Heme promotes sexual conversion of Plasmodium falciparum in human erythrocytes
- 1Center for Translational and International Hematology, Heart, Lung, Blood and Vascular Medicine Institute, Department of Medicine, University of Pittsburgh, Pittsburgh, PA, United States
- 2Department of Infectious Diseases and Microbiology, University of Pittsburgh School of Public Health, Pittsburgh, PA, United States
- 3Heart, Lung, Blood and Vascular Medicine Institute, Department of Medicine, University of Pittsburgh, Pittsburgh, PA, United States
- 4Division of Pulmonary, Allergy, and Critical Care Medicine, University of Pittsburgh, Pittsburgh, PA, United States
- 5Department of Immunology, Noguchi Memorial Institute for Medical Research, University of Ghana, Accra, Ghana
- 6School of Biomedical and Allied Health Sciences, University of Ghana, Accra, Ghana
- 7West African Genetic Medicine Centre, University of Ghana, Accra, Ghana
Between 2018 and 2020, the global population experienced a 55% increase in deaths attributed to the most prominent malaria-causing pathogen, Plasmodium falciparum. Transmission of P. falciparum from a human host to the mosquito vector is completely reliant on the formation of sexual stage gametocytes, which arise from asexually replicating parasites during the intraerythrocytic stage of infection. Defining specific factors that promote the formation of transmissible sexual stages from the disease-causing asexual stages is important for developing new malaria control methods. Malaria infection rates are known to be affected by genetic variation of the hemoglobin (Hb) protein, and epidemiological studies have shown that Hb variants may positively influence the production of sexual stage parasites. However, the mechanisms involved are poorly defined. Here, we show P. falciparum sexual conversion rates (SCR) are significantly higher in erythrocytes expressing Hb S compared to those that express Hb A. We then found parasitic enzyme-mediated digestion of Hb S to occur more rapidly than Hb A, suggesting an increased release of heme groups carried by each Hb subunit. Upon manipulating both intracellular and extracellular heme concentrations, we found significant increases in SCR, ultimately indicating heme acts as an inducer of sexual conversion (SC). As levels of both intracellular and extracellular heme are increased in individuals with Hb variants, we propose heme to be a contributing factor for increased sexual stage conversion observed in these populations. These findings support further investigation into how heme concentrations may be directly manipulated to prevent commitment to sexual-stage formation and ultimately disease transmission.
1 Introduction
Malaria inflicts a significant burden on at-risk populations at the clinical, social, and economic level. As over 40% of the global population is considered at-risk, malaria is amongst the leading concerns of global health today. Though there are five species of Plasmodium that cause malaria in humans, infection with P. falciparum is the most severe and is the primary cause of disease and mortality. Over the past two years, deaths attributed to P. falciparum have increased alarmingly, largely due to obstacles following the COVID-19 pandemic (Hogan et al., 2020; Weiss et al., 2021). To circumvent this surge and regain control in the effort to reduce cases and deaths, new methods to control parasite transmission are urgently needed.
Symptoms of falciparum malaria result from the intraerythrocytic phase of infection. During this phase, multiple rounds of asexual replication take place, each of which result in hemolysis and the release of daughter merozoites capable of invading new erythrocytes within circulation. In order for host to vector transmission to occur, sexually competent gametocytes must be produced. Gametocytes arise from intraerythrocytic asexually replicating parasites in a process termed sexual conversion (SC) and are the only stage of Plasmodium spp. that are viable for transmission to the mosquito vector.
The rate of parasites becoming sexually committed versus those that continue to replicate asexually has been shown to fluctuate, exhibiting seasonality, varying between parasite strains, and in response to certain environmental stimuli (Trager and Gill, 1992; Trager et al., 1999; Van Der Kolk et al., 2003; Peatey et al., 2009; Chaubey et al., 2014; Srivastava et al., 2015; Brancucci et al., 2017; Josling et al., 2018; Oduma et al., 2021). This ability to control reproductive investment is an important survival adaptation among Plasmodium spp., ultimately ensuring a balance between intra-host survival and inter-host transmission. Specific strategies of reproductive investment can be applied to Plasmodium spp. to provide a framework to explain these alterations in sexual conversion rate (SCR) (Pollitt et al., 2011; Schneider et al., 2018). For example, during conditions of extreme stress, P. falciparum has been shown to invest more highly in SC as opposed to asexual replication (Carter et al., 2013; Chaubey et al., 2014; Josling and Llinas, 2015; Usui and Williamson, 2021). This aligns with the terminal investment hypothesis, wherein organisms sacrifice intra-host survival for inter-host transmission (Carter and Miller, 1979; Pollitt et al., 2011; Carter et al., 2013; Schneider et al., 2018). During environments of manageable stress, P. falciparum have been shown to exhibit reproductive restraint, wherein diverting all resources into salvaging intra-host survival “pays-off” by increasing the likelihood of greater inter-host transmission in the future (Pollitt et al., 2011; Carter et al., 2013; Josling et al., 2018; Schneider et al., 2018).
Targeting gametocytes may be an effective strategy for developing host-directed methods to reduce parasite transmission. As such, the identification of factors that influence SC in P. falciparum has been an area of increased study. One observation that has arisen from this line of investigation is increased gametocyte carriage among individuals expressing variants of Hb compared to those with Hb A (Gouagna et al., 2010; Bougouma et al., 2012; Goncalves et al., 2017). The Hb protein contained within erythrocytes is an essential component during intraerythrocytic infection, as throughout the cycle most will be digested to sustain life within the cell (Thom et al., 2013). Genetic variants of the Hb protein are the most common monogenic disorders, affecting approximately 7% of the global human population (Weatherall, 2008). Though over 1000 different variants have currently been identified, the most common in P. falciparum endemic areas is the sickle Hb (Hb S). While Hb S trait (Hb AS) is known to provide a survival advantage against malaria, intraerythrocytic infection and transmission among these individuals still occurs (Allison, 1954). This selective advantage has led to the high prevalence of Hb S in many malaria-endemic regions. In fact, individuals heterozygous for Hb S account for an estimated 10-30% of the population in certain malaria-endemic regions and exceed 300 million globally (Heller et al., 1979; Piel et al., 2010; Grosse et al., 2011). While the Hb AS genotype has a balanced frequency due to the protective effects against malaria, the homozygous sickle cell disease has a lower frequency due to the associated health consequences of SCD, affecting an estimated 3% of births (Grosse et al., 2011).
The presence of Hb S in different populations has profound implications for malaria transmission dynamics. Considering the importance of Hb to the intraerythrocytic cycle, and the potential impact on malaria transmission, we sought to experimentally determine whether variants of the Hb protein influence the rate of SC in P. falciparum (Goldberg et al., 1990; Thom et al., 2013). Through the side-by-side culture of P. falciparum parasites in Hb A- expressing (Hb A-Ery), Hb S-expressing (Hb S-Ery), and Hb AS-expressing (Hb AS-Ery) erythrocytes, we compared the development of asexual and sexual stage parasites, to find Hb S-Ery cultures produce proportionally more sexual stages than Hb A-Ery cultures. To characterize factors which may be influencing this change in reproductive investment, we first focused on the direct impact of the Hb protein. After finding parasite-mediated digestion of Hb S is more efficient than Hb A, we implicate free heme, a powerful oxidant released during Hb digestion, as a potential factor for SC. We further reveal direct hemin exposure to promote SC. As heme is toxic to parasites, an increased or imbalanced concentration of the toxin may cause a strain on intraerythrocytic survival. Therefore, shifting reproductive effort towards the production of more transmissible sexual stages aligns with current theories of reproductive investment.
2 Materials and methods
2.1 Parasite culture
P. falciparum strain NF54 were obtained from MR4 and cultured in O+ erythrocytes. Cultures were maintained in modified RPMI containing 25 mm HEPES, L-glutamine, and 50 mg/L hypoxanthine (KD Medical), and supplemented with gentamicin, sodium bicarbonate, and 10% fresh human serum (complete parasite media (CPM)) and a gas mix containing 5% CO2, 5% O2, and 90% N2. Human blood for cultures was purchased from authorized collection facilities (Zen-Bio and Innovative Research) or collected from steady state SCD patients in accordance with the guidelines set by the Institutional Review Board at the University of Pittsburgh and the Declaration of Helsinki. All blood was washed in CPM upon receival, and Hb type was determined by Hb electrophoresis. All synchronization was performed by sorbitol lysis, wherein cultures were resuspended in 5% sorbitol, incubated for 10 min at 37°C, and washed with CPM. Synchronizations were performed 18-20 hrs apart to achieve 6-4 hr development windows respectively.
For all experiments involving hemin exposure, a hemin stock solution was prepared fresh on the day of treatment and as described (Vinchi et al., 2008; Larsen et al., 2010; Ghosh et al., 2013). Hemin was first dissolved in NaOH and the pH was then adjusted to 7.52 using HCl. The solution was then filtered to ensure no precipitate.
2.2 Sexual conversion assays
For comparison of SC between Hb A-Ery and Hb S-Ery, cultures were synchronized to a 6 hr development window prior to magnetic separation of mature schizonts using MACS LS columns (Miltenyi). Schizonts were eluted with CPM and re-applied to a fresh column. Schizont isolation was confirmed with a blood smear prior to inoculating Hb A-, Hb AS-, and Hb S-Ery cultures at a 4% HCT, and to a final parasitemia of 0.3% in t75 flasks. Media was changed daily. Cultures were periodically sampled for flow cytometry and blood smear analysis. On days four and seven of culture, blood smears were analyzed for the presence of stage II and III gametocytes respectively. SCR was determined by dividing total stage II/III gametocytemia on day four/seven by total parasitemia on day one.
For SC assays involving CQ and hemin exposure, cultures were synchronized to a 4 hr development window. Early rings (0-4 hr post-invasion) were plated at 1% parasitemia and 2% HCT in 12- well culture plates prior to vehicle, chloroquine diphosphate (CQ) (Sigma), or hemin (Frontier) treatment. SC was determined on day seven, after the addition of NAG at 48 hrs, and the removal of all treatment conditions.
2.3 Flow cytometry
All parasitemia and gametocytemia measurements for experiments involving CQ and hemin exposure were conducted through flow cytometry on a BD LSR Fortessa. Parasitemia and gametocytemia measurements by flow cytometry were used to determine infected cells. No stage distinction was made using this method (Supplementary Figure 1). For each sample, 10 μl of whole culture was resuspended in 20 μM Hoechst (Thermo Scientific). Samples were incubated for 30 min at 37°C and immediately analyzed. For each sample, 100,000 events were collected and gated for FSC and SSC, followed by doublets exclusion. Parasitemia was determined by Hoechst-positivity. Excitation of cells for Hoechst was performed with a UV laser (355 nm) and band pass filter 450/50 nm. A sample of uninfected erythrocytes was used as a negative control.
2.4 qRT-PCR
For RNA analysis, cultures were pelleted and resuspended in Qiazol (Qiagen), before frozen at -80°C. Upon thaw, chloroform was added, followed by centrifugation at 9,800 rpm for 15 min. The aqueous portion of the samples was then mixed with 70% ethanol. RNA was isolated with Qiagen RNeasy Mini columns with on-column DNase digestion (Qiagen). Samples were reverse transcribed (Applied Biosystems), diluted and analyzed for gene expression on Applied Biosystems StepOnePlus Real-Time PCR System using FAST Sybr Green Master Mix (Applied Biosystems). Primers for analysis are listed in Supplementary Table 1. All data is calculated using the relative quantity method (2-ΔΔCt) method and presented in fold-change relative to the reference gene seryl-tRNA synthetase.
2.5 Production of recombinant falcipain-2
Recombinant Falcipain-2 (PF3D7_1115700), was produced as described (Shenai et al., 2000; Sijwali and Rosenthal, 2004; Chugh et al., 2013). E. coli containing the plasmid construct for falcipain-2 (FP2) was obtained by the generous donation of Philip Rosenthal. Prior to producing the protein on a large scale, the construct was confirmed. After the construct was confirmed, bacteria were grown to mid-log phase and induced with IPTG for 3 hrs at 37°C. Cells were then harvested and washed in cold Tris-NaCl (20 mM, 150 mM). Samples were sonicated and centrifuged for 30 min at 17,000 rpm. Remaining pellets were solubilized in urea solution (8 M urea, 10 mM Tris, 200 mM Imidazole, pH 8.0). The insoluble material was then separated by centrifugation at 17,000 rpm for 30 min. Protein was purified from the supernatant using nickel-nitrilotriacetic acid (Ni-NTA+) resin (Qiagen). Bound protein was eluted (8 M urea, 10 mM Tris, 500 mM Imidazole) and quantified by nanodrop.
Protein refolding was performed overnight in folding buffer (100 mM Tris-HCI, 30% glycerol, 250 mM arginine, 1 mM EDTA, 1 mM GSH, 1 mM GSSG, pH 9.2) at 4°C. Refolded protein was then concentrated using 10 K centrifuge units (Thermo Scientific). Activity was confirmed with a fluorometric assay wherein the release of 7-amino-4-methyl coumarin (AMC) was monitored (excitation, 355 nm; emission, 460 nm) over the course of 30 min at 37°C in assay buffer (1 M DTT, 100 mM NaOAc, pH 5.5). After confirming activity, recombinant protein was filtered, stabilized, and stored at -80°C until future use.
2.6 Hemoglobin isolation
Hemoglobin was isolated from Townes mice (The Jackson Laboratory), which express exclusively human Hb. Procedures were reviewed and approved by the University of Pittsburgh IACUC. For Hb A, Hb S, and Hb AS samples, blood was collected from adult mice by cardiac puncture. The sample containing Hb A, Hb S, and Hb F was obtained from a pooled blood sample collected from two-day old pups. Townes pups still express high levels of Hb F, as well as Hb A and Hb S. All blood was thoroughly washed with PBS, followed by lysis in water. Lysed blood was then centrifuged at 10,000 rpm for 30 min prior to filtering and concentrating (Millipore). Hemoglobin concentration was measured with QuantiChrom Hemoglobin Assay Kit (BioAssay Systems).
2.7 Hemoglobin hydrolysis assay
To assess Hb degradation, 50 μM of FP2 and 0.12, 0.24, 0.36, and 0.5 μg/μl Hb, buffered in 100 mM NaOAc, and 5 mM GSH, pH 5.5 in a 250 μl volume, was placed in a 96-well plate and incubated for 24 hrs at 37°C. Spectrophotometric measurements were taken at 410 nM at 0 and 24 hrs of incubation, intending to determine total Hb independent of heme binding status. Samples were compared between Hb types, as well as to a no-enzyme control, wells which contained the Hb variant in assay buffer without the presence of FP2. At 24 hrs, 50 μl of each sample was removed and used for total Hb quantification by QuantiChrom Hemoglobin Assay Kit (BioAssay Systems).
2.8 Hemozoin quantification
For each treatment group, 2 mL of culture was collected, lysed, and washed in a 1% final concentration of Triton X-100. Pellets were centrifuged at 13,000 rpm for 45 min at 4°C and then washed three times in water. Each hemozoin pellet was then solubilized in a solution of NaOH, pyridine, and water (1:2:8 v/v). A 75 μl aliquot of each sample was then plated in duplicate. For determination of oxidized and reduced heme, 10 μl 2.5 mM potassium ferricyanide and sodium hydrosulfite were added respectively. Absorbance was measured at 560 nm with hemozoin calculated according to a hemin standard curve.
2.9 Chromatin immunoprecipitation with qPCR
ChIP-qPCR of H3K9me3 and H3K9Ac marks was performed as described (Hoeijmakers and Bartfai, 2018). Cultures were crosslinked with formaldehyde, prior to being washed, pelleted, and lysed in saponin (Sigma). Parasite isolates were then gently homogenized in cell lysis buffer (10 mM Tris-HCI pH 8.0, 3 mM MgCl2, 0.2% Nonidet P-40, protease inhibitor cocktail (Sigma)), and separated by a 0.25 M sucrose gradient. Nuclei were digested (50 mM Tris–HCl pH 7.4, 4 mM MgCl2, 1 mM CaCl2, 0.075% Nonidet P- 40, 1 mM DTT, protease inhibitor cocktail) by micrococcal nuclease and exonuclease (NEB) for 10 min and quenched (2% Triton X-100, 0.6% SDS, 300 mM NaCl, 6 mM EDTA), prior to a brief sonication (25 mM Tris pH 7.4, 1% Triton X-100, 0.3% SDS, 150 mM NaCl, 3 mM EDTA, 2 mM MgCl2, 0.5 mM CaCl2, protease inhibitor cocktail). After ensuring digestion efficiency by gel electrophoresis, 500 ng DNA was incubated with 2.5 μg Rabbit IgG (12-370, Milipore-Sigma), 2.5 μg anti-H3 (ab1791, Abcam), 5 μg anti-H3K9me3 (07-442, Milipore-Sigma), and 5 μg anti-H3K9Ac (06-942, Sigma-Aldrich). Antibodies were incubated with each sample for a minimum of 12 hrs at 4°C while under constant rotation. Protein A and Protein G Dynabeads (ThermoFisher) were added to each reaction and incubated for an additional 2 hrs. Samples were then washed as follows: two washes with 20 mM Tris–HCl pH 8.0, 2 mM EDTA, 1% Triton X-100, 0.1% SDS, 150 mM NaCl, two washes with 20 mM Tris pH 8.0, 2 mM EDTA, 1% Triton X-100, 0.1% SDS, 500 mM NaCl, and two washes with 10 mM Tris-HCl pH 8.0, 1 mM EDTA, prior to elution (1% SDS, 0.1 M NaHCO3). Samples were reverse crosslinked overnight at 45°C, followed by DNA isolation with Qiaquick PCR purification kit (Qiagen). Primers for qPCR analysis are listed in Supplementary Table 1. The amount of target DNA recovered after immunoprecipitation was directly compared to dilution of input DNA and defined as percentage of DNA present in the input sample.
2.10 Image analysis
Blood smears were prepared using 5 μl whole culture and stained for 10 min in 10% Giemsa (Sigma). All images were taken on an Olympus Provis microscope at 40x-100x magnification. All images were analyzed using ImageJ Fiji software and white balance was adjusted as needed.
2.11 Statistical analysis
GraphPad Prism 9 software was used for all statistical analysis. For comparison of significance between three or more means, a one-way ANOVA was used. For experiments with repeated measures (i.e., measurements across time), in which certain values were missing for random reasons, a mixed-effects analysis was completed. For comparison of multiple group means between two factors in which measures were not repeated, data were analyzed by an ordinary two-way ANOVA. For comparison of multiple group means between two factors in which measures were repeated, data were analyzed by repeated measured two-way ANOVA. For all data, statistical significance was based on a p- value of less than 0.05. Correlation was determined by Pearson correlation analysis.
3 Results
3.1 Hb S erythrocytes (Hb S-Ery) promote sexual conversion in P. falciparum
To investigate SC among Hb A-, Hb AS-, and Hb S- Ery cultures, we compared asexual and sexual stage parasite formation over the course of seven days (Figure 1A). Each culture was inoculated with magnetically isolated schizonts that had been synchronized to a six-hour development window. Total parasitemia was measured by microscopy and validated with flow cytometry (Figures 1B–D). Though parasitemia within Hb AS- and Hb S-Ery cultures was overall lower than the Hb A-Ery culture over the first replication cycle, notably, the cultures did sustain, and consistently maintained between a 0.5-2% parasitemia over the seven-day period for each donor sample analyzed (Figures 1B-D). Additionally, blood smears show normal development in all cultures through day two of culture (Figure 1E). Stage II and stage III gametocytemia was determined on day four and seven, respectively, and though total parasitemia was significantly different on both days, a non-significant difference in gametocytemia was observed between all groups (Figures 1C, D). From the stage II gametocytemia measurement on day four, we found a significantly increased SCR among Hb S- Ery cultures compared to Hb A-Ery (Figure 1C). This difference was sustained through day seven (Figure 1D).
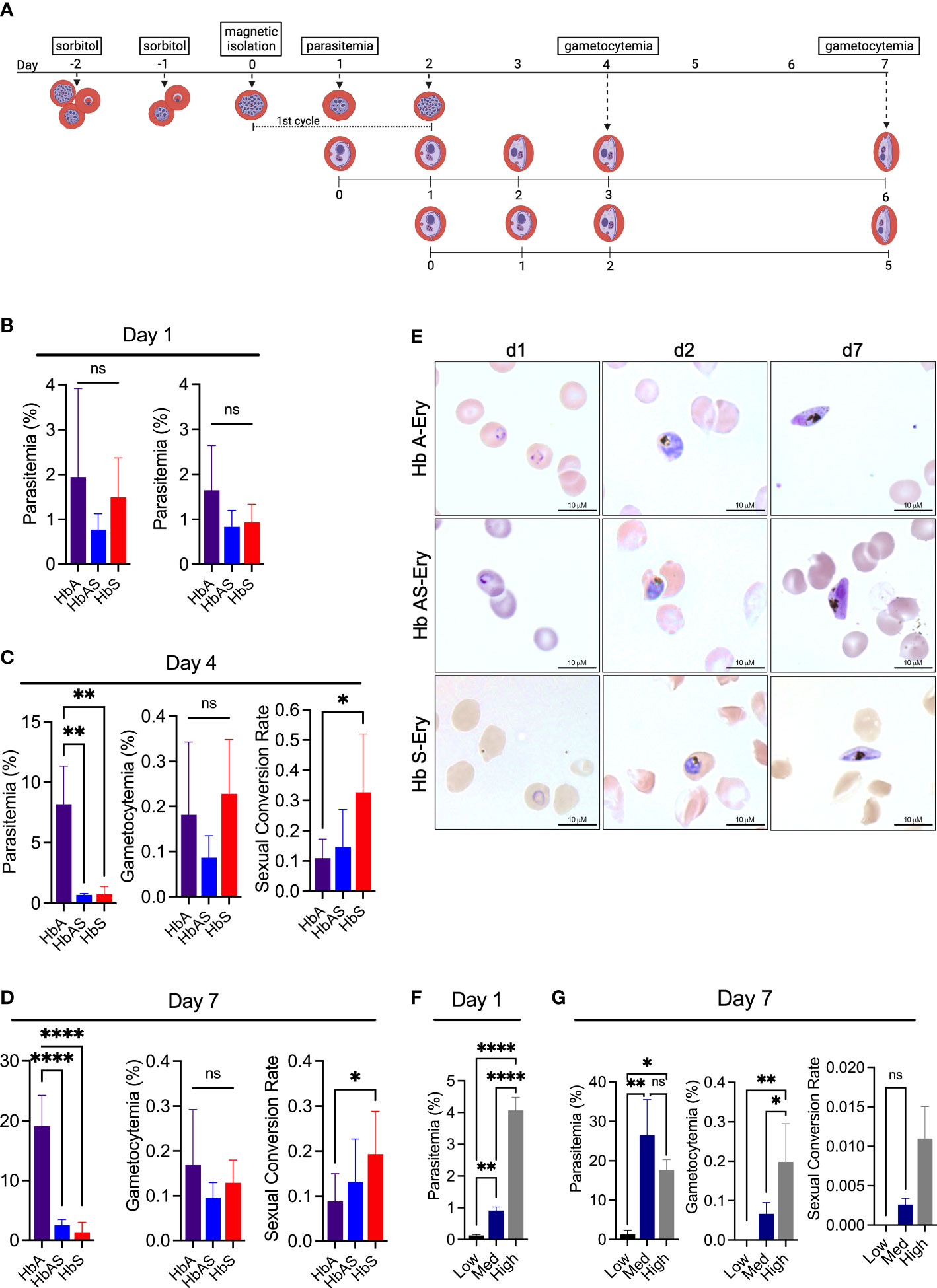
Figure 1 P. falciparum sexual conversion rate is positively influenced by Hb S-Ery. (A) Schematic of culture initiation and gametocyte development. Cultures were begun on day 0 with magnetically isolated schizonts that were synchronized to a six-hour development window by subsequent sorbitol synchronizations. Media was changed daily thereafter. Created with BioRender.com (B) Day one parasitemia measured by flow cytometry (left) and microscopy (right) 24 hours after inoculation (n=6) (C) Day four parasitemia (left), and stage II gametocytemia (middle) measured by microscopy. Resulting SCR (right), calculated from microscopy measurements of parasitemia at day one and stage II gametocytemia on day four (n=6) (D) Day seven parasitemia (left), and stage III gametocytemia (middle) measured by microscopy. Resulting SCR (right), calculated from microscopy measurements of parasitemia at day one and stage III gametocytemia on day seven (E) Representative images of cultures on day one, two, and seven of assay. Images captured using Olympus Provis microscope (100x) and obtained from blood smears stained with Giemsa. (F) Day one parasitemia measured by microscopy 24 hours after inoculation. Low, Medium, and High cultures started with 0.1%, 0.5%, and 1% schizonts respectively (n=3) (G) Day seven parasitemia (left), and stage III gametocytemia (middle) measured by microscopy. SCR (right) calculated from microscopy measurements of parasitemia at day one and stage III gametocytemia measurement at day seven (n=3). ns, non-significant, *p<0.05, **p<0.01, ****p<0.0001, one-way ANOVA.
To verify these results were not an artifact of an overall low parasitemia among the Hb S- and Hb AS-Ery cultures, we set up an additional experiment exclusively in Hb A-Ery. These cultures were inoculated with dilutions of the isolated schizont pellets, which generated varying levels of parasitemia on day one (represented as “Low”, “Medium”, and “High”) and mimicked the different parasitemia levels observed among the Hb S- and Hb AS-Ery cultures (Figure 1F). Among these experiments, we observed the opposite trend in gametocyte conversion, as the Low parasitemia group resulted in a lower rate of SC. No gametocytes were detected by microscopy in the low parasitemia group, and no change in SCR was observed among the Med and High parasitemia group (Figure 1G). Notably in this group of experiments, we observed a slightly lower SCR among the Med experimental group than the Hb A group in Figure 1F. Though the Med group had the most comparable parasitemia on day one, their day seven rates vary more significantly, potentially accounting for the difference in overall SCR. These results ultimately indicate the higher rate of sexual conversion observed among the Hb AS- and Hb S-Ery cultures was not due to the low parasitemia achieved by both cultures.
These results show that cells containing Hb S induce a greater rate of SC compared to cells containing Hb A. Interestingly, though the change in SCR between the Hb A and Hb AS cultures was not significant, rates were higher among the Hb AS group, and landed in between that of Hb S and Hb A. This gives the appearance of a dosing effect of Hb S on SC. We did observe a significant difference in parasite proliferation between Hb A and both the Hb AS and Hb S cultures, but intraerythrocytic development did not appear significantly impaired through the first replication cycle in which SCR was determined. Ultimately, these results indicate that Hb S is a driver of sexual stage conversion, and a modifier of P. falciparum’s reproductive investment strategy.
3.2 Parasitic enzyme-mediated digestion of Hb S is more efficient than Hb A
The change in reproductive investment witnessed among the Hb S-Ery cultures exemplifies a shift in investment from intra-host survival to inter-host transmission. This change in reproductive strategy is indicative of a factor restraining the success of intra-host survival, which we observed in the change in parasite proliferation over the culture period (Figures 1C, D). As Hb S appeared to have a dosing effect on SC, we next questioned the protein’s direct effect on the intraerythrocytic cycle. The digestion of Hb is a vital part of the intraerythrocytic cycle, providing the parasite with amino acids needed for protein synthesis and preventing the premature lysis of infected cells (Rudzinska et al., 1965; Shenai et al., 2000; Krugliak et al., 2002; Lew et al., 2003; Sijwali and Rosenthal, 2004; Chugh et al., 2013). Interestingly, it has been previously hypothesized that Hb S may be resistant to digestion by parasite hemoglobinases, ultimately limiting the parasite’s ability to sustain life within these cell environments (Pauling et al., 1949; Archer et al., 2018). Because of the importance of the Hb protein to intraerythrocytic survival, we next looked at the process of Hb digestion as a potential stressor that may prompt the observed change in reproductive investment.
To investigate Hb digestion between Hb A, Hb AS, and Hb S, we analyzed Hb hydrolysis with an in vitro assay (Shenai et al., 2000; Chugh et al., 2013). By combining a high pH, physiologically relevant reducing agent, and recombinant P. falciparum enzyme, falcipain-2 (FP2), this assay provides a clean examination of Hb digestion, while mimicking the essential components of the digestive vacuole environment. Using this assay, significant disparities in hydrolysis efficiency were observed between the Hb variants tested (Figure 2). Overall, we found Hb S to experience the highest rate of hydrolysis and a sample containing Hb A, Hb S, and Hb F (Hb ASF) to experience the most resistance to hydrolysis (Figure 2). Hb F (fetal hemoglobin) was included in analysis because it has previously been reported to be resistant to P. falciparum hemoglobinase activity, and therefore served as a control to demonstrate resistance (Shear et al., 1998). Hb F is an isoform of Hb that is produced during fetal development. Interestingly, though Hb F levels in individuals without hemoglobinopathies decrease to <1% by adulthood, individuals with SCD retain higher levels of Hb F, ranging from 4-10%, and therapeutic induction of Hb F with hydroxyurea has been suggested to provide protection against malaria disease (Archer et al., 2019; Tshilolo et al., 2019; Steinberg, 2020). Though notably a recent investigation has shown Hb F does not inhibit P. falciparum growth (Archer et al., 2019). The Hb ASF sample was obtained from transgenic SCD mice which naturally produce high levels of Hb F.
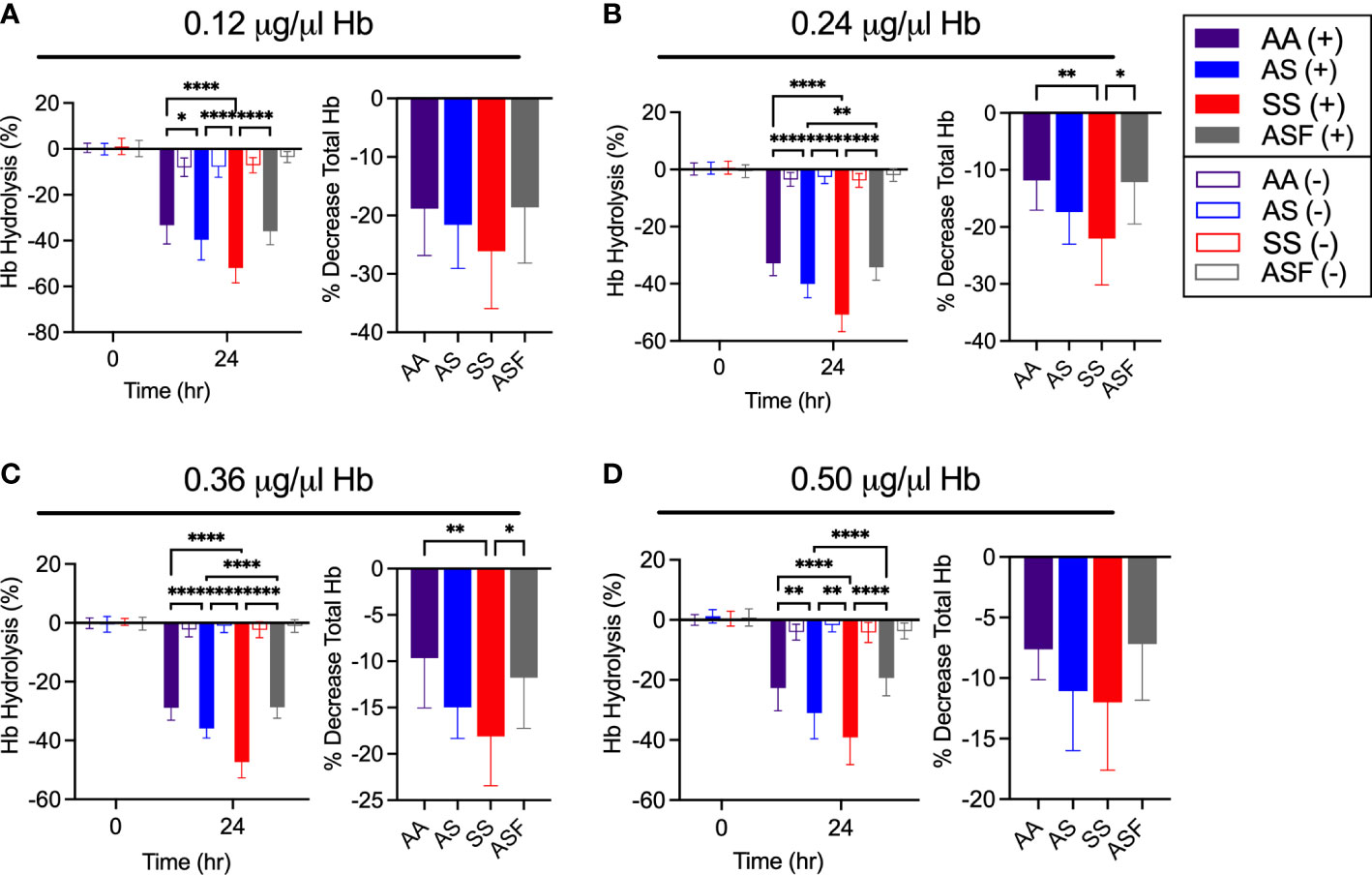
Figure 2 Hb S is more efficiently hydrolyzed by falcipain-2 compared to Hb A, Hb AS, and Hb ASF. Hemoglobin hydrolysis (left) and percent decrease in total Hb concentration (right) by 50 μM FP2 for (A) 0.12, (B) 0.24, (C) 0.36, and (D) 0.5 μg/μl Hb (AA, AS and SS, n=14) (ASF, n=11), *p<0.05, **p<0.01, ****p<0.0001, two-way and one-way ANOVA, respectively.
All results were consistent over the four concentrations tested and were confirmed by total Hb quantification at 24 hours (Figure 2). Negative controls, wells containing Hb in assay buffer without the presence of FP2, for all samples were used to verify the hydrolysis displayed was due to the action of FP2. As Hb S is known to be unstable at low concentrations, these data confirm FP2 had the most hydrolytic activity on Hb S (Perutz and Lehmann, 1968; Carrell and Lehmann, 1969; Rieder, 1970; Seakins et al., 1973).
These results demonstrate that the efficiency of Hb S degradation by FP2 is more rapid than Hb A. As Hb digestion in the parasite’s digestive vacuole results in the release of free heme, an increased rate of Hb hydrolysis would lead to a faster rate of heme release. Interestingly, Hb S cells are known to contain higher concentrations of heme at baseline (Bensinger and Gillette, 1974; Hebbel et al., 1988; Liu et al., 1988; Uzunova et al., 2010). As heme is a powerful oxidant, known to be toxic to both the human host and parasite, we questioned whether this alteration of Hb digestion and heme concentration may be a relevant factor to the previously observed change in SC.
3.3 Therapeutically disrupting Hb digestion promotes sexual conversion
To better understand the relationship between heme release and SC, we therapeutically altered Hb digestion using the antimalarial chloroquine diphosphate (CQ). Chloroquine functions by preventing P. falciparum from detoxifying heme released during Hb digestion (Yayon et al., 1984; Krogstad et al., 1985; Krogstad and Schlesinger, 1987; Slater and Cerami, 1992). Suboptimal doses can measurably limit heme detoxification without eliminating parasite viability. Interestingly, CQ has also been shown to increase rates of SC, which we confirm here by SC and gene expression analysis (Figure 3) (Peatey et al., 2009; Combrinck et al., 2013; Abshire et al., 2017). For these experiments, cultures were treated with CQ for 48 hours, followed by the addition of N-acetylglucosamine (NAG) to prevent further asexual growth (Figure 3A). The rate of SC was then calculated using the total gametocytemia captured on day seven from the point of NAG addition (Figure 3B).
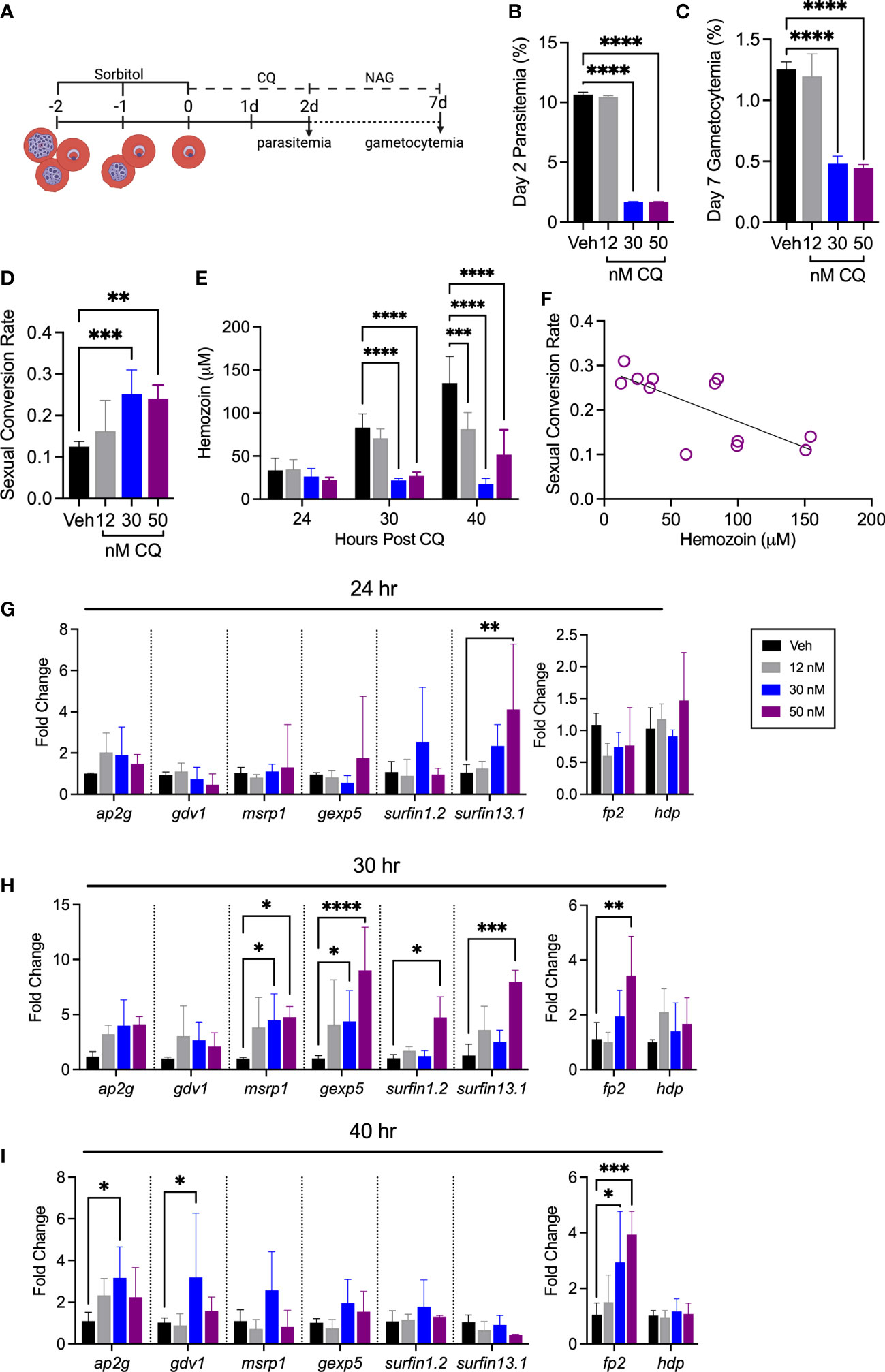
Figure 3 Sexual conversion rate is increased after treatment with chloroquine diphosphate. (A) Schematic of experimental design. Hb A-Ery cultures were synchronized to a four-hour window and plated during the early ring stage (0-4 hrs post infection). Media was changed daily, with NAG addition at 48 hours. Created with BioRender.com (B) Day two parasitemia and (C) day seven gametocytemia measured by flow cytometry. (D) SCR calculated from flow cytometry measurements of parasitemia at day two and gametocytemia measurement at day seven (n=6), **p<0.01, ***p<0.001, ****p<0.0001, one-way ANOVA. (E) Quantification of hemozoin content after CQ treatment in Hb A-Ery cultures (n=3), ***p<0.001, ****p<0.0001, two-way ANOVA. (F) Correlation between hemozoin content and SCR. Pearson r= -0.7244, **p<0.01. (G–I) Early gametocyte genes, ap2-g, gdv1, msrp1, gexp5, surfin 1.2, and surfin 13.1 (left), and genes relevant for the process of Hb digestion, falcipain-2 (FP2) and heme detoxification protein (hdp) (right) (G) 24 hours, (H) 30 hours, and (I) 40 hours post CQ treatment in Hb A-Ery cultures *p<0.05, **p<0.01, ****p<0.0001, two-way ANOVA.
No differences in total parasitemia were observed between all treatment groups through 40 hours of treatment (Supplementary Figure 2). Though the 30 nM treatment group appeared morphologically normal through 40 hours, total parasitemia was significantly lower at the point of NAG addition (Figure 3B, Supplementary Figure 2). The calculated rates of SC reveal a significant increase among the 30- and 50 nM treatment groups compared to the vehicle (Figure 3D). Furthermore, quantification of hemozoin content after CQ treatment revealed an overall negative correlation between hemozoin production and SCR (Figures 3E, F). Gene expression analysis of early gametocyte genes support this increase in SC, while analysis of genes involved with Hb digestion support a disruption of the Hb degradation process (Figures 3G-I). Interestingly, though appearing morphologically normal, the 30 nM treatment group displayed the largest decrease in total hemozoin content and the highest rate of SC.
These results are in line with other reports that have found an increase in SC with sub-optimal doses of CQ (Peatey et al., 2009). It is known intracellular heme levels are increased during CQ treatment, which in these experiments is supported in the 30 nM treatment group by the change in hemozoin content in the absence of a change in total parasitemia and parasite morphology (Supplementary Figure 2) (Abshire et al., 2017). Therefore, the increase in SC observed here supports a relationship between heme concentration and SC. Considering this, we next investigated whether direct hemin exposure on Hb A-Ery P. falciparum cultures would similarly impact SCR.
3.4 Hemin treatment increases sexual conversion in P. falciparum
To determine whether hemin influences SC, Hb A-Ery cultures were treated with hemin for 48 hours. SCR was determined as performed in the previous experiment, with NAG addition at 48 hours and final gametocytemia determined on day seven (Figures 4A-D). Representative images from day ten of culture are additionally presented to show mature gametocyte development in all cultures (Figure 4E). Interestingly, we found SC to increase significantly with doses of hemin starting at 10 μM (Figure 4B). This was further supported during quantification of day ten images, which followed the trend of increasing total gametocytemia with increasing doses of hemin (Figure 4F).
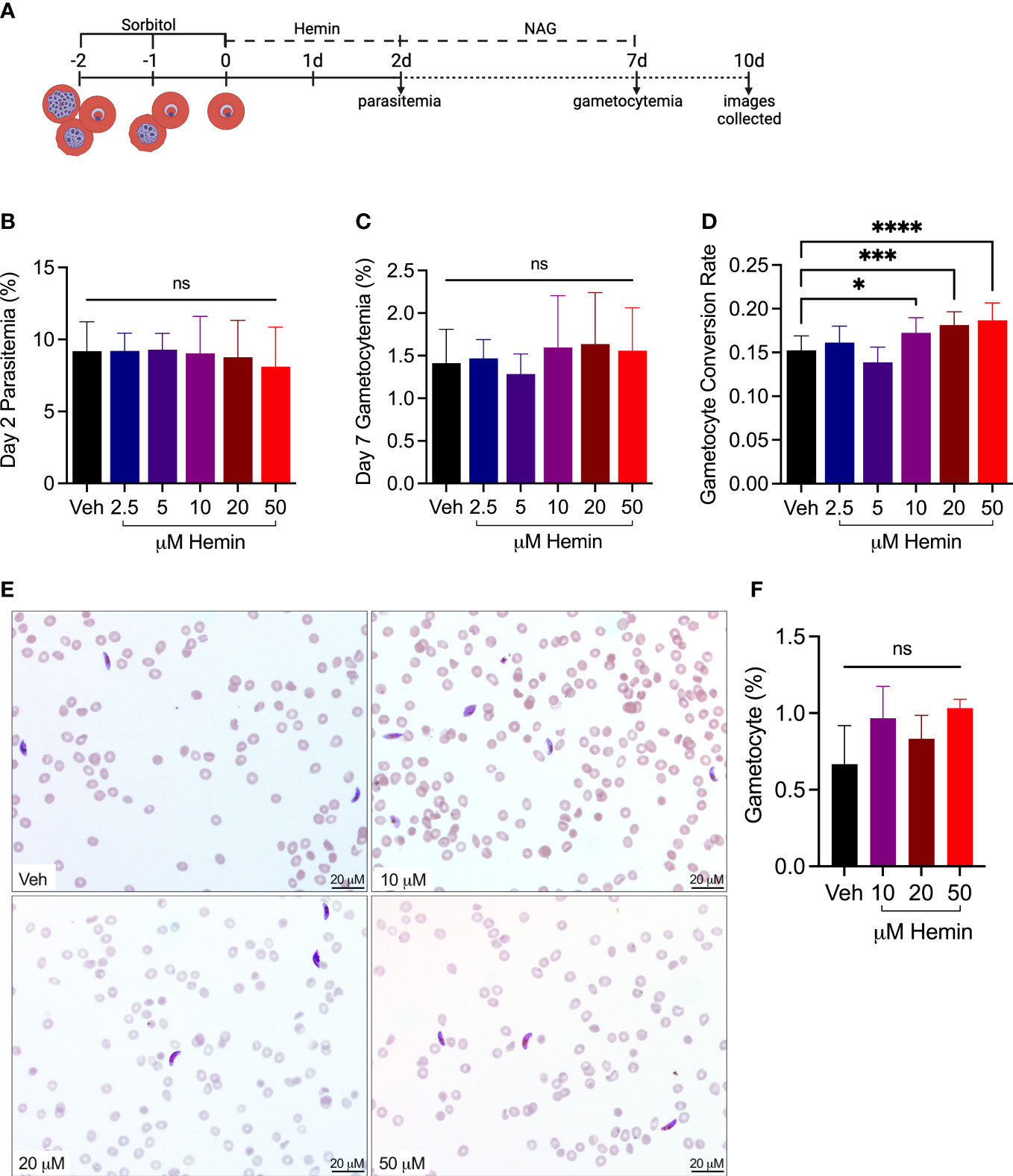
Figure 4 Treatment with hemin increases rate of sexual conversion. (A) Schematic of experimental design. Hb A-Ery cultures were synchronized to a 4 hour window and plated during the early ring stage (0-4 hrs post infection). Media was changed daily, with NAG addition at 48 hours. Created with BioRender.com (B) Day two parasitemia and (C) and day seven gametocytemia measured by flow cytometry. (D) SCR calculated from flow cytometry measurements of gametocytemia at day seven divided by the peak parasitemia at day two (n=12), ns, non-significant, *p<0.05, ***p<0.001, ****p<0.0001, one-way ANOVA. (E) Representative images of hemin-treated and vehicle Hb A-Ery cultures on day 10 of assay. Images captured using Olympus Provis microscope (40x) and obtained from blood smears stained with Giemsa. (F) Quantification of gametocytemia from representative images (n=3).
These results show exposure to increased levels of extracellular hemin increases the rate of SC in Hb A-Ery cultures. Notably, the rate of increase in the culture exposed to 50 μM was similar to that observed in the Hb S-Ery cultures.
3.5 Changes in ap2-g expression in response to hemin treatment are not reflected in the epigenetic landscape
Aiming to further confirm this change in SCR during hemin exposure, we investigated relevant gene expression and epigenetic marks associated with the process of SC. Total gene expression of ap2-g was increased among treatment groups between 48-64-, and 72-80-hours post exposure, overall supporting the observed increased rates of SC (Figure 5A). Going further, we attempted to identify changes in the epigenetic landscape of ap2-g. The ap2-g gene locus remains epigenetically repressed by H3K9me3 during asexual replication. De-repression of ap2-g is therefore marked by H3K9Ac. ChIP-qPCR of H3K9me3 and H3K9Ac revealed no differences in the epigenetic landscape during hemin treatment (Figures 5B, C). However, due to the overall rate of asexual replication remaining >80%, a modest difference, if any at all, was anticipated.
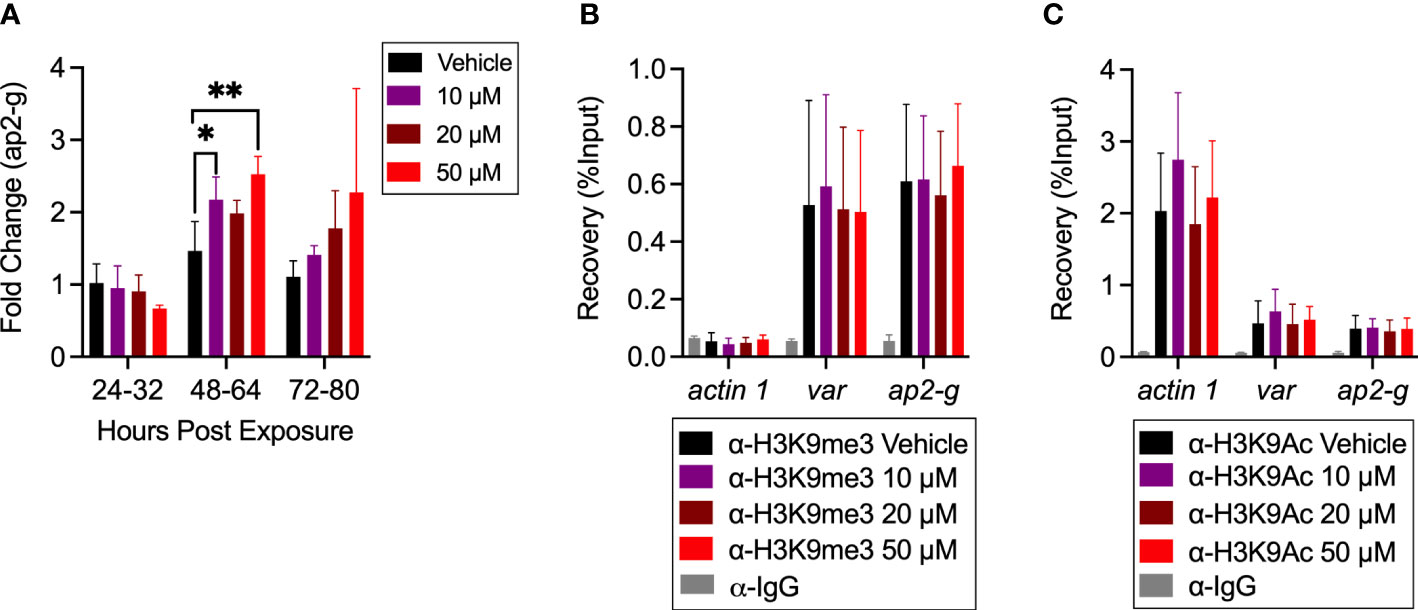
Figure 5 Investigation of epigenetic landscape after hemin treatment. (A) Gene expression of ap2-g at 24-32 hour, 48-64 hour, and 72-80 hour after hemin treatment in Hb A-Ery cultures (n=3), *p<0.05, **p<0.01, two-way ANOVA. (B, C) ChIP-qPCR represented as recovery of % input (n=6). (B) H3K9Me3 and (C) H3K9Ac occupancy at selected loci in vehicle and hemin treated Hb A-Ery cultures at 48-64 hours of hemin treatment. Tested loci include actin-1, used as a euchromatic control, and var used as a heterochromatic control. Anti-Rabbit IgG was used as a negative control. Results are obtained from biological replicates.
4 Discussion
Sexual conversion dynamics of P. falciparum are known to be influenced by different sources of environmental pressure, and the ability of Plasmodium spp. to alter its reproductive investment is an instrumental survival strategy in line with many evolutionary biology theories (Pollitt et al., 2011; Carter et al., 2013; Schneider et al., 2018). As specific sources of sexual stage initiation remain elusive, a growing understanding of how Plasmodium spp. are able to balance intra-host survival with inter-host transmission is critical to understanding trends and fluctuations in pathogen virulence (Josling and Llinas, 2015; Usui and Williamson, 2021).
In this study, we demonstrate that SC is positively influenced by the Hb S variant. As Hb hydrolysis efficiencies have previously been shown to vary between Hb types, and Hb degradation is integral to the intraerythrocytic cycle, we focus on this process as a potential factor for the observed change in reproductive effort. After revealing FP2-induced hydrolysis of Hb S is significantly more efficient than Hb A, and demonstrating the effect CQ has in increasing both SC and intracellular heme levels, we implicate heme, as a product of Hb hydrolysis, to be a potentially relevant component for SC. We go on to expose Hb A-Ery cultures to increasing levels of hemin and discover increased rates of SC with increasing exposure concentrations. This establishes heme as a potential factor which promotes SC.
To our knowledge, this is the only study directly comparing the generation of gametocytes in vitro between these two Hb types. Though it is known SCRs vary in vivo, the average commitment rate per asexual cycle has been estimated to be <1% (Cao et al., 2019). Therefore, a naturally occurring condition which we found to result in a SCR of 20% is striking. While we anticipated lower proliferation in the Hb S- and Hb AS-Ery cultures, we did not observe a significant difference in the total parasitemia on day one of the assay. Moreover, the lack of morphological differences on day two indicate the SCR determination from this first cycle was a point of valid comparison. We acknowledge SCR determination through microscopy presents sources of variability, however, we present parasitemia obtained from flow cytometry to show little variation between the two methods. Additionally, our reported SCRs, and the SCR trends observed among the CQ-treated experimental groups, are similar and consistent with what has been published in other studies (Brancucci et al., 2015; Brancucci et al., 2017; Usui and Williamson, 2021). Future experiments would benefit from the use of gametocyte-specific reporter strains for faster and simpler quantification.
As total parasitemia on day seven significantly differed between experimental groups, we further validated our calculated SCR by repeating the experiment in Hb A-Ery cultures with varying levels of parasitemia. While the low parasitemia group had a comparable total parasitemia to the Hb S- and Hb AS-Ery cultures, we were unable to identify any gametocytes. This establishes the calculated SCRs were not biased by differences in total parasitemia. Furthermore, these results together with the previous set of experiments demonstrate two defined strategies of reproductive investment by P. falciparum: terminal investment and reproductive restraint. The lack of proliferation coupled with the increased rate of SC in the Hb S- and Hb AS- Ery cultures indicate terminal investment, while the lack of SC during the same level of total parasitemia in the Hb A-Ery culture indicates reproductive restraint.
In considering possible explanations for this observed adjustment in reproductive investment, we first explored Hb. We were initially drawn to investigating Hb digestion because of the Hb AS results, which interestingly landed in between that of Hb A and Hb S. This gave the appearance of a direct effect due to the Hb S protein. Our analysis of Hb digestibility by P. falciparum FP2 revealed Hb S to be more readily hydrolyzed compared to Hb A. Though FP2 is one of several P. falciparum proteases known to be active in the Hb degradation process, it has been shown to be active during the early phases of Hb digestion and is an essential protease in the process, with disruption resulting in the accumulation of undigested Hb within the digestive vacuole (Sijwali and Rosenthal, 2004). We acknowledge this assay is a simplified version of Hb digestion, but it is worth noting knock-out studies of other hemoglobinases suggest more of a cooperative function to the overall process (Sijwali and Rosenthal, 2004; Liu et al., 2006).
The finding of increased hydrolysis of Hb S, which consequently indicates more heme release, presented the question of whether heme may be associated with SC. Using CQ to alter intracellular heme concentrations, we determined a negative correlation between SC and hemozoin content. This was particularly significant among the 30 nM treatment group which exhibited the largest increase in SC. Interestingly this group overall showed the least amount of total hemozoin production but little change in parasite morphology at the time of hemozoin sampling. This shows Hb digestion was still taking place and is an important designation, as a lack of hemozoin formation may also indicate a lack of parasite development. If Hb digestion is still taking place but hemozoin is not being produced, this indicates an increase in heme. In fact, previous studies have shown CQ concentrations of 40 nM to increase labile heme 2-fold (Abshire et al., 2017). This observation is additionally relevant to the first replication cycle of the Hb S-Ery cultures, as though we know asexual proliferation is impacted, within the first asexual cycle, we show little change in parasite morphology. This likewise indicates Hb digestion was taking place, though we were ultimately unable to measure corresponding heme levels or hemozoin content. The doses of CQ used in this study were based on a previous study which determined the IC10, IC50, and IC90 inhibitory concentrations (Peatey et al., 2009).
Upon directly manipulating levels of extracellular hemin, we found SC to increase. Interestingly, the observed increase was comparable to what was seen in the Hb S-Ery experiments. Though significant, on a whole-culture level more investment into asexual proliferation was still being made for all treatment groups, which explains the absence of a distinguishable difference in the epigenetic landscape related to SC. Overall, these results show hemin to promote SC, which could potentially be exploited for the development of transmission-blocking therapeutics. Importantly, extracellular heme concentrations have been shown to reach maximal levels of 100 μM during mild and severe malaria disease, making the concentrations used here relevant to disease states (Dalko et al., 2015; Elphinstone et al., 2015; Elphinstone et al., 2016). However, we acknowledge the limitation of quantifying the precise hemin concentrations due to serum protein binding.
The finding of increased SCR among Hb S-Ery cultures and during CQ exposure satisfies an evolutionary purpose, as impaired intra-host survival occurs during these conditions (Reece et al., 2009; Carter et al., 2013; Schneider et al., 2018). Intriguingly, though direct exposure to hemin did not appear to significantly alter intra-host survival during the first replication cycle, we still observed an increase in SCR. Over the course of chronic malaria infection, multiple rounds of asexual replication result in hemolysis and subsequent increase in free extracellular heme. Interestingly, chronic malaria infection is also associated with higher SC (Barry et al., 2021). Therefore, based on our findings, it is possible heme is a contributing factor for increasing rates of SC observed during chronic infection.
Our results provide experimental support for previous epidemiologic studies which have observed differences in gametocyte carriage among individuals with Hb variants (Ringelhann et al., 1976; Robert et al., 1996; Gouagna et al., 2010; Bougouma et al., 2012; Goncalves et al., 2017). Considering Hb S-Ery are known to have higher concentrations of intracellular heme, and Hb S-carrying individuals are known to contain higher concentrations of extracellular heme, we propose increased heme concentration as a potential explanation for this observation (Bensinger and Gillette, 1974; Hebbel et al., 1988; Uzunova et al., 2010). Exact mechanisms of how heme contributes to the initiation of SC is an area for future investigation. As methods of controlling asexual replication are increasingly becoming limited, deciphering new ways to control transmission, such as through the prevention of gametocyte transfer from human host to mosquito vector, provide new opportunities for the goal of ending malaria (Conrad and Rosenthal, 2019; Usui and Williamson, 2021).
Data availability statement
The original contributions presented in the study are included in the article/Supplementary Material. Further inquiries can be directed to the corresponding author.
Ethics statement
The studies involving human participants were reviewed and approved by University of Pittsburgh Institutional Review Board. The patients/participants provided their written informed consent to participate in this study.
Author contributions
Project conception was completed by BF and SO-A. Project design was completed by BF, LA, and SO-A. Vital resources and supervision were provided by MD and JT. Data curation, investigation, and analysis was completed by BF. Data interpretation and visualization was completed by BF and SO-A. Article drafting was completed by BF, with critical revision by SO-A. Funding was acquired by SO-A. All authors contributed to the article and approved the submitted version.
Funding
This work was supported by the National Institutes of Health (NIH), National Heart, Lung, and Blood Institute grant U54HL141011 (SO-A).
Acknowledgments
We thank Diane Lenhart and Rimi Hazra for technical support.
Conflict of interest
The authors declare that the research was conducted in the absence of any commercial or financial relationships that could be construed as a potential conflict of interest.
The authors JT and LA declared that they were editorial board members of Frontiers, at the time of submission. This had no impact on the peer review process and the final decision.
Publisher’s note
All claims expressed in this article are solely those of the authors and do not necessarily represent those of their affiliated organizations, or those of the publisher, the editors and the reviewers. Any product that may be evaluated in this article, or claim that may be made by its manufacturer, is not guaranteed or endorsed by the publisher.
Supplementary material
The Supplementary Material for this article can be found online at: https://www.frontiersin.org/articles/10.3389/fmala.2023.1161750/full#supplementary-material
References
Abshire J. R., Rowlands C. J., Ganesan S. M., So P. T., Niles J. C. (2017). Quantification of labile heme in live malaria parasites using a genetically encoded biosensor. Proc. Natl. Acad. Sci. U.S.A. 114, E2068–E2076. doi: 10.1073/pnas.1615195114
Allison A. C. (1954). Protection afforded by sickle-cell trait against subtertian malareal infection. Br. Med. J. 1, 290–294. doi: 10.1136/bmj.1.4857.290
Archer N. M., Petersen N., Clark M. A., Buckee C. O., Childs L. M., Duraisingh M. T. (2018). Resistance to plasmodium falciparum in sickle cell trait erythrocytes is driven by oxygen-dependent growth inhibition. Proc. Natl. Acad. Sci. U.S.A. 115, 7350–7355. doi: 10.1073/pnas.1804388115
Archer N. M., Petersen N., Duraisingh M. T. (2019). Fetal hemoglobin does not inhibit plasmodium falciparum growth. Blood Adv. 3, 2149–2152. doi: 10.1182/bloodadvances.2019000399
Barry A., Bradley J., Stone W., Guelbeogo M. W., Lanke K., Ouedraogo A., et al. (2021). Higher gametocyte production and mosquito infectivity in chronic compared to incident plasmodium falciparum infections. Nat. Commun. 12, 2443. doi: 10.1038/s41467-021-22573-7
Bensinger T. A., Gillette P. N. (1974). Hemolysis in sickle cell disease. Arch. Intern. Med. 133, 624–631. doi: 10.1001/archinte.1974.00320160118010
Bougouma E. C., Tiono A. B., Ouedraogo A., Soulama I., Diarra A., Yaro J. B., et al. (2012). Haemoglobin variants and plasmodium falciparum malaria in children under five years of age living in a high and seasonal malaria transmission area of Burkina Faso. Malar. J. 11, 154. doi: 10.1186/1475-2875-11-154
Brancucci N. M. B., Gerdt J. P., Wang C., De Niz M., Philip N., Adapa S. R., et al. (2017). Lysophosphatidylcholine regulates sexual stage differentiation in the human malaria parasite plasmodium falciparum. Cell 171, 1532–1544 e15. doi: 10.1016/j.cell.2017.10.020
Brancucci N. M., Goldowitz I., Buchholz K., Werling K., Marti M. (2015). An assay to probe plasmodium falciparum growth, transmission stage formation and early gametocyte development. Nat. Protoc. 10, 1131–1142. doi: 10.1038/nprot.2015.072
Cao P., Collins K. A., Zaloumis S., Wattanakul T., Tarning J., Simpson J. A., et al. (2019). Modeling the dynamics of plasmodium falciparum gametocytes in humans during malaria infection. Elife 8. doi: 10.7554/eLife.49058.033
Carrell R. W., Lehmann H. (1969). The unstable haemoglobin haemolytic anaemias. Semin. Hematol. 6, 116–132.
Carter L. M., Kafsack B. F., Llinas M., Mideo N., Pollitt L. C., Reece S. E. (2013). Stress and sex in malaria parasites: why does commitment vary? Evol. Med. Public Health 2013, 135–147. doi: 10.1093/emph/eot011
Carter R., Miller L. H. (1979). Evidence for environmental modulation of gametocytogenesis in plasmodium falciparum in continuous culture. Bull. World Health Organ 57 Suppl 1, 37–52.
Chaubey S., Grover M., Tatu U. (2014). Endoplasmic reticulum stress triggers gametocytogenesis in the malaria parasite. J. Biol. Chem. 289, 16662–16674. doi: 10.1074/jbc.M114.551549
Chugh M., Sundararaman V., Kumar S., Reddy V. S., Siddiqui W. A., Stuart K. D., et al. (2013). Protein complex directs hemoglobin-to-hemozoin formation in plasmodium falciparum. Proc. Natl. Acad. Sci. U.S.A. 110, 5392–5397. doi: 10.1073/pnas.1218412110
Combrinck J. M., Mabotha T. E., Ncokazi K. K., Ambele M. A., Taylor D., Smith P. J., et al. (2013). Insights into the role of heme in the mechanism of action of antimalarials. ACS Chem. Biol. 8, 133–137. doi: 10.1021/cb300454t
Conrad M. D., Rosenthal P. J. (2019). Antimalarial drug resistance in Africa: the calm before the storm? Lancet Infect. Dis. 19, e338–e351. doi: 10.1016/s1473-3099(19)30261-0
Dalko E., Das B., Herbert F., Fesel C., Pathak S., Tripathy R., et al. (2015). Multifaceted role of heme during severe plasmodium falciparum infections in India. Infect. Immun. 83, 3793–3799. doi: 10.1128/IAI.00531-15
Elphinstone R. E., Conroy A. L., Hawkes M., Hermann L., Namasopo S., Warren H. S., et al. (2016). Alterations in systemic extracellular heme and hemopexin are associated with adverse clinical outcomes in Ugandan children with severe malaria. J. Infect. Dis. 214, 1268–1275. doi: 10.1093/infdis/jiw357
Elphinstone R. E., Riley F., Lin T., Higgins S., Dhabangi A., Musoke C., et al. (2015). Dysregulation of the haem-haemopexin axis is associated with severe malaria in a case-control study of Ugandan children. Malar. J. 14, 511. doi: 10.1186/s12936-015-1028-1
Ghosh S., Adisa O. A., Chappa P., Tan F., Jackson K. A., Archer D. R., et al. (2013). Extracellular hemin crisis triggers acute chest syndrome in sickle mice. J. Clin. Invest. 123, 4809–4820. doi: 10.1172/JCI64578
Goldberg D. E., Slater A. F., Cerami A., Henderson G. B. (1990). Hemoglobin degradation in the malaria parasite plasmodium falciparum: an ordered process in a unique organelle. Proc. Natl. Acad. Sci. U.S.A. 87, 2931–2935. doi: 10.1073/pnas.87.8.2931
Goncalves B. P., Sagara I., Coulibaly M., Wu Y., Assadou M. H., Guindo A., et al. (2017). Hemoglobin variants shape the distribution of malaria parasites in human populations and their transmission potential. Sci. Rep. 7, 14267. doi: 10.1038/s41598-017-14627-y
Gouagna L. C., Bancone G., Yao F., Yameogo B., Dabire K. R., Costantini C., et al. (2010). Genetic variation in human HBB is associated with plasmodium falciparum transmission. Nat. Genet. 42, 328–331. doi: 10.1038/ng.554
Grosse S. D., Odame I., Atrash H. K., Amendah D. D., Piel F. B., Williams T. N. (2011). Sickle cell disease in Africa: a neglected cause of early childhood mortality. Am. J. Prev. Med. 41, S398–S405. doi: 10.1016/j.amepre.2011.09.013
Hebbel R. P., Morgan W. T., Eaton J. W., Hedlund B. E. (1988). Accelerated autoxidation and heme loss due to instability of sickle hemoglobin. Proc. Natl. Acad. Sci. U.S.A. 85, 237–241. doi: 10.1073/pnas.85.1.237
Heller P., Best W. R., Nelson R. B., Becktel J. (1979). Clinical implications of sickle-cell trait and glucose-6-phosphate dehydrogenase deficiency in hospitalized black male patients. N Engl. J. Med. 300, 1001–1005. doi: 10.1056/NEJM197905033001801
Hoeijmakers W., Bartfai R. (2018). Characterization of the nucleosome landscape by micrococcal nuclease-sequencing (MNase-seq). Methods Mol. Biol. 1689, 83–101. doi: 10.1007/978-1-4939-7380-4_8
Hogan A. B., Jewell B. L., Sherrard-Smith E., Vesga J. F., Watson O. J., Whittaker C., et al. (2020). Potential impact of the COVID-19 pandemic on HIV, tuberculosis, and malaria in low-income and middle-income countries: a modelling study. Lancet Glob. Health 8, e1132–e1141. doi: 10.1016/S2214-109X(20)30288-6
Josling G. A., Llinas M. (2015). Sexual development in plasmodium parasites: knowing when it's time to commit. Nat. Rev. Microbiol. 13, 573–587. doi: 10.1038/nrmicro3519
Josling G. A., Williamson K. C., Llinas M. (2018). Regulation of sexual commitment and gametocytogenesis in malaria parasites. Annu. Rev. Microbiol. 72, 501–519. doi: 10.1146/annurev-micro-090817-062712
Krogstad D. J., Schlesinger P. H. (1987). Acid-vesicle function, intracellular pathogens, and the action of chloroquine against plasmodium falciparum. N Engl. J. Med. 317, 542–549. doi: 10.1056/NEJM198708273170905
Krogstad D. J., Schlesinger P. H., Gluzman I. Y. (1985). Antimalarials increase vesicle pH in plasmodium falciparum. J. Cell Biol. 101, 2302–2309. doi: 10.1083/jcb.101.6.2302
Krugliak M., Zhang J., Ginsburg H. (2002). Intraerythrocytic plasmodium falciparum utilizes only a fraction of the amino acids derived from the digestion of host cell cytosol for the biosynthesis of its proteins. Mol. Biochem. Parasitol. 119, 249–256. doi: 10.1016/S0166-6851(01)00427-3
Larsen R., Gozzelino R., Jeney V., Tokaji L., Bozza F. A., Japiassu A. M., et al. (2010). A central role for free heme in the pathogenesis of severe sepsis. Sci. Transl. Med. 2, 51ra71. doi: 10.1126/scitranslmed.3001118
Lew V. L., Tiffert T., Ginsburg H. (2003). Excess hemoglobin digestion and the osmotic stability of plasmodium falciparum-infected red blood cells. Blood 101, 4189–4194. doi: 10.1182/blood-2002-08-2654
Liu J., Istvan E. S., Gluzman I. Y., Gross J., Goldberg D. E. (2006). Plasmodium falciparum ensures its amino acid supply with multiple acquisition pathways and redundant proteolytic enzyme systems. Proc. Natl. Acad. Sci. U.S.A. 103, 8840–8845. doi: 10.1073/pnas.0601876103
Liu S. C., Zhai S., Palek J. (1988). Detection of hemin release during hemoglobin s denaturation. Blood 71, 1755–1758. doi: 10.1182/blood.V71.6.1755.1755
Oduma C. O., Ogolla S., Atieli H., Ondigo B. N., Lee M. C., Githeko A. K., et al. (2021). Increased investment in gametocytes in asymptomatic plasmodium falciparum infections in the wet season. BMC Infect. Dis. 21, 44. doi: 10.1186/s12879-020-05761-6
Pauling L., Itano H. A., Singer S. J., Wells I. C. (1949). Sickle cell anemia, a molecular disease. Science 110, 543–548. doi: 10.1126/science.110.2865.543
Peatey C. L., Skinner-Adams T. S., Dixon M. W., Mccarthy J. S., Gardiner D. L., Trenholme K. R. (2009). Effect of antimalarial drugs on plasmodium falciparum gametocytes. J. Infect. Dis. 200, 1518–1521. doi: 10.1086/644645
Perutz M. F., Lehmann H. (1968). Molecular pathology of human haemoglobin. Nature 219, 902–909. doi: 10.1038/219902a0
Piel F. B., Patil A. P., Howes R. E., Nyangiri O. A., Gething P. W., Williams T. N., et al. (2010). Global distribution of the sickle cell gene and geographical confirmation of the malaria hypothesis. Nat. Commun. 1, 104. doi: 10.1038/ncomms1104
Pollitt L. C., Mideo N., Drew D. R., Schneider P., Colegrave N., Reece S. E. (2011). Competition and the evolution of reproductive restraint in malaria parasites. Am. Nat. 177, 358–367. doi: 10.1086/658175
Reece S. E., Ramiro R. S., Nussey D. H. (2009). Plastic parasites: sophisticated strategies for survival and reproduction? Evol. Appl. 2, 11–23. doi: 10.1111%2Fj.1752-4571.2008.00060.x
Rieder R. F. (1970). Hemoglobin stability: observations on the denaturation of normal and abnormal hemoglobins by oxidant dyes, heat, and alkali. J. Clin. Invest. 49, 2369–2376. doi: 10.1172/JCI106456
Ringelhann B., Hathorn M. K., Jilly P., Grant F., Parniczky G. (1976). A new look at the protection of hemoglobin AS and AC genotypes against plasmodium falciparum infection: a census tract approach. Am. J. Hum. Genet. 28, 270–279.
Robert V., Tchuinkam T., Mulder B., Bodo J. M., Verhave J. P., Carnevale P., et al. (1996). Effect of the sickle cell trait status of gametocyte carriers of plasmodium falciparum on infectivity to anophelines. Am. J. Trop. Med. Hyg. 54, 111–113. doi: 10.4269/ajtmh.1996.54.111
Rudzinska M. A., Trager W., Bray R. S. (1965). Pinocytotic uptake and the digestion of hemoglobin in malaria parasites. J. Protozool 12, 563–576. doi: 10.1111/j.1550-7408.1965.tb03256.x
Schneider P., Greischar M. A., Birget P. L. G., Repton C., Mideo N., Reece S. E. (2018). Adaptive plasticity in the gametocyte conversion rate of malaria parasites. PloS Pathog. 14, e1007371. doi: 10.1371/journal.ppat.1007371
Seakins M., Gibbs W. N., Milner P. F., Bertles J. F. (1973). Erythrocyte hb-s concentration. an important factor in the low oxygen affinity of blood in sickle cell anemia. J. Clin. Invest. 52, 422–432. doi: 10.1172/JCI107199
Shear H. L., Grinberg L., Gilman J., Fabry M. E., Stamatoyannopoulos G., Goldberg D. E., et al. (1998). Transgenic mice expressing human fetal globin are protected from malaria by a novel mechanism. Blood 92, 2520–2526. doi: 10.1182/blood.V92.7.2520
Shenai B. R., Sijwali P. S., Singh A., Rosenthal P. J. (2000). Characterization of native and recombinant falcipain-2, a principal trophozoite cysteine protease and essential hemoglobinase of plasmodium falciparum. J. Biol. Chem. 275, 29000–29010. doi: 10.1074/jbc.M004459200
Sijwali P. S., Rosenthal P. J. (2004). Gene disruption confirms a critical role for the cysteine protease falcipain-2 in hemoglobin hydrolysis by plasmodium falciparum. Proc. Natl. Acad. Sci. U.S.A. 101, 4384–4389. doi: 10.1073/pnas.0307720101
Slater A. F., Cerami A. (1992). Inhibition by chloroquine of a novel haem polymerase enzyme activity in malaria trophozoites. Nature 355, 167–169. doi: 10.1038/355167a0
Srivastava A., Creek D. J., Evans K. J., De Souza D., Schofield L., Muller S., et al. (2015). Host reticulocytes provide metabolic reservoirs that can be exploited by malaria parasites. PloS Pathog. 11, e1004882. doi: 10.1371/journal.ppat.1004882
Steinberg M. H. (2020). Fetal hemoglobin in sickle hemoglobinopathies: high HbF genotypes and phenotypes. J. Clin. Med. 9. doi: 10.3390/jcm9113782
Thom C. S., Dickson C. F., Gell D. A., Weiss M. J. (2013). Hemoglobin variants: biochemical properties and clinical correlates. Cold Spring Harb. Perspect. Med. 3, a011858. doi: 10.1101/cshperspect.a011858
Trager W., Gill G. S. (1992). Enhanced gametocyte formation in young erythrocytes by plasmodium falciparum in vitro. J. Protozool 39, 429–432. doi: 10.1111/j.1550-7408.1992.tb01476.x
Trager W., Gill G. S., Lawrence C., Nagel R. L. (1999). Plasmodium falciparum: enhanced gametocyte formation in vitro in reticulocyte-rich blood. Exp. Parasitol. 91, 115–118. doi: 10.1006/expr.1998.4347
Tshilolo L., Tomlinson G., Williams T. N., Santos B., Olupot-Olupot P., Lane A., et al. (2019). Hydroxyurea for children with sickle cell anemia in Sub-Saharan Africa. N Engl. J. Med. 380, 121–131. doi: 10.1056/NEJMoa1813598
Usui M., Williamson K. C. (2021). Stressed out about plasmodium falciparum gametocytogenesis. Front. Cell Infect. Microbiol. 11, 790067. doi: 10.3389/fcimb.2021.790067
Uzunova V. V., Pan W., Galkin O., Vekilov P. G. (2010). Free heme and the polymerization of sickle cell hemoglobin. Biophys. J. 99, 1976–1985. doi: 10.1016/j.bpj.2010.07.024
Van Der Kolk M., Tebo A. E., Nimpaye H., Ndombol D. N., Sauerwein R. W., Eling W. M. (2003). Transmission of plasmodium falciparum in urban Yaounde, Cameroon, is seasonal and age-dependent. Trans. R Soc. Trop. Med. Hyg. 97, 375–379. doi: 10.1016/S0035-9203(03)90059-9
Vinchi F., Gastaldi S., Silengo L., Altruda F., Tolosano E. (2008). Hemopexin prevents endothelial damage and liver congestion in a mouse model of heme overload. Am. J. Pathol. 173, 289–299. doi: 10.2353/ajpath.2008.071130
Weatherall D. J. (2008). Hemoglobinopathies worldwide: present and future. Curr. Mol. Med. 8, 592–599. doi: 10.2174/156652408786241375
Weiss D. J., Bertozzi-Villa A., Rumisha S. F., Amratia P., Arambepola R., Battle K. E., et al. (2021). Indirect effects of the COVID-19 pandemic on malaria intervention coverage, morbidity, and mortality in Africa: a geospatial modelling analysis. Lancet Infect. Dis. 21, 59–69. doi: 10.1016/S1473-3099(20)30700-3
Keywords: Heme, Plasmodium falciparum, hemoglobin S (HbS), sexual conversion rate, malaria transmission
Citation: Flage B, Dent MR, Tejero J, Amoah LE and Ofori-Acquah SF (2023) Heme promotes sexual conversion of Plasmodium falciparum in human erythrocytes. Front. Malar. 1:1161750. doi: 10.3389/fmala.2023.1161750
Received: 08 February 2023; Accepted: 06 June 2023;
Published: 26 June 2023.
Edited by:
Marion Avril, MalarVx, United StatesReviewed by:
Ariel H. Magallon-Tejada, Gorgas Memorial Institute of Health Studies, PanamaHarry Dailey, University of Georgia, United States
Copyright © 2023 Flage, Dent, Tejero, Amoah and Ofori-Acquah. This is an open-access article distributed under the terms of the Creative Commons Attribution License (CC BY). The use, distribution or reproduction in other forums is permitted, provided the original author(s) and the copyright owner(s) are credited and that the original publication in this journal is cited, in accordance with accepted academic practice. No use, distribution or reproduction is permitted which does not comply with these terms.
*Correspondence: Solomon Fiifi Ofori-Acquah, sfo2@pitt.edu