- 1State Key Laboratory of Dampness Syndrome of Chinese Medicine, The Second Affiliated Hospital of Guangzhou University of Chinese Medicine, Guangzhou, China
- 2The Second Clinical Medical College of Guangzhou University of Chinese Medicine, Guangzhou, China
- 3Guangdong Provincial Key Laboratory of Clinical Research on Traditional Chinese Medicine Syndrome, Guangzhou, China
Introduction: Renal podocyte injury, apoptosis and autophagy are involved in the occurrence and development of diabetic nephropathy (DN). Kaempferol (KPF) has the promotion of autophagy and inhibition of apoptosis properties in the development of miscellaneous diseases, but these functions in DN have not yet been elucidated.
Methods: We used db/db mice to evaluate the protective role of KPF on DN. The anti-DN effect of KPF was evaluated by urine albumin-to-creatinine ratio and pathological changes of kidney tissue. Injury of podocytes was observed through Transmission electron microscopy. Immunofluorescence, Western blot, and Immunohistochemistry were used to detect the protein expression of podocyte-associated molecules, autophagy, and AMPK/mTOR pathway.
Results: We demonstrated that KPF treatment significantly attenuated diabetes-induced albuminuria and glycolipid metabolism dysfunction. In addition, KPF alleviated mesangial matrix expansion, glomerular basement membrane thickening and loss or fusion of podocytes. Mechanistically, KPF treatment regulated the expression of autophagic proteins (upregulated LC3II, Beclin-1, Atg7 and Atg 5, and downregulated p62/SQSTM1), accompanied by inhibited renal apoptosis (downregulated Caspase 3 and Bax, and upregulated Bcl-2). KPF could significantly regulate the AMPK/mTOR signaling pathways by increasing p-AMPK and decreasing p-mTOR expressions.
Discussion: In conclusion, KPF might have a protective effect on DN through reduced apoptosis and enhanced podocytes autophagy, which were correlated with regulating AMPK/mTOR pathways.
Introduction
Diabetic nephropathy (DN) is a serious diabetic angiopathy, manifesting as microvascular complications, and a leading cause of end-stage renal disease (1). In the kidney glomerulus, podocytes are pivotal in maintaining glomerular filtration barrier function. The loss and dysfunction of podocytes are closely associated with proteinuria, the hallmark of early renal injury in DN (2), and contribute to aggravated glomerular injury and the progression of DN (3, 4).
Autophagy is the way of refreshing newer, healthier cells through catabolizing damaged cells. It is an essential self-repair mechanism in maintaining cell homeostasis (5). Podocytes show high rates of autophagy under normal conditions. Studies have confirmed that reduced podocytes autophagy induces podocyte function alteration that may exacerbate diabetic renal damage (6, 7). Most of the research in podocyte autophagy has focused on the mammalian target of rapamycin (mTOR) and AMP-activated protein kinase (AMPK) signaling pathways, which play an important role in accelerated podocyte injury in DN (8–11). AMPK is a key regulator of energy homeostasis and a vital energy sensor that can promote autophagy. On the other hand, mTOR can inhibit autophagy, which is a significant regulator of cellular and organismal growth (12). High glucose conditions can inhibit the AMPK signaling pathway and promote mTOR activation, thus inhibiting podocyte autophagy and aggravating kidney injury (13). Thus, autophagy regulation should be a therapeutic target to prevent DN. Agents that can regulate the activity of mTOR and AMPK may have a therapeutic effect on DN.
Kaempferol (KPF), which could be extracted from tea leaves, broccoli, hazelnuts, propolis, grapefruit and other green plants, belongs to the family of natural flavanol. It has been reported for its roles as a potential anti-diabetic, anti-obesity and anti-fibrosis agent, attributed to its potent anti-inflammatory, anti-oxidative, and anti-atherosclerotic effects (14–16). It has been shown in studies that KPF could promote autophagy and inhibit apoptosis via the regulation of AMPK/mTOR pathway in several conditions, including pancreatic β-cell dysfunction (17, 18), memory deficits (19), cerebral ischemic-reperfusion injury (20), hepatic cancer (21), and HeLa cells (22). The nephroprotective effect of KPF on streptozotocin induced type-1 DN mice has been reported in previous studies, due to its effect of ameliorating inflammation, which correlates with decreased TRAF6 levels (23), the upregulation of the Nrf-2/HO-1 axis (24), the enhanced GLP-1 and insulin release, and the inhibition of RhoA/Rho kinase activity (25). However, the renal protective effects of KPF on a type-2 DN model of db/db mice remain unclear. In addition, studies on whether KPF could prevent the progression of DN through regulating autophagy via AMPK/mTOR pathway are lacking.
Consequently, in our current study, we aim to investigate whether KPF treatment has therapeutic effects on type-2 DN model of db/db mice and to examine the mechanism underlying the protective effects of KPF on DN by focusing on the regulation of podocyte autophagy via AMPK/mTOR pathway.
Materials and methods
Reagents and chemicals
Kaempferol was purchased from Sigma-Aldrich (St. Louis, MO, USA) and was suspended in 1% sodium carboxymethyl cellulose (CMC-Na). The antibodies against SQSTM1/p62 (23214), Beclin1 (3738), LC3II (83506), Bcl-2 (3498), Bax (14796), Caspase3 (9662), Atg7 (8558), AMPK (5832), p-AMPK (50081), mTOR (2983), p-mTOR (5536, 2976), Horseradish Peroxidase (HRP)-linked anti-rabbit Immunoglobulin G (IgG) (7074), HRP-linked anti-mouse IgG (7076) antibodies, and DAB Substrate Kit were all purchased from Cell Signaling Technology. Atg5 (ab108327), the antibodies against Nephrin (ab216341) and Wilms’ tumor protein-1 (WT1) (ab89901) were purchased from Abcam. The antibody against β-actin (BM0627) and terminal deoxynucleotidyl transferase dUTP nick end labeling (TUNEL) apoptosis detection Kit (MK1018) were from Boster Biological Technology. Enhanced chemiluminescence (ECL) reagent was obtained from Millipore.
Experimental animals
Eight-week-old male C57BLKS/J db/db (n = 15) and db/m mice (n = 5) were obtained from the Model Animal Research Center of Nanjing University (experimental animal license No. 32002100006993). All mice were housed in the SPF level animal room, where the temperature was controlled at 23 ± 3°C and humidity at 55 ± 15% with a 12 h light and dark cycle and fed with a standard rodent diet and sterile water. After 1 week of acclimatization, the db/db mice were randomly divided into 3 groups (n = 5 each) db/db, db/db + Low Kaempferol (LKPF, Kaempferol 50 mg/kg/day dissolved in 1% CMC-Na) and db/db + High Kaempferol (HKPF, Kaempferol 100 mg/kg/day dissolved in 1% CMC-Na). KPF was administered through oral gavage, and the dose of KPF is referred to the previous study (26). The db/m mice were given the same daily volume of 1% CMC-Na solution. After 12 weeks of KPF treatment, all mice were sacrificed for the experiment after fasting overnight. The experimental procedures used in the present study were approved by the Animal Care and Ethics Committee of Guangzhou University of Chinese Medicine (ethical batch number: 2018076) and were in accordance with the internationally accepted principles for laboratory animal use and care.
Urine and serum measurement
All mice were weighed every week, and fasting plasma glucose (FPG) was tested every 2 weeks. We collected urine samples using metabolic cages every 4 weeks. Urine albumin-to-creatinine ratio (UACR) was measured using an automatic analyzer. The mice were sacrificed after KPF treatment for 12 weeks. Blood was sampled from eyeball blood collection. Serum creatinine (SCr), total cholesterol (TC), and low-density lipoprotein cholesterol (LDL-C) were detected using an automatic analyzer by the clinical laboratory of Guangdong Provincial Hospital of Chinese medicine. At the time of sacrifice, renal tissues were collected. Kidney tissues were either fixed in 4% paraformaldehyde for histological analysis or fast-frozen in liquid nitrogen and stored at –80°C for protein expression detection.
Histological analysis
The kidney tissues were fixed in 10% formalin buffer for 48 h and then embedded in paraffin, which were cut into 5-μm thick, and stained with periodic acid–Schiff (PAS) and Hematoxylin and Eosin (H&E) to determine the mesangial matrix expansion of glomerulus and to estimate the kidney damage. PAS and H&E-stained sections were observed under an Olympus IX71 microscope.
Immunohistochemistry
For Immunohistochemistry (IHC), 5-μm thick and paraffin-embedded kidney sections were separately stained with primary antibodies against nephrin (1:1000), Bcl-2 (1:500), Bax (1:500), Caspase3 (1:500), Atg5 (1:100), p-AMPK (1:100), and p-mTOR (1:100) at 4°C overnight. Next, incubated by the secondary antibody and 3,3′3′-Diaminobenzidine (DAB) kit, the sections were viewed under an Olympus IX71 microscope.
Immunofluorescence
Immunofluorescence (IF) assay was used to assess the expression of WT1, LC3II and nephrin protein in kidney tissues. A total of 0.25% Triton X-100 was used to permeabilize 5-μm thick kidney sections for 15 min, and 5% Bovine Serum Albumin (BSA) was used to block them for 1 h at room temperature. Then, the sections were stained with primary antibodies against WT1 (1:200), LC3II (1:200), and nephrin (1:500) overnight at 4°C, followed by secondary Cy3-labeled goat anti-rabbit IgG (A0516, Beyotime), Cy3-labeled goat anti-mouse IgG (A0521, Beyotime) or FITC-labeled goat anti-rabbit IgG (A0561, Beyotime). The cell nuclei were labeled with 4′,6-diamidino-2-phenylindole (DAPI), and images were obtained with fluorescence microscopy.
Terminal deoxynucleotidyl transferase dUTP nick end labeling (TUNEL) assay
The kidney sections were dewaxed and hydrated in graded alcohol series and were incubated with Proteinase K (1:200 diluted by 0.01 M Tris/Hcl, pH 7.5) for 15 min at 37°C to enhance tissue permeability. After rinsing 3 times with 0.01 M TBS, each section was added 20 μl labeling buffer to keep moist. The labeling buffer was composed of 1 μl Terminal deoxyribonuclease transferase (TDT), 1 μl Biotin-labeled DUTP (Bio-DUTP), and 18 μl buffer solution. After incubating in a humidified environment for 2 h at 37°C protecting from light, the sections were rinsed for 3 times, and each section was added 5% serum albumin fraction for blocking 30 min at room temperature. And then, each section was added SABC-FITC (1:200 diluted) for 30 min at 37°C. Rinsing 4 times with 0.01 M TBS, At last the cell nuclei were stained with DAPI, and images were obtained with the fluorescence microscopy.
Transmission electron microscopy
We used transmission electron microscopy (TEM) to evaluate ultrastructural. Kidney tissues were sliced into cubes and in fixation in 2.5% glutaraldehyde. Samples were handled in Wuhan service biotechnology and detected by transmission electron microscope (JEM-1400 PLUS, Tokyo, Japan).
Western blot analysis
Renal tissues were lysed in Radioimmunoprecipitation assay buffer (RIPA) buffer. A total of 30 μg per well protein were separated on 10 or 15% sodium dodecyl sulfate-polyacrylamide gel electrophoresis (SDS-PAGE) and subsequently immunoblotted on Polyvinylidene fluoride or polyvinylidene difluoride (PVDF) membranes. Densitometric quantification of the protein bands was analyzed by Image Lab System (Bio-Rad Laboratories, Inc.). The primary antibodies are listed as follows: nephrin, SQSTM1/p62, Beclin1, LC3II, Bcl-2, Bax, Caspase 3, Atg7, AMPK, p-AMPK, mTOR, p-mTOR, β-actin 1:1,000.
Statistical analysis
All results were expressed as the mean ± standard deviation (SD). Statistical analysis of all data was performed by using Graphpad Prism 7.0 software (San Diego, CA, USA). Statistical significance was determined using a one-way analysis of variance (ANOVA) in multiple comparisons. A value of p < 0.05 was considered to be statistically significant.
Results
Kaempferol decreased urine albumin-to-creatinine ratio and improved glycolipid metabolism dysfunction of db/db mice
In comparison with db/m mice, db/db mice had a significantly higher level of urine albumin-creatine ratio (UACR), body weight (BW), fasting blood glucose (FBG), TC, and low-density lipoprotein-cholesterol (LDL-C). A dose-dependent reduction in these indicators was observed in db/db mice after administration of KPF (either 50 or 100 mg/kg/day) for 12 weeks (Figure 1).
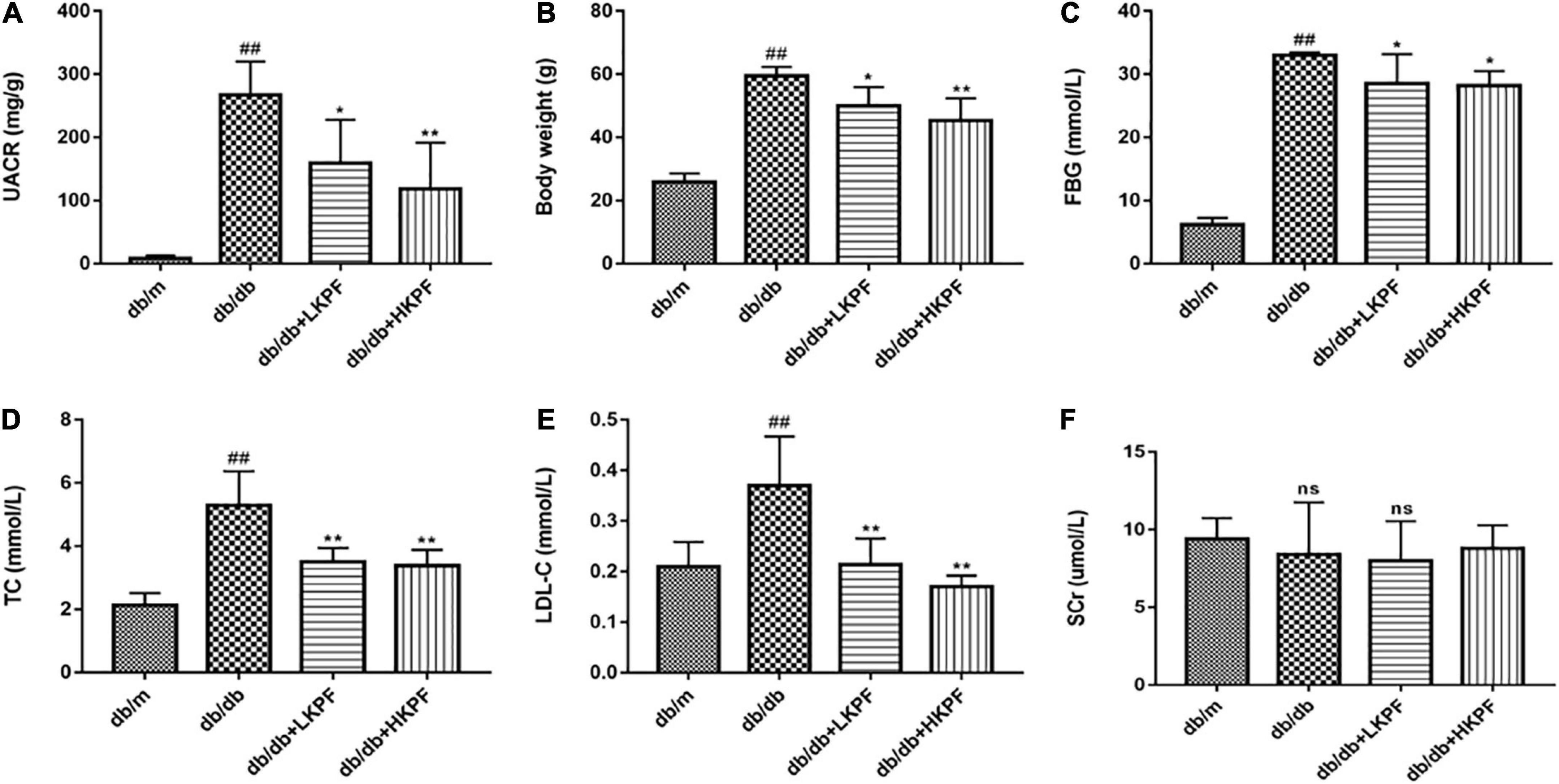
Figure 1. Kaempferol alleviated dysregulated UACR, BW, FBG and dyslipidemia levels of db/db mice. (A) Urine albumin-creatine ratio (UACR) (mg/g), (B) Body weight (g), (C) Fasting blood glucose (FBG) (mmol/L), (D) Total cholesterol (TC) (mmol/L), (E) Low density lipoprotein-cholesterol (LDL-C) (mmol/L), and (F) serum creatinine (SCr) (μmol/L) were measured after KPF treatment. The data were presented as the mean ± SD (n = 5, ##p < 0.01 vs. db/m mice; *p < 0.05 and **p < 0.01 vs. db/db mice; ns, not significant).
Kaempferol ameliorated renal histological damage in db/db mice
The morphological changes of the kidney were observed using PAS and H&E staining. Compared with db/m mice, the kidneys of db/db mice showed mesangial matrix expansion and extracellular matrix deposition. Treatment with KPF for 12 weeks significantly reduced the accumulation of extracellular matrix and the expansion of renal mesangial in db/db mice (Figure 2).
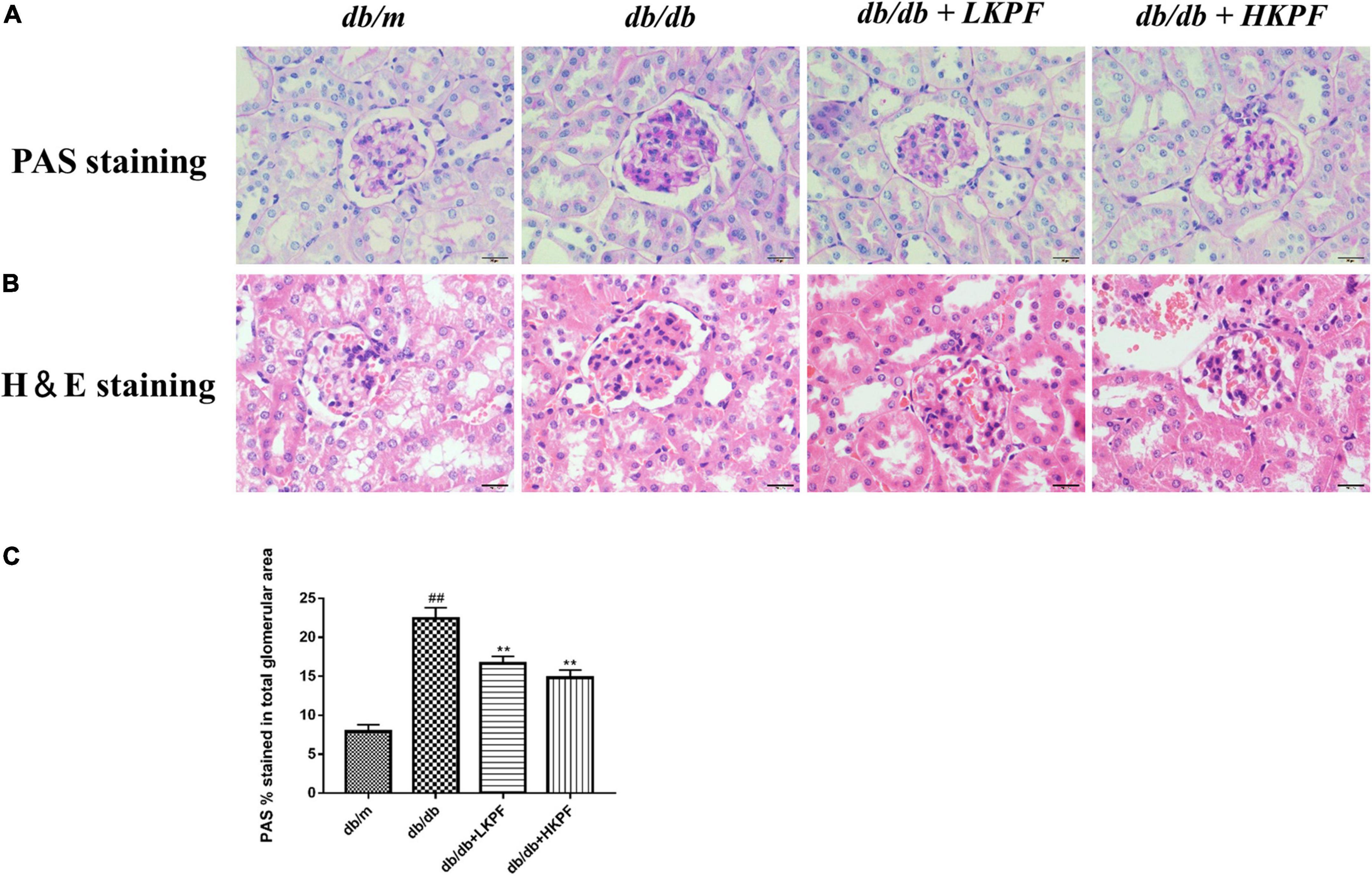
Figure 2. Kaempferol ameliorated renal histological damage in db/db mice (400×, bar = 20 μm). (A) Kaempferol treatment ameliorated glomerular mesangial matrix deposition in db/db mice (PAS staining). (B) Kaempferol treatment ameliorated morphology in the kidneys of db/db mice (H&E staining). (C) Quantification of PAS stained (the glomerular mesangial matrix area) for each group. The data were presented as the mean ± SD (n = 5, ##p < 0.01 vs. db/m mice; **p < 0.01 vs. db/db mice). H&E, Hematoxylin and Eosin; PAS, Periodic Acid–Schiff.
Kaempferol attenuated podocytes injury in db/db mice
Transmission electron microscopy revealed that podocytes were damaged with foot processes obvious loss and fusion, and the glomerular basement membrane thickening in db/db mice compared to db/m mice. However, these injuries were alleviated with KPF treatment (Figure 3A). Then, Western blot (WB) and IHC analysis were conducted to determine nephrin expression levels, while Immunofluorescence (IF) analysis determine WT1 expression levels. The levels of these two podocyte-specific proteins (nephrin and WT1) were significantly reduced in glomeruli of db/db mice relative to db/m mice, which was significantly ameliorated with KPF treatment (Figures 3B–D). These results indicated that KPF exerts a podocyte protection effect in a mice model of DN.
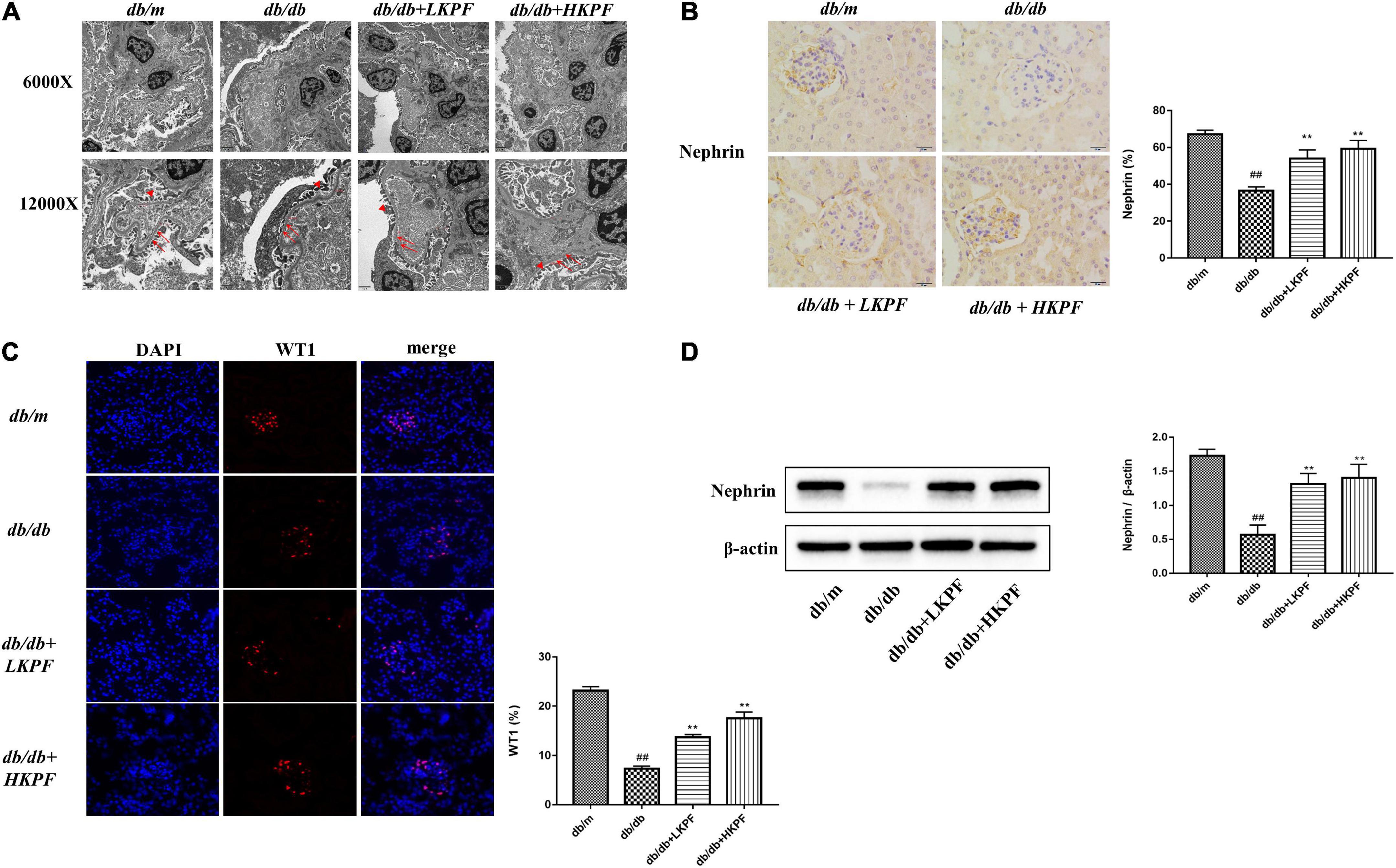
Figure 3. Kaempferol attenuated podocytes injury in db/db mice. (A) TEM (6000×, bar = 2 μm and 12000×, bar = 1 μm) micrograph, showing the GBM thickness (arrows) and FPs width (triangle). (B) Immunohistochemical (IHC) analysis of nephrin positive cells (400×, bar = 20 μm) and quantification of IHC staining for nephrin (n = 5). (C) IF analysis of podocyte nucleoprotein WT1 in kidney tissue. The tissues were stained with an antibody against WT1 (red), DAPI (blue) was used to stain the cellular nucleus (400×, n = 5). And quantification of IF stained for WT1 (n = 5). (D) WB assay and quantitative analysis of nephrin expression after KPF treatment, β-actin was used as internal references; data from each group are expressed as the mean ± SD (n = 3) from three repeated WB experiments. The data were presented as the mean ± SD (##p < 0.01 vs. db/m mice; **p < 0.01 vs. db/db mice). TEM, Transmission electron microscopy; IHC, Immunohistochemical; WB, Western blot; GBM, glomerular basement membrane; FPs, foot processes.
Kaempferol reduced apoptosis in the kidneys of db/db mice
TUNEL staining was used to assess cell apoptosis in the kidneys of db/db mice. Our results indicated that the number of TUNEL-positive cells significantly increased in the kidneys of db/db mice, whereas KPF treatment reduced kidney cell apoptosis (Figure 4A). To further determine the effect of KPF on apoptosis in the kidneys of db/db mice, the apoptosis-related proteins were assessed by WB. The pro-apoptotic proteins Bax and Caspase 3 were upregulated and the anti-apoptotic protein Bcl-2 was downregulated in db/db mice in comparison to db/m mice, which were reversed by KPF treatment (Figure 4B). Moreover, the results of IHC analysis were consistent with the WB results (Figure 4C), which revealed that the apoptosis process was increased in the kidneys of db/db mice and decreased by KPF treatment.
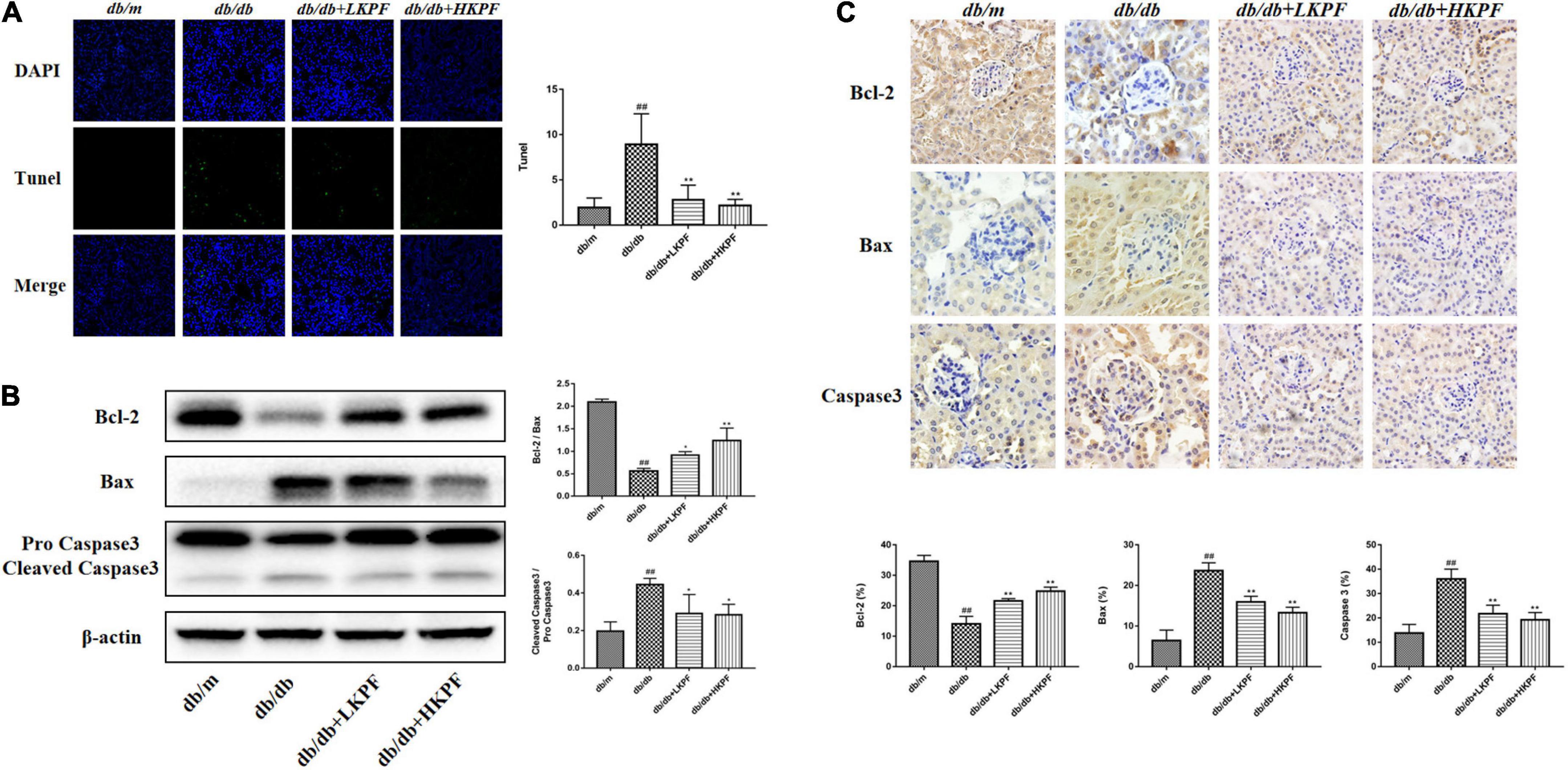
Figure 4. Kaempferol reduced apoptosis in the kidneys of db/db mice. (A) TUNEL-positive cells (green) were stained in glomeruli after Kaempferol treatment, DAPI (blue) was used to stain the cellular nucleus (400×, n = 3). (B) WB assay and quantitative analysis of apoptosis-related proteins Bcl-2, Bax, and Caspase 3 expressions after Kaempferol treatment, β-actin was used as internal references; data from each group were expressed as the mean ± SD (n = 3) from three repeated WB experiments. (C) IHC assay and quantitative analysis of apoptosis-related proteins: Bcl-2, Bax, and Caspase 3 (400×, n = 5). (##p < 0.01 vs. db/m mice; *p < 0.05 and **p < 0.01 vs. db/db mice). IHC, Immunohistochemical; WB, Western blot.
Kaempferol enhanced autophagy in podocytes and regulated AMPK/mTOR pathways in the kidneys of db/db mice
Transmission electron microscopy was used to detect the presence of autophagosomes, as shown by the red arrowheads in Figure 5A. The results revealed a decreased number of autophagosomes in podocytes in db/db mice compared with db/m mice, which was elevated with KPF treatment (Figure 5A). We also assessed the expression of LC3II in podocytes by IF, which was decreased in db/db mice in comparison with db/m mice and was reversed by KPF treatment (Figure 5B). The WB analysis of autophagy-related proteins (LC3II, p62, Beclin1, and Atg7) and IHC analysis of Atg5 supported the above results (Figures 5C,D). Furthermore, we assessed the protein expression of AMPK, mTOR and their phosphorylation protein both by WB and IHC. Compared with db/m mice, the expression of mTOR phosphorylation (p-mTOR) was increased and the expression of AMPK phosphorylation (p-AMPK) was decreased in db/db mice, which were reversed by KPF treatment (Figures 6A,B). These data indicated that KPF could enhance autophagy in podocytes and regulate AMPK/mTOR pathways in DN.
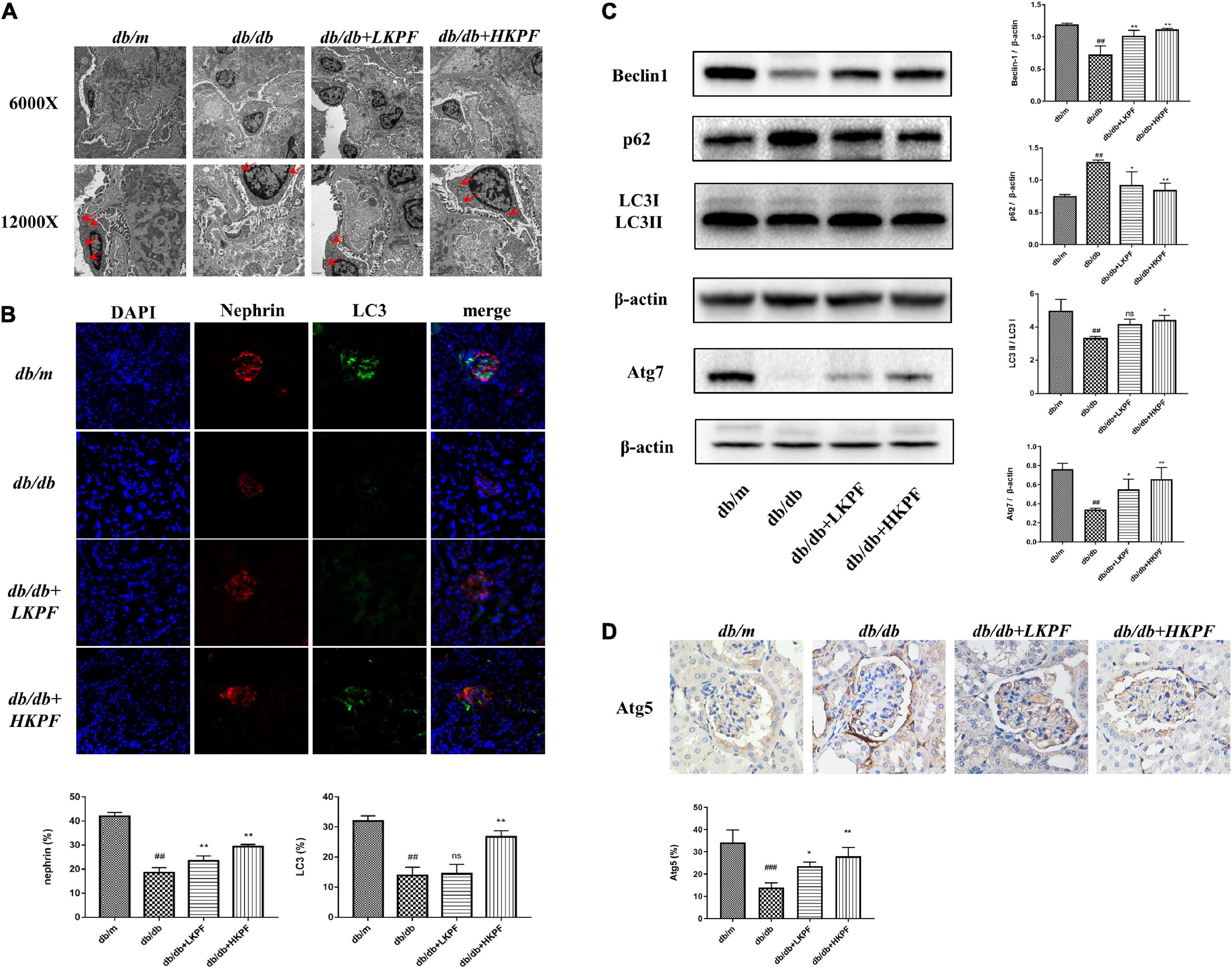
Figure 5. Kaempferol enhanced autophagy in podocytes in the kidneys of db/db mice. (A) Autophagosome formation was detected in kidneys by using TEM (6000×, bar = 2 μm and 12000×, bar = 1 μm), red arrows indicated autophagosomes. (B) IF analysis of the autophagosome marker LC3II and podocyte marker protein nephrin in kidney tissue. The tissues were stained with antibodies against LC3II (green) and nephrin (red). DAPI (blue) was used to stain the cellular nucleus (400×, n = 5). (C) WB assay and quantitative analysis of p62, Beclin1, LC3, and Atg7 expressions after Kaempferol treatment, β-actin was used as internal references; data from each group are expressed as the mean ± SD (n = 3) from three repeated WB experiments. (D) IHC assay and quantitative analysis of apoptosis-related protein: Atg5 (400×, n = 3). #p < 0.05, ##p < 0.01 and ###p < 0.001 vs. db/m mice; *p < 0.05 and **p < 0.01 vs. db/db mice; ns, not significant). TEM, Transmission electron microscopy; IF, Immunofluorescence; WB, Western blot.
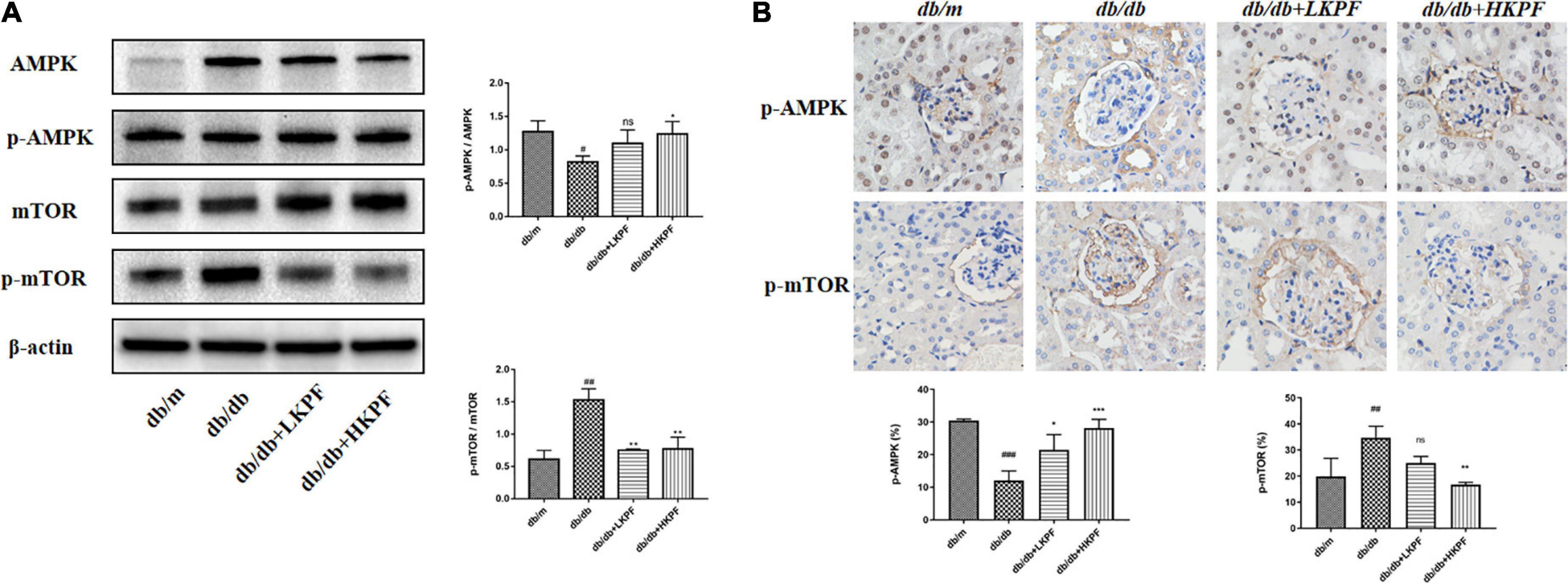
Figure 6. Kaempferol regulated AMPK/mTOR pathways in the kidneys of db/db mice. (A) WB assay and quantitative analysis of AMPK, p-AMPK, mTOR, and p-mTOR expressions after Kaempferol treatment, β-actin was used as internal references; data from each group are expressed as the mean ± SD (n = 3) from three repeated WB experiments. (B) IHC assay and quantitative analysis of p-AMPK and p-mTOR expressions (400×, n = 3). (##p < 0.01 and ###p < 0.001 vs. db/m mice; *p < 0.05, **p < 0.01, and ***p < 0.001 vs. db/db mice; ns, not significant). IHC, Immunohistochemical; WB, Western blot.
Discussion
This study demonstrated that Kaempferol (KPF) could significantly reduce UACR, improve glycolipid metabolism dysfunction, as well as reduce renal histopathological damage, especially podocytes injury of db/db mice via reducing apoptosis and promoting podocytes autophagy, which is correlated with the regulation of AMPK/mTOR pathways.
Proteinuria is the hallmark of DN and has been widely regarded as an independent risk factor for renal accelerated damage, which is caused by the damage of glomerular filtration barrier, including renal mesangial expansion, extracellular matrix accumulation, especially podocyte injury (27, 28). Podocyte dysfunction has been considered a breakthrough in deciphering the molecular mechanisms of DN (29).
Nephrin and WT1 have been used as biomarkers for the evaluation of podocyte damage. Our data showed decreased expressions of nephrin and WT1, accompanied by foot processes fusion and glomerular basement membrane thickening in the kidneys of db/db mice, which were significantly ameliorated with KPF treatment, showing the protective effect of KPF on podocyte in DN. This finding in our study was consistent with the previous findings by Yuanping Li et al. (30), and Xinyu Wang et al. (31), which showed the inhibition effect of KPF on podocyte apoptosis under hyperglycemic conditions in vitro. Impaired autophagy mediated imbalance of homeostasis in podocytes, contributing to podocyte dysfunction and further renal injury in DN (32). In the present study, indicators of autophagy such as light chain 3 (LC3), p62 (SQSTM1/sequestosome 1), Beclin-1, Atg5, and Atg7 were assessed. LC3 is now widely regarded as a monitor of autophagy activity. The detection of LC3 conversion (LC3-I to LC3-II) is the most reliable approach to evaluate autophagy activity (33). An alternative approach to detect autophagic flux is to measure the degradation of p62 (34). As a selective autophagy substrate, p62 can bind LC3, which indicates its significance in monitoring autophagy suppression (35). As one of the first autophagy effectors identified in mammals (36), Beclin-1 could interact with PtdIns (3)-kinase (Vps34) and initiate the nucleation step of autophagy to begin autophagic flux and also participates in following steps involving the fusion of autophagosomes to lysosomes (37). Atg5 and Atg7 are both essential for the formation of microtubule-associated protein 1 light chain 3-phosphatidylethanolamine (MAP1LC3-PE) conjugation and autophagosome formation (38, 39), mediating membrane expansion and maturation of autophagosomes. In our findings, KPF treatment increased the numbers of autophagosomes in podocytes, the double-membrane vesicles newly formed during autophagy. In addition, KPF effectively promoted the expression of LC3II in podocytes, with an increased expression of Beclin-1, Atg5, Atg7 and a decreased expression of p62 in the kidneys of db/db mice, suggesting that the KPF could prevent DN via regulating podocyte autophagy, which has not been reported by previous studies.
A multitude of signaling molecules is involved in podocyte autophagy, including mTOR and AMPK (40). The inhibitory function of mTOR might be linked to nutrient signals to regulation of autophagy (41). The hyperactivation of the mTOR pathway in DN is crucial to the injury of podocytes (42). AMPK could maintain energy homeostasis by serving as a cellular energy status sensor (43). AMPK has been implicated in autophagy induction in response to glucose starvation (44). Promoting AMPK activation could protect podocytes from high glucose-induced injury (45). Our results revealed the upregulation of AMPK phosphorylation as well as the downregulation of mTOR phosphorylation in KPF treated db/db mice. Overall, we demonstrated that KPF treatment alleviated podocyte autophagy dysfunction in db/db mice.
Collectively, our findings indicate the renal protective effect of KPF on type 2 DN db/db mice that KPF treatment reduced apoptosis and enhanced podocytes autophagy through AMPK/mTOR pathways. However, the exact mechanism of how KPF regulated AMPK/mTOR pathway remains to be investigated.
Conclusion
In summary, our study demonstrated that KPF could decrease UACR, improve glycolipid metabolism dysfunction and podocytes injury of db/db mice. Its protective effect might be attributed to reducing apoptosis and enhancing podocytes autophagy, which are correlated with regulating AMPK/mTOR pathways. Our findings may be implicated in the potential therapeutic role of KPF in future DN treatment. Further research on how KPF regulated AMPK/mTOR pathway is warranted.
Data availability statement
The original contributions presented in this study are included in the article/supplementary material, further inquiries can be directed to the corresponding author.
Ethics statement
The animal study was reviewed and approved by the Animal Care and Ethics Committee of Guangzhou University of Chinese Medicine.
Author contributions
LZ acquired funding for the research, contributed to the conception and design of the experiments, and analysis and interpretation of the data. HS drafted the manuscript. HS and DZ contributed to the performance of the experiments and the acquisition and analysis of data. DZ, JZ, YZ, and ZL contributed to the performance of some experiments and analysis of data. WM contributed to providing experimental suggestions. XL contributed to the design of the experiment and edited this manuscript. All authors reviewed the manuscript and approved the final version to be published.
Funding
This project was supported in part by grants from the State Key Laboratory of Dampness Syndrome of Chinese Medicine (Grant No: SZ2021ZZ16), National Natural Science Foundation of China (Grant No: 81603717), and Guangzhou Science and Technology Project (Grant No: 202206010076).
Conflict of interest
The authors declare that the research was conducted in the absence of any commercial or financial relationships that could be construed as a potential conflict of interest.
Publisher’s note
All claims expressed in this article are solely those of the authors and do not necessarily represent those of their affiliated organizations, or those of the publisher, the editors and the reviewers. Any product that may be evaluated in this article, or claim that may be made by its manufacturer, is not guaranteed or endorsed by the publisher.
References
1. Flyvbjerg A. The role of the complement system in diabetic nephropathy. Nat Rev Nephrol. (2017) 13:311–8. doi: 10.1038/nrneph.2017.31
2. Tagawa A, Yasuda M, Kume S, Yamahara K, Nakazawa J, Chin-Kanasaki M, et al. Impaired podocyte autophagy exacerbates proteinuria in diabetic nephropathy. Diabetes. (2016) 65:755–67. doi: 10.2337/db15-0473
3. Zhang P, Fang J, Zhang J, Ding S, Gan D. Curcumin inhibited podocyte cell apoptosis and accelerated cell autophagy in diabetic nephropathy via regulating Beclin1/UVRAG/Bcl2. Diabetes Metab Syndr Obes. (2020) 13:641–52. doi: 10.2147/DMSO.S237451
4. Fang L, Li X, Luo Y, He W, Dai C, Yang J, et al. Autophagy inhibition induces podocyte apoptosis by activating the pro-apoptotic pathway of endoplasmic reticulum stress. Exp Cell Res. (2014) 322:290–301. doi: 10.1016/j.yexcr.2014.01.001
5. Mizushima N, Levine B, Cuervo AM, Klionsky DJ. Autophagy fights disease through cellular self-digestion. Nature. (2008) 451:1069–75. doi: 10.1038/nature06639
6. Lenoir O, Jasiek M, Henique C, Guyonnet L, Hartleben B, Bork T, et al. Endothelial cell and podocyte autophagy synergistically protect from diabetes-induced glomerulosclerosis. Autophagy. (2015) 11:1130–45. doi: 10.1080/15548627.2015.1049799
7. Yang D, Livingston MJ, Liu Z, Dong G, Zhang M, Chen JK, et al. Autophagy in diabetic kidney disease: regulation, pathological role and therapeutic potential. Cell Mol Life Sci. (2018) 75:669–88. doi: 10.1007/s00018-017-2639-1
8. Ding DF, You N, Wu XM, Xu JR, Hu AP, Ye XL, et al. Resveratrol attenuates renal hypertrophy in early-stage diabetes by activating AMPK. Am J Nephrol. (2010) 31:363–74. doi: 10.1159/000300388
9. Fantus D, Rogers NM, Grahammer F, Huber TB, Thomson AW. Roles of mTOR complexes in the kidney: implications for renal disease and transplantation. Nat Rev Nephrol. (2016) 12:587–609. doi: 10.1038/nrneph.2016.108
10. Godel M, Hartleben B, Herbach N, Liu S, Zschiedrich S, Lu S, et al. Role of mTOR in podocyte function and diabetic nephropathy in humans and mice. J Clin Invest. (2011) 121:2197–209. doi: 10.1172/JCI44774
11. Puelles VG, van der Wolde JW, Wanner N, Scheppach MW, Cullen-McEwen LA, Bork T, et al. MTOR-mediated podocyte hypertrophy regulates glomerular integrity in mice and humans. JCI Insight. (2019) 4:e99271. doi: 10.1172/jci.insight.99271
12. Kim J, Kundu M, Viollet B, Guan KL. AMPK and mTOR regulate autophagy through direct phosphorylation of Ulk1. Nat Cell Biol. (2011) 13:132–41. doi: 10.1038/ncb2152
13. Hu Q, Qu C, Xiao X, Zhang W, Jiang Y, Wu Z, et al. Flavonoids on diabetic nephropathy: advances and therapeutic opportunities. Chin Med. (2021) 16:74. doi: 10.1186/s13020-021-00485-4
14. Calderon-Montano JM, Burgos-Moron E, Perez-Guerrero C, López-Lázaro M. A review on the dietary flavonoid kaempferol. Mini Rev Med Chem. (2011) 11:298–344. doi: 10.2174/138955711795305335
15. Gomez-Zorita S, Lasa A, Abendano N, Fernández-Quintela A, Mosqueda-Solís A, Garcia-Sobreviela MP, et al. Phenolic compounds apigenin, hesperidin and kaempferol reduce in vitro lipid accumulation in human adipocytes. J Transl Med. (2017) 15:237. doi: 10.1186/s12967-017-1343-0
16. Sharma D, Kumar TR, Kalia K. Kaempferol in ameliorating diabetes-induced fibrosis and renal damage: an in vitro and in vivo study in diabetic nephropathy mice model. Phytomedicine. (2020) 76:153235. doi: 10.1016/j.phymed.2020.153235
17. Varshney R, Varshney R, Mishra R, Gupta S, Sircar D, Roy P, et al. Kaempferol alleviates palmitic acid-induced lipid stores, endoplasmic reticulum stress and pancreatic beta-cell dysfunction through AMPK/mTOR-mediated lipophagy. J Nutr Biochem. (2018) 57:212–27. doi: 10.1016/j.jnutbio.2018.02.017
18. Varshney R, Gupta S, Roy P. Cytoprotective effect of kaempferol against palmitic acid-induced pancreatic beta-cell death through modulation of autophagy via AMPK/mTOR signaling pathway. Mol Cell Endocrinol. (2017) 448:1–20. doi: 10.1016/j.mce.2017.02.033
19. El-Kott AF, Bin-Meferij MM, Eleawa SM, Alshehri MM. Kaempferol protects against cadmium chloride-induced memory loss and hippocampal apoptosis by increased intracellular glutathione stores and activation of PTEN/AMPK induced inhibition of Akt/mTOR signaling. Neurochem Res. (2020) 45:295–309. doi: 10.1007/s11064-019-02911-4
20. Yuan Y, Xia F, Gao R, Chen Y, Zhang Y, Cheng Z, et al. Kaempferol mediated AMPK/mTOR signal pathway has a protective effect on cerebral ischemic-reperfusion injury in rats by inducing autophagy. Neurochem Res. (2022) 47:2187–97. doi: 10.1007/s11064-022-03604-1
21. Huang WW, Tsai SC, Peng SF, Lin MW, Chiang JH, Chiu YJ, et al. Kaempferol induces autophagy through AMPK and AKT signaling molecules and causes G2/M arrest via downregulation of CDK1/cyclin B in SK-HEP-1 human hepatic cancer cells. Int J Oncol. (2013) 42:2069–77. doi: 10.3892/ijo.2013.1909
22. Filomeni G, Desideri E, Cardaci S, Graziani I, Piccirillo S, Rotilio G, et al. Carcinoma cells activate AMP-activated protein kinase-dependent autophagy as survival response to kaempferol-mediated energetic impairment. Autophagy. (2010) 6:202–16. doi: 10.4161/auto.6.2.10971
23. Luo W, Chen X, Ye L, Chen X, Jia W, Zhao Y, et al. Kaempferol attenuates streptozotocin-induced diabetic nephropathy by downregulating TRAF6 expression: the role of TRAF6 in diabetic nephropathy. J Ethnopharmacol. (2021) 268:113553. doi: 10.1016/j.jep.2020.113553
24. Alshehri AS. Kaempferol attenuates diabetic nephropathy in streptozotocin-induced diabetic rats by a hypoglycaemic effect and concomitant activation of the Nrf-2/Ho-1/antioxidants axis. Arch Physiol Biochem. (2021) 24:1–14. doi: 10.1080/13813455.2021.1890129
25. Sharma D, Gondaliya P, Tiwari V, Kalia K. Kaempferol attenuates diabetic nephropathy by inhibiting RhoA/Rho-kinase mediated inflammatory signalling. Biomed Pharmacother. (2019) 109:1610–9. doi: 10.1016/j.biopha.2018.10.195
26. Alkhalidy H, Moore W, Wang Y, Luo J, McMillan RP, Zhen W, et al. The flavonoid kaempferol ameliorates streptozotocin-induced diabetes by suppressing hepatic glucose production. Molecules. (2018) 23:2338. doi: 10.3390/molecules23092338
27. Chang DY, Li MR, Yu XJ, Wang SX, Chen M, Zhao MH. Clinical and pathological characteristics of patients with nonproteinuric diabetic nephropathy. Front Endocrinol. (2021) 12:761386. doi: 10.3389/fendo.2021.761386
28. Zhou L, Chen X, Lu M, Wu Q, Yuan Q, Hu C, et al. Wnt/beta-catenin links oxidative stress to podocyte injury and proteinuria. Kidney Int. (2019) 95:830–45. doi: 10.1016/j.kint.2018.10.032
29. Wolf G, Chen S, Ziyadeh FN. From the periphery of the glomerular capillary wall toward the center of disease: podocyte injury comes of age in diabetic nephropathy. Diabetes. (2005) 54:1626–34. doi: 10.2337/diabetes.54.6.1626
30. Li Y, Zheng D, Shen D, Zhang X, Zhao X, Liao H. Protective effects of two safflower derived compounds, kaempferol and hydroxysafflor yellow A, on hyperglycaemic stress-induced podocyte apoptosis via modulating of macrophage M1/M2 polarization. J Immunol Res. (2020) 2020:2462039. doi: 10.1155/2020/2462039
31. Wang X, Xiang J, Huang G, Kang L, Yang G, Wu H, et al. Inhibition of podocytes DPP4 activity is a potential mechanism of Lobeliae chinensis herba in treating diabetic kidney disease. Front Pharmacol. (2021) 12:779652. doi: 10.3389/fphar.2021.779652
32. Shintani T, Klionsky DJ. Autophagy in health and disease: a double-edged sword. Science. (2004) 306:990–5. doi: 10.1126/science.1099993
33. Mizushima N, Yoshimori T. How to interpret LC3 immunoblotting. Autophagy. (2007) 3:542–5. doi: 10.4161/auto.4600
34. Bjorkoy G, Lamark T, Brech A, Outzen H, Perander M, Overvatn A, et al. p62/SQSTM1 forms protein aggregates degraded by autophagy and has a protective effect on huntingtin-induced cell death. J Cell Biol. (2005) 171:603–14. doi: 10.1083/jcb.200507002
35. Wang QJ, Ding Y, Kohtz DS, Mizushima N, Cristea IM, Rout MP, et al. Induction of autophagy in axonal dystrophy and degeneration. J Neurosci. (2006) 26:8057–68. doi: 10.1523/JNEUROSCI.2261-06.2006
36. Liang XH, Jackson S, Seaman M, Brown K, Kempkes B, Hibshoosh H, et al. Induction of autophagy and inhibition of tumorigenesis by beclin 1. Nature. (1999) 402:672–6. doi: 10.1038/45257
37. Matsunaga K, Saitoh T, Tabata K, Omori H, Satoh T, Kurotori N, et al. Two beclin 1-binding proteins, Atg14L and Rubicon, reciprocally regulate autophagy at different stages. Nat Cell Biol. (2009) 11:385–96. doi: 10.1038/ncb1846
38. Kuma A, Hatano M, Matsui M, Yamamoto A, Nakaya H, Yoshimori T, et al. The role of autophagy during the early neonatal starvation period. Nature. (2004) 432:1032–6. doi: 10.1038/nature03029
39. Huang Q, Liu Y, Zhang S, Yap YT, Li W, Zhang D, et al. Autophagy core protein ATG5 is required for elongating spermatid development, sperm individualization and normal fertility in male mice. Autophagy. (2021) 17:1753–67. doi: 10.1080/15548627.2020.1783822
40. Jia J, Abudu YP, Claude-Taupin A, Gu Y, Kumar S, Choi SW, et al. Galectins control MTOR and AMPK in response to lysosomal damage to induce autophagy. J Autophagy. (2019) 15:169–71. doi: 10.1080/15548627.2018.1505155
41. Chang YY, Juhasz G, Goraksha-Hicks P, Arsham AM, Mallin DR, Muller LK, et al. Nutrient-dependent regulation of autophagy through the target of rapamycin pathway. Biochem Soc Trans. (2009) 37(Pt. 1):232–6. doi: 10.1042/BST0370232
42. Chen JK, Chen J, Neilson EG, Harris RC. Role of mammalian target of rapamycin signaling in compensatory renal hypertrophy. J Am Soc Nephrol. (2005) 16:1384–91. doi: 10.1681/ASN.2004100894
43. Hardie DG. AMP-activated/SNF1 protein kinases: conserved guardians of cellular energy. Nat Rev Mol Cell Biol. (2007) 8:774–85. doi: 10.1038/nrm2249
44. Vingtdeux V, Giliberto L, Zhao H, Chandakkar P, Wu Q, Simon JE, et al. AMP-activated protein kinase signaling activation by resveratrol modulates amyloid-beta peptide metabolism. J Biol Chem. (2010) 285:9100–13. doi: 10.1074/jbc.M109.060061
Keywords: diabetic nephropathy, Kaempferol, apoptosis, autophagy, AMPK/mTOR pathway
Citation: Sheng H, Zhang D, Zhang J, Zhang Y, Lu Z, Mao W, Liu X and Zhang L (2022) Kaempferol attenuated diabetic nephropathy by reducing apoptosis and promoting autophagy through AMPK/mTOR pathways. Front. Med. 9:986825. doi: 10.3389/fmed.2022.986825
Received: 05 July 2022; Accepted: 14 November 2022;
Published: 30 November 2022.
Edited by:
Dong Zhou, University of Connecticut, United StatesReviewed by:
Yuan Gui, University of Connecticut, United StatesYuqun Zeng, Zhejiang Provincial People’s Hospital, China
Copyright © 2022 Sheng, Zhang, Zhang, Zhang, Lu, Mao, Liu and Zhang. This is an open-access article distributed under the terms of the Creative Commons Attribution License (CC BY). The use, distribution or reproduction in other forums is permitted, provided the original author(s) and the copyright owner(s) are credited and that the original publication in this journal is cited, in accordance with accepted academic practice. No use, distribution or reproduction is permitted which does not comply with these terms.
*Correspondence: Lei Zhang, emhhbmdsZWlAZ3p1Y20uZWR1LmNu
†These authors have contributed equally to this work