- 1College of Food Science and Engineering, Northwest A&F University, Xianyang, China
- 2National Engineering Research Center of Seafood, Collaborative Innovation Center of Seafood Deep Processing, Dalian Polytechnic University, Dalian, China
Cronobacter sakazakii (C. sakazakii) is an emerging opportunistic foodborne pathogen that can cause neonatal necrotizing enterocolitis, meningitis, sepsis in neonates and infants with a relatively high mortality rate. Bacterial transcytosis across the human brain microvascular endothelial cells (HBMEC) is vital for C. sakazakii to induce neonatal meningitis. However, few studies focus on the mechanisms by which C. sakazakii translocates HBMEC. In this study, the translocation processes of C. sakazakii on HBMEC were explored. C. sakazakii strains could effectively adhere to, invade and intracellularly survive in HBMEC. The strain ATCC 29544 exhibited the highest translocation efficiency across HBMEC monolayer among four tested strains. Bacteria-contained intracellular endosomes were detected in C. sakazakii-infected HBMEC by a transmission electron microscope. Endocytosis-related proteins CD44, Rab5, Rab7, and LAMP2 were increased after infection, while the level of Cathepsin L did not change. C. sakazakii induced TLR4/NF-κB inflammatory signal pathway activation in HBMEC, with increased NO production and elevated mRNA levels of IL-8, IL-6, TNF-α, IL-1β, iNOS, and COX-2. C. sakazakii infection also caused LDH release, caspase-3 activation, and HBMEC apoptosis. Meanwhile, increased Dextran-FITC permeability and decreased trans epithelial electric resistance indicated that C. sakazakii disrupted tight junction of HBMEC monolayers, which was confirmed by the decreased levels of tight junction-related proteins ZO-1 and Occludin. These findings suggest that C. sakazakii induced intracellular bacterial endocytosis, stimulated inflammation and apoptosis, disrupted monolayer tight junction in HBMEC, which all together contribute to bacterial translocation.
Introduction
Cronobacter sakazakii (C. sakazakii) is rod-shaped Gram-negative opportunistic pathogen, which is closely associated with neonatal necrotizing enterocolitis, sepsis, meningitis, and meningo-encephalitis in neonates and infants (Mullane et al., 2007; Weng et al., 2014). C. sakazakii could contaminate and survive in powdered infant formulas and infections in infants have been commonly associated with consumption of contaminated powdered infant formula (Hunter et al., 2008; Tall et al., 2014). Without appropriate treatment, C. sakazakii infection could lead to a relatively high mortality rate (approximately 33–80%) (Patrick et al., 2014; Lepuschitz et al., 2019; Elkhawaga et al., 2020).
Cronobacter sakazakii is one of the important pathogens implicated in neonatal meningitis (Ogrodzki and Forsythe, 2015). Previous studies have shown that several meningitis-related pathogens could penetrate the blood brain barrier (BBB) and invade the central nervous system (CNS). Once the pathogens enter the CNS, they could multiply and induce the release of proinflammatory cytokines and toxins (Sanders et al., 2011; Karassek et al., 2015), which leads to increased BBB permeability and the migration of lymphocytes, monocytes, and neutrophils across the BBB (pleocytosis), resulting in inflammatory responses in pallium tissues and meningitis (Mu et al., 2014). To cause infections in the brain, the pathogen needs to survive in the host bloodstream, cross the BBB and gain entry into the CNS (Van Sorge and Doran, 2012). C. sakazakii has been proved to be able to invade epithelial and endothelial cells and evade the immune responses, thereby leading to bacterial colonization and proliferation in the host CNS to induce meningitis (Almajed and Forsythe, 2016).
Blood brain barrier maintains the homeostasis of the CNS and is a critical line of defense against harmful microbial pathogens and other factors that potentially inflict damages on the host (Lemichez et al., 2010). BBB is mainly composed of endothelial cells and astrocytes lining the brain microvasculature (Abbott et al., 2010). Diverse factors facilitating the bacterial crossing of brain capillary endothelial cells have been identified, and several pathogens have been proved to cross BBB through both “transcellular” and “paracellular” routes (Neuhaus et al., 2018; Lu et al., 2019). Endocytosis plays an important role in translocation across BBB. For example, Escherichia coli K1 translocated HBMEC through endocytosis. The pathogen could adhere to receptors on the surface of HBMEC, stimulating the activation of the host molecules involved in a number of signaling pathways, including endocytosis-related proteins and kinases (Loh et al., 2017). A similar endocytosis process was also reported in Group B Streptococcus during the crossing of the BBB model (Benmimoun et al., 2020). Disruption of the BBB structure also facilitates the processes of bacterial translocation, and several inflammatory mediators (such as TNF-alpha, nitric oxide, and IL-6) contribute to HBMEC monolayer disruption (McLoughlin et al., 2017). Certain pathogens, like Neisseria meningitidis, could induce an increase of permeability in HBMEC, facilitating bacterial translocation across HBMEC monolayers (Schubert-Unkmeir et al., 2010). Some pathogens are proven to be able to disrupt endothelial tight junction structures by decreasing tight junction-related proteins (ZO-1, Occludin, and Claudin-5), which leads to an increase in bacterial translocation from the blood into CNS (Yuan et al., 2020).
Some studies have researched the mechanisms of Cronobacter pathogenesis in neonatal necrotizing enterocolitis, intestinal barrier translocation, and potential virulence factors (Liu Q. et al., 2012; Ye et al., 2016). For example, it has been proven that host cell actin rearrangements are required for Cronobacter sakazakii invasion into HBMEC (Li et al., 2010; Liu D. X. et al., 2012). However, the mechanisms by which C. sakazakii transverse HBMEC remain elusive.
This study aimed to decipher the routes through which C. sakazakii crosses HBMEC monolayers. Experiments were carried out to examine the bacterial endocytosis, inflammatory responses, endothelial monolayer permeability, cell apoptosis, and disruption of the tight junction in HBMEC infected by C. sakazakii.
Materials and Methods
Bacterial Strains and Cell Line
Cronobacter sakazakii strains (strain no. ATCC29544, ATCC12868, ATCC29004, and ATCCBAA-894) and human brain microvascular endothelial cells (HBMEC) were purchased from the American Type Culture Collection (ATCC). Bacterial strains were grown at 37°C in brain heart infusion (BHI) broth (Landbridge, Beijing, China) overnight. HBMEC were grown in DMEM (Dulbecco’s modified eagle medium, Gibco, Grand Island, NY, United States) with 10% FBS (Biological Industries, Kibbutz Beit Haemek, Israel) at 37°C and 5% CO2 for the indicated period of time in different experiments.
Bacterial Adhesion to HBMEC
Adhesion assays were performed as previously described with some modifications (Amalaradjou et al., 2014). Briefly, HBMEC were seeded into 24-well plates (105 cells per well), grown in DMEM (Dulbecco’s modified eagle medium) with 10% FBS at 37°C and 5% CO2 for 18 h (70–90% confluence in the well), and then rinsed twice with PBS (Beyotime, Shanghai, China). C. sakazakii strains (ATCC29544, 12868, 29004, BAA-894) were cultured in BHI broth at 37°C overnight. The bacterial suspensions were washed twice with PBS and resuspended in DMEM to approximately 107 CFU/mL. Then 1ml of C. sakazakii suspension was added to each well at a multiplicity of infection (MOI) of 100:1 and incubated at 37°C in a humidified 5% CO2 incubator for 2 h. After incubation, the infected monolayers of HBMEC were washed twice with ice-cold PBS and lysed with 1 mL 0.1% Triton X-100 (Beyotime) at 4°C for 25 min. The lysates were serially diluted, plated onto LB agar (Landbridge), and incubated at 37°C overnight for counting.
Invasion and Intracellular Survival
The invasion and intracellular survival were examined as previously described with minor modifications (Ryan et al., 2018). HBMEC and C. sakazakii samples were prepared as in the adhesion assay. After incubation with bacteria for 2 h, HBMEC monolayers were washed once with PBS and incubated for 40 min with DMEM containing gentamicin (100 μg/mL) to kill the extracellular bacteria. HBMEC were then washed and cultured with DMEM containing 10 μg/mL gentamicin for an additional 0, 2, 4, and 6 h. After being washed with PBS three times, HBMEC monolayers were lysed with 0.1% Triton X-100 at 4°C for 25 min. The sample dilutions were then plated on LB agar to count the invaded bacterial (0 h) and intracellularly survived bacteria (2, 4, and 6 h).
Transcytosis Assay
The transwell systems were employed for transcytosis assay as previously described (Chen et al., 2019), HBMEC were seeded (2 × 104 cells/insert) onto the inner surface of collagen-coated transwell inserts (24 well format, 3.0-μm pore size polycarbonate filter; Corning, NY, United States) which were placed in wells of a 24-well plate (Figure 1C). After growth in DMEM (containing 10% FBS) for 5 days, the HBMEC monolayers formed tight junctions, which was evidenced by TEER measurement. After washing with PBS three times, each transwell was moved to a new well containing 800 μL of fresh FBS-free DMEM medium, and the top well was replaced with 500 μL of C. sakazakii suspensions. The HBMEC monolayer was incubated at 37°C under 5% CO2 for 3 h, then the samples from the bottom well were serial diluted and plated on LB agar and cultured at 37°C overnight to count the number of translocated C. sakazakii.
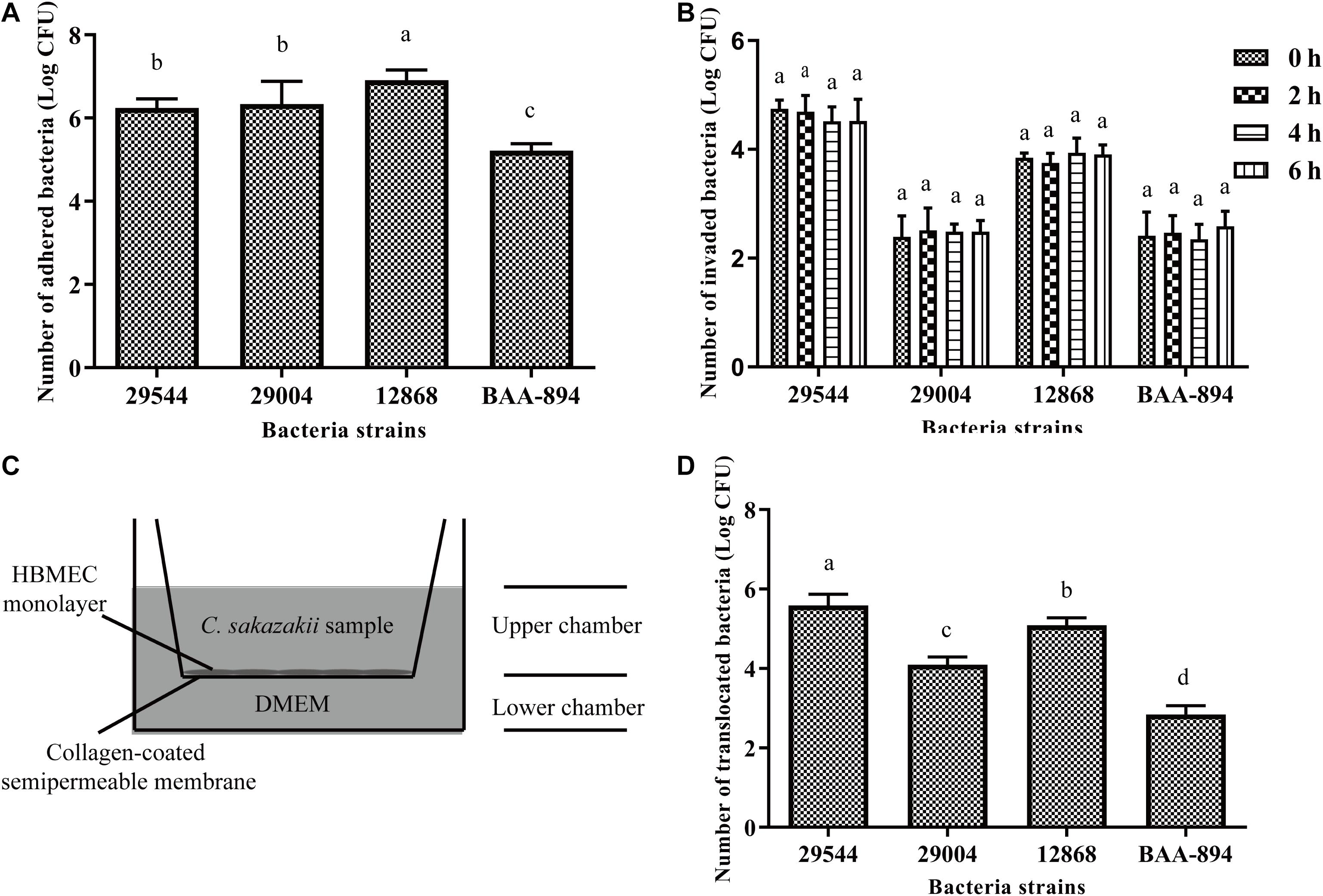
Figure 1. Adhesion, invasion, intracellular replication, and translocation of C. sakazakii in HBMEC. (A) The number of C. sakazakii cells that adhere to HBMEC. (B) The number of C. sakazakii cells in HBMEC after 2 h invasion and intracellular bacteria counts at 2, 4, and 6 h post-invasion. (C) HBMEC were incubated in transwell inserts. (D) The counts of C. sakazakii that translocated HBMEC monolayers were calculated. The strain ATCC 29544 performed the strongest invasion and translocation abilities compared to other strains (P < 0.01). Bars represent the means ± standard deviations (n = 3). Mean values with different lower-case letters are statistically different (P < 0.05).
Transmission Electron Microscope (TEM)
HBMEC were seeded into a 90-mm culture dish (105 per dish) and incubated at 37°C, 5% CO2 overnight to 70–90% confluence. Then HBMEC were infected with C. sakazakii ATCC 29544 for 0, 1, 2, and 3 h (MOI = 100). TEM samples were prepared as previously described (Lu et al., 2019). In brief, the infected cells were fixed with 2.5% glutaraldehyde for 6 h, followed by treatment with 1% osmic acid buffer for 2 h. Fixed HBMEC were dehydrated using an ethanol gradient (30, 50, 70, 80, 90, and 100%), then embedded and polymerized with the LR white with resin gradient to achieve final ultrathin sections (70 nm) on an ultramicrotome (UCT, Leica Microsystems). Ultrathin sections were mounted onto copper grids and stained with 4% uranyl acetate and lead citrate. Imaging was performed using a Transmission Electron Microscope (FEI TECNAI G2 SPIRIT BIO) operating at 120 kV. Digital images of HBMEC were captured using the Quemesa Bottom-Mount CCD and Morada G3 Side-Mount CCD Camera.
Immunofluorescence
Immunofluorescence assays were performed according to a previous study with some modifications (Mu et al., 2014). For bacterial visualization, a GFP-labeled plasmid pCA66-GFP was electrotransformated into C. sakazakii ATCC 29544. The plasmid did not affect the invasion abilities of the strain (data not shown). HBMEC were propagated on round glass coverslips within 24-well plates to 70–90% confluence and infected with GFP-labeled C. sakazakii (MOI = 100). After infection for 0, 1, 2, 3, and 4 h, the cells were washed with PBS and fixed with 4% paraformaldehyde at 4°C for 1 h. After blocking with QuickBlockTM Blocking Buffer (Beyotime, Shanghai, China) for 20 min at room temperature, the samples were incubated with the 1:100 dilutions of primary antibodies of CD44 (Proteintech, Wuhan, China), Rab5 (Beyotime), Rab7 (Beyotime), LAMP2 (Beyotime), Cathepsin L (Proteintech), and NF-κB p65 (Proteintech) at 4°C overnight. Cy-3 labeled secondary antibodies were used at a 1:500 dilution at room temperature. Samples on the slides were washed with PBS and mounted onto microscope slides with antifade reagent with DAPI (Beyotime). The images of protein expression and distribution in HBMEC were obtained with confocal laser scanning microscopy (Revolution-XD, Andor, England).
Western Blot
HBMEC were prepared as in TEM assay (for detection of tight junction related-proteins ZO-1 and Occludin, HBMEC were grown for 5 days to form tight junction) and then infected with C. sakazakii ATCC 29544 for 0, 1, 2, 3, and 4 h (MOI = 100). Then, the samples were washed twice with ice-cold PBS and the cell lysates were prepared in lysis buffer with proteinase inhibitor cocktails (Beyotime). The supernatants were centrifuged at 13000 × g for 10 min at 4°C, and the protein concentrations were measured with a Bradford assay kit (Beyotime). An equal amount of proteins (30 μg) from samples were resolved by SDS-polyacrylamide gel electrophoresis and electrotransferred onto a nitrocellulose (NC) membrane. After being blocked, the NC membrane was subsequently incubated with specific primary antibodies at 4°C overnight and then washed by TBST (Tris-buffered saline, Tween 20, Beyotime). Horseradish peroxidase-conjugated secondary antibodies (1:1000 in TBST) were added and the mixture was incubated for 90 min at room temperature. After washing, the blots were detected with ECL reagents (Beyotime) and ChemiDocXRS+System (Bio-Rad, CA, United States). ACTB (β-actin) was used as an internal standard, the expression levels of CD44, Cathepsin L, TLR4, IκBα, ZO-1, Occludin, Rab5, Rab7, and LAMP2 were detected.
Quantitative RT-qPCR
HBMEC were seeded in 60-mm dishes to 70–90% confluence and infected with C. sakazakii ATCC 29544 for 0, 1, 2, 3, and 4 h (MOI = 100). Total RNA was extracted from HBMEC using RNAeasyTM Animal RNA Isolation Kit R0026 (Beyotime), and then reverse transcribed to cDNA using Evo M-MLV RT Premix AG11706 (Accurate Biotechnology, Changsha, China) with the suppliers’ instructions. Quantitative Real-Time PCR was performed with SYBR® Green Premix Pro Taq HS qPCR Kit AG11701 (AG11701; Accurate Biotechnology) on a Bio-Rad iQ5 PCR system (Bio-Rad). The primer sequences for RT-PCR are shown in Table 1. ACTB was used as the control. The Ct values for RT-PCR samples were recorded. The relative mRNA levels were analyzed with the 2−ΔΔCt method.
Nitric Oxide Assay
To detect nitric oxide (NO) release from HBMEC, the cells were seeded into a 96-well plate (104 cells/well) and grown overnight to 70–90% confluence. Then cells were infected with C. sakazakii ATCC 29544 for 0, 1, 2, 3, and 4 h (MOI = 100). NO concentrations were quantified using a nitric oxide assay kit S0021S (Beyotime). Briefly, 50 μL of Griess reagent I and Griess reagent II were dripped into each well containing 50 μL of culture supernatants or NaNO2 standards. After reaction for 5 min, the absorbance of samples was measured at 540 nm utilizing a microplate reader (Victor X3; PE, Singapore) to determine the NO release.
Lactate Dehydrogenase (LDH) Release
The procedures for C. sakazakii infection of HBMEC were the same as the NO release assay. LDH release was detected using an LDH Cytotoxicity Assay Kit C0017 (Beyotime). The absorbance at 490 nm of samples was measured with a microplate reader (Victor X3; PE, Singapore) to evaluate LDH release after C. sakazakii infection. The percentage of LDH release was calculated as follows: % LDH release = (sample LDH activity-background LDH)/(total LDH activity-background LDH) ×100.
Caspase-3 Activity and Apoptosis Assay
HBMEC cells were seeded into a 60-mm dish and grown overnight to 70–90% confluence. Then cells were infected with C. sakazakii for 0, 1, 2, 3, and 4 h to detect Caspase-3 activity and apoptosis levels. Caspase-3 activity was detected with a Caspase-3 Activity Assay Kit C1115 (Beyotime). In brief, cells were lysed on an ice bath and centrifuged (18000 × g, 15 min, 4°C) to collect the supernatant. After incubation with Ac-DEVD-pNA for 20 h at 37°C, the samples were measured with a microplate reader (VictorX3, PE, Singapore) at an absorbance of 405 nm.
Apoptosis assay was performed with Annexin V-FITC/PI Apoptosis Detection Kit C1062S (Beyotime) according to the instructions. HBMEC were collected and washed twice with PBS after treatments, then the cells were stained with Annexin V-FITC and PI reagents. Cell apoptosis levels were measured with a flow cytometer (FACSAria TM III, BD, New Jersey, United States).
Trans Epithelial Electric Resistance (TEER) and Permeability of HBMEC Monolayers
HBMEC were cultured using a transwell system to form a tight junction as previously described. C. sakazakii suspension was added into the inner side of the transwell inserts and incubated for 0, 1, 2, 3, and 4 h. After treatments, the integrity of the HBMEC cellular monolayer was monitored for TEER using a MillicellR ERS-2 meter (Millipore, United States). The permeabilities of HBMEC monolayers were measured by the transport of 4 kDa Dextran-FITC (FD4; Sigma-Aldrich, St. Louis, MO, United States). Dextran-FITC was dissolved in FBS-free DMEM (1 mg/mL) and added to the PBS-washed apical surface of the transwell insert (400 μL per insert) after C. sakazakii infection, 800 μL of pure DMEM medium was added to the bottom side of the transwell systems. After 4 h incubation, fluorescence intensities of the medium from the transwell bottom sides were measured using a fluorescence microplate reader (485 nm excitation/520 nm emission).
Statistical Analysis
All experiments were performed in triplicate. Statistical analyses were performed in SPSS software (Version 22.0; SPSS, Inc., Chicago, IL, United States). The data are presented as the mean ± SD and differences between means were tested by one-way ANOVA. The p values < 0.05 were considered to be statistically significant.
Results
C. sakazakii Invaded, Survived in, and Translocated Across HBMEC
As shown in Figure 1, all four tested C. sakazakii strains (ATCC 29544, 29004, 12868, BAA-894) could adhere to (A) and invade (B) HBMEC cells. After C. sakazakii strains invasion, the number of intracellular survived C. sakazakii bacteria in HBMEC did not change significantly after 6 h (P > 0.1), which indicates intracellular survival without replication in HBMEC (Figure 1B). Four strains were also efficiently translocated across the HBMEC monolayers (Figure 1D). Among four strains, C. sakazakii ATCC 29544 was the most efficient in invading and translocating across HBMEC. Therefore, only the strain ATCC 29544 was used for further studies.
Endocytosis of C. sakazakii in HBMEC
Cronobacter sakazakii bacteria were found in vacuoles of the HBMEC by transmission electron microscopy (Figure 2B). From 1 h to 3 h post-infection, the presence of intracellular endosomes containing bacteria increased in HBMEC, presumably indicating that the strain is undergoing transcytosis.
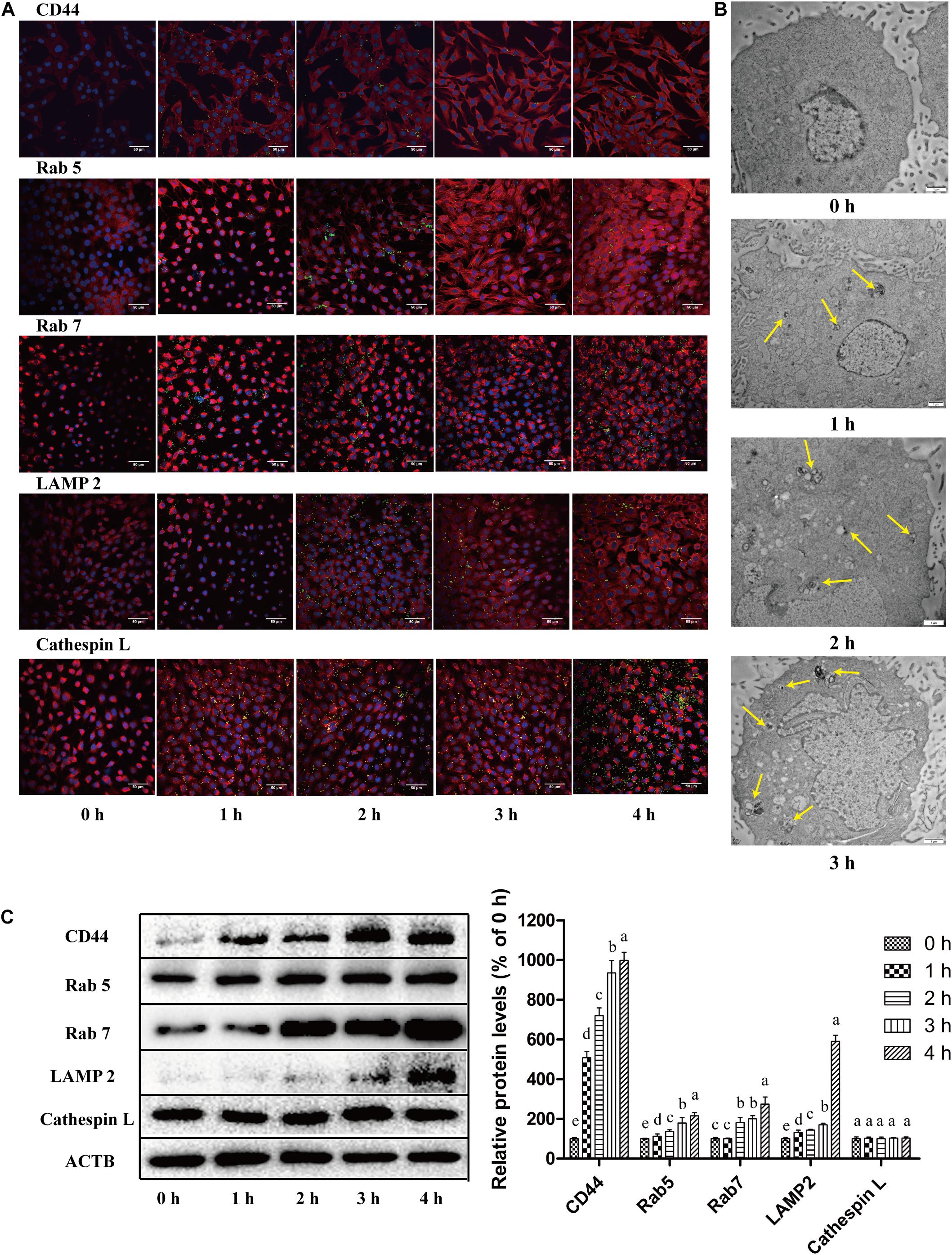
Figure 2. Bacterial endocytosis and intracellular transport of C. sakazakii in HBMEC after the invasion. (A) The expression and localization of endocytosis-related proteins (CD44, Rab5, Rab7, LAMP2, and Cathepsin L) (red fluorescence) in HBMEC (DPAI, blue fluorescence for cell nucleus) infected with C. sakazakii (labeled with GFP, green fluorescence) were analyzed with immunofluorescent staining and observed with a confocal laser scanning microscope. (B) The C. sakazakii-containing endocytosis vacuoles (yellow arrows) were examined in HBMEC using transmission electron microscopy after infection. (C) The expression levels of CD44, Rab5, Rab7, LAMP2, and Cathepsin L in C. sakazakii-infected HBMEC were detected with Western Blot using ACTB as a control. Representative blots for proteins (left) and quantitative analysis (right) were presented. Bars represent the means ± standard deviations (n = 3). Mean values with different lower-case letters are statistically different (P < 0.05).
To confirm the transport of C. sakazakii through endosomes, endocytosis-related proteins were measured with immunofluorescence (Figure 2A) and western blot (Figure 2C). Cell-surface glycoprotein CD44 (an adhesion receptor) was labeled red under fluorescence and its expression in infected cells was increased by 9 fold at 4 h post-infection compared to uninfected HBMEC. Expression of early (Rab5) and late (Rab7) endosomal markers also increased significantly in infected HBMEC (P < 0.05). C. sakazakii-containing intracellular vacuoles colocalized with Rab5 and Rab7. The expression of a lysosomal marker, LAMP2, which facilitates the fusion of intracellular vacuoles with lysosomes, was also enhanced in HBMEC after C. sakazakii infection. Additionally, C. sakazakii infection did not change the levels of the lysosomal enzyme Cathepsin L. Meanwhile, co-localization rates of GFP-labeled C. sakazakii with endocytosis-related proteins in HBMEC were analyzed with immunofluorescence (Table 2). Intracellular bacteria are closely associated with CD44, Rab5, Rab7, and LAMP2. However, intracellular C. sakazakii showed a limited association with Cathepsin L. Taken together, intracellular C. sakazakii ATCC 29544 is associated with endocytosis, which could contribute to bacterial translocation across HBMEC.
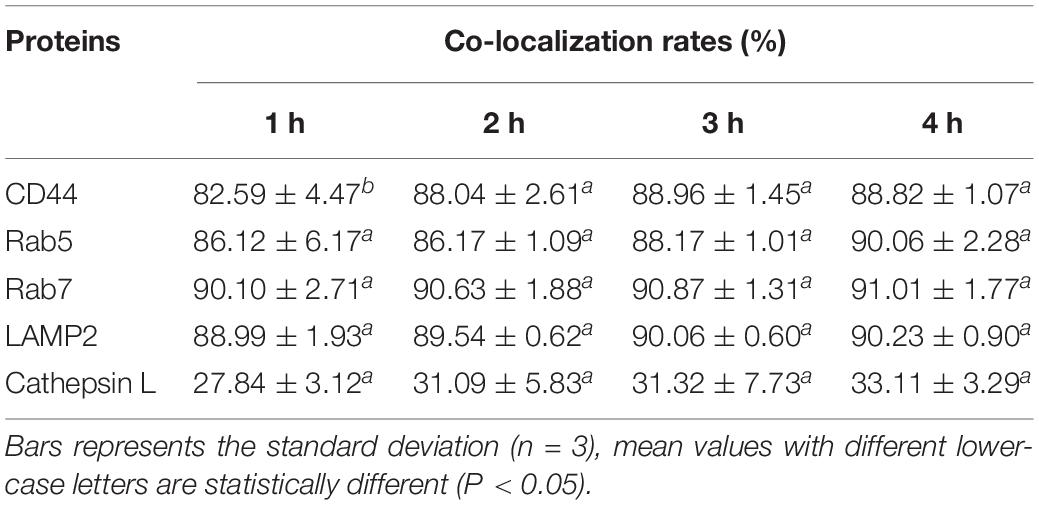
Table 2. Co-localization rates of GFP-labeled C. sakazakii with endocytosis-related proteins in HBMEC.
C. sakazakii Induced Inflammatory Responses in HBMEC
As shown in Figure 3A, C. sakazakii infection stimulated the translocation of NF-κB p65 from the cytoplasm to the nucleus in HBMEC. After infection for 4 h, 80.63% of HBMEC were positive for NF-κB p65 nuclear translocation (Figure 3B). To further demonstrate the activation of the inflammatory pathway, TLR4/NF-κB pathway related proteins were detected by western blot (Figure 3D). After infection for 4 h, relative expression levels of TLR4 in HBMEC increased 8.30 fold. Meanwhile, a significant reduction was observed for IκBα expression, contributing to the activation of the TLR4/NF-κB inflammation pathway.
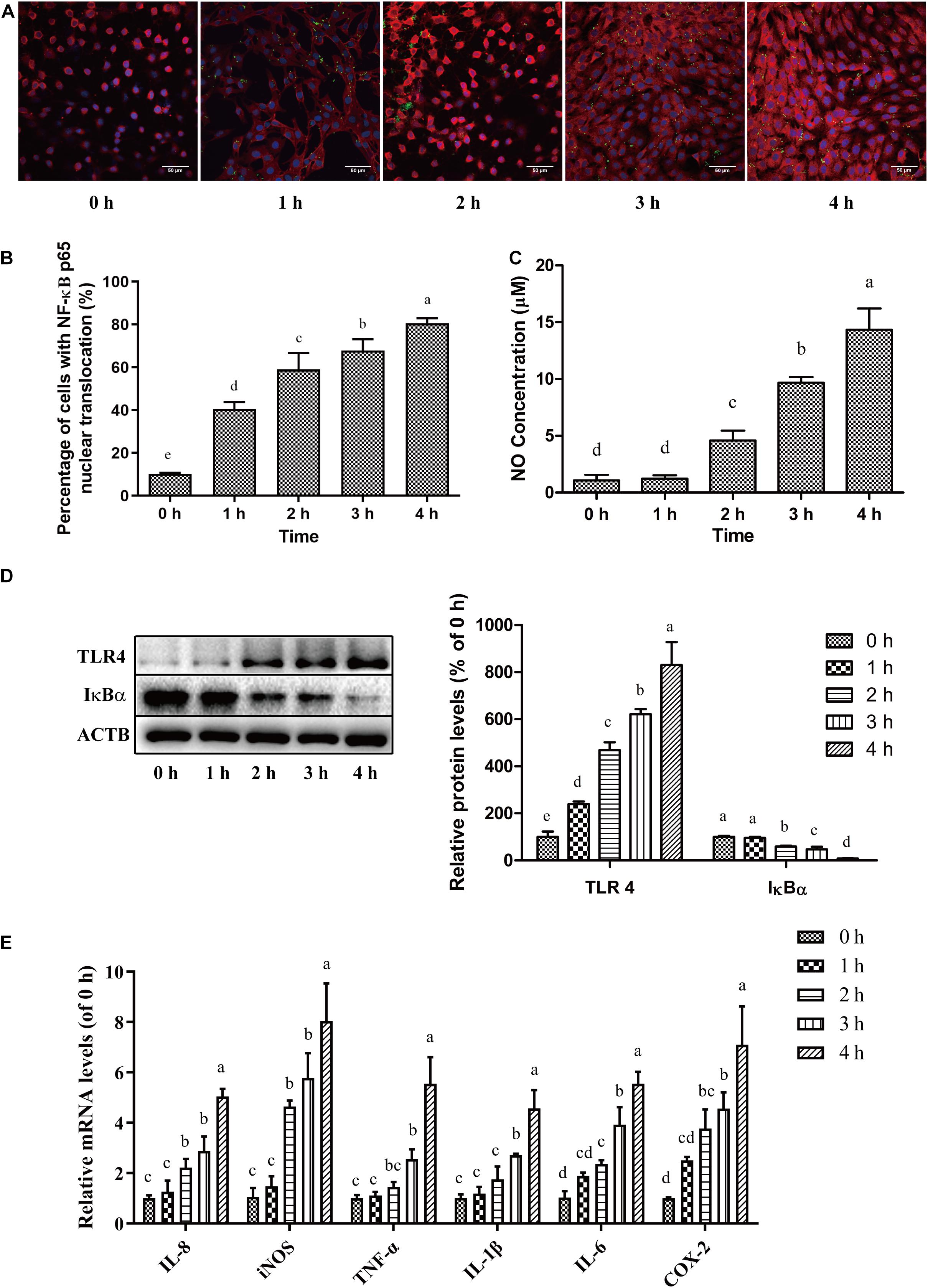
Figure 3. C. sakazakii infection induced inflammatory responses in HBMEC. After C. sakazakii infection, the nuclear translocation of NF-κB p65 in HBMEC was analyzed with immunofluorescent staining. (A) Representative fluorescence micrograph and (B) quantitative analysis were presented. (C) The concentrations of NO in culture medium supernatant was measured. (D) The blots (left) and quantitative analysis (right) for IκBα and TLR4 protein in HBMEC after C. sakazakii infection. (E) Relative mRNA levels of IL-8, IL-6, TNF-α, IL-1β, iNOS, and COX-2 in HBMEC after C. sakazakii infection for 0, 1, 2, 3, and 4 h. Error bars represent the standard deviation (n = 3). Mean values with different lower-case letters are statistically different from one another (P < 0.05).
Nitric oxide (NO), a cellular inflammatory response product, was also detected (Figure 3C). After C. sakazakii infection for 0, 1, 2, 3, and 4 h, the concentrations of NO were 1.08 ± 0.51, 1.22 ± 0.30, 4.59 ± 0.87, 9.68 ± 0.50, and 14.34 ± 1.87 μM, respectively. The expressions of inflammation-related genes were shown in Figure 3E. C. sakazakii infection up-regulated the transcriptional levels of 6 inflammation-associated genes. Exposure to C. sakazakii increased the mRNA expressions of cytokines such as IL-8, IL-6, TNF-α, IL-1β, and proinflammatory factors such as iNOS, COX-2 in HBMEC in a time-dependent manner.
Cytotoxic and Apoptotic Effect of C. sakazakii on HBMEC
After C. sakazakii infection, lactate dehydrogenase (LDH) was rapidly released into the cell culture supernatant from HBMEC (Figure 4C), relative LDH release increased to 29.77% after 4 h-infection, indicating damage in the cell plasma membrane. To investigate whether C. sakazakii infection induces the cytotoxic and apoptotic effect in HBMEC, caspase-3 activity and apoptosis levels were measured. The activity of caspase-3 in C. sakazakii-induced HBMEC was shown in Figure 4B. Compared to control HBMEC, caspase-3 activity in HBMEC infected with C. sakazakii significantly increased by 145.45% (P < 0.05) after 4 h.
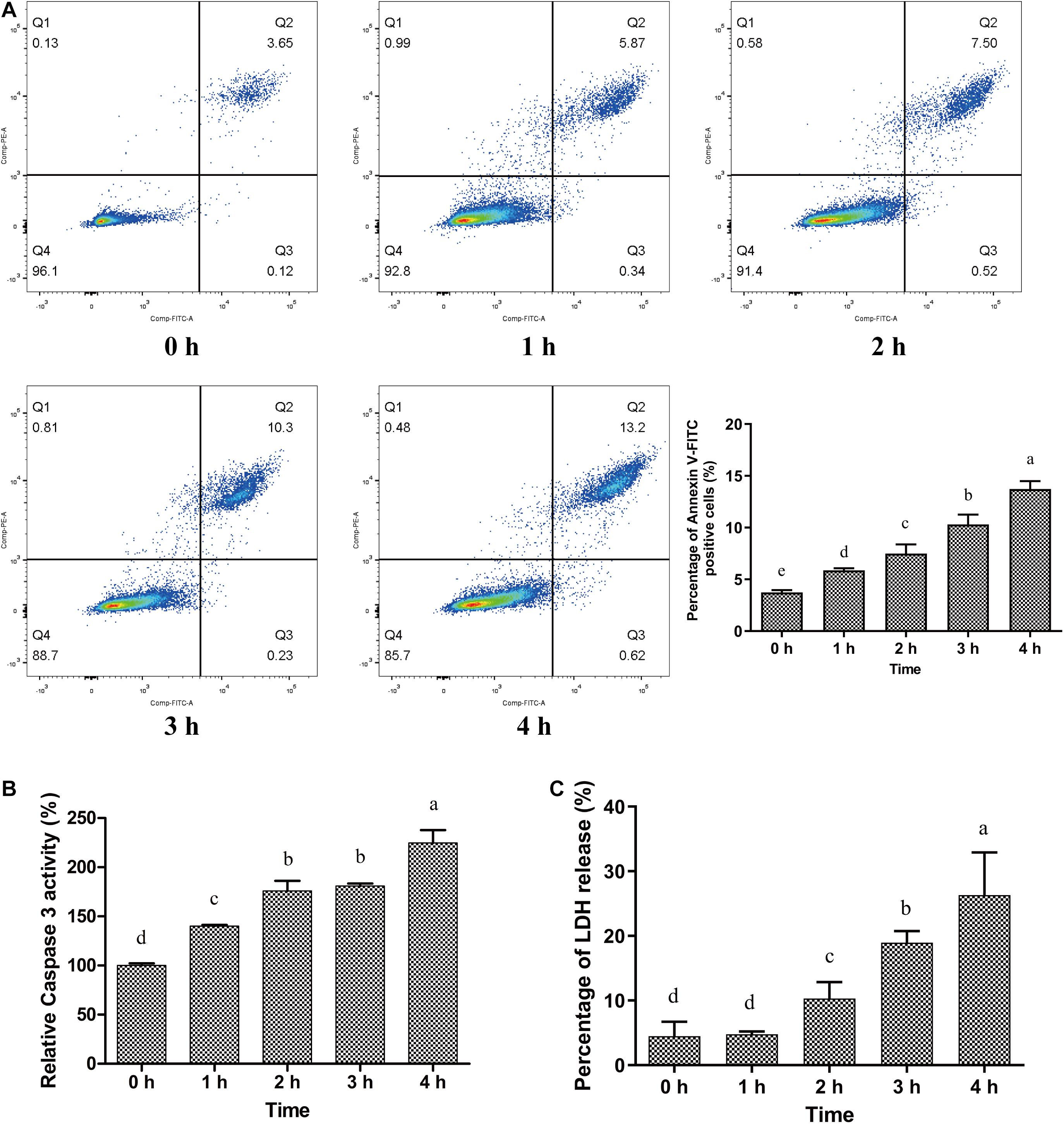
Figure 4. Cytotoxic and apoptotic effect of C. sakazakii on HBMEC. (A) C. sakazakii-infected HBMEC were stained with Annexin V-FITC/PI and analyzed with a flow cytometer. (B) Caspase-3 activity in HBMEC after C. sakazakii infection for 0, 1, 2, 3, and 4 h. (C) Relative LDH release from C. sakazakii–infected HBMEC. Error bars represent the standard deviation (n = 3), Mean values with different lower-case letters are statistically different from one another (P < 0.05).
The apoptotic HBMEC were stained with Annexin V/FITC and PI and analyzed using a flow cytometer. As shown in Figure 4A, only 3.74% of the control HBMEC were stained with Annexin V/FITC. After C. sakazakii infection for 1, 2, 3, and 4 h, the percentage of Annexin V/FITC positive HBMEC significantly increased to 5.88, 7.29, 10.31, and 13.73%, respectively (P < 0.05). Interestingly, nearly all Annexin V/FITC positive HBMEC were also stained with PI, which indicated that C. sakazakii induced late-stage apoptosis in HBMEC. Altogether, C. sakazakii strain infection caused cytotoxicity and apoptosis in HBMEC.
C. sakazakii Disrupted Tight Junction of HBMEC Monolayers
Trans-epithelial electrical resistance (TEER) of HBMEC monolayers (on a transwell system) before and after C. sakazakii infection are presented in Figure 5A. HBMEC monolayers without C. sakazakii infection demonstrated a stable TEER of around 306.75 ± 13.72 Ω cm2, which indicates the formation of a monolayer tight junction after 5 days of growth. After being challenged with C. sakazakii for 1–4 h, TEER of monolayers was reduced in a time-dependent manner and decreased to 182.60 ± 22.80 Ω cm2 after 4 h-infection (P < 0.05). The permeabilities of HBMEC monolayers, calculated based on the Dextran-FITC (4 kDa) transport, are presented in Figure 5B. Dextran permeability increased in a time-dependent manner after infection. After 4 h exposure to C. sakazakii, dextran permeability of HBMEC monolayers increased by 1.6 folds compared to control cells. In addition, protein expression of tight junction-related proteins ZO-1 and Occludin (Figure 5C) were significantly reduced in C. sakazakii infected cells. The relative expression of ZO-1 and Occludin significantly decreased to 39.54% and 56.73% of the control, respectively, in HBMEC after infection for 4 h (P < 0.05).
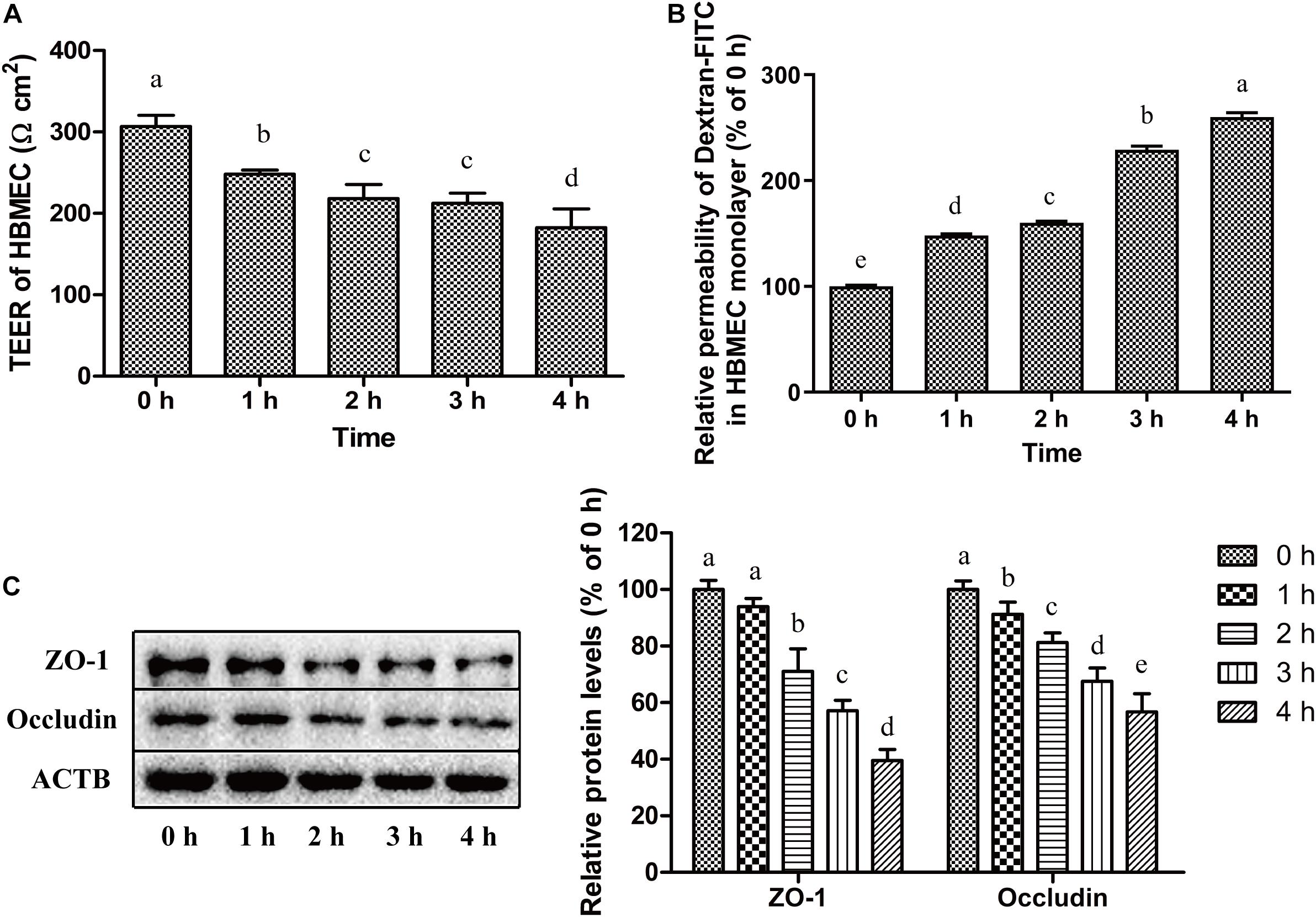
Figure 5. C. sakazakii disrupted tight junction of HBMEC monolayers. (A) The TEER of HBMEC monolayer after C. sakazakii infection for 0, 1, 2, 3, and 4 h. (B) Dextran-FITC (4 kDa) permeability in HBMEC monolayer infected with C. sakazakii. (C) The expression of tight junction-related proteins ZO-1 and Occludin in C. sakazakii-infected HBMEC were detected by Western Blot. Error bars represent the standard deviation (n = 3). Mean values with different lower-case letters are statistically different from one another (P < 0.05).
Discussion
Cronobacter sakazakii-contaminated powdered infant formulas have been associated with outbreaks of infant meningitis (Alzahrani et al., 2015). To cause meningitic infections, C. sakazakii is required to cross the blood brain barrier and gain access to the CNS (Townsend et al., 2007). Mange et al. (2006) showed that C. sakazakii isolates effectively adhered to the HEp-2 cells, Caco-2 cells, and HBMEC. In this study, four C. sakazakii strains were shown to not only adhere to and invaded HBMEC, but also traverse across HBMEC. Similarly, Escherichia coli K1 has been demonstrated to invade and translocate HBMEC monolayers (Zhang et al., 2002). It has also been proved that transcytosis is important for Streptococcus pneumoniae to cause bacteremia and meningitis (Brissac and Orihuela, 2019). Bacterial intracellular replication also plays an important role in some bacterial infections. For example, Citrobacter freundii and Neisseria meningitidis strains could survive intracellularly and replicate in HBMEC when translocating the monolayer (Badger et al., 1999; Nikulin et al., 2006). However, we observed that the number of intracellular C. sakazakii bacteria did not increase or reduce significantly in HBMEC during translocation.
Mehta et al. (2006) also reported that Mycobacterium tuberculosis could enter, internalize and translocate across human microvascular endothelial cells, but lack the ability to replicate intracellularly. Thus, the translocation of C. sakazakii in HBMEC seems not to be dependent on intracellular replication. Interestingly, C. sakazakii could survive intracellularly and replicate in macrophage cells (Shi et al.,2017a,b; Kim S. et al., 2017), and the immune-related pericytes and leucocytes are also essential for the stabilization of BBB. Further research will focus on the role of C. sakazakii intracellular survival in HBMEC monolayer during bacterial translocation.
Bacterial endocytosis and intracellular transport are important for certain pathogens to penetrate and cross the HBMEC monolayer. Loh et al. (2017) reported that Escherichia coli K1 utilized host macro-pinocytic pathways and intracellular vesicles for transcytosis in HBMEC. Using TEM, we discovered the presence of C. sakazakii–containing vacuoles in HBMEC after infection. Similarly, Giri et al. (2012) also demonstrated intracellular Cronobacter within host vacuoles in HBMEC during translocation. We discovered that C. sakazakii stimulated the expression of the transmembrane glycoprotein CD44 (an adhesion receptor for pathogen) in HBMEC, indicating the transmembrane invasion and transport of the pathogen. Similarly, a previous study showed that Shigella virulence proteins bound to CD44 on host cytomembrane for bacterial invasion (Skoudy et al., 2000).
Cronobacter sakazakii infection up-regulated the levels of endocytotic vesicle formation and maturation marker proteins Rab5 and Rab7 in HBMEC. It has been proved that Rab5 and Rab7 positively contributed to bacterial invasion into host cells (Mottola et al., 2014). In this study, it was shown that intracellular C. sakazakii existed in the Rab5 and Rab7 positive vacuole compartment. Previous studies have shown that rab5 and rab7 played an essential role in the trafficking of E. coli-containing vacuoles and intracellular bacterial survival in HBMEC (Kim et al., 2003; Mu et al., 2016). It can be inferred that endocytosis is an important strategy for C. sakazakii to translocate across HBMEC.
After C. sakazakii infection, lysosomal marker LAMP2 levels increased and relocalized, indicating that endosomes containing C. sakazakii mature into endolysosomes. The previous study demonstrated that Trypanosoma cruzi invasion into human epithelial HeLa cells was accomplished through recognition of gp82 by its receptor LAMP2 protein (Rodrigues et al., 2019). Kuhbacher et al. (2018) showed that when Listeria monocytogenes invaded epithelial cells, the lysosomal-associated membrane proteins LAMP1 and LAMP2 are translocated to the cellular surface, and the activation of several signaling pathways promoted escape of bacteria from endosomes and transcytosis of L. monocytogenes. As late endosomes/lysosomes are the major donor compartments of LAMP2, these organelles are likely to participate in C. sakazakii invasion and translocation in HBMEC. The lysosomal enzyme Cathepsin L is involved in cellular invasion and vesicle degradation (Xiong et al., 2017). Cathepsin L was activated in swine dendritic cells (DCs) and B cells after Mycoplasma hyopneumoniae infection, contributing to the inflammatory responses induced by pathogens (Zhang et al., 2019). In this work, the Cathepsin L in HBMEC were not activated by C. sakazakii. Similarly, Helicobacter pylori infection caused inflammation in gastric mucosa, but Cathepsin L expression in gastric mucosa epithelial cells was still at very low levels during Helicobacter pylori infection (Buhling et al., 2004). Pathogen-induced inflammatory signaling pathway activation and cytokines expressions in BBB-related cells mediated its intracellular transcytosis processes (Miller et al., 2005). We showed that C. sakazakii could stimulate NF-κB p65 nuclear translocation and TLR4 expression, suggesting NF-κB inflammation pathway activation. Some pathogens, like Neisseria meningitidis and Streptococcus pneumoniae could express microbial-associated molecular patterns, which are recognized by pattern recognition receptors (such as TLR4) on the cell surface. The binding recruits a cohort of signaling molecules and drives signaling pathway activation, which promotes bacterial translocation (Telleria-Orriols et al., 2014). It could be inferred that, once C. sakazakii interacts with TLR4, inflammation-related proteins were phosphorylated and disassociated, activating the NF-κB pathway and inducing nitric oxide release. NO regulated cellular inflammation and permeability.
A previous study has identified that lipoteichoic acid in Gram-positive pathogens activated glial cells to produce nitric oxide and pro-inflammatory cytokines, which contributed to the disruption of BBB structure and function in a concentration- and time-dependent manner (Boveri et al., 2006). An increase in the transcription of IL-8, IL-6, TNF-α, IL-1β, iNOS, and COX-2- mRNA was also noted. Previous studies showed that cytokines IL-6, TNF-α, and IL-1β and some other inflammatory factors regulated HBMEC apoptosis or monolayer tight junction disruption, and increased BBB permeability (Wong et al., 2004; Poller et al., 2010; Barichello et al., 2011). We observed that C. sakazakii induced inflammatory responses in HBMEC, and proinflammatory cytokines releases are likely to facilitate disruption of HBMEC monolayers integrity. It was reported that the different types of bacteria could interact with host BMEC in different ways, such as inflammatory responses or molecular receptor binding, which contributes to bacterial translocation across host BMEC monolayers (Al-Obaidi and Desa, 2018).
Castro-Garza et al. (2012) reported that bacterial cytotoxicity is dependent on strain virulence and host intracellular interaction (Castro-Garza et al., 2012). In our study, we found that C. sakazakii caused a cytotoxic response, including LDH release and caspase-3 activation. Lactate dehydrogenase (LDH), a stable cytoplasmic enzyme, is a marker for cytomembrane damage and cellular permeability increase. It was reported that Pseudomonas aeruginosa stimulated host cell LDH release, contributing to bacterial intracellular transcytosis (Elsahn et al., 2020). In this work, it was observed that LDH was rapidly released after C. sakazakii infection. Meanwhile, the pro-apoptotic caspase-3 activity and Annexin V-FITC positive apoptotic cells in infected HBMEC increased. These observations are similar to Listeria monocytogenes invasion into the host epithelial cells. L. monocytogenes-induced cell stress responses and apoptosis facilitated bacterial invasion and translocation in epithelial cells (Dos Santos et al., 2011). Inducing host cell apoptosis is also an essential pathway for Shigella flexneri invasion in hepatocytes and translocation in the Caco-2 monolayer barrier (Lima et al., 2013).
Additionally, cytotoxicity and apoptosis are closely associated with inflammatory responses. Previous research found that pro-inflammatory cytokines, like TNF-α, activated caspase-3 and cell apoptosis (Zhao et al., 2016). Interestingly, we observed an increase in mRNAs of TNF-α, IL-6, and caspase-3 activations in C. sakazakii-infected HBMEC. We speculated that pro-inflammatory cytokines might stimulate the activation of caspase-3 and subsequently induce HBMEC apoptosis, leading to C. sakazakii translocation. It was noted that C. sakazakii may directly induce late stage apoptosis in HBMEC. These results are similar to methylglyoxal-induced HBMEC injury and apoptosis (Zhou et al., 2015). It can be inferred that the bacteriotoxin released by C. sakazakii may induce apoptosis in HBMEC directly, following the disruption of HBEMC monolayers. Liu Q. et al. (2012) reported that Cronobacter sakazakii isolates induced the increase of intestinal epithelial cells monolayer permeability and cell apoptosis during bacterial translocation through intestinal barriers. Li et al. (2010) and Liu D. X. et al. (2012) justified C. sakazakii could stimulate cPLA(2)α-regulated Akt signaling pathway activation, PI3K-mediated actin filaments rearrangements, and cell apoptosis in HBMEC, which were required for C. sakazakii invasion in HBMEC.
Disruption of the BBB tight junction structure is one of the possible mechanisms for bacterial translocation. After exposure to E. coli for a long period of time, a widening of tight-junctions and formation of holes were observed in the Caco-2 monolayer, leading to a lower TEER and increased Dextran-FITC permeability (Yuan et al., 2020). In our study, C. sakazakii decreased the TEER and increased Dextran-FITC permeability. Similarly, Pseudomonas aeruginosa exoprotein induced mucosal barrier disruption evidenced by increased permeability of Dextran-FITC and decreased TEER after 4 h of challenge (Li et al., 2019). A reduction was observed in the protein levels of ZO-1 and Occludin among C. sakazakii-infected HBMEC. It was also proved that certain bacteria, like Group B Streptococcus agalactiae, disrupted the tight junction proteins claudin-5, Occludin, and ZO-1, which was necessary for bacterial translocation in BBB (Kim B. J. et al., 2017; Jiao et al., 2011). In a rat model, the reduced levels of ZO-1/Occludin increased the permeability of BBB and facilitated transcytosis (Wang et al., 2019). Taken together, C. sakazakii infection resulted in increased cellular permeability and disruption of the tight junction, which contributes to bacterial translocation across HBMEC monolayers.
In summary, C. sakazakii strains could invade and survive in HBMEC, and translocate across HBMEC monolayers. Endocytosis vesicles were observed in C. sakazakii- invaded HBMEC. C. sakazakii also induced inflammatory responses and apoptosis in HBMEC. Meanwhile, the permeability of HBMEC monolayers increased and the tight junction was disrupted after C. sakazakii infection. These findings reveal the possible transcellular and paracellular pathways for C. sakazakii ATCC 29544 to translocate across HBMEC monolayers. However, this in vitro study was performed mainly with one virulent strain and one cell line forming BBB. Future studies will test more C. sakazakii strains in in vitro and in vivo models to discover detailed mechanisms by which C. sakazakii translocates through HBMEC monolayers and BBB.
Data Availability Statement
The raw data supporting the conclusions of this article will be made available by the authors, without undue reservation.
Author Contributions
TJ and XX conceived and designed the experiments and wrote the manuscript. TJ, NG, and YD performed the experiments. XZ and JL analyzed the data. NG and XZ contributed reagents, materials, and analysis tools. All authors contributed to the article and approved the submitted version.
Funding
This work was supported partly by the National Natural Science Foundation of China (31772084), Shaanxi Key Research and Development project (2019SF-259), Science and Technology Research Program of the Liaoning Department of Education (J2020044), and LiaoNing Revitalization Talents Program (XLYC1807220).
Conflict of Interest
The authors declare that the research was conducted in the absence of any commercial or financial relationships that could be construed as a potential conflict of interest.
References
Abbott, N. J., Patabendige, A. A. K., Dolman, D. E. M., Yusof, S. R., and Begley, D. J. (2010). Structure and function of the blood-brain barrier. Neurobiol. Dis. 37, 13–25. doi: 10.1016/j.nbd.2009.07.030
Almajed, F. S., and Forsythe, S. J. (2016). Cronobacter sakazakii clinical isolates overcome host barriers and evade the immune response. Microb. Pathogen. 90, 55–63. doi: 10.1016/j.micpath.2015.11.014
Al-Obaidi, M. M. J., and Desa, M. N. M. (2018). Mechanisms of blood brain barrier disruption by different types of bacteria, and bacterial-host interactions facilitate the bacterial pathogen invading the brain. Cell. Mol. Neurobiol. 38, 1349–1368. doi: 10.1007/s10571-018-0609-2
Alzahrani, H., Winter, J., Boocock, D., De Girolamo, L., and Forsythe, S. J. (2015). Characterization of outer membrane vesicles from a neonatal meningitic strain of Cronobacter sakazakii. FEMS Microbiol. Lett. 362:fnv085. doi: 10.1093/femsle/fnv085
Amalaradjou, M. A. R., Kim, K. S., and Venkitanarayanan, K. (2014). Sub-inhibitory concentrations of trans-cinnamaldehyde attenuate virulence in Cronobacter sakazakii in vitro. Int. J. Mol. Sci. 15, 8639–8655. doi: 10.3390/ijms15058639
Badger, J. L., Stins, M. F., and Kim, K. S. (1999). Citrobacter freundii invades and replicates in human brain microvascular endothelial cells. Infect. Immun. 67, 4208–4215. doi: 10.1128/Iai.67.8.4208-4215.1999
Barichello, T., Pereira, J. S., Savi, G. D., Generoso, J. S., Cipriano, A. L., Silvestre, C., et al. (2011). A kinetic study of the cytokine/chemokines levels and disruption of blood-brain barrier in infant rats after pneumococcal meningitis. J. Neuroimmunol. 233, 12–17. doi: 10.1016/j.jneuroim.2010.10.035
Benmimoun, B., Papastefanaki, F., Périchon, B., Segklia, K., and Spéder, P. (2020). An original infection model identifies host lipoprotein import as a route for blood-brain barrier crossing. Nat. Commun. 11:6106. doi: 10.1038/s41467-020-19826-2
Boveri, M., Kinsner, A., Berezowski, V., Lenfant, A. M., Draing, C., Dehouck, M. P., et al. (2006). Highly purified lipoteichoic acid from Gram-positive bacteria induces in vitro blood-brain barrier disruption through GLIA activation: role of pro-inflammatory cytokines and nitric oxide. Neuroscience 137, 1193–1209. doi: 10.1016/j.neuroscience.2005.10.011
Brissac, T., and Orihuela, C. J. (2019). In vitro adhesion, invasion, and transcytosis of Streptococcus pneumoniae with host cells. Methods Mol. Biol. 1968, 137–146. doi: 10.1007/978-1-4939-9199-0_12
Brozek, W., Bises, G., Fabjani, G., Cross, H. S., and Peterlik, M. (2008). Clone-specific expression, transcriptional regulation, and action of interleukin-6 in human colon carcinoma cells. BMC Cancer 8:13. doi: 10.1186/1471-2407-8-13
Buhling, F., Peitz, U., Kruger, S., Kuster, D., Vieth, M., Gebert, I., et al. (2004). Cathepsins K, L, B, X and W are differentially expressed in normal and chronically inflamed gastric mucosa. Biol. Chem. 385, 439–445. doi: 10.1515/BC.2004.051
Castro-Garza, J., Swords, W. E., Karls, R. K., and Quinn, F. D. (2012). Dual mechanism for Mycobacterium tuberculosis cytotoxicity on lung epithelial cells. Can. J. Microbiol. 58, 909–916. doi: 10.1139/W2012-067
Chen, J., Sun, L., Ding, G. B., Chen, L., Jiang, L., Wang, J., et al. (2019). Oxygen-glucose deprivation/reoxygenation induces human brain microvascular endothelial cell hyperpermeability via VE-cadherin internalization: roles of RhoA/ROCK2. J. Mol. Neurosci. 69, 49–59. doi: 10.1007/s12031-019-01326-8
Dos Santos, S. A., de Andrade, D. R., and de Andrade, D. R. (2011). TNF-alpha production and apoptosis in hepatocytes after Listeria monocytogenes and Salmonella typhimurium invasion. Rev. Inst. Med. Trop. Sao Paulo 53, 107–112. doi: 10.1590/S0036-46652011000200009
Elkhawaga, A. A., Hetta, H. F., Osman, N. S., Hosni, A., and El-Mokhtar, M. A. (2020). Emergence of Cronobacter sakazakii in cases of neonatal sepsis in Upper Egypt: first report in North Africa. Front. Microbiol. 11:215. doi: 10.3389/fmicb.2020.00215
Elsahn, A., Cendra, M. D. M., Humbert, M. V., Christodoulides, M., and Hossain, P. (2020). Pseudomonas aeruginosa host-pathogen interactions in human corneal infection models. J. EuCornea 7, 8–16. doi: 10.1016/j.xjec.2020.02.002
Giri, C. P., Shima, K., Tall, B. D., Curtis, S., Sathyamoorthy, V., Hanisch, B., et al. (2012). Cronobacter spp. (previously Enterobacter sakazakii) invade and translocate across both cultured human intestinal epithelial cells and human brain microvascular endothelial cells. Microb. Pathog. 52, 140–147. doi: 10.1016/j.micpath.2011.10.003
Hunter, C. J., Petrosyan, M., Ford, H. R., and Prasadarao, N. V. (2008). Enterobacter sakazakii: an emerging pathogen in infants and neonates. Surg. Infect. 9, 533–539. doi: 10.1089/sur.2008.006
Jiao, H. X., Wang, Z. H., Liu, Y. H., Wang, P., and Xue, Y. X. (2011). Specific role of tight junction proteins claudin-5, Occludin, and ZO-1 of the blood-brain barrier in a focal cerebral ischemic insult. J. Mol. Neurosci. 44, 130–139. doi: 10.1007/s12031-011-9496-4
Karassek, S., Starost, L., Solbach, J., Greune, L., Sano, Y., Kanda, T., et al. (2015). Pertussis toxin exploits specific host cell signaling pathways for promoting invasion and translocation of Escherichia coli K1 RS218 in human brain-derived microvascular endothelial cells. J. Biol. Chem. 290, 24835–24843. doi: 10.1074/jbc.M115.650101
Kaulmann, A., Legay, S., Schneider, Y. J., Hoffmann, L., and Bohn, T. (2016). Inflammation related responses of intestinal cells to plum and cabbage digesta with differential carotenoid and polyphenol profiles following simulated gastrointestinal digestion. Mol. Nutr. Food Res. 60, 992–1005. doi: 10.1002/mnfr.201500947
Kim, B. J., Bee, O. B., Mcdonagh, M. A., Stebbins, M. J., and Shusta, E. V. (2017). Modeling group B Streptococcus and blood-brain barrier interaction by using induced pluripotent stem cell-derived brain endothelial cells. mSphere 2:e00398-17. doi: 10.1128/mSphere.00398-17
Kim, K. J., Elliott, S. J., Di Cello, F., Stins, M. F., and Kim, K. S. (2003). The K1 capsule modulates trafficking of E coli-containing vacuoles and enhances intracellular bacterial survival in human brain microvascular endothelial cells. Cell. Microbiol. 5, 245–252. doi: 10.1046/j.1462-5822.2003.t01-1-00271.x
Kim, S., Kim, Y. T., Yoon, H., Lee, J. H., and Ryu, S. (2017). The complete genome sequence of Cronobacter sakazakii ATCC 29544(T), a food-borne pathogen, isolated from a child’s throat. Gut Pathog. 9, 1–7. doi: 10.1186/S13099-016-0150-0
Kuhbacher, A., Novy, K., Quereda, J. J., Sachse, M., Moya-Nilges, M., Wollscheid, B., et al. (2018). Listeriolysin O-dependent host surfaceome remodeling modulates Listeria monocytogenes invasion. Pathog. Dis. 76:fty082. doi: 10.1093/femspd/fty082
Lemichez, E., Lecuit, M., Nassif, X., and Bourdoulous, S. (2010). Breaking the wall: targeting of the endothelium by pathogenic bacteria. Nat. Rev. Microbiol. 8, 93–104. doi: 10.1038/nrmicro2269
Lepuschitz, S., Ruppitsch, W., Pekard-Amenitsch, S., Forsythe, S. J., Cormican, M., Mach, R. L., et al. (2019). Multicenter study of Cronobacter sakazakii infections in humans, Europe, 2017. Emerg. Infect. Dis. 25, 515–522. doi: 10.3201/eid2503.181652
Li, J., Ramezanpour, M., Fong, S. A., Cooksley, C., Murphy, J., Suzuki, M., et al. (2019). Pseudomonas aeruginosa exoprotein-induced barrier disruption correlates with elastase activity and marks chronic Rhinosinusitis severity. Front. Cell Infect. Microbiol. 9:38. doi: 10.3389/Fcimb.2019.00038
Li, Q. A., Zhao, W. D., Zhang, K., Fan, W. G., Hu, Y., Wu, S. H., et al. (2010). PI3K-dependent host cell actin rearrangements are required for Cronobacter sakazakii invasion of human brain microvascular endothelial cells. Med. Microbiol. Immun. 199, 333–340. doi: 10.1007/s00430-010-0168-8
Lima, C. B. C., dos Santos, S. A., and de Andrade, D. R. (2013). Hypoxic Stress, Hepatocytes and Caco-2 viability and susceptibility to Shigella flexneri invasion. Rev. Inst. Med. Trop. Sao Paulo 55, 341–346. doi: 10.1590/S0036-46652013000500008
Liu, D. X., Zhao, W. D., Fang, W. G., and Chen, Y. H. (2012). cPLA(2)alpha-mediated actin rearrangements downstream of the Akt signaling is required for Cronobacter sakazakii invasion into brain endothelial cells. Biochem. Biophys. Res. Commun. 417, 925–930. doi: 10.1016/j.bbrc.2011.11.079
Liu, Q., Mittal, R., Emami, C. N., Iversen, C., Ford, H. R., and Prasadarao, N. V. (2012). Human isolates of Cronobacter sakazakii bind efficiently to intestinal epithelial cells in vitro to induce monolayer permeability and apoptosis. J. Surg. Res. 176, 437–447. doi: 10.1016/j.jss.2011.10.030
Loh, L. N., McCarthy, E. M. C., Narang, P., Khan, N. A., and Ward, T. H. (2017). Escherichia coli K1 utilizes host macropinocytic pathways for invasion of brain microvascular endothelial cells. Traffic 18, 733–746. doi: 10.1111/tra.12508
Lu, X. T., Qi, X. L., Yi, X. L., Jian, Z., and Gao, T. W. (2019). Transcellular traversal of the blood-brain barrier by the pathogenic Propionibacterium acnes. J. Cell. Biochem. 120, 8457–8465. doi: 10.1002/jcb.28132
Mange, J. P., Stephan, R., Borel, N., Wild, P., Kim, K. S., Pospischil, A., et al. (2006). Adhesive properties of Enterobacter sakazakii to human epithelial and brain microvascular endothelial cells. BMC Microbiol. 6:58. doi: 10.1186/1471-2180-6-58
McLoughlin, A., Rochfort, K. D., McDonnell, C. J., Kerrigan, S. W., and Cummins, P. M. (2017). Staphylococcus aureus-mediated blood-brain barrier injury: an in vitro human brain microvascular endothelial cell model. Cell. Microbiol. 19:12664. doi: 10.1111/cmi.12664
Mehta, P. K., Karls, R. K., White, E. H., Ades, E. W., and Quinn, F. D. (2006). Entry and intracellular replication of Mycobacterium tuberculosis in cultured human microvascular endothelial cells. Microb. Pathog. 41, 119–124. doi: 10.1016/j.micpath.2006.05.002
Miller, F., Fenart, L., Landry, V., Coisne, C., and Buée-Scherrer, V. (2005). The MAP kinase pathway mediates transcytosis induced by TNF-alpha in an in vitro blood-brain barrier model. Eur. J. Neurosci. 22, 835–844. doi: 10.1111/j.1460-9568.2005.04273.x
Mottola, G., Boucherit, N., Trouplin, V., Barry, A. O., Soubeyran, P., Mege, J. L., et al. (2014). Tropheryma whipplei, the agent of Whipple’s disease, affects the early to late phagosome transition and survives in a Rab5-and Rab7-positive compartment. PLoS One 9:e89367. doi: 10.1371/journal.pone.0089367
Mu, R., Cutting, A. S., Del Rosario, Y., Villarino, N., Stewart, L., Weston, T. A., et al. (2016). Identification of CiaR regulated genes that promote group B streptococcal virulence and interaction with brain endothelial cells. PLoS One 11:e0153891. doi: 10.1371/journal.pone.0153891
Mu, R., Kim, B. J., Paco, C., Del Rosario, Y., Courtney, H. S., and Doran, K. S. (2014). Identification of a Group B Streptococcal fibronectin binding protein, SfbA, that contributes to invasion of brain endothelium and development of meningitis. Infect. Immun. 82, 2276–2286. doi: 10.1128/Iai.01559-13
Mullane, N. R., Iversen, C., Healy, B., Walsh, C., Whyte, P., Wall, P. G., et al. (2007). Enterobacter sakazakii an emerging bacterial pathogen with implications for infant health. Minerva Pediatr. 59, 137–148.
Neuhaus, W., Piontek, A., Protze, J., Eichner, M., Mahringer, A., Subileau, E. A., et al. (2018). Reversible opening of the blood-brain barrier by claudin-5-binding variants of Clostridium perfringens enterotoxin’s claudin-binding domain. Biomaterials 161, 129–143. doi: 10.1016/j.biomaterials.2018.01.028
Nikulin, J., Panzner, U., Frosch, M., and Schubert-Unkmeir, A. (2006). Intracellular survival and replication of Neisseria meningitidis in human brain microvascular endothelial cells. Int. J. Med. Microbiol. 296, 553–558. doi: 10.1016/j.ijmm.2006.06.006
Ogrodzki, P., and Forsythe, S. (2015). Capsular profiling of the Cronobacter genus and the association of specific Cronobacter sakazakii and C. malonaticus capsule types with neonatal meningitis and necrotizing enterocolitis. BMC Genomics 16:758. doi: 10.1186/S12864-015-1960-Z
Patrick, M. E., Mahon, B. E., Greene, S. A., Rounds, J., Cronquist, A., Wymore, K., et al. (2014). Incidence of Cronobacter spp. infections, United States, 2003-2009. Emerg. Infect. Dis. 20, 1520–1523. doi: 10.3201/eid2009.140545
Poller, B., Drewe, J., Krahenbuhl, S., Huwyler, J., and Gutmann, H. (2010). Regulation of BCRP (ABCG2) and P-Glycoprotein (ABCB1) by cytokines in a model of the human blood-brain barrier. Cell. Mol. Neurobiol. 30, 63–70. doi: 10.1007/s10571-009-9431-1
Rodrigues, J. P. F., Onofre, T. S., Barbosa, B. C., Ferreira, E. R., Bonfim-Melo, A., and Yoshida, N. (2019). Host cell protein LAMP-2 is the receptor for Trypanosoma cruzi surface molecule gp82 that mediates invasion. Cell. Microbiol. 21:e13003. doi: 10.1111/cmi.13003
Ryan, D., Mukherjee, M., Nayak, R., Dutta, R., and Suar, M. (2018). Biological and regulatory roles of acid-induced small RNA RyeC in Salmonella typhimurium. Biochimie 150, 48–56. doi: 10.1016/j.biochi.2018.05.001
Sanders, M. S., Van Well, G. T. J., Ouburg, S., Morre, S. A., and Van Furth, A. M. (2011). Genetic variation of innate immune response genes in invasive pneumococcal and meningococcal disease applied to the pathogenesis of meningitis. Genes Immun. 12, 321–334. doi: 10.1038/gene.2011.20
Schubert-Unkmeir, A., Konrad, C., Slanina, H., Czapek, F., Hebling, S., and Frosch, M. (2010). Neisseria meningitidis induces brain microvascular endothelial cell detachment from the matrix and cleavage of Occludin: a role for MMP-8. PLoS Pathog. 6:e1000874. doi: 10.1371/journal.ppat.1000874
Shi, C., Sun, Y., Liu, Z. Y., Guo, D., Sun, H. H., Sun, Z., et al. (2017a). Inhibition of Cronobacter sakazakii virulence factors by citral. Sci. Rep. 7:43243. doi: 10.1038/srep43243
Shi, C., Yan, C. H., Sui, Y., Sun, Y., Guo, D., Chen, Y. F., et al. (2017b). Thymoquinone inhibits virulence related traits of Cronobacter sakazakii ATCC 29544 and has anti-biofilm formation potential. Front. Microbiol. 8:2220. doi: 10.3389/fmicb.2018.00290
Skoudy, A., Mounier, J., Aruffo, A., Ohayon, H., Gounon, P., Sansonetti, P., et al. (2000). CD44 binds to the Shigella IpaB protein and participates in bacterial invasion of epithelial cells. Cell. Microbiol. 2, 19–33. doi: 10.1046/j.1462-5822.2000.00028.x
Tall, B. D., Chen, Y., Yan, Q. Q., Gopinath, G. R., Grim, C. J., Jarvis, K. G., et al. (2014). Cronobacter: an emergent pathogen causing meningitis to neonates through their feeds. Sci. Prog. 97, 154–172. doi: 10.3184/003685014X13994743930498
Telleria-Orriols, J. J., Garcia-Salido, A., Varillas, D., Serrano-Gonzalez, A., and Casado-Flores, J. (2014). TLR2-TLR4/CD14 polymorphisms and predisposition to severe invasive infections by Neisseria meningitidis and Streptococcus pneumoniae. Med. Intensiva 38, 356–362. doi: 10.1016/j.medin.2013.08.006
Townsend, S. M., Hurrell, E., Gonzalez-Gomez, I., Lowe, J., Frye, J. G., Forsythe, S., et al. (2007). Enterobacter sakazakii invades brain capillary endothelial cells, persists in human macrophages influencing cytokine secretion and induces severe brain pathology in the neonatal rat. Microbiology (Reading) 153, 3538–3547. doi: 10.1099/mic.0.2007/009316-0
Van Sorge, N. M., and Doran, K. S. (2012). Defense at the border: the blood-brain barrier versus bacterial foreigners. Future Microbiol. 7, 383–394. doi: 10.2217/FMB.12.1
Wang, H. Y., Li, T., Zhao, L. L., Sun, M., Jian, Y. T., Liu, J., et al. (2019). Dynamic effects of ioversol on the permeability of the blood-brain barrier and the expression of ZO-1/Occludin in rats. J. Mol. Neurosci. 68, 295–303. doi: 10.1007/s12031-019-01305-z
Weng, M. Q., Ganguli, K., Zhu, W. S., Shi, H. N., and Walker, W. A. (2014). Conditioned medium from Bifidobacteria infantis protects against Cronobacter sakazakii-induced intestinal inflammation in newborn mice. Am. J. Physiol. Gastrointest. Liver Physiol. 306, G779–G787. doi: 10.1152/ajpgi.00183.2013
Wong, D., Dorovini-Zis, K., and Vincent, S. R. (2004). Cytokines, nitric oxide, and cGMP modulate the permeability of an in vitro model of the human blood-brain barrier. Exp. Neurol. 190, 446–455. doi: 10.1016/j.expneurol.2004.08.008
Wu, S. J., Wu, M., Tian, D. C., Qiu, L. Q., and Li, T. T. (2020). Effects of polystyrene microbeads on cytotoxicity and transcriptomic profiles in human Caco-2 cells. Environ. Toxicol. 35, 495–506. doi: 10.1002/tox.22885
Xiong, Y. J., Ji, W. J., Fei, Y., Zhao, Y. F., Wang, L., Wang, W. J., et al. (2017). Cathepsin L is involved in X-ray-induced invasion and migration of human glioma U251 cells. Cell. Signal. 29, 181–191. doi: 10.1016/j.cellsig.2016.10.012
Ye, Y. W., Li, H., Ling, N., Han, Y. J., Wu, Q. P., Xu, X. K., et al. (2016). Identification of potential virulence factors of Cronobacter sakazakii isolates by comparative proteomic analysis. Int. J. Food Microbiol. 217, 182–188. doi: 10.1016/j.ijfoodmicro.2015.08.025
Yuan, L., Van der Mei, H. C., Busscher, H. J., and Peterson, B. W. (2020). Two-stage interpretation of changes in TEER of intestinal epithelial layers protected by adhering Bifidobacteria during E. coli challenges. Front. Microbiol. 11:599555. doi: 10.3389/Fmicb.2020.599555
Zhang, G. W., Khan, N. A., Kim, K. J., Stins, M., and Kim, K. S. (2002). Transforming growth factor-beta increases Escherichia coli K1 adherence, invasion, and transcytosis in human brain microvascular endothelial cells. Cell Tissue Res. 309, 281–286. doi: 10.1007/s00441-002-0549-4
Zhang, N., Gao, P., Yin, B., Li, J. H., Wu, T., Kuang, Y., et al. (2019). Cathepsin L promotes secretory IgA response by participating in antigen presentation pathways during Mycoplasma Hyopneumoniae infection. PLoS One 14:e0215408. doi: 10.1371/journal.pone.0215408
Zhao, H., Zhang, M., Zhou, F. X., Cao, W., Bi, L. L., and Xie, Y. H. (2016). Cinnamaldehyde ameliorates LPS-induced cardiac dysfunction via TLR4-NOX4 pathway: the regulation of autophagy and ROS production. J. Mol. Cell. Cardiol. 101, 11–24. doi: 10.1016/j.yjmcc.2016.10.017
Keywords: Cronobacter sakazakii, human brain microvascular endothelial cells, transcytosis, apoptosis, tight junction
Citation: Jin T, Guan N, Du Y, Zhang X, Li J and Xia X (2021) Cronobacter sakazakii ATCC 29544 Translocated Human Brain Microvascular Endothelial Cells via Endocytosis, Apoptosis Induction, and Disruption of Tight Junction. Front. Microbiol. 12:675020. doi: 10.3389/fmicb.2021.675020
Received: 05 March 2021; Accepted: 23 April 2021;
Published: 07 June 2021.
Reviewed by:
Ariadnna Cruz-Córdova, Federico Gómez Children’s Hospital, MexicoPhilippe V. Afonso, Institut Pasteur, France
Copyright © 2021 Jin, Guan, Du, Zhang, Li and Xia. This is an open-access article distributed under the terms of the Creative Commons Attribution License (CC BY). The use, distribution or reproduction in other forums is permitted, provided the original author(s) and the copyright owner(s) are credited and that the original publication in this journal is cited, in accordance with accepted academic practice. No use, distribution or reproduction is permitted which does not comply with these terms.
*Correspondence: Xiaodong Xia, foodscixiaodong@yahoo.com