- 1Institute of Biology, University of Graz, Graz, Austria
- 2Tvärminne Zoological Station, University of Helsinki, Hanko, Finland
- 3Ecology and Genetics Research Unit, University of Oulu, Oulu, Finland
Ants face unique challenges regarding pathogens, as the sociality which has allowed them to form large and complex colonies also raises the potential for transmission of disease within them. To cope with the threat of pathogens, ants have developed a variety of behavioral and physiological strategies. One of these strategies is self-medication, in which animals use biologically active compounds to combat pathogens in a way which would be harmful in the absence of infection. Formica fusca are the only ants that have previously been shown to successfully self-medicate against an active infection caused by a fungal pathogen by supplementing their diet with food containing hydrogen peroxide. Here, we build on that research by investigating how the prevalence of disease in colonies of F. fusca affects the strength of the self-medication response. We exposed either half of the workers of each colony or all of them to a fungal pathogen and offered them different combinations of diets. We see that workers of F. fusca engage in self-medication behavior even if exposed to a low lethal dose of a pathogen, and that the strength of that response is affected by the prevalence of the disease in the colonies. We also saw that the infection status of the individual foragers did not significantly affect their decision to forage on either control food or medicinal food as uninfected workers were also foraging on hydrogen peroxide food, which opens up the possibility of kin medication in partially infected colonies. Our results further affirm the ability of ants to self-medicate against fungal pathogens, shed new light on plasticity of self-medication and raise new questions to be investigated on the role self-medication has in social immunity.
Introduction
Eusociality has been a key driver in why ants have become some of the most successful insects in the world, inhabiting most terrestrial environments on the planet (1). The high degree of cooperation and the division of labor of ants benefit the colonies in the form of more effective brood care, foraging and protection of resources, which has allowed ant colonies to grow large, often comprising of hundreds of thousands or even millions of individuals (2). Sociality also comes with costs. Ants live in dense colonies with a low genetic diversity among frequently interacting nestmates, which creates favorable conditions for pathogens to spread (3, 4). Ants also forage and interact with a wide variety of organisms in their environment, which makes them vulnerable to exposure to pathogens (5). The combination of high risk of exposure as well as favorable conditions for transmission of pathogens makes ants particularly susceptible for outbreaks of infectious diseases (3).
To cope with the high pathogen pressure they face, a wide variety of both physiological and behavioral strategies have evolved in ants. Ants share an innate immunity with other insects as a first line of defense, but also have unique adaptations in the form of anti-microbial gland exudates (6–8). Ants initially try to avoid pathogens, but if exposed they can mitigate the effects of them through hygienic behaviors such as grooming of both self and nestmates (9) and contain pathogen spread by corpse processing and cemetery formation and management (10). The extreme level of sociality also means that the anti-pathogenic strategies of individual ants in a colony lead to a whole which is larger than the sum of its parts, providing protection against pathogens on a colony level as a form of social immunity (11, 12).
As a complementary strategy, ants could potentially use self-medication to stave off the threat of disease. Self-medication is the use of biologically active compounds by animals to fight off disease in a way which would be harmful or costly for uninfected individuals (13, 14). The self-medication response is plastic and can take place either before infection takes place (prophylactic) or after (therapeutic) (15), and can be directed either at self or kin (13, 16). To be qualified as true self-medication, the behavior needs to fulfill four criteria: the compound must be deliberately contacted (I), the compound must be harmful for the pathogen (II), the behavior must lead to a higher fitness for infected individuals (III), the use of the compound must be costly for uninfected individuals (IV) (13). However, some studies using vertebrates have adapted slightly different criteria, possibly due to difficulties in carrying out field studies compared to controlled laboratory studies using insects (14, 16, 17).
Evidence of self-medication behavior in ants and other social insects is still scarce (18–20) and to date the clearest evidence for self-medication has been provided in solitary insects (15, 21–24). So far, there is only one demonstrated case of therapeutic self-medication in ants (20), where workers infected with a highly lethal dose of a generalist entomopathogenic fungus consumed food laced with hydrogen peroxide (H2O2), a reactive oxygen species (ROS) which is part of their natural immune responses (25–27), to increase their chances of survival. There is also a case to be made that the collection and processing of resin into the nest material is a form of prophylactic self-medication in ants (8). However, the extent of self-medication responses and how they play out in the colony foraging dynamics is still unknown in ants.
Due to the eusociality of ants, individual self-medication responses are likely to engage colony level processes which regulate the collection and distribution of medicinal compounds in the colony. The division of labor in ant colony means that the foragers, who commonly make up around 10–20% of the workers in the colony (1, 28–30), provide nutrition for the entire nest, and tune their foraging choices according to the nutritional needs of their nestmates, influenced by feedback-loops of food acceptance rates by other nestmates (31). The food is then distributed according to which groups or individuals have a higher need for specific nutrients (32). Therefore, if nestmates are battling disease, the nutritional needs of the colony change, and foragers should respond by foraging on medicinal compounds regardless of their individual infection status to distribute medicine to infected nestmates as a form of kin medication (13).
In this study, we tested how different levels of infection by the generalist entomopathogenic fungus Beauveria bassiana affect the self-medication behavior of Formica fusca ants in the form of foraging choices of colonies, and whether they lead to enhanced survival against the pathogen. The use of different levels of pathogen exposure combined with the use of either fixed food diets or a diet choice allowed us to explore questions relating to the plasticity of the self-medication response. We predict that as the prevalence of disease in a colony increases, so does the strength of the self-medication response. By using colonies in which only part of the workers is infected, we could also investigate a potential kin medication component to the self-medication response. We also predict that both infected and infection free workers will forage on medicinal food, with the task of distributing it to the rest of the colony. We used a dose of pathogen which had a low effect on mortality, which is considered to be more naturally relevant (33). Studies using highly lethal doses have also been shown to negatively affect normal immunologic behaviors such as grooming (34).
Materials and Methods
We collected 47 wild nests of Formica fusca from around the Hanko peninsula in southern Finland (59°54'46.9“N, 23°15'53.8”E). Formica fusca is a weakly polygynous species in this area, with between 40 and 45% of the nests being monogyne, and queen numbers of the nests varying between 1 and 26 (35, 36). Some of the colonies we collected contained several queens, but as precise numbers of queens were not relevant to this study, we did not aim to excavate whole colonies, but taking subsamples and allowing the rest of the nest to survive.
From the nests we made a total of 133 experimental colonies of one queen and 100 workers each and placed them in separate plastic containers (8 × 15 × 10 cm). Whenever several experimental colonies were made of a single nest, they were used in the different setups. The walls and lids of each container was lined with a 20% (w/v) mixture of ethanol and talcum powder to keep the ants from escaping (37). A small portion of the lid was cut off to get a clear view of the foraging area of the containers. Each container had a 2 cm deep plaster cast base with a 1 cm deep circular indentation (3 cm Ø) as a nesting site with a ceramic tile (4 × 4 cm) on top for shelter.
Experimental Setup
To study how the strength of the self-medication response of a colony changes with increasing disease prevalence, we set up an experiment using three different ratios of infected vs. non-infected workers and exposed all the treatments to three different feeding regimes. This way, we could study how the foraging activity changes on a colony level as well as how the infection status of individual foragers affects their foraging choices.
The 133 colonies were divided into three groups to be used in the three different infection ratios. Forty-four colonies were used as infection free sham-treated controls (0%), in 45 colonies half of the workers were infected and half sham-treated (50%), in the remaining 44 colonies, all of the workers were infected (100%). Queens were not subjected to any treatment in any of the colonies. To be able to distinguish infected ants from infection free ants in the 50% infected colonies, half of the workers were marked with a red dot on their abdomen using marking color for marking honeybee queens (Hunajayhtymä, product code: 16006). The marked individuals in the colonies were subjected to either the infection or control treatment to limit any differences caused by handling. To ensure that the marking would not cause differences in behavior due to handling in relation to the colonies in the other treatments, we marked half the workers in all of the experimental colonies the same way. The marking of ants also functioned as a blinding measure during the observational phase to limit observational bias.
Fifteen colonies of each infection ratio were given two Eppendorf tube caps of the Bhatkar and Whitcomb diet (38) as the control food. Fifteen colonies were given a food choice of one cap of control food and one cap containing control food containing 4% H2O2, the same concentration previously used by Bos et al. (20). The remaining 15 colonies were given a diet of two caps containing 4% H2O2 food (ROS diet). The experimental setup is visualized in Figure 1. Fresh food in Eppendorf caps were provided each morning at 11 a.m. Each colony also had an Eppendorf tube with water and cotton to drink from.
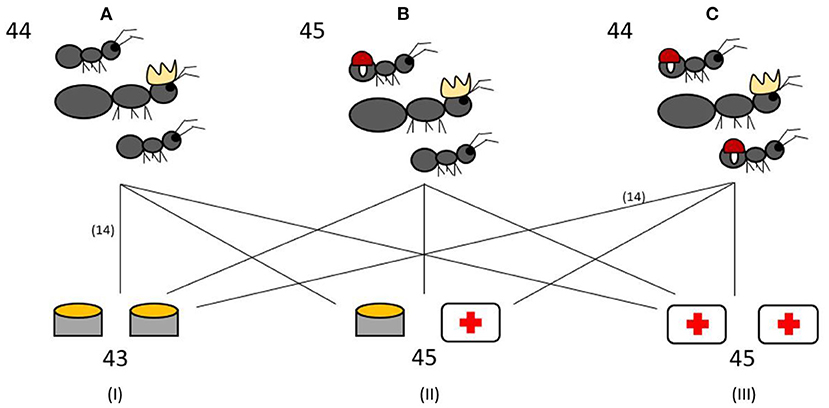
Figure 1. Experimental setup. The 133 experimental colonies were split into three different infection treatments: 0% workers infected (A), 50% workers infected (B), 100% workers infected (C). Colonies from each infection treatment were further assigned to one of three different diets: control food diet (I), food choice between control food and ROS food (4% H2O2) as medicine (II), ROS diet (III). The numbers indicate the total number of experimental colonies used in the different treatments and diets. The lines all represent 15 colonies, with the exception of two groups which have 14 colonies.
The colonies were given a day to settle in their nests, with both water and control food being provided, before we started to observe foraging behavior. The foraging was observed four times a day (at 10, 14, 18 and 22) for a total duration of 6 days. At each observation period, the colonies were photographed through the opening in the lid and the foraging was determined by analyzing the photographs. If an ant had contact with the food with either antenna or a leg, it was deemed as a forager. The strict criterion for foraging was to prevent other activity to be assessed as foraging. From each photograph, every forager was counted. We also made a note on what food was being foraged and whether the ants were marked or not. Photographs were analyzed blind with respect to the infection and diet treatment.
Infections
Beauveria bassiana is a common entomopathogenic fungus readily used in similar experiments on immunological behaviors in ants (12, 20, 39). In previous studies, it has been shown that B. bassiana kills ants of diverse species after 4 days (39), and the corpses killed by the fungus can further infect ants (36). We cultivated B. bassiana on petri dishes with Potato Glucose Agar, incubated in the dark at room temperature. To prevent any effect of local adaptations to B. bassiana, we used a Danish strain (KLV 03–90) of fungus which has been previously used with F. fusca (20). Spores of B. bassiana were collected from plates with visibly sporulating fungus by pipetting 10 mL of 1 × PBS on the plate and then carefully rubbing it with a sterile glass rod to collect spores. The solution of PBS containing spores was centrifuged in 3000 rpm for 3 min in 4°C. The supernatant was discarded, and the spores were then suspended in 10 mL of Milli-Q H2O. The spore concentration was determined using a haemacytometer.
To infect the ants, we submerged them in a solution containing 1 × 107 spores/mL of B. bassiana for 5 s. The control ants were all submerged in MilliQ-H2O for 5 s. Dead ants were not removed during the observation period to avoid interrupting the natural chain of infections and keep the pathogen pressure on the colonies high due to fungus killed corpses (40, 41) but were counted the morning after the observation period ended (day 7).
Statistical Analysis
All statistical analysis was done using the R software (version 4.1.2, R Core Team 2020).
The foraging data was analyzed using generalized linear mix models (GLMM) using the glmmTMB function from the glmmTMB package (42). All the models were fitted with a poisson distribution and were tested for deviations in dispersion using the DHARMa package for diagnostics for hierarchical regression models (43). Original nest and time nested within the experimental colony ID were used as random effects in all the models to account for nest-caused differences as well as pseudoreplication. Pairwise comparisons were conducted using the emmeans function from the emmeans package (44) using a Tukey's p-value adjustment when performing multiple comparisons.
The model for analyzing overall foraging activity used the number of ants foraging for food during the observational periods as the response variable. The infection treatment (0/50/100%) and diet (control diet/food choice/ROS diet) were used as fixed factors, as well as the interaction between them.
Foraging within the food choice diet was analyzed with a model using the total number of ants foraging on the different foods during the observational periods as the response variable, with the infection treatment and food type (control food/ROS food) and their interaction as fixed factors.
Whether the infection status of the individuals affects the choice on foraging on the different food types in the food choice diet was analyzed with a model using the total amount of ants foraging on the different foods within the 50% infection treatment as the response variable with the infection status of the individuals (infected/uninfected) and food type and their interaction as fixed factors.
The survival data was analyzed using a cox proportional hazard model from the coxme package (45). The numbers of individual ants who had died when the observation was terminated was used as the response variable, and the infection treatment and diet, as well as their interaction, were used as fixed factors. Original nest was used as a random effect to account for within nest differences. The experimental colony was also used as a random effect to account for pseudoreplication. Pairwise comparison between the groups was done using the emmeans function from the emmeans package (44) with a Tukey's p-value adjustment for multiple group comparisons.
Experimental colony 14 (infection free colony with ROS diet) experienced abnormally high mortality (77.08% compared to the average of 11.61%, SD ± 8.84, min = 2.88%, max = 26.92% of the other colonies with the same treatment combination) and was therefore omitted from the analysis. The queen died during the observational period in experimental colonies 70 (50% infected colony with food choice diet) and 75 (50% infected colony with ROS diet) and were omitted from the analysis due to possible alterations in the natural behavior of the colonies caused by death of the queen. The omission of these colonies did not affect the results in a significant way.
Results
Foraging
Access to the different diets had a significant effect on foraging activity in the colonies (foraging ~ diet, = 20.868, p < 0.001), but the different infection treatments did not (foraging ~ infection treatment, = 3.963, p = 0.138). The interaction between infection treatment and diet was not significantly affecting the overall foraging activity of the colonies (infection treatment × diet interaction on foraging, = 0.386, p = 0.984). Ants were foraging significantly less on a fixed ROS diet compared to both the control diet (t = 3.801, p < 0.001) and the food choice diet (t = 4.157, p < 0.001), but there was no difference in foraging activity when colonies had access to the control and food choice diets (t = −0.282, p = 0.957) (Figure 2).
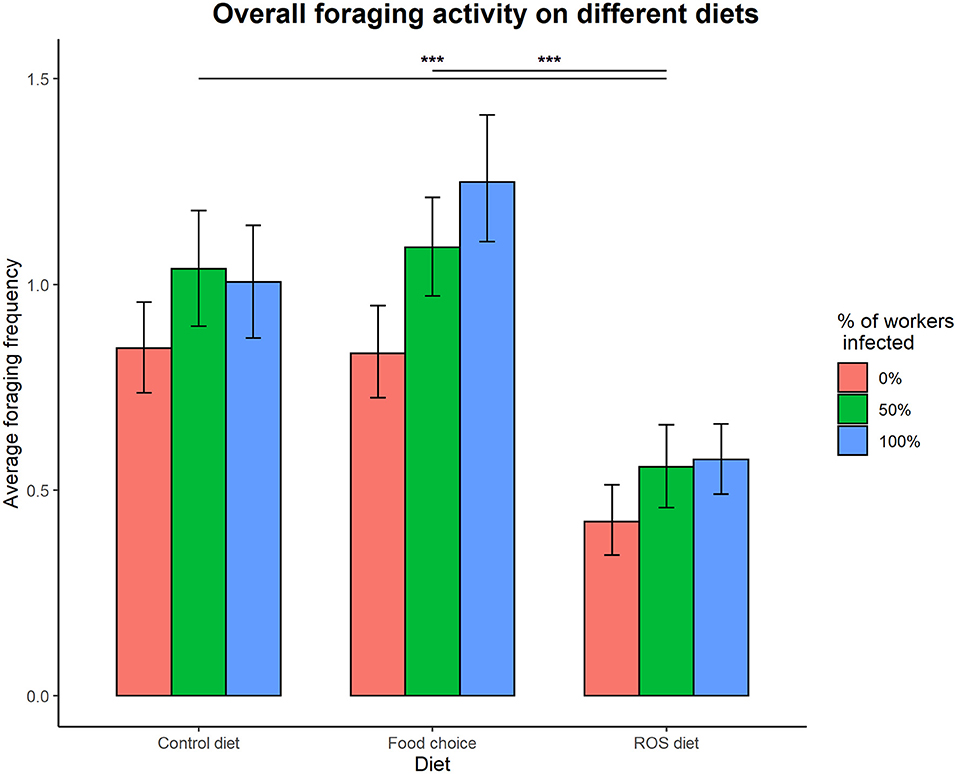
Figure 2. Overall foraging activity on different diets. Access to the different diets affected foraging activity in the colonies ( = 19.189, p < 0.001), as foraging on a ROS diet was significantly lower compared to both the control diet (t = 3.781, p < 0.001) and the food choice diet (t = 4.129, p < 0.001). The difference between foraging on the control diet and the food choice diet was not significant (t = −0.277, p = 0.959). Infection treatment did not affect foraging in a significant way ( = 3.865, p = 0.149). The error bars represent the 95% confidence intervals. *** denotes a significance p < 0.001.
Within the food choice diet, the effect of infection treatment on foraging activity depended on the type of food (control food vs. ROS food) (infection treatment × food type interaction on foraging, = 8.025, p = 0.018). Pairwise comparisons revealed that foraging on control food within the food choice diet was not affected by infection treatment in colonies (0:50%, t = −1.095, p = 0.518; 0%:100, t = −0.995, p = 0.580; 50:100%, t = 0.113, p = 0.993) (Figure 3A). However, foraging activity on ROS food was affected by the disease prevalence when presented with a food choice diet. One hundred percent infected colonies were foraging significantly more on ROS food compared to 0% infected colonies (t = −2.873, p = 0.012), and the differences in foraging between the 0 and 50% or the 50 and 100% colonies were not significant (0:50%, t = −2.873, p = 0.251; 50:100%, t = −1.311, p = 0.389) (Figure 3B). Foraging on control food compared to ROS food was much more common regardless of the infection treatment of the colonies (0%, t = 11.272, p < 0.001; 50%, t = 12.352, p < 0.001; 100%, t = 13.045, p < 0.001).
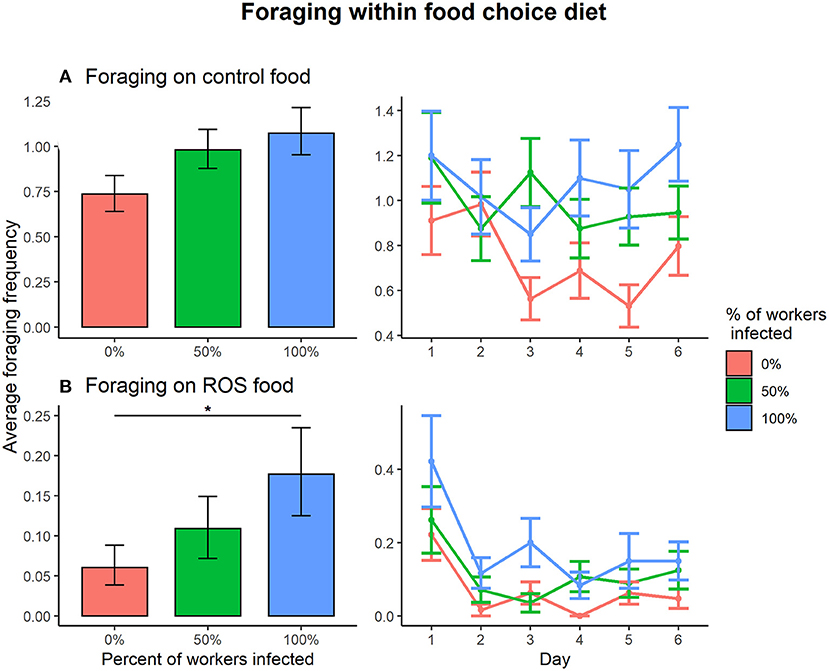
Figure 3. Foraging frequencies between the infection ratios 0% (red), 50% (green), 100% (blue) within the food choice setup. (A) There were no significant differences in foraging activity on control food between any of the infection treatments. (B) When foraging on ROS food, the 100% infected colonies were foraging significantly more compared to the 0% infected colonies (t = −2.873, p = 0.012), but the differences between 0:50% and 50:100% infected colonies were non-significant. The error bars represent the 95% confidence interval. * denotes a significance p < 0.05.
Infection status did not significantly affect the overall foraging activity (foraging ~ infection status, = 1.527, p = 0.217). Foraging activity on the different types of food (control food vs. ROS food) in the 50% colonies with access to a food choice was not dependent on the infection status (uninfected vs. infected) of the individuals (infection status × food type interaction on foraging, = 2.694, p = 0.101) (Figure 4).
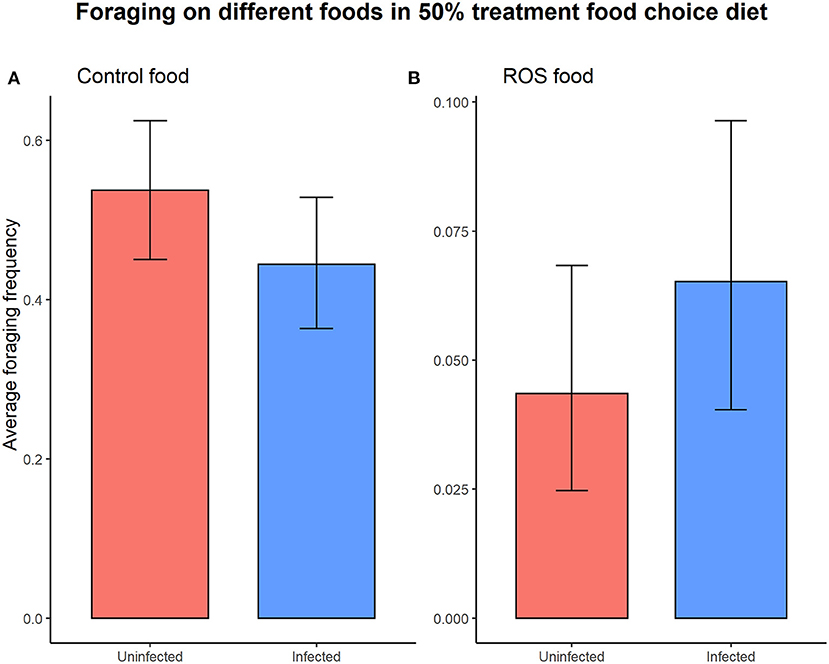
Figure 4. The infection status of the individual did not cause significant differences in foraging activity on either control food (t = 1.685, p = 0.092) (A) or ROS food (t = −1.175, p = 0.240) (B) when presented with a food choice diet. The error bars represent the 95% confidence interval.
Survival Analysis
Both the diet and the infection treatment in the colonies significantly affected the worker mortality in the colonies (diet, = 12.802, p = 0.002; infection treatment, = 9.148, p = 0.010), but the interaction of them did not affect mortality significantly (diet × infection treatment interaction on survival, = 3.006, p = 0.557).
Infecting of all the workers in the colony with B. bassiana significantly affected the mortality compared to infection free colonies when no medicinal food was present (0:100%, z = −2.638, p = 0.023). Infecting half of the workers in the colonies had no significant effect on survival compared to either infection free colonies (0:50%, z = −1.058, p = 0.540) or colonies where all the workers were exposed (50:100%, z = −1.591, p = 0.249) (Figure 5A).
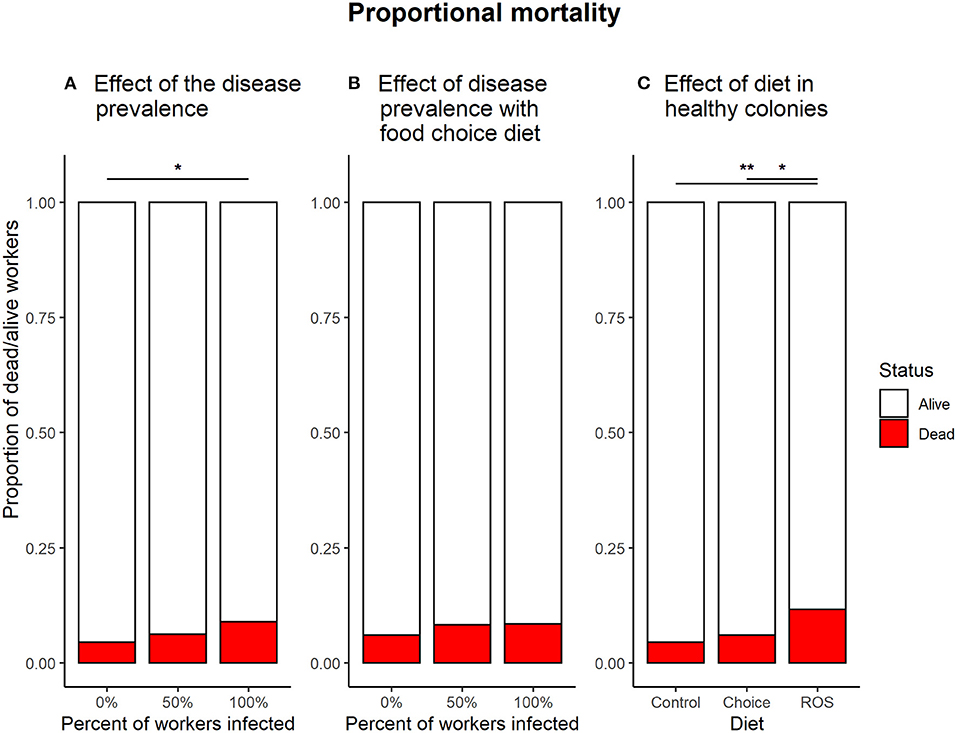
Figure 5. Proportional mortality in the different treatments. (A) Effect of the infection ratio on mortality in colonies fed with the control diet. The dose of pathogen used for the experiment had an overall low effect on mortality, but enough to cause a significant effect on mortality when all of the workers in the colony were infected with B. bassiana (z = −2.638, p = 0.023). (B) The effect of infection ratio in colonies with a food choice diet. When access to both foods, the 100% infected colonies no longer show a significantly higher mortality compared to infection free colonies (z = −1.456, p = 0.312). (C) The effect of the different diet treatments on mortality in infection free colonies. Colonies feeding on a fixed ROS diet had significantly higher mortality compared to colonies feeding on the control diet (z = −3.205, p = 0.004) or the food choice diet (z = −2.403, p = 0.043). * denotes a significance p < 0.05; ** denotes a significance p < 0.01.
If the ants had access to the food choice diet with both control food and medicinal food, the ratio of workers infected with B. bassiana did not affect survival (0:50%, z = −0.889, p = 0.648; 0:100%, z = −1.456, p = 0.312; 50:100%, z = −0.524, p = 0.860) (Figure 5B).
Infection free colonies feeding on a ROS diet have significantly higher mortality compared to colonies who have a control diet (z = 3.205, p = 0.004) or a food choice diet (z = −2.403, p = 0.043). No difference in mortality is observed between infection free colonies with the control diet or having a choice between the two types of food (z = −0.902, p = 0.639) (Figure 5C).
Discussion
Formica fusca ants have previously been shown to self-medicate by incorporating food containing ROS in the form of H2O2 into their diet in response to a highly lethal dose of B. bassiana (20). Here we show that a similar response is also induced with a much less lethal dose, and that the strength of self-medication response is modulated by the ratio of infected ants in the colony, as the colonies were choosing to forage increasingly on ROS food as disease prevalence increased in the colonies, as we predicted.
Adjusting foraging on ROS food according to pathogen prevalence implies plastic and deliberate use of the compound, which is in accordance with the first criterion of self-medication. Our experiment also clearly shows that another criterion is met: ROS food is harmful for healthy individuals. Simone-Finstrom and Spivak (18) have previously confirmed a further criterion, that H2O2 lowers the fitness of B. bassiana. The remaining criterion we cannot clearly prove in our experiment—i.e., the benefit of the dietary change as a response to the infection. We do see however, that when having access to a balanced diet of both medicinal and normal food, the level of infection does not affect the survival of ants in a significant way compared to healthy colonies, so there is some evidence for a benefit to changing the diet.
Two factors may have masked any benefits in survival caused by changes in foraging behavior: the low effect of the pathogen dose on ant mortality and the relatively short period of time observing the colonies. Observing mortality after seven days gives us only a view on the effects of the primary infection, but the threat of disease could still linger in the colonies due to the fungus killed corpses as dead ants were not removed from the colonies (40). Fungal growth on corpses is often visible 2 days after death (40), and ants can differentiate between fungus and freeze killed corpses (41), so it is likely that the self-medication response in the colony would remain upregulated even if the effects of the primary infection would decrease. Therefore, it is reasonable to expect, that the colony would continue to forage on medicinal food which due to its deleterious effects on survival could render short-term benefits low or unclear, but in a longer timeframe benefit the colonies in either worker survival or other measures of fitness. The issue of determining costs and benefits on more than just the short-term survival of individual ants has been raised before (46) and remains an important part in future studies on self-medication behavior.
An interesting result of the experiment was that also infection free colonies with a food choice diet were observed foraging on ROS food, even if this was rarer than in infected colonies. The reason for this behavior is speculative, but it could be possible that the ants are displaying a fixed prophylaxis behavior. de Roode and Lefèvre (47) argued, that if insects would face constant pathogen pressure, evolution would favor prophylaxis to become a fixed behavior. Although this experiment was not enough to provide strong enough evidence for it, Bos et al. (20) reported a similar observation of healthy colonies incorporating ROS food into their diet even if they were free of active infection. As ants are under constant threat of disease due to their behavior and the prevalence of pathogens in their nest and surroundings (3, 5, 48, 49), a fixed prophylaxis would be a feasible strategy for ants to stave off disease, and an interesting study into the extent of the self-medication behavior in ants.
Our results also suggest that obtaining ROS even when only part of a colony is exposed to pathogen infection might not only be an individual response, but instead a response on the colony level.
Foraging on H2O2 supplemented food in the 50% infected colonies was done by both uninfected and infected ants without showing any significant difference due to the health status of the foragers. As the foraging frequency was quite low overall, it is likely that all individual ants in the colonies were not individually foraging on the foods, but instead the foragers of the colony were distributing the food according to the needs of the colony. Buffin et al. (50) have previously shown how food is effectively distributed in colonies of F. fusca in a matter of a few hours. If the foragers are distributing medicinal food to infected nestmates as a form of therapeutics as well as uninfected nestmates as prophylaxis, then there could also be a kin medication aspect to the self-medication behavior of ant colonies, however this would have to be confirmed in a separate experiment.
The response of uninfected individuals in a colony to also forage on ROS food implies that there is simultaneous prophylactic as well as therapeutic self-medication within colonies. Thus, the self-medication response is active on both the individual as well as the colony level and opens up the possibility of active kin medication in colonies suffering from pathogen infection. This sort of kin medication also draws parallels with mass drug administration strategies (MDA) used by humans against diseases such as malaria (51, 52). In MDA, the entire population is treated against a prevalent disease regardless of whether they are actively infected or not, meaning that simultaneous therapeutic and prophylactic medication takes place, and it has been shown to be an efficient strategy to contain and eliminate the disease (51). Therefore, kin medication driven by foragers could also be a strategy also used by ants to combat pathogens as a part of their social immunity, which opens up an interesting avenue of self-medication research in ants as well as other social insects.
In conclusion, this experiment adds to the evidence of the ability of ants to self-medicate against disease. In our experiment, we found that the strength of the self-medication response is plastic and increases when a larger part of the colony is infected with a fungal pathogen. This experiment also takes into consideration the criticism of past experiments, where highly lethal doses of pathogens have been used, to provide a more natural level of exposure of pathogens. The fact that ants alter their foraging behavior in response to not only to prevalence of pathogens in their colonies, but also the quality of the medicinal food available and possibly the severity of the disease (20), means that ants have a highly complex understanding of their surroundings and changes in it caused by pathogens leads to intricate decisions on a colony level to respond to them.
Data Availability Statement
The data is now available on Dryad: DOI https://doi.org/10.5061/dryad.0zpc86707.
Author Contributions
JR developed the idea for the experiment, analyzed the data, and provided the first draft of the manuscript. JR, HH, and DF designed the experiment and carried it out. All authors worked on the revisions of the manuscript and approved the final version.
Conflict of Interest
The authors declare that the research was conducted in the absence of any commercial or financial relationships that could be construed as a potential conflict of interest.
Publisher's Note
All claims expressed in this article are solely those of the authors and do not necessarily represent those of their affiliated organizations, or those of the publisher, the editors and the reviewers. Any product that may be evaluated in this article, or claim that may be made by its manufacturer, is not guaranteed or endorsed by the publisher.
Acknowledgments
We thank Konsta Kesti and Laura Kares for their help with the field work and experiments. We would also like to thank the two reviewers for their valuable comments on the manuscript.
References
1. Hölldobler B, Wilson EO. The Superorganism: The Beauty, Elegance, and Strangeness of Insect Societies. London: WW Norton and Company (2009).
4. McCallum H, Barlow N, Hone J. How should pathogen transmission be modelled? Trends Ecol Evol. (2001) 16:295–300. doi: 10.1016/S0169-5347(01)02144-9
5. Hughes WOH, Thomsen L, Eilenberg J, Boomsma JJ. Diversity of entomopathogenic fungi near leaf-cutting ant nests in a neotropical forest, with particular reference to Metarhizium anisopliae var. anisopliae. J Invertebr Pathol. (2004) 85:46–53. doi: 10.1016/j.jip.2003.12.005
6. Veal DA, Trimble JE, Beattie AJ. Antimicrobial properties of secretions from the metapleural glands of Myrmecia gulosa (the Australian bull ant). J Appl Bacteriol. (1992) 72:188–94. doi: 10.1111/j.1365-2672.1992.tb01822.x
7. Tragust S, Mitteregger B, Barone V, Konrad M, Ugelvig LV, Cremer S. Ants disinfect fungus-exposed brood by oral uptake and spread of their poison. Curr Biol. (2013) 23:76–82. doi: 10.1016/j.cub.2012.11.034
8. Brütsch T, Jaffuel G, Vallat A, Turlings TCJ, Chapuisat M. Wood ants produce a potent antimicrobial agent by applying formic acid on tree-collected resin. Ecol Evol. (2017) 7:2249–54. doi: 10.1002/ece3.2834
9. Reber A, Purcell J, Buechel SD, Buri P, Chapuisat M. The expression and impact of antifungal grooming in ants. J Evol Biol. (2011) 24:954–64. doi: 10.1111/j.1420-9101.2011.02230.x
10. Maák I, Tóth E, Lenda M, Lorinczi G, Kiss A, Juhász O, et al. Behaviours indicating cannibalistic necrophagy in ants are modulated by the perception of pathogen infection level. Sci Rep. (2020) 10:1–13. doi: 10.1038/s41598-020-74870-8
11. Cremer S, Armitage SAO, Schmid-Hempel P. Social immunity. Curr Biol. (2007) 17:693–702. doi: 10.1016/j.cub.2007.06.008
12. Cremer S, Pull CD, Fürst MA. Social immunity: emergence and evolution of colony-level disease protection. Annu Rev Entomol. (2018) 63:105–23. doi: 10.1146/annurev-ento-020117-043110
13. Abbott J. Self-medication in insects: current evidence and future perspectives. Ecol Entomol. (2014) 39:273–80. doi: 10.1111/een.12110
14. Huffman MA. Current evidence for self-medication in primates: a multidisciplinary perspective. Am J Phys Anthropol. (1997) 140:171–200.
15. Singer MS, Mace KC, Bernays EA. Self-medication as adaptive plasticity: increased ingestion of plant toxins by parasitized caterpillars. PLoS ONE. (2009) 4:e4796. doi: 10.1371/journal.pone.0004796
16. de Roode JC, Lefevre T, Hunter MD. Self-medication in animals. Science. (2013) 340:150–1. doi: 10.1126/science.1235824
17. Huffman MA. Primate self-medication, passive prevention and active treatment - a brief review. Int J Multidiscip Studies. (2017) 3:1–10. doi: 10.4038/ijms.v3i2.1
18. Simone-Finstrom MD, Spivak M. Increased resin collection after parasite challenge: A case of self-medication in honey bees? PLoS ONE. (2012) 7:e34601. doi: 10.1371/journal.pone.0034601
19. Baracchi D, Brown MJF, Chittka L. Behavioural evidence for self-medication in bumblebees? F1000Res. (2015) 4:1–15. doi: 10.12688/f1000research.6262.2
20. Bos N, Sundström L, Fuchs S, Freitak D. Ants medicate to fight disease. Evolution. (2015) 69:2979–84. doi: 10.1111/evo.12752
21. Lee KP, Cory JS, Wilson K, Raubenheimer D, Simpson SJ. Flexible diet choice offsets protein costs of pathogen resistance in a caterpillar. Proc R Soc B Biol Sci. (2006) 273:823–9. doi: 10.1098/rspb.2005.3385
22. Lefèvre T, Oliver L, Hunter MD, de Roode JC. Evidence for trans-generational medication in nature. Ecol Lett. (2010) 13:1485–93. doi: 10.1111/j.1461-0248.2010.01537.x
23. Singer MS, Mason PA, Smilanich AM. Ecological immunology mediated by diet in herbivorous insects. Integr Comp Biol. (2014) 54:913–21. doi: 10.1093/icb/icu089
24. Poyet M, Eslin P, Chabrerie O, Prud'Homme SM, Desouhant E, Gibert P. The invasive pest Drosophila suzukii uses trans-generational medication to resist parasitoid attack. Sci Rep. (2017) 7:1–8. doi: 10.1038/srep43696
25. Ha EM, Oh CT, Ryu JH, Bae YS, Kang SW, Jang In-Hwan, et al. An antioxidant system required for host protection against gut infection in Drosophila. Dev Cell. (2005) 8:125–32. doi: 10.1016/j.devcel.2004.11.007
26. Molina-Cruz A, DeJong RJ, Charles B, Gupta L, Kumar S, Jaramillo-Gutierrez G, et al. Reactive oxygen species modulate anopheles gambiae immunity against bacteria and Plasmodium. J Biol Chem. (2008) 283:3217–23. doi: 10.1074/jbc.M705873200
27. Mikonranta L, Mappes J, Kaukoniitty M, Freitak D. Insect immunity: oral exposure to a bacterial pathogen elicits free radical response and protects from a recurring infection. Front Zool. (2014) 11:1–7. doi: 10.1186/1742-9994-11-23
28. Lewis T, Pollard GV, Dibley GC. Rhythmic Foraging in the Leaf-Cutting Ant Atta cephalotes (L) (Formicidae: Attini). J Anim Ecol. (1974) 43:129–41. doi: 10.2307/3162
29. Bruin MK-D, Rost LCM, Draisma FGAM. Estimates of the number of foraging ants with the lincoln-index method in relation to the colony size of formica polyctena. J Anim Ecol. (1977) 46:457–70. doi: 10.2307/3823
30. Porter SD, Jorgensen CD. Foragers of the harvester ant, pogonomyrmex owyheei: a disposable caste? Behav Ecol Sociobiol. (1981) 9:247–56. doi: 10.1007/BF00299879
31. Dussutour A, Simpson SJ. Communal nutrition in ants. Curr Biol. (2009) 19:740–4. doi: 10.1016/j.cub.2009.03.015
32. Howard DF, Tschinkel WR. The flow of food in colonies of the fire ant, solenopsis invicta: a multifactorial study. Physiol Entomol. (1981) 6:297–306. doi: 10.1111/j.1365-3032.1981.tb00274.x
33. Loreto RG, Hughes DP. Disease dynamics in ants. A critical review of the ecological relevance of using generalist fungi to study infections in insect societies. Adv Genet. (2016) 94:287–306. doi: 10.1016/bs.adgen.2015.12.005
34. Okuno M, Tsuji K, Sato H, Fujisaki K. Plasticity of grooming behavior against entomopathogenic fungus Metarhizium anisopliae in the ant Lasius japonicus. J Ethol. (2012) 30:23–7. doi: 10.1007/s10164-011-0285-x
35. Chernenko A, Helanterä H, Sundström L. Colony kin structure and queen recruitment in the ant Formica fusca (Hymenoptera: Formicidae). Myrmecological News. (2012) 16:93–100.
36. Hannonen M, Helanterä H, Sundström L. Habitat age, breeding system and kinship in the ant Formica fusca. Mol Ecol. (2004) 13:1579–88. doi: 10.1111/j.1365-294X.2004.02136.x
37. Ning D, Yang F, Xiao Q, Ran H, Xu Y. A simple and efficient method for preventing ant escape (Hymenoptera: Formicidae). Myrmecological News. (2019) 29:57–65. doi: 10.25849/myrmecol.news_029:057
38. Bhatkar A, Whitcomb WH. Artificial diet for rearing various species of ants. Fla Entomol. (1970) 53:229–32. doi: 10.2307/3493193
39. Bos N, Kankaanpää-Kukkonen V, Freitak D, Stucki D, Sundström L. Comparison of twelve ant species and their susceptibility to fungal infection. Insects. (2019) 10:271. doi: 10.3390/insects10090271
40. Loreto RG, Hughes DP. Disease in the society: infectious cadavers result in collapse of ant sub-colonies. PLoS ONE. (2016) 11:e0160820. doi: 10.1371/journal.pone.0160820
41. Qiu HL, Lu LH, Shi QX, Tu CC, Lin T, He YR. Differential necrophoric behaviour of the ant Solenopsis invicta towards fungal-infected corpses of workers and pupae. Bull Entomol Res. (2015) 105:607–14. doi: 10.1017/S0007485315000528
42. Brooks ME, Kristensen K, van Benthem KJ, Magnusson A, Berg CW, Nielsen A, et al. glmmTMB balances speed and flexibility among packages for zero-inflated generalized linear mixed modeling. R Journal. (2017) 9:378–400. doi: 10.32614/RJ-2017-066
43. Hartig F. DHARMa: Residual Diagnostics for Hierarchical (Multi-level/mixed) Regression Models. (2021). Available online at: https://CRAN.R-project.org/package=DHARMa (accessed March 31, 2022).
44. Lenth R. Emmeans: Estimated Marginal Means aka Least - Square Means. (2021). Available online at: https://CRAN.R-project.org/package=emmeans (accessed March 31, 2022).
45. Therneau TM. Coxme: Mixed Effects Cox Models. (2020). Available online at: https://CRAN.R-project.org/package=coxme (accessed March 31, 2022).
46. de Roode JC, Hunter MD. Self-medication in insects: when altered behaviors of infected insects are a defense instead of a parasite manipulation. Curr Opin Insect Sci. (2019) 33:1–6. doi: 10.1016/j.cois.2018.12.001
47. de Roode JC, Lefèvre T. Behavioral immunity in insects. Insects. (2012) 3:789–820. doi: 10.3390/insects3030789
48. Reber A, Chapuisat M. Diversity, prevalence and virulence of fungal entomopathogens in colonies of the ant Formica selysi. Insectes Soc. (2012) 59:231–9. doi: 10.1007/s00040-011-0209-3
49. Brahma A, Leon RG, Hernandez GL, Wurm Y. Larger, more connected societies of ants have a higher prevalence of viruses. Mol Ecol. (2022) 31:859–65. doi: 10.1111/mec.16284
50. Buffin A, Denis D, van Simaeys G, Goldman S, Deneubourg JL. Feeding and stocking up: Radio-labelled food reveals exchange patterns in ants. PLoS ONE. (2009) 4:e5919. doi: 10.1371/journal.pone.0005919
51. Hotez PJ. Mass drug administration and integrated control for the world's high-prevalence neglected tropical diseases. Clin Pharmacol Ther. (2009) 85:659–64. doi: 10.1038/clpt.2009.16
Keywords: self-medication, Formica fusca, ants, Beauveria bassiana, insect immunity, behavior
Citation: Rissanen J, Helanterä H and Freitak D (2022) Pathogen Prevalence Modulates Medication Behavior in Ant Formica fusca. Front. Insect Sci. 2:870971. doi: 10.3389/finsc.2022.870971
Received: 07 February 2022; Accepted: 15 March 2022;
Published: 27 April 2022.
Edited by:
Elke Genersch, Institute for Bee Research Hohen Neuendorf (LIB), GermanyReviewed by:
Michael A. Huffman, Kyoto University, JapanAnna Somogyi, Hungarian Natural History Museum, Hungary
Copyright © 2022 Rissanen, Helanterä and Freitak. This is an open-access article distributed under the terms of the Creative Commons Attribution License (CC BY). The use, distribution or reproduction in other forums is permitted, provided the original author(s) and the copyright owner(s) are credited and that the original publication in this journal is cited, in accordance with accepted academic practice. No use, distribution or reproduction is permitted which does not comply with these terms.
*Correspondence: Jason Rissanen, SmFzb24ucmlzc2FuZW5AdW5pLWdyYXouYXQ=