- 1Department of Urology, The Second Affiliated Hospital of Jiaxing University, Jiaxing, Zhejiang, China
- 2Department of Surgery, The Second Affiliated Hospital of Jiaxing University, Jiaxing, Zhejiang, China
Spindle Pole Body Component 25 (SPC25), a critical component of the NDC80 kinetochore complex, plays an essential role in maintaining chromosomal stability during mitosis. Recent studies have revealed its aberrant expression in various cancers, highlighting its potential as both a diagnostic biomarker and a therapeutic target. This review provides a comprehensive analysis of the molecular mechanisms underlying SPC25’s involvement in tumorigenesis, including its regulation of cell cycle progression and interaction with key oncogenic pathways. Furthermore, we discuss its prognostic significance across different cancer types and its potential impact on therapy resistance. The emerging evidence underscores SPC25’s multifaceted role in cancer biology, offering novel insights into its clinical applications. We conclude by exploring future research directions, emphasizing the need for in-depth studies to unravel the precise molecular functions of SPC25 and its therapeutic potential in cancer treatment.
1 Introduction
Cancer remains one of the leading causes of morbidity and mortality worldwide, characterized by its heterogeneity and complexity at the molecular, cellular, and clinical levels (1–3). Spindle Pole Body Component 25 (SPC25), a core subunit of the NDC80 kinetochore complex, is essential for accurate chromosome alignment and segregation during mitosis (4–6). By linking microtubules to kinetochores, SPC25 ensures proper attachment and tension generation, critical for the maintenance of genomic stability (7, 8). Aberrant expression of SPC25 disrupts these processes, leading to aneuploidy, which are frequently observed in malignant cells (9–11). Emerging evidence suggests that SPC25 is not merely a passive player in cell division but also participates in oncogenic signaling pathways, making it an intriguing target for cancer research (4, 9, 12).
In recent years, studies have highlighted the overexpression of SPC25 in various cancers, including lung, breast, gastric, and colorectal cancers (CRC) (10, 13–15). Its elevated levels are often associated with aggressive tumor phenotypes, poor prognosis, and resistance to therapy, underscoring its potential as a diagnostic and prognostic biomarker (15–17). Moreover, the role of SPC25 in modulating the tumor microenvironment (TME) and its crosstalk with other cellular pathways have further expanded its relevance in cancer biology (18, 19). Despite these advances, a comprehensive understanding of SPC25’s molecular mechanisms and clinical implications in cancer remains incomplete.
This review aims to provide a detailed examination of the current knowledge regarding SPC25 in cancer biology. We begin by exploring the structural and functional characteristics of SPC25 within the NDC80 complex, followed by its dysregulation in different tumor types and its impact on tumor progression. Furthermore, we discuss its emerging roles in cancer progression, including its involvement in oncogenic pathways and therapy resistance. Also, we highlight the potential of SPC25 as a biomarker and therapeutic target (Figure 1). Finally, we discuss future research directions and how to take full advantage of its clinical potential.
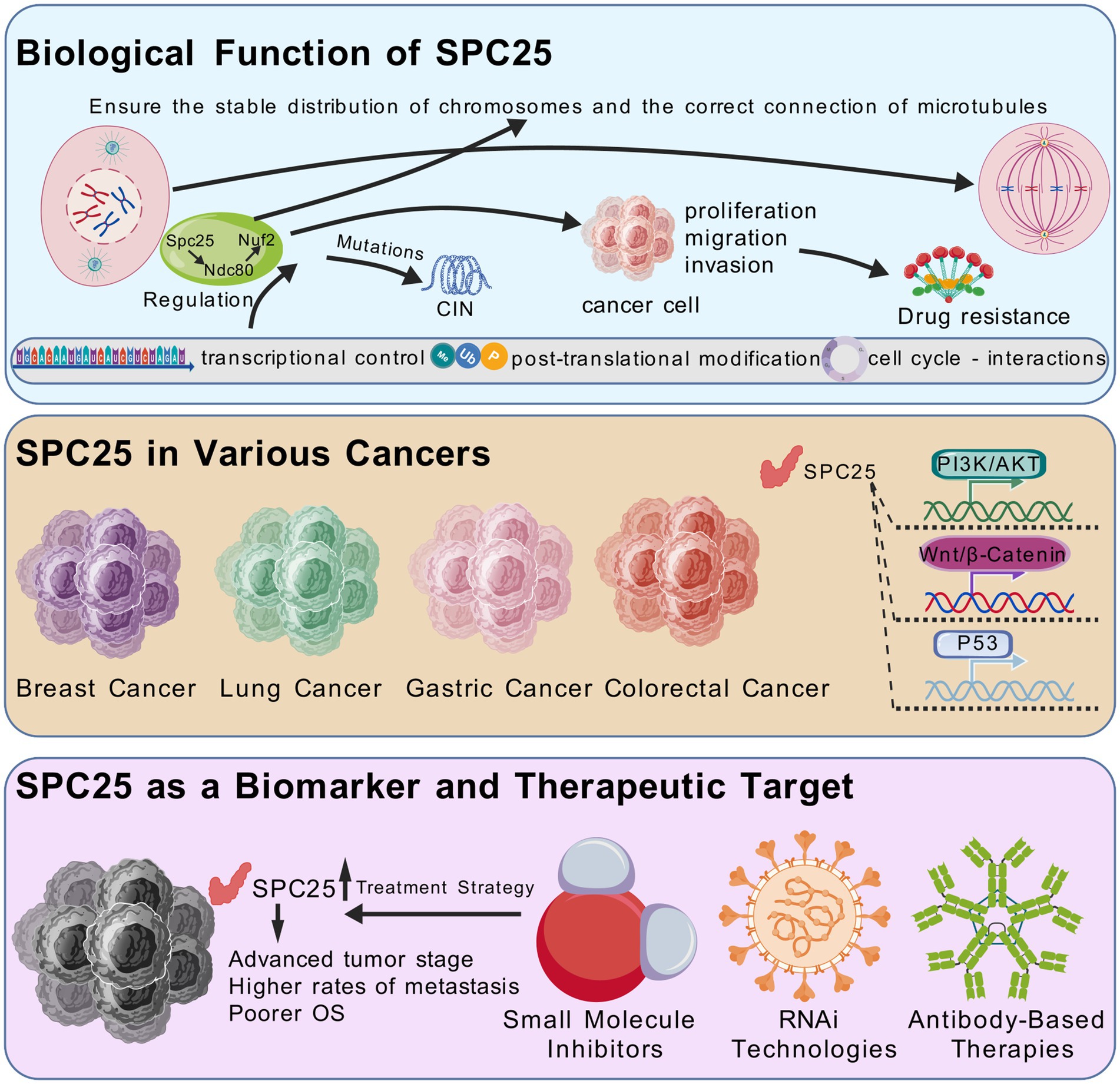
Figure 1. SPC25 is an important part of the NDC80 centromere complex. SPC25 ensures the stability of the centromate-microtubule attachment through interactions with other NDC80 complex components, thus maintaining chromosome integrity. Its function is strictly regulated by multiple layers of transcriptional regulation, post-translational modification, and cell cycle dependent interactions. SPC25 not only plays an important role in chromosome stability and cell division, but also interacts with a variety of key signaling pathways for tumor cell proliferation, survival and metastasis. These pathways include the Wnt/β-catenin pathway, PI3K/AKT pathway, and p53 signaling pathway. Thus, dysregulation of SPC25 in various cancers makes it a promising candidate for biomarker development and targeted therapy.
By consolidating existing evidence, this article seeks to bridge the gap between basic research and clinical applications, offering novel insights into the role of SPC25 in cancer and its implications for the development of innovative diagnostic and therapeutic strategies.
2 Biological function of SPC25
SPC25 is an essential component of the NDC80 kinetochore complex, which plays a central role in mitosis by mediating the attachment of chromosomes to the spindle microtubules (5, 12, 20–22). The NDC80 complex, consisting of NDC80, SPC25, SPC24, and HEC1, facilitates the proper alignment and segregation of chromosomes during cell division (23–27). SPC25, through its interactions with other NDC80 complex components, ensures the stability of kinetochore-microtubule attachments, thus maintaining chromosomal integrity (5).
2.1 SPC25 in mitosis and chromosome segregation
Accurate chromosome segregation during mitosis is critical for cell division and genetic stability (28, 29). SPC25 plays an essential role in this process by facilitating the proper attachment of chromosomes to the mitotic spindle microtubules through its interaction with the NDC80 complex. The NDC80 complex, which is a key component of the kinetochore, bridges the inner kinetochore to the outer microtubule-binding layer, ensuring stable chromosome alignment at the metaphase plate and segregation during anaphase (30). The NDC80 complex comprises four subunits, including SPC25, SPC24, NDC80, and NUF2, and is structured as an elongated rod with globular domains at each end, critical for its function in mitosis (31).
SPC25 interacts with SPC24 to stabilize the NDC80 complex, allowing it to maintain stable association with microtubules throughout mitosis (4, 25). The C-terminal domain of SPC25 directly binds to microtubules, facilitating dynamic regulation during cell division. This microtubule-binding interface involves a cooperative, electrostatic interaction, which is essential for kinetochore-microtubule attachment (32). Disruption of SPC25 function or its binding to microtubules leads to defective chromosome alignment and segregation (33–35).
Recent structural insights into the NDC80 complex have revealed that SPC25, along with SPC24, forms a globular structure that directly interacts with the microtubules, a critical process for accurate chromosome segregation. Mutations or malfunctions in SPC25 hinder this interaction, resulting in misaligned chromosomes and aneuploidy (20, 31).
2.2 Regulation of SPC25
The function of SPC25, as a core component of the NDC80 complex, is tightly regulated through multiple layers, including transcriptional control, post-translational modifications, and cell cycle-dependent interactions. Understanding these regulatory mechanisms is crucial to uncovering the full range of SPC25’s roles in mitosis.
The expression of SPC25 is primarily regulated at the transcriptional level, where various transcription factors likely play a role. Studies have suggested that certain oncogenic signaling pathways, such as PI3K/AKT and Wnt/β-catenin, can modulate the transcription of genes involved in mitotic progression, including SPC25 (17, 36–38). These pathways, which are frequently dysregulated in cancer, could lead to the overexpression of SPC25, contributing to tumor progression. Further studies are needed to identify the specific transcription factors or regulatory elements that control SPC25 expression in different cancer types, as well as how these pathways integrate with the cellular environment.
SPC25 is subject to various post-translational modifications, which are critical for its function in mitosis. Phosphorylation is one of the most prominent modifications regulating SPC25’s activity. Phosphorylation of SPC25 during mitosis is likely mediated by kinases such as Cyclin-dependent kinases and Aurora B kinase, which are essential for proper chromosome alignment and segregation (39, 40). Phosphorylation could regulate SPC25’s interaction with other components of the NDC80 complex or with microtubules, thus modulating its function during the mitotic phase (20, 36, 39–41).
Ubiquitination is another important modification that may control SPC25 stability and its turnover. The precise regulation of SPC25 degradation is crucial for maintaining mitotic fidelity, as premature degradation or accumulation of SPC25 could lead to chromosome missegregation (42, 43). But there is still no research to explore this in depth. Additionally, acetylation, methylation, and SUMOylation may also play roles in regulating SPC25 activity, although these modifications require further exploration. Such modifications can affect SPC25’s interactions with other mitotic regulators and may provide another layer of control in the maintenance of chromosomal stability.
SPC25’s activity is intricately linked to the progression of the cell cycle, with its function being highly dependent on cell cycle-dependent interactions with key mitotic proteins (4, 44). During mitosis, SPC25 forms stable interactions with its binding partners in the NDC80 complex, including SPC24, NDC80, and NUF2, to ensure proper chromosome alignment and segregation. These interactions are dynamically regulated, with SPC25 being recruited to the kinetochore at the onset of mitosis and dissociating during telophase (4, 5, 45, 46).
At specific stages of the cell cycle, SPC25 may also interact with other regulatory proteins to modulate its function. For example, interactions with Aurora B kinase during mitosis help to regulate the attachment of kinetochores to microtubules, ensuring proper chromosome alignment and preventing premature segregation (8, 39, 40). Similarly, SPC25 may interact with checkpoint proteins, such as Mad2, to monitor and ensure that all chromosomes are properly attached to the spindle before anaphase proceeds (33, 47). These interactions are essential for maintaining genomic stability, and any disruption of the cell cycle-dependent regulation of SPC25 can contribute to cancer progression.
In summary, the regulation of SPC25 through transcriptional control, post-translational modifications, and cell cycle-dependent interactions is essential for its role in mitosis and chromosomal stability. Dysregulation of these mechanisms can lead to SPC25 overexpression or malfunction, which contributes to cancer progression. Understanding these regulatory processes in greater detail will offer valuable insights into the therapeutic potential of targeting SPC25 in cancer.
2.3 SPC25 and cancer progression
Beyond its role in mitosis, SPC25 also contributes to the progression of cancer by influencing various cellular processes such as proliferation, migration, and invasion (48, 49). The overexpression of SPC25 in cancer cells enhances cell cycle progression, particularly through its involvement in the G2/M transition (50). SPC25 stabilizes the NDC80 complex and promotes the efficient separation of chromatids during mitosis, which accelerates cell division. This, in turn, facilitates the rapid proliferation of cancer cells.
In addition to promoting cell proliferation, SPC25 has been implicated in enhancing cancer cell migration and invasion, two key processes involved in tumor metastasis (15, 51, 52). Research has demonstrated that SPC25 interacts with signaling pathways known to regulate cell migration, such as the PI3K/AKT and MAPK pathways (36, 37, 53). These interactions may contribute to the ability of cancer cells to migrate through tissues and invade distant organs. Furthermore, SPC25’s role in maintaining genomic stability and promoting cell proliferation may help establish a TME conducive to the survival and expansion of cancer cells (54, 55).
2.4 SPC25 and chemotherapy resistance
In addition to its involvement in tumor progression, SPC25 plays a critical role in cancer cell resistance to chemotherapy and targeted therapies (14, 17, 56, 57). Chemotherapy agents that target microtubules, such as paclitaxel, work by stabilizing microtubules and disrupting their dynamic instability, leading to mitotic arrest and cell death (58, 59). However, cancer cells often develop resistance to these therapies through various mechanisms, including the dysregulation of mitotic regulators like SPC25.
SPC25 overexpression has been shown to enhance the stability of kinetochore-microtubule attachment, which can result in a more stable microtubule-kinetochore interaction (5, 9). While this stabilizing effect may be synergistic with the actions of paclitaxel in promoting microtubule stabilization, it can paradoxically reduce the effectiveness of paclitaxel by potentially mitigating the disruption of microtubule dynamics that is critical for drug efficacy. The enhanced stability of kinetochore-microtubule attachments may make it more difficult for paclitaxel to further disrupt the mitotic spindle, thereby impairing the drug’s ability to induce mitotic failure.
Additionally, SPC25 may contribute to resistance by regulating DNA repair mechanisms. Cancer cells with elevated SPC25 levels may be better equipped to repair DNA damage induced by chemotherapy, allowing them to survive and proliferate despite treatment (14, 60). This ability to overcome chemotherapy-induced cell death highlights SPC25 as a potential target for overcoming therapy resistance in cancer treatment.
3 SPC25 in different cancers: recent advances
Numerous studies have investigated the expression and functional implications of SPC25 across various tumor types, including breast cancer, lung cancer, gastric cancer (GC), and CRC. These studies have provided valuable insights into the clinical significance and molecular mechanisms of SPC25, as well as its interactions with key signaling pathways. A summary of these relevant studies is presented in Table 1, which highlights the expression levels, prognostic value, and functional roles of SPC25 in these cancers. This section will review the current knowledge regarding these aspects of SPC25, with a focus on its contribution to cancer progression and its potential as a therapeutic target.
3.1 SPC25 in various cancers
3.1.1 Breast cancer
Breast cancer, one of the most common malignancies worldwide, is characterized by high genomic instability, which plays a significant role in tumor progression (61–63). Studies have shown that SPC25 is overexpressed in a significant proportion of breast cancer cases. Overexpression of SPC25 has been associated with advanced tumor stages, higher histologic grades, and worse overall survival (OS) and disease-free survival in breast cancer patients (54, 56, 60). For instance, a study by Wang et al. demonstrated that SPC25 expression was significantly higher in breast cancer tissues compared to normal adjacent tissues, and its high expression was correlated with poor prognosis. Additionally, SPC25 overexpression may be linked to tumor evolution and resistance to treatment (11, 14, 56).
3.1.2 Lung cancer
Lung cancer is another malignancy in which SPC25 plays a pivotal role. Both non-small cell lung cancer (NSCLC) and small cell lung cancer exhibit elevated SPC25 levels. In NSCLC, SPC25 overexpression correlates with tumor size, lymph node metastasis, and poor prognosis (13, 15, 37). SPC25 is thought to promote lung cancer progression by regulating cell cycle progression and inhibiting apoptosis (52, 64, 65). For example, a study by Chen et al. found that SPC25 upregulation can increase cancer stem cell (CSC) properties in lung adenocarcinoma (LUAD) and independently predict poor survival in this histological subtype (15). Liu et al. showed that inhibiting SPC25 expression significantly affected the proliferation, migration and apoptosis of LUAD cells. Abnormal SPC25 expression levels can affect cell cycle progression, glycolysis capacity and regulation of ferroptosis (52). These findings suggest that SPC25 may serve as a potential biomarker for prognosis and a therapeutic target for lung cancer.
3.1.3 Gastric cancer
GC is one of the leading causes of cancer-related mortality globally, and its prognosis remains poor due to late-stage diagnosis and metastasis (66–68). Recent studies have revealed that SPC25 is frequently overexpressed in GC tissues. SPC25 overexpression has been associated with more aggressive tumor phenotypes, including larger tumor size, increased lymphatic invasion, and higher rates of metastasis (10, 13). A study by Zeng et al. reported that after the knockout of SPC25, the viability and proliferation of GC cells were significantly reduced (10). This suggests that SPC25 contributes to GC progression by promoting tumor cell proliferation and migration through its involvement in cell cycle regulation and mitotic progression.
3.1.4 Hepatocellular carcinoma
In hepatocellular carcinoma (HCC), SPC25 exhibits multifaceted oncogenic roles. Clinically, its high expression correlates with poor prognosis, metastasis, and early recurrence (36, 38). Mechanistically, SPC25 promotes DNA damage in HCC cells, activating the DNA-PK/Akt/Notch1 cascade. This triggers the NICD/RBP-Jκ complex to transcriptionally upregulate stemness factors SOX2 and NANOG, driving tumor proliferation and self-renewal (9). Additionally, SPC25 enhances metastasis by modulating ECM-integrin interactions: its silencing suppresses HCC cell invasion/migration via downregulating ITGB4, while rescuing ITGB4 restores FAK/PI3K/AKT phosphorylation, reactivating integrin signaling (36). Furthermore, SPC25 is integrated into a Wnt/PRC1-driven positive feedback loop – PRC1 (a Wnt target) transcriptionally regulates SPC25, KIF11, and KIF23, which collectively amplify Wnt signaling to accelerate HCC recurrence (38). These findings position SPC25 as both a prognostic biomarker and a multi-pathway therapeutic target in HCC. More studies are summarized in Table 1.
3.1.5 Colorectal cancer
In CRC, SPC25 has been implicated in both the development and progression of the disease (13, 69). Elevated SPC25 expression has been linked to poor prognosis and aggressive features in CRC, including advanced tumor stage and distant metastasis. A study by Naoyuki et al. showed that SPC25 was significantly overexpressed in CRC tissue samples compared to adjacent normal tissue. Additionally, the expression levels of tumor/normal ratios of CDCA1, KNTC2, SPC24 and SPC25 correlated with each other in CRCs, and the cell growths after the small interfering RNA (siRNA)-mediated knockdown were significantly suppressed (13). SPC25 contributes to CRC progression by promoting cell cycle progression, inhibiting apoptosis, and enhancing cell migration. Furthermore, SPC25’s interaction with microtubules in the mitotic spindle facilitates the maintenance of genomic stability, which may be a hallmark of CRC.
3.2 SPC25 and its interaction with key signaling pathways
SPC25 not only contributes to chromosomal stability and cell division but also interacts with various critical signaling pathways involved in cancer cell proliferation, survival, and metastasis. These pathways include the Wnt/β-catenin pathway, PI3K/AKT pathway, and p53 signaling pathway, all of which are frequently dysregulated in cancer (17, 36–38, 70–72).
3.2.1 Wnt/β-catenin signaling pathway
The Wnt/β-catenin signaling pathway is crucial for regulating cell proliferation, differentiation, and migration (73–75). Aberrant activation of Wnt signaling is commonly observed in many cancers, including colorectal, gastric, and breast cancers (76–78). SPC25 has been shown to interact with this pathway, promoting tumorigenesis by enhancing Wnt/β-catenin activity (Figure 2). In epithelial ovarian cancer, SPC25 acts as a scaffolding platform, orchestrating the assembly of an SPC25/RIOK1/MYH9 trimeric complex, triggering RIOK1-mediated phosphorylation of MYH9 at Ser1943. This prompts MYH9 to disengage from the cytoskeleton, augmenting its nuclear accumulation, thus potentiating CTNNB1 transcription and subsequent activation of Wnt/β-catenin signaling (17). It has also been reported that PRC1 controls the expression and function of wrrag such as FANCI, SPC25, KIF11, and KIF23 via Wnt signaling in hepatocellular carcinoma (HCC) (38).
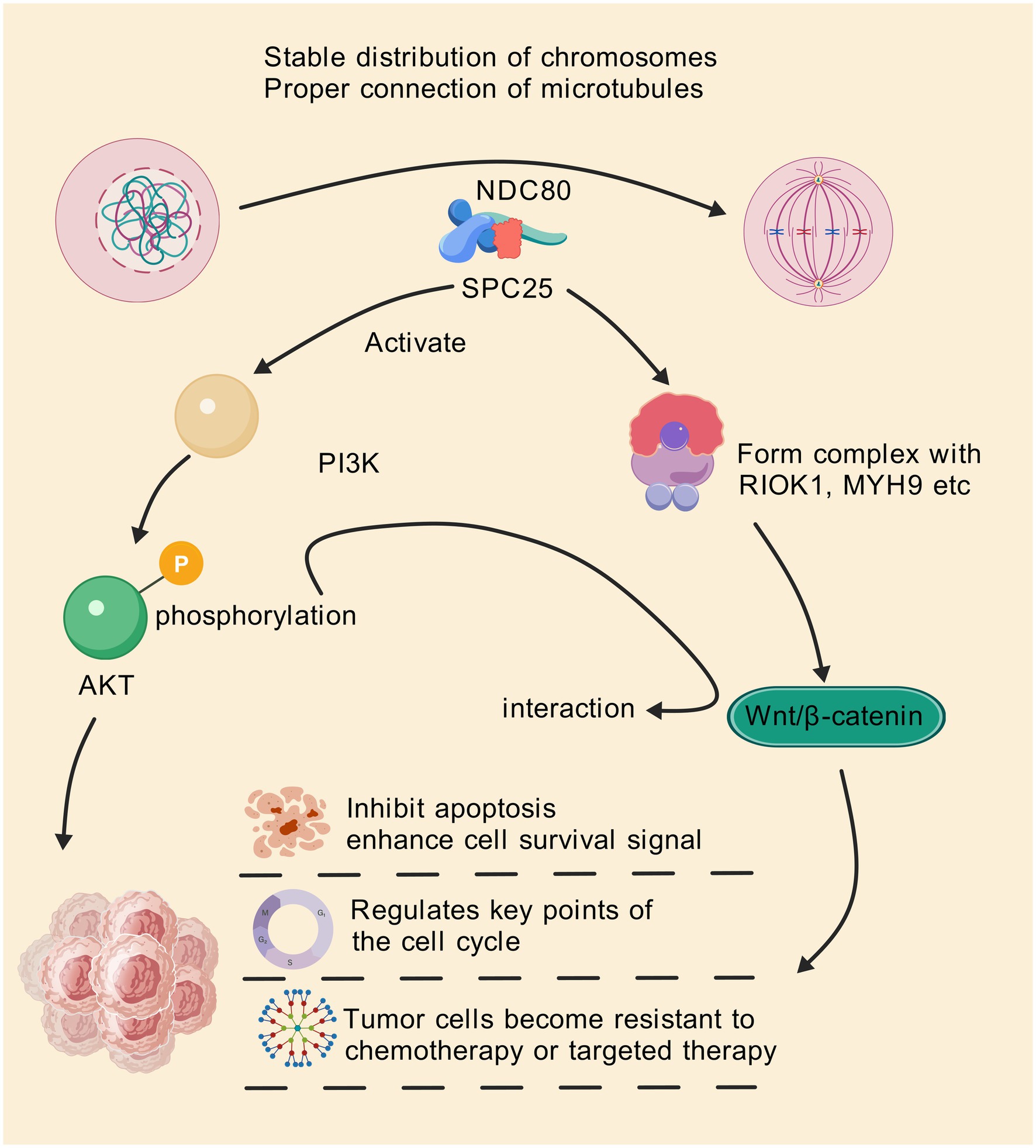
Figure 2. SPC25 can be used as a scaffolding platform to coordinate the assembly of the SPC25/RIOK1/MYH9 trimer complex, triggering RIOK1-mediated phosphorylation of MYH9 Ser1943. This prompts MYH9 to break away from the cytoskeleton and increase its nuclear accumulation, thereby enhancing CTNNB1 transcription and subsequent activation of the Wnt/β-catenin signaling pathway. In addition, SPC25 is also involved in abnormal activation of the PI3K/AKT pathway, which helps to improve cell survival and resist apoptosis. Through these pathways, SPC25 inhibits apoptosis of cancer cells and enhances survival signals of cancer cells, regulates key nodes in the cell cycle, and makes tumor cells resistant to therapy.
3.2.2 PI3K/AKT pathway
The PI3K/AKT signaling pathway is essential for regulating cell survival, growth, and metabolism (79–81). Aberrant activation of the PI3K/AKT pathway is a common feature of various cancers, including breast, lung, and colorectal cancers (80, 82).SPC25’s involvement in this pathway has been well-documented, with studies showing that SPC25 promotes PI3K/AKT activation in tumor cells, contributing to enhanced cell survival and resistance to apoptosis (36, 37, 53) (Figure 2). Study has reported that SPC25 is highly expressed in HCC, and high expression is associated with poor prognosis and metastasis. SPC25 preferentially affects the expression of genes associated with extracellular matrix (ECM) -integrin interactions, including the integrin subunit β4 (ITGB4), an upstream element of the integrin pathway. Up-regulation of ITGB4 partially reversed the decline in cell invasion and migration caused by SPC25 silencing. In addition, deletion of SPC25 and ITGB4 resulted in reduced phosphorylation of focal adhesion kinase (FAK), PI3K, and AKT, which are downstream elements of the integrin pathway. These results demonstrate the important role of SPC25 as a prognostic indicator and metastasis promoter in HCC, suggesting that SPC25 can be used as a biomarker and therapeutic intervention target for HCC treatment (36).
3.2.3 p53 pathway
The tumor suppressor p53 is a critical regulator of cell cycle arrest, DNA repair, and apoptosis. In many cancers, p53 is mutated or functionally inactivated, leading to unchecked cell proliferation (83–85). SPC25 has been found to interact with the p53 pathway, where its overexpression may inhibit p53, contributing to cancer cell survival. For example, in HCC, SPC25 knockdown can significantly reduce the proliferation and metastasis of HCC cells and increase the protein levels of p53 pathway components (70).
4 SPC25 as a biomarker and therapeutic target: potential and perspectives
SPC25, as an essential component of the NDC80 kinetochore complex, plays a pivotal role in mitosis, maintaining chromosomal stability, and promoting tumorigenesis. Its dysregulation in various cancers has positioned SPC25 as a promising candidate for both biomarker development and targeted therapy. In this section, we explore the potential of SPC25 as a biomarker for early diagnosis and prognosis, as well as its value as a therapeutic target in cancer treatment.
4.1 SPC25 as a biomarker for diagnosis and prognosis
The overexpression of SPC25 in numerous cancers, including breast, lung, gastric, and colorectal cancers, has highlighted its potential as a diagnostic and prognostic biomarker. Elevated SPC25 levels are frequently associated with advanced tumor stages, higher rates of metastasis, and poor OS in cancer patients. Studies have demonstrated that SPC25 is upregulated in tumor tissues compared to adjacent normal tissues, and its expression often correlates with aggressive tumor features, such as larger tumor size and lymph node involvement (17, 86).
For instance, in breast cancer, SPC25 expression has been shown to correlate with high histological grade and poor prognosis (54, 60). In lung cancer, SPC25 levels are positively correlated with tumor size and lymph node metastasis, indicating its potential to serve as a marker for tumor progression (15, 37, 64). In CRC, high SPC25 expression has been linked to poor survival outcomes, reinforcing its prognostic value (13). Given these associations, SPC25 could serve as a reliable biomarker for early detection of aggressive cancers, providing clinicians with valuable information to guide treatment strategies.
In addition, the use of SPC25 as a biomarker for liquid biopsy is also one of the potential areas of research. Liquid biopsies, which involve the analysis of circulating tumor DNA, exosomes, or proteins from blood or other bodily fluids, offer a non-invasive alternative to traditional tissue biopsies (87–89). Given its elevated expression in blood samples from cancer patients, SPC25 could potentially be detected in the circulation, offering a promising tool for monitoring disease progression, recurrence, and response to therapy (90, 91). Liquid biopsy assays targeting SPC25 could provide a real-time snapshot of tumor dynamics, enabling more personalized and timely treatment decisions.
4.2 Therapeutic targeting of SPC25: opportunities and challenges
The functional significance of SPC25 in regulating mitosis, chromosomal stability, and cell proliferation presents a compelling rationale for its targeting in cancer therapy. Given its central role in maintaining kinetochore-microtubule attachment during cell division, disrupting SPC25 function could lead to mitotic errors, cell cycle arrest, and apoptosis in rapidly proliferating cancer cells. Several therapeutic strategies are being explored to target SPC25 and its associated pathways, including small molecule inhibitors, RNA interference (RNAi) technologies, and immunotherapies.
4.2.1 Small molecule inhibitors
Small molecules designed to inhibit SPC25 could disrupt its interactions within the NDC80 complex or prevent its binding to microtubules, leading to mitotic defects and cell death. While direct targeting of SPC25 with small molecules remains an area of ongoing research, inhibitors of other mitotic regulators, such as Aurora kinases and Polo-like kinases, have demonstrated some progress (92, 93). These agents exert their effects by disrupting mitotic spindle assembly and promoting chromosomal misalignment, thereby inducing tumor cell death. Given the role of SPC25 in stabilizing the kinetochore-microtubule complex, small molecules targeting SPC25 could potentially mimic these effects, leading to mitotic catastrophe in cancer cells.
4.2.2 RNAi technologies
RNAi technologies, including siRNAs and short hairpin RNAs, offer another promising strategy to target SPC25. By silencing SPC25 expression, RNAi could impair its function, leading to cell cycle arrest and apoptosis (13, 38, 45). Several preclinical studies have demonstrated the effectiveness of RNAi-mediated knockdown of SPC25 in inhibiting cancer cell proliferation and reducing tumor growth (42, 94). The development of RNA-based therapeutics targeting SPC25 could offer a highly specific and effective treatment approach with minimal off-target effects, though challenges in delivery and stability remain.
4.2.3 Antibody-based therapies
Another potential therapeutic approach involves the use of monoclonal antibodies that specifically target SPC25. These antibodies could inhibit SPC25’s interaction with other mitotic machinery components or block its binding to microtubules, ultimately leading to impaired mitosis and cancer cell death. However, developing highly specific and potent antibodies against SPC25 may be challenging, given its involvement in essential cellular processes. Nevertheless, antibody-based therapies targeting other mitotic regulators, such as kinesins or microtubule-associated proteins, have shown promise in preclinical studies, providing a basis for developing similar strategies against SPC25 (95–97).
4.3 Clinical perspective: feasibility and challenges
Despite the promising therapeutic potential of targeting SPC25, several challenges remain before its clinical application can become a reality. One major hurdle is the identification of small molecules or biologics that can selectively target SPC25 without affecting other components of the cell cycle machinery, which are essential for normal cell function. Since SPC25 is involved in fundamental processes like mitosis, the risk of off-target effects and toxicity to normal, non-cancerous cells must be carefully evaluated.
Additionally, the development of effective delivery systems for SPC25-targeting agents is crucial. RNA-based therapies, such as siRNA, face challenges related to their stability, delivery to tumor cells, and off-target effects. Nanoparticle-based delivery systems have shown promise in overcoming these challenges by enabling the targeted delivery of RNAi molecules to tumor cells (98, 99). Similarly, small molecules or antibody-based therapies targeting SPC25 will require careful optimization to enhance their specificity, bioavailability, and therapeutic efficacy.
Finally, the heterogeneity of cancer cells and the potential for tumor cells to develop resistance to SPC25-targeting therapies are important considerations. Combining SPC25-targeted therapies with other therapeutic modalities, such as chemotherapy, immunotherapy, or checkpoint inhibitors, may enhance their efficacy and prevent resistance.
5 Challenges and future research directions
The growing body of evidence highlighting the role of SPC25 in cancer progression has brought this protein into the spotlight as both a potential biomarker and therapeutic target. However, despite promising data, several challenges remain that limit the full clinical translation of SPC25-targeted strategies. In this section, we discuss the limitations of current research and outline future research directions that could help address these challenges and further elucidate the role of SPC25 in cancer. While considerable progress has been made in understanding the role of SPC25 in various cancers, several limitations in current studies hinder a comprehensive understanding of its function and therapeutic potential.
One of the primary limitations of existing research is the relatively narrow range of cancer types in which SPC25 expression and function have been investigated. Most studies focus on commonly studied cancers such as liver, breast, lung, and colorectal cancers, with limited research on other malignancies like pancreatic cancer, and glioblastoma. The lack of comprehensive data across different cancer types restricts the generalizability of SPC25 as a universal biomarker or therapeutic target. Future studies should focus on expanding the range of cancers examined to provide a more holistic view of SPC25’s role in tumorigenesis and its potential as a target in diverse cancer types.
Although there is growing evidence linking SPC25 to key biological processes such as mitosis and chromosomal stability, the underlying molecular mechanisms remain insufficiently characterized. Many of the studies to date have focused on the correlation between SPC25 expression and tumor progression, but the specific signaling pathways or molecular interactions through which SPC25 exerts its oncogenic effects are not fully understood. For instance, while SPC25 is known to interact with the NDC80 complex and affect microtubule attachment, its precise involvement in modulating key cell cycle regulators and tumor suppressor pathways such as p53, Wnt/β-catenin, and PI3K/AKT requires further investigation. More in-depth studies are needed to uncover the detailed molecular networks that SPC25 is part of, which could offer more precise insights into how SPC25 contributes to cancer progression.
Despite the promising potential of SPC25 as a therapeutic target, the development of specific inhibitors against this protein is still in its infancy. Currently, no small molecule inhibitors or monoclonal antibodies specifically targeting SPC25 are available, and much of the preclinical evidence supporting its therapeutic targeting is based on knockdown or overexpression models. The lack of specific and selective inhibitors hampers the development of therapeutic strategies that can be clinically tested. Furthermore, while preclinical studies have demonstrated the potential of SPC25-targeted therapies, there is a significant gap in clinical trials and validation. Without robust clinical evidence, the translation of SPC25-targeted therapies from the laboratory to the clinic remains speculative.
To address these challenges, future research on SPC25 should focus on several key areas to further elucidate its role in cancer and improve its clinical applicability.
Future studies should broaden the scope of cancers examined for SPC25 expression and its clinical significance. In particular, cancers that are less well-studied, such as ovarian, and glioblastoma, should be investigated to determine whether SPC25 holds similar prognostic and therapeutic value. Clinical studies assessing the relationship between SPC25 expression levels and patient outcomes are essential for establishing its role as a reliable biomarker. Longitudinal studies with larger, diverse patient cohorts will be crucial for validating the clinical utility of SPC25 as a biomarker for early detection, prognosis, and therapeutic monitoring.
A critical area for future research is the detailed characterization of the molecular mechanisms through which SPC25 contributes to tumorigenesis. This includes identifying the upstream regulators and downstream effectors of SPC25 and understanding how it interacts with key signaling pathways involved in cancer progression, such as PI3K/AKT, MAPK, and p53. Furthermore, exploring how SPC25 modulates the TME, including interactions with stromal cells, immune cells, and EMC components, will provide valuable insights into its broader role in tumor progression and metastasis. By dissecting the molecular networks in which SPC25 participates, researchers can identify new therapeutic targets and biomarkers that can be used to enhance the efficacy of SPC25-targeted therapies.
Developing specific inhibitors of SPC25 is a critical next step in advancing its potential as a therapeutic target. High-throughput screening of small molecules or the development of monoclonal antibodies targeting SPC25 could lead to the identification of potent and selective inhibitors. These inhibitors could be tested in preclinical models to assess their ability to disrupt SPC25 function and inhibit tumor growth. Additionally, exploring novel drug delivery systems, such as nanoparticles or liposomes, may improve the bioavailability and specificity of SPC25-targeted therapies. Once validated in preclinical models, clinical trials assessing the safety and efficacy of SPC25-targeted therapies in cancer patients will be crucial for translating these findings into therapeutic applications.
The tumor immune microenvironment plays a crucial role in cancer progression and response to treatment, and recent studies suggest that SPC25 may influence immune cell function and immune evasion mechanisms (19, 54). Future research should focus on examining the interactions between SPC25 and various immune cell types within the tumor immune microenvironment, such as tumor-associated macrophages, T cells, and dendritic cells. Understanding how SPC25 modulates immune responses, including its potential to regulate immune checkpoint molecules like programmed cell death ligand 1 or cytotoxic T lymphocyte-associated protein 4, could provide new opportunities for combining SPC25-targeted therapies with immunotherapies, such as immune checkpoint inhibitors. At present, SPC25 has been shown to predict the efficacy of tumor immunotherapy, but the research on SPC25 inhibitors combined with immunotherapy is limited (54). Furthermore, investigating how SPC25 may contribute to chemotherapy and immunotherapy resistance could uncover new strategies to overcome treatment resistance and improve patient outcomes.
Cancer cells often develop resistance to chemotherapy, targeted therapies, and immunotherapies (100, 101), and SPC25 may play a role in this process. Research into the role of SPC25 in drug resistance mechanisms, including its involvement in DNA damage repair, cell cycle regulation, and apoptosis evasion, could provide new insights into how tumors become resistant to treatment. Understanding how SPC25 contributes to the development of resistance to specific therapies will be crucial for designing combination treatment strategies that target SPC25 alongside other resistance pathways.
While SPC25 is one component of the NDC80 complex, its structural features make it particularly attractive as a therapeutic target. Unlike other components such as SPC24 and HEC1, SPC25 has a unique C-terminal domain that directly interacts with microtubules, contributing to the stability of kinetochore-microtubule attachments during mitosis. This direct microtubule-binding ability distinguishes SPC25 from other NDC80 subunits, making it a key player in regulating the dynamics of chromosome segregation (33, 47, 102). Additionally, SPC25’s role in stabilizing the kinetochore-microtubule interface offers potential advantages in targeting mitotic progression, particularly in cancers where microtubule dynamics are disrupted by chemotherapy agents (4, 9). Targeting SPC25 could therefore offer a more specific approach to modulating mitotic functions compared to other NDC80 components, making it a promising candidate for therapeutic intervention in cancer treatment.
Although SPC25 has therapeutic potential in tumors as a key component of the NDC80 complex, its underlying function in normal mitosis may induce off-target toxicity. To improve targeting specificity, potential strategies can be based on the unique biology of cancer cells. The first is synthetic mortality: tumor cells are often accompanied by genomic instability (such as BRCA mutations or deletion of p53), making them more sensitive to microtubule-kinetosomal junction interference. Inhibition of SPC25 may form a synthetic lethal effect with a specific genetic background to selectively kill tumors (such as the synthetic lethal effect of PARP inhibitors and BRCA mutations (103)). Post-translational modification targeting is also one of the directions, but no studies have shown that SPC25-specific post-translational modifications (phosphorylation, ubiquitination) exist in cancer cells and can be used as targets. Local drug delivery or nanocarrier delivery is also a reliable option to limit the accumulation of inhibitors in tumor tissues through tumor microenvironment responsive drug delivery systems (such as PH-sensitive liposomes), reducing the risk of systemic exposure (104). But it’s worth noting. Current studies have not fully clarified the feasibility of the above strategy, and the future needs to be combined with conditional gene knockout models and tumor-specific promoter-driven inhibitor release techniques to verify its in vivo selectivity.
While significant progress has been made in understanding the role of SPC25 in cancer, substantial challenges remain in translating these findings into clinical applications. The expansion of cancer type studies, in-depth mechanistic research, and the development of specific inhibitors are all critical for advancing our understanding of SPC25 as a biomarker and therapeutic target. Future research should also focus on the relationship between SPC25 and the immune microenvironment, as well as its involvement in drug resistance mechanisms. By addressing these challenges and expanding the research on SPC25, we can unlock its full potential as a tool for cancer diagnosis, prognosis, and treatment.
Author contributions
YJ: Writing – review & editing, Writing – original draft. MC: Writing – original draft, Writing – review & editing. FC: Writing – original draft, Writing – review & editing. ZG: Writing – original draft, Writing – review & editing. XL: Writing – original draft, Writing – review & editing. LH: Writing – original draft, Writing – review & editing. DC: Writing – original draft, Conceptualization. SZ: Conceptualization, Writing – original draft, Visualization, Writing – review & editing. ZS: Visualization, Conceptualization, Writing – review & editing.
Funding
The author(s) declare that financial support was received for the research and/or publication of this article. This research was supported by the Medical Health Science and Technology Project of Zhejiang Provincial Health Commission (2022KY1246) and the Science and Technology Bureau of Jiaxing City (2023AZ31002 and 2022AZ10009).
Acknowledgments
We sincerely appreciate the potential editors and reviewers for their succinct comments on improving this manuscript, and Generic Diagramming Platform (GDP) (https://www.biogdp.com/) was used to create the figures (105).
Conflict of interest
The authors declare that the research was conducted in the absence of any commercial or financial relationships that could be construed as a potential conflict of interest.
Generative AI statement
The authors declare that no Gen AI was used in the creation of this manuscript.
Publisher’s note
All claims expressed in this article are solely those of the authors and do not necessarily represent those of their affiliated organizations, or those of the publisher, the editors and the reviewers. Any product that may be evaluated in this article, or claim that may be made by its manufacturer, is not guaranteed or endorsed by the publisher.
Abbreviations
SPC25, Spindle Pole Body Component 25; CRC, Colorectal cancer; TME, Tumor microenvironment; OS, Overall survival; NSCLC, Non-small cell lung cancer; CSC, Cancer stem cell; LUAD, Lung adenocarcinoma; GC, Gastric cancer; siRNA, Interfering RNA; HCC, Hepatocellular carcinoma; ECM, Extracellular matrix; ITGB4, Integrin subunit β4; FAK, Focal adhesion kinase; RNAi, RNA interference.
References
1. Sung, H, Ferlay, J, Siegel, RL, Laversanne, M, Soerjomataram, I, Jemal, A, et al. Global Cancer statistics 2020: GLOBOCAN estimates of incidence and mortality worldwide for 36 cancers in 185 countries. CA Cancer J Clin. (2021) 71:209–49. doi: 10.3322/caac.21660
2. Bray, F, Laversanne, M, Sung, H, Ferlay, J, Siegel, RL, Soerjomataram, I, et al. Global cancer statistics 2022: GLOBOCAN estimates of incidence and mortality worldwide for 36 cancers in 185 countries. CA Cancer J Clin. (2024) 74:229–63. doi: 10.3322/caac.21834
3. Hanahan, D, and Monje, M. Cancer hallmarks intersect with neuroscience in the tumor microenvironment. Cancer Cell. (2023) 41:573–80. doi: 10.1016/j.ccell.2023.02.012
4. McCleland, ML, Kallio, MJ, Barrett-Wilt, GA, Kestner, CA, Shabanowitz, J, Hunt, DF, et al. The vertebrate Ndc80 complex contains Spc24 and Spc25 homologs, which are required to establish and maintain kinetochore-microtubule attachment. Curr Biol. (2004) 14:131–7. doi: 10.1016/j.cub.2003.12.058
5. Kudalkar, EM, Scarborough, EA, Umbreit, NT, Zelter, A, Gestaut, DR, Riffle, M, et al. Regulation of outer kinetochore Ndc80 complex-based microtubule attachments by the central kinetochore Mis12/MIND complex. Proc Natl Acad Sci USA. (2015) 112:E5583–9. doi: 10.1073/pnas.1513882112
6. Nishino, T, Rago, F, Hori, T, Tomii, K, Cheeseman, IM, and Fukagawa, T. CENP-T provides a structural platform for outer kinetochore assembly. EMBO J. (2013) 32:424–36. doi: 10.1038/emboj.2012.348
7. Holmstrom, TH, Rehnberg, J, Ahonen, LJ, and Kallio, MJ. Ectopic expression of plasma membrane targeted subunits of the Ndc80-complex as a tool to study kinetochore biochemistry. Mol Oncol. (2009) 3:262–8. doi: 10.1016/j.molonc.2009.02.005
8. Zhao, G, Cheng, Y, Gui, P, Cui, M, Liu, W, Wang, W, et al. Dynamic acetylation of the kinetochore-associated protein HEC1 ensures accurate microtubule-kinetochore attachment. J Biol Chem. (2019) 294:576–92. doi: 10.1074/jbc.RA118.003844
9. Yang, J, Huang, Y, Song, M, Pan, Q, Zhao, J, He, J, et al. SPC25 promotes proliferation and stemness of hepatocellular carcinoma cells via the DNA-PK/AKT/Notch1 signaling pathway. Int J Biol Sci. (2022) 18:5241–59. doi: 10.7150/ijbs.71694
10. Zeng, Z, Zhang, X, Jiang, CQ, Zhang, YG, Wu, X, Li, J, et al. Identifying novel therapeutic targets in gastric cancer using genome-wide CRISPR-Cas9 screening. Oncogene. (2022) 41:2069–78. doi: 10.1038/s41388-022-02177-1
11. Jung, SY, Papp, JC, Sobel, EM, Pellegrini, M, and Yu, H. Genetic variants of glucose metabolism and exposure to smoking in African American breast cancer. Endocr Relat Cancer. (2023) 30:e220184. doi: 10.1530/ERC-22-0184
12. Asai, K, Zhou, Y, Takenouchi, O, and Kitajima, TS. Artificial kinetochore beads establish a biorientation-like state in the spindle. Science. (2024) 385:1366–75. doi: 10.1126/science.adn5428
13. Kaneko, N, Miura, K, Gu, Z, Karasawa, H, Ohnuma, S, Sasaki, H, et al. siRNA-mediated knockdown against CDCA1 and KNTC2, both frequently overexpressed in colorectal and gastric cancers, suppresses cell proliferation and induces apoptosis. Biochem Biophys Res Commun. (2009) 390:1235–40. doi: 10.1016/j.bbrc.2009.10.127
14. Pathania, R, Ramachandran, S, Mariappan, G, Thakur, P, Shi, H, Choi, JH, et al. Combined inhibition of DNMT and HDAC blocks the Tumorigenicity of Cancer stem-like cells and attenuates mammary tumor growth. Cancer Res. (2016) 76:3224–35. doi: 10.1158/0008-5472.CAN-15-2249
15. Chen, J, Chen, H, Yang, H, and Dai, H. SPC25 upregulation increases cancer stem cell properties in non-small cell lung adenocarcinoma cells and independently predicts poor survival. Biomed Pharmacother. (2018) 100:233–9. doi: 10.1016/j.biopha.2018.02.015
16. Arai, E, Gotoh, M, Tian, Y, Sakamoto, H, Ono, M, Matsuda, A, et al. Alterations of the spindle checkpoint pathway in clinicopathologically aggressive CpG island methylator phenotype clear cell renal cell carcinomas. Int J Cancer. (2015) 137:2589–606. doi: 10.1002/ijc.29630
17. Jiang, X, Yang, M, Zhang, W, Shi, D, Li, Y, He, L, et al. Targeting the SPC25/RIOK1/MYH9 Axis to overcome tumor Stemness and platinum resistance in epithelial ovarian Cancer. Adv Sci (Weinh). (2024) 11:e2406688. doi: 10.1002/advs.202406688
18. Cui, F, Xu, Z, Hu, J, and Lv, Y. Spindle pole body component 25 and platelet-derived growth factor mediate crosstalk between tumor-associated macrophages and prostate cancer cells. Front Immunol. (2022) 13:907636. doi: 10.3389/fimmu.2022.907636
19. Xia, F, Yang, H, Wu, H, and Zhao, B. Spindle component 25 predicts the prognosis and the immunotherapy response of cancers: a pan-cancer analysis. Sci Rep. (2024) 14:8452. doi: 10.1038/s41598-024-59038-y
20. Malvezzi, F, Litos, G, Schleiffer, A, Heuck, A, Mechtler, K, Clausen, T, et al. A structural basis for kinetochore recruitment of the Ndc80 complex via two distinct centromere receptors. EMBO J. (2013) 32:409–23. doi: 10.1038/emboj.2012.356
21. Wei, RR, Sorger, PK, and Harrison, SC. Molecular organization of the Ndc80 complex, an essential kinetochore component. Proc Natl Acad Sci USA. (2005) 102:5363–7. doi: 10.1073/pnas.0501168102
22. Roy, B, Varshney, N, Yadav, V, and Sanyal, K. The process of kinetochore assembly in yeasts. FEMS Microbiol Lett. (2013) 338:107–17. doi: 10.1111/1574-6968.12019
23. Sobajima, T, Kowalczyk, KM, Skylakakis, S, Hayward, D, Fulcher, LJ, Neary, C, et al. PP 6 regulation of Aurora A-TPX2 limits NDC80 phosphorylation and mitotic spindle size. J Cell Biol. (2023) 222:e202205117. doi: 10.1083/jcb.202205117
24. Zahm, JA, Stewart, MG, Carrier, JS, Harrison, SC, and Miller, MP. Structural basis of Stu 2 recruitment to yeast kinetochores. eLife. (2021) 10:e65389. doi: 10.7554/eLife.65389
25. Shin, J, Jeong, G, Park, JY, Kim, H, and Lee, I. MUN (MERISTEM UNSTRUCTURED), encoding a SPC24 homolog of NDC80 kinetochore complex, affects development through cell division in Arabidopsis thaliana. Plant J. (2018) 93:977–91. doi: 10.1111/tpj.13823
26. Hiruma, Y, Sacristan, C, Pachis, ST, Adamopoulos, A, Kuijt, T, Ubbink, M, et al. Competition between MPS1 and microtubules at kinetochores regulates spindle checkpoint signaling. Science. (2015) 348:1264–7. doi: 10.1126/science.aaa4055
27. Leem, J, Kim, JS, and Oh, JS. Oocytes can repair DNA damage during meiosis via a microtubule-dependent recruitment of CIP2A-MDC1-TOPBP1 complex from spindle pole to chromosomes. Nucleic Acids Res. (2023) 51:4899–913. doi: 10.1093/nar/gkad213
28. Quinton, RJ, DiDomizio, A, Vittoria, MA, Kotynkova, K, Ticas, CJ, Patel, S, et al. Whole-genome doubling confers unique genetic vulnerabilities on tumour cells. Nature. (2021) 590:492–7. doi: 10.1038/s41586-020-03133-3
29. Mazzagatti, A, Engel, JL, and Ly, P. Boveri and beyond: Chromothripsis and genomic instability from mitotic errors. Mol Cell. (2024) 84:55–69. doi: 10.1016/j.molcel.2023.11.002
30. Zahm, JA, Jenni, S, and Harrison, SC. Structure of the Ndc80 complex and its interactions at the yeast kinetochore-microtubule interface. Open Biol. (2023) 13:220378. doi: 10.1098/rsob.220378
31. Wei, RR, Schnell, JR, Larsen, NA, Sorger, PK, Chou, JJ, and Harrison, SC. Structure of a central component of the yeast kinetochore: the Spc24p/Spc25p globular domain. Structure. (2006) 14:1003–9. doi: 10.1016/j.str.2006.04.007
32. Ciferri, C, Pasqualato, S, Screpanti, E, Varetti, G, Santaguida, S, Dos Reis, G, et al. Implications for kinetochore-microtubule attachment from the structure of an engineered Ndc80 complex. Cell. (2008) 133:427–39. doi: 10.1016/j.cell.2008.03.020
33. Kim, S, Sun, H, Tomchick, DR, Yu, H, and Luo, X. Structure of human Mad1 C-terminal domain reveals its involvement in kinetochore targeting. Proc Natl Acad Sci USA. (2012) 109:6549–54. doi: 10.1073/pnas.1118210109
34. Rizzo, M, Stout, TAE, Cristarella, S, Quartuccio, M, Kops, G, and De Ruijter-Villani, M. Compromised MPS1 activity induces multipolar spindle formation in oocytes from aged mares: establishing the horse as a natural animal model to study age-induced oocyte meiotic spindle instability. Front Cell Dev Biol. (2021) 9:657366. doi: 10.3389/fcell.2021.657366
35. Rumpf, C, Cipak, L, Schleiffer, A, Pidoux, A, Mechtler, K, Tolic-Norrelykke, IM, et al. Laser microsurgery provides evidence for merotelic kinetochore attachments in fission yeast cells lacking pcs 1 or Clr 4. Cell Cycle. (2010) 9:3997–4004. doi: 10.4161/cc.9.19.13233
36. Shi, WK, Shang, QL, and Zhao, YF. SPC25 promotes hepatocellular carcinoma metastasis via activating the FAK/PI3K/AKT signaling pathway through ITGB4. Oncol Rep. (2022) 47:91. doi: 10.3892/or.2022.8302
37. Zhang, W, Yu, L, Xu, C, Tang, T, Cao, J, Chen, L, et al. PLEK2 activates the PI3K/AKT signaling pathway to drive lung adenocarcinoma progression by upregulating SPC25. Cell Biol Int. (2024) 48:1285–300. doi: 10.1002/cbin.12197
38. Chen, J, Rajasekaran, M, Xia, H, Zhang, X, Kong, SN, Sekar, K, et al. The microtubule-associated protein PRC1 promotes early recurrence of hepatocellular carcinoma in association with the Wnt/beta-catenin signalling pathway. Gut. (2016) 65:1522–34. doi: 10.1136/gutjnl-2015-310625
39. Cheeseman, IM, Chappie, JS, Wilson-Kubalek, EM, and Desai, A. The conserved KMN network constitutes the core microtubule-binding site of the kinetochore. Cell. (2006) 127:983–97. doi: 10.1016/j.cell.2006.09.039
40. Huis In 'T Veld, PJ, Volkov, VA, Stender, ID, Musacchio, A, and Dogterom, M. Molecular determinants of the Ska-Ndc80 interaction and their influence on microtubule tracking and force-coupling. eLife. (2019) 8:8. doi: 10.7554/eLife.49539
41. Wimbish, RT, DeLuca, KF, Mick, JE, Himes, J, Jimenez-Sanchez, I, Jeyaprakash, AA, et al. The Hec1/Ndc80 tail domain is required for force generation at kinetochores, but is dispensable for kinetochore-microtubule attachment formation and Ska complex recruitment. Mol Biol Cell. (2020) 31:1453–73. doi: 10.1091/mbc.E20-05-0286
42. Kim, S, Lau, TTY, Liao, MK, Ma, HT, and Poon, RYC. Coregulation of NDC80 complex subunits determines the Fidelity of the spindle-assembly checkpoint and mitosis. Mol Cancer Res. (2024) 22:423–39. doi: 10.1158/1541-7786.MCR-23-0828
43. Jiang, H, Jin, X, Gu, H, Li, B, Li, Z, and Sun, Y. SPC25 upregulates CCND1 to promote the progression of esophageal squamous cell carcinoma by inhibiting MDM2-mediated E2F1 ubiquitination. Transl Oncol. (2025) 53:102300:102300. doi: 10.1016/j.tranon.2025.102300
44. Li, J, Wang, Y, Zou, W, Jian, L, Fu, Y, and Zhao, J. AtNUF2 modulates spindle microtubule organization and chromosome segregation during mitosis. Plant J. (2021) 107:801–16. doi: 10.1111/tpj.15347
45. Sun, SC, Lee, SE, Xu, YN, and Kim, NH. Perturbation of Spc25 expression affects meiotic spindle organization, chromosome alignment and spindle assembly checkpoint in mouse oocytes. Cell Cycle. (2010) 9:4552–9. doi: 10.4161/cc.9.22.13815
46. Nilsson, J. Looping in on Ndc80 – how does a protein loop at the kinetochore control chromosome segregation? BioEssays. (2012) 34:1070–7. doi: 10.1002/bies.201200096
47. Vos, LJ, Famulski, JK, and Chan, GK. hZwint-1 bridges the inner and outer kinetochore: identification of the kinetochore localization domain and the hZw10-interaction domain. Biochem J. (2011) 436:157–68. doi: 10.1042/BJ20110137
48. Tran Nguyen, TM, Munhoven, A, Samel-Pommerencke, A, Kshirsagar, R, Cuomo, A, Bonaldi, T, et al. Methylation of CENP-A/Cse 4 on arginine 143 and lysine 131 regulates kinetochore stability in yeast. Genetics. (2023) 223:iyad028. doi: 10.1093/genetics/iyad028
49. Zhang, YJ, Sun, YZ, Gao, XH, and Qi, RQ. Integrated bioinformatic analysis of differentially expressed genes and signaling pathways in plaque psoriasis. Mol Med Rep. (2019) 20:225–35. doi: 10.3892/mmr.2019.10241
50. Cui, F, Hu, J, Fan, Y, Tan, J, and Tang, H. Knockdown of spindle pole body component 25 homolog inhibits cell proliferation and cycle progression in prostate cancer. Oncol Lett. (2018) 15:5712–20. doi: 10.3892/ol.2018.8003
51. Yamada, Y, Arai, T, Sugawara, S, Okato, A, Kato, M, Kojima, S, et al. Impact of novel oncogenic pathways regulated by antitumor miR-451a in renal cell carcinoma. Cancer Sci. (2018) 109:1239–53. doi: 10.1111/cas.13526
52. Liu, XS, Zhang, Y, Ming, X, Hu, J, Chen, XL, Wang, YL, et al. SPC25 as a novel therapeutic and prognostic biomarker and its association with glycolysis, ferroptosis and ce RNA in lung adenocarcinoma. Aging (Albany NY). (2024) 16:779–98. doi: 10.18632/aging.205418
53. Yu, W, Peng, X, Cai, X, Xu, H, Wang, C, Liu, F, et al. Transcriptome analysis of porcine oocytes during postovulatory aging. Theriogenology. (2024) 226:387–99. doi: 10.1016/j.theriogenology.2024.05.035
54. Liao, LX, Zhang, M, Xu, X, Zhang, S, and Guo, YZ. SPC25 functions as a prognostic-related biomarker, and its high expression correlates with tumor immune infiltration and UCEC progression. Front Biosci (Landmark Ed). (2024) 29:69. doi: 10.31083/j.fbl2902069
55. Liu, Y, Cheng, X, Xi, P, Zhang, Z, Sun, T, and Gong, B. Bioinformatic analysis highlights SNHG6 as a putative prognostic biomarker for kidney renal papillary cell carcinoma. BMC Urol. (2023) 23:54. doi: 10.1186/s12894-023-01218-5
56. Gao, C, Zhuang, J, Li, H, Liu, C, Zhou, C, Liu, L, et al. Development of a risk scoring system for evaluating the prognosis of patients with Her2-positive breast cancer. Cancer Cell Int. (2020) 20:121. doi: 10.1186/s12935-020-01175-1
57. Cui, F, Ning, S, Xu, Z, and Hu, J. Spindle pole body component 25 in the androgen-induced regression of castration-resistant prostate cancer. Transl Androl Urol. (2022) 11:519–27. doi: 10.21037/tau-22-214
58. Risinger, AL, Riffle, SM, Lopus, M, Jordan, MA, Wilson, L, and Mooberry, SL. The taccalonolides and paclitaxel cause distinct effects on microtubule dynamics and aster formation. Mol Cancer. (2014) 13:41. doi: 10.1186/1476-4598-13-41
59. Muller-Deku, A, Meiring, JCM, Loy, K, Kraus, Y, Heise, C, Bingham, R, et al. Photoswitchable paclitaxel-based microtubule stabilisers allow optical control over the microtubule cytoskeleton. Nat Commun. (2020) 11:4640. doi: 10.1038/s41467-020-18389-6
60. Wang, Q, Zhu, Y, Li, Z, Bu, Q, Sun, T, Wang, H, et al. Up-regulation of SPC25 promotes breast cancer. Aging (Albany NY). (2019) 11:5689–704. doi: 10.18632/aging.102153
61. Harbeck, N, and Gnant, M. Breast cancer. Lancet. (2017) 389:1134–50. doi: 10.1016/S0140-6736(16)31891-8
62. Barzaman, K, Karami, J, Zarei, Z, Hosseinzadeh, A, Kazemi, MH, Moradi-Kalbolandi, S, et al. Breast cancer: biology, biomarkers, and treatments. Int Immunopharmacol. (2020) 84:106535. doi: 10.1016/j.intimp.2020.106535
63. Cancer Genome Atlas Research NKandoth, C, Schultz, N, Cherniack, AD, Akbani, R, Liu, Y, et al. Integrated genomic characterization of endometrial carcinoma. Nature. (2013) 497:67–73. doi: 10.1038/nature12113
64. Sun, ZY, Wang, W, Gao, H, and Chen, QF. Potential therapeutic targets of the nuclear division cycle 80 (NDC80) complexes genes in lung adenocarcinoma. J Cancer. (2020) 11:2921–34. doi: 10.7150/jca.41834
65. Fukuizumi, A, Noro, R, Seike, M, Miyanaga, A, Minegishi, Y, Omori, M, et al. CADM1 and SPC25 gene mutations in lung Cancer patients with idiopathic pulmonary fibrosis. JTO Clin Res Rep. (2021) 2:100232. doi: 10.1016/j.jtocrr.2021.100232
66. Smyth, EC, Nilsson, M, Grabsch, HI, van Grieken, NC, and Lordick, F. Gastric cancer. Lancet. (2020) 396:635–48. doi: 10.1016/S0140-6736(20)31288-5
67. Chia, NY, and Tan, P. Molecular classification of gastric cancer. Ann Oncol. (2016) 27:763–9. doi: 10.1093/annonc/mdw040
68. Patel, TH, and Cecchini, M. Targeted therapies in advanced gastric Cancer. Curr Treat Options in Oncol. (2020) 21:70. doi: 10.1007/s11864-020-00774-4
69. Janke, C, Ortiz, J, Lechner, J, Shevchenko, A, Shevchenko, A, Magiera, MM, et al. The budding yeast proteins Spc24p and Spc25p interact with Ndc80p and Nuf 2p at the kinetochore and are important for kinetochore clustering and checkpoint control. EMBO J. (2001) 20:777–91. doi: 10.1093/emboj/20.4.777
70. Chen, F, Zhang, K, Huang, Y, Luo, F, Hu, K, and Cai, Q. SPC25 may promote proliferation and metastasis of hepatocellular carcinoma via p 53. FEBS Open Bio. (2020) 10:1261–75. doi: 10.1002/2211-5463.12872
71. Fang, Z, Wang, F, Zhang, M, Huang, H, and Lin, Z. Identification of co-expression modules and genes associated with tumor progression in Oral squamous cell carcinoma. Pathol Oncol Res. (2022) 28:1610481. doi: 10.3389/pore.2022.1610481
72. Zhang, Z, Wang, Q, Li, Y, Li, B, Zheng, L, and He, C. Hub genes and key pathways of intervertebral disc degeneration: bioinformatics analysis and validation. Biomed Res Int. (2021) 2021:5340449. doi: 10.1155/2021/5340449
73. Perugorria, MJ, Olaizola, P, Labiano, I, Esparza-Baquer, A, Marzioni, M, Marin, JJG, et al. Wnt-beta-catenin signalling in liver development, health and disease. Nat Rev Gastroenterol Hepatol. (2019) 16:121–36. doi: 10.1038/s41575-018-0075-9
74. Liu, J, Xiao, Q, Xiao, J, Niu, C, Li, Y, Zhang, X, et al. Wnt/beta-catenin signalling: function, biological mechanisms, and therapeutic opportunities. Signal Transduct Target Ther. (2022) 7:3. doi: 10.1038/s41392-021-00762-6
75. Schunk, SJ, Floege, J, Fliser, D, and Speer, T. WNT-beta-catenin signalling – a versatile player in kidney injury and repair. Nat Rev Nephrol. (2021) 17:172–84. doi: 10.1038/s41581-020-00343-w
76. Zhang, M, Weng, W, Zhang, Q, Wu, Y, Ni, S, Tan, C, et al. The lncRNA NEAT1 activates Wnt/beta-catenin signaling and promotes colorectal cancer progression via interacting with DDX5. J Hematol Oncol. (2018) 11:113. doi: 10.1186/s13045-018-0656-7
77. Peng, Y, Xu, Y, Zhang, X, Deng, S, Yuan, Y, Luo, X, et al. A novel protein AXIN1-295aa encoded by circAXIN1 activates the Wnt/beta-catenin signaling pathway to promote gastric cancer progression. Mol Cancer. (2021) 20:158. doi: 10.1186/s12943-021-01457-w
78. Sun, G, Wu, L, Sun, G, Shi, X, Cao, H, and Tang, W. WNT5a in colorectal Cancer: research Progress and challenges. Cancer Manag Res. (2021) 13:2483–98. doi: 10.2147/CMAR.S289819
79. Wang, J, Hu, K, Cai, X, Yang, B, He, Q, Wang, J, et al. Targeting PI3K/AKT signaling for treatment of idiopathic pulmonary fibrosis. Acta Pharm Sin B. (2022) 12:18–32. doi: 10.1016/j.apsb.2021.07.023
80. Sun, G, Zhao, S, Fan, Z, Wang, Y, Liu, H, Cao, H, et al. CHSY1 promotes CD8(+) T cell exhaustion through activation of succinate metabolism pathway leading to colorectal cancer liver metastasis based on CRISPR/Cas9 screening. J Exp Clin Cancer Res. (2023) 42:248. doi: 10.1186/s13046-023-02803-0
81. Yu, L, Wei, J, and Liu, P. Attacking the PI3K/Akt/mTOR signaling pathway for targeted therapeutic treatment in human cancer. Semin Cancer Biol. (2022) 85:69–94. doi: 10.1016/j.semcancer.2021.06.019
82. Zhang, Y, Kwok-Shing Ng, P, Kucherlapati, M, Chen, F, Liu, Y, Tsang, YH, et al. A Pan-Cancer Proteogenomic atlas of PI3K/AKT/mTOR pathway alterations. Cancer Cell. (2017) 31:820–832.e3. doi: 10.1016/j.ccell.2017.04.013
83. Hassin, O, and Oren, M. Drugging p53 in cancer: one protein, many targets. Nat Rev Drug Discov. (2023) 22:127–44. doi: 10.1038/s41573-022-00571-8
84. Levine, AJ. p53: 800 million years of evolution and 40 years of discovery. Nat Rev Cancer. (2020) 20:471–80. doi: 10.1038/s41568-020-0262-1
85. Liu, Y, Su, Z, Tavana, O, and Gu, W. Understanding the complexity of p53 in a new era of tumor suppression. Cancer Cell. (2024) 42:946–67. doi: 10.1016/j.ccell.2024.04.009
86. Xie, H, Guo, L, Wang, Z, Peng, S, Ma, Q, Yang, Z, et al. Assessing the potential prognostic and immunological role of TK1 in prostate Cancer. Front Genet. (2022) 13:778850. doi: 10.3389/fgene.2022.778850
87. Yu, W, Hurley, J, Roberts, D, Chakrabortty, SK, Enderle, D, Noerholm, M, et al. Exosome-based liquid biopsies in cancer: opportunities and challenges. Ann Oncol. (2021) 32:466–77. doi: 10.1016/j.annonc.2021.01.074
88. Nikanjam, M, Kato, S, and Kurzrock, R. Liquid biopsy: current technology and clinical applications. J Hematol Oncol. (2022) 15:131. doi: 10.1186/s13045-022-01351-y
89. Martin-Alonso, C, Tabrizi, S, Xiong, K, Blewett, T, Sridhar, S, Crnjac, A, et al. Priming agents transiently reduce the clearance of cell-free DNA to improve liquid biopsies. Science. (2024) 383:eadf2341. doi: 10.1126/science.adf2341
90. Zhang, S, Zheng, Y, Li, X, Zhang, S, Hu, H, and Kuang, W. Cellular senescence-related gene signature as a valuable predictor of prognosis in hepatocellular carcinoma. Aging (Albany NY). (2023) 15:3064–93. doi: 10.18632/aging.204658
91. Shim, SM, Kim, JH, and Jeon, JP. Effective litmus gene test for monitoring the quality of blood samples: application to Alzheimer's disease diagnostics. Sci Rep. (2017) 7:16848. doi: 10.1038/s41598-017-17293-2
92. Du, R, Huang, C, Liu, K, Li, X, and Dong, Z. Targeting AURKA in Cancer: molecular mechanisms and opportunities for Cancer therapy. Mol Cancer. (2021) 20:15. doi: 10.1186/s12943-020-01305-3
93. Luo, P, Yan, H, Du, J, Chen, X, Shao, J, Zhang, Y, et al. PLK1 (polo like kinase 1)-dependent autophagy facilitates gefitinib-induced hepatotoxicity by degrading COX6A1 (cytochrome c oxidase subunit 6A1). Autophagy. (2021) 17:3221–37. doi: 10.1080/15548627.2020.1851492
94. Chen, YJ, You, GR, Lai, MY, Lu, LS, Chen, CY, Ting, LL, et al. A combined systemic strategy for overcoming cisplatin resistance in head and neck Cancer: from target identification to drug discovery. Cancers (Basel). (2020) 12:3482. doi: 10.3390/cancers12113482
95. Wu, T, Luo, Y, Zhang, M, Chen, B, Du, X, Gu, H, et al. Mechanisms of minor pole-mediated spindle bipolarization in human oocytes. Science. (2024) 385:eado1022. doi: 10.1126/science.ado1022
96. Maccioni, RB, and Cambiazo, V. Role of microtubule-associated proteins in the control of microtubule assembly. Physiol Rev. (1995) 75:835–64. doi: 10.1152/physrev.1995.75.4.835
97. Yokoyama, H. Chromatin-binding proteins moonlight as mitotic microtubule regulators. Trends Cell Biol. (2016) 26:161–4. doi: 10.1016/j.tcb.2015.12.005
98. Kara, G, Calin, GA, and Ozpolat, B. RNAi-based therapeutics and tumor targeted delivery in cancer. Adv Drug Deliv Rev. (2022) 182:114113. doi: 10.1016/j.addr.2022.114113
99. Yoon, J, Shin, M, Lee, JY, Lee, SN, Choi, JH, and Choi, JW. RNA interference (RNAi)-based plasmonic nanomaterials for cancer diagnosis and therapy. J Control Release. (2022) 342:228–40. doi: 10.1016/j.jconrel.2022.01.012
100. Sun, G, Liu, H, Zhao, J, Zhang, J, Huang, T, Sun, G, et al. Macrophage GSK3beta-deficiency inhibits the progression of hepatocellular carcinoma and enhances the sensitivity of anti-PD1 immunotherapy. J Immunother Cancer. (2022) 10:e005655. doi: 10.1136/jitc-2022-005655
101. Cai, M, Song, XL, Li, XA, Chen, M, Guo, J, Yang, DH, et al. Current therapy and drug resistance in metastatic castration-resistant prostate cancer. Drug Resist Updat. (2023) 68:100962. doi: 10.1016/j.drup.2023.100962
102. Thapa, KS, Oldani, A, Pagliuca, C, De Wulf, P, and Hazbun, TR. The Mps1 kinase modulates the recruitment and activity of Cnn1 (CENP-T) at Saccharomyces cerevisiae kinetochores. Genetics. (2015) 200:79–90. doi: 10.1534/genetics.115.175786
103. Noordermeer, SM, and van Attikum, H. PARP inhibitor resistance: a tug-of-war in BRCA-mutated cells. Trends Cell Biol. (2019) 29:820–34. doi: 10.1016/j.tcb.2019.07.008
104. Ding, H, Tan, P, Fu, S, Tian, X, Zhang, H, Ma, X, et al. Preparation and application of pH-responsive drug delivery systems. J Control Release. (2022) 348:206–38. doi: 10.1016/j.jconrel.2022.05.056
105. Jiang, S, Li, H, Zhang, L, Mu, W, Zhang, Y, Chen, T, et al. Generic diagramming platform (GDP): a comprehensive database of high-quality biomedical graphics. Nucleic Acids Res. (2025) 53:D1670–6. doi: 10.1093/nar/gkae973
106. Liu, J, Wu, Z, Sun, R, Nie, S, Meng, H, Zhong, Y, et al. Using mRNAsi to identify prognostic-related genes in endometrial carcinoma based on WGCNA. Life Sci. (2020) 258:118231. doi: 10.1016/j.lfs.2020.118231
107. Zhang, B, Zhou, Q, Xie, Q, Lin, X, Miao, W, Wei, Z, et al. SPC25 overexpression promotes tumor proliferation and is prognostic of poor survival in hepatocellular carcinoma. Aging (Albany NY). (2020) 13:2803–21. doi: 10.18632/aging.202329
108. Yang, X, Sun, H, Song, Y, Yang, L, and Liu, H. Diagnostic and prognostic values of upregulated SPC25 in patients with hepatocellular carcinoma. PeerJ. (2020) 8:e9535. doi: 10.7717/peerj.9535
109. Wang, X, Qiao, J, and Wang, R. Exploration and validation of a novel prognostic signature based on comprehensive bioinformatics analysis in hepatocellular carcinoma. Biosci Rep. (2020) 40:BSR20203263. doi: 10.1042/BSR20203263
110. Gao, Y, Liu, J, Zhao, D, and Diao, G. A novel prognostic model for identifying the risk of hepatocellular carcinoma based on angiogenesis factors. Front Genet. (2022) 13:857215. doi: 10.3389/fgene.2022.857215
111. Guo, L, Wang, Z, Du, Y, Mao, J, Zhang, J, Yu, Z, et al. Random-forest algorithm based biomarkers in predicting prognosis in the patients with hepatocellular carcinoma. Cancer Cell Int. (2020) 20:251. doi: 10.1186/s12935-020-01274-z
112. Yin, Q, Chen, W, Zhang, C, and Wei, Z. A convolutional neural network model for survival prediction based on prognosis-related cascaded Wx feature selection. Lab Investig. (2022) 102:1064–74. doi: 10.1038/s41374-022-00801-y
113. Jeong, J, Keum, S, Kim, D, You, E, Ko, P, Lee, J, et al. Spindle pole body component 25 homolog expressed by ECM stiffening is required for lung cancer cell proliferation. Biochem Biophys Res Commun. (2018) 500:937–43. doi: 10.1016/j.bbrc.2018.04.205
114. Cui, F, Tang, H, Tan, J, and Hu, J. Spindle pole body component 25 regulates stemness of prostate cancer cells. Aging (Albany NY). (2018) 10:3273–82. doi: 10.18632/aging.101631
Keywords: SPC25, cancer progression, biomarker, therapeutic target, NDC80 complex
Citation: Jin Y, Chen M, Chen F, Gao Z, Li X, Hu L, Cai D, Zhao S and Song Z (2025) The multifaceted functions of SPC25 in cancer: from molecular pathways to targeted therapy. Front. Med. 12:1550901. doi: 10.3389/fmed.2025.1550901
Edited by:
Tanay Thakar, Broad Institute, United StatesReviewed by:
Neha Varshney, University of California, San Diego, United StatesArchana Krishnamoorthy, Dana-Farber Cancer Institution/Harvard Cancer Center, United States
Bernardo Gouveia, Harvard University, United States
Copyright © 2025 Jin, Chen, Chen, Gao, Li, Hu, Cai, Zhao and Song. This is an open-access article distributed under the terms of the Creative Commons Attribution License (CC BY). The use, distribution or reproduction in other forums is permitted, provided the original author(s) and the copyright owner(s) are credited and that the original publication in this journal is cited, in accordance with accepted academic practice. No use, distribution or reproduction is permitted which does not comply with these terms.
*Correspondence: Dandan Cai, NDA3NDIyNDI5QHFxLmNvbQ==; Siqi Zhao, NTQ2NTE3ODI3QHFxLmNvbQ==; Zhengwei Song, ZG9jdG9yc29uZ3p3QHpqeHUuZWR1LmNu
†These authors share first authorship