- 1CAS Key Laboratory of Green Process and Engineering, State Key Laboratory of Biochemical Engineering, Institute of Process Engineering, Chinese Academy of Sciences, Beijing, China
- 2College of Chemical Engineering, University of Chinese Academy of Sciences, Beijing, China
- 3Biofilm Centre, Aquatic Microbiology Department, Faculty of Chemistry, University Duisburg-Essen, Essen, Germany
- 4Department of Chemical Engineering, Wah Engineering College, University of Wah, Wah Cantt, Pakistan
- 5Chemistry and Chemical Engineering Guangdong Laboratory, Shantou, China
The widespread use of commercial polymers composed of a mixture of polylactic acid and polyethene terephthalate (PLA-PET) in bottles and other packaging materials has caused a massive environmental crisis. The valorization of these contaminants via cost-effective technologies is urgently needed to achieve a circular economy. The enzymatic hydrolysis of PLA-PET contaminants plays a vital role in environmentally friendly strategies for plastic waste recycling and degradation. In this review, the potential roles of microbial enzymes for solving this critical problem are highlighted. Various enzymes involved in PLA-PET recycling and bioconversion, such as PETase and MHETase produced by Ideonella sakaiensis; esterases produced by Bacillus and Nocardia; lipases produced by Thermomyces lanuginosus, Candida antarctica, Triticum aestivum, and Burkholderia spp.; and leaf-branch compost cutinases are critically discussed. Strategies for the utilization of PLA-PET’s carbon content as C1 building blocks were investigated for the production of new plastic monomers and different value-added products, such as cyclic acetals, 1,3-propanediol, and vanillin. The bioconversion of PET-PLA degradation monomers to polyhydroxyalkanoate biopolymers by Pseudomonas and Halomonas strains was addressed in detail. Different solutions to the production of biodegradable plastics from food waste, agricultural residues, and polyhydroxybutyrate (PHB)-accumulating bacteria were discussed. Fuel oil production via PLA-PET thermal pyrolysis and possible hybrid integration techniques for the incorporation of thermostable plastic degradation enzymes for the conversion into fuel oil is explained in detail.
Introduction: Plastic Waste, an Enormous Ecological Emergency
Global plastic production, including additives and fillers, has increased dramatically since the 1950s. Between 1950 and 2019, 8,100 Mt. of plastic was produced worldwide. Plastic production grew from 2 Mt. in 1950 to 450 Mt. in 2019 (Shanmugam et al., 2020). Currently, only 4 Mt. of biobased or biodegradable plastics are produced globally. They are used in almost every industry and household for disposable items such as wraps, plates, cups, and spoons (Song et al., 2009). Synthetic plastics are traditionally made from refined petroleum products in a controlled environment. These heavy crude oil-based plastics have the potential to contribute to the loss of resources, climate change, and greenhouse gas emissions (Brandt, 2011). However, the microbial degradation of plastic is determined by chemical bonding, rather than the source monomers (Blanco et al., 2020). As common plastics are composed of multiple repeats of small monomer units, they have a high molecular weight. Biological resources can be used to obtain polymers, including polyethylene (PE), polypropylene (PP), polyvinyl chloride (PVC), and polyethene terephthalate (PET), which all start with similar monomers. These plastics are not biodegradable, despite including biobased components (Alshehrei, 2017). For example, plastic materials are made of high molecular weight polymers and additives. They are indispensable in many applications owing to their versatility; they are used in the packaging, construction, automotive, electronic, agriculture, home, leisure, and sports industries, among others (Zhang et al., 2018; PlasticsEurope, 2019).
The demand for readily available plastic items continues to grow as the world’s population grows and lifestyles change. Thus, synthetic plastic manufacturing dominates global industries. Globally, 350–400 million tons of plastic waste is generated each year (Roser, 2018), with this value predicted to be 3-fold higher by 2050 (Macarthur et al., 2016). As present, the largest global waste challenges are food waste and plastic waste (Su et al., 2020a; Nanda and Berruti, 2021). Contamination resulting from plastic use is a global problem that occurs in all land areas and oceans, with an estimated 580,000 pieces of plastic per square kilometer (Wilcox et al., 2015). A more recent United Nations-commissioned study discovered that over 600 species of organisms, ranging from bacteria to whales, have been damaged by marine plastic waste; most of those organisms became ill after ingesting the plastic (Bhuyan et al., 2021). The most common food packaging products are high-density polyethylene (HDPE) flexible plastic packaging, polystyrene (PS) styrofoam boxes, PP rigid plastic containers, and PET soft drink bottles (Lim et al., 2018). The difference between the plastic waste produced globally and recycled waste is presented in Table 1. Various solutions have been proposed to deal with plastic waste, including waste sorting, value-added recycling, energy recovery, product bans, and biodegradable plastic production (Su et al., 2020b). The separation of various types of plastic waste from food waste is impossible, and this is likely to persist for an extended time (Gala et al., 2020). Organic residues in plastic-contaminated food waste can contaminate the soil, water, and air with pathogens, greenhouse gases, and unpleasant aromas (Zhang et al., 2021). Plastic and food waste can act as a catalyst for the dispersion of disease-causing microbes, such as viruses (Zhang et al., 2020). The costs and time requirements associated with cultivating, maintaining, and extracting enzymes from microorganisms are somewhat high. The process of enzymatic microbial degradation usually comprises the breaking of polymer bonds to produce smaller monomer components that can be processed further (Koshti et al., 2018). Petroleum-based polymers degrade slowly and research into their biodegradation is in its infancy, despite recent studies examining the biodegradation of plastic waste using specialized microbial strains (Baskaran and Rajamanickam, 2019; Gunawan et al., 2020; Li et al., 2020). A critical research gap is the lack of well-characterized microbes capable of digesting plastic that can be employed as model organisms for further investigations. Consequently, scientists should focus their efforts on the discovery of plastic-degrading bacteria to provide further groundwork with respect to the rapid biodegradation of polymers (Sánchez, 2020; Carmen, 2021; Matjašič et al., 2021). The total plastic waste and recycling development over the last decade are presented in Table 1.
According to “Plastics – the Facts (PlasticsEurope, 2016),” which examined data from 2006 to 2014, there has been a slight growth in waste generation and notable increase in recycling. However, the percentage difference between waste generation and recycling is still very large. This difference can be reduced by replacing plastic with biodegradable plastic and using biotechnology to enhance plastic biodegradation, which will reduce pollution and contribute to a healthy environment (PlasticsEurope, 2016). Only approximately 9% of all plastic is recycled after use, which is a major cause of global plastic pollution (Hopewell et al., 2009).
This review focuses on microbial hydrolase enzymes that have been shown to digest PET-polylactic acid (PLA) under anaerobic or aerobic conditions. PET and PLA are abundant and incredibly valuable materials that have a wide range of applications. Therefore, the development of technologies to valorize PET-PLA waste is needed to prevent plastic pollution and achieve a circular economy. Herein, we have discussed plastic biodegradation by I. sakaiensis, Bacillus, Thermomyces lanuginosus, Candida antarctica, Triticum aestivum, and Burkholderia spp., and Leaf-branch compost cutinases (LCC) for PET-PLA generated monomers that are directly upcycled into small molecules with added value, including cyclic acetals, 1,3-propanediol, and vanillin. Pseudomonas stutzeri, Pseudomonas aeruginosa, Streptomyces setonii, Streptomyces badius, Rhodococcus ruber, Comamonas acidovorans, Butyrivibrio fibrisolvens, and Clostridium thermocellum are the main bacterial species among the vast microbial populations linked to polymer degradation. Similarly, fungal species, including Aspergillus flavus, Aspergillus niger, Fusarium lini, Mucor rouxii, and Pycnoporus cinnabarinus, are discussed. We have also focused on fuel oil production using various methods, such as PLA-PET thermal pyrolysis/microwave-heated pyrolysis, self-made catalysts, fluidized-bed reactors, and in-house fabricated glass reactors. A possible hybrid integration technique to incorporate thermostable plastic degradation enzymes in the conversion into fuel oil will be explained in detail. This section discusses how biobased plastics can act as an alternative to fossil-based plastics. The challenges of PLA-PET waste bioconversion or thermal conversion into value-added products and fuel oils have been critically investigated.
Microbial Enzymes: a Potential Solution for Green Recycling
Most high molecular weight synthetic polymers can be biodegraded by microorganisms. Microbes have the ability to break down highly crystalline polymers; however, their utilization in commercial plastics is restricted. Microbiomes commonly interact with abiotic elements, such as heat and light, to alter polymer structures and enzymatic attack (Lear et al., 2021). Plastics are biodegraded by bacteria, fungi, and algae found in landfill leachate, sewage sludge, and compost. These microorganisms can degrade both natural and synthetic polymers (Sankhla et al., 2020).
Microbial biomass is a waste product of biodegradation. The waste products are the outcome of using organic polymers as energy and growth substrates, as shown in Figure 1. Enzymatic energy harvesting by microorganisms efficiently destroys natural polymers. Surprisingly, bacteria have evolved also these ancient decomposition pathways for use to degrade artificial plastic polymers. For example, Ideonella sakaiensis 201-F6 was shown to grow solely on PET. PETases and MHETases are two new enzymes catalyzed in nontoxic monomers by PET hydrolysis [for example, terephthalic acid (TPA) and ethylene glycol (EG); Yoshida et al., 2016]. In addition to I. sakaiensis, which biologically produces PETase, several bacterial systems, including Bacillus and Escherichia, have been studied as overexpressing PETase enzymes (Huang et al., 2018). Cutinase, lipase, and esterase are the most common enzymes studied for the degradation of synthetic oligomers or polymers (Danso et al., 2018). Multiple enzymes and metabolic pathways are frequently involved in the breakdown of polymers (Taniguchi et al., 2019). Enzymatic hydrolysis in stirred tanks and membrane bioreactors are the most commonly used techniques (Pino et al., 2018). High solid loadings are seen as an alternative to improve the biodegradation processes, whereas bubble columns and gas-lift bioreactors are used for enzymatic hydrolysis (EH).
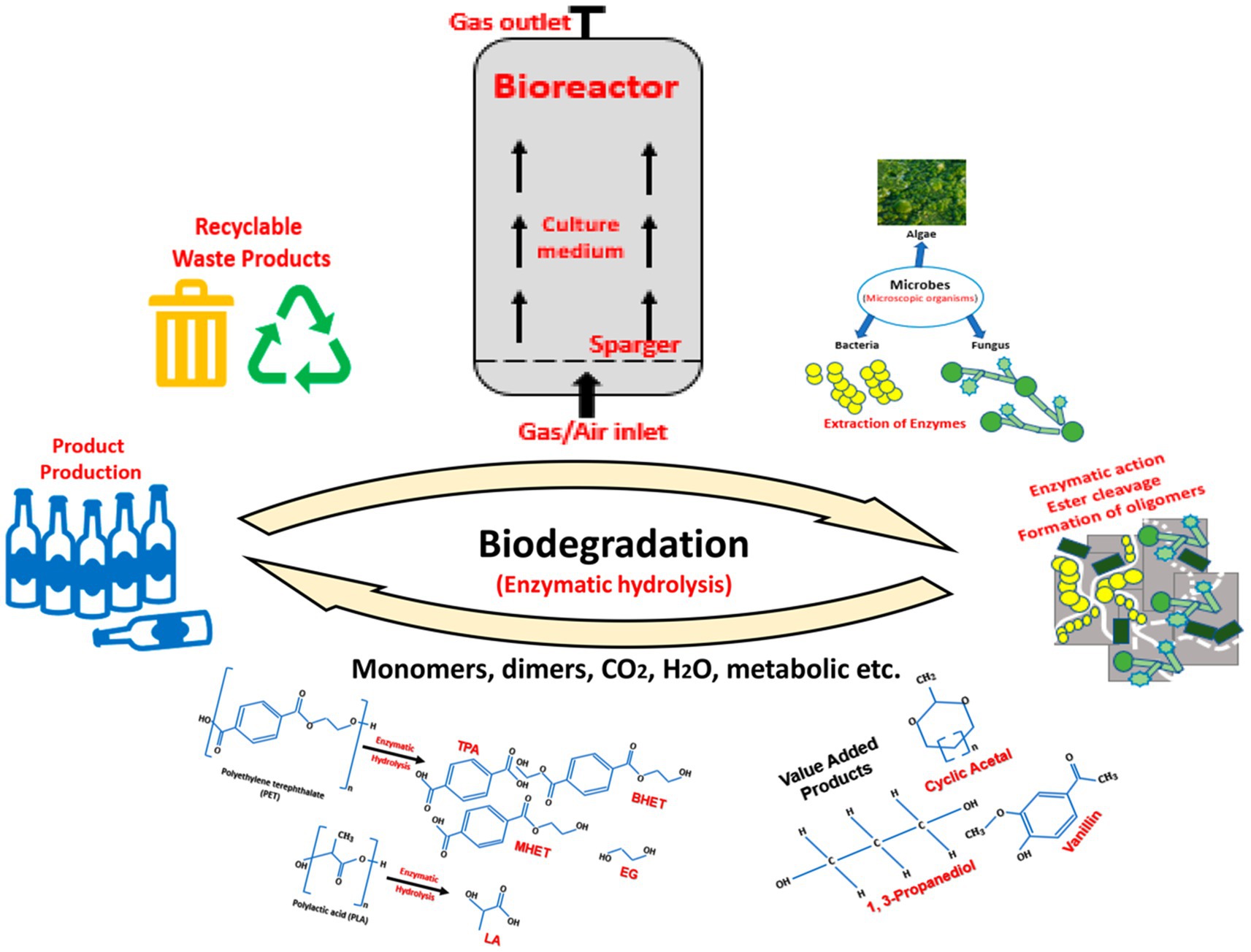
Figure 1. Schematic workflow of the biodegradation of plastic waste through enzymatic hydrolysis. The biodegradation of PLA-PET plastic waste is dependent on the diversity of the microorganisms and the environmental/experimental conditions. At a commercial level, this biological process occurs in bioreactors. The culture medium in the bioreactors results in the production of enzymes by the microbes. After excretion of the enzymes, they attach to the external surface of the plastic. As time passes, owing to their enzymatic activity, cracks appear on the polymer surface, subsequently causing it to decompose into lower molecular weight units, i.e., monomers and the other value-added products. The recycled monomers can be reused as a resin for polymer production.
Bacterial Degradation of PLA-PET Waste
Microbes degrade organic matter via enzymes. Carbon, nitrogen, and sulfur are transferred in elementary cycles via biodegradation and this procedure releases CO2, methane, and other biological components (Chandra and Rustgi, 1998). Microorganisms have been studied for their ability to decrease the strength of synthetic and natural polymers (Rujnić-Sokele and Pilipović, 2017). Biodegradability depends on the environment, because microorganisms and biodiversity are diverse. Plastic biodegradability is affected by the characteristics of both the microbe and the polymer (Tokiwa et al., 2009). The diversity of microbes that can degrade plastic is affected by various circumstances, including ambient conditions, temperature, and pH. In terms of environmental conditions, the moisture content of the soil is critical for microorganism growth. The soil’s water content is essential for microbial activation (Briški and Domanovac, 2017). The hydrolytic cleavage of microorganisms increases as the moisture content increases. Temperature and pH are essential elements in the breakdown process (Karigar and Rao, 2011; Mohanan et al., 2020). In addition to ambient conditions, temperature, and pH, microbial diversity also fluctuates. The soil moisture content is critical for microorganism growth (Jin and Kirk, 2018; Ratzke and Gore, 2018).
Under certain conditions, the chemical structure of degradable plastics will be changed significantly. In biodegradable plastics, this change is caused by the action of natural microorganisms (ASTM International, 2017). In principle, all organic compounds are biodegradable. However, to achieve this, different factors must be considered. The degradation of bioplastics via microorganisms and their degrading enzymes has emerged as a method of solving the unintended waste stream generated by large-scale plastic production. PET-PLA is an abundant and valuable material with a wide range of applications throughout society. Unfortunately, the global economy is not yet ready to fully embrace the benefits of recycling plastics because of the lack of cost effective equipment to measure the quality of post-consumer PET-PLA trash. PET and other materials may be recycled from PET by utilizing technologies such as monomer recycling, although recycling focus on repurposing the monomers to make additional PET.
Thermobifida fusca KW3, a gram-positive thermophilic actinomycete secretes a highly hydrophobic carboxylesterase (TfCa) that can hydrolyze PET (Oeser et al., 2010). To enhance TfCa enzyme expression and avoid the formation of inclusion bodies, its codon usage and induction conditions were optimized. The optimization conferred a 4,000-fold increase in TfCa activity. The TfCa protein concentration was found to be 41.6mg/L after culture in a pH-controlled bioreactor (Samak et al., 2020). The hydrophobic synthetic cyclic PET trimer was hydrolyzed by a carboxylesterase from Thermobifida fusca KW3, a thermophilic actinomycetes at 60°C and pH 6 (Billig et al., 2010). The values of Vmax and Km for the hydrolysis were 9.3mmol−1 min−1 mg−1 and 0.5mM, respectively. The esterase demonstrated a strong preference for short- and medium-chain fatty acyl esters of p-nitrophenol. The enzyme retained 37% of its activity after culture at 50°C and pH 8 for 96h. The process used a standard three-element catalytic system that includes the amino acids serine, glutamic acid, and histidine.
Acero et al. (2011) expressed cutinases from Thermobifida cellulosilytica DSM44535 (Thc_Cut1 and Thc_Cut2) and Thermobifida fusca DSM44342 (Thf42_Cut1) in Escherichia coli BL21-Gold(DE3) to hydrolyze PET. Two cutinases from T. fusca KW3 were compared with PHA depolymerase (ePhaZmcl) from Pseudomonas fluorescens. pNP-acetate and pNP-butyrate exhibited kcat and Km values in the ranges of 2.4–211.9s−1 and 127–200μM and 5.3 and 195.1s−1 and between 1,483 and 2,133μM, respectively. The PET-based products had a high conversion rate to MHET and TA. The water contact angle (WCA) could also change as a result of the change in the hydrophilicity.
Bacillus subtilis p-nitrobenzylesterase (BsEstB) was isolated and confirmed to hydrolyze PET (Ribitsch et al., 2011). The expressed BsEstB of 55.2kDa showed a specific activity of 77 and 108U/mg after reaction with p-nitrophenyl acetate (PNPA) and p-nitrophenyl butyrate (PNPB), respectively. BsEstB demonstrated strong enzyme activity at 40°C and pH 7.0 and good stability for several days at pH 7.0 and 37°C. The half-life was 3days at 40°C and 6h at 45°C. The principal conversion products of PET films with a lower WCA were the TA and MHET products, which decreased from 68.2°±1.7° to 62.6°±1.1°.
Thermobifida alba (Tha_Cut1) cutinase was overexpressed for PET hydrolysis (Ribitsch et al., 2012b). The Cut1 sample demonstrated a considerable degree of active PET surface hydrolysis, which improved hydrophilicity, as observed by a decrease in the WCA from 87.7° to 45°. The PNPB and PNPA values were 2.72 and 6.03s−1 for Km and 213 and 1933μM for the kcat, respectively. Tha_Cut1 primarily released 2-hydroxyethyl benzoate after interaction with bis(benzoyloxyethyl)terephthalate (3PET). Unlike the well-studied Humicula insolens cutinase (HiC), which preferably liberates 3PET terminal benzoic acid, this takes up all the carbon, oxygen, and nitrogen, rendering it very toxic to the environment.
In E. coli, the enzyme Thh_Est, which shares 87% homology with an esterase found in T. alba, was studied for its ability to surface-hydrolyze PLA and PET (Ribitsch et al., 2011). PET was digested by Thh_Est, which released TA and MHET in similar proportions (19.8 and 21.5mmol/mol of enzyme) without producing higher-order oligomers such as BHET. PLA was hydrolyzed, and lactic acid was released. Both the enzymatic and surface hydrolysis processes of PET and PLA caused an increase in hydrophilicity; the WCA decreased from 90.8° and 75.5° to 50.4°, respectively, and the water droplets spread entirely over the surface.
Tcur1278 and Tcur0390 from Thermomonospora curvata DSM43183 were found to have 61% sequence identity with the enzyme from T. fusca (Wei et al., 2014). The optimal reaction temperatures of Tcur1278 and Tcur0390 were 60 and 55°C, respectively. It was found that the optimum pH of both enzymes was 8.5. After incubation for 60min at 55°C, Tcur1278 retained more than 80% of its initial activity, and Tcur0390 had less than 10% of its initial activity. At reaction temperatures of up to 50°C, Tcur0390 was exhibited more effect hydrolysis of poly (ε-caprolactone) and PET nanoparticles than Tcur1278. At 55 and 60°C, only Tcur1278 exerted hydrolytic activity on PET nanoparticles. In molecular dynamics situations, Tcur1278 was demonstrated to have superior thermal stability, which is likely to be the main reason for its greater hydrolytic activity against PET.
The cutinase Cut190, from Saccharomonospora viridis, was expressed in E. coli (Kawai et al., 2014). The substitutions of Ser226 to Pro and Arg228 to Ser were shown to increase activity when operating at 65–75°C and pH 6.5–8.0 in the presence of 20% glycerol and Ca2+ ions. The mutant Cut190 could hydrolyze various aliphatic polyester materials. Yoshida et al. (2016) discovered a unique species that used PETase and MHETase to hydrolyze PET, enabling it to utilize plastic as its only energy and carbon source. The bacterium could therefore easily convert PET into TPA and EG, which was used for growth. PET film was hydrolyzed at 60°C using a dual enzyme system, including the KW3 strain of Thermobifida fusca TfCut2, and a metagenome-derived LC-cutinase (Barth et al., 2016). In the enzyme system, the amount of breakdown products formed was 2.4-fold greater when compared with TfCut2 after reaction for 24h.
Cutinase from Humicola insolens (De Castro et al., 2017) showed the best performance of 16 commercial lipases and was used for reactions with PET, solely or in combination with the efficient MHET-converting lipase from Candida antarctica. When two enzymes were used in combination, the concentration of the product was more than twice that when the enzyme was used alone, and the rate of terephthalic acid production was increased, reaching 9.36g/L. Carniel et al. (2017) screened 10 enzymes using BHET as the substrate and found that the combination of Candida antarctica lipase B (CALB) and HiC resulted in complete synergetic depolymerization of PET to TPA and a 7.7-fold increase in PET to TPA yield was achieved.
Four types of cutinase from Thermobifida cellulosilytica were employed to hydrolyze HMLS-PET cords (Vecchiato et al., 2017). -COOH of 0.51nmol mm−2 resulted in the highest degree of functionalization, with 1.35mM of soluble product was released after 72h. Compared with NaOH-based chemical hydrolysis, EH only solubilizes 0.7% of the polymer, without any reduction in mechanical characteristics or crystallinity. PET-TE membranes with a highly crystalline structure could be hydrolyzed by cutinases 1 and 2 from T. cellulosilytica and a fusion protein of cutinase 1 with the polymer-binding module from Alcaligenes faecalis (Thc Cut1 PBM) through a very narrow pore size distribution (Gamerith et al., 2017). By comparing the effects of two surface-functionalized versions of Thc_Cut1_PBM in the EH of PET-TE film on hydrophilic and hydrophobic surfaces, the influence of surface chemistry was also studied. The initial PET-TE membrane had the highest surface hydrolysis efficiency, resulting in a weight loss of 0.36%, which corresponded to the removal of approximately 3nm of PET from the entire porous membrane surface, which was well correlated with a 4-nm increase in the measured barrier aperture. A chemo-enzymatic treatment was developed to recover TPA and EG from PET (Quartinello et al., 2017). The chemical treatment, performed at 250°C and 40bar, converted PET into 85% TPA and other small oligomers. The hydrolysis in the second step using the HiC yielding 97% pure TPA, which was comparable with commercial synthetic grade TPA (98%). Biundo et al. (2018) improved the activity of an esterase (Cbotu_EstA) from Clostridium botulinum on PET by site-directed mutations in zinc-binding domain and deleted the domain consisting of 71 amino acids at the N-terminus. The zinc-binding domain of the native enzyme had 50-fold lower activity than the combination with truncations and substitution. Moreover, the kinetic parameters of Cbotu_EstA demonstrated a noticeable shift from water-soluble to insoluble polymer substrates.
The protein Sub1, a potential cutinase from Streptomyces scabies, was expressed in E. coli, purified, and characterized (Jabloune et al., 2020). Among the carbon short chain p-nitrophenyl esters, Sub1 had the strongest hydrolytic activity. The Vmax was 2.36mol g−1 min−1 for p-NPB. The Sub1 esterase hydrolyzed PET, as shown by the release of TA. The addition of Triton to Sub1 on PET led to an increase in activity, and the Sub1 activity was found to be stable at 37°C for at least 20days. The expression of BhrPETase in Bacillus subtilis was 0.66g/L, and the biochemical characteristics of BhrPETase showed that BhrPETase had stronger hydrolytic activity on amorphous PET than LCC and IsPETase, as previously reported, as expressed under the same conditions (Xi et al., 2021). The purified BhrPETase has a melting temperature of up to 101°C, making it the most heat-resistant bacterial PETase ever characterized.
Polylactic acid depolymerase from Paenibacillus amylolyticus showed degradation activities against PLA (Akutsu-Shigeno et al., 2003). Lactic acid was found to be a degradation product of PLA. The gene encoded 201 amino acid residues, including the conserved pentapeptide Ala-His-Ser-Met-Gly, which is present in the lipases of mesophilic Bacillus species. Kawai et al. (2014) (Kawai, 2010) reported that microorganisms and PLA depolymerases are capable of decomposing PLA. The finding offsets the fact that PLA is highly vitrifiable and that microbes capable of decomposing PLA can do so at temperatures as low as 37°C. In this case, members of the Nocardiaceae family are the putrefying microorganisms here. The Amycolatopsis PLA depolymerases were isolated and cloned as serine proteases. The thermophilic lipases used to make the PLA were derived from Bacillus thermophilus strains that could grow at 60°C. Enzymes such as proteases and lipases are widely used in industrial settings as PLA depolymerases. Protease-type depolymerases were enantioselective for L-PLA, but lipase-type depolymerases were enantioselective for D-PLA. Consequently, two unique types of PLA depolymerases degrade the lipases and proteases. The EH conditions of PLA fibers were optimized with lipaselipases from different sources (Lee and Song, 2011). Pseudozyma antarctica JCM 10317 from the Pseudozyma genus (PaE) showed high breakdown potential for biodegradable polymers (Shinozaki et al., 2013). Poly (butylene succinate-co-adipate) activity against phthalate esters (PaE) was 54.8±6.3U/mg. Moreover, it was found that PaE could also degrade PBS, PBSA, and PLA solid films.
Tchigvintsev et al. (2015) performed a search for novel carboxylesterases inside three marine metagenomic gene banks. They found 23 active clones, five of which were extremely active esters and, at 5°C, exhibited an ability to target both α-naphthalene and p-nitrophenyl esters. MGS0010 exhibited a 2-fold increase in activity in the presence of 3.5M NaCl or KCl; however, the other four esterases were inhibited to various degrees. Esterases may also hydrolyze PLA polyester substrates, suggesting that they may be suitable for depolymerizing polyester. To enhance the amount of hydroxyl and carboxylic groups on the polymer chains, H. insolens cutinase (Pellis et al., 2015) was employed to hydrolyze PLA film. Another change in WCA was found, as it decreased from 74.6 to 33.1°, and roughness and waviness were 10 times higher than in than the control samples.
The structures of two native cutinases from Thermobifida cellulosilytica, named Thc_Cut1 and Thc_Cut2, as well as of two variants were investigated by Ribitsch et al. (2017). They showed different activities toward PLA. Although, the crystal structure analysis did not reveal significant conformational differences, unique SAXS scattering data collected from enzymes in the solution showed significant surface charge differences among the different cutinases. The differences in surface electrostatic potential and hydrophobicity could explain the different binding patterns of the four cutinases on PLA, and thus explain their different activities. Est119 from Thermobifida alba AHK119 can degrade polymer substrates, including PLA. The PLA-degrading mechanism by Est119 was clarified by Kitadokoro et al. (2019), who found that the amino acid residues responsible for substrate interaction were substantially conserved in the enzymes of Ideonella sakaiensis, including cutinases and PETase. The bacterial enzymes in literature for the biodegradation are briefly mentioned in Table 2.
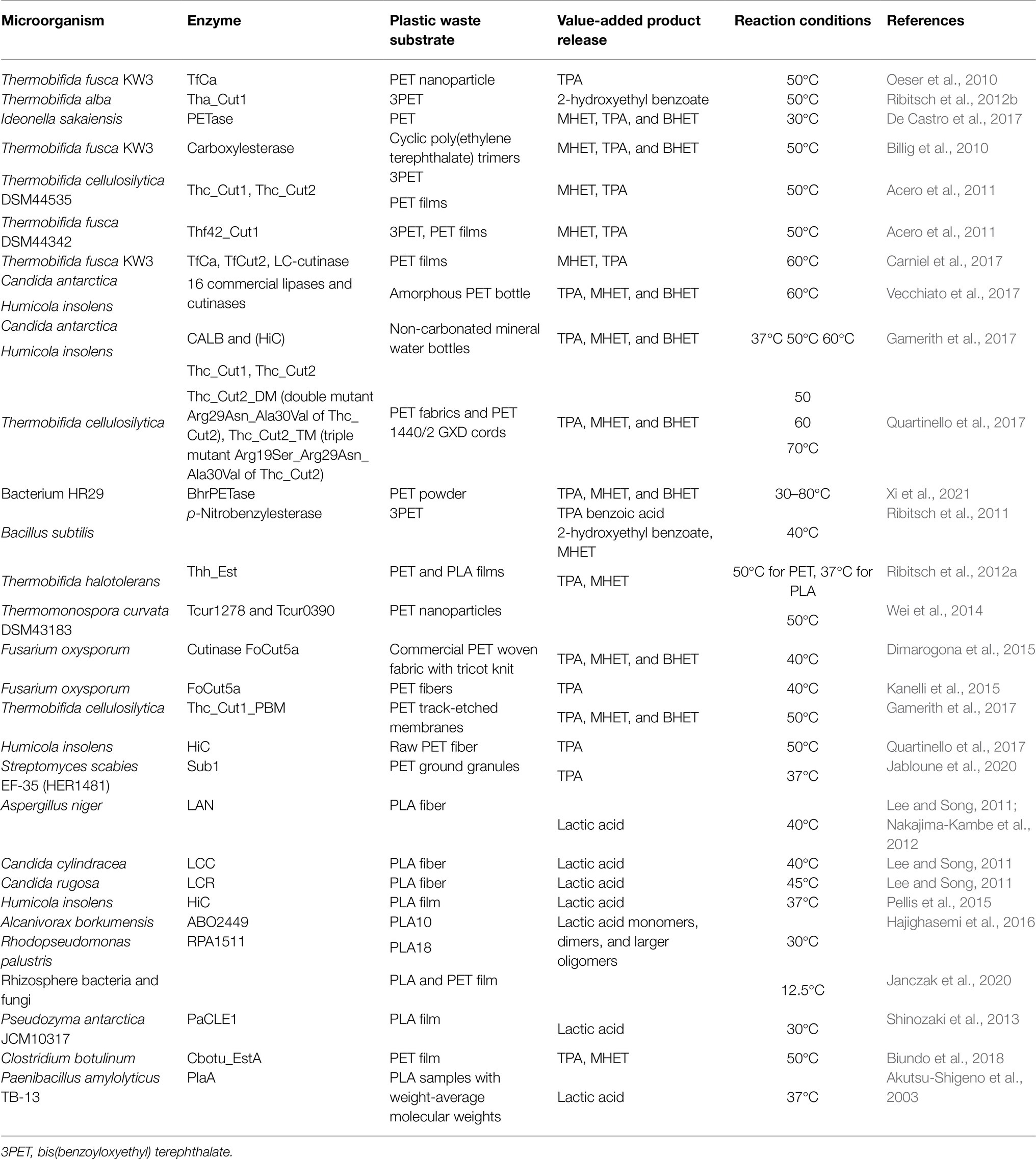
Table 2. Summary of polylactic acid and polyethene terephthalate (PLA-PET) degrading enzymes, their microbial sources, and the value-added products released from the biodegradation process.
Fungal Degradation of PLA-PET Waste
The fungal kingdom comprises an exceedingly diverse and fascinating eukaryotic collection of aerobic microorganisms varying from simple yeast cells to highly mycelial molds (Rossman, 1995). Single-celled or pseudomycelia growth is the standard for yeasts, although molds usually grow as mycelia. Because fungi, like bacteria, decompose and mix with organic substances, they are vital to the breakdown of the C–C bonds in the biosphere. Different species of bacteria and fungi can indeed grow in conditions with less moisture and in solutions with a lower pH, which helps organic materials decompose (Spellman, 2007). Fungi are incredibly active in decomposing natural polymeric materials owing to their extracellular multienzyme complexes. They are also capable of rapidly colonizing and penetrating substrates via their hyphal systems and transporting and redistributing nutrients within their mycelium via their hyphal systems (Matavulj and Molitoris, 2009). A diverse range of fungal strains from various classes, ecologies, and morphologies has been demonstrated to destroy plastics.
Fungi are highly capable of degrading many materials, including lignin, and are used in remediating contaminants (Fritsche and Hofrichter, 2005), as indicated in Table 3. New studies have indicated that the Aspergillus genus is the most prevalent of all the fungal groups appropriate for the biodegradation of synthetic plastics. Aspergillus clavatus, Aspergillus fumigatus, and Aspergillus niger are three different species of Aspergillus that have been found in terrestrial habitats around the world and are known to degrade PE, PU, and PP (Abraham et al., 2016). Neosartorya, Amorphoteca, and Talaromyces were deserted from soil polluted with petroleum and demonstrated to be promising hydrocarbon degradation organisms (Chaillan et al., 2004). In addition, it was discovered that the collectively, a number of individuals of fungi, namely Penicillium, Cephalosporium, and Aspergillus, are capable degraders of crude oil hydrocarbons (Singh, 2006). Alternaria solani, Fusarium solani, Spicaria spp., Geomyces pannorum, Phoma sp., and Penicillium spp. are additional fungal species with a high degree of plastic degradability (Sowmya et al., 2015). Contrary to popular belief, different fungal consortia have been proven to destroy various polymers, such as PET and PLA, through synergistic action (Ghosh et al., 2019; Nikolaivits et al., 2021). Before eventual depolymerization, as well as their potential to release hydrophobin for improved hyphal adhesion to hydrophobic substrates, fungal hyphae are critical in both the initial colonization and the subsequent depolymerization of the fungus (Wang et al., 2018; Quarantin et al., 2019). Pretreatment factors such as exposure to light and temperature and acid pretreatment and various additives have been used to enhance fungal biodegradation of plastics (Montazer et al., 2020). The hydrolysis of PLA fibers by lipases was greatest at 40°C for 60min at pH 7.5 for A. niger lipase, 40°C for 120min at pH 8.0 for Candida cylindracea lipase, and 45°C for 120min at pH 8.0 for Candida rugosa lipase. Lipase from Aspergillus niger MTCC 2594 was 53.8-fold purified by hydrophobic chromatography after interaction with octyl sepharose, and the enzyme revealed two protein bands with apparent molecular masses of 35 and 37kDa (Nakajima-Kambe et al., 2012). The lipase had maximum activity at pH 7.0 and 37°C, and was stable between pH 4.0 and 10.0, as well as temperatures up to 50°C. Olive oil, as a substrate, yielded values of Km and Vmax of 3.83mM and 32.21μmol/min/mg, respectively. The lipase has the potential to degrade PLA and poly(ε-caprolactone). A Fusarium oxysporum cutinase, FoCut5a, was expressed in E. coli BL21 (Dimarogona et al., 2015). The highest production of recombinant FoCut5a was achieved using periplasmic expression at 16°C. The X-ray structure was determined at 1.9Å resolution. The crystal structure was highly similar to that of Fusarium solani cutinase. FoCut5a showed absolute activity at 40°C and pH 8.0, while it was active on three p-nitrophenyl synthetic esters of aliphatic acids, with the maximum catalytic efficiency for the hydrolysis of butyl ester. FoCut5a cutinase is capable of modifying the surface of PET fabrics. The optimal parameters were found to be 40°C, pH 8, and 1.92mg of enzyme loading per gram of fabric (Kanelli et al., 2015). The analysis of tensile test and dyeability proved that the surface treatment did not harm the quality of the original material. The S-2 strain of Cryptococcus sp. had remote similarity to cutinases, but not lipases (Masaki et al., 2005). This enzyme can degrade PLA and other biodegradable plastics such as poly(butylene succinate) and poly(3-hydroxybutyrate).
Algae as a Promising Candidate for Plastic Degradation
Microalgae are unicellular photosynthetic organisms capable of converting water and CO2 into nutrients such as proteins, amino acids, lipids, polysaccharides, and carotenoids. They are a good candidate for a “microbial factory” because they can use readily accessible materials, such as inorganic nutrients (CO2), water, sunlight, and wastewater, with little or no effort. The identification of algae and their toxins that can biologically degrade polymeric materials can help reduce white pollution (Bhuyar et al., 2019). In contrast, bioplastics made from algae offer the same qualities as petroleum-based polymers while being naturally biodegradable (Chia et al., 2020). They can be grown on ponds and do not require specialized fertile land or freshwaters. Thus, microalgal biomass is an ideal low-cost bioprocess system for the production of PHA (Arora et al., 2021). Among them, only a few eukaryotic algae, such as Chlorella, have been reported to produce PHA (Roja et al., 2019). Cyanobacteria are some of the oldest and first photoautotrophic microorganisms on earth. They contribute to the global carbon/oxygen cycle and helped to evolve life by creating an oxygen-rich environment. The phycocyanin pigment confers their characteristic blue-green color, hence the name blue-green algae (Kaewbai-Ngam et al., 2016). The growth of microalgae in a closed laboratory photobioreactor, wastewater, or open ponds can yield higher biomass. This biomass can be harvested and processed for use as a substrate by other heterotrophic bacteria and produce PHA (Rahman et al., 2014). Microalgae cultures can use inorganic nitrogen and phosphorus for growth, making them a perfect solution for tertiary and quaternary treatments. Moreover, their ability to remove heavy metals and some toxic organic compounds prevents the secondary pollution (Abdel-Raouf et al., 2012). Microalgae, like bacteria, are a biological pollutant owing to their endotoxins and carbon demand (Yan et al., 2016). Microalgae grow in natural and laboratory environments with bacteria in mutually beneficial relationships. Bacteria benefit from the oxygen and extracellular substances of microalgae and return the favor with CO2 and vitamins (Han et al., 2016). Organic pollution can harm water quality in many ways. Algal wastewater treatment systems are now widely accepted as an effective conventional treatment system. Algal wastewater treatment systems are a significant low-cost alternative to complex, expensive treatment systems, particularly for municipal wastewater purification (Sen et al., 2013). Phaeodactylum tricornutum, a photosynthetic chassis, produces a microbial cell factory that develops an engineered form of PETase, which is secreted into the surrounding culture medium to degrade PET in aquatic environments (Moog et al., 2019). To better predict how climate change will affect aquatic microbial ecosystems, we need to understand community resilience. Interlevel interactions in a mixed community of microalgae and bacteria have a large impact on system resilience (Sörenson et al., 2021). The potential of the microalgal species Chlorella vulgaris with pretreatment, marked by its effectiveness in cracking and changing polymers, aids the growth of microorganisms on fractured surfaces (Falah et al., 2020). They found that the microbial species could produce the biodegradation products found in PET, such as alkane esters, fatty acids, benzoic acid, and aromatics, and the most toxic biodegradation product is bis(2-ethyl hexyl phthalate). In total, 10 algal species belonging to nine families, seven orders, and 10 genera were identified from various polyethylene-degrading locations (Sharma et al., 2014). Microalgae produce enzymes that reduce reaction activation energy and weaken polymer chemical bonds, promoting biodegradation. Microalgae have the ability to convert plastics into metabolites such as CO2, H2O, and new cell biomass (i.e., mineralization).
Biomass gasification converts biomass into CH4, H, CO2, and NH3. The gas produced from this process can be used as fuel gas, engine fuel, or as a feedstock for other industrial processes (synthetic gas; Mckendry, 2002). Microalgae are productive (12.5kg m−2 year−1) compared with most other plants (Shay, 1993). As reported by Goudriaan et al. (2000), a 75% thermal efficiency was achieved in a 10kg dry weight h−1 pilot plant during the hydrothermal upgrading process of biomass. Bio-crude oil comprises approximately 45% of the whole feedstock weight and has a lower heat capacity of 30–35MJ kg−1, enabling it to work with diesel fuel and be improved further.
The liquefaction of biomass by direct heating is very well studied. A handful of methods rely on algal biomass for their technology. Minowa et al. (1995) performed direct hydrothermal liquefaction at temperatures of 300°C and pressures of 10MPa to achieve a moisture content of 78.4wt% and an oil yield of roughly 37% for Dunaliella tertiolecta. After 60min of holding time at 350°C, the oil obtained had viscosity in the range of 150–330mPa and calorific values in the range of 36–40kJ g−1. These values were close to those of fuel oil. As shown by the energy balance, the liquefaction method is a total energy producer.
Similarly, Demirbas (2010) achieved a maximum output of 64% dry wt. based on oil liquefaction at 300°C catalyzed by sodium carbonate in a study on oil recovery using Botryococcus braunii. The development of microalgae occurs much faster than on land. It is predicted that 20,000–80,000L of oil are produced annually per acre of algae, which is 7–31 times greater than the next best crop of palm oil. Open ponds, photobioreactors, and closed systems are industrial reactors for algae culture.
Aresta et al. (2005) examined various conversion strategies to manufacture microalgal biodiesel, including organic solvent extraction, supercritical CO2, pyrolysis, and hydrothermal technology. For the mining of microalgae biodiesel, the hydrothermal-liquefaction approach proved more successful than utilizing supercritical CO2. It is reasonable to assume that hydrothermal liquefaction is the most effective technological solution for producing biodiesel from algae among the selected methods.
The incorporation of nanosized materials, such as nanofibers, nanoparticles, nanotubes, nanosheets, and other nanostructures. A novel method incorporating aluminum sulfate-coated magnetic particles was highly effective at isolating microalgae species of the Anabaena and the Aphanizomenon genus from a mixed culture. The use of silver nanoparticles to increase the harvest of Cyanothece 51,142 and Chlamydomonas reinhardtii microalgae resulted in a 3-fold increase (Pattarkine and Pattarkine, 2012; Safarik et al., 2016).
The following enzymes, used in conjunction with polymer nanoparticles such as polyacrylonitrile nanofibers, iron oxide, and nanoporous gold; silica nanoparticles with lipase from Rhizopus miehei; ferric silica and magnetic nanoparticles with lipase from Burkholderia sp.; and polyacrylonitrile nanofibers, successfully transformed plant oils into biodiesel via the transesterification process: Pseudomonas cepacia lipase, Rizopus miehei lipase, ferric silica, and magnetic nanoparticles with lipase from Burkholderia sp., and polyacrylonitrile nanofibers (Palaniappan, 2017). In addition, a nanomagnetic biocatalyst, composed of lithium-doped CaO, KF/CaO–Fe3O4, Fe2O3–CaO, and sulfate, integrated sodium titanate, zirconium, and carbon-based nanotubes and nanoparticles to yield up to 95% or more biodiesel from a variety of biomass and biodegradable waste (Liu et al., 2018).
Apart from increasing the efficiency of biodiesel production, a type of NP called zeolite has been employed commercially as an absorbent within the transesterification process. Zeolites consumed the unwanted moisture content (4–6%) from biodiesel. Mesoporous nanoparticles have also demonstrated a critical aptitude for regular microalgal-biofuel synthesis without cell lysis. Additionally, zeolites extract lipids from the microalgae cell membrane (Liu et al., 2008).
Moog et al. (2019) created a microbial cell factory utilizing the photosynthetic microalga Phaeodactylum tricornutum to produce and secrete an engineered version of PETase into the surrounding culture medium. Initial studies utilizing 3D culture weightless at 30°C examined PETase activity vs. PET and the copolymer polyethylene terephthalate glycol (PETG); the low-crystallinity PETG had an 80-fold higher turnover than bottle-grade PET. The breakdown products of the PET substrate were TPA and MHET.
Chlorella pyrenoidosa was grown to produce biofuel and bioplastic. The maximal growth took 10days. The strain grew faster in the presence of CO2. The chemically processed algal biomass produced bioplastic. The 14-day-old algal culture contained 27% polyhydroxybutyrate (PHB). This ensures that the microalgae-derived bioplastic is biodegradable. The microalgae detoxified chromium and nickel by 11.24 and 33.89% (Das et al., 2018). Table 4 summarizes the literature on biodegradable algae enzymes.
Factors Affecting Microbial Degradation of PLA-PET Waste
Environmental variables, such as pH, humidity, temperature, salinity, quantity of oxygen, sunshine, water, stress, and culture conditions, have a meaningful effect on the microbial population and enzyme activity (Oliveira et al., 2020). Owing to their metabolic machinery, as well as their ability to adapt to hostile settings, microorganisms can destroy a wide range of organic contaminants. Consequently, microbes play an important role in site rehabilitation. However, their efficiency is conditional on a range of factors, including the chemical composition and quantity of pollutants, their presence in microorganisms, and the physical and chemical features of the environment. These are microbiological and genetic factors or environmental influences (Gupta et al., 2017).
When fungi grow at the lowest pH possible, the most CO2 is produced, and the strongest lignolytic activity occurs (Kumar and Chandra, 2020). The biodegradability of polyester is dominated by chemical factors and the physical characteristics of polyester. A significant aspect related to plastic biodegradation is the molecular weight. A low molecular weight is beneficial for biodegradation. The increases in the molecular weight of poly (e-caprolactone) diols resulted in an increase in the rate of EH of poly (e-caprolactone) diol by Rhizopus delemar lipase (Valderrama et al., 2019). The melting point of the polymers strongly impacts polymer enzymatic degradability. Polyester is usually more durable and less biodegradable when it has a higher melting point. Polymer degradation was decreased with the help of improvements in crystallinity and flexibility (Siracusa, 2019). Additives, stabilizers, and antioxidants used in the manufacture of polymers can delay degradation and may be toxic to microorganisms (Allen and Edge, 2021). Apart from structural considerations (linearity and branching in polymers, bond types, such as carbon–carbon, amide, and ester), the physical form of the polymer (powder, films, pellets, and fibers) may also affect a polymer’s biodegradability. Ultimately, the degradation rate is determined by the mechanism of degradation and the process acceleration (Vohlídal, 2021).
Different plastic polymers are electromagnetic radiation sensitive owing to their ability to absorb intense solar radiation (Hegazy et al., 2009). Chemical reagents included in polymeric structures can alter functional groups along with hydrophilicity/hydrophobicity (additives). It has been demonstrated that hydrogen peroxide, hydrochloric acid, sulfuric acid, and nitric acid all oxidize polymer surfaces (Moharir and Kumar, 2019). The observed increase in mineralization rate and carbon-fixing during PE degradation was ascribed to the use of chemicals that enhanced polymer oxidation (Jakubowicz, 2003).
Potential Biogas Production Using PLA-PET Plastic Waste
Food waste is used to produce biogas, which is made up of enormous amounts of methane (Nanda and Berruti; Luo and Wong, 2019). Nevertheless, anaerobic digestion (AD) digesters are not typically used for the breakdown of plastic (Sánchez, 2020). In anaerobic conditions only, the methanogenic stage of the AD process is used for simple substrates (Treu et al., 2019; Yan et al., 2019). In addition, typical AD systems often keep the working pH at 6.8–8.0, which is not favorable for the breakdown of plastic when contrasted with a pH range with a lower value (e.g., pH ~4–6; Pathak and Navneet, 2017; Srisowmeya et al., 2020). A field emission-scanning electron microscope was used to analyze the time taken for disposable plastics (e.g., PP, PS, and HDPE) to degrade in a typical AD process, which showed that the procedure had limited success in degrading plastics (Lim et al., 2018). More recently (Zhang et al., 2018, 2019), following a 65-day anaerobic breakdown of plastic, it was revealed that the percentage of solid material in LDPE and PP was lowered by 1.5 and 2.0%, respectively. Regardless of how plastic waste is biodegraded, with some microorganisms emerging as a study subject, there is still a long way to go before petroleum-based plastics can be considered biodegradable (Baskaran and Rajamanickam, 2019; Gunawan et al., 2020; Li et al., 2020). A major research gap that has been identified is a microorganism capable of digesting plastic is as a model organism for further detailed research. Researchers should focus on finding potential bacteria that degrade plastics so that the biodegradation of plastics can be made highly efficient (Sánchez, 2020; Carmen, 2021; Matjašič et al., 2021).
The physical and chemical properties of the polymers are generally assessed through the direct observation of the anaerobic degradation process (Day et al., 1994). A number of the evaluated properties, including mass loss, mechanical property reduction, altered thermal behavior through scanning electron microscopy, infrared spectroscopy, gel permeation chromatography, and exclusion chromatography, all revealed microbial invasion (Rivard et al., 1995; Massardier-Nageotte et al., 2006; Yagi et al., 2009, 2012, 2014; Hermanová et al., 2015; Selke et al., 2015; Lee et al., 2016). These methods provide evidence of breakdown, but they cannot ascertain the degree of degradation or tell whether it was caused by microbes or other activities.
Anaerobic degradation is a type of nonoxygenated biological deterioration. It uses organic matter as an electron donor or acceptor, whereas anaerobic respiration uses CO2, SO4−2, and NO3−. Hydration, acidification, acetogenesis, and methanogenesis are the four steps. Fermentation creates two-thirds of the CH4, whereas respiration produces one-third. Acidity, temperature, redox potential, and hydrogen concentration all affect anaerobic biodegradation efficiency. It also depends on the microbe concentration, nutritional levels, and substrate qualities (Mohee et al., 2008). The waste in landfills is diverse, complicating biodegradation. Anaerobic decomposition has five stages. The initial hydrolysis reduces pH, increases CO2 demand, and concentrates volatile fatty acids, ammonia, and sulfates. Step 2 is acidogenesis, which generates H2. Acetate, hydrogen, and CO2 are produced in Step 3. Step 4 is methanogenesis, which produces biogas. In Step 5, stabilization reduces biogas generation and increases the N2 concentration. Abiotic and biotic factors influence biodegradation. However, the treatment and recirculating of leachate has been demonstrated to increase biodegradation rates, enhancing the role of landfill as a bioreactor (Cossu et al., 2018). The differences between the biodegradation processes of PLA-PET waste under aerobic and anaerobic conditions are shown in Figure 2.
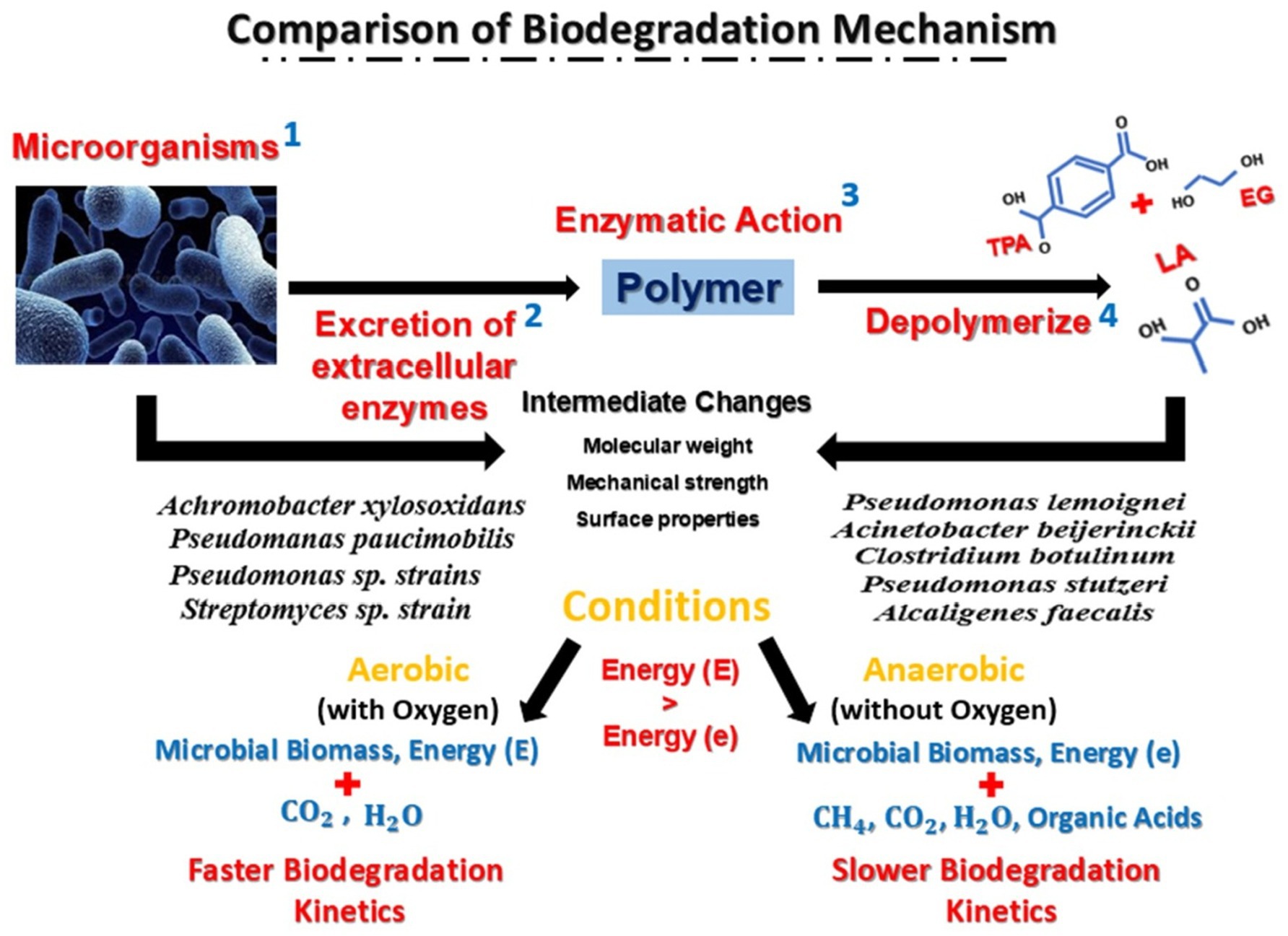
Figure 2. Biodegradation processes of PLA-PET plastic waste under different conditions. Biodegradation occurs under two main conditions: aerobic and anaerobic. For aerobic degradation, oxygen is necessary; in contrast, for anaerobic degradation, oxygen is needed at the start of the process, but not needed after a while. Both conditions use different enzymes that affect the molecular weight, mechanical strength, and surface properties. In one comparison, aerobic degradation occurs much faster than anaerobic degradation (Glaser, 2019). In anaerobic conditions, less energy is released compared with in aerobic conditions. The end products of the degradation are microbial biomass, CO2, H2O, and energy in aerobic digestion and extra products, namely CH4 and organic acids in anaerobic digestion.
The breakdown of plastics results from various causes that may be physical, chemical, or biological. The mechanism is material- and condition-dependent. Exposure to UV light, temperature, mechanical stress, oxidative, hydrolytic, and biodegradative conditions causes damage. Plastics break down anaerobically in enclosed vessels or spaces; therefore, oxygen and ozone oxidation are irrelevant. For polyolefins and PS, free radicals are thought to cause consecutive cleavages along the polymeric chain. Free radicals are created through a UV-stimulated radiation process for polyethylene (Singh and Sharma, 2008).
Temperature and pH control the rate of degradation. The proposed biodegradation processes for polymers, such as PHBV, include colonization, hydrolysis, the erosion of exposed sections, saturation, and fragmentation. Microbes attack amorphous starch zones on polyethylene–starch copolymers, allowing entrance into interior holes. Therefore, the degradation of the polyethylene matrix occurs after starch consumption (Singh and Sharma, 2008). Depending on the test settings, plastics degrade at varying rates. Compared with other PHAs, this material increased biogas generation by 62%. In contrast, PHB decomposed 90% in 14days at 55°C, which was equivalent to cellulose. However, only 23% of anaerobic sludge degradation was achieved in 10weeks (Yagi et al., 2014). When eggshell was utilized to make a biocomposite, the degradation of PCL rose from 80% over 50days to negligible at 55°C. PBS was also degraded at 25.8 and 32.5% under thermophilic circumstances, but did not mineralize when tested at 55°C (Petit et al., 2015).
Polylactic acid biodegradation has been reported as anything from total mineralization to complete biodegradation, with complete biodegradation taking place in under 100days (Selke et al., 2015). The L-lactic acid treatment of PET waste resulted in a random distribution of co-monomeric units (Hermanová et al., 2015). Hydrolytic and/or biodegradable co-polyesters were created by combining aromatic and aliphatic units. Sample A generated a theoretical yield of 69% methane-rich biogas, whereas sample D generated 34%. The starting content and chain length of aromatic sequences reduced the anaerobic biodegradation rate. Notably, insoluble degradation intermediates were expected alongside soluble intermediates, depending on how long it takes to hydrolyze aromatic trimers and oligomers to dimers and monomers. Aromatic oligomers and monomers degrade in sludge.
The biodegradable starch-based polymers Mater Bi and Ecostarplus behaved differently when biodegraded anaerobically (Day et al., 1994). In the Mater Bi samples, it appeared that the starch was readily attacked by bacteria, leading to significant CH4 evolution and weight loss. However, the Ecostarplus polymer demonstrated minimal CH4 evolution and weight reduction.
Many biocomposites include starch because of its inexpensive cost, high permeability, and natural biodegradability by microorganisms. Biodegradation occurs solely for starch in polyolefin composites, indicating that these composites were not fundamentally biodegradable. The starch in these composites only partially biodegrades owing to the presence of a starch matrix. Biodegradation rates for composites with a biodegradable matrix ranged from 10.2 to 53% (Yagi et al., 2014). During polymer degradation, new products such as CO2, H2O, or CH4 are formed, resulting in the formation of process end products (Lucas et al., 2008). Aerobic degradation necessitates the presence of oxygen as the final electron acceptor. During the degradation of plastics, aerobic conditions lead to the production of CO2 and H2O, as well as cellular biomass from microorganisms. When polymer biodegradation occurs in sulfidogenic conditions, CO2 and H2O are produced as byproducts. Anaerobic polymer degradation yields organic acids, H2O, CO2, and methane as byproducts. Anaerobic decomposition is less efficient for energy production than aerobic degradation, involving CO2 and SO4−2, because of the absence of O2, which serves as an electron acceptor (Gottschalk, 1986). Anaerobic degradation occurs through a different method, i.e., the burial of the polymer sets off a complex chain of chemical and biological reactions. Aerobic bacteria deplete oxygen in the buried materials. The following oxygen-depleted conditions initiate anaerobic biodegradation. In most cases, 3-m–thick layers prevent oxygen replenishment. It may begin with alternative electron acceptors, such as nitrate, sulfate, or methane. Any further addition of oxygen will stop the anaerobic degradation process (Glaser, 2019).
Environmental Waste: Biodegradable Plastic Production
Among all the environmental pollutants and contamination-causing agents, the accumulation of single-use plastic made from nonbiodegradable materials in the marine and terrestrial environments is very harmful and has toxic effects on human and marine health. Biodegradable plastic and bioplastic, which includes plastics composed of biodegradable polymers, as well as effective biobased recycling strategies offer the only realistic alternative that can achieve a safe and sustainable environment for humans and animals (Emadian et al., 2017; Abdelmoez et al., 2021). In an effort to reduce the massive plastic problem, the production and use of biodegradable plastic is more important role than other existing strategies. Many responsible countries, including the United States and China, have planned to increase the production facilities for biodegradable plastics and reduce the production of traditional, single-use plastics (Ma et al., 2020; Shen et al., 2020). Biodegradable plastics are mainly made from renewable resources, including biobased and fossil-fuel sources, such as food waste and agricultural residues (Havstad, 2020). Biodegradable plastics are the only realistic solution to the problem of plastic pollution in the environment.
Food Waste
Food waste is one of the main spreading waste worldwide and it is considered a cheap carbon source for the production of PHAs. High-quality poly-L-lactate (PLLA) biodegradable plastic was produced from food wastes through a combination of chemical processes and fermentation. A novel system composed of D, L-lactic acid removal by Propionibacterium from minced food waste, which was followed by the purification, esterification, and polymerization of L-lactic acid (Sakai et al., 2003). This process produced high quantities of PLLA with high activity, but used relatively little energy and had limited emissions. Furthermore, there was no difference in the physical properties of PLLA, produced by this method and PLLA produced commercial from L-lactic acid. Researchers have put significant effort into the production of PHA from food waste as the production of PHA from food waste is the best alternative to waste disposal.
Palm oil waste has been used as a carbon source for the synthesis of mcl-polyhydroxyalkanoates (mcl-PHAs) from the Pseudomonas sp. Gl01 strain (Fukui and Doi, 1998). The characterization showed that this polymer consisted of C6 to C16 monomers. Another study examined genetic modifications of E. coli that may enhance the synthesis of PHA from cheese whey and reduce the production of organic acid. P(3HB) homopolymer and P(3HB-co-5mol% 3HHx) copolymer were effectively synthesized by providing soybean oil as a carbon source to Cupriavidus necator H16 and its recombinant strain (Murugan et al., 2016). The malt waste from a beer brewery plant, soya waste, and sucrose were also investigated substrates for the production of PHAs; malt waste resulted in the highest production of polymers (Yu et al., 1999).
Agricultural Residues
Compared with traditional plastic, the production of biodegradable plastic consumes less energy and reduces waste, CO2, and greenhouse gas emissions. Biodegradable plastics produced from agricultural residue can enhance soil fertility. In the agricultural sector, biodegradable mulch films offer an environmentally friendly alternative to traditional plastic mulch film. Problems associated with traditional plastic mulch film, such as reduced fertilizer effectiveness, poor soil fertility, and malnutrition, can be prevented by using biodegradable mulch film. The production of biodegradable plastic from agricultural residues for the mulch film is an interesting solution to related problems. The plastic products used in our daily life can be produced from polymers that can rapidly degrade, reducing the pressure of landfill sites. Biodegradable polymers produced from renewable resources are likely to prove irreplaceable in high-value markets, such as medical sutures, drug-delivery materials, and orthopedic needles (Shen et al., 2009, 2020).
The production of PHA using cane molasses and the waste from ethanol production as a substrate has been reported from different parts of plants (Verlinden et al., 2007; Khiyami et al., 2011). PHB was produced from wheat straw and bagasse hydrolysate, which are renewable sources of agriculture residue (Yu and Chen, 2008; Galia, 2010). Xie et al. (2020) also studied the preparation of biopolymers film containing potato peel powder, curcumin, and bacterial cellulose. Their results showed that the incorporation of bacterial cellulose in the PP matrix increased the mechanical properties of the biopolymer films, reduced water vapor and oxygen permeability, and reduced light transmission.
Accumulating Bacteria
Scientists in this area of research prefer the use of microbes for the production of biodegradable plastic owing to their easy handling, maintenance, purification, and susceptibility to genetic modification for the desired properties (Mostafa et al., 2020; Narayanan et al., 2021). Polyhydroxybutyrates are macromolecules synthesized by bacteria. These are inclusion bodies accumulate when bacteria are subjected to stress. Polyhydroxybutyrates have similar properties to synthetic thermoplastics and good mechanical, physical, and immunological properties that make them an excellent biopolymer (Verlinden et al., 2007; Galia, 2010; Khiyami et al., 2011). To achieve industrial production, different microorganisms are being studied for their ability to produce different qualities and quantities of polyhydroxybutyrates with different decomposition rates (Natarajan, 2011; Juengert et al., 2018). Bacteria are most often used for their fabrication as they are able to produce the greatest biomass in the shortest time, and have simple downstream processes and more possibilities for gene modification compared with fungi, yeasts, and algae (Kumarasamy et al., 2020; Soman et al., 2020a,b). Azoto-bacter vinelandii, E. coli, Pseudomonas oleovorans, Halomonas campaniensis, Alteromonas lipolytica, Aeromonas sp., Bacillus megaterium, Methylobacteria sp., Herbaspirillum seropedicae, and Alcaligenes latus are the most commonly used of the 300+ bacterial species that can accumulate polyhydroxybutyrates and polyhydroxyalkanoates (Rendón-Villalobos et al., 2016; Shi et al., 2017; Tufail et al., 2017; Gabr, 2018). Potential PHB-producing bacterial strains were isolated and the production of PHB was evaluated using agricultural residues as the carbon source (Getachew and Woldesenbet, 2016). The optimum conditions for PHB production were pH 7, 37°C, and an incubation period of 48h with shaking at 120rpm.
Pyrolysis: Waste Into Energy
Oil can be described as a dark blood that flows through the veins of the global elite. As a major power source, it has brought prosperity to the developing world. However, the continued resources of oil are uncertain and can be expected to fluctuate dramatically owing to restrictions on supply. Power is important to all areas of society. The major improvements and developments in society over the past 200years have been driven by the use of nonrenewable energy resources. The maximum usage of petroleum products will soon be reached. Therefore, sound strategies for determined new sources are essential, especially for unrefined oil. It is important to consider several sources potential sources of oil; however, to obtain a realistic viewpoint, the factors that affect the production of hydrocarbons must be considered. The use of plastic waste and EH may play an important role in the production of bio-oil. In addition, changing the structure or the surface functionality of plastic using enzyme hydrolysis may improve the quality and yield of pyrolytic bio-oil.
Thermal Pyrolysis of PLA-PET
Polyethene terephthalate is an essential substance that is abundant in a variety of areas, such as food, electrical cables, and scientific equipment. Bioaccumulation and poisonous gasoline when igniting are common problems (Zimmermann, 2020). Therefore, as a necessity, the problem of plastic waste needs to be solved to prevent climate change and to improve human health. The typical recycling processes for plastic waste are shown in Figure 3. There are exclusive disposal strategies for PET waste, although all modern techniques have regulations. For example, incineration is a regular disposal method for solid urban waste (MSW; Kim et al., 2010). Although it may not be difficult to handle, and that it prevents an opportunity to reduce plastic waste, air pollutants such as dioxins, furans, mercury, and polychlorinated biphenyls are released (Diaz-Silvarrey et al., 2018). It could also result in the destruction of potential material that could have been disposed of as PET waste (Verma et al., 2016). In addition, constructs that live after PETs have been burned pollute the soil by leaching out metals such as lead and cadmium (Raheem et al., 2019). Reuse is also an option, and is considered as it is very environmentally friendly and prevents the destruction of items (Jamdar et al., 2017). In addition, sorting plastic before reuse requires extra labor (Gurgul et al., 2018). Therefore, a more feasible and reliable approach to PET waste disposal is required.
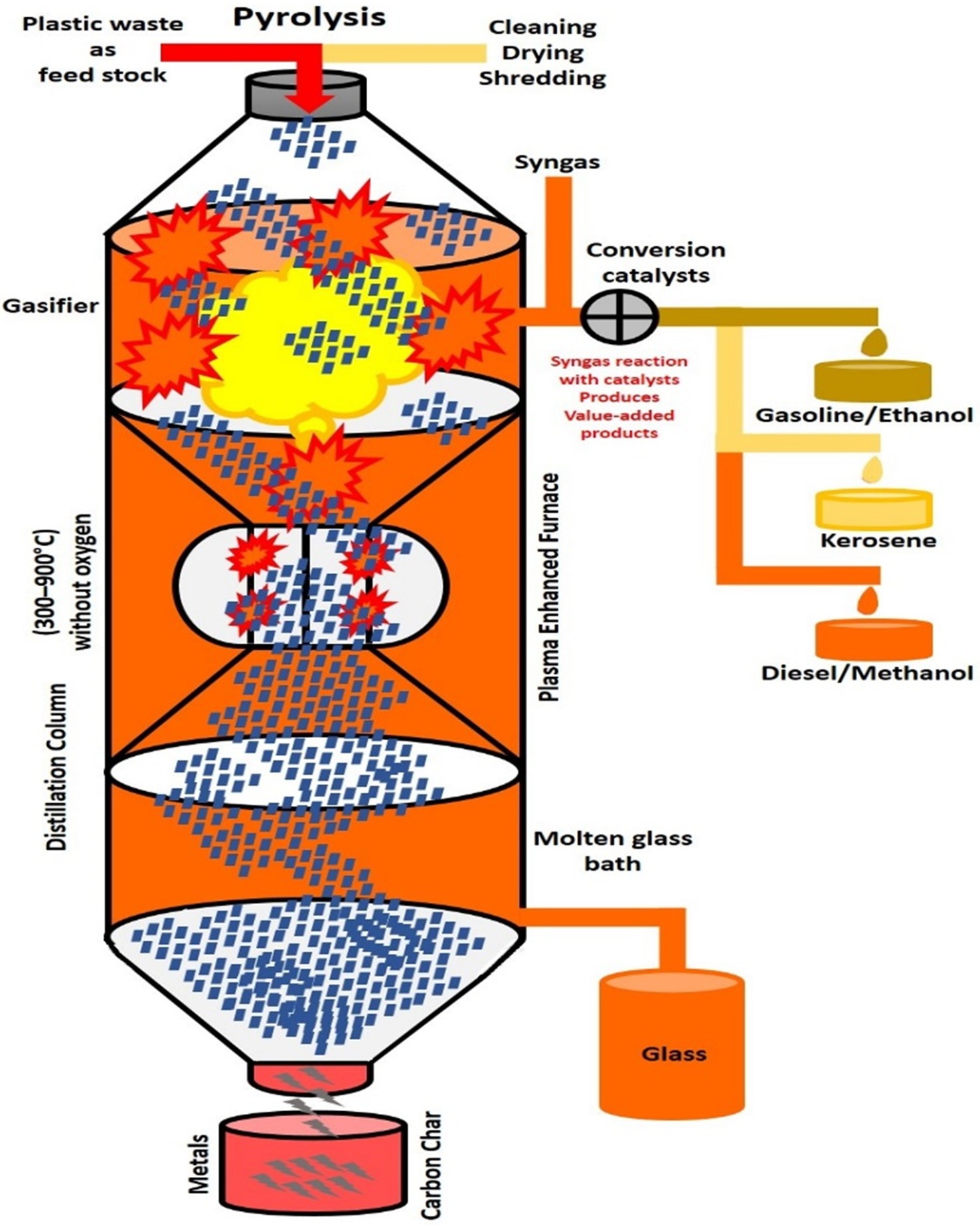
Figure 3. Pyrolytic process for the conversion of plastic waste into energy. Plastic to oil or other fuel (PTF) conversion technology is discussed here, as well as the possible reductions in CO2 emissions and other environmental advantages that might result if the Resources Recovery Authorities implemented these technologies. Plastics can be converted to oil or other fuels via pyrolysis. Before pyrolysis, plastic waste is shredded and cleaned for pretreatment, followed by the conversion of plastic waste to gas. The gas is converted to a liquid via distillation, and the acids formed during the breakdown of certain scrap plastics will be removed to prevent any corrosion of the PTF systems and engines. The final steps are the separating, refining, and blending of the fuel.
Another treatment method is pyrolysis, i.e., thermal treatment without oxygen (Kim et al., 2020c). This method has been used for various waste materials, including plastic (Kwon et al., 2019). The advantages are that it can reduce the amount of waste and transfer the estimated substances introduced by means of combined heat and power (Kim et al., 2020c). Valuable objects such as pyrolysis oil and gasoline have too great a thermal power, and this can be converted into a pyrolysis plant to satisfy the power requirements (Bridgwater and Peacocke, 2000). Heat treatment is considered because it is preferred not to require complex skills or extreme work cycles; moreover, it could not use plastic in any way like traditional techniques (Adrados et al., 2012). Although pyrolysis has several advantages over other methods of plastic waste recycling, its drawback is the generation of hazardous substances, such as polycyclic compounds and biphenyls, which can have to serious impact on ecological systems and human health if not properly managed (Kim et al., 2020d). For example, polycyclic aromatic compounds (PAHs) can be exchanged with particulate oxygen-containing PAHs, which are sometimes present in natural smoke concentrates, through a reaction with NOx and O3 (Gbeddy et al., 2020). To ensure a strong, natural approach to PET waste disposal, the whole process must be considered and polycyclic fragments should be avoided.
The pyrolysis of reagents has been used to study thermal efficiency and the incorporation of alternative pyrolytic objects (Lee et al., 2020). Although most studies on the pyrolysis of reagents have used zeolites, because of the current impetus to increase the yield of bio-oil and, similarly, to increase the reaction yield (Kim et al., 2019a). In addition, owing to the coke reinforcement, zeolites are not available at excessively high temperatures (Kim et al., 2019b). Upheld’s decent metal pulses are thermally stable and show excessive movement at excessive temperatures (Kim et al., 2020b); however, from now on they can be used in pyrolysis, which is conducted at high temperatures. For example, a Pt pulse, maintained in activated carbon, has been used extensively in the pyrolysis of food waste to reduce the build-up of harmful artificial species during pyrolysis (He et al., 2012).
Catalytic Fast Pyrolysis of PLA-PET
The biotreatment process of plastic waste is believed to enhance the performance of pyrolysis, including increased thermal conversion rate and selectivity, and a decrease in the required temperature. Many recent reports have discussed the importance of enzymatic treatment before the pyrolysis process for lignin, which is high molecular weight insoluble polymer. Unfortunately, there are no reports discussing the importance of enzymatic treatment before thermal pyrolysis of plastic waste. Enzymatic treatment of plastic waste before thermal pyrolysis can help to decrease the activation energy and pre-exponential factor of the thermal pyrolysis of plastic waste. Wang et al. (2020) used the enzymatic treatment of lignin before thermal pyrolysis, which significantly enhanced the performance and selectivity of pyrolysis at lower temperatures and decreased the activation energy.
Thermochemical measures (ignition, gasification, pyrolysis, and torrefaction) are among the best techniques to gain additional energy from starch biomass (Bridgwater, 2012). One of the more promising thermochemical methods, pyrolysis, produces a liquid powder. Rapid pyrolysis occurs between 300 and 500°C in an oxygen-free or oxygen-restricted atmosphere with high heat loads (>1,000°C/min) and a rapid vapor cooling period. Bio-oil yields in the range of 60–70% by weight cannot always be realized.
Bio-oil is a complex natural mixture that contains low-molecular-weight biomass. Normally, it is refined for different utilizations (Bridgwater et al., 2009). Lignocellulosic biomass feedstocks, such as rural deposits, are a water-based mixture of cellulose, hemicellulose, lignin, extractives, and minerals (P, K, Ca, Mg, and Na).
Many of these minerals help to catalyze secondary responses that alter the yields of liquid, solid, and gaseous products during fast pyrolysis (Akhtar and Amin, 2012). Excellent organic oil at some point in the pyrolysis depends on the desired product and the degree of oxygen-containing compounds, specific conditions were used, particularly to intensify the deoxygenation reactions at a certain stage of the pyrolysis, e.g., Zeolites and steel oxides (Zhang et al., 2009). After the oil has accumulated, various measures, such as hydrocracking, esterification, reagent degradation, etc. (Goldberg et al., 2015) were used to refine the bio-oil. In addition, the oil fit is critical to capacity and transportation so that it does not deteriorate with unpredictable additional polymer designs (Carlson et al., 2008). In one model, Atadana (2010) performed pyrolysis of the reagent with HZSM5 zeolite in biomass washed with NaOH to improve the yields of levoglucosan and caramel odor compounds. It was found that this reaction drove the formation of water at a certain stage of pyrolysis, which results in an excessive moisture content in the oil. In addition, zeolite pulses are quite expensive, which makes a cheaper alternative very attractive.
Lu et al. (2010) studied metal oxide nanoparticles in pyrolyzed wood waste (MgO, CaO, TiO2, Fe2O3, NiO, and ZnO). When all the solids were removed, it was discovered that the calcium oxide underwent major alterations and that the admission of anhydrous phenols, acids, and sugars had stopped. Pen tested the effect of calcium and potassium salts in the pyrolysis of biomass and a large number of carboxylic acids and furans and a lower oxygen content in the oil (Goldberg et al., 2015). Mullen et al. (2011) conducted a brief pyrolysis of sawdust in a fluidized-bed reactor using two successive Ca pulses. The two CO and CO2 pulses used in conjunction decreased the oxygen concentration of the oil. In addition, they found a reduced mean output with natural caustic. Wang et al. (2010) conducted investigations with two pulse types: acid zeolite MCM41 and essential CaO. The pyrolysis was performed on a TGA-FTIR for the advantageous cluster area, although it is not always a short pyrolysis because the heating load is only 90°C/min. Their results verified that the yield of corrosives decreased, along with a range of hydrocarbons. Acid production was reduced by the use of CaO, and the CaCO3 that was created had the potential to degrade into ketones and water. Calcium oxide has been reported by Lin et al. (2010) to induce the pyrolysis of pine sawdust in a fluidized-bed reaction on the scale of a study facility (180–1,000g/h). The percentage of biomass/pulse was modified (0–30%). The yields of formic acid, levoglucosan, phenol, acetone, and guaiacol decreased as the content of CaO was increased, and various mineral impulses were also tested (Beaumont and Schwob, 1984). Overall, reactive pyrolysis is attractive when you consider that it benefits from the warm inert temperature of the vapors after pyrolysis.
During a synergistic in situ pyrolysis the impulse fragments are mixed with (Lin et al., 2009) or essentially displace the fluidizing medium (Williams and Nugranad, 2000). Unfortunately, this type of association typically has problems with catalyst deactivation and requires chronic pulse regeneration and replacement. In contrast, ex situ reagent pyrolysis structures generally have a solid cushion after the fluidization sector of the reactor. At the same time, this type of structure has much smaller deactivation momentum, which increases domestic smoke cases and improves response to polling breaks. This shows the potential of in situ synergistic pyrolysis to provide higher caliber oils.
Challenges Associated With Thermal Pyrolysis of PLA-PET
Recycling plastic waste has emerged as one method of reducing plastic pollution; however, this generally requires large labor costs and, at some stages in processing, create ignited water. If plastic recycling can support the liquids with tribological effects, such as lubricating oils, this is a convenient pathway to eliminate plastics in the environment. To understand potential tribological uses of recycled plastic, it is important to study techniques that can be used to convert waste plastic into completely petroleum-based oils. The viscosity, density, and friction of pyrolyzed waste plastic oils are examined and evaluated in comparison to commercial lubricants to determine their function and lubrication effects. Segregation strategies, catalytic isomerization dewaxing, and the Fischer-Tropsch method for recycling plastic waste have also been examined to obtain insight into techniques for converting pyrolyzed plastic waste into lubricants (Sikdar et al., 2020).
The separation of other plastics from PLA and PET can be difficult can be difficult; naturally, this impacts the ability to recycle these materials. The purpose of this test is to examine the feasibility of recycling mixed PLA and PET waste via a copyrolysis method. Samples containing significant levels of pre-processed PLA and PET waste were subjected to the relevant studies using thermogravimetric (TG) and reaction kinetic analyses. Finally, pyrolytic studies based solely on the determined TG reaction conditions were completed to investigate the yields of the pyrolytic reactions. The results indicated that the HHV of PLA and domestic animal was approximately 18.26 and 22.85MJ/kg, respectively, and that of the combinations fell between those values. Each sample has a combustible fraction of more than 96% and a maximum decomposition temperature between 618 and 736K. Additional PET ratios were determined to lead to higher activation energies and pre-exponential elements. In addition, the PLA ratios were correlated with the mass yield of carbonated goods, whereas the PET ratios were correlated with the yields of resistant and condensing products. To avoid potential issues with strength and performance, the copyrolysis of PLA and PET can also mitigate the need to decompose PLA and PET, which can contribute to a reduction in plastic waste (Tai, 2015).
The linear economic system for plastic packaging, which currently results in the emission of too much CO2 and the release of plastic into the environment, needs to be reformed into a more spherical and ecologically focused model that is resource- and environmentally friendly throughout the plastics value chain, from product develop, to recycling, and with the abandonment of existing alternatives. This review highlights areas in which those who are inexperienced in chemistry can make contributions. Replacing plastics derived from fossil fuel sources with fully renewable biobased options can reduce greenhouse gas emissions and produce plastics that are much less complex to recycle and biodegrade at the end of their useful existence in the environment. The underlying chemical and biocatalytic production for plastic production and recycling has been reviewed and priorities for future improvements have been identified (Sheldon and Norton, 2020).
Polymers such as polyamides or nylon, PET, polycarbonate (PC), and polyurethane (PE) are known as condensation polymers because, in addition to carbon (C) atoms, they consist of certain atoms such as oxygen (O) and nitrogen (N). They can be degraded by hydrolytic cleavage as hydrophilic ester or amide bonds are present in their monomers. Biodegradation begins at the same time that the molecular weight of the polymers reaches several thousands, which is usually a low value for commercial polymers (Bonhomme et al., 2003). Another difficulty affecting the biodegradation and pyrolysis of polymers is their crystallinity. The specific physical phenomena that occur within the pyrolysis pathway depend on the material. Thermosetting polymers are insoluble and therefore their modification phase is not feasible during pyrolysis. However, thermoplastics can be softened within the pyrolysis reaction with reversible phase modifications until a minimum pyrolysis temperature is reached (Brostow and Lobland, 2016). There are no completely crystalline polymers, but there are completely amorphous polymers. A crystalline material under a given voltage has a single melting temperature, but no variety; similarly, the glass transition occurs over a variety of temperatures, but not at a single temperature.
The biodegradation of polymers is based on the chemical and physical properties of the polymer. The crystallinity and molecular weight of the polymer are key factors that help biodegradation. Increasing the molecular weight of a polymer reduces its degradability (Tokiwa et al., 2009). For example, polymeric polycaprolactone (PCL) with a molecular weight of >4,000 is slowly degraded by a hydrolysis pathway using a Rhizopus delemar lipase, unlike PCL that has a lower molecular weight. Various enzymes from microorganisms are a useful resource for the biodegradation of polymers through oxidation and hydrolysis processes. Crystallinity also affects the rate of biodegradation in biopolymers. In the absence of additives, many commercial polymeric substances may encounter obstacles to their use. However, it was found that additives such as phthalates and bisphenol can damage human health through their impact on natural reactions (Meeker et al., 2009).
Conclusion and Future Perspective
The enormous problems arising from the accumulation of different plastic wastes has attracted the attention of scientists worldwide. The correct use of this waste may be a “hidden treasure,” especially for the conversion and production of various value-added products. A variety of plastic-degrading enzymes have been recently discovered, widening the potential applications for mixed-plastic waste biodegradations. Moreover, there is a very urgent need for safe and nontoxic alternatives to fossil fuel-based plastics, such as biodegradable plastics like polyhydroxyalkanoates, which can be more easily degraded by microorganisms. Diethyl-2,5-furandicarboxylate and diethyl isophthalate are also promising alternatives to fossil fuel-based plastics and can be easily produced by enzymatic catalysis. Biodegradable plastics can be produced from food waste, agricultural residues, and polyhydroxybutyrate-accumulating bacteria. The thermal pyrolysis of mixed-plastic waste is also a very important method that may overcome the depletion of natural crude oil. A possible hybrid integration technique to incorporate thermostable plastic degradation enzymes in the conversion into fuel oils requires further investigations. The discovery of highly thermostable enzymes is essential for the development of mixed-plastic waste thermal pyrolysis.
Author Contributions
MT, NS, YJ, MM, HS, MB, and JX wrote the manuscript. MT drew all the figures. NS and JX designed the paper concept and revised the manuscript. All authors contributed to the article and approved the submitted version.
Funding
This article acknowledges the funding support provided by the National Natural Science Foundation of China (grant numbers 31961133017, 31961133018, and 31961133019). These grants are part of the “MIXed plastics biodegradation and UPcycling using microbial communities” MIX-UP research project, which is a joint NSFC and EU H2020 collaboration. In Europe, MIX-UP has received funding from the European Union’s Horizon 2020 research and innovation program under grant agreement no. 870294.
Conflict of Interest
The authors declare that the research was conducted in the absence of any commercial or financial relationships that could be con.strued as a potential conflict of interest.
Publisher’s Note
All claims expressed in this article are solely those of the authors and do not necessarily represent those of their affiliated organizations, or those of the publisher, the editors and the reviewers. Any product that may be evaluated in this article, or claim that may be made by its manufacturer, is not guaranteed or endorsed by the publisher.
Acknowledgments
We would like to acknowledge the Chinese Government and the University of Chinese Academy of Sciences for the financial support of the Ph.D. scholarship awarded to MT.
References
Abdelmoez, W., Dahab, I., Ragab, E. M., Abdelsalam, O. A., and Mustafa, A. (2021). Bio-and oxo-degradable plastics: insights on facts and challenges. Polym. Adv. Technol. 32, 1981–1996. doi: 10.1002/pat.5253
Abdel-Raouf, N., Al-Homaidan, A. A., and Ibraheem, I. B. M. (2012). Microalgae and wastewater treatment. Saudi J. Biol. Sci. 19, 257–275. doi: 10.1016/j.sjbs.2012.04.005
Abraham, J., Ghosh, E., Mukherjee, P., and Gajendiran, A. (2016). Microbial degradation of low density polyethylene. Environ. Prog. Sustain. Energy 36, 147–154. doi: 10.1002/ep.12467
Acero, E. H., Ribitsch, D., Steinkellner, G., Gruber, K., Greimel, K., Eiteljoerg, I., et al. (2011). Enzymatic surface hydrolysis of PET: effect of structural diversity on kinetic properties of cutinases from Thermobifida. Macromolecules 44, 4632–4640. doi: 10.1021/ma200949p
Adrados, A., De Marco, I., Caballero, B. M., López, A., Laresgoiti, M. F., and Torres, A. (2012). Pyrolysis of plastic packaging waste: a comparison of plastic residuals from material recovery facilities with simulated plastic waste. Waste Manag. 32, 826–832. doi: 10.1016/j.wasman.2011.06.016
Akhtar, J., and Amin, N. S. (2012). A review on operating parameters for optimum liquid oil yield in biomass pyrolysis. Renew. Sust. Energ. Rev. 16, 5101–5109. doi: 10.1016/j.rser.2012.05.033
Akutsu-Shigeno, Y., Teeraphatpornchai, T., Teamtisong, K., Nomura, N., Uchiyama, H., Nakahara, T., et al. (2003). Cloning and sequencing of a poly(DL-lactic acid) depolymerase gene from Paenibacillus amylolyticus strain TB-13 and its functional expression in Escherichia coli. Appl. Environ. Microbiol. 69, 2498–2504. doi: 10.1128/AEM.69.5.2498-2504.2003
Allen, N. S., and Edge, M. (2021). Perspectives on additives for polymers. 1. Aspects of stabilization. J. Vinyl Addit. Technol. 27, 5–27. doi: 10.1002/vnl.21807
Alshehrei, F. (2017). Biodegradation of synthetic and natural plastic by microorganisms. J. Appl. Environ. Microbiol. 5, 8–19. doi: 10.12691/jaem-5-1-2
Aresta, M., Dibenedetto, A., and Barberio, G. (2005). Utilization of macro-algae for enhanced CO2 fixation and biofuels production: development of a computing software for an LCA study. Fuel Process. Technol. 86, 1679–1693. doi: 10.1016/j.fuproc.2005.01.016
Arora, K., Kaur, P., Kumar, P., Singh, A., Patel, S. K. S., Li, X., et al. (2021). Valorization of wastewater resources into biofuel and value-added products using microalgal system. Front. Energy Res. 9:119. doi: 10.3389/fenrg.2021.646571
ASTM International (2017). "ASTM D883-17 Standard Terminology Relating to Plastics." Pennsylvania, PA, USA.
Atadana, F. W. (2010). Catalytic pyrolysis of cellulose, hemicellulose and lignin model compounds. Virginia Tech.
Barth, M., Honak, A., Oeser, T., Wei, R., Belisário-Ferrari, M. R., Then, J., et al. (2016). A dual enzyme system composed of a polyester hydrolase and a carboxylesterase enhances the biocatalytic degradation of polyethylene terephthalate films. Biotechnol. J. 11, 1082–1087. doi: 10.1002/biot.201600008
Baskaran, D., and Rajamanickam, R. (2019). Aerobic biodegradation of trichloroethylene by consortium microorganism from Turkey litter compost. J. Environ. Chem. Eng. 7:103260. doi: 10.1016/j.jece.2019.103260
Beaumont, O., and Schwob, Y. (1984). Influence of physical and chemical parameters on wood pyrolysis. Ind. Eng. Chem. Process. Des. Dev. 23, 637–641. doi: 10.1021/i200027a002
Bhuyan, M. S., Venkatramanan, S., Selvam, S., Szabo, S., Hossain, M. M., Rashed-Un-Nabi, M., et al. (2021). Plastics in marine ecosystem: a review of their sources and pollution conduits. Reg. Stud. Mar. Sci. 41:101539. doi: 10.1016/j.rsma.2020.101539
Bhuyar, P., Muniyasamy, S., and Govindan, N. (2019). Biodegradation of plastic waste by using microalgae and their toxins. J. Biotechnol. Biomater. 8:55.
Billig, S., Oeser, T., Birkemeyer, C., and Zimmermann, W. (2010). Hydrolysis of cyclic poly(ethylene terephthalate) trimers by a carboxylesterase from Thermobifida fusca KW3. Appl. Microbiol. Biotechnol. 87, 1753–1764. doi: 10.1007/s00253-010-2635-y
Biundo, A., Reich, J., Ribitsch, D., and Guebitz, G. M. (2018). Synergistic effect of mutagenesis and truncation to improve a polyesterase from Clostridium botulinum for polyester hydrolysis. Sci. Rep. 8:3745. doi: 10.1038/s41598-018-21825-9
Blanco, I., Pantani, R., Pizzi, A., and Sorrentino, A. (2020). State-of-the-art polymer science and technology in Italy. Polymers 12:1721. doi: 10.3390/polym12081721
Bonhomme, S., Cuer, A., Delort, A., Lemaire, J., Sancelme, M., and Scott, G. (2003). Environmental biodegradation of polyethylene. Polym. Degrad. Stab. 81, 441–452. doi: 10.1016/S0141-3910(03)00129-0
Brandt, A. R. (2011). Oil depletion and the energy efficiency of oil production: the case of California. Sustainability 3, 1833–1854. doi: 10.3390/su3101833
Bridgwater, A. V. (2012). Review of fast pyrolysis of biomass and product upgrading. Biomass Bioenergy 38, 68–94. doi: 10.1016/j.biombioe.2011.01.048
Bridgwater, A., Hofbauer, H., and Van Loo, S. (2009). Thermal Biomass Conversion. United Kingdom: CPL Press.
Bridgwater, A., and Peacocke, G. (2000). Fast pyrolysis processes for biomass. Renew. Sust. Energ. Rev. 4, 1–73. doi: 10.1016/S1364-0321(99)00007-6
Briški, F., and Domanovac, M. V. (2017). Environmental microbiology. Phys. Sci. Rev. 2:20160118. doi: 10.1515/psr-2016-0118
Brostow, W., and Lobland, H. E. H. (2016). Materials: Introduction and Applications. United States: John Wiley & Sons.
Carlson, T. R., Vispute, T. P., and Huber, G. W. (2008). Green gasoline by catalytic fast pyrolysis of solid biomass derived compounds. ChemSusChem 1, 397–400. doi: 10.1002/cssc.200800018
Carmen, S. (2021). Microbial capability for the degradation of chemical additives present in petroleum-based plastic products: a review on current status and perspectives. J. Hazard. Mater. 402:123534. doi: 10.1016/j.jhazmat.2020.123534
Carniel, A., Valoni, É., Nicomedes, J., Gomes, A. D. C., and Castro, A. M. D. (2017). Lipase from Candida antarctica (CALB) and cutinase from Humicola insolens act synergistically for PET hydrolysis to terephthalic acid. Process Biochem. 59, 84–90. doi: 10.1016/j.procbio.2016.07.023
Chaillan, F., Le Flèche, A., Bury, E., Phantavong, Y. H., Grimont, P., Saliot, A., et al. (2004). Identification and biodegradation potential of tropical aerobic hydrocarbon-degrading microorganisms. Res. Microbiol. 155, 587–595. doi: 10.1016/j.resmic.2004.04.006
Chandra, R., and Rustgi, R. (1998). Biodegradable polymers. Prog. Polym. Sci. 23, 1273–1335. doi: 10.1016/S0079-6700(97)00039-7
Chia, W. Y., Tang, D. Y. Y., Khoo, K. S., Lup, A. N. K., and Chew, K. W. (2020). Nature’s fight against plastic pollution: algae for plastic biodegradation and bioplastics production. Environ. Sci. Ecotechnol. 4:100065. doi: 10.1016/j.ese.2020.100065
Cossu, R., Morello, L., and Stegmann, R. (2018). “Biochemical processes in landfill,” in Solid Waste Landfilling: Concepts, Processes, Technologies. eds. R. Cossu and R. Stegmann (Amsterdam, The Netherlands: Elsevier), 91–115.
Danso, D., Schmeisser, C., Chow, J., Zimmermann, W., Wei, R., Leggewie, C., et al. (2018). New insights into the function and global distribution of polyethylene terephthalate (PET)-degrading bacteria and enzymes in marine and terrestrial metagenomes. Appl. Environ. Microbiol. 84, e02773–e02717. doi: 10.1128/AEM.02773-17
Das, S. K., Sathish, A., and Stanley, J. (2018). Production of biofuel and bioplastic from Chlorella pyrenoidosa. Mater. Today Proc. 5, 16774–16781. doi: 10.1016/j.matpr.2018.06.020
Day, M., Shaw, K., and Cooney, D. (1994). Biodegradability: an assessment of commercial polymers according to the Canadian method for anaerobic conditions. J. Environ. Polym. Degrad. 2, 121–127. doi: 10.1007/BF02074780
De Castro, A. M., Carniel, A., Junior, J. N., Da Conceição Gomes, A., and Valoni, É. (2017). Screening of commercial enzymes for poly(ethylene terephthalate; PET) hydrolysis and synergy studies on different substrate sources. J. Ind. Microbiol. Biotechnol. 44, 835–844. doi: 10.1007/s10295-017-1942-z
Demirbas, A. (2010). Use of algae as biofuel sources. Energy Convers. Manag. 51, 2738–2749. doi: 10.1016/j.enconman.2010.06.010
Diaz-Silvarrey, L. S., Mcmahon, A., and Phan, A. N. (2018). Benzoic acid recovery via waste poly (ethylene terephthalate)(PET) catalytic pyrolysis using sulphated zirconia catalyst. J. Anal. Appl. Pyrolysis 134, 621–631. doi: 10.1016/j.jaap.2018.08.014
Dimarogona, M., Nikolaivits, E., Kanelli, M., Christakopoulos, P., Sandgren, M., and Topakas, E. (2015). Structural and functional studies of a Fusarium oxysporum cutinase with polyethylene terephthalate modification potential. Biochim. Biophys. Acta 1850, 2308–2317. doi: 10.1016/j.bbagen.2015.08.009
Emadian, S. M., Onay, T. T., and Demirel, B. (2017). Biodegradation of bioplastics in natural environments. Waste Manag. 59, 526–536. doi: 10.1016/j.wasman.2016.10.006
Falah, W., Chen, F.-J., Zeb, B., Hayat, T., Mahmood, Q., Ebadi, A., et al. (2020). Polyethylene terephthalate degradation by microalga Chlorella vulgaris along with pretreatment. Mater. Plast. 57, 260–270. doi: 10.37358/MP.20.3.5398
Fritsche, W., and Hofrichter, M. (2005). “Aerobic degradation of recalcitrant organic compounds by microorganisms,” in Environmental Biotechnology Concepts and Applications. eds. H. J. Jördening and J. Winter (Weinheim, Germany: Wiley-VCH), 203–227.
Fukui, T., and Doi, Y. (1998). Efficient production of polyhydroxyalkanoates from plant oils by Alcaligenes eutrophus and its recombinant strain. Appl. Microbiol. Biotechnol. 49, 333–336. doi: 10.1007/s002530051178
Gabr, G. A. (2018). Isolation and identification of bacterial strains able to biopolymer polyhydroxybutyrate (Phb) production from soil of Al-Kharj probes, Saudi Arabia. J. Pharm. Res. Int. 21, 1–11. doi: 10.9734/JPRI/2018/39532
Gala, A., Guerrero, M., and Serra, J. M. (2020). Characterization of post-consumer plastic film waste from mixed MSW in Spain: a key point for the successful implementation of sustainable plastic waste management strategies. Waste Manag. 111, 22–33. doi: 10.1016/j.wasman.2020.05.019
Galia, M. B. (2010). “Isolation and analysis of storage compounds,” in Handbook of Hydrocarbon and Lipid Microbiology. ed. K. N. Timmis (Berlin: Springer), 3725–3741.
Gamerith, C., Gajda, M., Ortner, A., Acero, E. H., Guebitz, G. M., and Ulbricht, M. (2017). Enzymatic hydrolysis of poly(ethyleneterephthalate) used for and analysed by pore modification of track-etched membranes. New Biotechnol. 39, 42–50. doi: 10.1016/j.nbt.2017.06.007
Gbeddy, G., Goonetilleke, A., Ayoko, G. A., and Egodawatta, P. (2020). Transformation and degradation of polycyclic aromatic hydrocarbons (PAHs) in urban road surfaces: influential factors, implications and recommendations. Environ. Pollut. 257:113510. doi: 10.1016/j.envpol.2019.113510
Getachew, A., and Woldesenbet, F. (2016). Production of biodegradable plastic by polyhydroxybutyrate (PHB) accumulating bacteria using low cost agricultural waste material. BMC Res. Notes 9:509. doi: 10.1186/s13104-016-2321-y
Ghosh, S., Qureshi, A., and Purohit, H. (2019). “Microbial degradation of plastics: biofilms and degradation pathways,” in Contaminants in Agriculture and Environment: Health Risks and Remediation. Vol. 1. eds. V. Kumar, R. Kumar, J. Singh, and P. Kumar (Haridwar, India: Agro Environ Media), 184–199.
Glaser, J. (2019). “Biological degradation of polymers in the environment,” in Plastics in the Environment. ed. A. Gomiero (London, United Kingdom: IntechOpen).
Goldberg, N. M., Dallmer, M. F., Boateng, A. A., Mullen, C. A., and Milhalcik, D. J. (2015). "Fast pyrolysis catalytic cracking pipe for producing bio-oils." Google Patents.
Gottschalk, G. (1986). “Bacterial fermentations,” in Bacterial Metabolism. New York: Springer, 208–282.
Goudriaan, F., Naber, J. A., Van De Beld, B., Boerefijn, F. R., Van Der Wal, S., Bos, G. M., et al. (2000). “Thermal efficiency of the HTU process for biomass liquefaction,” in Progress in Thermochemical Biomass Conversion. ed. A. Bridgwater (Austria: Tyrol), 1312–1325.
Griswold, K., Mahmood, N., Iverson, B., and Georgiou, G. (2003). Effects of codon usage versus putative 5'-mRNA structure on the expression of Fusarium solani cutinase in the Escherichia coli cytoplasm. Protein Expr. Purif. 27, 134–142. doi: 10.1016/S1046-5928(02)00578-8
Gunawan, N. R., Tessman, M., Schreiman, A. C., Simkovsky, R., Samoylov, A. A., Neelakantan, N. K., et al. (2020). Rapid biodegradation of renewable polyurethane foams with identification of associated microorganisms and decomposition products. Bioresour. Technol. Rep. 11:100513. doi: 10.1016/j.biteb.2020.100513
Gupta, A., Gupta, R., and Singh, R. L. (2017). “Microbes and environment,” in Principles and Applications of Environmental Biotechnology for a Sustainable Future. ed. R. L. Singh (Singapore: Springer Singapore), 43–84.
Gurgul, A., Szczepaniak, W., and Zabłocka-Malicka, M. (2018). Incineration and pyrolysis vs. steam gasification of electronic waste. Sci. Total Environ. 624, 1119–1124. doi: 10.1016/j.scitotenv.2017.12.151
Hajighasemi, M., Nocek, B. P., Tchigvintsev, A., Brown, G., Flick, R., Xu, X., et al. (2016). Biochemical and structural insights into enzymatic depolymerization of polylactic acid and other polyesters by microbial carboxylesterases. Biomacromolecules 17, 2027–2039. doi: 10.1021/acs.biomac.6b00223
Han, J., Zhang, L., Wang, S., Yang, G., Zhao, L., and Pan, K. (2016). Co-culturing bacteria and microalgae in organic carbon containing medium. J. Biol. Res. 23:8. doi: 10.1186/s40709-016-0047-6
Havstad, M. R. (2020). “Biodegradable plastics,” in Plastic Waste and Recycling. ed. T. Letcher (Amsterdam: Elsevier), 97–129.
He, C., Li, J., Zhang, X., Yin, L., Chen, J., and Gao, S. (2012). Highly active Pd-based catalysts with hierarchical pore structure for toluene oxidation: catalyst property and reaction determining factor. Chem. Eng. J. 180, 46–56. doi: 10.1016/j.cej.2011.10.099
Hegazy, E. A., Abdel-Rehim, H., Diaa, D. A., and El-Barbary, A. (2009). Controlling of Degradation Effects in Radiation Processing of Polymers. Vienna, Austria: International Atomic Energy Agency, 67–84.
Hermanová, S., Šmejkalová, P., Merna, J., and Zarevúcka, M. (2015). Biodegradation of waste PET based copolyesters in thermophilic anaerobic sludge. Polym. Degrad. Stab. 111, 176–184. doi: 10.1016/j.polymdegradstab.2014.11.007
Hopewell, J., Dvorak, R., and Kosior, E. (2009). Plastics recycling: challenges and opportunities. Philos. Trans. R. Soc. Lond. Ser. B Biol. Sci. 364, 2115–2126. doi: 10.1098/rstb.2008.0311
Huang, X., Cao, L., Qin, Z., Li, S., Kong, W., and Liu, Y. (2018). Tat-independent secretion of polyethylene terephthalate hydrolase PETase in Bacillus subtilis 168 mediated by its native signal peptide. J. Agric. Food Chem. 66, 13217–13227. doi: 10.1021/acs.jafc.8b05038
Jabloune, R., Khalil, M., Ben Moussa, I. E., Simao-Beaunoir, A. M., Lerat, S., Brzezinski, R., et al. (2020). Enzymatic degradation of p-nitrophenyl esters, polyethylene terephthalate, cutin, and suberin by Sub1, a suberinase encoded by the plant pathogen streptomyces scabies. Microbes Environ. 35:ME19086. doi: 10.1264/jsme2.ME19086
Jakubowicz, I. (2003). Evaluation of degradability of biodegradable polyethylene (PE). Polym. Degrad. Stab. 80, 39–43. doi: 10.1016/S0141-3910(02)00380-4
Jamdar, V., Kathalewar, M., Dubey, K. A., and Sabnis, A. (2017). Recycling of PET wastes using electron beam radiations and preparation of polyurethane coatings using recycled material. Prog. Org. Coat. 107, 54–63. doi: 10.1016/j.porgcoat.2017.02.007
Janczak, K., Dąbrowska, G. B., Raszkowska-Kaczor, A., Kaczor, D., Hrynkiewicz, K., and Richert, A. (2020). Biodegradation of the plastics PLA and PET in cultivated soil with the participation of microorganisms and plants. Int. Biodeterior. Biodegradation 155:105087. doi: 10.1016/j.ibiod.2020.105087
Jarerat, A., and Tokiwa, Y. (2001). Degradation of poly(L-lactide) by a fungus. Macromol. Biosci. 1, 136–140. doi: 10.1002/1616-5195(20010601)1:4<136::AID-MABI136>3.0.CO;2-3
Jin, Q., and Kirk, M. F. (2018). pH as a primary control in environmental microbiology: 1. Thermodynamic perspective. Front. Environ. Sci. 6:21. doi: 10.3389/fenvs.2018.00021
Juengert, J. R., Bresan, S., and Jendrossek, D. (2018). Determination of polyhydroxybutyrate (PHB) content in Ralstonia eutropha using gas chromatography and Nile red staining. Bio. Protoc. 8:e2748. doi: 10.21769/BioProtoc.2748
Kaewbai-Ngam, A., Incharoensakdi, A., and Monshupanee, T. (2016). Increased accumulation of polyhydroxybutyrate in divergent cyanobacteria under nutrient-deprived photoautotrophy: an efficient conversion of solar energy and carbon dioxide to polyhydroxybutyrate by Calothrix scytonemicola TISTR 8095. Bioresour. Technol. 212, 342–347. doi: 10.1016/j.biortech.2016.04.035
Kanelli, M., Vasilakos, S., Nikolaivits, E., Ladas, S., Christakopoulos, P., and Topakas, E. (2015). Surface modification of poly(ethylene terephthalate; PET) fibers by a cutinase from Fusarium oxysporum. Process Biochem. 50, 1885–1892. doi: 10.1016/j.procbio.2015.08.013
Karigar, C. S., and Rao, S. S. (2011). Role of microbial enzymes in the bioremediation of pollutants: a review. Enzyme Res. 2011:805187. doi: 10.4061/2011/805187
Kawai, F. (2010). "Polylactic acid (PLA)-degrading microorganisms and PLA depolymerases," in ACS Symposium Series.
Kawai, F., Oda, M., Tamashiro, T., Waku, T., Tanaka, N., Yamamoto, M., et al. (2014). A novel Ca2+−activated, thermostabilized polyesterase capable of hydrolyzing polyethylene terephthalate from Saccharomonospora viridis AHK190. Appl. Microbiol. Biotechnol. 98, 10053–10064. doi: 10.1007/s00253-014-5860-y
Khiyami, M. A., Al-Fadual, S. M., and Bahklia, A. H. (2011). Polyhydroxyalkanoates production via Bacillus plastic composite support (PCS) biofilm and date palm syrup. J. Med. Plant Res. 5, 3312–3320.
Kim, S., Kwon, E. E., Kim, Y. T., Jung, S., Kim, H. J., Huber, G. W., et al. (2019a). Recent advances in hydrodeoxygenation of biomass-derived oxygenates over heterogeneous catalysts. Green Chem. 21, 3715–3743. doi: 10.1039/C9GC01210A
Kim, S., Lee, C.-G., Kim, Y. T., Kim, K.-H., and Lee, J. (2020b). Effect of Pt catalyst on the condensable hydrocarbon content generated via food waste pyrolysis. Chemosphere 248:126043. doi: 10.1016/j.chemosphere.2020.126043
Kim, S., Lee, Y., Lin, K.-Y. A., Hong, E., Kwon, E. E., and Lee, J. (2020c). The valorization of food waste via pyrolysis. J. Clean. Prod. 259:120816. doi: 10.1016/j.jclepro.2020.120816
Kim, S., Park, C., and Lee, J. (2020d). Reduction of polycyclic compounds and biphenyls generated by pyrolysis of industrial plastic waste by using supported metal catalysts: a case study of polyethylene terephthalate treatment. J. Hazard. Mater. 392:122464. doi: 10.1016/j.jhazmat.2020.122464
Kim, J. W., Park, S.-B., Tran, Q.-G., Cho, D.-H., Choi, D.-Y., Lee, Y. J., et al. (2020a). Functional expression of polyethylene terephthalate-degrading enzyme (PETase) in green microalgae. Microb. Cell Factories 19:97. doi: 10.1186/s12934-020-01355-8
Kim, S., Tsang, Y. F., Kwon, E. E., Lin, K.-Y. A., and Lee, J. (2019b). Recently developed methods to enhance stability of heterogeneous catalysts for conversion of biomass-derived feedstocks. Korean J. Chem. Eng. 36, 1–11. doi: 10.1007/s11814-018-0174-x
Kim, S. B., Yi, N. H., Kim, H. Y., Kim, J.-H. J., and Song, Y.-C. (2010). Material and structural performance evaluation of recycled PET fiber reinforced concrete. Cement Concrete Comp. 32, 232–240. doi: 10.1016/j.cemconcomp.2009.11.002
Kitadokoro, K., Kakara, M., Matsui, S., Osokoshi, R., Thumarat, U., Kawai, F., et al. (2019). Structural insights into the unique polylactate-degrading mechanism of Thermobifida alba cutinase. FEBS J. 286, 2087–2098. doi: 10.1111/febs.14781
Koshti, R., Mehta, L., and Samarth, N. (2018). Biological recycling of polyethylene terephthalate: a mini-review. J. Polym. Environ. 26, 3520–3529. doi: 10.1007/s10924-018-1214-7
Kumar, A., and Chandra, R. (2020). Ligninolytic enzymes and its mechanisms for degradation of lignocellulosic waste in environment. Heliyon 6:e03170. doi: 10.1016/j.heliyon.2020.e03170
Kumarasamy, S., Subramanian, V., Narayanan, M., and Ranganathan, M. (2020). Microbial stereo inversion of (R) 3 chloro-1, 2-propandiol by Wickerhamomyces anomalous MGR6-KY209903. Bioin. Res. App. Chem. 10, 6157–6166. doi: 10.33263/BRIAC105.61576166
Kwon, E. E., Kim, S., and Lee, J. (2019). Pyrolysis of waste feedstocks in CO2 for effective energy recovery and waste treatment. J. CO2 Util. 31, 173–180. doi: 10.1016/j.jcou.2019.03.015
Lear, G., Kingsbury, J. M., Franchini, S., Gambarini, V., Maday, S. D. M., Wallbank, J. A., et al. (2021). Plastics and the microbiome: impacts and solutions. Environ. Microb. 16:2. doi: 10.1186/s40793-020-00371-w
Lee, J., Kwon, E. E., and Park, Y.-K. (2020). Recent advances in the catalytic pyrolysis of microalgae. Catal. Today 355, 263–271. doi: 10.1016/j.cattod.2019.03.010
Lee, J. C., Moon, J. H., Jeong, J.-H., Kim, M. Y., Kim, B. M., Choi, M.-C., et al. (2016). Biodegradability of poly(lactic acid; PLA)/lactic acid (LA) blends using anaerobic digester sludge. Macromol. Res. 24, 741–747. doi: 10.1007/s13233-016-4100-y
Lee, S. H., and Song, W. S. (2011). Enzymatic hydrolysis of polylactic acid fiber. Appl. Biochem. Biotechnol. 164, 89–102. doi: 10.1007/s12010-010-9117-7
Li, J., Kim, H. R., Lee, H. M., Yu, H. C., Jeon, E., Lee, S., et al. (2020). Rapid biodegradation of polyphenylene sulfide plastic beads by Pseudomonas sp. Sci. Total Environ. 720:137616. doi: 10.1016/j.scitotenv.2020.137616
Liebminger, S., Eberl, A., Sousa, F., Heumann, S., Fischer-Colbrie, G., Cavaco-Paulo, A., et al. (2009). Hydrolysis of PET and bis-(benzoyloxyethyl) terephthalate with a new polyesterase from Penicillium citrinum. Biocatal. Biotransformation 25, 171–177. doi: 10.1080/10242420701379734
Lim, J. W., Ting, D. W. Q., Loh, K.-C., Ge, T., and Tong, Y. W. (2018). Effects of disposable plastics and wooden chopsticks on the anaerobic digestion of food waste. Waste Manag. 79, 607–614. doi: 10.1016/j.wasman.2018.08.033
Lin, Y.-C., Cho, J., Tompsett, G. A., Westmoreland, P. R., and Huber, G. W. (2009). Kinetics and mechanism of cellulose pyrolysis. J. Phys. Chem. 113, 20097–20107. doi: 10.1021/jp906702p
Lin, Y., Zhang, C., Zhang, M., and Zhang, J. (2010). Deoxygenation of bio-oil during pyrolysis of biomass in the presence of CaO in a fluidized-bed reactor. Energy Fuel 24, 5686–5695. doi: 10.1021/ef1009605
Liu, G., Liao, Y., Wu, Y., and Ma, X. (2018). Synthesis gas production from microalgae gasification in the presence of Fe2O3 oxygen carrier and CaO additive. Appl. Energy 212, 955–965. doi: 10.1016/j.apenergy.2017.12.110
Liu, X., Piao, X., Wang, Y., Zhu, S., and He, H. (2008). Calcium methoxide as a solid base catalyst for the transesterification of soybean oil to biodiesel with methanol. Fuel 87, 1076–1082. doi: 10.1016/j.fuel.2007.05.059
Lu, Q., Zhang, Z.-F., Dong, C.-Q., and Zhu, X.-F. (2010). Catalytic upgrading of biomass fast pyrolysis vapors with nano metal oxides: an analytical Py-GC/MS study. Energies 3, 1805–1820. doi: 10.3390/en3111805
Lucas, N., Bienaime, C., Belloy, C., Queneudec, M., Silvestre, F., and Nava-Saucedo, J.-E. (2008). Polymer biodegradation: mechanisms and estimation techniques—a review. Chemosphere 73, 429–442. doi: 10.1016/j.chemosphere.2008.06.064
Luo, L., and Wong, J. W. C. (2019). Enhanced food waste degradation in integrated two-phase anaerobic digestion: effect of leachate recirculation ratio. Bioresour. Technol. 291:121813. doi: 10.1016/j.biortech.2019.121813
Ma, Q., Chen, Z., and Favoino, E. (2020). Biodegradable Plastics: Breaking Down the Facts. Greenpeace East Asia, China. Available at: https://www.greenpeace.org/static/planet4-eastasia-stateless/84075f56-biodegradable-plastics-report.pdf
Ma, M. M., Wang, L. Y., and Zhu, H. Y. (2011). Enzymatic degradation of polyester-nanoparticles by lipases and adsorption of lipases on the polyester-nanoparticles. Adv. Mater. Res. 418-420, 2302–2307. doi: 10.4028/www.scientific.net/AMR.418-420.2302
Macarthur, D. E., Waughray, D., and Stuchtey, M. R. (2016). The New Plastics Economy, Rethinking the Future of Plastics. Ellen Macarthur Foundation, United Kingdom.
Masaki, K., Kamini, N. R., Ikeda, H., and Iefuji, H. (2005). Cutinase-like enzyme from the yeast Cryptococcus sp. strain S-2 hydrolyzes polylactic acid and other biodegradable plastics. Appl. Environ. Microbiol. 71, 7548–7550. doi: 10.1128/AEM.71.11.7548-7550.2005
Massardier-Nageotte, V., Pestre, C., Cruard-Pradet, T., and Bayard, R. (2006). Aerobic and anaerobic biodegradability of polymer films and physico-chemical characterization. Polym. Degrad. Stab. 91, 620–627. doi: 10.1016/j.polymdegradstab.2005.02.029
Matavulj, M., and Molitoris, H. P. (2009). Marine fungi: degraders of poly-3-hydroxyalkanoate based plastic materials. Zbornik Matice Srpske za Prirodne Nauke 116, 253–265. doi: 10.2298/ZMSPN0916253M
Matjašič, T., Simčič, T., Medvešček, N., Bajt, O., Dreo, T., and Mori, N. (2021). Critical evaluation of biodegradation studies on synthetic plastics through a systematic literature review. Sci. Total Environ. 752:141959. doi: 10.1016/j.scitotenv.2020.141959
Mckendry, P. (2002). Energy production from biomass (part 2): conversion technologies. Bioresour. Technol. 83, 47–54. doi: 10.1016/S0960-8524(01)00119-5
Meeker, J. D., Sathyanarayana, S., and Swan, S. H. (2009). Phthalates and other additives in plastics: human exposure and associated health outcomes. Philos. Trans. R. Soc. Lond. Ser. B Biol. Sci. 364, 2097–2113. doi: 10.1098/rstb.2008.0268
Minowa, T., Yokoyama, S.-Y., Kishimoto, M., and Okakura, T. (1995). Oil production from algal cells of Dunaliella tertiolecta by direct thermochemical liquefaction. Fuel 74, 1735–1738. doi: 10.1016/0016-2361(95)80001-X
Mohanan, N., Montazer, Z., Sharma, P. K., and Levin, D. B. (2020). Microbial and enzymatic degradation of synthetic plastics. Front. Microbiol. 11:580709. doi: 10.3389/fmicb.2020.580709
Moharir, R. V., and Kumar, S. (2019). Challenges associated with plastic waste disposal and allied microbial routes for its effective degradation: a comprehensive review. J. Clean. Prod. 208, 65–76. doi: 10.1016/j.jclepro.2018.10.059
Mohee, R., Unmar, G. D., Mudhoo, A., and Khadoo-Jeetah, P. (2008). Biodegradability of biodegradable/degradable plastic materials under aerobic and anaerobic conditions. Waste Manag. 28, 1624–1629. doi: 10.1016/j.wasman.2007.07.003
Montazer, Z., Najafi, M. B. H., and Levin, D. B. (2020). Challenges with verifying microbial degradation of polyethylene. Polymers 12:123. doi: 10.3390/polym12010123
Moog, D., Schmitt, J., Senger, J., Zarzycki, J., Rexer, K.-H., Linne, U., et al. (2019). Using a marine microalga as a chassis for polyethylene terephthalate (PET) degradation. Microb. Cell Factories 18:171. doi: 10.1186/s12934-019-1220-z
Mostafa, Y. S., Alrumman, S. A., Otaif, K. A., Alamri, S. A., Mostafa, M. S., and Sahlabji, T. (2020). Production and characterization of bioplastic by polyhydroxybutyrate accumulating Erythrobacter aquimaris isolated from mangrove rhizosphere. Molecules 25:179. doi: 10.3390/molecules25010179
Mullen, C. A., Boateng, A. A., Mihalcik, D. J., and Goldberg, N. M. (2011). Catalytic fast pyrolysis of white oak wood in a bubbling fluidized bed. Energy Fuel 25, 5444–5451. doi: 10.1021/ef201286z
Murugan, P., Chhajer, P., Kosugi, A., Arai, T., Brigham, C. J., and Sudesh, K. (2016). Production of P (3HB-co-3HHx) with controlled compositions by recombinant Cupriavidus necator Re2058/pCB113 from renewable resources. CLEAN Soil Air Water 44, 1234–1241. doi: 10.1002/clen.201500714
Nagata, M., Kiyotsukuri, T., Minami, S., Tsutsumi, N., and Sakai, W. (1997). Enzymatic degradation of poly(ethylene terephthalate) copolymers with aliphatic dicarboxylic acids and/or poly(ethylene glycol). Eur. Polym. J. 33, 1701–1705. doi: 10.1016/S0014-3057(97)00063-3
Nakajima-Kambe, T., Edwinoliver, N. G., Maeda, H., Thirunavukarasu, K., Gowthaman, M. K., Masaki, K., et al. (2012). Purification, cloning and expression of an Aspergillus niger lipase for degradation of poly(lactic acid) and poly(ε-caprolactone). Polym. Degrad. Stab. 97, 139–144. doi: 10.1016/j.polymdegradstab.2011.11.009
Nanda, S., and Berruti, F. (2021). A technical review of bioenergy and resource recovery from municipal solid waste. J. Hazard. Mater. 403:123970. doi: 10.1016/j.jhazmat.2020.123970
Narayanan, M., Ranganathan, M., Kandasamy, G., and Kumarasamy, S. (2021). Evaluation of interaction among indigenous rhizobacteria and Vigna unguiculata on remediation of metal-containing abandoned magnesite mine tailing. Arch. Microbiol. 203, 1399–1410. doi: 10.1007/s00203-020-02115-3
Natarajan, D. (2011). Assessment of physicochemical and heavy metals from waste dumps of magnesite and bauxite mines. Electron. J. Environ. Agric. Food Chem. 10, 3076–3082.
Nikolaivits, E., Pantelic, B., Azeem, M., Taxeidis, G., Babu, R., Topakas, E., et al. (2021). Progressing plastics circularity: a review of mechano-biocatalytic approaches for waste plastic (re)valorization. Front. Bioeng. Biotechnol. 9:696040. doi: 10.3389/fbioe.2021.696040
Nimchua, T., Punnapayak, H., and Zimmermann, W. (2007). Comparison of the hydrolysis of polyethylene terephthalate fibers by a hydrolase from Fusarium oxysporum LCH I and Fusarium solani f. sp. pisi. Biotechnol. J. 2, 361–364. doi: 10.1002/biot.200600095
Oeser, T., Wei, R., Baumgarten, T., Billig, S., Föllner, C., and Zimmermann, W. (2010). High level expression of a hydrophobic poly(ethylene terephthalate)-hydrolyzing carboxylesterase from Thermobifida fusca KW3 in Escherichia coli BL21(DE3). J. Biotechnol. 146, 100–104. doi: 10.1016/j.jbiotec.2010.02.006
Oliveira, J., Belchior, A., Da Silva, V. D., Rotter, A., Petrovski, Ž., Almeida, P. L., et al. (2020). Marine environmental plastic pollution: mitigation by microorganism degradation and recycling valorization. Front. Mar. Sci. 7:1007. doi: 10.3389/fmars.2020.567126
Palaniappan, K. (2017). An overview of applications of nanotechnology in biofuel production. World Appl. Sci. J. 35, 1305–1311. doi: 10.5829/idosi.wasj.2017.1305.1311
Pathak, V. M., and Navneet, (2017). Review on the current status of polymer degradation: a microbial approach. Bioresour. Bioprocess. 4:15. doi: 10.1186/s40643-017-0145-9
Pattarkine, M. V., and Pattarkine, V. M. (2012). “Nanotechnology for algal biofuels,” in The Science of Algal Fuels. eds. R. Gordon and J. Seckbach (Dordrecht, The Netherlands: Springer), 147–163.
Pellis, A., Acero, E. H., Weber, H., Obersriebnig, M., Breinbauer, R., Srebotnik, E., et al. (2015). Biocatalyzed approach for the surface functionalization of poly(L-lactic acid) films using hydrolytic enzymes. Biotechnol. J. 10, 1739–1749. doi: 10.1002/biot.201500074
Petit, M. G., Correa, Z., and Sabino, M. A. (2015). Degradation of a polycaprolactone/eggshell biocomposite in a bioreactor. J. Polym. Environ. 23, 11–20. doi: 10.1007/s10924-014-0655-x
Pino, M. S., Rodríguez-Jasso, R. M., Michelin, M., Flores-Gallegos, A. C., Morales-Rodriguez, R., Teixeira, J. A., et al. (2018). Bioreactor design for enzymatic hydrolysis of biomass under the biorefinery concept. Chem. Eng. J. 347, 119–136. doi: 10.1016/j.cej.2018.04.057
PlasticsEurope (2016). An Analysis of European Plastics Production, Demand and Waste Data. Brussels, Belgium: Wemmel-Belgium.
PlasticsEurope (2019). "Plastics-the Facts 2019 an Analysis of European Plastics Production, Demand and Waste Data" (Wemmel-Belgium: Brussels, Belgium).
Quarantin, A., Hadeler, B., Kröger, C., Schäfer, W., Favaron, F., Sella, L., et al. (2019). Different hydrophobins of Fusarium graminearum are involved in hyphal growth, attachment, water-air interface penetration and plant infection. Front. Microbiol. 10:751. doi: 10.3389/fmicb.2019.00751
Quartinello, F., Vajnhandl, S., Valh, J. V., Farmer, T. J., Vončina, B., Lobnik, A., et al. (2017). Synergistic chemo-enzymatic hydrolysis of poly(ethylene terephthalate) from textile waste. Microb. Biotechnol. 10, 1376–1383. doi: 10.1111/1751-7915.12734
Raheem, A. B., Noor, Z. Z., Hassan, A., Hamid, M. K. A., Samsudin, S. A., and Sabeen, A. H. (2019). Current developments in chemical recycling of post-consumer polyethylene terephthalate wastes for new materials production: a review. J. Clean. Prod. 225, 1052–1064. doi: 10.1016/j.jclepro.2019.04.019
Rahman, A., Anthony, R. J., Sathish, A., Sims, R. C., and Miller, C. D. (2014). Effects of wastewater microalgae harvesting methods on polyhydroxybutyrate production. Bioresour. Technol. 156, 364–367. doi: 10.1016/j.biortech.2014.01.034
Ratzke, C., and Gore, J. (2018). Modifying and reacting to the environmental pH can drive bacterial interactions. PLoS Biol. 16:e2004248. doi: 10.1371/journal.pbio.2004248
Rendón-Villalobos, R., Ortíz-Sánchez, A., Tovar-Sánchez, E., and Flores-Huicochea, E. (2016). “The role of biopolymers in obtaining environmentally friendly materials,” in Composites From Renewable and Sustainable Materials. Vol. 151. ed. M. Poletto (London, United Kingdom: IntechOpen).
Ribitsch, D., Acero, E. H., Greimel, K., Dellacher, A., Zitzenbacher, S., Marold, A., et al. (2012a). A new esterase from Thermobifida halotolerans hydrolyses polyethylene terephthalate (PET) and polylactic acid (PLA). Polymers 4, 617–629. doi: 10.3390/polym4010617
Ribitsch, D., Acero, E. H., Greimel, K., Eiteljoerg, I., Trotscha, E., Freddi, G., et al. (2012b). Characterization of a new cutinase from Thermobifida alba for PET-surface hydrolysis. Biocatal. Biotransformation 30, 2–9. doi: 10.3109/10242422.2012.644435
Ribitsch, D., Heumann, S., Trotscha, E., Acero, E. H., Greimel, K., Leber, R., et al. (2011). Hydrolysis of polyethyleneterephthalate by p-nitrobenzylesterase from Bacillus subtilis. Biotechnol. Prog. 27, 951–960. doi: 10.1002/btpr.610
Ribitsch, D., Hromic, A., Zitzenbacher, S., Zartl, B., Gamerith, C., Pellis, A., et al. (2017). Small cause, large effect: structural characterization of cutinases from Thermobifida cellulosilytica. Biotechnol. Bioeng. 114, 2481–2488. doi: 10.1002/bit.26372
Rivard, C., Moens, L., Roberts, K., Brigham, J., and Kelley, S. (1995). Starch esters as biodegradable plastics: effects of ester group chain length and degree of substitution on anaerobic biodegradation. Enzym. Microb. Technol. 17, 848–852. doi: 10.1016/0141-0229(94)00120-G
Roja, K., Sudhakar, D. R., Anto, S., and Mathimani, T. (2019). Extraction and characterization of polyhydroxyalkanoates from marine green alga and cyanobacteria. Biocatal. Agric. Biotechnol. 22:101358. doi: 10.1016/j.bcab.2019.101358
Roser, H. R. (2018). "Plastic Pollution". Published Online at OurWorldInData.org. Available at: https://ourworldindata.org/plastic-pollution
Rossman, A. Y. (1995). “Microfungi: molds, mildews, rusts, and smuts,” in Our Living Resources: A Report to the Nation on the Distribution, Abundance, and Health of US Plants, Animals, and Ecosystems. eds. E. T. LaRoe, G. S. Farris, C. E. Puckett, P. D. Doran, and M. J. Mac, 190–192.
Rujnić-Sokele, M., and Pilipović, A. (2017). Challenges and opportunities of biodegradable plastics: a mini review. Waste Manag. Res. 35, 132–140. doi: 10.1177/0734242X16683272
Safarik, I., Prochazkova, G., Pospiskova, K., and Branyik, T. (2016). Magnetically modified microalgae and their applications. Crit. Rev. Biotechnol. 36, 931–941. doi: 10.3109/07388551.2015.1064085
Sakai, K., Taniguchi, M., Miura, S., Ohara, H., Matsumoto, T., and Shirai, Y. (2003). Making plastics from garbage. J. Ind. Ecol. 7, 63–74. doi: 10.1162/108819803323059406
Samak, N. A., Jia, Y., Sharshar, M. M., Mu, T., Yang, M., Peh, S., et al. (2020). Recent advances in biocatalysts engineering for polyethylene terephthalate plastic waste green recycling. Environ. Int. 145:106144. doi: 10.1016/j.envint.2020.106144
Sánchez, C. (2020). Fungal potential for the degradation of petroleum-based polymers: an overview of macro- and microplastics biodegradation. Biotechnol. Adv. 40:107501. doi: 10.1016/j.biotechadv.2019.107501
Sankhla, I. S., Sharma, G., and Tak, A. (2020). “Fungal degradation of bioplastics: an overview,” in New and Future Developments in Microbial Biotechnology and Bioengineering. eds. J. Singh and P. Gehlot (Amsterdam, Netherlands: Elsevier), 35–47.
Selke, S., Auras, R., Nguyen, T. A., Aguirre, E. C., Cheruvathur, R., and Liu, Y. (2015). Evaluation of biodegradation-promoting additives for plastics. Environ. Sci. Technol. 49, 3769–3777. doi: 10.1021/es504258u
Sen, B., Alp, M., Sönmez, F., Koçer, M., and Canpolat, O. (2013). “Relationship of algae to water pollution and waste water treatment,” in Water Treatment. eds. W. Elshorbagy and R. K. Chowdhury (London, UK: InTech), 335–354.
Shanmugam, V., Das, O., Neisiany, R. E., Babu Nb, K., Singh, S., Hedenqvist, M., et al. (2020). Polymer recycling in additive manufacturing: an opportunity for the circular economy. Mater. Circul. Econ. 2:11. doi: 10.1007/s42824-020-00012-0
Sharma, M., Dubey, A., and Pareek, A. (2014). Algal flora on degrading polythene waste. CIBTech J. Microbiol. 3, 43–47.
Shay, E. G. (1993). Diesel fuel from vegetable oils: status and opportunities. Biomass Bioenergy 4, 227–242. doi: 10.1016/0961-9534(93)90080-N
Sheldon, R. A., and Norton, M. (2020). Green chemistry and the plastic pollution challenge: towards a circular economy. Green Chem. 22, 6310–6322. doi: 10.1039/D0GC02630A
Shen, L., Haufe, J., and Patel, M. K. (2009). Product overview and market projection of emerging bio-based plastics PRO-BIP 2009. Report for European Polysaccharide Network of Excellence (EPNOE) and European Bioplastics.
Shen, M., Song, B., Zeng, G., Zhang, Y., Huang, W., Wen, X., et al. (2020). Are biodegradable plastics a promising solution to solve the global plastic pollution? Environ. Pollut. 263:114469. doi: 10.1016/j.envpol.2020.114469
Shi, X.-L., Wu, Y.-H., Jin, X.-B., Wang, C.-S., and Xu, X.-W. (2017). Alteromonas lipolytica sp. nov., a poly-beta-hydroxybutyrate-producing bacterium isolated from surface seawater. Int. J. Syst. Evol. Microbiol. 67, 237–242. doi: 10.1099/ijsem.0.001604
Shinozaki, Y., Morita, T., Cao, X. H., Yoshida, S., Koitabashi, M., Watanabe, T., et al. (2013). Biodegradable plastic-degrading enzyme from Pseudozyma antarctica: cloning, sequencing, and characterization. Appl. Microbiol. Biotechnol. 97, 2951–2959. doi: 10.1007/s00253-012-4188-8
Sikdar, S., Siddaiah, A., and Menezes, P. L. (2020). Conversion of waste plastic to oils for tribological applications. Lubricants 8:78. doi: 10.3390/lubricants8080078
Singh, H. (2006). Mycoremediation: Fungal Bioremediation. United States of America: John Wiley & Sons.
Singh, B., and Sharma, N. (2008). Mechanistic implications of plastic degradation. Polym. Degrad. Stab. 93, 561–584. doi: 10.1016/j.polymdegradstab.2007.11.008
Siracusa, V. (2019). Microbial degradation of synthetic biopolymers waste. Polymers 11:1066. doi: 10.3390/polym11061066
Soman, S., Kumarasamy, S., Narayanan, M., and Ranganathan, M. (2020a). Biocatalyst: phytase production in solid state fermentation by OVAT strategy. Bioin. Res. Appl. Chem. 10, 6119–6127. doi: 10.33263/BRIAC105.61196127
Soman, S., Suresh, K., Mathiyazhagan, N., and Muthusamy, R. (2020b). Chemically defined medium for the production of phytase by Hanseniaspora guilliermondii S1, Pichia fermentans S2 and its secondary structure prediction of 16S rRNA. Bioin. Res. App. Chem 10, 6262–6272. doi: 10.33263/BRIAC105.62626272
Song, J. H., Murphy, R. J., Narayan, R., and Davies, G. B. H. (2009). Biodegradable and compostable alternatives to conventional plastics. Philos. Trans. R. Soc. Lond. Ser. B Biol. Sci. 364, 2127–2139. doi: 10.1098/rstb.2008.0289
Sörenson, E., Capo, E., Farnelid, H., Lindehoff, E., and Legrand, C. (2021). Temperature stress induces shift from co-existence to competition for organic carbon in microalgae-bacterial photobioreactor community—enabling continuous production of microalgal biomass. Front. Microbiol. 12:607601. doi: 10.3389/fmicb.2021.607601
Sowmya, H. V., Bellibatlu, R., Nayanashree, G., Basaiah, T., and Krishnappa, M. (2015). Polyethylene degradation by fungal consortium. Int. J. Environ. Res. 9, 823–830. doi: 10.22059/IJER.2015.969
Srisowmeya, G., Chakravarthy, M., and Nandhini Devi, G. (2020). Critical considerations in two-stage anaerobic digestion of food waste—a review. Renew. Sust. Energ. Rev. 119:109587. doi: 10.1016/j.rser.2019.109587
Su, H., Kanchanatip, E., Wang, D., Zheng, R., Huang, Z., Chen, Y., et al. (2020a). Production of H2-rich syngas from gasification of unsorted food waste in supercritical water. Waste Manag. 102, 520–527. doi: 10.1016/j.wasman.2019.11.018
Su, H., Liao, W., Wang, J., Hantoko, D., Zhou, Z., Feng, H., et al. (2020b). Assessment of supercritical water gasification of food waste under the background of waste sorting: influences of plastic waste contents. Int. J. Hydrog. Energy 45, 21138–21147. doi: 10.1016/j.ijhydene.2020.05.256
Tai, H.-S. (2015). Co-pyrolysis study of polylactic acid and polyethylene terephthalate plastic wastes. J. Solid Waste Technol. Manag. 41, 189–202. doi: 10.5276/JSWTM.2015.189
Taniguchi, I., Yoshida, S., Hiraga, K., Miyamoto, K., Kimura, Y., and Oda, K. (2019). Biodegradation of PET: current status and application aspects. ACS Catal. 9, 4089–4105. doi: 10.1021/acscatal.8b05171
Tchigvintsev, A., Tran, H., Popovic, A., Kovacic, F., Brown, G., Flick, R., et al. (2015). The environment shapes microbial enzymes: five cold-active and salt-resistant carboxylesterases from marine metagenomes. Appl. Microbiol. Biotechnol. 99, 2165–2178. doi: 10.1007/s00253-014-6038-3
Tokiwa, Y., Calabia, B. P., Ugwu, C. U., and Aiba, S. (2009). Biodegradability of plastics. Int. J. Mol. Sci. 10, 3722–3742. doi: 10.3390/ijms10093722
Torres, A., Li, S. M., Roussos, S., and Vert, M. (1996). Screening of microorganisms for biodegradation of poly (lactic-acid) and lactic acid-containing polymers. Appl. Environ. Microbiol. 62, 2393–2397. doi: 10.1128/aem.62.7.2393-2397.1996
Treu, L., Tsapekos, P., Peprah, M., Campanaro, S., Giacomini, A., Corich, V., et al. (2019). Microbial profiling during anaerobic digestion of cheese whey in reactors operated at different conditions. Bioresour. Technol. 275, 375–385. doi: 10.1016/j.biortech.2018.12.084
Tufail, S., Munir, S., and Jamil, N. (2017). Variation analysis of bacterial polyhydroxyalkanoates production using saturated and unsaturated hydrocarbons. Braz. J. Microbiol. 48, 629–636. doi: 10.1016/j.bjm.2017.02.008
Urbanek, A. K., Rymowicz, W., Strzelecki, M. C., Kociuba, W., Franczak, Ł., and Mirończuk, A. M. (2017). Isolation and characterization of Arctic microorganisms decomposing bioplastics. AMB Express 7, 1–10. doi: 10.1186/s13568-017-0448-4
Valderrama, M. A. M., Van Putten, R.-J., and Gruter, G.-J. M. (2019). The potential of oxalic—and glycolic acid based polyesters (review). Towards CO2 as a feedstock (carbon capture and utilization—CCU). Eur. Polym. J. 119, 445–468. doi: 10.1016/j.eurpolymj.2019.07.036
Vecchiato, S., Ahrens, J., Pellis, A., Scaini, D., Mueller, B., Acero, E. H., et al. (2017). Enzymatic functionalization of HMLS-polyethylene terephthalate fabrics improves the adhesion to rubber. ACS Sustain. Chem. Eng. 5, 6456–6465. doi: 10.1021/acssuschemeng.7b00475
Verlinden, R. A., Hill, D. J., Kenward, M., Williams, C. D., and Radecka, I. (2007). Bacterial synthesis of biodegradable polyhydroxyalkanoates. J. Appl. Microbiol. 102, 1437–1449. doi: 10.1111/j.1365-2672.2007.03335.x
Verma, R., Vinoda, K., Papireddy, M., and Gowda, A. (2016). Toxic pollutants from plastic waste-a review. Procedia Environ. Sci. 35, 701–708. doi: 10.1016/j.proenv.2016.07.069
Vohlídal, J. (2021). Polymer degradation: a short review. Chem. Teach. Int. 3, 213–220. doi: 10.1515/cti-2020-0015
Wang, K.-F., Guo, C., Ju, F., Samak, N. A., Zhuang, G.-Q., and Liu, C.-Z. (2018). Farnesol-induced hyperbranched morphology with short hyphae and bulbous tips of Coriolus versicolor. Sci. Rep. 8, 1–12. doi: 10.1038/s41598-018-33435-6
Wang, L., Ni, H., Zhang, J., Shi, Q., Zhang, R., Yu, H., et al. (2020). Enzymatic treatment improves fast pyrolysis product selectivity of softwood and hardwood lignin. Sci. Total Environ. 717:137241. doi: 10.1016/j.scitotenv.2020.137241
Wang, D., Xiao, R., Zhang, H., and He, G. (2010). Comparison of catalytic pyrolysis of biomass with MCM-41 and CaO catalysts by using TGA–FTIR analysis. J. Anal. Appl. Pyrolysis 89, 171–177. doi: 10.1016/j.jaap.2010.07.008
Wei, R., Oeser, T., Then, J., Kühn, N., Barth, M., Schmidt, J., et al. (2014). Functional characterization and structural modeling of synthetic polyester-degrading hydrolases from Thermomonospora curvata. AMB Express 4, 1–10. doi: 10.1186/s13568-014-0044-9
Wilcox, C., Sebille, E., and Hardesty, B. (2015). Threat of plastic pollution to seabirds is global, pervasive, and increasing. Proc. Natl. Acad. Sci. U. S. A. 112, 11899–11904. doi: 10.1073/pnas.1502108112
Williams, P. T., and Nugranad, N. (2000). Comparison of products from the pyrolysis and catalytic pyrolysis of rice husks. Energy 25, 493–513. doi: 10.1016/S0360-5442(00)00009-8
Xi, X., Ni, K., Hao, H., Shang, Y., Zhao, B., and Qian, Z. (2021). Secretory expression in Bacillus subtilis and biochemical characterization of a highly thermostable polyethylene terephthalate hydrolase from bacterium HR29. Enzym. Microb. Technol. 143:109715. doi: 10.1016/j.enzmictec.2020.109715
Xie, Y., Niu, X., Yang, J., Fan, R., Shi, J., Ullah, N., et al. (2020). Active biodegradable films based on the whole potato peel incorporated with bacterial cellulose and curcumin. Int. J. Biol. Macromol. 150, 480–491. doi: 10.1016/j.ijbiomac.2020.01.291
Yagi, H., Ninomiya, F., Funabashi, M., and Kunioka, M. (2009). Anaerobic biodegradation tests of poly(lactic acid) and polycaprolactone using new evaluation system for methane fermentation in anaerobic sludge. Polym. Degrad. Stab. 94, 1397–1404. doi: 10.1016/j.polymdegradstab.2009.05.012
Yagi, H., Ninomiya, F., Funabashi, M., and Kunioka, M. (2012). Anaerobic biodegradation of poly (lactic acid) film in anaerobic sludge. J. Polym. Environ. 20, 673–680. doi: 10.1007/s10924-012-0472-z
Yagi, H., Ninomiya, F., Funabashi, M., and Kunioka, M. (2014). Mesophilic anaerobic biodegradation test and analysis of eubacteria and archaea involved in anaerobic biodegradation of four specified biodegradable polyesters. Polym. Degrad. Stab. 110, 278–283. doi: 10.1016/j.polymdegradstab.2014.08.031
Yan, N., Fan, C., Chen, Y., and Hu, Z. (2016). The potential for microalgae as bioreactors to produce pharmaceuticals. Int. J. Mol. Sci. 17:962. doi: 10.3390/ijms17060962
Yan, M., Fotidis, I. A., Tian, H., Khoshnevisan, B., Treu, L., Tsapekos, P., et al. (2019). Acclimatization contributes to stable anaerobic digestion of organic fraction of municipal solid waste under extreme ammonia levels: focusing on microbial community dynamics. Bioresour. Technol. 286:121376. doi: 10.1016/j.biortech.2019.121376
Yoshida, S., Hiraga, K., Takehana, T., Taniguchi, I., Yamaji, H., Maeda, Y., et al. (2016). A bacterium that degrades and assimilates poly (ethylene terephthalate). Science 351, 1196–1199. doi: 10.1126/science.aad6359
Yu, J., and Chen, L. X. L. (2008). The greenhouse gas emissions and fossil energy requirement of bioplastics from cradle to gate of a biomass refinery. Environ. Sci. Technol. 42, 6961–6966. doi: 10.1021/es7032235
Yu, P. H., Chua, H., Huang, A.-L., and Ho, K.-P. (1999). Conversion of industrial food wastes by Alcaligenes latus into polyhydroxyalkanoates. Appl. Biochem. Biotechnol. 78, 445–454. doi: 10.1385/ABAB:78:1-3:445
Zhang, X., Chen, J., and Li, J. (2020). The removal of microplastics in the wastewater treatment process and their potential impact on anaerobic digestion due to pollutants association. Chemosphere 251:126360. doi: 10.1016/j.chemosphere.2020.126360
Zhang, W., Heaven, S., and Banks, C. J. (2018). Degradation of some EN13432 compliant plastics in simulated mesophilic anaerobic digestion of food waste. Polym. Degrad. Stab. 147, 76–88. doi: 10.1016/j.polymdegradstab.2017.11.005
Zhang, L., Loh, K.-C., Kuroki, A., Dai, Y., and Tong, Y. W. (2021). Microbial biodiesel production from industrial organic wastes by oleaginous microorganisms: current status and prospects. J. Hazard. Mater. 402:123543. doi: 10.1016/j.jhazmat.2020.123543
Zhang, W., Torrella, F., Banks, C. J., and Heaven, S. (2019). Data related to anaerobic digestion of bioplastics: images and properties of digested bioplastics and digestate, synthetic food waste recipe and packaging information. Data Brief 25:103990. doi: 10.1016/j.dib.2019.103990
Zhang, H., Xiao, R., Huang, H., and Xiao, G. (2009). Comparison of non-catalytic and catalytic fast pyrolysis of corncob in a fluidized bed reactor. Bioresour. Technol. 100, 1428–1434. doi: 10.1016/j.biortech.2008.08.031
Keywords: PLA-PET waste, bioconversion, polyhydroxyalkanoates, circular economy, fuel oil
Citation: Tamoor M, Samak NA, Jia Y, Mushtaq MU, Sher H, Bibi M and Xing J (2021) Potential Use of Microbial Enzymes for the Conversion of Plastic Waste Into Value-Added Products: A Viable Solution. Front. Microbiol. 12:777727. doi: 10.3389/fmicb.2021.777727
Edited by:
El-Sayed Salama, Lanzhou University, ChinaReviewed by:
Huadong Peng, Imperial College London, United KingdomMin Jiang, Nanjing Tech University, China
Copyright © 2021 Tamoor, Samak, Jia, Mushtaq, Sher, Bibi and Xing. This is an open-access article distributed under the terms of the Creative Commons Attribution License (CC BY). The use, distribution or reproduction in other forums is permitted, provided the original author(s) and the copyright owner(s) are credited and that the original publication in this journal is cited, in accordance with accepted academic practice. No use, distribution or reproduction is permitted which does not comply with these terms.
*Correspondence: Nadia A. Samak, nadia@ipe.ac.cn; Jianmin Xing, jmxing@ipe.ac.cn