- 1School of Biological, Environment and Earth Sciences, The University of Southern Mississippi, Hattiesburg, MS, United States
- 2Department of Medicine and Pediatrics, University of North Carolina, Chapel Hill, NC, United States
Introduction: Alpha-Gal Syndrome (AGS) is a delayed allergic reaction due to specific IgE antibodies targeting galactose-α-1,3-galactose (α-gal), a carbohydrate found in red meat. This condition has gained significant attention globally due to its increasing prevalence, with more than 450,000 cases estimated just in the United States alone. Previous research has established a connection between AGS and tick bites, which sensitize individuals to α-gal antigens and elevate the levels of specific IgE. However, the precise mechanism by which tick bites influence the host’s immune system and contribute to the development of AGS remains poorly understood. This study investigates various factors related to ticks and the host associated with the development of AGS following a tick bite, using mice with a targeted disruption of alpha-1,3-galactosyltransferase (AGKO) as a model organism.
Methods: Lone-star tick (Amblyomma americanum) and gulf-coast tick (Amblyomma maculatum) nymphs were used to sensitize AGKO mice, followed by pork meat challenge. Tick bite site biopsies from sensitized and non-sensitized mice were subjected to mRNA gene expression analysis to assess the host immune response. Antibody responses in sensitized mice were also determined.
Results: Our results showed a significant increase in the total IgE, IgG1, and α-gal IgG1 antibodies titers in the lone-star tick-sensitized AGKO mice compared to the gulf-coast tick-sensitized mice. Pork challenge in Am. americanum -sensitized mice led to a decline in body temperature after the meat challenge. Gene expression analysis revealed that Am. americanum bites direct mouse immunity toward Th2 and facilitate host sensitization to the α-gal antigen.
Conclusion: This study supports the hypothesis that specific tick species may increase the risk of developing α-gal-specific IgE and hypersensitivity reactions or AGS, thereby providing opportunities for future research on the mechanistic role of tick and host-related factors in AGS development.
Introduction
Alpha-gal syndrome (AGS) is an atypical allergic reaction to galactose-α-1,3-galactose (α-gal), a disaccharide moiety on the end of the glycan present in all mammals except for catarrhine primates (1, 2). The enzymes responsible for producing these glycoconjugates are mainly found in the cells, tissues, and fluids of mammals, excluding humans, apes, and old-world monkeys (3–7). The deactivation of α-1,3-galactosyl transferase (α-1,3GT) in humans is believed to be the reason for developing an immune response to α-gal upon exposure to glycoconjugates containing α-gal antigens (8, 9). Deactivation of α-1,3GT gene in an ancestral Old-World species explains why humans, unlike other mammals, lack α-gal (1). As a result, the α-gal moiety becomes clinically significant because it triggers the production of anti-Gal antibodies in humans, including immunoglobulin M, A, and G (1, 10–12). AGS, in contrast, is caused by a specific immunoglobulin E (sIgE) antibody response in sensitized hosts directed against α-gal. It usually leads to allergic reactions 2-6 hours after consuming red meat or its derivatives (2, 8, 13–15). The synthesis of α-gal-containing glycoconjugates involves a diverse family of glycosyltransferase enzymes (16, 17).
Ticks are ectoparasites that can transmit various disease-causing pathogens, macromolecules, and other substances to humans (18–20). Numerous scientific studies conducted globally have provided evidence that establishes a link between tick bites and the development of AGS (13, 21–24). The rising prevalence of this emerging allergy has been observed in specific global regions, such as the United States (~450,000 estimated cases (25, 26), where the increased tick population and their migration to new areas present a significant public health issue (26–28). In certain major regions of the Southeastern U.S., it is estimated that up to 3% of the population has been affected by AGS, resulting in anaphylactic reactions (www.alphagalinformation.org, 2023). Furthermore, several other tick species worldwide, including Ixodes holocyclus in Australia, Ixodes ricinus and Rhipicephalus bursa in Europe, Hyalomma marginatum in Europe, Haemaphysalis longicornis in Japan, and Amblyomma sculptum in Brazil, have been identified as potential contributors to the development of AGS (9).
The precise mechanism by which tick bites sensitize humans and contribute to the development of AGS is not fully understood. It is hypothesized that tick saliva, which contains α-gal antigens and salivary components, may trigger a host immune response and skew the immune system toward a TH2 response, resulting in the production of IgE antibodies that target α-gal (23, 29, 30). In fact, repeated tick bites have been observed to enhance the existing specific IgE antibody response (21, 31–33). However, the relationship between glycosylated proteins containing α-gal in tick saliva and the process of α-gal sensitization or AGS induction in hosts requires further investigation, as these salivary factors may not be the sole determinant. It is worth noting that N-glycome profiling and proteome analysis have demonstrated the α-gal antigen in both salivary gland extracts and saliva of the lone-star tick (Am. americanum) and the black-legged tick (Ix. scapularis), while it is absent in Am. maculatum (29). Indeed, previous research has demonstrated exposure to Am. americanum salivary gland extracts can induce the development of AGS in an AGKO mouse model (30). Recently, a case-control study provided evidence of an 11-fold increased risk of AGS in human hosts reporting tick bites (34). Nevertheless, the specific conditions under which ticks or other exposures trigger sIgE antibody production against α-gal, resulting in AGS, remain unclear. Consequently, it is essential to further investigate the tick and host-related factors associated with AGS induction after tick bites. This study aims to explore the role of tick bites in AGS development using AGKO mice and nymphal ticks, as well as examine how tick bites influence the host’s immune response and contribute to AGS development.
Materials and methods
Ethics statement
All animal studies were conducted in strict accordance with the recommendations in the Guide for the Care and Use of Laboratory Animals of the National Institutes of Health, USA. The protocols for tick blood-feeding on mice and sheep were approved by the Institutional Animal Care and Use Committee (IACUC) of the University of Southern Mississippi (protocol #15101501.2, #19041801.2). All efforts were made to minimize animal distress and ensure their well-being throughout the procedures.
Ticks and other animals
The lone-star tick (Amblyomma americanum), hereafter Aa or LST, and Gulf-Coast tick (Amblyomma maculatum), hereafter Am or GST, were maintained at the University of Southern Mississippi according to established methods (35). Unfed lone-star ticks (Amblyomma americanum) were purchased from Oklahoma State University’s tick-rearing facility (Stillwater, OK, USA), and Ecto Services (Henderson, NC, USA). Immature and mature developmental stages of ticks were maintained at the University of Southern Mississippi using the previously described method (35). Ticks were kept at room temperature at approximately 90% humidity with a photoperiod of 14 hours of light and 10 hours of darkness before infestation on mice. The nymph ticks were fed on mice for biopsy tissue collection, depending on the experimental plan.
Mice
Alpha-1,3-galactosyltransferase knockout (AGKO) mice on C57BL/6 background were obtained from Dr. Anthony D’Apice (36). AGKO mice were bred and maintained in pathogen-free rooms under protocols approved by the University of Southern Mississippi, Animal Care and Use Committee (IACUC). Euthanasia was performed by anesthetizing animals with an intraperitoneal injection of 1.25% avertin (125-250 mg/kg body weight) followed by cervical dislocation.
Mice sensitization and food challenge
Eight- to ten-week-old AGKO mice were used for the sensitization experiment; control (n=10), Aa infestation (n=10), and Am infestation (n=6). During the sensitization experiment, mice were anesthetized by intraperitoneal injection of a 10 mg/kg ketamine/xylazine mixture, and 15 nymphal ticks (Am. americanum and Am. maculatum) were infested on a mouse ear days 0, 7, 21, 28 (Figure 1). Ticks were permitted to attach on the mice before being housed in individual cages with wire platforms above water to capture the engorged ticks. For repeat tick exposures in mice, ticks were allowed to feed till repletion and rested for an additional three days post-drop-off before the next challenge. Biopsy samples were collected during the partial blood-feeding phase of nymphs (3 days post infestation). Mouse blood was collected on days 3, 10, 24, and 31 to quantify total IgE, IgG, α-gal IgG, and α-gal sIgE titers. Mice sensitized to α-gal and control mice were orally challenged with 400 mg of cooked pork kidney homogenate (PKH) in phosphate-buffered saline. Pork kidney was prepared the method described earlier (30). Core body temperature was measured with a rectal probe (Fisher Scientific, USA), before and after the meat challenge every 15 minutes for 2 hours. Mice were subjected to repeated rectal probe insertion and were conditioned before the food challenge to mitigate temperature variation induced by the insertion of the rectal probe.
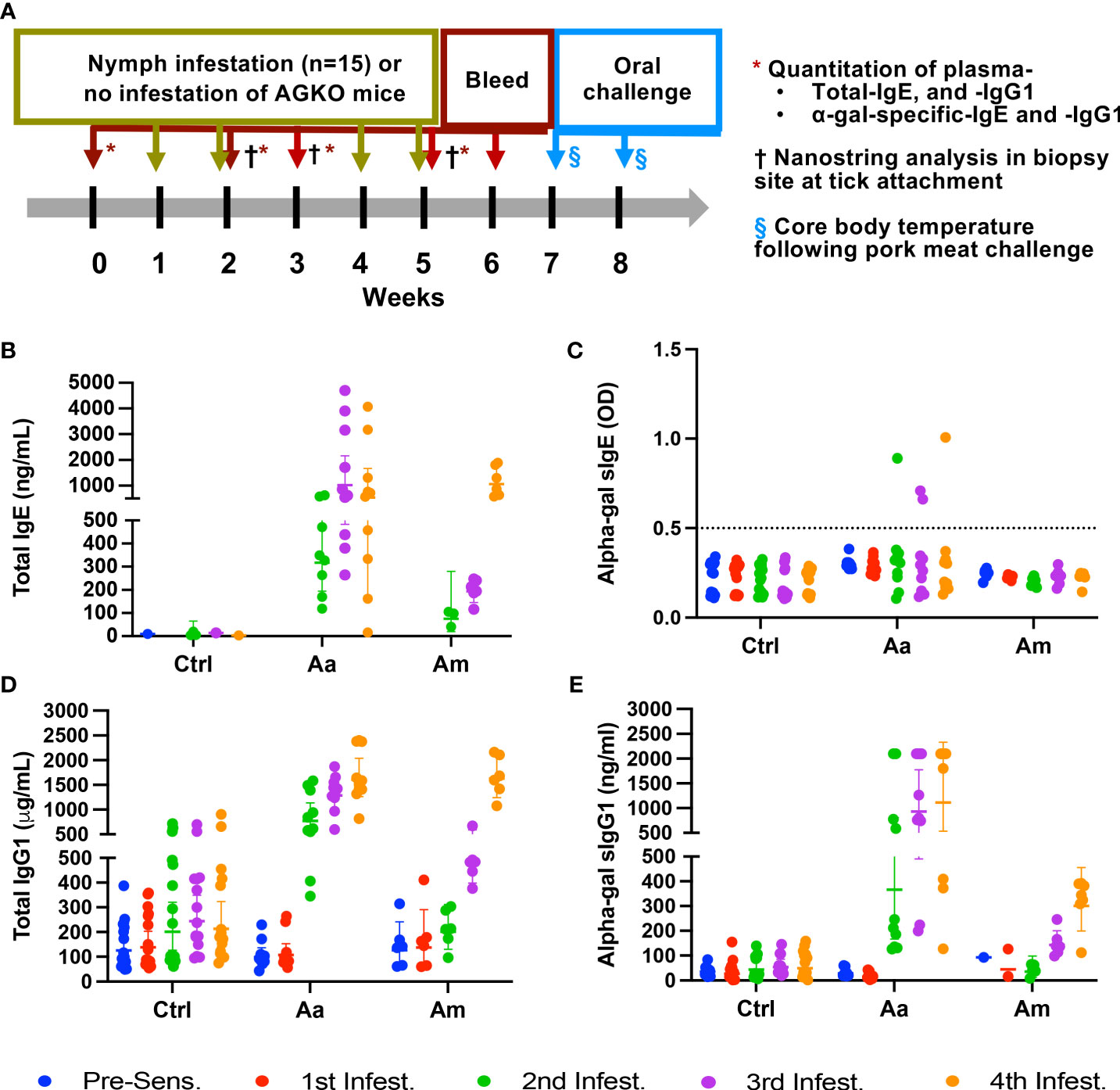
Figure 1 Sensitization of AGKO mice with tick nymphs. Schematics of tick-bite induced sensitization, collection of tick-bite site biopsy site for immune gene expression, and oral meat challenge (A). Quantitation of total IgE (B), alpha-gal specific IgE (C), total IgG1 (D), and alpha-gal specific IgG1 (E). Each dot represents an individual mouse and error bars represent the geometric mean with a 95% confidence interval. The dotted line shows a limit of detection of alpha-gal sIgE. Aa represents a group of mice (N=10) infested with Amblyomma americanum, Am represents a group of mice (N=6) infested with Amblyomma maculatum and Ctrl represents a group of mice with no infestation (N=16). Mice without nymph infestation exhibited untraceable total IgE, even at 60-fold plasma dilutions. IgE analysis plot includes six pooled replicate samples (from N=16) demonstrating IgE detection. The analysis plot for both total IgG1 and α-gal-specific IgG1 incorporated the examination of 10 replicate samples from control mice and those infested with Aa, along with 6 replicate samples from mice infested with Am. Fifteen nymphs were used per mouse in each round of infestation.
Quantitation of specific immunoglobulins
IgE was quantitated using the IgE Max Standard Set from Biolegend (San Diego, CA) according to the manufacturer’s instructions. Nunc Maxisorp plates were coated with 1X capture antibody or cetuximab (20 µg/mL) in carbonate-bicarbonate coating buffer to quantitate total IgE and α-gal IgE respectively. Briefly, plates were coated overnight at 4°C, received four washes with PBS containing 0.05% Tween 20 (PBST; Sigma-Aldrich) and were blocked with 1% BSA in PBST for 90 minutes (min). Plasma samples (1:60 dilution for total IgE, 1:2 dilution for α-gal specific IgE) or standard were added to the plate and incubated for 2 hours (h) at room temperature (RT). Samples were incubated with 1X detection antibody for 1 h and avidin-HRP at RT in the dark for 30 min. 3,3’,5,5’-Tetramethylbenzidine (TMB) Peroxidase Substrate and Stop Solution (KPL, Gaithersburg, MD) was used to develop an enzymatic colored reaction. To quantitate IgG1 and α-gal sIgG1, plates were coated with capture antibody (goat anti-mouse IgG1, 1 μg/L, SouthernBiotech) or cetuximab (20 µg/mL) respectively in carbonate-bicarbonate coating buffer overnight at 4°C. Plates received four washes with PBST and were blocked with 3% fetal bovine serum (FBS) in PBST. Plasma samples (1:20,000 dilution in PBS containing 1% FBS for total IgG, 1:10 dilution for α-gal specific IgG) were incubated for 90 min at RT, and ELISAs were detected with HRP-conjugated goat-anti-mouse IgG1-HRP (Southern Biotech). To develop the enzymatic reaction TMB was used as described above. Plates were read on an Epoch Microplate Spectrophotometer (BioTek Instruments, Winooski, VT) and analyzed using Gen5 software. Four-parameter logistic regression was used to calculate the unknown concentration using the standard concentration from 80 ng - 0.312 ng with two-fold serial dilution for IgE and 200 ng - 0.3906 ng for IgG1. Our limit of detection at the above-mentioned dilutions of unknown samples was 4 ng for total IgE and 7 μg for total IgG1. Antibody titer data further were analyzed using GraphPad Prism 9 (La Jolla CA). The two-way ANOVA with a mixed-model analysis and Tukey’s multiple comparison test was performed for statistical significance involving more than two groups while the Mann-Whitney test was performed for a single comparison.
Skin biopsies, RNA Extraction, NanoString immunological assays, and analysis
Mice were anesthetized by intraperitoneal injection of a 10 mg/kg ketamine/xylazine mixture. Biopsy samples using 3 mm biopsy punches (Miltex, USA) were collected at the tick bite sites (1 site/animal) and stored in RNAlater Stabilization Solution (ThermoFisher, Waltham, MA, USA). During the partial feeding phase of ticks (3 days after infestation of mice), biopsy samples were obtained at the 1st, 2nd, and 3rd sensitization stages. To extract RNA from the skin biopsies, the RNeasy Plus kit (specifically, the Quick RNA miniprep plus kit, Zymo, USA) was utilized and RNA was quantitated with Qubit RNA HS (High Sensitivity) assay kit (Invitrogen, Waltham, MA). For the NanoString nCounter assay, 100 ng of RNA in 5 μL per sample was used for the Gene Expression Panel/CodeSet Hybridization protocol and each cartridge was hybridized for 18 hours on a thermal cycler set to 65°C with a lid heated to 70°C. Once hybridization was complete, cartridges were moved to a nCounter Prep station and processed using the High Sensitivity protocol and then analyzed on the nCounter Pro Analysis System (NanoString Technologies, Seattle, WA, USA). NanoString Data was further analyzed by ROSALIND® (https://rosalind.bio/), with a HyperScale architecture developed by ROSALIND, Inc. (San Diego, CA). Read Distribution percentages, violin plots, identity heatmaps, and sample MDS plots were generated as part of the QC step. Normalization, fold changes, and p-values were calculated using criteria provided by Nanostring. ROSALIND® follows the nCounter® Advanced Analysis protocol of dividing counts within a lane by the geometric mean of the normalizer probes from the same lane. Housekeeping probes to be used for normalization are selected based on the geNorm algorithm as implemented in the NormqPCR R library1. An abundance of various cell populations is calculated on ROSALIND using the Nanostring Cell Type Profiling Module. ROSALIND performs a filtering of Cell Type Profiling results to include results that have scores with a p-value greater than or equal to 0.05. Fold changes and p-values are calculated using the fast method as described in the nCounter® Advanced Analysis 2.0 User Manual. P-value adjustment is performed using the Benjamini-Hochberg method of estimating false discovery rates (FDR).
Data availability
The data presented in this study are deposited in the NCBI GEO repository, accession number GSE249650 (GSM7956829-64).
Results
Bites of Aa but not Am nymphs cause a robust production of IgE, IgG1 and α-gal-directed sIgG1 in an Alpha-gal KO mice mouse model
Pruritic reactions at the site of tick bites correlate with increased production of α-gal sIgE in humans, implying that sensitization to tick bites containing α-gal epitope or α-gal containing proteins as foundin saliva may require repeated tick bites (29, 32, 37). We used nymphs of two tick species- Aa, known to secrete α-gal containing epitopes in the saliva, and Am, deficient in epitopes containing α-gal. Tick bite increased total and specific IgE and IgG1 production following sensitization (N=15 ticks infested, in an average total 7, ticks fed to repletion and dropped off per infestation) in AGKO mice (Figure 1A). In control mice with no nymph infestation, total IgE was not detected in several mice at 60-fold dilutions of plasma (data not shown). We, therefore, pooled the plasma samples across all four control infestations and measured total IgE (geometric mean (GM) = 7.44 ng/mL (n=6) with a 95% confidence interval (CI) of 3.44 to 16.06 ng/mL; Figure 1B, Table 1). In mice with Aa and Am nymphs infested, total IgE increased significantly following the second infestation compared to the control. Notably, the increase in total IgE was 4.2-fold higher in Aa-infested mice than in Am-infested mice (p<0.05) and peaked following the third infestation with Aa (GM = 1022.53 ng/mL, CI 483.45 to 2162.75) and was significantly higher than Am-infested mice (GM = 194.16, CI 145.61 to 258.90). No significant difference in total IgE between Aa- and Am-infested mice was noted following the fourth infestation. We further detected α-gal specific IgE (sIgE) in three out of ten Aa-infested mice (Figure 1C). In contrast, none of the Am-infested mice produced α-gal sIgE. An increase in IgG1 is associated with Th2 polarization and anaphylaxis in mice (38, 39). We, therefore, quantitated IgG1 and observed increased titer following tick infestation in both Aa- and Am-infested mice (Figure 1D, Table 1). Total IgG1 concentration following second and third infestations was significantly higher in Aa-infested mice (GM = 769.57, CI 522.06-1134.41 and GM = 1286.43, CI 1021.30- 1620.40) in comparison to both Am-infested (GM = 200.94, CI 129.67-311.37 and 484.3, CI 397.86-589.53) and control (GM = 201.19, CI 126.38-320.28 and GM = 243.81, CI 170.48-348.69) mice. In contrast to the Aa-infested mice, the increase in total IgG1 titer remained low in Am-infested mice until the fourth infestation compared to control cohorts. Notably, α-gal specific IgG1 (sIgG1) was 13.1-fold, 6.5-fold, and 3.7-fold higher in Aa-infested mice than Am-infested mice following second, third, and fourth infestation, respectively; and these differences were significant (Figure 1E, Table 1).
Tick bite-sensitized mice exhibit a drop in core body temperature after the red meat challenge
We have previously reported that oral challenge of sensitized mice with pork kidney causes a more consistent and faster reaction (less than 2 hours) due to the high content of α-gal in heavily glycosylated proteins such as angiotensin I-converting enzyme (ACE I) and aminopeptidase N (AP-N) found in pork kidney (30). We, therefore, challenged tick bite-sensitized AGKO mice with 400 mg of cooked pork kidney homogenate (PKH) orally to study the allergic response to α-gal. Body temperature decline was measured every 15 minutes over two hours with a rectal thermometer. A drop in mean body temperature between 1.5-3.0°C was taken as a sign of mild anaphylaxis and below 3.0°C as severe anaphylaxis (40). In Aa-infested mice, severe anaphylaxis was noted at 30 min after PKH with a mean temperature decline of 5.8°C. It was significantly different from control mice or Am-infested mice (Figure 2). The body temperature reached its nadir 60 minutes after the challenge, and the mice showed symptoms of reduced activity and labored breathing (data not shown). In contrast, no significant decline in body temperature was observed in control and Am-infested mice, where a drop in the body temperature was less than 1.5°C following the PKH challenge. Our results suggest that infestation with Aa but not Am causes anaphylaxis in AGKO mice.
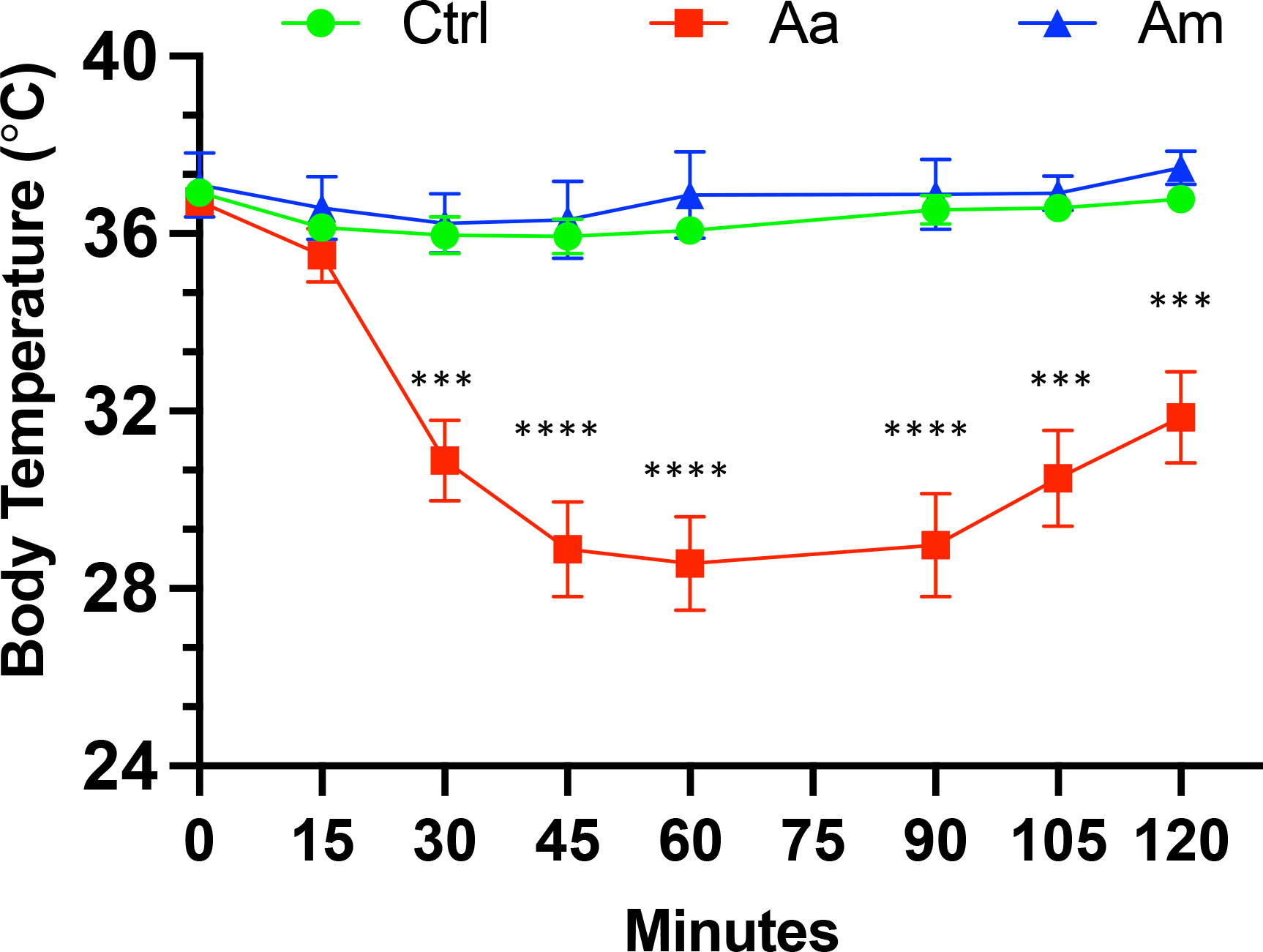
Figure 2 Anaphylaxis in tick infested mice following post-oral challenge with 400 mg of cooked pork kidney homogenates. Body temperature was recorded at baseline and post-oral challenge and a drop in body temperature >3 was considered as a sign of severe anaphylaxis. The two-way ANOVA with Tukey’s multiple comparison test was performed for statistical significance; *** P<0.001; **** P<0.0001. Aa, Amblyomma americanum; Am, Amblyomma maculatum; Ctrl, no infestation.
AGKO mice sensitization using repeat tick infestation and nymph engorgement rate
To investigate whether repeated tick exposure in AGKO mice leads to acquired tick resistance (ATR) and host rejection, we conducted an experiment involving sensitizing ticks to AGKO mice through four repeated infestations and determined the total weight of nymphs dropped off after each infestation. The engorgement data indicated that repeated exposure to Aa ticks resulted in a small but significant decline in nymph body weight following the second infestation. The Aa nymph body weight decline peaked following the third infestation; no further reduction was noted at the fourth infestation (Figure 3A). In contrast, no significant impact on tick engorgement was observed in Am nymphs (Figure 3B).
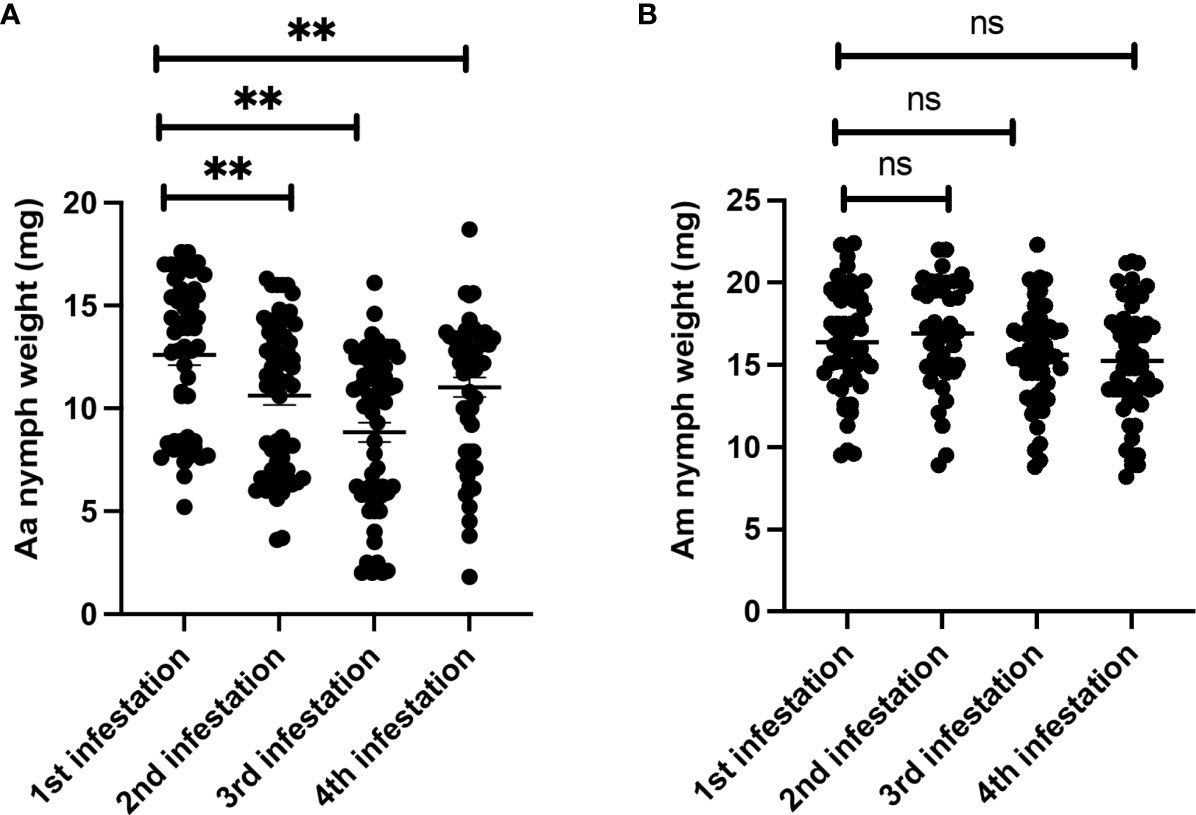
Figure 3 Repeated sensitization of AGKO mice via nymph tick bite and analysis of tick engorgement (A) Engorgement weight of Amblyomma americanum nymph tick recovered during different stage of sensitization of AGKO mice (N=5). (B) Engorgement weight of Amblyomma maculatum nymph tick recovered during different stage of sensitization of AGTKO mice (N=5). Statistical test-students t-test. **, p<0.01, ns not significant.
Differential gene expression at tick bite site following 1st, 2nd, and 3rd infestation of Aa nymphs
Skin biopsy was performed at the site of Aa tick attachment; RNA was extracted and quantitated as described in the Methods section. The Mouse Host Response Panel supplemented with primers for Bach2, Clec7a, Ighg1, Mmp13, Mmp8, Rorc, S100a, Sp140, TSLP, and Ybx3, was used for expression profiling of 783 genes via the digital multiplexed NanoString™ nCounter analysis system. Data were analyzed using the Rosalind NanoString™ Gene Expression platform. A sample correlation heatmap was drawn in which the data matrix contains correlation values between samples, with the darkest blue representing the strongest correlation (Figure 4A). Replicates of tick-infested mice strongly correlated; however, some correlation was also observed between control and 3/18 tick-infested samples. Fold change and p-values were calculated using the fast method, and p-value adjustment was performed using the Benjamini-Hochberg method of estimating false discovery rates (FDR). Setting a filter at fold change to ≥ 1.5 and ≤ -1.5 and p-adj to 0.05, first-, second-, and third-tick infestation resulted in gene expression change of 283 (162 upregulated, 111 downregulated), 158 (122 upregulated, 36 downregulated, and 313 (248 upregulated, 65 downregulated) genes respectively. When data were compared for all three tick infestations versus control, 329 genes were differentially expressed, of which 237 were upregulated, and 92 were downregulated. Volcano plot and heat map (Figures 4B, C) show that data with a fold change of ≥2.0 and ≤ -2.0 and p-adj to 0.01 that identified 169 upregulated and 53 downregulated genes. A list of differentially expressed genes with values is provided in Supplementary Table S1.
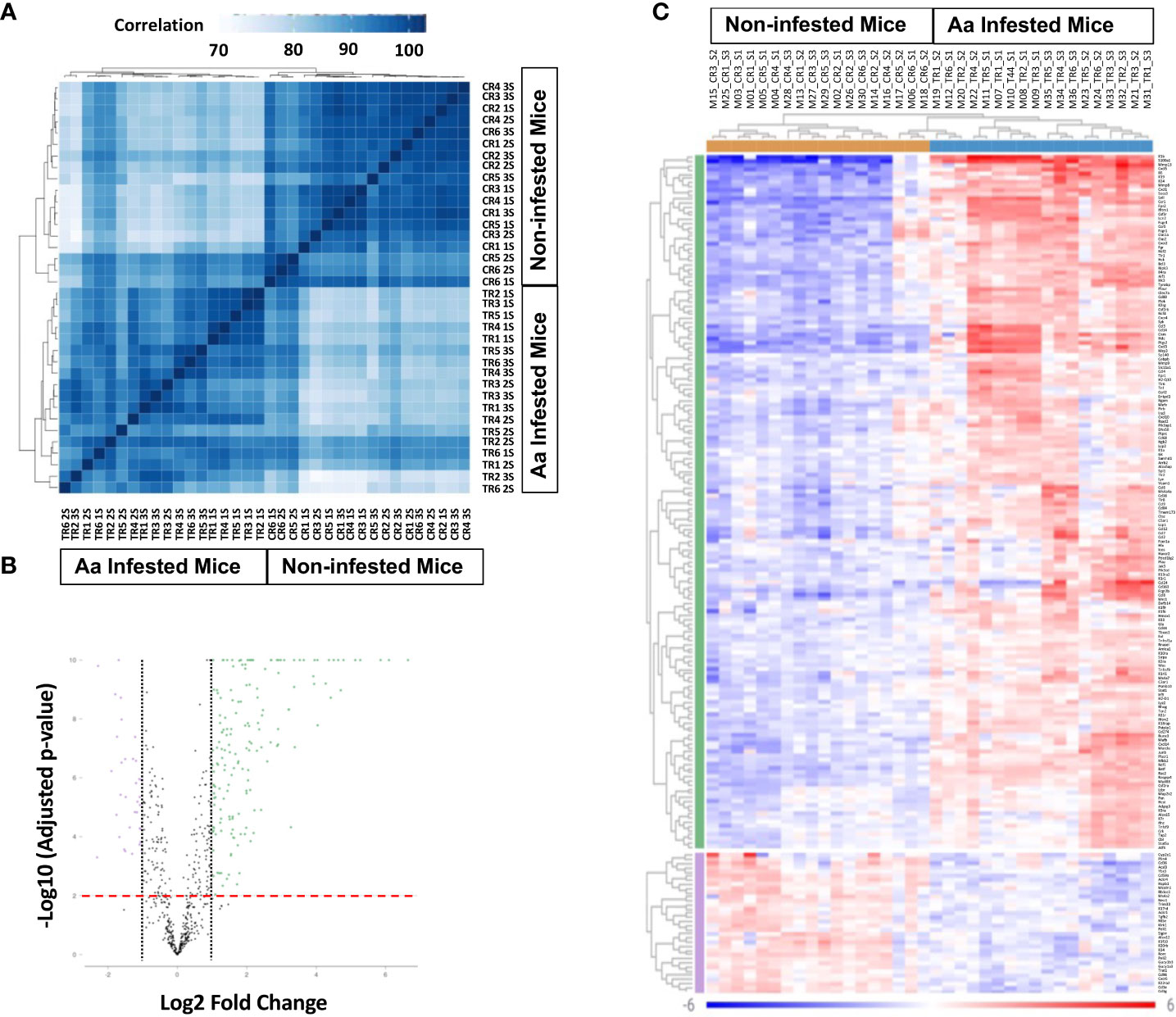
Figure 4 Differential gene transcription in biopsy site at tick attachment. (A) Sample correlation heatmap. (B) Volcano plot with dotted line displays mean gene expression fold change ≥ 2.0 and ≤ -2.0 between tick-infested mice and control infestation. The dashed red line shows where adjusted p value = 0.01. (C) Heat map of genes displaying two-fold change and p-Adjusted value ≥0.01. The legend displays mapping to row-wise Z-scores. Aa, Amblyomma americanum; 1S, first sensitization; 2S, second sensitization; 3S, third sensitization; TR1-TR6, Aa infested mice; CR1-CR6, non-infested mice.
Regulation of chemokine, cell adhesion, vascular permeability, and leucocyte migration
Cell type profiling was based on the NanoString™ Cell Type Profiling Module and filtered using Rosalind to include data with a p-value < 0.05 demonstrating that monocytes and NK56dim cells were predominantly detected at the site of tick attachment in comparison to no tick infestation (Figure 5A). In keeping with this, we observed an upregulation of monocyte chemokine genes Ccl3 and Ccl4 (Figure 5B). The induction of Ccl3 (35-fold) and Ccl4 (21-fold) peaked following the 1st tick attachment with a concomitant increase in their receptor, CCR1. Other CC chemokines (Ccl2, Ccl7, Ccl8, Ccl12, Ccl24) were also robustly induced; however, expression of these peaked following additional tick infestations. The induction of Cxcl1(12-fold), Cxcl3 (64-fold), and Cxcl10 (6-fold) peaked following the 1st tick infestation, while Cxcl5 expression rose 39-fold following the 3rd tick infestation. Gene sets associated with tissue damage and inflammation were induced in tick-attached mice. Sell, which encodes the adhesion molecule L-selection, was highly upregulated following tick infestation (Figure 5C). Plasminogen activator Plau and plasminogen activator Plaur, and the matrix metallopeptidases Mmp8, Mmp9, and Mmp13 were all induced several folds (Figure 5D). Furthermore, we observed increased transcription of Ptgs2, a key enzyme in prostaglandin biosynthesis and associated receptor Ptger4 and bradykinin receptor Bdkrb1.
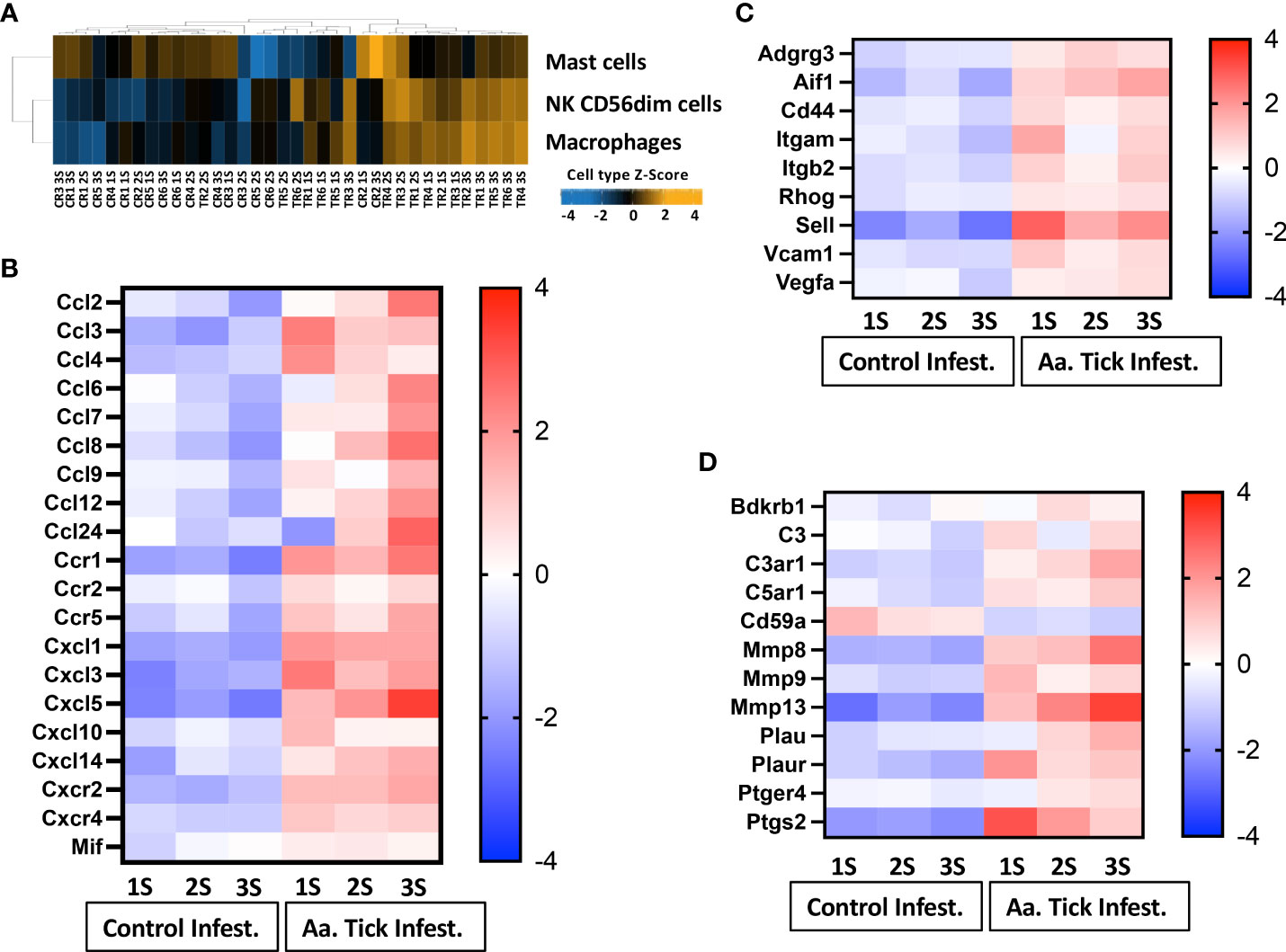
Figure 5 Heat map representation of differentially transcribed genes involved in cell migration and recruitment of leucocytes to tick-infestation sites. (A) Cell population present on site of tick attachment based on gene characteristics determined by NanoString cell type profiler. (B) Chemokine and chemokine receptors, (C) Cell adhesion, vascular permeability and leucocyte migration. (D) Plasminogen and complement activation. Aa, Amblyomma americanum; 1S, first sensitization; 2S, second sensitization; 3S, third sensitization.
Induction of cytokines, interferons, inflammasome and other inflammatory mediator genes
Infestation of mice with ticks resulted in a massive induction of genes involved in IL-1β secretion and activation at the first timepoint (IL-1β increased 831-fold; Figure 6A). Induction of IL-1β coincided with upregulation of pattern recognition receptors (PRR) of distinct classes which included the following: (i) Toll-like receptors (TLR) members Tlr1, Tlr2, Tlr5, Tlr6 and Tlr8 (ii) Nod-like receptor (NLR) member Nod2 and C-type lectin receptor (CLR) member Clec7a (Figure 6B). CD14, which is also a co-receptor for bacterial lipopolysaccharide (LPS) was induced 20-fold (41). MyD88 transcripts were induced, and this critical member of TLR signaling is involved in both NF-kB and AP-1 activation (42). Downstream transcription factors Map2k2, Mapkapk2, Batf and Junb, involved in the nuclear translocation of AP-1 and Nfkb2, Nfkbia and Bcl3, which activate NF-kB, were all induced (Figure 6C). Furthermore, upregulation of Tnfα (six-fold), and its receptor Tnfrsf1a were noted, which could activate NF-κB downstream targets as well. Induction of pro-IL-1β by both NF-kB and MAPK pathways was supported by NOD [nucleotide oligomerization domain]-, LRR [leucine-rich repeat]-, and PYD [pyrin domain]-containing protein 3 (NLPR3) inflammasome activation; transcription of Nlrp3 was induced 45-fold following the 1st infestation of mice with ticks (Figure 6B) (43–45).
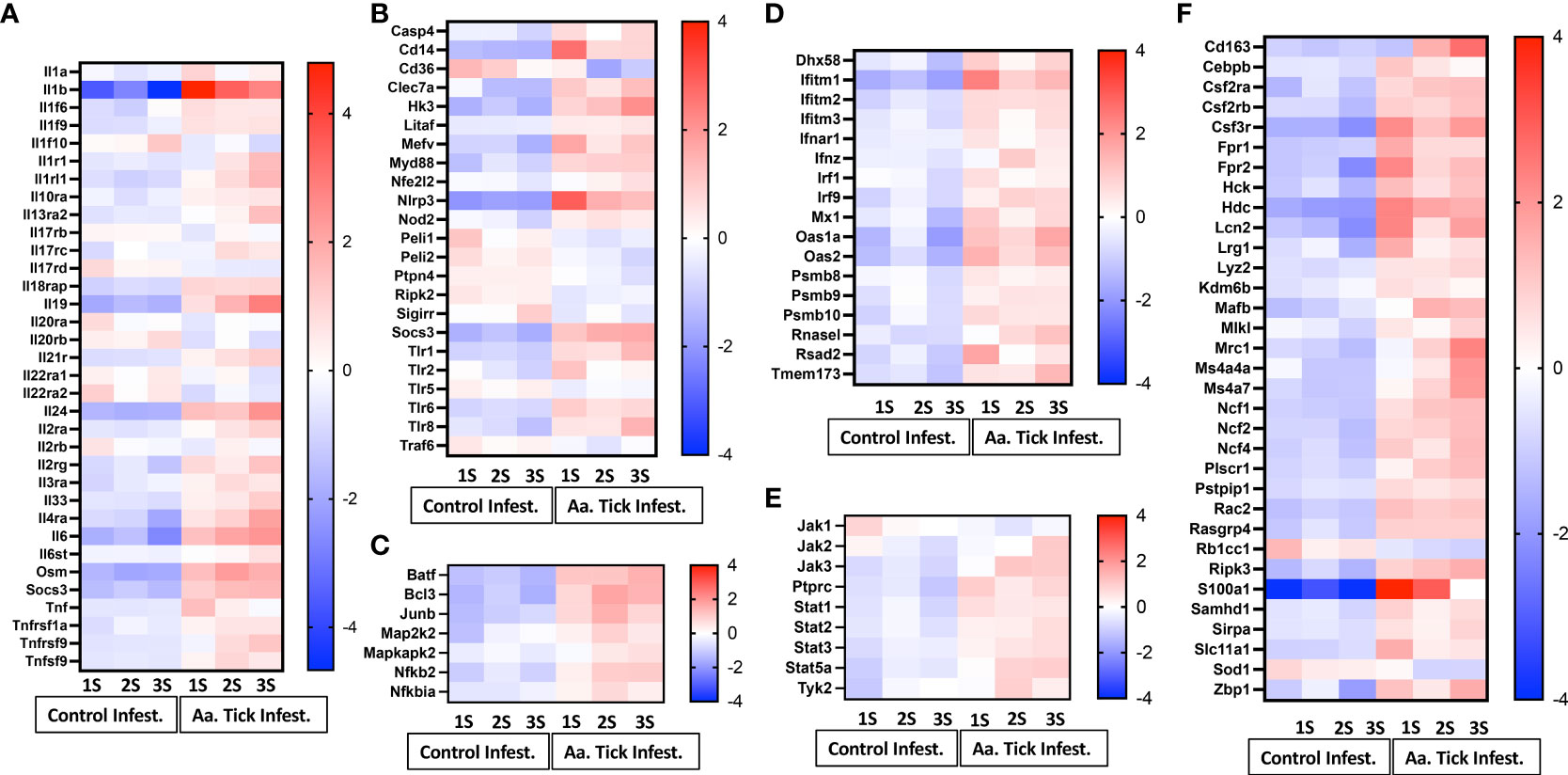
Figure 6 Heat map representation of differentially transcribed genes involved in inflammatory and anti-inflammatory responses. (A) Cytokines and cytokine receptors. (B) Pattern recognizing receptor (PRR), toll-like receptor (TLR) and inflammasome activation. (C) MAPK and NF-κB signaling pathway. (D) Interferon and antiviral responses. (E) JAK-STAT signaling pathway. (F) Other inflammatory response genes.
Several negative modulators of TLR signaling were induced, which would lead to inhibition of both the signaling complex formation of pro-IL-1β transcription as well as inflammasome inactivation (Figure 6B). Traf6, which is involved in both MyD88-dependent and TRIF-dependent TLR signaling pathways was downregulated, whereas Socs3, which suppresses the activation of the MyD88-dependent pathway, was upregulated. The IL-20 subfamily of the IL-10 cytokine family elicits innate defense mechanisms from epithelial cells against extracellular pathogens (46). Following the first tick infestation, two members of this subfamily Il19 and Il24 were induced and further increased following more tick infestations (Figure 6A). Il6 and Osm, which belong to the IL-6 cytokine family, were induced following the first tick infestation and their transcription increased following subsequent tick infestation along with their receptor Il6st (Figure 6A).
Consistent with the induction of PRR and TLR-mediated signaling, all three members of the CD225 superfamily of interferons: Ifitm1, Iftim2, and Ifitm3 were upregulated (Figure 6D) (47). JAK/STAT signaling pathway is critical to cytokine-receptor signaling and induces key mediators of inflammations (48). Tick infestation resulted in the upregulation of Stat1, Stat2, Stat3 and Stat5a (Figure 6E). Three members of the Janus kinase family Jak2, Jak3 and Tyk2 were upregulated while Jak1 was downregulated. During an inflammatory state, common β- chain cytokines such as GM-CSF, IL-3 and IL-5 further regulate the inflammatory response in a cell- and tissue-specific manner. We observed an increase in the transcription of Csf2ra, Csf2rb and Csf3r of GM-CSF and G-CSF which promote the generation of granulocytes and antigen-presenting cells (Figure 6F). Neutrophils and monocytes are the first leucocytes to be recruited to an inflammatory site. Transcripts of Fpr1 and Fpr2, the high-affinity receptors for N-formyl-methionyl peptides (fMLP), a powerful neutrophil chemotactic factor, were upregulated (49).
Antigen presentation and Th2 polarization
Following the second infestation of mice with ticks, indicators of a switch in the immune response from an innate to an adaptive response were present. Cd6, which expresses a costimulatory molecule to promote T-cell activation in response to PRR, was induced (Figure 7A) (50). Several MHC class I transcripts, including H2-D1, H2-K1, H2-M3, H2-Q10, and H2-T23, were upregulated as well. Rorc was downregulated and, as a suppressor of IL-2 in mice, this decrease would lead to further T cell activation (51). Importantly, the transcription factor Foxp3 was significantly induced following the 2nd infestation, consistent with a Treg response to suppress inflammation (Figure 7B) (52). No significant change in gene expression was observed for Th1 transcription factor Tbx21 (Figure 7C) (53). MHC class II molecules are involved in Th2 response, and Cd209e, which is involved in dendritic cell-mediated APC, was induced (Figure 7A) (54). Further, the transcript for cathepsin, Ctss, involved in removing the invariant chain from MHC class II molecules and MHC class II antigen presentation was upregulated (55). Several receptors and costimulatory molecules, such as Icos, Cd40, Cd40lg, and Cd80 were induced for efficient antibody response to T-cell-dependent antigens (Figure 8A). Cd84, which prolongs T-cell: B-cell contact, optimal T follicular helper function, and germinal center formation, was induced (56). In keeping with the switch to an adaptive response, induction of Il6 was observed that supports the differentiation of B cells into immunoglobulin-secreting cells as well as the development of T follicular helper (Tfh) cells (57, 58). Cytokines IL-4, IL-13, IL-21, and IL-33 are involved in different aspects of immunoglobulin secretion, such as Th2 differentiation, generation of Tfh cells, and formation of the germinal center (58–61). We observed an increase in transcripts of the pro-Th2 cytokine Il33 and its receptors Il1rl1, and Il4 and its receptors Il4ra, Il21r, and Il13ra2 (Figures 8A–G). Transcription of Il21 was significantly induced following the 2nd tick infestation; however, no induction was observed for Il13 (data not shown). Maf, which activates the expression of IL4 in Th2 cells, Pik3ap1, which contributes to PI3K-Akt-mediated BCR signaling, and Pik3cd, which mediates proliferative of B cells in response CD40 and IL-4 stimulation, were all induced (62–64). Consistent with the induction of Th2 differentiation genes, increased expression of the transcripts of Fc receptors such as Fcer1a, Fgr1, Fcgr2bm, Fcgr4, and Ms4a2 were observed. Furthermore, increased gene expression of Alox15, Atf4, and Xbp1, involved in ER stress response and immunoglobulin secretion, was observed (65). Our data suggest that Th2 differentiation and immunoglobulin production predominate the immune response following the 2nd tick infestation.
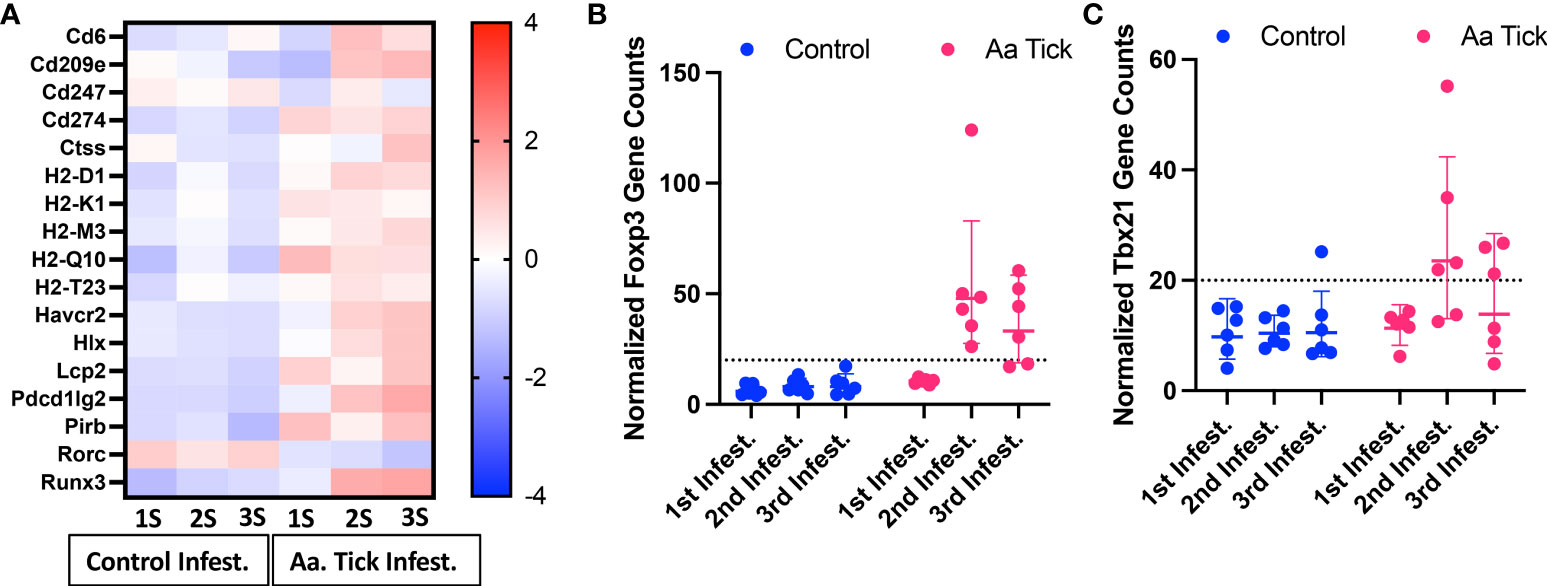
Figure 7 Modulation of genes involved in antigen presentation and Th1 response. (A) Heat map showing representation of differentially transcribed genes involved in antigen presentation. (B, C) Dot plots showing transcript counts of Treg transcription factor Foxp3 and Th1 transcription factor Tbx21. Each dot represents an individual mouse. The dotted line shows the limit of detection.
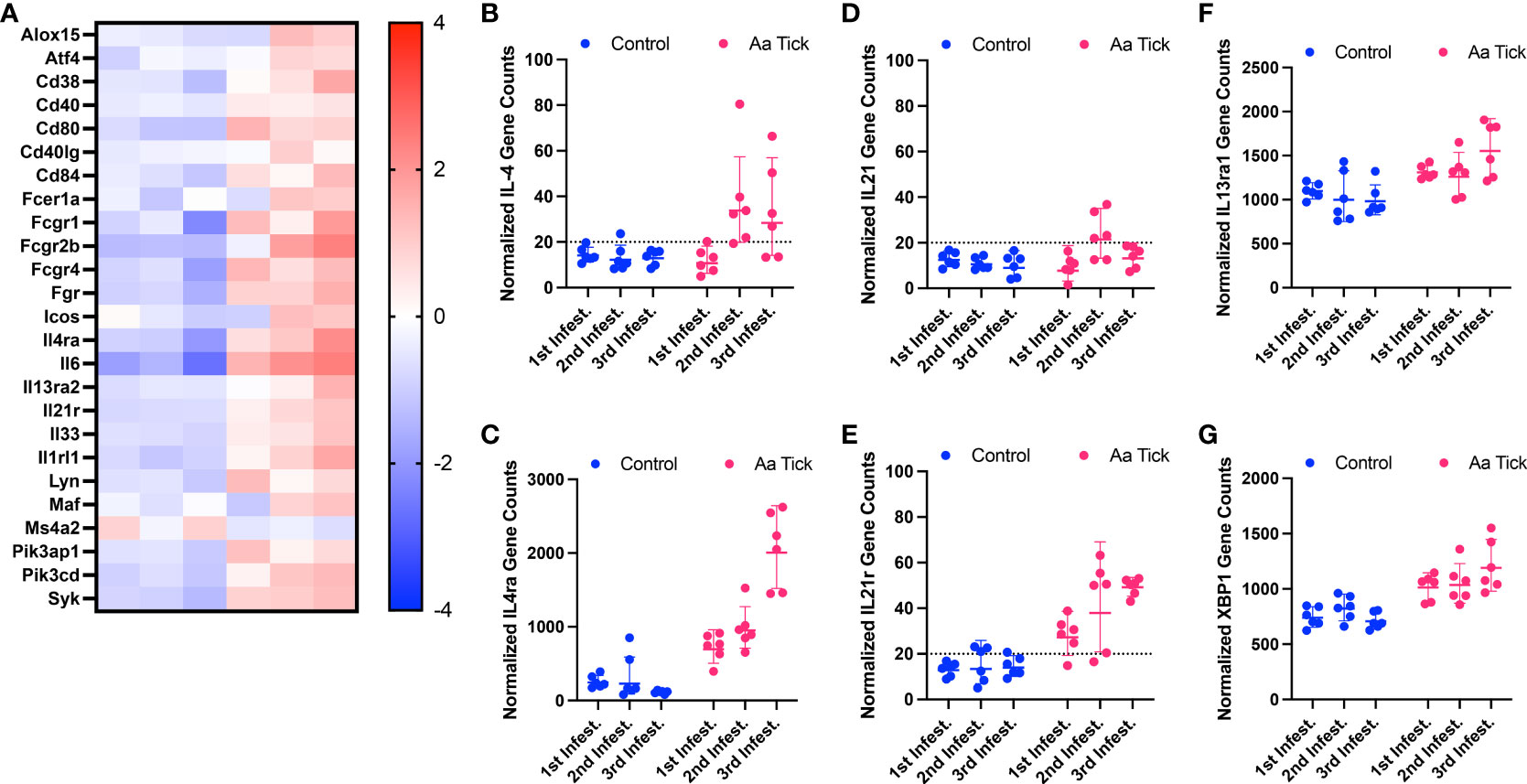
Figure 8 Modulation of genes involved in B cell signaling and Th2 response. Heat map showing representation of differentially transcribed genes involved in B cell signaling and Th2 response (A). Dot plot showing differential transcription of cytokine IL-4 and its receptor (B, C), cytokine IL-21 and its receptor (D, E), cytokine IL-13 receptor (F) and ER stress response factor XBP-1 (G). Each dot represents an individual mouse. The dotted line shows the limit of detection.
Discussion
The current study reports the first small animal model that utilizes live, attached immature developmental stage of ticks to induce IgE immune response against tick bite and AGS as observed in humans. Through this, we tested the hypothesis that lone-star tick or Aa bite induces a Th2 immune response in the mammalian host, reorienting the immune system to produce IgE antibodies responsible for AGS. We compared immune-related gene expression profiles of the tick-sensitized and unsensitized AGKO murine samples to identify relevant pathways involved in the IgE response following tick bites. Based on AGS patient tick bite histories, tick species prevalence, in the regions with high AGS incidences, it is thought that α‐gal sIgE develops in humans after being bitten by certain tick species. For instance, Am. americanum is associated with this response in the United States, while in Australia, Europe, Japan, and Brazil, Ixodes holocyclus, Ixodes ricinus, Haemaphysalis longicornis, and Amblyomma sculptum, respectively, have been implicated in AGS (9, 21, 29, 66, 67).
Interestingly, epidemiologic surveillance studies have found a correlation between the geographic distribution of Aa ticks, high titer of α‐gal sIgE antibody in AGS patients (8, 34). Beyond epidemiologic evidence from a recent case-control study, no direct and definitive evidence of tick bite induced AGS exists in humans. In keeping with this, the mechanisms by which Aa tick bites induce high titer sIgE production and initiate delayed allergic response after exposure to red meat in humans are poorly understood. Among available allergy mouse models, we and others have previously utilized an AGKO mouse model, which mimics humans as an “alpha‐gal‐deficient host” and reported the induction of α-gal sIgE following immunization using partially fed salivary gland extracts and larval protein extracts with adjuvants, respectively (30, 68). These sensitization methods have their own set of limitations. For example, employing tick salivary extract or larval homogenate for sensitization permits the utilization of salivary protein extracts and offers evidence suggesting ticks’ recognition of their role in AGS development. Despite their utility, these methods fail to replicate the intricate natural process of tick attachment and the secretion of tick salivary factors during feeding, which is crucial for host sensitization.
Additionally, the salivary extract contains several proteins; many of those are non-secretory glycosylated proteins and may not be part of tick saliva but still can drive antibody response against the α-gal antigen in the host. Consequently, the host response driven by cutaneous injection may not truly reflect the sensitization process during prolonged and repeated tick feeding. Furthermore, no direct evidence demonstrates that mouse sensitization following repeated tick feeding causes AGS induction in the AGKO murine model. Therefore, we aimed to develop a tick sensitization model by sensitizing AGKO mice through repeated nymphal tick feeding. This model was used to study the host’s immune response to tick bites using tick species Aa and Am to determine their role in inducing the sIgE response. The alpha-gal signature in the saliva and salivary glands of the lone-star tick has been reported (9, 29, 69), and subcutaneous injection of tick salivary glands extract has also been reported to induce high titer IgE response in AGKO murine model (30). Figure 1 shows a gradual increase in the total IgE and IgG1 levels in tick-sensitized mice compared to the control mice group.
Intriguingly, the total IgG titer of Am-sensitized mice was similar following 4th sensitization however in the Aa sensitized mice, total IgG titer peak earlier (from 3rd sensitization). It is expected to see an elevated IgG titer after any tick species bite; however, Am bite-induced high titer may correlate with the voracious and aggressive feeding behavior and high amount of salivary protein injected into the host compared to Aa. Noticeably, Aa-sensitized mice showed a significantly higher α-gal IgG1 antibody titer after 3rd and 4th infestation, indicating salivary antigens from Aa boosts anti-α-gal host responses (Figure 1B). The total IgE titer significantly increased after the 4th infestation of Am nymphs compared to control mice (Figure 1B). These results align with the trend observed in subcutaneous sensitization of mice injected with Aa TSGE (30). Though we observed that α-gal specific IgG1 increased gradually during repeated sensitization, the level of α-gal specific IgE during tick feeding did not follow the same increasing pattern with Am. americanum repeated nymph sensitization. Low levels of sIgE were detected in a few Aa-sensitized mice as well as in Am-sensitized mice. Previous studies reported a correlation between elevated α-gal specific IgG1 and high IgE titer in AGS patients (70). Despite elevated levels of α-gal IgG1, high titer α-gal IgE was not observed in Aa nymph-sensitized mice. The most likely explanation is that tick salivary factors inoculated at the bite site inhibited the alpha-gal-sIgE-driven inflammatory response in the later infestations and aided in tick feeding (71). Mice sensitization results imply tick bites of Aa can have low anti-alpha-gal IgE response and consistently elevated levels of alpha-gal IgG1 response. On the other hand, Am sensitization generated a low level of α-gal the IgE response, and α-gal the IgG titer remained extremely low. These trends suggest that the elevation in the titer of α-gal specific IgE following tick bites may be attributed to antibody switching induced by tick salivary prostaglandins, a phenomenon believed to play a role in driving IgE class switching and IgE production (72). Since α-gal alone does not induce an IgE response (68), there must be sensitization driven by antigen and tick salivary factors such as prostaglandin E2 (PGE2) stimulating the synthesis of the α-gal IgE antibodies (73). Thus, an “atypical” Th2-like host response may occur during tick sensitization to produce α-gal IgE. In addition, another possible mechanism is the presence of α-gal bound lipids in the tick saliva, which could potentially trigger the release of Th2 biasing cytokines from NKT cells. As reported earlier, this immune response skewing towards Th2 could contribute to the production of α-gal IgE antibodies (74).
In this study, we presented evidence that alpha-gal-specific immune response of the host triggered by tick bite-sensitized mice led to an allergic reaction upon challenge with pork kidney meat. This indicates that α-gal specific sensitization of the host is caused or boosted by a particular tick expressing α-gal in saliva during a tick bite. It is also important to note that the dose of the allergen can also vary during repeat tick sensitization as nymphs are in an undifferentiated developmental stage, and lightweight engorged nymphs molt into male adults after feeding on the host (75).
We investigated host response analysis using a NanoString approach to understand how Aa tick bite shapes AGS development in a murine model. Infestation of AGKO mice with ticks resulted in an initial burst of proinflammatory response characterized by a robust increase in the transcript of IL-1β as well as components of the NLRP3 inflammasome complex that is essential for IL-1β activation (43). Among inflammasome receptors, NLRP3 is unique because it is activated by diverse pathogen-associated molecular patterns (PAMP) and damage-associated molecular patterns (DAMP) from dying or injured cells. It is intriguing to speculate that an increase in HK3 could also activate the inflammasome in a PAMP-independent manner (76). In sum, tick infestation in mice resulted in a massive induction of pro-IL-1β by both NF-κB and MAPK pathways and its cleavage to an active form can occur by the NLPR3 inflammasome, which was also markedly upregulated in skin biopsy samples from tick bitten mice.
The IL-6 family of cytokines plays an important role in antimicrobial and antiviral immunity and provides tissue protection from infection-related injury (77). These cytokines often control the recruitment, adhesion, survival and effector activities of neutrophils, tissue-resident and inflammatory monocytes, and innate lymphoid cell populations including NK cells. We observed robust upregulation of both Osm and Il6 and several of their downstream targets, specifically neutrophil-activating chemokines Cxcl1 and Cxcl5, adhesion molecules Icam1 and Vcam1 (78). While these molecules facilitate the effector function of neutrophils and the acute phase response, they also serve as lymphokines to promote the differentiation of Th1 or Th2 cells (58, 79–81). We further observed changes in gene signatures involved in suppressing inflammation and inducing immune tolerance. While no significant change was observed in Tbx21, critical to Th1 differentiation in tick-infested mice, the transcription factor Foxp3 was significantly induced following the 2nd infestation, consistent with a Treg response to suppress inflammation (52). We also observed increased transcription of Havcr2, which is expressed on Treg cells and could inhibit both Th1 and Th17 responses (82, 83).
Repeated exposure to tick saliva has been suggested to skew polarization of the immune response toward a Th2 profile, leading to the development of allergies and suppression of proinflammatory response. In this context, we observed increased transcripts of Th2 cytokines Il33 and Il4, as well as their receptors, following subsequent tick infestations that were also associated with increased production of IgG and IgE measured by ELISA (59, 61) (Figure 6). Further, we also found evidence of tick bite induced robust Tlr1, Tlr2, Tlr6, and Tlr8 signaling and MyD88 induction, both of which could contribute to IgE production as suggested by Chandrasekhar and colleagues (68). An increase in Alox15, Atf4 and Xbp1 transcripts which modulate ER stress response for efficient immunoglobulins secretion was further noted (65).
Prostaglandin E2 (PGE2) is one of the most abundant bioactive molecules in tick saliva and has been implicated in driving IgE class switching and the production of IgE (72). We observed upregulation of Ptgs2 transcription, a key enzyme in prostaglandin biosynthesis, as well as prostaglandin E receptor Ptger4 and bradykinin receptor Bdkrb1 (84). Bradykinin can lead to the release of prostaglandins (85). It is tempting to speculate that the upregulation of Ptger4 and Bdkrb1, and the production of PGE2, might have contributed to class switching from existing B cell clones producing anti-alpha-Gal IgM and/or IgG to anti-alpha-Gal IgE-producing B cells (72, 84–88).
IL-20 subfamily of cytokines protects epithelial cells against extracellular pathogens and plays a vital role in wound healing and homeostasis of the tissue epithelial layer (46). IL-19 and IL-24 are produced primarily by myeloid cells principally through TLR activation, although epithelial cells and Th2 cells can also generate both cytokines under certain conditions (89). We observed increased transcription of Il19 and Il24 following tick bites, which peaked following the 3rd infestation. Lipocalin 2 (Lcn2), which is important in controlling bacterial infection in mice (90) and could be induced by the IL-20 subfamily was upregulated (91). IL-24 suppresses IL-1β expression in keratinocytes to dampen inflammatory responses and stimulate keratinocytes for tissue repair (92). Further, it induces factors that play critical roles in modulating inflammatory responses, enhancing granulation tissue formation, and inducing angiogenesis.
The complement cascade offers the first line of defense against bites from ectoparasitic ticks and has been shown to play a part in acquired tick resistance (ATR) (93). Following infestation of AGKO mice with Aa, complement component 3 (C3) and complement receptors C3ar1 and C5ar1 were upregulated while Cd59a, a potent complement membrane attack system inhibitor, was downregulated. We observed upregulation of Plau and Plaur which could cause activation of plasminogen into plasmin (PL), a broad-spectrum serine-protease. Interestingly, plasmin could initiate a classical complement cascade resulting in complement activation and might contribute to the recruitment of basophils as observed in tick attachment sites in guinea pig models 94). We observed upregulation of several genes such as Il3ra, Hdc, Lyn, Syk, Fcer1a and Pik3cd which prime mature basophils and mast cells resulting in degranulation following IgE binding or exposure to anaphylatoxins and therefore might also contribute to ATR (95).
Conclusion
Here we describe key immune determinants linked to host response and AGS induction following Am. americanum repeated tick bite using AGTKO mice model. We also demonstrated and established a method that included the use of nymph to investigate the role of ticks in the induction of AGS—following tick bite. We also reported that presence of α-gal antigens in tick plays a critical role in the sensitization of host against α-gal following tick bite. This NanoString dataset will be important for understanding critical host pathways, immune gene linked to AGS induction in host following tick bite. Our study also validated the fact that high α-gal IgG1 titer is an indicator of AGS development in the host and tick bite plays role in boosting host response against α-gal antigen leading to sensitization. Our findings also demonstrated that presence of α-gal moieties in tick saliva is a critical factor for sensitization against α-gal in host during tick bite.
Data availability statement
The data presented in this study are deposited in the NCBI GEO repository, accession number GSE249650 (GSM7956829-64).
Ethics statement
The animal study was approved by The Institutional Animal Care and Use Committee of the University of Southern Mississippi. The study was conducted in accordance with the local legislation and institutional requirements.
Author contributions
SS: Formal analysis, Investigation, Methodology, Visualization, Writing – original draft, Writing – review & editing. SKC: Data curation, Formal analysis, Investigation, Methodology, Visualization, Writing – original draft, Writing – review & editing. JV: Data curation, Investigation, Methodology, Writing, reviewing & editing. SPC: Data curation, Funding acquisition, Investigation, Resources, Validation, Visualization, Writing – original draft, Writing – review & editing. SK: Conceptualization, Data curation, Funding acquisition, Investigation, Methodology, Project administration, Resources, Supervision, Validation, Visualization, Writing – original draft, Writing – review & editing.
Funding
The author(s) declare financial support was received for the research, authorship, and/or publication of this article. This research was principally supported by USDA NIFA award # 2017-67017-26171, 2016-67030-24576, and NIH NIAID award # AI128182 & R01 AI135049. The funders played no role in the study design, data collection and analysis, decision to publish, or manuscript preparation.
Acknowledgments
We thank the staff of UNC Respiratory TRACTS Core Laboratory for NanoString immunological assays.
Conflict of interest
The authors declare that the research was conducted in the absence of any commercial or financial relationships that could be construed as a potential conflict of interest.
The author(s) declared that they were an editorial board member of Frontiers, at the time of submission. This had no impact on the peer review process and the final decision.
Publisher’s note
All claims expressed in this article are solely those of the authors and do not necessarily represent those of their affiliated organizations, or those of the publisher, the editors and the reviewers. Any product that may be evaluated in this article, or claim that may be made by its manufacturer, is not guaranteed or endorsed by the publisher.
Supplementary material
The Supplementary Material for this article can be found online at: https://www.frontiersin.org/articles/10.3389/fimmu.2023.1336883/full#supplementary-material
References
1. Macher BA, Galili U. The Galalpha1,3Galbeta1,4GlcNAc-R (alpha-Gal) epitope: a carbohydrate of unique evolution and clinical relevance. Biochim Biophys Acta (2008) 1780(2):75–88. doi: 10.1016/j.bbagen.2007.11.003
2. Commins SP, Satinover SM, Hosen J, Mozena J, Borish L, Lewis BD, et al. Delayed anaphylaxis, angioedema, or urticaria after consumption of red meat in patients with IgE antibodies specific for galactose-alpha-1,3-galactose. J Allergy Clin Immunol (2009) 123(2):426–33. doi: 10.1016/j.jaci.2008.10.052
3. Galili U, Avila JL. α-gal and anti-gal, α1,3-galactosyltransferase, α-gal epitopes, and the natural anti-gal antibody subcellular biochemistry Vol. 32. Boston, MA: Springer US (1999). doi: 10.1007/978-1-4615-4771-6
4. Apostolovic D, Tran TA, Hamsten C, Starkhammar M, Cirkovic Velickovic T, van Hage M. Immunoproteomics of processed beef proteins reveal novel galactose-α-1,3-galactose-containing allergens. Allergy (2014) 69(10):1308–15. doi: 10.1111/all.12462
5. Takahashi H, Chinuki Y, Tanaka A, Morita E. Laminin γ-1 and collagen α-1 (VI) chain are galactose-α-1,3-galactose-bound allergens in beef. Allergy (2014) 69(2):199–207. doi: 10.1111/all.12302
6. Iweala OI, Choudhary SK, Addison CT, Batty CJ, Kapita CM, Amelio C, et al. Glycolipid-mediated basophil activation in alpha-gal allergy. J Allergy Clin Immunol (2020) 146(2):450–2. doi: 10.1016/j.jaci.2020.02.006
7. Hilger C, Fischer J, Swiontek K, Hentges F, Lehners C, Eberlein B, et al. Two galactose-α-1,3-galactose carrying peptidases from pork kidney mediate anaphylactogenic responses in delayed meat allergy. Allergy (2016) 71(5):711–9. doi: 10.1111/all.12835
8. Commins SP, James HR, Stevens W, Pochan SL, Land MH, King C, et al. Delayed clinical and ex vivo response to mammalian meat in patients with IgE to galactose-alpha-1,3-galactose. J Allergy Clin Immunol (2014) 134(1):108–15. doi: 10.1016/j.jaci.2014.01.024
9. Sharma SR, Karim S. Tick saliva and the alpha-gal syndrome: finding a needle in a haystack. Front Cell infection Microbiol (2021) 11:680264. doi: 10.3389/fcimb.2021.680264
10. Galili U. Evolution of alpha 1,3galactosyltransferase and of the alpha-Gal epitope. Sub-cellular Biochem (1999) 32:1–23. doi: 10.1007/978-1-4615-4771-6_1
11. Galili U. The alpha-gal epitope and the anti-Gal antibody in xenotransplantation and in cancer immunotherapy. Immunol Cell Biol (2005) 83(6):674–86. doi: 10.1111/j.1440-1711.2005.01366.x
12. Galili U, Anaraki F, Thall A, Hill-Black C, Radic M. One percent of human circulating B lymphocytes are capable of producing the natural anti-Gal antibody. Blood (1993) 82(8):2485–93. doi: 10.1182/blood.V82.8.2485.2485
13. Fischer J, Hebsaker J, Caponetto P, Platts-Mills TA, Biedermann T. Galactose-alpha-1,3-galactose sensitization is a prerequisite for pork-kidney allergy and cofactor-related mammalian meat anaphylaxis. J Allergy Clin Immunol (2014) 134(3):755–759.e1. doi: 10.1016/j.jaci.2014.05.051
14. Hamanova M, Chmelikova M, Nentwich I, Thon V, Lokaj J. Anti-Gal IgM, IgA and IgG natural antibodies in childhood. Immunol Lett (2015) 164(1):40–3. doi: 10.1016/j.imlet.2015.02.001
15. Hilger C, Fischer J, Wölbing F, Biedermann T. Role and mechanism of galactose-alpha-1,3-galactose in the elicitation of delayed anaphylactic reactions to red meat. Curr Allergy Asthma Rep (2019) 19(1):3. doi: 10.1007/s11882-019-0835-9
16. Berg EA, Platts-Mills TA, Commins SP. Drug allergens and food–the cetuximab and galactose-α-1,3-galactose story. Ann Allergy Asthma Immunol (2014) 112(2):97–101. doi: 10.1016/j.anai.2013.11.014
17. Roseman S. Reflections on glycobiology. J Biol Chem (2001) 276(45):41527–42. doi: 10.1074/jbc.R100053200
18. Chmelař J, Kotál J, Kopecký J, Pedra JHF, Kotsyfakis M. All for one and one for all on the tick-host battlefield. Trends Parasitol (2016) 32(5):368–77. doi: 10.1016/j.pt.2016.01.004
19. Bullard R, Sharma SR, Das PK, Morgan SE, Karim S. Repurposing of glycine-rich proteins in abiotic and biotic stresses in the lone-star tick (Amblyomma americanum). Front Physiol (2019) 10:744. doi: 10.3389/fphys.2019.00744
20. Adegoke A, Kumar D, Bobo C, Rashid MI, Durrani AZ, Sajid MS, et al. Tick-borne pathogens shape the native microbiome within tick vectors. Microorganisms (2020) 8(9):1299. doi: 10.3390/microorganisms8091299
21. Commins SP, James HR, Kelly LA, Pochan SL, Workman LJ, Perzanowski MS, et al. The relevance of tick bites to the production of IgE antibodies to the mammalian oligosaccharide galactose-α-1,3-galactose. J Allergy Clin Immunol (2011) 127(5):1286–93.e6. doi: 10.1016/j.jaci.2011.02.019
22. Hamsten C, Starkhammar M, Tran TA, Johansson M, Bengtsson U, Ahlén G, et al. Identification of galactose-α-1,3-galactose in the gastrointestinal tract of the tick Ixodes ricinus; possible relationship with red meat allergy. Allergy (2013) 68(4):549–52. doi: 10.1111/all.12128
23. Araujo RN, Franco PF, Rodrigues H, Santos LCB, McKay CS, Sanhueza CA, et al. Amblyomma sculptum tick saliva: α-Gal identification, antibody response and possible association with red meat allergy in Brazil. Int J Parasitol (2016) 46(3):213–20. doi: 10.1016/j.ijpara.2015.12.005
24. van Nunen S. Tick-induced allergies: mammalian meat allergy, tick anaphylaxis and their significance. Asia Pac Allergy (2015) 5(1):3–16. doi: 10.5415/apallergy.2015.5.1.3
25. Thompson JM, Carpenter A, Kersh GJ, Wachs T, Commins SP, Salzer JS. Geographic distribution of suspected alpha-gal syndrome cases - United States, january 2017-december 2022. MMWR. Morbidity mortality weekly Rep (2023) 72(30):815–20. doi: 10.15585/mmwr.mm7230a2
26. Binder AM, Commins SP, Altrich ML, Wachs T, Biggerstaff BJ, Beard CB, et al. Diagnostic testing for galactose-alpha-1,3-galactose, United States 2010 to 2018. Ann allergy Asthma immunology: Off Publ Am Coll Allergy Asthma Immunol (2021) 126(4):411–416.e1. doi: 10.1016/j.anai.2020.12.019
27. Monzón JD, Atkinson EG, Henn BM, Benach JL. Population and evolutionary genomics of amblyomma americanum, an expanding arthropod disease vector. Genome Biol Evol (2016) 8(5):1351–60. doi: 10.1093/gbe/evw080
28. Raghavan RK, Peterson AT, Cobos ME, Ganta R, Foley D. Current and future distribution of the lone star tick, amblyomma americanum (L.) (Acari: ixodidae) in North America. PloS One (2019) 14(1):e0209082. doi: 10.1371/journal.pone.0209082
29. Crispell G, Commins SP, Archer-Hartman SA, Choudhary S, Dharmarajan G, Azadi P, et al. Discovery of alpha-gal-containing antigens in north american tick species believed to induce red meat allergy. Front Immunol (2019) 10:1056. doi: 10.3389/fimmu.2019.01056
30. Choudhary SK, Karim S, Iweala OI, Choudhary S, Crispell G, Sharma SR, et al. Tick salivary gland extract induces alpha-gal syndrome in alpha-gal deficient mice. Immun. Inflamm Dis (2021) 9(3):984–90. doi: 10.1002/iid3.457
31. Commins SP, Platts-Mills TA. Delayed anaphylaxis to red meat in patients with IgE specific for galactose alpha-1,3-galactose (alpha-gal). Curr Allergy Asthma Rep (2013) 13(1):72–7. doi: 10.1007/s11882-012-0315-y
32. Hashizume H, Fujiyama T, Umayahara T, Kageyama R, Walls AF, Satoh T. Repeated Amblyomma testudinarium tick bites are associated with increased galactose-α-1,3-galactose carbohydrate IgE antibody levels: A retrospective cohort study in a single institution. J Am Acad Dermatol (2018) 78(6):1135–1141.e3. doi: 10.1016/j.jaad.2017.12.028
33. Kim MS, Straesser MD, Keshavarz B, Workman L, McGowan EC, Platts-Mills TAE, et al. IgE to galactose-α-1,3-galactose wanes over time in patients who avoid tick bites. J Allergy Clin Immunol In Pract (2020) 8(1):364–367.e2. doi: 10.1016/j.jaip.2019.08.045
34. Kersh GJ, Salzer J, Jones ES, Binder AM, Armstrong PA, Choudhary SK, et al. Tick bite as a risk factor for alpha-gal-specific immunoglobulin E antibodies and development of alpha-gal syndrome. Ann allergy Asthma immunology: Off Publ Am Coll Allergy Asthma Immunol (2023) 130(4):472–8. doi: 10.1016/j.anai.2022.11.021
35. Patrick CD, Hair JA. Laboratory rearing procedures and equipment for multi-host ticks (Acarina: Ixodidae). J Med Entomol (1975) 12(3):389–90. doi: 10.1093/jmedent/12.3.389
36. Tearle RG, Tange MJ, Zannettino ZL, Katerelos M, Shinkel TA, Van Denderen BJ, et al. The alpha-1,3-galactosyltransferase knockout mouse. Implications for xenotransplantation. Transplantation (1996) 61(1):13–9. doi: 10.1097/00007890-199601150-00004
37. Wilson JM, Keshavarz B, Retterer M, Workman LJ, Schuyler AJ, McGowan EC, et al. A dynamic relationship between two regional causes of IgE-mediated anaphylaxis: α-Gal syndrome and imported fire ant. J Allergy Clin Immunol (2021) 147(2):643–652.e7. doi: 10.1016/j.jaci.2020.05.034
38. Snapper CM, Paul WE. Interferon-gamma and B cell stimulatory factor-1 reciprocally regulate Ig isotype production. Sci (New York N.Y.) (1987) 236(4804):944–7. doi: 10.1126/science.3107127
39. Finkelman FD. Anaphylaxis: lessons from mouse models. J Allergy Clin Immunol (2007) 120(3):506–17. doi: 10.1016/j.jaci.2007.07.033
40. Orgel K, Smeekens JM, Ye P, Fotsch L, Guo R, Miller DR, et al. Genetic diversity between mouse strains allows identification of the CC027/GeniUnc strain as an orally reactive model of peanut allergy. J Allergy Clin Immunol (2019) 143(3):1027–1037.e7. doi: 10.1016/j.jaci.2018.10.009
41. Wright SD, Ramos RA, Tobias PS, Ulevitch RJ, Mathison JC. CD14, a receptor for complexes of lipopolysaccharide (LPS) and LPS binding protein. Sci (New York N.Y.) (1990) 249(4975):1431–3. doi: 10.1126/science.1698311
42. Kawasaki T, Kawai T. Toll-like receptor signaling pathways. Front Immunol (2014) 5:461. doi: 10.3389/fimmu.2014.00461
43. Jo EK, Kim JK, Shin DM, Sasakawa C. Molecular mechanisms regulating NLRP3 inflammasome activation. Cell Mol Immunol (2016) 13(2):148–59. doi: 10.1038/cmi.2015.95
44. Rühl S, Broz P. Caspase-11 activates a canonical NLRP3 inflammasome by promoting K(+) efflux. Eur J Immunol (2015) 45(10):2927–36. doi: 10.1002/eji.201545772
45. Wagner RN, Proell M, Kufer TA, Schwarzenbacher R. Evaluation of Nod-like receptor (NLR) effector domain interactions. PloS One (2009) 4(4):e4931. doi: 10.1371/journal.pone.0004931
46. Rutz S, Wang X, Ouyang W. The IL-20 subfamily of cytokines–from host defence to tissue homeostasis. Nat Rev Immunol (2014) 14(12):783–95. doi: 10.1038/nri3766
47. Yánez DC, Ross S, Crompton T. The IFITM protein family in adaptive immunity. Immunology (2020) 159(4):365–72. doi: 10.1111/imm.13163
48. Tangye SG, Pathmanandavel K, Ma CS. Cytokine-mediated STAT-dependent pathways underpinning human B-cell differentiation and function. Curr Opin Immunol (2023) 81:102286. doi: 10.1016/j.coi.2023.102286
49. Boulay F, Tardif M, Brouchon L, Vignais P. The human N-formylpeptide receptor. Characterization of two cDNA isolates and evidence for a new subfamily of G-protein-coupled receptors. Biochemistry (1990) 29(50):11123–33. doi: 10.1021/bi00502a016
50. Sarrias MR, Farnós M, Mota R, Sánchez-Barbero F, Ibáñez A, Gimferrer I, et al. CD6 binds to pathogen-associated molecular patterns and protects from LPS-induced septic shock. Proc Natl Acad Sci United States America (2007) 104(28):11724–9. doi: 10.1073/pnas.0702815104
51. He YW, Deftos ML, Ojala EW, Bevan MJ. RORgamma t, a novel isoform of an orphan receptor, negatively regulates Fas ligand expression and IL-2 production in T cells. Immunity (1998) 9(6):797–806. doi: 10.1016/s1074-7613(00)80645-7
52. Ono M, Yaguchi H, Ohkura N, Kitabayashi I, Nagamura Y, Nomura T, et al. Foxp3 controls regulatory T-cell function by interacting with AML1/Runx1. Nature (2007) 446(7136):685–9. doi: 10.1038/nature05673
53. Szabo SJ, Kim ST, Costa GL, Zhang X, Fathman CG, Glimcher LH. A novel transcription factor, T-bet, directs Th1 lineage commitment. Cell (2000) 100(6):655–69. doi: 10.1016/s0092-8674(00)80702-3
54. Angel CE, Chen CJ, Horlacher OC, Winkler S, John T, Browning J, et al. Distinctive localization of antigen-presenting cells in human lymph nodes. Blood (2009) 113(6):1257–67. doi: 10.1182/blood-2008-06-165266
55. Baranov MV, Bianchi F, Schirmacher A, van Aart MAC, Maassen S, Muntjewerff EM, et al. The phosphoinositide kinase PIKfyve promotes cathepsin-S-mediated major histocompatibility complex class II antigen presentation. iScience (2019) 11:160–77. doi: 10.1016/j.isci.2018.12.015
56. Cannons JL, Qi H, Lu KT, Dutta M, Gomez-Rodriguez J, Cheng J, et al. Optimal germinal center responses require a multistage t cell:B cell adhesion process involving integrins, SLAM-associated protein, and CD84. Immunity (2010) 32(2):253–65. doi: 10.1016/j.immuni.2010.01.010
57. Eto D, Lao C, DiToro D, Barnett B, Escobar TC, Kageyama R, et al. IL-21 and IL-6 are critical for different aspects of B cell immunity and redundantly induce optimal follicular helper CD4 T cell (Tfh) differentiation. PloS One (2011) 6(3):e17739. doi: 10.1371/journal.pone.0017739
58. Dienz O, Eaton SM, Bond JP, Neveu W, Moquin D, Noubade R, et al. The induction of antibody production by IL-6 is indirectly mediated by IL-21 produced by CD4+ t cells. J Exp Med (2009) 206(1):69–78. doi: 10.1084/jem.20081571
59. Coffman RL, Ohara J, Bond MW, Carty J, Zlotnik A, Paul WE. B cell stimulatory factor-1 enhances the IgE response of lipopolysaccharide-activated B cells. J Immunol (Baltimore Md.: 1950) (1986) 136(12):4538–41. doi: 10.4049/jimmunol.136.12.4538
60. DeFrance T, Carayon P, Billian G, Guillemot JC, Minty A, Caput D, et al. Interleukin 13 is a B cell stimulating factor. J Exp Med (1994) 179(1):135–43. doi: 10.1084/jem.179.1.135
61. Schmitz J, Owyang A, Oldham E, Song Y, Murphy E, McClanahan TK, et al. IL-33, an interleukin-1-like cytokine that signals via the IL-1 receptor-related protein ST2 and induces T helper type 2-associated cytokines. Immunity (2005) 23(5):479–90. doi: 10.1016/j.immuni.2005.09.015
62. Ho IC, Lo D, Glimcher LH. c-maf promotes T helper cell type 2 (Th2) and attenuates Th1 differentiation by both interleukin 4-dependent and -independent mechanisms. J Exp Med (1998) 188(10):1859–66. doi: 10.1084/jem.188.10.1859
63. Troutman TD, Hu W, Fulenchek S, Yamazaki T, Kurosaki T, Bazan JF, et al. Role for B-cell adapter for PI3K (BCAP) as a signaling adapter linking Toll-like receptors (TLRs) to serine/threonine kinases PI3K/Akt. Proc Natl Acad Sci United States America (2012) 109(1):273–8. doi: 10.1073/pnas.1118579109
64. Avery DT, Kane A, Nguyen T, Lau A, Nguyen A, Lenthall H, et al. Germline-activating mutations in PIK3CD compromise B cell development and function. J Exp Med (2018) 215(8):2073–95. doi: 10.1084/jem.20180010
65. So JS. Roles of endoplasmic reticulum stress in immune responses. Molecules Cells (2018) 41(8):705–16. doi: 10.14348/molcells.2018.0241
66. Commins SP. Diagnosis & Management of alpha-gal syndrome: lessons from 2,500 patients. Expert rev. Clin Immunol (2020) 16(7):667–77. doi: 10.1080/1744666X.2020.1782745
67. Van Nunen SA, O'Connor KS, Clarke LR, Boyle RX, Fernando SL. An association between tick bite reactions and red meat allergy in humans. Med J Aust (2009) 190(9):510–1. doi: 10.5694/j.1326-5377.2009.tb02533.x
68. Chandrasekhar JL, Cox KM, Loo WM, Qiao H, Tung KS, Erickson LD. Cutaneous exposure to clinically relevant lone star ticks promotes igE production and hypersensitivity through CD4+ T cell- and myD88-dependent pathways in mice. J Immunol (Baltimore Md.: 1950) (2019) 203(4):813–24. doi: 10.4049/jimmunol.1801156
69. Park Y, Kim D, Boorgula GD, De Schutter K, Smagghe G, Šimo L, et al. Alpha-gal and cross-reactive carbohydrate determinants in the n-glycans of salivary glands in the lone star tick, amblyomma americanum. Vaccines (2020) 8(1):18. doi: 10.3390/vaccines8010018
70. Joral A, Azketa N, Sanchez P, Vélez-Del-Burgo A, Aranzabal-Soto MA, Lizarza S, et al. The quantification of igG specific to α-gal could be used as a risk marker for suffering mammalian meat allergy. Foods (2022) 11(3):466. doi: 10.3390/foods11030466
71. Kotál J, Langhansová H, Lieskovská J, Andersen JF, Francischetti IM, Chavakis T, et al. Modulation of host immunity by tick saliva. J Proteomics (2015) 128:58–68. doi: 10.1016/j.jprot.2015.07.005
72. Cabezas-Cruz A, Mateos-Hernández L, Chmelař J, Villar M, de la Fuente J. Salivary prostaglandin E2: role in tick-induced allergy to red meat. Trends Parasitol (2017) 33(7):495–8. doi: 10.1016/j.pt.2017.03.004
73. Carvalho-Costa TM, Mendes MT, da Silva MV, da Costa TA, Tiburcio MG, Anhê AC, et al. Immunosuppressive effects of amblyomma cajennense tick saliva on murine bone marrow-derived dendritic cells. Parasit Vectors (2015) 8:22. doi: 10.1186/s13071-015-0634-7
74. Van Kaer L, Wu L. Therapeutic potential of invariant natural killer T cells in autoimmunity. Front Immunol (2018) 9:519. doi: 10.3389/fimmu.2018.00519
75. Karim S, Singh P, Ribeiro JM. A deep insight into the sialotranscriptome of the gulf coast tick, amblyomma maculatum. PloS One (2011) 6(12):e28525. doi: 10.1371/journal.pone.0028
76. Wolf AJ, Reyes CN, Liang W, Becker C, Shimada K, Wheeler ML, et al. Hexokinase is an innate immune receptor for the detection of bacterial peptidoglycan. Cell (2016) 166(3):624–36. doi: 10.1016/j.cell.2016.05.076
77. Tanaka T, Narazaki M, Kishimoto T. IL-6 in inflammation, immunity, and disease. Cold Spring Harbor Perspect Biol (2014) 6(10):a016295. doi: 10.1101/cshperspect.a016295
78. Heinze DM, Wikel SK, Thangamani S, Alarcon-Chaidez FJ. Transcriptional profiling of the murine cutaneous response during initial and subsequent infestations with Ixodes scapularis nymphs. Parasites Vectors (2012) 5:26. doi: 10.1186/1756-3305-5-26
79. Jones SA, Jenkins BJ. Recent insights into targeting the IL-6 cytokine family in inflammatory diseases and cancer. Nat Rev Immunol (2018) 18(12):773–89. doi: 10.1038/s41577-018-0066-7
81. Malandain H. IgE-reactive carbohydrate epitopes–classification, cross-reactivity, and clinical impact. Eur Ann Allergy Clin Immunol (2005) 37(4):122–8.
82. Sánchez-Fueyo A, Tian J, Picarella D, Domenig C, Zheng XX, Sabatos CA, et al. Tim-3 inhibits T helper type 1-mediated auto- and alloimmune responses and promotes immunological tolerance. Nat Immunol (2003) 4(11):1093–101. doi: 10.1038/ni987
83. Gautron AS, Dominguez-Villar M, de Marcken M, Hafler DA. Enhanced suppressor function of TIM-3+ FoxP3+ regulatory T cells. Eur J Immunol (2014) 44(9):2703–11. doi: 10.1002/eji.201344392
84. Kosaka T, Miyata A, Ihara H, Hara S, Sugimoto T, Takeda O, et al. Characterization of the human gene (PTGS2) encoding prostaglandin-endoperoxide synthase 2. Eur J Biochem (1994) 221(3):889–97. doi: 10.1111/j.1432-1033.1994.tb18804.x
85. Jose PJ, Page DA, Wolstenholme BE, Williams TJ, Dumonde DC. Bradykinin-stimulated prostaglandin E2 production by endothelial cells and its modulation by antiinflammatory compounds. Inflammation (1981) 5(4):363–78. doi: 10.1007/BF00911100
86. Qian Y, Essenberg RC, Dillwith JW, Bowman AS, Sauer JR. A specific prostaglandin E2 receptor and its role in modulating salivary secretion in the female tick, Amblyomma americanum (L.). Insect Biochem Mol Biol (1997) 27(5):387–95. doi: 10.1016/s0965-1748(97)00010-6
87. Poole NM, Mamidanna G, Smith RA, Coons LB, Cole JA. Prostaglandin E(2) in tick saliva regulates macrophage cell migration and cytokine profile. Parasites Vectors (2013) 6(1):261. doi: 10.1186/1756-3305-6-261
88. Li H, Edin ML, Gruzdev A, Cheng J, Bradbury JA, Graves JP, et al. Regulation of T helper cell subsets by cyclooxygenases and their metabolites. Prostaglandins Other Lipid Mediat (2013) 104-105:74–83. doi: 10.1016/j.prostaglandins.2012.11.002
89. Ouyang W, Rutz S, Crellin NK, Valdez PA, Hymowitz SG. Regulation and functions of the IL-10 family of cytokines in inflammation and disease. Annu Rev Immunol (2011) 29:71–109. doi: 10.1146/annurev-immunol-031210-101312
90. Wang Q, Li S, Tang X, Liang L, Wang F, Du H. Lipocalin 2 protects against escherichia coli infection by modulating neutrophil and macrophage function. Front Immunol (2019) 10:2594. doi: 10.3389/fimmu.2019.02594
91. Behnsen J, Jellbauer S, Wong CP, Edwards RA, George MD, Ouyang W, et al. The cytokine IL-22 promotes pathogen colonization by suppressing related commensal bacteria. Immunity (2014) 40(2):262–73. doi: 10.1016/j.immuni.2014.01.003
92. Myles IA, Fontecilla NM, Valdez PA, Vithayathil PJ, Naik S, Belkaid Y, et al. Signaling via the IL-20 receptor inhibits cutaneous production of IL-1β and IL-17A to promote infection with methicillin-resistant Staphylococcus aureus. Nat Immunol (2013) 14(8):804–11. doi: 10.1038/ni.2637
93. Kitsou C, Fikrig E, Pal U. Tick host immunity: vector immunomodulation and acquired tick resistance. Trends Immunol (2021) 42(7):554–74. doi: 10.1016/j.it.2021.05.005
94. Allen JR, Khalil HM, Graham JE. The location of tick salivary antigens, complement and immunoglobulin in the skin of Guinea-pigs infested with Dermacentor andersoni larvae. Immunology (1979) 38(3):467–72.
Keywords: alpha-gal, tick, Amblyomma americanum, alpha-gal knockout mice, delayed allergic responses, food allergy, mammalian meat, red meat allergy
Citation: Sharma SR, Choudhary SK, Vorobiov J, Commins SP and Karim S (2024) Tick bite-induced alpha-gal syndrome and immunologic responses in an alpha-gal deficient murine model. Front. Immunol. 14:1336883. doi: 10.3389/fimmu.2023.1336883
Received: 11 November 2023; Accepted: 26 December 2023;
Published: 08 February 2024.
Edited by:
Reginaldo G. Bastos, Washington State University, United StatesReviewed by:
Fábio Mambelli Silva, University of São Paulo, BrazilDanijela Apostolovic, Karolinska Institutet (KI), Sweden
Copyright © 2024 Sharma, Choudhary, Vorobiov, Commins and Karim. This is an open-access article distributed under the terms of the Creative Commons Attribution License (CC BY). The use, distribution or reproduction in other forums is permitted, provided the original author(s) and the copyright owner(s) are credited and that the original publication in this journal is cited, in accordance with accepted academic practice. No use, distribution or reproduction is permitted which does not comply with these terms.
*Correspondence: Shahid Karim, Shahid.Karim@usm.edu
†These authors have contributed equally to this work