- 1The First Clinical Medical College, Lanzhou University, Lanzhou, China
- 2Department of General Surgery, The First Hospital of Lanzhou University, Lanzhou, China
- 3Key Laboratory of Biological Therapy and Regenerative Medicine Transformation Gansu Province, Lanzhou, China
- 4Department of Gastroenterology, The First Hospital of Lanzhou University, Lanzhou, China
- 5Key Laboratory for Gastrointestinal Diseases of Gansu Province, Lanzhou University, Lanzhou, China
- 6Department of Medical Oncology, The First Hospital of Lanzhou University, Lanzhou, China
- 7Storr Liver Centre, Westmead Institute for Medical Research, University of Sydney at Westmead Clinical School, Westmead, NSW, Australia
Liver diseases are a major health concern globally, and are associated with poor survival and prognosis of patients. This creates the need for patients to accept the main alternative treatment of liver transplantation to prevent progression to end-stage liver disease. Investigation of the molecular mechanisms underpinning complex liver diseases and their pathology is an emerging goal of stem cell scope. Human induced pluripotent stem cells (hiPSCs) derived from somatic cells are a promising alternative approach to the treatment of liver disease, and a prospective model for studying complex liver diseases. Here, we review hiPSC technology of cell reprogramming and differentiation, and discuss the potential application of hiPSC-derived liver cells, such as hepatocytes and cholangiocytes, in refractory liver-disease modeling and treatment, and drug screening and toxicity testing. We also consider hiPSC safety in clinical applications, based on genomic and epigenetic alterations, tumorigenicity, and immunogenicity.
Introduction
Liver disease causes ~2 million deaths annually worldwide. Cirrhosis-related complications account for 50% of deaths, and viral hepatitis and hepatocellular carcinoma (HCC) together account for the other 50% of deaths annually worldwide. Cirrhosis is currently the 11th most common cause of death globally, and liver cancer is the 16th leading cause of death. Cirrhosis and HCC together account for 3.5% of all deaths worldwide (1). Currently, liver transplantation is the most effective treatment option for patients with end-stage liver disease (ESLD). However, <10% of global liver transplantation needs are met (2). The shortage of donor organs and transplant costs are the major limiting factors (3). In addition, the recipient's immune system may reject the transplanted organ (4). Therefore, the development of alternative therapeutic strategies for patients with chronic liver disease is of utmost importance.
Human induced pluripotent stem cells (hiPSCs) are pluripotent stem cells that are induced and reprogrammed from adult cells into undifferentiated cells by exposure to specific mediators. They have the capacity for self-renewal and differentiation into a variety of somatic cells, such as embryonic stem cells (5). HiPSC-derived hepatocyte-like cells (hiPSC-HLCs) exhibit morphological and phenotypic characteristics of primary human hepatocytes (PHHs) (6). hiPSC-HLCs should be incorporated into future studies of liver diseases to solve the problems of organ shortage and prevent recipient immunorejection of PHHs. hiPSC-HLCs can be considered as an ideal source of hepatocytes. In addition, these cells may enable cell-based therapy, exploration of liver disease models, in vitro models for pharmacology and toxicology studies (7) and ESLD treatment.
In this review article, we aimed to provide a perspective on the current organization and provision of transplant services based on specific challenges and environmental settings. We review how these specific requirements are addressed by the existing cell culture systems for the generation of hiPSC-derived hepatocytes, describe their applications for modeling hepatic disorders, and discuss future directions for the use of hiPSCs in the study and treatment of liver diseases.
Recent Developments in the iPSC Reprogramming Methodology
Protocols for somatic cells transformed into induced pluripotent stem cells are widely used for iPSC reprogramming method. The key to the success of these protocols is the ability to efficiently induce pluripotent cells to adopt a definitive endoderm fate. Earlier studies have shown that mouse fibroblasts can be reprogrammed to develop embryonic stem cell (ESC)-like features, and grow in the presence of four factors: Oct3/4, Sox2, c-Myc, and Klf4 (8). These induced cells were named iPSCs. These insights opened the door to further refinements in the cell differentiation methodology and enabled the derivation of visceral, endodermal-derived tissues. The same protocol was used to successfully generate iPSCs from adult human skin cells (9). In addition, human somatic cells were transformed into PSCs by inducing the expression of a new set of four factors: Oct4, Sox2, Nanog, and Lin28 (10). Many optimization steps have been devised to improve the efficiency of reprogramming factors, such as the use of integration-free methods (8, 11). Episomal vectors, Sendai viruses, and synthetic mRNAs are among the most commonly used methods for generating hiPSCs (12–14) without modifying the host genome, which could interfere with disease modeling or experimental outcomes.
Current Approaches for Generation of hiPSC-HLCs and Cholangiocyte-Like Cells
In 2009, hiPSCs were induced to differentiate into hepatic cells for the first time, via a timed administration of various growth factors. The expression of hepatocyte markers and liver-related functions in hiPSC-HLCs was monitored and compared with those in differentiated human ESCs and PHHs (15). This revealed that hepatic cells could be generated from iPSCs but the process took more than 20 d. To overcome this, the differentiation step was revised to a more efficient three-step protocol, allowing rapid generation of HLCs from hiPSCs (Figure 1) (16). Hepatic progenitor-like cells derived from hiPSCs possess the potential for bipotent differentiation into HLCs and cholangiocyte-like cells (17).
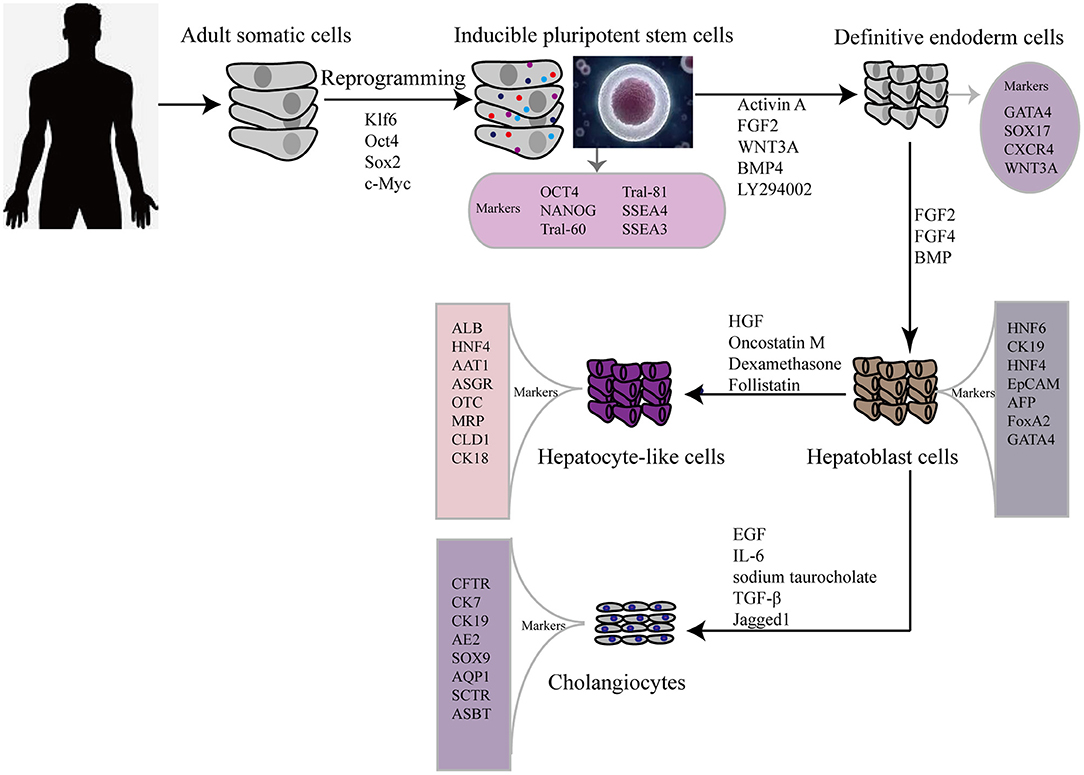
Figure 1. Flow diagram showing typical protocol for iPSCs reprogramming and hepatic differentiation of human into hepatocyte-like cells in vitro. OCT 4, octamer binding transcription factor 3/4; SSEA, stage-specific embryonic antigen; TRA, Tumor resistance antigen 1-60; SOX2 sex determining region Y box 2; KLF4 (Kruppel-like factor 4); GATA4, GATA binding protein 4; CXCR4, C-X-C chemokine receptor type 4; FGF, fibroblast growth factor; HGF, hepatocyte growth factor; BMP, bone morphogenetic protein; HNF6, Hepatocyte nuclear factor 6; CK, cytokeratin; EpCAM, Epithelial cell adhesion molecule; AFP, alpha-fetoprotein; AAT1, α1-anti-tripsin; ALB, albumin; ASGR, asialoglycoprotein receptor; MRP, multidrug resistance protein; CLD, claudin; EGF, epidermal growth factor; IL-6, interleukin 6; TGF-β, transforming growth factor beta; CK7, cytokeratin 7; CK19, cytokeratin 19; AE2, chloride/bicarbonate anion exchanger 2; ASBT, apical sodium-dependent bile acid transporter; CFTR, cystic fibrosis transmembrane conductance regulator; AQP1, aquaporin-1; SOX9, SRY-box 9; SCTR, secretin receptor.
For clinical applications, differentiated cells should be assessed by comparing them with primary liver-derived cells, to verify their morphology and expression of liver-specific proteins, such as alpha-fetoprotein (AFP) and albumin. However, neither ESCs nor iPSCs can differentiate into fully mature hepatocytes in vitro. As a consequence of an emerging interest in using iPSCs in regenerative medicine to treat liver diseases, many researchers are focused on developing the most efficient and reproducible approaches for the derivation of high-quality hiPSC-HLCs. Currently, the differentiation strategies for obtaining hiPSC-HLCs generally require a stepwise induction of definitive endoderm, hepatocyte specification, and hepatoblast expansion into mature HLCs. Activin A, Wnt3a (18)and fibroblast growth factor 2 (FGF) signaling (19) play important roles in hiPSC differentiation toward hepatic endoderm, whereas hepatocyte growth factor (HGF) promotes the growth of hepatoblast cells (20, 21). Another factor, the interleukin (IL) 6 family cytokine oncostatin M (OSM), combined with the glucocorticoid dexamethasone (DEX), accelerates the maturation of hepatocytes. Meanwhile, many protocols for iPSC differentiation to hepatocytes have been reported, which involve the use of various growth factors and cytokines, plating techniques, and transduction of key liver-specific transcription factors (22).
Other studies have reported strategies for the generation of cholangiocyte-like cells from hiPSCs. The first differentiation of cholangiocyte-like cells from hiPSC hepatoblasts was induced in the presence of growth hormone, epidermal growth factor (EGF), IL-6, and sodium taurocholate (23). Subsequently, efficient differentiation of cholangiocytes from iPSCs was improved by 3D co-culture of hepatoblasts and OP9 stromal cells in the presence of HGF, EGF, and TGF-β (24), and by stimulating cholangiocyte progenitor specification using FGF10, activin A, and retinoic acid (25). Yet another stepwise cholangiocyte differentiation approach involves a definitive endoderm–hepatic specification–hepatic progenitor–cholangiocyte procedure, with Jagged1 and TGF-β supplementation being key for the promotion of iPSC-cholangiocyte formation (26). These cells were subsequently characterized in vitro and in vivo. Induced-cholangiocytes show mature markers, such as SOX9, CK7, CK19, CFTR, AE2, ASBT, AQP1, and SCTR and they are negative for the hepatocyte marker HNF4a.
Features and Functions oF hiPSC-HLCs
The liver performs a wide range of fundamental functions, including metabolic, nutrient storage, and detoxification functions. Hence, each protocol for an efficient maturation of iPSCs into HLCs needs to incorporate a thorough and critical evaluation of the hepato-specific transcriptome and enzymatic activities of the obtained cells. Drug metabolizing capacity, urea cycle activity, or bile acid and lipoprotein synthesis and excretion need to be evaluated to ascertain HLC maturation (Figure 2). By contrast, most of the current reports on HLC generation show that HLCs express genes at levels that are characteristic for the fetal liver and display a fetal-like phenotype. According to one study (26), HLC mitochondria display specific morphological changes, such as elongation, swollen cristae, dense matrix, and cytoplasmic migration, with an increased expression of mitochondrial DNA transcription and replication-related genes, and increased oxygen consumption. Following differentiation, HLCs express liver-specific proteins, including albumin and hepatocyte nuclear factor 4 alpha, and show intrinsic hepatocyte functions, including CYP450 activity. However, HLCs also express high levels of AFP, suggesting a persistent immature phenotype or inability to turn off early-stage genes. Furthermore, albumin production, urea production, CYP450 activity, and mitochondrial function of HLCs are significantly lower than those of primary human hepatocytes. Functional indicators, urea synthesis, glycogen synthesis, lipid storage, indocyanine green intake, and low-density lipoprotein intake, are also used to evaluate the function of HLCs (27–31) (Table 1). Liver function after HLC transplantation in disease-specific models in mouse is assessed using glycogen content synthesis, low-density lipoprotein, and indocyanine green intake (32).
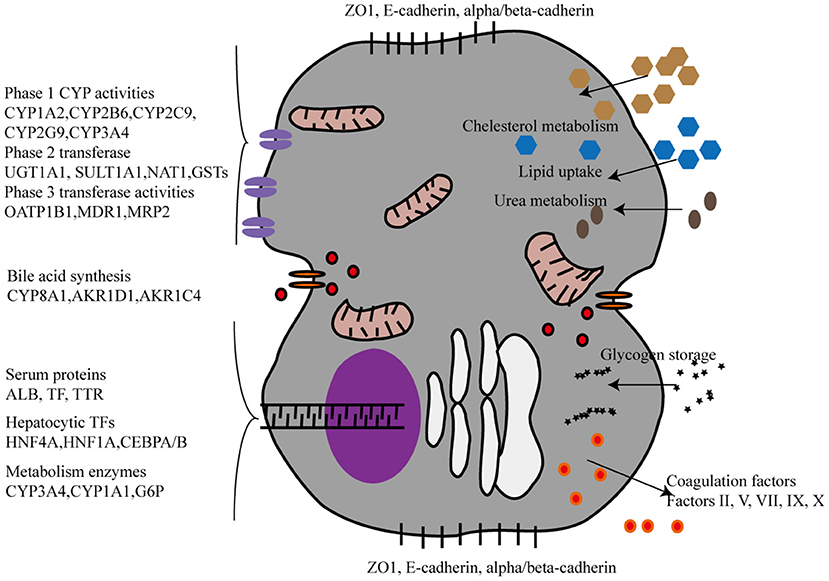
Figure 2. The phenotypic characterization of Hepatocyte-like cells derived from iPSCs. CYP, CYPs cytochromes P450; UGT, UDP glucuronosyltransferase. SULT1A1, Sulfotransferase Family 1A Member 1; NAT1, N-Acetyltransferase 1; GSTs, Glutathione S-Transferase; OATP, organic anion-transporting polypeptide; MDR, Multiple drug resistance; MRP2:multidrug resistance protein 2; AKR1D1, aldo-keto reductase family 1 member D1, AKR1C4, aldo-keto reductase family 1 member C4, TTR:Transthyretin, TP, Total protein, ALB, Albumin, HNF, Hepatocyte nuclear factor, CEBPA/B, CCAAT Enhancer Binding Protein Alpha/Beta, G6P, Glucose-6-Phosphatase.
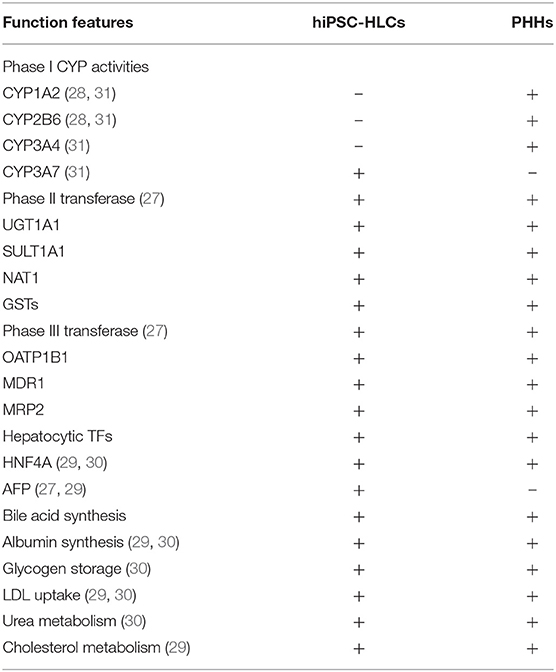
Table 1. Functional features of hepatocyte-like cells (HLCs) derived from human induced pluripotent stem cells.
Hepatocyte isolation is not easy to perform, and requires expertise and access to primary human liver tissue. An alternative strategy for the acquisition of hepatocytes may grant the researchers access to important control material, thanks to commercially available cryopreserved hepatocytes, or as an alternative to using human liver tissue. Recently, an important and detailed report describing the expression of more than 60 hepatic, pluripotency, and developmental genes has been published to map their changes during liver cell isolation and stem cell maturation into HLC (33). In this report, the specific traits of iPSC-HLC were compared with those of adult liver and fetal liver cells, proving that several hepatic enzymes, whose expression is limited during the fetal period and absent at the postnatal stage, are highly expressed in iPSC-HLCs. In the study, CYP3A4 and CYP3A7 genes were used for a convenient and quick evaluation of hepatic maturation.
hiPSC-HLCs for the Treatment of ESLD
At present, some promising stem cell-based transportation treatments for ESLD have been reported. Several studies have demonstrated the restoration of liver function in hematopoietic stem cells (HSCs), mesenchymal stem cells (MSCs), and endothelial progenitor cells (EPCs) in acute liver failure (34–37). Although alleviation of liver dysfunction was observed in patients who have received these treatments in clinical trials, the safety and short-term efficacy of stem cell-based transplantation were not explored in detail and should be further evaluated in large-scale prospective cohort studies (38). In fact, stem cell transplantation as an alternative treatment for ESLD cannot be used for remodeling injured hepatic structure. In theory, iPSC-HLCs can be regarded as the optimal cell replacement treatment for acute liver failure (ALF) and ESLD. Meanwhile, HLCs derived from patient-specific iPSCs are an unlimited source of hepatocytes to treat liver failure.
To date, the application of iPSCs in ALF and liver failure has been tested in animal models. Indeed, iPSC-HLC transplantation improves the general condition in a mouse model of CCl4-induced liver injury (39). Further, the survival rate of immunodeficient mice with ALF significantly increased and liver fibrosis levels in mice with chronic liver injury significantly decreased after hiPSC-HLC transplantation (40). According to another study, hiPSC-HLCs rescue drug-induced ALF in rodents, and hiPSC-HLCs have functional and proliferative potential for liver regeneration after transplantation in an ALF model (41, 42). These studies indicated the potential of these cells in regenerative medicine for future clinical applications. Of note, the aforementioned studies only focused on rodent models rather than primates. To date, no studies have illustrated the application of iPSC-HLCs in human liver failure.
The first transplantation of hiPSCs in regenerative medicine took place in 2014, and was performed to treat a 77-year-old female patient with polypoidal choroidal vasculopathy in both eyes. Autologous iPSCs were generated from the patient's skin fibroblasts, differentiated into retinal pigment epithelium, and then transplanted into the eye. Immune response to autologous transplantation was observed 18 months postoperatively, and the results indicated no complications. The retinal pigment epithelial sheet that had been transplanted survived well, and the corrected visual acuity of the treated eye did not improve or worsen.
Unfortunately, not all cases are as successful as the autologous transplant discussed above. Another clinical trial involving three patients with age-related macular degeneration involved intravitreal administration of stem cells derived from autologous adipose tissue. Post-treatment observation revealed vision loss associated with ocular hypertension, hemorrhagic retinopathy, vitreous hemorrhage, combined traction and rhegmatogenous retinal detachment, or lens dislocation (43).
The above trials demonstrate the need for further investigation of stem cell therapy and, more specifically, the use of hiPSCs in regenerative medicine to treat ESLD.
hiPSC-HLC Modeling of Liver Disease Development
Infectious Liver Diseases
Hepatitis B virus (HBV) and hepatitis C virus (HCV) are the most prevalent agents of infectious liver diseases. Approximately 520 million people suffer from infections caused by one of these viruses worldwide, including 170 million people with HBV and 350 million people with HCV infections (44). The infected population will develop virus-related cirrhosis and liver cancer (45). At present, the interaction between these viruses and host hepatocytes remains unclear because of the absence of viral culture models to reflect the infection process and reproduce the molecular events within an infected host cell.
PHHs are the gold standard for studying the physiopathology of liver infections. However, low cell viability and yield caused by a rapid loss of hepatic phenotype upon isolation from the liver microenvironment are the main limitations of their application. As an alternative to overcome these limitations, iPSC-HLCs could be used to study the viral infection process and virus–host interactions, as well as the viral life cycle, to ultimately identify efficacious drugs for infectious liver diseases.
In the past, iPSC-HLCs have been successfully used as an in vitro system for modeling hepatitis virus infections and virus–host interactions. When HCV entry and genomic replication is stimulated, iPSC-HLCs more commonly express HCV receptors and show increased susceptibility to HCV infection than PHHs (46). Further, HCV entry inhibitor (CD81 antibody) and HCV genomic replication inhibitor (interferon) attenuate HCV pseudovirus entry and HCV sub-genomic replication, respectively, in iPSC-HLCs. It has been reported that hiPSC-HLCs support the entire life cycle of HCV, including the inflammatory response to infection and host genetics impacting viral pathogenesis (31). Furthermore, iPSC-HLCs that show an appropriate antiviral response produce interferon (47, 48) and survive in vitro for up to 1 week after inoculation with HCV (47), comprising a superior model for observing hepatocyte function during a relatively long-term infection. Detailed molecular mechanisms allowing viral infection can also be investigated using this model (49).
The above observations suggest that iPSC-HLCs can act as a promising cell model for analyzing hepatocyte responses to viral infection, as well as an ideal platform for drug target discovery for HCV therapy. However, some limitations should be considered. For example, some studies have reported lower virus titers in culture supernatants of HBV-infected iPSC-HLCs than those in PHHs (50), and suggested lack of functional maturation of iPSC-HLCs obtained using various differentiation protocols. Finally, it is necessary to increase the diversity of hiPSC lines used in similar such analyses to assess the impact of the host genetic background on the cellular response and efficiency of infection.
Inherited Metabolic Disorders of the Liver (IMDs)
The liver is vital for metabolic homeostasis. Approximately 70% of patients with IMDs are affected by liver tissue damage. α1-Antitrypsin deficiency (AATD) and familial hypercholesterolemia (FH) are common IMDs that have been extensively studied. AATD results from a single base-pair mutation (leading to Glu342Lys substitution in the protein product), known as the Z mutation, in the SERPINA1 gene. The substitution causes the protein to misfold and be retained in the endoplasmic reticulum (ER), with the formed protein polymers inducing hepatocyte death (51). By contrast, FH is an autosomal dominant hypercholesterolemia caused by mutations in a gene for the low-density lipoprotein receptor (LDLR) or LDLR-related genes. FH is characterized by elevated serum levels of low-density lipoprotein (LDL)-cholesterol (C), which lead to xanthoma formation and premature cardiovascular disease (52, 53).
The use of iPSC-HLCs as a novel model of the above monogenic diseases and iPSC-HLC application for a gene correction therapy for the generation of disease-free autologous cells are attracting increasing attention. For instance, accumulation of A1AT variant polymers in the endoplasmic reticulum of iPSC-HLCs was observed when iPSCs from AATD patients were differentiated into HLCs (54). Further, biochemical features and morphological manifestations of iPSC-HLC models generated from cells from AATD patients with and without severe liver disease (SLD) were explored (55). The analysis of individual disease phenotypes of AATD patients revealed rapid degradation of misfolded α1-antitrypsin Z (ATZ) and no globular inclusions in cells from patients in which the liver disease had been ameliorated (55). Similar, the main pathological characteristic of FH was recapitulated by inducing iPSCs obtained from patients with FH to form HLCs (54). In addition, analysis of iPSC-HLCs obtained from an FH patient with a mutation in the LDLR gene demonstrated that FH-derived iPSC-HLCs are unable to take up LDL-C, and secrete more apolipoprotein B-100 than the controls (56). Furthermore, these HLCs do not respond to statin treatment (56). Together, these observations demonstrate that IMD-hiPSCs effectively model the pathological features of IMD.
Animal models have been used to assess the efficacy of iPSC-HLC therapy in IMDs (57). The first reported targeted gene correction of AATD in iPSCs was achieved by bi-allelic correction of mutated loci by using zinc finger nucleases (ZFNs) and PB technology to correct the A1AT gene in hiPSCs (58). Genetic correction of iPSC-HLCs restored the normal structure and function of the A1AT protein in vitro and in vivo. Gene correction for AATD has been also successfully attempted using the TALEN approach, which is more efficient than ZEN (59). In addition, the use of CRISPR/Cas9 gene editing technology to correct point mutations in specific genes has been reported (60) and experimental data have highlighted the advantages of using the CRISPR/Cas9 system for allele-specific genome targeting and for gene disruption mediated by non-homologous end joining. Indeed, CRISPR/Cas9 genome editing was used to permanently correct a 3-bp homozygous deletion in LDLR exon 4 in patient-derived homozygous FH (HoFH)-iPSCs (61). This genetic correction restored LDLR-mediated endocytosis in FH-HLCs and is a proof-of-principle that CRISPR-mediated genetic modification can be successfully used to normalize HoFH cholesterol metabolism deficiency at the cellular level. The above findings clearly demonstrate that genome-editing technology can be used to achieve functional correction of patient-derived iPSC-HLCs.
Non-alcoholic Fatty Liver Disease (NAFLD)
NAFLD is becoming a serious clinical concern because of its severe morbidity and potential progression to ESLD, such as liver cirrhosis and HCC (62). The current global prevalence of NAFLD is estimated to be 25.24% (63) and NAFLD is the second most common cause of liver transplantation (64). The disease develops when chronic hepatic lipid accumulation stimulates an overload of metabolic alterations, including mitochondrial dysfunction, endoplasmic reticulum stress, and hepatic insulin resistance, and induces an inflammatory response (65–68). It is not possible to predict the progression of NAFLD because of adverse events and sampling variability of the currently used invasive diagnostic methods (liver biopsy) (69).
Several studies have reported cell models of NAFLD that use HCC cell lines or immortalized primary hepatocytes (70, 71) Differences in cell function and gene expression between the two have been demonstrated. However, the main limitation of these models is that cultivation of liver biopsy-derived primary hepatocytes for NAFLD modeling takes several days. Hence, using iPSC-HLCs to model NAFLD and non-alcoholic steatohepatitis (NASH) would facilitate research on molecular diagnosis and prognosis, disease progression, and drug development for NAFLD. hiPSC-HLCs have been used as an in vitro model to first demonstrate intracellular lipid accumulation in NAFLD (72). Major changes in the expression of metabolism-associated genes and upregulation of the lipid droplet-coating protein Perilipin2 (PLIN2) were detected in the model. Upregulation of the expression of numerous genes of the peroxisome proliferator-activated receptor (PPAR) pathway, constituting a regulatory hub for metabolic processes, was also detected. Taking previous studies into consideration, an iPSC-HLCs model with the PNPLA3 genotype that is closely associated with hepatic steatosis in moderate (30–40% fatty changes) and severe (70% fatty changes) NAFLD patients was established and characterized (73, 74). Other studies, on the association between endoplasmic reticulum stress response and hepatocyte metabolism disorders in hiPSC-HLCs have been published (75). ER stress pathways play an important role in lipid metabolism, and ER stress enhances lipid accumulation ~5-fold in hiPSC-HLCs compared to their respective controls (71).
Taken together, the hiPSC-HLC model of NAFLD can be used to characterize some of the metabolic features of NAFLD. However, the pathological progression of lipotoxicity in NAFLD involves not only lipid accumulation but also a complex pathophysiological response, such as inflammation and the immune response. Future studies should highlight the applicability of hiPSC-HLCs as a discovery platform for the exploration of molecular events and aid in drug development for NAFLD.
Liver Cirrhosis
Liver cirrhosis is characterized by the presence of diffuse, chronic necro-inflammatory, and fibrogenetic hepatocytes, ultimately leading to the development of features of chronic liver injury, such as structurally abnormal nodules, dense fibrotic septa, concomitant parenchymal exhaustion, and collapse of the liver tissue (76). Chronic liver injury remains one of the most common causes of death in the Western world (77). Currently, modeling liver cirrhosis in vitro to unveil the intricate cellular interactions underlying its pathological development is challenging. Traditional approaches involve treatment of cell culture models with toxic drugs or compounds that are often not specific to liver disease (78). Meanwhile, 3D models of primary hepatocyte co-culture with stellate cells are limited by the availability of tissue samples (79). 3D-Organoid cell culture models using iPSC-HLCs, stromal cells, and Kupffer cells (80, 81) can be an excellent approach to study the cellular interactions underlying the pathophysiology of liver cirrhosis. However, some chronic liver injuries, such as cardiac cirrhosis, are difficult to study in an in vitro setting and require major advances in 3D co-culture systems.
Previous studies have demonstrated enhanced liver regeneration in mouse model (42), reduced murine liver fibrosis (82) and stabilization of chronic liver disease (39) following iPSC-HLC administration, highlighting its potential as a therapeutic strategy for liver cirrhosis. Recently, the anti-fibrotic properties of iPSCs were demonstrated. Namely, iPSC-derived extracellular vesicles (EVs) were shown to regulate hepatic stellate cell activation and have anti-fibrotic effects (83). Consequently, the use of iPSC-EVs is regarded as a novel anti-fibrotic approach that may reduce or reverse liver fibrosis in patients with chronic liver disease. Furthermore, the therapeutic potential of iPSC-HLCs in liver fibrosis was explored by generation of iPSC-HLCs from mouse embryonic fibroblasts by using a reprogramming technology, and migrating iPSC-HLCs cluster to the intra-spleen and the liver (84). Transplantation of iPSC-HLCs significantly attenuated liver fibrosis induced by CCL4 (80); hence, iPSC-HLCs may be used as a novel therapeutic strategy for the treatment of liver fibrosis. However, while a cell-based therapy for cirrhosis can temporarily relieve cellular hepatocyte injury, it cannot eliminate collagen deposits or restore the original liver structure. Further detailed studies could bring about a novel method by which fibrogenic cells can be reprogrammed into hepatic parenchymal cells in the cirrhotic liver (85).
Liver Cancer
HCC is the third leading cause of cancer-related deaths worldwide and the sixth most common malignancy (86, 87). Liver cancer research mainly focuses on the molecular pathways and treatments for HCC. Within the cancer stem cell (CSC) research, previous reports have explored the possibility of generating liver CSCs by the induction of reprogramming-related factors, such as Oct4 or Nanog (88, 89). However, the induction of HCC cells into liver CSCs using pluripotency-related transcription factors has not yet been widely studied. According to one report, reprogramming can be achieved in tumor cells by retroviral induction of reprograming-associated genes (76). Interestingly, the reprogrammed pluripotent cancer cells (iPCs) were very different from the original cancer cells in terms of colony shape and gene expression of tumor markers. Further, the induction of pluripotent liver cancer cells is correlated with the p53 status, suggesting that varying the gene expression level of p53 may affect the reprogramming process.
In one study, potential tumorigenicity of hiPSC-HLCs during differential induction from hiPSCs to HLC was observed after knockdown of p21 (90). The authors of that study also investigated whether hepatoma-like cells derived from hiPSCs of HCC patients can be transformed into normal hepatocyte cells upon treatment with acyclic retinoid and AKR1B10 inhibitor (tolestat). Combining acyclic retinoid (10 μM) with tolestat (10 μM) is considered to be an appropriate regimen for inducing differentiation of hepatoma-like cells into hepatocytes. The efficacy and toxicity of this combination therapy for individual patients with HCC will be evaluated in the near future.
Previously, iPSCs have been used to treat HCC. More specifically, the therapeutic effect of IPS cell-derived myeloid lineage cells (iPS-ML) and their ability to produce interferon (IFN) β in primary and metastatic liver cancer were reported in mice xenograft model of liver metastasis (91). Further, iPS-ML producing IFN-β injection hindered cancer progression and increased the survival rate in a mouse model.
In summary, at present, the applicability of iPSC technology for HCC treatment mainly focuses on cell reprogramming from HCC to CSCs, and the search for novel HCC treatments. The above studies provide valuable insights for studying and treating HCC.
hiPSC-Cholangiocyte Modeling of Cholangiocyte Disease Development
Cystic fibrosis (CF) is a single-gene inherited disease characterized by mutations in the cystic fibrosis transmembrane conductance regulator gene (CFTR), affecting the function of chloride ion channels, with intrahepatic bile stasis. The iPSC-cholangiocytes are superior to other cells from the perspective of the exploration of these diseases and drug discovery. In 2015, two studies reported generation of cholangiocyte organoids from CF patient-iPSCs using their iPSC-cholangiocytes protocols (24, 92). The disease phenotype was modeled in these studies by exploring non-functional CFTR proteins, with subsequent chloride channel impairment and the inability of fluid secretion to form cysts. The effects of the drug VX809 on CF were also tested, demonstrating a functional rescue of impaired CFTR proteins. Both disease models illustrate valid applications of iPSCs, not only as a proof of pathophysiological interactions, but also for biliary-specific pharmacological screening.
Currently, no other types of cholangiopathy have been modeled using iPSC-cholangiocytes, modeling these disease-types rely on animal models of a deficiency or mutation of disease-specific genes. It may be easier to characterize disease pathophysiology and obtain detailed drug test information if cholangiocytes derived from patient-related iPSC have direct disease-specific genes information. The etiology of biliary atresia, primary biliary cholangitis, primary sclerosing cholangitis, and cholangiocarcinoma is still unknown because of the intricate interaction between the environment and the genes. Therefore, iPSC cholangiocytes derived from disease-specific cases can be studied to confirm the etiological hypotheses and identify potential therapeutic targets.
Drug Discovery and Toxicity Testing
Another important application of iPSCs is assessing the therapeutic effect and toxicity of drugs, as has been reported in many studies (93, 94). The effect of drugs is influenced by the genetic background and other complex factors. It is easier to screen drugs using iPSCs than via pharmacological testing of animals. Consequently, organoids, as an innovative technique for drug screening and toxicity assessment, have been extensively researched. However, iPSC-derived organoids in 2D culture cannot be used for drug screening because iPSCs in such culture receive similar stimuli as those in monolayer and cannot be used to model the physiological microenvironment. By contrast, 3D culture models the in vivo microenvironment and approximates the physiological conditions. Many studies have reported high-throughput screening of small molecule libraries for drug development and toxicity assessment for liver diseases using iPSC-hepatocytes (59, 95, 96). Generation of organoids in several liver cell co-cultures yields a more sensitive cellular model than that constructed using single cell type. Recently, Broutier et al. (97) developed organoids representing the tumor structure and reflecting the expression profile of hepatocellular carcinoma, cholangiocarcinoma, and hepatocellular cholangiocarcinoma. These organoids open up new opportunities for drug testing and personalized medicine, and can be used for the generation of tumor bio-banks to be used as screening platforms (98). Additionally, “liver-on-a-chip” as a platform for drug development and toxicology testing can be used in pharmacokinetic and pharmacodynamic studies. In one study, liver organoids were generated using hepatocytes and cholangiocytes on a perfusable microcapillary chip (99). These organoids were then used to test the dose- and time-dependent hepatotoxic effects of acetaminophen. Thus, “organ-on-a-chip” represents a novel and valid platform for drug testing.
Safety Evaluation of hiPSC-HLCs in Various Applications
While iPSCs have considerable applications in regenerative medicine, the genomic stability of these cells, such as the occurrence of genomic and epigenetic aberrations, copy number variation (CNV), and single-nucleotide polymorphisms (SNPs), is a matter of concern. Genomic aberrations in human PSCs (hPSCs) include abnormal karyotypes, such as recurrent trisomy of chromosomes 12, 17, or X, and aneuploidies of sub-chromosomal regions, such as duplications of the 12p, 17q, or 20q11.21 loci (100). Some studies have demonstrated that iPSCs, unlike somatic cells, contain regions of uniparental disomy (UPD), sharing the equivalent chromosomal and sub-chromosomal characteristics (101). These abnormalities may confer a selective advantage to certain genes during prolonged culturing.
In addition to genomic aberrations, other studies have focused on epigenetic aberrations, but the possibility of hiPSC application for complex diseases remains unknown (102). Epigenetic variations are often observed in different iPSC lines, and prolonged periods of culture (103) may affect disease modeling and clinical applications. For example, reactivating X-chromosome inactivation (XCI) and erosion of inactive X chromosome (Xi) silencing in female hiPSCs during iPSC reprogramming may trigger phenotypic and epigenetic changes in iPSCs, reducing their differentiation potential and increasing their tumorigenicity (104). Furthermore, local epigenetic variations in iPSCs, such as cellular memory and aberrant methylation loci, have been noted. Cellular memory leads to incomplete reprogramming, as DNA hypomethylation and histone modification at specific loci render iPSCs remain similar to the source cell. Further, iPSCs with cellular memory are susceptible to preferential differentiation into the cell type that they had been derived from (105–108). The iPSC-associated methylated loci contain certain imprinted loci and other genomic regions. Some alteration of genomic imprinted loci occurs during cell reprogramming or long-term culture (109–112). For instance, aberrant silencing of the D1K1-Dio3 imprinted locus is functionally associated with the failure to generate iPSCs in mouse during reprogramming (113, 114). Methylome profiling has been used to detect differentially methylated regions (DMRs) in hESCs and hiPSCs (115). In the study, all hypermethylated CG DMRs in hiPSCs were recognized as reprogramming-induced aberrancies.
CNVs and SNVs can be introduced into iPSCs as they may already exist in source cell lines or be acquired during the reprogramming process. hiPSCs contain more CNVs than hESCs, source cells, and somatic cells (116). Therefore, it is possible that iPSCs are more susceptible to CNV generation during cell reprogramming. However, sequencing-based findings suggest the occurrence of few or no detectable de novo CNVs in iPSCs (117–121). By contrast, low-grade genetic mosaicism of CNVs in source cells was tracked to iPSC derivation. Hence, it is possible that low-grade genetic mosaicism of somatic cells is the major source of CNVs in iPSCs. In addition, several studies have discussed the potential relationship between SNVs and iPSC generation. Most studies revealed no specific functional enrichment of genes with SNVs in iPSCs (117, 120, 122, 123). Most iPSC-manifested SNVs appear to be randomly distributed in the genome and are functionally irrelevant to iPSC generation. Therefore, the safety of iPSCs for use in regenerative medicine still faces many challenges.
Another safety concern has been raised regarding immunogenicity and tumorigenicity of the iPSC technology. Both genetic and epigenetic instability arising from iPSC reprogramming increases the risk of immunogenicity and tumorigenicity in vivo during iPSC-derived hepatic cell-associated therapy. Theoretically, the recipients of autologous iPSC-HLC transplants should not reject the transplants (124). However, in a teratoma mouse model, immune rejection in recipients was observed after iPSC transplantation (125). Undifferentiated PSCs, which possess the privilege property of immune tolerance because of low MHC-I antigen expression and the absence of MCH-II antigen expression, are expected to be less immunogenic than iPSCs (126–129). However, abnormal epigenetic differences between iPSCs and PSCs could contribute to the expression of immunogenic antigens during iPSC differentiation (128). Further, iPSC tumorigenicity poses a challenge for the development of individualized iPSC-HLC therapy. Pluripotency acquired by somatic cells upon reprogramming methods can increase genomic instability on the chromosomal and sub-chromosomal levels, contributing to the risk of tumorigenic transformation (130). Therefore, before the clinical development of iPSCs, current reprogramming technologies need to be optimized to minimize the occurrence of immunogenicity and tumorigenicity (Figure 3).
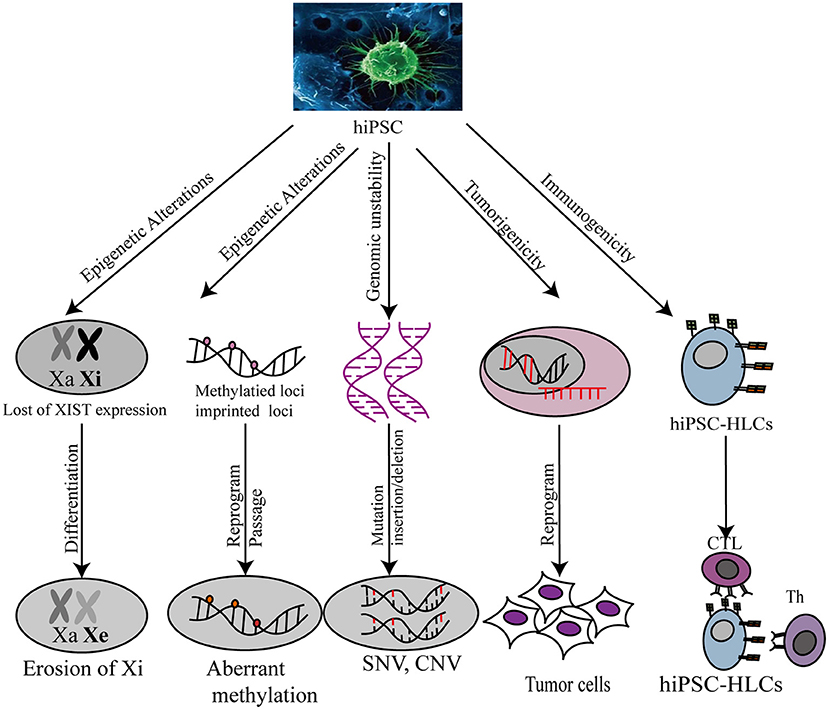
Figure 3. Safety evaluation of hiPSC-HLC in various applications. Xa, X chromosome activity; Xi, X chromosome inactivity; XIST, X-inactive specific transcripts; CNV, copy number variation; SNP, single nucleotide polymorphism.
Conclusion
Together with the burgeoning application of stem cell-based techniques, iPSC technology has been incorporated into new approaches such as -omics–related research, nuclear reprogramming, gene-editing technology, RNAi, tissue engineering, medical devices, high-throughput screens (HTS), and humanized chimeric animal models. iPSCs provide promising opportunities to study novel therapies for liver diseases using cell properties of self-renewal and differentiation for the generation of HLCs. Recent studies have demonstrated that iPSC-derived hepatocytes are applicable for in vitro studies of complex liver disorders, such as viral hepatitis, inherited metabolic disorders, non-alcoholic liver diseases, cirrhosis, and HCC (Figure 4). HLCs can also be used as cell therapy to repair and regenerate liver mass to treat liver disease and prolong patient survival. The genetic and molecular mechanisms underlying liver disorders are an emerging area of research. By studying iPSC-HLCs as a possible treatment option, researchers are able to gain valuable insight into in vitro disease modeling and personalized medicine.
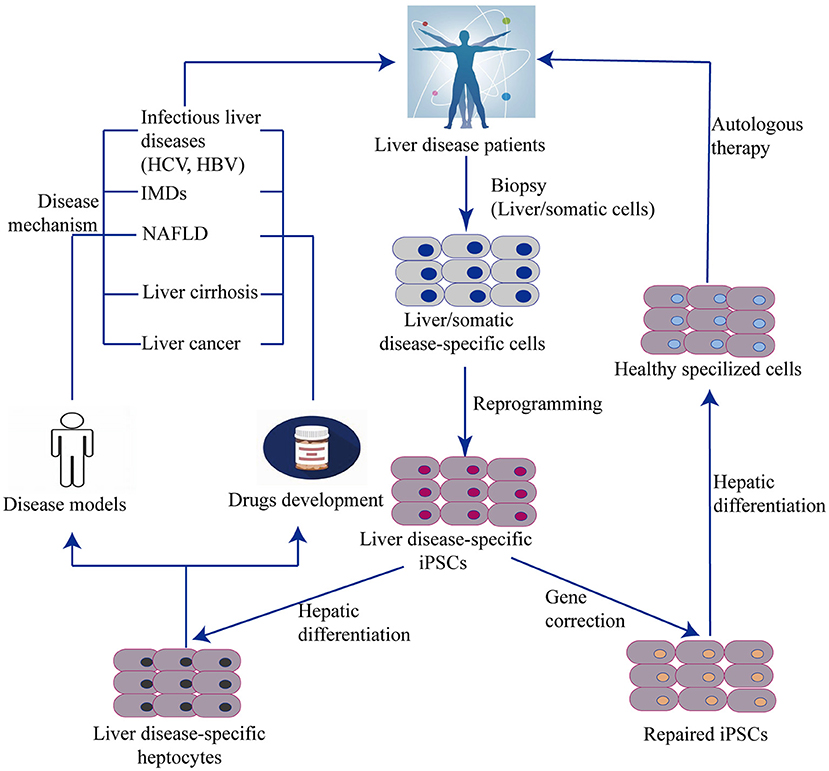
Figure 4. Generation of liver cells from iPSC and their applications. The scheme illustrates an overview of iPSC and organoid technology in relation to liver diseases. Disease specific iPSCs are generated by reprogramming technology from a biopsy of patients. One hand, iPSCs with disease–related gene mutation can be corrected by genome editing and then differentiated into functional disease-specific liver cells in vitro. These corrected cells are used to autologous cell therapy. Another hand, Non-corrected iPSC-derived disease-specific liver cells can be remodeled liver disease phenotypes, pathogenesis, and drug testing. IMD, Inherit metabolism disease; NAFLD, Non-alcoholic fatty liver disease.
Nevertheless, several limitations still exist that prevent the clinical application of iPSC technology. The protocols for reprogramming and differentiation of hiPSCs into HLCs should be optimized and standardized to increase the efficiency of inducibility and promote HLC maturation. The risk of potential tumorigenicity associated with genomic and epigenetic variations should also be assessed. In addition, to commercialize iPSCs for clinical applications, further investigation into the limitations and challenges of using iPSCs is needed. Very few scientific reports have tested HLC injection/transplantation in life-threatening models of congenital diseases. To the best of our knowledge, to date, no single study has been able to demonstrate correction of amino acid or neurotransmitter abnormalities by injecting iPSC-HLCs. Nonetheless, with rapid technological advances in stem cell therapy, hiPSCs are likely to become effective and safe treatment of liver diseases in the future.
Author Contributions
LQ provided the concept of this manuscript and prepared the figures. LZ, KP, and XL drafted this manuscript and prepared the figures. SDWB, RN, SB, and YL revised the manuscript. All authors contributed to the article and approved the submitted version.
Funding
This study was supported by grants from the Talent Innovation and Entrepreneurship Project of Lanzhou City (2019-RC-34) and Science and Technology Project of ChengGuan District, Lanzhou (2020SHFZ0029).
Conflict of Interest
The authors declare that the research was conducted in the absence of any commercial or financial relationships that could be construed as a potential conflict of interest.
Abbreviations
iPSCs, induced pluripotent stem cell; HLC, hepatic like cell; PHH, primary human hepatocytes; confidence interval; ESLD, end-stage liver disease; ESC, embryonic stem cells; IMD, metabolic disorder of liver; HCC, Hepatocellular carcinoma; NAFLD, non-alcoholic fatty liver disease; NASH, Non-alcoholic steatohepatitis; HBV, hepatitis B virus; HCV, hepatitis C virus; AATD, α1-antitrypsin deficiency; FH, familial hypercholesterolemia; EVs, extracellular vesicles; HSC, hepatic stellate cell; CSC, cancer stem cells; XCI, X-chromosome inactivation; CNV, copy number variations; SNV, single-nucleotide variations.
References
1. Asrani SK, Devarbhavi H, Eaton J, Kamath PS. Burden of liver diseases in the world. J Hepatol. (2019) 70:151–71. doi: 10.1016/j.jhep.2018.09.014
2. Global Observatory on Donation and Transplantation (2019). Available online at: http://www.transplant-observatory.org/
3. Trotter JF, Cardenas A. Liver transplantation around the world. Liver Transpl. (2016) 22:1059–61. doi: 10.1002/lt.24508
4. Soltys KA, Setoyama K, Tafaleng EN, Soto Gutierrez A, Fong J, Fukumitsu K, et al. Host conditioning and rejection monitoring in hepatocyte transplantation in humans. J Hepatol. (2017) 66:987–1000. doi: 10.1016/j.jhep.2016.12.017
5. Sampaziotis F, Segeritz CP, Vallier L. Potential of human induced pluripotent stem cells in studies of liver disease. Hepatology. (2015) 62:303–11. doi: 10.1002/hep.27651
6. Nakamori D, Takayama K, Nagamoto Y, Mitani S, Sakurai F, Tachibana M, et al. Hepatic maturation of human iPS cell-derived hepatocyte-like cells by ATF5, c/EBPalpha, and PROX1 transduction. Biochem Biophys Res Commun. (2016) 469:424–9. doi: 10.1016/j.bbrc.2015.12.007
7. Schwartz RE, Fleming HE, Khetani SR, Bhatia SN. Pluripotent stem cell-derived hepatocyte-like cells. Biotechnol Adv. (2014) 32:504–13. doi: 10.1016/j.biotechadv.2014.01.003
8. Takahashi K, Yamanaka S. Induction of pluripotent stem cells from mouse embryonic and adult fibroblast cultures by defined factors. Cell. (2006) 126:663–76. doi: 10.1016/j.cell.2006.07.024
9. Takahashi K, Tanabe K, Ohnuki M, Narita M, Ichisaka T, Tomoda K, et al. Induction of pluripotent stem cells from adult human fibroblasts by defined factors. Cell. (2007) 131:861–72. doi: 10.1016/j.cell.2007.11.019
10. Yu J, Vodyanik MA, Smuga-Otto K, Antosiewicz-Bourget J, Frane JL, Tian S, et al. Induced pluripotent stem cell lines derived from human somatic cells. Science. (2007) 318:1917–20. doi: 10.1126/science.1151526
11. Takahashi K, Yamanaka S. A decade of transcription factor-mediated reprogramming to pluripotency. Nat Rev Mol Cell Biol. (2016) 17:183–93. doi: 10.1038/nrm.2016.8
12. Fusaki N, Ban H, Nishiyama A, Saeki K, Hasegawa M. Efficient induction of transgene-free human pluripotent stem cells using a vector based on Sendai virus, an RNA virus that does not integrate into the host genome. Proc Jpn Acad Ser B Phys Biol Sci. (2009) 85:348–62. doi: 10.2183/pjab.85.348
13. Okita K, Matsumura Y, Sato Y, Okada A, Morizane A, Okamoto S, et al. A more efficient method to generate integration-free human iPS cells. Nat Methods. (2011) 8:409–12. doi: 10.1038/nmeth.1591
14. Warren L, Manos PD, Ahfeldt T, Loh YH, Li H, Lau F, et al. Highly efficient reprogramming to pluripotency and directed differentiation of human cells with synthetic modified mRNA. Cell Stem Cell. (2010) 7:618–30. doi: 10.1016/j.stem.2010.08.012
15. Song Z, Cai J, Liu Y, Zhao D, Yong J, Duo S, et al. Efficient generation of hepatocyte-like cells from human induced pluripotent stem cells. Cell Res. (2009) 19:1233–42. doi: 10.1038/cr.2009.107
16. Chen YF, Tseng CY, Wang HW, Kuo HC, Yang VW, Lee OK. Rapid generation of mature hepatocyte-like cells from human induced pluripotent stem cells by an efficient three-step protocol. Hepatology. (2012) 55:1193–203. doi: 10.1002/hep.24790
17. Yanagida A, Ito K, Chikada H, Nakauchi H, Kamiya A. An in vitro expansion system for generation of human iPS cell-derived hepatic progenitor-like cells exhibiting a bipotent differentiation potential. PLoS ONE. (2013) 8:e67541. doi: 10.1371/journal.pone.0067541
18. Hay DC, Fletcher J, Payne C, Terrace JD, Gallagher RC, Snoeys J, et al. Highly efficient differentiation of hESCs to functional hepatic endoderm requires ActivinA and Wnt3a signaling. Proc Natl Acad Sci USA. (2008) 105:12301–6. doi: 10.1073/pnas.0806522105
19. Morrison GM, Oikonomopoulou I, Migueles RP, Soneji S, Livigni A, Enver T, et al. Anterior definitive endoderm from ESCs reveals a role for FGF signaling. Cell Stem Cell. (2008) 3:402–15. doi: 10.1016/j.stem.2008.07.021
20. Zaret KS. Regulatory phases of early liver development: paradigms of organogenesis. Nat Rev Genet. (2002) 3:499–512. doi: 10.1038/nrg837
21. Schmidt C, Bladt F, Goedecke S, Brinkmann V, Zschiesche W, Sharpe M, et al. Scatter factor/hepatocyte growth factor is essential for liver development. Nature. (1995) 373:699–702. doi: 10.1038/373699a0
22. Hansel MC, Davila JC, Vosough M, Gramignoli R, Skvorak KJ, Dorko K, et al. The use of induced pluripotent stem cells for the study and treatment of liver diseases. Curr Protoc Toxicol. (2016) 67:14.13.1–14.13.27. doi: 10.1002/0471140856.tx1413s67
23. Dianat N, Dubois-Pot-Schneider H, Steichen C, Desterke C, Leclerc P, Raveux A, et al. Generation of functional cholangiocyte-like cells from human pluripotent stem cells and HepaRG cells. Hepatology. (2014) 60:700–14. doi: 10.1002/hep.27165
24. Ogawa M, Ogawa S, Bear CE, Ahmadi S, Chin S, Li B, et al. Directed differentiation of cholangiocytes from human pluripotent stem cells. Nat Biotechnol. (2015) 33:853–61. doi: 10.1038/nbt.3294
25. Sampaziotis F, de Brito MC, Madrigal P, Bertero A, Saeb-Parsy K, Soares FAC, et al. Cholangiocytes derived from human induced pluripotent stem cells for disease modeling and drug validation. Nat Biotechnol. (2015) 33:845–52. doi: 10.1038/nbt.3275
26. Yu Y, Liu H, Ikeda Y, Amiot BP, Rinaldo P, Duncan SA, et al. Hepatocyte-like cells differentiated from human induced pluripotent stem cells: relevance to cellular therapies. Stem Cell Res. (2012) 9:196–207. doi: 10.1016/j.scr.2012.06.004
27. Roy-Chowdhury N, Wang X, Guha C, Roy-Chowdhury J. Hepatocyte-like cells derived from induced pluripotent stem cells. Hepatol Int. (2017) 11:54–69. doi: 10.1007/s12072-016-9757-y
28. Lu J, Einhorn S, Venkatarangan L, Miller M, Mann DA, Watkins PB, et al. Morphological and functional characterization and assessment of ipsc-derived hepatocytes for in vitro toxicity testing. Toxicol Sci. (2015) 147:39–54. doi: 10.1093/toxsci/kfv117
29. Larsen LE, Smith MA, Abbey D, Korn A, Reeskamp LF, Hand NJ, et al. Hepatocyte-like cells derived from induced pluripotent stem cells: A versatile tool to understand lipid disorders. Atherosclerosis. (2020) 303:8–14. doi: 10.1016/j.atherosclerosis.2020.03.014
30. Grandy R, Tomaz RA, Vallier L. Modeling disease with human inducible pluripotent stem cells. Annu Rev Pathol. (2019) 14:449–68. doi: 10.1146/annurev-pathol-020117-043634
31. Gao X, Liu Y. A transcriptomic study suggesting human iPSC-derived hepatocytes potentially offer a better in vitro model of hepatotoxicity than most hepatoma cell lines. Cell Biol Toxicol. (2017) 33:407–21. doi: 10.1007/s10565-017-9383-z
32. Chen Y, Li Y, Wang X, Zhang W, Sauer V, Chang CJ, et al. Amelioration of hyperbilirubinemia in gunn rats after transplantation of human induced pluripotent stem cell-derived hepatocytes. Stem Cell Rep. (2015) 5:22–30. doi: 10.1016/j.stemcr.2015.04.017
33. Zabulica M, Srinivasan RC, Vosough M, Hammarstedt C, Wu T, Gramignoli R, et al. Guide to the assessment of mature liver gene expression in stem cell-derived hepatocytes. Stem Cells Dev. (2019) 28:907–19. doi: 10.1089/scd.2019.0064
34. Nakamura T, Torimura T, Sakamoto M, Hashimoto O, Taniguchi E, Inoue K, et al. Significance and therapeutic potential of endothelial progenitor cell transplantation in a cirrhotic liver rat model. Gastroenterology. (2007) 133:91–107. doi: 10.1053/j.gastro.2007.03.110
35. Fang B, Shi M, Liao L, Yang S, Liu Y, Zhao RC. Systemic infusion of FLK1(+) mesenchymal stem cells ameliorate carbon tetrachloride-induced liver fibrosis in mice. Transplantation. (2004) 78:83–8. doi: 10.1097/01.TP.0000128326.95294.14
36. Aziz MTA, Atta HM, Mahfouz S, Fouad HH, Roshdy NK, Ahmed HH, et al. Therapeutic potential of bone marrow-derived mesenchymal stem cells on experimental liver fibrosis. Clin Biochem. (2007) 40:893–9. doi: 10.1016/j.clinbiochem.2007.04.017
37. Sakaida I, Terai S, Yamamoto N, Aoyama K, Ishikawa T, Nishina H, et al. Transplantation of bone marrow cells reduces CCl4-induced liver fibrosis in mice. Hepatology. (2004) 40:1304–11. doi: 10.1002/hep.20452
38. Amer MEM, El-Sayed SZ, El-Kheir WA, Gabr H, Gomaa AA, El-Noomani N, et al. Clinical and laboratory evaluation of patients with end-stage liver cell failure injected with bone marrow-derived hepatocyte-like cells. Eur J Gastroenterol Hepatol. (2011) 23:936–41. doi: 10.1097/MEG.0b013e3283488b00
39. Asgari S, Moslem M, Bagheri-Lankarani K, Pournasr B, Miryounesi M, Baharvand H. Differentiation and transplantation of human induced pluripotent stem cell-derived hepatocyte-like cells. Stem Cell Rev Rep. (2013) 9:493–504. doi: 10.1007/s12015-011-9330-y
40. Takayama K, Akita N, Mimura N, Akahira R, Taniguchi Y, Ikeda M, et al. Generation of safe and therapeutically effective human induced pluripotent stem cell-derived hepatocyte-like cells for regenerative medicine. Hepatol Commun. (2017) 1:1058–69. doi: 10.1002/hep4.1111
41. Isobe K, Cheng Z, Ito S, Nishio N. Aging in the mouse and perspectives of rejuvenation through induced pluripotent stem cells (iPSCs). Results Probl Cell Differ. (2012) 55:413–27. doi: 10.1007/978-3-642-30406-4_21
42. Espejel S, Roll GR, McLaughlin KJ, Lee AY, Zhang JY, Laird DJ, et al. Induced pluripotent stem cell-derived hepatocytes have the functional and proliferative capabilities needed for liver regeneration in mice. J Clin Invest. (2010) 120:3120–6. doi: 10.1172/JCI43267
43. Kuriyan AE, Albini TA, Townsend JH, Rodriguez M, Pandya HK, Leonard RE, et al. Vision loss after intravitreal injection of autologous “Stem Cells” for AMD. N Engl J Med. (2017) 376:1047–53. doi: 10.1056/NEJMoa1609583
44. Evans AA, London WT, Gish RG, Cohen C, Block TM. Chronic HBV infection outside treatment guidelines: is treatment needed? Antivir Ther. (2013) 18:229–35. doi: 10.3851/IMP2325
45. Yang JD, Roberts LR. Hepatocellular carcinoma: a global view. Nat Rev Gastroenterol Hepatol. (2010) 7:448–58. doi: 10.1038/nrgastro.2010.100
46. Yoshida T, Takayama K, Kondoh M, Sakurai F, Tani H, Sakamoto N, et al. Use of human hepatocyte-like cells derived from induced pluripotent stem cells as a model for hepatocytes in hepatitis C virus infection. Biochem Biophys Res Commun. (2011) 416:119–24. doi: 10.1016/j.bbrc.2011.11.007
47. Liu H, Ye Z, Kim Y, Sharkis S, Jang YY. Generation of endoderm-derived human induced pluripotent stem cells from primary hepatocytes. Hepatology. (2010) 51:1810–9. doi: 10.1002/hep.23626
48. Furuyama K, Kawaguchi Y, Akiyama H, Horiguchi M, Kodama S, Kuhara T, et al. Continuous cell supply from a Sox9-expressing progenitor zone in adult liver, exocrine pancreas and intestine. Nat Genet. (2011) 43:34–41. doi: 10.1038/ng.722
49. Si-Tayeb K, Noto FK, Nagaoka M, Li J, Battle MA, Duris C, et al. Highly efficient generation of human hepatocyte-like cells from induced pluripotent stem cells. Hepatology. (2010) 51:297–305. doi: 10.1002/hep.23354
50. Sakurai F, Mitani S, Yamamoto T, Takayama K, Tachibana M, Watashi K, et al. Human induced-pluripotent stem cell-derived hepatocyte-like cells as an in vitro model of human hepatitis B virus infection. Sci Rep. (2017) 7:45698. doi: 10.1038/srep45698
51. Kaserman JE, Wilson AA. Patient-derived induced pluripotent stem cells for alpha-1 antitrypsin deficiency disease modeling and therapeutic discovery. Chronic Obstr Pulm Dis. (2018) 5:258–66. doi: 10.15326/jcopdf.5.4.2017.0179
52. Rader DJ, Cohen J, Hobbs HH. Monogenic hypercholesterolemia: new insights in pathogenesis and treatment. J Clin Invest. (2003) 111:1795–803. doi: 10.1172/JCI200318925
53. Harada-Shiba M, Arai H, Ishigaki Y, Ishibashi S, Okamura T, Ogura M, et al. Guidelines for diagnosis and treatment of familial hypercholesterolemia 2017. J Atheroscler Thromb. (2018) 25:751–70. doi: 10.5551/jat.CR003
54. Rashid ST, Corbineau S, Hannan N, Marciniak SJ, Miranda E, Alexander G, et al. Modeling inherited metabolic disorders of the liver using human induced pluripotent stem cells. J Clin Invest. (2010) 120:3127–36. doi: 10.1172/JCI43122
55. Tafaleng EN, Chakraborty S, Han B, Hale P, Wu W, Soto-Gutierrez A, et al. Induced pluripotent stem cells model personalized variations in liver disease resulting from alpha1-antitrypsin deficiency. Hepatology. (2015) 62:147–57. doi: 10.1002/hep.27753
56. Cayo MA, Cai J, DeLaForest A, Noto FK, Nagaoka M, Clark BS, et al. JD induced pluripotent stem cell-derived hepatocytes faithfully recapitulate the pathophysiology of familial hypercholesterolemia. Hepatology. (2012) 56:2163–71. doi: 10.1002/hep.25871
57. Chun YS, Chaudhari P, Jang YY. Applications of patient-specific induced pluripotent stem cells; focused on disease modeling, drug screening and therapeutic potentials for liver disease. Int J Biol Sci. (2010) 6:796–805. doi: 10.7150/ijbs.6.796
58. Yusa K, Rashid ST, Strick-Marchand H, Varela I, Liu PQ, Paschon DE, et al. Targeted gene correction of alpha1-antitrypsin deficiency in induced pluripotent stem cells. Nature. (2011) 478:391–4. doi: 10.1038/nature10424
59. Choi SM, Kim Y, Shim JS, Park JT, Wang RH, Leach SD, et al. Efficient drug screening and gene correction for treating liver disease using patient-specific stem cells. Hepatology. (2013) 57:2458–68. doi: 10.1002/hep.26237
60. Smith C, Abalde-Atristain L, He C, Brodsky BR, Braunstein EM, Chaudhari P, et al. Efficient and allele-specific genome editing of disease loci in human iPSCs. Mol Ther. (2015) 23:570–7. doi: 10.1038/mt.2014.226
61. Omer L, Hudson EA, Zheng S, Hoying JB, Shan Y, Boyd NL. CRISPR correction of a homozygous low-density lipoprotein receptor mutation in familial hypercholesterolemia induced pluripotent stem cells. Hepatol Commun. (2017) 1:886–98. doi: 10.1002/hep4.1110
62. Starley BQ, Calcagno CJ, Harrison SA. Nonalcoholic fatty liver disease and hepatocellular carcinoma: a weighty connection. Hepatology. (2010) 51:1820–32. doi: 10.1002/hep.23594
63. Younossi ZM, Koenig AB, Abdelatif D, Fazel Y, Henry L, Wymer M. Global epidemiology of nonalcoholic fatty liver disease-meta-analytic assessment of prevalence, incidence, and outcomes. Hepatology. (2016) 64:73–84. doi: 10.1002/hep.28431
64. Wong RJ, Aguilar M, Cheung R, Perumpail RB, Harrison SA, Younossi ZM, et al. Nonalcoholic steatohepatitis is the second leading etiology of liver disease among adults awaiting liver transplantation in the United States. Gastroenterology. (2015) 148:547–55. doi: 10.1053/j.gastro.2014.11.039
65. Fon Tacer K, Rozman D. Nonalcoholic Fatty liver disease: focus on lipoprotein and lipid deregulation. J Lipids. (2011) 2011:783976. doi: 10.1155/2011/783976
66. Grochowski CM, Loomes KM, Spinner NB. Jagged1 (JAG1): structure, expression, and disease associations. Gene. (2016) 576:381–4. doi: 10.1016/j.gene.2015.10.065
67. Guegan K, Stals K, Day M, Turnpenny P, Ellard S. JAG1 mutations are found in approximately one third of patients presenting with only one or two clinical features of Alagille syndrome. Clin Genet. (2012) 82:33–40. doi: 10.1111/j.1399-0004.2011.01749.x
68. Tiniakos DG, Vos MB, Brunt EM. Nonalcoholic fatty liver disease: pathology and pathogenesis. Annu Rev Pathol. (2010) 5:145–71. doi: 10.1146/annurev-pathol-121808-102132
69. Pu K, Wang Y, Bai S, Wei H, Zhou Y, Fan J, et al. Diagnostic accuracy of controlled attenuation parameter (CAP) as a non-invasive test for steatosis in suspected non-alcoholic fatty liver disease: a systematic review and meta-analysis. BMC Gastroenterol. (2019) 19:51. doi: 10.1186/s12876-019-0961-9
70. De Gottardi A, Vinciguerra M, Sgroi A, Moukil M, Ravier-Dall'Antonia F, Pazienza V, et al. Microarray analyses and molecular profiling of steatosis induction in immortalized human hepatocytes. Lab Invest. (2007) 87:792–806. doi: 10.1038/labinvest.3700590
71. Ricchi M, Odoardi MR, Carulli L, Anzivino C, Ballestri S, Pinetti A, et al. Differential effect of oleic and palmitic acid on lipid accumulation and apoptosis in cultured hepatocytes. J Gastroenterol Hepatol. (2009) 24:830–40. doi: 10.1111/j.1440-1746.2008.05733.x
72. Graffmann N, Ring S, Kawala MA, Wruck W, Ncube A, Trompeter HI, et al. Modeling nonalcoholic fatty liver disease with human pluripotent stem cell-derived immature hepatocyte-like cells reveals activation of PLIN2 and confirms regulatory functions of peroxisome proliferator-activated receptor alpha. Stem Cells Dev. (2016) 25:1119–33. doi: 10.1089/scd.2015.0383
73. Graffmann N, Bohndorf M, Ncube A, Kawala MA, Wruck W, Kashofer K, et al. Establishment and characterization of an iPSC line from a 35years old high grade patient with nonalcoholic fatty liver disease (30-40% steatosis) with homozygous wildtype PNPLA3 genotype. Stem Cell Res. (2018) 31:113–6. doi: 10.1016/j.scr.2018.07.015
74. Graffmann N, Bohndorf M, Ncube A, Wruck W, Kashofer K, Zatloukal K, et al. Establishment and characterization of an iPSC line from a 58years old high grade patient with nonalcoholic fatty liver disease (70% steatosis) with homozygous wildtype PNPLA3 genotype. Stem Cell Res. (2018) 31:131–4. doi: 10.1016/j.scr.2018.07.011
75. Parafati M, Kirby RJ, Khorasanizadeh S, Rastinejad F, Malany S. A nonalcoholic fatty liver disease model in human induced pluripotent stem cell-derived hepatocytes, created by endoplasmic reticulum stress-induced steatosis. Dis Model Mech. (2018) 11:33530. doi: 10.1242/dmm.033530
76. Romanelli RG, Stasi C. Recent advancements in diagnosis and therapy of liver cirrhosis. Curr Drug Targets. (2016) 17:1804–17. doi: 10.2174/1389450117666160613101413
77. Murray KF, Carithers RL Jr., Aasld. AASLD practice guidelines: evaluation of the patient for liver transplantation. Hepatology. (2005) 41:1407–32. doi: 10.1002/hep.20704
78. Van de Bovenkamp M, Groothuis GM, Meijer DK, Olinga P. Liver fibrosis in vitro: cell culture models and precision-cut liver slices. Toxicol In Vitro. (2007) 21:545–57. doi: 10.1016/j.tiv.2006.12.009
79. Guyot C, Lepreux S, Combe C, Doudnikoff E, Bioulac-Sage P, Balabaud C, et al. Hepatic fibrosis and cirrhosis: the (myo)fibroblastic cell subpopulations involved. Int J Biochem Cell Biol. (2006) 38:135–51. doi: 10.1016/j.biocel.2005.08.021
80. Bhatia SN, Balis UJ, Yarmush ML, Toner M. Effect of cell-cell interactions in preservation of cellular phenotype: cocultivation of hepatocytes and nonparenchymal cells. FASEB J. (1999) 13:1883–900. doi: 10.1096/fasebj.13.14.1883
81. Takebe T, Sekine K, Enomura M, Koike H, Kimura M, Ogaeri T, et al. Vascularized and functional human liver from an iPSC-derived organ bud transplant. Nature. (2013) 499:481–4. doi: 10.1038/nature12271
82. Chen Z, Qi LZ, Zeng R, Li HY, Dai LJ. Stem cells and hepatic cirrhosis. Panminerva Med. (2010) 52:149–65.
83. Povero D, Pinatel EM, Leszczynska A, Goyal NP, Nishio T, Kim J, et al. Human induced pluripotent stem cell-derived extracellular vesicles reduce hepatic stellate cell activation and liver fibrosis. JCI Insight. (2019) 5:e125652. doi: 10.1172/jci.insight.125652
84. Park S, In Hwang S, Kim J, Hwang S, Kang S, Yang S, et al. The therapeutic potential of induced hepatocyte-like cells generated by direct reprogramming on hepatic fibrosis. Stem Cell Res Ther. (2019) 10:21. doi: 10.1186/s13287-018-1127-3
85. Choi SM, Kim Y, Liu H, Chaudhari P, Ye Z, Jang YY. Liver engraftment potential of hepatic cells derived from patient-specific induced pluripotent stem cells. Cell Cycle. (2011) 10:2423–7. doi: 10.4161/cc.10.15.16869
86. Ferlay J, Shin HR, Bray F, Forman D, Mathers C, Parkin DM. Estimates of worldwide burden of cancer in 2008: GLOBOCAN (2008). Int J Cancer. (2010) 127:2893–917. doi: 10.1002/ijc.25516
87. Forner A, Bruix J. Biomarkers for early diagnosis of hepatocellular carcinoma. Lancet Oncol. (2012) 13:750–1. doi: 10.1016/S1470-2045(12)70271-1
88. Sun C, Liu YK. Induced pluripotent cancer cells: progress and application. J Cancer Res Clin Oncol. (2011) 137:1–8. doi: 10.1007/s00432-010-0955-z
89. Zheng YW, Nie YZ, Taniguchi H. Cellular reprogramming and hepatocellular carcinoma development. World J Gastroenterol. (2013) 19:8850–60. doi: 10.3748/wjg.v19.i47.8850
90. Moriguchi H, Chung RT, Sato C. An identification of novel therapy for human hepatocellular carcinoma by using human induced pluripotent stem cells. Hepatology. (2010) 51:1090–1. doi: 10.1002/hep.23418
91. Sakisaka M, Haruta M, Komohara Y, Umemoto S, Matsumura K, Ikeda T, et al. Therapy of primary and metastatic liver cancer by human iPS cell-derived myeloid cells producing interferon-beta. J Hepatobiliary Pancreat Sci. (2017) 24:109–19. doi: 10.1002/jhbp.422
92. Sampaziotis F, de Brito MC, Geti I, Bertero A, Hannan NR, Vallier L. Directed differentiation of human induced pluripotent stem cells into functional cholangiocyte-like cells. Nat Protoc. (2017) 12:814–27. doi: 10.1038/nprot.2017.011
93. Sayed N, Liu C, Wu JC. Translation of human-induced pluripotent stem cells: from clinical trial in a dish to precision medicine. J Am Coll Cardiol. (2016) 67:2161–76. doi: 10.1016/j.jacc.2016.01.083
94. Shi Y, Inoue H, Wu JC, Yamanaka S. Induced pluripotent stem cell technology: a decade of progress. Nat Rev Drug Discovery. (2017) 16:115–30. doi: 10.1038/nrd.2016.245
95. Cayo MA, Mallanna SK, Di Furio F, Jing R, Tolliver LB, Bures M, et al. A drug screen using human iPSC-derived hepatocyte-like cells reveals cardiac glycosides as a potential treatment for hypercholesterolemia. Cell Stem Cell. (2017) 20:478–89. doi: 10.1016/j.stem.2017.01.011
96. Jing R, Corbett JL, Cai J, Beeson GC, Beeson CC, Chan SS, et al. A screen using iPSC-derived hepatocytes reveals NAD(+) as a potential treatment for mtDNA depletion syndrome. Cell Rep. (2018) 25:1469–84. doi: 10.1016/j.celrep.2018.10.036
97. Broutier L, Mastrogiovanni G, Verstegen MM, Francies HE, Gavarró LM, Bradshaw CR, et al. Human primary liver cancer-derived organoid cultures for disease modeling and drug screening. Nat Med. (2017) 23:1424–35. doi: 10.1038/nm.4438
98. Artegiani B, Clevers H. Use and application of 3D-organoid technology. Human Mol Genet. (2018) 27:R99–107. doi: 10.1093/hmg/ddy187
99. Wang Y, Wang H, Deng P, Chen W, Guo Y, Tao T, et al. In situ differentiation and generation of functional liver organoids from human iPSCs in a 3D perfusable chip system. Lab Chip. (2018) 18:3606–16. doi: 10.1039/C8LC00869H
100. Draper JS, Smith K, Gokhale P, Moore HD, Maltby E, Johnson J, et al. Recurrent gain of chromosomes 17q and 12 in cultured human embryonic stem cells. Nat Biotechnol. (2004) 22:53–4. doi: 10.1038/nbt922
101. Hannoun Z, Steichen C, Dianat N, Weber A, Dubart-Kupperschmitt A. The potential of induced pluripotent stem cell derived hepatocytes. J Hepatol. (2016) 65:182–99. doi: 10.1016/j.jhep.2016.02.025
102. Panopoulos AD, Ruiz S, Izpisua Belmonte JC. iPSCs: induced back to controversy. Cell Stem Cell. (2011) 8:347–8. doi: 10.1016/j.stem.2011.03.003
103. Liang G, Zhang Y. Genetic and epigenetic variations in iPSCs: potential causes and implications for application. Cell Stem Cell. (2013) 13:149–59. doi: 10.1016/j.stem.2013.07.001
104. Anguera MC, Sadreyev R, Zhang Z, Szanto A, Payer B, Sheridan SD, et al. Molecular signatures of human induced pluripotent stem cells highlight sex differences and cancer genes. Cell Stem Cell. (2012) 11:75–90. doi: 10.1016/j.stem.2012.03.008
105. Polo JM, Liu S, Figueroa ME, Kulalert W, Eminli S, Tan KY, et al. Cell type of origin influences the molecular and functional properties of mouse induced pluripotent stem cells. Nat Biotechnol. (2010) 28:848–55. doi: 10.1038/nbt.1667
106. Kim K, Zhao R, Doi A, Ng K, Unternaehrer J, Cahan P, et al. Donor cell type can influence the epigenome and differentiation potential of human induced pluripotent stem cells. Nat Biotechnol. (2011) 29:1117–9. doi: 10.1038/nbt.2052
107. Kim K, Doi A, Wen B, Ng K, Zhao R, Cahan P, et al. Epigenetic memory in induced pluripotent stem cells. Nature. (2010) 467:285–90. doi: 10.1038/nature09342
108. Bar-Nur O, Russ HA, Efrat S, Benvenisty N. Epigenetic memory and preferential lineage-specific differentiation in induced pluripotent stem cells derived from human pancreatic islet beta cells. Cell Stem Cell. (2011) 9:17–23. doi: 10.1016/j.stem.2011.06.007
109. Pick M, Stelzer Y, Bar-Nur O, Mayshar Y, Eden A, Benvenisty N. Clone- and gene-specific aberrations of parental imprinting in human induced pluripotent stem cells. Stem Cells. (2009) 27:2686–90. doi: 10.1002/stem.205
110. Nishino K, Toyoda M, Yamazaki-Inoue M, Fukawatase Y, Chikazawa E, Sakaguchi H, et al. DNA methylation dynamics in human induced pluripotent stem cells over time. PLoS Genet. (2011) 7:e1002085. doi: 10.1371/journal.pgen.1002085
111. Nazor KL, Altun G, Lynch C, Tran H, Harness JV, Slavin I, et al. Recurrent variations in DNA methylation in human pluripotent stem cells and their differentiated derivatives. Cell Stem Cell. (2012) 10:620–34. doi: 10.1016/j.stem.2012.02.013
112. Chamberlain SJ, Chen PF, Ng KY, Bourgois-Rocha F, Lemtiri-Chlieh F, Levine ES, et al. Induced pluripotent stem cell models of the genomic imprinting disorders Angelman and Prader-Willi syndromes. Proc Natl Acad Sci USA. (2010) 107:17668–73. doi: 10.1073/pnas.1004487107
113. Stadtfeld M, Apostolou E, Akutsu H, Fukuda A, Follett P, Natesan S, et al. Aberrant silencing of imprinted genes on chromosome 12qF1 in mouse induced pluripotent stem cells. Nature. (2010) 465:175–81. doi: 10.1038/nature09017
114. Liu L, Luo GZ, Yang W, Zhao X, Zheng Q, Lv Z, et al. Activation of the imprinted Dlk1-Dio3 region correlates with pluripotency levels of mouse stem cells. J Biol Chem. (2010) 285:19483–90. doi: 10.1074/jbc.M110.131995
115. Lister R, Pelizzola M, Kida YS, Hawkins RD, Nery JR, Hon G, et al. Hotspots of aberrant epigenomic reprogramming in human induced pluripotent stem cells. Nature. (2011) 471:68–73. doi: 10.1038/nature09798
116. Hussein SM, Batada NN, Vuoristo S, Ching RW, Autio R, Narva E, et al. Copy number variation and selection during reprogramming to pluripotency. Nature. (2011) 471:58–62. doi: 10.1038/nature09871
117. Young MA, Larson DE, Sun CW, George DR, Ding L, Miller CA, et al. Background mutations in parental cells account for most of the genetic heterogeneity of induced pluripotent stem cells. Cell Stem Cell. (2012) 10:570–82. doi: 10.1016/j.stem.2012.03.002
118. Quinlan AR, Boland MJ, Leibowitz ML, Shumilina S, Pehrson SM, Baldwin KK, et al. Genome sequencing of mouse induced pluripotent stem cells reveals retroelement stability and infrequent DNA rearrangement during reprogramming. Cell Stem Cell. (2011) 9:366–73. doi: 10.1016/j.stem.2011.07.018
119. Gore A, Li Z, Fung HL, Young JE, Agarwal S, Antosiewicz-Bourget J, et al. Somatic coding mutations in human induced pluripotent stem cells. Nature. (2011) 471:63–7. doi: 10.1038/nature09805
120. Cheng L, Hansen NF, Zhao L, Du Y, Zou C, Donovan FX, et al. Low incidence of DNA sequence variation in human induced pluripotent stem cells generated by nonintegrating plasmid expression. Cell Stem Cell. (2012) 10:337–44. doi: 10.1016/j.stem.2012.01.005
121. Abyzov A, Mariani J, Palejev D, Zhang Y, Haney MS, Tomasini L, et al. Somatic copy number mosaicism in human skin revealed by induced pluripotent stem cells. Nature. (2012) 492:438–42. doi: 10.1038/nature11629
122. Ruiz S, Gore A, Li Z, Panopoulos AD, Montserrat N, Fung HL, et al. Analysis of protein-coding mutations in hiPSCs and their possible role during somatic cell reprogramming. Nat Commun. (2013) 4:1382. doi: 10.1038/ncomms2381
123. Ji J, Ng SH, Sharma V, Neculai D, Hussein S, Sam M, et al. Elevated coding mutation rate during the reprogramming of human somatic cells into induced pluripotent stem cells. Stem Cells. (2012) 30:435–40. doi: 10.1002/stem.1011
124. Araki R, Uda M, Hoki Y, Sunayama M, Nakamura M, Ando S, et al. Negligible immunogenicity of terminally differentiated cells derived from induced pluripotent or embryonic stem cells. Nature. (2013) 494:100–4. doi: 10.1038/nature11807
125. Zhao T, Zhang ZN, Rong Z, Xu Y. Immunogenicity of induced pluripotent stem cells. Nature. (2011) 474:212–5. doi: 10.1038/nature10135
126. Tan Y, Ooi S, Wang L. Immunogenicity and tumorigenicity of pluripotent stem cells and their derivatives: genetic and epigenetic perspectives. Curr Stem Cell Res Ther. (2014) 9:63–72. doi: 10.2174/1574888X113086660068
127. Säljö K, Barone A, Mölne J, Rydberg L, Teneberg S, Breimer ME. HLA and histo-blood group antigen expression in human pluripotent stem cells and their derivatives. Sci Rep. (2017) 7:13072. doi: 10.1038/s41598-017-12231-8
128. Zhao T, Zhang ZN, Westenskow PD, Todorova D, Hu Z, Lin T, et al. Humanized mice reveal differential immunogenicity of cells derived from autologous induced pluripotent stem cells. Cell Stem Cell. (2015) 17:353–9. doi: 10.1016/j.stem.2015.07.021
129. Chen HF, Yu CY, Chen MJ, Chou SH, Chiang MS, Chou WH, et al. Characteristic expression of major histocompatibility complex and immune privilege genes in human pluripotent stem cells and their derivatives. Cell Transplant. (2015) 24:845–64. doi: 10.3727/096368913X674639
Keywords: HLCs, primary human hepatocytes, liver disease, clinical application, IPS cell
Citation: Zhang L, Pu K, Liu X, Bae SDW, Nguyen R, Bai S, Li Y and Qiao L (2021) The Application of Induced Pluripotent Stem Cells Against Liver Diseases: An Update and a Review. Front. Med. 8:644594. doi: 10.3389/fmed.2021.644594
Received: 21 December 2020; Accepted: 04 June 2021;
Published: 01 July 2021.
Edited by:
Heather Francis, Indiana University, United StatesReviewed by:
Yuyan Han, University of Northern Colorado, United StatesRoberto Gramignoli, Karolinska Institutet (KI), Sweden
Copyright © 2021 Zhang, Pu, Liu, Bae, Nguyen, Bai, Li and Qiao. This is an open-access article distributed under the terms of the Creative Commons Attribution License (CC BY). The use, distribution or reproduction in other forums is permitted, provided the original author(s) and the copyright owner(s) are credited and that the original publication in this journal is cited, in accordance with accepted academic practice. No use, distribution or reproduction is permitted which does not comply with these terms.
*Correspondence: Liang Qiao, bGlhbmcucWlhb0BzeWRuZXkuZWR1LmF1
†These authors have contributed equally to this work