- 1Department of Gastroenterology and Hepatology, University of Groningen, University Medical Center Groningen, Groningen, Netherlands
- 2Department of Medical Microbiology and Infection Prevention, University of Groningen, University Medical Center Groningen, Groningen, Netherlands
The intestinal mucosa is constantly exposed to commensal microbes, opportunistic pathogens, toxins, luminal components and other environmental stimuli. The intestinal mucosa consists of multiple differentiated cellular and extracellular components that form a critical barrier, but is also equipped for efficient absorption of nutrients. Combination of genetic susceptibility and environmental factors are known as critical components involved in the pathogenesis of intestinal diseases. The innate immune system plays a critical role in the recognition and elimination of potential threats by detecting pathogen-associated molecular patterns (PAMPs) and damage-associated molecular patterns (DAMPs). This host defense is facilitated by pattern recognition receptors (PRRs), in which the cyclic GMP-AMP synthase-stimulator of interferon genes (cGAS-STING) pathway has gained attention due to its role in sensing host and foreign double-stranded DNA (dsDNA) as well as cyclic dinucleotides (CDNs) produced by bacteria. Upon binding with dsDNA, cGAS converts ATP and GTP to cyclic GMP-AMP (cGAMP), which binds to STING and activates TANK binding kinase 1 (TBK1) and interferon regulatory factor 3 (IRF3), inducing type I interferon (IFN) and nuclear factor kappa B (NF-κB)-mediated pro-inflammatory cytokines, which have diverse effects on innate and adaptive immune cells and intestinal epithelial cells (IECs). However, opposite perspectives exist regarding the role of the cGAS-STING pathway in different intestinal diseases. Activation of cGAS-STING signaling is associated with worse clinical outcomes in inflammation-associated diseases, while it also plays a critical role in protection against tumorigenesis and certain infections. Therefore, understanding the context-dependent mechanisms of the cGAS-STING pathway in the physiopathology of the intestinal mucosa is crucial for developing therapeutic strategies targeting the cGAS-STING pathway. This review aims to provide insight into recent findings of the protective and detrimental roles of the cGAS-STING pathway in intestinal diseases.
Introduction
The intestinal mucosa is a selectively permeable barrier where nutrients are absorbed and immunologic tolerance is induced, but also a physical barrier that prevents against direct tissue injury caused by gut luminal content (1). The intestinal mucosa consists of multiple differentiated cellular and extracellular components: 1) a single-polarized layer of IECs with specialized subtypes (e.g., enterocytes, Paneth cells, goblet cells and enteroendocrine cells) attached to each other and to basal membrane; 2) cells within the subepithelial lamina propria (such as stromal cells and immune cells); 3) biochemical elements secreted by those cells (e.g. regenerating islet-derived protein 3 gamma (Reg3γ) and defensins) (2). Combinations of genetic susceptibility and environmental and lifestyle factors, including dietary and microbiome changes, alcohol consumption, exposure to toxins or detergents, infections and smoking, contribute to intestinal barrier dysfunction, mucosal inflammation and dysbiosis. These factors are increasingly acknowledged as critical mechanisms involved in the development of intestinal diseases (3).
As the first line of defense against complex exposures and a bridge to the adaptive system, the innate immune system has evolved various sensing mechanisms modulated by PRRs to detect and counteract microbial invasion by recognizing PAMPs and DAMPs (4). PRRs mainly include Toll-like receptors (TLRs) (5), retinoic acid-inducible gene I (RIG-I)-like receptors (RLRs) (6), nucleotide oligomerization domain (NOD)-like receptors (NLRs) (7), C-type lectin receptors (CLRs) (8), absent in melanoma-2 (AIM2)-like receptors (ALRs) (9), as well as other DNA and RNA sensors (10–15). The competence of sensing microbial pathogens through recognition of their nucleic acids (RNA and DNA) has been considered as a key feature of innate immunity in mammalian cells (16, 17). One major family of cytokines fundamentally involved in antimicrobial host defense are the type I IFN, which exerts antiviral, anti-proliferative, anti-tumor and a multitude of regulatory actions on innate and adaptive immune responses, involving antigen presentation and the differentiation of both CD4+ and CD8+ T cells (18, 19). Just over a decade ago, a signaling pathway that controls the production of type I IFN was discovered, which consists of the cGAS for generating the second messenger cGAMP and cGAMP receptor STING (20, 21). The nucleic acids of bacteria, viruses and parasites can enter cells through various mechanisms, such as phagocytosis, endocytosis and membrane fusion (22–24). Pathogen-related infections also causes injury to host cell and release of their genomic and mitochondrial DNA (mtDNA) (25–27). Upon direct binding with host or foreign dsDNA, cytosolic cGAS undergoes a switch-like conformational change that activates its enzymatic activity, converting GTP and ATP to the second messenger cGAMP (28, 29). Subsequently, cGAMP binds to STING, a transmembrane adaptor located at the endoplasmic reticulum (ER), to promote the phosphorylation of TBK1 and IRF3, and thereby inducing type I IFN production and NF-κB-dependent expression of pro-inflammatory cytokines in the innate immune system (30). In addition to cGAMP synthesized by cGAS, the bacterial-produced CDNs (c-di-AMP and c-di-GMP) and canonical cGAMP (e.g. 3’3’-cGAMP) can be directly sensed by STING as well, which is reviewed in detail by Liu et al. (31). Multiple studies have demonstrated the critical role of the cGAS-STING signaling in intestinal pathophysiology, however, both protective and detrimental roles of cGAS-STING signaling have been reported. On the one hand, the activation of cGAS-STING signaling is associated with the severity of intestinal inflammation. On the other hand, it plays a critical role in maintaining the intestinal structure and environment, as well as protecting against tumorigenesis and infections. It is known that inflammation displays opposing effects in cancer development and immunotherapy, such as immune checkpoint inhibitors and cancer vaccines. Inflammation can facilitate tumorigenesis and immune resistance but can also induce differentiation of immune cells and antigen presentation to perform an anti-cancer immune response (32–34). Therefore, it is necessary to elucidate the shift from a promoter to a protector role of the cGAS-STING pathway in the transition from intestinal inflammation, cancer, infections and tissue repair. Understanding the intricate mechanisms of the cGAS-STING pathway in the pathophysiology of the intestinal mucosa is crucial for the development of effective therapeutic interventions targeting this signaling pathway. In this review, we describe recent findings of the cGAS-STING pathway according to its protective and detrimental roles in intestinal homeostasis and diseases.
The cGAS-STING signaling pathway
The cGAS-STING signaling pathway is graphically presented in Figure 1. The MB21D1 gene (also known as C6orf150), located on chromosome 6q13, is one of the most rapidly diverging genes in the human genome. It encodes the 60 kDa nucleotidyltransferase enzyme cGAS of 522 amino acids and is activated by endogenous dsDNA (e.g., damaged genomic DNA and mtDNA) and exogenous dsDNA (from viruses, bacteria and parasites) (35, 36). cGAS belongs to the structurally conserved cGAS/DncV-like nucleotidyltransferase superfamily, which consists of a positively charged N-terminal domain (aa1-160) and conserved C-terminal domain (aa161-522) that includes the NTase core (aa160-330) and the male abnormal 21 (Mab21) domain (aa213-513) with zinc-ribbon insertion (37–39). Human cGAS has three DNA-binding sites, defined as site A, B and C, where cGAS interacts with dsDNA via electrostatic interactions and hydrogen bonds. The interface of cGAS and dsDNA form salt bridges with the phosphate backbone of the DNA and then form a 2:2 complex with dsDNA in an asymmetrical manner (29, 40). The binding of cGAS to DNA depends on the DNA length in a sequence-independent manner (41, 42). Human cGAS K187 and L195 are two substitutions in the cGAS DNA-binding surface that enhance the ability of cGAS to distinguish short (~20 bp) and long (>45 bp) DNAs (40). The activity of cGAS is dependent on the length of the dsDNA and some species-specific differences have been reported (29, 43). While the catalytic activity of mouse cGAS activity is strongly induced by short dsDNA of ~18 bp (29), long dsDNA (>45 bp) more efficiently promotes human cGAS enzymatic activity than the shorter counterparts. Enzymatic activity of human cGAS appeared also dependent on the concentrations of zinc (43). The C-terminal domain of cGAS is critical for activating the enzymatic activity upon dsDNA binding through triggering conformational changes (42, 44, 45), in which the conversion of ATP and GTP to cGAMP happens (21, 44, 46). Under physiological conditions, the continuous cytosolic clearance of endogenous DNA via cytosolic 3-prime repair exonuclease 1 (TREX1) and lysosomal DNase II is important to avoid the overactivation of the cGAS-STING signaling (47, 48). Notably, other DNA sensors, such as IFI16, DDX41, DAI (DLM-1/ZBP1) and MRE11 might also be involved in the modulation of the cGAS-STING-type I IFN pathway (10–13). For instance, IFN-γ inducible protein 16 (IFI16) can bind to exogenous DNA and form a complex with cGAS, synergizing cGAMP-induced phosphorylation and translocation of STING (13). Detailed analysis interaction between these other DNA sensors and the cGAS-STING signaling pathway is needed to better understand the context-specific role of cGAS-STING activation.
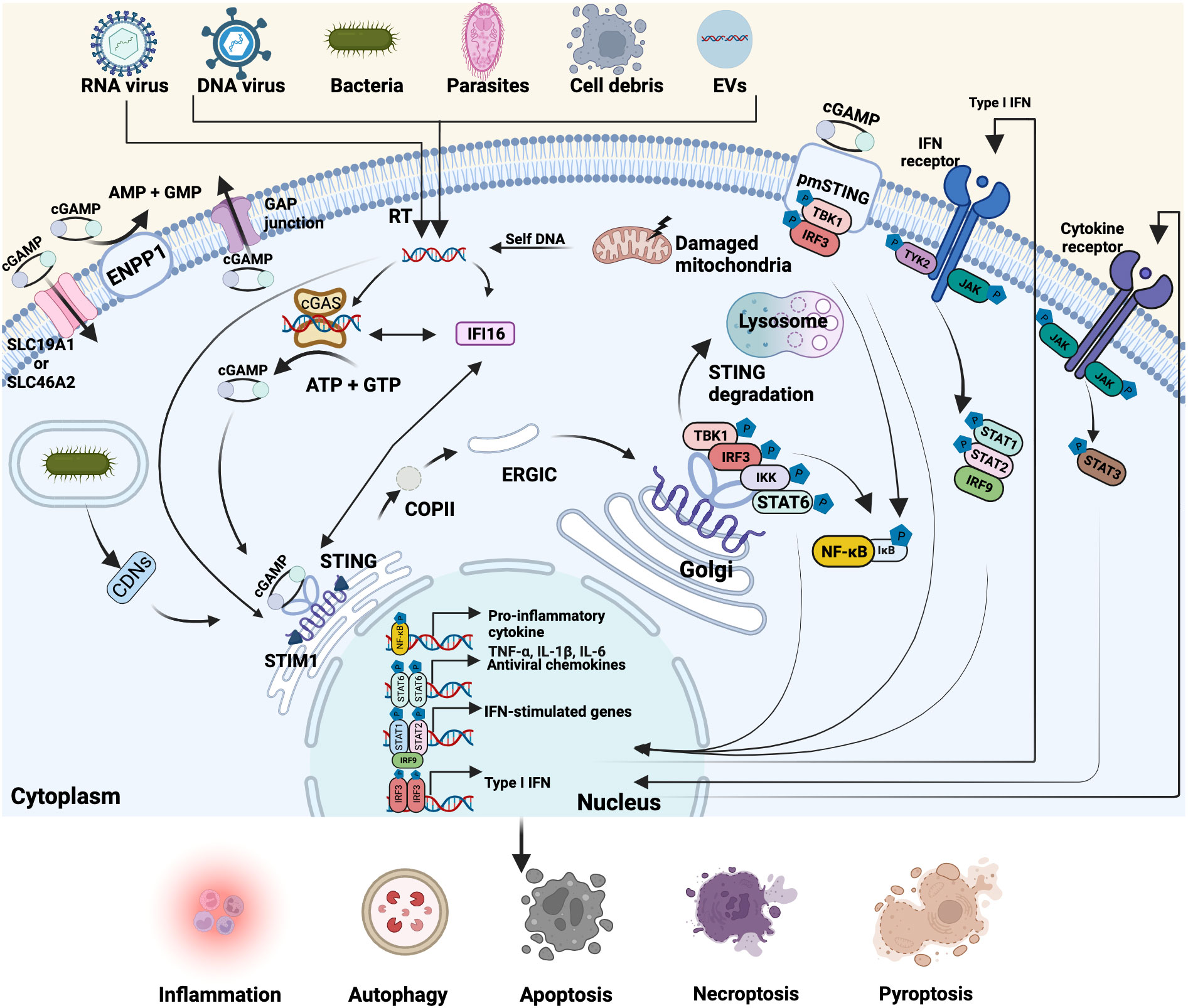
Figure 1 Overview of the cGAS-STING pathway. Cyclic GMP-AMP synthase (cGAS)-stimulator of interferon genes (STING) pathway is involved in multiple biological processes. Cytoplasmic cGAS can recognize exogenous and endogenous double-strand DNA (dsDNA) from various sources, including DNA viruses, reverse-transcription of RNA viruses, bacteria, parasites, cell debris, extracellular vesicles (EVs), as well as the damaged mitochondria- and self-DNA from the nucleus. Interferon-gamma inducible protein 16 (IFI16) directly interacts with cytosolic dsDNA, cGAS and STING, facilitating the activation of cGAS and STING. Activated cGAS then catalyzes the synthesis of the second messenger cyclic GMP-AMP (cGAMP) from ATP and GTP. Cyclic dinucleotides (CDNs) of bacteria, cGAMP or dsDNA binds to endoplasmic reticulum (ER)-located STING that is retained by stromal interaction molecule 1 (STIM1) and initiates STING translocation to the Golgi apparatus via coat protein complex II (COPII) and ER-Golgi intermediate compartment (ERGIC). Then STING recruits and phosphorylates TBK1 and IRF3 and simultaneously activates IΚB kinase (IKK) and signal transducer and activator of transcription 6 (STAT6), triggering the transcription of pro-inflammatory cytokines, antiviral chemokines, IFN-stimulated genes and Type I IFN. In parallel, some cGAMP can be delivered to the outside of cells through the GAP junction, binding with the plasmatic membrane (pmSTING) and driving the same activation response or enter other cells through solute carrier family 19 member 1 (SLC19A1) and SLC46A2. The rest of cGAMP can be degraded by membrane hydrolase ectonucleotide pyrophosphatase phosphodiesterase 1 (ENPP1). Post-Golgi STING vesicles are either fused or encapsulated by lysosomes and gradually eliminated. After type I IFN and cytokines (e.g. IL-6) are secreted outside, they can bind to IFN receptor and cytokine receptor (e.g. IL-6R/GP130) on the membrane respectively and then activate Janus kinases (JAK)-STAT signaling pathway. Created with BioRender.com.
STING (also named MITA, MPYS and ERIS), encoded by the TMEM173 gene, is an evolutionary conserved transmembrane protein consisting of 379 amino acids with a molecular weight of 42 kDa, widely existing in diverse species and cell lineages, including endothelial cells, epithelial cells, immune cells (T cells, macrophages, dendritic cells (DCs)) and hematopoietic cells (20, 49, 50). STING predominantly resides in the ER and forms a highly structured wing-like hydrophobic dimer facing toward the cytosol (51). The N-terminal domain of STING includes four transmembrane helices to anchor this adaptor protein to the ER membrane, and the C-terminal domain of STING comprises a ligand-binding domain and a C-terminal tail, which are responsible for binding cGAMP, CDNs and TBK1 (52–54). Recently, plasmatic membrane STING (pmSTING), an alternatively-spliced STING isoform, located in the cytoplasmic membrane with C-terminal tail projecting outside the cell, has been identified in many species, including humans. Human pmSTING can sense extracellular cGAMP and activates TBK1-IRF3-type I IFN signaling the same way as the canonical one (55). Since the subcellular localization of STING can influence its function and interaction with cellular and extracellular components, further studies on the functional differences of ER-located STING and pmSTING are needed. During the resting/non-activated state, STING is retained in the ER by associating with stromal interaction molecule 1 (STIM1), which acts as an ER retention factor to suppress the activity of STING (56). Viral infection begins with the attachment of the virus to the surface of host cells mediated by viral entry proteins and host surface receptors (e.g. proteins, carbohydrates or lipids). Once attached, viruses can enter cells and release genetic material by direct fusion of the viral envelope with the membrane of host cells or endocytosis (57). Also, bacteria and parasites possess various exquisite tools for cell adhesion and host cell invasion (58, 59). Upon binding with dsDNA from pathogens or damaged cells, cytoplasmic cGAS undergoes a conformational change and forms a sandwiched-like structure with dsDNA (28, 60), which induces liquid-liquid phase separation and the formation of liquid-like droplets that function as micro-reactors to enhance cGAMP production by concentrating enzymes and reactants, which promotes the conversion of ATP and GTP to cGAMP (21, 43, 44). The binding of cGAMP to STING leads to a conformational change of the ligand-binding domain of STING, triggering a release of the C-terminal domain of STING, and subsequently, a disulfide-stabilized polymer is formed for interacting with TBK1 and IRF3 (20, 54, 61). The cGAMP-mediated disruption of the interaction between STING and ER-resident protein STIM1 initiates the intracellular trafficking of STING from ER to the Golgi apparatus through the coat protein complex II (COP-II)-vesicles and ER-Golgi intermediate compartment (ERGIC) (31). Meanwhile, many positive regulators of STING trafficking from the ER to Golgi are involved in this process, which have been extensively reviewed by Taguchi et al. (62). For example, STING ER exit protein (STEEP) interacts with STING and promotes trafficking from the ER to Golgi through promoting the accumulation of phosphatidylinositol-3-phosphate (PI3P) and ER membrane curvature (63). The palmitoylation of STING at cysteines 88 and 91 is essential for the location of STING in the Golgi and the initiation of downstream signal cascades (54, 64). In addition to recognizing cGAMP produced by cGAS, STING also binds dsDNA and c-di-GMP directly (61, 65), while the direct binding of c-di-AMP to STING remains unclear so far. Then STING oligomers recruit TBK1 via its highly conserved PLPLRT/SD motif in the C-terminal tail and induce clustering and trans-autophosphorylation of TBK1 (66, 67). Activated TBK1 phosphorylates STING at the C-terminal pLxIS motif (p, hydrophilic residue; x, any residue; S, phosphorylation site), which allows STING to recruit IRF3 and subsequently binds to the conserved, positively charged surfaces of IRF3, thereby promoting its phosphorylation and activation by TBK1. The phosphorylated IRF3 dissociates from STING, undergoes dimerization, and finally translocates to the nucleus (67, 68). In the nucleus, the phosphorylated IRF3 homodimers bind to DNA at the interferon-stimulated response elements (ISREs) and orchestrate the expression of type I IFN (69). Eventually, when type I IFN are secreted outside the cells, they can be recognized by their receptors. This activates Janus kinases (JAKs) and tyrosine kinase 2 (TYK2), followed by the phosphorylation of signal transducer and activator of transcription protein 1 (STAT1), STAT2 and IRF9, which drives the production of interferon-stimulated genes (ISGs) to establish antipathogenic immunity functions via JAK-STAT signaling pathways (70). In addition, STING may also activate STAT6 independent of JAKs through phosphorylating its Ser407 via TBK1 (71). Apart from eliciting type I IFN response, STING activation can also trigger NF-κB signaling (72). Recent evidence indicated that TBK1/IKKϵ redundantly induces phosphorylation of STING and promotes the recruitment and phosphorylation of transforming growth factor-β-activated kinase 1 (TAK1) and inhibitor of NF-κB kinase subunit beta (IKKβ). This leads to the phosphorylation and degradation of IκB inhibitory proteins, which further promotes the translocation of NF-κB (p65 and p50) into the nucleus and triggers NF-κB signaling activation (73, 74). Interestingly, compared with human and mouse STING, zebrafish STING contains a unique C-terminal tail module that is responsible for the enhanced NF-κB activation. Further investigation showed that zebrafish STING sequence contains the closest homology with TRAF6 recruitment motifs, which is indispensable for zebrafish STING-induced NF-κB signaling activation, but not for human and mouse, indicating there might be some species-specific differences in cGAS-STING-induced NF-κB activation (73, 75). Moreover, the activation of the cGAS-STING pathway facilitates non-canonical NF-κB signaling by recruiting mitogen-activated protein kinase kinase kinase 14 (MAP3K14/NIK) and NIK-STING interaction might act to synergize STING-dependent type I IFN response (76–78). Those activated molecules induce a set of cytokines, chemokines and immunomodulatory genes that are responsible for inflammatory or immune responses, ultimately establishing an antipathogen state (79). For instance, after IL-6 are secreted outside, they bind to their cytokine receptors (IL-6R/GP130) on the membrane of cells, leading to the homodimer formation of STAT3, which in turn triggers target gene expression to influence cell growth and survival (80, 81). As canonical NF-κB activation has been reported to enhance STING signaling through blocking STING degradation by regulating microtubule-mediated STING trafficking from the Golgi apparatus to lysosomes (82), and activation of non-canonical NF-κB pathway resulted in decreased type I IFN expression (83), suggesting canonical and non-canonical NF-κB pathway might play a different role in modulating STING signaling. Interestingly, cGAMP is synthesized in the cytosol and cannot cross the cell membrane due to its two negative charges. However, it was recently shown that cGAMP can be exported to the extracellular space through gap junctions (84), exosomes (85), LRRC8 (86), multidrug-resistance-associated protein 1 (MRP1) (87) and recognized by pmSTING to signal other types of cells in a paracrine manner. With the help of cGAMP importers SLC19A1 and SLC46A2, it is likely that extracellular cGAMP can also be detected by ER-located STING (88, 89). Extracellular cGAMP can also be degraded by the membrane hydrolase ectonucleotide pyrophosphatase phosphodiesterase 1 (ENPP1) that has a catalytically active domain toward extracellular through hydrolyzing the 2’,5’-phosphodiester linkage and 3’,5’-phosphodiester linkage of cGAMP (90, 91). In ENPP1 knockout 293T cells, a significant increase of extracellular cGAMP molecules was detected (92), demonstrating the potential of ENPP1 in regulating the cGAS-STING signaling. After fulfilling its role, post-Golgi STING vesicles are either fused or encapsulated by lysosomes and gradually eliminated (93).
The cGAS-STING pathway in intestinal homeostasis
The intestinal mucosa is exposed to commensal microbiota and potential pathogenic microbiota, their metabolites and a multitude of ingested luminal factors. The intestinal epithelial barrier is the first line defense against gut microbes, which is composed of stem cells, differentiated epithelial cells and their secreted products (e.g., mucins produced by the goblet cells and antimicrobial peptides produced by Paneth cells), and immune cells (e.g., intraepithelial lymphocytes (IELs)) (94). Additionally, cytokines and chemokines released by immune cells in the lamina propria (LP), such as mononuclear phagocytes and antigen-presenting cells (e.g., macrophages and DCs), as well as antibodies (e.g., Immunoglobulin A (IgA)) produced by plasma cells, protect the host against infections and harmful antigens (95). The antipathogenic response by epithelial and immune cells is mediated by the recognition PAMPs or DAMPs (e.g., lipopolysaccharides (LPS) and dsDNA) through diverse PRRs (96). From the various PRRs, increasing evidence shows that STING plays a central role in modulating and maintaining intestinal homeostasis (97–100). Whole-body STING knockout (Sting-/-) mice displayed alterations in the mucosa and intestinal microbiota when compared to WT mice, while no obvious weight loss and length of the colon were observed (left panel in Figure 2). Elongated villi in the small intestine and shorter crypts in the colon were observed. In the colon, there were also decreases in induced IELs (expressing TCRαβ), goblet cells, mucin production (MUC-1 and MUC-2) and secreted IgA (sIgA). In the lamina propria, there were elevations in innate lymphoid cells (ILC) 1 and ILC3, as well as reductions in ILC2 and anti-inflammatory T regulatory (Treg) cells. IL17 secretion, which is commonly associated with an inflammatory response, was upregulated, and accompanied by a decrease in cytokines associated with tissue regeneration and anti-inflammatory function (101). With regard to microbiota alterations, a reduction in Bifidobacterium (Actinobacteria phylum) and Allobaculum (Firmicutes phylum) and an increase in Desulfovibrio genus (Proteobacteria phylum) were observed in Sting-/- mice, suggesting a shift to a pro-inflammatory microbiota profile (101). Furthermore, a decrease in serum and fecal IgA, short-chain fatty acid (SCFA)-producing bacteria and SCFA fermentation were observed in Sting-/- mice (102). STING also enhanced the production of the antimicrobial peptide Reg3γ in a type I IFN-dependent manner, which could limit damage to intestinal tissues by sustaining a protective shield against bacterial colonization and translocation (102). In addition to Sting-/- mice, also mice with constitutive-active STING have been generated and analyzed for intestinal functions. STING gain-of-function Sting+/N153s (N153S) mice presented weight loss and reduction of colon length compared to WT mice, together with loss of intestinal barrier integrity as shown by higher intestinal permeability, lower level of ZO-1, loss of goblet cells along with their products (MUC2 and TFF3), as well as a decrease in induced IELs along with increased defensin and Reg3γ production (right panel in Figure 2). STING gain function caused a decrease in Treg cells and Th17 in the lamina propria, but an increase in other pro-inflammatory cells, such as neutrophils, macrophages and Th1 cells. Notably, N153S mice displayed excessive extracellular matrix (ECM) deposition in the colon (103). The changes in the number of stem cells, Paneth cells and enteroendocrine cells have not been described in either of the 2 mouse models, which can be interesting perspectives in exploring the function of STING in the epithelial cells. N153S mice also showed altered gut microbiota composition compared to WT mice, displaying an increase in the bacterial families Enterobacteriaceae, Helicobacteraceae, Lactobacillaceae, Peptostreptococcaceae and a decrease in Lachnospiraceae and Rikenellaceae (103). In addition, the cGAS-STING-type I IFN axis promoted intestinal regeneration after irradiation injury (104, 105). These studies indicate that intrinsic STING signaling is important to maintain mucosal homeostasis and adequate symbiosis with gut microbiota (Figure 3A).
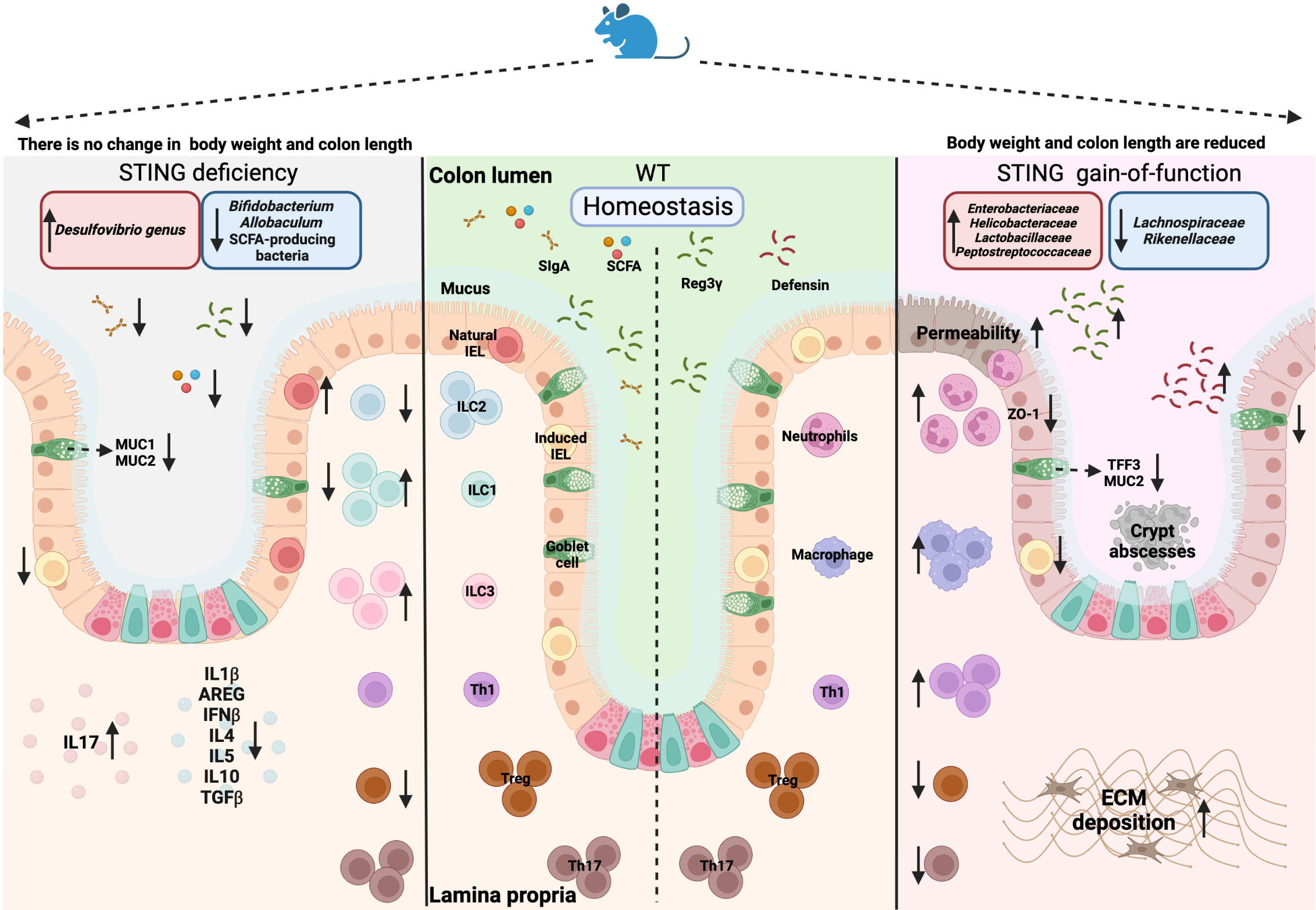
Figure 2 Overview of the STING deficiency (Sting-/-) and STING gain-of-function (Sting+/N153s) on mice models. Created with BioRender.com.
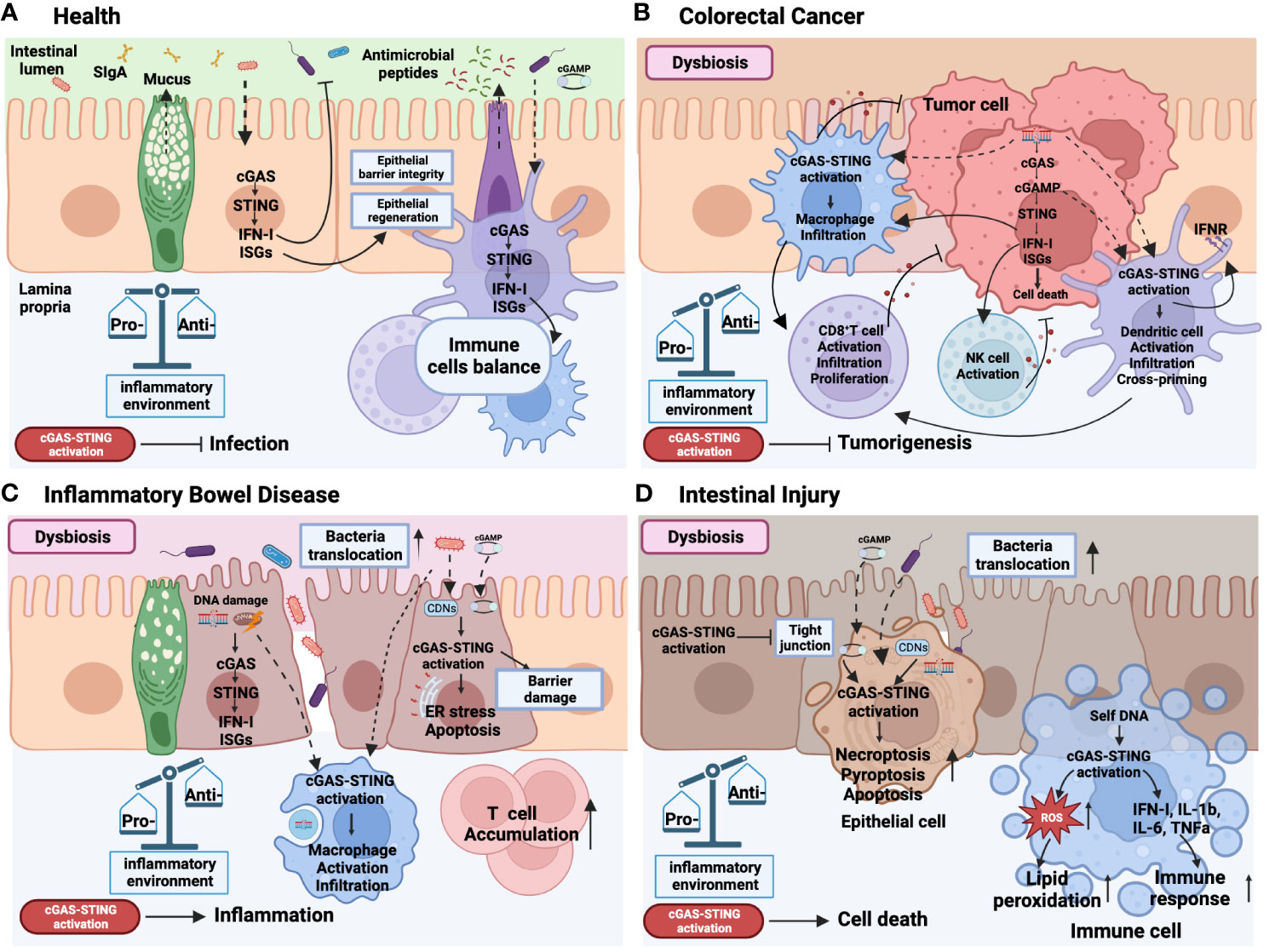
Figure 3 Role of cGAS-STING signaling activation in health and intestinal diseases. In the context of health (A), the intricate symbiotic interplay between the intestinal microbiota and the colonic mucosa relies on the intrinsic STING pathway present in both epithelial and immune cells. This dynamic interaction facilitates the defense against pathogenic infections by stimulating the secretion of type I interferons (IFN-I) and Interferon-stimulated gene (ISGs), reinforcing the integrity and regeneration of the epithelial barrier, promoting the production of antimicrobial peptides by Paneth cells, mucus synthesis by goblet cells, secretory IgA (SIgA) expression by plasma cells. This mutually beneficial relationship is crucial for maintaining immune cell homeostasis and establishing a harmonious and balanced gut environment. Colorectal cancer (B) is marked by an imbalanced state of the microbiota. Within this context, the release of DNA from dead tumor cells triggers the activation of the STING pathway in tumor cells and immune cells. This dual activation cascade promotes a pro-inflammatory immune response within the tumor microenvironment, effectively impeding tumorigenesis. Notably, STING activation facilitates the infiltration, activation, proliferation, and cross-priming of dendritic cells, macrophages, CD8+ T cells, and NK cells. Inflammatory bowel disease (IBD) (C) also manifests dysbiosis and the translocation of pathogenic microorganisms into the lamina propria (LP) due to a compromised epithelial barrier. Bacterial DNA or cyclic dinucleotides (CDNs) and self-DNA derived from damaged cells, including double-stranded DNA (dsDNA) and mitochondrial DNA (mtDNA), induce the activation of STING in both epithelial and immune cells, thereby exacerbating intestinal mucosal inflammation. Furthermore, STING activation and its downstream effectors elicit endoplasmic reticulum (ER) stress, and apoptosis, contributing to epithelial barrier damage. Consequently, macrophage infiltration and activation, along with T cell accumulation in the LP, are promoted by STING activation, perpetuating the imbalance of pro-inflammatory cells and cytokines in IBD. Similar to IBD, intestinal injury (D) is characterized by dysbiosis, epithelial damage, and the translocation of bacteria. Bacterial DNA or CDNs and self-DNA from damaged or dead cells play a significant role in activating the STING pathway, leading to cellular death, inflammation, and intestinal barrier disruption. Furthermore, activation of the STING pathway in immune cells elevates reactive oxygen species (ROS) levels, subsequently causing lipid peroxidation and triggering the production of IFN-I and pro-inflammatory cytokines. This collective response perpetuates a state of sustained inflammation within the intestinal environment. The solid line represents effects, the dotted line represents translocation. Created with BioRender.com.
The cGAS-STING pathway in colorectal cancer
Colorectal cancer (CRC) is the third most common cancer worldwide associated with high mortality. Although treatment of CRC has slowly but steadily improved in many countries, its 5-year survival rate is still less than 50% in low-income countries. The biological heterogeneity and complex tumor microenvironment of CRC impede the development of efficient and personalized therapeutic strategies (106). Recently, several studies have shown that the cGAS-STING signaling is critical to protect against tumorigenesis (Figure 3B) and preserve the efficacy of anti-tumor therapies such as immune checkpoint therapy, chemotherapy and radiotherapy (Table 1). Many colon adenocarcinoma cell lines show poor response to dsDNA-induced activation of the STING pathway and type I IFN production. Epigenetic silencing of STING and cGAS inhibited tumor cell death and anti-tumor response (126). STING knockout CT26 colon carcinoma cells significantly promoted tumor growth in immune-competent C57BL/6 mice and BALB/c mice compared to parent CT26 cells (122). CRC patients with high STING expression exhibited increased intratumoral CD8+ T cell infiltration and less frequent lymphovascular invasion in the early stage of cancer and had longer overall and recurrence-free survival compared to CRC patients with low STING expression (124), which has also been confirmed by Yang et al. (116). These findings suggest that the level of STING can be used as a clinical predictor for CRC progression. Further research indicated that the growth of MC38 tumors can be effectively suppressed by intratumoral treatment with the STING agonist 3’3’-cGAMP leading to enhanced intratumoral infiltration and activation of CD8+ T cells (124), suggesting that the ability of STING to suppress tumor growth may rely on regulating immune cells and the activation of STING could be a therapeutic strategy for CRC treatment. The anti-tumor role of STING has also been observed in the mouse model of azoxymethane/dextran sodium sulfate (AOM/DSS)-induced colitis-associated cancer (CAC) (108–110). Stinggt is an I199N missense mutant allele leading to inactive STING. Homozygous Stinggt/gt mice do not produce IFN-β in response to cyclic dinucleotides or Listeria monocytogenes infection (127). Stinggt/gt mice housed in a specific pathogen-free (SPF) facility are highly susceptible to AOM/DSS-induced CAC. These mice demonstrated more severe colonic hyperplasia during the early stages of tumorigenesis and failed to restrict activation of the NF-kB- and STAT3-signaling pathways and expressed increased levels of the pro-inflammatory cytokines IL-6 and KC (a chemokine that is capable of recruiting neutrophils) (109). Exacerbating CAC has been also reported in AOM/DSS-induced Sting-/- mice as well (108). The protective effect of STING was also observed in AOM/DSS-induced Sting-/- mice housed in the environment containing Helicobacter spp. (Helico) that is known to influence the outcome of colitis (110). Regarding that AOM/DSS-induced Sting-/- mice housed in Helico-SPF barrier failed to produce IL-10 compared with WT mice, STING may also interact with opportunistic pathogens to produce anti-inflammatory cytokines for maintaining gut immune homeostasis (110). However, the role of cGAS in tumorigenesis is controversial. AOM/DSS-treated Cgas-/- mice showed no obvious increase in polyp formation compared to WT mice regardless of the Helico status in their study (110), whereas in another study, Cgas-/- mice displayed higher mortality upon AOM/DSS treatment compared with Stinggt/gt and interferon alpha and beta receptor (Ifnar)-/- mice (107). Since the above studies used different drug delivery strategies and different mice lineages, the discrepancies between these studies are not clarified yet. Thus, more studies are needed to investigate the difference and association of cGAS, STING and IFNAR in the progression of CRC.
In addition, the efficacy of anti-tumor therapies is dependent on the cGAS-STING signaling. Evidence has shown that higher STING expression in human CRC specimens was associated with better survival and responsiveness to chemotherapy 5‐Fluorouracil (5‐FU) (112), a widely used chemotherapeutic drug, whose responsiveness requires a fully functional cancer cell-intrinsic cGAS-STING pathway, as well as IFN‐sensing by bone‐marrow‐derived cells (BMDCs) (112). Also, cGAS-STING-mediated type I IFN production in the host immune system was required for radiotherapy-induced adaptive immune responses (128) and anti-signal regulatory protein α (SIRPα)/anti-programmed cell death protein 1 (PD-1)/radiotherapy treatment (118). Additionally, it suggested that the capability of Midostaurin (PKC412) to restrict colorectal carcinoma cell growth is associated with the activation of the cGAS-STING signaling (113). Src homology-2-containing protein tyrosine phosphatase 2 (SHP2), encoded by the PTPN11 gene, plays a critical role in the tumor microenvironment by regulating T cell activation, as well as polarization, phagocytosis, anti-inflammatory cytokine expression of macrophages (129). CRC patients with microsatellite stable phenotypes displayed increased CD68+ macrophage infiltration and enhanced SHP2 phosphorylation than microsatellite instability-high phenotypes, suggesting inhibition of SHP2 could be a promising strategy for CRC therapy. As an SHP2 allosteric inhibitor, SHP099 was shown to arrest the malignant evolution of tumor cells by activating STING-TBK1-IRF3-mediated type I IFN signaling in macrophages (120). In colon cancer cells, SHP2 was indicated to dephosphorylate poly (ADP-ribose) polymerase 1 (PARP-1) and thus to suppress DNA repair while promoting the cGAS-STING activation to elevate the sensitization of chemotherapy in colon cancer cells (111, 130). Although different mechanisms of SHP2-mediated STING activation in cancer cells and immune cells were suggested, the cGAS-STING signaling appears to be critically involved in anti-cancer immunotherapy.
As DNA and CDNs from bacteria are triggers of the cGAS-STING pathway, the regulatory roles of gut microbiota on the effects of STING signaling during the development of CRC also gained attention. AOM/DSS-treated Sting-/- mice housed under Helico+ barrier facility showed significant bacterial differences in Turicibacter and Odoribacter species compared to that of healthy WT mice and antibiotic treatment eliminated the observed colitis and CAC in this model compared to untreated Sting-/- mice (110). On the other hand, no obvious changes in Prevatollaceae, Bacteroides, mouse intestinal Bacteroides (MIB), segmented filamentous bacteria (SFB) and TM7 were observed between Stinggt/gt and WT mice housed in an SPF facility (109). Therefore, the role of STING in CAC development might be dependent on the presence of specific commensal bacteria and opportunistic pathogens. Also, the regulatory role of intestinal microbiota that influences the efficacy of immunotherapies in a STING-dependent manner has been observed. Cluster of differentiation 47 (CD47), a ligand of SIRPα, is an immunoglobulin-like transmembrane protein that is elevated in many cancer cells (131–134), including colon cancer cells (135–137). The recognition of tumors presenting CD47 macrophages and dendritic cells is impaired, therefore, anti-CD47 immunotherapy is a potential therapeutic target of cancer treatment (138, 139). Administration of a Bifidobacterium cocktail (B. bifidum, B. longum, B. lactis, and B. breve) or STING agonist DMXAA synergized the anti-tumor efficacy of CD47 blockade in mice non-responders in a STING-dependent way. The potential of Bifidobacterium to enhance anti-tumor efficacy might be dependent on type I IFN signaling in DC and CD8+ T cell activation (114). In addition to anti-CD47 immunotherapy, bacteria can also increase the efficacy of anti-PD‐1/PD‐L1 therapy. Oral administration of probiotic Lactobacillus rhamnosus GG (LGG) augmented the anti-tumor responses to anti-PD-1 in colon cancer model in a cGAS-STING-dependent way (115). Moreover, tumor sensitivity to PD-1/PD-L1 checkpoint blockade can be enhanced by F. nucleatum supplementation, which induced PD-L1 expression by activating STING signaling and increasing the abundance of IFN-γ+CD8+ tumor-infiltrating lymphocytes in the tumor tissues of BALB/c mice during treatment with PD-L1 blockade (119). Since the application of PD-L1 blockade in CRC was not effective in clinical trials (140, 141), exposure to specific microorganisms might be a promising strategy to improve the efficacy of cancer immunotherapies. It is worth noting that although supplementation of certain bacteria can synergize immunotherapies, bacteria such as F. nucleatum, have been reported to be positively associated with the recurrence of CRC after chemotherapy by promoting chemoresistance, as well as increasing proliferation and invasive activities of CRC cell lines via activation of TLR4 and MYD88 signaling (142, 143). This suggests that STING signaling could have opposing roles in cancer immunity compared to other PRRs involved in microbiota recognition, and thus thoughtful investigation is required when using bacteria as adjuncts to activate STING-dependent immune response in CRC treatment. Therefore, more investigations are needed on how specific bacteria or their products can stimulate the cGAS-STING pathway and enhance the anti-tumor immune response, which may promote the development of novel therapeutic strategies or interventions for CRC treatment. In addition, applying STING agonists alone or combined with traditional CRC immunotherapies is also a promising strategy to suppress tumorigenesis. Treatment with the STING agonist DMXAA in a murine CAC model reduced structural heteromorphism, the number of adenocarcinomas and increased immune cell infiltration. Increased Syk-mediated pyroptosis in tumor cells was found in an ex vivo study (108). After intertumoral injection of cGAMP, a macrophage phenotype-like cell subset that produced TNF-α, displayed phagocytic activity and expressed high levels of T-cell recruiting chemokines, were transiently accumulated in the tumor microenvironment of CRC mice model (123). Also, the STING agonist diABZI combined with the indoleamine 2,3 dioxygenase (IDO) inhibitor 1-MT significantly inhibited tumor growth and promoted the recruitment and activation of IFN-γ+CD8+ T cells and DCs, and significantly decreased myeloid-derived suppressor cells that exerted immunosuppressive function (125). STING agonist (cGAMP or RR-CDA) treatment combined with VEGFR2 blockade (DC101) can induce tumor regression in the CT26 colon cancer mouse model, which was largely dependent on IFNAR and CD8+ T cells (116). Intraperitoneal application of the STING agonist RR-CDA (also known as MIW815 or ADU-S100) alone or combined with an anti-PD-1 antibody enhanced antitumor immunity and normalized aberrant angiogenesis in peritoneal carcinomatosis of colon cancer (144).
Thus, applying STING agonists or bacteria that can enhance the cGAS-STING signaling in combination with traditional anti-tumoral therapies could be a strategy for CRC treatment in the future. Recently, phase I clinical trials with the STING agonists MIW815 (ADU-S100) and SYNB1891 (an engineered Escherichia coli Nissle strain 1917 expressing STING agonist CDNs under hypoxia) as monotherapy or in combination with immune checkpoint inhibitors (anti-PD1 or anti-PD-L1) have been conducted in patients with solid tumors (including CRC), lymphomas or advanced/metastatic cancers (145–147). These clinical trials have shown immune response activation and good tolerance in patients, but the anti-tumor effect was limited. Moreover, there are registered clinical studies in phase I and phase II using STING agonists, such as SNX281, TAK-500 and IMSA101 in patients with solid tumors or lymphomas (ClinicalTrials.gov identifiers: NCT04609579; NCT05070247; NCT05846659). Other clinical trials supporting the use of STING agonists in cancer immunotherapy have recently been reviewed and discussed by Hines et al. (148). These investigations will contribute to a better understanding of the cGAS-STING pathway activation as a therapeutic target in cancer. However, advances in basic and clinical research on optimal therapeutic dose, delivery system, and possibly drug combinations are needed to improve the anti-tumor effects of STING agonists to achieve an effective and safe therapy. Further understanding the interaction of cGAS-STING signaling, gut bacteria and the tumor microenvironment is critical to evaluate the possibility of targeting the cGAS-STING pathway in clinical implications.
The cGAS-STING signaling pathway in intestinal inflammatory bowel diseases
Inflammatory bowel diseases (IBD), including ulcerative colitis (UC) and Crohn’s disease (CD), are long-lasting, relapsing-remitting, and chronic inflammatory diseases of the intestinal tract. The complex multifactorial etiology of IBD poses a great challenge to prevent disease progression and develop effective therapeutic strategies (149, 150). Multiple studies have suggested the pro-inflammatory role of cell-free DNA in IBD. Plasma mtDNA levels were increased in the DSS-induced colitis mouse model as well as in both UC and CD patients, in which mtDNA levels were positively correlated with disease severity (151–153). Increased DNA damage and accumulation of cytosolic DNA were also observed in the colon of colitis mice model and IBD patients (154, 155). Microbiota dysbiosis that influences the level of CDNs has been shown to be involved in the pathogenesis of IBD as well (156–158). Therefore, due to its capacity of sensing dsDNA and CDNs from bacteria, increasing research focus on exploring the role of the cGAS-STING pathway in IBD (Table 2). Several studies reported that the cGAS-STING pathway is activated in UC patients with active disease. Protein levels of cGAS, p-STING, p-TBK1 and p-IRF3 were increased in the colon of UC patients with active disease compared to patients in remission and healthy controls (159). In addition, the expression of STING was increased in inflamed regions of the colon in UC patients compared with healthy control and noninflamed flanking areas (103). Although STING, TBK1, and IRF3 mRNA levels were unchanged in a study by Chen et al. (159), Flood et al. reported a significant increase of STING, TBK1, IRF3, IFNB1 gene levels in active UC, which supports that the STING-TBK1-IRF3 axis was activated in UC (165). Similarly, a significant increase of p-STING, p-IRF3 and p-p65 protein levels were detected in colon tissues of active CD patients (153). Autophagy related 16 like 1 (ATG16L1) is a genetic risk factor of CD (166). Mice with a deletion of Atg16l1 in the IECs develop spontaneous ileitis phenocopying ileal CD (167). An in vitro study showed that cell death was significantly enhanced in Atg16l1ΔIEC organoids treated with IFN-β or IL-22 that activate STING signaling. Blocking the IFN receptor led to amelioration of the disease severity in mice (168). In addition, the stabilization of STING protein via bacteria-induced ubiquitination in mice challenged with colitis inducer Salmonella typhimurium, Citrobacter rodentium further exacerbated colitis in mice (103).
In contrast to the protective role of the cGAS-STING pathway in CRC cancer, the cGAS-STING pathway appears to promote inflammation and intestinal barrier dysfunction in IBD (Figure 3C). For instance, extracellular vesicles (EVs) carrying dsDNA from the plasma of active CD patients and damaged CT26 cells triggered STING activation in bone marrow-derived macrophages (BMDMs) in vitro and promoted the expression of pro-inflammatory cytokines, while this was not observed in Sting-deficient macrophages. And the level of intestinal apoptosis and pro-inflammatory cytokines were reduced in Sting-deficient mice compared with DSS-treated wild-type mice (153). The detrimental effects of overactivated STING signaling in IBD have also been investigated in the N153S mice model in which STING is constitutively activated. N153S mice spontaneously develop severe colitis and intestinal fibrosis with barrier dysfunction, which could be associated with the predominant presence of active STING in myeloid cells (103). It is worth noting that STING gain-of-function mutation in human causes STING-associated vasculopathy with onset in infancy (SAVI), a rare disease associated with systemic inflammation, resulting in peripheral vasculopathy, interstitial lung disease, polyarthritis and respiratory failure (169, 170). Unlike its mice model, the gastrointestinal involvement in SAVI has not been addressed. Although SAVI has distinct underlying mechanisms and clinical presentations compared with UC, there is a connection between them in terms of the potential use of JAK inhibitors for treatment (171). In apparent agreement, Sting-/- mice appear resistant to DSS treatment, exhibiting less weight loss, disease activity, and longer colon length than DSS-treated WT controls (108). Treatment with the STING agonist DMXAA significantly decreased expression of the tight junction proteins (TJPs) ZO-1 and occludin in human colorectal adenocarcinoma cell HT-29 and in the DSS-induced colitis mouse model when compared to DSS treatment alone (159). Il-10 deficiency is a genetic mouse model that causes chronic enterocolitis (172), and Sting deficiency in Il-10-/- mice ameliorated the severity of inflammation via reducing pro-inflammatory cytokines expression (110). Atrial Natriuretic Peptide (ANP) treatment displayed its ability to restrict ER stress-induced autophagy and restore TJPs level via restricting the cGAS-STING pathway in colon tissues of DSS-induced mouse model (159). Unlike Sting deficiency, the absence of Cgas worsened colitis and decreased autophagy in the DSS-induced mouse model independent of microbiota (173), although cGAS inhibitor illustrated benefit in restricting inflammation in DSS-induced colitis mouse model (162).
Overall, the above results suggest that gut microbiota is involved in the modulation of the cGAS-STING pathway in the context of inflammation. Lowering the production of cell-free DNA and CDNs, restricting the cGAS-STING as well as regulating intestinal flora might be potentially helpful in modulating inflammation and barrier dysfunction in IBD. Still, while inhibiting the overactivation of STING signaling might suppress intestinal inflammatory immune response and IBD progression, total blockade of the cGAS-STING activity may also induce intestinal pathologies, as suggested by studies with Sting-/- mice.
The cGAS-STING signaling in intestinal ischemia-reperfusion and sepsis
Intestinal ischemia/reperfusion (I/R) injury is caused by an insufficient blood supply (ischemia) followed by a restoration of blood flow (reperfusion) in the intestinal tissue, as during septic shock, severe trauma and gastrointestinal surgeries like intestinal resection or small intestine transplantation. Intestinal I/R injury leads to an increase in oxidative stress, cell death, and epithelial barrier breakdown with the consequent release of intracellular components, known as DAMPs. Bacteria translocation from the lumen to the lamina propria can result in intestinal complications, affecting distant organs (e.g. acute liver injury) or leading to a systemic inflammatory response syndrome (SIRS) or multiple organ dysfunction syndrome (174, 175). As a DAMP and also ligand of STING signaling, mtDNA released from damaged and stressed mitochondria were increased in patients after gastrointestinal surgery with SIRS (176), in a mouse and cell model of intestinal I/R (177), and in cecal ligation perforation (CLP)-induced sepsis in mice (Table 3) (178). Furthermore, the expression of STING and its downstream effectors p-IRF3, NF-κB, inflammatory cytokines (TNF-α, IL-1β, IL-6), apoptosis and epithelial injury were increased in intestinal tissues from patients with abdominal sepsis (178). While Sting deficiency or DNAse I treatment mitigated CLP-induced inflammation, IEC apoptosis and intestinal barrier damage in mice (178). Further research indicated that mtDNA can promote necroptosis signaling in IECs and therefore exacerbate intestinal injury in WT mice. Compared with WT mice, Sting-/- mice exhibited improved intestinal morphology and reduced intestinal inflammation and necroptosis after the intraperitoneal injection of mtDNA (181). Moreover, the absence of Sting ameliorated damage to liver and lung as well as lipid peroxidation in intestinal I/R mice model (179) (Figure 3D). The STING antagonist H151 was also shown to ameliorate lung and intestinal injury, as well as systemic inflammation after intestinal I/R (182). However, it remains unclear which types of cells are responsible for the therapeutic effects of H151. These results suggest that the combination of oxidative stress inhibitors (antioxidants), STING antagonists, and the elimination of mtDNA could be a potential therapeutic strategy for restoring intestinal barrier disruption and therefore to improve intestinal I/R injury.
Taken together, the involvement of the STING pathway in intestinal damage reveals a dual function depending on the causes underlying the intestinal disturbance. On the one hand, the STING pathway triggered by released mtDNA following intestinal I/R injury causes a local and/or systemic inflammatory response. On the other hand, as we mentioned before, the immune response generated by the STING pathway following acute damage caused by irradiation led to the resolution of inflammation and epithelial regeneration (104). Further investigation into the mechanisms under different types of acute or chronic intestinal injury will clarify when interventions with DAMPs inhibitors, STING agonists, or antagonists are most effective.
STING in intestinal parasite and viral infections
Intestinal parasite infections have a high morbidity and mortality rate in endemic countries and are the most common and prevalent infections worldwide (183, 184). Parasite infections can cause intestinal symptoms, such as diarrhea, abdominal pain, inflammation and bleeding, which vary depending on the specific parasite involved and the severity of the infection. Many reports have shown that the cGAS-STING signaling can be triggered by parasites including Plasmodium (185), Toxoplasma gondii (186), Leishmania (187), Trypanosoma (188), Schistosoma (189), while the role of the cGAS-STING pathway in parasite infections remains controversial. Wang et al. found that cGAS was required for the activation of the immune signaling defense against Toxoplasma gondii in a mouse model (186). Also, STING agonist c-di-AMP could protect against Trypanosoma cruzi infection by promoting Th1/2/17 cells and CD8+ T cells responses (188). However, although S. japonicum and S. mansoni can activate cGAS-STING-dependent type I IFN response, and surprisingly, Cgas-/- mice and Sting-/- mice were more resistant to S. japonicum and S. mansoni infection (189, 190). The resistance of Cgas or Sting deficiency to parasite infection has also been observed in Malaria infections caused by Plasmodium. Plasmodium yoelii and Plasmodium falciparum malaria infection can cause intestinal pathological changes, such as intestinal shortening, intestinal barrier damage, increased mucus thickness and gut dysbiosis (191, 192). Mice deficient in Cgas, Sting, or Ifnar were resistant to Plasmodium yoelii infection (193, 194). As shown by Du et al. (195), the immune response in Cgas or Sting-deficient mice resistant to lethal malaria strains Plasmodium yoelii N67C and YM infection was characterized by an elevation of type I IFN in the early stages of infection and a reduction of IL-6 in the late stages. In the context of cGAS-STING activation, late IL-6 production was promoted in macrophages via MyD88-p38 signaling, resulting in the expansion of proinflammatory monocytes (CD11b+Ly6Chi) and the suppression of T cell proliferation, resulting in loss of the immune defense against malaria. Therefore, more studies are required to illustrate the role of the cGAS-STING pathway in intestinal mucosa in the context of specific parasite infections for developing targeted therapeutic interventions.
Rotavirus, norovirus, adenovirus, and astroviruses are the most common causes of acute viral gastroenteritis worldwide, presenting symptoms such as vomiting, diarrhea, nausea and abdominal pain (196, 197), where the STING pathway protects against viral infections (27, 198–200). In addition, although Human Herpesviruses (HHV) were rarely found in the gastrointestinal tract in adults (201, 202), it is positively associated with morbidity and mortality in patients with IBD, especially in immunocompromised individuals (203). Moreover, persistent infection of the intestinal epithelial cells and systemic dysfunction was observed in murine gammaherpesvirus-68 (γHV-68)-exposed mice, a model to understand the pathogenicity of HHV-8 (204). It was reported that HHV such as Herpes simplex virus-1 (HSV-1) can infect enteric neurons and recruit activated CD3+CD8+ lymphocytes, resulting in gastrointestinal neuromuscular dysfunction in mice model (205). Sting-/- and Cgas-/- mice appeared highly susceptible to HSV-1 infection. Also, the STING pathway seemed to enhance the adjuvant activity of DNA-vaccine-induced T cell activation and antibody production in response to antigen (49, 206). The fact that Hepatitis B virus (HBV) and Hepatitis C virus (HCV) led to the compositional and functional alterations in the gut microbiota, which further impairs the intestinal barrier and nutrition absorption, has been considered to promote the progression of hepatitis (207, 208). Although it has been shown that STING activation-mediated autophagic flux inhibited inflammasome activation in macrophages, ameliorated liver injury and fibrosis in mouse model induced by HBV (209), it remains to be explored that whether STING pathway-mediated attenuation of hepatitis is associated with restoration of gut dysbiosis. Furthermore, it is well known that influenza virus causes infectious respiratory disease with common symptoms such as cough, fever, headache, while it also impaired gut barrier and immunity, leading to gastroenteritis-like symptoms (210, 211). The STING-dependent antiviral response is essential for limiting virus propagation (212, 213). The STING pathway also contributed to IFN response to the severe acute respiratory syndrome coronavirus 2 (SARS-CoV-2/COVID-19) (214, 215), causing intestinal ischemia and thrombosis (216, 217), mesenteric vascular endothelial cells damages (218) and increased risk of post-acute gastrointestinal pathologies (219). These data illustrate that the STING pathway is necessary for host defense against viral infections and understanding how STING signaling responds to viral infection might be important in the development of anti-viral therapies.
It is worth noting that viruses have developed multiple mechanisms to fight the host IFN responses that are controlled by STING. The HSV-1 ICP27 protein interacted with STING and TBK1 to inhibit the expression of type I IFN in human macrophages (220). HSV-1 can also evade the antiviral immune response by inducing miR-24 that binds to the 3’untranslated region of the STING mRNA and inhibit its translation (221). Human cytomegalovirus (HCMV), another virus that belongs to Herpesviridae, whose tegument protein pUL83 (also known as pp65) and UL82 (also known as pp71) can suppress the immune response by interacting with the IFI16 pyrin domain (222) and impair the trafficking of STING from the ER to perinuclear microsomes as well as the recruitment of TBK1 and IRF3 to the STING complex (223), thereby promoting HCMV replication and immune evasion. Additionally, the NS4B protein of HCV can disrupt the interactions between STING and TBK1 and thereby block the host interferon signaling responses (224). Influenza virus is able to increase cytosolic levels of mtDNA and cGAMP, leading to stimulation of cGAS-STING-dependent IFN-β expression. The NS1 protein of influenza virus was associated with mtDNA through its RNA-binding domain to evade the STING-dependent antiviral immunity (213). Therefore, targeting viral proteins involved in virus infection while combining with enhancing STING signaling might be a more efficient antiviral strategy. Controversially, a recent study showed that STING can facilitate nuclear import of HCMV and HSV-1 dsDNA during infection, rendering originally HCMV-insusceptible cells susceptible to the virus. The reduction of HSV-1 gene expression and HSV-1 viral DNA in the nuclear fraction were confirmed in STING-/- HepG2 cells as well. STING deficiency negatively regulated the establishment of HCMV latency and reactivation in monocytes, the latent reservoirs of HCMV (225), suggesting that STING might have a proviral effect in DNA virus infection and more investigations in different types of cells are required. Overall, the STING pathway is essential in DNA and RNA virus defense and could be a target for antiviral therapies. Although virus infections frequently cause intestinal manifestation, the cellular mechanism of the cGAS-STING pathway in intestinal mucosa during virus infection needs more research.
Conclusion and future perspectives
Ever since the initial discoveries that the cytosolic DNA sensor cGAS produces cGAMP to activate STING (20, 226), the cGAS-STING pathway has gained increased attention due to its critical role in regulating immune responses and has emerged as a promising therapeutic target in intestinal diseases. Detailed analysis of this pathway led us to better understand the host immune system in response to genetic susceptibility and environmental complexity. In this review, we focused on the role of the cGAS-STING pathway in intestinal homeostasis and diseases, which included their role in normal gut physiology, CRC, IBD, intestinal IR injury and intestinal infections caused by viruses, bacteria and parasites. Data so far show that the cGAS-STING pathway exhibits opposite effects in the progression of IBD and colon cancer. In IBD, activation of the cGAS-STING pathway promotes the production of pro-inflammatory cytokines and contributes to inflammation in the gut, while in colon cancer, its role skews to recruit immune cells to the tumor microenvironment and suppress tumor growth. These distinct effects might involve many factors, such as genetics, microbiota, tumor microenvironment and immune context. Precisely modulating the cGAS-STING pathway in cancer therapeutic interventions could be crucial to limit its potential adverse effects. Similarly, restricting overactivated STING signaling seems to be a promising therapeutic target for IBD, but likely some basal level of STING activation needs to remain to maintain gut homeostasis. Despite the growing therapeutic interest in this signaling cascade, several limitations hinder a comprehensive understanding of this pathway. Firstly, the majority of studies have primarily focused on immune cells, such as macrophages, dendritic cells, NK cells and T cells, while the influence of the cGAS-STING pathway in other cells, including epithelial cells and stromal cells, remains relatively unexplored. Expanding investigations to non-immune cells in intestinal diseases might be helpful in understanding the intricate interaction and communication of cells and provide insight into developing cell-targeted therapies and interventions. Secondly, it is known that there are several DNA sensors that play crucial roles in detecting and responding to different types of DNA in the host immune system and they are also involved in the progress of diseases, such as autoimmune diseases and cancer (227). Analyzing the crosstalk between the cGAS-STING pathway and other DNA sensors might provide key insights into manipulating host immune responses. Also, a thorough investigation of the potential synergistic or antagonistic interplay between cGAS-STING and other PRRs could offer novel strategies for modulating intestinal disorders. Thirdly, we need better mechanistic understanding of the regulatory effects of post-translational modifications of STING. SUMOylation, ubiquitination, phosphorylation, and palmitoylation affect oligomerization and activation of STING (228–231), while dephosphorylation, deubiquitination, carbonylation, deSUMOylation may inhibit the dimerization, decrease the oligomerization or inhibit the residence of STING (230, 232–234). Deeper understanding of these post-translational modifications in intestinal diseases might provide novel perspectives in drug development.
Author contributions
YY contributed to the manuscript concept and structure. YY, LW, IP-G and DP-V each wrote and contributed to sections of literature search, manuscript drafts, figures drafts and review. KF and GD contributed to the correction and supervision of the manuscript. All authors contributed to the article and approved the submitted version.
Funding
This work was supported by the China Scholarship Council (No. 202006350025 and No. 202108310110) to YY and LW. IP-G was supported by CONAHCYT (Consejo Nacional de Humanidades, Ciencias y Tecnologías, CVU: 710722. Scholarship Number: 297024). YY, LW, IP-G and DP-V were all supported by UMCG and RUG.
Acknowledgments
Figures were created with BioRender.com.
Conflict of interest
The authors declare that the research was conducted in the absence of any commercial or financial relationships that could be construed as a potential conflict of interest.
Publisher’s note
All claims expressed in this article are solely those of the authors and do not necessarily represent those of their affiliated organizations, or those of the publisher, the editors and the reviewers. Any product that may be evaluated in this article, or claim that may be made by its manufacturer, is not guaranteed or endorsed by the publisher.
References
1. Turner JR. Intestinal mucosal barrier function in health and disease. Nat Rev Immunol (2009) 9(11):799–809. doi: 10.1038/nri2653
2. Okumura R, Takeda K. Maintenance of intestinal homeostasis by mucosal barriers. Inflamm Regener (2018) 38(1):5. doi: 10.1186/s41232-018-0063-z
3. Ramos GP, Papadakis KA. Mechanisms of disease: Inflammatory bowel diseases. Mayo Clin Proc (2019) 94(1):155–65. doi: 10.1016/j.mayocp.2018.09.013
4. Takeuchi O, Akira S. Pattern recognition receptors and inflammation. Cell (2010) 140(6):805–20. doi: 10.1016/j.cell.2010.01.022
5. Duan T, Du Y, Xing C, Wang HY, Wang RF. Toll-like receptor signaling and its role in cell-mediated immunity. Front Immunol (2022) 13:812774. doi: 10.3389/fimmu.2022.812774
6. Rehwinkel J, Gack MU. Rig-I-like receptors: Their regulation and roles in rna sensing. Nat Rev Immunol (2020) 20(9):537–51. doi: 10.1038/s41577-020-0288-3
7. Claes AK, Zhou JY, Philpott DJ. Nod-like receptors: Guardians of intestinal mucosal barriers. Physiol (Bethesda) (2015) 30(3):241–50. doi: 10.1152/physiol.00025.2014
8. Li M, Zhang R, Li J, Li J. The role of C-type lectin receptor signaling in the intestinal microbiota-inflammation-cancer axis. Front Immunol (2022) 13:894445. doi: 10.3389/fimmu.2022.894445
9. Hu S, Peng L, Kwak YT, Tekippe EM, Pasare C, Malter JS, et al. The DNA sensor aim2 maintains intestinal homeostasis via regulation of epithelial antimicrobial host defense. Cell Rep (2015) 13(9):1922–36. doi: 10.1016/j.celrep.2015.10.040
10. Zhang Z, Yuan B, Bao M, Lu N, Kim T, Liu YJ. The helicase ddx41 senses intracellular DNA mediated by the adaptor sting in dendritic cells. Nat Immunol (2011) 12(10):959–65. doi: 10.1038/ni.2091
11. Kondo T, Kobayashi J, Saitoh T, Maruyama K, Ishii KJ, Barber GN, et al. DNA damage sensor mre11 recognizes cytosolic double-stranded DNA and induces type I interferon by regulating sting trafficking. Proc Natl Acad Sci USA (2013) 110(8):2969–74. doi: 10.1073/pnas.1222694110
12. Takaoka A, Wang Z, Choi MK, Yanai H, Negishi H, Ban T, et al. Dai (Dlm-1/zbp1) is a cytosolic DNA sensor and an activator of innate immune response. Nature (2007) 448(7152):501–5. doi: 10.1038/nature06013
13. Almine JF, O'Hare CA, Dunphy G, Haga IR, Naik RJ, Atrih A, et al. Ifi16 and cgas cooperate in the activation of sting during DNA sensing in human keratinocytes. Nat Commun (2017) 8:14392. doi: 10.1038/ncomms14392
14. Zierhut C, Funabiki H. Regulation and consequences of cgas activation by self-DNA. Trends Cell Biol (2020) 30(8):594–605. doi: 10.1016/j.tcb.2020.05.006
15. Maelfait J, Liverpool L, Bridgeman A, Ragan KB, Upton JW, Rehwinkel J. Sensing of viral and endogenous rna by zbp1/dai induces necroptosis. EMBO J (2017) 36(17):2529–43. doi: 10.15252/embj.201796476
16. Roers A, Hiller B, Hornung V. Recognition of endogenous nucleic acids by the innate immune system. Immunity (2016) 44(4):739–54. doi: 10.1016/j.immuni.2016.04.002
17. de Oliveira Mann CC, Hornung V. Molecular mechanisms of nonself nucleic acid recognition by the innate immune system. Eur J Immunol (2021) 51(8):1897–910. doi: 10.1002/eji.202049116
18. González-Navajas JM, Lee J, David M, Raz E. Immunomodulatory functions of type I interferons. Nat Rev Immunol (2012) 12(2):125–35. doi: 10.1038/nri3133
19. Di Franco S, Turdo A, Todaro M, Stassi G. Role of type I and ii interferons in colorectal cancer and melanoma. Front Immunol (2017) 8:878. doi: 10.3389/fimmu.2017.00878
20. Ishikawa H, Barber GN. Sting is an endoplasmic reticulum adaptor that facilitates innate immune signalling. Nature (2008) 455(7213):674–8. doi: 10.1038/nature07317
21. Wu J, Sun L, Chen X, Du F, Shi H, Chen C, et al. Cyclic gmp-amp is an endogenous second messenger in innate immune signaling by cytosolic DNA. Science (2013) 339(6121):826–30. doi: 10.1126/science.1229963
22. Watson RO, Manzanillo PS, Cox JS. Extracellular M. Tuberculosis DNA targets bacteria for autophagy by activating the host DNA-sensing pathway. Cell (2012) 150(4):803–15. doi: 10.1016/j.cell.2012.06.040
23. Chong A, Celli J. The francisella intracellular life cycle: Toward molecular mechanisms of intracellular survival and proliferation. Front Microbiol (2010) 1:138. doi: 10.3389/fmicb.2010.00138
24. Harrison SC. Viral membrane fusion. Nat Struct Mol Biol (2008) 15(7):690–8. doi: 10.1038/nsmb.1456
25. West AP, Shadel GS, Ghosh S. Mitochondria in innate immune responses. Nat Rev Immunol (2011) 11(6):389–402. doi: 10.1038/nri2975
26. Liu H, Zhu Z, Xue Q, Yang F, Li Z, Xue Z, et al. Innate sensing of picornavirus infection involves cgas-sting-mediated antiviral responses triggered by mitochondrial DNA release. PLoS Pathog (2023) 19(2):e1011132. doi: 10.1371/journal.ppat.1011132
27. Jahun AS, Sorgeloos F, Chaudhry Y, Arthur SE, Hosmillo M, Georgana I, et al. Leaked genomic and mitochondrial DNA contribute to the host response to noroviruses in a sting-dependent manner. Cell Rep (2023) 42(3):112179. doi: 10.1016/j.celrep.2023.112179
28. Zhang X, Wu J, Du F, Xu H, Sun L, Chen Z, et al. The cytosolic DNA sensor cgas forms an oligomeric complex with DNA and undergoes switch-like conformational changes in the activation loop. Cell Rep (2014) 6(3):421–30. doi: 10.1016/j.celrep.2014.01.003
29. Li X, Shu C, Yi G, Chaton CT, Shelton CL, Diao J, et al. Cyclic gmp-amp synthase is activated by double-stranded DNA-induced oligomerization. Immunity (2013) 39(6):1019–31. doi: 10.1016/j.immuni.2013.10.019
30. Motwani M, Pesiridis S, Fitzgerald KA. DNA sensing by the cgas-sting pathway in health and disease. Nat Rev Genet (2019) 20(11):657–74. doi: 10.1038/s41576-019-0151-1
31. Gui X, Yang H, Li T, Tan X, Shi P, Li M, et al. Autophagy induction via STING trafficking is a primordial function of the cGAS pathway. Nature (2019) 567(7747):262–6. doi: 10.1038/s41586-019-1006-9
32. Zhao H, Wu L, Yan G, Chen Y, Zhou M, Wu Y, et al. Inflammation and tumor progression: Signaling pathways and targeted intervention. Signal Transduct Targeted Ther (2021) 6(1):263. doi: 10.1038/s41392-021-00658-5
33. Rubin DC, Shaker A, Levin MS. Chronic intestinal inflammation: Inflammatory bowel disease and colitis-associated colon cancer. Front Immunol (2012) 3:107. doi: 10.3389/fimmu.2012.00107
34. Lucafo M, Curci D, Franzin M, Decorti G, Stocco G. Inflammatory bowel disease and risk of colorectal cancer: An overview from pathophysiology to pharmacological prevention. Front Pharmacol (2021) 12:772101. doi: 10.3389/fphar.2021.772101
35. Barber GN. Sting: infection, inflammation and cancer. Nat Rev Immunol (2015) 15(12):760–70. doi: 10.1038/nri3921
36. Ke X, Hu T, Jiang M. Cgas-sting signaling pathway in gastrointestinal inflammatory disease and cancers. FASEB J (2022) 36(1):e22029. doi: 10.1096/fj.202101199R
37. Whiteley AT, Eaglesham JB, de Oliveira Mann CC, Morehouse BR, Lowey B, Nieminen EA, et al. Bacterial cgas-like enzymes synthesize diverse nucleotide signals. Nature (2019) 567(7747):194–9. doi: 10.1038/s41586-019-0953-5
38. Kranzusch PJ, Lee AS, Berger JM, Doudna JA. Structure of human cgas reveals a conserved family of second-messenger enzymes in innate immunity. Cell Rep (2013) 3(5):1362–8. doi: 10.1016/j.celrep.2013.05.008
39. Wu X, Wu FH, Wang X, Wang L, Siedow JN, Zhang W, et al. Molecular evolutionary and structural analysis of the cytosolic DNA sensor cgas and sting. Nucleic Acids Res (2014) 42(13):8243–57. doi: 10.1093/nar/gku569
40. Zhou W, Whiteley AT, de Oliveira Mann CC, Morehouse BR, Nowak RP, Fischer ES, et al. Structure of the human cgas-DNA complex reveals enhanced control of immune surveillance. Cell (2018) 174(2):300–11.e11. doi: 10.1016/j.cell.2018.06.026
41. Andreeva L, Hiller B, Kostrewa D, Lässig C, de Oliveira Mann CC, Jan Drexler D, et al. Cgas senses long and hmgb/tfam-bound U-turn DNA by forming protein-DNA ladders. Nature (2017) 549(7672):394–8. doi: 10.1038/nature23890
42. Civril F, Deimling T, de Oliveira Mann CC, Ablasser A, Moldt M, Witte G, et al. Structural mechanism of cytosolic DNA sensing by cgas. Nature (2013) 498(7454):332–7. doi: 10.1038/nature12305
43. Du M, Chen ZJ. DNA-induced liquid phase condensation of cgas activates innate immune signaling. Science (2018) 361(6403):704–9. doi: 10.1126/science.aat1022
44. Gao P, Ascano M, Wu Y, Barchet W, Gaffney BL, Zillinger T, et al. Cyclic [G(2',5')Pa(3',5')P] is the metazoan second messenger produced by DNA-activated cyclic gmp-amp synthase. Cell (2013) 153(5):1094–107. doi: 10.1016/j.cell.2013.04.046
45. Bai J, Liu F. Nuclear cgas: Sequestration and beyond. Protein Cell (2022) 13(2):90–101. doi: 10.1007/s13238-021-00869-0
46. Ablasser A, Goldeck M, Cavlar T, Deimling T, Witte G, Röhl I, et al. Cgas produces a 2'-5'-linked cyclic dinucleotide second messenger that activates sting. Nature (2013) 498(7454):380–4. doi: 10.1038/nature12306
47. Ablasser A, Hemmerling I, Schmid-Burgk JL, Behrendt R, Roers A, Hornung V. Trex1 deficiency triggers cell-autonomous immunity in a cgas-dependent manner. J Immunol (2014) 192(12):5993–7. doi: 10.4049/jimmunol.1400737
48. Gao D, Li T, Li XD, Chen X, Li QZ, Wight-Carter M, et al. Activation of cyclic gmp-amp synthase by self-DNA causes autoimmune diseases. Proc Natl Acad Sci USA (2015) 112(42):E5699–705. doi: 10.1073/pnas.1516465112
49. Ishikawa H, Ma Z, Barber GN. Sting regulates intracellular DNA-mediated, type I interferon-dependent innate immunity. Nature (2009) 461(7265):788–92. doi: 10.1038/nature08476
50. Kranzusch PJ, Wilson SC, Lee AS, Berger JM, Doudna JA, Vance RE. Ancient origin of cgas-sting reveals mechanism of universal 2',3' Cgamp signaling. Mol Cell (2015) 59(6):891–903. doi: 10.1016/j.molcel.2015.07.022
51. Ergun SL, Li L. Structural insights into sting signaling. Trends Cell Biol (2020) 30(5):399–407. doi: 10.1016/j.tcb.2020.01.010
52. Cavlar T, Deimling T, Ablasser A, Hopfner KP, Hornung V. Species-specific detection of the antiviral small-molecule compound cma by sting. EMBO J (2013) 32(10):1440–50. doi: 10.1038/emboj.2013.86
53. Chin KH, Tu ZL, Su YC, Yu YJ, Chen HC, Lo YC, et al. Novel C-di-gmp recognition modes of the mouse innate immune adaptor protein sting. Acta Crystallogr D Biol Crystallogr (2013) 69(Pt 3):352–66. doi: 10.1107/s0907444912047269
54. Zhang X, Bai XC, Chen ZJ. Structures and mechanisms in the cgas-sting innate immunity pathway. Immunity (2020) 53(1):43–53. doi: 10.1016/j.immuni.2020.05.013
55. Li X, Zhu Y, Zhang X, An X, Weng M, Shi J, et al. An alternatively spliced STING isoform localizes in the cytoplasmic membrane and directly senses extracellular cGAMP. J Clin Invest (2022) 132(3):e144339. doi: 10.1172/jci144339
56. Srikanth S, Woo JS, Wu B, El-Sherbiny YM, Leung J, Chupradit K, et al. The ca(2+) sensor stim1 regulates the type I interferon response by retaining the signaling adaptor sting at the endoplasmic reticulum. Nat Immunol (2019) 20(2):152–62. doi: 10.1038/s41590-018-0287-8
57. Dimitrov DS. Virus entry: Molecular mechanisms and biomedical applications. Nat Rev Microbiol (2004) 2(2):109–22. doi: 10.1038/nrmicro817
58. Pizarro-Cerdá J, Cossart P. Bacterial adhesion and entry into host cells. Cell (2006) 124(4):715–27. doi: 10.1016/j.cell.2006.02.012
59. Walker DM, Oghumu S, Gupta G, McGwire BS, Drew ME, Satoskar AR. Mechanisms of cellular invasion by intracellular parasites. Cell Mol Life Sci (2014) 71(7):1245–63. doi: 10.1007/s00018-013-1491-1
60. Pan J, Fei CJ, Hu Y, Wu XY, Nie L, Chen J. Current understanding of the cgas-sting signaling pathway: Structure, regulatory mechanisms, and related diseases. Zool Res (2023) 44(1):183–218. doi: 10.24272/j.issn.2095-8137.2022.464
61. Burdette DL, Monroe KM, Sotelo-Troha K, Iwig JS, Eckert B, Hyodo M, et al. Sting is a direct innate immune sensor of cyclic di-gmp. Nature (2011) 478(7370):515–8. doi: 10.1038/nature10429
62. Taguchi T, Mukai K, Takaya E, Shindo R. Sting operation at the er/golgi interface. Front Immunol (2021) 12:646304. doi: 10.3389/fimmu.2021.646304
63. Zhang BC, Nandakumar R, Reinert LS, Huang J, Laustsen A, Gao ZL, et al. Steep mediates sting er exit and activation of signaling. Nat Immunol (2020) 21(8):868–79. doi: 10.1038/s41590-020-0730-5
64. Mukai K, Konno H, Akiba T, Uemura T, Waguri S, Kobayashi T, et al. Activation of sting requires palmitoylation at the golgi. Nat Commun (2016) 7:11932. doi: 10.1038/ncomms11932
65. Abe T, Harashima A, Xia T, Konno H, Konno K, Morales A, et al. Sting recognition of cytoplasmic DNA instigates cellular defense. Mol Cell (2013) 50(1):5–15. doi: 10.1016/j.molcel.2013.01.039
66. Zhang C, Shang G, Gui X, Zhang X, Bai XC, Chen ZJ. Structural basis of sting binding with and phosphorylation by tbk1. Nature (2019) 567(7748):394–8. doi: 10.1038/s41586-019-1000-2
67. Zhao B, Du F, Xu P, Shu C, Sankaran B, Bell SL, et al. A conserved plplrt/sd motif of sting mediates the recruitment and activation of tbk1. Nature (2019) 569(7758):718–22. doi: 10.1038/s41586-019-1228-x
68. Liu S, Cai X, Wu J, Cong Q, Chen X, Li T, et al. Phosphorylation of innate immune adaptor proteins mavs, sting, and trif induces irf3 activation. Science (2015) 347(6227):aaa2630. doi: 10.1126/science.aaa2630
69. Dalskov L, Narita R, Andersen LL, Jensen N, Assil S, Kristensen KH, et al. Characterization of distinct molecular interactions responsible for irf3 and irf7 phosphorylation and subsequent dimerization. Nucleic Acids Res (2020) 48(20):11421–33. doi: 10.1093/nar/gkaa873
70. Schneider WM, Chevillotte MD, Rice CM. Interferon-stimulated genes: A complex web of host defenses. Annu Rev Immunol (2014) 32:513–45. doi: 10.1146/annurev-immunol-032713-120231
71. Chen H, Sun H, You F, Sun W, Zhou X, Chen L, et al. Activation of stat6 by sting is critical for antiviral innate immunity. Cell (2011) 147(2):436–46. doi: 10.1016/j.cell.2011.09.022
72. Hopfner KP, Hornung V. Molecular mechanisms and cellular functions of cgas-sting signalling. Nat Rev Mol Cell Biol (2020) 21(9):501–21. doi: 10.1038/s41580-020-0244-x
73. Balka KR, Louis C, Saunders TL, Smith AM, Calleja DJ, D’Silva DB, et al. Tbk1 and ikkϵ Act redundantly to mediate sting-induced nf-κb responses in myeloid cells. Cell Rep (2020) 31(1):107492. doi: 10.1016/j.celrep.2020.03.056
74. Zandi E, Rothwarf DM, Delhase M, Hayakawa M, Karin M. The ikappab kinase complex (Ikk) contains two kinase subunits, ikkalpha and ikkbeta, necessary for ikappab phosphorylation and nf-kappab activation. Cell (1997) 91(2):243–52. doi: 10.1016/s0092-8674(00)80406-7
75. de Oliveira Mann CC, Orzalli MH, King DS, Kagan JC, Lee ASY, Kranzusch PJ. Modular architecture of the sting C-terminal tail allows interferon and nf-κb signaling adaptation. Cell Rep (2019) 27(4):1165–75.e5. doi: 10.1016/j.celrep.2019.03.098
76. Wang Y, Luo J, Alu A, Han X, Wei Y, Wei X. Cgas-sting pathway in cancer biotherapy. Mol Cancer (2020) 19(1):136. doi: 10.1186/s12943-020-01247-w
77. Parvatiyar K, Pindado J, Dev A, Aliyari SR, Zaver SA, Gerami H, et al. A traf3-nik module differentially regulates DNA vs rna pathways in innate immune signaling. Nat Commun (2018) 9(1):2770. doi: 10.1038/s41467-018-05168-7
78. Galluzzi L, Vanpouille-Box C, Bakhoum SF, Demaria S. Snapshot: Cgas-sting signaling. Cell (2018) 173(1):276–.e1. doi: 10.1016/j.cell.2018.03.015
79. Cheng Z, Dai T, He X, Zhang Z, Xie F, Wang S, et al. The interactions between cgas-sting pathway and pathogens. Signal Transduct Target Ther (2020) 5(1):91. doi: 10.1038/s41392-020-0198-7
80. Hong C, Schubert M, Tijhuis AE, Requesens M, Roorda M, van den Brink A, et al. Cgas-sting drives the il-6-dependent survival of chromosomally instable cancers. Nature (2022) 607(7918):366–73. doi: 10.1038/s41586-022-04847-2
81. Yu H, Pardoll D, Jove R. Stats in cancer inflammation and immunity: A leading role for stat3. Nat Rev Cancer (2009) 9(11):798–809. doi: 10.1038/nrc2734
82. Zhang L, Wei X, Wang Z, Liu P, Hou Y, Xu Y, et al. Nf-κb activation enhances sting signaling by altering microtubule-mediated sting trafficking. Cell Rep (2023) 42(3):112185. doi: 10.1016/j.celrep.2023.112185
83. Hou Y, Liang H, Rao E, Zheng W, Huang X, Deng L, et al. Non-canonical nf-κb antagonizes sting sensor-mediated DNA sensing in radiotherapy. Immunity (2018) 49(3):490–503.e4. doi: 10.1016/j.immuni.2018.07.008
84. Chen Q, Boire A, Jin X, Valiente M, Er EE, Lopez-Soto A, et al. Carcinoma-astrocyte gap junctions promote brain metastasis by cgamp transfer. Nature (2016) 533(7604):493–8. doi: 10.1038/nature18268
85. Gentili M, Kowal J, Tkach M, Satoh T, Lahaye X, Conrad C, et al. Transmission of innate immune signaling by packaging of cgamp in viral particles. Science (2015) 349(6253):1232–6. doi: 10.1126/science.aab3628
86. Zhou C, Chen X, Planells-Cases R, Chu J, Wang L, Cao L, et al. Transfer of cgamp into bystander cells via lrrc8 volume-regulated anion channels augments sting-mediated interferon responses and anti-viral immunity. Immunity (2020) 52(5):767–81.e6. doi: 10.1016/j.immuni.2020.03.016
87. Maltbaek JH, Cambier S, Snyder JM, Stetson DB. ABCC1 transporter exports the immunostimulatory cyclic dinucleotide cGAMP. Immunity (2022) 55(10):1799–812.e4. doi: 10.1016/j.immuni.2022.08.006
88. Ritchie C, Cordova AF, Hess GT, Bassik MC, Li L. Slc19a1 is an importer of the immunotransmitter cgamp. Mol Cell (2019) 75(2):372–81.e5. doi: 10.1016/j.molcel.2019.05.006
89. Cordova AF, Ritchie C, Böhnert V, Li L. Human slc46a2 is the dominant cgamp importer in extracellular cgamp-sensing macrophages and monocytes. ACS Cent Sci (2021) 7(6):1073–88. doi: 10.1021/acscentsci.1c00440
90. Li L, Yin Q, Kuss P, Maliga Z, Millán JL, Wu H, et al. Hydrolysis of 2'3'-cgamp by enpp1 and design of nonhydrolyzable analogs. Nat Chem Biol (2014) 10(12):1043–8. doi: 10.1038/nchembio.1661
91. Kato K, Nishimasu H, Oikawa D, HIrano S, HIrano H, Kasuya G, et al. Structural insights into cgamp degradation by ecto-nucleotide pyrophosphatase phosphodiesterase 1. Nat Commun (2018) 9(1):4424. doi: 10.1038/s41467-018-06922-7
92. Carozza JA, Böhnert V, Nguyen KC, Skariah G, Shaw KE, Brown JA, et al. Extracellular cgamp is a cancer cell-produced immunotransmitter involved in radiation-induced anti-cancer immunity. Nat Cancer (2020) 1(2):184–96. doi: 10.1038/s43018-020-0028-4
93. Gonugunta VK, Sakai T, Pokatayev V, Yang K, Wu J, Dobbs N, et al. Trafficking-mediated sting degradation requires sorting to acidified endolysosomes and can be targeted to enhance anti-tumor response. Cell Rep (2017) 21(11):3234–42. doi: 10.1016/j.celrep.2017.11.061
94. Okumura R, Takeda K. Roles of intestinal epithelial cells in the maintenance of gut homeostasis. Exp Mol Med (2017) 49(5):e338. doi: 10.1038/emm.2017.20
95. Shi N, Li N, Duan X, Niu H. Interaction between the gut microbiome and mucosal immune system. Mil Med Res (2017) 4:14. doi: 10.1186/s40779-017-0122-9
96. Li D, Wu M. Pattern recognition receptors in health and diseases. Signal Transduct Target Ther (2021) 6(1):291. doi: 10.1038/s41392-021-00687-0
97. Hu Q, Zhou Q, Xia X, Shao L, Wang M, Lu X, et al. Cytosolic sensor sting in mucosal immunity: A master regulator of gut inflammation and carcinogenesis. J Exp Clin Cancer Res (2021) 40(1):39. doi: 10.1186/s13046-021-01850-9
98. Qing F, Xie T, Xie L, Guo T, Liu Z. How gut microbiota are shaped by pattern recognition receptors in colitis and colorectal cancer. Cancers (2022) 14(15):3821. doi: 10.3390/cancers14153821
99. Metzger R, Krug A, Eisenächer K. Enteric virome sensing—Its role in intestinal homeostasis and immunity. Viruses (2018) 10(4):146. doi: 10.3390/v10040146
100. Xing C, Du Y, Duan T, Nim K, Chu J, Wang HY, et al. Interaction between microbiota and immunity and its implication in colorectal cancer. Front Immunol (2022) 13:963819. doi: 10.3389/fimmu.2022.963819
101. Canesso MCC, Lemos L, Neves TC, Marim FM, Castro TBR, Veloso ES, et al. The cytosolic sensor sting is required for intestinal homeostasis and control of inflammation. Mucosal Immunol (2018) 11(3):820–34. doi: 10.1038/mi.2017.88
102. Yu T, Yang W, Yao S, Yu Y, Wakamiya M, Golovko G, et al. STING promotes intestinal IgA production by regulating acetate-producing bacteria to maintain host-microbiota mutualism. Inflamm Bowel Dis (2023) 29(6):946–59. doi: 10.1093/ibd/izac268
103. Shmuel-Galia L, Humphries F, Lei X, Ceglia S, Wilson R, Jiang Z, et al. Dysbiosis exacerbates colitis by promoting ubiquitination and accumulation of the innate immune adaptor sting in myeloid cells. Immunity (2021) 54(6):1137–53.e8. doi: 10.1016/j.immuni.2021.05.008
104. Leibowitz BJ, Zhao G, Wei L, Ruan H, Epperly M, Chen L, et al. Interferon B drives intestinal regeneration after radiation. Sci Adv (2021) 7(41):eabi5253. doi: 10.1126/sciadv.abi5253
105. Fischer JC, Bscheider M, Eisenkolb G, Lin CC, Wintges A, Otten V, et al. RIG-I/MAVS and STING signaling promote gut integrity during irradiation- and immune-mediated tissue injury. Sci Transl Med (2017) 9(386):eaag2513. doi: 10.1126/scitranslmed.aag2513
106. Brenner H, Kloor M, Pox CP. Colorectal cancer. Lancet (2014) 383(9927):1490–502. doi: 10.1016/s0140-6736(13)61649-9
107. Hu S, Fang Y, Chen X, Cheng T, Zhao M, Du M, et al. cGAS restricts colon cancer development by protecting intestinal barrier integrity. Proc Natl Acad Sci USA (2021) 118(23):e2105747118. doi: 10.1073/pnas.2105747118
108. Gong W, Liu P, Zhao F, Liu J, Hong Z, Ren H, et al. Sting-mediated syk signaling attenuates tumorigenesis of colitis−Associated colorectal cancer through enhancing intestinal epithelium pyroptosis. Inflamm Bowel Dis (2022) 28(4):572–85. doi: 10.1093/ibd/izab217
109. Zhu Q, Man SM, Gurung P, Liu Z, Vogel P, Lamkanfi M, et al. Cutting edge: Sting mediates protection against colorectal tumorigenesis by governing the magnitude of intestinal inflammation. J Immunol (2014) 193(10):4779–82. doi: 10.4049/jimmunol.1402051
110. Ahn J, Son S, Oliveira SC, Barber GN. Sting-dependent signaling underlies il-10 controlled inflammatory colitis. Cell Rep (2017) 21(13):3873–84. doi: 10.1016/j.celrep.2017.11.101
111. Wei B, Xu L, Guo W, Wang Y, Wu J, Li X, et al. Shp2-mediated inhibition of DNA repair contributes to cgas-sting activation and chemotherapeutic sensitivity in colon cancer. Cancer Res (2021) 81(12):3215–28. doi: 10.1158/0008-5472.Can-20-3738
112. Tian J, Zhang D, Kurbatov V, Wang Q, Wang Y, Fang D, et al. 5-fluorouracil efficacy requires anti-tumor immunity triggered by cancer-cell-intrinsic sting. EMBO J (2021) 40(7):e106065. doi: 10.15252/embj.2020106065
113. Lai CT, Chi CW, Wu SH, Shieh HR, Yen JC, Chen YJ. Midostaurin modulates tumor microenvironment and enhances efficacy of anti-PD-1 against colon cancer. Cancers (Basel) (2022) 14(19):4847. doi: 10.3390/cancers14194847
114. Shi Y, Zheng W, Yang K, Harris KG, Ni K, Xue L, et al. Intratumoral accumulation of gut microbiota facilitates CD47-based immunotherapy via STING signaling. J Exp Med (2020) 217(5):e20192282. doi: 10.1084/jem.20192282
115. Si W, Liang H, Bugno J, Xu Q, Ding X, Yang K, et al. Lactobacillus rhamnosus GG induces cGAS/STING- dependent type I interferon and improves response to immune checkpoint blockade. Gut (2021) 71(3):521–33. doi: 10.1136/gutjnl-2020-323426
116. Yang H, Lee WS, Kong SJ, Kim CG, Kim JH, Chang SK, et al. Sting activation reprograms tumor vasculatures and synergizes with vegfr2 blockade. J Clin Invest (2019) 129(10):4350–64. doi: 10.1172/jci125413
117. Hu Z, Teng XL, Zhang T, Yu X, Ding R, Yi J, et al. Senp3 senses oxidative stress to facilitate sting-dependent dendritic cell antitumor function. Mol Cell (2021) 81(5):940–52.e5. doi: 10.1016/j.molcel.2020.12.024
118. Hsieh RC, Krishnan S, Wu RC, Boda AR, Liu A, Winkler M, et al. Atr-mediated CD47 and pd-L1 up-regulation restricts radiotherapy-induced immune priming and abscopal responses in colorectal cancer. Sci Immunol (2022) 7(72):eabl9330. doi: 10.1126/sciimmunol.abl9330
119. Gao Y, Bi D, Xie R, Li M, Guo J, Liu H, et al. Fusobacterium nucleatum enhances the efficacy of pd-L1 blockade in colorectal cancer. Signal Transduct Target Ther (2021) 6(1):398. doi: 10.1038/s41392-021-00795-x
120. Gao J, Wu Z, Zhao M, Zhang R, Li M, Sun D, et al. Allosteric inhibition reveals shp2-mediated tumor immunosuppression in colon cancer by single-cell transcriptomics. Acta Pharm Sin B (2022) 12(1):149–66. doi: 10.1016/j.apsb.2021.08.006
121. Zhong G, Peng C, Chen Y, Li J, Yang R, Wu M, et al. Expression of sting and pd-L1 in colorectal cancer and their correlation with clinical prognosis. Int J Clin Exp Pathol (2018) 11(3):1256–64.
122. Zheng H, Wu L, Xiao Q, Meng X, Hafiz A, Yan Q, et al. Epigenetically suppressed tumor cell intrinsic sting promotes tumor immune escape. BioMed Pharmacother (2023) 157:114033. doi: 10.1016/j.biopha.2022.114033
123. Ohkuri T, Kosaka A, Ishibashi K, Kumai T, Hirata Y, Ohara K, et al. Intratumoral administration of cgamp transiently accumulates potent macrophages for anti-tumor immunity at a mouse tumor site. Cancer Immunol Immunother (2017) 66(6):705–16. doi: 10.1007/s00262-017-1975-1
124. Chon HJ, Kim H, Noh JH, Yang H, Lee WS, Kong SJ, et al. Sting signaling is a potential immunotherapeutic target in colorectal cancer. J Cancer (2019) 10(20):4932–8. doi: 10.7150/jca.32806
125. Shi J, Liu C, Luo S, Cao T, Lin B, Zhou M, et al. Sting agonist and ido inhibitor combination therapy inhibits tumor progression in murine models of colorectal cancer. Cell Immunol (2021) 366:104384. doi: 10.1016/j.cellimm.2021.104384
126. Xia T, Konno H, Ahn J, Barber GN. Deregulation of sting signaling in colorectal carcinoma constrains DNA damage responses and correlates with tumorigenesis. Cell Rep (2016) 14(2):282–97. doi: 10.1016/j.celrep.2015.12.029
127. Sauer JD, Sotelo-Troha K, von Moltke J, Monroe KM, Rae CS, Brubaker SW, et al. The N-ethyl-N-nitrosourea-induced goldenticket mouse mutant reveals an essential function of sting in the in vivo interferon response to listeria monocytogenes and cyclic dinucleotides. Infect Immun (2011) 79(2):688–94. doi: 10.1128/iai.00999-10
128. Deng L, Liang H, Xu M, Yang X, Burnette B, Arina A, et al. Sting-dependent cytosolic DNA sensing promotes radiation-induced type I interferon-dependent antitumor immunity in immunogenic tumors. Immunity (2014) 41(5):843–52. doi: 10.1016/j.immuni.2014.10.019
129. Liu Q, Qu J, Zhao M, Xu Q, Sun Y. Targeting shp2 as a promising strategy for cancer immunotherapy. Pharmacol Res (2020) 152:104595. doi: 10.1016/j.phrs.2019.104595
130. Ray Chaudhuri A, Nussenzweig A. The multifaceted roles of parp1 in DNA repair and chromatin remodelling. Nat Rev Mol Cell Biol (2017) 18(10):610–21. doi: 10.1038/nrm.2017.53
131. Candas-Green D, Xie B, Huang J, Fan M, Wang A, Menaa C, et al. Dual blockade of CD47 and her2 eliminates radioresistant breast cancer cells. Nat Commun (2020) 11(1):4591. doi: 10.1038/s41467-020-18245-7
132. Golubovskaya V, Berahovich R, Zhou H, Xu S, Harto H, Li L, et al. CD47-CAR-T cells effectively kill target cancer cells and block pancreatic tumor growth. Cancers (Basel) (2017) 9(10):139. doi: 10.3390/cancers9100139
133. Kim H, Bang S, Jee S, Paik SS, Jang K. Clinicopathological significance of CD47 expression in hepatocellular carcinoma. J Clin Pathol (2021) 74(2):111–5. doi: 10.1136/jclinpath-2020-206611
134. Weiskopf K, Jahchan NS, Schnorr PJ, Cristea S, Ring AM, Maute RL, et al. CD47-blocking immunotherapies stimulate macrophage-mediated destruction of small-cell lung cancer. J Clin Invest (2016) 126(7):2610–20. doi: 10.1172/jci81603
135. Hu T, Liu H, Liang Z, Wang F, Zhou C, Zheng X, et al. Tumor-intrinsic CD47 signal regulates glycolysis and promotes colorectal cancer cell growth and metastasis. Theranostics (2020) 10(9):4056–72. doi: 10.7150/thno.40860
136. Sugimura-Nagata A, Koshino A, Inoue S, Matsuo-Nagano A, Komura M, Riku M, et al. Expression and prognostic significance of CD47-SIRPA macrophage checkpoint molecules in colorectal cancer. Int J Mol Sci (2021) 22(5):2690. doi: 10.3390/ijms22052690
137. Oh HH, Park YL, Park SY, Myung E, Im CM, Yu HJ, et al. CD47 mediates the progression of colorectal cancer by inducing tumor cell apoptosis and angiogenesis. Pathol Res Pract (2022) 240:154220. doi: 10.1016/j.prp.2022.154220
138. Hayat SMG, Bianconi V, Pirro M, Jaafari MR, Hatamipour M, Sahebkar A. CD47: Role in the immune system and application to cancer therapy. Cell Oncol (Dordr) (2020) 43(1):19–30. doi: 10.1007/s13402-019-00469-5
139. Weiskopf K. Cancer immunotherapy targeting the CD47/sirpα Axis. Eur J Cancer (2017) 76:100–9. doi: 10.1016/j.ejca.2017.02.013
140. Llosa NJ, Cruise M, Tam A, Wicks EC, Hechenbleikner EM, Taube JM, et al. The vigorous immune microenvironment of microsatellite instable colon cancer is balanced by multiple counter-inhibitory checkpoints. Cancer Discov (2015) 5(1):43–51. doi: 10.1158/2159-8290.Cd-14-0863
141. Topalian SL, Hodi FS, Brahmer JR, Gettinger SN, Smith DC, McDermott DF, et al. Safety, activity, and immune correlates of anti-PD-1 antibody in cancer. N Engl J Med (2012) 366(26):2443–54. doi: 10.1056/NEJMoa1200690
142. Yu T, Guo F, Yu Y, Sun T, Ma D, Han J, et al. Fusobacterium nucleatum promotes chemoresistance to colorectal cancer by modulating autophagy. Cell (2017) 170(3):548–63.e16. doi: 10.1016/j.cell.2017.07.008
143. Yang Y, Weng W, Peng J, Hong L, Yang L, Toiyama Y, et al. Fusobacterium nucleatum increases proliferation of colorectal cancer cells and tumor development in mice by activating toll-like receptor 4 signaling to nuclear factor-κb, and up-regulating expression of microrna-21. Gastroenterology (2017) 152(4):851–66.e24. doi: 10.1053/j.gastro.2016.11.018
144. Lee SJ, Yang H, Kim WR, Lee YS, Lee WS, Kong SJ, et al. STING activation norMalizes the intraperitoneal vascular-immune microenvironment and suppresses peritoneal carcinomatosis of colon cancer. J Immunother Cancer (2021) 9(6):e002195. doi: 10.1136/jitc-2020-002195
145. Meric-Bernstam F, Sweis RF, Hodi FS, Messersmith WA, Andtbacka RHI, Ingham M, et al. Phase I dose-escalation trial of miw815 (Adu-S100), an intratumoral sting agonist, in patients with advanced/metastatic solid tumors or lymphomas. Clin Cancer Res (2022) 28(4):677–88. doi: 10.1158/1078-0432.Ccr-21-1963
146. Meric-Bernstam F, Sweis RF, Kasper S, Hamid O, Bhatia S, Dummer R, et al. Combination of the sting agonist miw815 (Adu-S100) and pd-1 inhibitor spartalizumab in advanced/metastatic solid tumors or lymphomas: An open-label, multicenter, phase ib study. Clin Cancer Res (2023) 29(1):110–21. doi: 10.1158/1078-0432.Ccr-22-2235
147. Luke JJ, Piha-Paul SA, Medina T, Verschraegen CF, Varterasian M, Brennan AM, et al. Phase I study of synb1891, an engineered E. Coli nissle strain expressing sting agonist, with and without atezolizumab in advanced Malignancies. Clin Cancer Res (2023) 29(13):2435–44. doi: 10.1158/1078-0432.Ccr-23-0118
148. Hines JB, Kacew AJ, Sweis RF. The development of sting agonists and emerging results as a cancer immunotherapy. Curr Oncol Rep (2023) 25(3):189–99. doi: 10.1007/s11912-023-01361-0
149. Kaplan GG, Ng SC. Understanding and preventing the global increase of inflammatory bowel disease. Gastroenterology (2017) 152(2):313–21 e2. doi: 10.1053/j.gastro.2016.10.020
150. Park SJ, Kim WH, Cheon JH. Clinical characteristics and treatment of inflammatory bowel disease: A comparison of eastern and western perspectives. World J Gastroenterol (2014) 20(33):11525–37. doi: 10.3748/wjg.v20.i33.11525
151. Boyapati RK, Dorward DA, Tamborska A, Kalla R, Ventham NT, Doherty MK, et al. Mitochondrial DNA is a pro-inflammatory damage-associated molecular pattern released during active ibd. Inflamm Bowel Dis (2018) 24(10):2113–22. doi: 10.1093/ibd/izy095
152. Vrablicova Z, Tomova K, Tothova L, Babickova J, Gromova B, Konecna B, et al. Nuclear and mitochondrial circulating cell-free DNA is increased in patients with inflammatory bowel disease in clinical remission. Front Med (Lausanne) (2020) 7:593316. doi: 10.3389/fmed.2020.593316
153. Zhao F, Zheng T, Gong W, Wu J, Xie H, Li W, et al. Extracellular vesicles package dsdna to aggravate crohn’s disease by activating the sting pathway. Cell Death Dis (2021) 12(9):1022. doi: 10.1038/s41419-021-04101-z
154. Wu X, Chen X, Liu H, He ZW, Wang Z, Wei LJ, et al. Rescuing dicer expression in inflamed colon tissues alleviates colitis and prevents colitis-associated tumorigenesis. Theranostics (2020) 10(13):5749–62. doi: 10.7150/thno.41894
155. Pereira C, Grácio D, Teixeira JP, Magro F. Oxidative stress and DNA damage: Implications in inflammatory bowel disease. Inflammation Bowel Dis (2015) 21(10):2403–17. doi: 10.1097/mib.0000000000000506
156. Ni J, Wu GD, Albenberg L, Tomov VT. Gut microbiota and ibd: Causation or correlation? Nat Rev Gastroenterol Hepatol (2017) 14(10):573–84. doi: 10.1038/nrgastro.2017.88
157. Putignani L, Del Chierico F, Vernocchi P, Cicala M, Cucchiara S, Dallapiccola B. Gut microbiota dysbiosis as risk and premorbid factors of ibd and ibs along the childhood-adulthood transition. Inflammation Bowel Dis (2016) 22(2):487–504. doi: 10.1097/mib.0000000000000602
158. Rashed R, Valcheva R, Dieleman LA. Manipulation of gut microbiota as a key target for crohn's disease. Front Med (Lausanne) (2022) 9:887044. doi: 10.3389/fmed.2022.887044
159. Chen C, Zhang Y, Tao M, Zhao X, Feng Q, Fei X, et al. Atrial natriuretic peptide attenuates colitis via inhibition of the cgas-sting pathway in colonic epithelial cells. Int J Biol Sci (2022) 18(4):1737–54. doi: 10.7150/ijbs.67356
160. Gong LK, Yang X, Yang J, Wu S, Chen Y, Zhang JT, et al. Low-dose ganciclovir ameliorates dextran sulfate sodium-induced ulcerative colitis through inhibiting macrophage sting activation in mice. Front Pharmacol (2022) 13:1020670. doi: 10.3389/fphar.2022.1020670
161. Cai Y, Xu B, Zhou F, Wu J, Li S, Zheng Q, et al. Si-Ni-San ameliorates chronic colitis by modulating type I interferons-mediated inflammation. Phytomedicine (2021) 84:153495. doi: 10.1016/j.phymed.2021.153495
162. Song J, Yang RR, Chang J, Liu YD, Lu CH, Chen LF, et al. Discovery and characterization of a novel cgas covalent inhibitor for the treatment of inflammatory bowel disease. Acta Pharmacol Sin (2023) 44(4):791–800. doi: 10.1038/s41401-022-01002-5
163. Aden K, Tran F, Ito G, Sheibani-Tezerji R, Lipinski S, Kuiper JW, et al. Atg16l1 orchestrates interleukin-22 signaling in the intestinal epithelium Via cgas-sting. J Exp Med (2018) 215(11):2868–86. doi: 10.1084/jem.20171029
164. Ma C, Yang D, Wang B, Wu C, Wu Y, Li S, et al. Gasdermin d in macrophages restrains colitis by controlling cgas-mediated inflammation. Sci Adv (2020) 6(21):eaaz6717. doi: 10.1126/sciadv.aaz6717
165. Flood P, Fanning A, Woznicki JA, Crowley T, Christopher A, Vaccaro A, et al. DNA sensor-associated type I interferon signaling is increased in ulcerative colitis and induces jak-dependent inflammatory cell death in colonic organoids. Am J Physiol Gastrointest Liver Physiol (2022) 323(5):G439–g60. doi: 10.1152/ajpgi.00104.2022
166. Rioux JD, Xavier RJ, Taylor KD, Silverberg MS, Goyette P, Huett A, et al. Genome-wide association study identifies new susceptibility loci for crohn disease and implicates autophagy in disease pathogenesis. Nat Genet (2007) 39(5):596–604. doi: 10.1038/ng2032
167. Adolph TE, Tomczak MF, Niederreiter L, Ko HJ, Böck J, Martinez-Naves E, et al. Paneth cells as a site of origin for intestinal inflammation. Nature (2013) 503(7475):272–6. doi: 10.1038/nature12599
168. Aden K, Tran F, Ito G, Sheibani-Tezerji R, Lipinski S, Kuiper JW, et al. Atg16l1 orchestrates interleukin-22 signaling in the intestinal epithelium via cgas–sting. J Exp Med (2018) 215(11):2868–86. doi: 10.1084/jem.20171029
169. Liu Y, Jesus AA, Marrero B, Yang D, Ramsey SE, Sanchez GAM, et al. Activated sting in a vascular and pulmonary syndrome. N Engl J Med (2014) 371(6):507–18. doi: 10.1056/NEJMoa1312625
170. Frémond ML, Hadchouel A, Berteloot L, Melki I, Bresson V, Barnabei L, et al. Overview of sting-associated vasculopathy with onset in infancy (Savi) among 21 patients. J Allergy Clin Immunol Pract (2021) 9(2):803–18.e11. doi: 10.1016/j.jaip.2020.11.007
171. Alexander M, Luo Y, Raimondi G, O'Shea JJ, Gadina M. Jakinibs of all trades: Inhibiting cytokine signaling in immune-mediated pathologies. Pharm (Basel) (2021) 15(1):48. doi: 10.3390/ph15010048
172. Kuhn R, Lohler J, Rennick D, Rajewsky K, Muller W. Interleukin-10-deficient mice develop chronic enterocolitis. Cell (1993) 75(2):263–74. doi: 10.1016/0092-8674(93)80068-p
173. Khan S, Mentrup HL, Novak EA, Siow VS, Wang Q, Crawford EC, et al. Cyclic gmp-amp synthase contributes to epithelial homeostasis in intestinal inflammation via beclin-1-mediated autophagy. FASEB J (2022) 36(5):e22282. doi: 10.1096/fj.202200138R
174. Grootjans J, Lenaerts K, Derikx JP, Matthijsen RA, de Bruïne AP, van Bijnen AA, et al. Human intestinal ischemia-reperfusion-induced inflammation characterized: Experiences from a new translational model. Am J Pathol (2010) 176(5):2283–91. doi: 10.2353/ajpath.2010.091069
175. Wang J, Zhang W, Wu G. Intestinal ischemic reperfusion injury: Recommended rats model and comprehensive review for protective strategies. BioMed Pharmacother (2021) 138:111482. doi: 10.1016/j.biopha.2021.111482
176. Kong C, Song W, Ren J, Zhou D, Li J, Xiang R, et al. Circulating mtDNA and impaired intestinal barrier after gastrointestinal surgery are correlated with postoperative SIRS. Genes (Basel) (2022) 13(11):1933. doi: 10.3390/genes13111933
177. Hu Q, Ren H, Ren J, Liu Q, Wu J, Wu X, et al. Released mitochondrial DNA following intestinal ischemia reperfusion induces the inflammatory response and gut barrier dysfunction. Sci Rep (2018) 8(1):7350. doi: 10.1038/s41598-018-25387-8
178. Hu Q, Ren H, Li G, Wang D, Zhou Q, Wu J, et al. Sting-mediated intestinal barrier dysfunction contributes to lethal sepsis. EBioMedicine (2019) 41:497–508. doi: 10.1016/j.ebiom.2019.02.055
179. Wu J, Liu Q, Zhang X, Wu X, Zhao Y, Ren J. Sting-dependent induction of lipid peroxidation mediates intestinal ischemia-reperfusion injury. Free Radic Biol Med (2021) 163:135–40. doi: 10.1016/j.freeradbiomed.2020.12.010
180. Yang M, Ma YX, Zhi Y, Wang HB, Zhao L, Wang PS, et al. Inhibitors of ifn gene stimulators (Sting) improve intestinal ischemia-Reperfusion-Induced acute lung injury by activating ampk signaling. Eur J Med Res (2022) 27(1):79. doi: 10.1186/s40001-022-00703-1
181. Zhang X, Wu J, Liu Q, Li X, Li S, Chen J, et al. mtDNA-STING pathway promotes necroptosis-dependent enterocyte injury in intestinal ischemia reperfusion. Cell Death Dis (2020) 11(12):1050. doi: 10.1038/s41419-020-03239-6
182. Kobritz M, Borjas T, Patel V, Coppa G, Aziz M, Wang P. H151, a small molecule inhibitor of sting as a novel therapeutic in intestinal ischemia-reperfusion injury. Shock (2022) 58(3):241–50. doi: 10.1097/shk.0000000000001968
183. Faria CP, Zanini GM, Dias GS, da Silva S, de Freitas MB, Almendra R, et al. Geospatial distribution of intestinal parasitic infections in rio de janeiro (Brazil) and its association with social determinants. PLoS Negl Trop Dis (2017) 11(3):e0005445. doi: 10.1371/journal.pntd.0005445
184. Verjee MA. Schistosomiasis: Still a cause of significant morbidity and mortality. Res Rep Trop Med (2019) 10:153–63. doi: 10.2147/rrtm.S204345
185. Gallego-Marin C, Schrum JE, Andrade WA, Shaffer SA, Giraldo LF, Lasso AM, et al. Cyclic gmp-amp synthase is the cytosolic sensor of plasmodium falciparum genomic DNA and activates type I ifn in malaria. J Immunol (2018) 200(2):768–74. doi: 10.4049/jimmunol.1701048
186. Wang P, Li S, Zhao Y, Zhang B, Li Y, Liu S, et al. The gra15 protein from toxoplasma gondii enhances host defense responses by activating the interferon stimulator sting. J Biol Chem (2019) 294(45):16494–508. doi: 10.1074/jbc.RA119.009172
187. Yilmaz IC, Dunuroglu E, Ayanoglu IC, Ipekoglu EM, Yildirim M, Girginkardesler N, et al. Leishmania kinetoplast DNA contributes to parasite burden in infected macrophages: Critical role of the cgas-sting-tbk1 signaling pathway in macrophage parasitemia. Front Immunol (2022) 13:1007070. doi: 10.3389/fimmu.2022.1007070
188. Sanchez Alberti A, Bivona AE, Matos MN, Cerny N, Schulze K, Weißmann S, et al. Mucosal heterologous prime/boost vaccination induces polyfunctional systemic immunity, improving protection against trypanosoma cruzi. Front Immunol (2020) 11:128. doi: 10.3389/fimmu.2020.00128
189. Souza C, Sanches RCO, Assis NRG, Marinho FV, Mambelli FS, Morais SB, et al. The role of the adaptor molecule sting during schistosoma mansoni infection. Sci Rep (2020) 10(1):7901. doi: 10.1038/s41598-020-64788-6
190. Liang L, Shen Y, Hu Y, Liu H, Cao J. Cgas exacerbates schistosoma japonicum infection in a sting-type I ifn-dependent and independent manner. PLoS Pathog (2022) 18(2):e1010233. doi: 10.1371/journal.ppat.1010233
191. Taniguchi T, Miyauchi E, Nakamura S, Hirai M, Suzue K, Imai T, et al. Plasmodium berghei anka causes intestinal malaria associated with dysbiosis. Sci Rep (2015) 5(1):15699. doi: 10.1038/srep15699
192. Denny JE, Powers JB, Castro HF, Zhang J, Joshi-Barve S, Campagna SR, et al. Differential sensitivity to plasmodium yoelii infection in C57bl/6 mice impacts gut-liver axis homeostasis. Sci Rep (2019) 9(1):3472. doi: 10.1038/s41598-019-40266-6
193. Yu X, Cai B, Wang M, Tan P, Ding X, Wu J, et al. Cross-regulation of two type I interferon signaling pathways in plasmacytoid dendritic cells controls anti-malaria immunity and host mortality. Immunity (2016) 45(5):1093–107. doi: 10.1016/j.immuni.2016.10.001
194. Spaulding E, Fooksman D, Moore JM, Saidi A, Feintuch CM, Reizis B, et al. Sting-licensed macrophages prime type I ifn production by plasmacytoid dendritic cells in the bone marrow during severe plasmodium yoelii malaria. PLoS Pathog (2016) 12(10):e1005975. doi: 10.1371/journal.ppat.1005975
195. Du Y, Luo Y, Hu Z, Lu J, Liu X, Xing C, et al. Activation of cgas-sting by lethal malaria N67c dictates immunity and mortality through induction of cd11b(+) ly6c(Hi) proinflammatory monocytes. Adv Sci (Weinh) (2022) 9(22):e2103701. doi: 10.1002/advs.202103701
196. Stuempfig ND, Seroy J. Viral gastroenteritis. In: Statpearls. Treasure Island (FL: StatPearls Publishing (2023).
197. Yang S, He Y, Zhang J, Zhang D, Wang Y, Lu X, et al. Viral metagenomics reveals diverse viruses in the fecal samples of children with diarrhea. Virol Sin (2022) 37(1):82–93. doi: 10.1016/j.virs.2022.01.012
198. Ding S, Diep J, Feng N, Ren L, Li B, Ooi YS, et al. Stag2 deficiency induces interferon responses via cgas-sting pathway and restricts virus infection. Nat Commun (2018) 9(1):1485. doi: 10.1038/s41467-018-03782-z
199. Stein SC, Falck-Pedersen E. Sensing adenovirus infection: Activation of interferon regulatory factor 3 in raw 264.7 cells. J Virol (2012) 86(8):4527–37. doi: 10.1128/jvi.07071-11
200. Kolawole AO, Mirabelli C, Hill DR, Svoboda SA, Janowski AB, Passalacqua KD, et al. Astrovirus replication in human intestinal enteroids reveals multi-cellular tropism and an intricate host innate immune landscape. PLoS Pathog (2019) 15(10):e1008057. doi: 10.1371/journal.ppat.1008057
201. Colemont LJ, Pen JH, Pelckmans PA, Degryse HR, Pattyn SR, Van Maercke YM. Herpes simplex virus type 1 colitis: An unusual cause of diarrhea. Am J Gastroenterol (1990) 85(9):1182–5.
202. diaAsano Y, Nakashima T, Yoshikawa T, Suga S, Yazaki T. Severity of human herpesvirus-6 viremia and clinical findings in infants with exanthem subitum. J Pediatr (1991) 118(6):891–5. doi: 10.1016/s0022-3476(05)82200-0
203. Schunter MO, Walles T, Fritz P, Meyding-Lamadé U, Thon KP, Fellermann K, et al. Herpes simplex virus colitis complicating ulcerative colitis: A case report and brief review on superinfections. J Crohns Colitis (2007) 1(1):41–6. doi: 10.1016/j.crohns.2007.06.004
204. Peacock JW, Bost KL. Infection of intestinal epithelial cells and development of systemic disease following gastric instillation of murine gammaherpesvirus-68. J Gen Virol (2000) 81(Pt 2):421–9. doi: 10.1099/0022-1317-81-2-421
205. Brun P, Conti J, Zatta V, Russo V, Scarpa M, Kotsafti A, et al. Persistent herpes simplex virus type 1 infection of enteric neurons triggers cd8(+) T cell response and gastrointestinal neuromuscular dysfunction. Front Cell Infect Microbiol (2021) 11:615350. doi: 10.3389/fcimb.2021.615350
206. Li XD, Wu J, Gao D, Wang H, Sun L, Chen ZJ. Pivotal roles of cgas-cgamp signaling in antiviral defense and immune adjuvant effects. Science (2013) 341(6152):1390–4. doi: 10.1126/science.1244040
207. Preveden T, Scarpellini E, Milić N, Luzza F, Abenavoli L. Gut microbiota changes and chronic hepatitis C virus infection. Expert Rev Gastroenterol Hepatol (2017) 11(9):813–9. doi: 10.1080/17474124.2017.1343663
208. Wang J, Wang Y, Zhang X, Liu J, Zhang Q, Zhao Y, et al. Gut microbial dysbiosis is associated with altered hepatic functions and serum metabolites in chronic hepatitis B patients. Front Microbiol (2017) 8:2222. doi: 10.3389/fmicb.2017.02222
209. Li Y, He M, Wang Z, Duan Z, Guo Z, Wang Z, et al. Sting signaling activation inhibits hbv replication and attenuates the severity of liver injury and hbv-induced fibrosis. Cell Mol Immunol (2022) 19(1):92–107. doi: 10.1038/s41423-021-00801-w
210. Wang J, Li F, Wei H, Lian ZX, Sun R, Tian Z. Respiratory influenza virus infection induces intestinal immune injury via microbiota-mediated th17 cell-dependent inflammation. J Exp Med (2014) 211(12):2397–410. doi: 10.1084/jem.20140625
211. Sencio V, Gallerand A, Gomes MaChado M, Deruyter L, Heumel S, Soulard D, et al. Influenza virus infection impairs the gut's barrier properties and favors secondary enteric bacterial infection through reduced production of short-chain fatty acids. Infect Immun (2021) 89(9):e0073420. doi: 10.1128/iai.00734-20
212. Holm CK, Rahbek SH, Gad HH, Bak RO, Jakobsen MR, Jiang Z, et al. Influenza a virus targets a cgas-independent sting pathway that controls enveloped rna viruses. Nat Commun (2016) 7:10680. doi: 10.1038/ncomms10680
213. Moriyama M, Koshiba T, Ichinohe T. Influenza a virus M2 protein triggers mitochondrial DNA-mediated antiviral immune responses. Nat Commun (2019) 10(1):4624. doi: 10.1038/s41467-019-12632-5
214. Li H, Zhou F, Zhang L. Sting, a critical contributor to Sars-Cov-2 immunopathology. Signal Transduct Target Ther (2022) 7(1):106. doi: 10.1038/s41392-022-00967-3
215. Domizio JD, Gulen MF, Saidoune F, Thacker VV, Yatim A, Sharma K, et al. The cgas-sting pathway drives type I ifn immunopathology in Covid-19. Nature (2022) 603(7899):145–51. doi: 10.1038/s41586-022-04421-w
216. Chiu CY, Sarwal A, Mon AM, Tan YE, Shah V. Gastrointestinal: Covid-19 related ischemic bowel disease. J Gastroenterol Hepatol (2021) 36(4):850. doi: 10.1111/jgh.15254
217. Wu X, Jing H, Wang C, Wang Y, Zuo N, Jiang T, et al. Intestinal damage in Covid-19: Sars-Cov-2 infection and intestinal thrombosis. Front Microbiol (2022) 13:860931. doi: 10.3389/fmicb.2022.860931
218. Varga Z, Flammer AJ, Steiger P, Haberecker M, Andermatt R, Zinkernagel AS, et al. Endothelial cell infection and endotheliitis in Covid-19. Lancet (2020) 395(10234):1417–8. doi: 10.1016/s0140-6736(20)30937-5
219. Xu E, Xie Y, Al-Aly Z. Long-term gastrointestinal outcomes of Covid-19. Nat Commun (2023) 14(1):983. doi: 10.1038/s41467-023-36223-7
220. Christensen MH, Jensen SB, Miettinen JJ, Luecke S, Prabakaran T, Reinert LS, et al. Hsv-1 icp27 targets the tbk1-activated sting signalsome to inhibit virus-induced type I ifn expression. EMBO J (2016) 35(13):1385–99. doi: 10.15252/embj.201593458
221. Sharma N, Wang C, Kessler P, Sen GC. Herpes simplex virus 1 evades cellular antiviral response by inducing microrna-24, which attenuates sting synthesis. PLoS Pathog (2021) 17(9):e1009950. doi: 10.1371/journal.ppat.1009950
222. Li T, Chen J, Cristea IM. Human cytomegalovirus tegument protein pul83 inhibits ifi16-mediated DNA sensing for immune evasion. Cell Host Microbe (2013) 14(5):591–9. doi: 10.1016/j.chom.2013.10.007
223. Fu YZ, Su S, Gao YQ, Wang PP, Huang ZF, Hu MM, et al. Human cytomegalovirus tegument protein ul82 inhibits sting-mediated signaling to evade antiviral immunity. Cell Host Microbe (2017) 21(2):231–43. doi: 10.1016/j.chom.2017.01.001
224. Ding Q, Cao X, Lu J, Huang B, Liu YJ, Kato N, et al. Hepatitis C virus ns4b blocks the interaction of STING and TBK1 to evade host innate immunity. J Hepatol (2013) 59(1):52–8. doi: 10.1016/j.jhep.2013.03.019
225. Hong Y, Jeong H, Park K, Lee S, Shim JY, Kim H, et al. Sting facilitates nuclear import of herpesvirus genome during infection. Proc Natl Acad Sci USA (2021) 118(33). doi: 10.1073/pnas.2108631118
226. Sun L, Wu J, Du F, Chen X, Chen ZJ. Cyclic gmp-amp synthase is a cytosolic DNA sensor that activates the type I interferon pathway. Science (2013) 339(6121):786–91. doi: 10.1126/science.1232458
227. Briard B, Place DE, Kanneganti TD. DNA sensing in the innate immune response. Physiol (Bethesda) (2020) 35(2):112–24. doi: 10.1152/physiol.00022.2019
228. Konno H, Konno K, Barber GN. Cyclic dinucleotides trigger ulk1 (Atg1) phosphorylation of sting to prevent sustained innate immune signaling. Cell (2013) 155(3):688–98. doi: 10.1016/j.cell.2013.09.049
229. Zhang ZD, Xiong TC, Yao SQ, Wei MC, Chen M, Lin D, et al. Rnf115 plays dual roles in innate antiviral responses by catalyzing distinct ubiquitination of mavs and mita. Nat Commun (2020) 11(1):5536. doi: 10.1038/s41467-020-19318-3
230. Hu MM, Yang Q, Xie XQ, Liao CY, Lin H, Liu TT, et al. Sumoylation promotes the stability of the DNA sensor cgas and the adaptor sting to regulate the kinetics of response to DNA virus. Immunity (2016) 45(3):555–69. doi: 10.1016/j.immuni.2016.08.014
231. Haag SM, Gulen MF, Reymond L, Gibelin A, Abrami L, Decout A, et al. Targeting sting with covalent small-molecule inhibitors. Nature (2018) 559(7713):269–73. doi: 10.1038/s41586-018-0287-8
232. Li Z, Liu G, Sun L, Teng Y, Guo X, Jia J, et al. Ppm1a regulates antiviral signaling by antagonizing tbk1-mediated sting phosphorylation and aggregation. PLoS Pathog (2015) 11(3):e1004783. doi: 10.1371/journal.ppat.1004783
233. Bodda C, Reinert LS, Fruhwürth S, Richardo T, Sun C, Zhang BC, et al. HSV1 VP1-2 deubiquitinates STING to block type I interferon expression and promote brain infection. J Exp Med (2020) 217(7):e20191422. doi: 10.1084/jem.20191422
Keywords: cGAS, STING, inflammatory bowel diseases, colorectal cancer, intestinal infections, intestinal ischemia/reperfusion
Citation: Yang Y, Wang L, Peugnet-González I, Parada-Venegas D, Dijkstra G and Faber KN (2023) cGAS-STING signaling pathway in intestinal homeostasis and diseases. Front. Immunol. 14:1239142. doi: 10.3389/fimmu.2023.1239142
Received: 12 June 2023; Accepted: 18 August 2023;
Published: 14 September 2023.
Edited by:
Chaofeng Han, Second Military Medical University, ChinaReviewed by:
Tianhao Duan, University of Southern California, United StatesGuijun Shang, Shanxi Academy of Advanced Research and Innovation, China
Copyright © 2023 Yang, Wang, Peugnet-González, Parada-Venegas, Dijkstra and Faber. This is an open-access article distributed under the terms of the Creative Commons Attribution License (CC BY). The use, distribution or reproduction in other forums is permitted, provided the original author(s) and the copyright owner(s) are credited and that the original publication in this journal is cited, in accordance with accepted academic practice. No use, distribution or reproduction is permitted which does not comply with these terms.
*Correspondence: Klaas Nico Faber, k.n.faber@umcg.nl