of short-term heat stress on growth, physiology and antioxidative defence system in wheat seedlings. Acta Physiol. Plant. 35, 1837–1842. doi:10.1007/s11738-013-1221-1
Hamada, A. M. (2001). Alteration in growth and some relevant metabolic processes of broad bean plants during extreme temperatures exposure. Acta Physiol. Plant 23, 193–200. doi:10.1007/s11738-001-0008-y
Hanif, A., and Wahid, A. (2018). Seed yield loss in mungbean is associated to heat stress induced oxidative damage and loss of photosynthetic capacity in proximal trifoliate leaf. Pak. J. Agric. Sci. 55.
HanumanthaRao, B., Nair, R. M., and Nayyar, H. (2016). Salinity and high temperature tolerance in mungbean [Vigna radiata (L) Wilczek] from a physiological perspective. Front. Plant Sci. 7, 957. doi:10.3389/fpls.2016.00957
Hare, P. D., Cress, W. A., and Van Staden, J. (1998). Dissecting the roles of osmolyte accumulation during stress. Plant Cell. Environ. 21, 535–553. doi:10.1046/j.1365-3040.1998.00309.x
Hasanuzzaman, M., Hossain, M. A., and Fujita, M. (2011). Nitric oxide modulates antioxidant defense and the methylglyoxal detoxification system and reduces salinity-induced damage of wheat seedlings. Plant Cell. Rep. 5, 353–365. doi:10.1007/s11816-011-0189-9
Hasanuzzaman, M., Hossain, M. A., da Silva, J. A. T., and Fujita, M. (2012). “Plant response and tolerance to abiotic oxidative stress: Antioxidant defense is a key factor,” in Crop stress and its management: Perspectives and strategies. Editors B. Venkateswarlu, A. Shanker, C. Shanker, and M. Maheswari (Dordrecht: Springer). doi:10.1007/978-94-007-2220-0_8
Hasanuzzaman, M., Nahar, K., Alam, M. M., Roychowdhury, R., and Fujita, M. (2013). Physiological, biochemical, and molecular mechanisms of heat stress tolerance in plants. Int. J. Mol. Sci. 14, 9643–9684. doi:10.3390/ijms14059643
Havaux, M. (1998). Carotenoids as membrane stabilizers in chloroplasts. Trends Plant Sci. 3, 147–151. doi:10.1016/s1360-1385(98)01200-x
Heath, R. L., and Packer, L. (1968). Photoperoxidation in isolated chloroplasts: I. Kinetics and stoichiometry of fatty acid peroxidation. Arch. Biochem. Biophys. 125, 189–198. doi:10.1016/0003-9861(68)90654-1
Howarth, C. J. (2005). “Genetic improvements of tolerance to high temperature,” in Abiotic stresses: Plant resistance through breeding and molecular approaches. Editors M. Ashraf, and P. J. C. Harris (New York: Howarth Press Inc.).
Hurkman, W. J., Vensel, W. H., Tanaka, C. K., Whitehand, L., and Altenbach, S. B. (2009). Effect of high temperature on albumin and globulin accumulation in the endosperm proteome of the developing wheat grain. J. Cereal Sci. 49, 12–23. doi:10.1016/j.jcs.2008.06.014
Iqbal, M., Ahmad, M., and Mustafa, G. (2015). Impact of farm households’ adaptations to climate change on food security: Evidence from different agro-ecologies of Pakistan. Pak. Dev. Rev. 55 (4), 561–588. Part-II (Winter 2016). doi:10.30541/v55i4i-iipp.561-588
Islam, M. T. (2015). Effects of high temperature on photosynthesis and yield in mungbean. Bangladesh J. Bot. 44, 451–454. doi:10.3329/bjb.v44i3.38553
Jha, U. C., Bohra, A., and Singh, N. P. (2014). Heat stress in crop plants: Its nature, impacts and integrated breeding strategies to improve heat tolerance. Plant Breed. 133, 679–701. doi:10.1111/pbr.12217
Jha, U. C., Bohra, A., Parida, S. K., and Jha, R. (2017). Integrated “omics” approaches to sustain global productivity of major grain legumes under heat stress. Plant Breed. 136, 437–459. doi:10.1111/pbr.12489
Jha, U. C., Nayyar, H., Palakurthi, R., Jha, R., Valluri, V., Bajaj, P., et al. (2021). Major QTLs and potential candidate genes for heat stress tolerance identified in chickpea (Cicer arietinum L). Front. Plant Sci. 12, 655103. doi:10.3389/fpls.2021.655103
Jincy, M., Jeyakumar, P., Boominathan, P., Manivannan, N., Varanavasiappan, S., and Rajendraprasad, V. B. (2019). Effect of drought and high temperature stress on greengram (Vigna radiata (L) Wilczek) at vegetative stage. Pharma Innov. 8, 647–650.
Jincy, M., Prasad, V. B. R., Senthil, A., Jeyakumar, P., and Manivannan, N. (2020). Physiological divergence in green gram [Vigna radiata (L) wilczek] genotypes for drought and high temperature stress tolerance during flowering phase. Legume Res. 45, 960. doi:10.18805/LR-4314
Kalaji, H. M., Jajoo, A., Oukarroum, A., Brestic, M., Zivcak, M., Samborska, I. A., et al. (2016). Chlorophyll a fluorescence as a tool to monitor physiological status of plants under abiotic stress conditions. Acta Physiol. Plant 38, 102–111. doi:10.1007/s11738-016-2113-y
Kang, Y. J., Kim, S. K., Kim, M. Y., Lestari, P., Kim, K. H., Ha, B.-K., et al. (2014). Genome sequence of mungbean and insights into evolution within Vigna species. Nat. Commun. 5, 5443. doi:10.1038/ncomms6443
Karim, M. A., Fukamachi, H., Komori, S., Ogawa, K., and Hidaka, T. (2003). Growth, yield and photosynthetic activity of Vigna radiata L. grown at different temperature and light levels. Plant Prod. Sci. 6, 43–49. doi:10.1626/pps.6.43
Kaur, S., and Nayyar, H. (2015). Selenium fertilization to salt-stressed mungbean (Vigna radiata L. Wilczek) plants reduces sodium uptake, improves reproductive function, pod set and seed yield. Sci. Hortic. 197, 304–317. doi:10.1016/j.scienta.2015.09.048
Kaur, R., Bains, T. S., Bindumadhava, H., and Nayyar, H. (2015). Responses of mungbean (Vigna radiata L) genotypes to heat stress: Effects on reproductive biology, leaf function and yield traits. Sci. Hortic. 197, 527–541. doi:10.1016/j.scienta.2015.10.015
Kaushal, N., Awasthi, R., Gupta, K., Gaur, P., Siddique, K. H., and Nayyar, H. (2013). Heat-stress-induced reproductive failures in chickpea (Cicer arietinum) are associated with impaired sucrose metabolism in leaves and anthers. Funct. Plant Biol. 40, 1334–1349. doi:10.1071/FP13082
Kaushal, N., Bhandari, K., Siddique, K. H., and Nayyar, H. (2016). Food crops face rising temperatures: An overview of responses, adaptive mechanisms, and approaches to improve heat tolerance. Cogent food Agric. 2, 1134380. doi:10.1080/23311932.2015.1134380
Keatinge, J. D. H., Easdown, W. J., Yang, R. Y., Chadha, M. L., and Shanmugasundaram, S. (2011). Overcoming chronic malnutrition in a future warming world: The key importance of mungbean and vegetable soybean. Euphytica 180, 129–141. doi:10.1007/s10681-011-0401-6
Klimenko, S. B., Peshkova, A. A., and Dorofeev, N. V. (2006). Nitrate reductase activity during heat shock in winter wheat. J. Stress Physiol. Biochem. 2, 50–55.
Königshofer, H., and Lechner, S. (2002). Are polyamines involved in the synthesis of heat-shock proteins in cell suspension cultures of tobacco and alfalfa in response to high-temperature stress? Plant Physiol. biochem. 40, 51–59. doi:10.1016/S0981-9428(01)01347-X
Kotak, S., Larkindale, J., Lee, U., von Koskull-Döring, P., Vierling, E., and Scharf, K.-D. (2007). Complexity of the heat stress response in plants. Curr. Opin. Plant Biol. 10, 310–316. doi:10.1016/j.pbi.2007.04.011
Kumar, S. V., and Wigge, P. A. (2010). H2A.Z-Containing nucleosomes mediate the thermosensory response in arabidopsis. Cell. 140, 136–147. doi:10.1016/j.cell.2009.11.006
Kumar, S., Kaur, R., Kaur, N., Bhandhari, K., Kaushal, N., Gupta, K., et al. (2011). Heat-stress induced inhibition in growth and chlorosis in mungbean (Phaseolus aureus Roxb) is partly mitigated by ascorbic acid application and is related to reduction in oxidative stress. Acta Physiol. Plant. 33, 2091–2101. doi:10.1007/s11738-011-0748-2
Kumar, P., Pal, M., Joshi, R., and Sairam, R. K. (2013). Yield, growth and physiological responses of mung bean [Vigna radiata (L) Wilczek] genotypes to waterlogging at vegetative stage. Physiol. Mol. Biol. Plants. 19, 209–220. doi:10.1007/s12298-012-0153-3
Kumar, R. R. (2012). Protection against heat stress in wheat involves change in cell membrane stability, antioxidant enzymes, osmolyte, H2O2 and transcript of heat shock protein. Int. J. Plant Physiol. Biochem. 4 (4), 83–91. doi:10.5897/IJPPB12.008
Kumari, P., and Varma, S. K. (1983). Genotypic differences in flower production/shedding and yield in mungbean (Vigna radiata). Acta Physiol. Plant 4, 402–405.
Landi, S., Hausman, J.-F., Guerriero, G., and Esposito, S. (2017). Poaceae vs. Abiotic stress: Focus on drought and salt stress, recent insights and perspectives. Front. Plant Sci. 8, 1214. doi:10.3389/fpls.2017.01214
Levine, A., Tenhaken, R., Dixon, R., and Lamb, C. (1994). H2O2 from the oxidative burst orchestrates the plant hypersensitive disease resistance response. Cell. 79, 583–593. doi:10.1016/0092-8674(94)90544-4
Li, S., Zhou, X., Chen, L., Huang, W., and Yu, D. (2010). Functional characterization of Arabidopsis thaliana WRKY39 in heat stress. Mol. Cells 29, 475–483. doi:10.1007/s10059-010-0059-2
Li, S., Fu, Q., Chen, L., Huang, W., and Yu, D. (2011). Arabidopsis thaliana WRKY25, WRKY26, and WRKY33 coordinate induction of plant thermotolerance. Planta 233, 1237–1252. doi:10.1007/s00425-011-1375-2
Li, W., Chen, Y., Ye, M., Lu, H., Wang, D., and Chen, Q. (2020). Evolutionary history of the C-repeat binding factor/dehydration-responsive element-binding 1 (CBF/DREB1) protein family in 43 plant species and characterization of CBF/DREB1 proteins in Solanum tuberosum. BMC Evol. Biol. 20, 142. doi:10.1186/s12862-020-01710-8
Lin, C.-Y., Roberts, J. K., and Key, J. L. (1984). Acquisition of thermotolerance in soybean seedlings: Synthesis and accumulation of heat shock proteins and their cellular localization. Plant Physiol. 74, 152–160. doi:10.1104/pp.74.1.152
Liu, X., and Huang, B. (2000). Heat stress injury in relation to membrane lipid peroxidation in creeping bentgrass. Crop Sci. 40, 503–510. doi:10.2135/cropsci2000.402503x
Liu, Z.-W., Wu, Z.-J., Li, X.-H., Huang, Y., Li, H., Wang, Y.-X., et al. (2016). Identification, classification, and expression profiles of heat shock transcription factors in tea plant (Camellia sinensis) under temperature stress. Gene 576, 52–59. doi:10.1016/j.gene.2015.09.076
Loreto, F., Förster, A., Dürr, M., Csiky, O., and Seufert, G. (1998). On the monoterpene emission under heat stress and on the increased thermotolerance of leaves of Quercus ilex L. fumigated with selected monoterpenes. Plant Cell. Environ. 21, 101–107. doi:10.1046/j.1365-3040.1998.00268.x
Lucas, M. R., Ehlers, J. D., Huynh, B.-L., Diop, N.-N., Roberts, P. A., and Close, T. J. (2013). Markers for breeding heat-tolerant cowpea. Mol. Breed. 31, 529–536. doi:10.1007/s11032-012-9810-z
Maavimani, M., and Saraswathi, R. (2014). Anther characteristics and spikelet fertility in rice (Oryza sativa L) under high temperature stress at anthesis. Indian J. Genet. Plant. Breed. 74, 300–308. doi:10.5958/0975-6906.2014.00847.5
Mandhania, S., Madan, S., and Sawhney, V. (2006). Antioxidant defense mechanism under salt stress in wheat seedlings. Biol. Plant. 50, 227–231. doi:10.1007/s10535-006-0011-7
Mansoor, S., and Naqvi, F. N. (2013). Effect of heat stress on lipid peroxidation and antioxidant enzymes in mung bean (Vigna radiata L) seedlings. Afr. J. Biotechnol. 12. doi:10.4314/ajb.v12i21
Mason, R. E., and Singh, R. P. (2014). Considerations when deploying canopy temperature to select high yielding wheat breeding lines under drought and heat stress. Agronomy 4, 191–201. doi:10.3390/agronomy4020191
Mason, R. E., Mondal, S., Beecher, F., Pacheco, A., Jampala, B., Ibrahim, A., et al. (2010). QTL associated with heat susceptibility index in wheat (Triticum aestivum L) under short-term reproductive stage heat stress. Euphytica 174, 423–436. doi:10.1007/s10681-010-0151-x
Mason, R. E., Hays, D. B., Mondal, S., Ibrahim, A. M. H., and Basnet, B. R. (2013). QTL for yield, yield components and canopy temperature depression in wheat under late sown field conditions. Euphytica 194, 243–259. doi:10.1007/s10681-013-0951-x
Mathur, S., Agrawal, D., and Jajoo, A. (2014). Photosynthesis: Response to high temperature stress. J. Photochem. Photobiol. B Biol. 137, 116–126. doi:10.1016/j.jphotobiol.2014.01.010
Maulana, F., Ayalew, H., Anderson, J. D., Kumssa, T. T., Huang, W., and Ma, X.-F. (2018). Genome-Wide association mapping of seedling heat tolerance in winter wheat. Front. Plant Sci. 9, 1272. doi:10.3389/fpls.2018.01272
Miller, G., Suzuki, N., Rizhsky, L., Hegie, A., Koussevitzky, S., and Mittler, R. (2007). Double mutants deficient in cytosolic and thylakoid ascorbate peroxidase reveal a complex mode of interaction between reactive oxygen species, plant development, and response to abiotic stresses. Plant Physiol. 144, 1777–1785. doi:10.1104/pp.107.101436
Mishra, D., Shekhar, S., Singh, D., Chakraborty, S., and Chakraborty, N. (2018). “Heat shock proteins and abiotic stress tolerance in plants,” in Regulation of heat shock protein responses. Editors A. Asea, and P. Kaur (Cham: Springer) 13. doi:10.1007/978-3-319-74715-6_3
Mittler, R., Finka, A., and Goloubinoff, P. (2012). How do plants feel the heat? Trends biochem. Sci. 37, 118–125. doi:10.1016/j.tibs.2011.11.007
Mohammed, A. R., and Tarpley, L. (2010). Effects of high night temperature and spikelet position on yield-related parameters of rice (Oryza sativa L) plants. Eur. J. Agron. 33, 117–123. doi:10.1016/j.eja.2009.11.006
Monterroso, V. A., and Wien, H. C. (1990). Flower and pod abscission due to heat stress in beans. J. Am. Soc. Hortic. Sci. 115, 631–634. doi:10.21273/jashs.115.4.631
Nair, R. M., Pandey, A. K., War, A. R., Hanumantharao, B., Shwe, T., Alam, A., et al. (2019). Biotic and abiotic constraints in mungbean production-progress in genetic improvement. Front. Plant Sci. 10, 1340. doi:10.3389/fpls.2019.01340
Nakamoto, H., and Hiyama, T. (1999). “Heat-shock proteins and temperature stress,” in Handbook of plant and crop stress (New York: Marcel Dekker), 399–416.
Nawaz, A., Farooq, M., Cheema, S. A., and Wahid, A. (2013). Differential response of wheat cultivars to terminal heat stress. Int. J. Agric. Biol. 15.
Neumann, D., Emmermann, M., Thierfelder, J.-M., Zur Nieden, U., Clericus, M., Braun, H.-P., et al. (1993). HSP68-a DnaK-like heat-stress protein of plant mitochondria. Planta 190, 32–43. doi:10.1007/BF00195672
Njuki, J., Benin, S., Marivoet, W., Ulimwengu, J. M., Mwongera, C., Breisinger, C., et al. (2022). “Regional developments [in 2022 global food policy report],” in IFPRI book chapters, 114–145.
Ottaviano, E., Sari Gorla, M., Pe, E., and Frova, C. (1991). Molecular markers (RFLPs and HSPs) for the genetic dissection of thermotolerance in maize. Theor. Appl. Genet. 81, 713–719. doi:10.1007/BF00224979
Parihar, A. K., Kumar, A., Dixit, G. P., and Gupta, S. (2017). Seasonal effects on outbreak of yellow mosaic disease in released cultivars of mungbean (Vigna radiata) and urdbean (Vigna mungo). Indian J. Agric. Sci. 87, 734–738. doi:10.56093/ijas.v87i6.70938
Patriyawaty, N. R., Rachaputi, R. C., and George, D. (2018). Physiological mechanisms underpinning tolerance to high temperature stress during reproductive phase in mungbean (Vigna radiata (L) Wilczek). Environ. Exp. Bot. 150, 188–197. doi:10.1016/j.envexpbot.2018.03.022
Paul, S., Das, M. K., Baishya, P., Ramteke, A., Farooq, M., Baroowa, B., et al. (2017). Effect of high temperature on yield associated parameters and vascular bundle development in five potato cultivars. Sci. Hortic. 225, 134–140. doi:10.1016/j.scienta.2017.06.061
Paul, P. J., Samineni, S., Thudi, M., Sajja, S. B., Rathore, A., Das, R. R., et al. (2018). Molecular mapping of QTLs for heat tolerance in chickpea. Int. J. Mol. Sci. 19, 2166. doi:10.3390/ijms19082166
Peleg, Z., and Blumwald, E. (2011). Hormone balance and abiotic stress tolerance in crop plants. Curr. Opin. Plant Biol. 14, 290–295. doi:10.1016/j.pbi.2011.02.001
Pincus, D., Chevalier, M. W., Aragón, T., van Anken, E., Vidal, S. E., El-Samad, H., et al. (2010). BiP binding to the ER-stress sensor Ire1 tunes the homeostatic behavior of the unfolded protein response. PLoS Biol. 8, e1000415. doi:10.1371/journal.pbio.1000415
Pinto, R. S., Lopes, M. S., Collins, N. C., and Reynolds, M. P. (2016). Modelling and genetic dissection of staygreen under heat stress. Theor. Appl. Genet. 129, 2055–2074. doi:10.1007/s00122-016-2757-4
Polle, A. (1997). Defense against photooxidative damage in plants. Cold Spring Harb. Monogr. Ser. 34, 623–666.
Pottorff, M., Roberts, P. A., Close, T. J., Lonardi, S., Wanamaker, S., and Ehlers, J. D. (2014). Identification of candidate genes and molecular markers for heat-induced Brown discoloration of seed coats in cowpea [Vigna unguiculata (L) Walp]. BMC genomics 15, 328. doi:10.1186/1471-2164-15-328
Prasad, P. V. V., Djanaguiraman, M., Prasad, P. V. V., and Djanaguiraman, M. (2011). High night temperature decreases leaf photosynthesis and pollen function in grain sorghum. Funct. Plant Biol. 38, 993–1003. doi:10.1071/FP11035
Priya, M., Pratap, A., Sengupta, D., Singh, N. P., Jha, U. C., Siddique, K., et al. (2020). “Mungbean and high temperature stress: Responses and strategies to improve heat tolerance,” in Heat stress in food grain crops: Plant breeding and omics research. Editors U. C. Jha, H. Nayyar, and S. Gupta (Singapore: Bentham Science Publishers), 144–170.
Punia, S., Punia, M., and Yadav, R. (2019). Drought and heat tolerance in mungbean. Proceedings of national conference on harmony with nature in context of resource conservation and climate change (HARMONY - 2016), 22–24.
Rainey, K. M., and Griffiths, P. D. (2005). Differential response of common bean genotypes to high temperature. J. Am. Soc. Hortic. Sci. 130, 18–23. doi:10.21273/jashs.130.1.18
Ramani, H. R., Mandavia, M. K., Dave, R. A., Bambharolia, R. P., Silungwe, H., and Garaniya, N. H. (2017). Biochemical and physiological constituents and their correlation in wheat (Triticum aestivum L) genotypes under high temperature at different development stages. Int. J. Plant Physiol. Biochem. 9, 1–8. doi:10.5897/ijppb2015.0240
Rasheed, R. (2009). Salinity and extreme temperature effects on sprouting buds of sugarcane (Saccharum officinarum L): Some histological and biochemical studies. PhD Thesis. Faisalabad Pakistan: University of Agriculture.
Reardon, M. E., and Qaderi, M. M. (2017). Individual and interactive effects of temperature, carbon dioxide and abscisic acid on mung bean (Vigna radiata) plants. J. Plant. Interact. 12, 295–303. doi:10.1080/17429145.2017.1353654
Rivero, R. M., Ruiz, J. M., and Romero, L. (2004). Oxidative metabolism in tomato plants subjected to heat stress. J. Hortic. Sci. Biotechnol. 79, 560–564. doi:10.1080/14620316.2004.11511805
Rodríguez, M., Canales, E., and Borrás-Hidalgo, O. (2005). Molecular aspects of abiotic stress in plants. Biotecnol. Apl. 22, 1–10.
Rodriguez, R. J., Henson, J., Van Volkenburgh, E., Hoy, M., Wright, L., Beckwith, F., et al. (2008). Stress tolerance in plants via habitat-adapted symbiosis. ISME J. 2, 404–416. doi:10.1038/ismej.2007.106
Sairam, R. K., and Tyagi, A. (2004). Physiology and molecular biology of salinity stress tolerance in plants. Curr. Sci. 86, 407–421.
Sakamoto, A., and Murata, N. (2002). The role of glycine betaine in the protection of plants from stress: Clues from transgenic plants. Plant, Cell. and Environ. 25, 163–171. doi:10.1046/j.0016-8025.2001.00790.x
Sakata, T., and Higashitani, A. (2008). Male sterility accompanied with abnormal anther development in plants-genes and environmental stresses with special reference to high temperature injury. Int. J. Plant Dev. Biol. 2, 42–51.
Samakovli, D., Tichá, T., Vavrdová, T., Ovečka, M., Luptovčiak, I., Zapletalová, V., et al. (2020). YODA-HSP90 module regulates phosphorylation-dependent inactivation of SPEECHLESS to control stomatal development under acute heat stress in Arabidopsis. Mol. Plant. 13, 612–633. doi:10.1016/j.molp.2020.01.001
Sangwan, V., Örvar, B. L., Beyerly, J., Hirt, H., and Dhindsa, R. S. (2002). Opposite changes in membrane fluidity mimic cold and heat stress activation of distinct plant MAP kinase pathways. Plant J. 31, 629–638. doi:10.1046/j.1365-313x.2002.01384.x
Sangwan, S., Munjal, R., Ram, K., and Kumar, N. (2019). QTL mapping for morphological and physiological traits in RILs of spring wheat population of WH1021 × WH711. J. Environ. Biol. 40, 674–682. doi:10.22438/jeb/40/4/mrn-1002
Sarieva, G. E., Kenzhebaeva, S. S., and Lichtenthaler, H. K. (2010). Adaptation potential of photosynthesis in wheat cultivars with a capability of leaf rolling under high temperature conditions. Russ. J. Plant Physiol. 57, 28–36. doi:10.1134/S1021443710010048
Scandalios, J. G., Guan, L., and Polidoros, A. N. (1997). Catalases in plants: Gene structure, properties, regulation, and expression. Cold Spring Harb. Protoc. 34, 343–406.
Schoffl, F., Prandl, R., and Reindl, A. (1998). Regulation of the heat-shock response. Plant Physiol. 117, 1135–1141. doi:10.1104/pp.117.4.1135
Schreinemachers, P., Sequeros, T., Rani, S., Rashid, M. A., Gowdru, N. V., Rahman, M. S., et al. (2019). Counting the beans: Quantifying the adoption of improved mungbean varieties in South Asia and Myanmar. Food Secur. 11, 623–634. doi:10.1007/s12571-019-00926-x
Sehgal, A., Sita, K., and Nayyar, H. (2016). Heat stress in plants: Sensing and defense mechanisms. J. Plant. Sci. Res. 32, 195.
Sekhar, S., Kumar, J., Mohanty, S., Mohanty, N., Panda, R. S., Das, S., et al. (2021). Identification of novel QTLs for grain fertility and associated traits to decipher poor grain filling of basal spikelets in dense panicle rice. Sci. Rep. 11 (1), 13617. doi:10.1038/s41598-021-93134-7
Shamsudin, N. A. A., Swamy, B. P. M., Ratnam, W., StaCruz, Ma. T., Raman, A., and Kumar, A. (2016). Marker assisted pyramiding of drought yield QTLs into a popular Malaysian rice cultivar, MR219. BMC Genet. 17, 30. doi:10.1186/s12863-016-0334-0
Sharma, D. K., Fernández, J. O., Rosenqvist, E., Ottosen, C.-O., and Andersen, S. B. (2014). Genotypic response of detached leaves versus intact plants for chlorophyll fluorescence parameters under high temperature stress in wheat. J. Plant Physiol. 171, 576–586. doi:10.1016/j.jplph.2013.09.025
Sharma, L., Priya, M., Bindumadhava, H., Nair, R. M., and Nayyar, H. (2016). Influence of high temperature stress on growth, phenology and yield performance of mungbean [Vigna radiata (L) Wilczek] under managed growth conditions. Sci. Hortic. 213, 379–391. doi:10.1016/j.scienta.2016.10.033
Shen, H. F., Zhao, B., Xu, J. J., Liang, W., Huang, W. M., and Li, H. H. (2017). Effects of heat stress on changes in physiology and anatomy in two cultivars of Rhododendron. S. Afr. J. Bot. 112, 338–345. doi:10.1016/j.sajb.2017.06.018
Sheoran, S., Gupta, M., Kumari, S., Kumar, S., and Rakshit, S. (2022). Meta-QTL analysis and candidate genes identification for various abiotic stresses in maize (Zea mays L) and their implications in breeding programs. Mol. Breed. 42, 26. doi:10.1007/s11032-022-01294-9
Siddiqui, M. H., Al-Khaishany, M. Y., Al-Qutami, M. A., Al-Whaibi, M. H., Grover, A., Ali, H. M., et al. (2015). Morphological and physiological characterization of different genotypes of faba bean under heat stress. Saudi J. Biol. Sci. 22, 656–663. doi:10.1016/j.sjbs.2015.06.002
Simões-Araújo, J. L., Rodrigues, R. L., de, A., Gerhardt, L. B., Mondego, J. M. C., Alves-Ferreira, M., et al. (2002). Identification of differentially expressed genes by cDNA-AFLP technique during heat stress in cowpea nodules. FEBS Lett. 515, 44–50. doi:10.1016/S0014-5793(02)02416-X
Simões-Araújo, J. L., Rumjanek, N. G., and Margis-Pinheiro, M. (2003). Small heat shock proteins genes are differentially expressed in distinct varieties of common bean. Braz. J. Plant Physiol. 15, 33–41. doi:10.1590/S1677-04202003000100005
Singh, D. P., Singh, B. B., and Pratap, A. (2016). Genetic improvement of mungbean and urdbean and their role in enhancing pulse production in India. Indian J. Genet. Plant. Breed. 76, 550–567. doi:10.5958/0975-6906.2016.00072.9
Singh, H., Kaur, P., Bal, S. K., and Choudhury, B. U. (2021). Effect of elevated temperature on green gram [Vigna radiata (L) Wilczek] performance under temperature gradient tunnel (TGT) environment in Punjab. J. Agric. Meteorol. 23, 3–13. doi:10.54386/jam.v23i1.82
Singh, C. M., Kumar, M., Pratap, A., Tripathi, A., Singh, S., Mishra, A., et al. (2022). Genome-Wide analysis of late embryogenesis abundant protein gene family in vigna species and expression of VrLEA encoding genes in Vigna glabrescens reveal its role in heat tolerance. Front. Plant Sci. 13, 843107. doi:10.3389/fpls.2022.843107
Sinsawat, V., Leipner, J., Stamp, P., and Fracheboud, Y. (2004). Effect of heat stress on the photosynthetic apparatus in maize (Zea mays L) grown at control or high temperature. Environ. Exp. Bot. 52, 123–129. doi:10.1016/j.envexpbot.2004.01.010
Sita, K., Sehgal, A., HanumanthaRao, B., Nair, R. M., Vara Prasad, P. V., Kumar, S., et al. (2017). Food legumes and rising temperatures: Effects, adaptive functional mechanisms specific to reproductive growth stage and strategies to improve heat tolerance. Front. Plant Sci. 8, 1658. doi:10.3389/fpls.2017.01658
Solai, M., Vijayalakshmi, C., and Basu, P. S. (2015). Effect of high temperature on flowering pattern, pollen germination and pod setting in green gram (Vigna radiata (L) Wilczek). Int. J. Plant Biol. 7, 59–66.
Song, L., Ding, W., Zhao, M., Sun, B., and Zhang, L. (2006). Nitric oxide protects against oxidative stress under heat stress in the calluses from two ecotypes of reed. Plant Sci. 171, 449–458. doi:10.1016/j.plantsci.2006.05.002
Song, J., Jiao, L. F., Xiao, K., Luan, Z. S., Hu, C. H., Shi, B., et al. (2013). Cello-oligosaccharide ameliorates heat stress-induced impairment of intestinal microflora, morphology and barrier integrity in broilers. Anim. Feed Sci. Technol. 185, 175–181. doi:10.1016/j.anifeedsci.2013.08.001
Spiertz, J. H. J., Hamer, R. J., Xu, H., Primo-Martin, C., Don, C., and van der Putten, P. E. L. (2006). Heat stress in wheat (Triticum aestivum L): Effects on grain growth and quality traits, modelling quality traits and their genetic variability for wheat. Eur. J. Agron. 25, 89–95. doi:10.1016/j.eja.2006.04.012
Srivastava, S., Pathak, A. D., Gupta, P. S., Shrivastava, A. K., and Srivastava, A. K. (2012). Hydrogen peroxide-scavenging enzymes impart tolerance to high temperature induced oxidative stress in sugarcane. J. Environ. Biol. 33 (3), 657–661.
Stefanov, D., Petkova, V., and Denev, I. D. (2011). Screening for heat tolerance in common bean (Phaseolus vulgaris L) lines and cultivars using JIP-test. Sci. Hortic. 128, 1–6. doi:10.1016/j.scienta.2010.12.003
Sugio, A., Dreos, R., Aparicio, F., and Maule, A. J. (2009). The cytosolic protein response as a subcomponent of the wider heat shock response in arabidopsis. Plant Cell. 21, 642–654. doi:10.1105/tpc.108.062596
Suzuki, N., Miller, G., Morales, J., Shulaev, V., Torres, M. A., and Mittler, R. (2011). Respiratory burst oxidases: The engines of ROS signaling. Curr. Opin. Plant Biol. 14, 691–699. doi:10.1016/j.pbi.2011.07.014
Suzuki, N., Koussevitzky, S., Mittler, R., and Miller, G. (2012). ROS and redox signalling in the response of plants to abiotic stress. Plant Cell. Environ. 35, 259–270. doi:10.1111/j.1365-3040.2011.02336.x
Taiz, L., Zeiger, E., Møller, I. M., and Murphy, A. (2015). Plant physiol. and develop. Sunderland, USA: Sinauer Associates Incorporated.
Tamindžić, G., Ignjatov, M., Miljaković, D., Červenski, J., Milošević, D., Nikolić, Z., et al. (2023). Seed priming treatments to improve heat stress tolerance of garden pea (Pisum sativum L). Agriculture 13 (2), 439. doi:10.3390/agriculture13020439
Tangphatsornruang, S., Somta, P., Uthaipaisanwong, P., Chanprasert, J., Sangsrakru, D., Seehalak, W., et al. (2009). Characterization of microsatellites and gene contents from genome shotgun sequences of mungbean (Vigna radiata (L) Wilczek). BMC Plant Biol. 9, 137. doi:10.1186/1471-2229-9-137
Thomas, H., and Ougham, H. (2014). The stay-green trait. J. Exp. Bot. 65, 3889–3900. doi:10.1093/jxb/eru037
Tian, Y., Chen, J., Chen, C., Deng, A., Song, Z., Zheng, C., et al. (2012). Warming impacts on winter wheat phenophase and grain yield under field conditions in Yangtze Delta Plain, China. Field Crops Res. 134, 193–199. doi:10.1016/j.fcr.2012.05.013
Tiwari, M., Kumar, R., Min, D., and Jagadish, S. V. K. (2022). Genetic and molecular mechanisms underlying root architecture and function under heat stress—a hidden story. Plant Cell. Environ. 45, 771–788. doi:10.1111/pce.14266
Tzudir, L., Bera, P. S., and Chakraborty, P. K. (2014). Impact of temperature on the reproductive development in mungbean (Vigna radiata) varieties under different dates of sowing. Int. J. Bio-resour. Stress Manag. 5, 194. doi:10.5958/0976-4038.2014.00555.7
Valliyodan, B., and Nguyen, H. T. (2006). Understanding regulatory networks and engineering for enhanced drought tolerance in plants. Curr. Opin. 9, 189–195. doi:10.1016/j.pbi.2006.01.019
Vargas, Y., Mayor-Duran, V. M., Buendia, H. F., Ruiz-Guzman, H., and Raatz, B. (2021). Physiological and genetic characterization of heat stress effects in a common bean RIL population. PLOS ONE 16, e0249859. doi:10.1371/journal.pone.0249859
Vierling, E. (1991). The roles of heat shock proteins in plants. Annu. Rev. Plant Physiol. Plant Mol. Biol. 42, 579–620. doi:10.1146/annurev.pp.42.060191.003051
Vollenweider, P., and Günthardt-Goerg, M. S. (2005). Diagnosis of abiotic and biotic stress factors using the visible symptoms in foliage. Environ. Pollut. 137, 455–465. doi:10.1016/j.envpol.2005.01.032
Wahid, A., Gelani, S., Ashraf, M., and Foolad, M. R. (2007). Heat tolerance in plants: An overview. Environ. Exp. Bot. 61, 199–223. doi:10.1016/j.envexpbot.2007.05.011
Wang, W., Vinocur, B., Shoseyov, O., and Altman, A. (2004). Role of plant heat-shock proteins and molecular chaperones in the abiotic stress response. Trends Plant Sci. 9, 244–252. doi:10.1016/j.tplants.2004.03.006
Wang, J., Wu, B., Yin, H., Fan, Z., Li, X., Ni, S., et al. (2017). Overexpression of CaAPX induces orchestrated reactive oxygen scavenging and enhances cold and heat tolerances in tobacco. Bio. Med. Res. Int. 2017, e4049534. doi:10.1155/2017/4049534
Wang, Y., Reiter, R. J., and Chan, Z. (2018). Phytomelatonin: A universal abiotic stress regulator. J. Exp. Bot. 69, 963–974. doi:10.1093/jxb/erx473
Wang, Y., Yu, Y., Huang, M., Gao, P., Chen, H., Liu, M., et al. (2020). Transcriptomic and proteomic profiles of II YOU 838 (Oryza sativa) provide insights into heat stress tolerance in hybrid rice. PeerJ 8, e8306. doi:10.7717/peerj.8306
Wang, Q., Lu, X., Chen, X., Zhao, L., Han, M., Wang, S., et al. (2021). Genome-wide identification and function analysis of HMAD gene family in cotton (Gossypium spp). BMC Plant Biol. 21, 386. doi:10.1186/s12870-021-03170-8
Waraich, E. A., Ahmad, R., Halim, A., and Aziz, T. (2012). Alleviation of temperature stress by nutrient management in crop plants: A review. J. Soil Sci. Plant Nutr. 12, 221–244. doi:10.4067/S0718-95162012000200003
Waseem, M., Ali, A., Tahir, M., Nadeem, M. A., Ayub, M., Tanveer, A., et al. (2011). Mechanism of drought tolerance in plant and its management through different methods. C. J. Agric. Sci. 5, 10–25.
Willekens, H., Inzé, D., Van Montagu, M., and van Camp, W. (1995). Catalases in plants. Mol. Breed. 1, 207–228. doi:10.1007/BF02277422
Xia, X.-J., Zhou, Y.-H., Shi, K., Zhou, J., Foyer, C. H., and Yu, J.-Q. (2015). Interplay between reactive oxygen species and hormones in the control of plant development and stress tolerance. J. Exp. Bot. 66, 2839–2856. doi:10.1093/jxb/erv089
Yang, J., Sears, R. G., Gill, B. S., and Paulsen, G. M. (2002). Quantitative and molecular characterization of heat tolerance in hexaploid wheat. Euphytica 126, 275–282. doi:10.1023/A:1016350509689
Yang, J.-D., Yun, J.-Y., Zhang, T.-H., and Zhao, H.-L. (2006). Presoaking with nitric oxide donor SNP alleviates heat shock damages in mung bean leaf discs. Bot. Stud. 47, 129–136.
Young, N. D., and Udvardi, M. (2009). Translating Medicago truncatula genomics to crop legumes. Curr. Opin. Plant Biol. 12, 193–201. doi:10.1016/j.pbi.2008.11.005
Yuan, Z.-C., Haudecoeur, E., Faure, D., Kerr, K. F., and Nester, E. W. (2008). Comparative transcriptome analysis of Agrobacterium tumefaciens in response to plant signal salicylic acid, indole-3-acetic acid and γ-amino butyric acid reveals signaling cross-talk and Agrobacterium-plant co-evolution. Cell. Microbiol. 10, 2339–2354. doi:10.1111/j.1462-5822.2008.01215.x
Zandalinas, S. I., Balfagón, D., Arbona, V., and Gómez-Cadenas, A. (2017). Modulation of antioxidant defense system is associated with combined drought and heat stress tolerance in citrus. Front. Plant Sci. 8, 953. doi:10.3389/fpls.2017.00953
Zhang, J.-H., Huang, W.-D., Liu, Y.-P., and Pan, Q.-H. (2005). Effects of temperature acclimation pretreatment on the ultrastructure of mesophyll cells in young grape plants (Vitis vinifera L. Cv. Jingxiu) under cross-temperature stresses. J. Integr. Plant Biol. 47, 959–970. doi:10.1111/j.1744-7909.2005.00109.x
Zhang, X., Li, J., Liu, A., Zou, J., Zhou, X., Xiang, J., et al. (2012). Expression profile in rice panicle: Insights into heat response mechanism at reproductive stage. PLOS ONE 7, e49652. doi:10.1371/journal.pone.0049652
Zheng, H., Wang, W., Xu, K., Xu, Y., Ji, D., Chen, C., et al. (2020). Ca2+ influences heat shock signal transduction in Pyropia haitanensis. Aquaculture 516, 734618. doi:10.1016/j.aquaculture.2019.734618
Zhou, J., Wang, J., Yu, J.-Q., and Chen, Z. (2014). Role and regulation of autophagy in heat stress responses of tomato plants. Front. Plant Sci. 5, 174. doi:10.3389/fpls.2014.00174
Keywords: greengram, heat stress signaling pathways, oxidative stress, mungbean germplasm, adaptative traits
Citation: Bhardwaj R, Lone JK, Pandey R, Mondal N, Dhandapani R, Meena SK, Khan S and Gayacharan (2023) Insights into morphological and physio-biochemical adaptive responses in mungbean (Vigna radiata L.) under heat stress. Front. Genet. 14:1206451. doi: 10.3389/fgene.2023.1206451
Received: 15 April 2023; Accepted: 05 June 2023;
Published: 15 June 2023.
Edited by:
Mahmoud Magdy, Ain Shams University, EgyptReviewed by:
Chao Wu, Guangxi Institute of Botany (CAS), ChinaAnandan Annamalai, Indian Institute of Seed Science, India
Copyright © 2023 Bhardwaj, Lone, Pandey, Mondal, Dhandapani, Meena, Khan and Gayacharan. This is an open-access article distributed under the terms of the Creative Commons Attribution License (CC BY). The use, distribution or reproduction in other forums is permitted, provided the original author(s) and the copyright owner(s) are credited and that the original publication in this journal is cited, in accordance with accepted academic practice. No use, distribution or reproduction is permitted which does not comply with these terms.
*Correspondence: Gayacharan, Z2F5YWNoYXJhbkBpY2FyLmdvdi5pbg==
†These authors have contributed equally to this work