- 1Department of Neurology, Heinrich-Heine University Düsseldorf, Medical Faculty, Düsseldorf, Germany
- 2Department of Neurology, Maria Hilf Clinic, Moenchengladbach, Germany
- 3Department of Neurology, Palacky University in Olomouc, Olomouc, Czechia
- 4Department of Neurology, Barzilai Medical Center, Ashkelon, Israel
- 5Brain and Mind Center, Medical Faculty, The University of Sydney, Sydney, NSW, Australia
Cumulative evidence along several lines indicates that B cells play an important role in the pathological course of multiple sclerosis (MS), neuromyelitisoptica spectrum disorders (NMOSD) and related CNS diseases. This has prompted extensive research in exploring the utility of targeting B cells to contain disease activity in these disorders. In this review, we first recapitulate the development of B cells from their origin in the bone marrow to their migration to the periphery, including the expression of therapy-relevant surface immunoglobulin isotypes. Not only the ability of B cells to produce cytokines and immunoglobulins seems to be essential in driving neuroinflammation, but also their regulatory functions strongly impact pathobiology. We then critically assess studies of B cell depleting therapies, including CD20 and CD19 targeting monoclonal antibodies, as well as the new class of B cell modulating substances, Bruton´s tyrosinekinase (BTK) inhibitors, in MS, NMOSD and MOGAD.
1 Introduction
The fundamental role of B cells in the pathogenesis of inflammatory central nervous system (CNS) disease has emerged through extensive studies in the last 10-15 years. However, the exact role of B cells in the development of these disorders and the mechanisms of the drugs targeting B lymphocytes still remain, at least in parts, unclear.
Multiple Sclerosis (MS) is an inflammatory, autoimmune disorder of the CNS characterized by demyelination and axonal loss. While demyelination, in principle, is reversible, neuroaxonal degeneration is almost invariably permanent. Therefore, new therapies are urgently needed to effectively prevent chronic neurodegeneration as the main determination of long term disability. Immunpathological changes in MS are generally characterized by activity and complex interactions of T-cells, myeloid-cells, and B cells (1, 2).
For a long time, neuromyelitisoptica (NMO), was considered to be a rare, special variant of MS. However, in 2004, a specific antibody neuromyelitisoptica immunoglobulin G (NMO-IgG) (3), one year later identified to be directed against the water channel aquaporin-4 (AQP-4) was discovered (4). This established NMO as a distinct entity in its own right and later allowed to broaden its clinical manifestation for which the term neuromyelitisoptica spectrum disorders was coined. These observations fundamentally changed diagnostics and treatment of this group of inflammatory CNS disorders. Further research revealed elevated B cell and plasmablast activity and attenuated B cell regulatory function and complement-mediated astrocyte damage (5) underlying the pathobiology of NMOSD (6). In addition, the detection of a specific antibody in NMOSD stimulated efforts to look for specific markers and subtypes in MS that continue to this day (7).
Similar to MS, myelin oligodendrocyte glycoprotein (MOG)-antibody-associated autoimmune disease (MOGAD) is an inflammatory, demyelinating disease of the CNS with reference to oligodendrocytes, which is primarily characterized by (mostly relapsing) optic neuritis, myelitis and brainstem encephalitis or acute disseminated encephalomyelitis (ADEM) in children (8–11). In contrast to MS and MOGAD, NMOSD particularly affects astrocytes. On the other hand, NMOSD and MOGAD, with their predilection sites in the optic nerves, cerebellum, brain stem and spinal cord (causing long spinal cord lesions), have more in common with each other than with MS (10).In terms of specific MRI features, MOGAD is characterized by anterior participation of the optic nerve involving the peribulbar fat, poorly delineated (‘fluffy’) lesions and central grey matter of the spinal cord (in axial imaging - ‘H-sign’). However, in MOGAD, MRI lesions may regress and a positive MOG-Ab status may transform to seronegativity (11). Neuropathologically, CD4+ T-cells dominate MOGAD lesions, whereas MS is dominated by CD8+ cells. To date, there is no female predominance in MOGAD, which distinguishes the disease from MS and NMOSD (11). Overall, MOGAD is an independent clinical entity that sits between MS and NMOSD in the spectrum of autoimmune inflammatory diseases. It represents around 40% of the patients presenting as NMOSD patients who are AQP4 antibody negative (9).
In the following review, we provide a brief recapitulation of B cell development, their involvement in the pathology of MS and other inflammatory demyelinating CNS disease and provide a detailed overview of B cell depleting therapies and key clinical studies.
2 B cell development – from bone marrow to periphery
Myeloid and erythroid progenitor cells as well as lymphoid progenitor cells differentiate from self-renewing pluripotent haematopoietic stem cells of the bone marrow, whereas from the latter, mature B cells develop through various intermediate stages. Up to the stage of “immature B cell”, rearrangement of the immunoglobulin segment genes occurs, resulting in the expression of a mature B cell receptor (BCR) that consists of two heavy and two light chains (12–14).
In the pro-B cell stage, the gene segments of the heavy chain are rearranged and ultimately expressed as a µ-chain. While the µ-chain represents the heavy chain of the final BCR product, the CD19 antigen is already expressed on the pro-B cell (15). In the pre-B cell stage, CD20 is already displayed on the cell surface and the light chain gene segments of the BCR are reorganized, however not yet integrated into the receptor. Among other actions the signaling pathway of Bruton’s tyrosine kinase (BTK) stimulates the development of the immature B cell, in which light and heavy chains are combined to form the BCR on the cell surface (16–19). The immature B cells then undergo two selection processes: First, they interact with endogenous antigens (AG) of the bone marrow. Afterwards, the immature B cells migrate from the bone marrow and are challenged with exogenous AGs in the spleen and secondary lymphatic organs by contact with macrophages, amongst others. In the course of this process, signaling with B cell activating factor receptor (BAFF-R), B cell maturation antigen receptors (BCMA-R) and transmembrane activator and CAML interactor receptors (TACI-R) leads to the development of an immature B cell to a mature-naïve B cell. By interaction with follicular T helper cells, differentiation into memory B cells and short-lived AG-producing plasmablasts is initiated. In this process, BAFF-R, BCMA-R and TACI-R are also heavily involved. While TACI-R is mainly expressed on memory cells, BCMA-R is found on plamablasts/plasma cells, BAFF-R is expressed on cells from the immature B cell onwards (20–22). Memory cells express CD19 and CD20, whereas only CD19 is found on plasmablast (12, 13, 15, 17, 19, 23–26) (Figure 1).
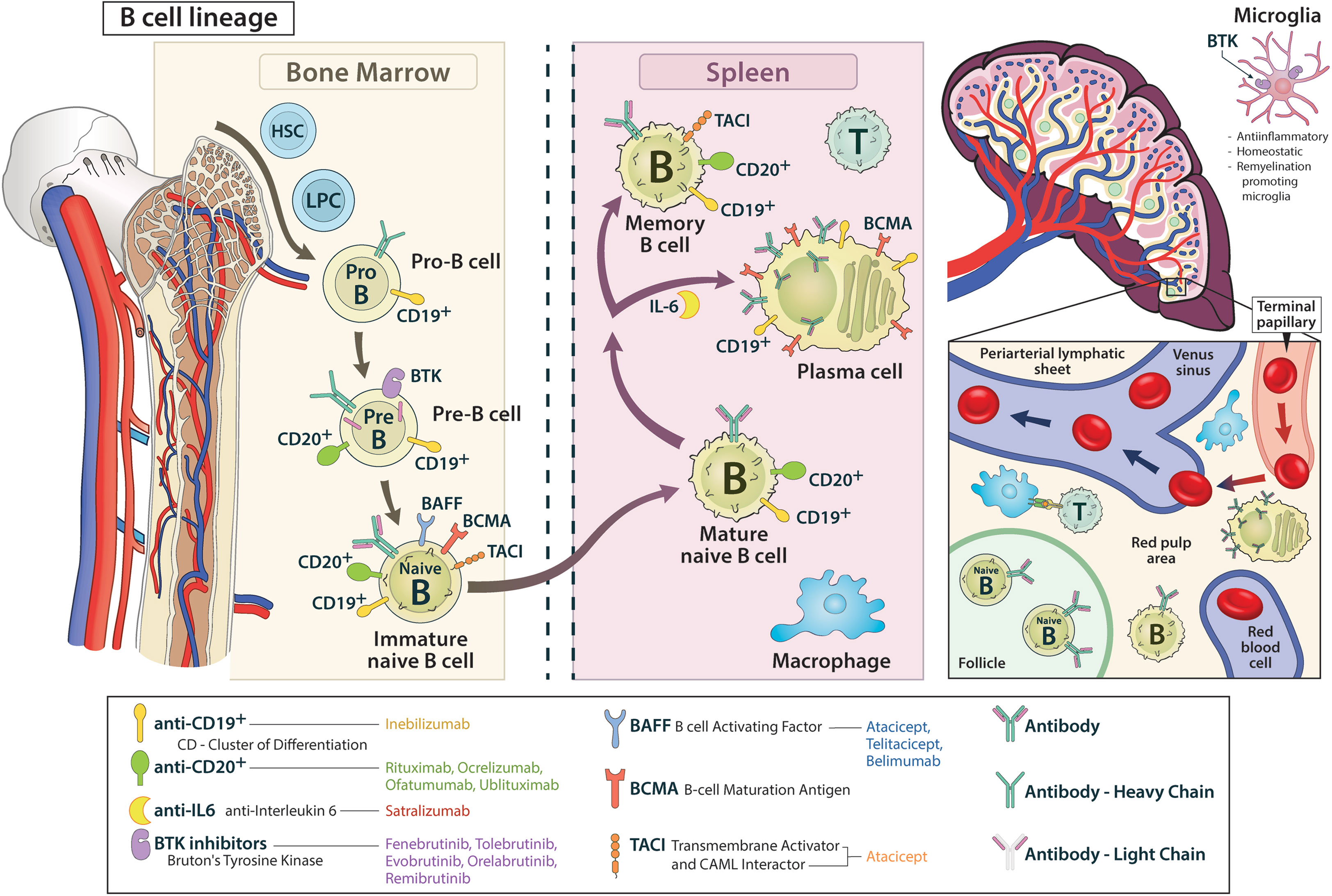
Figure 1 The B cell lineage from bone marrow to the periphery is shown, including relevant surface proteins and their targeting by corresponding drugs.
3 Complex immunological role of B cells in MS, NMOSD and MOGAD
The presence of oligoclonal bands in the cerebrospinal fluid, detection of circulating CNS-reactive antibodies and demonstration of immunoglobulins deposited in CNS lesions of MS patients directed for long the focus of interest primarily on the antibody-producing function of B cells. The impressive and rapid improvement observed after drug-induced depletion of B cells in MS prompted reconsideration of the key role of B lymphocytes in the immunopathogenesis of MS. Along with other evidence, these effects suggested a role of B cells as antigen presenting cells that would initiate or amplify T-cell responses to CNS antigens and emphasized their roles as complex players diversely interacting with and modulating several other components of the immune system (16, 19, 23, 25, 27–32) and which can now be traced even at the epigenetic level (33).
The pathobiology of MS is assumed to involve interactions of multiple intrinsic (e.g. genetic variants) and extrinsic (e.g. Epstein-Barr-virus - EBV - infections, vitamin D deficiency) factors. This leads to an autoreactive activation of peripheral immune cells such as CD8+ T-cells and CD4+ T-cells (e. g. TH-17 cells as a subset of CD4+ T-cells) paralleled by a breakdown of immune-tolerance. These cells migrate via meningeal vessels or choroid plexus structures across the blood-CSF barrier into the brain, get reactivated by local CNS autoantigens presented by resident glial and other myeloid cells and attack endogenous CNS structures (1, 34–39). The activation process of autoreactive T-cells is flanked by a deficiency of immune-inhibitory components, with both a quantitative deficiency of T-reg cells and their dysfunction due to a newly developed resistance of autoreactive T-cells (34, 37). Beyond the blood-brain barrier, innate microglia also play an important role in the progression of MS, although this role is not yet fully understood. On the one hand, microglial cells can act in a regenerative manner by promoting neuron recovery and remyelination via phagocytosis of cell debris or modulation of synaptic connections. In addition these cells can also exert CNS-destructive actions via the regulation of cytokine and chemokine release (40). This leads to an additional recruitment of macrophages and lymphocytes, which amplifies the already existing autoimmune inflammatory processes in the CNS (35, 41–45).
B cells are also essentially involved in the outlined process. Through internalization and consecutive antigen presentation via the major histocompatibility complex II (MHC II), B cells stimulate and activate T-cells. In addition, they secrete pro- (e.g. TNF-α, GM-CSF) and anti-inflammatory (e.g. IL-10, IL-35) cytokines that modulate the invasion and behavior of both, the innate immune system in the form of macrophages or microglia and the adaptive immune system such as T-cells (19, 23, 25, 27–31). Recently, the importance of the dura with its lymphatic vessels has been recognized as a particularly relevant site for the residency and development of B cells, enabling communication across the blood-brain barrier (23, 32, 46, 47). Late-stage tertiary follicles of B cells in the meninges of MS patients have been demonstrated to maintain and modulate continuous inflammation (15, 19, 48, 49). Complementing this, the gut-brain axis is also coming into focus as an important component of the B-cell modulated autoimmune processes underlying MS. The determination of IgA-bound specific intestinal taxa in MS patients and experiments in animal models indicate an immunomodulatory function of IgA-producing B cells that receive their imprinting in the intestine and migrate to the CNS (50, 51). Unaffected by this, the ability of B cells to produce antibodies seems to play a subordinate role. The IgG antibodies detected in the CSF are rather to be regarded as a reaction to ubiquitous intracellular proteins that are produced as part of the CNS destruction process occurring during the disease (28, 30) (Figure 2, right).
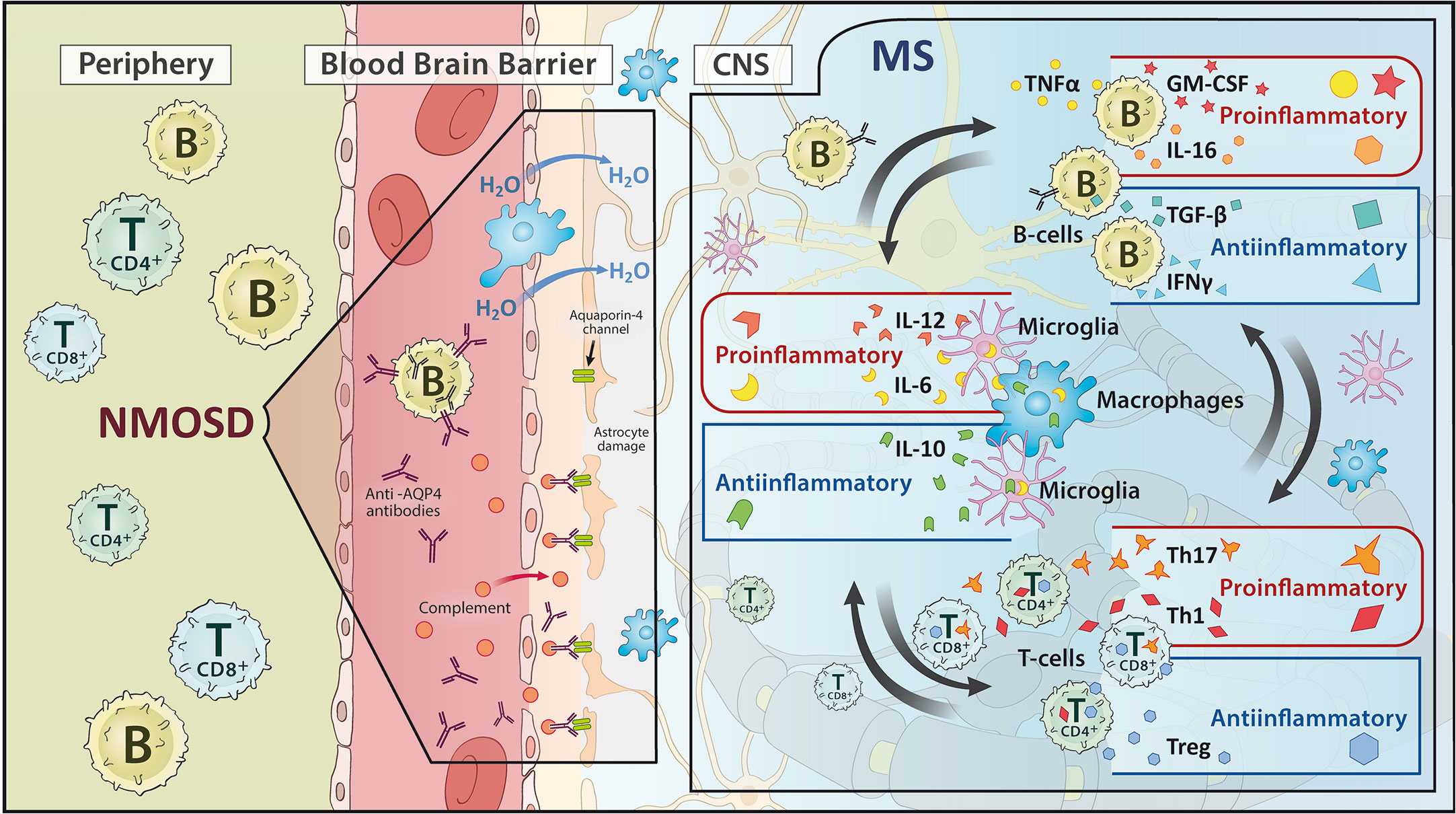
Figure 2 Schematic representation of the pathomechanism of (left) neuromyelitis optica spectrum disorders (NMOSD) and (right) multiple sclerosis (MS). While NMOSD focuses on an antibody reaction, MS shows an interplay of pro- and anti-inflammatory processes between B cells, T cells and microglia/macrophages.
Nevertheless, recent experiments have demonstrated that monoclonal-Ab of B cells from CSF and serum of MS patients bind the EBV transcription factor EBNA1 with high affinity. They also show molecular mimicry with CNS cell adhesion molecule GlialCAM. This observation leads to the hypothesis that after contact with EBV, B cells enter the CNS/CSF space, encounter GlialCAM antigen and then undergo affinity maturation, producing high-affinity clones for anti-GlialCAM-Ab (52). This may confirm the long-established link between MS and EBV infection, which has recently been established in a cohort of 10 million young adults (53).
The situation is different, however, in NMOSD. In 70-80% of all NMOSD patients, aquaporin-4 immunoglobulin G antibodies (AQP4-Ab) can be detected, which are directed against the body’s endogenous AQP4 antigen. Up to 42% of AQP4 IgG negative patients harbor IgG antibodies to Myelin Oligodendrocyte Glycoprotein (MOG) (54). There may be overlap with or genuine MOG antibody associated disease (MOGAD) (55). AQP4 is found mainly in the central nervous system on astrocytes near the blood-brain barrier and function as a water channel, whereas the binding of AP4-Ab to AQP4 channels causes their downregulation. This leads to intra-myelinoedema due to disturbed water homeostasis and to an activation of the complement system with assembly of the terminal complement complex C5b-9 and consecutive necrosis of the cells expressing AQP4. Leukocytes migrate through the pre-damaged blood-brain barrier, further promoting the pathological process (15, 25) (Figure 2, left). Interestingly, there is also information on the possible involvement of molecular mimicry in the pathogenesis of NMOSD. Reports have appeared on the autoimmune cross-reactivity of T cells with the protein adenosine triphosphate-binding cassette of the intestinal bacterium Clostridium perfringens (56). Evidence how this impinges B cell responses has not yet been put forth.
4 Targets of B cell therapies
Several B cell targeting strategies have been approved in recent years, some of which are the most effective in treating MS and other inflammatory CNS disease. In the following, the B cell depleting therapies are listed, with the key studies, also summarized in Supplementary Table 1.
4.1 CD20 antigen related drugs
CD20 is a four-transmembrane protein expressed on the surface of pre-B cells up to memory cells, but not on the long-lived and antibody-producing plasma cells or stem cells and pro-B cells. Thus, targeting CD20 does not interfere directly with antibody production or generation of new B cells in the bone marrow (Figure 3).
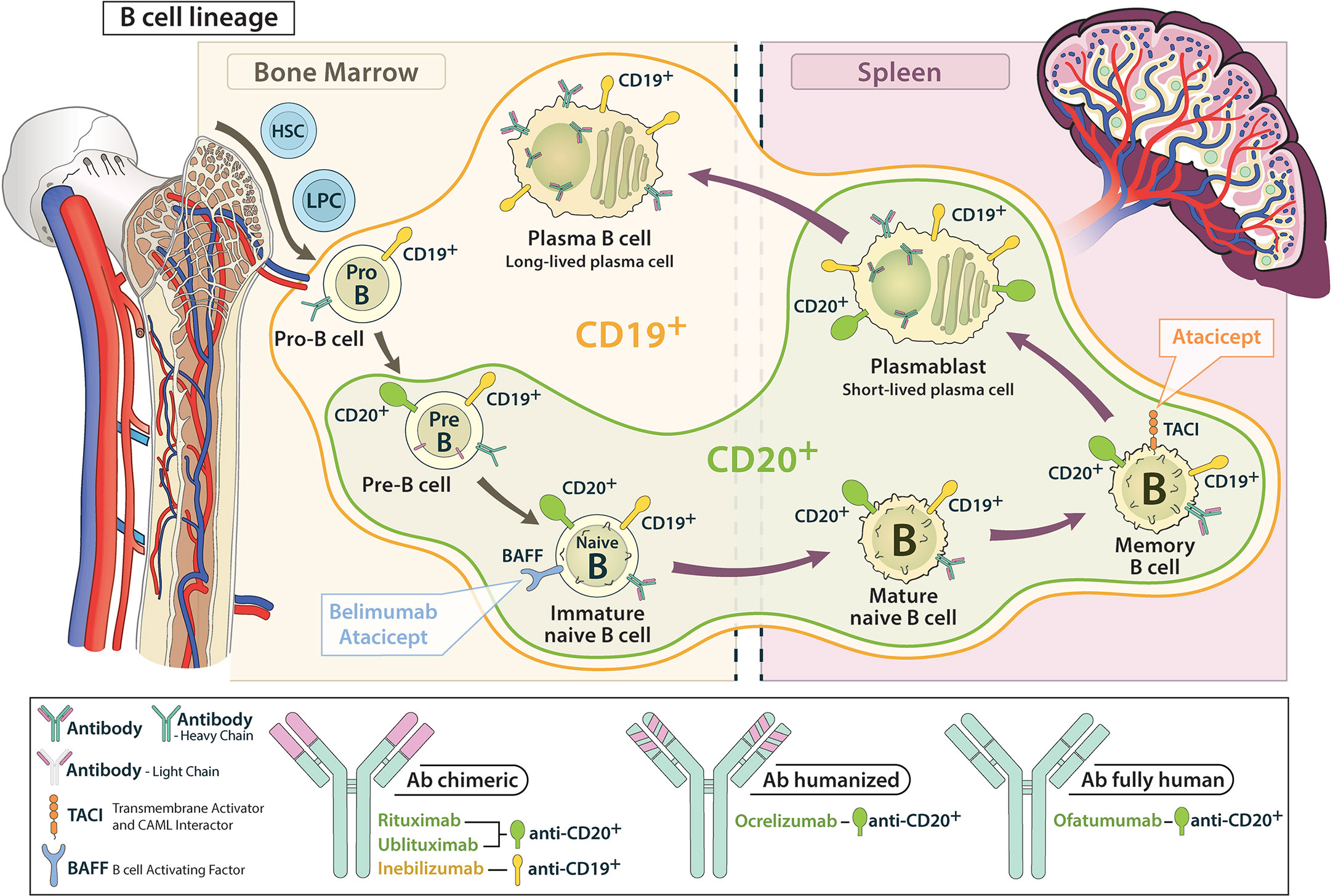
Figure 3 Schematic representation of the attack range of CD 19 and CD 20 antibodies in the B cell lineage. CD 19 antibodies cover earlier and later developmental stages compared to CD 20 antibodies.
4.1.1 Rituximab
Rituximab was the first CD20-targeting antibody to be tested in people with MS. As a chimeric monoclonal antibody (Ab), it depletes B cells primarily via complement-dependent cytotoxicity (CDC) (28–30, 57).
In 2008, a phase 1 study confirmed the safety of two cycles of rituximab in 26 relapsing-remitting multiple sclerosis (RMS) patients. The results for the secondary endpoint also showed a decrease in gadolinium-enhancing T1 lesions and new T2 lesions down to zero at 72 weeks (58). In the double-blind phase 2 HERMES trail, superiority of rituximab over placebo was demonstrated for the primary endpoint namely reduction in the total number of gadolinium-enhancing lesions on MRI at 12, 16, 20 and 24 weeks. In addition, an improvement in clinical outcomes such as the reduction in relapse rate within the first 24 weeks could be achieved as secondary endpoints (59).
The positive outcome of the RMS studies could not be replicated in studies of patients with primary progressive multiple sclerosis (PPMS). In the double-blind phase 2/3 OLYMPUS trial, there was no significant difference between placebo and rituximab treated patients in the primary endpoint of time to confirmed disease progression. However, in a subgroup analysis, rituximab attenuated disease progression in PPMS patients younger than 51 years of age with gadolinium-enhancing lesions on MRI compared to placebo (60).
In an initial open-label pilot trial in patients with NMO in 2005 rituximab demonstrated beneficial effects in 8 patients (61). Subsequently, a number of retro- and prospective studies followed. However, no randomized double-blind studies were conducted (62, 63) until 2020 when the Japanese RIN-1 study with rituximab in 38 patients with NMOSD was published. The primary endpoint in this study, time to first relapse within 72 weeks, was reached. While no patient relapsed on rituximab, 7 patients suffered attacks on placebo (62, 63). Due to the limitation of the small number of patients and the lack of an independent relapse assessment as well as the exclusive inclusion of aquaporin-4-antibody positive patients the study leaves several questions unanswered (62, 63).
A similar conclusion regarding the efficacy of rituximabin myelin-oligodendrocyte-glycoprotein-immunoglobulinG-associated disorders (MOGAD) can be drawn. After numerous smaller studies (64), a prospective study comprising 102 paediatric MOGAD patients from 8 countries compared the different treatment protocols of the respective centers along with their outucomes between 2014 and 2016. Treatment of 9 children with rituximab resulted in a decrease in the annualized relapse rate from 2.12 to 0.67, with the median Expanded Disability Status Scale (EDSS) score remaining stable (65). In 2020, a retrospective study of 121 pediatric and adult patients from 13 countries found rituximab to be effective. In the group of patients who had relapsed before switching to rituximab, 70.0% remained relapse-free for the next 11.2 months, and in the group of patients with two or more relapses, 52.5% did not experience an attack within the subsequent 12.1 months (66). In 2022, a first systematic meta-analysis of 13 studies and a total of 238 patients was published. In the analyzed data, 55% of the patients treated with rituximab did not have a relapse within the observed study periods (67). The following rituximab-specific adverse reactions have manifested in the pivotal trials and in clinical use: Infusion-associated reactions such as fever, tachycardia and hypotension, upper respiratory tract infections and changes in the blood count in a sense of neutro- or thrombocytopenia (16, 23, 28, 67). In summary, the current data principally supports the efficacy of rituximab in MOGAD, however, the exact mode of action in this disease still remains unclear, as relapses occurred despite a significantly reduced number of B lymphocytes (41, 43).
Regardless of the above, there is currently a lack of randomized controlled trials on therapeutic options for MOGAD. A 152-patient MOGAD trial is currently recruiting to test the efficacy and safety profile of the interleukin-6 antagonist sartralizumab against placebo (ClinicalTrials.gov Identifier: NCT05271409). Currently, treatment is guided according to expert opinion and case series: Acute MOGAD attacks in adults are treated with an i.v. steroid course followed by a 2-3 months tapering. Depending on the clinical situation, a maintenance therapy with rituximab, azathioprine, mycophenolatemofetil or IVIG follows. This does not apply to children, who often have a monophasic course. Both the phasing out of cortisone with regard to possible side effects and the initiation of a basic therapy must be critically discussed (10, 68, 69).
4.1.2 Ocrelizumab
Ocrelizumab was developed based on the experience with rituximab and was the first CD20-based receive approval for MS. This humanized monoclonal antibody (Ab) depletes B cells predominantly via antibody-dependent cellular cytotoxicity (ADCC) (29, 30, 70).
In 2011, a double-blind randomized phase 2 trial tested ocrelizumab at doses of 600 and 2000 mg for efficacy compared to placebo or intramuscular interferon β (IFNβ)-1a in 220 study participants with RMS for a total of 48 weeks. The number of gadolinium-positive T1 lesions at weeks 12, 16, 20 and 24 (the primary endpoint) were significantly lower in the two ocrelizumab groups compared to the placebo and comparator groups. A significant difference in efficacy between the two doses of ocrelizumab could not be determined (71). This trial added support to the role of B cells in MS pathogenesis and prompted the double-blind, randomized, double-blind OPERA-I and II phase 3 trials in 1656 relapsing MS patients, which led to the approval of ocrelizumab by the regulatory authorities FDA, European Medicines Agency (EMA), Health Canada and Therapeutic Goods Administration for the treatment of relapsing MS. After 96 weeks, the primary endpoint of a lower annualized relapse rate compared to IFNβ-1a was achieved. Ocrelizumab also proved to be superior to IFN-β in most secondary clinical and MRI endpoints. However, superiority in the items of improvement in multiple sclerosis function score, including Short Form Health Survey-36 (SF-36 score), and total brain volume loss were not significant. In addition, a higher number of infusion reactions and twice the absolute number of neoplasia manifestations (4 vs. 2) occurred with ocrelizumab compared to interferon-β. Consequently, the study addressed the need for further long-term follow-up observations of the patients treated with ocrelizumab (72). In the open-label extension patients treated initially with IFNβ were switched to ocrelizumab. After 3 years, the population treated with ocrelizumab from the beginning had a lower percentage of disease progression over 24 weeks and a reduced loss of brain volume (-1.87% versus -2.15%). Importantly, the safety profile, now collected over the course of 5 years, was similar to the core study (73).
Using the evidence from the OLYMPUS study the double-blind, randomized ORATORIO 2017 trial investigated the effect of ocrelizumab compared to placebo in 732 patients with PPMS (27). The percentage of patients on ocrelizumab who experienced disability progression at 12 weeks (primary endpoint) was 32.9% compared to 39.3% in the placebo group (24% reduction, p=0.03). In addition, ocrelizumab met all secondary clinical and MRI endpoints except for an improvement in the SF-36. Compared to the placebo group, more infusion reactions up to the intermediate category and an increased incidence of neoplasia were observed with ocrelizumab (74). It should be noted that ORATORIO primarily included PPMS patients with short disease duration (16). However, recent post-hoc data suggest efficacy of ocrelizumab even in the presence of increased disability due to MS (75). In an open-label ORATORIO follow-up study, a significant improvement in 24-week confirmed disability progression was still demonstrated with ocrelizumab for up to 6.5 years after the start of the study compared to patients who switched from placebo to ocrelizumab after the official end of ORATORIO (33.3% versus 44.7%). There were no new safety findings (76).
Direct comparisons of ocrelizumab with other disease-modifying therapies (DMT) in a double-blind and randomized study design are still lacking, with the exception of IFNβ. A comparative study between rituximab and ocrelizumab is recruiting (ClinicalTrials.gov Identifier: NCT04578639). Currently, a systematic meta-analysis from 2019 provides the most robust data. It confirms, that ocrelizumab has an efficacy and safety profile that is superior or comparable to other available DMT at all endpoints, with the exception of natalizumab and alemtuzumab (77). The efficacy of switching to ocrelizumab after treatment failure with another DMT has been explored in two prospective non-randomized studies. The North American CHORDS study includes a population of 576 patients with RMS with a clinically or radiologically objectified relapse on one or more DMTs for at least 6 months. The primary endpoint “No evidence of disease activity” (NEDA) includes the absence of protocol-defined relapses, confirmed disability progression, T1 Gd-enlarging lesions and new/enlarging T2 lesions. At 96 months, 48.1% of patients reached this point on ocrelizumab (78). The European counterpart study CASTING, which included 680 patients with the same baseline characteristics, 74.8% achieved NEDA after 96 weeks (79). Independently of this, a non-blinded open-label study on the safety and efficacy of the use of ocrelizumab in particularly early stages of RMS is currently ongoing (EudraCT Number: 2016-002937-31).In 2020, a sub-study of CASTING, called ENSEMBLE PLUS, showed that shortening the infusion time for the second administration of ocrelizumab from 3.5 to 2 hours was associated with a similar percentage of infusion-related adverse events (23.1% in the 3.5-hour group and 24.6% in the 2-hour group) (80).
In the pivotal studies and clinical use, the following ocrelizumab-specific adverse reactions have been noted: Infusion-associated reactions such as fever, tachycardia and hypotension, upper respiratory tract infections, increased herpes and influenza infection rates and opportunistic infections including PML (81, 82).
4.1.3 Ofatumumab
Ofatumumab is a fully humanCD-20-monoclonal antibody that, unlike ocrelizumab, is administered subcutaneously at monthly intervals. It acts primarily through CDC and was approved by the FDA in August 2020 and by EMA in March 2021 for the treatment of MS (16, 19, 28–30, 58, 83).
The MIRROR phase 2 study demonstrated efficacy of ofatumumab at doses of 3, 30 or 60 mg every 12 weeks or 60 mg every 4 weeks compared to placebo in the primary endpoint, the cumulative number of new gadolinium-enhancing T1 lesions at weeks 4, 8, and 12. The cumulative number of new lesions was reduced by 65% in all ofatumumab dose groups compared to placebo at 12 weeks. Interestingly, all ofatumumab doses achieved radiological efficacy, and the authors concluded that complete depletion of CD19-B cells was not necessary for a robust effect (84). The twin studies ASCLEPIOS I/II, a randomized-blinded and comparator-controlled phase 3 study with ofatumumab in patients with RMS was published in 2020. In this 30-month period, ofatumumab versus teriflunomide met its primary endpoint, the adjusted annualized relapse rate (0.11/0.1 for ofatumumab versus 0.22/0.25 for teriflunomide). Among neurofilament light chains (Nfl) in the serum of ofatumumab patients was reduced compared to teriflunomide at 3, 12 and 24 months. However, this sign of a neuroprotective effect of ofatumumab contrasts with the lack of difference between the two drug groups in terms of brain volume change over time (85). The phase 3b ALITHOS study, designed as an open-label, long-term study, collected cumulative data from 1969 patients from the previously mentioned studies. It included patients who continued treatment with ofatumumab after ASCLEPIOSI/II and the phase 2 trials or who were switched from teriflunomide to ofatumumab after the end of ASCLEPIOS. In the cumulative patient data with ofatumumab exposure of up to 3.5 years, there were no new safety signals compared to those in the ASCLEPIOSI/II trial (83).
The SOSTOS trial (ClinicalTrials.gov Identifier: NCT05090371) is currently recruiting patients who switch from platform therapies to after a proven increase in Nfl concentration. Under the name ARTIOS, the switch from dimethyl fumarate or fingolimod to ofatumumab due to persistent disease activity is being investigated in a planned trial of 555 people (ClinicalTrials.gov Identifier: NCT04353492). In addition, the OKLIOS trial is recruiting patients to evaluate the impact of switching from another CD20-mAB (most likely ocrelizumab) to ofatumumab in terms of safety and efficacy (ClinicalTrials.gov Identifier: NCT04486716). Results are currently pending.
According to studies and clinical use, the following adverse reactions should be considered when using ofatumumab: an increased rate of infection, especially of the upper respiratory tract and in pneumonia and urinary tract infections, systemic and local reaction to the administering injection, a lowered IgM level and hepatitis B reactivation (81, 83).
4.1.4 Ublituximab
Ublituximab is a chimeric glycoengineered IgG1 monoclonal antibody that has been modified at the constant fragment (Fc) region and acts mainly via ADCC on CD20-positive cells. It has been argued that the higher binding affinity will result in dose reduction and faster infusion times compared to previous anti-CD20 therapies (16, 30, 86, 87). In December 2022, the drug was approved by the FDA for the treatment of patients with RMS (88).
In a phase 2 study published in 2021 (n = 48 RMS patients), total doses over 24 weeks of 1050 to 1350 mg with infusion times of 1 to 4 hours were applied and each dose/infusion time group was controlled against a placebo group. The primary endpoint, the proportion of patients treated with ublituximab with ≧̸95% peripheral CD19+ B-cell depletion at 2 weeks after ublituximab application, was met at 100% by all dose/infusion time groups. Furthermore, an increase in infusion-related systematic reactions was not found to be related to higher doses or shorter infusion times (87). The results of 2 phase 3 randomized, double-blind twin trials (ULTIMATEI/II) were published in 2022. 1094 RMS patients were treated with either ublituximab or teriflunomide for 96 weeks according to a standardized protocol. The primary endpoint, annualized relapse rate over 96 weeks, was met by ublituximab at 0.08 and 0.09 (ULTIMATEI/II) versus teriflunomide at 0.19 and 0.18 respectively. In the secondary endpoints, although ublituximab resulted in fewer MRI brain lesions compared to teriflunomide with effective CD19 depletion, significant differences in disability worsening could not be substantiated, probably due to the low rate of disability progression in both groups (86).
Looking at applications in NMOSD, Mealy et al. presented a phase 1 study in 2019 in which active NMOSD patients were administered a single dose of ublituximab in addition to 1 g of methylprednisolone for 5 days. In the absence of serious adverse events within 90 days, the primary endpoint of safety of additional ublituximab use was met. The secondary endpoints showed a decrease in the median EDSS score from 6.5 to 4.0 within 90 days. However, with a group size of only 5 AQP4-IgG-seropositive NMOSD patients and the phase 1 study design, the significance of the study is limited (89).
Based on the data to date, the safety profile of ublituximab is similar to that of the other CD20 antibodies: leading adverse events are infusion-related reactions with fever and tachycardia, upper respiratory tract infections and headache (86). An open-label observational study is currently being conducted following ULTIMATEI/II to better explore the long-term safety profile of ublituximab (ClinicalTrials.gov Identifier: NCT04130997).
4.1.5 Covid 19 and CD20 depleting basic therapies
The question of what the pandemic means for MS patients on immunomodulatory therapy arose early in Covid-19. Retrospective data indicate that factors such as female gender, comorbidities, previous drug escalation of DMT and hospitalization predispose MS patients to infection with covid-19 (90). In particular, the question is about the influence of DMT on the efficacy of vaccination. It is known from studies that various aspects such as vaccination timing and type of vaccine differ between the different basic therapy classes. In particular, a reduced humoral response after vaccination has already been described for CD20-depleting drugs and sphingosine-1-phosphate receptor modulators (91). This has also been confirmed in numerous prospective, retrospective and experimental studies: Under CD20-depleting and sphingosine-phosphate-modulating therapies, there is a reduced humoral response compared to vaccine naïve subjects compared to the other MS medications (91–99). At the same time, there is evidence that the reduced humoral response is accompanied by an increased T-cell response (95, 99). Whether the increased T-cell response can compensate for the decreased humoral response in relation to covid infection remains unclear and is currently the subject of research (92, 98). With regard to patients on anti-CD20 therapy, the current literature contains conflicting statements on whether CD20-depleting therapy leads to an increased likelihood of hospitalization (100, 101). Remarkable are new findings on different vaccination regimens that have direct therapeutic consequences. It could be shown that, especially with CD20-depleting therapies, different vaccines have more or less strong effects on the long-term humoral response after vaccination. The choice of a specific vaccine is therefore of particular importance (97). On the other hand, after a third Covid booster vaccination, a significant increase in both the humoral - and the T-cell response is observed (94).
4.2 CD19 antigen directed drugs
CD19 is a type I transmembrane glycoprotein that, in contrast to CD20, is already expressed on both early pro-B cells and late antibody-producing plasmablasts and some plasma cells during B-cell development. Accordingly, CD19 is thought to be more important in mainly antibody-driven diseases such as NMOSD (15, 25, 64, 102, 103).
4.2.1 Inebilizumab
Inebilizumab is a CD-19 directed humanized monoclonal antibody that has an increased affinity to the Fc region due to its glycosylation and it eliminates CD-19 positive B cells primarily via ADCC. It was approved by the FDA in 2020 for the treatment of seropositive NMOSD patients, in 2021 in Japan for the prevention of clinical relapses in NMOSD and by EMA as monotherapy for the treatment of adult patient with seropostive NMOSD in 2022 (15, 25, 64, 102, 103).
In 2017, a phase 1 study assessed the safety and tolerability of intravenous or subcutaneous inebilizumab (at 30, 100, 600 mg iv - 60, 300 mg sc) compared to a corresponding placebo group. With good tolerability, the most common side effects were nasopharyngitis, upper respiratory tract infection, urinary tract infection and infusion/injection-related side effects. With decreasing immunoglobulin levels, there was no change in pre-existing anti tetanus toxoid IgG levels (103). The randomized multicenter phase 2/3 N-MOmentum trial (2019) tested the efficacy of inebilizumab versus placebo in a population of 230 mostly seropositive but also seronegative NMOSD patients. The primary endpoint of the study was the number of days to clinical relapse within the observed time point. Within the observation period, 12% of the inebilizumab group relapsed compared to 39% of the placebo group. There were no new safety profile findings, although one death occurred in aninebilizumab-treated patient after initial administration, with two new lesions on MRI involving both white and grey matter. The presence of progressive multifocal leukencephalopathy could neither be excluded nor confirmed with certainty. Irrespective of this, the antitetanus toxoid IgG levels were also stable in N-MOmentum, therefore it can be assumed that there is no influence on the vaccination response achieved before the initial administration of inebilizumab (104). As rituximab alone was approved for the treatment of NMOSD prior to the FDA approval of inebilizumab, it is relevant to ask whether inebilizumab could be considered as an alternative drug for NMOSD patients who had relapsed on rituximab. In a post-hoc analysis of the N-MOmentum data from 2022 including 17 patients who had initially taken rituximab, the efficacy of inebilizumab as a second therapy was confirmed. However, this patient population showed an increased susceptibility to infections (102).
The previous findings on side effects of inebilizumab were based on 4 years of drug use, which emerged in a post hoc analysis on data from the N-MOmentum study and its extension (26): As known from anti-CD20-Ab’s, infusion-related reactions with fever and tachycardia, upper respiratory tract infections as well as urinary tract infections are worth mentioning. However, there was no case of progressive multifocal leukencephalopathy.
4.3 Cytokine antagonists
BAFF (B-Cell Activating Factor) and APRIL (A proliferation inducing ligand), members of the TNF supra family, are important regulatory cytokines in B cell development and activation. They exert their effect upon interaction with receptors of the BAFF, TACI and BCMA signaling pathways (19, 22, 30). Attempts have been made to interfere with these pathways in the treatment of MS with the drugs atacicept and belimumab. Interleukin 6 represents an important component in the pathogenesis of NMOSD, addressing B cells among others (105). Sartralizumab is an approved drug for the treatment of NMOSD.
4.3.1 Atacicept
Atacicept is a recombinant fusion protein of the extracellular TACI domain and human Fc IgG moiety that binds to the cytokines BAFF and APRIL, preventing their interaction with the B-cell surface receptors (8, 19, 41, 72). It acts selectively on B-cell development by blocking plasma cells and late B-cell development, but has no effect on B-cell progenitors or memory cells (106).
In the ATAMS phase 2 study, 255 RMS patients were randomized 1:1:1:1 to three dose groups of atacicept (25 mg, 75 mg, 150 mg) and a placebo group. The primary endpoint was the change in the mean number of gadolinium-enhancing lesions on T1-weighted MRI per patient. However, there was early study discontinuation due to increased annualized relapse rates in all atacicept groups compared to placebo (atacicept 25 mg 0.86, 75 mg 0.79, 150 mg 0.98 versus placebo 0.38). Also, no significant group differences were found in the number of gadolinium-enhancing T1 lesions (107). After these negative results emerged, the phase 2 ATON trial, which was conducted at the same time, was reviewed. This was a randomized clinical trial that tested atacicept against placebo in 34 patients with unilateral optic neuritis without a definitive MS diagnosis. The review of the study results led to early discontinuation by the sponsor. Although there was evidence of reduced axonal retinal layer thickness loss in the atacicept group (-8.6 µm versus -17.3 µm in the placebo group), there was a concurrent significantly increased conversion rate to definitive MS in the same group (35.3% versus 17.6%) (46).
Numerous hypotheses have been proposed regarding the reasons for MS worsening with atacicept. It is now generally agreed that atacicept, through its binding of APRIL, shifts the immunological balance of inhibition and excitation of the immune system to the disadvantage of inhibition (19, 22, 30, 108). APRIL has a higher affinity for TACI and BMCA-R and is, if this interaction is disturbed, an increased interaction of T and B cells and consequently, increased MS activity, occurs. Insights to confirm this presumed mechanism could be gained by considering pure BAFF antagonists such as tabalumab and belimumab, which do not compromise APRIL interactions. For example, belimumab was approved for the treatment of systemic lupus due to its efficacy, but studies of its use in MS showed neither improvement nor worsening (22). In addition, unlike CD20 monoclonal antibodies, atacicept does not deplete memory B-cells, which are also known to modulate T-cells. Consequently, these elective interference with the immunological balance is again discussed as a reason for worsening of the MS progression with atacicept (20, 22, 30, 108). Other possible explanations for the failure of Atacicept include the reduction in plasma cells that secrete the regulatory cytokine IL 35 (109).
4.3.2 Telitacicept
Telitacicept, as an analogue of atacicept, is also a recombinant fusion protein of the extracellular TACI domain and the humanized Fc-IgG part, which binds to the cytokines BAFF and APRIL and thus prevents their interactions with B-cells (110). Unlike atacicept, it has a longer TACI fragment (111).
In an open-label, uncontrolled phase 2 trial in China, 8 patients with relapsing NMOSD were treated with telitacicept once weekly for an additional 46 weeks following three cycles of plasma separation. The primary endpoint was the time to first relapse during 48 weeks of observation. Two patients (25%) relapsed and five patients (63%) remained relapse-free after 48 weeks of treatment. The relapse of the two patients occurred after a longer inter-relapse interval than prior to study inclusion (112). Thus, this cytokine antagonist could be a valuable treatment for NMOSD, probably due to the different underlying pathology of MS and NMOSD and its effect on antibody production. A phase 3 trial is currently recruiting to test the safety and efficacy of telitacicept against placebo in a cohort of 166 NMOSD patients (ClinicalTrials.gov Identifier: NCT03330418).
4.3.3 Satralizumab
Satralizumab is a humanized monoclonal recycling antibody against the interleukin-6 (IL-6) receptor that is approved for the treatment of NMOSD in Canada, the USA, Japan, Switzerland, Europe and other countries by their respective regulatory authorities (113). By binding to the IL-6R, it reduces B cell-derived plasmablasts in the periphery and AQP4-Ab secretion by B cells. Both effects explain the therapeutic effect in NMOSD (105, 114).
In a randomized controlled trial involving 83 NMOSD patients (the Sakura-Sky trial, 70% positive to AQP4 Ab’s), sartralizumab was tested as an add-on therapy to conventional immunosuppressive treatment against placebo. The relapse rate of 20% for sartralizumab was significantly lower than that for placebo (43%). The percentage of patients who were free from relapse at 96 weeks were 78% (sartralizumab) and 59% (placebo).In the secondary endpoints, sartralizumab did not reduce pain and fatigue symptoms (115).One year later, results were available for a 95-patient NMOSD (the Sakura-Star) trial testing sartralizumab as monotherapy against placebo. With a relapse rate of 30%, sartralizumab was superior to 50% with placebo, although this significant result was not noted in the subgroup of seronegative patients. Among the seropositive participants, 77% had no relapse after 96 weeks with sartralizumab, whereas only 41% had no relapse with placebo. Again, no robust difference between the substances could be objectified with regard to pain and fatigue (116).
4.4 Bruton’s tyrosine kinase inhibitors
BTK inhibitors are a relatively new class of small molecules explored for utility in the treatment of MS. By inhibiting this tyrosine kinase, they have an effect on the innate immune system including microglial cells and monocytes/macrophages as well as on the adaptive immune response by modulating B cells proliferation and activation. Recent work found evidence that BTK inhibition drives myeloid cells into a regulatory phenotype, promoting myelin repair (40, 117). BTK inhibitors may impact the innate immune system through interactions with the surface receptors of the Toll-like group and modification of upstream proteins such as Mal/TIRAP or Toll-like receptor 13 d. In addition, inflammasomes such as the multi-protein complex NLRP3 are regarded as components of BTK signalling and hence may be downregulated by BTK inhibitors (118–122). BTK inhibitors are considered promising candidates for MS therapy, as they can cross the blood-brain barrier (123) and, through their additional effect on the innate immune system, they may also address compartmentalized inflammation, which is of particular importance in mediating brain damage and driving disability of MS (119, 124–127).
4.4.1 Iribrutinib
Iribrutinib, the first market-ready BTK inhibitor, was developed in 2007 and approved by the FDA in 2013 for the treatment of mantle cell lymphoma and chronic lymphocytic leukemia. Due to its irreversible and non-specific binding to cysteine residue-481 of the kinase domain, it interacts with other kinases such as EGFR, JAK3, HER2 and TEC (off-target effects). This results in drug side effects such as cardiac arrhythmias, diarrhea, bleeding, hypertension and arthralgia (118). New generations of BTK inhibitors, which are also being tested in MS studies, are designed to reduce these effects.
4.4.2 Evobrutinib
Evobrutinib binds irreversibly to cysteine residue 481 of the kinase domain, but increases its selectivity to avoid off-target effects by additionally interacting with threonine residue 474 (128).
A double-blind, placebo- and comparator-controlled (dimethyl fumarate) phase 2 study of 267 RMS patients yielded complex results. The daily dose of 75 mg met the primary endpoint of cumulative total number of gadolinium-enhancing T1 lesions at weeks 12 to 24. Surprisingly, both the lower (25 mg) and higher (75 mg twice daily) dose of evobrutinib compared to the placebo group showed no significant difference with respect to the primary endpoint (129). The currently ongoing phase 3 evolution RMS 1 trial may shed a different light on the effect of the BTK inhibitor. In this trial, the agents evobrutinib and teriflunomide are being compared in a placebo-controlled manner in a planned population of 898 RMS patients (ClinicalTrials.gov identifier: NCT04338022 - first results expected in September 2023). According to current experience from studies in MS, an increased number of nasopharingitides and elevated liver enzymes are side effects of evobrutinib (129).
4.4.3 Tolebrutinib
Tolebrutinib functions as a covalent irreversible BTK inhibitor that specifically binds to cysteine residue 481 of the kinase domain (130).
The results of a randomized, double-blind, placebo-controlled, 16-week phase 2 trial in 126 RMS or SPMS patients have been published in 2021 (120). A 4-week treatment with placebo at the start or end of the 16-week treatment was combined with a 12-week treatment with tolebrutinib at doses of 5, 15, 30 or 60 mg per day. The primary endpoint was the number of new gadolinium-enhancing lesions after 12 weeks of tolebrutinib treatment, which was achieved by all dose groups, while the strongest effect in the 60 mg group. In these patients, there was a relative reduction of new gadolinium-enhancing lesions of 85% compared to placebo. The observed mean number of lesions was 0.13 for tolebrutinib 60 mg versus 1.03 for placebo (120). Encouraged by the results, four phase III trials are currently underway: The GEMINI I/II twin trials recruited a total of 900 RMS patients with randomized to receive either tolebrutinib or teriflunomide (ClinicalTrials.gov identifier: NCT04410991 - first results are expected in August 2023). The HERCULES trial is testing tolebrutinib against placebo in a planned cohort of 1290 non-relapsing SPMS patients (ClinicalTrials.gov Identifier: NCT04411641 - first results expected in August 2024). 990 PPMS patients are planned to receive tolebrutinib or placebo in the ongoing PERSEUS trial (ClinicalTrials.gov Identifier: NCT04458051 - first results expected in August 2024).
Based on the study results to date, headache and questionable elevation of liver enzymes are potential side effects of tolebrutinib (120). In June 2022, the FDA temporarily placed a partial clinical hold on the trial in this regard (131).
4.4.4 Fenebrutinib
Fenebrutinib is a non-covalent reversible BTK inhibitor that exerts its effect through hydrogen bonding with lysion-430, methionine-477 and apsartate-539 of the kinase domain. Due to the alternative mechanism of action, off-target effects may be avoided (118, 121).
Two phase III trials are currently underway. In the FENtrepid study, placebo-controlled fenebrutinib and ocrelizumabare being tested for their effect in a planned group of 946 PPMS patients (ClinicalTrials.gov Identifier: NCT04544449 - first results expected in October 2025). 734 RMS patients are being compared against the comparator teriflunomide in the FENhance trial (ClinicalTrials.gov Identifier: NCT04586023 - first results expected in October 2025).
Side effects observed in previous studies in rheumatoid arthritis, include nausea, headache, anemia and upper respiratory tract infections (121).
4.4.5 Orelabrutinib
Orelabrutinib irreversibly binds to the kinase domain with exceptionally high selectivity and has been shown to have little binding to other kinases (132, 133).
A phase 2 trial of 160 RMS patients is currently underway, testing three different dose concentrations against placebo (ClinicalTrials.gov identifier: NCT04711148 - first results expected in July 2023).
From previous phase 1 trials, petechiae and headache are coming into focus as possible side effects (133).
4.4.6 Remibrutinib
Remibrutinib is an orally administered irreversible covalently binding BTK inhibitor (127, 134).
Results from MS studies have not yet been presented, but the safety and efficacy profile of the compound have been investigated in a phase I study in healthy volunteers with and without atopic diathesis (134). Encouraged by the results, a phase III trial is currently taking place in which remibrutinib is being tested against the comparator teriflunomide in a collective of 800 RMS patients (ClinicalTrials.gov Identifier: NCT05147220/NCT05156281 - first results expected in October 2025).
5 Conclusion
In summary, important milestones in the drug therapy of MS have been achieved in the last 10 years. While the focus was initially on controlling acute relapses of MS, efforts are increasingly moving in the direction of establishing therapy options for progressive disease. With the CD20-depleting antibody ocrelizumab and the sphingosine receptor modulator siponimod, drugs for primary and secondary chronic MS are available for the first time. Great hopes are pinned on the class of Bruton’s tyrosine kinase inhibitors, which address not only the adaptive but also the innate immune system, which is thought to be responsible for the maintenance of chronic MS disease progression. The results the ongoing phase 3 trials with BTKi’s are eagerly awaited. Meanwhile, the understanding of the pathogenesis of MS, MOGAD and NMOSD continues to deepen. Even though the study situation on drug therapy options in NMOSD and MOGAD has not yet reached the breadth of those in MS, the fields of cytokine antagonists and CD19+ B cell depletors offer candidates for further therapeutic options. All in all, there seems to be reason for cautious optimism that the therapeutic successes of the last 10 years in the field of inflammatory autoimmune CNS diseases can be continued.
Author contributions
Study concept and design: MF, RM, H-PH. Acquisition of data: MF, RM, H-PH. Analysis and interpretation of data: MF, MD, JM, RM, H-PH. Drafting of the manuscript: MF, MD, JM, RM, H-PH. Critical revision of the manuscript for important intellectual content: SM, PA, JM. Graphical support: HB. Study supervision: RM, H-PH.All authors contributed to the article and approved the submitted version.
Funding
SM: research is funded by the German Ministry for Education and Research BMBF, Deutsche Forschungsgemeinschaft DFG, Else Kröner Fresenius Foundation, German Academic Exchange Service, Hertie Foundation, Interdisciplinary Center for Clinical Studies IZKF Muenster, German Foundation Neurology, and by Almirall. The funders were not involved in the study design, collection, analysis, interpretation of data, the writing of this article, or the decision to submit it for publication.
Conflict of interest
SM: received honoraria for lecturing and travel expenses for attending meetings from Almirall, Amicus Therapeutics Germany, Bayer Health Care, Biogen, Celgene, Diamed, Genzyme, MedDay Pharmaceuticals, Merck Serono, Novartis, Novo Nordisk, ONO Pharma, Roche, Sanofi- Aventis, Chugai Pharma, QuintilesIMS, and Teva. PA is employed by the Clinic Maria Hilf GmbH, Germany. He received a research grant from Ipsen Pharma to support the analysis of the data of patients with spasticity and a grant from Merz Pharmaceuticals for funding of NAB-testing. Besides this, he reports grants, personal fees and non-financial support from Allergan, Biogen, Ipsen, Merz Pharmaceuticals, Merck, Novartis, and Roche, personal fees and non-financial support from Bayer Healthcare, and non-financial support from Sanofi-Aventis/Genzyme. RM reports personal fees from Actelion, Biogen and Medison Pharma; grants, personal fees and other from Merck Serono and Teva; personal fees and other from Novartis, Roche; other from TG Therapeutics and MAPI-Pharma, outside the submitted work. H-PH: has received fees for consulting, speaking, and serving on steering committees from Bayer HealthCare, Biogen Idec, Celgene Receptos, CSL Behring, GeNeuro, Genzyme, Horizon Therapeutics formerly Viela Bio, MedDay, MedImmune, Merck Serono, Novartis, Roche, Sanofi, and TG Therapeutics with approval by the Rector of Heinrich Heine University Düsseldorf. JM: presentations and participation in Advisory Boards: Biogen, Novartis, Roche, Sanofi, Merck and Teva.
The remaining authors declare that the research was conducted in the absence of any commercial or financial relationships that could be constructed as a potential conflict of interest.
Publisher’s note
All claims expressed in this article are solely those of the authors and do not necessarily represent those of their affiliated organizations, or those of the publisher, the editors and the reviewers. Any product that may be evaluated in this article, or claim that may be made by its manufacturer, is not guaranteed or endorsed by the publisher.
Supplementary material
The Supplementary Material for this article can be found online at: https://www.frontiersin.org/articles/10.3389/fimmu.2023.1129906/full#supplementary-material
Abbreviations
Ab, antibodies; APRIL, A proliferation inducing ligand; AP-4-Ab, Aquaporin-4-immunoglobulin-G-antibodies; BAFF-R, B cell activating factor receptor; BCMA-R, B cell maturation antigen receptor; BCR, B-cell receptor; BTK, Bruton tyrosine kinase; CD, Cluster of differentiation; DMT, Disease modifying therapy; EBV, Epstein-barr-virus; EDSS, Expanded-disability-status-score, EMA, European Medicines Agency; FDA, Food and drug administration, mAb, Monoclonal antibody, MHC II, Major histocompatibility complex II, NEDA, No evidence of disease activity, NMOSD, Neuromyelitisoptica spectrum disorders, MOGAD, Myelin oligodendrocyte glycoprotein associated autoimmune disease, RMS, Relapsing-remitting multiple sclerosis, PPMS, Primary progressive multiple sclerosis, SPMS, Secondary progressive multiple sclerosis, TACI-R, Transmembran activator and CAML interactor receptor.
References
1. Reich DS, Lucchinetti CF, Calabresi PA. Multiple sclerosis. N Engl J Med (2018) 378:169–80. doi: 10.1056/NEJMra1401483
2. Bar-Or A, Li R. Cellular immunology of relapsing multiple sclerosis: interactions, checks, and balances. Lancet Neurol (2021) 20:470–83. doi: 10.1016/S1474-4422(21)00063-6
3. Lennon VA, Wingerchuk DM, Kryzer TJ, Pittock SJ, Lucchinetti CF, Fujihara K, et al. A serum autoantibody marker of neuromyelitis optica: distinction from multiple sclerosis. Lancet (2004) 364:2106–12. doi: 10.1016/S0140-6736(04)17551-X
4. Lennon VA, Kryzer TJ, Pittock SJ, Verkman AS, Hinson SR. IgG marker of optic-spinal multiple sclerosis binds to the aquaporin-4 water channel. J Exp Med (2005) 202:473–7. doi: 10.1084/jem.20050304
5. Wingerchuk DM, Banwell B, Bennett JL, Cabre P, Carroll W, Chitnis T, et al. International consensus diagnostic criteria for neuromyelitis optica spectrum disorders. Neurology (2015) 85:177–89. doi: 10.1212/WNL.0000000000001729
6. Pittock SJ, Zekeridou A, Weinshenker BG. Hope for patients with neuromyelitis optica spectrum disorders - from mechanisms to trials. Nat Rev Neurol (2021) 17:759–73. doi: 10.1038/s41582-021-00568-8
7. Höftberger R, Lassmann H, Berger T, Reindl M. Pathogenic autoantibodies in multiple sclerosis - from a simple idea to a complex concept. Nat Rev Neurol (2022) 18:681–8. doi: 10.1038/s41582-022-00700-2
8. Oh J, Levy M. Neuromyelitis optica: an antibody-mediated disorder of the central nervous system. Neurol Res Int (2012) 2012:460825. doi: 10.1155/2012/460825
9. Narayan R, Simpson A, Fritsche K, Salama S, Pardo S, Mealy M, et al. MOG antibody disease: A review of MOG antibody seropositive neuromyelitis optica spectrum disorder. Multiple Sclerosis Related Disord (2018) 25:66–72. doi: 10.1016/j.msard.2018.07.025
10. Marignier R, Hacohen Y, Cobo-Calvo A, Pröbstel A-K, Aktas O, Alexopoulos H, et al. Myelin-oligodendrocyte glycoprotein antibody-associated disease. Lancet Neurol (2021) 20:762–72. doi: 10.1016/S1474-4422(21)00218-0
11. Sechi E, Cacciaguerra L, Chen JJ, Mariotto S, Fadda G, Dinoto A, et al. Myelin oligodendrocyte glycoprotein antibody-associated disease (MOGAD): A review of clinical and MRI features, diagnosis, and management. Front Neurol (2022) 13:885218. doi: 10.3389/fneur.2022.885218
12. Abbas AK, Murphy KM, Sher A. Functional diversity of helper T lymphocytes. Nature (1996) 383:787–93. doi: 10.1038/383787a0
13. Sagaert X, de Wolf-Peeters C. Classification of b-cells according to their differentiation status, their micro-anatomical localisation and their developmental lineage. Immunol Lett (2003) 90:179–86. doi: 10.1016/j.imlet.2003.09.007
14. Jackson TR, Ling RE, Roy A. The origin of b-cells: Human fetal b cell development and implications for the pathogenesis of childhood acute lymphoblastic leukemia. Front Immunol (2021) 12:637975. doi: 10.3389/fimmu.2021.637975
15. Tullman MJ, Zabeti A, Vuocolo S, Dinh Q. Inebilizumab for treatment of neuromyelitis optica spectrum disorder. Neurodegenerative Dis Manage (2021) 11. doi: 10.2217/nmt-2021-0017
16. Graf J, Mares J, Barnett M, Aktas O, Albrecht P, Zamvil SS, et al. Targeting b cells to modify MS, NMOSD, and MOGAD: Part 1. Neurol Neuroimmunol Neuroinflamm (2021) 8. doi: 10.1212/NXI.0000000000000918
17. Leandro MJ. B-cell subpopulations in humans and their differential susceptibility to depletionwith anti-CD20 monoclonal antibodies. Arthritis Res Ther (2013) 15:S3. doi: 10.1186/ar3908
18. Singh SP, Dammeijer F, Hendriks RW. Role of bruton’s tyrosine kinase in b cells and malignancies. Mol Cancer (2018) 17:1–23. doi: 10.1186/s12943-018-0779-z
19. Rahmanzadeh R, Weber MS, Brück W, Navardi S, Sahraian MA. B cells in multiple sclerosis therapy-a comprehensive review. Acta Neurol Scand (2018) 137:544–56. doi: 10.1111/ane.12915
20. Baker D, Pryce G, James LK, Schmierer K, Giovannoni G. Failed b cell survival factor trials support the importance of memory b cells in multiple sclerosis. Eur J Neurol (2020) 27:221–8. doi: 10.1111/ene.14105
21. Kumar G, Maria Z, Kohli U, Agasing A, Quinn JL, Ko RM, et al. CNS autoimmune responses in BCMA-deficient mice provide insight for the failure of atacicept in MS. Neurol Neuroimmunol Neuroinflamm (2021) 8. doi: 10.1212/NXI.0000000000000973
22. Vincent FB, Saulep-Easton D, Figgett WA, Fairfax KA, Mackay F. The BAFF/APRIL system: emerging functions beyond b cell biology and autoimmunity. Cytokine Growth Factor Rev (2013) 24:203–15. doi: 10.1016/j.cytogfr.2013.04.003
23. Gelfand JM, Cree BA, Hauser SL. Ocrelizumab and other CD20+ b-Cell-Depleting therapies in multiple sclerosis. Neurotherapeutics (2017) 14:835–41. doi: 10.1007/s13311-017-0557-4
24. Nutt SL, Hodgkin PD, Tarlinton DM, Corcoran LM. The generation of antibody-secreting plasma cells. Nat Rev Immunol (2015) 15:160–71. doi: 10.1038/nri3795
25. Forsthuber TG, Cimbora DM, Ratchford JN, Katz E, Stüve O. B cell-based therapies in CNS autoimmunity: differentiating CD19 and CD20 as therapeutic targets. Ther Adv Neurol Disord (2018) 11:1756286418761697. doi: 10.1177/1756286418761697
26. Rensel M, Zabeti A, Mealy MA, Cimbora D, She D, Drappa J, et al. Long-term efficacy and safety of inebilizumab in neuromyelitis optica spectrum disorder: Analysis of aquaporin-4–immunoglobulin G–seropositive participants taking inebilizumab for ≧̸4 years in the n-MOmentum trial. Mult Scler (2021) 28:925–32. doi: 10.1177/13524585211047223
27. Comi G, Bar-Or A, Lassmann H, Uccelli A, Hartung HP, Montalban X, et al. The role of b cells in multiple sclerosis and related disorders. Ann Neurol (2020) 89:1–11. doi: 10.1002/ana.25927
28. Greenfield AL, Hauser SL. B-cell therapy for multiple sclerosis: Entering an era. Ann Neurol (2018) 83:13–26. doi: 10.1002/ana.25119
29. Milo R. Therapeutic strategies targeting b-cells in multiple sclerosis. Autoimmun Rev (2016) 15:714–8. doi: 10.1016/j.autrev.2016.03.006
30. Milo R. Therapies for multiple sclerosis targeting b cells. Croat Med J (2019) 60:87–98. doi: 10.3325/cmj.2019.60.87
31. Shen P, Fillatreau S. Antibody-independent functions of b cells: a focus on cytokines. Nat Rev Immunol (2015) 15:441–51. doi: 10.1038/nri3857
32. Schafflick D, Wolbert J, Heming M, Thomas C, Hartlehnert M, Börsch A-L, et al. Single-cell profiling of CNS border compartment leukocytes reveals that b cells and their progenitors reside in non-diseased meninges. Nat Neurosci (2021) 24:1225–34. doi: 10.1038/s41593-021-00880-y
33. Ma Q, Caillier SJ, Muzic S, Wilson MR, Henry RG, Cree BA, et al. Specific hypomethylation programs underpin b cell activation in early multiple sclerosis. Proc Natl Acad Sci U.S.A. (2021) 118:e2111920118. doi: 10.1073/pnas.2111920118
34. Compston A, Coles A. Multiple sclerosis. Lancet (2008) 372:1502–17. doi: 10.1016/S0140-6736(08)61620-7
35. Goldmann T, Prinz M. Role of microglia in CNS autoimmunity. Clin Dev Immunol (2013) 2013:1–8. doi: 10.1155/2013/208093
36. Klineova S, Lublin FD. Clinical course of multiple sclerosis. Cold Spring Harb Perspect Med (2018) 8:a028928. doi: 10.1101/cshperspect.a028928
37. Dendrou CA, Fugger L, Friese MA. Immunopathology of multiple sclerosis. Nat Rev Immunol (2015) 15:545–58. doi: 10.1038/nri3871
38. Kezuka T, Usui Y, Goto H. Analysis of the pathogenesis of experimental autoimmune optic neuritis. J BioMed Biotechnol (2011) 2011:294046. doi: 10.1155/2011/294046
39. Berer K, Gerdes LA, Cekanaviciute E, Jia X, Xiao L, Xia Z, et al. Gut microbiota from multiple sclerosis patients enables spontaneous autoimmune encephalomyelitis in mice. Proc Natl Acad Sci U.S.A. (2017) 114:10719–24. doi: 10.1073/pnas.1711233114
40. Yong VW. Microglia in multiple sclerosis: Protectors turn destroyers. Neuron (2022) 110:3534–48. doi: 10.1016/j.neuron.2022.06.023
41. Lee M, Lee Y, Song J, Lee J, Chang S-Y. Tissue-specific role of CX3CR1 expressing immune cells and their relationships with human disease. Immune Netw (2018) 18:1–19. doi: 10.4110/in.2018.18.e5
42. Nayak D, Roth TL, McGavern DB. Microglia development and function. Annu Rev Immunol (2014) 32:367–402. doi: 10.1146/annurev-immunol-032713-120240
43. Silverman SM, Wong WT. Microglia in the retina: Roles in development, maturity, and disease. Annu Rev Vision Sci (2018) 4:45–77. doi: 10.1146/annurev-vision-091517-034425
44. Cruz-Herranz A, Oertel FC, Kim K, Cantó E, Timmons G, Sin JH, et al. Distinctive waves of innate immune response in the retina in experimental autoimmune encephalomyelitis. JCI Insight (2021) 6:e149228. doi: 10.1172/jci.insight.149228
45. Luo C, Jian C, Liao Y, Huang Q, Wu Y, Liu X, et al. The role of microglia in multiple sclerosis. Neuropsychiatr Dis Treat (2017) 13:1661–7. doi: 10.2147/NDT.S140634
46. Sergott RC, Bennett JL, Rieckmann P, Montalban X, Mikol D, Freudensprung U, et al. ATON: results from a phase II randomized trial of the b-cell-targeting agent atacicept in patients with optic neuritis. J Neurological Sci (2015) 351:174–8. doi: 10.1016/j.jns.2015.02.019
47. Jain RW, Yong VW. B cells in central nervous system disease: diversity, locations and pathophysiology. Nat Rev Immunol (2022) 22:513–24. doi: 10.1038/s41577-021-00652-6
48. Bhargava P, Hartung HP, Calabresi PA. Contribution of b cells to cortical damage in multiple sclerosis. Brain (2022) 145:3363–73. doi: 10.1093/brain/awac233
49. Bierhansl L, Hartung H-P, Aktas O, Ruck T, Roden M, Meuth SG. Thinking outside the box: non-canonical targets in multiple sclerosis. Nat Rev Drug Discovery (2022) 21:578–600. doi: 10.1038/s41573-022-00477-5
50. Rojas OL, Pröbstel A-K, Porfilio EA, Wang AA, Charabati M, Sun T, et al. Recirculating intestinal IgA-producing cells regulate neuroinflammation via IL-10. Cell (2019) 176:610–624.e18. doi: 10.1016/j.cell.2018.11.035
51. Pröbstel AK, Zhou X, Baumann R, Wischnewski S, Kutza M, Rojas OL, et al. Gut microbiota-specific IgA+ b cells traffic to the CNS in active multiple sclerosis. Sci Immunol (2020) 5:eabc7191. doi: 10.1126/sciimmunol.abc7191
52. Lanz TV, Brewer RC, Ho PP, Moon J-S, Jude KM, Fernandez D, et al. Clonally expanded b cells in multiple sclerosis bind EBV EBNA1 and GlialCAM. Nature (2022) 603:321–7. doi: 10.1038/s41586-022-04432-7
53. Bjornevik K, Cortese M, Healy BC, Kuhle J, Mina MJ, Leng Y, et al. Longitudinal analysis reveals high prevalence of Epstein-Barr virus associated with multiple sclerosis. Science (2022) 375:296–301. doi: 10.1126/science.abj8222
54. Contentti EC, Correale J. Neuromyelitis optica spectrum disorders: from pathophysiology to therapeutic strategies. J Neuroinflamm (2021) 18:208. doi: 10.1186/s12974-021-02249-1
55. Banwell B, Bennett JL, Marignier R, Kim HJ, Brilot F, Flanagan EP, et al. Diagnosis of myelin oligodendrocyte glycoprotein antibody-associated disease: International MOGAD panel proposed criteria. Lancet Neurol (2023) 22:268–82. doi: 10.1016/S1474-4422(22)00431-8
56. Varrin-Doyer M, Spencer CM, Schulze-Topphoff U, Nelson PA, Stroud RM, Cree BA, et al. Aquaporin 4-specific T cells in neuromyelitis optica exhibit a Th17 bias and recognize clostridium ABC transporter. Ann Neurol (2012) 72:53–64. doi: 10.1002/ana.23651
57. Chisari CG, Sgarlata E, Arena S, Toscano S, Luca M, Patti F. Rituximab for the treatment of multiple sclerosis: a review. J Neurol (2022) 269:159–83. doi: 10.1007/s00415-020-10362-z
58. Bar-Or A, Calabresi PA, Arnold D, Arnlod D, Markowitz C, Shafer S, et al. Rituximab in relapsing-remitting multiple sclerosis: a 72-week, open-label, phase I trial. Ann Neurol (2008) 63:395–400. doi: 10.1002/ana.21363
59. Hauser SL, Waubant E, Arnold DL, Vollmer T, Antel J, Fox RJ, et al. B-cell depletion with rituximab in relapsing-remitting multiple sclerosis. N Engl J Med (2008) 358:676–88. doi: 10.1056/NEJMoa0706383
60. Hawker K, O’Connor P, Freedman MS, Calabresi PA, Antel J, Simon J, et al. Rituximab in patients with primary progressive multiple sclerosis: results of a randomized double-blind placebo-controlled multicenter trial. Ann Neurol (2009) 66:460–71. doi: 10.1002/ana.21867
61. Cree BA, Lamb S, Morgan K, Chen A, Waubant E, Genain C. An open label study of the effects of rituximab in neuromyelitis optica. Neurology (2005) 64:1270–2. doi: 10.1212/01.WNL.0000159399.81861.D5
62. Hartung H-P, Aktas O. Old and new breakthroughs in neuromyelitis optica. Lancet Neurol (2020) 19:280–1. doi: 10.1016/S1474-4422(20)30062-4
63. Tahara M, Oeda T, Okada K, Kiriyama T, Ochi K, Maruyama H, et al. Safety and efficacy of rituximab in neuromyelitis optica spectrum disorders (RIN-1 study): a multicentre, randomised, double-blind, placebo-controlled trial. Lancet Neurol (2020) 19:298–306. doi: 10.1016/S1474-4422(20)30066-1
64. Graf J, Mares J, Barnett M, Aktas O, Albrecht P, Zamvil SS, et al. Targeting b cells to modify MS, NMOSD, and MOGAD: Part 2. Neurol Neuroimmunol Neuroinflamm (2021) 8:e919. doi: 10.1212/NXI.0000000000000919
65. Hacohen Y, Wong YY, Lechner C, Jurynczyk M, Wright S, Konuskan B, et al. Disease course and treatment responses in children with relapsing myelin oligodendrocyte glycoprotein antibody-associated disease. JAMA Neurol (2018) 75:478–87. doi: 10.1001/jamaneurol.2017.4601
66. Whittam DH, Cobo-Calvo A, Lopez-Chiriboga AS, Pardo S, Gornall M, Cicconi S, et al. Treatment of MOG-IgG-associated disorder with rituximab: An international study of 121 patients. Multiple Sclerosis Related Disord (2020) 44:102251. doi: 10.1016/j.msard.2020.102251
67. Nepal G, Kharel S, Coghlan MA, Rayamajhi P, Ojha R. Safety and efficacy of rituximab for relapse prevention in myelin oligodendrocyte glycoprotein immunoglobulin G (MOG-IgG)-associated disorders (MOGAD): A systematic review and meta-analysis. J Neuroimmunol (2022) 364:577812. doi: 10.1016/j.jneuroim.2022.577812
68. Ramanathan S, Mohammad S, Tantsis E, Nguyen TK, Merheb V, Fung VS, et al. Clinical course, therapeutic responses and outcomes in relapsing MOG antibody-associated demyelination. J Neurol Neurosurg Psychiatry (2018) 89:127–37. doi: 10.1136/jnnp-2017-316880
69. Ambrosius W, Michalak S, Kozubski W, Kalinowska A. Myelin oligodendrocyte glycoprotein antibody-associated disease: Current insights into the disease pathophysiology, diagnosis and management. Int J Mol Sci (2020) 22:100. doi: 10.3390/ijms22010100
70. Morschhauser F, Marlton P, Vitolo U, Linden O, Seymour J, Crump M, et al. Interim results of a phase I/II study of ocrelizumab, a new humanised anti-CD20 antibody in patients with Relapsed/Refractory follicular non-hodgkin’s lymphoma. Blood (2007) 110:645. doi: 10.1182/blood.V110.11.645.645
71. Kappos L, Li D, Calabresi PA, O’Connor P, Bar-Or A, Barkhof F, et al. Ocrelizumab in relapsing-remitting multiple sclerosis: a phase 2, randomised, placebo-controlled, multicentre trial. Lancet (2011) 378:1779–87. doi: 10.1016/S0140-6736(11)61649-8
72. Hauser SL, Bar-Or A, Comi G, Giovannoni G, Hartung H-P, Hemmer B, et al. Ocrelizumab versus interferon beta-1a in relapsing multiple sclerosis. N Engl J Med (2017) 376:221–34. doi: 10.1056/NEJMoa1601277
73. Hauser SL, Kappos L, Arnold DL, Bar-Or A, Brochet B, Naismith RT, et al. Five years of ocrelizumab in relapsing multiple sclerosis: OPERA studies open-label extension. Neurology (2020) 95:e1854–67. doi: 10.1212/WNL.0000000000010376
74. Montalban X, Hauser SL, Kappos L, Arnold DL, Bar-Or A, Comi G, et al. Ocrelizumab versus placebo in primary progressive multiple sclerosis. N Engl J Med (2017) 376:209–20. doi: 10.1056/NEJMoa1606468
75. Wolinsky JS, Engmann NJ, Pei J, Pradhan A, Markowitz C, Fox EJ. An exploratory analysis of the efficacy of ocrelizumab in patients with multiple sclerosis with increased disability. Mult Scler J Exp Transl Clin (2020) 6:2055217320911939. doi: 10.1177/2055217320911939
76. Wolinsky JS, Brochet B, Hartung HP, Naismith RT, Airas L, Coutant K, et al. Sustained reduction in confirmed disability progression after 6.5 study-years of ocrelizumab treatment in patients with primary progressive multiple sclerosis. J Neurological Sci (2019) 405:31. doi: 10.1016/j.jns.2019.10.269
77. McCool R, Wilson K, Arber M, Fleetwood K, Toupin S, Thom H, et al. Systematic review and network meta-analysis comparing ocrelizumab with other treatments for relapsing multiple sclerosis. Multiple Sclerosis Related Disord (2019) 29:55–61. doi: 10.1016/j.msard.2018.12.040
78. Weinstock-Guttman B, Bermel R, Cutter G, Freedman MS, Leist TP, Ma X, et al. Ocrelizumab treatment for relapsing-remitting multiple sclerosis after a suboptimal response to previous disease-modifying therapy: A nonrandomized controlled trial. Mult Scler (2022) 28:790–800. doi: 10.1177/13524585211035740
79. Vermersch P, Oreja-Guevara C, Siva A, van Wijmeersch B, Wiendl H, Wuerfel J, et al. Efficacy and safety of ocrelizumab in patients with relapsing-remitting multiple sclerosis with suboptimal response to prior disease-modifying therapies: A primary analysis from the phase 3b CASTING single-arm, open-label trial. Eur J Neurol (2022) 29:790–801. doi: 10.1111/ene.15171
80. Hartung H-P. Ocrelizumab shorter infusion: Primary results from the ENSEMBLE PLUS substudy in patients with MS. Neurol Neuroimmunol Neuroinflamm (2020) 7:e807. doi: 10.1212/NXI.0000000000000807
81. Wiendl H, Gold R, Berger T, Derfuss T, Linker R, Mäurer M, et al. Multiple sclerosis therapy consensus group (MSTCG): position statement on disease-modifying therapies for multiple sclerosis (white paper). Ther Adv Neurol Disord (2021) 14:17562864211039648. doi: 10.1177/17562864211039648
82. Hauser SL, Kappos L, Montalban X, Craveiro L, Chognot C, Hughes R, et al. Safety of ocrelizumab in patients with relapsing and primary progressive multiple sclerosis. Neurology (2021) 97:e1546–59. doi: 10.1212/WNL.0000000000012700
83. Hauser SL, Cross AH, Winthrop K, Wiendl H, Nicholas J, Meuth SG, et al. Safety experience with continued exposure to ofatumumab in patients with relapsing forms of multiple sclerosis for up to 3.5 years. Mult Scler (2022) 28:1576–90. doi: 10.1177/13524585221079731
84. Bar-Or A, Grove RA, Austin DJ, Tolson JM, VanMeter SA, Lewis EW, et al. Subcutaneous ofatumumab in patients with relapsing-remitting multiple sclerosis: The MIRROR study. Neurology (2018) 90:e1805–14. doi: 10.1212/WNL.0000000000005516
85. Hauser SL, Bar-Or A, Cohen JA, Comi G, Correale J, Coyle PK, et al. Ofatumumab versus teriflunomide in multiple sclerosis. N Engl J Med (2020) 383:546–57. doi: 10.1056/NEJMoa1917246
86. Steinman L, Fox E, Hartung H-P, Alvarez E, Qian P, Wray S, et al. Ublituximab versus teriflunomide in relapsing multiple sclerosis. N Engl J Med (2022) 387:704–14. doi: 10.1056/NEJMoa2201904
87. Fox E, Lovett-Racke AE, Gormley M, Liu Y, Petracca M, Cocozza S, et al. A phase 2 multicenter study of ublituximab, a novel glycoengineered anti-CD20 monoclonal antibody, in patients with relapsing forms of multiple sclerosis. Mult Scler (2021) 27:420–9. doi: 10.1177/1352458520918375
88. FDA Roundup: December 30, 2022. Available at: https://www.fda.gov/news-events/press-announcements/fda-roundup-december-30-2022.
89. Mealy MA, Levy M. A pilot safety study of ublituximab, a monoclonal antibody against CD20, in acute relapses of neuromyelitis optica spectrum disorder. Med (Baltimore) (2019) 98:e15944. doi: 10.1097/MD.0000000000015944
90. Iaffaldano P, Lucisano G, Manni A, Paolicelli D, Patti F, Capobianco M, et al. Risk of getting COVID-19 in people with multiple sclerosis: A case-control study. Neurol Neuroimmunol Neuroinflamm (2022) 9:e1141. doi: 10.1212/NXI.0000000000001141
91. Winkelmann A, Loebermann M, Barnett M, Hartung H-P, Zettl UK. Vaccination and immunotherapies in neuroimmunological diseases. Nat Rev Neurol (2022) 18:289–306. doi: 10.1038/s41582-022-00646-5
92. Disanto G, Sacco R, Bernasconi E, Martinetti G, Keller F, Gobbi C, et al. Association of disease-modifying treatment and anti-CD20 infusion timing with humoral response to 2 SARS-CoV-2 vaccines in patients with multiple sclerosis. JAMA Neurol (2021) 78:1529–31. doi: 10.1001/jamaneurol.2021.3609
93. Wu X, Wang L, Shen L, Tang K. Response of COVID-19 vaccination in multiple sclerosis patients following disease-modifying therapies: A meta-analysis. EBioMedicine (2022) 81:104102. doi: 10.1016/j.ebiom.2022.104102
94. Madelon N, Heikkilä N, Sabater Royo I, Fontannaz P, Breville G, Lauper K, et al. Omicron-specific cytotoxic T-cell responses after a third dose of mRNA COVID-19 vaccine among patients with multiple sclerosis treated with ocrelizumab. JAMA Neurol (2022) 79:399–404. doi: 10.1001/jamaneurol.2022.0245
95. Madelon N, Lauper K, Breville G, Sabater Royo I, Goldstein R, Andrey DO, et al. Robust T-cell responses in anti-CD20-Treated patients following COVID-19 vaccination: A prospective cohort study. Clin Infect Dis (2022) 75:e1037–45. doi: 10.1093/cid/ciab954
96. Sormani MP, de Rossi N, Schiavetti I, Carmisciano L, Cordioli C, Moiola L, et al. Disease-modifying therapies and coronavirus disease 2019 severity in multiple sclerosis. Ann Neurol (2021) 89:780–9. doi: 10.1002/ana.26028
97. Sormani MP, Inglese M, Schiavetti I, Carmisciano L, Laroni A, Lapucci C, et al. Effect of SARS-CoV-2 mRNA vaccination in MS patients treated with disease modifying therapies. EBioMedicine (2021) 72:103581. doi: 10.1016/j.ebiom.2021.103581
98. Sormani MP, Schiavetti I, Inglese M, Carmisciano L, Laroni A, Lapucci C, et al. Breakthrough SARS-CoV-2 infections after COVID-19 mRNA vaccination in MS patients on disease modifying therapies during the delta and the omicron waves in Italy. EBioMedicine (2022) 80:104042. doi: 10.1016/j.ebiom.2022.104042
99. Räuber S, Korsen M, Huntemann N, Rolfes L, Müntefering T, Dobelmann V, et al. Immune response to SARS-CoV-2 vaccination in relation to peripheral immune cell profiles among patients with multiple sclerosis receiving ocrelizumab. J Neurol Neurosurg Psychiatry (2022) 93:978–85. doi: 10.1136/jnnp-2021-328197
100. Hughes R, Whitley L, Fitovski K, Schneble H-M, Muros E, Sauter A, et al. COVID-19 in ocrelizumab-treated people with multiple sclerosis. Multiple Sclerosis Related Disord (2021) 49:102725. doi: 10.1016/j.msard.2020.102725
101. Simpson-Yap S, de Brouwer E, Kalincik T, Rijke N, Hillert JA, Walton C, et al. Associations of disease-modifying therapies with COVID-19 severity in multiple sclerosis. Neurology (2021) 97:e1870–85. doi: 10.1212/WNL.0000000000012753
102. Flanagan EP, Levy M, Katz E, Cimbora D, Drappa J, Mealy MA, et al. Inebilizumab for treatment of neuromyelitis optica spectrum disorder in patients with prior rituximab use from the n-MOmentum study. Multiple Sclerosis Related Disord (2022) 57:103352. doi: 10.1016/j.msard.2021.103352
103. Agius MA, Klodowska-Duda G, Maciejowski M, Potemkowski A, Li J, Patra K, et al. Safety and tolerability of inebilizumab (MEDI-551), an anti-CD19 monoclonal antibody, in patients with relapsing forms of multiple sclerosis: Results from a phase 1 randomised, placebo-controlled, escalating intravenous and subcutaneous dose study. Mult Scler (2017) 25:235–45. doi: 10.1177/1352458517740641
104. Cree BA, Bennett JL, Kim HJ, Weinshenker BG, Pittock SJ, Wingerchuk DM, et al. Inebilizumab for the treatment of neuromyelitis optica spectrum disorder (N-MOmentum): a double-blind, randomised placebo-controlled phase 2/3 trial. Lancet (2019) 394:1352–63. doi: 10.1016/S0140-6736(19)31817-3
105. Ringelstein M, Ayzenberg I, Lindenblatt G, Fischer K, Gahlen A, Novi G, et al. Interleukin-6 receptor blockade in treatment-refractory MOG-IgG-Associated disease and neuromyelitis optica spectrum disorders. Neurol Neuroimmunol Neuroinflamm (2022) 9:e1100. doi: 10.1212/NXI.0000000000001100
106. Hartung H-P, Kieseier BC. Atacicept: targeting b cells in multiple sclerosis. Ther Adv Neurol Disord (2010) 3:205–16. doi: 10.1177/1756285610371146
107. Kappos L, Hartung H-P, Freedman MS, Boyko A, Radü EW, Mikol DD, et al. Atacicept in multiple sclerosis (ATAMS): a randomised, placebo-controlled, double-blind, phase 2 trial. Lancet Neurol (2014) 13:353–63. doi: 10.1016/S1474-4422(14)70028-6
108. Lühder F, Gold R. Trial and error in clinical studies: lessons from ATAMS. Lancet Neurol (2014) 13:340–1. doi: 10.1016/S1474-4422(14)70050-X
109. Shen T, You Y, Arunachalam S, Fontes A, Liu S, Gupta V, et al. Differing structural and functional patterns of optic nerve damage in multiple sclerosis and neuromyelitis optica spectrum disorder. Ophthalmology (2018) 126:1–9. doi: 10.1016/j.ophtha.2018.06.022
110. Dhillon S. Telitacicept: First approval. Drugs (2021) 81:1671–5. doi: 10.1007/s40265-021-01591-1
111. Shi F, Xue R, Zhou X, Shen P, Wang S, Yang Y. Telitacicept as a BLyS/APRIL dual inhibitor for autoimmune disease. Immunopharmacol Immunotoxicol (2021) 43:666–73. doi: 10.1080/08923973.2021.1973493
112. Ding J, Jiang X, Cai Y, Pan S, Deng Y, Gao M, et al. Telitacicept following plasma exchange in the treatment of subjects with recurrent neuromyelitis optica spectrum disorders: A single-center, single-arm, open-label study. CNS Neurosci Ther (2022) 28:1613–23. doi: 10.1111/cns.13904
113. Heo Y-A. Correction to: Satralizumab: First approval. Drugs (2020) 80:1483. doi: 10.1007/s40265-020-01391-z
114. Chihara N, Aranami T, Sato W, Miyazaki Y, Miyake S, Okamoto T, et al. Interleukin 6 signaling promotes anti-aquaporin 4 autoantibody production from plasmablasts in neuromyelitis optica. Proc Natl Acad Sci U.S.A. (2011) 108:3701–6. doi: 10.1073/pnas.1017385108
115. Yamamura T, Kleiter I, Fujihara K, Palace J, Greenberg B, Zakrzewska-Pniewska B, et al. Trial of satralizumab in neuromyelitis optica spectrum disorder. N Engl J Med (2019) 381:2114–24. doi: 10.1056/NEJMoa1901747
116. Traboulsee A, Greenberg BM, Bennett JL, Szczechowski L, Fox E, Shkrobot S, et al. Safety and efficacy of satralizumab monotherapy in neuromyelitis optica spectrum disorder: a randomised, double-blind, multicentre, placebo-controlled phase 3 trial. Lancet Neurol (2020) 19:402–12. doi: 10.1016/S1474-4422(20)30078-8
117. Martin E, Aigrot MS, Grenningloh R, Stankoff B, Lubetzki C, Boschert U, et al. Bruton’s tyrosine kinase inhibition promotes myelin repair. Brain Plasticity (Amsterdam Netherlands) (2020) 5:123–33. doi: 10.3233/BPL-200100
118. García-Merino A. Bruton’s tyrosine kinase inhibitors: A new generation of promising agents for multiple sclerosis therapy. Cells (2021) 10:2560. doi: 10.3390/cells10102560
119. Jayagopol LA, Zabad RK. Bruton tyrosine kinase inhibition in multiple sclerosis. the missing link for treatment optimization? Pract Neurol, 29–32. Available at: https://practicalneurology.com/articles/2022-feb/bruton-tyrosine-kinase-inhibition-in-multiple-sclerosis/pdf.
120. Reich DS, Arnold DL, Vermersch P, Bar-Or A, Fox RJ, Matta A, et al. Safety and efficacy of tolebrutinib, an oral brain-penetrant BTK inhibitor, in relapsing multiple sclerosis: a phase 2b, randomised, double-blind, placebo-controlled trial. Lancet Neurol (2021) 20:729–38. doi: 10.1016/S1474-4422(21)00237-4
121. Cohen S, Tuckwell K, Katsumoto TR, Zhao R, Galanter J, Lee C, et al. Fenebrutinib versus placebo or adalimumab in rheumatoid arthritis: A randomized, double-blind, phase II trial. Arthritis Rheumatol (2020) 72:1435–46. doi: 10.1002/art.41275
122. Weber ANR, Bittner Z, Liu X, Dang T-M, Radsak MP, Brunner C. Bruton’s tyrosine kinase: An emerging key player in innate immunity. Front Immunol (2017) 8:1454. doi: 10.3389/fimmu.2017.01454
123. Zhang M, Chang Y-C, Shankara S, Jacobs A, Godin J, Klinger K, et al. Poster, LB315, Comparative Transcriptome Analysis of Gene Co-Expression Networks in Relapsing-Remitting Multiple Sclerosis Patients and Healthy Controls. ACTRIMS 2019 - late breaking news posters. Mult Scler (2019) 25:157–65. doi: 10.1177/1352458519843084
124. de Gracia P, Gallego BI, Rojas B, Ramírez AI, de Hoz R, Salazar JJ, et al. Automatic counting of microglial cells in healthy and glaucomatous mouse retinas. PloS One (2015) 10:1–16. doi: 10.1371/journal.pone.0143278
125. Frenger MJ, Hecker C, Sindi M, Issberner A, Hartung H-P, Meuth SG, et al. Semi-automated live tracking of microglial activation in CX3CR1GFP mice during experimental autoimmune encephalomyelitis by confocal scanning laser ophthalmoscopy. Front Immunol (2021) 12:761776. doi: 10.3389/fimmu.2021.761776
126. Yong HY, Yong VW. Mechanism-based criteria to improve therapeutic outcomes in progressive multiple sclerosis. Nat Rev Neurol (2022) 18:40–55. doi: 10.1038/s41582-021-00581-x
127. Geladaris A, Torke S, Weber MS. Bruton’s tyrosine kinase inhibitors in multiple sclerosis: Pioneering the path towards treatment of progression? CNS Drugs (2022) 36:1019–30. doi: 10.1007/s40263-022-00951-z
128. Haselmayer P, Camps M, Liu-Bujalski L, Nguyen N, Morandi F, Head J, et al. Efficacy and pharmacodynamic modeling of the BTK inhibitor evobrutinib in autoimmune disease models. J Immunol (2019) 202:2888–906. doi: 10.4049/jimmunol.1800583
129. Montalban X, Arnold DL, Weber MS, Staikov I, Piasecka-Stryczynska K, Willmer J, et al. Placebo-controlled trial of an oral BTK inhibitor in multiple sclerosis. N Engl J Med (2019) 380:2406–17. doi: 10.1056/NEJMoa1901981
130. Owens TD, Smith PF, Redfern A, Xing Y, Shu J, Karr DE, et al. Phase 1 clinical trial evaluating safety, exposure and pharmacodynamics of BTK inhibitor tolebrutinib (PRN2246, SAR442168). Clin Transl Sci (2022) 15:442–50. doi: 10.1111/cts.13162
131. Media update: Patient enrollment of phase III tolebrutinib trials paused in the U.S. - sanofi (2022.000Z) (2022). Available at: https://www.sanofi.com/en/media-room/press-releases/2022/2022-06-30-05-30-00-2471767.
132. Dhillon S. Orelabrutinib: First approval. Drugs (2021) 81:503–7. doi: 10.1007/s40265-021-01482-5
133. Zhang B, Zhao R, Liang R, Gao Y, Liu R, Chen X, et al. Abstract CT132: Orelabrutinib, a potent and selective bruton’s tyrosine kinase inhibitor with superior safety profile and excellent PK/PD properties. Cancer Res (2020) 80:CT132–2. doi: 10.1158/1538-7445.AM2020-CT132
Keywords: B cell depletion, multiple sclerosis (MS), neuromyelitisoptica spectrum disorders (NMOSD), myelin oligodendrocyte glycoprotein associated autoimmune disease (MOGAD), autoimmune disease of the central nervous system
Citation: Furman MJ, Meuth SG, Albrecht P, Dietrich M, Blum H, Mares J, Milo R and Hartung H-P (2023) B cell targeted therapies in inflammatory autoimmune disease of the central nervous system. Front. Immunol. 14:1129906. doi: 10.3389/fimmu.2023.1129906
Received: 22 December 2022; Accepted: 23 February 2023;
Published: 09 March 2023.
Edited by:
Rui Li, University of Pennsylvania, United StatesReviewed by:
Javier Ochoa-Repáraz, Boise State University, United StatesBobby Kwanghoon Han, University of Washington, United States
Copyright © 2023 Furman, Meuth, Albrecht, Dietrich, Blum, Mares, Milo and Hartung. This is an open-access article distributed under the terms of the Creative Commons Attribution License (CC BY). The use, distribution or reproduction in other forums is permitted, provided the original author(s) and the copyright owner(s) are credited and that the original publication in this journal is cited, in accordance with accepted academic practice. No use, distribution or reproduction is permitted which does not comply with these terms.
*Correspondence: Hans-Peter Hartung, hartunghp@gmail.com
†These authors share senior authorship