- 1Division of Microbiology and Fermentation, Department of Food Science, University of Copenhagen, Frederiksberg, Denmark
- 2Department of Food Science and Nutrition, Faculty of Food Engineering, University of Campinas, Campinas, Brazil
- 3Department of Food Engineering, School of Animal Science and Food Engineering, University of São Paulo, Pirassununga, Brazil
The increased consumption of plant-based foods has intensified the concern related to mycotoxin intoxication. This study aimed to investigate the effect of selected lactic acid bacteria (LAB) strains on the growth of Aspergillus parasiticus NRRL 2999 and its production of aflatoxin (AF). The ability of the heat-killed (100°C for 1 h) LAB strains to bind aflatoxin M1 (AFM1) in milk and aflatoxin B1 (AFB1), ochratoxin A (OTA), and zearalenone (ZEN) in potassium phosphate buffer (PPB) was also evaluated in vitro. Ten LAB strains were tested individually, by inoculating them simultaneously with the fungus or after incubation of the fungus for 24 or 48 h at 25°C. Double layer yeast extract sucrose (YES) agar, de Man Rogosa and Sharpe (MRS) agar, and YES broth were incubated for 7 days at 25°C to follow the development of the fungus. Levilactobacillus spp. 3QB398 and Levilactobacillus brevis 2QB422 strains were able to delay the growth of A. parasiticus in YES broth, even when these strains were inoculated 24 h after the fungus. The inhibitory effect of these LAB strains was confirmed by the reduction of fungus colony size, suggesting dominance of LAB by competition (a Lotka-Voltera effect). The production of AFB1 by A. parasiticus was inhibited when the fungus was inoculated simultaneously with Lactiplantibacillus plantarum 3QB361 or L. plantarum 3QB350. No AFB1 was found when Levilactobacillus spp. 2QB383 was present, even when the LAB was inoculated 48 h after the fungus. In binding studies, seven inactivated LAB strains were able to promote a reduction of at least 50% the level of AFB1, OTA, and ZEN. This reduction varied depending on the pH of the PPB. In milk, however, only two inactivated LAB strains were able to reduce AFM1, with a reduction of 33 and 45% for Levilactobacillus spp. 3QB398 (Levilactobacillus spp.) and L. brevis 2QB422, respectively. Nevertheless, these results clearly indicate the potential of using LAB for mycotoxin reduction.
Introduction
An important food safety concern is the presence of natural contaminants such as the mycotoxins (Schatzmayr and Streit, 2013; Eskola et al., 2020; Baazeem et al., 2021). In 2019, mycotoxins were reported to be the main hazard in food products at EU borders (RASFF, 2020). As recently as 2020, a survey of global food crop contamination reported an incidence of 25% of mycotoxins above the EU and Codex limits (Eskola et al., 2020). The potential toxic effects of mycotoxins including immunosuppression and carcinogenic effects have stimulated considerable research efforts aiming at reducing the mycotoxin levels in foods (Corassin et al., 2013; Oliveira et al., 2014; Gummadidala et al., 2019; Cuevas-González et al., 2020; Gao et al., 2020). In particular, the aflatoxins (AFs) B1 (AFB1) and M1 (AFM1) were classified in Group 1 (human carcinogen) by the International Agency for Research on Cancer (2012), while ochratoxin A (OTA) was categorized in Group 2B, probable carcinogen to humans (International Agency for Research on Cancer, 1993). The incidence of AFM1 in milk is of concern, because milk is the main nutritional source for infants and children (Conteçotto et al., 2021). AFM1 in the milk is derived from the biotransformation of AFB1 by the cow (Barnes, 1970; Galvano et al., 1996; Pauletto et al., 2020). Since AFM1 binds to the casein in the milk, it may be carried into high-content protein products such as cheeses, and this is worrying considering the highly consumption of cheeses by people of all ages (Khaneghah et al., 2021). Considering the health risks posed by AFM1, several countries have set maximum permissible levels (MPL) for this toxin in milk. The European Union and the United States Food and Drug Administration (FDA) have set MPLs of 0.05 and 0.5 μg L−1 for AFM1 in milk, respectively (Oliveira et al., 2014).
Many genera of molds have the ability to produce mycotoxins (Campagnollo et al., 2020). The main genera of mycotoxin-producing fungi include Aspergillus, Penicillium, Fusarium, and Alternaria (Egbuta et al., 2017). The mycotoxins produced by these genera include the AFs, fumonisins (FBs), OTA, zearalenone (ZEN), and trichothecenes such as deoxynivalenol (DON; Palumbo et al., 2020). Levels of these mycotoxins in food are regulated in many countries because of their frequent occurrence and their high toxigenic potential (Oliveira et al., 2014; Franco et al., 2019). The main food commodities produced worldwide and associated with mycotoxin contamination are mainly cereals, seeds, nuts, fruit, vegetables, herbs, and spices (RASFF, 2020).
The present situation clearly shows that mycotoxin occurrence is an increasing worldwide problem despite the many developments of good agricultural and manufacturing practices in the food chain. The impact of mycotoxins on human and animal health, as well as on economic losses is significant (Eskola et al., 2020). According to a recent estimation (World Health Organization, 2016), the mycotoxin contamination problem is especially evident in developing countries, where 500 million people are exposed to mycotoxins, and 160 million children under the age of five suffer from stunting associated to exposure to these toxins. Besides the high cost related to research and regulatory activities, the yearly direct impact associated with mycotoxins in food and feed production is immense, with the extent varying according to the region and the weather (Gbashi et al., 2018; Agriopoulou et al., 2020). In the United States, the aflatoxin-associated losses in food and feed crops are estimated to be between 300 million and 1.7 billion USD per year (Pitt and Miller, 2017). While 900 million USD per year are the total social costs of aflatoxin estimated to the Philippines, Thailand, and India (European Commission, 2016). The annual cost related to crops contaminated with aflatoxins in Africa is estimated to be between 670 and 750 million USD (Gbashi et al., 2018). The detailed data related to economic impact in the EU are not reported. Avoidance of mycotoxigenic fungi in the field is of extreme importance as the level of mycotoxins in the plants increases due to the environmental conditions (temperature and humidity) and management practices, especially during storage after harvest. Since mycotoxins are stable and cannot be reduced during processing, they end up in the crops used in the production of plant-based foods (Bullerman and Bianchini, 2007; Cinar and Onbaşı, 2019). Natural solutions have been proposed to prevent mycotoxin formation and to degrade or avoid the bioavailability of mycotoxins in foods (Avantaggiato et al., 2014; Agriopoulou et al., 2020; Muhialdin et al., 2020). Efforts to investigate the suitability of lactic acid bacteria (LAB) have been proposed since the 1960s (Ciegler et al., 1966), but the potential of these bacteria to reduce the risk associated with mycotoxins is still at an early research stage. In this context, three main mechanisms have been proposed for mycotoxin reduction by LAB: (i) binding of the mycotoxin to the bacterial cell wall components, (ii) degradation of the mycotoxins into less toxic substances by the LAB, or (iii) inhibition of the mold growth or aflatoxin biosynthesis by specific bacterial metabolites (Ben Taheur et al., 2019a).
Many LAB are regarded by the FDA and general recognized as safe (GRAS), while the European Food Safety Authority (EFSA) applies the qualified presumption of safety (QPS) principle (EFSA Panel on Biological Hazards (BIOHAZ) et al., 2017; FDA, 2018; Hill et al., 2018). Their nature of non-pathogenicity and non-toxicity allows many applications of LAB with the purpose of improving safety and quality in food processing. In a recent study, Gonçalves et al. (2020) successfully used heat-killed cells of LAB (Lacticaseibacillus rhamnosus and Lactococcus lactis) and Saccharomyces cerevisiae, alone or in combination, for binding up to 100% of AFM1 in cheese. Furthermore, a Lentilactobacillus kefiri strain was able to reduce 80–100% of AFB1, OTA, and ZEN through binding in milk (Ben Taheur et al., 2017). Despite the reported positive effect of LAB in the reduction of mycotoxins, the usefulness of LAB it is still uncertain when the level of mycotoxins is high and well-established in the food matrix (Ben Taheur et al., 2019a). Intracellular and extracellular extracts of microorganisms, like Pediococcus parvulus, Bacillus subtilis, and Candida utilis have proven to be important sources of enzymes that are able to biotransform some of the well-known mycotoxins, such as OTA (Abrunhosa et al., 2014), ZEN, and AFB1 (Huang et al., 2018). Various pathways have been proposed for mycotoxin biodegradation (Zhang et al., 2018; Ben Taheur et al., 2019a).
The purpose of this work was to screen the ability of the selected LAB strains to inhibit growth of A. parasiticus and to reduce the formation of AFB1, AFB2, AFG1, and AFG2. In addition, the ability of the strains to reduce the levels of the mycotoxins in spiked buffer models (AFB1, OTA, and ZEN) and milk (AFM1) was examined.
Materials and Methods
Lactic Acid Bacteria Strains
Ten LAB isolates from Brazilian artisanal cheeses (isolation conditions indicated in Table 1) were selected; four strains of Lactiplantibacillus plantarum (formerly Lactobacillus plantarum, comprising strains 1QB147, 1QB314, 3QB350, and QB361), two strains of Levilactobacillus brevis (formerly Lactobacillus brevis, comprising strains 2QB422 and 3QB446), and four strains of Levilactobacillus spp. (formerly Lactobacillus spp., comprising strains 1QB4593, 2QB383, 3QB167, and QB398). The identity of the isolates was confirmed using the MALDI-TOF MS Biotyper by Margalho et al. (2020).
Growth Experiments
Preparation of LAB Inoculum
Inocula of the 10 strains were prepared according to Møller et al. (2021). Briefly, 100 μl of each of the thawed frozen stocks (−80°C, in 20% glycerol) were individually inoculated into 10 ml MRS broth, and incubated at 30°C in a Biological Oxygen Demand (BOD) incubator (Tecnal, Sao Paulo, Brazil) for 24 h, at static condition in the dark. The grown culture was centrifuged (6,000 × g for 10 min at 4°C), and the cell pellet was resuspended in 10 ml 0.9% NaCl and centrifuged (6,000 × g for 10 min at 4°C). The washed, resuspended culture was adjusted to 0.5 MCFarland (~ 108 CFU/ml) and diluted in 0.9% NaCl in order to obtain a final cell density of 5.0 log10 CFU g−1 (checked by plate count).
Preparation of the Conidial Suspension
Aspergillus parasiticus NRRL 2999 (a strain with the ability to produce mycotoxins such as AFB1, AFB2, AFG1, and AFG2) was kindly donated by the United States Department of Agriculture. This strain was isolated from soil in Uganda as reported by Singh and Cotty (2019). The strain was prepared according to Wigmann et al. (2016) by inoculating it in Malt Extract Agar (MEA; Acumedia, Lansing, Michigan, United States; Malt Extract: 20.0 g; Peptone: 1.0 g; Glucose: 30.0 g; Agar: 20 g; and Distilled Water: 1 L) and incubating it aerobically at 25°C in a BOD incubator for 5 days, at static condition in the dark. Conidia were collected by scraping the mycelium from each plate with sterile distilled water containing 0.1% Tween 80 (Labsynth, Diadema, Brazil). Subsequently, they were filtered and then centrifuged at 11,000 g three consecutive times for 15 min at 4°C (Sorvall Legend XTR, Thermo Scientific, Hampton, Unites States). The final concentration of conidia in the fungal suspension was determined using a Neubauer chamber (Sigma-Aldrich, Darmstadt, Germany). The conidial suspension was diluted and used to reach a final concentration of 5.0 log10 cfu ml−1 in the growth trials (checked by plate count).
Incubation of LAB With A. parasiticus in Broth
The method was adapted from Bouillon et al. (2019) and performed in 200 μl YES broth using 96-well microtiter plates. Negative control (50 μl of non-inoculated 0.9% saline), positive control of the fungus (25 μl of the fungus suspension plus 25 μl of 0.9% saline), and positive control of each LAB (25 μl inoculum of the individual LAB strain plus 25 μl of 0.9% saline) were added to 200 μl YES broth. When the fungus was tested in combination with the respective LAB strain, a 25 μl inoculum of each microorganism was added to 200 μl YES broth, and a final volume of 250 μl was reached. The final concentration was 5.0 log10 cfu ml−1 for the LAB and/or 5.0 log10 spores ml−1 for the fungus. Three independent trials were performed in triplicate. The microtiter plates were incubated at 25°C in a BOD incubator (Tecnal, Sao Paulo, Brazil) for 7 days, at static condition in the dark. Growth of the fungus in the YES broth was evaluated after incubation by visual inspection of the microplates. The treatments were compared relatively, and the effect of each tested combination was ranked in relation to the fungus positive control. Symbols were assigned according to the effect of each LAB in comparison with the growth of A. parasiticus alone (positive control of the fungus). (+++) indicates the maximum effect on growth without total inhibition of the fungus growth by LAB and (+) indicates very little effect of LAB on growth of the fungus.
Incubation of LAB With A. parasiticus Using an Agar Plate Technique
A double-layer agar technique was adapted from Aunsbjerg et al. (2015) and applied in triplicate using petri dishes (8.5 cm round shape). One milliliter inoculum of the individual LAB strains (final concentration of 5 log10 cfu mL−1) was covered with 10 ml MRS agar, which when solidified was covered with 10 ml YES Agar (Samson et al., 2002). Ten microliters of the fungus suspension (final concentration of 5 log10 spores ml−1) were drop plated into the center of the plate of the solidified YES agar. The development of the fungus was followed by measuring the diameter of fungal growth every 24 h over a 7 days incubation period at 25°C. Plates were incubated in a BOD incubator (Tecnal, Sao Paulo, Brazil), at static condition in the dark.
Inhibition of A. parasiticus Growth and Mycotoxins Production in Broth
The 10 LAB strains were applied individually at the level of 5.0 log10 cfu ml−1 at each of the following three inoculum time-points to the YES broth (10 ml): (a) simultaneously with the A. parasiticus (5.0 log10 spores ml−1); (b) after 24 h of incubation of the A. parasiticus; or (c) after 48 h of incubation of the A. parasiticus. All incubations were performed at 25°C, at static condition in the dark. Samples (1 ml) for mycotoxin determination were taken just after inoculation with A. parasiticus alone (control) or combined with the tested LAB strain. Further 1 ml samples were taken 7 days after the inoculation of the fungus. Samples were dispersed in Eppendorf tubes, centrifuged at 3,000 × g for 15 min, and the supernatants were kept at −20°C until analysis. Samples were prepared by diluting 20 μl of supernatants in 2 ml of milli-Q water (Millipore, Burlington, MA, United States). Then, 100 μl of the diluted supernatant were mixed with 900 μl acetonitrile:water (50:50), and 500 μl were transferred to a new Eppendorf tube containing 200 μl hexane and 100 μl trifluoroacetic acid for derivatization of AFB1 and AFG1 (Oliveira et al., 2008). The mixture was kept at 35°C for 10 min, then evaporated to near-dryness, and diluted in 500 μl acetonitrile:water (50:50). Final extracts were filtered through a 0.45 μm PTFE membrane and subjected to AF determination using HPLC according to Oliveira et al. (2008). Triplicate samples were analyzed by injecting 20 μl of sample with an isocratic elution by water:acetonitrile:methanol (60:20:20) for a run time of 10 min (flow rate of 1.0 ml min−1) in a Shimadzu 10VP liquid chromatograph (Kyoto, Japan) with a 10 AXL fluorescence detector (excitation at 360 nm and emission above 440 nm). A Kinetex C18 column (Phenomenex, Torrance, CA, United States) 4.6 × 150 mm, 2.6 μm particle size and an in-line filter of 0.5 μm were used. The limit of detection (LOD) and the limit of quantification (LOQ) were calculated from matrix matched calibration curves using signal to noise ratios of 3 and 10, respectively. Under these conditions, the LOD and LOQ values for each aflatoxin (AFB1, AFB2, AFG1, or AFG2) were 0.1 and 0.3 μg ml−1, respectively.
Testing the Reduction of Mycotoxins by the Binding Ability of LAB
Preparing the LAB Cells
The LAB strains were also investigated for their potential to reduce levels of mycotoxins in spiked buffer and milk. Preparation of the cells was performed according to Bovo et al. (2013). The LAB inocula were obtained as described in section “Preparation of the conidial suspension” (before washing steps). The 1 ml bacterial suspensions (9.0 log10 cfu ml−1) were dispersed in Eppendorf tubes, centrifuged at 3,000 × g for 15 min, and the cell pellets exposed to 100°C for 1 h. The heat-treated cell pellets were then washed twice with 0.9% NaCl and kept at −20°C until execution of the binding studies.
Binding Studies in Milk Spiked With Aflatoxin M1
One milliliter skimmed milk spiked with AFM1 at the level of 500 ng L−1 was added to the bacterial cell pellet (obtained from 1 ml of the 9.0 log10 cfu ml−1 bacterial suspension, inactivated as described in section “Preparing the LAB cells”) and incubated at 37°C for 15 min (Ismail et al., 2017). Negative controls (milk + LAB cells) and positive controls (milk + AFM1) were also prepared. All incubations were performed in triplicate. After incubation, the cells were removed by centrifugation (3,000 × g for 15 min) and the supernatant was collected for quantification of the remaining AFM1.
Binding Studies in Buffer Spiked With AFB1, OTA, and ZEN
One milliliter samples of 0.1 M potassium phosphate buffer (PPB) with different pH values (3.0 or 6.5) and spiked with AFB1, OTA (at the level of 1.0 μg ml−1), and ZEN (2.0 μg ml−1), were added to the bacterial cells pellet (obtained from 1 ml of the 9.0 log10 cfu ml−1 bacterial suspension, inactivated as described in section “Preparing the LAB cells”) and incubated at 37°C for 5 as well as for 15 min (Campagnollo et al., 2015). Negative controls (PPB + LAB cells) and positive controls (PPB + mycotoxin mixture) were also prepared. All incubations were performed in triplicate. After incubation, the cells were removed by centrifugation at 3,000 × g and the supernatants were collected for quantification of remaining AFB1, OTA, and ZEN for each treatment.
Quantification of Mycotoxins
Aflatoxin M1 was extracted from supernatants of spiked milk samples as described by Flores-Flores and González-Peñas (2017). Supernatants from spiked buffer samples were individually diluted in acetonitrile (100 μl in 10 ml), and after filtration (0.22 μm) were again diluted in acetonitrile (100 μl in 1.0 ml), and then collected for quantification of AFB1, OTA, and ZEN according to Franco et al. (2019). Five microliter of each final extract from milk samples or diluted buffer samples were injected into a Waters Acquity I-Class system (Waters, Milford, MA, United States) equipped with a BEH C18 column (2.1 × 50 mm, 1.7 μm) and coupled to a Xevo TQ-S® mass spectrometer (Waters, Milford, MA, United States). The chromatographic conditions were as described by Franco et al. (2019), with a flow rate of 0.5 ml min−1 (total chromatographic run time of 10 min). MS/MS parameters were as stated in Table 2.
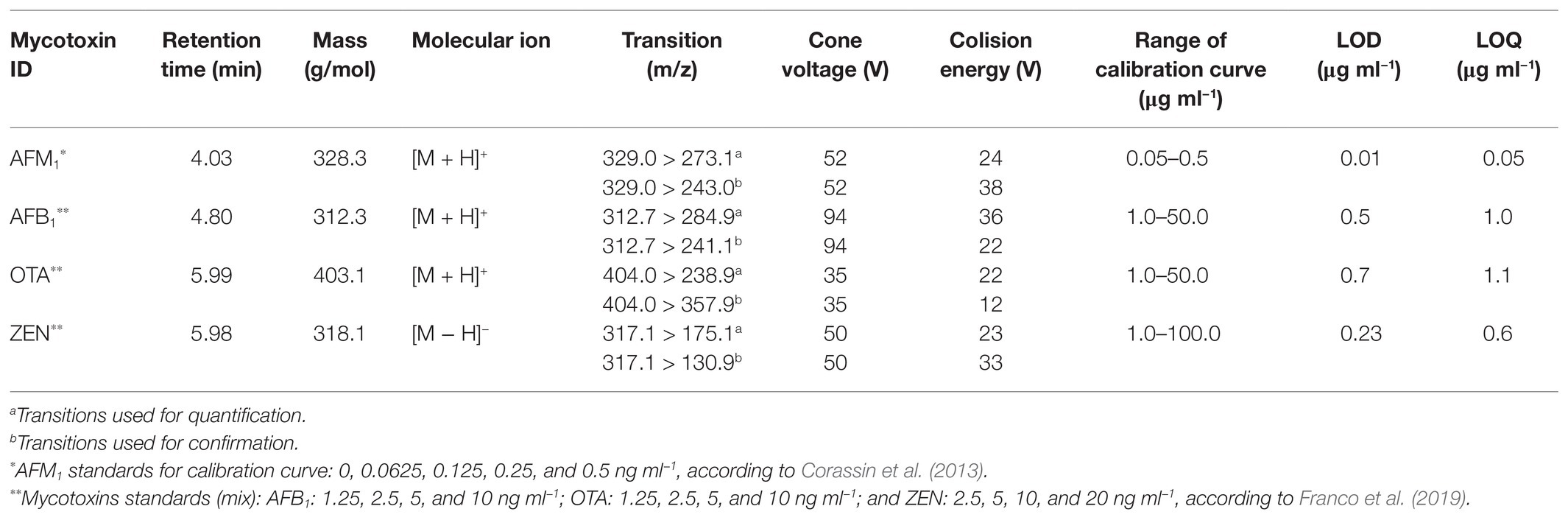
Table 2. LC-MS/MS conditions for investigating the levels of aflatoxin (AF) M1 (AFM1) in skimmed spiked milk (5.0 ng L−1), as well as aflatoxin B1 (AFB1) ochatoxin A (OTA), and zearalenone (ZEN) in potassium phosphate buffer (PPB) spiked samples (5.0 ng L−1), after treatment with LAB heat inactivated cells.
Data Analysis
The growth of the fungus was modeled by applying the adapted version (Equation 1) of the primary model developed by Baranyi et al. (1993) and Dantigny et al. (2005), to allow accessing of the effect of LAB on growth of the mycotoxigenic fungus.
Where r is the colony diameter (cm) as a function of time (t), μ is the multiplication rate (cm day−1), and λ is the time obtained by the intersection (days).
Normality test was performed to verify variability in the samples, the Friedman test was applied due to a not normal distribution of data, and evaluation of the differences in the kinetic parameters of the A. parasiticus was performed according to the approach suggested by Ferreira (2011).
The effect of LAB on inhibition of mycotoxins production was evaluated by comparing median, minimum, and maximum values of each treatment (performed in triplicate) to the levels of mycotoxins produced by the non-treated fungus in YES. Medians of AFs levels were compared using Mood Median test of XLStat software (version 2020.1.1) at 5% significance level (Addinsoft, 2021).
The reduction of mycotoxins after the treatment of spiked “in vitro” models and milk, performed in triplicate, were subjected to ANOVA using the General Linear Model of SAS® (SAS Institute, 1992). When applicable, means showing significant differences were compared using the Fisher protected least significant difference test, considering the 0.05 level of probability.
Results and Discussion
Effect of Lactic Acid Bacteria on Growth of Mycotoxigenic Aspergillus parasiticus
The screening of LAB performed in microtiter plate (250 μl) was not conclusive but it gave an indication that all tested LAB strains had an impact on the growth of the fungus (Table 3). While strains 1QB459 (Levilactobacillus spp.), 1QB314 (L. plantarum), and 2QB446 (L. brevis) had a greater impact (+++, Table 3) on growth of A. parasiticus, a lower impact was seen for strains 3QB398 (Levilactobacillus spp.), 3QB167 (Levilactobacillus spp.), and 3QB361 (L. plantarum; +, Table 3). By scaling-up the assay to 10 ml (in test tubes), it was confirmed that strain 2QB422 (L. brevis; Table 3) had the potential to reduce the fungal growth not only when inoculated simultaneously with the fungus but also when it was inoculated at 24 and 48 h after the fungus was incubated. This finding indicates the potential of the strain to inhibit the growth of the fungus, even after the adaptation phase of the fungus to the environment. A possible explanation of the fungal growth inhibition could be the production of antimicrobial peptides, as reported in the literature (Dalié et al., 2010; Muhialdin et al., 2011; Hashemi and Gholamhosseinpour, 2019). While strain 3QB398 (Levilactobacillus spp.) was shown to have inhibition activity against the growth of the fungus at earlier stages of the fungus incubation (0 and 24 h), strains 3QB350 (L. plantarum) and 1QB314 (L. plantarum) were effective in reducing growth when inoculated at the later stages (24 and 48 h) after the incubation of the fungus. It is speculated that while the mechanism of inhibition of strain 3QB398 (Levilactobacillus spp.) may be related to fast production of metabolites in high concentration during growth of the strain, the mechanism of inhibition for strains 3QB350 (L. plantarum) and 1QB314 (L. plantarum) may be due to the slower production of metabolites as well as lower toxicity of the compounds, or even competition for nutrients. These mechanisms of action of LAB have been reported by Ben Said et al. (2019). Furthermore, they also highlighted the capacity of some LAB to adhere strongly to surfaces and survive there for long periods of time. Margalho et al. (2020) investigated the bacteriocin-forming ability of the LAB strains tested in the present study. The bacteriocin-forming ability may be related to the inhibition mechanism of LAB strains such as the strain with the greatest impact on growth of A. parasiticus, which in our study was shown to be L. brevis 2QB422 in all stages of application (Table 3). This strain was also found to be positive for bacteriocin production and effective against different strains of Listeria monocytogenes (Margalho et al., 2020). Similar to our work, Ghanbari et al. (2018) observed that L. plantarum and Lactobacillus delbrueckii subsp. lactis significantly reduced the mycelial growth of A. parasiticus. Luz et al. (2017) also described antifungal activities of L. plantarum CECT 749 against A. parasiticus in potato dextrose agar (PDA), while no activity was observed with Lb. delbrueckii subsp. bulgaricus CECT 4005. Also in agreement with the present study, Poornachandra Rao et al. (2019) showed that a cell-free supernatant of L. plantarum MYS44 inhibited A. parasiticus through general morphological changes, such as destruction of the hyphae wall and inhibition of the germination of the conidia. Strains of Lacticaseibacillus casei and Lactobacillus acidophilus have also shown to have inhibition effects against A. parasiticus (Abbaszadeh et al., 2015). LAB have been reported to produce compounds during growth with potent antifungal effects which may damage the fungal membrane and cell wall structure, resulting in lysis of hyphae and spores (de Souza de Azevedo et al., 2020; Li et al., 2021). As indicated by the two first assays, L. brevis 2QB422 was the most effective strain in suppressing the growth of A. parasiticus, which reached a maximum diameter of 4.91 ± 0.6 cm (Table 3; Figure 1), followed by the range of 5.26–5.46 cm for strains 3QB361 (Lactiplantibacillus plantarum), 3QB398 (Levilactobacillus spp.), 2QB383 (Levilactobacillus spp.), and 2QB446 (L. brevis). In order to clarify the full inhibition potential of the tested LAB strains on the fungus, the diameter was followed daily for 7 days at 25°C (Figure 2). The parameters obtained for the growth rate of the fungus (Table 3), also showed the same effect when comparing the maximum growth reached after 7 days incubation. Since growth of LAB was much faster and the maximum population density was reached at earlier stages of the fungus growth, microbial interaction modeling was not possible. However, it is clear that inhibition was not related to the Jameson effect, which describes the phenomena of the dominant microorganism reaching the maximum population and completely interrupting the growth phase of the other population (Jameson, 1962). In fact, the growth patterns shown in the present study indicate a Lotka-Volterra competition (Lotka, 1956), which describes the phenomena of the dominant microorganism reaching the maximum population and lowering the growth rate of the other microorganism (Table 3). While growth rates below 1.0 cm day−1 were reached by treatment with strains having inhibiting activity against A. parasiticus growth, rates equal and higher than 1.0 cm day−1 were reached when no effect of the strains on the growth of the fungus was shown. Even though the LAB strains were not able to completely suppress the growth of the A. parasiticus, it is clear that they have the potential to extend the shelf life and possibly improve safety of food matrices contaminated with this fungus. Studies in regional cheeses where the LAB strains were isolated are rare and Margalho et al. (2020) are one of the first investigations already reported, which highlights the importance of the present study. Interactions of LAB with mycotoxigenic aspergilli have been proposed to be good examples of biological control in the field. However the need for more research to deeper understand the mechanisms and to develop novel biocontrol technologies remains to be explored (Pócsi et al., 2020).
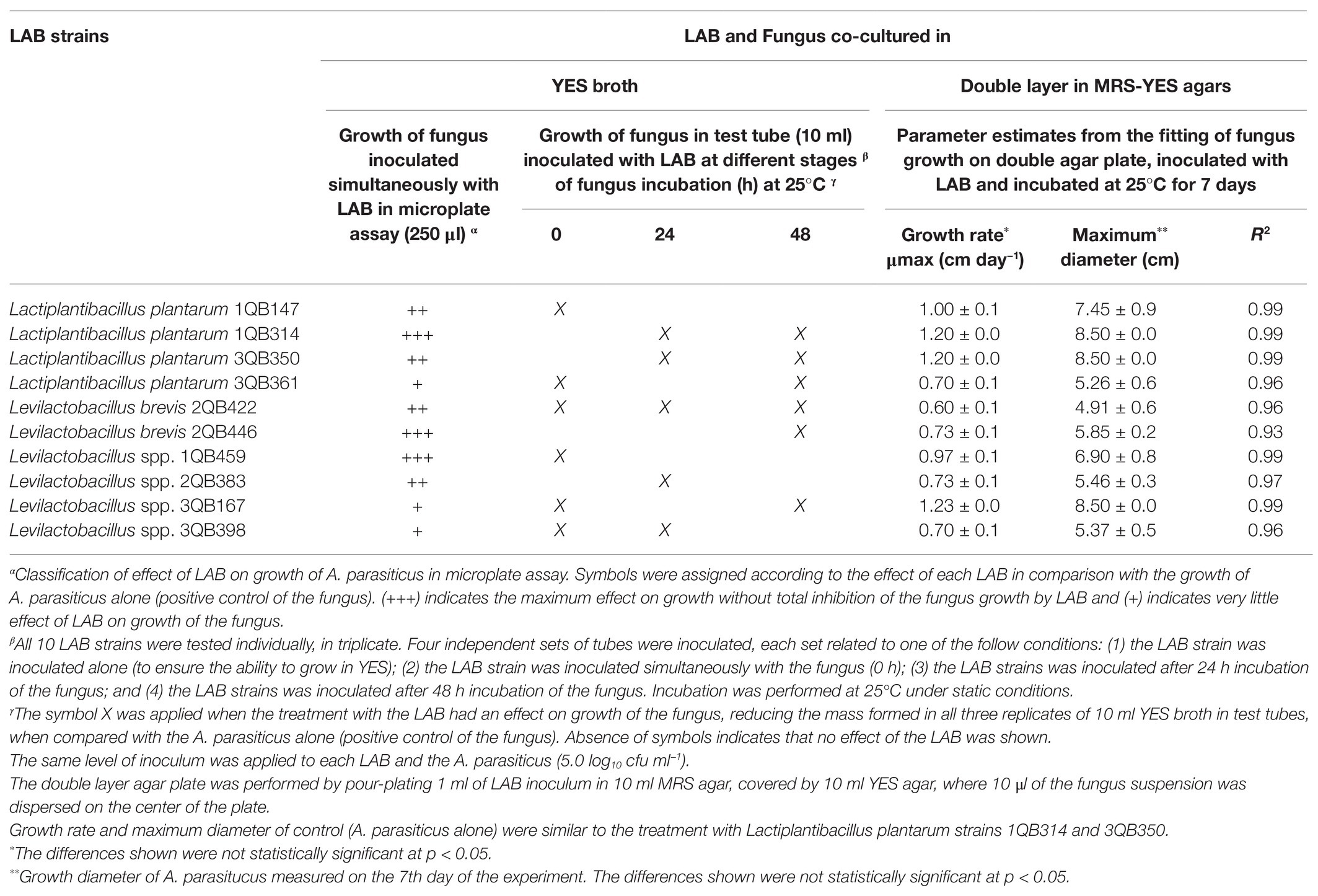
Table 3. Effect of LAB on the growth of mycotoxigenic Aspergillus parasiticus NRRL 2999, investigated in yeast extract sucrose (YES) broth and double layer agar plates with de Man Rogosa and Sharpe (MRS) and YES agars.
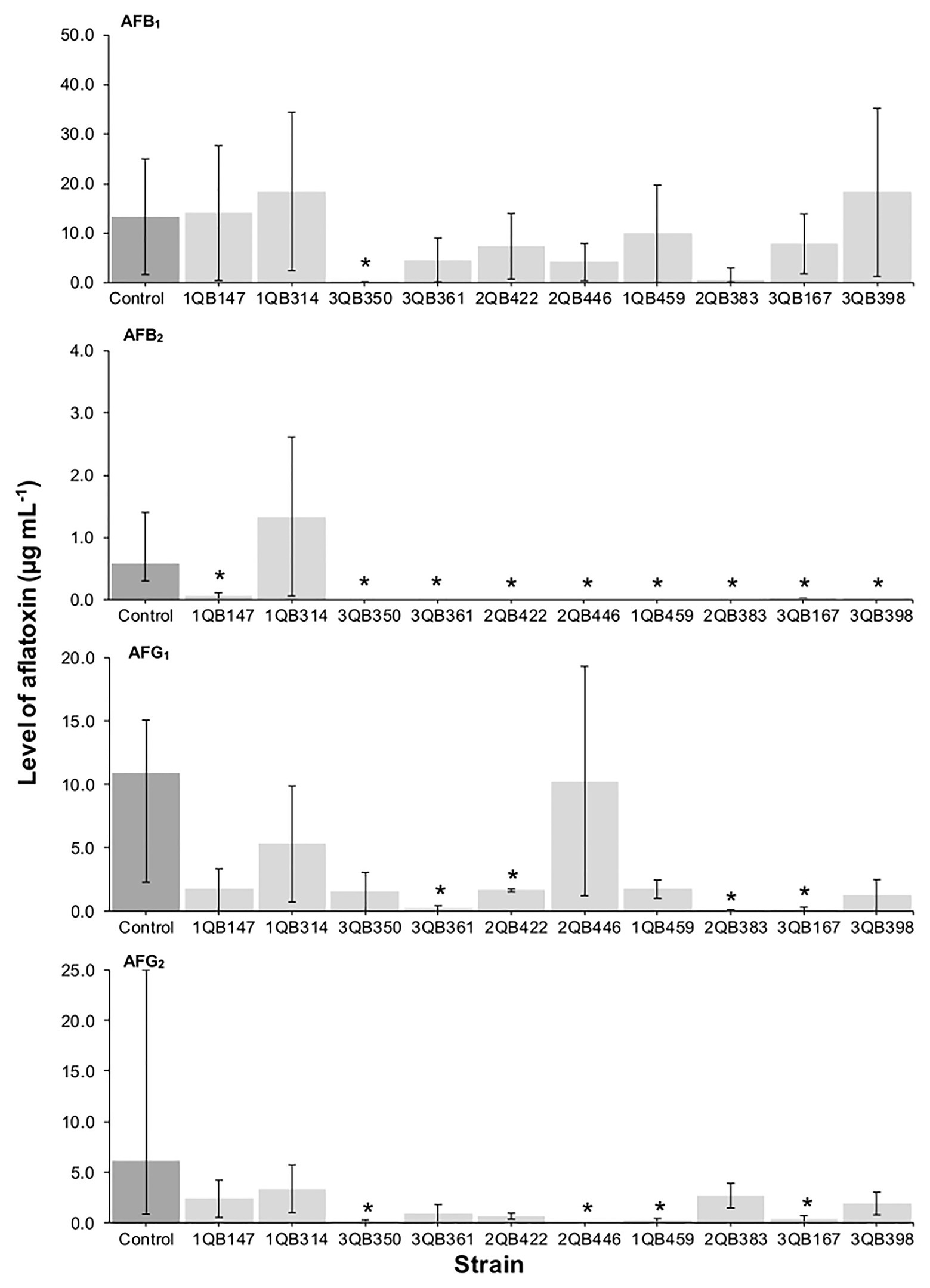
Figure 1. Effect of LAB on production of aflatoxins B1 (AFB1), B2 (AFB2), G1 (AFG1), and G2 (AFG2), by A. parasiticus NRRL 2999 in co-cultured YES broth after 7 days of incubation at 25°C. LAB was inoculated (5.0 log10 cfu ml−1) simultaneously with the fungus (Inoculation = T 0 h). The individually tested LAB included: four strains of Lactiplantibacillus plantarum (former Lactobacillus plantarum, comprising strains 1QB147, 1QB314, 3QB350, and QB361), two strains of Levilactobacillus brevis (former Lactobacillus brevis, comprising strains 2QB422 and 3QB446), and four strains of Levilactobacillus spp. (former Lactobacillus sp., comprising strains 1QB4593, 2QB383, 3QB167, and QB398). Results are expressed as median values of three independent experiments, with minimum and maximum values indicated as error bars. *Median values with asterisks differ significantly from controls (p < 0.05).
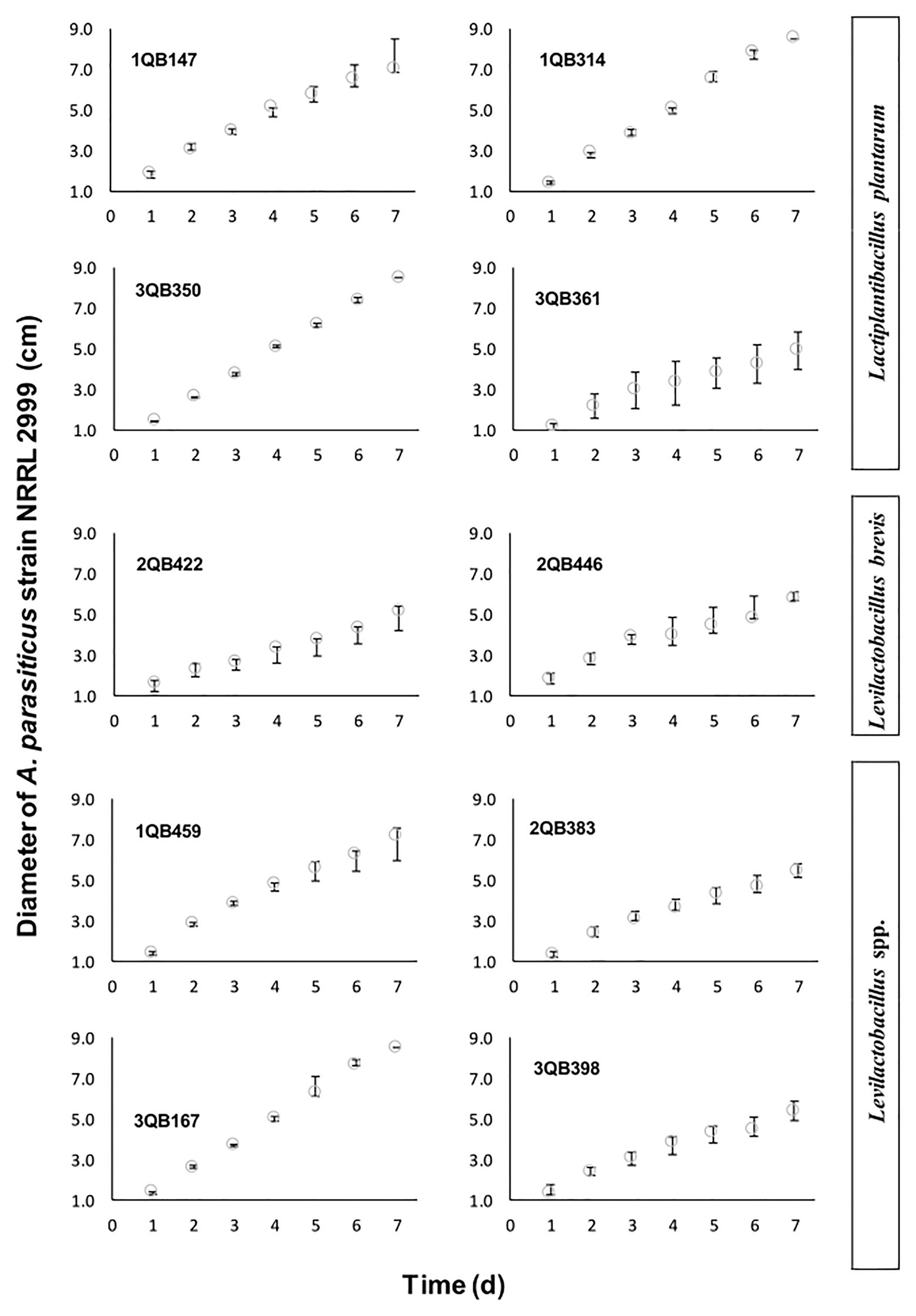
Figure 2. Diameter increase of A. parasiticus NRRL 2999 drop plated (inoculated at the level of 105 log10 cfu ml−1) on the center of the YES agar, and incubated for 7 days at 25°C. The YES agar was covering a layer of MRS agar pour plated with one of the tested LAB (5.0 log10 cfu ml−1), and included: four strains of Lactiplantibacillus plantarum (former Lactobacillus plantarum, comprising strains 1QB147, 1QB314, 3QB350, and QB361), two strains of Levilactobacillus brevis (former Lactobacillus brevis, comprising strains 2QB422 and 3QB446), and four strains of Levilactobacillus spp. (former Lactobacillus sp., comprising strains 1QB4593, 2QB383, 3QB167, and QB398). Results are expressed as median values of three independent experiments, with minimum and maximum values indicated as error bars.
Effect of LAB on the Production of Aflatoxins AFB1, AFB2, AFG1, and AFG2 by A. parasiticus
The effect of the LAB strains on the mycotoxin production by A. parasiticus was also investigated in YES broth over 7 days at 25°C. Aspergillus parasiticus strains are known to produce AFBs and AFGs, while another Aspergillus species commonly found in foodstuffs, Aspergillus flavus produce only AFBs (Oliveira et al., 2014). As shown in Figures 1, 3, 4, the moment of inoculation of the LAB strains on the development stage of the fungus, and thus on supressing mycotoxins production was critical. When inoculating the LAB strains at an earlier stage of development of the fungus, nearly all strains reduced the level of the four mycotoxins produced. Greatest inhibition of AFB1, AFB2, and AFG2 production was achieved with strain 3QB350 (L. plantarum), followed by 2QB383 (Levilactobacillus spp.) and 2QB446 (L. brevis). An impressive inhibition ability was shown by 2QB383 (Levilactobacillus spp.) toward AFB1 (max value of 2.97 μg ml−1, while max value of 25.00 μg ml−1 was produced by the control without LAB, Figures 1, 4) and AFB2 (not detected, Figures 1, 3, 4), and by strain 1QB459 (Levilactobacillus spp.) toward AFB2 (not detected, Figures 1, 3) and AFG2 (max values of 0.41 μg ml−1, while max value of 25.00 μg ml−1 was produced by the control without LAB, Figure 1). While nearly all strains were able to suppress the production of AFB2, only 3QB167 (Levilactobacillus spp.), 3QB361 (L. plantarum), and 2QB383 (Levilactobacillus spp.) were able to inhibit completely the formation of AFG1 (Figure 1). Lower effectiveness for strains, as well as larger variation on mycotoxin production was found when they were inoculated 24 h after incubation of the fungus (Figure 1). The varying effect of LAB on mycotoxin production was also reported by Fernández-Juri et al. (2011) when growing A. parasiticus NRRL 2999 together with different LAB strains at 25°C for 7 days. These authors reported that AFB1 in the control (fungus alone) reached 4.46 ± 0.29 μg g−1, but when grown with LAB, the level of AFB1 varied between 0.12 ± 0.04 and 7.72 ± 0.56 μg g−1, and the variation was dependent of the specific LAB strain. In our study, a similar large difference in the effect of LAB strains on mycotoxin production was found. Mycotoxin production by A. parasiticus NRRL 2999 alone (control) was found to vary greatly (dark columns in Figures 1, 3, 4), with median values of 13.31 (AFB1), 0.58 (AFB2), 19.91 (AFG1), and 6.12 μg ml (AFG2), but with minimum and maximum values deviating more than 38.1% of the median values (error bars in Figures 1, 3, 4). A 30% variation on mycotoxins production as obtained in our study is considered acceptable due to the intrinsic properties of the fungus (EC, 2002, 2006). This variability is strain-specific and occurs even when the strain is subjected to similar experimental conditions such as performed here (Whiting and Golden, 2002; Freire et al., 2018). Also in agreement with our results, several reports indicate that, once the mycotoxins have reached a maximum production, a decline of its levels occurs, possibly because of the ability of the fungus to degrade or convert the mycotoxins into other metabolites (Freire et al., 2018; Kowalczyk et al., 2019). Furthermore, Greeff-Laubscher et al. (2020) showed that the level of mycotoxin production by a fungus can vary largely within the replicates of a trial as well as length of time of incubation of the fungus, with episodes of levels increasing and decreasing over the whole period. This could explain the increased level of AFs vs. the control for some strains such as 3QB361 (Figure 3) and 2QB446 for AFG1 (Figure 4). The largest variation of mycotoxin production was shown in the present study when the LAB strains were inoculated 48 h after the inoculation of the fungus (Figure 4). The number of LAB strains able to inhibit mycotoxin production was lower when the inoculation of the strains was performed after 24 h of the fungus inoculation (Figure 3). The antifungal activity of LAB varies widely according to the species, the incubation conditions (pH, time, and temperature), and the substrate/mycotoxin produced. Generally, the maximum production of anti-fungal compounds occurs at the beginning of the stationary growth phase and decreases over incubation time (Dalié et al., 2010; Gomaa et al., 2018). In a study performed by Sangmanee and Hongpattarakere (2014), L. plantarum k35 reached its maximum fungicidal activity at 37°C. This activity was reduced when the strain was incubated at 25°C (temperature at which grains and cereals are generally stored). Strains of L. brevis and L. plantarum appear to be best aflatoxin inhibitors. Gomaa et al. (2018) investigated a L. brevis strain capable of reducing the production of AFB1 by 90.4%, and of decreasing the amount of mycelial biomass. In addition to the antifungal effect, bacteria can modify mycotoxins through their enzymatic activity and defense mechanisms and therefore, apparent reduction in the level of mycotoxins is evident. However, the mechanisms of this biotransformation have not yet been elucidated (Freire and Sant’Ana, 2018).
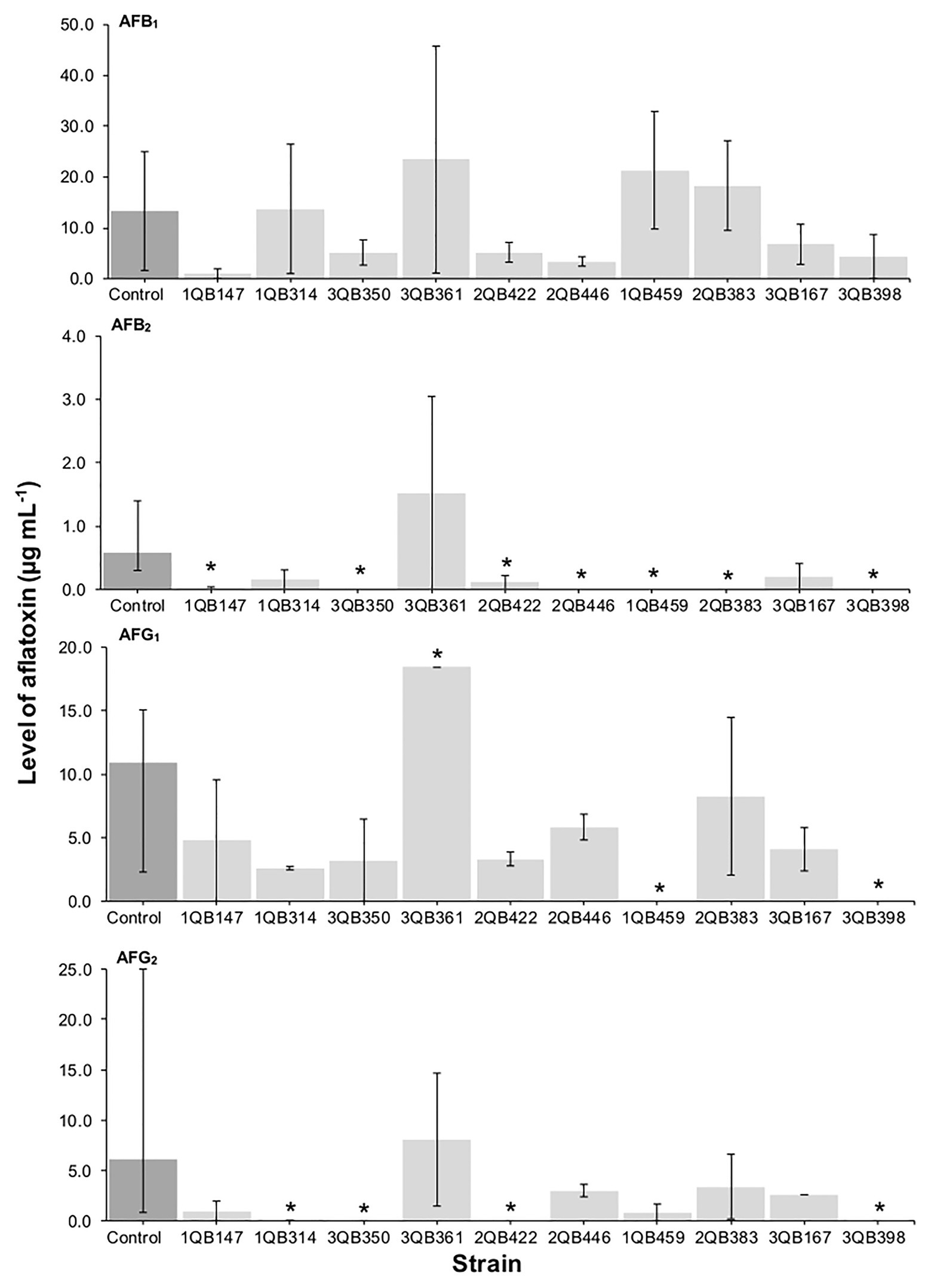
Figure 3. Effect of LAB on production of AFB1, AFB2, AFG1, and AFG2 by A. parasiticus NRRL 2999 in co-cultured YES broth after 7 days of incubation at 25°C. LAB was inoculated (5 log10 cfu ml−1) after 24 h (Inoculation = T 24 h) incubation of the fungus. The individually tested LAB included: four strains of Lactiplantibacillus plantarum (former Lactobacillus plantarum, comprising strains 1QB147, 1QB314, 3QB350, and QB361), two strains of Levilactobacillus brevis (former Lactobacillus brevis, comprising strains 2QB422 and 3QB446), and four strains of Levilactobacillus spp. (former Lactobacillus sp., comprising strains 1QB4593, 2QB383, 3QB167, and QB398). Results are expressed as median values of three independent experiments, with minimum and maximum values indicated as error bars. *Median values with asterisks differ significantly from controls (p < 0.05).
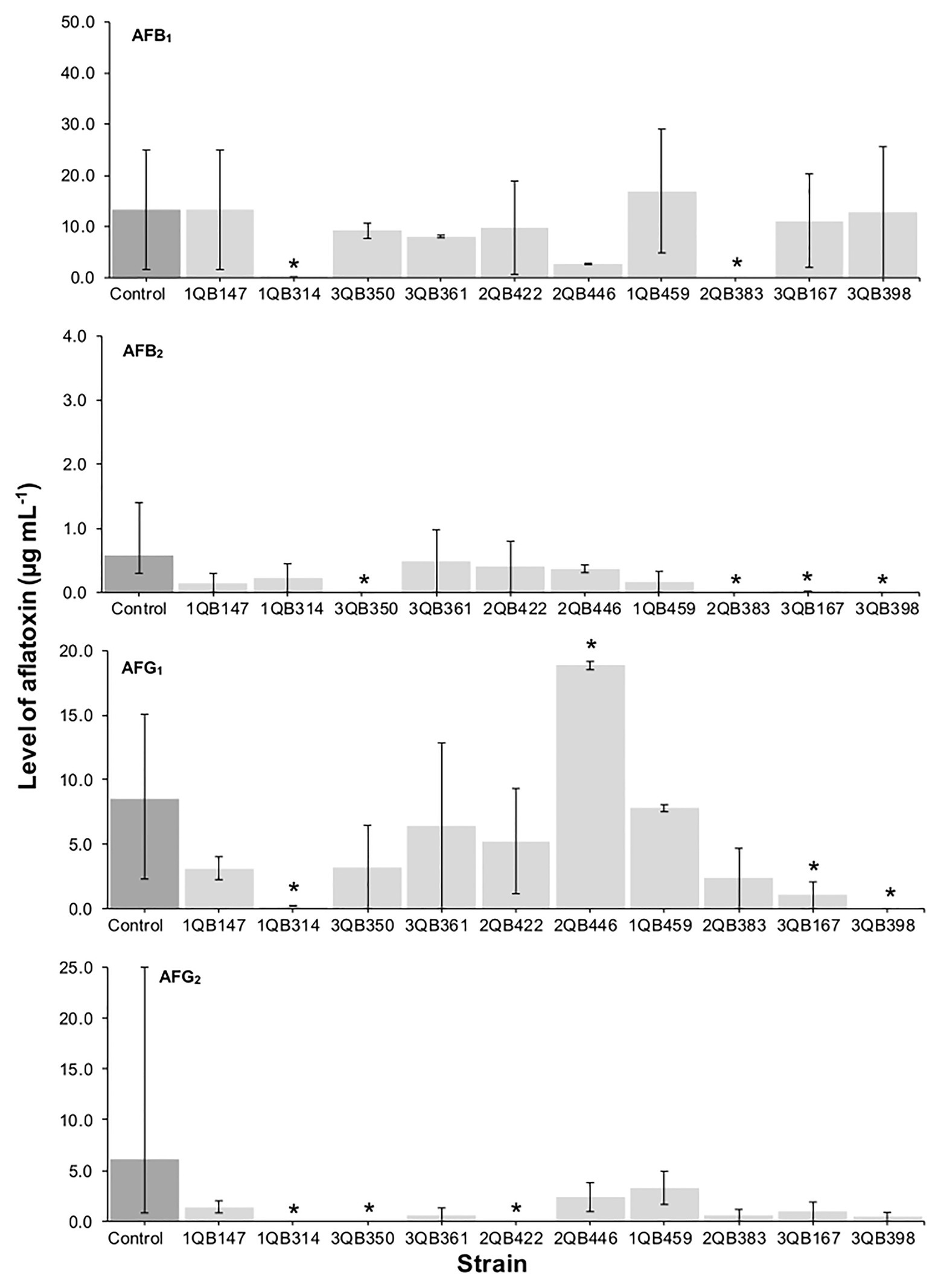
Figure 4. Effect of LAB on production of AFB1, AFB2, AFG1, and AFG2 by A. parasiticus NRRL 2999 in co-cultured YES broth after 7 days of incubation at 25°C. LAB was inoculated (5 log10 cfu ml−1) after 48 h (Inoculation = T 48 h) incubation of the fungus. The individually tested LAB included: four strains of Lactiplantibacillus plantarum (former Lactobacillus plantarum, comprising strains 1QB147, 1QB314, 3QB350, and QB361), two strains of Levilactobacillus brevis (former Lactobacillus brevis, comprising strains 2QB422 and 3QB446), and four strains of Levilactobacillus spp. (former Lactobacillus sp., comprising strains 1QB4593, 2QB383, 3QB167, and QB398). Results are expressed as median values of three independent experiments, with minimum and maximum values indicated as error bars. *Median values with asterisks differ significantly from controls (p < 0.05).
A positive correlation was found between the inhibition of the fungus growth and the inhibition of mycotoxin production (Table 3; Figures 1, 3, 4). An overview of the combined effect of the LAB strains on fungus growth and reduction of aflatoxin production by A. parasiticus is shown in Table 4. LAB strains affecting the growth of the fungus, such as strains 2QB422 (L. brevis), 2QB383 (Levilactobacillus spp), 2QB446 (L. brevis), and 3QB398 (Levilactobacillus spp.), were also able to inhibit by 50% the production of at least three of the tested aflatoxins. This correlation between inhibition of growth and suppression ability of mycotoxin production by aspergilli was also reported by Ben Taheur et al. (2019b), with a L. kefiri strain. Surprisingly, despite no clear inhibition effect of the LAB strains on fungus growth, strong inhibition potential (>50%) on all four aflatoxins was shown by strain 3QB350 (L. plantarum), and on at least three of the tested aflatoxins by strains 1QB314 (L. plantarum) and 3QB167 (Levilactobacillus spp.), at some point of the fungus life cycle (when LAB was inoculated simultaneously with the fungus in Figure 1, when it was inoculated at 24 and 48 h after the fungus was incubated in Figures 3, 4, respectively). This is of particular importance for selection of the best time-point of application of the LAB to obtain the optimum effect and minimize mycotoxin formation in a food matrix. When linked to plant-based foods, the efficiency of strains at earlier stages of the fungus development could be an indication that application on the seeds (instead any other stage of plant development) would be appropriate. Strains more robust and able to affect mycotoxin formation in the later stages of the fungus development, could be an alternative to the application when the fungus is already established in the plant. A similar inhibitory effect of actinomycetes isolates on aflatoxins B1 and B2, but with limited impact on growth of A. flavus has been reported (Verheecke et al., 2014). Effect of pomegranate peel extract on AFB1 production, but without interfering on fungus growth, was shown by Sadhasivam et al. (2019) when investigating the growth and mycotoxin-producing ability of A. flavus. This indicates the relevance of applying selected LAB strains as biological control agents in order to prevent aflatoxin formation. Understanding the mechanisms of this inhibitory effect could be used to optimize the conditions for application purpose.
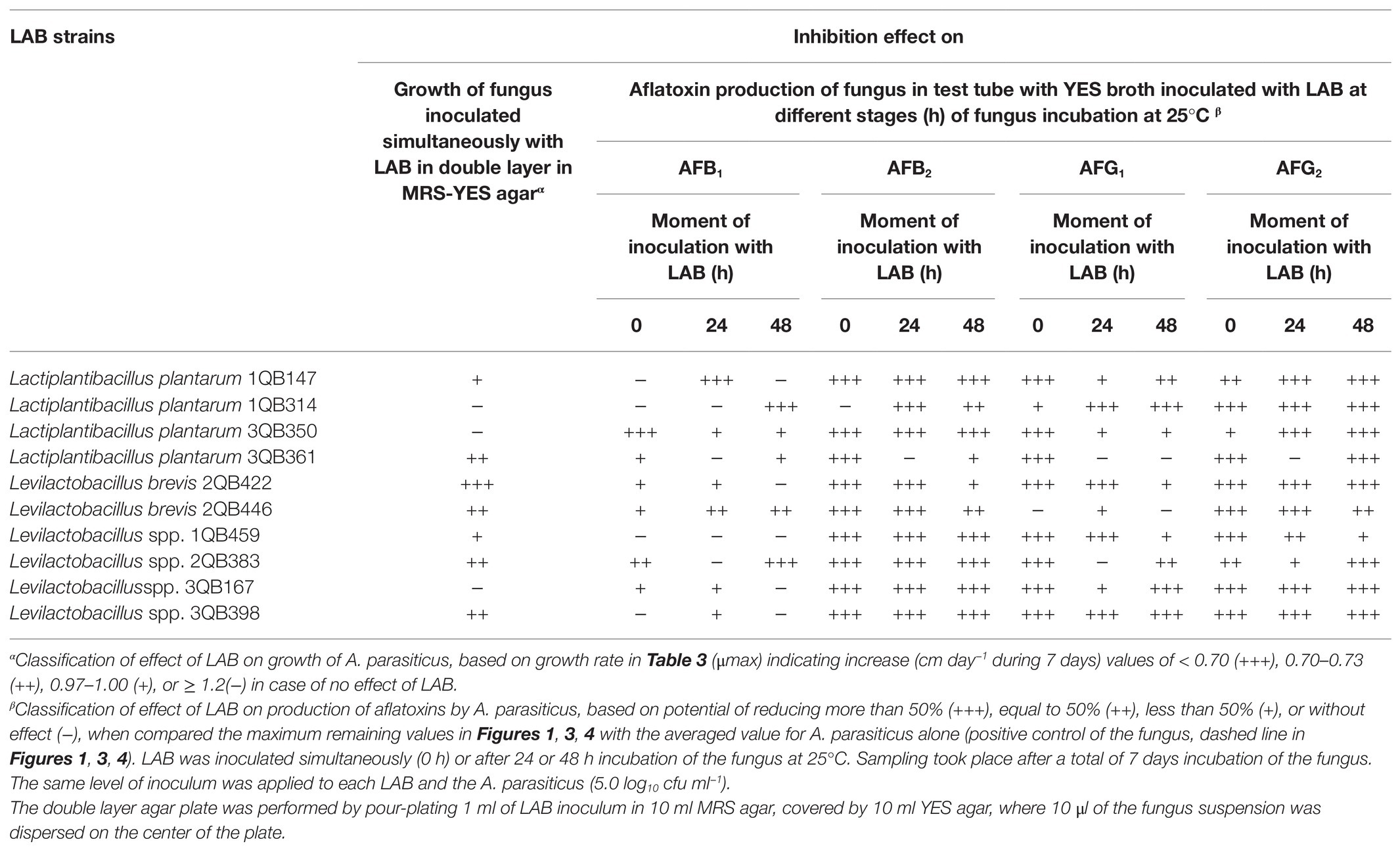
Table 4. Overview of effect of LAB on inhibition of mycotoxigenic A. parasiticus NRRL 2999 growth and aflatoxins (AF) production (AFB1, AFB2, AFG1, and AFG2), investigated in double layer agar plates with MRS and YES agars and in YES broth, respectively.
Effect of LAB on Reduction of Aflatoxin M1 in Spiked Milk
After treatment with the heat-inactivated LAB cells, the remaining levels of mycotoxins in spiked milk samples (Figure 5) indicated that a small reduction (about 18–25%) was achieved with strains 3QB361 (L. plantarum), 1QB147 (L. plantarum), 2QB293 (Levilactobacillus spp.), 3QB350 (L. plantarum), and 2QB446 (L. brevis). Greater reductions of 33 and 45% were achieved by treatment with strains 3QB398 (Levilactobacillus spp.) and 2QB422 (L. brevis), respectively. Our results were similar to those of Bovo et al. (2013), who under similar conditions, reported reductions ranging from 12.42 to 45.67% for inactivated cells and between 5.60 and 33.54% for viable cells. In a study performed by Hashemi and Gholamhosseinpour (2019), all strains of Levilactobacillus spp. reduced the amount of AFM1 by 26–52% in fermented cream. Mycotoxin binding with LAB cells is essentially a surface phenomenon, which involves non-covalent bonds between the cell wall components and the mycotoxin dissolved in the liquid medium (Haskard et al., 2001). Kuharić et al. (2018) selected, isolated, and identified 10 LAB species from raw milk with the objective to select a strain to efficiently bind AFM1. In addition, they observed a binding efficiency ranging from 21 to 92% for viable cells, and from 26 to 95% for thermally treated cells. Similar to our results, Corassin et al. (2013) observed that a pool of heat-killed cells of LAB strains (Lb. rhamnosus, Lb. delbrueckii spp. bulgaricus, and Bifidobacterium lactis) bound 11.5–11.7% of AFM1 in ultra-high temperature skim milk after 30–60 min. The binding percentages of heat-killed cells of Lb. bulgaricus, Lb. rhamnosus, and B. lactis to AFM1 in UHT skimmed milk were 13.5, 19.7, and 37.7%, respectively (Bovo et al., 2013), which are in range of the values described in the present work.
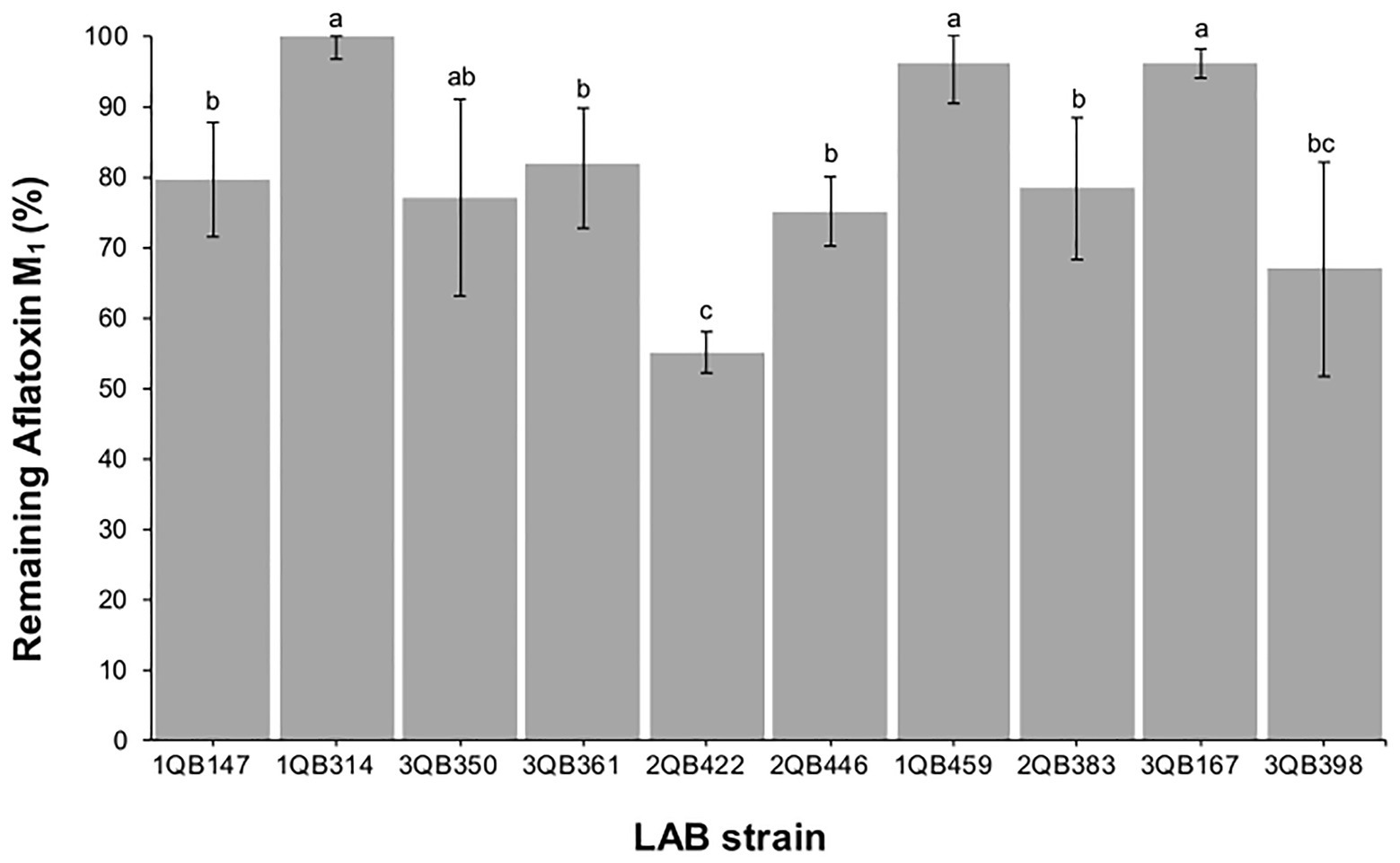
Figure 5. Remaining aflatoxin M1 in skimmed milk samples spiked at 0.5 μg ml−1, after 15 min contact time with heat-inactivated cells of individually tested LAB including: four strains of Lactiplantibacillus plantarum (former Lactobacillus plantarum, comprising strains 1QB147, 1QB314, 3QB350, and QB361), two strains of Levilactobacillus brevis (former Lactobacillus brevis, comprising strains 2QB422 and 3QB446), and four strains of Levilactobacillus spp. (former Lactobacillus sp., comprising strains 1QB4593, 2QB383, 3QB167, and QB398). Results (% in relation to controls) are expressed as mean values of three independent experiments, with SDs indicated as error bars. Bars with no common letters differ significantly (p < 0.05).
Effect of LAB on Reduction of AFB1, OTA, and ZEN in Buffer
When testing the effect of LAB strains on the reduction of AFB1, OTA, and ZEN in spiked buffer, the effect of time of exposure with the inactivated LAB cells was important for the robustness of the reduction. The mycotoxin decline was more robust with less variation when the spiked buffer was treated for 15 min (Figure 6), in comparison to a treatment for 5 min (Figure 7). The incubation period was chosen based on the experiments described by Bovo et al. (2013), who showed that the viable and heat-killed cells of LAB have the same ability to bind to AFM1 in 15 min and 24 h of contact. In addition, a recent review by Muaz et al. (2021) indicated that the process of decontamination is a rapid phenomenon during which the binding occurs within the first few minutes, so a longer incubation time may not result in any significant difference among decontamination levels. In addition to the effect of time of exposure, the reduction of the mycotoxins was greatest at pH 6.5 (D, E, and F in Figures 6, 7). The LAB strain 1QB147 (L. plantarum) was the most effective in reducing the level of AFB1 (Figures 6, 7), independent of the pH, and duration of treatment. LAB strain 3QB361 (L. plantarum) was the most effective strain in reducing the levels of OTA (Figures 6, 7) at pH 3.0 (independent of the duration of treatment). LAB strains 3QB398 (Levilactobacillus spp.) and 1QB459 (Levilactobacillus spp.) resulted in a greater decline of OTA at pH 6.5 when treated for 5 (Figure 7) and 15 min (Figure 6), respectively. Regardless of the pH and duration of treatment, strain 3QB361 (L. plantarum) had the highest impact on reducing ZEN (C and F in Figures 6, 7), followed by strains 1QB147 (L. plantarum) and 1QB459 (Levilactobacillus spp.). The high mycotoxin binding efficiencies of some LAB strains evaluated in the present study highlight their potential use as additives for reducing the bioavailability of mycotoxins in foods. In this context, LAB have been tested for reducing mycotoxins in foods, such as artificially contaminated almonds and peanuts, with L. kefiri FR7 able to decrease by 85.3% the level of AFB1 and by 83.9% the level of AFB2 in almonds, in addition to reducing by 25% OTA in peanuts (Ben Taheur et al., 2019b). Ben Taheur et al. (2017) demonstrated a high mycotoxin binding capacity of Kefir-originated strains of L. kefiri, Kazachstania servazziia, and Acetobacter syzygii, with L. kefiri KFLM3 reducing approximately 82% of ABF1, 94% of OTA, and 100% of ZEN in milk. In addition, another study demonstrated that strains of L. plantarum in PPB and MRS medium were efficient in decreasing ZEN after 48 h of incubation (Zhao et al., 2015). ZEN reduction of up to 30% through adsorption was observed with L. lactis (Rogowska et al., 2019). Thus, taking together, our findings and the previous reports indicate that LAB may be a potential tool for reducing mycotoxins in foods.
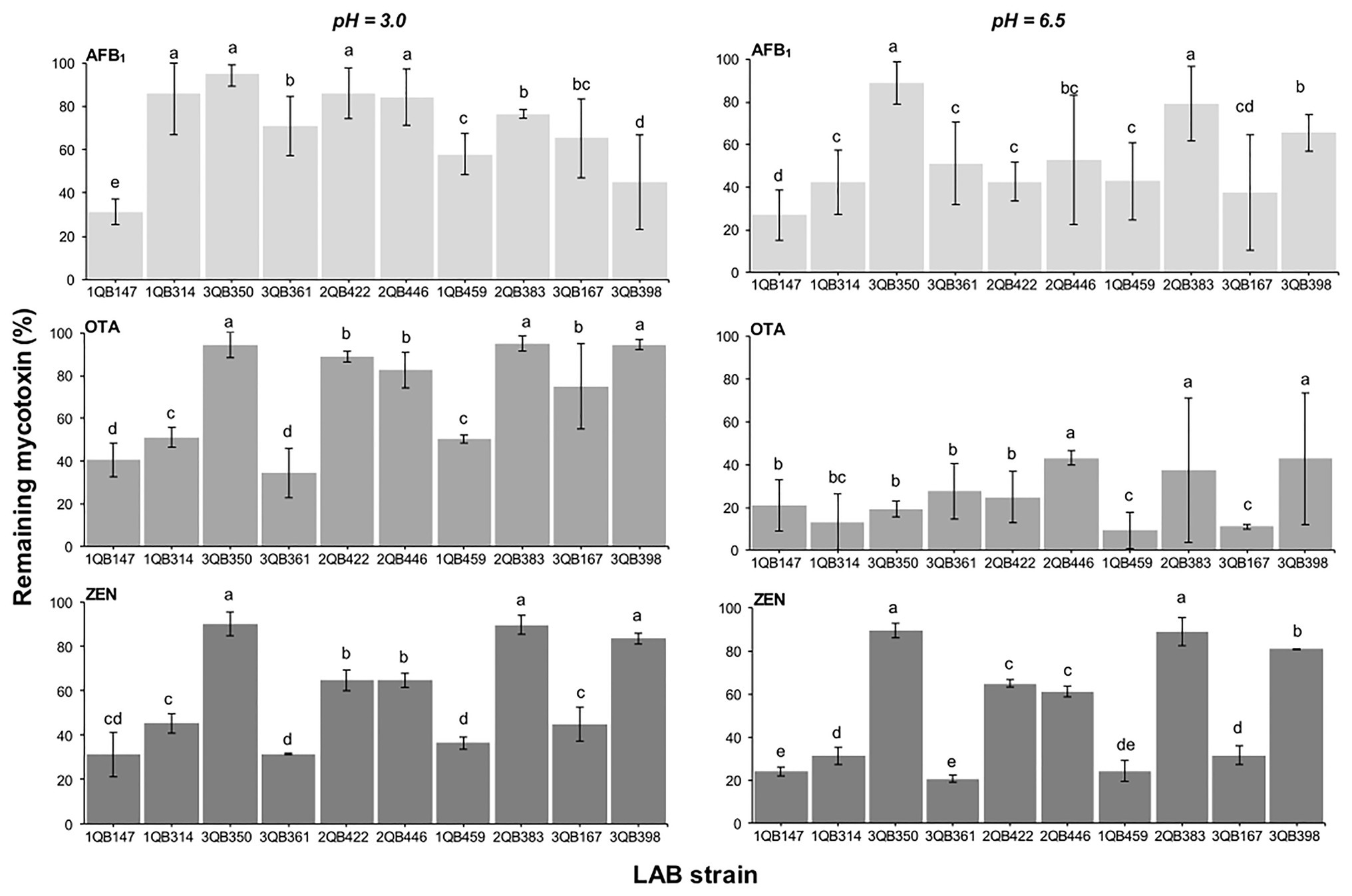
Figure 6. Remaining AFB1, OTA, and ZEN in PPB at pH 3.0 and 6.5, after 15 min contact time with heat-inactivated LAB strains including: four strains of Lactiplantibacillus plantarum (former Lactobacillus plantarum, comprising strains 1QB147, 1QB314, 3QB350, and QB361), two strains of Levilactobacillus brevis (former Lactobacillus brevis, comprising strains 2QB422 and 3QB446), and four strains of Levilactobacillus spp. (former Lactobacillus sp., comprising strains 1QB4593, 2QB383, 3QB167, and QB398). Initial concentrations of mycotoxins in PPB were 1.0 ng ml−1 (AFB1, OTA) and 2.0 μg ml−1 (ZEN). Results (% in relation to controls) are expressed as mean values of three independent experiments, with SDs indicated as error bars. Bars with no common letters differ significantly (p < 0.05).
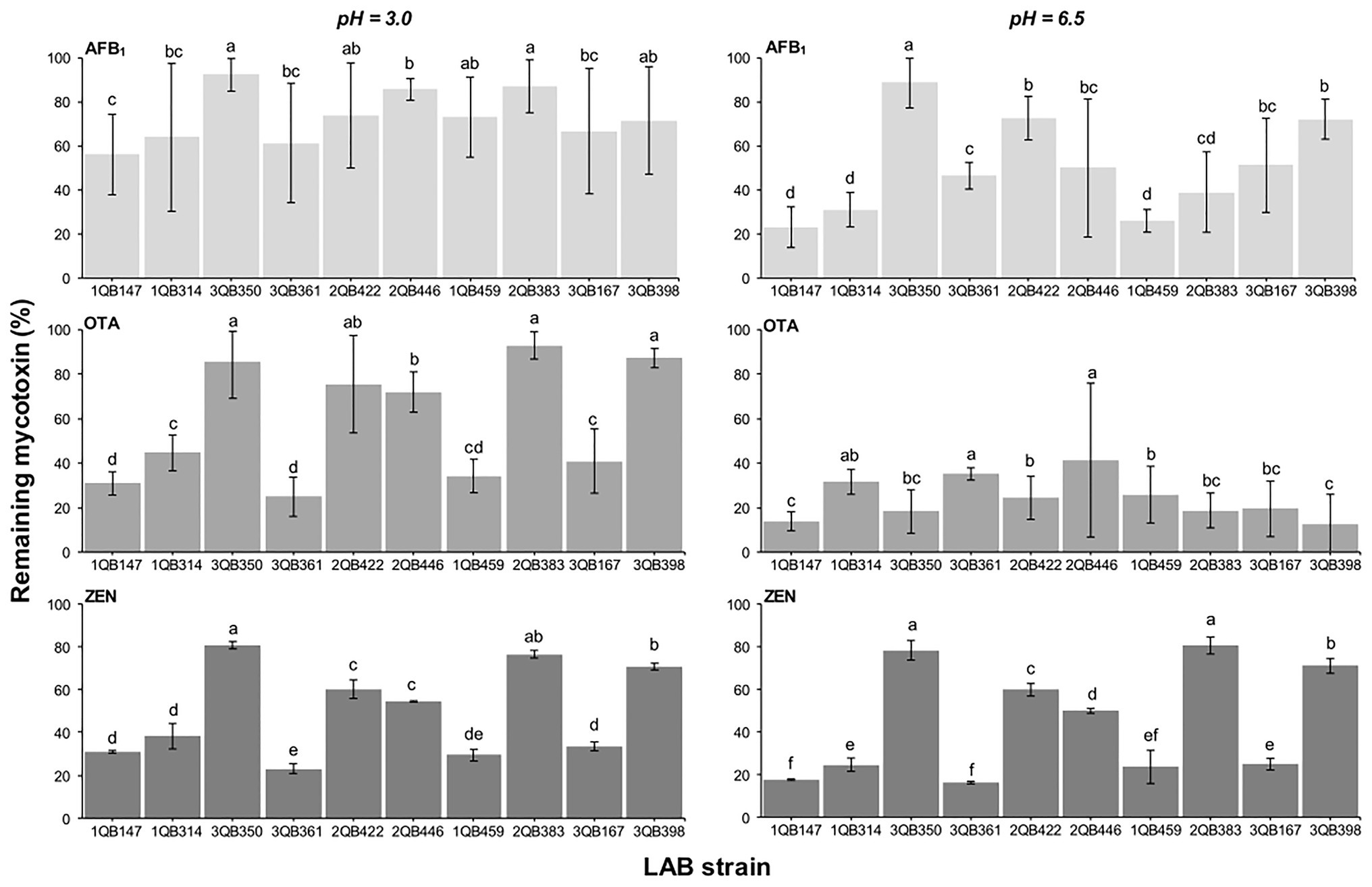
Figure 7. Remaining AFB1, OTA, and ZEN in PPB at pH 3.0 and 6.5, after 5 min contact time with heat-inactivated LAB strains including: four strains of Lactiplantibacillus plantarum (former Lactobacillus plantarum, comprising strains 1QB147, 1QB314, 3QB350, and QB361), two strains of Levilactobacillus brevis (former Lactobacillus brevis, comprising strains 2QB422 and 3QB446), and four strains of Levilactobacillus spp. (former Lactobacillus sp., comprising strains 1QB4593, 2QB383, 3QB167, and QB398). Initial concentrations of mycotoxins in PPB were 1.0 μg ml−1 (AFB1, OTA) and 2.0 μg ml−1 (ZEN). Results (% in relation to controls) are expressed as mean values of three independent experiments, with SDs indicated as error bars. Bars with no common letters differ significantly (p < 0.05).
The removal of mycotoxins by LAB usually occurs by adsorption (Bueno et al., 2007), in which mycotoxin molecules bind reversibly to the structures present in the bacterial cell wall (peptidoglycans, carbohydrates, polysaccharides, and teichoic and lipoteichoic acids) through ion exchange, complexation, and hydrophobic iterations (Dalié et al., 2010; Ben Taheur et al., 2017; Afshar et al., 2020). Because it is a physical phenomenon, most chemical and physical treatments (e.g., application of heat) intended to kill cells do not affect this property and, in some cases, even increase its performance, due to the exposure of the binding sites (Dalié et al., 2010). Another factor that can contribute to the reduction of aflatoxins by adsorption is the production of exopolysaccharides (Ben Taheur et al., 2017), a characteristic present in three of the four LAB mentioned above (Levilactobacillus spp. 1QB459, L. plantarum 3QB361, and Levilactobacillus spp. 3QB398; see supplementary material in Margalho et al., 2020).
Conclusion
From the 10 LAB strains tested in the present study, all had an effect on mycotoxins. Some strains suppressed the growth and/or aflatoxins (AFB1, AFB2, AFG1, and AFG2) production ability of A. parasiticus, to different degrees. Some strains were able to bind with mycotoxins, such as AFB1, OTA, and ZEN, at different levels of efficiency, which was influenced by the applied conditions (pH and time of treatment), as well as the mycotoxin and the matrix used (milk or buffer). The findings of the present study are very relevant, especially considering the critical toxic effect of mycotoxins as well as the increasing mycotoxin occurrence worldwide. The purpose of screening for LAB with the ability to minimize mycotoxins in milk and in vitro models was demonstrated. Despite challenging and time consuming, applicability and efficiency of these LAB in different food matrices are worthy of investigation. It is important to consider that the LAB strains tested in the present study were isolated from cheese, and they have the ability to control aflatoxins in foodstuff such as milk, and this may be considered as an asset for the development of integrated management systems for aflatoxin reduction in cheese production. The results clearly indicate the promising potential of LAB in reducing the risk related to mycotoxins. This potential could even be explored further for the safer production of plant-based foods.
Data Availability Statement
The original contributions presented in the study are included in the article/supplementary material; further inquiries can be directed to the corresponding author.
Author Contributions
CM designed the experimental work as well as acquired and analyzed the data, and wrote and reviewed the manuscript. LF contributed by acquiring and modeling the data from the growth experiments, and reviewing the manuscript. RR acquired the data using LC-LC/MS. LM, CB, and LTF acquired the data and reviewed the manuscript. AS, FR, and CC supervised the work in their respective labs, and reviewed the manuscript. CO conceptualized the work, supervised the project, interpreted the data, and reviewed the manuscript. All authors contributed to the article and approved the submitted version.
Funding
This research was supported by the Danish Ministry of Higher Education and Science (grant number 116004), Conselho Nacional de Desenvolvimento Científico e Tecnológico – CNPq (grant numbers 302763/2014-7, 305804/2017-0, and 306304/2017-1), Coordenação de Aperfeiçoamento de Pessoal de Nível Superior – CAPES (grant numbers code 001), and Fundação de Amparo à Pesquisa do Estado de São Paulo – FAPESP (Grants 2015/25641-4, 2016/21041-5, 2017/20081-6, 2017/03899-5, and 2018/24540-8).
Conflict of Interest
The authors declare that the research was conducted in the absence of any commercial or financial relationships that could be construed as a potential conflict of interest.
References
Abbaszadeh, S., Tavakoli, R., Sharifzadeh, A., and Shokri, H. (2015). Lactic acid bacteria as functional probiotic isolates for inhibiting the growth of Aspergillus flavus, A. parasiticus, A. niger and Penicillium chrysogenum. J. Mycol. Med. 25, 263–267. doi: 10.1016/j.mycmed.2015.10.011
Abrunhosa, L., Inês, A., Rodrigues, A. I., Guimarães, A., Pereira, V. L., Parpot, P., et al. (2014). Biodegradation of ochratoxin A by Pediococcus parvulus isolated from Douro wines. Int. J. Food Microbiol. 188, 45–52. doi: 10.1016/j.ijfoodmicro.2014.07.019
Addinsoft (2021). XLSTAT Statistical and Data Analysis Solution. New York, USA. Available at: https://www.xlstat.com (Accessed January 05, 2021).
Afshar, P., Shokrzadeh, M., Raeisi, S. N., Ghorbani-HasanSaraei, A., and Nasiraii, L. R. (2020). Aflatoxins biodetoxification strategies based on probiotic bacteria. Toxicon 178, 50–58. doi: 10.1016/j.toxicon.2020.02.007
Agriopoulou, S., Stamatelopoulou, E., and Varzakas, T. (2020). Advances in occurrence, importance, and mycotoxin control strategies: prevention and detoxification in foods. Foods 9:137. doi: 10.3390/foods9020137
Aunsbjerg, S. D., Honoré, A. H., Marcussen, J., Ebrahimi, P., Vogensen, F. K., Benfeldt, C., et al. (2015). Contribution of volatiles to the antifungal effect of Lactobacillus paracasei in defined medium and yogurt. Int. J. Food Microbiol. 194, 46–53. doi: 10.1016/j.ijfoodmicro.2014.11.007
Avantaggiato, G., Greco, D., Damascelli, A., Solfrizzo, M., and Visconti, A. (2014). Assessment of multi-mycotoxin adsorption efficacy of grape pomace. J. Agric. Food Chem. 62, 497–507. doi: 10.1021/jf404179h
Baazeem, A., Garcia-Cela, E., Medina, A., and Magan, N. (2021). Interacting abiotic factors affect growth and aflatoxin B1 production profiles of Aspergillus flavus strains on pistachio-based matrices and pistachio nuts. Front. Microbiol. 11:624007. doi: 10.3389/fmicb.2020.624007
Baranyi, J., Roberts, T. A., and McClure, P. (1993). A non-autonomous differential equation to model bacterial growth. Food Microbiol. 10, 43–59. doi: 10.1006/fmic.1993.1005
Barnes, J. M. (1970). Aflatoxin as a health hazard. J. Appl. Bacteriol. 33, 285–298. doi: 10.1111/j.1365-2672.1970.tb02200.x
Ben Said, L., Gaudreau, H., Dallaire, L., Tessier, M., and Fliss, I. (2019). Bioprotective culture: a new generation of food additives for the preservation of food quality and safety. Ind. Biotechnol. 15, 138–147. doi: 10.1089/ind.2019.29175.lbs
Ben Taheur, F., Fedhila, K., Chaieb, K., Kouidhi, B., Bakhrouf, A., and Abrunhosa, L. (2017). Adsorption of aflatoxin B1, zearalenone and ochratoxin A by microorganisms isolated from Kefir grains. Int. J. Food Microbiol. 251, 1–7. doi: 10.1016/j.ijfoodmicro.2017.03.021
Ben Taheur, F., Kouidhi, B., Al Qurashi, Y. M. A., Ben Salah-Abbès, J., and Chaieb, K. (2019a). Review: biotechnology of mycotoxins detoxification using microorganisms and enzymes. Toxicon 160, 12–22. doi: 10.1016/j.toxicon.2019.02.001
Ben Taheur, F., Mansour, C., Kouidhi, B., and Chaieb, K. (2019b). Use of lactic acid bacteria for the inhibition of Aspergillus flavus and Aspergillus carbonarius growth and mycotoxin production. Toxicon 166, 15–23. doi: 10.1016/j.toxicon.2019.05.004
Bouillon, G. A., Gåserød, O., and Rattray, F. P. (2019). Evaluation of the inhibitory effect of alginate oligosaccharide on yeast and mould in yoghurt. Int. Dairy J. 99:104554. doi: 10.1016/j.idairyj.2019.104554
Bovo, F., Corassin, C. H., Rosim, R. E., and de Oliveira, C. A. F. (2013). Efficiency of lactic acid bacteria strains for decontamination of aflatoxin M1 in phosphate buffer saline solution and in skimmed milk. Food Bioprocess Technol. 6, 2230–2234. doi: 10.1007/s11947-011-0770-9
Bueno, D. J., Casale, C. H., Pizzolitto, R. P., Salvano, M. A., and Oliver, G. (2007). Physical adsorption of aflatoxin B1 by lactic acid bacteria and Saccharomyces cerevisiae: a theoretical model. J. Food Prot. 70, 2148–2154. doi: 10.4315/0362-028X-70.9.2148
Bullerman, L. B., and Bianchini, A. (2007). Stability of mycotoxins during food processing. Int. J. Food Microbiol. 119, 140–146. doi: 10.1016/j.ijfoodmicro.2007.07.035
Campagnollo, F. B., Franco, L. T., Rottinghaus, G. E., Kobashigawa, E., Ledoux, D. R., Dakovic, A., et al. (2015). In vitro evaluation of the ability of beer fermentation residue containing Saccharomyces cerevisiae to bind mycotoxins. Food Res. Int. 77, 643–648. doi: 10.1016/j.foodres.2015.08.032
Campagnollo, F. B., Mousavi Khaneghah, A., Borges, L. L., Bonato, M. A., Fakhri, Y., Barbalho, C. B., et al. (2020). In vitro and in vivo capacity of yeast-based products to bind to aflatoxins B1 and M1 in media and foodstuffs: a systematic review and meta-analysis. Food Res. Int. 137:109505. doi: 10.1016/j.foodres.2020.109505
Ciegler, A., Lillehoj, E. B., Peterson, R. E., and Hall, H. H. (1966). Microbial detoxification of aflatoxin. Appl. Microbiol. 14, 934–939.
Cinar, A., and Onbaşı, E. (2019). “Mycotoxins: the Hidden Danger in Foods,” in Mycotoxins and Food Safety. ed. S. Sabuncuoglu (London, UK: Intechopen).
Conteçotto, A. C. T., Pante, G. C., Castro, J. C., Souza, A. A., Lini, R. S., Romoli, J. C. Z., et al. (2021). Occurrence, exposure evaluation and risk assessment in child population for aflatoxin M1 in dairy products in Brazil. Food Chem. Toxicol. 148:111913. doi: 10.1016/j.fct.2020.111913
Corassin, C. H., Bovo, F., Rosim, R. E., and Oliveira, C. A. F. (2013). Efficiency of Saccharomyces cerevisiae and lactic acid bacteria strains to bind aflatoxin M1 in UHT skim milk. Food Control 31, 80–83. doi: 10.1016/j.foodcont.2012.09.033
Cuevas-González, P. F., González-Córdova, A. F., Vallejo-Cordoba, B., Aguilar-Toalá, J. E., Hall, F. G., Urbizo-Reyes, U. C., et al. (2020). Protective role of lactic acid bacteria and yeasts as dietary carcinogen-binding agents–a review. Crit. Rev. Food Sci. Nutr. doi: 10.1080/10408398.2020.1813685 [Epub ahead of print]
Dalié, D. K. D., Deschamps, A. M., and Richard-Forget, F. (2010). Lactic acid bacteria—potential for control of mould growth and mycotoxins: a review. Food Control 21, 370–380. doi: 10.1016/j.foodcont.2009.07.011
Dantigny, P., Guilmart, A., and Bensoussan, M. (2005). Basis of predictive mycology. Int. J. Food Microbiol. 100, 187–196. doi: 10.1016/j.ijfoodmicro.2004.10.013
de Souza de Azevedo, P. O., Mendonça, C. M. N., Moreno, A. C. R., Bueno, A. V. I., de Almeida, S. R. Y., Seibert, L., et al. (2020). Antibacterial and antifungal activity of crude and freeze-dried bacteriocin-like inhibitory substance produced by Pediococcus pentosaceus. Sci. Rep. 10:12291. doi: 10.1038/s41598-020-68922-2
EC (2002). 96/23/EC COMMISSION DECISION of 12 August 2002 implementing Council Directive 96/23/EC concerning the performance of analytical methods and the interpretation of results (notified under document number C(2002) 3044)(Text withEEA relevance; 2002/657/EC). 96/23/Ec Comm. Decis. 29. Available at: https://op.europa.eu/en/publication-detail/-/publication/ed928116-a955-4a84-b10a-cf7a82bad858/language-en (Accessed January 05, 2021).
EC (2006). Commission Regulation (EC) No 401/2006 of 23 February 2006 laying down the methods of sampling and analysis for the official control of the levels of mycotoxins in foodstuffs. Official Journal of the European Union 70, 12–34. Available at: http://eur-lex.europa.eu/legal-content/EN/TXT/PDF/?uri=CELEX:32006R0401&qid=1490946469496&from=NL (Accessed January 05, 2021).
EFSA Panel on Biological Hazards (BIOHAZ), Ricci, A., Allende, A., Bolton, D., Chemaly, M., Davies, R., et al. (2017). Update of the list of QPS-recommended biological agents intentionally added to food or feed as notified to EFSA 5: suitability of taxonomic units notified to EFSA until September 2016. EFSA J. 15:e04663. doi: 10.2903/j.efsa.2017.4663
Egbuta, M. A., Mwanza, M., and Babalola, O. O. (2017). Health risks associated with exposure to filamentous fungi. Int. J. Environ. Res. Public Health 14:719. doi: 10.3390/ijerph14070719
Eskola, M., Kos, G., Elliott, C. T., Hajšlová, J., Mayar, S., and Krska, R. (2020). Worldwide contamination of food-crops with mycotoxins: validity of the widely cited ‘FAO estimate’ of 25%. Crit. Rev. Food Sci. Nutr. 60, 2773–2789. doi: 10.1080/10408398.2019.1658570
European Commission (2016). Impact Assessment Report on Criteria to dentify EDs. Defining criteria for identifying endocrine disruptors in the context of the implementation of the plant protection products regulation and biocidal product regulation. Avaliable at: https://eur-lex.europa.eu/resource.html?uri=cellar:8d56d9fd-339d-11e6-969e-01aa75ed71a1.0001.02/DOC_7&format=PDF
FDA (2018). Microorganisms & microbial-derived ingredients used in food. U.S. Food & Drug Administration. Available at: https://www.fda.gov/food/generally-recognized-safe-gras/microorganisms-microbial-derived-ingredients-used-food-partial-list (Accessed January 6, 2021).
Fernández-Juri, M. G., Muzzolón, J. A., Dalcero, A. M., and Magnoli, C. E. (2011). Effect of acid lactic bacteria isolated from faeces of healthy dogs on growth parameters and aflatoxin B1 production by Aspergillus species in vitro. Mycotoxin Res. 27, 273–280. doi: 10.1007/s12550-011-0104-9
Ferreira, D. F. (2011). Sisvar: a computer statistical analysis system review. Sisvar: um sistema computacional de análise estatística. Available at: www.dex.ufla.br/~danielff
Flores-Flores, M. E., and González-Peñas, E. (2017). An LC–MS/MS method for multi-mycotoxin quantification in cow milk. Food Chem. 218, 378–385. doi: 10.1016/j.foodchem.2016.09.101
Franco, L. T., Petta, T., Rottinghaus, G. E., Bordin, K., Gomes, G. A., Alvito, P., et al. (2019). Assessment of mycotoxin exposure and risk characterization using occurrence data in foods and urinary biomarkers in Brazil. Food Chem. Toxicol. 128, 21–34. doi: 10.1016/j.fct.2019.03.046
Freire, L., Guerreiro, T. M., Pia, A. K. R., Lima, E. O., Oliveira, D. N., Melo, C. F. O. R., et al. (2018). A quantitative study on growth variability and production of ochratoxin A and its derivatives by A. carbonarius and A. niger in grape-based medium. Sci. Rep. 8:14573. doi: 10.1038/s41598-018-32907-z
Freire, L., and Sant’Ana, A. S. (2018). Modified mycotoxins: an updated review on their formation, detection, occurrence, and toxic effects. Food Chem. Toxicol. 111, 189–205. doi: 10.1016/j.fct.2017.11.021
Galvano, F., Galofaro, V., and Galvano, G. (1996). Occurrence and stability of aflatoxin M1 in milk and milk products: a worldwide review. J. Food Prot. 59, 1079–1090. doi: 10.4315/0362-028X-59.10.1079
Gao, Y., Ye, Q., Bao, X., Huang, X., Wang, J., and Zheng, N. (2020). Transcriptomic and proteomic profiling reveals the intestinal immunotoxicity induced by aflatoxin M1 and ochratoxin A. Toxicon 180, 49–61. doi: 10.1016/j.toxicon.2020.03.008
Gbashi, S., Madala, N. E., De Saeger, S., De Boevre, M., Adekoya, I., Adebo, O. A., et al. (2018). “The Socio-Economic Impact of Mycotoxin Contamination in Africa,” in Mycotoxins - Impact and Management Strategies. eds. P. B. Njobeh and F. Stepman (London, UK: Intechopen).
Ghanbari, R., Molaee Aghaee, E., Rezaie, S., Jahed Khaniki, G., Alimohammadi, M., Soleimani, M., et al. (2018). The inhibitory effect of lactic acid bacteria on aflatoxin production and expression of aflR gene in Aspergillus parasiticus. J. Food Saf. 38, 2–7. doi: 10.1111/jfs.12413
Gomaa, E. Z., Abdelall, M. F., and El-Mahdy, O. M. (2018). Detoxification of aflatoxin B1 by antifungal compounds from Lactobacillus brevis and Lactobacillus paracasei, isolated from dairy products. Probiotics Antimicrob. Proteins 10, 201–209. doi: 10.1007/s12602-017-9350-2
Gonçalves, B. L., Muaz, K., Coppa, C. F. S. C., Rosim, R. E., Kamimura, E. S., Oliveira, C. A. F., et al. (2020). Aflatoxin M1 absorption by non-viable cells of lactic acid bacteria and Saccharomyces cerevisiae strains in Frescal cheese. Food Res. Int. 136:e12413. doi: 10.1016/j.foodres.2020.109604
Greeff-Laubscher, M. R., Beukes, I., Marais, G. J., and Jacobs, K. (2020). Mycotoxin production by three different toxigenic fungi genera on formulated abalone feed and the effect of an aquatic environment on fumonisins. Mycology 11, 105–117. doi: 10.1080/21501203.2019.1604575
Gummadidala, P. M., Omebeyinje, M. H., Burch, J. A., Chakraborty, P., Biswas, P. K., Banerjee, K., et al. (2019). Complementary feeding may pose a risk of simultaneous exposures to aflatoxin M1 and deoxynivalenol in Indian infants and toddlers: lessons from a mini-survey of food samples obtained from Kolkata, India. Food Chem. Toxicol. 123, 9–15. doi: 10.1016/j.fct.2018.10.006
Hashemi, S. M. B., and Gholamhosseinpour, A. (2019). Fermentation of table cream by Lactobacillus plantarum strains: effect on fungal growth, aflatoxin M1 and ochratoxin A. Int. J. Food Sci. Technol. 54, 347–353. doi: 10.1111/ijfs.13943
Haskard, C. A., El-Nezami, H. S., Kankaanpää, P. E., Salminen, S., and Ahokas, J. T. (2001). Surface binding of aflatoxin B1 by lactic acid bacteria. Appl. Environ. Microbiol. 67, 3086–3091. doi: 10.1128/AEM.67.7.3086–3091.2001
Hill, D., Sugrue, I., Tobin, C., Hill, C., Stanton, C., and Ross, R. P. (2018). The Lactobacillus casei group: history and health related applications. Front. Microbiol. 9:2107. doi: 10.3389/fmicb.2018.02107
Huang, W., Chang, J., Wang, P., Liu, C., Yin, Q., Zhu, Q., et al. (2018). Effect of the combined compound probiotics with mycotoxin-degradation enzyme on detoxifying aflatoxin B1 and zearalenone. J. Toxicol. Sci. 43, 377–385. doi: 10.2131/jts.43.377
International Agency for Research on Cancer (1993). IARC monographs on the evaluation of carcinogenic risks to humans. Vol. 56. 245–395. Available at: http://monographs.iarc.fr/ENG/Monographs/vol56/mono56.pdf (Accessed January 19, 2020).
International Agency for Research on Cancer (2012). IARC Monographs on the Evaluation of Carcinogenic Risks to Humans/World Health Organization, International Agency for Research on Cancer, 100, 9–562.
Ismail, A., Levin, R. E., Riaz, M., Akhtar, S., Gong, Y. Y., and de Oliveira, C. A. F. (2017). Effect of different microbial concentrations on binding of aflatoxin M1 and stability testing. Food Control 73, 492–496. doi: 10.1016/j.foodcont.2016.08.040
Jameson, J. E. (1962). A discussion of the dynamics of Salmonella enrichment. J. Hyg. 60, 193–207. doi: 10.1017/s0022172400039462
Khaneghah, A. M., Moosavi, M., Omar, S. S., Oliveira, C. A. F., Karimi-Dehkordi, M., Fakhri, Y., et al. (2021). The prevalence and concentration of aflatoxin M1 among different types of cheeses: a global systematic review, meta-analysis, and meta-regression. Food Control 125:107960. doi: 10.1016/j.foodcont.2021.107960
Kowalczyk, J. E., Peng, M., Pawlowski, M., Lipzen, A., Ng, V., Singan, V., et al. (2019). The white-rot basidiomycete dichomitus squalens shows highly specific transcriptional response to lignocellulose-related aromatic compounds. Front. Bioeng. Biotechnol. 7:229. doi: 10.3389/fbioe.2019.00229
Kuharić, Ž., Jakopović, Ž., Čanak, I., Frece, J., Bošnir, J., Pavlek, Ž., et al. (2018). Removing aflatoxin M1 from milk with native lactic acid bacteria, centrifugation, and filtration. Arh. Hig. Rada Toksikol. 69, 334–339. doi: 10.2478/aiht-2018-69-3160
Li, L., Yang, B., Humza, M., Geng, H., Wang, G., Zhang, C., et al. (2021). A novel strain Lactobacillus brevis 8-2B inhibiting Aspergillus carbonarius growth and ochratoxin a production. LWT Food Sci. Technol. 136:110308. doi: 10.1016/j.lwt.2020.110308
Luz, C., Saladino, F., Luciano, F. B., Mañes, J., and Meca, G. (2017). In vitro antifungal activity of bioactive peptides produced by Lactobacillus plantarum against Aspergillus parasiticus and Penicillium expansum. LWT Food Sci. Technol. 81, 128–135. doi: 10.1016/j.lwt.2017.03.053
Margalho, L. P., Feliciano, M. D. E., Silva, C. E., Abreu, J. S., Piran, M. V. F., and Sant’Ana, A. S. (2020). Brazilian artisanal cheeses are rich and diverse sources of nonstarter lactic acid bacteria regarding technological, biopreservative, and safety properties—insights through multivariate analysis. J. Dairy Sci. 103, 7908–7926. doi: 10.3168/jds.2020-18194
Møller, C. O. D. A., Christensen, B. B., and Rattray, F. P. (2021). Modelling the biphasic growth of non-starter lactic acid bacteria on starter-lysate as a substrate. Int. J. Food Microbiol. 337:108937. doi: 10.1016/j.ijfoodmicro.2020.108937
Muaz, K., Riaz, M., Oliveira, C. A. F., Akhtar, S., Ali, S. W., Nadeem, H., et al. (2021). Aflatoxin M1 in milk and dairy products: global occurrence and potential decontamination strategies. Toxin Rev. 1–18. doi: 10.1080/15569543.2021.1873387
Muhialdin, B. J., Hassan, Z., and Sadon, S. K. (2011). Antifungal activity of Lactobacillus fermentum Te007, Pediococcus pentosaceus Te010, Lactobacillus pentosus G004, and L. paracasi D5 on selected foods. J. Food Sci. 76, 493–499. doi: 10.1111/j.1750-3841.2011.02292.x
Muhialdin, B. J., Saari, N., and Meor Hussin, A. S. (2020). Review on the biological detoxification of mycotoxins using lactic acid bacteria to enhance the sustainability of foods supply. Molecules 25:2655. doi: 10.3390/molecules25112655
Oliveira, C. A. F., Corassin, C. H., Corrêa, B., and Oswald, I. P. (2014). “Animal Health: Mycotoxins,” in Encyclopedia of Agriculture and Food Systems. Vol. 1. ed. N. K. Van Alfen (San Diego: Elsevier), 358–377.
Oliveira, C. A. F., Sebastião, L. S., Fagundes, H., Rosim, R. E., and Fernandes, A. M. (2008). Aflatoxins and cyclopiazonic acid in feed and milk from dairy farms in são Paulo, Brazil. Food Addit. Contam. Part B. Surveill. 1, 147–152. doi: 10.1080/02652030802382865
Palumbo, R., Crisci, A., Venâncio, A., Abrahantes, J. C., Dorne, J. L., Battilani, P., et al. (2020). Occurrence and co-occurrence of mycotoxins in cereal-based feed and food. Microorganisms 8:74. doi: 10.3390/microorganisms8010074
Pauletto, M., Giantin, M., Tolosi, R., Bassan, I., Barbarossa, A., Zaghini, A., et al. (2020). Curcumin mitigates AFB1-induced hepatic toxicity by triggering cattle antioxidant and anti-inflammatory pathways: a whole transcriptomic in vitro study. Antioxidants 9:1059. doi: 10.3390/antiox9111059
Pitt, J. I., and Miller, J. D. (2017). A concise history of mycotoxin research. J. Agric. Food Chem. 65, 7021–7033. doi: 10.1021/acs.jafc.6b04494
Pócsi, I., Giacometti, F., Ambrus, Á., and Logrieco, A. F. (2020). Editorial: Aspergillus-derived mycotoxins in the feed and food chain. Front. Microbiol. 11:606108. doi: 10.3389/fmicb.2020.606108
Poornachandra Rao, K., Deepthi, B. V., Rakesh, S., Ganesh, T., Achar, P., and Sreenivasa, M. Y. (2019). Antiaflatoxigenic potential of cell-free supernatant from Lactobacillus plantarum MYS44 against Aspergillus parasiticus. Probiotics Antimicrob. Proteins 11, 55–64. doi: 10.1007/s12602-017-9338-y
RASFF (2020). The rapid alert system for food and feed—Annual Report 2019. Available at: https://op.europa.eu/en/publication-detail/-/publication/2c5c7729-0c31-11eb-bc07-01aa75ed71a1/language-en/format-PDF/source-174742448 (Accessed January 05, 2021).
Rogowska, A., Pomastowski, P., Walczak, J., Railean-Plugaru, V., Rudnicka, J., and Buszewski, B. (2019). Investigation of zearalenone adsorption and biotransformation by microorganisms cultured under cellular stress conditions. Toxins 11:463. doi: 10.3390/toxins11080463
Sadhasivam, S., Shapiro, O. H., Ziv, C., Barda, O., Zakin, V., and Sionov, E. (2019). Synergistic inhibition of mycotoxigenic fungi and mycotoxin production by combination of pomegranate peel extract and azole fungicide. Front. Microbiol. 10:1919. doi: 10.3389/fmicb.2019.01919
Samson, R. A., Hoekstra, E. S., Frisvad, J. C., and Filtenborg, O. (2002). Introduction to Food- and Airborne Fungi. The Netherlands: CBS.
Sangmanee, P., and Hongpattarakere, T. (2014). Inhibitory of multiple antifungal components produced by Lactobacillus plantarum K35 on growth, aflatoxin production and ultrastructure alterations of Aspergillus flavus and Aspergillus parasiticus. Food Control 40, 224–233. doi: 10.1016/j.foodcont.2013.12.005
Schatzmayr, G., and Streit, E. (2013). Global occurrence of mycotoxins in the food and feed chain: facts and figures. World Mycotoxin J. 6, 213–222. doi: 10.3920/WMJ2013.1572
Singh, P., and Cotty, P. J. (2019). Characterization of Aspergilli from dried red chilies (Capsicum spp.): insights into the etiology of aflatoxin contamination. Int. J. Food Microbiol. 289, 145–153. doi: 10.1016/j.ijfoodmicro.2018.08.025
Verheecke, C., Liboz, T., Darriet, M., Sabaou, N., and Mathieu, F. (2014). In vitro interaction of actinomycetes isolates with Aspergillus flavus: impact on aflatoxins B1 and B2 production. Lett. Appl. Microbiol. 58, 597–603. doi: 10.1111/lam.12233
Whiting, R. C., and Golden, M. H. (2002). Variation among Escherichia coli O157:H7 strains relative to their growth, survival, thermal inactivation, and toxin production in broth. Int. J. Food Microbiol. 75, 127–133. doi: 10.1016/S0168-1605(02)00003-X
Wigmann, É. F., Moreira, R. C., Alvarenga, V. O., Sant’Ana, A. S., and Copetti, M. V. (2016). Survival of Penicillium spp. conidia during deep-frying and baking steps of frozen chicken nuggets processing. Food Microbiol. 55, 1–6. doi: 10.1016/j.fm.2015.11.010
World Health Organization (2016). New IARC report urges action against widespread mycotoxin contamination in developing countries. IARC WHO Press Release 2016, 9, 2015–2016. Available at: https://www.iarc.who.int/wp-content/uploads/2018/07/pr242_E.pdf (Accessed January 05, 2021).
Zhang, X., Yang, H., Apaliya, M. T., Zhao, L., Gu, X., Zheng, X., et al. (2018). The mechanisms involved in ochratoxin A elimination by Yarrowia lipolytica Y-2. Ann. Appl. Biol. 173, 164–174. doi: 10.1111/aab.12452
Keywords: Lactobacillus, Aspergillus, microbial interaction, mycotoxigenic fungus, mycotoxin inhibition, mycotoxin reduction
Citation: Møller COdA, Freire L, Rosim RE, Margalho LP, Balthazar CF, Franco LT, Sant’Ana AS, Corassin CH, Rattray FP and Oliveira CAFd (2021) Effect of Lactic Acid Bacteria Strains on the Growth and Aflatoxin Production Potential of Aspergillus parasiticus, and Their Ability to Bind Aflatoxin B1, Ochratoxin A, and Zearalenone in vitro. Front. Microbiol. 12:655386. doi: 10.3389/fmicb.2021.655386
Edited by:
Marco Camardo Leggieri, Catholic University of the Sacred Heart, ItalyReviewed by:
Carol Verheecke-Vaessen, Cranfield University, United KingdomMichail D. Kaminiaris, Agricultural University of Athens, Greece
Copyright © 2021 Møller, Freire, Rosim, Margalho, Balthazar, Franco, Sant’Ana, Corassin, Rattray and Oliveira. This is an open-access article distributed under the terms of the Creative Commons Attribution License (CC BY). The use, distribution or reproduction in other forums is permitted, provided the original author(s) and the copyright owner(s) are credited and that the original publication in this journal is cited, in accordance with accepted academic practice. No use, distribution or reproduction is permitted which does not comply with these terms.
*Correspondence: Cleide Oliveira de Almeida Møller, cleide@food.ku.dk