- 1Lanzhou University Second Hospital, Lanzhou, China
- 2Orthopaedics Key Laboratory of Gansu Province, Lanzhou, China
- 3The 947th Army Hospital of the Chinese PLA, Kashgar, China
- 4People's Hospital of Ningxia Hui Autonomous Region, Yinchuan, China
Periostin, an extracellular matrix protein, is widely expressed in a variety of tissues and cells. It has many biological functions and is related to many diseases: for example, it promotes cell proliferation and differentiation in osteoblasts, which are closely related to osteoporosis, and mediates cell senescence and apoptosis in chondrocytes, which are involved in osteoarthritis. Furthermore, it also plays an important role in mediating inflammation and reconstruction during bronchial asthma, as well as in promoting bone development, reconstruction, repair, and strength. Therefore, periostin has been explored as a potential biomarker for various diseases. Recently, periostin has also been found to be expressed in intervertebral disc cells as a component of the intervertebral extracellular matrix, and to play a crucial role in the maintenance and degeneration of intervertebral discs. This article reviews the biological role of periostin in bone marrow-derived mesenchymal stem cells, osteoblasts, osteoclasts, chondrocytes, and annulus fibrosus and nucleus pulposus cells, which are closely related to spinal degenerative diseases. The study of its pathophysiological effects is of great significance for the diagnosis and treatment of spinal degeneration, although additional studies are needed.
Introduction
Each vertebra of the spine includes two parts: an intervertebral disc (IVD) and a bony vertebral body. The IVD consists of a central jelly-like nucleus pulposus (NP), a rigid annulus fibrous (AF), and upper and lower cartilaginous endplates, which connect the upper and lower vertebral bodies and withstand conditions such as compression, extension, bending, and torsion, and thus play an important role in bearing weight and cushioning pressure loads (1, 2). Spinal degeneration is a common clinical disease that includes vertebral body and intervertebral disc degeneration (IVDD). Disc degeneration is a common and important form of degeneration (3, 4). Long-term low back pain is a common clinical manifestation of chronic disease (1, 5). According to the Global Burden of Disease Study 2017, low back pain is a common condition that can affect up to 80% of the global population (6). It not only affects the life and work of patients but also causes heavy economic burdens on patients, their families, and society.
In recent years, the role of the extracellular matrix (ECM) in modulating the activity of tissue cells has been extensively studied. ECM proteins act not only as scaffolds for the organization of cells, but also as multifunctional regulators of cell behavior. For example, ECM proteins can modulate the activity or bioavailability of extracellular signaling molecules, such as growth factors, cytokines, chemokines, and extracellular enzymes, or directly bind to signals through cell surface receptors to regulate cell functions (7, 8). In addition, it is increasingly acknowledged that ECM proteins can alter local endogenous signal transduction responses to growth factors and cytokines (9). Therefore, ECM proteins have potential as biomarkers and precise molecular targets for therapeutic intervention in many chronic tissue diseases and even tumors (10, 11). Similar to most other tissues, IVD cells also rely on synergistic synthesis, maintenance, and degradation with the ECM to regulate and maintain the health of the IVD (12, 13).
Periostin (POSTN), an ECM protein, is the first adhesion molecule identified in the mouse osteoblast cell line MC3T3-E1, and is also called osteoblast-specific factor 2 (OSF-2). It is closely homologous to the insect cell adhesion molecule Fasciclin 1 (14). Although POSTN is not directly involved in the formation of the ECM, it plays a dynamic role in mediating the communication between cells and the surrounding microenvironment, and usually triggers specific effects (15). For example, POSTN can participate in ECM remodeling by interacting with collagen, fibronectin, tenascin C, and POSTN itself in a multi-affinity homotypic manner (16, 17). According to published reports, POSTN is also expressed in intervertebral cells, mesenchymal stem cells (MSCs), and osteoblasts, and plays a vital role in regulating bone density and IVD homeostasis (18, 19).
In short, the role of POSTN in affecting IVD cell function and vertebral body mass and density has been explored in vivo and in vitro. POSTN expression has been shown to be related to spinal degeneration and to play an important role in the activation and progression of related cell differentiation and pathology. Therefore, understanding its functions and signaling properties is essential to improve our knowledge of healthy cell–matrix interactions, although it is clear that more studies are needed to elucidate this.
Structure and Function of POSTN
POSTN was originally identified as OSF-2. Later, because it is preferentially expressed in the periosteum and periodontal ligaments and plays a key regulatory role in the formation and maintenance of bones and teeth, it was renamed periostin by Takeshita et al. (20) and Horiuchi et al. (21). POSTN is an ECM protein with a molecular weight of 90 kDa. Its protein structure consists of an amino-terminal EMI domain, a tandem repeat of four FAS1 domains, and a carboxy-terminal domain (CTD) (22). These distinct domains have been demonstrated to bind to many proteins, including ECM proteins, and have different biological functions (23). It is precisely because of this special multi-domain structure that POSTN can help ECM proteins assemble into highly complex extracellular network structures. Therefore, the multi-domain structure of POSTN serves as the basis for its role in scaffolding in the ECM environment (24).
The EMI domain, belonging to the EMILIN family, consists of approximately 80 amino acid residues, including six highly conserved cysteine residues (25). It can bind to collagen types I, II, and IV (26) and to fibronectin (27), and promote the infiltration and contraction of the ECM (22, 28). Moreover, the EMI domain is essential for POSTN multimerization, and promotes collagen cross-linking by forming a network structure with fibronectin and tenascin C (29). The FAS1 domain is composed of four repeated FAS1 structural sequences in tandem, and was originally found in Fasciclin 1, a nerve cell adhesion molecule of Drosophila (30). The four tandem repeats of FAS1 are thought to exist only in TGFB1 (βigh3), stabilins, and POSTN in humans (31, 32). The FAS1 domain is a member of the Fasciclin 1 family and can bind to tenascin C, bone morphogenetic protein 1 (BMP-1), and cellular communication network factor 3 (CCN3), a member of the CCN family (33). The function of the FAS1 domain is to act as an anchor point to mediate the interaction of the EMI domain with other ECM components. The CTD includes an arginine-rich heparin binding site, which makes it possible to purify POSTN using heparin columns (34). Different isomers of POSTN, all having cell adhesion functions, can be formed according to the extent of C-terminal shearing (35). The heparin binding site is responsible for binding to proteoglycans (36, 37). It plays an important role in regulating cell processes such as cell migration and growth factor signal transduction by interacting with heparan sulfate proteoglycans on the cell surface (38). The structure of POSTN and a schematic diagram of its different domains interacting with other ECM proteins are shown in Figure 1.
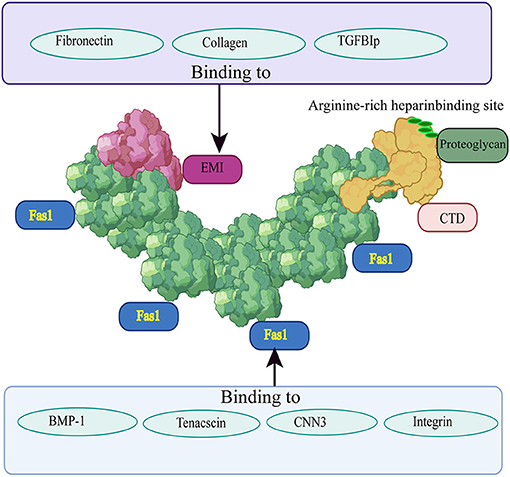
Figure 1. Structure of POSTN and its interactions with other ECM proteins. The EMI domain is depicted in red, the four-repeat FAS1 domain is shown in green, and the CTD is highlighted in yellow. Fibronectin, collagen II, and TGFB1 can bind to the EMI domain; the four repeated FAS1 domains can interact with BMP-1, tenascin C, CCN3, and integrin; the CTD includes an arginine-rich heparin binding site which is responsible for binding to proteoglycans. Different regions bind to different proteins and play different roles.
POSTN is Involved in Regulating Vertebral Body Bone Mass and Density
Spinal degeneration includes bony vertebral body and IVD degeneration, which is not only related to the physiological process of aging (39), but can also be promoted by many other factors, such as mechanical pressure, inflammatory factors, and oxidative stress (40), resulting in an increased risk of spinal imbalance, osteoporosis, and even degenerative fractures (41). Among the factors affecting vertebral degeneration, osteogenic differentiation of bone marrow-derived mesenchymal stem cells (BMSCs) and the regulation of the distribution of osteoblasts and osteoclasts are the most important (42).
BMSCs are known as key factors in the strategy of bone tissue engineering because of their self-renewal and multi-differentiation abilities (43). It is well-known that bone formation or ossification occurs through intramembranous or intrachondral ossification, and intramembranous ossification occurs via differentiation of BMSCs into osteocytes (44). In addition, BMSCs in the vertebral body can differentiate into osteoblasts and adipocytes. When the ability of BMSCs to differentiate into osteoblasts is weakened, while their ability to differentiate into adipocytes is enhanced, adipose tissue accumulates in the vertebral body, while the bone mass, density, and stiffness of the vertebral body are reduced. Therefore, osteogenic differentiation of BMSCs is necessary for the maintenance of normal bone homeostasis and bone defect repair (45, 46).
Osteoblasts are critical components of the bone multicellular unit and play an important role in bone remodeling, which is essential for maintaining the structural integrity and metabolic capacity of the skeleton (47). The accumulation of osteoclasts increases the osteoclast effect, which promotes bone metabolism and reduces bone formation, and ultimately affects the bone mass and density of the vertebral body (48, 49). In the elderly, vertebral body degeneration, for instance during osteoporosis or upon osteoporotic fractures, is caused by the deregulation of bone metabolism due to the imbalance of osteoblasts and osteoclasts. Therefore, as one of the main manifestations of spinal degeneration, bony vertebral body degeneration is closely related to the direction of BMSC differentiation and the balance between osteoblasts and osteoclasts (50–52). In the following sections we describe the effect of POSTN on the determination of vertebral body bone mass and density through its activity in BMSCs, osteoblasts, osteoclasts, and osteocytes.
POSTN Induces Osteogenic Differentiation of BMSCs
By analyzing the secretome of MSCs via proteomics methods combined with two-dimensional gel electrophoresis and tandem mass spectrometry, Coutu et al. showed for the first time that POSTN is highly expressed in MSCs and plays a key role in the mineralization of the ECM (53). This conclusion was confirmed by another study. Interestingly, the authors of the latter concluded that POSTN not only is secreted from BMSCs, but also supports tendon formation when overexpressed (54). In addition, POSTN can also bind to integrin α6β4, which is very important for the survival and differentiation of BMSCs (55).
These findings have prompted several studies on the mechanism of POSTN-mediated BMSC differentiation. For example, Zhang et al. (56) examined POSTN expression in three groups of human BMSCs: wild-type MSCs, erythropoietin-producing hepatocyte B4 (EPHB4)-overexpressing MSCs, and MSCs with siRNA-mediated inhibition of EPHB4 expression. After stimulation with ephrin B2-FC, POSTN expression was significantly increased in the first two groups, but not in the third. EPHB4 is a receptor expressed in MSCs/pre-osteoblasts and can interact with ephrin B2 to forward signals that promote osteogenic differentiation (57). This finding suggests that POSTN is a downstream mediator of EPHB4 implied in the induction of the osteogenic differentiation of BMSCs. Moreover, these authors studied the effects of POSTN and ephrin B2-FC on the osteogenic differentiation of BMSCs by measuring the level of the osteogenic marker alkaline phosphatase (ALP) and counting bone nodules, and regarded BMSCs with stimulated POSTN expression and blocked integrin αvβ3 expression as positive and negative controls, respectively. The results showed that both ephrin B2-FC and POSTN could induce osteogenic differentiation of BMSCs. Finally, these authors also studied the osteogenic differentiation mechanism mediated by POSTN and EPHB4. The results showed that both POSTN and EPHB4 could increase the expression of p-GSK3-β (Ser9) and β-catenin, as well as that of the osteogenic markers type I collagen, RUNX2, and ALP, but not in the EPHB4 siRNA-treated group and the negative control group. These results indicate that the expression of POSTN induced by the EPHB4 signaling pathway downregulates GSK-3-β (Ser9) activity through the Wnt pathway to promote the osteogenic differentiation of BMSCs (56).
In a subsequent study, the effect of POSTN on the osteogenic differentiation of MSCs bearing a modified cytotoxic T-lymphocyte associated protein 4 (MSCs-CTLA4) was examined by establishing a MSCs-CTLA4 model, because previous studies found that MSCs-CTLA4-based xenografts had better osteogenic ability than MSC-based xenografts (58). Immunohistochemical analysis showed that POSTN was expressed in both MSCs and MSCs-CTLA4 under tissue-engineered bone and immune-activated conditions, but showed higher expression in MSCs-CTLA4; moreover, collagen type I expression was consistent with this pattern. Further confirming the role of POSTN in inducing osteogenesis in MSCs-CTLA4 cells, the expression of osteogenic markers, including RUNX family transcription factor 2 (RUNX2), collagen type I, osteocalcin, osterix, and ALP, as well as the formation of calcium nodules, in MSCs increased after treatment with soluble POSTN. However, when soluble POSTN was neutralized, these effects were diminished (59). These results indicate that CTLA4 can promote ectopic osteogenesis by maintaining POSTN expression in xenotransplants. However, the specific mechanism of action remained unclear until another study demonstrated that CTLA4 maintains POSTN expression by regulating the phosphorylation of LDL receptor related protein 6 (LRP6) and activating the Wnt/β-catenin signaling pathway under immune activation (60).
In addition, when exploring the role of POSTN in the regulation of bone formation by 17β-estradiol (17β-E2), Li et al. (61) found that the expression of POSTN in BMSCs from mice subjected to ovariectomy and treated with 17β-E2 was higher than that in BMSCs from mice belonging to the sham and ovariectomy only groups. In order to study the role of POSTN in the regulation of bone formation by 17β-E2, they overexpressed POSTN by transfecting POSTN-GFP lentivirus. And RT-PCR and ALP staining were performed 7 days after osteogenic induction to investigate the osteoblastic differentiation. The results showed that the expressions of POSTN, Wnt3a and β-catenin in the Lev-POSTN group were higher than those in the control group. The overexpression of POSTN partially reversed the levels of RUNX2 and ALP, and partially restored the ALP activity of OVX-BMSCs. Then, they also treated OVX-BMSCs with Postn-siRNA to reduce the expression of POSTN in order to further evaluate the relationship between POSTN and Wnt/β-catenin signaling pathway under 17β-E2 stimulation. The expression of POSTN, Wnt3a and β-catenin was measured by Western blotting. The results showed that the expression of POSTN, Wnt3a and β-catenin protein in the Postn-siRNA group was lower than that of the negative control siRNA group. Finally, it was concluded that 17β-E2 can induce osteogenic differentiation of BMSCs in ovariectomized rats through the POSTN-Wnt/β-catenin pathway to promote osteogenic effects and reduce osteoporosis. POSTN may be a new target gene for anti-osteoporosis treatment, and, due to its wide expression profile, its role in the regulation of stem cell function is emerging (62). The mechanism by which POSTN regulates the osteogenic differentiation of BMSCs is shown in Figure 2A.
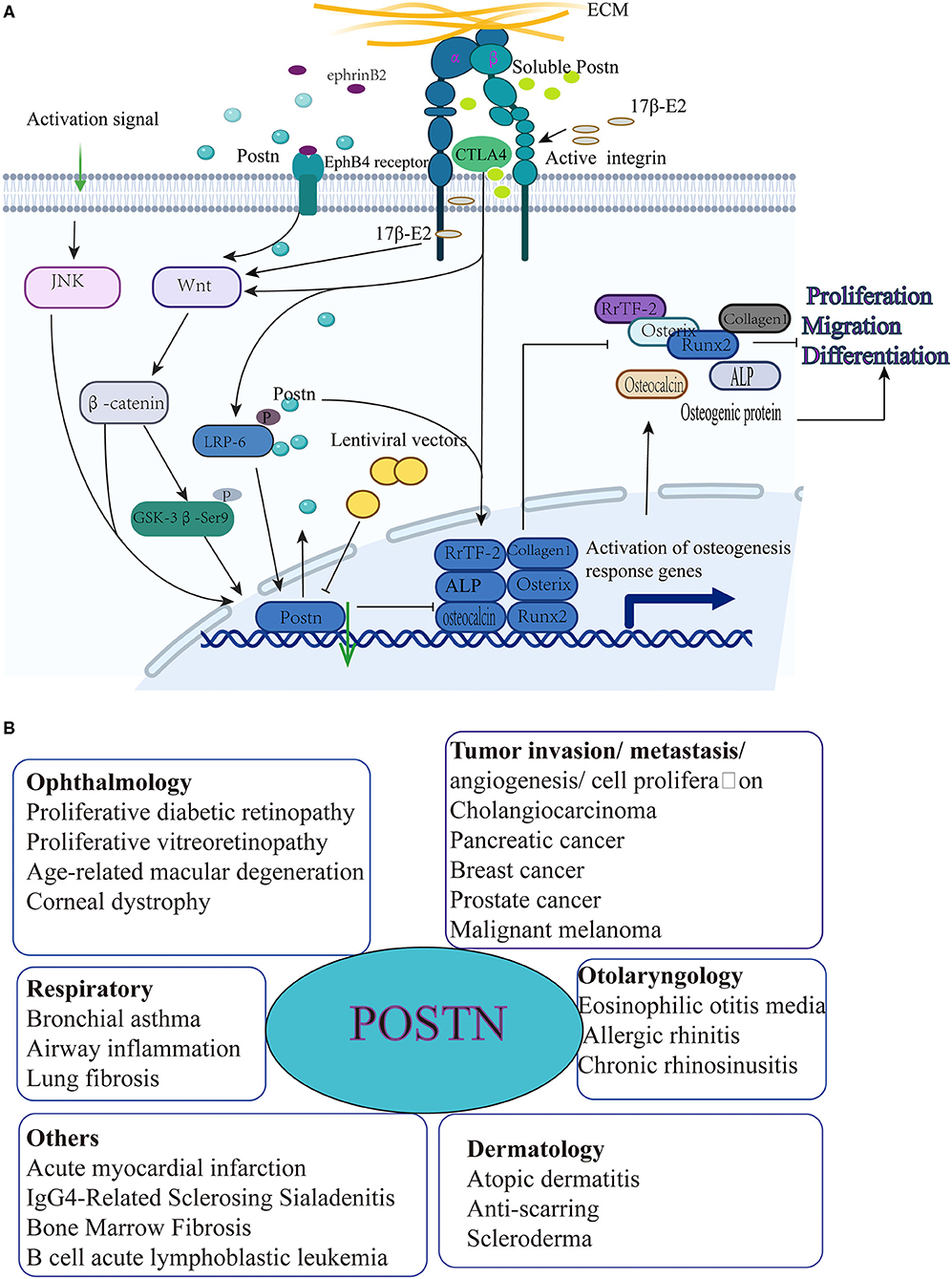
Figure 2. (A) POSTN induces osteogenic differentiation, migration, and proliferation of MSCs. (1) The receptor erythropoietin-producing hepatocyte B4 (EPHB4) interacts with ephrin B2, thereby inducing POSTN expression, which in turn promotes the expression of p-GSK-3-β (Ser9); this leads to the downregulation of GSK-3-β (Ser9) activity through the Wnt pathway to increase collagen type I, RUNX2, and ALP expression in order to promote the osteogenic differentiation of BMSCs. (2) CTLA4 maintains POSTN expression by regulating the phosphorylation of LDL receptor related protein 6 (LRP6) and activating the Wnt/β-catenin signaling pathway under immune activation conditions to promote RUNX family transcription factor 2 (RUNX2), collagen I, osteocalcin, osterix, and ALP expression, as well as calcium nodule formation in BMSCs. (3) The expression of osteogenic markers in MSCs increases after treatment with soluble POSTN. However, when soluble POSTN is neutralized, these effects are diminished. (4) 17β-estradiol (17β-E2) induces osteogenic differentiation of BMSCs in ovariectomized rats through the POSTN-Wnt/β-catenin pathway to promote osteogenic effects. (B) Periostin and associated diseases [adapted from (23)].
Apart from BMSCs, POSTN also acts in other types of stem cells. For example, POSTN interacts with integrin αv in hematopoietic stem cells (HSCs) to regulate HSC homeostasis in the bone marrow niche (63). POSTN has also been reported to promote the migration and proliferation potential of periodontal ligament mesenchymal stem cells (PDLSCs) via the JNK signaling pathway, revealing the underlying mechanism by which POSTN enhances the function of MSCs (64). POSTN is also expressed in neural stem cells (NSCs), and the interaction between different niches that POSTN mediates is crucial in the adult neurogenic niche (65). Similar to its role in other adult stem cells, POSTN can participate in the regulation of NSC function through integrin signals and promote the proliferation and differentiation of NSCs. Moreover, POSTN supports the regeneration potential of neurons through the maintenance or activation of stem cells (66). Cancer stem cells (CSCs) have been shown to express POSTN via either autocrine or paracrine pathways; this process plays an important role in the functional maintenance of CSCs. For example, POSTN secretion increases the invasiveness of CSCs and promotes metastasis (67). In many cancer models, the expression of POSTN and its receptors also plays a crucial role in tumorigenesis (68).
In general, POSTN is considered to be a key molecule that plays a promoting role in the proliferation, migration, and osteogenic differentiation of MSCs, although these effects may be modulated through the regulation of autocrine or paracrine pathways (64, 69); moreover, POSTN indirectly affects the bone density and quality of the vertebral body, and ultimately participates in the establishment and progression of IVDD. In addition to IVDD, POSTN plays an extremely important role in various other systemic diseases, such as asthma fibrosis, infarcted myocardial scar formation, and tumorigenesis (70). In a recent study, POSTN was shown to promote the progression of B-cell acute lymphoblastic leukemia (B-ALL) by regulating the expression of monocyte chemoattractant protein 1 (MCP-1 or CCL2) in leukemia cells (71). The mechanisms by which POSTN participates in its related diseases are shown in Figure 2B.
Activity of POSTN in Osteoblasts and Osteoclasts
POSTN was originally identified in MC3T3-E1 osteoblasts and was found to play a role in mediating transforming signal response to growth factor-β (TGF-β) and cell adhesion (21). Later, Litvin et al. isolated an isoform of POSTN from osteoclasts called periostin-like factor and found that this played an important role in osteoblast differentiation (72). A recent study analyzed the effects of POSTN on the differentiation of osteoblasts by examining three osteoblast models isolated from the mouse periosteum, calvaria, and MC3T3-E1 cell line. The results showed that Postn was expressed in all three models during the whole differentiation process in a time-dependent manner. In the differentiation process of the calvaria and MC3T3-E1 models, the expression of Postn first appeared on the third day, while resulting detectable only on the seventh day in the mouse periosteum model; furthermore, its expression increased by 3–10 times between the sixth and the 14th day, when it reached its peak. After differentiation was complete and mineralization begun, the expression of Postn rapidly declined (73). Subsequent studies also confirmed that POSTN secreted by osteoblasts participates in the early stages of bone development and bone formation by promoting osteoblast differentiation and survival, and is a key regulator of bone microarchitecture, strength, and mass (55, 74). However, the expression of the osteoblast differentiation markers collagen type I, osteocalcin, osteopontin, ALP, and RUNX2 was decreased in an osteoblast model with Postn knockout. This indicates that inhibition of Postn can suppress cell proliferation and differentiation, resulting in decreased bone mass and strength, decreased collagen cross-linking and demineralization, and increased micro-damage (75).
Nevertheless, the mechanism by which POSTN induces osteoblast differentiation was unknown until Zhang et al. (76) studied the expression of Postn and semaphorin 3A in osteoblasts under mechanical stress in vitro. Semaphorin 3A is an axon-guiding molecule secreted by osteoblasts and osteocytes, and can enhance bone formation, increase bone volume, and accelerate bone regeneration by inhibiting bone resorption (77, 78). It was found that the gene expression of Postn and semaphorin 3A in MC3T3-E1 cells was increased under mechanical stimulation. When an MC3T3-E1 cell culture was treated with recombinant POSTN, the level of semaphorin 3A in its supernatant was significantly increased in a dose-dependent manner, promoting the activation and differentiation of osteoblasts through the Wnt/β-catenin signaling pathway. Moreover, previous studies have confirmed that high levels of mechanical stretch can induce osteoclast apoptosis (79, 80), but the intensity of the apoptosis signal is weakened after POSTN preconditioning. A subsequent study revealed that POSTN expression increased in osteoblast-like MG-63 cells subjected to mechanical stretch through the activation of the TGF-β signaling pathway (81). This finding suggests that POSTN protects osteoblasts from mechanical stretch-induced apoptosis (81).
In the same year, Meng et al. found that apoptosis of osteoblasts was induced by melatonin through the activation of the endoplasmic reticulum stress (ERS)-associated eukaryotic initiation factor 2α (eIF2α)-activating transcription factor 4 (ATF4) pathway; subsequently, the cascade effects of ccAAT/enhanced binding protein homologous protein (CHOP), caspase 3, and c-Jun N-terminal kinase (JNK) were triggered to promote apoptosis. Similarly, overexpressed POSTN can act as a protective factor for osteoblasts because it can hamper melatonin-induced apoptosis by inhibiting the eIF2α-ATF4 pathway through the suppression of the protein kinase R-like endoplasmic reticulum kinase (PERK) pathway (82).
In addition, Qin et al. constructed a postn knockout (KO) model in MC3T3-E1 osteoblasts using a lentiviral vector to study the effect of Postn inhibition on autophagy in osteoblasts. The results showed that the expression of the autophagy-related protein beclin 1 in osteoblasts was increased, suggesting that the autophagy function of osteoblasts was enhanced. This also indicates that POSTN inhibits autophagy in osteoblasts, although the specific signaling pathway mediating this effect has not yet been identified (83). In a subsequent study, these authors demonstrated that the protein expression of RUNX2 and RANKL was significantly reduced after Postn gene silencing, and speculated that this process was achieved through the NF-κB signaling pathway (84). The mechanisms of action of POSTN in osteoblasts are shown in Figure 3.
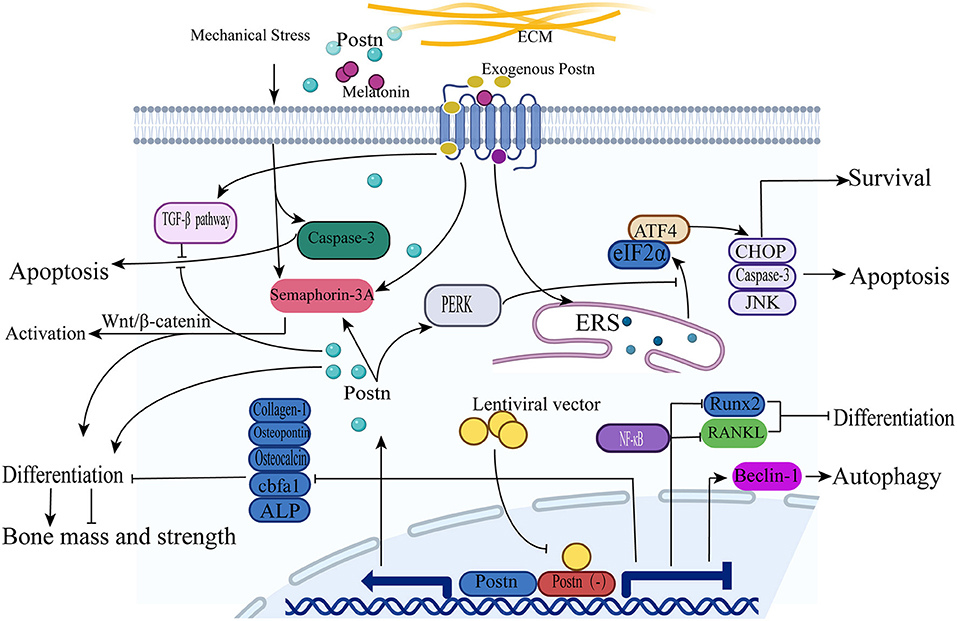
Figure 3. POSTN induces osteoblast differentiation, survival, and apoptosis. (1) Upon treatment with recombinant POSTN, the expression of semaphorin 3A is increased and promotes the activation and differentiation of osteoblasts through the Wnt/β-catenin signaling pathway. (2) The intensity of osteoclast apoptosis signals is weakened by POSTN expression through the TGF-β signaling pathway under a high level of mechanical stress. (3) The expression of the autophagy-related protein beclin 1 in osteoblasts is increased after Postn silencing. (4) The expression of the proteins RUNX2 and RANKL is significantly reduced after Postn gene silencing through the activation of the NF-κB signaling pathway. (5) Osteoblast apoptosis is induced by melatonin through the activation of the ERS-eIF2α-ATF4 pathway; subsequently, the cascade effects of CHOP, caspase 3, and JNK are triggered to promote apoptosis. POSTN overexpression can inhibit the eIF2α-ATF4 pathway by suppressing the PERK pathway to protect osteoblasts from apoptosis.
According to the literature, there appears to be no relationship between POSTN expression and osteoclasts (75, 85). In fact, although cortical bone remodeling was increased in postn KO mice at 1 year of age, there was no change in osteoclast activity and number in long bones of postn KO mice (86). However, subsequent studies have redefined the relationship between POSTN and osteoclasts, and Postn has been shown to be expressed at low levels in mouse long-bone osteoclasts differentiated in vitro (73). Moreover, the number and activity of wild-type osteoclasts co-cultured with postn KO mouse primary osteoblasts were higher than those of wild-type osteoblasts co-cultured with postn KO mouse primary osteoblasts. In turn, the addition of recombinant POSTN to osteoclast cultures reduced the number and activity of osteoclasts (87).
POSTN Exerts an Anti-apoptotic Effect on Osteocytes and Affects Bone Metabolism
Although POSTN is not expressed in bone cells (88), the relationship between POSTN and bone cells has been demonstrated by several studies. For instance, POSTN has been shown to interact with Notch receptors and to exert an anti-apoptotic effect on osteocytes under mechanical stress (89). Similarly, intraperitoneal injection of a high dose of zoledronate sodium into wild-type and postn KO mice treated with a high dose of zoledronate for 3 months reduced the number of osteocytes in both groups, but the number of empty osteocyte lacunae increased in the latter; this result confirmed that POSTN exerts an anti-apoptotic effect in osteocytes (85). POSTN also plays a vital role in bone formation, and in determining its mass and density (90). The observation that the quality of cortical bone decreases due to the reduction of periosteal bone formation in a postn KO mouse model supports this view (55, 91). Another strong piece of evidence is that in mouse strains where Wnt16 gene KO leads to a decrease in cortical bone mass, the expression of POSTN in periosteal cells is reduced (92). POSTN expression also modulates bone homeostasis (93).
The mechanism by which POSTN influences bone formation, quality, metabolism, and density has been revealed in follow-up studies. For example, POSTN directly stimulates the Wnt signaling pathway and osteoblast function while inhibiting the expression of sclerostin (SOST) to mediate the anabolic response of the bone to parathyroid hormone. Therefore, defective POSTN expression impairs bone formation, as parathyroid hormone cannot effectively improve its cortical structure and strength. Moreover, POSTN expression may be involved in the remodeling of bone cells near the inner cortical ridge (91).
In addition, recent data indicated that cathepsin K, a protease involved in the process of bone resorption, may also modulate periosteal bone formation by regulating POSTN expression (94, 95). In this context, the drug odanacatib, a cathepsin K inhibitor, is effective in reducing osteoporotic fractures and stimulating the formation of periosteal bone in ovariectomized adult monkeys (96). In another study, the anti-osteoporosis drug teriparatide, an anabolic drug that promotes bone remodeling in patients with osteoporosis, was found to trigger the secretion of POSTN to promote bone formation and counteract osteoporosis. Moreover, after teriparatide treatment, the level of POSTN in the circulation of patients increased significantly (97). Finally, POSTN plays a different role in each stage of bone repair: in the early stage of repair, it activates periosteal stem cells; during the active period, it can improve the deposition of fracture callus cartilage and bone; in the final stage, it induces bone bridging and rebuilding of the stem cell pool in the periosteum (98, 99).
In summary, these studies effectively illustrated that POSTN is associated with bone mass, density, and metabolism as it indirectly affects osteocyte apoptosis, although it is not directly expressed in osteocytes. Moreover, POSTN is not only a regulator of bone formation and bone mineral density but also a potential biomarker for osteoporosis and fracture. It plays an irreplaceable role in the onset and development of vertebral degeneration, for instance during osteoporosis or upon osteoporotic fracture.
The Activity of POSTN in IVD Cells
IVD cells can be distinguished into three main groups: NP cells, AF cells, and superior and inferior endplate chondrocytes. Altogether, these cells constitute the IVD, a flexible joint localized between adjacent spinal vertebrae (2, 100). The tough AF is the circular exterior of the IVD and surrounds the inner soft NP. Its function is to prevent the NP from herniating or leaking out of the disc by sealing the nucleus and distributing any pressure and force imposed on the IVD (101, 102). The elastic NP is mainly composed of water (70–90%), NP cells, proteoglycan, and type II collagen (3). When the spine moves, the spherical structure of the NP contributes significantly to dispersing pressure, supporting large-angle and high-frequency movement, and assisting other parts of the spine in the completion of physiological activities (103, 104). Finally, it has been suggested that the cartilage endplates absorb the small molecules and nutrients required for disc cells (39). In summary, the dysfunction of IVD cells causes loss of balance and reduces the effect of balancing pressure, leading to decreased function of the IVD and its eventual degeneration. In the following sections we describe the role of POSTN in each of the three above-mentioned IVD cell types, which mediates its indirect influence on the occurrence and development of IVDD.
POSTN Induces Chondrocyte Apoptosis and Cartilage Degradation
Although the mechanisms involved in the binding of POSTN to the cartilage endplate of the IVD have not been analyzed in detail, studies on cartilage of patients with osteoarthritis (OA) have extensively reported that POSTN can interact with chondrocytes and enhance cell proliferation and survival, migration, and metastasis. For example, Chen et al. (105), while exploring the molecular differences between light and dark hypertrophic chondrocytes by qPCR, found that the most highly differentially expressed dark cell-specific gene was Postn. Other studies also detected a significant increase in the protein expression of POSTN between OA and normal chondrocytes by immunohistochemistry (106, 107). Moreover, the POSTN isoforms 1 and 8 have been reported to be significantly highly expressed in chondrocytes, whereas others are mainly expressed in anterior cruciate ligament remnants, suggesting that differential expression patterns of POSTN and its splice variants exist in various tissues and cell types (19). POSTN was also found to contribute to the maturation and shape retention of tissue-engineered cartilage by promoting conformational changes in collagen molecules during tissue engineering (108). This newly discovered effect of POSTN could be useful in clinical applications in the future. These findings demonstrated that POSTN is not only expressed in chondrocytes and synovial fluid (SF) from OA patients but may also be a potential biomarker and contributor to the progression of OA. Most importantly, POSTN also has potential value for applications in tissue repair and regeneration.
With the deepening of research, especially on the role of chondrocytes in OA, a large number of studies have revealed the role and mechanism of POSTN activity in chondrocytes. For example, a recent study reported that POSTN is upregulated in human and rodent OA chondrocytes. To promote OA, POSTN induces the expression of matrix metallopeptidase 13 (MMP13) and ADAMTS4 in a dose- and time-dependent manner. Moreover, MMP13 levels are regulated by endogenous POSTN, because when the Postn gene was inhibited by siRNA transfection, the levels of both endogenous POSTN and MMP13 in chondrocytes were reduced. This process was accomplished through the selective activation of Wnt/β-catenin signaling without the activation of the ERK1/2, p38, and NF-κB pathways (109). However, another study in the same year showed that the upregulation of IL-6, IL-8, MMP1, MMP3, MMP13, and nitric oxide synthase 2 (NOS2), which are induced by POSTN and known to be related to OA pathogenesis, was suppressed by NF-κB inactivation in chondrocytes. It has been suggested that POSTN triggers the activation of the NF-κB signaling pathway, denoted by the nuclear translocation of p65, and subsequently upregulates inflammatory factors and MMPs to induce chondrocyte apoptosis and accelerate cartilage degeneration (110). In human OA synovial cells, researchers not only detected higher gene and protein expression of POSTN than in normal cells, but also observed increased secretion of POSTN under the stimulation of the inflammatory cytokine IL-13, suggesting that both POSTN and IL-13 are involved in the pathological progression of OA. Mechanistically, IL-13 promotes the phosphorylation of signal transducer and activator of transcription 6 (STAT6) and activates STAT6 to upregulate POSTN expression. Moreover, POSTN expression was significantly inhibited by dexamethasone and leflunomide (111, 112). Although these results are inconsistent with the hypothesis of the selective activation of the Wnt/β-catenin signaling pathway, the results of POSTN overexpression in OA chondrocytes and SF are consistent with the previous hypothesis.
In addition, Chinzei et al. (113) studied the effects of anabolic (TGF-β1) and catabolic (IL-1β) factors on the expression of POSTN and found that the expression of POSTN did not change after treatment with either TGF-β1 or IL-1β. However, these factors affected chondrocyte metabolism. These authors also observed that exogenous POSTN treatment of chondrocytes stimulated the expression of MMP13, but did not alter Postn gene expression, while siRNA-mediated POSTN ablation inhibited expression of the POSTN and MMP13 genes, consistent with prior results (113). These findings suggest that exogenous POSTN can act as a catabolic factor promoting cartilage degradation. Subsequent studies revealed the following mechanism: POSTN-induced upregulation of MMP13 requires its interaction with and activation of discoidin domain receptor 1 (DDR1). DDR1 is a receptor tyrosine kinase that signals through the AKT-Wnt/β-catenin pathway to induce the degradation of collagen and proteoglycans in the cartilage (114).
In summary, POSTN, previously reported as an osteoblast-specific factor, has been shown to be upregulated in both human and rodent OA chondrocytes. In addition, it is a catabolic factor promoting collagen and proteoglycan degradation to accelerate the pathogenesis of OA, and may act as a novel therapeutic target to prevent OA progression. The mechanisms of POSTN-mediated chondrocyte apoptosis and ECM degradation leading to cartilage degeneration and promotion of OA are shown in Figure 4A.
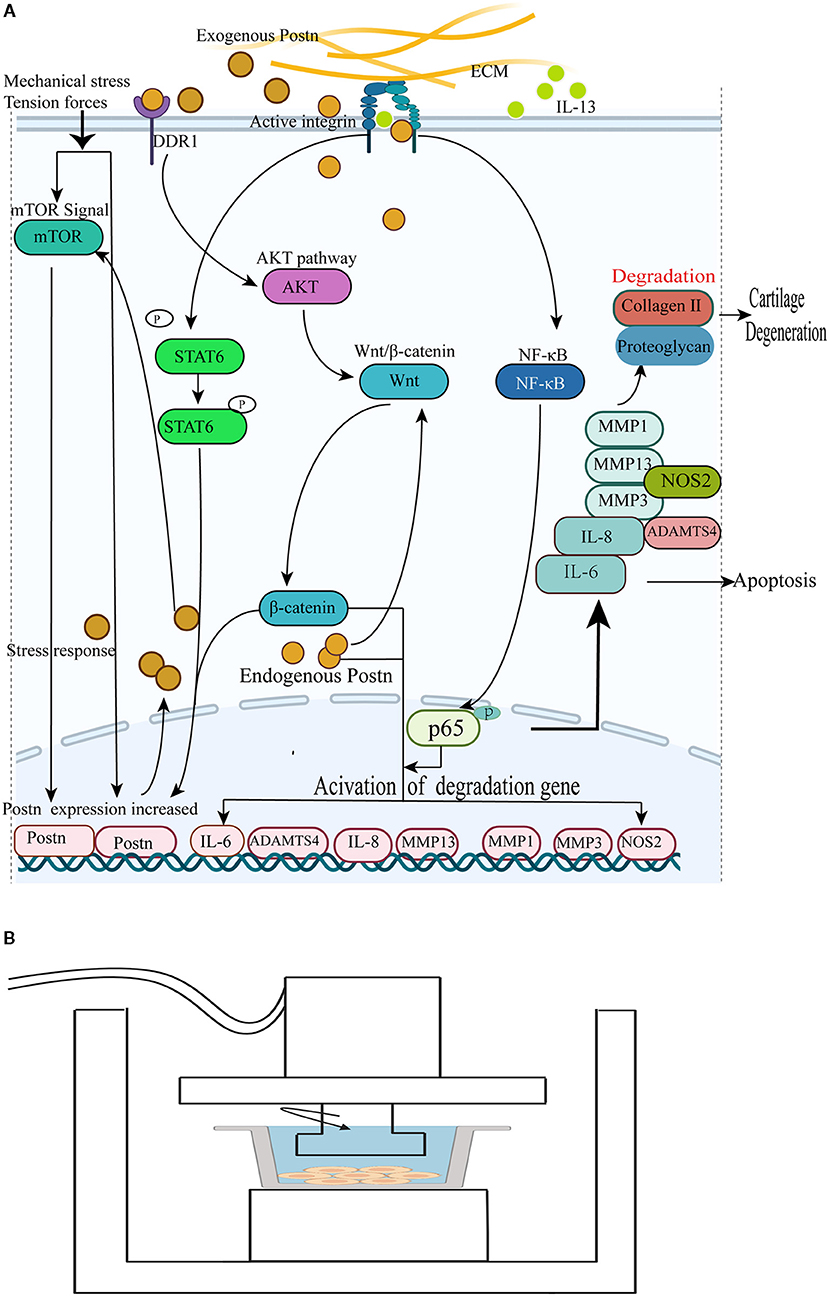
Figure 4. (A) POSTN promotes collagen and proteoglycan degradation to accelerate cartilage degeneration and loss. (1) Endogenous POSTN can promote the expression of MMP13 and ADAMTS4 by activating the canonical Wnt/β-catenin signal pathway to accelerate cartilage degeneration and chondrocyte apoptosis. (2) POSTN leads to the activation of the NF-κB signaling pathway, denoted by the nuclear translocation of p65, and subsequently upregulates IL-6, IL-8, MMP1, MMP3, MMP13, and NOS2 to induce chondrocyte apoptosis and accelerate cartilage degeneration. (3) IL-13 promotes the phosphorylation of STAT6 and activates STAT6 to upregulate POSTN expression, contributing to the pathological progression of OA. (4) Exogenous POSTN-induced upregulation of MMP13 requires its interaction with and activation of DDR1 to induce the degradation of collagen and proteoglycans in the cartilage through the AKT-Wnt/β-catenin pathway. (5) Mechanical stress can induce POSTN expression to increase chondrocyte apoptosis. The expression of POSTN increases in response to tendon forces via the mTOR pathway; in turn, POSTN enhances mTOR signals. (B) Schematic diagram of a mechanical stress induction device. The arrow indicates the direction of the mechanical force generated by the device. The gray line represents a culture dish with a monolayer of nucleus pulposus cells (115).
Expression of POSTN in Annulus Fibrous and Nucleus Pulposus Cells
POSTN is predominantly expressed in collagen-rich fibrous connective tissues that are subjected to constant mechanical stress (17). Because of this characteristic, one could speculate that POSTN is also expressed in the IVD, especially in AF and NP cells. As expected, through the immunolocalization of POSTN in 11 specimens of surgical or donor lumbar IVD tissues, a study revealed that POSTN exists in the cytoplasm of some cells in the human inner and outer rings, as well as in the NP (18). The greatest proportion of POSTN-expressing cells was present in the outer annulus (88.8%), while the inner annulus tissue and the NP contained an average of 61.4 and 18.5% POSTN-expressing cells, respectively. Similar cellular patterns of POSTN localization have been observed in lumbar discs from sand rats (18). Moreover, additional studies have shown that the percentage of POSTN-positive cells in the inner ring is significantly negatively correlated with the age of the subjects (7, 18). However, a later study demonstrated that there is a positive relationship between POSTN expression and NP cell degeneration. Its authors measured POSTN gene expression in degenerative and non-degenerative NP cells by PCR, and the results showed that the mRNA and protein levels of POSTN increased in the degeneration group. Histological examination showed that, compared with non-degenerative IVD, human IVD displayed more fibrosis together with structural disorders and fragments. Finally, POSTN positive staining increased in human tissues and degenerated IVD of rat tails subjected to acupuncture (115).
Recently, using microarray and bioinformatics analyses, abnormal gene expression during aging of rat NP cells has been observed; among downregulated genes, Postn was the most significantly altered, a result confirmed by real-time PCR. This finding contradicts the results of previous studies showing that the mRNA and protein level of POSTN in IVD cells increases during IVDD (115, 116). The authors explained such discrepancy by discussing that the acupuncture mouse model represents a rapid damage of the IVD, which stimulates the expression of POSTN to regulate the structure of the ECM. However, the model in their experiment was natural aging, which is more similar to the process of NP degradation. Consistent with these results, Graja et al. (117) reported that POSTN loss in aging adipose tissue is closely related to its age-related ECM changes. In addition, bioinformatics analysis revealed that the KEGG pathways that were enriched with the highest score in the gene module relative to the NP cell aging process were “ECM–receptor interaction,” “local adhesion,” and “PI3K-Akt signaling pathway”; however, more experiments are needed to confirm these results (116, 118).
In summary, these studies have demonstrated the relationship between POSTN expression and IVDD, and the potential mechanism of NP cell senescence has been studied on a genome-wide scale. This suggests that POSTN may be a promising new prognostic marker and therapeutic target, and further studies are needed to expand our understanding of its role in IVDD. However, it is regrettable that, despite reports that POSTN is highly expressed in the AF, there is a lack of further literature on this topic.
The Role of POSTN in the Maintenance of the Extracellular Matrix
In addition to the well-known NP cells, annulus fibroblasts, and endplate chondrocytes, the IVD also includes ECM components. The ECM is a mixture of molecules secreted by cells and provides biochemical and structural support for cells, tissues, and organs (11). A large number of existing studies have demonstrated that the occurrence and development of IVDD is closely related to excessive ECM catabolism. An increasing amount of direct genetic evidence links the development, maintenance, and repair of the IVD with the coordinated interaction of the ECM components in the respective IVD niches (119). Therefore, to delay the progression of IVDD, it is necessary not only to regulate the function of NP cells but also to restore the metabolic balance of the ECM (120). Thus, it has been suggested that the ECM may be a new biomarker for the treatment of IVDD or a major candidate target for treatment.
POSTN is an ECM protein composed of multiple domains, as described previously (20). It has different physiological functions in tissues when combined with other ECM molecules. It has been proven that POSTN can not only serve as the basis of complex ECM architecture, but also be used as a scaffold for assembling ECM and accessory proteins through its multi-domain structure (29, 121, 122). These distinct domains have been shown to bind to many proteins, including ECM proteins (collagen types I and V, fibronectin, tenascin, and laminin), matricellular proteins (CCN3 and βig-h3), and enzymes that catalyze covalent cross-linking between ECM proteins (lysyl oxidase and BMP-1) (9, 24). For example, Kii et al. (27) observed that the proximal end of POSTN binding to fibronectin is located in the endoplasmic reticulum of fibroblasts. Moreover, POSTN can enhance the secretion of fibronectin from the endoplasmic reticulum to the extracellular environment. It was revealed that POSTN interacts directly with fibronectin before secretion and plays an important role in fibronectin secretion.
POSTN has also been found to interact with collagen and form a complex with type I collagen. It can also directly bind to type V collagen and plays an important role in the formation of collagen fibers (16, 17). Although the binding site of collagen has not been determined, the process of collagen fiber formation is important for tissue repair and IVDD. In this process, the interaction of POSTN with fibronectin is a key factor (123). Moreover, tenascin C interacts with and binds to the FAS1 domain of POSTN. Tenascin C is an ECM protein that can form six-arm oligomers by multimerization through disulfide bonds at its amino terminus (29, 124). In the process of collagen production, POSTN and intracellular tenascin C form a network structure that combines with fibronectin to form a cross-linked type I collagen scaffold (9).
In addition, POSTN interacts with BMP-1, a pro-collagen processing enzyme that removes the carboxy-terminal propeptide of several proteins (125). Experiments by Maruhashi and Hwang et al. showed that POSTN directly binds to and interacts with BMP-1 (28, 126). The result of this interaction is the enhanced proteolytic activation of lysine oxidase (LOX), an enzyme that catalyzes the intermolecular cross-linking of collagen molecules and is essential for the formation of collagen fibers (127). Interestingly, LOX can also be connected to the EMI domain of POSTN via fibronectin (126, 128).
Finally, Takayama et al. (33) proved that POSTN can interact with CCN3 to enhance its deposition in the ECM. CCN3 includes four domains: an insulin-like growth factor-binding protein-like domain (IGFBP), a von Willebrand type C-like domain (VWC), a thrombospondin type 1-like domain (TSP1), and a carboxy-terminal domain (CT). In this assembly process, the TSP1 and CT domains of CCN3 interact with the EMI domain of POSTN.
In addition to the proteins described above, POSTN has been reported to interact with members of the integrin superfamily. Although direct evidence of the interaction between POSTN and integrins has not been provided, POSTN is believed to interact with integrins, including αVβ3, αVβ5, and α6β4, that promote cell proliferation, cell migration, epithelial-to-mesenchymal transformation, and modulation of the biomechanical properties of connective tissues (63, 129, 130).
In conclusion, POSTN interacts with multiple ECM proteins, as shown in Table 1 and Figure 1. POSTN interacts with a variety of ECM proteins, as shown in Table 1 and Figure 1, possibly anchoring them to the ECM. It may contribute to IVD structure by interacting with different ECM and accessory proteins to maintain their stability and metabolic balance; in turn, these proteins maintain the conditions for the pathophysiological functioning of IVD tissues, and participate in tissue regeneration and fibrosis after injury.
POSTN Responds to Mechanical Stress
The magnitude and duration of mechanical stress is positively correlated with the rate of IVD cell apoptosis, which is an important factor leading to IVDD and herniation (4). POSTN, taking its first name from the periosteum and periodontal ligament, is considered sensitive to mechanical stress because the supraosseous periosteum and periodontal ligament can promptly respond to mechanical stress to help tissue regeneration and development (131, 132). A study by Rani et al. confirmed that stress or pressure overload can induce the expression of POSTN, and that IVD overuse and injury are associated with its higher expression (133).
In osteocytes, the degradation of POSTN by cathepsin K plays a central role in the regulation of the biomechanical response in bone tissues and controls cortical bone formation (134). Moreover, Bonnet et al. (135) demonstrated that cortical bone microarchitecture and bending strength related to vertebral degeneration were closely altered in Postn-deficient mice. In the cartilage, POSTN contributed to retaining the shape of a biodegradable polymer scaffold by increasing the mechanical strength of the surrounding fibrous tissues, consisting of a POSTN-mediated collagen structure (136), and enhanced chondrogenesis in a 3D culture embedded within collagen gel; this indicates that conformational changes in collagen induced by POSTN can alter cell adhesion to the substratum and affect signal transduction in chondrocytes (108, 137).
In addition, Stansfield et al. applied a model, as shown in Figure 4B, to investigate the effect of stress on POSTN expression in human NP cells. Following stimulation by mechanical stress for 6 h, the effects of shear stress on the expression of POSTN in degenerative and non-degenerative NP cells were examined by RT-PCR (115). The results revealed that the expression of POSTN and MMP2 was upregulated by mechanical stress, with a significantly higher magnitude of response in human degenerative NP cells compared with non-degenerative cells. This provided an explanation for the susceptibility of degenerative disc cells to stress-induced damage (115). Moreover, Rosselli-Murai et al. (138) found that both POSTN and mammalian target of rapamycin (mTOR) are coordinately upregulated by tension forces during wound healing to induce cell proliferation and migration, indicating that the same signal derived from tendon forces activates the expression of both POSTN and mTOR; in turn, POSTN enhances mTOR signaling.
In conclusion, POSTN expression either promotes or inhibits cellular biological functions to stimulate or hamper irregular collagen fibrillogenesis and ECM organization, in order to maintain or abolish tissue homeostasis in response to mechanical stress, as shown in Figure 4A.
Pro-Inflammatory Cytokines Inducing POSTN Expression
It is well-known that hypoxia and inflammation are two important characteristics of the environment of IVDD (139). Indeed, high levels of pro-inflammatory cytokines such as tumor necrosis factor (TNF)-α, interleukin (IL)-1 α/β, IL-6, and IL-17 secreted by disc cells induce degeneration of the IVD, which is the major contributor to back/neck and radicular pain (140, 141). These cytokines promote matrix degradation, chemokine production, and changes in cell phenotype (40, 142). Among these, IL-1β and TNF-α are the most important pro-inflammatory cytokines in the inflammatory process because they exert powerful pro-inflammatory activities and can promote the secretion of a variety of pro-inflammatory mediators (143, 144).
Originally, POSTN was identified as a TGF-β-inducible gene as its expression increased in a TGF-β-dose-dependent manner (21). Attur et al. (109) found that TGF-β1 can induce POSTN expression in a time-dependent manner, whereas this is inhibited by IL-1β. POSTN was then found to be induced by various stimuli, including IL-4, IL-13, TNF-β, angiotensin II, connective tissue growth factor 2, BMP-2, and cancer-derived factors (70, 145). Upon stimulation with TNF-α, wild-type and Postn-deficient fibroblasts showed different behaviors. Indeed, the expression of chemokines was significantly upregulated in wild-type fibroblasts, whereas the expression of Ccl2, Ccl7, Cxcl1, and Cxcl2 was markedly downregulated in Postn-deficient fibroblasts (146). This is sufficient and direct evidence of an association between inflammatory cytokines and POSTN expression.
In subsequent studies, POSTN was found to be associated with many chronic inflammatory diseases due to its interaction with inflammatory cytokines. In particular, it was found that POSTN can cooperate with TNF-α or IL-1α derived from epithelial cells or inflammatory cells to activate the NF-κB pathway in fibroblasts, leading to the subsequent induction of various chemokines/inflammatory cytokines including IL-1β, which recruits neutrophils and macrophages and accelerates pulmonary fibrosis (147, 148). Furthermore, the pro-inflammatory cytokines TNF-α and IL-17 work synergistically to enhance the expression of POSTN; this suggests a potential role of POSTN in liver inflammatory injury that eventually leads to fibrosis (149). Not just limited to the liver, the synergistic effect of TNF-α and IL-17 has also been noted in other inflammatory diseases, such as psoriasis, in which it leads to altered keratinocyte differentiation (150). In myocardial ischemia-reperfusion injury (MIRI), POSTN overexpression promotes caspase 1-mediated pyroptosis by activating NLRP3 (151).
Moreover, another study showed that TNF-α inhibited the expression of POSTN at the protein level in PDLSCs (64). POSTN can promote the migration and osteogenic differentiation of PDLSCs by activating the JNK signaling pathway in response to TNF-α challenge. POSTN is also highly expressed during chronic inflammatory diseases such as asthma (152), atopic dermatitis (153), eosinophilic chronic sinusitis/chronic rhinosinusitis with nasal polyps (154), and allergic conjunctivitis (155), and plays important roles in the pathogenesis of these diseases. For instance, POSTN has emerged as a novel biomarker for type 2 inflammation in allergic diseases (148, 154). The interaction between POSTN and inflammatory factors mediates many chronic inflammatory responses; its mechanism of action in this process is shown in Figure 5.
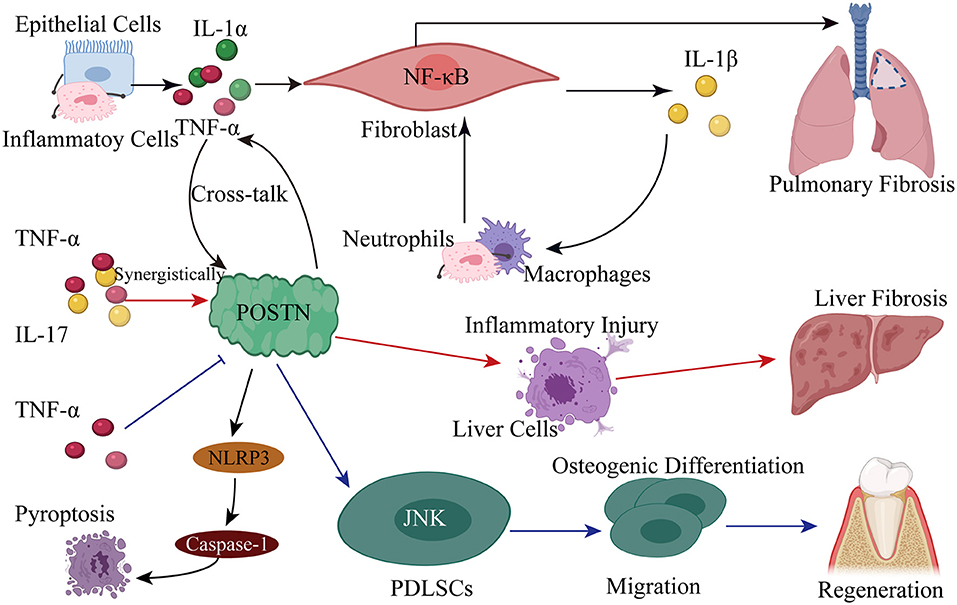
Figure 5. Pro-inflammatory cytokines induce POSTN expression, revealing the underlying mechanism of many chronic inflammatory diseases. (1) POSTN cross-talks with TNF-α or IL-1α derived from epithelial cells or inflammatory cells to induce various chemokines/inflammatory cytokines, including IL-1β, by activating the NF-κB pathway in fibroblasts; this leads to the recruitment of neutrophils and macrophages, which accelerates pulmonary fibrosis. (2) The pro-inflammatory cytokines TNF-α and IL-17 work synergistically to promote the expression of POSTN in order to induce liver inflammatory injury eventually leading to fibrosis. (3) POSTN overexpression promotes caspase 1-mediated pyroptosis by activating NLRP3 in MIRI. (4) POSTN promotes the migration and osteogenic differentiation of PDLSCs by activating the JNK signaling pathway in response to inflammatory stimuli.
In addition, when Zhao et al. (156) studied the mechanism of CYP1B1 mutation and congenital glaucoma, they found that Cyp1b1−/− mice presented ultrastructural irregular collagen distribution in their trabecular meshwork tissue along with increased oxidative stress and decreased levels of POSTN. Interestingly, Postn-deficient mice exhibited similar ultrastructural abnormalities in the trabecular meshwork tissue of Cyp1b1−/− mice. Therefore, they concluded that the metabolic activity of CYP1B1 contributes to the oxidative homeostasis and the ultrastructural organization and function of TM tissue by regulating the expression of POSTN. In another in vitro study, Wu et al. (157) found that oxidative stress-induced periosteal protein is involved in myocardial fibrosis and hypertension. And in a recent study, Liu et al. (158) found that POSTN can inhibit hypoxia-induced oxidative stress and apoptosis in human periodontal ligament fibroblasts via p38 MAPK signaling pathway.
However, to date, there is still no description of the relationship between POSTN and pro-inflammatory cytokines and hypoxia-induced oxidative stress in IVD cells. Although research on how POSTN and pro-inflammatory cytokines lead to IVDD is still lacking, the cross-talk of POSTN with TNF-α and IL-1β, which are the most potent inflammatory cytokines, has been suggested as the underlying mechanism of many chronic inflammatory diseases. In this regard, we can learn from previous studies to outline the possible role of POSTN in the pathophysiology of IVDD.
POSTN Interacts With Signaling Pathways in IVDD
Current knowledge about POSTN signaling mainly comes from studies aiming to elucidate its role in BMSCs, osteoblasts, chondrocytes, as well as its interaction with inflammatory cytokines and mechanical stimulation. Mechanical stress, chemokines, and changes in ECM composition trigger signaling pathways that induce POSTN expression and subsequent secretion. As a potent modulator of cell–matrix interactions, POSTN has been implicated in the cross-talk between multiple signaling pathways that regulate cell migration, adhesion, and proliferation.
First, the most studied pathway associated with POSTN expression is the TGF-β pathway in osteoblasts cells, in which POSTN acts as a focal contributor of osteogenesis and differentiation in response to mechanical stress (21). Moreover, the binding of POSTN to integrins initiates a cross-talk between integrins and receptor tyrosine kinases, such as EGFR, at the plasma membrane. This crosstalk activates the Akt signaling pathway (159). Akt functions as a cardinal node for the transduction of extracellular and intracellular signals. It is now generally accepted that the PI3K/Akt survival pathway is a central regulator of cell survival and proliferation (160, 161). However, this pathway interacts with the Wnt/β-catenin pathway to induce collagen and proteoglycan degradation in the cartilage (114). POSTN is also capable of activating canonical Wnt/β-catenin signaling in BMSCs and chondrocytes, resulting in induced MMP13 and ADAMTS4 expression to accelerate the pathogenesis of OA, and mediates estrogen-induced osteogenic differentiation of BMSCs in ovariectomized rats to reduce osteoporosis (60, 61, 109).
Not surprisingly, POSTN expression has been shown to activate NF-κB (84, 110, 147, 148), JNK (64), and PERK (82) signaling in BMSCs, osteoblasts, and chondrocytes during development and disease. Finally, mechanical stress activates mTOR signaling to increase the expression of both POSTN and mTOR; in turn, POSTN enhances mTOR signaling (138). In addition, POSTN has been reported to induce MMP2 expression via the avβ3 integrin/ERK pathway in human periodontal ligament cells (162). In a preclinical in vivo murine model of human melanoma, POSTN promoted angiogenesis by interacting with integrins avβ3 and avβ5 via its FAS1-2 domain (163). The signaling pathways interacting with POSTN and their effects are shown in Table 2.
Potential of POSTN as a Biomarker
POSTN has been intensively studied as a biomarker for inflammatory and allergic diseases. In addition to its role in respiratory diseases such as asthma and allergic pneumonia (147, 148), it was found that plasma and SF POSTN levels were positively correlated with the radiographic severity of knee OA, and POSTN expression was increased in synoviocytes and SF from OA patients (106, 164). Moreover, a clinical study directly showed that serum POSTN (sPOSTN) levels were associated with the prevalence and risk of development and progression of knee OA in women (165). Additionally, POSTN variants are also associated with the course and severity of juvenile idiopathic arthritis, according to the literature (166).
Significantly, an increasing amount of information is available about the role of POSTN in fractures and osteoporosis, which is the most common age-related bone disease characterized by bone resorption (50). A longitudinal case-control study of women over 70 years of age with acute osteoporotic hip fractures suggested that high levels of sPOSTN are associated with bone density loss, because women with hip fractures exhibited higher sPOSTN levels (167). Furthermore, high sPOSTN levels were regarded as independent predictors of femoral neck bone mineral density in older women with acute hip fractures (168). In addition, sPOSTN levels were higher in ankylosing spondylitis patients with higher disease activity and higher systemic inflammation and were independently associated with C-reactive protein levels (169). This study has shown for the first time a positive association among POSTN expression, inflammatory markers, and disease activity. Finally, sPOSTN levels are even increased in fractures during the early healing phase, possibly implying that POSTN plays a role in bone repair (167). When vertebral and non-vertebral fractures were analyzed separately, it was found that POSTN levels were associated only with the latter fracture type, in agreement with the predominant role of POSTN in cortical bone metabolism established by a study on the role of sPOSTN in postmenopausal women (87, 168).
Therefore, in view of the characteristics of POSTN in clinical diseases, an increasing number of scholars have proposed POSTN as a biomarker for predicting the severity of OA and diagnosing osteoporosis and the outcome of fracture repair (170, 171).
Conclusion and Perspectives
In this article, we described the roles of POSTN in BMSCs, osteoblasts, osteocytes, and IVD cells, as well as its interactions with inflammatory cytokines and mechanical stimulation, and the potential of POSTN in acting as a biomarker of some diseases. POSTN was originally identified in an osteoblastic cell line (20) and its expression was found to be prominent in the bone periosteum, indicating its involvement in bone tissue development and function (21). POSTN plays a critical role in regulating bone microarchitecture, strength, and mass by promoting BMSC and osteoblast differentiation and survival, and regulating collagen fibrillogenesis and ECM assembly (62, 122). On the other hand, increased POSTN expression could contribute to bone mass protection in hyperparathyroidism (91). Furthermore, POSTN is present in disc cells and can promote the degradation of cartilage ECM and induce chondrocyte apoptosis (109, 110). Finally, the cross-talk of POSTN with pro-inflammatory cytokines reveals the underlying mechanism of many chronic inflammatory diseases (40, 142, 149).
Although the study of POSTN has developed dramatically in the past several years, several problems connected to its role in IVDD have remained unresolved: (a) POSTN expression has been officially reported in NP, AF, and osteoclasts. However, almost nothing is known about the mechanism by which POSTN interacts with these cell types to mediate degeneration. (b) Increased POSTN expression was observed in both SF and serum of patients with higher disease activity and higher systemic inflammation, and thus this gene can potentially be used as a biomarker. Therefore, there is a relationship between sPOSTN levels and the Pfirrmann grade of spinal degeneration. In brief, sPOSTN can also be used as a biomarker for spinal degenerative diseases. (c) Many studies have suggested that inflammation is an important factor that leads to herniation, hypoxia, and IVDD. Therefore, it is important to investigate the underlying mechanism by which POSTN responds to inflammatory cytokines during IVDD. (d) POSTN is sensitive to mechanical stress. POSTN expression is increased under mechanical stress as well as under overstimulation and injury. In the process of IVDD, the apoptosis of NP cells caused by external mechanical stress is the most important; therefore, the role of POSTN in the process of mechanical stress-induced apoptosis of NP cells needs further study. However, studies in these areas have been lacking so far. We hope that in the near future novel studies will provide an improved and broader understanding of POSTN activity in the pathogenesis of spinal degenerative diseases, yielding useful diagnostic or therapeutic agents to counteract them.
Author Contributions
DZ, ZW, and WZ contributed equally to the writing of this article, conceived and designed the study, and drafted the manuscript. YW, ML, and GZ referenced manuscript analysis. XG and XK edited and revised the manuscript. All authors contributed to the article and approved the submitted version.
Funding
This work was supported by the special fund project for doctoral training program of Lanzhou University Second Hospital (YJS-BD-09), Lanzhou University Innovation and Entrepreneurship Cultivation Project (cxcy201906), the Chinese Medicine Administration Research Project of Gansu province (GZK-2019-46), the Cuiying Technology Innovation Project of Lanzhou University Second Hospital (CY2019-MS10), and Key R&D Program of Ningxia Hui Autonomous Region (General Project) (2019BEG03031).
Conflict of Interest
The authors declare that the research was conducted in the absence of any commercial or financial relationships that could be construed as a potential conflict of interest.
Publisher's Note
All claims expressed in this article are solely those of the authors and do not necessarily represent those of their affiliated organizations, or those of the publisher, the editors and the reviewers. Any product that may be evaluated in this article, or claim that may be made by its manufacturer, is not guaranteed or endorsed by the publisher.
Abbreviations
AF, Fibrous annulus; BMSCs, Bone-marrow-derived mesenchymal stem cells; CTD, C-terminal end; ECM, Extracellular matrix; HSCs, Hematopoietic stem cells; IVDD, Intervertebral discs degeneration; IVD, Intervertebral discs; MMP, Matrix metalloproteinase; NP, Nucles pulposus; OA, Osteoarthritis; POSTN, Periostin.
References
1. Vadalà G, Russo F, Di Martino A, Denaro V. Intervertebral disc regeneration: from the degenerative cascade to molecular therapy and tissue engineering. J Tissue Eng Regen Med. (2015) 9:679–90. doi: 10.1002/term.1719
2. Molladavoodi S, McMorran J, Gregory D. Mechanobiology of annulus fibrosus and nucleus pulposus cells in intervertebral discs. Cell Tissue Res. (2020) 379:429–44. doi: 10.1007/s00441-019-03136-1
3. Roberts S, Menage J, Duance V, Wotton S, Ayad S. 1991 Volvo Award in basic sciences. Collagen types around the cells of the intervertebral disc and cartilage end plate: an immunolocalization study. Spine. (1991) 16:1030–8. doi: 10.1097/00007632-199109000-00003
4. Lotz JC, Chin JR. Intervertebral disc cell death is dependent on the magnitude and duration of spinal loading. Spine. (2000) 25:1477–83. doi: 10.1097/00007632-200006150-00005
5. Kalichman L, Kim DH, Li L, Guermazi A, Hunter DJ. Computed tomography-evaluated features of spinal degeneration: prevalence, intercorrelation, and association with self-reported low back pain. Spine J. (2010) 10:200–8. doi: 10.1016/j.spinee.2009.10.018
6. GBD 2016 Disease and Injury Incidence and Prevalence Collaborators. Global, regional, and national incidence, prevalence, and years lived with disability for 328 diseases and injuries for 195 countries, 1990-2016: a systematic analysis for the Global Burden of Disease Study 2016. Lancet. (2017) 390:1211–59. doi: 10.1016/S0140-6736(17)32154-2
7. Bedore J, Leask A, Seguin CA. Targeting the extracellular matrix: matricellular proteins regulate cell-extracellular matrix communication within distinct niches of the intervertebral disc. Matrix Biol. (2014) 37:124–30. doi: 10.1016/j.matbio.2014.05.005
8. Neidlinger-Wilke C, Galbusera F, Pratsinis H, Mavrogonatou E, Mietsch A, Kletsas D, et al. Mechanical loading of the intervertebral disc: from the macroscopic to the cellular level. Eur Spine J. (2014) 23(Suppl 3):S333–43. doi: 10.1007/s00586-013-2855-9
9. Kudo A, Kii I. Periostin function in communication with extracellular matrices. J Cell Commun Signal. (2018) 12:301–8. doi: 10.1007/s12079-017-0422-6
10. Jun J-I, Lau LF. Taking aim at the extracellular matrix: CCN proteins as emerging therapeutic targets. Nat Rev Drug Discov. (2011) 10:945–63. doi: 10.1038/nrd3599
11. Murphy-Ullrich JE, Sage EH. Revisiting the matricellular concept. Matrix Biol. (2014) 37:1–14. doi: 10.1016/j.matbio.2014.07.005
12. Vo NV, Hartman RA, Yurube T, Jacobs LJ, Sowa GA, Kang JD. Expression and regulation of metalloproteinases and their inhibitors in intervertebral disc aging and degeneration. Spine J. (2013) 13:331–41. doi: 10.1016/j.spinee.2012.02.027
13. Yan Z, Pan Y, Wang S, Cheng M, Kong H, Sun C, et al. Static compression induces ECM remodeling and integrin α2β1 expression and signaling in a rat tail caudal intervertebral disc degeneration model. Spine. (2017) 42:E448–58. doi: 10.1097/BRS.0000000000001856
14. Kudo A. Naming, history, future. Adv Exp Med Biol. (2019) 1132:3–4. doi: 10.1007/978-981-13-6657-4_1
15. Bornstein P, Sage EH. Matricellular proteins: extracellular modulators of cell function. Curr Opin Cell Biol. (2002) 14:608–16. doi: 10.1016/S0955-0674(02)00361-7
16. Takayama G, Arima K, Kanaji T, Toda S, Tanaka H, Shoji S, et al. Periostin: a novel component of subepithelial fibrosis of bronchial asthma downstream of IL-4 and IL-13 signals. J Allergy Clin Immunol. (2006) 118:98–104. doi: 10.1016/j.jaci.2006.02.046
17. Norris RA, Damon B, Mironov V, Kasyanov V, Ramamurthi A, Moreno-Rodriguez R, et al. Periostin regulates collagen fibrillogenesis and the biomechanical properties of connective tissues. J Cell Biochem. (2007) 101:695–711. doi: 10.1002/jcb.21224
18. Gruber HE, Norris RA, Kern MJ, Hoelscher GL, Ingram JA, Zinchenko N, et al. Periostin is expressed by cells of the human and sand rat intervertebral discs. Biotech Histochem. (2011) 86:199–206. doi: 10.3109/10520291003722774
19. Cai L, Brophy RH, Tycksen ED, Duan X, Nunley RM, Rai MF. Distinct expression pattern of periostin splice variants in chondrocytes and ligament progenitor cells. FASEB J. (2019) 33:8386–405. doi: 10.1096/fj.201802281R
20. Takeshita S, Kikuno R, Tezuka K, Amann E. Osteoblast-specific factor 2: cloning of a putative bone adhesion protein with homology with the insect protein fasciclin I. Biochem J. (1993) 294(Pt. 1):271–8. doi: 10.1042/bj2940271
21. Horiuchi K, Amizuka N, Takeshita S, Takamatsu H, Katsuura M, Ozawa H, et al. Identification and characterization of a novel protein, periostin, with restricted expression to periosteum and periodontal ligament and increased expression by transforming growth factor beta. J Bone Miner Res. (1999) 14:1239–49. doi: 10.1359/jbmr.1999.14.7.1239
22. Kudo A. The structure of the periostin gene, its transcriptional control and alternative splicing, and protein expression. Adv Exp Med Biol. (2019) 1132:7–20. doi: 10.1007/978-981-13-6657-4_2
23. Kudo A. Introductory review: periostin-gene and protein structure. Cell Mol Life Sci. (2017) 74:4259–68. doi: 10.1007/s00018-017-2643-5
24. Kii I, Ito H. Periostin and its interacting proteins in the construction of extracellular architectures. Cell Mol Life Sci. (2017) 74:4269–77. doi: 10.1007/s00018-017-2644-4
25. Doliana R, Bot S, Bonaldo P, Colombatti A. EMI, a novel cysteine-rich domain of EMILINs and other extracellular proteins, interacts with the gC1q domains and participates in multimerization. FEBS Lett. (2000) 484:164–8. doi: 10.1016/S0014-5793(00)02140-2
26. Hashimoto K, Noshiro M, Ohno S, Kawamoto T, Satakeda H, Akagawa Y, et al. Characterization of a cartilage-derived 66-kDa protein (RGD-CAP/beta ig-h3) that binds to collagen. Biochim Biophys Acta. (1997) 1355:303–14. doi: 10.1016/S0167-4889(96)00147-4
27. Kii I, Nishiyama T, Kudo A. Periostin promotes secretion of fibronectin from the endoplasmic reticulum. Biochem Biophys Res Commun. (2016) 470:888–93. doi: 10.1016/j.bbrc.2016.01.139
28. Hwang EY, Jeong MS, Park EK, Kim JH, Jang SB. Structural characterization and interaction of periostin and bone morphogenetic protein for regulation of collagen cross-linking. Biochem Biophys Res Commun. (2014) 449:425–31. doi: 10.1016/j.bbrc.2014.05.055
29. Kii I, Nishiyama T, Li M, Matsumoto K-I, Saito M, Amizuka N, et al. Incorporation of tenascin-C into the extracellular matrix by periostin underlies an extracellular meshwork architecture. J Biol Chem. (2010) 285:2028–39. doi: 10.1074/jbc.M109.051961
30. Clout NJ, Tisi D, Hohenester E. Hohenester E. Novel fold revealed by the structure of a FAS1 domain pair from the insect cell adhesion molecule fasciclin I. Structure. (2003) 11:197–203. doi: 10.1016/S0969-2126(03)00002-9
31. Kim JE, Kim SJ, Lee BH, Park RW, Kim KS, Kim IS. Identification of motifs for cell adhesion within the repeated domains of transforming growth factor-beta-induced gene, betaig-h3. J Biol Chem. (2000) 275:30907–15. doi: 10.1074/jbc.M002752200
32. Politz O, Gratchev A, McCourt PAG, Schledzewski K, Guillot P, Johansson S, et al. Stabilin-1 and−2 constitute a novel family of fasciclin-like hyaluronan receptor homologues. Biochem J. (2002) 362(Pt. 1):155–64. doi: 10.1042/bj3620155
33. Takayama I, Tanabe H, Nishiyama T, Ito H, Amizuka N, Li M, et al. Periostin is required for matricellular localization of CCN3 in periodontal ligament of mice. J Cell Commun Signal. (2017) 11:5–13. doi: 10.1007/s12079-016-0371-5
34. Sugiura T, Takamatsu H, Kudo A, Amann E. Expression and characterization of murine osteoblast-specific factor 2 (OSF-2) in a baculovirus expression system. Protein Expr Purif. (1995) 6:305–11. doi: 10.1006/prep.1995.1040
35. Hoersch S, Andrade-Navarro MA. Periostin shows increased evolutionary plasticity in its alternatively spliced region. BMC Evol Biol. (2010) 10:30. doi: 10.1186/1471-2148-10-30
36. Zhang J, Liu X, Ma K, Chen M, Xu H, Niu X, et al. Collagen/heparin scaffold combined with vascular endothelial growth factor promotes the repair of neurological function in rats with traumatic brain injury. Biomater Sci. (2021) 9:745–64. doi: 10.1039/C9BM01446B
37. Zhang J, Wang R-J, Chen M, Liu X-Y, Ma K, Xu H-Y, et al. Collagen/heparan sulfate porous scaffolds loaded with neural stem cells improve neurological function in a rat model of traumatic brain injury. Neural Regener Res. (2021) 16:1068–77. doi: 10.4103/1673-5374.300458
38. Rios HF, Bonewald LF, Conway SJ. Lessons from the matricellular factor periostin. J Dent Res. (2014) 93:843–5. doi: 10.1177/0022034514543017
39. Moore RJ. The vertebral endplate: disc degeneration, disc regeneration. Eur Spine J. (2006) 15(Suppl 3):S333–7. doi: 10.1007/s00586-006-0170-4
40. Wang J, Markova D, Anderson DG, Zheng Z, Shapiro IM, Risbud MV. TNF-α and IL-1β promote a disintegrin-like and metalloprotease with thrombospondin type I motif-5-mediated aggrecan degradation through syndecan-4 in intervertebral disc. J Biol Chem. (2011) 286:39738–49. doi: 10.1074/jbc.M111.264549
41. Pang S, Su Z, Leung S, Nachum IB, Chen B, Feng Q, et al. Direct automated quantitative measurement of spine by cascade amplifier regression network with manifold regularization. Med Image Anal. (2019) 55:103–15. doi: 10.1016/j.media.2019.04.012
42. Müller U, Imwinkelried T, Horst M, Sievers M, Graf-Hausner U. Do human osteoblasts grow into open-porous titanium? Eur Cell Mater J. (2006) 11:8–15. doi: 10.22203/eCM.v011a02
43. Chen Q, Shou P, Zheng C, Jiang M, Cao G, Yang Q, et al. Fate decision of mesenchymal stem cells: adipocytes or osteoblasts? Cell Death Differ. (2016) 23:1128–39. doi: 10.1038/cdd.2015.168
44. Pajarinen J, Lin T, Gibon E, Kohno Y, Maruyama M, Nathan K, et al. Mesenchymal stem cell-macrophage crosstalk and bone healing. Biomaterials. (2019) 196:80–9. doi: 10.1016/j.biomaterials.2017.12.025
45. Muruganandan S, Roman AA, Sinal CJ. Adipocyte differentiation of bone marrow-derived mesenchymal stem cells: cross talk with the osteoblastogenic program. Cell Mol Life Sci. (2009) 66:236–53. doi: 10.1007/s00018-008-8429-z
46. Kokabu S, Lowery JW, Jimi E. Cell fate and differentiation of bone marrow mesenchymal stem cells. Stem Cells Int. (2016) 2016:3753581. doi: 10.1155/2016/3753581
47. Papachroni KK, Karatzas DN, Papavassiliou KA, Basdra EK, Papavassiliou AG. Mechanotransduction in osteoblast regulation and bone disease. Trends Mol Med. (2009) 15:208–16. doi: 10.1016/j.molmed.2009.03.001
48. Hu L, Yin C, Zhao F, Ali A, Ma J, Qian A. Mesenchymal stem cells: cell fate decision to osteoblast or adipocyte and application in osteoporosis treatment. Int J Mol Sci. (2018) 19:360. doi: 10.3390/ijms19020360
49. Li L, Wang P, Hu K, Wang X, Cai W, Ai C, et al. PFMG1 promotes osteoblast differentiation and prevents osteoporotic bone loss. FASEB J. (2018) 32:838–49. doi: 10.1096/fj.201700422R
50. Rachner TD, Khosla S, Hofbauer LC. Osteoporosis: now and the future. Lancet. (2011) 377:1276–87. doi: 10.1016/S0140-6736(10)62349-5
51. An J, Yang H, Zhang Q, Liu C, Zhao J, Zhang L, et al. Natural products for treatment of osteoporosis: the effects and mechanisms on promoting osteoblast-mediated bone formation. Life Sci. (2016) 147:46–58. doi: 10.1016/j.lfs.2016.01.024
52. Ensrud KE, Crandall CJ. Osteoporosis. Ann Intern Med. (2017) 167:ITC17–32. doi: 10.7326/AITC201708010
53. Coutu DL, Wu JH, Monette A, Rivard GE, Blostein MD, Galipeau J. Periostin, a member of a novel family of vitamin K-dependent proteins, is expressed by mesenchymal stromal cells. J Biol Chem. (2008) 283:17991–8001. doi: 10.1074/jbc.M708029200
54. Noack S, Seiffart V, Willbold E, Laggies S, Winkel A, Shahab-Osterloh S, et al. Periostin secreted by mesenchymal stem cells supports tendon formation in an ectopic mouse model. Stem Cells Dev. (2014) 23:1844–57. doi: 10.1089/scd.2014.0124
55. Merle B, Garnero P. The multiple facets of periostin in bone metabolism. Osteoporos Int. (2012) 23:1199–212. doi: 10.1007/s00198-011-1892-7
56. Zhang F, Zhang Z, Sun D, Dong S, Xu J, Dai F. Periostin: a downstream mediator of EphB4-induced osteogenic differentiation of human bone marrow-derived mesenchymal stem cells. Stem Cells Int. (2016) 2016:7241829. doi: 10.1155/2016/7241829
57. Matsuo K, Irie N. Osteoclast-osteoblast communication. Arch Biochem Biophys. (2008) 473:201–9. doi: 10.1016/j.abb.2008.03.027
58. Zhang F, Zhang Z, Sun D, Dong S, Xu J, Dai F. EphB4 promotes osteogenesis of CTLA4-modified bone marrow-derived mesenchymal stem cells through cross talk with Wnt pathway in xenotransplantation. Tissue Eng Part A. (2015) 17–18:2404–16. doi: 10.1089/ten.tea.2015.0012
59. Zhang F, Rong Z, Wang Z, Zhang Z, Sun D, Dong S, et al. Periostin promotes ectopic osteogenesis of CTLA4-modified bone marrow mesenchymal stem cells. Cell Tissue Res. (2017) 370:143–51. doi: 10.1007/s00441-017-2655-3
60. Zhang F, Luo K, Rong Z, Wang Z, Luo F, Zhang Z, et al. Periostin upregulates Wnt/β-catenin signaling to promote the osteogenesis of CTLA4-modified human bone marrow-mesenchymal stem cells. Sci Rep. (2017) 7:41634. doi: 10.1038/srep41634
61. Li C, Li X, Wang X, Miao P, Liu J, Li C, et al. Periostin mediates oestrogen-induced osteogenic differentiation of bone marrow stromal cells in ovariectomised rats. Biomed Res Int. (2020) 2020:9405909. doi: 10.1155/2020/9405909
62. Suresh A, Biswas A, Perumal S, Khurana S. Periostin and integrin signaling in stem cell regulation. Adv Exp Med Biol. (2019) 1132:163–76. doi: 10.1007/978-981-13-6657-4_16
63. Khurana S, Schouteden S, Manesia JK, Santamaria-Martínez A, Huelsken J, Lacy-Hulbert A, et al. Outside-in integrin signalling regulates haematopoietic stem cell function via Periostin-Itgav axis. Nat Commun. (2016) 7:13500. doi: 10.1038/ncomms13500
64. Tang Y, Liu L, Wang P, Chen D, Wu Z, Tang C. Periostin promotes migration and osteogenic differentiation of human periodontal ligament mesenchymal stem cells via the Jun amino-terminal kinases (JNK) pathway under inflammatory conditions. Cell Prolif. (2017) 50. doi: 10.1111/cpr.12369
65. Kazanis I, Ffrench-Constant C. Extracellular matrix and the neural stem cell niche. Dev Neurobiol. (2011) 71:1006–17. doi: 10.1002/dneu.20970
66. Ma S-M, Chen L-X, Lin Y-F, Yan H, Lv J-W, Xiong M, et al. Periostin promotes neural stem cell proliferation and differentiation following hypoxic-ischemic injury. PLoS ONE. (2015) 4:e0123585. doi: 10.1371/journal.pone.0123585
67. Xu D, Xu H, Ren Y, Liu C, Wang X, Zhang H, et al. Cancer stem cellrelated gene periostin: a novel prognostic marker for breast cancer. PLoS ONE. (2012) 10:e46670. doi: 10.1371/journal.pone.0046670
68. Malanchi I, Santamaria-Martínez A, Susanto E, Peng H, Lehr HA, Delaloye JF, et al. Interactions between cancer stem cells and their niche govern metastatic colonization. Nature. (2011) 481:85–9. doi: 10.1038/nature10694
69. Panchamanon P, Pavasant P, Leethanakul C. Periostin plays role in force-induced stem cell potential by periodontal ligament stem cells. Cell Biol Int. (2019) 43:506–15. doi: 10.1002/cbin.11116
70. Conway SJ, Izuhara K, Kudo Y, Litvin J, Markwald R, Ouyang G, et al. The role of periostin in tissue remodeling across health and disease. Cell Mol Life Sci. (2014) 71:1279–88. doi: 10.1007/s00018-013-1494-y
71. Ma Z, Zhao X, Deng M, Huang Z, Wang J, Wu Y, et al. Bone marrow mesenchymal stromal cell-derived periostin promotes B-ALL progression by modulating CCL2 in leukemia cells. Cell Rep. (2019) 26:1533–43.e1534. doi: 10.1016/j.celrep.2019.01.034
72. Litvin J, Selim A-H, Montgomery MO, Lehmann K, Rico MC, Devlin H, et al. Expression and function of periostin-isoforms in bone. J Cell Biochem. (2004) 92:1044–61. doi: 10.1002/jcb.20115
73. Merle B, Bouet G, Rousseau JC, Bertholon C, Garnero P. Periostin and transforming growth factor beta-induced protein (TGFbetaIp) are both expressed by osteoblasts and osteoclasts. Cell Biol Int. (2014) 38:398–404. doi: 10.1002/cbin.10219
74. Rani S, Barbe MF, Barr AE, Litivn J. Role of TNF alpha and PLF in bone remodeling in a rat model of repetitive reaching and grasping. J Cell Physiol. (2010) 225:152–67. doi: 10.1002/jcp.22208
75. Bonnet N, Gineyts E, Ammann P, Conway SJ, Garnero P, Ferrari S. Periostin deficiency increases bone damage and impairs injury response to fatigue loading in adult mice. PLoS ONE. (2013) 8:e78347. doi: 10.1371/journal.pone.0078347
76. Zhang M, Ishikawa S, Inagawa T, Ikemoto H, Guo S, Sunagawa M, et al. Influence of mechanical force on bone matrix proteins in ovariectomised mice and osteoblast-like MC3T3-E1 cells. In Vivo. (2017) 31:87–95. doi: 10.21873/invivo.11029
77. Hayashi M, Nakashima T, Taniguchi M, Kodama T, Kumanogoh A, Takayanagi H. Osteoprotection by semaphorin 3A. Nature. (2012) 485:69–74. doi: 10.1038/nature11000
78. Tse MT. Bone diseases: SEMA3A strikes a balance in bone homeostasis. Nat Rev Drug Discov. (2012) 11:442. doi: 10.1038/nrd3759
79. Chen YJ, Chang MC, Jane Yao CC, Lai HH, Chang JZ, Jeng JH. Mechanoregulation of osteoblast-like MG-63 cell activities by cyclic stretching. J Formos Med Assoc. (2014) 113:447–53. doi: 10.1016/j.jfma.2012.10.003
80. Matsui H, Fukuno N, Kanda Y, Kantoh Y, Chida T, Nagaura Y, et al. The expression of Fn14 via mechanical stress-activated JNK contributes to apoptosis induction in osteoblasts. J Biol Chem. (2014) 289:6438–50. doi: 10.1074/jbc.M113.536300
81. Yu K, Yao C, Jeng J, Shieh H, Chen Y. Periostin inhibits mechanical stretch-induced apoptosis in osteoblast-like MG-63 cells. J Formos Med Assoc. (2018) 117:292–300. doi: 10.1016/j.jfma.2017.12.008
82. Meng X, Zhu Y, Tao L, Zhao S, Qiu S. Periostin has a protective role in melatonininduced cell apoptosis by inhibiting the eIF2alphaATF4 pathway in human osteoblasts. Int J Mol Med. (2018) 41:1003–12. doi: 10.3892/ijmm.2017.3300
83. Qin H, Cai J. Effect of periostin silencing on the autophagy of osteoblasts. Cell Reprog. (2019) 21:122–8. doi: 10.1089/cell.2018.0051
84. Cai J, Qin H, Yu G. Effect of periostin silencing on Runx2, RANKL and OPG expression in osteoblasts. J Orofac Orthop. 82:82–91. (2020) doi: 10.1007/s00056-020-00253-3
85. Bonnet N, Lesclous P, Saffar JL, Ferrari S. Zoledronate effects on systemic and jaw osteopenias in ovariectomized periostin-deficient mice. PLoS ONE. (2013) 8:e58726. doi: 10.1371/journal.pone.0058726
86. Gerbaix M, Vico L, Ferrari SL, Bonnet N. Periostin expression contributes to cortical bone loss during unloading. Bone. (2015) 71:94–100. doi: 10.1016/j.bone.2014.10.011
87. Bonnet N, Biver E, Durosier C, Chevalley T, Rizzoli R, Ferrari S. Additive Genetic Effects on Circulating Periostin Contribute to the Heritability of Bone Microstructure. J Clin Endocrinol Metab. (2015) 100:E1014–21. doi: 10.1210/jc.2015-1183
88. Brown JM, Mantoku A, Sabokbar A, Oppermann U, Hassan AB, Kudo A, et al. Periostin expression in neoplastic and non-neoplastic diseases of bone and joint. Clin Sarcoma Res. (2018) 8:18. doi: 10.1186/s13569-018-0105-y
89. Canalis E, Economides AN, Gazzerro E. Bone morphogenetic proteins, their antagonists, and the skeleton. Endocr Rev. (2003) 24:218–35. doi: 10.1210/er.2002-0023
90. Rios H, Koushik SV, Wang H, Wang J, Zhou H-M, Lindsley A, et al. periostin null mice exhibit dwarfism, incisor enamel defects, and an early-onset periodontal disease-like phenotype. Mol Cell Biol. (2005) 25:11131–44. doi: 10.1128/MCB.25.24.11131-11144.2005
91. Bonnet N, Conway SJ, Ferrari SL. Regulation of beta catenin signaling and parathyroid hormone anabolic effects in bone by the matricellular protein periostin. Proc Natl Acad Sci USA. (2012) 109:15048–53. doi: 10.1073/pnas.1203085109
92. Wergedal JE, Kesavan C, Brommage R, Das S, Mohan S. Role of WNT16 in the regulation of periosteal bone formation in female mice. Endocrinology. (2015) 156:1023–32. doi: 10.1210/en.2014-1702
93. Brommage R, Ohlsson C. Translational studies provide insights for the etiology and treatment of cortical bone osteoporosis. Best Pract Res Clin Endocrinol Metab. (2018) 32:329–40. doi: 10.1016/j.beem.2018.02.006
94. Novinec M, Lenarčič B. Cathepsin K: a unique collagenolytic cysteine peptidase. Biol Chem. (2013) 394:1163–79. doi: 10.1515/hsz-2013-0134
95. Drake MT, Clarke BL, Oursler MJ, Khosla S. Cathepsin K inhibitors for osteoporosis: biology, potential clinical utility, and lessons learned. Endocr Rev. (2017) 38:325–50. doi: 10.1210/er.2015-1114
96. Gossiel F, Scott JR, Paggiosi MA, Naylor KE, McCloskey EV, Peel NFA, et al. Effect of teriparatide treatment on circulating periostin and its relationship to regulators of bone formation and BMD in postmenopausal women with osteoporosis. J Clin Endocrinol Metab. (2018) 103:1302–9. doi: 10.1210/jc.2017-00283
97. Pennypacker BL, Chen CM, Zheng H, Shih M-S, Belfast M, Samadfam R, et al. Inhibition of cathepsin K increases modeling-based bone formation, and improves cortical dimension and strength in adult ovariectomized monkeys. J Bone Mineral Res. (2014) 29:1847–58. doi: 10.1002/jbmr.2211
98. Idolazzi L, Ridolo E, Fassio A, Gatti D, Montagni M, Caminati M, et al. Periostin: the bone and beyond. Eur J Intern Med. (2017) 38:12–6. doi: 10.1016/j.ejim.2016.11.015
99. Duchamp de Lageneste O, Colnot C. Periostin in bone regeneration. Adv Exp Med Biol. (2019) 1132:49–61. doi: 10.1007/978-981-13-6657-4_6
100. Ding F, Shao ZW, Xiong LM. Cell death in intervertebral disc degeneration. Apoptosis. (2013) 18:777–85. doi: 10.1007/s10495-013-0839-1
101. Bron JL, Helder MN, Meisel H-J, Van Royen BJ, Smit TH. Repair, regenerative and supportive therapies of the annulus fibrosus: achievements and challenges. Eur Spine J. (2009) 18:301–13. doi: 10.1007/s00586-008-0856-x
102. Kanayama M, Togawa D, Takahashi C, Terai T, Hashimoto T. Cross-sectional magnetic resonance imaging study of lumbar disc degeneration in 200 healthy individuals. J Neurosurg Spine. (2009) 11:501–7. doi: 10.3171/2009.5.SPINE08675
103. Pattappa G, Li Z, Peroglio M, Wismer N, Alini M, Grad S. Diversity of intervertebral disc cells: phenotype and function. J Anat. (2012) 221:480–96. doi: 10.1111/j.1469-7580.2012.01521.x
104. Chen S, Fu P, Wu H, Pei M. Meniscus, articular cartilage and nucleus pulposus: a comparative review of cartilage-like tissues in anatomy, development and function. Cell Tissue Res. (2017) 370:53–70. doi: 10.1007/s00441-017-2613-0
105. Chen KS, Tatarczuch L, Mirams M, Ahmed YA, Pagel CN, Mackie EJ. Periostin expression distinguishes between light and dark hypertrophic chondrocytes. Int J Biochem Cell Biol. (2010) 42:880–9. doi: 10.1016/j.biocel.2010.01.018
106. Lourido L, Calamia V, Mateos J, Fernandez-Puente P, Fernandez-Tajes J, Blanco FJ, et al. Quantitative proteomic profiling of human articular cartilage degradation in osteoarthritis. J Proteome Res. (2014) 13:6096–106. doi: 10.1021/pr501024p
107. Brophy RH, Cai L, Duan X, Zhang Q, Townsend RR, Nunley RM, et al. Proteomic analysis of synovial fluid identifies periostin as a biomarker for anterior cruciate ligament injury. Osteoarthritis Cartilage. (2019) 27:1778–89. doi: 10.1016/j.joca.2019.08.002
108. Ryoko I, Yuko F, Akira K, Masaki M, Atsuhiko H, Tsuyoshi T, et al. Periostin contributes to the maturation and shape retention of tissue-engineered cartilage. Sci Rep. (2018) 8:11210. doi: 10.1038/s41598-018-29228-6
109. Attur M, Yang Q, Shimada K, Tachida Y, Nagase H, Mignatti P, et al. Elevated expression of periostin in human osteoarthritic cartilage and its potential role in matrix degradation via matrix metalloproteinase-13. FASEB J. (2015) 29:4107–21. doi: 10.1096/fj.15-272427
110. Ryota C, Yasuo K, Yoshiaki T, Norimasa N, Tetsuya T, Yoshikawa H. Expression and pathological effects of periostin in human osteoarthritis cartilage. BMC Musculoskelet Disord. (2015) 21:215. doi: 10.1186/s12891-015-0682-3
111. Moue T, Tajika Y, Ishikawa S, Kanada Y, Okumo T, Asano K, et al. Influence of IL13 on periostin secretion by synoviocytes in osteoarthritis. In Vivo. (2017) 31:79–85. doi: 10.21873/invivo.11028
112. Tajika Y, Moue T, Ishikawa S, Asano K, Okumo T, Takagi H, et al. Influence of periostin on synoviocytes in knee osteoarthritis. In Vivo. (2017) 31:69–77. doi: 10.21873/invivo.11027
113. Chinzei N, Brophy RH, Duan X, Cai L, Nunley RM, Sandell LJ, et al. Molecular influence of anterior cruciate ligament tear remnants on chondrocytes: a biologic connection between injury and osteoarthritis. Osteoarthritis Cartilage. (2018) 26:588–99. doi: 10.1016/j.joca.2018.01.017
114. Han T, Mignatti P, Abramson SB, Attur M. Periostin interaction with discoidin domain receptor-1 (DDR1) promotes cartilage degeneration. PLoS ONE. (2020) 15:e0231501. doi: 10.1371/journal.pone.0231501
115. Tsai T-T, Lai P-L, Liao J-C, Fu T-S, Niu C-C, Chen L-H, et al. Increased periostin gene expression in degenerative intervertebral disc cells. Spine J. (2013) 13:289–98. doi: 10.1016/j.spinee.2013.01.040
116. Cheng S, Li X, Lin L, Jia Z, Zhao Y, Wang D, et al. Identification of aberrantly expressed genes during aging in rat nucleus pulposus cells. Stem Cells Int. (2019) 2019:2785207. doi: 10.1155/2019/2785207
117. Graja A, Garcia-Carrizo F, Jank A-M, Gohlke S, Ambrosi TH, Jonas W, et al. Loss of periostin occurs in aging adipose tissue of mice and its genetic ablation impairs adipose tissue lipid metabolism. Aging Cell. (2018) 17:e12810. doi: 10.1111/acel.12810
118. Duchamp de Lageneste O, Julien A, Abou-Khalil R, Frangi G, Carvalho C, Cagnard N, et al. Periosteum contains skeletal stem cells with high bone regenerative potential controlled by Periostin. Nat Commun. (2018) 9:773. doi: 10.1038/s41467-018-03124-z
119. Zhang F, Zhao X, Shen H, Zhang C. Molecular mechanisms of cell death in intervertebral disc degeneration (Review). Int J Mol Med. (2016) 37:1439–48. doi: 10.3892/ijmm.2016.2573
120. Emanuel KS, Mader KT, Peeters M, Kingma I, Rustenburg CME, Vergroesen PPA, et al. Early changes in the extracellular matrix of the degenerating intervertebral disc, assessed by Fourier transform infrared imaging. Osteoarthr Cartil. (2018) 26:1400–8. doi: 10.1016/j.joca.2018.06.003
121. Elliott CG, Hamilton DW. Deconstructing fibrosis research: do pro-fibrotic signals point the way for chronic dermal wound regeneration? J Cell Commun Signal. (2011) 5:301–15. doi: 10.1007/s12079-011-0131-5
122. Kii I. periostin functions as a scaffold for assembly of extracellular proteins. Adv Exp Med Biol. (2019) 1132:23–32. doi: 10.1007/978-981-13-6657-4_3
123. Kadler KE, Hill A, Canty-Laird EG. Collagen fibrillogenesis: fibronectin, integrins, and minor collagens as organizers and nucleators. Curr Opin Cell Biol. (2008) 20:495–501. doi: 10.1016/j.ceb.2008.06.008
124. Midwood KS, Chiquet M, Tucker RP, Orend G. Tenascin-C at a glance. J Cell Sci. (2016) 129:4321–7. doi: 10.1242/jcs.190546
125. Vadon-Le Goff S, Hulmes DJS, Moali C. BMP-1/tolloid-like proteinases synchronize matrix assembly with growth factor activation to promote morphogenesis and tissue remodeling. Matrix Biol. (2015) 44–46:14–23. doi: 10.1016/j.matbio.2015.02.006
126. Maruhashi T, Kii I, Saito M, Kudo A. Interaction between periostin and BMP-1 promotes proteolytic activation of lysyl oxidase. J Biol Chem. (2010) 285:13294–303. doi: 10.1074/jbc.M109.088864
127. Trackman PC. Enzymatic and non-enzymatic functions of the lysyl oxidase family in bone. Matrix Biol. (2016) 52–4. doi: 10.1016/j.matbio.2016.01.001
128. Garnero P. The contribution of collagen crosslinks to bone strength. Bonekey Rep. (2012) 1:182. doi: 10.1038/bonekey.2012.182
129. Ghatak S, Misra S, Norris RA, Moreno-Rodriguez RA, Hoffman S, Levine RA, et al. Periostin induces intracellular cross-talk between kinases and hyaluronan in atrioventricular valvulogenesis. J Biol Chem. (2014) 289:8545–61. doi: 10.1074/jbc.M113.539882
130. Sugiyama A, Kanno K, Nishimichi N, Ohta S, Ono J, Conway SJ, et al. Periostin promotes hepatic fibrosis in mice by modulating hepatic stellate cell activation via alphav integrin interaction. J Gastroenterol. (2016) 51:1161–74. doi: 10.1007/s00535-016-1206-0
131. Nishida M, Sato Y, Uemura A, Narita Y, Tozaki-Saitoh H, Nakaya M, et al. P2Y6 receptor-Galpha12/13 signalling in cardiomyocytes triggers pressure overload-induced cardiac fibrosis. EMBO J. (2008) 27:3104–15. doi: 10.1038/emboj.2008.237
132. Stansfield WE, Andersen NM, Tang R-H, Selzman CH. Periostin is a novel factor in cardiac remodeling after experimental and clinical unloading of the failing heart. Ann Thorac Surg. (2009) 88:1916–21. doi: 10.1016/j.athoracsur.2009.07.038
133. Rani S, Barbe MF, Barr AE, Litvin J. Periostin-like-factor and Periostin in an animal model of work-related musculoskeletal disorder. Bone. (2009) 44:502–12. doi: 10.1016/j.bone.2008.11.012
134. Bonnet N, Brun J, Rousseau J-C, Duong LT, Ferrari SL. Cathepsin K Controls cortical bone formation by degrading periostin. J Bone Mineral Res. (2017) 32:1432–41. doi: 10.1002/jbmr.3136
135. Bonnet N, Standley KN, Bianchi EN, Stadelmann V, Foti M, Conway SJ, et al. The matricellular protein periostin is required for sost inhibition and the anabolic response to mechanical loading and physical activity. J Biol Chem. (2009) 284:35939–50. doi: 10.1074/jbc.M109.060335
136. Yoshiba N, Yoshiba K, Hosoya A, Saito M, Yokoi T, Okiji T, et al. Association of TIMP-2 with extracellular matrix exposed to mechanical stress and its co-distribution with periostin during mouse mandible development. Cell Tissue Res. (2007) 330:133–45. doi: 10.1007/s00441-007-0439-x
137. Ferretti C, Mattioli-Belmonte M. Periosteum derived stem cells for regenerative medicine proposals: boosting current knowledge. World J Stem Cells. (2014) 6:266–77. doi: 10.4252/wjsc.v6.i3.266
138. Rosselli-Murai LK, Almeida LO, Zagni C, Galindo-Moreno P, Padial-Molina M, Volk SL, et al. Periostin responds to mechanical stress and tension by activating the MTOR signaling pathway. PLoS ONE. (2013) 8:e83580. doi: 10.1371/journal.pone.0083580
139. Kadow T, Sowa G, Vo N, Kang JD. Molecular basis of intervertebral disc degeneration and herniations: what are the important translational questions? Clin Orthop Relat Res. (2015) 473:1903–12. doi: 10.1007/s11999-014-3774-8
140. Purmessur D, Walter BA, Roughley PJ, Laudier DM, Hecht AC, Iatridis J. A role for TNFα in intervertebral disc degeneration: a non-recoverable catabolic shift. Biochem Biophys Res Commun. (2013) 433:151–6. doi: 10.1016/j.bbrc.2013.02.034
141. Johnson ZI, Schoepflin ZR, Choi H, Shapiro IM, Risbud MV. Disc in flames: roles of TNF-α and IL-1β in intervertebral disc degeneration. Eur Cells Mater. (2015) 30. doi: 10.22203/eCM.v030a08
142. Risbud MV, Shapiro IM. Role of cytokines in intervertebral disc degeneration: pain and disc content. Nat Rev Rheumatol. (2014) 10:44–56. doi: 10.1038/nrrheum.2013.160
143. Tian Y, Yuan W, Fujita N, Wang J, Wang H, Shapiro IM, et al. Inflammatory cytokines associated with degenerative disc disease control aggrecanase-1 (ADAMTS-4) expression in nucleus pulposus cells through MAPK and NF-κB. Am J Pathol. (2013) 182:2310–21. doi: 10.1016/j.ajpath.2013.02.037
144. Wang Y, Che M, Xin J, Zheng Z, Li J, Zhang S. The role of IL-1β and TNF-α in intervertebral disc degeneration. Biomed Pharmacother. (2020) 131:110660. doi: 10.1016/j.biopha.2020.110660
145. Masuoka M, Shiraishi H, Ohta S, Suzuki S, Arima K, Aoki S, et al. Periostin promotes chronic allergic inflammation in response to Th2 cytokines. J Clin Invest. (2012) 122:2590–600. doi: 10.1172/JCI58978
146. Uchida M, Shiraishi H, Ohta S, Arima K, Taniguchi K, Suzuki S, et al. Periostin, a matricellular protein, plays a role in the induction of chemokines in pulmonary fibrosis. Am J Respir Cell Mol Biol. (2012) 46:677–86. doi: 10.1165/rcmb.2011-0115OC
147. Izuhara K, Nunomura S, Nanri Y, Ogawa M, Ono J, Mitamura Y, et al. Periostin in inflammation and allergy. Cell Mol Life Sci. (2017) 74:4293–303. doi: 10.1007/s00018-017-2648-0
148. Izuhara K, Nunomura S, Nanri Y, Ono J, Takai M, Kawaguchi A. Periostin: an emerging biomarker for allergic diseases. Allergy. (2019) 74:2116–28. doi: 10.1111/all.13814
149. Amara S, Lopez K, Banan B, Brown SK, Whalen M, Myles E, et al. Synergistic effect of pro-inflammatory TNFα and IL-17 in periostin mediated collagen deposition: potential role in liver fibrosis. Mol Immunol. (2015) 64:26–35. doi: 10.1016/j.molimm.2014.10.021
150. Chiricozzi A, Guttman-Yassky E, Suárez-Fariñas M, Nograles KE, Tian S, Cardinale I, et al. Integrative responses to IL-17 and TNF-α in human keratinocytes account for key inflammatory pathogenic circuits in psoriasis. J Invest Dermatol. (2011) 131:677–87. doi: 10.1038/jid.2010.340
151. Yao L, Song J, Meng XW, Ge JY, Du BX, Yu J, et al. Periostin aggravates NLRP3 inflammasome-mediated pyroptosis in myocardial ischemia-reperfusion injury. Mol Cell Probes. (2020) 53:101596. doi: 10.1016/j.mcp.2020.101596
152. Burgess JK, Jonker MR, Berg M, Ten Hacken NTH, Meyer KB, van den Berge M, et al. Periostin: contributor to abnormal airway epithelial function in asthma? Eur Respir J. (2020) 57:2001286. doi: 10.1183/13993003.01286-2020
153. Arima K, Ohta S, Takagi A, Shiraishi H, Masuoka M, Ontsuka K, et al. Periostin contributes to epidermal hyperplasia in psoriasis common to atopic dermatitis. Allergol Int. (2015) 64:41–8. doi: 10.1016/j.alit.2014.06.001
154. Ho J, Earls P, Harvey RJ. Systemic biomarkers of eosinophilic chronic rhinosinusitis. Curr Opin Allergy Clin Immunol. (2020) 20:23–9. doi: 10.1097/ACI.0000000000000602
155. Asada Y, Okano M, Ishida W, Iwamoto S, Fukuda K, Hirakata T, et al. Periostin deletion suppresses late-phase response in mouse experimental allergic conjunctivitis. Allergol Int. (2019) 68:233–9. doi: 10.1016/j.alit.2018.09.007
156. Zhao Y, Wang S, Sorenson CM, Teixeira L, Dubielzig RR, Peters DM, et al. Cyp1b1 mediates periostin regulation of trabecular meshwork development by suppression of oxidative stress. Mol Cell Biol. (2013) 33:4225–40. doi: 10.1128/MCB.00856-13
157. Wu H, Chen L, Xie J, Li R, Li GN, Chen QH, et al. Periostin expression induced by oxidative stress contributes to myocardial fibrosis in a rat model of high salt-induced hypertension. Mol Med Rep. (2016) 14:776–82. doi: 10.3892/mmr.2016.5308
158. Liu H, Wang Y, Yue Y, Zhang P, Sun Y, Chen Q. [Periostin inhibits hypoxia-induced oxidative stress and apoptosis in human periodontal ligament fibroblasts via p38 MAPK signaling pathway]. Nan Fang Yi Ke Da Xue Xue Bao. (2020) 40:942–8. doi: 10.12122/j.issn.1673-4254.2020.07.05
159. Khwaja A, Rodriguez-Viciana P, Wennström S, Warne PH, Downward J. Matrix adhesion and Ras transformation both activate a phosphoinositide 3-OH kinase and protein kinase B/Akt cellular survival pathway. EMBO J. (1997) 16:2783–93. doi: 10.1093/emboj/16.10.2783
160. Song G, Ouyang G, Bao S. The activation of Akt/PKB signaling pathway and cell survival. J Cell Mol Med. (2005) 9:59–71. doi: 10.1111/j.1582-4934.2005.tb00337.x
161. Cheng GZ, Park S, Shu S, He L, Kong W, Zhang W, et al. Advances of AKT pathway in human oncogenesis and as a target for anti-cancer drug discovery. Curr Cancer Drug Targets. (2008) 8:2–6. doi: 10.2174/156800908783497159
162. Watanabe T, Yasue A, Fujihara S, Tanaka E. Periostin regulates MMP-2 expression via the αvβ3 integrin/ERK pathway in human periodontal ligament cells. Arch Oral Biol. (2012) 57:52–9. doi: 10.1016/j.archoralbio.2011.07.010
163. Orecchia P, Conte R, Balza E, Castellani P, Borsi L, Zardi L, et al. Identification of a novel cell binding site of periostin involved in tumour growth. Eur J Cancer. (2011) 47:2221–9. doi: 10.1016/j.ejca.2011.04.026
164. Honsawek S, Wilairatana V, Udomsinprasert W, Sinlapavilawan P, Jirathanathornnukul N. Association of plasma and synovial fluid periostin with radiographic knee osteoarthritis: cross-sectional study. Joint Bone Spine. (2015) 82:352–5. doi: 10.1016/j.jbspin.2015.01.023
165. Rousseau JC, Sornay-Rendu E, Bertholon C, Garnero P, Chapurlat R. Serum periostin is associated with prevalent knee osteoarthritis and disease incidence/progression in women: the OFELY study. Osteoarthr Cartil. (2015) 23:1736–42. doi: 10.1016/j.joca.2015.05.015
166. Covone AE, Solari N, Malattia C, Pop V, Martini A, Ravelli A, et al. Periostin gene variants are associated with disease course and severity in juvenile idiopathic arthritis. Clin Exp Rheumatol. (2014) 32:747–53.
167. Yan J, Liu HJ, Li H, Chen L, Bian YQ, Zhao B, et al. Circulating periostin levels increase in association with bone density loss and healing progression during the early phase of hip fracture in Chinese older women. Osteoporos Int. (2017) 28:2335–41. doi: 10.1007/s00198-017-4034-z
168. Garnero P. The utility of biomarkers in osteoporosis management. Mol Diagn Ther. (2017) 21:401–18. doi: 10.1007/s40291-017-0272-1
169. Sakellariou GT, Anastasilakis AD, Bisbinas I, Oikonomou D, Gerou S, Polyzos SA, et al. Circulating periostin levels in patients with AS: association with clinical and radiographic variables, inflammatory markers and molecules involved in bone formation. Rheumatology. (2015) 54:908–14. doi: 10.1093/rheumatology/keu425
170. Varughese R, Semprini R, Munro C, Fingleton J, Holweg C, Weatherall M, et al. Serum periostin levels following small bone fractures, long bone fractures and joint replacements: an observational study. Allergy Asthma Clin Immunol. (2018) 14:30. doi: 10.1186/s13223-018-0254-9
Keywords: periostin, osteoarthritis, extracellular matrix, spinal degeneration, signaling pathways
Citation: Zhu D, Zhou W, Wang Z, Wang Y, Liu M, Zhang G, Guo X and Kang X (2021) Periostin: An Emerging Molecule With a Potential Role in Spinal Degenerative Diseases. Front. Med. 8:694800. doi: 10.3389/fmed.2021.694800
Received: 20 April 2021; Accepted: 23 July 2021;
Published: 27 August 2021.
Edited by:
Weiren Luo, The Second Affiliated Hospital of Southern University of Science and Technology, ChinaReviewed by:
Jian Zhang, Institute of Neurotrauma Repair, ChinaQingbing Meng, Fudan University, China
Copyright © 2021 Zhu, Zhou, Wang, Wang, Liu, Zhang, Guo and Kang. This is an open-access article distributed under the terms of the Creative Commons Attribution License (CC BY). The use, distribution or reproduction in other forums is permitted, provided the original author(s) and the copyright owner(s) are credited and that the original publication in this journal is cited, in accordance with accepted academic practice. No use, distribution or reproduction is permitted which does not comply with these terms.
*Correspondence: Xuewen Kang, ZXJ5X2thbmd4d0BsenUuZWR1LmNu; Zhen Wang, eGlqaW5nX2hAdmlwLnRvbS5jb20=
†These authors have contributed equally to this work and share first authorship