- 1Mildred Stahlman Division of Neonatology, Department of Pediatrics, Vanderbilt University, Nashville, TN, United States
- 2Institute for Lung Biology and Disease and Comprehensive Pneumology Center With the CPC-M bioArchive, Helmholtz Center Munich, Member of the German Center for Lung Research (DZL), Munich, Germany
- 3Center for Comprehensive Developmental Care (CDeCLMU), University Hospital Ludwig-Maximilian University, Munich, Germany
- 4Division of Respiratory Medicine, NIHR Nottingham Biomedical Research Centre, School of Medicine, University of Nottingham, City Hospital NUH Campus, Nottingham, United Kingdom
Infants suffering from neonatal chronic lung disease, i.e., bronchopulmonary dysplasia, are facing long-term consequences determined by individual genetic background, presence of infections, and postnatal treatment strategies such as mechanical ventilation and oxygen toxicity. The adverse effects provoked by these measures include inflammatory processes, oxidative stress, altered growth factor signaling, and remodeling of the extracellular matrix. Both, acute and long-term consequences are determined by the capacity of the immature lung to respond to the challenges outlined above. The subsequent impairment of lung growth translates into an altered trajectory of lung function later in life. Here, knowledge about second and third hit events provoked through environmental insults are of specific importance when advocating lifestyle recommendations to this patient population. A profound exchange between the different health care professionals involved is urgently needed and needs to consider disease origin while future monitoring and treatment strategies are developed.
Disease Characteristics and Predispositions
As the most common chronic lung disease in infants, Bronchopulmonary Dysplasia (BPD) is associated with long-term sequelae that persist into adulthood (1, 2). Despite significant improvements in perinatal care, i.e., surfactant and antenatal corticosteroid treatment together with improved ventilation strategies, the incidence of BPD has remained unchanged or even increased amongst the most immature infants (3). This is presumably due to a significant reduction of mortality rates together with an increase in the overall number of treated infants born significantly premature. The varying incidence of BPD between newborn care centers closely reflects differences in patient population and infant management practices (4–7). Recent publications report an incidence of BPD of up to 68% in very low birth weight infants (401–1,500 g) born prior to 29 weeks of gestation, or up to 77% in infants born at <32 weeks of gestation with a birth weight below 1 kg (5, 8, 9), with numbers predominantly derived from high-income countries.
The disease is classified into three different severity grades (mild, moderate, severe) according to the need for supplemental oxygen and/or ventilator support for >28 days of life, or beyond 36 weeks postmenstrual age (PMA) (2). Environmental insults associated with preterm birth sum up to sustained inflammation and extensive matrix remodeling resulting in substantial changes to the scaffold provided for the developing organ in concert with functional abnormalities as a consequence of diffuse fibrotic changes and increased smooth muscle hypertrophy in small pulmonary arteries and airways (10). The characteristic histopathologic changes of impaired alveolarization and vasculogenesis (2) are clinically mirrored by signs of impaired respiratory gas exchange, i.e., alveolar hypoventilation with resultant hypercapnia and hypoxemia leading to a mismatch of ventilation and perfusion (11).
Large clinical trials have identified numerous risk factors for the development of BPD, including congenital and nosocomial infections, mechanical ventilation, and oxygen toxicity (12–17). Poor nutritional support, vitamin deficiency as well as insufficient adrenal and thyroid hormone release in the very premature infant further increase the risk after birth (18–20). Prenatal risk factors influence the capacity of the developing lung to respond to these injuries. Preeclampsia is known as an independent risk factor not only for preterm delivery, but more importantly also for BPD, despite its underlying molecular mechanisms remaining elusive (21, 22). Intrauterine growth retardation increases the risk of BPD 3- to 4-fold (23–27), most likely through their impact on altered growth factor signaling and subsequently impaired alveolar and vascular development (28). Exposure to prenatal smoke, although largely underestimated, has been shown to significantly contribute to disease development, potentially beyond growth restriction (29, 30), and even the prenatal application of established therapeutic measures has to be critically reviewed. Here, antenatal betamethasone, despite its broad prenatal application to enhance lung maturation and to prevent respiratory distress while reducing BPD rates (31, 32), has been shown to increase indicators of lipid membrane peroxidation (33). This word of caution is in line with observations on behalf of postnatal dexamethasone treatment, where adverse effects on cardiac function, life expectancy, and neurologic development have been observed (34, 35). The broad use of antenatal maternal antibiotic treatment on the other hand not only significantly affects the bacterial flora of the child (36) but leads to sustained alterations of immune functions, e.g., in the response of invariant natural killer T cells, as indicated by studies in mice (37). Although prenatal exposure to smoke and antibiotics were shown to provoke lung changes on the molecular level as shown by studies in mice, further investigations are needed in order to establish these risk factors clinically, as prior published data from preterm infants are inconsistent (38, 39).
With regard to the genetic background impacting on the clinical course, prior work demonstrates that gene polymorphisms account for 53% of the variance in BPD (40). Identified genetic abnormalities include mutations in genes associated with surfactant biogenesis, innate immune response (41, 42), and superoxide dismutase (43), with details of the possible pathophysiology explained in the paragraphs below. The higher risk for the development of BPD and pulmonary arterial hypertension (PAH) in male preterm infants (44) has been associated with differences in hormonal regulation (45), although longer term, females with a history of BPD seem to be more severely affected (46).
From Cause to Consequence: Biological Properties, Pulmonary Structure, and Lung Function
Inflammation and Oxidative Stress Response
The sustained inflammatory processes that characterize BPD are caused by pre- as well as postnatal mechanisms with key players highlighted in the paragraph above. Both, infections as well as the corresponding immature immune response play an important role in the initiation and perpetuation of inflammatory processes characterizing and driving BPD development (14, 47–49). Perinatal processes, e.g., the fetal inflammatory response syndrome (FIRS), chorioamnionitis, or their development into congenital and nosocomial infections results in neutrophil influx into the immature lung, with an increased number of monocytes and macrophages during the so called “second wave” of inflammation (27, 50, 51). Here, specific pathogens such as Gram-negative bacteria play an important role, clearly increasing the risk for neonatal chronic lung disease (52). Later nosocomial infections are caused by a different, “non-maternal” spectrum of pathogens (e.g., Staphylococcus epidermidis, Escherichia coli) that are likewise associated with BPD development (53, 54).
Postnatally, non-infectious causes such as baro- and volutrauma during mechanical ventilation in concert with the effects caused by moderate or severe hyperoxia further contribute to or even initiate the inflammatory processes locally and on a systemic level (55–58). Ongoing studies addressing the debate, whether recruitment of inflammatory cells to the injured lung or activation of resident cells are primarily responsible to start and drive the vicious circle of inflammation (59–62) will give important insight for mechanistic understanding and development of targeted therapies. At the same time, extracellular matrix (ECM) remodeling itself further promotes lung inflammation through the release of proteases and inflammatory mediators such as transforming growth factor beta (63–65). The regulation of NF-kB signaling in inflammatory processes (66) and its lately discovered role in alveolo- and vasculogenesis that may even bear therapeutic potential (67, 68) demonstrate the close relation of lung inflammation during organ development to fundamental and long-lasting structural changes.
The degree of lung inflammation and ECM remodeling as well as early alveolar epithelial dysfunction is directly related to the cellular capacity to respond to postnatal environmental challenges. The relative deficiency of antioxidants and inhibitors of proteolytic enzymes render the immature lung especially vulnerable to the effects of inflammatory mediators and toxic oxygen metabolites (69–72). Different markers have been investigated to indicate enhanced oxidative stress in the preterm infant. Elevated urinary malondialdehyde concentrations in the first week of life, generated by peroxidation of lipid membranes after oxidant-mediated injury, were correlated with the risk for oxygen radical diseases including BPD (33). In a murine model, reduced superoxide dismutase 3 (SOD3) in reaction to postnatal hyperoxia was associated with alveolar injury, whereas overexpression of SOD3 attenuated hyperoxic injury in an alveolar epithelial cell line (73). Decreased pulmonary antioxidant concentrations have also been measured in the lavage of preterm infants (74). In line with this, intratracheal application of recombinant human CuZn superoxide dismutase at birth improved pulmonary outcome in high-risk premature infants at 1 year corrected age (75). Studies indicated that adolescent BPD patients have evidence of heightened oxidative stress in the airway, suggesting that long-term respiratory abnormalities after preterm birth align with sustained alterations of the oxidative stress response (76). These effects might even translate into altered responses to viral infections in later life (77).
The increased susceptibility of the developing organ to environmental challenges and the induction of long-term consequences are supported by the observation that significant maturational differences exist between neonatal and adult lung cells in response to lung injury. While chronic oxygen exposure (60% for 14 days) enhances lung vascular and airway smooth muscle contraction and reduces nitric-oxide relaxation in the neonatal rat lung, the opposite occurs in adults (78). In line with the observations obtained in neonatal mice, long-term effects of hyperoxia exposure in the first week of life (100% for 4 days) include increased mortality associated with pulmonary vascular disease and the development of right ventricular strain and PAH in mice (79). The alteration of bone morphogenic protein (BMP) signaling likely contributes to this adult lung phenotype. Other mechanisms explaining the increased susceptibility to injury and the subsequent likelihood of long-term effects observed in the newborn lung are suggested from studies by Balasubramaniam et al. showing that hyperoxia reduces bone marrow derived, circulating, and lung endothelial progenitor cells in the developing organ in contrast to adult mice (80), indicating early exhaustion of repair and regeneration capacities. The risk for long-term effects is furthermore mitigated by the affection of central processes such as cell cycle regulation, i.e., upregulation of P21 by hyperoxia exposure together with decreased histone deacetylase activity (81). The studied effects of excessive oxygen exposure on DNA methylation further contribute to the picture of accumulating damage in the face of reduced compensatory mechanisms in the immature lung (82).
The outlined injury effects ultimately result in the impairment of lung growth based on significantly imbalanced growth factor signaling. Orchestrating the interaction of the epithelial, mesenchymal, and endothelial cell compartment during the fine-tuned development of the gas exchange area, Notch and Wingless Int-1 (Wnt), the fibroblast and platelet derived growth factor (FGF, PDGF) as well as the BMP and the vascular endothelial growth factor (VEGF) play a critical role (83–89).
Morphogenetic Changes to the Pulmonary Cellular and Extracellular Matrix
The release of cytokines such as the transforming growth factor (TGF) –β, tumor necrosis factor (TNF) alpha and interleukins, e.g., IL-1beta in response to lung inflammation and subsequent events such as ECM remodeling and cellular injury significantly contributes to the imbalance in growth factor signaling and leads to the activation of different transcription factors enhancing apoptosis in numerous cell types (90–92). The interference with these transcription factors disrupts normal lung morphogenesis (67) and drives the onset of chronic bronchial inflammation and subsequent pulmonary emphysema in the adult organ (93). In the developing organ, the altered regulation of transcription leads to long-term effects such as the impairment in alveolar and vascular development resulting from nuclear factor kappa B (NF-kB) suppression (66, 68, 94), unequivocally linking key processes in BPD development such as infection and inflammation with altered growth factor signaling and transcriptional regulation. The characteristic co-existence of defective alveolar and capillary formation is determined by impaired angiogenic growth factor signaling (67) and ultimately results in sustained vascular disease, in many cases presenting as PAH and/or impaired lung lymphatic drainage (95, 96). The typical reduction in pulmonary expression levels of VEGF and its receptors (97–99), accompanied by diminished endothelial nitric oxide synthase (eNOS) and soluble guanylate cyclase (sGC) in lung blood vessels and airways (100, 101) reflects the expression pattern observed in aged mice (102) and likely contributes to the reduced plasticity of lung capillaries (103). Treatment with recombinant human VEGF during or after hyperoxia exposure improved not only vessel growth but also alveolarization in the lungs of newborn rats (104).
Both direct effects of shear stress, oxygen toxicity, inflammation, and hormonal regulation as well as subsequent impairment of growth factor signaling alters critical events in endoderm to mesoderm transition and myofibroblast proliferation and leads to severe alterations of the pulmonary scaffold (105, 106). Increased matrix remodeling characterized by the greater abundance and abnormal distribution of elastin together with the deformation of collagen scaffolding has been demonstrated in humans and animal models (107–109). The degradation of lung elastin is indicated by e.g., increased urinary excretion of desmosine, preceded, and paralleled by increased elastase activity (110–112). Desmosine, a breakdown product of the mature elastic fiber was found to predict disease severity and outcome in adult patients with acute respiratory distress syndrome (ARDS) (113), indicating the importance of the delicate balance of proteases and their inhibitors. Complicating the definition of friend or foe in the developing organ, the presence of elastases including metalloproteinase activity is crucial as evidenced by studies showing that complete matrix-metalloproteinase deficiency promotes lung remodeling resembling BPD (114).
The significant changes to the structural integrity of the ECM not only affect its function as a scaffold for the formation of alveoli and capillaries but as well-reveal a significant memory function through defining the fate of cells populating the developing organ (115, 116). The sustained and irreversible reorganization will furthermore result in long term effects with regard to the lung's repair and regeneration capacity, its potential for immune cell interaction, thereby determining its coping with environmental challenges, exacerbation episodes and physiologic aging (117, 118).
Short and Long-Term Pulmonary Function in Preterm Infants With BPD
With increasing survival of infants with BPD, attempts to minimize long-term pulmonary impairment (and associated neurologic complications) has become the main focus of perinatal care (119, 120). Nonetheless, respiratory symptom presentation and suboptimal lung function are manifesting in adult life, and where detected, can be misclassified as more common respiratory diagnoses such as asthma or COPD, particularly if the early life events are not known or asked about (121, 122). In many cases, respiratory disease is not detected until acute presentation or much later in life. As respiratory function serves as a good predictor of later morbidity and mortality (123), knowledge about early changes seems crucial.
After birth, early pulmonary dysfunction is characterized by diminished lung compliance, tachypnea, and increased minute ventilation resulting in increased work of breathing with or without subsequent oxygen dependency. This clinical picture can be accompanied by an increase in lung microvascular filtration pressure that may lead to interstitial pulmonary edema as shown in animal experiments (124). The increased lung vascular resistance, typically associated with impaired responsiveness to inhaled nitric oxide and other vasodilators, can progress to reversible or sustained PAH and right heart failure (95, 96). Early measurement of lung function provides prognostic information and has shown that postnatal development of severe lung disease more likely develops into chronic disease at term (125). At this time point BPD infants present with increased respiratory tract resistance and hyper-reactive airways (126), subsequently leading to frequent episodes of bronchoconstriction and cyanosis after discharge often resulting in hospital readmission. The proportion of underlying vascular disease playing a role in these clinical manifestations beyond the effects caused by the Euler-Liljestrand mechanism (hypoxic pulmonary vasoconstriction) often remains unclear as sensitive diagnostic tools are missing (127).
In the later course following discharge, infants with BPD may remain oxygen dependent for months or years, although only a minority remains oxygen dependent beyond 2 years of age (128, 129). Oxygen dependency indicates the most severe lung disease, as these infants require hospital readmission twice as often in comparison to infants without home oxygen therapy. However, even after having outgrown oxygen dependency, patients with moderate or severe BPD still require outpatient clinic visits, readmissions, and medication in up to 70% of the cases and 30% need three readmissions in the first 2 years of life (130). A major predictor for readmission due to respiratory causes or the need for subsequent mechanical ventilation is the pCO2 at discharge (131). After the second year of life, hospitalization rates decline (132). Related to prematurity beyond BPD status, lower respiratory tract infections resulting from respiratory syncytial virus remain the major cause for readmission amongst preterm infants (133).
In the later course of disease, BPD is a significant risk factor for persistent wheeze and the need for inhalation therapy (odds ratio 2.7 and 2.4, respectively) affecting about 20–30% of infants with BPD at 6 and 12 months of age (134, 135). Respiratory symptoms remain common at preschool and school age (128, 136). Up to 80% of preterm infants, particularly those who presented with wheezing, demonstrate airway obstruction in early childhood and adolescence, the majority of whom are symptomatic (137–139). Important data on long-term pulmonary function in BPD patients were generated by the EPICure study (140), showing significantly lower peak oxygen consumption, forced expiratory volume at 1 second (FEV1) and gas transfer for those born extremely premature at school age when compared to age matched controls, not considering BPD status. Mean difference in FEV1 sum up to a total of 600 ml when comparing infants with extreme prematurity at birth and the respective healthy controls. Significantly lower peak workload and higher respiratory rates in combination with lower tidal volumes during peak exercise and increased residual capacity in these infants may reflect the effect of hyperinflation due to airway obstruction and/or altered pulmonary chemoreceptor function, and suggest the presence of persistent airflow limitations and reductions in alveolar surface area.
In most severe cases symptoms either persist into adulthood (141) or show transient “improvement” reflected by the absence of symptoms, later resulting in the “reappearance” of disease as a consequence of lung function decline below a clinical (or individual) threshold when the disease associated reduction in lung volume and function is met by aging processes or a newly occurring mismatch of the lung-body mass ratio and/or energy expenditure. Altered lung volumes and decreased gas mixing efficiency in BPD has been confirmed by various studies, reflecting abnormalities in lung growth (142, 143), resulting in suboptimal airway function (judging from impaired FEV1 and FEV1/forced vital capacity) in young adults (144, 145), also manifesting in suboptimal exercise capacity (145). While a diverging path in lung growth during adolescence according to spirometric measures was reported by one group (144), the EPICure study revealed no catch-up of the suboptimal lung growth from 11 to 19 years in adolescents following extreme prematurity irrespective of BPD status and even showed significant impairment in all lung function parameters in 19 year old patients born extremely premature (145). Meanwhile, Vollsaeter et al. reported parallel trajectories of lung function in early adult life (146), reflecting the overall need for more robust and contemporaneous studies.
Taken together, the functional data available suggest that early lung injury in preterm infants leads to abnormalities in lung function (and immunity) in infancy and early childhood with significant pulmonary problems persisting in the most severely affected infants. The predisposition for lung function decline in adult survivors of preterm birth is suggested by data obtained in early and later adulthood with lung function measurements in infancy being an important predictor for later lung disease and the risk for (early) lung function decline. If performed during infancy and childhood, pulmonary function tests have the potential to identify individuals at risk of long-term respiratory sequelae and will help to elucidate the impact of secondary injuries, e.g., first and second hand smoke as well as viral infections on this trajectory (147). Importantly, lung function has to be interpreted in the context of the era in which BPD was diagnosed, thus taking both the underlying BPD definition as well as the standards of perinatal care into account (2, 148, 149).
Conclusion
To conclude, the unique response of the developing lung to early postnatal injury is characterized by sustained inflammatory processes, ECM remodeling and a pronounced alteration in growth factor signaling that ultimately results in the characteristic histopathologic picture of impaired alveolar and vascular development. These processes are critically determined by the pulmonary capacity to respond to and compensate for environmental challenges inducing oxidative stress and imbalance in growth factor signaling (Figure 1). We now understand that the effects provoked through early organ injury lead to a characteristic response to challenges later in life and an altered lung function trajectory due to differences in the pulmonary aging process. Subsequently, treatment strategies and life-style recommendations advocated to this patient population need to acknowledge the pulmonary “memory” effects that results from early injury as well as later disease characteristics and co-morbidity development (150, 151). Therefore, pediatricians, primary care physicians and adult pulmonologists need a close and iterative knowledge exchange to adequately address these topics, including the role of second and third hits in lung function decline (152, 153).
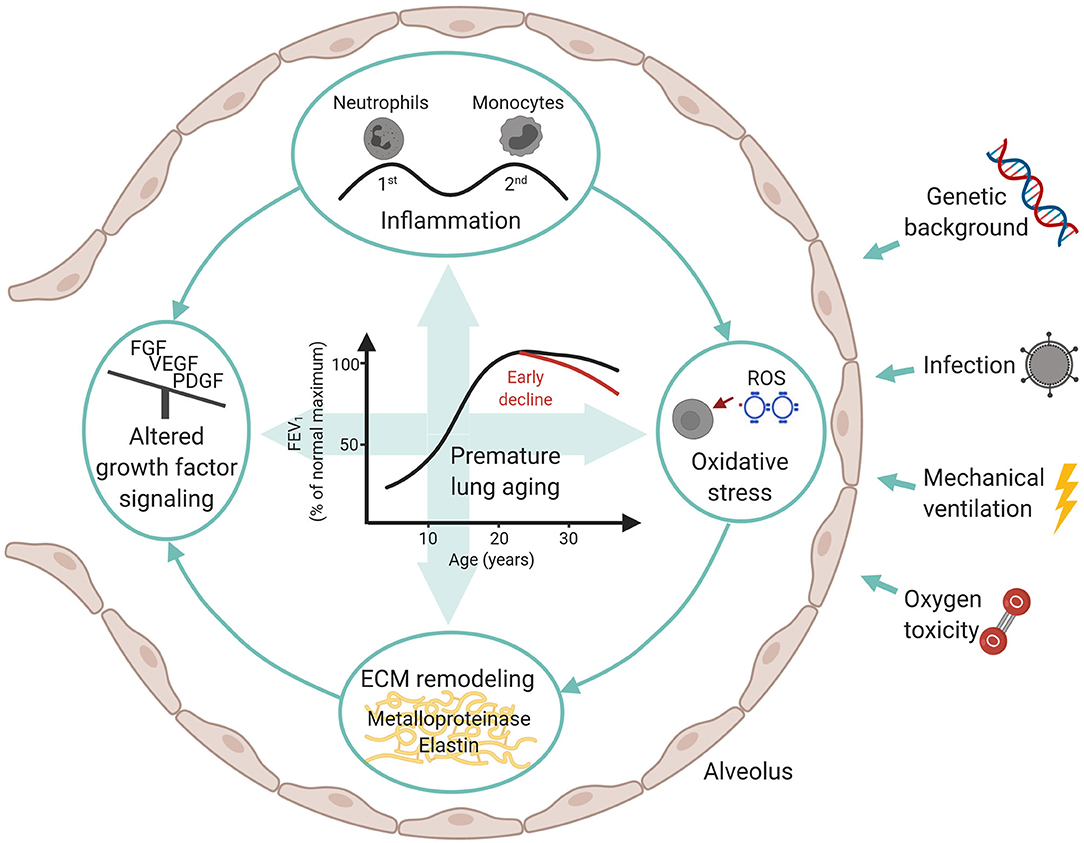
Figure 1. Overview over influencing factors and molecular mechanisms in BPD development with a focus on long-term consequences.
Author Contributions
All authors listed have made a substantial, direct and intellectual contribution to the work, and approved it for publication.
Funding
The present publication was supported by the Helmholtz Zentrum Muenchen, Germany, the German Center for Lung Research (DZL) as well as the Research Training Group Targets in Toxicology (GRK2338) of the German science and research organization (DFG) and the doctoral study program Molecular and clinical-translational science (FöFoLe) of the Ludwig-Maximilian University Munich.
Conflict of Interest
The authors declare that the research was conducted in the absence of any commercial or financial relationships that could be construed as a potential conflict of interest.
Acknowledgments
Figure 1 was created with BioRender.com. We published a short educational article about the long-term consequences of BPD in the Swiss Journal “InFo Pneumologie & Allergologie” (in German) to raise more awareness amongst pediatricians and students.
References
1. Duijts L, van Meel ER, Moschino L, Baraldi E, Barnhoorn M, Bramer WM, et al. European Respiratory Society guideline on long-term management of children with bronchopulmonary dysplasia. Eur Respir J. (2020) 55:1900788. doi: 10.1183/13993003.00788-2019
2. Jobe AH, Bancalari E. Bronchopulmonary dysplasia. Am J Respir Crit Care Med. (2001) 163:1723–9. doi: 10.1164/ajrccm.163.7.2011060
3. Doyle LW. Evaluation of neonatal intensive care for extremely-low-birth-weight infants. Semin Fetal Neonatal Med. (2006) 11:139–45. doi: 10.1016/j.siny.2005.11.009
4. Gortner L, Misselwitz B, Milligan D, Zeitlin J, Kollee L, Boerch K, et al. Rates of bronchopulmonary dysplasia in very preterm neonates in Europe: results from the MOSAIC cohort. Neonatology. (2011) 99:112–7. doi: 10.1159/000313024
5. Stoll BJ, Hansen NI, Bell EF, Shankaran S, Laptook AR, Walsh MC, et al. Neonatal outcomes of extremely preterm infants from the NICHD Neonatal Research Network. Pediatrics. (2010) 126:443–56. doi: 10.1542/peds.2009-2959
6. Vachon E, Bourbonnais Y, Bingle CD, Rowe SJ, Janelle MF, Tremblay GM. Anti-inflammatory effect of pre-elafin in lipopolysaccharide-induced acute lung inflammation. Biol Chem. (2002) 383:1249–56. doi: 10.1515/BC.2002.138
7. Van Marter LJ, Pagano M, Allred EN, Leviton A, Kuban KC. Rate of bronchopulmonary dysplasia as a function of neonatal intensive care practices. J Pediatr. (1992) 120:938–46. doi: 10.1016/S0022-3476(05)81968-7
8. Ehrenkranz RA, Walsh MC, Vohr BR, Jobe AH, Wright LL, Fanaroff AA, et al. Validation of the National Institutes of Health consensus definition of bronchopulmonary dysplasia. Pediatrics. (2005) 116:1353–60. doi: 10.1542/peds.2005-0249
9. Johnson AH, Peacock JL, Greenough A, Marlow N, Limb ES, Marston L, et al. High-frequency oscillatory ventilation for the prevention of chronic lung disease of prematurity. N Engl J Med. (2002) 347:633–42. doi: 10.1056/NEJMoa020432
10. Husain AN, Siddiqui NH, Stocker JT. Pathology of arrested acinar development in postsurfactant bronchopulmonary dysplasia. Hum Pathol. (1998) 29:710–7. doi: 10.1016/S0046-8177(98)90280-5
11. Lopez E, Mathlouthi J, Lescure S, Krauss B, Jarreau PH, Moriette G. Capnography in spontaneously breathing preterm infants with bronchopulmonary dysplasia. Pediatr Pulmonol. (2011) 46:896–902. doi: 10.1002/ppul.21445
12. Clyman R, Cassady G, Kirklin JK, Collins M, Philips JB III. The role of patent ductus arteriosus ligation in bronchopulmonary dysplasia: reexamining a randomized controlled trial. J Pediatr. (2009) 154:873–6. doi: 10.1016/j.jpeds.2009.01.005
13. Korhonen P, Tammela O, Koivisto AM, Laippala P, Ikonen S. Frequency and risk factors in bronchopulmonary dysplasia in a cohort of very low birth weight infants. Early Hum Dev. (1999) 54:245–58. doi: 10.1016/S0378-3782(98)00101-7
14. Kramer BW. Antenatal inflammation and lung injury: prenatal origin of neonatal disease. J Perinatol. (2008) 28(Suppl 1):S21–7. doi: 10.1038/jp.2008.46
15. Mittendorf R, Covert R, Montag AG, elMasri W, Muraskas J, Lee KS, et al. Special relationships between fetal inflammatory response syndrome and bronchopulmonary dysplasia in neonates. J Perinat Med. (2005) 33:428–34. doi: 10.1515/JPM.2005.076
16. Oh W, Poindexter BB, Perritt R, Lemons JA, Bauer CR, Ehrenkranz RA, et al. Association between fluid intake and weight loss during the first ten days of life and risk of bronchopulmonary dysplasia in extremely low birth weight infants. J Pediatr. (2005) 147:786–90. doi: 10.1016/j.jpeds.2005.06.039
17. Stevens TP, Harrington EW, Blennow M, Soll RF. Early surfactant administration with brief ventilation vs. selective surfactant and continued mechanical ventilation for preterm infants with or at risk for respiratory distress syndrome. Cochrane Database Syst Rev. (2007) 2007:CD003063. doi: 10.1002/14651858.CD003063.pub3
18. Biniwale MA, Ehrenkranz RA. The role of nutrition in the prevention and management of bronchopulmonary dysplasia. Semin Perinatol. (2006) 30:200–8. doi: 10.1053/j.semperi.2006.05.007
19. Shenai JP, Chytil F, Stahlman MT. Vitamin A status of neonates with bronchopulmonary dysplasia. Pediatr Res. (1985) 19:185–8. doi: 10.1203/00006450-198502000-00007
20. Watterberg KL, Scott SM. Evidence of early adrenal insufficiency in babies who develop bronchopulmonary dysplasia. Pediatrics. (1995) 95:120–5.
21. Hansen AR, Barnes CM, Folkman J, McElrath TF. Maternal preeclampsia predicts the development of bronchopulmonary dysplasia. J Pediatr. (2010) 156:532–6. doi: 10.1016/j.jpeds.2009.10.018
22. Rocha G, de Lima FF, Machado AP, Guimaraes H Collaborators of the Hypertensive Disorders of Pregnancy Study G. Preeclampsia predicts higher incidence of bronchopulmonary dysplasia. J Perinatol. (2018) 38:1165–73. doi: 10.1038/s41372-018-0133-8
23. Bose C, Van Marter LJ, Laughon M, O'Shea TM, Allred EN, Karna P, et al. Fetal growth restriction and chronic lung disease among infants born before the 28th week of gestation. Pediatrics. (2009) 124:e450–8. doi: 10.1542/peds.2008-3249
24. Regev RH, Lusky A, Dolfin T, Litmanovitz I, Arnon S, Reichman B, et al. Excess mortality and morbidity among small-for-gestational-age premature infants: a population-based study. J Pediatr. (2003) 143:186–91. doi: 10.1067/S0022-3476(03)00181-1
25. Reiss I, Landmann E, Heckmann M, Misselwitz B, Gortner L. Increased risk of bronchopulmonary dysplasia and increased mortality in very preterm infants being small for gestational age. Arch Gynecol Obstet. (2003) 269:40–4. doi: 10.1007/s00404-003-0486-9
26. Rieger-Fackeldey E, Schulze A, Pohlandt F, Schwarze R, Dinger J, Lindner W. Short-term outcome in infants with a birthweight less than 501 grams. Acta Paediatr. (2005) 94:211–6. doi: 10.1080/08035250410023223
27. Walsh MC, Yao Q, Horbar JD, Carpenter JH, Lee SK, Ohlsson A. Changes in the use of postnatal steroids for bronchopulmonary dysplasia in 3 large neonatal networks. Pediatrics. (2006) 118:e1328–35. doi: 10.1542/peds.2006-0359
28. Rozance PJ, Seedorf GJ, Brown A, Roe G, O'Meara MC, Gien J, et al. Intrauterine growth restriction decreases pulmonary alveolar and vessel growth and causes pulmonary artery endothelial cell dysfunction in vitro in fetal sheep. Am J Physiol Lung Cell Mol Physiol. (2011) 301:L860–71. doi: 10.1152/ajplung.00197.2011
29. Gilliland FD, Berhane K, McConnell R, Gauderman WJ, Vora H, Rappaport EB, et al. Maternal smoking during pregnancy, environmental tobacco smoke exposure and childhood lung function. Thorax. (2000) 55:271–6. doi: 10.1136/thorax.55.4.271
30. Vogt Isaksen C. Maternal smoking, intrauterine growth restriction, and placental apoptosis. Pediatr Dev Pathol. (2004) 7:433–42. doi: 10.1007/s10024-004-0105-1
31. Hennessy EM, Bracewell MA, Wood N, Wolke D, Costeloe K, Gibson A, et al. Respiratory health in pre-school and school age children following extremely preterm birth. Arch Dis Child. (2008) 93:1037–43. doi: 10.1136/adc.2008.140830
32. Merritt TA, Deming DD, Boynton BR. The ‘new' bronchopulmonary dysplasia: challenges and commentary. Semin Fetal Neonatal Med. (2009) 14:345–57. doi: 10.1016/j.siny.2009.08.009
33. Weinberger B, Anwar M, Henien S, Sosnovsky A, Hiatt M, Jochnowitz N, et al. Association of lipid peroxidation with antenatal betamethasone and oxygen radial disorders in preterm infants. Biol Neonate. (2004) 85:121–7. doi: 10.1159/000074968
34. Bal MP, de Vries WB, van Oosterhout MF, Baan J, van der Wall EE, van Bel F, et al. Long-term cardiovascular effects of neonatal dexamethasone treatment: hemodynamic follow-up by left ventricular pressure-volume loops in rats. J Appl Physiol. (2008) 104:446–50. doi: 10.1152/japplphysiol.00951.2007
35. Kamphuis PJ, de Vries WB, Bakker JM, Kavelaars A, van Dijk JE, Schipper ME, et al. Reduced life expectancy in rats after neonatal dexamethasone treatment. Pediatr Res. (2007) 61:72–6. doi: 10.1203/01.pdr.0000249980.95264.dd
36. Stoll BJ, Hansen N, Fanaroff AA, Wright LL, Carlo WA, Ehrenkranz RA, et al. Changes in pathogens causing early-onset sepsis in very-low-birth-weight infants. N Engl J Med. (2002) 347:240–7. doi: 10.1056/NEJMoa012657
37. Olszak T, An D, Zeissig S, Vera MP, Richter J, Franke A, et al. Microbial exposure during early life has persistent effects on natural killer T cell function. Science. (2012) 336:489–93. doi: 10.1126/science.1219328
38. Group E. Incidence of and risk factors for neonatal morbidity after active perinatal care: extremely preterm infants study in Sweden. (EXPRESS). Acta Paediatr. (2010) 99:978–92. doi: 10.1111/j.1651-2227.2010.01846.x
39. Isayama T, Shah PS, Ye XY, Dunn M, Da Silva O, Alvaro R, et al. Adverse impact of maternal cigarette smoking on preterm infants: a population-based cohort study. Am J Perinatol. (2015) 32:1105–11. doi: 10.1055/s-0035-1548728
40. Bhandari V, Bizzarro MJ, Shetty A, Zhong X, Page GP, Zhang H, et al. Familial and genetic susceptibility to major neonatal morbidities in preterm twins. Pediatrics. (2006) 117:1901–6. doi: 10.1542/peds.2005-1414
41. Hallman M, Haataja R. Genetic influences and neonatal lung disease. Semin Neonatol. (2003) 8:19–27. doi: 10.1016/S1084-2756(02)00196-3
42. Hilgendorff A, Heidinger K, Pfeiffer A, Bohnert A, Konig IR, Ziegler A, et al. Association of polymorphisms in the mannose-binding lectin gene and pulmonary morbidity in preterm infants. Genes Immun. (2007) 8:671–7. doi: 10.1038/sj.gene.6364432
43. Poggi C, Giusti B, Vestri A, Pasquini E, Abbate R, Dani C. Genetic polymorphisms of antioxidant enzymes in preterm infants. J Matern Fetal Neonatal Med. (2012) 25(Suppl 4):131–4. doi: 10.3109/14767058.2012.714976
44. Binet ME, Bujold E, Lefebvre F, Tremblay Y, Piedboeuf B, Canadian Neonatal N. Role of gender in morbidity and mortality of extremely premature neonates. Am J Perinatol. (2012) 29:159–66. doi: 10.1055/s-0031-1284225
45. Trotter A, Maier L, Kron M, Pohlandt F. Effect of oestradiol and progesterone replacement on bronchopulmonary dysplasia in extremely preterm infants. Arch Dis Child Fetal Neonatal Ed. (2007) 92:F94–8. doi: 10.1136/adc.2006.097170
46. Vrijlandt EJ, Gerritsen J, Boezen HM, Duiverman EJ, Dutch P-CSG. Gender differences in respiratory symptoms in 19-year-old adults born preterm. Respir Res. (2005) 6:117. doi: 10.1186/1465-9921-6-117
47. Stoll BJ, Gordon T, Korones SB, Shankaran S, Tyson JE, Bauer CR, et al. Early-onset sepsis in very low birth weight neonates: a report from the National Institute of Child Health and Human Development Neonatal Research Network. J Pediatr. (1996) 129:72–80. doi: 10.1016/S0022-3476(96)70192-0
48. Watterberg KL, Demers LM, Scott SM, Murphy S. Chorioamnionitis and early lung inflammation in infants in whom bronchopulmonary dysplasia develops. Pediatrics. (1996) 97:210–5.
49. Yoon BH, Romero R, Jun JK, Park KH, Park JD, Ghezzi F, et al. Am J Obstet Gynecol. (1997) 177:825–30. doi: 10.1016/S0002-9378(97)70276-X
50. Speer CP. Inflammation and bronchopulmonary dysplasia: a continuing story. Semin Fetal Neonatal Med. (2006) 11:354–62. doi: 10.1016/j.siny.2006.03.004
51. Todd DA, Earl M, Lloyd J, Greenberg M, John E. Cytological changes in endotracheal aspirates associated with chronic lung disease. Early Hum Dev. (1998) 51:13–22. doi: 10.1016/S0378-3782(97)00069-8
52. Klinger G, Levy I, Sirota L, Boyko V, Lerner-Geva L, Reichman B, et al. Outcome of early-onset sepsis in a national cohort of very low birth weight infants. Pediatrics. (2010) 125:e736–40. doi: 10.1542/peds.2009-2017
53. Dell'Orto V, Raschetti R, Centorrino R, Montane A, Tissieres P, Yousef N, et al. Short- and long-term respiratory outcomes in neonates with ventilator-associated pneumonia. Pediatr Pulmonol. (2019) 54:1982–8. doi: 10.1002/ppul.24487
54. Marshall DD, Kotelchuck M, Young TE, Bose CL, Kruyer L, O'Shea TM. Risk factors for chronic lung disease in the surfactant era: a North Carolina population-based study of very low birth weight infants. North Carolina Neonatologists Association. Pediatrics. (1999) 104:1345–50. doi: 10.1542/peds.104.6.1345
55. Bose CL, Laughon MM, Allred EN, O'Shea TM, Van Marter LJ, Ehrenkranz RA, et al. Systemic inflammation associated with mechanical ventilation among extremely preterm infants. Cytokine. (2013) 61:315–22. doi: 10.1016/j.cyto.2012.10.014
56. Jobe AH, Kallapur SG. Long term consequences of oxygen therapy in the neonatal period. Semin Fetal Neonatal Med. (2010) 15:230–5. doi: 10.1016/j.siny.2010.03.007
57. Kroon AA, Wang J, Huang Z, Cao L, Kuliszewski M, Post M. Inflammatory response to oxygen and endotoxin in newborn rat lung ventilated with low tidal volume. Pediatr Res. (2010) 68:63–9. doi: 10.1203/PDR.0b013e3181e17caa
58. Stoll BJ, Hansen NI, Bell EF, Shankaran S, Laptook AR, Walsh MC, et al. Pathology of arrested acinar development in postsurfactant bronchopulmonary dysplasia. Hum Pathol. (1998) 29:710–7.
59. Polglase GR, Hillman NH, Ball MK, Kramer BW, Kallapur SG, Jobe AH, et al. Lung and systemic inflammation in preterm lambs on continuous positive airway pressure or conventional ventilation. Pediatr Res. (2009) 65:67–71. doi: 10.1203/PDR.0b013e318189487e
60. Lechner AJ, Driver IH, Lee J, Conroy CM, Nagle A, Locksley RM, et al. Recruited monocytes and type 2 immunity promote lung regeneration following pneumonectomy. Cell Stem Cell. (2017) 21:120–34.e7. doi: 10.1016/j.stem.2017.03.024
61. Maus UA, Janzen S, Wall G, Srivastava M, Blackwell TS, Christman JW, et al. Resident alveolar macrophages are replaced by recruited monocytes in response to endotoxin-induced lung inflammation. Am J Respir Cell Mol Biol. (2006) 35:227–35. doi: 10.1165/rcmb.2005-0241OC
62. McQuattie-Pimentel AC, Budinger GRS, Ballinger MN. Monocyte-derived alveolar macrophages: the dark side of lung repair? Am J Respir Cell Mol Biol. (2018) 58:5–6. doi: 10.1165/rcmb.2017-0328ED
63. Zaslona Z, Przybranowski S, Wilke C, van Rooijen N, Teitz-Tennenbaum S, Osterholzer JJ, et al. Resident alveolar macrophages suppress, whereas recruited monocytes promote, allergic lung inflammation in murine models of asthma. J Immunol. (2014) 193:4245–53. doi: 10.4049/jimmunol.1400580
64. Atochina-Vasserman EN, Bates SR, Zhang P, Abramova H, Zhang Z, Gonzales L, et al. Early alveolar epithelial dysfunction promotes lung inflammation in a mouse model of Hermansky-Pudlak syndrome. Am J Respir Crit Care Med. (2011) 184:449–58. doi: 10.1164/rccm.201011-1882OC
65. Hilgendorff A, Parai K, Ertsey R, Jain N, Navarro EF, Peterson JL, et al. Inhibiting lung elastase activity enables lung growth in mechanically ventilated newborn mice. Am J Respir Crit Care Med. (2011) 184:537–46. doi: 10.1164/rccm.201012-2010OC
66. Robertson IB, Horiguchi M, Zilberberg L, Dabovic B, Hadjiolova K, Rifkin DB. Latent TGF-beta-binding proteins. Matrix Biol. (2015) 47:44–53. doi: 10.1016/j.matbio.2015.05.005
67. Alvira CM, Abate A, Yang G, Dennery PA, Rabinovitch M. Nuclear factor-kappaB activation in neonatal mouse lung protects against lipopolysaccharide-induced inflammation. Am J Respir Crit Care Med. (2007) 175:805–15. doi: 10.1164/rccm.200608-1162OC
68. Iosef C, Alastalo TP, Hou Y, Chen C, Adams ES, Lyu SC, et al. Inhibiting NF-kappaB in the developing lung disrupts angiogenesis and alveolarization. Am J Physiol Lung Cell Mol Physiol. (2012) 302:L1023–36. doi: 10.1152/ajplung.00230.2011
69. McKenna S, Michaelis KA, Agboke F, Liu T, Han K, Yang G, et al. Sustained hyperoxia-induced NF-kappaB activation improves survival and preserves lung development in neonatal mice. Am J Physiol Lung Cell Mol Physiol. (2014) 306:L1078–89. doi: 10.1152/ajplung.00001.2014
70. Bose CL, Dammann CE, Laughon MM. Bronchopulmonary dysplasia and inflammatory biomarkers in the premature neonate. Arch Dis Child Fetal Neonatal Ed. (2008) 93:F455–61. doi: 10.1136/adc.2007.121327
71. Rose MJ, Stenger MR, Joshi MS, Welty SE, Bauer JA, Nelin LD. Inhaled nitric oxide decreases leukocyte trafficking in the neonatal mouse lung during exposure to >95% oxygen. Pediatr Res. (2010) 67:244–9. doi: 10.1203/PDR.0b013e3181ca0d93
72. Vento G, Tirone C, Lulli P, Capoluongo E, Ameglio F, Lozzi S, et al. Bronchoalveolar lavage fluid peptidomics suggests a possible matrix metalloproteinase-3 role in bronchopulmonary dysplasia. Intensive Care Med. (2009) 35:2115–24. doi: 10.1007/s00134-009-1646-6
73. Watterberg KL, Carmichael DF, Gerdes JS, Werner S, Backstrom C, Murphy S. Secretory leukocyte protease inhibitor and lung inflammation in developing bronchopulmonary dysplasia. J Pediatr. (1994) 125:264–9. doi: 10.1016/S0022-3476(94)70209-8
74. Poonyagariyagorn HK, Metzger S, Dikeman D, Mercado AL, Malinina A, Calvi C, et al. Superoxide dismutase 3 dysregulation in a murine model of neonatal lung injury. Am J Respir Cell Mol Biol. (2014) 51:380–90. doi: 10.1165/rcmb.2013-0043OC
75. Collard KJ, Godeck S, Holley JE, Quinn MW. Pulmonary antioxidant concentrations and oxidative damage in ventilated premature babies. Arch Dis Child Fetal Neonatal Ed. (2004) 89:F412–6. doi: 10.1136/adc.2002.016717
76. Davis JM, Parad RB, Michele T, Allred E, Price A, Rosenfeld W, et al. Pulmonary outcome at 1 year corrected age in premature infants treated at birth with recombinant human CuZn superoxide dismutase. Pediatrics. (2003) 111:469–76. doi: 10.1542/peds.111.3.469
77. Filippone M, Bonetto G, Corradi M, Frigo AC, Baraldi E. Evidence of unexpected oxidative stress in airways of adolescents born very pre-term. Eur Respir J. (2012) 40:1253–9. doi: 10.1183/09031936.00185511
78. Buczynski BW, Yee M, Paige Lawrence B, O'Reilly MA. Lung development and the host response to influenza A virus are altered by different doses of neonatal oxygen in mice. Am J Physiol Lung Cell Mol Physiol. (2012) 302:L1078–87. doi: 10.1152/ajplung.00026.2012
79. Belik J, Jankov RP, Pan J, Tanswell AK. Chronic O2 exposure enhances vascular and airway smooth muscle contraction in the newborn but not adult rat. J Appl Physiol. (2003) 94:2303–12. doi: 10.1152/japplphysiol.00820.2002
80. Yee M, White RJ, Awad HA, Bates WA, McGrath-Morrow SA, O'Reilly MA. Neonatal hyperoxia causes pulmonary vascular disease and shortens life span in aging mice. Am J Pathol. (2011) 178:2601–10. doi: 10.1016/j.ajpath.2011.02.010
81. Balasubramaniam V, Mervis CF, Maxey AM, Markham NE, Abman SH. Hyperoxia reduces bone marrow, circulating, and lung endothelial progenitor cells in the developing lung: implications for the pathogenesis of bronchopulmonary dysplasia. Am J Physiol Lung Cell Mol Physiol. (2007) 292:L1073–84. doi: 10.1152/ajplung.00347.2006
82. Londhe VA, Sundar IK, Lopez B, Maisonet TM, Yu Y, Aghai ZH, et al. Hyperoxia impairs alveolar formation and induces senescence through decreased histone deacetylase activity and up-regulation of p21 in neonatal mouse lung. Pediatr Res. (2011) 69(5 Pt 1):371–7. doi: 10.1203/PDR.0b013e318211c917
83. Nanduri J, Makarenko V, Reddy VD, Yuan G, Pawar A, Wang N, et al. Epigenetic regulation of hypoxic sensing disrupts cardiorespiratory homeostasis. Proc Natl Acad Sci USA. (2012) 109:2515–20. doi: 10.1073/pnas.1120600109
84. Alejandre-Alcazar MA, Kwapiszewska G, Reiss I, Amarie OV, Marsh LM, Sevilla-Perez J, et al. Hyperoxia modulates TGF-beta/BMP signaling in a mouse model of bronchopulmonary dysplasia. Am J Physiol Lung Cell Mol Physiol. (2007) 292:L537–49. doi: 10.1152/ajplung.00050.2006
85. Chao CM, Moiseenko A, Kosanovic D, Rivetti S, El Agha E, Wilhelm J, et al. Impact of Fgf10 deficiency on pulmonary vasculature formation in a mouse model of bronchopulmonary dysplasia. Hum Mol Genet. (2019) 28:1429–44. doi: 10.1093/hmg/ddy439
86. Jankov RP, Keith Tanswell A. Growth factors, postnatal lung growth and bronchopulmonary dysplasia. Paediatr Respir Rev. (2004) 5(Suppl A):S265–75. doi: 10.1016/S1526-0542(04)90050-4
87. Li C, Smith SM, Peinado N, Gao F, Li W, Lee MK, et al. WNT5a-ROR signaling is essential for alveologenesis. Cells. (2020) 9:384. doi: 10.3390/cells9020384
88. Oak P, Hilgendorff A. The BPD trio? Interaction of dysregulated PDGF, VEGF, and TGF signaling in neonatal chronic lung disease. Mol Cell Pediatr. (2017) 4:11. doi: 10.1186/s40348-017-0076-8
89. Sucre JM, Wilkinson D, Vijayaraj P, Paul M, Dunn B, Alva-Ornelas JA, et al. A three-dimensional human model of the fibroblast activation that accompanies bronchopulmonary dysplasia identifies Notch-mediated pathophysiology. Am J Physiol Lung Cell Mol Physiol. (2016) 310:L889–98. doi: 10.1152/ajplung.00446.2015
90. Sucre JMS, Deutsch GH, Jetter CS, Ambalavanan N, Benjamin JT, Gleaves LA, et al. A shared pattern of beta-catenin activation in bronchopulmonary dysplasia and idiopathic pulmonary fibrosis. Am J Pathol. (2018) 188:853–62. doi: 10.1016/j.ajpath.2017.12.004
91. Kazzi SN, Kim UO, Quasney MW, Buhimschi I. Polymorphism of tumor necrosis factor-alpha and risk and severity of bronchopulmonary dysplasia among very low birth weight infants. Pediatrics. (2004) 114:e243–8. doi: 10.1542/peds.114.2.e243
92. Kunzmann S, Speer CP, Jobe AH, Kramer BW. Antenatal inflammation induced TGF-beta1 but suppressed CTGF in preterm lungs. Am J Physiol Lung Cell Mol Physiol. (2007) 292:L223–31. doi: 10.1152/ajplung.00159.2006
93. Nold MF, Mangan NE, Rudloff I, Cho SX, Shariatian N, Samarasinghe TD, et al. Interleukin-1 receptor antagonist prevents murine bronchopulmonary dysplasia induced by perinatal inflammation and hyperoxia. Proc Natl Acad Sci USA. (2013) 110:14384–9. doi: 10.1073/pnas.1306859110
94. Didon L, Roos AB, Elmberger GP, Gonzalez FJ, Nord M. Lung-specific inactivation of CCAAT/enhancer binding protein alpha causes a pathological pattern characteristic of COPD. Eur Respir J. (2010) 35:186–97. doi: 10.1183/09031936.00185008
95. Yang G, Abate A, George AG, Weng YH, Dennery PA. Maturational differences in lung NF-kappaB activation and their role in tolerance to hyperoxia. J Clin Invest. (2004) 114:669–78. doi: 10.1172/JCI200419300
96. Kinsella JP, Greenough A, Abman SH. Bronchopulmonary dysplasia. Lancet. (2006) 367:1421–31. doi: 10.1016/S0140-6736(06)68615-7
97. Steinhorn RH. Neonatal pulmonary hypertension. Pediatr Crit Care Med. (2010) 11(2 Suppl):S79–84. doi: 10.1097/PCC.0b013e3181c76cdc
98. De Paepe ME, Greco D, Mao Q. Angiogenesis-related gene expression profiling in ventilated preterm human lungs. Exp Lung Res. (2010) 36:399–410. doi: 10.3109/01902141003714031
99. De Paepe ME, Mao Q, Powell J, Rubin SE, DeKoninck P, Appel N, et al. Growth of pulmonary microvasculature in ventilated preterm infants. Am J Respir Crit Care Med. (2006) 173:204–11. doi: 10.1164/rccm.200506-927OC
100. Thebaud B. Angiogenesis in lung development, injury and repair: implications for chronic lung disease of prematurity. Neonatology. (2007) 91:291–7. doi: 10.1159/000101344
101. Bland RD, Ling CY, Albertine KH, Carlton DP, MacRitchie AJ, Day RW, et al. Pulmonary vascular dysfunction in preterm lambs with chronic lung disease. Am J Physiol Lung Cell Mol Physiol. (2003) 285:L76–85. doi: 10.1152/ajplung.00395.2002
102. Vyas-Read S, Shaul PW, Yuhanna IS, Willis BC. Nitric oxide attenuates epithelial-mesenchymal transition in alveolar epithelial cells. Am J Physiol Lung Cell Mol Physiol. (2007) 293:L212–21. doi: 10.1152/ajplung.00475.2006
103. Ito Y, Betsuyaku T, Nagai K, Nasuhara Y, Nishimura M. Expression of pulmonary VEGF family declines with age and is further down-regulated in lipopolysaccharide. (LPS)-induced lung injury. Exp Gerontol. (2005) 40:315–23. doi: 10.1016/j.exger.2005.01.009
104. Kamba T, Tam BY, Hashizume H, Haskell A, Sennino B, Mancuso MR, et al. VEGF-dependent plasticity of fenestrated capillaries in the normal adult microvasculature. Am J Physiol Heart Circ Physiol. (2006) 290:H560–76. doi: 10.1152/ajpheart.00133.2005
105. Kunig AM, Balasubramaniam V, Markham NE, Seedorf G, Gien J, Abman SH. Recombinant human VEGF treatment transiently increases lung edema but enhances lung structure after neonatal hyperoxia. Am J Physiol Lung Cell Mol Physiol. (2006) 291:L1068–78. doi: 10.1152/ajplung.00093.2006
106. Mizikova I, Morty RE. The extracellular matrix in bronchopulmonary dysplasia: target and source. Front Med. (2015) 2:91. doi: 10.3389/fmed.2015.00091
107. Torday JS, Rehan VK. Mechanotransduction determines the structure and function of lung and bone: a theoretical model for the pathophysiology of chronic disease. Cell Biochem Biophys. (2003) 37:235–46. doi: 10.1385/CBB:37:3:235
108. Pierce RA, Albertine KH, Starcher BC, Bohnsack JF, Carlton DP, Bland RD. Chronic lung injury in preterm lambs: disordered pulmonary elastin deposition. Am J Physiol. (1997) 272(3 Pt 1):L452–60. doi: 10.1152/ajplung.1997.272.3.L452
109. Thibeault DW, Mabry SM, Truog WE. Lung elastic tissue maturation and perturbations during the evolution of chronic lung disease. Pediatrics. (2000) 106:1452–9. doi: 10.1542/peds.106.6.1452
110. Thibeault DW, Mabry SM, Zhang X, Truog WE. Collagen scaffolding during development and its deformation with chronic lung disease. Pediatrics. (2003) 111(4 Pt 1):766–76. doi: 10.1542/peds.111.4.766
111. Bruce MC, Schuyler M, Martin RJ, Starcher BC, Tomashefski JF, Wedig KE. Risk factors for the degradation of lung elastic fibers in the ventilated neonate. Implications for impaired lung development in bronchopulmonary dysplasia. Am Rev Respir Dis. (1992) 146:204–12. doi: 10.1164/ajrccm/146.1.204
112. Bruce MC, Wedig KE, Jentoft N, Martin RJ, Cheng PW, Boat TF, et al. Altered urinary excretion of elastin cross-links in premature infants who develop bronchopulmonary dysplasia. Am Rev Respir Dis. (1985) 131:568–72. doi: 10.1164/arrd.1985.131.4.568
113. Merritt TA, Cochrane CG, Holcomb K, Bohl B, Hallman M, Strayer D, et al. Elastase and alpha 1-proteinase inhibitor activity in tracheal aspirates during respiratory distress syndrome. Role of inflammation in the pathogenesis of bronchopulmonary dysplasia. J Clin Invest. (1983) 72:656–66. doi: 10.1172/JCI111015
114. Tenholder MF, Rajagopal KR, Phillips YY, Dillard TA, Bennett LL, Mundie TG, et al. Urinary desmosine excretion as a marker of lung injury in the adult respiratory distress syndrome. Chest. (1991) 100:1385–90. doi: 10.1378/chest.100.5.1385
115. Lukkarinen H, Hogmalm A, Lappalainen U, Bry K. Matrix metalloproteinase-9 deficiency worsens lung injury in a model of bronchopulmonary dysplasia. Am J Respir Cell Mol Biol. (2009) 41:59–68. doi: 10.1165/rcmb.2008-0179OC
116. Bonvillain RW, Danchuk S, Sullivan DE, Betancourt AM, Semon JA, Eagle ME, et al. A nonhuman primate model of lung regeneration: detergent-mediated decellularization and initial in vitro recellularization with mesenchymal stem cells. Tissue Eng Part A. (2012) 18:2437–52. doi: 10.1089/ten.tea.2011.0594
117. Jensen T, Roszell B, Zang F, Girard E, Matson A, Thrall R, et al. A rapid lung de-cellularization protocol supports embryonic stem cell differentiation in vitro and following implantation. Tissue Eng Part C Methods. (2012) 18:632–46. doi: 10.1089/ten.tec.2011.0584
118. Meiners S, Eickelberg O, Konigshoff M. Hallmarks of the ageing lung. Eur Respir J. (2015) 45:807–27. doi: 10.1183/09031936.00186914
119. Meiners S, Hilgendorff A. Early injury of the neonatal lung contributes to premature lung aging: a hypothesis. Mol Cell Pediatr. (2016) 3:24. doi: 10.1186/s40348-016-0052-8
120. Jobe AH, Ikegami M. Prevention of bronchopulmonary dysplasia. Curr Opin Pediatr. (2001) 13:124–9. doi: 10.1097/00008480-200104000-00006
121. Newman JB, DeBastos AG, Batton D, Raz S. Neonatal respiratory dysfunction and neuropsychological performance at the preschool age: a study of very preterm infants with bronchopulmonary dysplasia. Neuropsychology. (2011) 25:666–78. doi: 10.1037/a0023895
122. Bolton CE, Bush A, Hurst JR, Kotecha S, McGarvey L. Lung consequences in adults born prematurely. Thorax. (2015) 70:574–80. doi: 10.1136/thoraxjnl-2014-206590
123. McGeachie MJ, Yates KP, Zhou X, Guo F, Sternberg AL, Van Natta ML, et al. Patterns of growth and decline in lung function in persistent childhood asthma. N Engl J Med. (2016) 374:1842–52. doi: 10.1056/NEJMoa1513737
124. Miller MR, Pedersen OF, Lange P, Vestbo J. Improved survival prediction from lung function data in a large population sample. Respir Med. (2009) 103:442–8. doi: 10.1016/j.rmed.2008.09.016
125. Bland RD, Albertine KH, Carlton DP, Kullama L, Davis P, Cho SC, et al. Chronic lung injury in preterm lambs: abnormalities of the pulmonary circulation and lung fluid balance. Pediatr Res. (2000) 48:64–74. doi: 10.1203/00006450-200007000-00013
126. May C, Kennedy C, Milner AD, Rafferty GF, Peacock JL, Greenough A. Lung function abnormalities in infants developing bronchopulmonary dysplasia. Arch Dis Child. (2011) 96:1014–9. doi: 10.1136/adc.2011.212332
127. Hilgendorff A, Reiss I, Gortner L, Schuler D, Weber K, Lindemann H. Impact of airway obstruction on lung function in very preterm infants at term. Pediatr Crit Care Med. (2008) 9:629–35. doi: 10.1097/PCC.0b013e31818d17c8
128. Mourani PM, Sontag MK, Younoszai A, Ivy DD, Abman SH. Clinical utility of echocardiography for the diagnosis and management of pulmonary vascular disease in young children with chronic lung disease. Pediatrics. (2008) 121:317–25. doi: 10.1542/peds.2007-1583
129. Greenough A, Alexander J, Burgess S, Bytham J, Chetcuti PA, Hagan J, et al. Preschool healthcare utilisation related to home oxygen status. Arch Dis Child Fetal Neonatal Ed. (2006) 91:F337–41. doi: 10.1136/adc.2005.088823
130. Greenough A, Alexander J, Burgess S, Chetcuti PA, Cox S, Lenney W, et al. Home oxygen status and rehospitalisation and primary care requirements of infants with chronic lung disease. Arch Dis Child. (2002) 86:40–3. doi: 10.1136/adc.86.1.40
131. Greenough A, Cox S, Alexander J, Lenney W, Turnbull F, Burgess S, et al. Health care utilisation of infants with chronic lung disease, related to hospitalisation for RSV infection. Arch Dis Child. (2001) 85:463–8. doi: 10.1136/adc.85.6.463
132. Kovesi T, Abdurahman A, Blayney M. Elevated carbon dioxide tension as a predictor of subsequent adverse events in infants with bronchopulmonary dysplasia. Lung. (2006) 184:7–13. doi: 10.1007/s00408-005-2556-1
133. Doyle LW, Cheung MM, Ford GW, Olinsky A, Davis NM, Callanan C. Birth weight <1501 g and respiratory health at age 14. Arch Dis Child. (2001) 84:40–4. doi: 10.1136/adc.84.1.40
134. Broughton S, Roberts A, Fox G, Pollina E, Zuckerman M, Chaudhry S, et al. Prospective study of healthcare utilisation and respiratory morbidity due to RSV infection in prematurely born infants. Thorax. (2005) 60:1039–44. doi: 10.1136/thx.2004.037853
135. Baraldi E, Carraro S, Filippone M. Bronchopulmonary dysplasia: definitions and long-term respiratory outcome. Early Hum Dev. (2009) 85(10 Suppl):S1–3. doi: 10.1016/j.earlhumdev.2009.08.002
136. Greenough A, Limb E, Marston L, Marlow N, Calvert S, Peacock J. Risk factors for respiratory morbidity in infancy after very premature birth. Arch Dis Child Fetal Neonatal Ed. (2005) 90:F320–3. doi: 10.1136/adc.2004.062018
137. Gross SJ, Iannuzzi DM, Kveselis DA, Anbar RD. Effect of preterm birth on pulmonary function at school age: a prospective controlled study. J Pediatr. (1998) 133:188–92. doi: 10.1016/S0022-3476(98)70219-7
138. Broughton S, Thomas MR, Marston L, Calvert SA, Marlow N, Peacock JL, et al. Very prematurely born infants wheezing at follow-up: lung function and risk factors. Arch Dis Child. (2007) 92:776–80. doi: 10.1136/adc.2006.112623
139. Pelkonen AS, Hakulinen AL, Turpeinen M. Bronchial lability and responsiveness in school children born very preterm. Am J Respir Crit Care Med. (1997) 156(4 Pt 1):1178–84. doi: 10.1164/ajrccm.156.4.9610028
140. Yuksel B, Greenough A. Relationship of symptoms to lung function abnormalities in preterm infants at follow-up. Pediatr Pulmonol. (1991) 11:202–6. doi: 10.1002/ppul.1950110304
141. Welsh L, Kirkby J, Lum S, Odendaal D, Marlow N, Derrick G, et al. The EPICure study: maximal exercise and physical activity in school children born extremely preterm. Thorax. (2010) 65:165–72. doi: 10.1136/thx.2008.107474
142. Northway WH, Moss RB, Carlisle KB, Parker BR, Popp RL, Pitlick PT, et al. Late pulmonary sequelae of bronchopulmonary dysplasia. N Engl J Med. (1990) 323:1793–9. doi: 10.1056/NEJM199012273232603
143. Greenough A, Dimitriou G, Bhat RY, Broughton S, Hannam S, Rafferty GF, et al. Lung volumes in infants who had mild to moderate bronchopulmonary dysplasia. Eur J Pediatr. (2005) 164:583–6. doi: 10.1007/s00431-005-1706-z
144. Hjalmarson O, Sandberg KL. Lung function at term reflects severity of bronchopulmonary dysplasia. J Pediatr. (2005) 146:86–90. doi: 10.1016/j.jpeds.2004.08.044
145. Doyle LW, Faber B, Callanan C, Freezer N, Ford GW, Davis NM. Bronchopulmonary dysplasia in very low birth weight subjects and lung function in late adolescence. Pediatrics. (2006) 118:108–13. doi: 10.1542/peds.2005-2522
146. Hurst JR, Beckmann J, Ni Y, Bolton CE, McEniery CM, Cockcroft JR, et al. Respiratory and cardiovascular outcomes in survivors of extremely preterm birth at 19 years. Am J Respir Crit Care Med. (2020) 202:422–32. doi: 10.1164/rccm.202001-0016OC
147. Vollsaeter M, Roksund OD, Eide GE, Markestad T, Halvorsen T. Lung function after preterm birth: development from mid-childhood to adulthood. Thorax. (2013) 68:767–76. doi: 10.1136/thoraxjnl-2012-202980
148. Baraldi E, Filippone M. Chronic lung disease after premature birth. N Engl J Med. (2007) 357:1946–55. doi: 10.1056/NEJMra067279
149. Jobe AH. The new bronchopulmonary dysplasia. Curr Opin Pediatr. (2011) 23:167–72. doi: 10.1097/MOP.0b013e3283423e6b
150. Northway WH Jr, Rosan RC, Porter DY. Pulmonary disease following respirator therapy of hyaline-membrane disease. Bronchopulmonary dysplasia. N Engl J Med. (1967) 276:357–68. doi: 10.1056/NEJM196702162760701
151. Bolton CE, Bush A, Hurst JR, Kotecha S, McGarvey L, Stocks J, et al. Are early life factors considered when managing respiratory disease? A British Thoracic Society survey of current practice. Thorax. (2012) 67:1110. doi: 10.1136/thoraxjnl-2012-202637
152. Crump C. Medical history taking in adults should include questions about preterm birth. BMJ. (2014) 349:g4860. doi: 10.1136/bmj.g4860
Keywords: neonatal chronic lung disease, bronchopulmonary dysplasia, lung aging, inflammation, mechanical ventilation, oxygen toxicity, lung function, preterm infant
Citation: Sucre J, Haist L, Bolton CE and Hilgendorff A (2021) Early Changes and Indicators Characterizing Lung Aging in Neonatal Chronic Lung Disease. Front. Med. 8:665152. doi: 10.3389/fmed.2021.665152
Received: 07 February 2021; Accepted: 04 May 2021;
Published: 31 May 2021.
Edited by:
Stefanie Krick, University of Alabama at Birmingham, United StatesReviewed by:
Jonathan L. Slaughter, The Research Institute at Nationwide Children's Hospital, United StatesJason Gien, University of Colorado, United States
Copyright © 2021 Sucre, Haist, Bolton and Hilgendorff. This is an open-access article distributed under the terms of the Creative Commons Attribution License (CC BY). The use, distribution or reproduction in other forums is permitted, provided the original author(s) and the copyright owner(s) are credited and that the original publication in this journal is cited, in accordance with accepted academic practice. No use, distribution or reproduction is permitted which does not comply with these terms.
*Correspondence: Anne Hilgendorff, YW5uZS5oaWxnZW5kb3JmZkBtZWQudW5pLW11ZW5jaGVuLmRl