- 1Postgraduate Course in Health Sciences, Federal University of Triângulo Mineiro, Uberaba, Brazil
- 2Biosciences Unit, Centro Universitário de Mineiros, Mineiros, Brazil
- 3Postgraduate Course in Tropical Medicine and Infectology, Federal University of Triângulo Mineiro, Uberaba, Brazil
- 4Cell Biology Laboratory, Institute of Biological and Natural Sciences of the Federal University of Triângulo Mineiro, Uberaba, Brazil
In Chagas disease, the initial responses of phagocyte-mediated innate immunity are strongly associated with the control of Trypanosoma cruzi and are mediated by various signaling pathways, including the inducible nitric oxide synthetase (iNOS) pathway. The clinical and laboratory manifestations of Chagas disease depend on the parasite–host relationship, i.e., the responsive capacity of the host immune system and the immunogenicity of the parasite. Here, we evaluated effect sizes in clinical and laboratory parameters mediated by acute infection with different concentrations of T. cruzi inoculum in mice immunosuppressed via iNOS pathway inactivation. Infection was induced in C57BL/6 wild-type and iNOS-/- mice with the “Y” strain of T. cruzi at three inoculum concentrations (3 × 102, 3 × 103, and 3 × 104). Parasitemia and mortality in both mouse strains were monitored. Immunohistochemistry was performed to quantify amastigotes in cardiac tissues and cardiac musculature cells. Biochemical parameters, such as blood urea nitrogen, sodium, albumin, and globulin concentrations, among others, were measured, and cytokine concentrations were also measured. Effect sizes were determined by the eta squared formula. Compared with that in wild-type animals, mice with an absence of iNOS expression demonstrated a greater parasite load, with earlier infection and a delayed parasitemia peak. Inoculum concentration was positively related to death in the immunosuppressed subgroup. Nineteen parameters (hematological, biochemical, cytokine-related, and histopathological) in the immunocompetent subgroup and four in the immunosuppressed subgroup were associated with parasitemia. Parasitemia, biochemical parameters, and hematological parameters were found to be predictors in the knockout group. The impact of effect sizes on the markers evaluated based on T. cruzi inoculum concentration was notably high in the immunocompetent group (Cohen’s d = 88.50%; p <.001). These findings contribute to the understanding of physiopathogenic mechanisms underlying T. cruzi infection and also indicate the influence of the concentration of T. cruzi during infection and the immunosuppression through the iNOS pathway in clinical laboratory heterogeneity reported in acute Chagas disease.
Introduction
Trypanosoma cruzi is a protozoan with vast genetic variability and the ability to infect humans and cause Chagas disease (1, 2). It is estimated that around 6 to 7 million individuals worldwide, primarily in Latin America, are infected with T. cruzi, and chronic infection can cause cardiac alterations in 30% of patients or neurological or mixed alterations in 10% of patients (3).
While parasitic infection can occur via different mechanisms, its development depends on methods of invasion and intracellular division of the parasite. As part of the immune response, macrophages stand out among infected cells (4, 5). Parasites are internalized by macrophage phagosomes, wherein they may be eliminated or evade the cytosol to undergo replication, which subsequently disrupts host cells and can lead to infection of other cells or tissues (6, 7). Macrophages play a key role in inhibiting T. cruzi proliferation (8, 9).
The destruction of parasites within macrophages of the vertebrate host is mediated by certain known mechanisms, such as nitric oxide (NO) production (10, 11). Upon elevation in the levels of proinflammatory proteins, such as interferon gamma (IFN-γ), produced by natural killer lymphocytes in response to the initial immunostimulation mediated by innate immunity, the parasite can induce the trypanocidal activity of macrophages mediated by NO release in the phagosomes (12). Inducible NO synthase (iNOS) is responsible for NO synthesis. NO directly or indirectly modulates the effector functions aimed at eliminating the parasite, which involves trypanocidal effects mediated by an increase in the levels of toxic free radicals, such as peroxynitrite and superoxide, or aggravation of the proinflammatory response (10).
In contrast to the antiparasitic effects mediated by immune cell signaling processes, tissue damage is induced by the proinflammatory proteins produced in host cells (13). However, the lack of expression of proinflammatory cytokines in acute T. cruzi infection has been confirmed to serve as a limiting factor to survival as well as to infection suppression (14).
The intensity of the immune response to infection is variable and depends on factors such as the antigenic and/or phenotypic characteristics of the parasite (15, 16), the T. cruzi load during infection (17, 18), and the responsiveness of the host’s immune system (19). The inoculum concentration has previously been shown to interfere with the pathophysiology of the disease and modify the intensity of the outcomes observed in the heart (20), intestines (21), and kidneys (22) in experimental models. However, the intensity of effect sizes in clinical and laboratory parameters has not been reported, and the influence of immunosuppression mediated by the absence of iNOS pathway activation on systemic and cardiac effects is not well understood.
In general, the host–parasite relationship is directly associated with clinical and laboratory parameters observed at different stages of Chagas disease. These parameters are the result of inflammatory components generated after the invasion by T. cruzi, and limiting factors to the initial or acute phase characterization of the disease are discrepancies in or heterogeneity of clinical and laboratory parameters observed in T. cruzi infection (23). However, different biochemical markers or hematological parameters may consistently reflect the acute state of the disease in study models with controlled management provided that the inoculum concentration or parasite load, the type of parasite strain, and the characteristics of the host are established (22, 24, 25). This relationship is very useful because it makes it possible to assess the prognosis of the infection, where greater changes are associated with worse prognosis. The heterogeneity of the disease at first makes it difficult in clinical practice to relate the changes of laboratory markers with the characteristics of T. cruzi infection and the patient’s immune status. However, we believe that well-established experimental definitions of host–parasite relationships and laboratory markers may help in the development of future computational models or machine learning that will guide the diagnosis and prognosis of T. cruzi infection in humans, as already established for other diseases (26–28).
This study aimed to determine predictors of disease in a controlled model of acute T. cruzi infection by evaluating the relationship between acute infection-mediated effect sizes in clinical and laboratory parameters and different T. cruzi inoculum concentrations in mice with immunosuppression caused by iNOS pathway inactivation. The results helped to understand the aspects of the heterogeneity of the disease and its clinical laboratory relationships.
Materials and Methods
Experimental Design
An explanatory/analytical experimental study was performed in a mouse model of acute T. cruzi infection. All stages of the study were blinded. The animals in each group were allocated randomly using a table of random numbers. The animals were previously subjected to a one-week period of acclimatization in their new groups. Environmental enrichment was performed through the provision of sunflower seeds and changes in food distribution (height and disposition).
The animal groups underwent a new evaluation to ensure equality in weight and food intake between groups and to reduce confounding factors for outcomes post-infection. Research evaluations were performed by at least two evaluators with prior independent training, and data replicability was assessed using the kappa coefficient of agreement (kappa > 0.90). The survey results independently reflect data from duplicate experiments.
Animals
Male C57BL/6 wild-type and iNOS-/- mice (n = 80, 40 animals from each lineage), aged 10 weeks and weighing 20–30 g, were housed in temperature-controlled rooms (22–25°C) with ad libitum access to water and food (Nuvilab-CR1, NUVITAL, Nutrients Veterinary Products Ltda, Curitiba, PR, Brazil) in the animal facilities of the Laboratory of Cell Biology, Institute of Biological and Natural Sciences, Federal University of Triângulo Mineiro (UFTM), Uberaba, Minas Gerais, Brazil. The protocols for all experiments involving mice were evaluated and approved by the UFTM Institutional Animal Care and Use Committee (protocol number 293/2013). No mouse was included in more than one experimental group.
Parasite Strain and Experimental Groups
The “Y” strain of T. cruzi was used in experimental studies. C57BL/6 mice (ten animals per group) were injected subcutaneously with blood-derived trypomastigotes (MHOM/BR/00Y; T. cruzi II) according to methods reported in a previous study (29, 30); the strain was kindly provided by the University of São Paulo (Brazil) and maintained at the Department of Cell Biology at UFTM. The mice used in this study were divided into the following groups: uninfected or infected with 3 × 102 (low), 3 × 103 (medium), or 3 × 104 (high) trypomastigotes (20–22). Each group comprised ten animals.
Parasitemia and Survival
Parasitemia was quantified in infected mice according to Brener’s technique (31). Briefly, were counted parasites present in 50 microscopic fields of a wet preparation containing 5 μL of blood collected from of the distal portion of the tail after cleaning and a small incision (around 3 mm) followed by dressing of the lesion. Microscopic blood parasite examinations were performed daily until day 12 of infection, and results were expressed as the number of parasites per milliliter. In other experiments, mice were infected with 3 × 102, 3 × 103, or 3 × 104 trypomastigotes, and the animals were followed for 22 days to assess the outcome of death after infection. Each death was reported after finding cardiorespiratory arrest and absence of diaphragmatic contraction and arterial pulse.
Sample Collection
We performed 24 h urine collection in metabolic cages (days 11 to 12 of infection). After 12 days of infection the animals were sacrificed (n = 10 animals for each subgroup were evaluated, total n = 80 animals). The animals were fasted for 4 h and heparinized afterwards with 40 units of Hemofol (5000 IU/mL). Unconsciousness and analgesia were induced with carbon dioxide, and all organs (including the heart) and blood (drawn through the ophthalmic plexus) were removed for evaluation. The euthanization procedures were performed in an environment different from the experimentation environment, with no contact among the animals during euthanasia. In addition, the euthanization process occurred simultaneously for at least one animal from each group, selected through randomization.
Histological and Immunohistochemical Analyses
The mouse hearts were washed with 0.9% saline solution at 5°C, and a cross-sectional dissection was performed along the long axis of the ventricle, which produced a 2 mm-thick slice corresponding to one-third of the tissue. The slices were immediately inserted in a solution containing a protease inhibitor and frozen at -80°C for cytokine evaluation. The remaining two-thirds of the cardiac tissue was placed in methacarn for 24 h and then stored in 70% alcohol. Subsequently, the heart tissue was subjected to dehydration with an ethyl alcohol series and diaphanization in xylene and embedded in paraffin for microtomy. Sections with a thickness of 6 µm were obtained in a rotating microtome Leica RM2245 (Leica Microsystems, Wetzlar, Germany) and mounted on slides previously treated with a silane adhesive. The cuts were serialized at 60-µm intervals on the same slide, and 10 slides were obtained with four cuts in each. A part of the tissue was used for staining with hematoxylin (32), and the remaining tissue was used for immunohistochemistry.
After endogenous peroxidase and nonspecific binding blockade and antigen recovery, the sections were treated for 2 h with rabbit anti-T. cruzi antibody (dilution 1:250) at 25°C. Later, the slides were washed with PBS, treated with protein A conjugated with peroxidase (1:100), and developed with DAB-diaminobenzidine in Tris-HCl buffer (pH 7.4). The sections were counterstained with hematoxylin, and the slides were mounted with Entellan for analysis under an ordinary light microscope (33).
For morphometry, the inflammatory infiltrates and T. cruzi nests were quantified using a color digital video camera (Evolution MP 5.0, Media Cybernetics, Rockville, MD, USA) coupled to a light microscope (Eclipse 50i, Nikon, Kawasaki, Japan) that relayed the images to a computer. Images were captured using the ImagePro Plus program (Media Cybernetics) and analyzed using ImageJ software (http://rsb.info.nih.gov/ij/). The images (2560 × 1920 pixels) were calibrated using a blade (Leica) with a ruler with graduations of 2 mm divided into units of 10 µm for a 10× objective for immunohistochemistry and 20× objective for cell quantitation.
For the quantification of cardiac tissue cells and inflammatory infiltrate, 10 images selected at random were used and distributed equally in the right and left ventricular region of each animal in duplicate. Each image had dimensions of 724.45 µm × 543.34 µm and an area of 393,625.63 µm2. In total, an area of 3,936,256.3 µm2 was analyzed, corresponding to 3.93 mm2 per duplicate.
To determine the number of cells, the semi-automatic mode of ImageJ was used after the nuclei of cardiac tissue cells were identified. The cells in the uninfected group were used to standardize the mean number of cells in the tissue, following which the mice infected with different inoculum concentrations were evaluated. The result was expressed in terms of the ratio of the number of cells divided by the area in mm2.
To determine the number of T. cruzi nests, an average of 218 fields per animal were tested, corresponding to a total area of 10.67 mm2. Each area of a field corresponded to 48,858.16 µm2. The images were distributed in equal numbers in the right and left ventricular regions of each animal. In the end, the ratio of the area occupied by T. cruzi nests to the total area analyzed was determined, and the results were expressed in cm2.
Blood Cell and Reticulocyte Count
After collection, 100 μL of whole blood was aliquoted into a tube containing 5 μL of 10% EDTA and analyzed using a hemocytometer (ABX MICROS 60, Horiba ABX Diagnostics, Montpellier, France). This device determined the following hematological parameters: red blood cell count, hematocrit, mean corpuscular volume, hemoglobin level, mean corpuscular hemoglobin, erythrocyte volume distribution amplitude, and total leukocyte count. The result corresponded to the average of two readings from the same sample. Next, blood smear slides stained with Panótico (NewProv, Pinhais, Brazil) were prepared for platelet counting and the leukocyte differential test. Subsequently, 15 μL of whole blood was incubated with 15 μL of brilliant cresyl blue dye (Laborclin, Campo Novo do Parecis, Brazil) at 37°C for 20 min to prepare the slides for reticulocyte counting.
Evaluation of Biochemical Parameters
Blood and urine samples were centrifuged at 1831 × g at 4°C for 10 min to obtain the plasma and supernatant. The plasma concentrations of blood urea nitrogen (BUN), sodium, potassium, chlorine, glutamic-oxaloacetic transaminase (GOT), glutamic-pyruvic transaminase (SGPT), alkaline phosphatase (ALP), creatine phosphokinase (CPK), creatine kinase myocardial band, total protein, albumin, and globulin and the albumin-to-globulin ratio (A/G ratio) were determined. The urinary concentrations of sodium, potassium, chlorine, urea, and creatinine were measured. In addition, the glomerular filtration rate was estimated by determining creatinine clearance in mL/min × 0.006179 (34). Measurements were performed via spectrophotometry using an automated device (COBAS INTEGRA 400, Roche Diagnostics Corp., Indianapolis, IN, USA).
Quality Control
In all stages of the study (pre-analytical, analytical, and post-analytical), internal quality control processes were implemented. The objectives, procedures, standards, criteria for tolerance limits, corrective actions, and registration of activities were reported, and the use of controls for evaluating the imprecision of analyses was stated and monitored. Control charts, such as the Levey-Jennings chart and multiple Westgard Rules, were also used (34, 35).
Cytokine Measurement
Cytokines were measured using an enzyme-linked immunosorbent assay according to the manufacturer’s instructions (OptEIATM Kit, Pharmingen, San Diego, CA, USA). High-affinity polystyrene plates (Corning Costar Europe, Badhoevedorp, The Netherlands) were sensitized with a specific capture antibody for each cytokine (50 mL/well) diluted in 0.1 M carbonate–bicarbonate buffer (pH 9.6) and then incubated for 24 h at 4°C. Next, the plates were washed with PBS-T and incubated with 1× PBS along with 10% inactivated fetal bovine serum (blocking solution) (Sigma-Aldrich, St. Louis, MO, USA) for 1 h at 25°C. Known concentrations of recombinant cytokines (for the standard curve) and the sample to be analyzed were added to 96-well ELISA plates in duplicate and incubated at 25°C for 2 h. Subsequently, the plates were washed with PBS-T. Then, secondary biotinylated antibodies (detection antibody) for each cytokine, pre-incubated for 15 min with peroxidase-conjugated avidin, were diluted; this solution was added to the plates and incubated at 25°C for 1 h. After a washing step, tetramethylbenzidine (Pierce Biotechnology, Waltham, MA, USA) as a developer and H2O2 as substrate were added. The reactions were blocked after 20 min using 2 M sulfuric acid, and readings were measured at 450 nm by a microplate reader (Power Wave X, BioTek Instruments, Inc., Winooski, VT, USA). The concentrations of IL-12 p40, IL-10, IFN-γ, TNF-α, and IL-17 were measured with reference to the standard curve generated from serial dilutions of the recombinant cytokines. Cytokine concentration was expressed in pg/mL/g.
Statistical Analysis
G*Power version 3.1.7 (Uiversität Kiel, Kiel, Germany) was used for sampling estimates and power of inferences. Data were tabulated in the Microsoft® Excel program and analyzed using IBM SPSS Statistics 21 (IBM Corp., Armonk, NY, USA) and jamovi 1.6.15 (36, 37). Survival differences among groups were verified using the log-rank test. Parasitemia and the effects of different concentrations of T. cruzi inocula were evaluated with respect to distribution using the Shapiro–Wilk test and homoscedasticity using Levene’s test. Welch correction was used for cases of unequal variances. A one-way analysis of variance was used with Tukey’s post-test for equal variances or with the Games–Howell test for unequal variances. The effect size was determined using the eta squared (η2) formula.
A multinomial logistic regression model was used to predict and estimate the effects [odds ratios (OR) and confidence intervals (CIs)] of different inoculum concentrations on laboratory parameters and their association with the parasite load. The parasite load was subdivided into four potential categories: undetected (absence of parasites), light (50,000–200,000 parasites/mL), moderate (201,000–500,000 parasites/mL), and high (above 501,000 parasites/mL). Bionomial logistic regression modeling was also performed for the outcome of death in the immunosuppressed subgroup. The parameters selected for modeling fulfilled three criteria: the effects among different inoculum concentrations were statistically significant (p <.05), the power of inferences was >70%, and the parameter exhibited multicollinearity (tolerance of 80%). The Akaike information criterion (AIC), Schwarz Bayesian criterion (BIC), and R² of McKelvey were used to assess the complexity and adherence of the models.
Lastly, the relationship between the effect sizes of the two mouse strains was obtained for each parameter analyzed in the study. To compare possible differences in the distributions of effect sizes for each parameter, the distributions of delta variables (paired samples t-test) were evaluated, and the effect size was determined using Cohen’s d. A significance level of 5% was considered in all analyses (38, 39). The experimental design, database, methods and data analysis were confirmed by a statistician.
Results
Effects of Different T. cruzi Inocula on Parasitemia and Survival of Wild-Type and iNOS-/- C57BL/6 Mice
Parasitemia was evaluated daily until day 12 of infection. Throughout the evaluation period, the mean distribution of the number of parasites/mL was greater for the highest inoculum concentration than for the lower inoculum concentrations for both mouse strains. On the third day after infection, the high groups in both mouse strains tested positive, confirming the presence of T. cruzi. The number of iNOS-/- animals that tested positive was greater than 3× the number of wild-type animals that tested positive. The differences in parasitemia between the two mouse strains followed during the experimentation period. Statistically significant differences between the iNOS-/- profile in the high group and that in the low group were reported on day 5, whereas for the wild-type profile, the same differences were observed on day 6 after infection. The permanence of the parasite load in the iNOS-/- group culminated in the peak of parasitemia on day 10 after infection, whereas in the wild-type group, the peak was inoculum-dependent on days 8 (high), 9 (medium), and 10 (low) (Figures 1A, B).
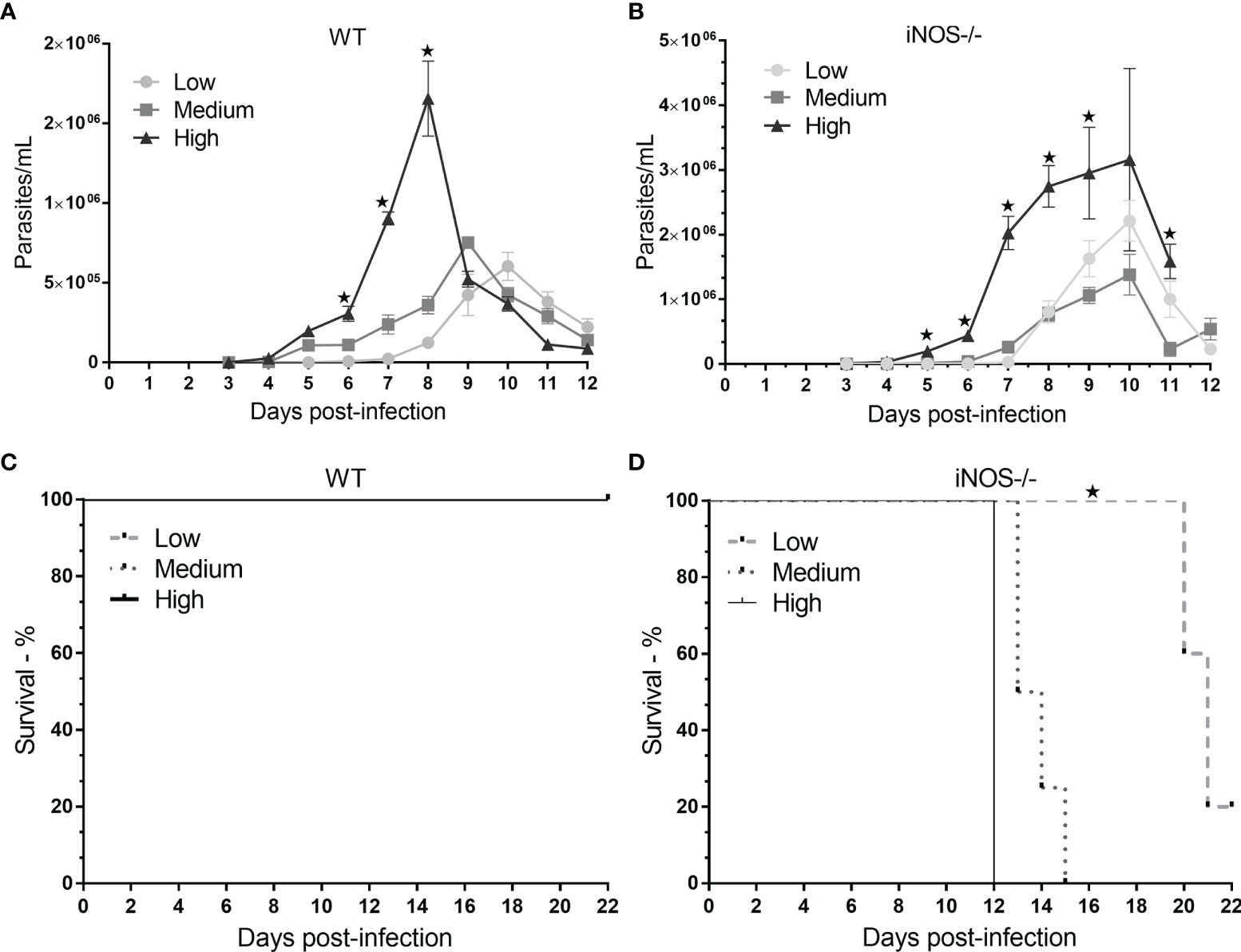
Figure 1 Evaluation of parasitemia and survival in wild-type (WT) and knockout (iNOS-/-) mice infected with different Trypanosoma cruzi inoculum concentrations. C57Bl/6 WT and iNOS-/- mice were infected with low (3 × 102), medium (3 × 103), or high (3 × 104) trypomastigote inoculum concentrations. In (A, B), parasitemia was evaluated daily for the different profiles and research groups for 12 days. The number of parasites per milliliter was expressed in terms of mean and standard deviation. (C, D) shows the survival of the subgroups; the animals were followed until day 22 (censorship point), with the same experimental conditions for each phenotypic profile and inoculum. Survival is represented in percentages. The * represents significant differences (p <.05) (ANOVA followed by Tukey’s or Games–Howell test for parasitemia and log-rank test for survival).
After the parasitemia of each strain was determined, the survival of infected animals was reported until day 22 in a different experiment (Figures 1C, D). The survival pattern was similar between the different experiments. There were no deaths in the wild-type groups, whereas, in the knockout group, 100% of the animals died in the high and medium subgroups after 12 and 15 days of infection, respectively, and 80% of the deaths observed in the low subgroup had occurred by day 22 after infection (p <.05).
Influence of T. cruzi Inoculum Concentration on the Effects of Differences in Clinical Laboratory Markers, Inflammatory, and Histopathological Parameters
Different clinical laboratory markers, inflammatory, and histopathological parameters were evaluated in experimental models of acute Chagas disease to determine the statistical effect (η2) of different parasite loads on infection in immunocompetent and immunosuppressed mice (Table 1). The greatest changes were observed in the subgroups that received a high load of of T. cruzi. In the wild-type C57BL/6 mice lineage, infection was related to a significant increase (p <.05) in BUN levels, plasma GOT, plasma globulin, reticulocytes, and monocytes in blood and inflammatory cytokine levels (TNF-α, IL -10, IFN-γ, IL-12p40, and IL-17) and the number of cells and amastigote nests in cardiac tissues. These alterations were inoculum-dependent. Reduction in different parameters dependent on the T. cruzi inoculums, such as plasma potassium, plasma SGPT, plasma ALP, plasma total protein, plasma albumin, A/G ratio, urinary sodium, urinary potassium, urinary urea, erythrocytes, hemoglobin, hematocrit, total leukocyte count, and lymphocyte count (p <.05), was also observed.
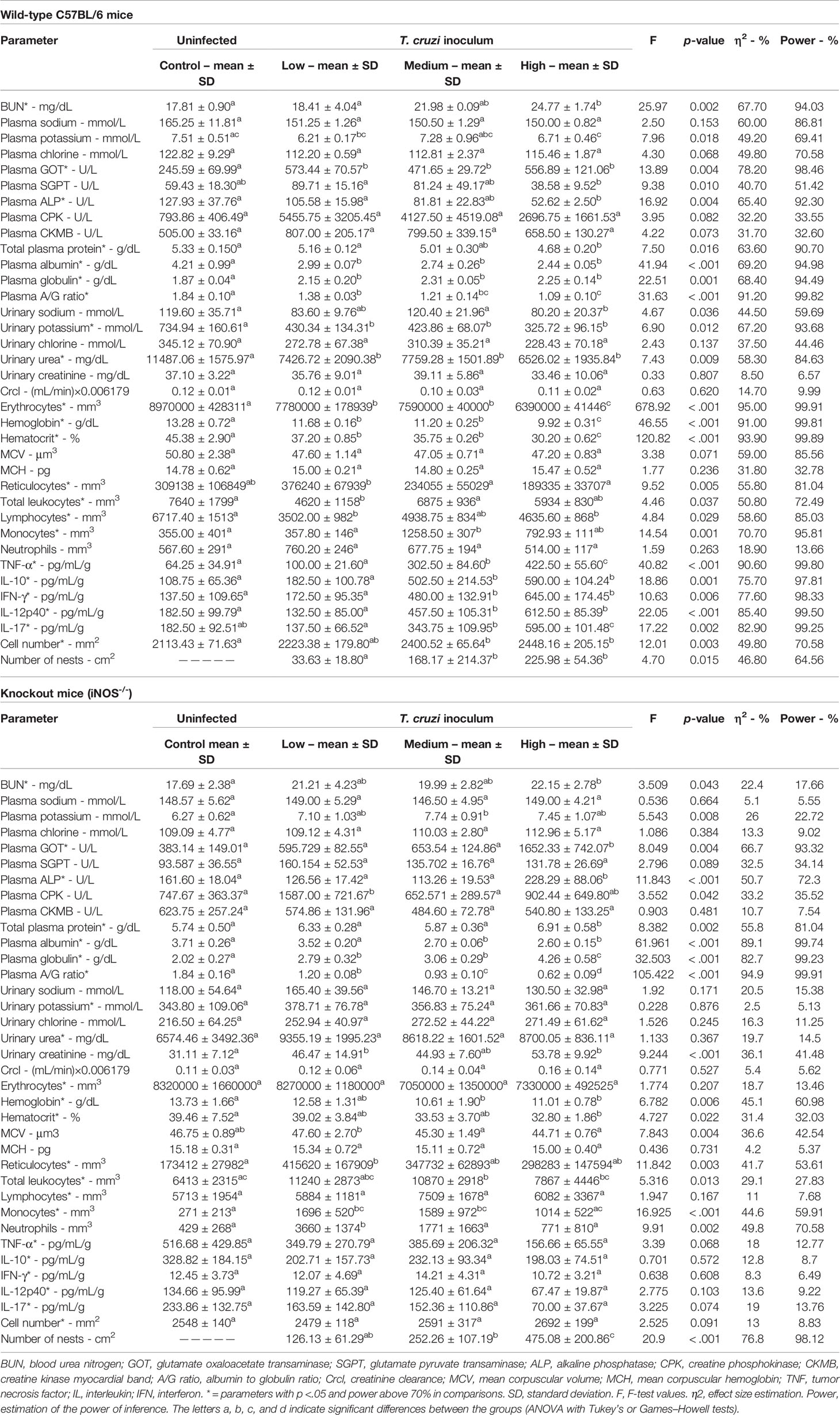
Table 1 Effects of different Trypanosoma cruzi inoculum concentrations (low, medium, and high) of the “Y” strain on clinical laboratory markers, inflammatory, and histopathological parameters in wild-type C57BL/6 and knockout (iNOS-/-) mice in the acute phase of infection (after 12 days of infection).
In the population of immunosuppressed animals (iNOS-/-), similar to that in the population of immunocompetent animals, inoculum-dependent increases in the levels of BUN, plasma GOT, and plasma globulin and the numbers of reticulocytes, monocytes, and amastigote nests were observed, alongside decreases in plasma albumin, A/G ratio, hemoglobin, and hematocrit (p <.05). However, unlike the wild-type animal subgroup, the knockout animal subgroup showed significant inoculum concentration-dependent increases in the levels of plasma potassium, ALP, CPK, and total protein; urinary creatinine; total leukocytes; and neutrophilic cell count (p <.05) with the onset of infection. The power for each inference was determined, as was the effect size for the observed differences (Table 1).
Association of Clinical Laboratory Markers, Inflammatory, and Histopathological Parameters With the T. cruzi Load in Acute Infection in the Blood of Wild-Type C57BL/6 and Knockout (iNOS-/-) Mice Infected With Different Inoculum Concentrations
After evaluating and determining the effects of different T. cruzi inoculum concentrations on clinical and laboratory parameters, the variables that showed significant differences between the subgroups with an estimated power ≥70% were selected to compose a predictive model of the rates of T. cruzi infection in the blood; the reticulocyte parameter was excluded based on the collinearity effect presented in the distributions (Table 2).
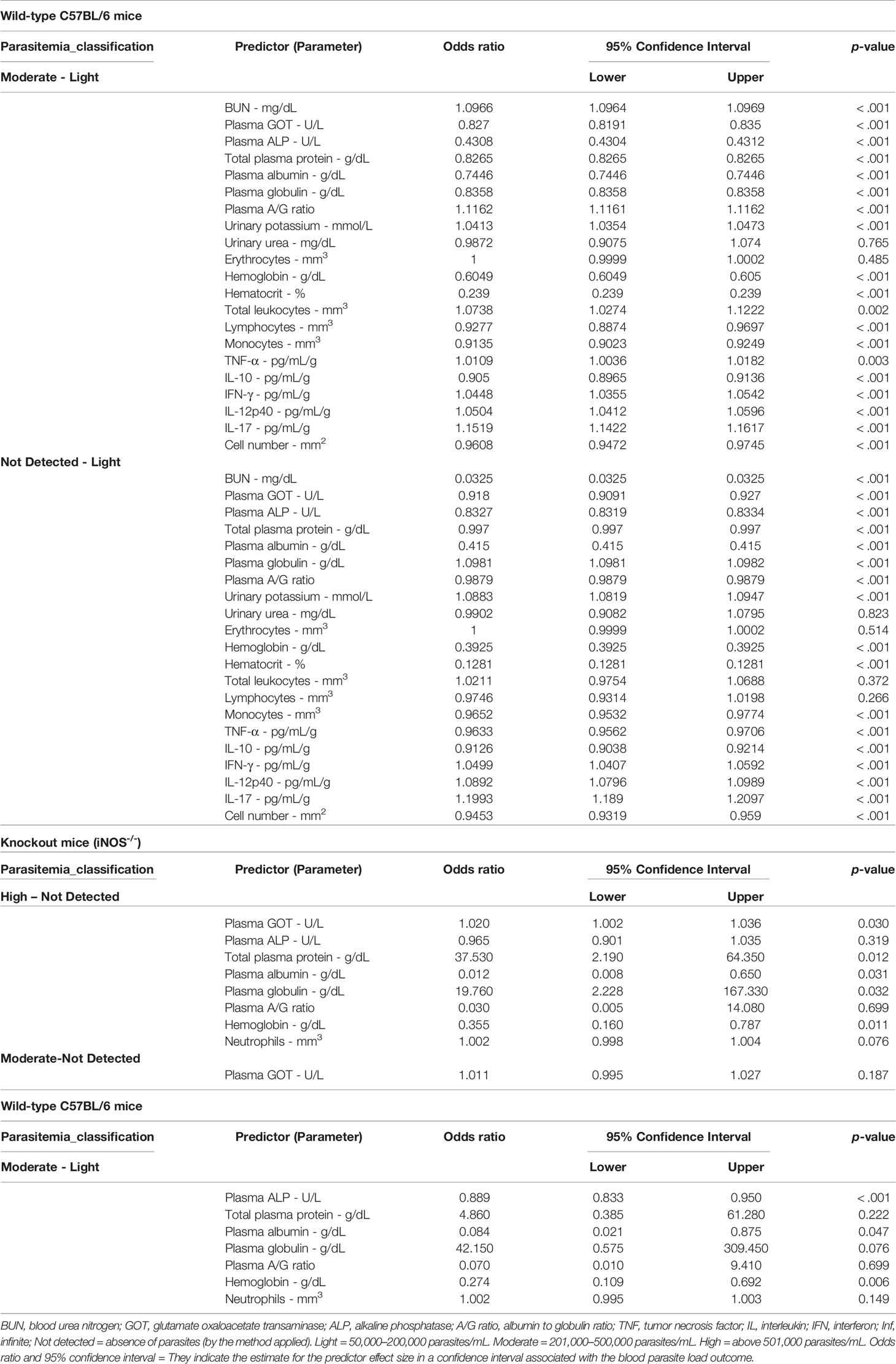
Table 2 Estimation of parameters in a multinomial logistic regression model for the classification of Trypanosoma cruzi (strain “Y”) load in the blood (undetected, light, moderate, and high) in wild-type C57BL/6 and knockout (iNOS-/-) mice.
Given the large number of parameters, the model was considerably complex. For the wild-type C57BL/6 group, AIC = 64, BIC = 111, and R² of McKelvey = 1, the “high” categorie was not found. The model could be fitted better using only parameters directly related to immunity, such as leukocyte count and cytokine levels (AIC = 40; BIC = 69.30). In the knockout mice group, the model showed AIC = 36, BIC = 56.40, and R² of McKelvey = 1, the “light” category was not found. In the knockout group, the model was affected by numerical variations within each explanatory variable. Heterogeneity within the explanatory variables caused amplitude expansion of confidence intervals. The variability was a reflection of the immunological imbalance expected for the biological model of infection.
In the immunocompetent group, animals with mild and moderate parasitemia could be identified. Increases in some parameters were related to an increased likelihood of enhanced parasitemia for the moderate classification (201 to 500 thousand parasites/mL). The moderate classification of parasitemia was associated with increased BUN levels, A/G ratio, urinary potassium, total leukocyte content, and levels of the cytokines TNF-α, IFN-γ, IL-12p40, and IL-17 (Table 2). Furthermore, decreases in some parameters, such as plasma GOT, plasma ALP, total plasma protein, plasma albumin, plasma globulin, hemoglobin, hematocrit, lymphocyte count, monocyte count, IL-10, and cardiac tissue cell number, were linked to greater chances of more severe parasitemia under the moderate classification (p <.001) (Table 2).
Decreases in BUN levels, plasma GOT, plasma ALP, plasma total protein, plasma albumin, the A/G ratio, hemoglobin, hematocrit, monocytes, TNF-α levels, IL-10 levels, and cardiac tissue cell number and increases in plasma globulin, urinary potassium, IFN-γ levels, IL-12p40 levels, and IL-17 levels were used to distinguish animals without parasite detection from animals presenting with mild parasitemia (p <.001) (Table 2).
In the immunosuppressed group, the increase in plasma GOT, total plasma protein and plasma globulin levels was related to an increase in parasitemia to levels >501,000 per mL (high classification) (p <.05). Furthermore, decreased albumin and hemoglobin were associated with an high parasite load in the blood (p <.05). The plasma ALP, albumin and hemoglobin showed good sensitivity to the increase in parasitemia since its reduction was related to moderated blood parasite loads (p <.05) (Table 2).
Association of Laboratory Parameters With the Outcome of Death in Mice With iNOS-Mediated Immunosuppression
The association of specific laboratory parameters with the outcome of death in the knockout animal subgroup could be estimated after the relationship of T. cruzi infection with different inoculum concentrations and the effect sizes for each of these variables were determined. A power of inferences ≥70% was used. Ten parameters were used, and although not all variables were directly associated with death, the model showed good explanatory adherence (AIC = 22, BIC = 34.5, and R² of McKelvey = 1).
The T. cruzi load in the blood (the mean number of parasites during infection) was a determining factor for death, as an increase in the number of parasites by 1 increased the chances of death by 1%. Elevations in plasma GOT levels and the plasma globulin ratio were also positively associated with death. Conversely, a protective effect was associated with increases in the plasma albumin concentration, A/G ratio, and hemoglobin levels, whereas decreases in these parameters were associated with death (p <.05) (Table 3).
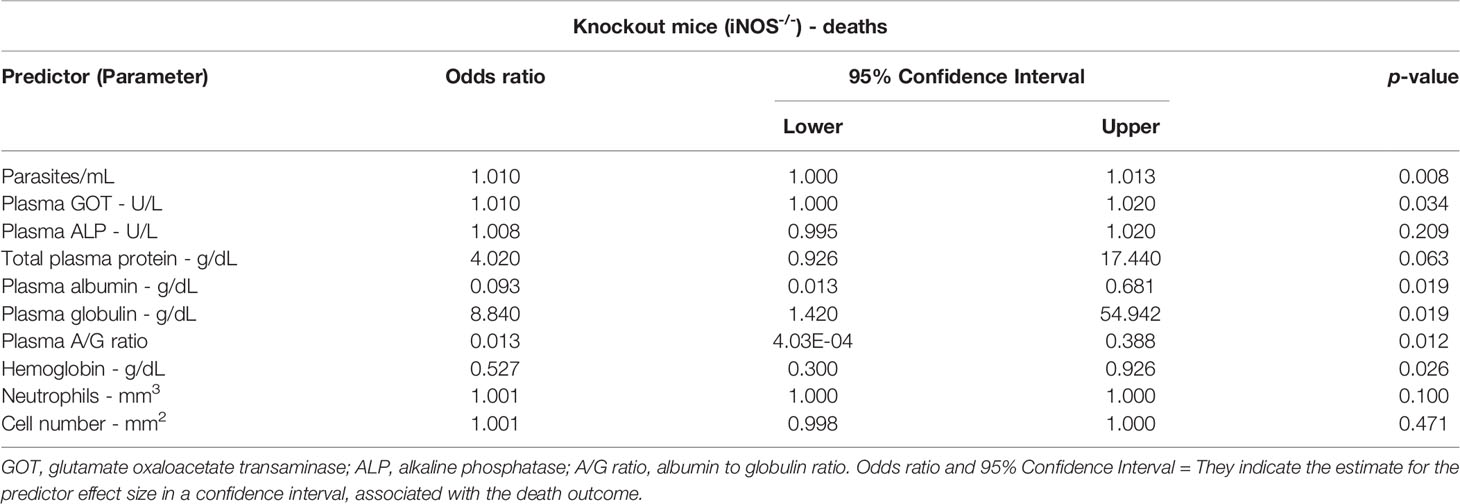
Table 3 Binomial logistic regression model to estimate parameters associated with the outcome of death in knockout mice (iNOS-/-) infected with different inoculum concentrations of Trypanosoma cruzi (“Y” strain) in the acute phase (after 12 days of infection).
Effect of iNOS Immunosuppression on Clinical Laboratory Markers, Inflammatory, and Histopathological Parameters
After the effect sizes to each parameter evaluated under acute T. cruzi infection in each subgroup (wild-type and knockout) were determined, the impact of immunosuppression on each effect size could be assessed (Table 4).
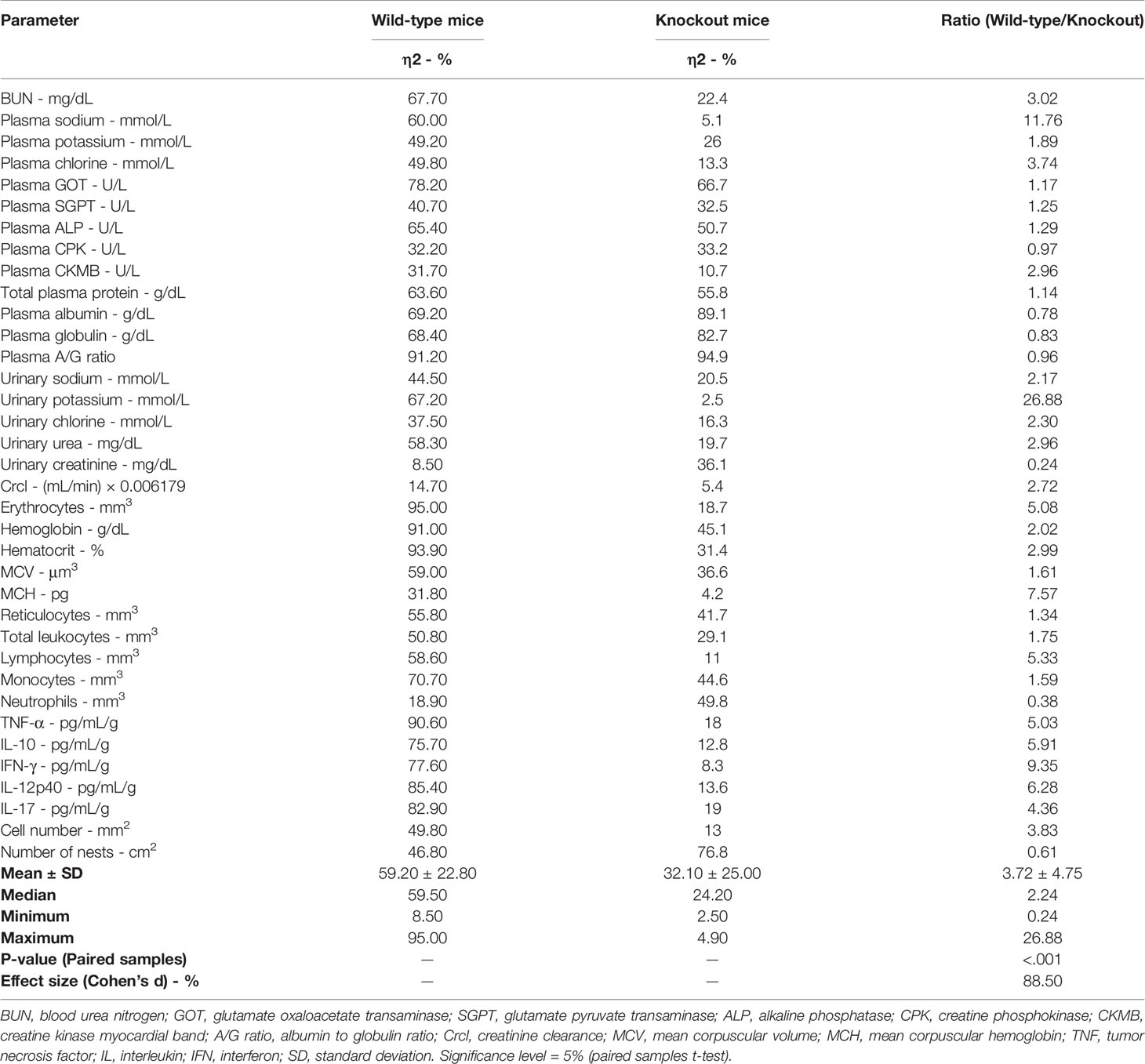
Table 4 Determination of differences in the effects of Trypanosoma cruzi inoculum concentrations (low, medium, and high) of “Y” strain on clinical laboratory markers, inflammatory, and histopathological parameters between wild-type and knockout (iNOS-/-) mice with acute infection.
In paired analysis, significant differences were observed between the effect sizes of the two strains (p <.001), with a Cohen’s d of 88.50%. The mean of effect sizes for the wild-type subgroup was 59.20 (SD = ± 22.80), whereas for the knockout group it was 32.10 (SD = ± 25.00), with a difference of 54.22% between the means. For 29 of the 36 parameters evaluated (80.55%), the relationship between the effect sizes in the wild-type and knockout groups was greater than 1 (Table 4).
Conversely, the effect sizes for plasma CPK (1.03×), plasma albumin (1.29×), plasma globulin (1.21×), the A/G ratio (1.04), urinary creatinine (4.25×), neutrophil count (2.63×), and cardiac tissue cell number (1.64×) were higher in the immunosuppressed subgroup (Table 4).
Discussion
Chagas disease is a diversified form of anthropozoonosis in terms of its ability to generate lesions owing to variability in pathophysiological processes. Attempts have been made to elucidate different mechanisms underlying the pathogenesis of Chagas disease to develop more assertive interventions or efficient prophylactic measures. In the present study, we reported the impact of T. cruzi inoculum concentration on the effect sizes of clinical laboratory parameters in acute infection in mice with immunosuppression due to iNOS pathway inactivation.
The imbalance of laboratory markers or the intensity of inflammation in acute T. cruzi infection was observed to be inoculum-dependent: the greater the inoculum load, the more severe the changes observed. High inoculum concentrations have previously been reported to correspond to a greater degree of changes in immunocompetent mice, although the responsiveness was reported to be greater at intermediate (medium) or low concentrations in some studies (20–22). Some parameters in immunocompetent animals garnered attention because their effect sizes were affected by more than 80% by the inoculum; some examples are the decreases in the A/G ratio (91.20%) or hematological parameters such as the number of erythrocytes (95%), hemoglobin concentration (91%), hematocrit percentage (93.90%), and concentrations of proinflammatory cytokines such as TNF-α (90.60%) and IL-17 (82.90%).
In the present study, two factors were observed to contribute to a reduction in the A/G ratio. The first was the reduction in plasma albumin concentrations, which may have resulted from an increase in the mobilization of amino acid residues for the synthesis of other proteins and an increase in albumin uptake for mediating different functions, primarily transport. In a study conducted on children under 13 years of age in the acute phase of Chagas disease in an endemic area of Bolivia, a significant increase in alpha-2-macroglobulin and C-reactive protein concentrations was observed (40); this mobilization probably directly affected the albumin levels. The elevation in globulin concentration was an additional indirect contributing factor in the relationship observed in our study. Globulin concentration is expected to be elevated in certain types of infections, including T. cruzi infection, wherein there is a consistent increase in the γ-globulin fraction (41, 42), which also affects the reduction in the A/G ratio. Furthermore, we believe that the monitoring and regulation of albumin concentration, if necessary, can favor certain metabolic processes in patients with acute Chagas disease, as has been reported in interactions involved in the mobilization of hematopoietic stem cells in mice (43); however, other types of research, including clinical studies, are warranted.
Hematological alterations caused by T. cruzi infection were reported in an evaluation of the medical records of 103 patients in Colombia; the authors observed anemia, along with leukocytosis (17.4% of patients), leukopenia (7.7% of patients), and increased GOT (68.9% of patients), SGPT (50.5%), and creatinine (48.5%) levels in 22.3% of patients (23). In the present study, we confirmed the relationship between hematological effects and T. cruzi infection and reported the variability of these effects based on T. cruzi inoculum concentration. One of the factors potentially affecting the parameters of the red blood cells is the elevation in the levels of cytokines, such as TNF-α. TNF-α has previously been reported to be associated with the onset of anemia owing to its ability to decrease the survival of erythrocytes and affect the medullary bioavailability of iron (44). The onset of anemia is observed in inflammatory bowel disease, wherein TNF-α concentrations also increase; anti-TNF monoclonal antibody therapy reportedly improves the anemic state in this disease (45).
Several systemic and local changes observed in acute T. cruzi infection are mediated by the expression and release of pro-inflammatory cytokines. Observed in the initial phase of the infection are typical macrophage activity, driven by IFN-γ elevation and potentiated by TNF-α activity, and elevation in the levels of other cytokines, such as IL-17, in response to parasitemia (46). The intensity of the immune response is influenced by the availability of antigens with high immunogenicity and the host’s responsive capacity and thus varies for different T. cruzi strains and host profiles. In addition, the present study demonstrated the discrepancies in the concentrations of proinflammatory cytokines among subgroups infected with different inoculum concentrations. Cytokine elevation is important for reactive oxygen species (ROS) generation and for increasing the bioavailability of cytokines in phagosomes containing the parasite (14). Consequently, it is essential to assess the impact of these alterations in animals immunosuppressed via inactivation of the iNOS pathway, which participates in the production of ROS, including NO (47).
NO affects T. cruzi by chemically modifying cysteine-containing proteins and/or by binding to metalloproteins that mediate crucial metabolic processes (10). Other mechanisms that contribute to increased free radical production as well as parasitemia control are phagocytic activity and pathogen pattern recognition, which trigger the assembly of the NADPH oxidase 2 complex and induce the formation of flavocytochrome b558. The active site of the NADPH oxidase 2 complex is oriented toward the phagosome lumen and catalyzes high levels of superoxide production at the expense of oxygen and NADPH; this induces moderate direct toxicity against T. cruzi. In addition, the pathway contributes to the generation of second derivatives of ROS such as hydrogen peroxide, which, at high concentrations, promote critical oxidative modifications (48–50). Conversely, among the different mechanisms adopted, the parasite’s adaptations for immune system evasion indicate its attempt to regulate the iNOS expression pathway. The growth of T. cruzi in macrophages is dependent on several factors, including its ability to upregulate arginase activity, which consequently leads to competition with iNOS for L-arginine, leading to production of L-ornithine and urea and reduction in NO levels (51, 52). However, despite the clear association between increases in ROS levels and control of parasitemia, a relationship has been established between the absence of ROS and decreases in intracellular parasite multiplication in macrophages (5), which generates doubts about the actual role of the effects of ROS in the infection. Another theory suggests that the increase in parasitism may be associated with the induction of the Wnt signaling pathway by T. cruzi. On one hand, the activation of the Wnt pathway corresponds to the replication of the parasite, and on the other hand, the inhibition of the pathway restricts replication and weakens the induction of lethality (53).
In the model of acute T. cruzi infection induced by iNOS knockout, similar changes were observed in albumin concentrations, globulin concentrations, and the A/G ratio: albumin concentrations and the A/G ratio decreased, while globulin concentrations increased in an inoculum concentration-dependent manner with effect sizes greater than 80%. Unlike the immunocompetent subgroup, the knockout subgroup did not present effect sizes greater than 80% for any other parameter evaluated.
The wild-type subgroup showed peak parasitemia for the highest inoculum concentration on day 8 after infection, whereas a similar concentration was observed in the knockout subgroup on day 6 of infection, and the peak extended to day 10. In addition to the blood parasites, the amastigote nests in the knockout subgroup occupied a larger area (effect size = 76.8%) than those in the wild-type subgroup. These changes influenced the mortality rates in the knockout subgroup.
The role of deficient iNOS-mediated NO production in the susceptibility of experimental models to T. cruzi infection is conflicting. In a study evaluating an iNOS-/- model infected with a reticulotropic lineage strain (T. cruzi Tulahuen) for 17 days, the authors reported high susceptibility owing to the absence of NO production, with deaths caused even by intraperitoneal inoculum of low concentrations, and severe histopathological changes (12). Conversely, another study reported that iNOS deficiency was not a limiting factor for resistance to T. cruzi infection compared with resistance in wild animals (54). In our evaluation, the absence of iNOS pathway activation affected the relationship between the effect sizes of clinical and laboratory parameters relative to that in the immunocompetent model. In the immunosuppressive model established via iNOS pathway inactivation, peripheral monocytes were mobilized, but the inflammatory activity induced by cytokines and associated with cell migration to the target tissues was noticeably affected.
The reduction in the levels of parameters associated with NO production via iNOS was also associated with greater vulnerability to T. cruzi infection. Reportedly, supplementation with L-arginine increased the effectiveness of immune responses to parasitemia; L-arginine is a semi-essential amino acid necessary for cell proliferation and is the substrate of arginase 1 and iNOS (55).
The major limitations of this study are associated with the molecular complexity of T. cruzi infection and the development of Chagas disease. The modification of the evaluated lineage or host immune profile can be linked to other effect sizes with the same parameters described here. Likewise, the effect sizes reported in this study will have limited reproducibility in human models of Chagas disease. However, modeling strategies that take into account the discrepancies in clinical and laboratory parameters can be optimized for and applied to future standardizations, and the models can also be used as indicators of the parasite–host relationship as well as disease prognosis. Therefore, the present study not only contributes to the understanding of the physiopathogenic mechanisms underlying T. cruzi infection but also indicate the influence of the concentration of T. cruzi during infection and the immunosuppression through the iNOS pathway in clinical laboratory heterogeneity reported in acute Chagas disease.
Data Availability Statement
The original contributions presented in the study are included in the article/supplementary material. Further inquiries can be directed to the corresponding author.
Ethics Statement
The protocols for all experiments involving mice were evaluated and approved by the UFTM Institutional Animal Care and Use Committee (protocol number 293/2013).
Author Contributions
JL-C designed the experiments. WR, CM, LM, TC, and MA performed the experiments. WR, CM, CO, and JL-C analyzed the data. WR, CM, LM, TC, MA, CO, and JL-C wrote the manuscript. All authors contributed to the article and approved the submitted version.
Funding
CM received doctoral fellowships from CAPES. WR received postdoctoral fellowships from the National Postdoctoral Program of CAPES (Social Demand/PNPD/CAPES). The funders had no role in the study design, data collection, and analysis, decision to publish, or preparation of the manuscript.
Conflict of Interest
The authors declare that the research was conducted in the absence of any commercial or financial relationships that could be construed as a potential conflict of interest.
Publisher’s Note
All claims expressed in this article are solely those of the authors and do not necessarily represent those of their affiliated organizations, or those of the publisher, the editors and the reviewers. Any product that may be evaluated in this article, or claim that may be made by its manufacturer, is not guaranteed or endorsed by the publisher.
Acknowledgments
The authors thank Prof. Dr. João Santana da Silva for providing iNOS-/- knockout mice through the University of São Paulo at Ribeirão Preto (Faculty of Medicine).
Abbreviations
iNOS, inducible nitric oxide synthetase; NO, nitric oxide; IFN-γ, interferon-gamma; ROS, reactive oxygen species; BUN, blood urea nitrogen; GOT, glutamic-oxaloacetic transaminase; SGPT, glutamic-pyruvic transaminase; ALP, alkaline phosphatase; CPK, creatine phosphokinase; A/G ratio, albumin-to-globulin ratio; AIC, Akaike information criterion; BIC, Schwarz Bayesian criterion; OR, odds ratio; CI, confidence interval.
References
1. Cordero-Montoya G, Flores-Villegas AL, Salazar-Schettino PM, Vences-Blanco MO, Rocha-Ortega M, Gutiérrez-Cabrera AE, et al. The Cost of Being a Killer's Accomplice: Trypanosoma Cruzi Impairs the Fitness of Kissing Bugs. Parasitol Res (2019) 118:2523–9. doi: 10.1007/s00436-019-06413-8
2. Messenger LA, Miles MA, Bern C. Between a Bug and a Hard Place: Trypanosoma Cruzi Genetic Diversity and the Clinical Outcomes of Chagas Disease. Expert Rev Anti Infect Ther (2015) 13:995–1029. doi: 10.1586/14787210.2015.1056158
3. World Health Organization. Chagas Disease (2021). Available at: https://www.who.int/news-room/fact-sheets/detail/chagas-disease-(american-trypanosomiasis) (Accessed July 05, 2021).
4. Kierszenbaum F, Knecht E, Budzko DB, Pizzimenti MC. Phagocytosis: A Defense Mechanism Against Infection With Trypanosoma Cruzi. J Immunol (1974) 112:1839–44.
5. Goes GR, Rocha PS, Diniz AR, Aguiar PH, Machado CR. And Vieira, L.QTrypanosoma Cruzi Needs a Signal Provided by Reactive Oxygen Species to Infect Macrophages. PloS Negl Trop Dis (2016) 10:e0004555. doi: 10.1371/journal.pntd.0004555
6. Romano PS, Cueto JA, Casassa AF, Vanrell MC, Gottlieb RA, Colombo MI. Molecular and Cellular Mechanisms Involved in the Trypanosoma Cruzi/Host Cell Interplay. IUBMB Life (2012) 64:387–96. doi: 10.1002/iub.1019
7. Cardillo F, de Pinho RT, Antas PR, Mengel J. Immunity and Immune Modulation in Trypanosoma Cruzi Infection. Pathog Dis (2015) 73:ftv082. doi: 10.1093/femspd/ftv082
8. Golden JM, Tarleton RL. Trypanosoma Cruzi: Cytokine Effects on Macrophage Trypanocidal Activity. Exp Parasitol (1991) 72:391–402. doi: 10.1016/0014-4894(91)90085-b
9. Cerbán FM, Stempin CC, Volpini X, Carrera Silva EA, Gea S, Motran CC. Signaling Pathways That Regulate Trypanosoma Cruzi Infection and Immune Response. Biochim Biophys Acta Mol Basis Dis (2020) 1866:165707. doi: 10.1016/j.bbadis.2020.165707
10. Gutierrez FR, Mineo TW, Pavanelli WR, Guedes PM, Silva JS. The Effects of Nitric Oxide on the Immune System During Trypanosoma Cruzi Infection. Mem Inst Oswaldo Cruz (2009) 104 Suppl 1:236–45. doi: 10.1590/s0074-02762009000900030
11. Mesías AC, Garg NJ, Zago MP. Redox Balance Keepers and Possible Cell Functions Managed by Redox Homeostasis in Trypanosoma Cruzi. Front Cell Infect Microbiol (2019) 20:435. doi: 10.3389/fcimb.2019.00435
12. Hölscher C, Köhler G, Müller U, Mossmann H, Schaub GA, Brombacher F. Defective Nitric Oxide Effector Functions Lead to Extreme Susceptibility of Trypanosoma Cruzi-Infected Mice Deficient in Gamma Interferon Receptor or Inducible Nitric Oxide Synthase. Infect Immun (1998) 66:1208–15. doi: 10.1128/IAI.66.3.1208-1215.1998
13. Gibaldi D, Vilar-Pereira G, Pereira IR, Silva AA, Barrios LC, Ramos IP, et al. CCL3/macrophage Inflammatory Protein-1α Is Dually Involved in Parasite Persistence and Induction of a TNF-And Ifnγ-Enriched Inflammatory Milieu in Trypanosoma Cruzi-Induced Chronic Cardiomyopathy. Front Immunol (2020) 11:306. doi: 10.3389/fimmu.2020.00306
14. Silva JS, Vespa GN, Cardoso MA, Aliberti JC, Cunha FQ. Tumor Necrosis Factor Alpha Mediates Resistance to Trypanosoma Cruzi Infection in Mice by Inducing Nitric Oxide Production in Infected Gamma Interferon-Activated Macrophages. Infect Immun (1995) 63:4862–7. doi: 10.1128/iai.63.12.4862-4867.1995
15. Zingales B. Trypanosoma Cruzi Genetic Diversity: Something New for Something Known About Chagas Disease Manifestations, Serodiagnosis and Drug Sensitivity. Acta Trop (2018) 184:38–52. doi: 10.1016/j.actatropica.2017.09.017
16. Domingues CS, Cardoso FO, Hardoim DJ, Pelajo-Machado M, Bertho AL, Calabrese K. Host Genetics Background Influence in the Intragastric Trypanosoma Cruzi Infection. Front Immunol (2020) 11:566476. doi: 10.3389/fimmu.2020.566476
17. Caldas IS, Menezes A, Diniz LF, Nascimento Á, Novaes RD, Caldas S, et al. Parasitaemia and Parasitic Load Are Limited Targets of the Aetiological Treatment to Control the Progression of Cardiac Fibrosis and Chronic Cardiomyopathy in Trypanosoma Cruzi-Infected Dogs. Acta Trop (2019) 189:30–8. doi: 10.1016/j.actatropica.2018.09.015
18. Covarrubias C, Cortez M, Ferreira D, Yoshida N. Interaction With Host Factors Exacerbates Trypanosoma Cruzi Cell Invasion Capacity Upon Oral Infection. Int J Parasitol (2007) 37:1609–16. doi: 10.1016/j.ijpara.2007.05.013
19. Teixeira AR, Hecht MM, Guimaro MC, Sousa AO, Nitz N. Pathogenesis of Chagas' Disease: Parasite Persistence and Autoimmunity. Clin Microbiol Rev (2011) 24:592–630. doi: 10.1128/CMR.00063-10
20. Borges DC, Araújo NM, Cardoso CR, Lazo Chica JE. Different Parasite Inocula Determine the Modulation of the Immune Response and Outcome of Experimental Trypanosoma Cruzi Infection. Immunology (2013) 138:145–56. doi: 10.1111/imm.12022
21. Vazquez BP, Vazquez TP, Miguel CB, Rodrigues WF, Mendes MT, de Oliveira CJ, et al. Inflammatory Responses and Intestinal Injury Development During Acute Trypanosoma Cruzi Infection Are Associated With the Parasite Load. Parasitol Vectors (2015) 8:206. doi: 10.1186/s13071-015-0811-8
22. Lemos JR, Rodrigues WF, Miguel CB, Parreira RC, Miguel RB, de Paula Rogerio A, et al. Influence of Parasite Load on Renal Function in Mice Acutely Infected With Trypanosoma Cruzi. PloS One (2013) 8:e71772. doi: 10.1371/journal.pone.0071772
23. Rincón-Acevedo CY, Parada-García AS, Olivera MJ, Torres-Torres F, Zuleta-Dueñas LP, Hernández C, et al. Clinical and Epidemiological Characterization of Acute Chagas Disease in Casanare, Eastern Colombi–2020. Front Med (Lausanne) (2021) 8:681635. doi: 10.3389/fmed.2021.681635
24. Duz AL, Vieira PM, Roatt BM, Aguiar-Soares RD, Cardoso JM, Oliveira FC, et al. The TcI and TcII Trypanosoma Cruzi Experimental Infections Induce Distinct Immune Responses and Cardiac Fibrosis in Dogs. Mem Inst Oswaldo Cruz (2014) 109:1005–13. doi: 10.1590/0074-02760140208
25. Philot Pavão B, Demarque KC, Meuser Batista M, Melo de Oliveira G, França da Silva C, Guedes da Silva FH, et al. Impact of Autologous Whole Blood Administration Upon Experimental Mouse Models of Acute Trypanosoma Cruzi Infection. J Venom Anim Toxins Incl Trop Dis (2018) 24:25. doi: 10.1186/s40409-018-0157-8
26. De Bruyne S, Speeckaert MM, Van Biesen W, Delanghe JR. Recent Evolutions of Machine Learning Applications in Clinical Laboratory Medicine. Crit Rev Clin Lab Sci (2020) 58:131–52. doi: 10.1080/10408363.2020.1828811
27. Pulgar-Sánchez M, Chamorro K, Fors M, Mora FX, Ramírez H, Fernandez-Moreira E, et al. Biomarkers of Severe COVID-19 Pneumonia on Admission Using Data-Mining Powered by Common Laboratory Blood Tests-Datasets. Comput Biol Med (2021) 136:104738. doi: 10.1016/j.compbiomed.2021.104738
28. Molina A, Alférez S, Boldú L, Acevedo A, Rodellar J, Merino A. Sequential Classification System for Recognition of Malaria Infection Using Peripheral Blood Cell Images. J Clin Pathol (2020) 73:665–70. doi: 10.1136/jclinpath-2019-206419
29. Da Silva LHP, Nussenzweig V. A Strain of Trypanosoma Cruzi Highly Virulent for Mice. Folia Clin Biol (1953) 20:191–207.
30. Andrade SG, Magalhães JB. Biodemes and Zymodemes of Trypanosoma Cruzi Strains: Correlations With Clinical Data and Experimental Pathology. Rev Soc Bras Med Trop (1997) 30:27–35. doi: 10.1590/s0037-86821997000100006
31. Brener Z. Therapeutic Activity and Criterion of Cure on Mice Experimentally Infected With Trypanosoma cruzi. Rev Inst Med Trop Sao Paulo (1962) 4:389–96.
32. Bancroft JD, Gamble M. Theory and Practice of Histological Techniques. New York, NY, USA: Elsevier Health Sciences (2008).
33. Miguel CB, Aparecido da silva T, Rodrigues WF, Oliveira-Brito PKM, Roque-Barreira MC, Lazo-Chica JE. Administration of Artinm Lectin Reduces the Severity of the Acute Phase Infection With Trypanosoma Cruzi. FASEB Bioadv (2021) 3:295–304. doi: 10.1096/fba.2020-00065
34. Rodrigues WF, Miguel CB, Napimoga MH, Oliveira CJ, Lazo-Chica JE. Establishing Standards for Studying Renal Function in Mice Through Measurements of Body Size-Adjusted Creatinine and Urea Levels. BioMed Res Int (2014) 2014:872827. doi: 10.1155/2014/872827
35. Westgard JO, Barry PL, Hunt MR, Groth T. A Multi-Rule Shewhart Chart for Quality Control in Clinical Chemistry. Clin Chem (1981) 27:493–501. doi: 10.1093/clinchem/27.3.493
37. The Jamovi Project. Jamovi. (Version 1.6) (2021). Available at: https://www.jamovi.org.
38. R Core Team. R: A Language and Environment for Statistical Computing. (Version 4.0) (2020). Available at: https://cran.r-project.org (Accessed July 20, 2021). R packages retrieved from MRAN snapshot 2020-08-24.
39. Arango HG. Bioestatística: Teórica E Computacional. Rio de Janeiro: Editora Guanabara Koogan S.A (2001).
40. Medrano NM, Luz MR, Cabello PH, Tapia GT, Van Leuven F, Araújo-Jorge TC. Acute Chagas' Disease: Plasma Levels of Alpha-2-Macroglobulin and C-Reactive Protein in Children Under 13 Years in a High Endemic Area of Bolivia. J Trop Pediatr (1996) 42:68–74. doi: 10.1093/tropej/42.2.68
41. Berra HH, Piaggio E, Revelli SS, Luquita A. Blood Viscosity Changes in Experimentally Trypanosoma Cruzi-Infected Rats. Clin Hemorheol Microcirc (2005) 32:175–82.
42. Luquetti AO, Schijman AG. Diagnosis of Chagas Disease. In: Altcheh J, Freilij H, editors. Chagas Disease. Birkhäuser Advances in Infectious Diseases. Cham, Switzerland: Springer International Publishing (2019). p. 141–58.
43. Danner E, Bonig H, Wiercinska E. Albumin Modifies Responses to Hematopoietic Stem Cell Mobilizing Agents in Mice. Cells (2020) 9:4. doi: 10.3390/cells9010004
44. Moldawer LL, Marano MA, Wei H, Fong Y, Silen ML, Kuo G, et al. Cachectin/tumor Necrosis Factor-Alpha Alters Red Blood Cell Kinetics and Induces Anemia In Vivo. FASEB J (1989) 3:1637–43. doi: 10.1096/fasebj.3.5.2784116
45. Shu W, Pang Z, Xu C, Lin J, Li G, Wu W, et al. Anti-TNF-α Monoclonal Antibody Therapy Improves Anemia Through Downregulating Hepatocyte Hepcidin Expression in Inflammatory Bowel Disease. Mediators Inflamm (2019) 2019:4038619. doi: 10.1155/2019/4038619
46. Miyazaki Y, Hamano S, Wang S, Shimanoe Y, Iwakura Y, Yoshida H. IL-17 Is Necessary for Host Protection Against Acute-Phase Trypanosoma Cruzi Infection. J Immunol (2010) 185:1150–7. doi: 10.4049/jimmunol.0900047
47. Rojas Márquez JD, Ana Y, Baigorrí RE, Stempin CC, Cerban FM. Mammalian Target of Rapamycin Inhibition in Trypanosoma Cruzi-Infected Macrophages Leads to an Intracellular Profile That Is Detrimental for Infection. Front Immunol (2018) 20:313. doi: 10.3389/fimmu.2018.00313
48. Martínez A, Prolo C, Estrada D, Rios N, Alvarez MN, Piñeyro MD, et al. Cytosolic Fe-Superoxide Dismutase Safeguards Trypanosoma Cruzi From Macrophage-Derived Superoxide Radical. Proc Natl Acad Sci USA (2019) 116:8879–88. doi: 10.1073/pnas.1821487116
49. Manni ML, Tomai LP, Norris CA, Thomas LM, Kelley EE, Salter RD, et al. Extracellular Superoxide Dismutase in Macrophages Augments Bacterial Killing by Promoting Phagocytosis. Am J Pathol (2011) 178:2752–9. doi: 10.1016/j.ajpath.2011.02.007
50. Prolo C, Estrada D, Piacenza L, Benítez D, Comini MA, Radi R, et al. Nox2-Derived Superoxide Radical Is Crucial to Control Acute Trypanosoma Cruzi Infection. Redox Biol (2021) 46:102085. doi: 10.1016/j.redox.2021.102085
51. Munder M, Eichmann K, Modolell M. Alternative Metabolic States in Murine Macrophages Reflected by the Nitric Oxide Synthase/Arginase Balance: Competitive Regulation by CD4+ T Cells Correlates With Th1/Th2 Phenotype. J Immunol (1998) 160:5347–54.
52. Stempin C, Giordanengo L, Gea S, Cerbán F. Alternative Activation and Increase of Trypanosoma Cruzi Survival in Murine Macrophages Stimulated by Cruzipain, a Parasite Antigen. J Leukoc Biol (2002) 72:727–34. doi: 10.1189/jlb.72.4.727
53. Volpini X, Ambrosio LF, Fozzatti L, Insfran C, Stempin CC, Cervi L, et al. Trypanosoma Cruzi Exploits Wnt Signaling Pathway to Promote Its Intracellular Replication in Macrophages. Front Immunol (2018) 9:859. doi: 10.3389/fimmu.2018.00859
54. Cummings KL, Tarleton RL. Inducible Nitric Oxide Synthase Is Not Essential for Control of Trypanosoma Cruzi Infection in Mice. Infect Immun (2004) 72:4081–9. doi: 10.1128/IAI.72.7.4081-4089.2004
Keywords: Trypanosoma cruzi, inoculum concentration, inducible nitric oxide synthetase, immunosuppression, statistical modeling
Citation: Rodrigues WF, Miguel CB, Marques LC, da Costa TA, de Abreu MCM, Oliveira CJF and Lazo-Chica JE (2022) Predicting Blood Parasite Load and Influence of Expression of iNOS on the Effect Size of Clinical Laboratory Parameters in Acute Trypanosoma cruzi Infection With Different Inoculum Concentrations in C57BL/6 Mice. Front. Immunol. 13:850037. doi: 10.3389/fimmu.2022.850037
Received: 07 January 2022; Accepted: 24 February 2022;
Published: 18 March 2022.
Edited by:
Michele Mortarino, University of Milan, ItalyReviewed by:
Stella Maris González Cappa, University of Buenos Aires, ArgentinaGalia Andrea Ramirez-Toloza, University of Chile, Chile
Jyothi Nagajyothi, Hackensack University Medical Center, United States
Rita Rizzi, University of Milan, Italy
Copyright © 2022 Rodrigues, Miguel, Marques, da Costa, de Abreu, Oliveira and Lazo-Chica. This is an open-access article distributed under the terms of the Creative Commons Attribution License (CC BY). The use, distribution or reproduction in other forums is permitted, provided the original author(s) and the copyright owner(s) are credited and that the original publication in this journal is cited, in accordance with accepted academic practice. No use, distribution or reproduction is permitted which does not comply with these terms.
*Correspondence: Wellington Francisco Rodrigues, pos-doc.rodrigues@uftm.edu.br
†These authors have contributed equally to this work