- 1Department of Veterinary Clinical Medicine, College of Veterinary Medicine, The University of Illinois at Urbana-Champaign, Urbana-Champaign, IL, United States
- 2Department of Animal Medicine, Internal Medicine, Faculty of Veterinary Medicine, Benha University, Benha, Egypt
Ozone (O3) is an attractive alternative antimicrobial in the poultry processing industry. The optimal operational conditions of O3 for improving food safety concerns are poorly understood. The main objective of this study was therefore to characterize the microbial killing capacity of aqueous O3 and O3–lactic acid blend (O3–LA) at different operational conditions on chicken drumsticks contaminated with high Salmonella load using sequential soaking and spraying approaches. Four hundred forty-eight chicken drumsticks (280–310 g) were soaked into two-strain Salmonella cocktail, and the initial load on the surface of the skin was 6.9-log10 cell forming unit (CFU)/cm2 [95% confidence interval (CI), 6.8–7.0]. The contaminated drumsticks were then sequentially (10×) soaked and sprayed with aqueous O3 (8 ppm) and O3–LA. Following O3 exposure, quantitative bacterial cultures were performed on the post-soaking and post-spraying water, skin surface, and subcutaneous (SC) of each drumstick using 3MTM PetrifilmTM Rapid Aerobic Count Plate (RAC) and plate reader. The average killing capacity of aqueous O3/cycle on the skin surface was 1.6-log10/cm2 (95% CI, 1.5–1.8-log10/cm2) and 1.2-log10/cm2 (95% CI, 1.0–1.4-log10/cm2), and it was 1.1-log10/cm2 (95% CI, 0.9–1.3-log10/cm2) and 0.9-log10/cm2 (95% CI, 0.7–1.1-log10/cm2) in SC for soaking and spraying approaches, respectively. Six sequential soaking and seven sequential spraying cycles with ozonated water of 8 ppm reduced the heavy Salmonella load below the detectable limit on the skin surface and SC of drumsticks, respectively. Addition of LA seems to increase the microbial killing capacity of aqueous O3 with average differences of 0.3-log10/cm2 (P = 0.08) and 0.2-log10/cm2 (P = 0.12) on the skin surface using soaking and spraying approaches, respectively. Aqueous O3 did not cause any significant changes in the drumstick skin color. The Salmonella load of < 4.5-log10/cm2 was a strong predictor for the reduction rate (P < 0.001, R2 = 0.64). These results provide important information that helps the poultry processing facilities for selecting the optimal operational strategy of O3 as an effective antimicrobial.
Introduction
Chicken meat is the second most popular meat in the world, which accounts for ∼30% of meat production preceded by pork with 38% of the total meat production. In the United States, poultry meat is the most popular consumed meat, and the consumption has steadily increased in recent years. The annual poultry meat consumption per capita has increased from 27 lb in 1970 to 60 lb in 2005 (National Chicken Council, 2011). The reasons for the popularity of this kind of meat are the competitive price, absence of cultural and religious obstacles, fast preparation, low fat content, and the high nutritional value (Food and Agriculture Organization of the United Nations [FAO], 2014). The chicken meat market has changed from whole birds to chicken parts over the years. In the last decades, 83% of broilers were marketed as whole birds, whereas 15% were marketed as parts. In 2009, 12% of broilers were consumed as whole birds, and 42% as parts (National Chicken Council, 2011). Chicken meat supports bacterial growth due to high water content, large amounts of variable nutrients, and high pH resulting in short shelf-life of products (Lawrie, 1998). In the last few years, consumers are more concerned about food safety than ever before. The US Department of Agriculture, Food Safety and Inspection Service (USDA-FSIS) recommendations are to control pathogens prevalence in the products that are most often purchased by consumers (United States Department of Agriculture, Food Safety, and Inspection Service, 2015). The new standards include culture for foodborne pathogens at the end of the chilling line and in the cut-up room (United States Department of Agriculture, Food Safety, and Inspection Service, 2015).
In the United States, foodborne diseases cause 8 million cases annually, 128,000 hospitalizations, and 3,000 deaths (Centers for Disease Control and Prevention, 2011). The total annual economic burden of illness calculated based on the enhanced cost-of-illness model is $77.7 billion (Scharff, 2012). Salmonella is the most frequently reported cause of foodborne illness in the United States with roughly 1.2 million cases annually, ∼20,000 hospitalizations, and ∼400 deaths (Scallan et al., 2011). The total annual cost including medical costs and loss of productivity has been estimated to be about 3.3 billion (Hoffmann et al., 2012). Salmonella is the most commonly associated pathogen with poultry meat and poultry products (Hoffmann et al., 2012). Consequently, it is considered a good indicator of the hygienic conditions in the poultry processing facilities (Kottwitz et al., 2010; Silva et al., 2010; Jakobsen et al., 2012). The chicken carcass is considered spoiled or deteriorated when the microbial count reaches 106–109 cell forming unit (CFU)/cm2 (Russell, 2001). Therefore, the safe elimination or, at least, reduction to a safe level is an immediate challenge in the poultry processing facilities. Currently, bacterial contamination is removed or controlled during the carcass processing using a combination of thermal treatment, water, and chemical antimicrobials, such as peracetic acid (PAA) (Northcutt et al., 2005; Dittoe et al., 2019). However, these chemical antimicrobials are faced with challenges including last for several hours, and most of them can be toxic before and after the breakdown (Megahed et al., 2018a,b, 2019). Additionally, these chemical antimicrobials can produce undesired color and texture effects and development of off-flavors (Qiao et al., 2002). Accordingly, the utilization of other antimicrobials with a high killing capacity, short half-life, and decompose to non-toxic molecules is a priority goal for the poultry industry.
Ozone (O3) is known for its strong oxidative power with an oxidative potential of 2.07 V, nearly twice the oxidizing potential of chlorine (1.36) and greater than the oxidizing potential of PAA (1.81) (Russel et al., 1999). Because of its strong oxidation potential, O3 is very toxic to bacteria, even at concentrations as low as 0.01 ppm (Qingshi et al., 1989). Additionally, O3 did not show any negative impact on the final appearance of poultry products (Mancini and Hunt, 2005). In 2002, the USDA approved O3 as a safe and suitable ingredient used in the production of meat and poultry (United States Department of Agriculture, 2002). In 1979, Yang and Chen reported approximately 3-log10 microbial reduction on chicken necks immersed in ozonated water of 2.48 ppm for 9 min exposure time (Yang and Chen, 1979). In another study, ozonated water did not show significant microbial reduction rate (1-log10) on chicken carcasses chilled with water containing 3.0–4.5 ppm of O3 for 45 min exposure time (Sheldon and Brown, 1986). This indicated that the microbial killing capacity of aqueous O3 on Salmonella-contaminated chicken carcasses is still an unresolved issue. Among the potential decontamination protocol is the blend of organic acid solutions and oxidizing agents that have been evaluated on the poultry products and showed variable results. A combination of lactic acid (LA) and PAA reduced Escherichia coli O157 and organic materials to a safe level in the fresh-cut leafy vegetables (Haute et al., 2015). To the best of our knowledge, no study evaluated the combination of O3–LA for reducing the pathogens prevalence and levels in the chicken cuts. Accordingly, the primary objective of the present study was to characterize the microbial killing capacity of aqueous O3 and O3–LA blend at different operational conditions on chicken drumsticks contaminated with high Salmonella load using sequential soaking and spraying approaches. We also hypothesized that the bacterial load has a significant impact on the microbial killing capacity of aqueous O3 (Megahed et al., 2018a,b). The secondary objective was therefore to use the segmented linear regression to determine the effect of skin surface microbial load on the microbial killing capacity of aqueous O3.
Materials and Methods
Preparation of Inoculated Samples
Four hundred forty-eight chicken drumsticks with an average weight ranging from 280 to 310 g obtained from local grocery stores were used in this study. Sample size was determined from the effect size and variation observed in a preliminary, unreported trial. Sampling at each intervention was based on a statistical power of 80%, an expected standard deviation of 0.8-log10 CFU/ml, and a desired reduction rate of 0.7-log10 CFU/ml. All drumsticks were kept in a freezer (−20°C) from the time of reception until used. Before the inoculation process, the enclosed drumsticks bags were thawed in a refrigerator at 4°C for 12 h and then held at room temperature for 1 h.
To confirm no Salmonella background on the drumsticks before inoculation, each drumstick sample was first rinsed with buffered peptone water (BPW; Difco, BD, Sparks, MD, United States) in a labeled sterile Nasco WHIRL-PAK bag by manually shaking the bag for 1 min. An area of approximately 6 cm2 of the skin surface and subcutaneous (SC) was also swabbed with sterile cotton swabs (Pur-Wraps®; Puritan Medical Products, Guilford, ME, United States). One ml of the rinsate solution and swabs were added to 9 ml of BPW. One ml of the mixture was streaked on Tryptic Soy Agar plates (TSA W/5% sheep blood agar; Remel, Lenexa, KS, United States) for bacterial identification using matrix-assisted laser desorption ionization-time of flight (MALDI-TOF) mass spectrometry (Bruker Daltonik, Bremen, Germany) at the Veterinary Diagnostic Laboratory of the University of Illinois Urbana-Champaign. Detailed bacterial identification using MALDI-TOF mass spectrometry has been previously mentioned in our previous work (Megahed et al., 2018a,b). Drumsticks with background bacteria were thoroughly washed with aqueous O3 (8 ppm) in a labeled sterile Nasco WHIRL-PAK bag until we get bacteria-free drumsticks. The clean drumsticks were then held in a laminar airflow hood (Baker, Sanford, ME, United States) for 30 min to ensure O3 destruction (Hirahara et al., 2019).
Avirulent live Salmonella typhimurium and Salmonella choleraesuis (aSTC) vaccine (Enterisol® Salmonella T/C vaccine; Boehringer Ingelheim Vetmedica, St. Joseph, MO, United States) was used as a source of two Salmonella strains. The two Salmonella strains were revitalized and activated to be used as a model to characterize the Salmonella killing capacity of aqueous O3 (Wigley et al., 2005; Lottenbach et al., 2013). Salmonella was recovered using BPW in 50 ml sterile conical polypropylene tubes equipped with a lid (Thermo Fisher Scientific, Waltham, MA, United States) at 1:9 (vaccine/BPW) ratio. The mixture was incubated at 37°C for 48 h to ensure that all injured cells were recovered. A serial 10-fold dilution was used in order to determine the level of inoculum by spreading 1 ml from each dilution on 3MTM PetrifilmTM Rapid Aerobic Count Plate (RAC; 3MTM Microbiology, St. Paul, MN, United States) using 3MTM PetrifilmTM spreader (3MTM Microbiology, St. Paul, MN, United States). 3MTM PetrifilmTM RAC was used for enumeration of aSTC as it is accurate, easy to use, more efficient, commercially available, enumeration medium has a quick turn-around time, and reading results are automated (Nelson et al., 2013; Kumar et al., 2020). One ml of the mixture was also spread on TSA for bacterial identification to confirm the presence of only aSTC using MALDI-TOF mass spectrometry. A Salmonella cocktail mixture of an inoculum level of 107 CFU/ml was used for contamination of drumsticks.
Clean drumsticks were soaked in aSTC cocktail with an inoculum level of 07 CFU/ml for 5 min, the worst-case scenario, at a room temperature of 20°C and relative humidity of 55–60%. The soaked samples were aerated in a clean laminar airflow hood (Baker, Sanford, ME, United States) for 30 min to evaporate the peptone water and allow for bacterial attachment. After drying, aseptic techniques were used to place each drumstick into a labeled sterile Nasco WHIRL-PAK bag. The initial inoculum on the surface of the skin was then detected by swabbing the skin surface with sterile cotton swabs. The culture protocol and cell count quantification were similar as that described above.
O3 Generation
The aqueous O3 with a concentration of 8 ppm was obtained using an OOG1 × 0 O3 generator manufactured by Origin, Inc. (Princeton, NJ, United States) as previously described in our work (Megahed et al., 2018a,b, 2019).
Sequential Soaking Approach
This experiment was designed to characterize the microbial killing capacity of aqueous O3 and O3–LA on aSTC heavily contaminated drumsticks using multi-sequential soaking approach. Fifty inoculated drumsticks were randomly assigned into four groups, aqueous O3 treatment (n = 15), O3–LA treatment (n = 15), positive control (n = 15), and negative control (n = 5). The negative control drumsticks were used to detect the presence of background Salmonella. The aqueous O3-treated drumsticks were sequentially soaked (10-serial washes) with 500 ml of water containing 8 ppm of O3 for 4 min exposure each (Megahed et al., 2018a,b, 2019). A waiting time of 30 min between cycles was performed in order to ensure the destruction of O3 (Hirahara et al., 2019). The O3–LA-treated drumsticks were sequentially soaked (10-serial washes) with 500 ml of ozonated water containing 0.3% L-lactic acid (Sigma Aldrich, St. Louis, MO, United States) for 4 min exposure. The concentration of LA was selected to achieve a pH range from 2 to 3 to provide an optimum condition to maximize the oxidative power of O3 (Van Netten et al., 1994). A volume of 500 ml was used as the optimal volume enough to completely cover the drumstick in the WHIRL-PAK bag. The temperature of the ozonated water was 10–12°C. The bags were gently shaken for the exposure period. The positive control drumsticks were sequentially soaked (10-serial washes) with 500 ml autoclave sterilized distilled water (DW) for the same exposure time.
Two milliliters of soaking water was aspirated each soaking cycle and used for culture. One milliliter was spread directly on RAC PetrifilmTM plate, and 1 ml was then serially diluted (fivefold dilutions) in 9 ml of BPW. An area of approximately 6 cm2 of the skin surface and SC was also swabbed with sterile cotton swabs at different points each soaking cycle. Each swab was washed in 10 ml of BPW, and 1 ml was then serially diluted (threefold dilutions) in 9 ml of BPW. One milliliter from each dilution was spread on RAC PetrifilmTM plate. All RAC PetrifilmTM plates were incubated at 37°C for 24 h. One milliliter from each dilution was also grown on TSA for bacterial identification using MALDI-TOF mass spectrometry for confirming only Salmonella isolates. The colony forming units were counted using an automated counter (3M Petrifilm Plate Reader; 3MTM Microbiology, St. Paul, MN, United States). The plates were incubated for further 2–3 days in order to remove the effect of recovery of injured cells. To accommodate the effective reading range of the plate reader (maximum reading < 999/plate), only plates with 30–300 colonies were used for calculating the bacterial reduction factor (RF).
Skin Color
The color of the skin surface of aqueous O3 and O3–LA-treated drumsticks was measured quantitatively by determining the RGB measurement using the ImageJ software program (version 1.50i; National Institutes of Health, Bethesda, MD, United States). Digital images were taken for the drumsticks each washing cycle using the Canon PowerShot SX420 IS 20.0 MP camera (Canon USA Inc., Lake Success, NY, United States). The drumsticks were hanged vertically in front of a white background and exposed to diffuse light to ensure the same amount of light distributed over the drumstick. A region of interest (ROI) of 20 × 20 mm was defined.
Determination of Ozonated Water Volume for Spraying Approach
This experiment was designed to determine the reliable ozonated water volume used for spraying approach to decontaminate the aSTC-contaminated drumsticks. The inoculated-drumsticks were sprayed with 100 (n = 6), 200 (n = 6), 300 (n = 6), and 500 ml (n = 6) of water containing 8 ppm of O3 using a veterinary spray nozzle (Sunny Farms, San Francisco, CA, United States) attached to 500-cc syringe. The spray protocol was done by one operator (AM) in order to eliminate the potential for subjective operator-to-operator differences. The drumsticks were hanged in labeled sterile Nasco WHIRL-PAK bags and sprayed for 5 s. The spray solution was equally distributed over the surface of drumsticks by locating the syringe tips 5 cm above the drumsticks. The control drumsticks were sprayed with 100 (n = 6), 200 (n = 6), 300 (n = 6), and 500 ml (n = 6) DW. The culture protocol from spraying water, skin, and SC swabbing and quantification of cell count were similar as that described above in the first experiment.
Sequential Spraying Approach
This experiment was designed to characterize the microbial killing capacity of aqueous O3 and O3–LA blend on the aSTC-contaminated drumsticks using multi-sequential spraying approach. The control and treated drumsticks were sequentially sprayed (10-serial sprays) with 100 ml. The spraying protocol was similar as that described above. The culture and cell count quantification were similar as that described above.
Effect of Bacterial Load
This experiment was designed to characterize the impact of bacterial load on the microbial killing capacity of aqueous O3 using soaking and spraying approaches. Three hundred drumsticks were used for both washing (150) and spraying (150) approaches. The skin surfaces of drumsticks were contaminated with five different aSTC loads (3.0, 4.0, 5.0, 6.0, and 7.0-log10/ml). The soaking, spraying, culture, and cell count quantification protocol were similar as that described above.
Data and Statistical Analyses
The log10 aSTC density for each drumstick was calculated using the formula presented in the ASTM method E2871-12 (ASTM E2871–12, 2012), as follows:
We added 1 in such way log10(x + 1) in order to overcome the problem of log-transformation of 0 CFUs. RF was also calculated for the first washing and/or spraying cycle by using an equation presented in the ASTM method E2871-12 (ASTM E2871–12, 2012), as follows:
For subsequent soaking and spraying cycles, RF was calculated by subtraction of the aSTC count of the current cycle from the aSTC count of the previous cycle.
Statistical analyses were performed using SAS 9.4 software (SAS Inc., Cary NC, United States), RStudio (version 1.1.383; R Studio, Inc., Boston, MA, United States), and Excel spreadsheet (Microsoft Corporation, Redmond, WA, United States). Data were expressed as median and range or as mean ± 95% confidence interval (CI) based on testing for normality by calculating the Shapiro–Wilk Statistic or based on testing equality of variances using Levene’s test. P < 0.05 was considered significant. For comparison between groups, Kruskal–Wallis One Way Analysis of Variance on Ranks was used for non-normal distribution or unequal variances data. For post hoc comparisons, P-values were adjusted for multiple comparisons according to Tukey. Mixed models analysis (PROC MIXED) of variance was used to detect differences in log10 RF between treatments (three levels; O3, O3–LA, and DW), sequential cycles, and the interaction between treatment and cycles. Cycles were included in the model as a repeated variable. Drumstick nested within treatment was included in the model as a random effect whenever the between subjects variation was determined to be significant. Whenever the F-test was significant, Tukey-adjusted P-values were used to assess differences between different treatments at a specific soaking or spraying cycle and between sequential cycles.
Univariate regression was used to characterize the association between RF and ozonated water volume for spraying approach. Mixed model segmented linear regression (PROC NLMIXED, SAS 9.4) was used to characterize the relationship between the bacterial reduction rate and the aSTC load (Gonçalves et al., 2016; Trefz et al., 2018). A mixed model approach using the adaptive Gauss–Hermitage quadrature approximation method for the marginal likelihood function was applied using quasi-Newton optimization, with μ0 representing the random background coefficient assuming the distribution of random effects to be normal with mean (μ0) = 0 and variance = s2e. The model equations assumed a constant value for RF when aSTC load < the cut point identified by segmented regression (Xc) and a negative linear relationship between the bacterial reduction rate and the aSTC load when aSTC load < Xc, such that: RF = (b0 + μ0) when aSTC load ≥ Xc and [b0 + μ0 − b1 × (aSTC load-Xc)] when aSTC load ≤ Xc. The segmented linear regression approach permitted the objective identification of increasing aSTC reduction when aSTC load started to decrease.
Results
The background bacteria identified by direct MALDI-TOF mass spectrometry were Bacillus thermosphacta, Enterobacteriaceae, and E. coli. The results of direct MALDI-TOF mass spectrometry confirmed the presence of only aSTC during the whole experiment. The average of the initial bacterial load after contamination with aSTC was 6.94-log10 (95% CI, 6.87–7.0).
Salmonella Reduction by Sequential Soaking Approach
On the skin surface, the first soaking cycle using ozonated water of 8 ppm reduced the aSTC load from 6.9 to 5.7-log10/cm2 with an average RF of 1.2-log10/cm2 (mean, 95% CI, 0.9–1.5). The average RF was then gradually increased in the subsequent soaking cycles until it reached a peak of 2.5-log10/cm2 (95% CI, 2.1–2.8) at the fourth soaking cycle, where the aSTC load reduced from 2.8 to 0.4-log10/cm2. Five sequential soaking cycles were sufficient to reduce the heavy bioload of aSTC (6.9-log10/ml) below the detectable limit on the skin surface (Supplementary Table S1 and Figure 1A). However, the O3–LA blend reduced the aSTC load from 6.9-log10 to 5.4-log10/cm2 in the first soaking cycle with an average RF of 1.5-log10/cm2 (95% CI, 1.1–1.9), reaching a peak of 1.9-log10 (95% CI, 1.5–2.4) at the fourth soaking cycle, where the aSTC load decreased from 1.9-log10/cm2 to the level below the detectable limit. O3–LA blend showed higher aSTC killing capacity than aqueous O3 with a total average difference of 0.3-log10/cm2 (P = 0.08), where four sequential soaking cycles were sufficient to reduce the heavy bioload of aSTC below the detectable limit (Supplementary Table S1 and Figure 1A).
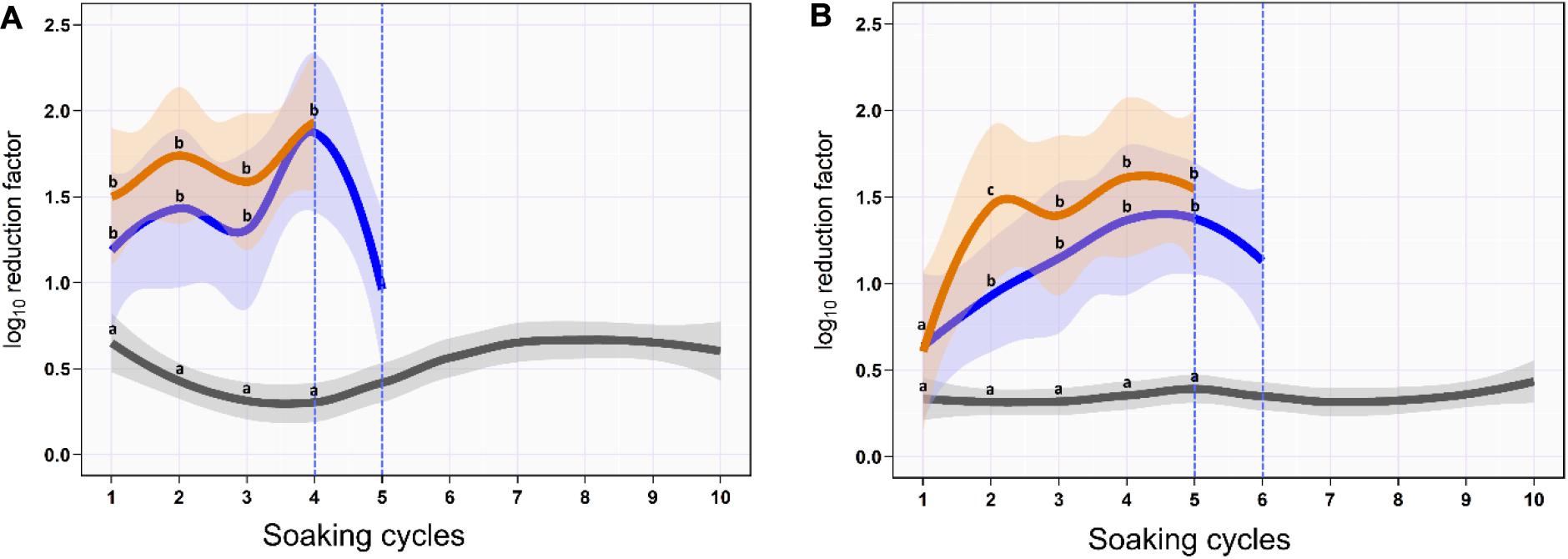
Figure 1. Mean and 95% CI of the log10 reduction in Salmonella typhimurium–choleraesuis (aSTC) cell count on the skin surface (A) and subcutaneous (B) of drumstick soaked with distilled water (gray), ozonated water of 8 ppm (blue), and ozone–lactic acid blend (orange) using multi-sequential soaking approach. The vertical dashed blue line indicates that no aSTC was detected.
In SC, ozonated water of 8 ppm reduced the aSTC load 0.6-log10 (95% CI, 0.3–1.0) in the first soaking cycle, where the aSTC load decreased from 6.8 to 6.2-log10. The average RF was then gradually increased in the subsequent soaking cycles until it reached a peak of 1.4-log10 (95% CI, 0.8–2.0) at the fifth soaking cycle, where the aSTC load was decreased from 3.9-log10 to 2.5-log10. Six sequential soaking cycles were sufficient to reduce the heavy bioload of aSTC (6.8-log10) below the detectable limit (Supplementary Table S1 and Figure 1B). However, O3–LA blend reduced the aSTC load from 6.8 to 6.2-log10 in the first soaking cycle with an average RF of 0.6-log10 (95% CI, 0.2–1.0), reaching a peak of 1.6-log10 (95% CI, 1.0–2.2) at the fifth soaking cycle, where the aSTC load was decreased from 2.5-log10 to 0.9-log10. Addition of LA with low concentration to aqueous O3 seems to intensify the O3 microbial killing capacity, where five sequential soaking cycles were sufficient to reduce the heavy bioload of aSTC below the detectable limit (Supplementary Table S1 and Figure 1B).
Surface Damage
The results of the RGB measurements showed no difference between the drumsticks treated with ozonated water of 8 ppm and DW (Figure 2).
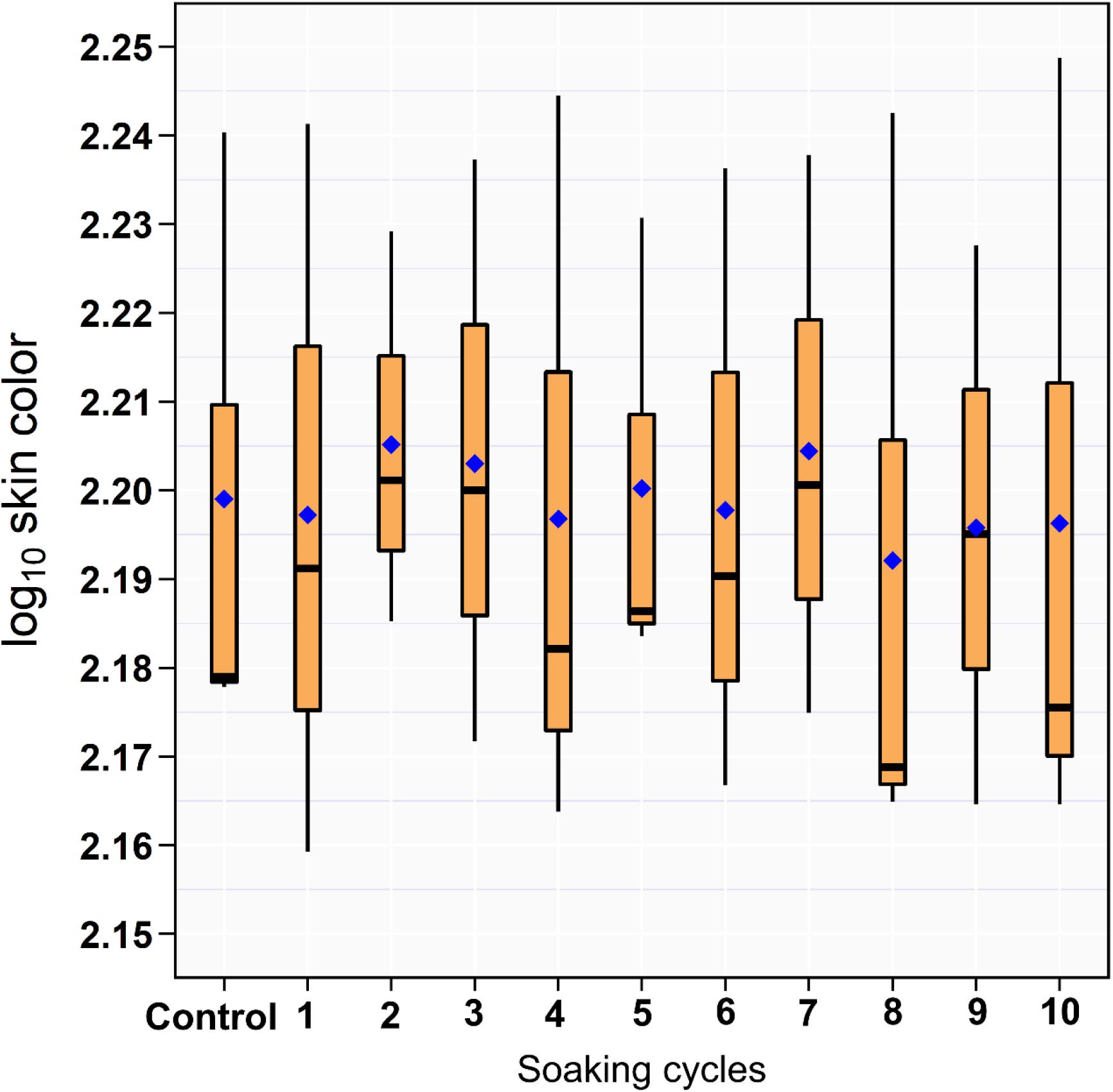
Figure 2. Box and whiskers plot of log10 RGB of the drumstick skin color across the 10 sequential soaking cycles.
Effect of Aqueous O3 Volume on Salmonella Reduction Using Sequential Spraying Approach
Univariate analysis indicated that the reduction in aSTC cell count when exposed to ozonated water of 8 ppm using spraying approach was not dependent on the volume of ozonated water (P = 0.16, R2 = 0.01; Table 1 and Figure 3). Distinct differences in the aSTC reduction were reported between different sampling locations (spraying water, skin, and SC; P ≤ 0.001, R2 = 0.38; Supplementary Table S2 and Figure 3).
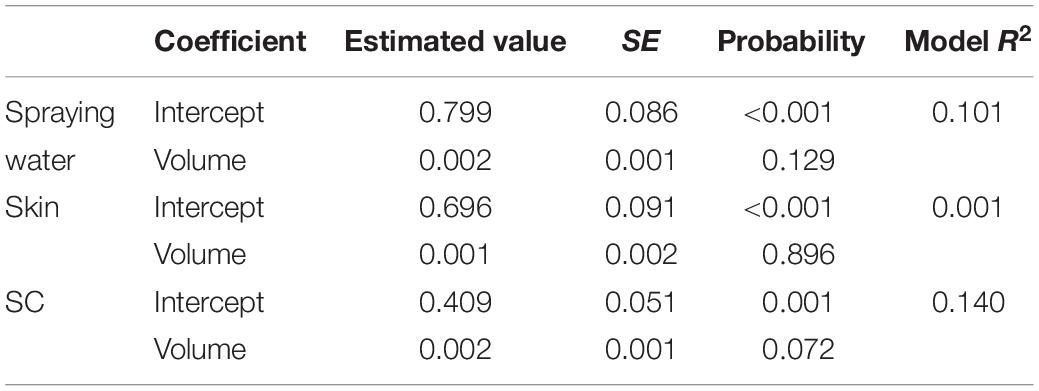
Table 1. Univariate linear regression model for predicting Salmonella typhimurium–choleraesuis reduction in spraying water, skin surface, and subcutaneous of drumsticks exposed to 100, 200, 300, and 500 ml ozonated water of 8 ppm.
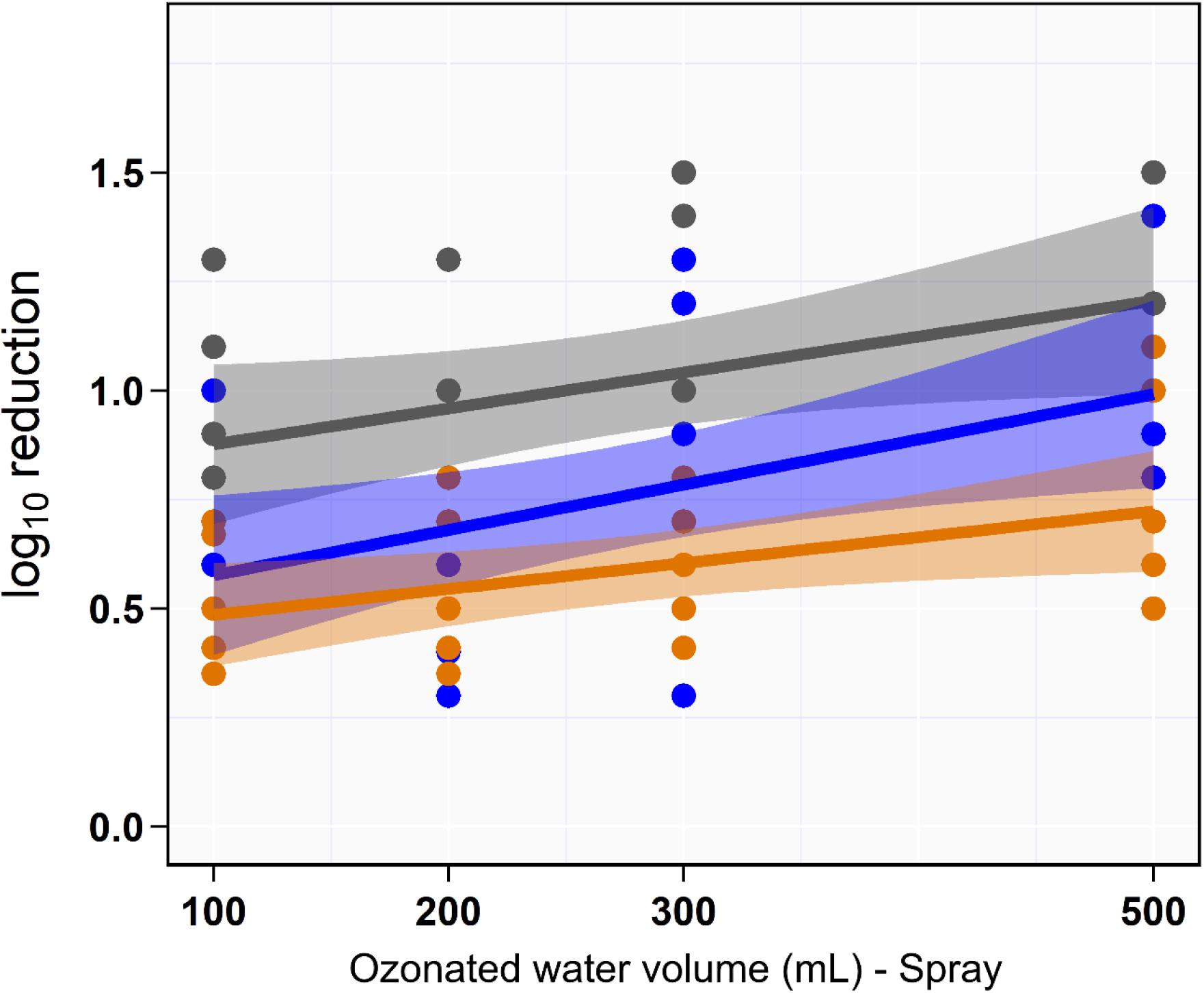
Figure 3. Scatterplot of the linear relationship between volume of aqueous ozone and rate of Salmonella typhimurium–choleraesuis reduction in spraying water (gray), skin surface (blue), and subcutaneous (orange).
Salmonella Reduction Using Sequential Spraying Approach
On the skin surface, the first spraying cycle using ozonated water of 8 ppm reduced the aSTC load from 6.9 to 6.3-log10/cm2 with an average RF of 0.6-log10/cm2 (95% CI, 0.4–0.8). The average RF was then gradually increased in the subsequent spraying cycles until it reached a peak of 1.7-log10/cm2 (95% CI, 0.9–2.5) at the fourth spraying cycle, where the aSTC load reduced from 3.4 to 1.7-log10/cm2. Six sequential spraying cycles were sufficient to reduce the heavy bioload of aSTC (6.9-log10/ml) below the detectable limit (Supplementary Table S3 and Figure 4A). However, O3–LA blend reduced the aSTC load from 6.9 to 6.0-log10/cm2 in the first washing cycle with an average RF of 0.9-log10/cm2 (95% CI, 0.6–1.3), reaching a peak of 2.2-log10/cm2 (95% CI, 1.5–2.7) at the fourth spraying cycle, where the aSTC load decreased from 2.9 to 0.7-log10/cm2. O3–LA blend showed higher reduction in the aSTC load than aqueous O3 with a total average difference of 0.2-log10/cm2 (P = 0.12), where five sequential spraying cycles were sufficient to reduce the heavy bioload of aSTC below the detectable limit (Supplementary Table S3 and Figure 4A).
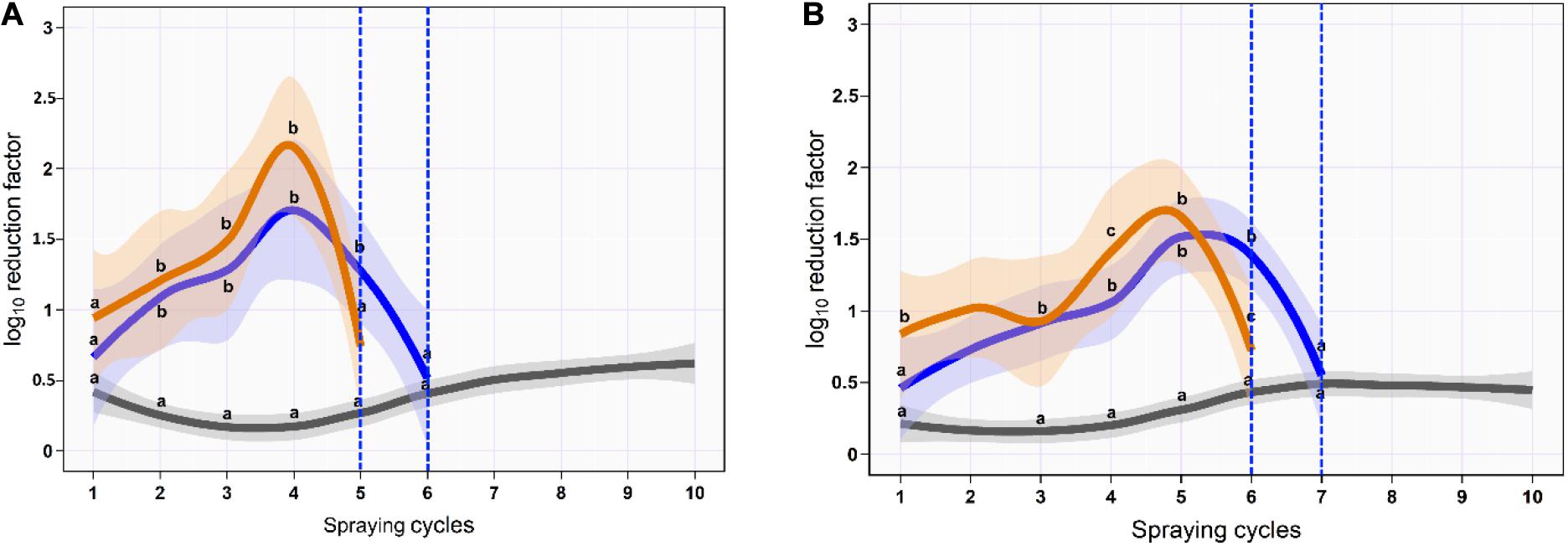
Figure 4. Mean and 95% CI of the log10 reduction in Salmonella typhimurium–choleraesuis (aSTC) cell count on skin surface (A) and subcutaneous (B) of drumstick sprayed with distilled water (gray), ozone of 8 ppm (blue), and ozone–lactic acid blend (orange) using multi-sequential spraying approach. The vertical dashed blue line indicates that no aSTC was detected.
In SC, ozonated water of 8 ppm reduced the aSTC load to 0.4-log10 (95% CI, 0.1–0.7) in the first spraying cycle, where aSTC load decreased from 6.8 to 6.4-log10. The average RF was then gradually increased in the subsequent spraying cycles until it reached a peak of 1.5-log10 (95% CI, 1.0–1.9) at the fifth spraying cycle, where the aSTC load was decreased from 3.7-log10 to 2.2-log10. Seven sequential washing cycles were sufficient to reduce the heavy bioload of aSTC (6.8-log10/ml) below the detectable limit (Supplementary Table S1 and Figure 1B). However, O3–LA blend reduced the aSTC load from 6.8 to 5.9-log10 with an average RF of 0.8 (95% CI, 0.4–1.1) in the first spraying cycle, reaching a peak of 1.9-log10 (95% CI, 1.4–2.4) at the fifth spraying cycle, where the aSTC load decreased from 2.4-log10 to 0.7-log10. O3–LA blend showed higher reduction in the aSTC load than aqueous O3 where sex sequential spraying cycles were sufficient to reduce the heavy bioload of aSTC below the detectable limit in SC (Supplementary Table S3 and Figure 4B).
Effect of Salmonella Load on the Reduction Rate
For soaking approach, segmented mixed models regression fitted two lines to the aSTC load–reduction rate relationship for 150 samples (skin surface) and identified a break point at aSTC load = 4.5-log10/cm2 (95% CI, 1.7–7.3). This break point represented the value for aSTC load where the reduction rate of aSTC increased markedly. The model equations were the reduction rate = 0.96 + 0.04 × aSTC load when aSTC load ≥ 4.5-log10/cm2 and the reduction rate increased in a linear manner after this cut point, such that: the reduction rate = 0.94 − 0.71 aSTC load. The 95% CI for the slope coefficient (b1) was 22–39 and < 4.5-log10/cm2. The 95% CI for the second slope was −0.96 to −0.57 (Figure 5A).
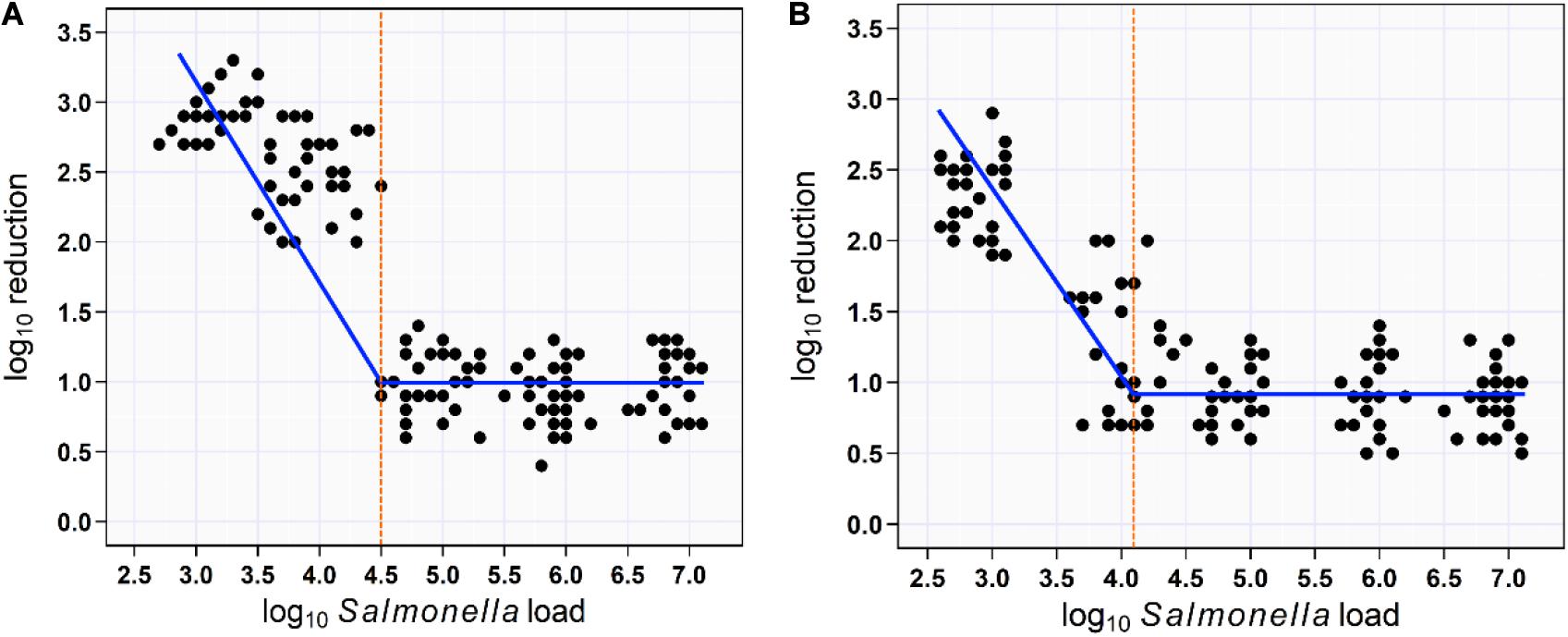
Figure 5. Scatterplot of the relationship between aSTC load and reduction rate. Segmented mixed models regression fitted two lines to the aSTC load–reduction rate relationship and identified a break point (aSTC load = 4.5-log10) where the reduction rate increased markedly using multi-sequential soaking approach (A). Segmented mixed models regression fitted two lines to the aSTC load–reduction rate relationship and identified a break point (aSTC load = 4.2-log10) where the reduction rate increased markedly using multi-sequential spraying approach (B).
For spraying approach, segmented mixed models regression fitted two lines to the aSTC load–reduction rate relationship for 150 samples (skin surface) and identified a break point at aSTC load = 4.2-log10 (95% CI, −1.5 to 9.9). This break point represented the value for aSTC load where the reduction rate of aSTC increased markedly. The reduction rate remained constant at 0.8-log10 (95% CI, 0.1–2.1) until 4.2-log10. The reduction rate increased in a linear manner after this cut point, such that: the reduction rate = 0.8 − 0.9 × aSTC load. The 95% CI for the second slope (−1.1 to −0.7) included 1, indicating that all of the increase in the reduction rate was due to aSTC load (Figure 5B).
Discussion
In the last few years, a great attention has been provided for the utilization of O3 in the poultry processing industry to reduce the contamination with foodborne pathogens, such as Salmonella and Campylobacter in the final product. The main goal of this study was to evaluate the microbial killing capacity of aqueous O3 and O3–LA blend on the drumsticks contaminated with heavy bioload of Salmonella using multi-sequential soaking or spraying approaches. Additionally, this study aimed to utilize the segmented linear regression to evaluate the effect of microbial load on the microbial killing capacity of O3 using soaking or spraying approaches. The first major finding of the study reported here was that the six sequential washing and seven sequential spraying cycles with 8 ppm ozonated water provide an efficient method for reducing 7.0-log10 aSTC load-contaminated drumsticks (the worst-case scenario) below the detectable limit. The second major finding was that the addition of 0.3% L-lactate seems to increase the decontamination power of aqueous O3 on aSTC-contaminated drumsticks. The third major finding was that the bacterial load on chicken carcass has a significant impact on the microbial killing capacity of aqueous O3.
It is important to identify the potential intervention of aqueous O3 to meet the bacterial load reduction requirements in the poultry processing industry. Multi-sequential application of antimicrobials is a common approach used in the poultry processing industry to overcome the physical properties of the skin surface that provides a complex surface niche for bacterial colonization, in addition to the opening, exposing channels, and crevices in the chicken skin that protect bacteria from the effects of antimicrobial interventions (Thomas and McMeekin, 1980; Veerkamp, 1990; Franchin et al., 2010; Benli et al., 2011; Tan et al., 2014). To the best of our knowledge, this is the first study that provides information about the microbial killing capacity of ozonated water on the heavily Salmonella-contaminated cut up parts using multi-sequential soaking and spraying approaches. The results of this study showed a higher aSTC reduction on the skin surface than SC during the first washing cycle that is considered a sensible result because of the high number of bacteria vulnerable to O3 molecules and the physical removal from washing (Franchin et al., 2010; Benli et al., 2011). In the subsequent soaking cycles, the reduction rate was fluctuated. In addition to the physical properties of the skin surface, this is also might be because the organic compounds found on the drumsticks surface prevent the penetration of O3 molecules to deeper layers (Restaino et al., 1995), and the high bacterial load makes bacteria accumulate over each other, creating hard penetrating masses (Megahed et al., 2018a,b). The continuous soaking results in decreasing the bacterial load and helping in destroying the complex colloid of bacteria and organic matter and exposing the underneath hidden organisms to O3 molecules (Li and Ji, 2017). Six washing cycles of 500 ml of ozonated water of 8 ppm are therefore recommended to decontaminate the drumsticks from high bioload of Salmonella. This scenario has been supported by the results of segmented linear regression that showed no association between aSTC load of ≥ 4.5-log10 and the reduction rate; however, aSTC load of < 4.5-log10 showed significant negative association with the aSTC reduction rate.
Addition of LA seems to increase the microbial killing capacity of aqueous O3. To the best of our knowledge, this is the first study to evaluate the decontamination power of low pH ozonated water by adding a low dose of LA on the heavily Salmonella-contaminated drumsticks. The antimicrobial power of O3 is affected by several factors including O3 dose, contact time, temperature, pH, and the presence of organic and inorganic matter (Castillo et al., 2003). Organic acids, such as acetic, lactic, and citric acids, have received the most attention among the sanitizers for their marked bactericidal effects (Dickson and Anderson, 1992). LA seems promising for implementation in the poultry processing facilities because of its availability, cost-effectiveness, ease of use, decontamination potential, and generally recognized as a safe antimicrobial (Ramirez-Hernandez et al., 2018). A decrease in pH of ozonated water by adding LA should intensify the microbial killing capacity of O3 through two mechanisms; first, low pH decreases the rate of O3 degradation (Gardoni et al., 2012); second, soaking/spraying with low pH ozonated water causes cytoplasmic acidification resulting in malfunction of the energy and regulatory parameters and accumulation of free acid anions that kill or retard the microbial growth and survival (Alonso-Calleja et al., 2004; Cardenas et al., 2011; Sohaib et al., 2016). The average reduction in this study from using O3–LA blend is consistent with previous studies that reported an average reduction of 0.8-log10/cycle produced by 100 ml ozonated water (8 ppm) in combination with 0.3% LA (pH 2–3) for 15 s exposure time (Dickson and Anderson, 1992; Gardoni et al., 2012; Ramirez-Hernandez et al., 2018). This reduction is considered an efficient reduction level for post-chilling spraying step under commercial condition (Dickson and Anderson, 1992).
The average reductions of 1.5-log10/ml on the skin surface and 1.0-log10/ml in SC that have been reported in the soaking approach are inconsistent with an earlier study by Sheldon and Brown in 1986 that reported 0.6-log10 reduction in Salmonella after exposing to ozonated water with a concentration ranging from 3.0 to 4.5 ppm for 25 min exposure (Yang and Chen, 1979). In 2012, Trindade and colleagues reported 2.0–3.0-log10 reduction in the Salmonella-contaminated chicken carcass after 30 min exposure to 6.0 ppm ozonated water (Trindade et al., 2012). In 1979, Yang and Chen reported a reduction rate of 7.08-log10 in Salmonella load for ozonated water of 19.0 ppm with a maximum reduction occurring between 2 and 3 min of exposure (United States Department of Agriculture, 2002). It seems that the concentration of O3 is a key factor affecting its microbial killing capacity (Megahed et al., 2018a). O3 might interact with the organic chemical compounds, such as albumin, that may be present on the chicken carcass surface resulting in consuming the O3 molecules and prevent the antimicrobial efficacy from being applied to pathogens. Therefore, decontamination of meat surface requires an additional O3 dose rate to have the desired biocide efficacy (Franchin et al., 2010).
The color of chicken carcass is an important quality factor that potentially affects the consumer-purchasing decisions (Sanchez-Escalante et al., 2001). The utilization of O3 in the meat industry is challenging because its strong oxidative power can cause damage to meat through the destruction of fatty acids and cellular proteins (Haute et al., 2015). The mechanism of color changes is related to the oxidation of myoglobin with O3 molecules producing metmyoglobin that causes discoloration by reducing the redness of meat (Mancini and Hunt, 2005; Bekhit et al., 2013). O3 exposure produced changes in the color of beef meat (Stivarius et al., 2002; Cardenas et al., 2011). However, the data of this study did not show any effects of O3 on the RGB measurements of drumsticks similar to that observed in several earlier studies (Stivarius et al., 2002; Chen et al., 2014). This might be because the chicken meat is categorized as a white meat due to the low content of myoglobin (Guastalli et al., 2016); therefore, O3 has a minimal effect on the skin color appearance of chicken carcass.
The decontamination of chicken carcass in the poultry processing plants mostly occurs at three levels: (1) carcass rinse in the pre-chilling tanks, (2) water chilling step, and (3) spray or drench post-chilling process (Chen et al., 2014). The average exposure to antimicrobials in the poultry processing facilities is approximately 30 min (Guastalli et al., 2016). The average spraying volume of the combination of water and antimicrobials that is mostly used to decontaminate the chicken parts at the post-chilling process is approximately 100–200 ml for 15 s exposure (Ramirez-Hernandez et al., 2018). Interestingly, the data of the spraying protocol reported in this study did not show a significant impact of the ozonated water volume on the aSTC reduction rate. One important concern of food processing industries is water conservation goals (BR50). Therefore, the results of this study suggest reducing the volume of ozonated water in post-chilling spray of drumsticks to meet the USDA requirements for water conservation goals (BR50). The frozen chicken parts showed growth of aerobic bacteria from 2.9 to 3.5-log10 CFU/cm2 (Yang and Chen, 1979; Jindal et al., 1995). The reduction rate in both studies (Yang and Chen, 1979; Jindal et al., 1995) ranged from 1.0 to 1.2-log10 that is consistent with the reduction rate reported in this study. However, both studies used different O3 doses, 3.88 ppm of aqueous O3 for 20 min (Yang and Chen, 1979) and 0.44–0.54 ppm of aqueous O3 for 45 min (Jindal et al., 1995).
In our previous studies, we reported a significant effect of the bacterial load on the microbial killing capacity of ozonated water where we found that there is a direct relationship between the bacterial load and the reduction rate (Megahed et al., 2018a,b). This is a physically sensible relationship as the more bacteria, the more complex bacterial mass formed, the more O3 consumed (Megahed et al., 2018a,b). To the best of our knowledge, this is the first study that used the segmented linear regression to determine the level of bacterial load at which the O3 molecule has a direct effect on bacteria. This information can serve as a guide to identify the optimal operational conditions of O3 that should be used to achieve a safe level of Salmonella reduction in the poultry processing facilities. The limitation of this study is that this study is conducted in a laboratory area that is considered a controlled environment. Additionally, this study artificially contaminated the drumsticks end-product with no assessment of the aqueous O3 on the naturally Salmonella-contaminated chicken, that is considered a different story because of the attachment of Salmonella to the skin surface and inside the feather follicles. Therefore, additional studies are indicated to determine the external validity of the results.
Conclusion
Six sequential soaking and seven sequential spraying cycles with ozonated water of 8 ppm provide an efficient safe protocol to reduce the high Salmonella load that contaminated the skin surface and SC of chicken parts below the detectable limits. Addition of LA to ozonated water seems to enhance the decontamination power of O3. Bacterial load is an important factor controlling the microbial killing capacity of O3 on the surface of chicken carcasses.
Data Availability Statement
The original contributions presented in the study are included in the article/Supplementary Material, further inquiries can be directed to the corresponding author/s.
Author Contributions
AM did conceptualization, data curation, formal analysis, investigation, methodology, software, validation, visualization, and writing original draft. BA did supervision, methodology, visualization, and review & editing. JL did conceptualization, data curation, formal analysis, investigation, methodology, project administration, resources, software, supervision, validation, visualization, and writing original draft. All authors contributed to the article and approved the submitted version.
Funding
The authors declare that this study received funding from Origin, Inc. Princeton, NJ, United States—https://www.originww.com/. The funder was not involved in the study design, collection, analysis, interpretation of data, the writing of this article or the decision to submit it for publication.
Conflict of Interest
The authors declare that the research was conducted in the absence of any commercial or financial relationships that could be construed as a potential conflict of interest.
Supplementary Material
The Supplementary Material for this article can be found online at: https://www.frontiersin.org/articles/10.3389/fmicb.2020.593911/full#supplementary-material
References
Alonso-Calleja, C., Martínez-Fernàndez, B., Prieto, M., and Capita, R. (2004). Microbiological quality of vacuum-packed retail ostrich meat in Spain. Food Microbiol. 21:241e246.
ASTM E2871–12 (2012). Standard Test Method for Evaluating Disinfectant Efficacy Against Pseudomonas aeruginosa Biofilm Grown in the CDC Biofilm Reactor Using the Single Tube Method. West Conshohocken: ASTM International.
Bekhit, A. A., Hopkins, D. L., Fahri, F. T., and Ponnampalam, E. N. (2013). Oxidative processes in muscle systems and fresh meat: sources, markers, and remedies. Compr. Rev. Food Sci. Food Saf. 12, 565–597. doi: 10.1111/1541-4337.12027
Benli, H., Sanchez-Plata, M. X., and Keeton, J. T. (2011). Efficacy of epsilon-polylysine, lauric arginate, or acidic calcium sulfate applied sequentially for Salmonella reduction on membrane filters and chicken carcasses. J. Food Prot. 74, 743–750. doi: 10.4315/0362-028x.jfp-10-463
Cardenas, F. C., Andres, S., Giannuzi, L., and Zaritzky, N. (2011). Antimicrobial action and effects on beef quality attributes of a gaseous ozone treatment at refrigeration temperatures. Food Control. 22, 1442–1447. doi: 10.1016/j.foodcont.2011.03.006
Castillo, A., Mckenzie, K. S., Lucia, L. M., and Acuff, G. R. (2003). Ozone treatment for reduction of Escherichia coli O157:H7 and Salmonella serotype Typhimurium on beef carcass surfaces. J. Food Prot. 66, 775–779. doi: 10.4315/0362-028x-66.5.775
Centers for Disease Control and Prevention (2011). Multistate Outbreak of Human Salmonella Heidelberg Infections Linked to Ground Turkey. Atlanta: CDC.
Chen, X. I., Bauermeister, L. J., Hill, G. N., Singh, M., Bilgili, S. F., and McKee, S. R. (2014). Efficacy of various antimicrobials on reduction of Salmonella and Campylobacter and quality attributes of ground chicken obtained from poultry parts treated in a postchill decontamination tank. J. Food Prot. 77, 1882–1888. doi: 10.4315/0362-028x.jfp-14-114
Dickson, J. S., and Anderson, M. E. (1992). Microbiological decontamination of food animal carcasses by washing and sanitizing systems: a review. J. Food Prot. 55, 133–140. doi: 10.4315/0362-028x-55.2.133
Dittoe, D. K., Feye, K. M., Peyton, B., Worlie, D., Draper, M. J., and Ricke, S. C. (2019). The addition of ViriditecTM aqueous ozone to peracetic acid as an antimicrobial spray increases air quality while maintaining Salmonella Typhimurium, non-pathogenic Escherichia coli, and Campylobacter jejuni reduction on whole carcasses. Front. Microbiol. 9:3180. doi: 10.3389/fmicb.2018.03180
Food and Agriculture Organization of the United Nations [FAO] (2014). FISHSTAT. Global Aquaculture Production. Rome: FAO.
Franchin, P. R., Battistella, P. D., and Vieira, C. W. (2010). Evaluation of multi-sequential interventions with water to reduce microbial loading as applied to chicken carcasses during slaughtering – A review. World Poult. Sci. J. 66, 203–2014. doi: 10.1017/s0043933910000267
Gardoni, D., Vailati, A., and Canziani, R. (2012). Decay of ozone in water. Ozone Sci. Eng. 34, 233–242. doi: 10.1080/01919512.2012.686354
Gonçalves, M. A., Bello, N. M., Dritz, S. S., Tokach, M. D., DeRouchey, J. M., and Woodworth, J. C. (2016). An update on modeling dose–response relationships: accounting for correlated data structure and heterogeneous error variance in linear and nonlinear mixed models. J. Anim. Sci. 94, 1940–1950. doi: 10.2527/jas.2015-0106
Guastalli, B. H. L., Batista, D. F. A., Souza, A. I. S., Guastalli, E. A. L., Lopes, P. D., Almeida, A. M., et al. (2016). Evaluation of disinfectants used in pre-chilling water tanks of poultry processing plants. Rev. Bras. Cienc Avic. 18, 217–223. doi: 10.1590/1806-9061-2015-0110
Haute, S. V., López-Gálvez, F., Gómez-López, V. M., Eriksson, M., Devlieghere, F., Allende, A., et al. (2015). Methodology for modeling the disinfection efficiency of fresh-cut leafy vegetables wash water applied on peracetic acid combined with lactic acid. Int. J. Food Microbiol. 208, 102–113. doi: 10.1016/j.ijfoodmicro.2015.05.020
Hirahara, Y., Iwata, K., and Nakamuro, K. (2019). effect of citric acid on prolonging the half-life of dissolved ozone in water. Food Saf. 7, 90–94. doi: 10.14252/foodsafetyfscj.d-19-00005
Hoffmann, S., Batz, M. B., and Morris, J. G. (2012). Annual cost of illness and quality-adjusted life year losses in the United States due to 14 foodborne pathogens. J. Food Prot. 75, 1292–1302. doi: 10.4315/0362-028x.jfp-11-417
Jakobsen, L., Garneau, P., Bruant, G., Harel, J., Olsen, S. S., Porsbo, L. J., et al. (2012). Is Escherichia coli urinary tract infection a zoonosis? Proof of direct link with production animals and meat. Eur. J. Clin. Microbiol. Infect. Dis. 31, 1121–1129. doi: 10.1007/s10096-011-1417-5
Jindal, V., Waldroup, A. L., Forsythe, R. H., and Miller, M. J. (1995). Ozone and improvement of quality and shelf-life of poultry products. J. Appl. Poult. Res. 4, 239–248. doi: 10.1093/japr/4.3.239
Kottwitz, L. M., Oliveira, T. M., Alcocer, I., Farah, S. S., Abrahão, W. M., and Rodrigues, D. P. (2010). Avaliação epidemiológica de salmonelose ocorridos no período de 1999 a 2008 no estado do Paraná, Brasil. Acta Scientiarum Health Sci. 32, 9–15.
Kumar, S., Singh, M., Cosby, D. E., Cox, N. A., and Thippareddi, H. (2020). Efficacy of peroxy acetic acid in reducing Salmonella and Campylobacter spp. populations on chicken breast fillets. Poult. Sci. 99, 2655–2661. doi: 10.1016/j.psj.2019.12.045
Li, X., and Ji, G. (2017). Evaluation of the direct relationship between bacterial load on contaminated stainless steel surgical instruments and the holding time prior to disinfection and also to analyse the efficacy of different disinfecting solutions. Biomed. Res. 28, 4680–4687.
Lottenbach, R., Kelly-Aehle, S. M., Brenneman, K. E., Curtiss, R., and Freya, S. E. (2013). Rapid, sensitive recovery of recombinant attenuated Salmonella enterica serovar typhi vaccine strains from human blood. Clin. Vaccine Immunol. 9, 1473–1478. doi: 10.1128/cvi.00331-13
Mancini, R. A., and Hunt, M. C. (2005). Current research in meat color. Rev. Meat Sci. 71, 100–121. doi: 10.1016/j.meatsci.2005.03.003
Megahed, A., Aldridge, B., and Lowe, J. (2018a). The microbial killing capacity of aqueous and gaseous ozone on different surfaces contaminated with dairy cattle manure. PLoS One 13:e0196555. doi: 10.1371/journal.pone.0196555
Megahed, A., Brian, A., and James, L. (2018b). Evaluation of the decontamination power of aqueous and gaseous ozone on various contaminated surfaces with cattle manure containing Salmonella. J. Adv. Dairy Res. 6:205.
Megahed, A. A., Aldridge, B., and Lowe, J. F. (2019). Comparative study on the efficacy of sodium hypochlorite, aqueous ozone, and peracetic acid in the elimination of various Salmonella typhimurium from cattle manure contaminated surfaces supported by Bayesian analysis. PLoS One 14:e0217428. doi: 10.1371/journal.pone.0217428
National Chicken Council (2011). How broilers are marketed. Daily Livestock Report. Available online at: http://www.nationalchickencouncil.org/about-the-industry/statistics/how-broilersare-marketed/ (Accessed May 10, 2013).
Nelson, M., LaBudde, R. A., Tomasino, S. F., and Pines, R. M. (2013). Comparison of 3MTM PetrifilmTM Aerobic count plates to standard plating methodology for use with AOAC antimicrobial efficacy methods 955.14, 955.15, 964.02, and 966.04 as an alternative enumeration procedure: collaborative study. J. AOAC Int. 96, 717–722. doi: 10.5740/jaoacint.12-469
Northcutt, J. K., Smith, D. P., Musgrove, M. T., Ingram, K. D., and Hinton, A. (2005). microbiological impact of spray washing broiler carcasses using different chlorine concentrations and water temperatures. Poult. Sci. 84, 1648–1652. doi: 10.1093/ps/84.10.1648
Qiao, M., Fletcher, D. L., Northcutt, J. K., and Smith, D. P. (2002). The realtionship between raw broiler breast meat color and composition. Poult. Sci. 81, 422–427. doi: 10.1093/ps/81.3.422
Qingshi, Z., Cunli, L., and Zhengyu, X. (1989). A study of contacting systems in water and wastewater disinfection by ozone. 1. Mechanism of ozone transfer and inactivation related to the contacting method selection. Ozone Sci. Engrg. 11, 169–188. doi: 10.1080/01919518908552434
Ramirez-Hernandez, A., Brashears, A. M., and Sanchez-Plat, M. X. (2018). Efficacy of lactic acid, lactic acid–acetic acid blends, and peracetic acid to reduce Salmonella on chicken parts under simulated commercial processing conditions. J. Food Prot. 81, 17–24. doi: 10.4315/0362-028x.jfp-17-087
Restaino, L., Frampton, E. W., Hemphill, J. B., and Palnikar, P. (1995). Efficacy of ozonated water against various food-related microorganisms. Appl. Environ. Microbiol. 61, 3471–3475. doi: 10.1128/aem.61.9.3471-3475.1995
Russel, A. D., Hugo, W. B., and Avliffe, G. A. (1999). Principles and Practice of Disinfection, Preservation, and Sterilization. Oxford: Blackwell Science.
Russell, S. M. (2001). “Spoilage bacteria associated with poultry,” in Poultry Meat Processing, ed. A. R. Sams (Boca Raton, FL: CRC Press), 159–179.
Sanchez-Escalante, A., Djenane, D., Torrescano, G., Beltran, A., and Roncales, P. (2001). The effects of ascorbic acid, taurine, carnosine and rosemary powder on colour and lipid stability of beef patties packaged in modified atmosphere. Meat Sci. 58, 421–429. doi: 10.1016/s0309-1740(01)00045-6
Scallan, E., Hoekstra, R. M., Angulo, F. J., Tauxe, R. V., Widdowson, M. A., Roy, S. L., et al. (2011). Foodborne illness acquired in the United States–major pathogens. Emerg. Infect. Dis. 17, 7–15. doi: 10.3201/eid1701.p11101
Scharff, R. L. (2012). Economic burden from health losses due to foodborne illness in the United States. J. Food Prot. 75, 123–131. doi: 10.4315/0362-028x.jfp-11-058
Sheldon, B. W., and Brown, A. L. (1986). Efficacy of ozone as a disinfectant for poultry carcasses and chill water. Food Sci. 51, 305–309. doi: 10.1111/j.1365-2621.1986.tb11116.x
Silva, E. P., Bergamini, A. M. M., and Oliveira, M. A. (2010). Alimentos e agentes etiológicos envolvidos em toxinfecções na região de Ribeirão Preto, SP, Brasil –2005 a 2008. Boletim Epidemiol. Paulista 7, 4–10.
Sohaib, M., Anjum, F. M., Arshad, M. S., and Rahman, U. U. (2016). Postharvest intervention technologies for safety enhancement of meat and meat based products; a critical review. J. Food Sci. Technol. 53, 19–30. doi: 10.1007/s13197-015-1985-y
Stivarius, M. R., Pohlman, F., Mcelyea, K., and Apple, J. K. (2002). Microbial, instrumental and sensory color and odour characteristics of ground beef produced from beef trimmings treated with ozone and chlorine dioxide. Meat Sci. 60, 299–305. doi: 10.1016/s0309-1740(01)00139-5
Tan, S. M., Lee, S. M., and Dykes, G. A. (2014). Buffering effect of chicken skin and meat protects Salmonella enterica strains against hydrochloric acid but not organic acid treatment. Food Control. 42, 329–334. doi: 10.1016/j.foodcont.2014.02.031
Thomas, C. J., and McMeekin, T. A. (1980). Contamination of broiler carcass skin during commercial processing procedures: an electron microscopy study. Appl. Environ. Microbiol. 40, 133–144. doi: 10.1128/aem.40.1.133-144.1980
Trefz, F. M., Lorenz, I., and Constable, P. D. (2018). Electrocardiographic findings in hospitalized neonatal calves with diarrhea and associated potassium balance disorders. J. Vet. Int. Med. 32, 1447–1461. doi: 10.1111/jvim.15220
Trindade, M. A., Kushida, M. M., Villanueva, N. D. M., Pereira, D. S., and Oliveira, C. L. (2012). Comparison of ozone and chlorine in low concentrations as sanitizing agents of chicken carcasses in the water immersion chiller. J. Food Prot. 75, 1139–1143. doi: 10.4315/0362-028x.jfp-11-288
United States Department of Agriculture (2002). The Use of Chlorine Dioxide As An Antimicrobial Agent in Poultry Processing in the United States. Washington, DC: USDA-FSIS, Office of International Affairs.
United States Department of Agriculture, Food Safety, and Inspection Service (2015). Changes to the Salmonella and Campylobacter verification testing program: proposed performance standards for Salmonella and Campylobacter in not-ready-to-eat comminuted chicken and turkey products and raw chicken parts and related agency verification procedures and other changes to agency sampling. Docket FSIS-2014-0023. Fed. Regist. 80, 3940–3950.
Van Netten, P., Huis Veld, J., and Mossel, D. A. (1994). The immediate bactericidal effect of lactic acid on meat-borne pathogens. J. Appl. Bact. 77, 490–496. doi: 10.1111/j.1365-2672.1994.tb04392.x
Veerkamp, C. H. (1990). Chilling of Poultry and Poultry Products, in Chilled Foods – the State of Art. New York, NY: Elsevier Applied Sciences, 147–158.
Wigley, P., Hulme, S., Powers, C., Beal, R., Smith, A., and Barrow, P. (2005). Oral infection with the Salmonella enterica serovar Gallinarum 9R attenuated live vaccine as a model to characterize immunity to fowl typhoid in the chicken. BMC Vet. Res. 1:2. doi: 10.1186/1746-6148-1-2
Keywords: aqueous ozone, ozone–lactic acid blend, sequential soaking, sequential spray, Salmonella, bacterial load, chicken parts
Citation: Megahed A, Aldridge B and Lowe J (2020) Antimicrobial Efficacy of Aqueous Ozone and Ozone–Lactic Acid Blend on Salmonella-Contaminated Chicken Drumsticks Using Multiple Sequential Soaking and Spraying Approaches. Front. Microbiol. 11:593911. doi: 10.3389/fmicb.2020.593911
Received: 16 August 2020; Accepted: 16 November 2020;
Published: 14 December 2020.
Edited by:
Julio Parra-Flores, University of the Bío Bío, ChileReviewed by:
Alejandro Castillo, Texas A&M University, United StatesGilles Salvat, Agence Nationale de Sécurité Sanitaire de l’Alimentation, de l’Environnement et du Travail (ANSES), France
Copyright © 2020 Megahed, Aldridge and Lowe. This is an open-access article distributed under the terms of the Creative Commons Attribution License (CC BY). The use, distribution or reproduction in other forums is permitted, provided the original author(s) and the copyright owner(s) are credited and that the original publication in this journal is cited, in accordance with accepted academic practice. No use, distribution or reproduction is permitted which does not comply with these terms.
*Correspondence: James Lowe, jlowe@illinois.edu