- 1Department of Tumor Biological Treatment, The Third Affiliated Hospital of Soochow University, Changzhou, Jiangsu, China
- 2Jiangsu Engineering Research Center for Tumor lmmunotherapy, The Third Affiliated Hospital of Soochow University, Changzhou, Jiangsu, China
- 3Institute of Cell Therapy, The Third Affiliated Hospital of Soochow University, Changzhou, Jiangsu, China
Serving as a pivotal immunotherapeutic approach against tumors, anti-PD-1/PD-L1 therapy amplifies the immune cells’ capability to eliminate tumors by obstructing the interaction between PD-1 and PD-L1. Research indicates that immune checkpoint inhibitors are effective when a patient’s gut harbors unique beneficial bacteria. As such, it has further been revealed that the gut microbiome influences tumor development and the efficacy of cancer treatments, with metabolites produced by the microbiome playing a regulatory role in the antitumor efficacy of Immune checkpoint inhibitors(ICBs). This article discusses the mechanism of anti-PD-1 immunotherapy and the role of intestinal flora in immune regulation. This review focuses on the modulation of intestinal flora in the context of PD-1 immunotherapy, which may offer a new avenue for combination therapy in tumor immunotherapy.
1 Introduction
The treatment strategies for tumors are combination of multiple therapies, including surgery, chemotherapy, radiotherapy, and immunotherapy (1). Recently, researchers have paid more attention to immunotherapy, making immune checkpoint inhibitors an important treatment for most types of tumors (2).
Extensive research has delved into the effectiveness of the PD-1/PD-L1 pathway in cancer. Anti-PD-1 treatment hinders the liberation of negative modulators in the immune checkpoint, a function with broad applicability across malignancies, offering a sustained response in contrast to conventional therapies (3). PD-1 is a widely studied inhibitory regulatory receptor, indispensable in maintaining immune system homeostasis and regulating the function of T cells. Although anti-PD-1/PD-L1 therapy has demonstrated its effectiveness against tumors in certain patients, its efficacy is not universal, encountering challenges related to treatment resistance in some cases (4). In fact, it may also be necessary to regulate cancer immune checkpoints, promote immune tolerance to those immune checkpoints, and control abnormal angiogenesis, among other factors (5). Therefore, a combination treatment strategy is considered a reasonable and feasible way to achieve the most efficacious therapeutic effect.
Intestinal flora can influence the therapeutic effectiveness of various cancer treatments, including chemotherapy, radiation, and immunotherapy (6). Currently, there is widespread concern about whether the gut microbiome can modify the innate immune response to tumors and influence the effectiveness of immunotherapy (7). Studies have uncovered that the gut microbiome has the capacity to impact both tumor progression and the effectiveness of cancer treatments (8). The success of ICB therapy hinges on the existence of particular beneficial bacteria in the patient’s gut, and certain microbial metabolites have been identified as regulators of ICB efficacy. This review focuses on the regulation of intestinal flora against PD-1 immunotherapy, which may provide a new avenue for combination therapy in tumor immunotherapy.
2 Results
2.1 Specific elucidation and research progress of anti-PD-1 mechanism
2.1.1 The typical pathways of anti-PD-1 therapy
Programmed cell death protein 1 (PD-1) is a transmembrane protein of type I, encoded by the PDCD1 gene (9). Its primary expression occurs on activated T cells (CD4+ T cells, CD8+ T cells). However, it is also found on B cells, macrophages, monocytes, natural killer T cells, and dendritic cells (DCs). PD-1 stands out as one of the extensively studied inhibitory regulatory receptors, functioning as a crucial immune checkpoint that governs T cell and B cell antigen responses. Its role is indispensable in precisely modulating T cell function and preserving the balance of the immune system (9). The interaction between PD-1 and its ligand programmed cell death protein 1 ligand 1(PD-L1) can lead to T-cell exhaustion and the suppression T-cell function (10).
Within the cytoplasmic domain of PD-1, two tyrosine residues are present: an immunoreceptor tyrosine inhibitory motif (ITIM) and an immunoreceptor tyrosine switching motif (ITSM) (11). Upon binding to a ligand, primarily PD-L1, the tyrosine residues situated in the PD-1 ITSM undergo phosphorylation, recruiting protein tyrosine phosphatases (PTPs). These PTPs antagonize the signals generated by T cell receptor (TCR) and CD28, inhibiting the effect of TCR activation (12). For example, shp2 inhibits the RAS-ERK pathway, and ZAP-70, the PI3K-AKT pathway. Therefore, PD-1 binding to PD-L1 reduces transcription factor activation, resulting in the inhibition of proliferation, activation, and cytokine production in T cells (Figure 1). This culminates in the inhibition of metabolic alterations and the impairment of cytotoxic T lymphocytes (CTLs) killing function, ultimately leading to the demise of activated T cells (13). The PD-L1 signaling pathway is implicated in various cell types, encompassing dendritic cells, macrophages, T cells, B cells, and mast cells. Beyond hematopoietic cells, nonhematopoietic cells such as vascular endothelium, islet cells, placental trophoblasts, and keratinocytes are also involved. In healthy tissues, the predominant mechanism for maintaining physiological peripheral immune tolerance involves the elevated expression of PD-L1. This control mechanism mitigates tissue autoimmunity following prolonged inflammatory responses to tissue injury. However, heightened PD-L1 expression diminishes T-cell-mediated cytotoxicity, facilitating evasion from the immune system (14).Tumor-infiltrating lymphocytes (TILs) stand out as the principal effector cells within the tumor tissue microenvironment, expressing PD-1 (15). Throughout tumor progression, tumor cells express negative checkpoint regulators that stifle immunity, allowing them to elude immune surveillance (16). Furthermore, interactions involving anti-PD-1/PD-L1 also contribute to the restoration of CD8+ T cell function within tumors (17).
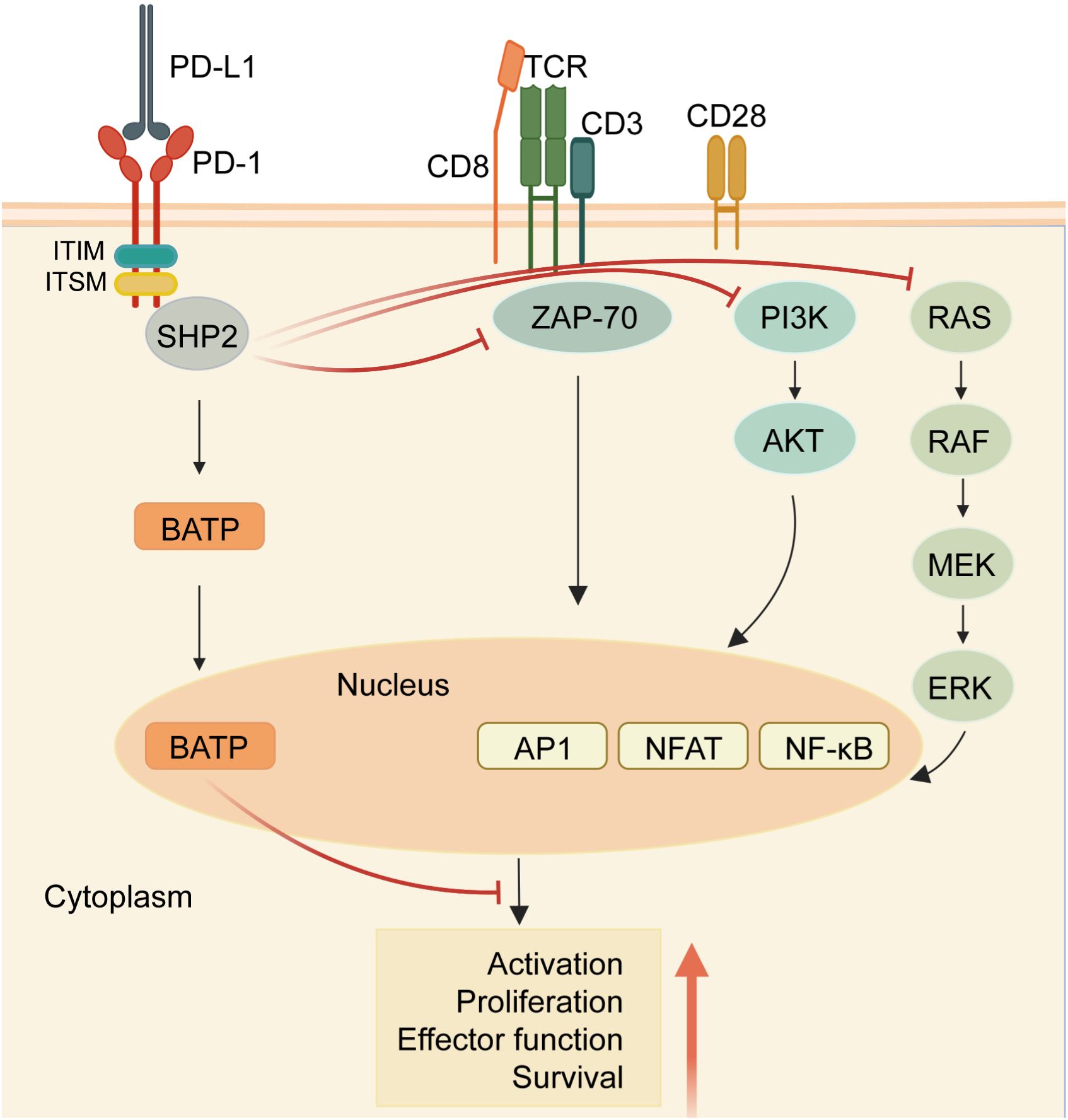
Figure 1 PD-1/PD-L1 signaling in T cells. When the receptor PD-1 binds to its ligand PD-L1, the PD-1 ITSM is phosphorylated at tyrosine residues, leading to the recruitment of protein tyrosine phosphatases (PTPs) such as SHP2. SHP2 dephosphorylates ZAP70 and antagonizes positive signals through the TCR and CD28 receptors, thereby inhibiting T cell function and T cell exhaustion. In addition, PD-1 can inhibit T cell function by up-regulating the expression of the transcription factor BATF. (Created with BioRender.com).
Crucially, the inhibition of the PD-1/PD-L1 pathway instigates the activation of tumor-specific cytotoxic T cells within the tumor microenvironment (15). The coalescence of PD-L1 and PD-1 fosters the induction of interleukin-10 and T cell apoptosis, leading to inactivation and depletion of T cells (15, 18). Additionally, PD-L1 serves as a protective “shield” for tumor cells against the cytotoxic response mediated by CD8+ T cells (19). Furthermore, CD80, expressed on activated T cells and antigen-presenting cells (APCs), acts as a receptor for PD-L1, transmitting inhibitory signals upon binding to PD-L1 (18). Moreover, PD-L1 can function as a receptor, transmitting signals from T cells to tumor cells, resulting in resistance of tumor cells to lysis. Consequently, the blockade of PD-1 and PD-L1 interaction effectively enhances the cytotoxicity of tumor-infiltrating lymphocytes(TILs) against tumor cells (20).
2.1.2 Progress in anti-PD-1 research and its combined therapy
The effectiveness of the PD-1/PD-L1 pathway has undergone thorough examination in the realm of cancer. Anti-PD-1 treatment, by impeding the release of negative modulators within the immune checkpoint, exhibits functionality with broad relevance across malignancies, yielding a sustained response when contrasted with conventional therapies (3). Employing the blockade of the PD-1/PD-L1 checkpoint has become the standard therapeutic approach for various cancers. Moreover, the inhibition of PD-1/PD-L1 is currently under intense scrutiny in clinical trials for the treatment of numerous other diseases (21). Based on clinical trial results, antibodies against PD-1 and PD-L1 are currently being used to treat multiple cancers (Table 1).
Despite the robust anti-tumor effects demonstrated in certain patients by α-PD-1/PD-L1 therapy, the majority are unable to derive sole benefits from this treatment, owing to the existence of various primary and secondary immune escape mechanisms (4). In fact, it may also be necessary to regulate cancer immune checkpoints and promote immune tolerance towards those immune checkpoints, as well as control abnormal angiogenesis, and so on (5). Eliminating these negative factors could raise the efficacy and mitigate drug resistance. In turn, reinforcing positive factors could improve the response to anti-PD-1/PD-L1 medical treatment (41). Therefore, a combination treatment strategy is considered to be a reasonable and feasible way to achieve the most efficacious therapeutic effect. It has been identified that some combination therapies can work synergistically with anti-PD-1/PD-L1 by raising the antigen release, and/or APC function and activity (42).
Studies have uncovered that the microbiome residing in the gut has the capacity to impact both tumor development and the effectiveness of cancer treatment (8). The success of ICB therapy hinges on the existence of particular beneficial bacteria in the patient’s gut, and certain microbial metabolites have been identified as regulators of the efficacy of ICBs. When dietary intake is degraded by the gut microbiome, various metabolites are produced, and liquid chromatography-tandem mass spectrometry can accurately screen the metabolites of intestinal flora (43). Some of these metabolites can trigger an inflammatory response, which aids the regulation of immune function (44). The presence of specific intestinal flora can cause an increase in the efficacy of PD-1 drugs four-fold. For example, certain bacteria (Lactobacillus johnsonii, Bifidobacterium pseudolongum, Olsenella species) produce inosine. In combination with checkpoint blockade immunotherapy, inosine can increase its effectiveness, promote the anti-tumor capacity of T cells, and ultimately inhibit tumor development (44). In summary, a deeper understanding of the interactions between the gut microbiome and the host immune system is of great importance for developing new cancer treatment strategies.
2.2 Gut microbiota and anti-tumor immunity
Tumor and gut microbiota determine cancer progression and response to treatment through their effects on innate and adaptive immunity (45). Intestinal flora can directly affect the progression and metastasis of tumors. Consequently, the presence and advancement of intestinal tumors stimulate the activity of intestinal flora. The interaction between tumors and gut microbiota plays a pivotal role in influencing cancer progression and the response to treatment, exerting effects on both innate and adaptive immunity (45). Intestinal flora can directly affect the progression and metastasis of tumors. As such, intestinal flora are stimulated by the occurrence and development of intestinal tumors.
2.2.1 Gut microbiota regulates innate immunity
The gut microbiota modulates innate immunity by regulating various immune cells, such as dendritic cells (DCs), monocytes, and natural killer (NK) cells.
The gut microbiota plays a regulatory role in DCs, which constitute a specialized group of antigen-presenting cells crucial for T cell activation and anti-tumor immunity (46). When Bifidobacterium is orally administered, it fosters the activation of DCs, consequently enhancing tumor-specific CD8+ T cell responses (47). Additionally, Bacteroides fragilis can promote the maturation of DCs and augment the IL-12-dependent Th1 cell immune response, thereby amplifying the anti-tumor efficacy of anti-CTLA-4 treatment (48).
Moreover, the gut microbiome exerts control over monocytes. Monocolonization with cdAMP-producing Akkermansia muciniphila(AKK) triggers a cascade involving monocytes, IFN-I, NK cells, and DCs, ultimately reinforcing anti-tumor responses (49). Similarly, Bifidobacterium, through STING signaling, supports CD47-based immunotherapy (50). Furthermore, Bacteroides fragilis is implicated in the up-regulation of the proportion of M1 macrophages, enhancing the expression of CD80 and CD86 on the cell surface and thus promoting innate immunity (51).
The gut microbiota plays a role in regulating NK cells, which influence anti-tumor immunity by modulating the abundance of DCs and CD8+ T cells in the TME (46). Lactobacillus plantarum has been shown to elevate NK cell activity by up-regulating the expression of natural cytotoxic receptor (NCR) protein (52). Furthermore, the intratumoral presence of Bifidobacterium and the subsequent heightened activation of NK cells have been demonstrated to induce anti-tumor immunity (53).
2.2.2 Intestinal microbiota regulates adaptive immunity
The gut microbiota exerts control over CD8+ T cells. In a melanoma clinical trial, a high abundance of Clostridiales, Ruminococcaceae, or Faecalibacterium is linked to increased antigen presentation. In comparison to patients with low abundance, this results in enhanced functionality of CD4+ and CD8+ T cells in both peripheral blood and the TME. Such enhancement is advantageous for the anti-tumor effectiveness of immune checkpoint inhibitors (ICI) (8). Another clinical trial provides evidence that the relative abundance of Enterococcus increases in patients’ tumors after receiving fecal microbiota transplantation (FMT) and anti-PD-1 treatment. This increase correlates with intratumoral CD8+ T cell infiltration and tumor cell necrosis (54). Furthermore, Bifidobacterium has been shown to increase the abundance of CD8+ T cells and enhance the efficacy of ICI treatment (47).
The gut microbiome exercises control over CD4+ T cells. The colonization of segmented filamentous bacteria (SFB) is particularly effective in inducing the growth of Th17 cells within the small intestine (55). Th17 cells, a vital component of CD4+ effector T cells, are proficient producers of IL-17, which plays a crucial role in host defense against extracellular pathogens (56). SFB induces small intestinal epithelial cells to produce serum amyloid A (SAA), which then activates CX3CR1+ phagocytes to produce IL-1β and IL-23. The synergistic action of IL-1β and IL-23 promotes the production of IL-22 in group 3 innate lymphoid cells (ILC3). Subsequently, IL-22 positively influences phagocytes, mediating IL-1β production and ultimately elevating IL-17 production in RORγt+ CD4+ T cells (55).Studies have shown that gut microbes exert control over the functionality of Treg cells by producing metabolites, including short-chain fatty acids (SCFAs), providing protection against colitis in mice through a mechanism dependent on Ffar2 (GPR43) (57). The genus Clostridium, especially Clostridium leptum and Clostridium coccoides, demonstrates the ability to induce Treg activity in both humans and mice (58). Moreover, SCFAs, particularly butyrate, possess the capability to augment the acetylation of Foxp3 sites in Tregs (59).
2.2.3 Regulatory mechanisms of immune cells by gut microbiota metabolites
Microbiota metabolism generates a multitude of metabolites, serving as crucial signaling factors and energy substrates that influence intestinal tumors (60). The key metabolites of gut microbiota encompass inosine, short-chain fatty acids (SCFAs), and anacardic acid, among others.
Inosine, a purine metabolite derived from gut microbes AKK and Bifidobacterium pseudolongum (B. pseudolongum), exhibits synergistic antitumor effects when combined with ICI therapy (44). Firstly, the treatment of tumor cells with inosine enhances the activation of IFN-γ and TNFα signaling pathways. IFN-γ, in turn, boosts the release of perforin and granzyme, augmenting antigen presentation and ultimately fostering anti-tumor immunity (61). Secondly, inosine enhances the efficacy of ICI by influencing the adenosine 2A receptor (A2AR) on T lymphocytes (44). Lastly, inosine can also serve as an alternative carbon source, providing energy for CD8+ T cells (62).
SCFAs, metabolites of gut microbiota, are also implicated in cancer antibody therapy (63). Firstly, Faecalibaculum rodentium PB1 and Helicia biformis (H. biformis) metabolize butyrate, which inhibits histone deacetylase (HDAC) and activates the NFATc3 transcription factor, leading to the inhibition of tumor cells (64). Secondly, SCFAs enhance anti-tumor immune responses. Butyrate, for instance, upregulates the expression of inhibiting DNA binding 2 (ID2) in CD8+ T cells, thereby augmenting the anti-tumor cytotoxicity of CD8+ T cells (65). Finally, SCFAs can serve as an energy source for anti-tumor immune cells by modulating metabolic pathways such as glycolysis, the tricarboxylic acid (TCA) cycle, and β-oxidation (66).
In addition to purines and SCFAs, several studies have verified that anacardic acid can also boost antitumor immunity. Anacardic acid activates innate immunity by phosphorylating mitogen-activated protein kinases (MAPKs), initiating the classical activation pathway of macrophages (67). Furthermore, in certain breast cancer models, it has been confirmed that anacardic acid elevates the levels of tumor-infiltrating NK cells and CTLs, inducing apoptosis in tumor cells (68).
Additionally, certain gut microbiota metabolites have been identified to dampen antitumor immune responses. For instance, some gut microbes generate the bile acid metabolite 3-oxolithocholic acid, which hinders the differentiation of Th17 cells (69). Moreover, the secondary bile acid 3β-hydroxydeoxycholic acid has been found to attenuate the immune activity of DCs, increase the number of Tregs, and facilitate immune escape (70).
These discoveries provide critical insights into regulatory mechanisms of immune cells by gut microbial metabolites, offering potential directions for developing novel anti-tumor therapies in the future.
2.2.4 Relationship between the gut microbiota and irAEs
The relationship between gut microbiota and immune-related adverse events (irAEs) triggered by immune checkpoint inhibitors (ICIs) is increasingly gaining attention. A specific category of toxicities resulting from the immune system’s hyperactivation induced by ICIs is termed irAEs (71). The gut microbiota is linked to the occurrence, type, and severity of these irAEs. This connection is particularly evident in cases of immune-related colitis, where it is speculated to operate through the regulation of metabolic pathways (72).
In a research endeavor involving 93 fecal samples obtained from 37 cancer patients undergoing anti-PD-1 treatment, individuals experiencing irAEs exhibit lower levels of Bifidobacterium, Faecalibacterium, and Agathobacter. Conversely, the abundance of Erysipelatoclostridium is notably higher in these patients. Those with colitis-type irAEs showcase reduced abundance of Bacteroides and Bifidobacterium, while Enterococcus has a higher prevalence (72). A thorough examination of the gut microbiota in 150 patients post-ICI treatment reveals that those with severe irAEs display elevated levels of Streptococcus, Paecalibacterium, and Stenotrophomonas. In contrast, patients with mild irAEs exhibit heightened levels of Faecalibacterium and unidentified Lachnospiraceae (73).
Analysis of gut microbiota in patients after ICI therapy has shown significant differences in the abundance of certain microbial communities between severe and mild irAEs, indicating that the diversity and abundance of gut microbiota may serve as important predictive indicators for the occurrence of irAEs (74). Particularly in patients with ICI-related colitis, changes in the gut microbiota are closely associated with disease progression (75). These findings highlight the potential role of gut microbiota in ICI therapy and offer a new perspective for a better understanding of the mechanisms, prevention, and treatment of irAEs.
2.3 Regulation of intestinal flora in anti-PD-1 immunotherapy
The effectiveness of utilizing ICBs to augment immunotherapy has been documented to diminish in the presence of antibiotics, while a heightened effectiveness has been noted in the presence of specific gut microbiota (76). The manipulation of gut microbiota composition has been shown to enhance the effectiveness of anti-PD-1/PD-L1 therapies (77). Numerous clinical studies have reported that FMT can regulate the intestinal microbiota in patients and effectively mitigating resistance to anti-PD-1/PD-L1 treatment in melanoma (78). The presence of an abundance of Clostridium or Difilibacterium in the gut of melanoma patients indicates a favorable response to anti-PD-1 therapy (8) (Table 2).
2.3.1 Enhancing the impact of intestinal flora on anti-PD-1 immunotherapy
Anti-PD-1/PD-L1 treatment inhibits the negative signaling of the PD-1 intracellular domain (ITIM, ITSM) transduction (11). Biomarkers highly correlated with anti-PD-1/PD-L1 therapeutic efficacy include PD-L1 expression levels and TILs status (83). Crucially, the gut microbiome plays a vital role in influencing the effectiveness of immunotherapy (84).
The transfer of fecal microbiota from cancer patients who exhibited a positive response to ICIs into germ-free mice or mice treated with broad-spectrum antibiotics has enhanced the anti-tumor efficacy of anti-PD-1. Conversely, FMT from non-responsive patients did not yield the same improvement (76). At present, through 16s rRNA gene sequencing, nine strains have been identified that substantially enhance the efficacy of anti-PD-1 (Figure 2).
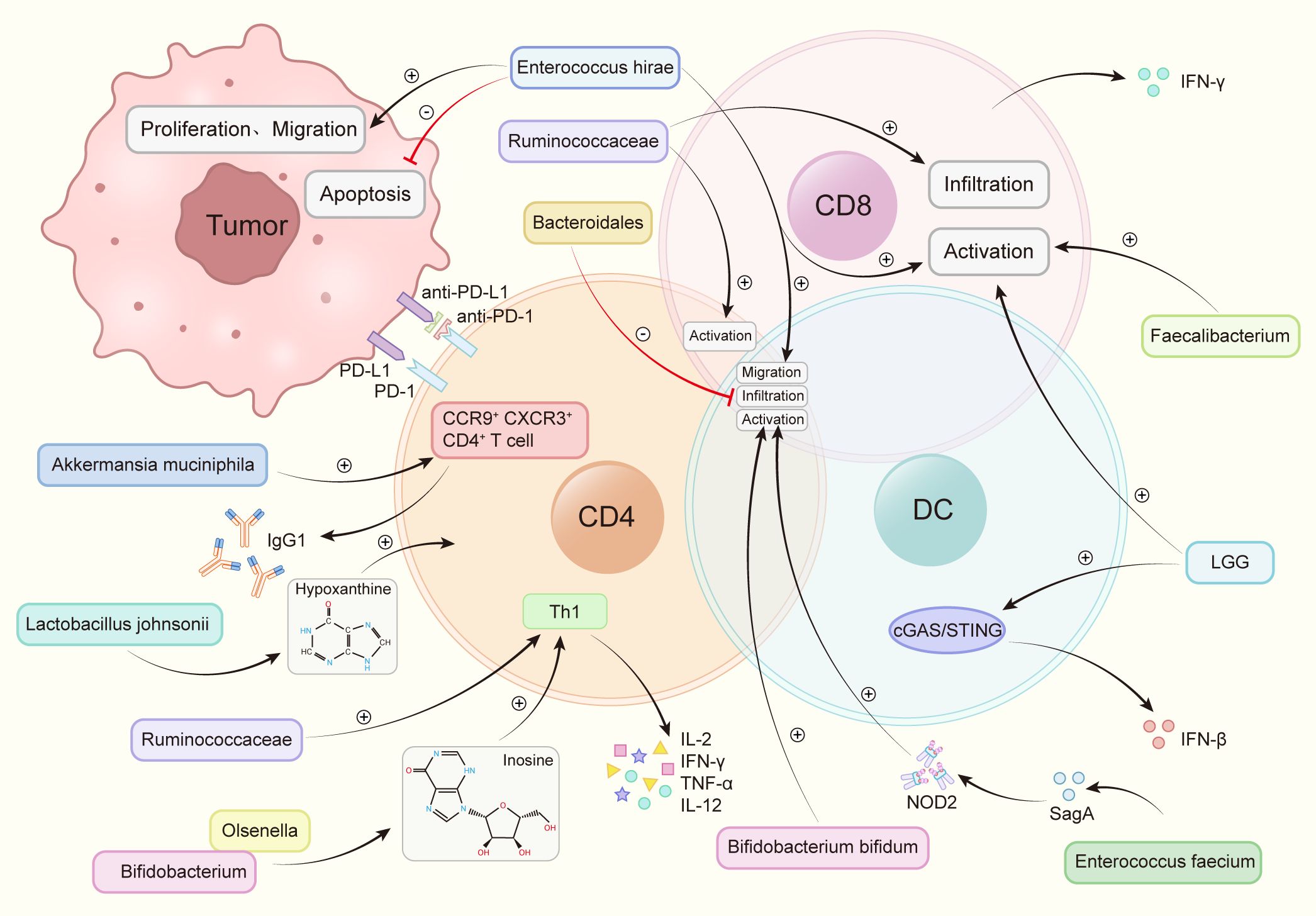
Figure 2 Intestinal flora increases the anti-PD-1 therapeutic effect by regulating immune cells. Akkermansia muciniphila accelerated the activity of CXCR3+CCR9+CD4+T cells, produced more IFN-γ. Bacteroidales inhibited the infiltration of B cells and T cells. Bifidobacterium and Olsenella produced metabolites inosine and increase the levels of interleukin-12 (IL-12), interferon γ (IFN-γ), TNF-a and interleukin-2 (IL-2). Bifidobacterium bifidum promotes the activation of T cells and B cells. Enterococcus faecium releases the metabolite SagA, produces NOD2 active polypeptide, and promotes the activation of B cells, T cells. Enterococcus hirae promotes tumor cell apoptosis, weakens the proliferation and migration of tumor cells, promotes the infiltration of T cells, B cells, promotes the activation of CD8+ T cells. Faecalibacterium promotes the activation of CD8+ T cells. Lactobacillus rhamnosus GG (LGG), induces IFN-β production, promotes CD8+ T cell activation via the cGAS/STING pathway in DC. Lactobacillus johnsonii enhances the immunotherapy effect through the metabolite hypoxanthine. Ruminococcaceae increases the number of effector T cells, induces the activation of effector CD4+ and CD8+ T cells, increases tumor CD8+ T cell infiltration.
2.3.1.1 Bifidobacterium bifidum
The promotion of DC maturation, increased cytokine secretion, and stimulation of the DC-IL-12-Th1-skewing immune response are reported as effects of Bifidobacterium bifidum. Moreover, this genus is found to enhance the overall function of DC, fostering the activation and survival of tumor-specific T cells. The Th1-skewing activation mediated by the DC-IL-12 axis contributes to the improvement of the therapeutic response to PD-1 blockade (47). Interferon-γ(IFN-γ) production can be improved by enhancing the biosynthesis of immune-stimulating molecules and metabolites, and oral bifidobacterium enhances tumor suppression to the same extent as specific antibody PD-L1 immunotherapy. Bifidobacterium bifidum significantly upregulates the secretion levels of IFN-γ and promotes the production of tumor-specific CD8+ T cells (47). Activation of CD8+ T cells and DCs results in enhanced efficacy of immunotherapy against melanoma, NSCLC, and Renal Cell Carcinoma (RCC) (47, 76, 85). Furthermore, Bifidobacterium pseudocatenulatum is found to secrete inosine, amplifying the impact of immunotherapy. The combination of inosine with immunotherapy significantly boosts the anti-tumor activity of T cells across various tumor types (44).
2.3.1.2 Akkermansia muciniphila(AKK):
A metagenomic examination of fecal samples from cancer patients reveals a robust connection between the clinical effectiveness of ICIs and the prevalence of AKK bacteria. Through the facilitation of the recruitment of CCR9+CXCR3+CD4+ T lymphocytes to the tumor tissue in mice, AKK bacteria can initiate an antigen-specific T cell response. This process promotes the production of IgG1 antibodies and reinstates the therapeutic efficacy of anti-PD-1 treatment for RCC, melanoma, and NSCLC in an IL-12-dependent manner (76).
2.3.1.3 Enterococcus faecium
Enterococcus faecium, expressed NlpC/p60 peptidoglycan hydrolase SagA, can release peptidoglycan fragments, produce Nucleotide-binding oligomerization domain 2 (NOD2) active polypeptides, activate innate immunity, induce a microenvironment conducive to anti-PD-1 immunotherapy, enhance the effectiveness of melanoma immunotherapy (86).
2.3.1.4 Enterococcus hirae
The antitumor mechanism of Enterococcus hirae involves inhibiting tumor proliferation, promoting a proinflammatory state, and promoting tumor cell apoptosis. Enterococcus hirae can further promote the migration of immune cells to promote anti-tumor effect of immune-targeted drugs. This genus inhibited the proliferation and metastasis of tumor cells like B16F10 melanoma, HT-29 human colon cancer, HepG-2 liver cancer cells (87). Studies have revealed that Enterococcus hirae can additionally induce CD8+ T cells, a function positively correlated with a good prognosis in HBV-associated hepatocellular carcinoma (HCC) (88).
2.3.1.5 Lactobacillus johnsonii
Lactobacillus Johnsoni produces the metabolite hypoxanthine, which then binds to inosine receptors, improving the effectiveness of anti-PD-1 therapy against MC38 colon cancer (44).
2.3.1.6 Olsenella
Much like the situation with Bifidobacterium pseudocatenulatum, Olsenella amplifies the therapeutic effects by metabolizing inosine. The immunotreatment-induced reduction in intestinal barrier function heightens the systemic transport of inosine, fostering the anti-tumor activation of Th1 cells. This robustly augments the anti-tumor functionality of T cells across diverse tumor types (44). Additionally, the effectiveness of anti-PD-1 therapy in NSCLC is elevated by augmenting the release of IL-12 and IFN-γ (80).
2.3.1.7 Lactobacillus rhamnosus GG (LGG)
LGG heightens the production of tumor-infiltrating DC cells and T cells. When administered in combination with anti-PD-1, LGG expedites interferon (IFN) production in DCs, enhancing the generation of anti-tumor CD8+ T cells. Consequently, this fosters immune cell activation and augments the immune effectiveness of anti-PD-1 therapy in colorectal cancer(CRC) and melanoma (82).
2.3.1.8 Ruminococcaceae
Ruminococcaceae improves the infiltration of CD8+ T cells within tumors, induces the differentiation of effector CD4+ and CD8+ T cells, and enhances the ratio of CD8+/FOXP3+CD4+ in tumor-infiltrating T cells. As a result, this increases the immune efficacy of anti-PD-1 therapy in melanoma and NSCLC (8, 89).
2.3.1.9 Faecalibacterium
Faecalibacterium is associated with the increase in CTL concentration in the tumor, subsequently potentiating the immune efficacy of anti-PD-1 immunotherapy on melanoma (8).
The aforementioned flora has all been revealed to activate immune cells through distinct mechanisms of action, thereby enhancing the efficacy of immunotherapy. Individuals who possess these microorganisms in their gut microbiome tend to be more responsive to PD-1 therapy compared to those who do not.
2.3.2 Inhibitory impact of intestinal flora on anti-PD-1 immunotherapy
Certain bacteria tend to accumulate in patients who show lower responsiveness to anti-PD-1 drugs, and these bacteria might directly diminish the effectiveness of PD-1 drugs. For example, Bacteroidales restrict the infiltration of lymphocytes and myeloid cells in tumors, dampen antigen presentation activity, and lead to impaired systemic immune and anti-tumor immune responses (8). In melanoma patients with short progression-free survival (PFS), there is a notable abundance of Bacteroides ovoides (90). Bacteroides ovatus, on the other hand, may influence the immune system by inducing IgA, among other mechanisms (91). A reduction in Bacteroides ovatus has been suggested to contribute to the enhancement of the efficacy of anti-PD-1 treatment in CRC (92). Conversely, Bacteroides thetaiotaomicron, Escherichia coli, Anaerotruncus colihominis, Ruminococcus obeum, and Roseburia intestinalis were found to be enriched in patients resistant to anti-PD-1 immunotherapy (8, 79). However, the specific mechanisms through which these intestinal flora diminish the efficacy of anti-PD-1 therapy remain to be further investigated.
2.3.3 Application of gut microbiota in mouse anti-tumor model.
Currently, several bacteria exhibit anti-tumor effects in mouse tumor models. It has been verified that both endogenous and externally introduced Akk significantly impede tumorigenesis in the Lewis lung cancer mouse model (93). Pasteurized AKK has been shown to mitigate colitis and colitis-associated CRC by regulating CTLs in a mouse model induced by dextran sulfate sodium (94, 95). Oral administration of Bifidobacterium alone enhances tumor control in a mouse model of B16 melanoma, and combined treatment with a PD-L1-specific antibody virtually eliminates tumor growth (47). Furthermore, B. adolescents effectively counters Dextran Sulfate Sodium Salt (DSS)-induced chronic colitis by stimulating protective Treg/Th2 responses and remodeling the gut microbiota. Regular administration of B. adolescents may enhance treatment outcomes in inflammatory bowel disease (IBD) (96). Bifidobacterium longum, Collinsella aerofaciens, and Enterococcus faecium have also demonstrated efficacy in alleviating colitis in mice, suggesting their potential use as alternative or adjunct therapies for IBD (97). In the MCA205 mouse tumor model, Enterococcus hirae is found to increase the intratumoral CD8/Treg ratio and enhance the effectiveness of the anti-cancer immunomodulator cyclophosphamide (CTX) (98). Lactobacillus johnsonii inhibited tumor growth in a mouse CRC model (99). Additionally, LGG recruits a substantial number of neutrophils and macrophages to the tumor site in a mouse orthotopic Bladder Cancer(BC) tumor model and is being explored as a potential substitute for BCG immunotherapy in the treatment of BC (100).
3 Discussion
The gut microbiota assumes a crucial role in regulating tumor responses to cancer immunotherapy (79, 92). Clinical approaches to improving the efficacy of tumor therapy have focused on providing microbial formulations with immune-stimulating properties (FMT and other more defined probiotic strains) or improving immunotherapy outcomes through targeted depletion of immunosuppressive bacteria. FMT can modify the balance between beneficial and detrimental bacteria in the gut microbiome during anti-PD-1 therapy, leading to an enhancement in therapeutic efficacy (92). The role of gut microbes is gaining prominence in the treatment of Non-Small Cell Lung Cancer (NSCLC), RCC, Urothelial Carcinoma (UC), and HCC (76, 101).
Currently, research regarding intestinal flora is primarily challenged by the following complications. Further study on immunomodulatory mechanism could facilitate the precise identification of immune-promoting and immunosuppressive bacterial strains or pathways, which could improve therapeutic efficacy, This may further aid in the avoidance of adverse events such as infections caused by FMT. The symbiotic nature of the microbiome means that it also influences tumor development, suggesting that individuals at high risk of cancer could be screened by the composition of their microbiome, and cancer be prevented by modulating the microbiome of high-risk individuals (102). At present, more accurate detection methods are required for detecting intestinal flora, these could be applied to the detection of intestinal flora in cancer patients to accurately predict the reaction or drug resistance of different intestinal flora to ICI. Similarly, correlation does not imply causation, and therefore, to overcome individual differences and biases, continued clinical trials are required to verify and ultimately achieve the deconvolution of any causal relationship between intestinal flora and immunotherapy effect to improve the effectiveness of ICB cancer treatment.
Author contributions
XG: Conceptualization, Investigation, Writing – original draft, Writing – review & editing. JJ: Conceptualization, Investigation, Methodology, Supervision, Writing – review & editing.
Funding
The author(s) declare financial support was received for the research, authorship, and/or publication of this article. This work was supported by the National Natural Science Foundation of China under Grant [32270955, 81972869]; Jiangsu Province Key R&D Plan Special Fund Project Clinical Frontier Technology under Grant [BE2022719]; Special research project of Jiangsu traditional Chinese medicine science and technology development plan under Grant [ZT202115]; Changzhou social development science and technology support project under Grant [CE20215030]; Jiangsu Provincial Medical Key Discipline under Grant [YXZDXK202236]; Changzhou Clinical Medical Center under Grant [CZZX202201].
Conflict of interest
The authors declare that the research was conducted in the absence of any commercial or financial relationships that could be construed as a potential conflict of interest.
Publisher’s note
All claims expressed in this article are solely those of the authors and do not necessarily represent those of their affiliated organizations, or those of the publisher, the editors and the reviewers. Any product that may be evaluated in this article, or claim that may be made by its manufacturer, is not guaranteed or endorsed by the publisher.
References
1. Joshi SS, Badgwell BD. Current treatment and recent progress in gastric cancer. CA Cancer J Clin. (2021) 71:264–79. doi: 10.3322/caac.21657
2. Sharma P, Allison JP. The future of immune checkpoint therapy. Science. (2015) 348:56–61. doi: 10.1126/science.aaa8172
3. Cristescu R, Mogg R, Ayers M, Albright A, Murphy E, Yearley J, et al. Pan-tumor genomic biomarkers for PD-1 checkpoint blockade-based immunotherapy. Science. (2018) 362. doi: 10.1126/science.aar3593
4. Kim JM, Chen DS. Immune escape to PD-L1/PD-1 blockade: seven steps to success (or failure). Ann Oncol. (2016) 27:1492–504. doi: 10.1093/annonc/mdw217
5. Wu Q, Li B, Li J, Sun S, Yuan J, Sun S. Cancer-associated adipocytes as immunomodulators in cancer. biomark Res. (2021) 9:2. doi: 10.1186/s40364-020-00257-6
6. Erny D, Dokalis N, Mezö C, Castoldi A, Mossad O, Staszewski O, et al. Microbiota-derived acetate enables the metabolic fitness of the brain innate immune system during health and disease. Cell Metab. (2021) 33(11):2260–76.e7. doi: 10.1016/j.cmet.2021.10.010
7. Takahashi D, Hoshina N, Kabumoto Y, Maeda Y, Suzuki A, Tanabe H, et al. Microbiota-derived butyrate limits the autoimmune response by promoting the differentiation of follicular regulatory T cells. EBioMedicine. (2020) 58:102913. doi: 10.1016/j.ebiom.2020.102913
8. Gopalakrishnan V, Spencer CN, Nezi L, Reuben A, Andrews MC, Karpinets TV, et al. Gut microbiome modulates response to anti-PD-1 immunotherapy in melanoma patients. Science. (2018) 359(6371):97–103. doi: 10.1126/science.aan4236
9. Ishida Y, Agata Y, Shibahara K, Honjo T. Induced expression of PD-1, a novel member of the immunoglobulin gene superfamily, upon programmed cell death. EMBO J. (1992) 11:3887–95. doi: 10.1002/embj.1992.11.issue-11
10. Filippone A, Lanza M, Mannino D, Raciti G, Colarossi C, Sciacca D, et al. PD1/PD-L1 immune checkpoint as a potential target for preventing brain tumor progression. Cancer Immunol Immunother. (2022) 71:2067–75. doi: 10.1007/s00262-021-03130-z
11. Daëron M, Jaeger S, Du Pasquier L, Vivier E. Immunoreceptor tyrosine-based inhibition motifs: a quest in the past and future. Immunol Rev. (2008) 224:11–43. doi: 10.1111/j.1600-065X.2008.00666.x
12. Arm JP, Nwankwo C, Austen KF. Molecular identification of a novel family of human Ig superfamily members that possess immunoreceptor tyrosine-based inhibition motifs and homology to the mouse gp49B1 inhibitory receptor. J Immunol. (1997) 159:2342–9. doi: 10.4049/jimmunol.159.5.2342
13. Sordo-Bahamonde C, Lorenzo-Herrero S, Granda-Díaz R, Martínez-Pérez A, Aguilar-García C, Rodrigo JP, et al. Beyond the anti-PD-1/PD-L1 era: promising role of the BTLA/HVEM axis as a future target for cancer immunotherapy. Mol Cancer. (2023) 22:142. doi: 10.1186/s12943-023-01845-4
14. Yamaguchi H, Hsu J-M, Yang W-H, Hung M-C. Mechanisms regulating PD-L1 expression in cancers and associated opportunities for novel small-molecule therapeutics. Nat Rev Clin Oncol. (2022) 19:287–305. doi: 10.1038/s41571-022-00601-9
15. Chen L, Han X. Anti-PD-1/PD-L1 therapy of human cancer: past, present, and future. J Clin Invest. (2015) 125:3384–91. doi: 10.1172/JCI80011
16. Ren Y, Kumar A, Das JK, Peng H-Y, Wang L, Balllard D, et al. Tumorous expression of NAC1 restrains antitumor immunity through the LDHA-mediated immune evasion. J Immunother Cancer. (2022) 10. doi: 10.1136/jitc-2022-004856
17. Kamphorst AO, Wieland A, Nasti T, Yang S, Zhang R, Barber DL, et al. Rescue of exhausted CD8 T cells by PD-1-targeted therapies is CD28-dependent. Science. (2017) 355:1423–7. doi: 10.1126/science.aaf0683
18. Hsieh RC-E, Krishnan S, Wu R-C, Boda AR, Liu A, Winkler M, et al. ATR-mediated CD47 and PD-L1 up-regulation restricts radiotherapy-induced immune priming and abscopal responses in colorectal cancer. Sci Immunol. (2022) 7:eabl9330. doi: 10.1126/sciimmunol.abl9330
19. Azuma T, Yao S, Zhu G, Flies AS, Flies SJ, Chen L. B7-H1 is a ubiquitous antiapoptotic receptor on cancer cells. Blood. (2008) 111:3635–43. doi: 10.1182/blood-2007-11-123141
20. Wu Y, Gu W, Li J, Chen C, Xu ZP. Silencing PD-1 and PD-L1 with nanoparticle-delivered small interfering RNA increases cytotoxicity of tumor-infiltrating lymphocytes. Nanomed (Lond). (2019) 14:955–67. doi: 10.2217/nnm-2018-0237
21. Wu X, Gu Z, Chen Y, Chen B, Chen W, Weng L, et al. Application of PD-1 blockade in cancer immunotherapy. Comput Struct Biotechnol J. (2019) 17:661–74. doi: 10.1016/j.csbj.2019.03.006
22. Finn RS, Qin S, Ikeda M, Galle PR, Ducreux M, Kim T-Y, et al. Atezolizumab plus bevacizumab in unresectable hepatocellular carcinoma. N Engl J Med. (2020) 382:1894–905. doi: 10.1056/NEJMoa1915745
23. Powles T, Park SH, Voog E, Caserta C, Valderrama BP, Gurney H, et al. Avelumab maintenance therapy for advanced or metastatic urothelial carcinoma. N Engl J Med. (2020) 383:1218–30. doi: 10.1056/NEJMoa2002788
24. Markham A, Keam SJ. Camrelizumab: first global approval. Drugs. (2019) 79:1355–61. doi: 10.1007/s40265-019-01167-0
25. Chen Z, Lu X, Koral K. The clinical application of camrelizumab on advanced hepatocellular carcinoma. Expert Rev Gastroenterol Hepatol. (2020) 14:1017–24. doi: 10.1080/17474124.2020.1807939
26. Migden MR, Rischin D, Schmults CD, Guminski A, Hauschild A, Lewis KD, et al. PD-1 blockade with cemiplimab in advanced cutaneous squamous-cell carcinoma. N Engl J Med. (2018) 379:341–51. doi: 10.1056/NEJMoa1805131
27. Mirza MR, Chase DM, Slomovitz BM, dePont Christensen R, Novák Z, Black D, et al. Dostarlimab for primary advanced or recurrent endometrial cancer. N Engl J Med. (2023) 388:2145–58. doi: 10.1056/NEJMoa2216334
28. Johnson ML, Cho BC, Luft A, Alatorre-Alexander J, Geater SL, Laktionov K, et al. Durvalumab with or without tremelimumab in combination with chemotherapy as first-line therapy for metastatic non-small-cell lung cancer: the phase III POSEIDON study. J Clin Oncol. (2023) 41:1213–27. doi: 10.1200/JCO.22.00975
29. Ieranò C, Righelli D, D'Alterio C, Napolitano M, Portella L, Rea G, et al. In PD-1+ human colon cancer cells NIVOLUMAB promotes survival and could protect tumor cells from conventional therapies. J Immunother Cancer. (2022) 10(6264):1079–84 doi: 10.1136/jitc-2021-004032
30. Friedman CF, Spencer C, Cabanski CR, Panageas KS, Wells DK, Ribas A, et al. Ipilimumab alone or in combination with nivolumab in patients with advanced melanoma who have progressed or relapsed on PD-1 blockade: clinical outcomes and translational biomarker analyses. J Immunother Cancer. (2022) 10(21):5338–56.e21. doi: 10.1136/jitc-2021-003853
31. Mei MG, Lee HJ, Palmer JM, Chen R, Tsai N-C, Chen L, et al. Response-adapted anti-PD-1-based salvage therapy for Hodgkin lymphoma with nivolumab alone or in combination with ICE. Blood. (2022) 139:3605–16. doi: 10.1182/blood.2022015423
32. Liu WN, So WY, Harden SL, Fong SY, Wong MXY, Tan WWS, et al. Successful targeting of PD-1/PD-L1 with chimeric antigen receptor-natural killer cells and nivolumab in a humanized mouse cancer model. Sci Adv. (2022) 8:eadd1187. doi: 10.1126/sciadv.add1187
33. Shen X, Zhao B. Efficacy of PD-1 or PD-L1 inhibitors and PD-L1 expression status in cancer: meta-analysis. BMJ. (2018) 362:k3529. doi: 10.1136/bmj.k3529
34. Kwon M, An M, Klempner SJ, Lee H, Kim K-M, Sa JK, et al. Determinants of response and intrinsic resistance to PD-1 blockade in microsatellite instability-high gastric cancer. Cancer Discovery. (2021) 11:2168–85. doi: 10.1158/2159-8290.CD-21-0219
35. Fuchs CS, Doi T, Jang RW, Muro K, Satoh T, MaChado M, et al. Safety and efficacy of pembrolizumab monotherapy in patients with previously treated advanced gastric and gastroesophageal junction cancer: phase 2 clinical KEYNOTE-059 trial. JAMA Oncol. (2018) 4:e180013. doi: 10.1001/jamaoncol.2018.0013
36. Tjulandin S, Demidov L, Moiseyenko V, Protsenko S, Semiglazova T, Odintsova S, et al. Novel PD-1 inhibitor prolgolimab: expanding non-resectable/metastatic melanoma therapy choice. Eur J Cancer. (2021) 149:222–32. doi: 10.1016/j.ejca.2021.02.030
37. Shi Y, Su H, Song Y, Jiang W, Sun X, Qian W, et al. Circulating tumor DNA predicts response in Chinese patients with relapsed or refractory classical hodgkin lymphoma treated with sintilimab. EBioMedicine. (2020) 54:102731. doi: 10.1016/j.ebiom.2020.102731
38. Wang J, Lu S, Yu X, Hu Y, Sun Y, Wang Z, et al. Tislelizumab plus chemotherapy vs chemotherapy alone as first-line treatment for advanced squamous non-small-cell lung cancer: A phase 3 randomized clinical trial. JAMA Oncol. (2021) 7:709–17. doi: 10.1001/jamaoncol.2021.0366
39. Zhang L, Hao B, Geng Z, Geng Q. Toripalimab: the first domestic anti-tumor PD-1 antibody in China. Front Immunol. (2021) 12:730666. doi: 10.3389/fimmu.2021.730666
40. Lin N, Zhang M, Bai H, Liu H, Cui J, Ke X, et al. Efficacy and safety of GLS-010 (zimberelimab) in patients with relapsed or refractory classical Hodgkin lymphoma: A multicenter, single-arm, phase II study. Eur J Cancer. (2022) 164:117–26. doi: 10.1016/j.ejca.2021.07.021
41. Smyth MJ, Ngiow SF, Ribas A, Teng MWL. Combination cancer immunotherapies tailored to the tumour microenvironment. Nat Rev Clin Oncol. (2016) 13:143–58. doi: 10.1038/nrclinonc.2015.209
42. Yi M, Zheng X, Niu M, Zhu S, Ge H, Wu K. Combination strategies with PD-1/PD-L1 blockade: current advances and future directions. Mol Cancer. (2022) 21:28. doi: 10.1186/s12943-021-01489-2
43. Mirji G, Worth A, Bhat SA, El Sayed M, Kannan T, Goldman AR, et al. The microbiome-derived metabolite TMAO drives immune activation and boosts responses to immune checkpoint blockade in pancreatic cancer. Sci Immunol. (2022) 7:eabn0704. doi: 10.1126/sciimmunol.abn0704
44. Mager LF, Burkhard R, Pett N, Cooke NCA, Brown K, Ramay H, et al. Microbiome-derived inosine modulates response to checkpoint inhibitor immunotherapy. Science. (2020) 369:1481–9. doi: 10.1126/science.abc3421
45. Hezaveh K, Shinde RS, Klötgen A, Halaby MJ, Lamorte S, Ciudad MT, et al. Tryptophan-derived microbial metabolites activate the aryl hydrocarbon receptor in tumor-associated macrophages to suppress anti-tumor immunity. Immunity. (2022) 55(3):511–24. doi: 10.1016/j.immuni.2022.01.006
46. Böttcher JP, Bonavita E, Chakravarty P, Blees H, Cabeza-Cabrerizo M, Sammicheli S, et al. NK Cells Stimulate Recruitment of cDC1 into the Tumor Microenvironment Promoting Cancer Immune Control. Cell. (2018) 172(5):988–1000.e7. doi: 10.1016/j.cell.2018.01.004
47. Sivan A, Corrales L, Hubert N, Williams JB, Aquino-Michaels K, Earley ZM, et al. Commensal Bifidobacterium promotes antitumor immunity and facilitates anti-PD-L1 efficacy. Science. (2015) 350:1084–9. doi: 10.1126/science.aac4255
48. Vétizou M, Pitt JM, Daillère R, Lepage P, Waldschmitt N, Flament C, et al. Anticancer immunotherapy by CTLA-4 blockade relies on the gut microbiota. Science. (2015) 350:1079–84. doi: 10.1126/science.aad1329
49. Lam KC, Araya RE, Huang A, Chen Q, Di Modica M, Rodrigues RR, et al. Microbiota triggers STING-type I IFN-dependent monocyte reprogramming of the tumor microenvironment. Cell. (2021) 184. doi: 10.1016/j.cell.2021.09.019
50. Shi Y, Zheng W, Yang K, Harris KG, Ni K, Xue L, et al. Intratumoral accumulation of gut microbiota facilitates CD47-based immunotherapy via STING signaling. J Exp Med. (2020) 217(7903):907–12. doi: 10.1084/jem.20192282
51. Deng H, Li Z, Tan Y, Guo Z, Liu Y, Wang Y, et al. A novel strain of Bacteroides fragilis enhances phagocytosis and polarises M1 macrophages. Sci Rep. (2016) 6:29401. doi: 10.1038/srep29401
52. Qiu Y, Jiang Z, Hu S, Wang L, Ma X, Yang X. Lactobacillus plantarum Enhanced IL-22 Production in Natural Killer (NK) Cells That Protect the Integrity of Intestinal Epithelial Cell Barrier Damaged by Enterotoxigenic Escherichia coli. Int J Mol Sci. (2017) 18. doi: 10.3390/ijms18112409
53. Rizvi ZA, Dalal R, Sadhu S, Kumar Y, Kumar S, Gupta SK, et al. High-salt diet mediates interplay between NK cells and gut microbiota to induce potent tumor immunity. Sci Adv. (2021) 7:eabg5016. doi: 10.1126/sciadv.abg5016
54. Baruch EN, Youngster I, Ben-Betzalel G, Ortenberg R, Lahat A, Katz L, et al. Fecal microbiota transplant promotes response in immunotherapy-refractory melanoma patients. Science. (2021) 371:602–9. doi: 10.1126/science.abb5920
55. Atarashi K, Tanoue T, Ando M, Kamada N, Nagano Y, Narushima S, et al. Th17 cell induction by adhesion of microbes to intestinal epithelial cells. Cell. (2015) 163:367–80. doi: 10.1016/j.cell.2015.08.058
56. Vanaudenaerde BM, Verleden SE, Vos R, De Vleeschauwer SI, Willems-Widyastuti A, Geenens R, et al. Innate and adaptive interleukin-17-producing lymphocytes in chronic inflammatory lung disorders. Am J Respir Crit Care Med. (2011) 183:977–86. doi: 10.1164/rccm.201007-1196PP
57. Smith PM, Howitt MR, Panikov N, Michaud M, Gallini CA, Bohlooly-Y M, et al. The microbial metabolites, short-chain fatty acids, regulate colonic Treg cell homeostasis. Science. (2013) 341:569–73. doi: 10.1126/science.1241165
58. Round JL, Lee SM, Li J, Tran G, Jabri B, Chatila TA, et al. The Toll-like receptor 2 pathway establishes colonization by a commensal of the human microbiota. Science. (2011) 332:974–7. doi: 10.1126/science.1206095
59. Furusawa Y, Obata Y, Fukuda S, Endo TA, Nakato G, Takahashi D, et al. Commensal microbe-derived butyrate induces the differentiation of colonic regulatory T cells. Nature. (2013) 504:446–50. doi: 10.1038/nature12721
60. Monte MJ, Marin JJG, Antelo A, Vazquez-Tato J. Bile acids: chemistry, physiology, and pathophysiology. World J Gastroenterol. (2009) 15:804–16. doi: 10.3748/wjg.15.804
61. Harjes U. Tumour-reactive T cells work remotely using IFNγ. Nat Rev Cancer. (2020) 20:261. doi: 10.1038/s41568-020-0255-0
62. Wang T, Gnanaprakasam JNR, Chen X, Kang S, Xu X, Sun H, et al. Inosine is an alternative carbon source for CD8+-T-cell function under glucose restriction. Nat Metab. (2020) 2:635–47. doi: 10.1038/s42255-020-0219-4
63. Nomura M, Nagatomo R, Doi K, Shimizu J, Baba K, Saito T, et al. Association of short-chain fatty acids in the gut microbiome with clinical response to treatment with nivolumab or pembrolizumab in patients with solid cancer tumors. JAMA Netw Open. (2020) 3:e202895. doi: 10.1001/jamanetworkopen.2020.2895
64. Zagato E, Pozzi C, Bertocchi A, Schioppa T, Saccheri F, Guglietta S, et al. Endogenous murine microbiota member Faecalibaculum rodentium and its human homologue protect from intestinal tumour growth. Nat Microbiol. (2020) 5:511–24. doi: 10.1038/s41564-019-0649-5
65. He Y, Fu L, Li Y, Wang W, Gong M, Zhang J, et al. Gut microbial metabolites facilitate anticancer therapy efficacy by modulating cytotoxic CD8+ T cell immunity. Cell Metab. (2021) 33(2):352–9. doi: 10.1016/j.cmet.2021.03.002
66. Donohoe DR, Garge N, Zhang X, Sun W, O'Connell TM, Bunger MK, et al. The microbiome and butyrate regulate energy metabolism and autophagy in the mammalian colon. Cell Metab. (2011) 13:517–26. doi: 10.1016/j.cmet.2011.02.018
67. Gnanaprakasam JNR, Estrada-Muñiz E, Vega L. The anacardic 6-pentadecyl salicylic acid induces macrophage activation via the phosphorylation of ERK1/2, JNK, P38 kinases and NF-κB. Int Immunopharmacol. (2015) 29:808–17. doi: 10.1016/j.intimp.2015.08.038
68. Gnanaprakasam JNR, López-Bañuelos L, Vega L. Anacardic 6-pentadecyl salicylic acid induces apoptosis in breast cancer tumor cells, immunostimulation in the host and decreases blood toxic effects of taxol in an animal model. Toxicol Appl Pharmacol. (2021) 410:115359. doi: 10.1016/j.taap.2020.115359
69. Paik D, Yao L, Zhang Y, Bae S, D'Agostino GD, Zhang M, et al. Human gut bacteria produce TH17-modulating bile acid metabolites. Nature. (2022) 603:907–12. doi: 10.1038/s41586-022-04480-z
70. Campbell C, McKenney PT, Konstantinovsky D, Isaeva OI, Schizas M, Verter J, et al. Bacterial metabolism of bile acids promotes generation of peripheral regulatory T cells. Nature. (2020) 581:475–9. doi: 10.1038/s41586-020-2193-0
71. Ramos-Casals M, Brahmer JR, Callahan MK, Flores-Chávez A, Keegan N, Khamashta MA, et al. Immune-related adverse events of checkpoint inhibitors. Nat Rev Dis Primers. (2020) 6:38. doi: 10.1038/s41572-020-0160-6
72. Liu X, Tang H, Zhou Q, Zeng Y, Lu B, Chen D, et al. Gut microbiota composition in patients with advanced Malignancies experiencing immune-related adverse events. Front Immunol. (2023) 14:1109281. doi: 10.3389/fimmu.2023.1109281
73. Liu W, Ma F, Sun B, Liu Y, Tang H, Luo J, et al. Intestinal microbiome associated with immune-related adverse events for patients treated with anti-PD-1 inhibitors, a real-world study. Front Immunol. (2021) 12:756872. doi: 10.3389/fimmu.2021.756872
74. Chau J, Yadav M, Liu B, Furqan M, Dai Q, Shahi S, et al. Prospective correlation between the patient microbiome with response to and development of immune-mediated adverse effects to immunotherapy in lung cancer. BMC Cancer. (2021) 21:808. doi: 10.1186/s12885-021-08530-z
75. Chaput N, Lepage P, Coutzac C, Soularue E, Le Roux K, Monot C, et al. Baseline gut microbiota predicts clinical response and colitis in metastatic melanoma patients treated with ipilimumab. Ann Oncol. (2017) 28:1368–79. doi: 10.1093/annonc/mdx108
76. Routy B, Le Chatelier E, Derosa L, Duong CPM, Alou MT, Daillère R, et al. Gut microbiome influences efficacy of PD-1-based immunotherapy against epithelial tumors. Science. (2018) 359:91–7. doi: 10.1126/science.aan3706
77. Huang J, Liu D, Wang Y, Liu L, Li J, Yuan J, et al. Ginseng polysaccharides alter the gut microbiota and kynurenine/tryptophan ratio, potentiating the antitumour effect of antiprogrammed cell death 1/programmed cell death ligand 1 (anti-PD-1/PD-L1) immunotherapy. Gut. (2022) 71:734–45. doi: 10.1136/gutjnl-2020-321031
78. Davar D, Dzutsev AK, McCulloch JA, Rodrigues RR, Chauvin J-M, Morrison RM, et al. Fecal microbiota transplant overcomes resistance to anti-PD-1 therapy in melanoma patients. Science. (2021) 371:595–602. doi: 10.1126/science.abf3363
79. Matson V, Fessler J, Bao R, Chongsuwat T, Zha Y, Alegre M-L, et al. The commensal microbiome is associated with anti-PD-1 efficacy in metastatic melanoma patients. Science. (2018) 359:104–8. doi: 10.1126/science.aao3290
80. He D, Li X, An R, Wang L, Wang Y, Zheng S, et al. Response to PD-1-based immunotherapy for non-small cell lung cancer altered by gut microbiota. Oncol Ther. (2021) 9:647–57. doi: 10.1007/s40487-021-00171-3
81. Miller PL, Carson TL. Mechanisms and microbial influences on CTLA-4 and PD-1-based immunotherapy in the treatment of cancer: a narrative review. Gut Pathogens. (2020) 12:43. doi: 10.1186/s13099-020-00381-6
82. Si W, Liang H, Bugno J, Xu Q, Ding X, Yang K, et al. Lactobacillus rhamnosus GG induces cGAS/STING- dependent type I interferon and improves response to immune checkpoint blockade. Gut. (2022) 71:521–33. doi: 10.1136/gutjnl-2020-323426
83. Yi M, Jiao D, Xu H, Liu Q, Zhao W, Han X, et al. Biomarkers for predicting efficacy of PD-1/PD-L1 inhibitors. Mol Cancer. (2018) 17:129. doi: 10.1186/s12943-018-0864-3
84. Li X, Zhang S, Guo G, Han J, Yu J. Gut microbiome in modulating immune checkpoint inhibitors. EBioMedicine. (2022) 82:104163. doi: 10.1016/j.ebiom.2022.104163
85. Lee S-H, Cho S-Y, Yoon Y, Park C, Sohn J, Jeong J-J, et al. Bifidobacterium bifidum strains synergize with immune checkpoint inhibitors to reduce tumour burden in mice. Nat Microbiol. (2021) 6:277–88. doi: 10.1038/s41564-020-00831-6
86. Griffin ME, Espinosa J, Becker JL, Luo J-D, Carroll TS, Jha JK, et al. Enterococcus peptidoglycan remodeling promotes checkpoint inhibitor cancer immunotherapy. Science. (2021) 373:1040–6. doi: 10.1126/science.abc9113
87. Yang Q, He Y, Tian L, Zhang Z, Qiu L, Tao X, et al. Anti-tumor effect of infant-derived Enterococcus via the inhibition of proliferation and inflammation as well as the promotion of apoptosis. Food Funct. (2023) 14:2223–38. doi: 10.1039/D2FO03045D
88. Rong Y, Dong Z, Hong Z, Jin Y, Zhang W, Zhang B, et al. Reactivity toward Bifidobacterium longum and Enterococcus hirae demonstrate robust CD8+ T cell response and better prognosis in HBV-related hepatocellular carcinoma. Exp Cell Res. (2017) 358:352–9. doi: 10.1016/j.yexcr.2017.07.009
89. Messaoudene M, Pidgeon R, Richard C, Ponce M, Diop K, Benlaifaoui M, et al. A natural polyphenol exerts antitumor activity and circumvents anti-PD-1 resistance through effects on the gut microbiota. Cancer Discovery. (2022) 12:1070–87. doi: 10.1158/2159-8290.CD-21-0808
90. Peters BA, Wilson M, Moran U, Pavlick A, Izsak A, Wechter T, et al. Relating the gut metagenome and metatranscriptome to immunotherapy responses in melanoma patients. Genome Med. (2019) 11:61. doi: 10.1186/s13073-019-0672-4
91. Yang C, Mogno I, Contijoch EJ, Borgerding JN, Aggarwala V, Li Z, et al. Fecal IgA levels are determined by strain-level differences in bacteroides ovatus and are modifiable by gut microbiota manipulation. Cell Host Microbe. (2020) 27. doi: 10.1016/j.chom.2020.01.016
92. Huang J, Zheng X, Kang W, Hao H, Mao Y, Zhang H, et al. Metagenomic and metabolomic analyses reveal synergistic effects of fecal microbiota transplantation and anti-PD-1 therapy on treating colorectal cancer. Front Immunol. (2022) 13:874922. doi: 10.3389/fimmu.2022.874922
93. Zhu Z, Cai J, Hou W, Xu K, Wu X, Song Y, et al. Microbiome and spatially resolved metabolomics analysis reveal the anticancer role of gut Akkermansia muciniphila by crosstalk with intratumoral microbiota and reprogramming tumoral metabolism in mice. Gut Microbes. (2023) 15:2166700. doi: 10.1080/19490976.2023.2166700
94. Wang L, Tang L, Feng Y, Zhao S, Han M, Zhang C, et al. A purified membrane protein from Akkermansia muciniphila or the pasteurised bacterium blunts colitis associated tumourigenesis by modulation of CD8+ T cells in mice. Gut. (2020) 69:1988–97. doi: 10.1136/gutjnl-2019-320105
95. Qu S, Fan L, Qi Y, Xu C, Hu Y, Chen S, et al. Akkermansia muciniphila alleviates dextran sulfate sodium (DSS)-induced acute colitis by NLRP3 activation. Microbiol Spectr. (2021) 9:e0073021. doi: 10.1128/Spectrum.00730-21
96. Fan L, Qi Y, Qu S, Chen X, Li A, Hendi M, et al. B. adolescentis ameliorates chronic colitis by regulating Treg/Th2 response and gut microbiota remodeling. Gut Microbes. (2021) 13:117–26. doi: 10.1080/19490976.2020.1826746
97. Yao S, Zhao Z, Wang W, Liu X. Bifidobacterium longum: protection against inflammatory bowel disease. J Immunol Res. (2021) 2021:8030297. doi: 10.1155/2021/8030297
98. Daillère R, Vétizou M, Waldschmitt N, Yamazaki T, Isnard C, Poirier-Colame V, et al. Enterococcus hirae and Barnesiella intestinihominis Facilitate Cyclophosphamide-Induced Therapeutic Immunomodulatory Effects. Immunity. (2016) 45:931–43. doi: 10.1016/j.immuni.2016.09.009
99. Cao Q, Zhao M, Su Y, Liu S, Lin Y, Da H, et al. Chronic stress dampens Lactobacillus johnsonii-mediated tumor suppression to enhance colorectal cancer progression. Cancer Res. (2024) 382(20): 1894–905. doi: 10.1158/0008-5472.CAN-22-3705
100. Seow SW, Cai S, Rahmat JN, Bay BH, Lee YK, Chan YH, et al. Lactobacillus rhamnosus GG induces tumor regression in mice bearing orthotopic bladder tumors. Cancer Sci. (2010) 101:751–8. doi: 10.1111/j.1349-7006.2009.01426.x
101. Zheng Y, Wang T, Tu X, Huang Y, Zhang H, Tan D, et al. Gut microbiome affects the response to anti-PD-1 immunotherapy in patients with hepatocellular carcinoma. J Immunother Cancer. (2019) 7:193. doi: 10.1186/s40425-019-0650-9
Keywords: PD-1, anti-PD-1, intestinal flora, ICI, immunotherapy
Citation: Gao X and Jiang J (2024) Exploring the regulatory mechanism of intestinal flora based on PD-1 receptor/ligand targeted cancer immunotherapy. Front. Immunol. 15:1359029. doi: 10.3389/fimmu.2024.1359029
Received: 20 December 2023; Accepted: 18 March 2024;
Published: 28 March 2024.
Edited by:
Leming Sun, Northwestern Polytechnical University, ChinaReviewed by:
Ravi Verma, Baylor College of Medicine, United StatesRajeev Kumar Pandey, Thermo Fisher Scientific, India
Copyright © 2024 Gao and Jiang. This is an open-access article distributed under the terms of the Creative Commons Attribution License (CC BY). The use, distribution or reproduction in other forums is permitted, provided the original author(s) and the copyright owner(s) are credited and that the original publication in this journal is cited, in accordance with accepted academic practice. No use, distribution or reproduction is permitted which does not comply with these terms.
*Correspondence: Jingting Jiang, jiangjingting@suda.edu.cn