- 1Department of Ocean Science, The Hong Kong University of Science and Technology, Kowloon, Hong Kong SAR, China
- 2Southern Marine Science and Engineering Guangdong Laboratory (Guangzhou), Guangzhou, China
- 3State Key Laboratory of Marine Pollution, Hong Kong SAR, China
Mixotrophic protists are widely observed in the aquatic ecosystems, while how they respond to inorganic nutrient imbalance and ocean warming remains understudied. We conducted a series of experiments on a mixotrophic dinoflagellate Lepidodinium sp. isolated from subtropical coastal waters to investigate the combined effect of temperature and medium nitrate to phosphate ratio (N:P ratio) on the ingestion activities of mixotrophic protists. We found Lepidodinium sp. displayed selective feeding behaviour with a higher ingestion rate on high-N prey (N-rich Rhodomonas salina) when the ambient inorganic N:P ratio was equal to or below the Redfield ratio. The Chesson selectivity index α increased with increasing temperature, suggesting that warming exacerbated the selective feeding of Lepidodinium sp. Under inorganic nitrogen sufficient conditions (N:P ratio = 64), no selective feeding was observed at 25 and 28°C, while it occurs at 31°C, which also indicates that warming alters the feeding behaviour of Lepidodinium sp. In addition, our results revealed that the total ingestion rate of Lepidodinium sp. under the condition with normal inorganic nutrients (Redfield ratio) was significantly lower than that under nutrient-imbalanced conditions, which indicates that Lepidodinium sp. developed compensatory feeding to balance their cellular stoichiometry and satisfy their growth. Our study is the first attempt on revealing the selective feeding behaviours of mixotrophic protists on prey under different inorganic nutrient environments and rising temperatures, which will contribute to our understanding of the response of marine plankton food web to projected climate changes.
Introduction
Mixotrophy is a nutrition strategy combining phototrophy and phagotrophy within one organism, ubiquitous among protists in aquatic ecosystems (Flynn and Mitra, 2009; Mitra et al., 2016). Phagotrophic algae, one common type of mixotrophs, are capable of acquiring carbon from photosynthesis as well as ingestion of prey (Stoecker, 1998; Mitra et al., 2016). Many studies have found that phagotrophic algae can benefit from prey ingestion, which allows them to thrive in the waters where the resource availability (e.g., inorganic nutrients and light) is limited to strict autotrophs (Wilken et al., 2014; Moeller et al., 2019). Although there is increasing recognition and understanding of the ecological significance of mixotrophic protists, it remains substantially understudied how phagotrophic algae take advantage of mixotrophic metabolisms in rapidly changing environments.
Phagotrophic algae are able to adjust their grazing activities according to the ambient inorganic nutrient concentrations. For instance, Gyrodinium galatheanum increases ingestion rate with decreasing inorganic phosphorus concentrations (Li et al., 2000; Smalley and Coats, 2002). Mixotrophic dinoflagellate Heterocapsa triquetra only ingests prey under nutrient-depleted conditions (Legrand et al., 1998). However, what the mixotrophic algae face in the changing ocean is not only the alteration in absolute inorganic nutrient concentrations but also the increasing imbalance in nutrient ratios due to anthropogenic activities (Li et al., 2000; Wickham and Wimmer, 2019).
The aquatic ecosystems have long been enriched with more N relative to P because of the sharp increase in the global use of N as well as the aggressive removal of P loads, which changes the nutrient stoichiometry and leads to the nutrient imbalance in aquatic ecosystems, especially in coastal waters (Huang et al., 2003; Glibert et al., 2013, 2014). Based on the framework of ecological stoichiometry, photoautotrophs have high plasticity in elemental composition and are apt to alter their elemental contents when the environment changes, whereas heterotrophic protists are prone to maintain the stoichiometry homeostatically (Sterner and Elser, 2002; Moreno and Martiny, 2018). Similar to heterotrophic protists, the phagocytosis of mixotrophs may enable them to maintain their cellular stoichiometry (Moorthi et al., 2017). Some studies have found that phagotrophic mixotrophs can regulate their phagotrophy in nutrient-imbalanced environments (Raven, 1997; Stoecker, 1998; Li et al., 2000; Smalley and Coats, 2002). For instance, the ingestion of Gyrodinium galatheanum increased as the ambient N:P ratios deviated from the Redfield ratio (Li et al., 2000).
The changing environmental N:P ratios also result in the variation of prey quality, affecting the ingestion activities of herbivorous consumers. To keep their elemental contents constant, consumers may adjust their feeding behaviour either through ingesting more favourable food to extract the limiting element more efficiently (i.e., selective feeding) or through an increase of food uptake and a reduction of handling time to extract the limiting nutrient only from the readily available parts (i.e., compensatory feeding; Knisely and Geller, 1986; Cruz-Rivera and Hay, 2000; Raubenheimer and Jones, 2006; Montagnes et al., 2008; Meunier et al., 2012). Such pre-gut selection mechanisms are usually assessed by the differences in food uptake. In addition, the consumers can use post-ingestion mechanisms (e.g., excretion of excess elements) to balance the nutrient-imbalanced food (Frost et al., 2005). The selective feeding behaviours have been widely observed in heterotrophic protists, such as Oxyrrhis marina, which can select P-rich prey from the mixture of prey with different N:P ratios (Montagnes et al., 2008; Meunier et al., 2012). Regarding mixotrophs, recent studies had found that mixotrophic flagellates in oligotrophic lakes showed prey preference when more than one kind of prey was available (Ballen-Segura et al., 2017; Gerea et al., 2019). Such prey preference was assumed to arise from different C:N:P ratios of prey (Ballen-Segura et al., 2017). However, more direct evidence is still needed for understanding how mixotrophs respond to prey with various nutritional qualities. Therefore, in the current study, we aim to investigate the selective feeding behaviours of mixotrophic protists and the potential influences of the ambient N:P ratio on their ingestion activities.
Lepidodinium sp. is a mixotrophic dinoflagellate isolated from subtropical coastal waters. It has the innate ability to photosynthesize but can ingest prey even under nutrient replete conditions. Their ingestion behaviour can be regulated according to the changes in inorganic nutrient concentrations (Liu et al., 2021). Our previous study also found that Lepidodinium sp. enhanced their ingestion rate and shifted towards more heterotrophy under warming conditions (Liu et al., 2021). If warming drives mixotrophs to behave more like heterotrophic protists, the effect of both inorganic nutrient concentrations and prey quality on the ingestion activities of mixotrophs could change accordingly. Thus, in this study, we used Lepidodinium sp. to investigate the selective feeding behaviour of phagotrophic algae under both nutrient-imbalanced and warming conditions. We conducted factorial experiments with three factors (i.e., inorganic N:P ratios, prey quality and temperature) to investigate (1) whether Lepidodinium sp. can undergo both compensatory feeding and selective feeding when provided prey of different nutritional quality; (2) how the inorganic N:P ratio affects the ingestion rate and prey selectivity of Lepidodinium sp.; and (3) whether rising temperature increases the ingestion rate of Lepidodinium sp. and enhances their prey selectivity.
Materials and Methods
Algae Cultures
Lepidodinium sp. used in this study was isolated from the Port shelter region of the Hong Kong Eastern area. Species identification was conducted by microscopic observation and 18 s rDNA sequencing. Based on BLAST search, our species showed 99% similarity to Lepidodinium sp. (MH 360; Ng et al., 2017). Lepidodinium sp. was grown under a 12:12 light cycle at 100 μmol photons m−2 s−1 in F/20 autoclaved filtered seawater medium. We added the Rhodomonas salina, which was grown in F/2 medium as prey, and checked the cultures every day to ensure sufficient prey for the Lepidodinium sp.
Culture Pre-condition
The Lepidodinium sp. was grown and acclimated under different inorganic nutrient conditions, including Nitrogen-rich (Nhigh, N:P ratio = 64), normal Nitrogen (NRedfield, N:P ratio = 16) and Nitrogen-limited (Nlow, N:P ratio = 4). The detailed nutrient concentrations are presented in Table 1. The Rhodomonas salina grown in F/2 medium was added to all cultures as prey for the Lepidodinium sp. All the above cultures with the three different inorganic nutrient conditions were acclimated at 25, 28 and 31°C for at least 2 weeks. We used semi-continuous cultures (i.e., transfer every 4 days) during the acclimation to keep the Lepidodinium sp. growing in an exponential growth phase.
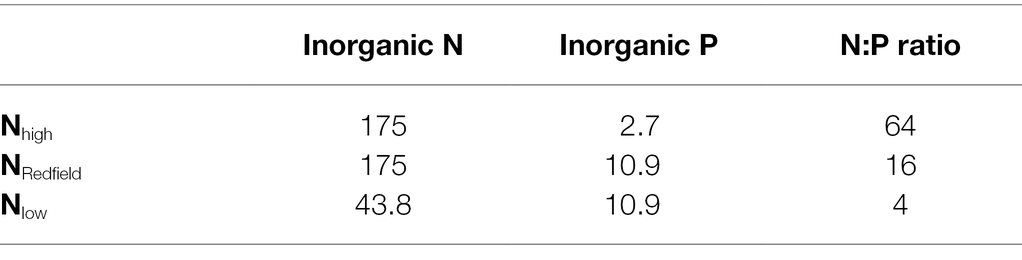
Table 1. The nitrate (N) and phosphorus (P) concentrations (μmol L−1) of the culture medium used for Lepidodinium sp. culture mediums.
Selective Feeding Experiments
We have conducted two rounds of selective feeding experiments. One round was carried out under three inorganic nutrient conditions at 25°C to investigate the ingestion behaviour of the mixotrophic Lepidodinium sp. The other round was conducted under three inorganic nutrient conditions at three temperatures (25, 28 and 31°C) to investigate the combined effect of temperature and inorganic nutrients on the ingestion behaviour of Lepidodinium sp.
The prey with different nutrition values was prepared by cultivating the Rhodomonas salina in the nitrogen-repleted and nitrogen-depleted mediums. The F/2 medium and F/2 medium without adding NO3− were used for the high-N prey and low-N prey, respectively. All cultures were maintained under the same light condition (100 μmol photons m−2 s−1) at 22°C. The Rhodomonas salina cells during the stationary phase were used in the selective feeding experiments to ensure a significant difference between high-N and low-N preys. It has been found that the colour of Rhodomonas salina cultures grown in nitrogen-limited mediums turned to be green or yellow, which is different from the cultures in nitrogen-replete mediums (red). This difference is caused by a lack of phycoerythrin in nitrogen-limited Rhodomonas salina cells (John and Davidson, 2001). It can be distinguished by flow cytometry with different fluorescence signals (Supplementary Figure S1). The cellular contents and C:N:P ratios were also significantly different when grown in these two mediums, while the cell size was similar (Table 2; Supplementary Figure S1), which renders the Rhodomonas salina as ideal prey for selective feeding experiments (Meunier et al., 2016).
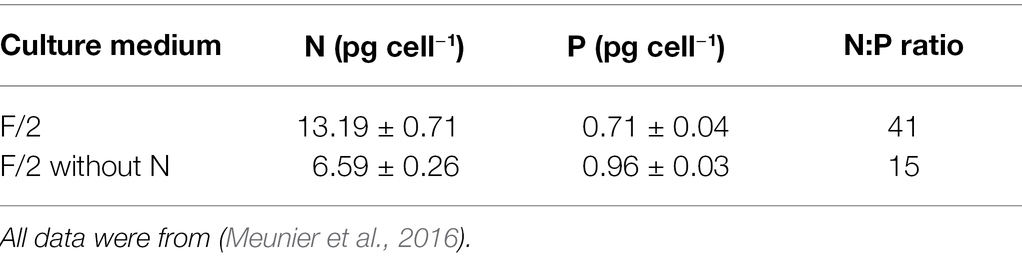
Table 2. The N and P contents (mean of four replicates ± SD) of Rhodomonas salinia cultured in F/2 medium and F/2 medium without N addition, respectively.
The selective feeding experiments were designed as feeding the Lepidodinium sp. grown in three nutrient conditions with mixed preys (high-N: low-N = 1:1). Before the selective feeding experiments, the Lepidodinium sp. cultures were starved for 2 days to ensure all Rhodomonas salina in the pre-conditioned cultures had been consumed and the food vacuole of Lepidodinium sp. was emptied, which minimised the influence of undigested food on the grazing selectivity of grazers. The Lepidodinium sp. cultures were starved for 1 day in the first-round experiments conducted at 25°C.
Upon the experiments, the Lepidodinium sp. cultures were filtered through 10 μm polycarbonate membrane filters (GVS Corporation) and resuspended into nutrient-free sterile artificial seawater to eliminate the remaining nutrient and prey. The high-N and low-N Rhodomonas salina used in the selective feeding experiments were centrifuged with a speed of 800 rpm for 5 min at 22°C and then washed and resuspended in nutrient-free autoclaved artificial seawater. The Lepidodinium sp. and Rhodomonas salina were observed under a microscope to guarantee that they were in good status after the treatments. The Lepidodinium sp. with a final concentration of 500 cells ml−1 (~1,000 cells ml−1 in first-round experiments) were transferred to 10 ml mediums with sufficient prey (100,000 ~ 160,000 cell ml−1). The nutrient concentration of the experimental mediums was the same as the pre-conditions. For instance, the N:P ratio of the experimental medium was set to be 16 when the ratio of pre-condition was 16. The prey was a mixture of high-N and low-N Rhodomonas salina in a ratio of 1:1. The control groups were set up with the same nutrient and prey concentrations but without grazers. All experimental and control groups were set up in triplicates. To prevent dramatic changes in nutrient concentrations and the depletion of prey, the grazing experiments lasted for 6 h (Båmstedt et al., 2000; Wilken et al., 2013), and the prey with different N contents (i.e., high-N prey and low-N prey) can be well distinguished after 6 h incubation (Supplementary Figure S1). In the first-round experiment conducted at 25°C, samples for measuring prey and predator concentrations were collected at four time points (0, 2, 4, 6 h), while in the second-round experiments conducted at three temperatures, the samples were collected only at 0 and 6 h of each experiment. Subsamples (2 ml) for counting the cell abundance of Lepidodinium sp. were collected, fixed by Lugol’s solution (final concentration 2%) and observed under a microscope. Other subsamples (1.8 ml) for prey concentration were collected, fixed by 50 μl 20% paraformaldehyde solution (0.5% final concentration) and analysed using a Becton-Dickinson FACSCalibur flow cytometer. The samples were run for 5 min at a high flow rate (57 ~ 60 μl/min) to enable sufficient events for calculations and minimise the measurement errors.
To compare the ingestion responses of mixotrophic species and heterotrophic species, we conducted another extra selective feeding experiment at 25°C using a heterotrophic species, O. marina. The experimental treatments of medium conditions and prey quality were the same as the abovementioned for Lepidodinium sp.
Estimate of Ingestion Rate and Prey Selectivity Index
Ingestion rates of Lepidodinium sp. and O. marina (I, prey predator h−1) in grazing experiments were calculated referring to the formula (Båmstedt et al., 2000):
where C0 and Ct are the prey concentrations at the beginning and end of the experiment (i.e., 0 and 6 h), respectively; [C] is the mean prey concentration; t is incubation time; n is the cell concentration of Lepidodinium sp. we set in the experiment as the Lepidodinium sp. concentration did not vary a lot over 6 h incubation (Supplementary Figure S2); and k is the instantaneous growth coefficient of the prey calculated by Eq. (2). In Eq. (2), Rt and R0 are the cell abundances of Rhodomonas salina in the control bottles without grazers at the end and beginning of the experiments, respectively. The ingestion rates for high-N and low-N preys were calculated using the corresponding prey concentrations at the beginning and end of experiments, respectively. This equation assumes a linear food reduction as a function of incubation time, which is confirmed by the trajectory of prey in the first-round experiments (Supplementary Figure S2). We then estimated the ingestion using Eq. (1) based on the reasonable prey reduction over 6 h incubation (Supplementary Figures S2, S3).
To evaluate the selectivity of Lepidodinium sp. towards the Rhodomonas salina with two different nutrition qualities, we calculated Manly’s α preference index, which compares the proportion of one prey in the diet with its proportion in the environment and is also known as Chesson’s index (Manly, 1974; Chesson, 1978, 1983):
where ri was the proportion of prey i in the diet, ni was the proportion of prey i in the environment, which was calculated by the abundance of prey i divided by the total sum of available prey abundance. The selectivity index α varies between 0 and 1, and αi = 0.5 indicated nonselective feeding towards the prey i. The Chesson’s index has been widely used to evaluate the prey selectivity of predators in aquatic ecosystems, such as insects (e.g., Klecka and Boukal, 2012), zooplankton (e.g., Meunier et al., 2016) and heterotrophic dinoflagellates (e.g., Hansen and Calado, 1999; Meunier et al., 2012).
Statistical Analysis
All data are expressed as the mean ± SD unless otherwise indicated. Differences in ingestion rate and prey selectivity among the treatments were tested using one-way ANOVA after grouping data by either temperature or nutrient condition. The Tukey’s Honest Significant Difference test (i.e., Tukey HSD test) was conducted following one-way ANOVA to examine the difference between specific groups by comparing all possible pairs of means. The effect of temperature and nutrient condition on ingestion rate and selectivity index was examined by two-way ANOVA. The student’s t-test was used to investigate whether the Chesson selectivity index α is significantly different from 0.5. All analyses were considered significant at p < 0.05 and conducted using GraphPad Prism (Version 8.3.0.) and R 3.4.3 (R Core Team, 2017).
Results
Compensatory Feeding and Selective Feeding Behaviour of Lepidodinium sp.
The total ingestion rate of Lepidodinium sp. on Rhodomonas salina (high-N + low-N) under NRedfield (N:P = 16) and Nlow (N:P = 4) conditions was significantly lower than the Nhigh (N:P = 64) conditions at 25°C in the first-round experiments (Tukey HSD test, p < 0.01; Figure 1A), which indicates that the Lepidodinium sp. may conduct compensatory feeding under Nhigh condition at 25°C. In this experiment, the ingestion rate of Lepidodinium sp. on high-N prey was significantly higher than on low-N prey under NRedfield and Nlow conditions where the nitrogen could be limited for Lepidodinium sp. (Tukey HSD test, p < 0.01; Figure 1B). Under these two situations (NRedfield and Nlow), Lepidodinium sp. exhibited selective feeding behaviour towards high-N prey, with the Chesson selectivity index α of 0.75 ± 0.01 and 0.70 ± 0.05, respectively, which were significantly different from 0.5 (Student’s t-test, p < 0.05; Figure 1C). By contrast, the ingestion rate on high-N prey and low-N prey was not significantly different when the inorganic N was sufficient with the N:P ratio of 64 (Tukey HSD test, p > 0.05; Figure 1B), although the Chesson selectivity index α is a bit higher than 0.5 (0.60 ± 0.06; p > 0.05; Figure 1C).
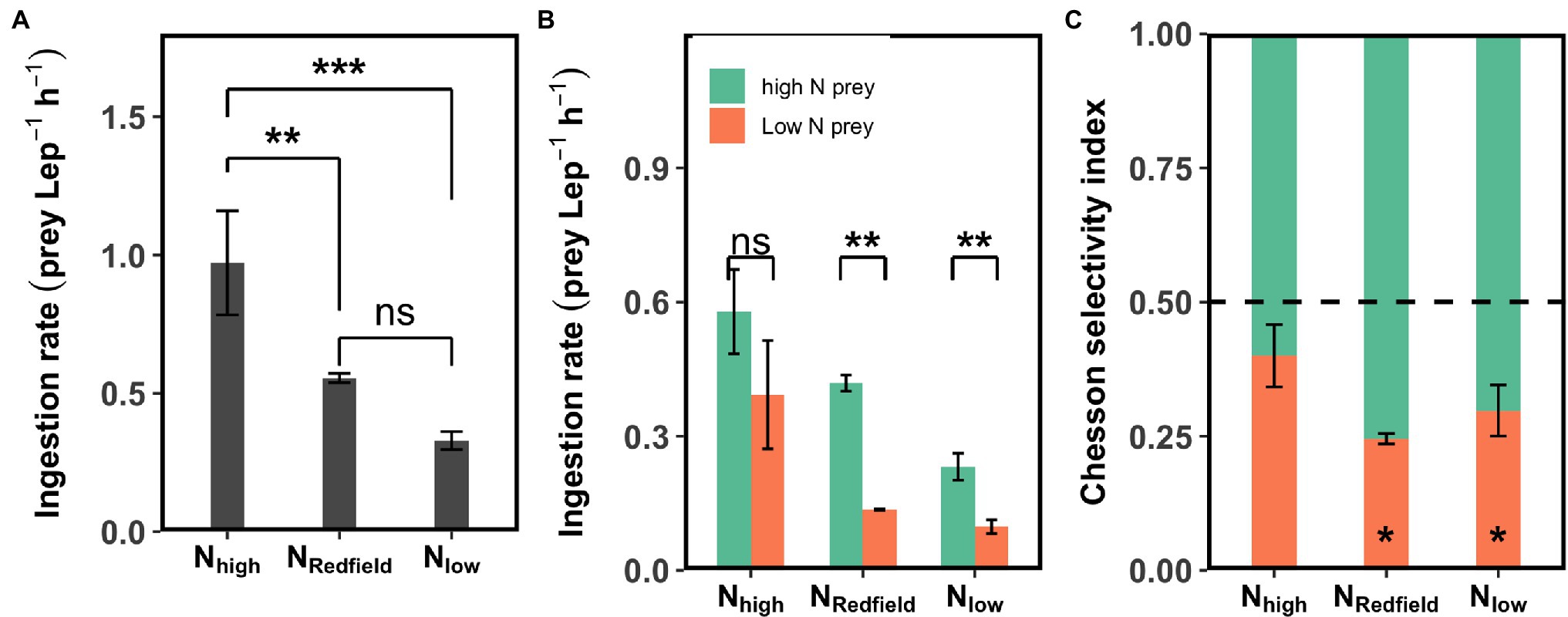
Figure 1. The results of first-round selective feeding experiments conducted at 25°C. The total ingestion rate (A), the ingestion rate on high-N and low-N prey (B), and the Chesson selectivity index (C) of Lepidodinium sp. under Nhigh (N:P ratio = 64), NRedfield (N:P ratio = 16), and Nlow (N:P ratio = 4) conditions at 25°C. The asterisk in (A) and (B) indicates the significant difference between specific groups, e.g., the high-N prey and low-N prey (Tukey HSD Test following a one-way ANOVA); the asterisk in (C) shows the difference of Chesson selectivity index from 0.5 (Student’s t-test; *p < 0.05; **p < 0.01; ***p < 0.001; and ns: p > 0.05).
The compensatory feeding and selective feeding behaviours were also observed at three temperatures in the second-round experiments. The patterns showed at 25°C in the two rounds of experiments were similar (Figures 1, 2, 3A), although the ingestion rate was lower in the first-round experiments because the initial concentration of Lepidodinium sp. was higher, and they were starved for only 1 day before experiments. At 28 and 31°C, the total ingestion rates under Nhigh and Nlow conditions were significantly higher than NRedfield conditions (Tukey HSD test, p < 0.05; Figure 2). The significant higher total ingestion rate under Nhigh and Nlow conditions were also observed at 19, 22 and 25°C in our preliminary experiments (Supplementary Figure S4), suggesting that Lepidodinium sp. ingest more prey when the inorganic nutrient ratio was imbalanced.
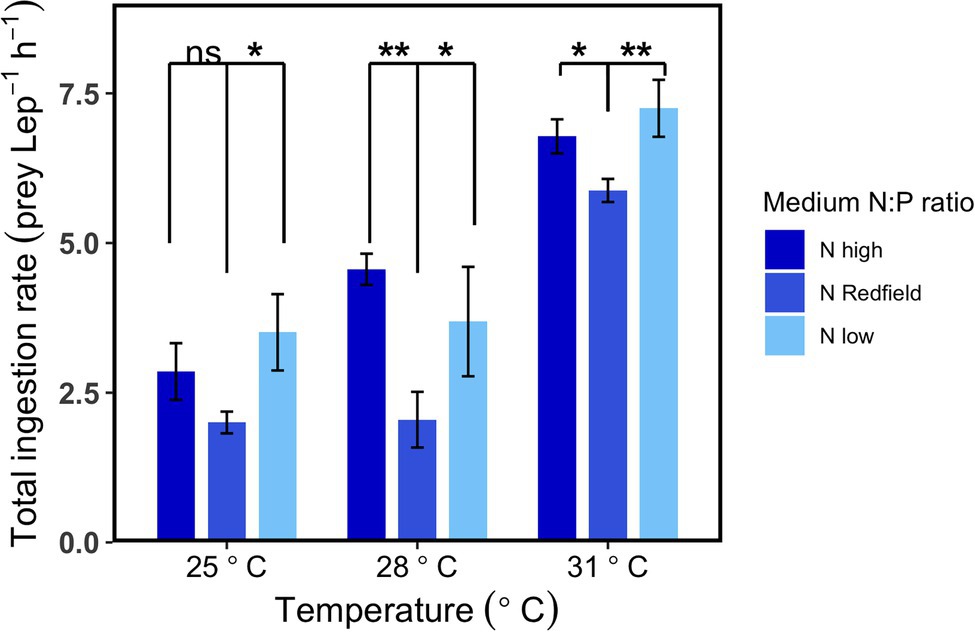
Figure 2. Total ingestion rate of Lepidodinium sp. under three different nutrient treatments (Nhigh, NRedfield and Nlow) at three different temperatures (25, 28 and 31°C) in the second-round experiments. The asterisk indicates significant difference from NRedfield conditions at each temperature (Tukey HSD Test following a one-way ANOVA; *p < 0.05; **p < 0.01; ***p < 0.001; and ns: p > 0.05).
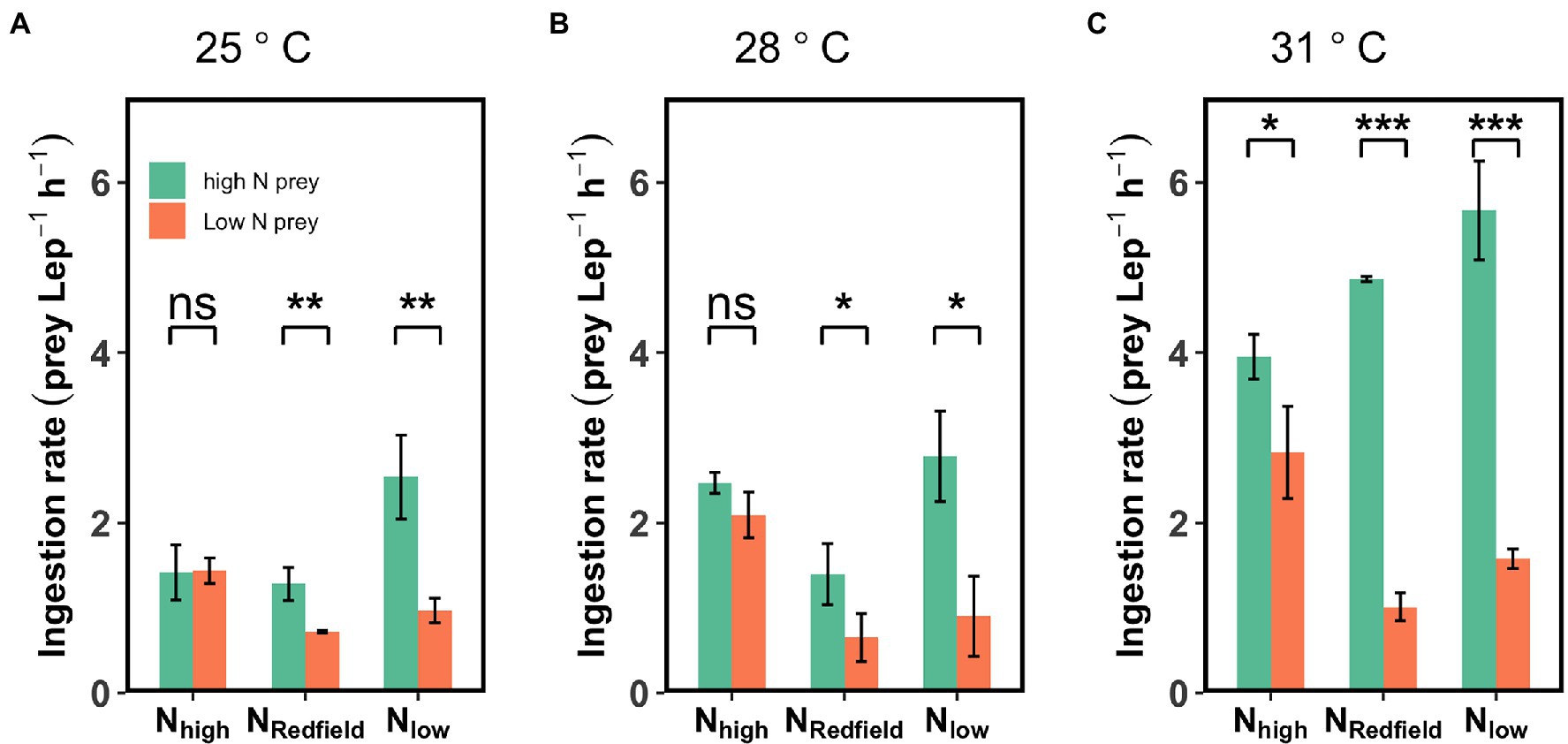
Figure 3. Ingestion rate of Lepidodinium sp. on high-N prey and low-N prey under three different nutrient treatments (Nhigh, NRedfield and Nlow) at 25°C (A), 28°C (B) and 31°C (C). The asterisk indicates the significant difference between high-N prey and low-N prey (Tukey HSD Test following a one-way ANOVA; *p < 0.05; **p < 0.01; ***p < 0.001; and ns: p > 0.05).
At 28°C, the difference between the ingestion rate on high-N prey and low-N prey was significant under NRedfield and Nlow conditions (Tukey HSD test; p < 0.05) but not the Nhigh conditions (p > 0.05; Figure 3B). While at 31°C, the selective feeding behaviour of Lepidodinium sp. not only occurred under NRedfield and Nlow but also under Nhigh conditions, as the ingestion rate on high-N prey was significantly higher than on low-N prey (Tukey HSD test; p < 0.05; Figure 3C).
In comparison with the mixotrophic Lepidodinium sp., although the heterotrophic dinoflagellate O. marina had a higher ingestion rate, they did not exhibit selective feeding on the Rhodomonas salina under three nutrient conditions. The Chesson selectivity index α was all about 0.5 (0.48 ± 0.05, 0.46 ± 0.07 and 0.45 ± 0.02 for Nhigh, NRedfield and Nlow, respectively; Supplementary Figure S5).
Effect of Temperature on the Total Ingestion Rate and Selective Feeding of Lepidodinium sp.
The total ingestion rate increased with increasing temperature under the three inorganic nutrient conditions (Figure 2; Supplementary Figure S4). The total ingestion rate of Lepidodinium sp. was affected by both temperature (two-way ANOVA, p < 0.001) and environmental inorganic nutrient conditions (two-way ANOVA, p < 0.01, Table 3).
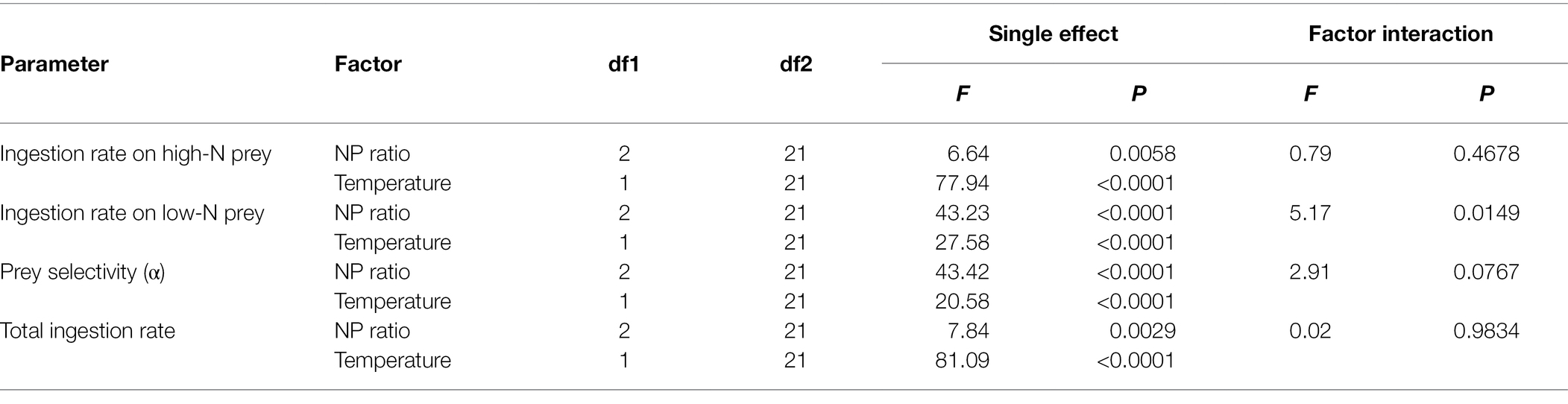
Table 3. Summary of the two-way ANOVA analysis on the effects of medium N:P ratios and temperature on the ingestion rate of different prey (high N and low N) and prey selectivity of Lepidodinium sp.
Increasing temperature not only increased the total ingestion rate of the Lepidodinium sp. but also exacerbated their feeding selectivity (Figures 3, 4). Under NRedfield and Nlow conditions, the ingestion rate of Lepidodinium sp. on the low-N prey remained unchanged at the three temperatures (one-way ANOVA, p > 0.05, Figure 3; Supplementary Figure S6). Nevertheless, the ingestion rate on high-N prey significantly increased when temperature increased from 28°C to 31°C under NRedfiled and Nlow conditions (Tukey HSD test; p < 0.05; Figure 3; Supplementary Figure S6). Therefore, the Chesson’s selectivity index α increased significantly from 0.64 ± 0.1 to 0.83 ± 0.02 under NRedfiled condition (Tukey HSD test; p < 0.0001; Figure 4). While rising temperature did not significantly affect prey selectivity of Lepidodinium sp. under Nlow condition because the selectivity index kept high (one-way ANOVA, p > 0.05; Figure 4). The Chesson selectivity index α was 0.76 ± 0.08 and 0.78 ± 0.03 under Nlow conditions at 28°C and 31°C, respectively (Figure 4). Under the Nhigh condition, Lepidodinium sp. increased their ingestion on both high-N and low-N prey when the temperature increased (Figure 3; Supplementary Figure S6). However, the increase in the ingestion rate on high-N prey was more significant, resulting in selective feeding and a slight increase in the Chesson’s selectivity index α at 31°C (0.58 ± 0.06; Figure 4).
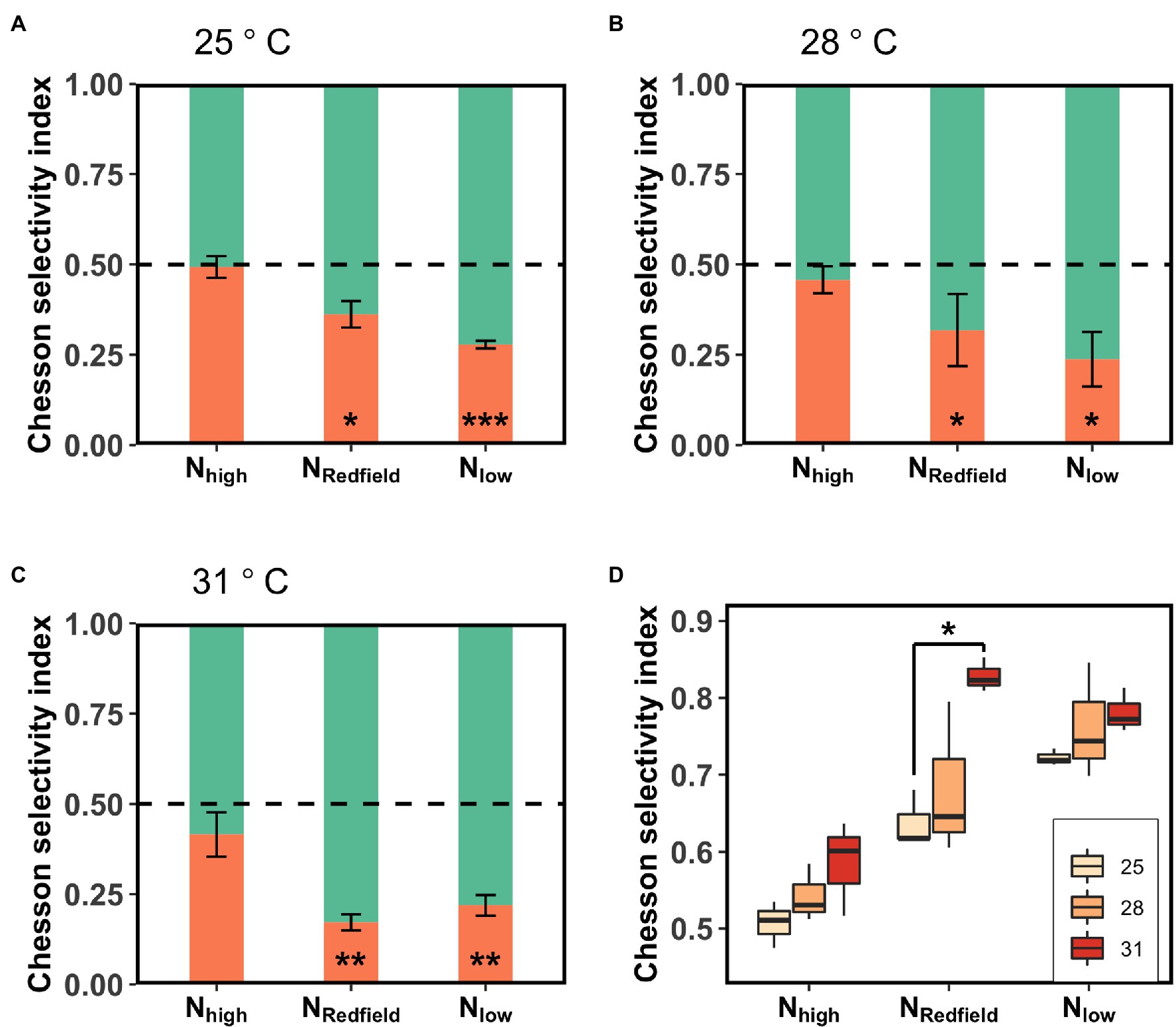
Figure 4. The Chesson selectivity index of Lepidodinium sp. under three different nutrient treatments (Nhigh, NRedfield and Nlow) at 25°C (A), 28°C (B) and 31°C (C). The green and orange bars represent the Chesson selectivity index towards high-N prey and low-N prey, respectively. The asterisk shows the difference of Chesson selectivity index from 0.5 (Student’s t-test; *p < 0.05; **p < 0.01; ***p < 0.001; and ns: p > 0.05). The data grouped by nutrient treatments was showed in (D). The asterisk indicates the significant difference between two temperatures (Tukey HSD Test following a one-way ANOVA).
The prey selectivity of Lepidodinium sp. was significantly regulated by both temperature (two-way ANOVA, p = 0.012 < 0.05) and pre-conditions with different medium N:P ratios (two-way ANOVA, p < 0.0001; Table 3).
Discussion
Although mixotrophs have long been recognised as widespread and critical components in planktonic communities and the aquatic food web (Mitra et al., 2016; Stoecker et al., 2017), how they respond to changing environments remains substantially understudied. In the current study, we investigated the ingestion activities of a mixotrophic dinoflagellate under various conditions with strong implications relevant to the response of similar mixotrophic protists to warming and the growing nutrient imbalance in aquatic ecosystems.
Compensatory Feeding and Selective Feeding of Mixotrophic Dinoflagellate Under Nutrient-Imbalanced Conditions
Lepidodinium sp. is a facultative phagotrophic dinoflagellate, of which photosynthesis is obligate and phagotrophy is facultative (Liu et al., 2021). Different from many mixotrophic algae that consume prey when inorganic nutrients are limited, Lepidodinium sp. can ingest prey even when nutrients are sufficient for their photosynthesis (Liu et al., 2021). Our study also observed the ingestion behaviours under NRedfiled conditions in which the nutrient concentrations (~20% f/2 medium) did not limit the growth of Lepidodinium sp. (Liu et al., 2021). This result suggests that the ingestion is advantageous to Lepidodinium sp. for more reasons than the supply of N or P. For instance, the ingestion could be the means for maintaining the internal stoichiometric balance (Stoecker et al., 2017). Moreover, Lepidodinium sp. increased their ingestion rate significantly when the inorganic N:P ratio in ambient water deviated from the Redfield ratio (i.e., Nhigh and Nlow conditions; Figures 1A, 2; Supplementary Figure S4). As the inorganic N:P ratios were achieved by manipulating either N or P concentrations, the increase in the ingestion rate could arise from the potential N or P limitations. It is likely that Lepidodinium sp. ingested more prey to acquire more P under Nhigh conditions and more N under Nlow conditions. The acquisition of P or N through enhanced ingestion then supports photosynthesis and growth. Thus, mixotrophy not only benefits the acquisition of carbon but also provides an important channel to replenish the nutrients (Glibert and Burkholder, 2011).
However, another explanation for the increasing ingestion rate under nutrient-imbalanced conditions is the lopsided N:P ratio rather than the limiting factors. In fact, the nutrient concentrations we used (2.7 μmol L−1 P for Nhigh and 43.8 μmol L−1 N for Nlow) were actually sufficient for Lepidodinium sp. to grow autotrophically (Liu et al., 2021). As such, the upregulation in the ingestion rate of Lepidodinium sp. may be for the sake of maintaining the cellular elemental balance, which has also been observed in other mixotrophic dinoflagellates such as Gyrodinium galatheanum and Prymnesium parvum. They also increased their feeding rate when grown under imbalanced N:P ratio conditions (Li et al., 2000; Lundgren et al., 2016). The feeding strategy is similar to the ‘compensatory feeding’ of heterotrophic consumers, which increase food uptake to extract the limiting nutrients only from the readily available parts (Raubenheimer and Jones, 2006; Montagnes et al., 2008; Meunier et al., 2012).
In addition to the ‘compensatory feeding’ behaviour, we also found that Lepidodinium sp. exhibits significant selective feeding behaviour towards N-rich prey under NRedfiled and Nlow conditions (Figures 1, 3, 4). Although the inorganic nutrient concentrations of NRedfiled condition were sufficient for the growth of Lepidodinium sp. (Liu et al., 2021), the N:P ratio may not be optimal, which renders Lepidodinium sp. to graze more N-rich prey to achieve the cellular optimal N:P ratios. Under the Nlow condition, the relatively low N may not satisfy the demand of Lepidodinium sp.; therefore, they grazed more N-rich prey to extract the limiting element more efficiently. It is also likely that the relatively low N disrupts the balance of the cellular stoichiometry of Lepidodinium sp., driving them to ingest more N to achieve the balance. By contrast, we did not observe the selective feeding behaviour of Lepidodinium sp. under Nhigh condition at 25 and 28°C because the inorganic N was in excess, and they do not need to ingest more N to supplement photosynthesis or for stoichiometric balance. Nevertheless, it could be possible that there was less P or P limitation under Nhigh condition, so Lepidodinium sp. fed more Rhodomonas salina to extract P contents (Figures 2, 3). Whereas the grazing was indiscriminate because the P contents of the two kinds of prey were similar (Table 2). Therefore, the selective feeding behaviour of mixotrophic protists is regulated by both inorganic nutrient conditions and the prey quality.
For comparison, we also examined the feeding behaviours of a heterotrophic protist (i.e., O. marina) under the same conditions. As the stoichiometric balance of heterotrophic protists mainly depends on ingestion activities, they may be more susceptible to prey quality and environmental changes. Nevertheless, we did not observe any selective feeding of O. marina under three N:P ratio conditions (Supplementary Figure S5). Also, prey with different N contents did not trigger their selective feeding behaviour. The result is consistent with a previous study, which found selective feeding of O. marina on P-rich prey but not on N-rich prey (Meunier et al., 2012). The results of the comparison suggest that mixotrophic protists might be more apt to selectively feed on different prey due to the influence of inorganic nutrients, which may help them adapt to the environmental changes. The ability of selective feeding and compensatory feeding may endow them with a competitive edge in nutrient-limited and -imbalanced environments. In such environments, the growth rate of mixotrophic protists could be higher than the comparable autotrophic and heterotrophic ones (Lundgren et al., 2016; Lin et al., 2018). Thus, the mixotrophs could become increasingly important in aquatic environments, especially in coastal waters with increasing nutrient imbalance (Mitra et al., 2014).
The feeding strategy (i.e., selective feeding or compensatory feeding) is commonly used by metazoan in aquatic ecosystems such as copepods (Boersma et al., 2016; Meunier et al., 2016) and Daphnia sp. (DeMott, 1998), which is conducive to compensate the elemental or biochemical deficiencies. The feeding behaviour of consumers strongly influences the environmental nutrient composition and prey community, such as the phytoplankton (Elser and Urabe, 1999; Vanni, 2002). Our study substantiated that phagotrophic algae also have the ability to use feeding strategy to compensate for the elemental deficiencies to maintain their internal balance, which gives feedback to the environments at the same time. By selectively feeding on the scarce elements, for instance, feeding more N-rich prey in low N:P ratio environments, Lepidodinium sp. may remove the limiting element from the phytoplankton community, which may indirectly reshape the community. Although the same prey with different nutritional contents designed in our experiments rarely occurs in nature, our results suggest that mixotrophic protists may selectively feed on prey with various C:N:P ratios under different conditions, potentially influencing the plankton community and food web structure (Ballen-Segura et al., 2017; Gerea et al., 2019).
Warming Exacerbates Selective Feeding Towards High-N Prey
The increase of nutrient imbalance in aquatic ecosystems is usually accompanied by temperature rise under the context of climate changes. In the current study, we examined the effect of nutrient imbalance on mixotrophs at different temperatures and found that warming exacerbates the selective feeding activities of Lepidodinium sp. (Figures 3, 4). This could be a result of the increasing contribution of ingestion activity to the growth of Lepidodinium sp. (Liu et al., 2021). Several empirical evidence has proved that warming shifts mixotrophs towards more heterotrophic (Wilken et al., 2013; Liu et al., 2021). According to the metabolic theory of ecology (i.e., MTE; Allen et al., 2005; Chen et al., 2012; Liu et al., 2019), heterotrophic ingestion is more sensitive to temperature changes than autotrophic photosynthesis. As such, phagotrophy would become more important for mixotrophs as temperature increases. In the current study, we observed that Lepidodinium sp. has significantly increased its ingestion rate to satisfy its growth demand (Figure 2; Supplementary Figure S4). Moreover, the increases in the ingestion rate with increasing temperature were all contributed by the ingestion on the high-N prey, while the ingestion on the low-N prey remained unchanged, leading to higher Chesson’s selectivity index under NRedfiled and Nlow conditions (Figure 4). As warming may change the internal N:P ratios and break the balance (Thrane et al., 2017), Lepidodinium sp. tended to graze more N-rich food to achieve stoichiometry balance as temperature increased (Figure 3). Thus, warming exacerbates Lepidodinium sp.’s selective feeding towards high-N prey.
Also, under the high N:P ratio condition, Lepidodinium sp. started to show selective feeding behaviour when temperature increased (Figures 3, 4). Although the demand for N for growth increased with increasing temperature, the concentration we set was relatively high (175 μmol/l, Table 1), which should be sufficient for Lepidodinium sp., even at high temperatures. It is possible that nitrogen uptake and photosynthesis of Lepidodinium sp. are constrained at high temperatures. In our previous study, we found that the autotrophic growth determined by photosynthesis was significantly lower than the mixotrophic growth determined by both ingestion and photosynthesis at high temperatures, which suggested that photosynthesis may be constrained by high temperature and phagocytosis contributed more to the growth of the mixotrophs (Liu et al., 2021). To maintain the stoichiometric balance that may be shifted by the increase of temperature, Lepidodinium sp. ingested more N-rich food to compensate for the deficiency of photosynthesis. That may explain the selective feeding towards high-N prey of mixotrophic protists even under inorganic N replete conditions as temperature increases, which points to an increase in heterotrophic activity of mixotrophs in the warmer ocean.
Conclusion
In the past four decades, seawater N:P ratios in many coasts and estuaries increased dramatically due to increasing nitrogen fertiliser and domestic and industrial wastewater discharge (Glibert et al., 2013, 2014). This might cause an increase in the ingestion rate of mixotrophs with a similar ecological niche as Lepidodinium sp. in the future and subsequently shift their functional role from primary producers to consumers (Mitra et al., 2014; Leles et al., 2018). Moreover, selective feeding towards prey with high nutritional quality may influence the community composition of phytoplankton. A warmer ocean could exacerbate such trends that cause the mixotrophs to become more heterotrophic and consequently change the structure of the planktonic food web and carbon and nutrient cycling in marine ecosystems. It should be pointed out that there are many types of mixotrophs, from highly autotrophic to highly heterotrophic (Stoecker, 1998; Mitra et al., 2016; Stoecker et al., 2017). More studies should focus on the response of different types of mixotrophic species to various environmental factors to elucidate and predict the ecological roles of the mixotrophs in marine ecosystems under projected climate warming.
Data Availability Statement
The raw data supporting the conclusions of this article will be made available by the authors, without undue reservation.
Author Contributions
HN, KL and HL conceived the study and designed the experiments. HN conducted the experiments. ZG helped repeated the experiments. KL and HN wrote the draft. KL and HL revised and improved the manuscript. All authors contributed to the article and approved the submitted version.
Funding
This study was supported by the Research Grants Council of Hong Kong (16101318 and T21/602/16), the Key Special Project for Introduced Talents Team of Southern Marine Science and Engineering Guangdong Laboratory (Guangzhou) (GML2019ZD0409), and the Hong Kong Branch of the Southern Marine Science and Engineering Guangdong Laboratory (Guangzhou; SMSEGL20SC01).
Conflict of Interest
The authors declare that the research was conducted in the absence of any commercial or financial relationships that could be construed as a potential conflict of interest.
Publisher’s Note
All claims expressed in this article are solely those of the authors and do not necessarily represent those of their affiliated organizations, or those of the publisher, the editors and the reviewers. Any product that may be evaluated in this article, or claim that may be made by its manufacturer, is not guaranteed or endorsed by the publisher.
Acknowledgments
The authors sincerely thank two reviewers for their helpful comments.
Supplementary Material
The Supplementary Material for this article can be found online at https://www.frontiersin.org/articles/10.3389/fmicb.2022.805306/full#supplementary-material
References
Allen, A. P., Gillooly, J. F., and Brown, J. H. (2005). Linking the global carbon cycle to individual metabolism. Funct. Ecol. 19, 202–213. doi: 10.1111/j.1365-2435.2005.00952.x
Ballen-Segura, M., Felip, M., and Catalan, J. (2017). Some mixotrophic flagellate species selectively graze on archaea. Appl. Environ. Microbiol. 83, e02317–e02316. doi: 10.1128/AEM.02317-16
Båmstedt, U., Gifford, D. J., Irigoien, X., Atkinson, A., and Roman, M. (2000). “Feeding,” in ICES Zooplankton Methodology Manual. eds. R. Harris, P. Wiebe, J. Lenz, H. R. Skjoldal, and M. Huntley (Unites States: Academic Press), 297–380.
Boersma, M., Mathew, K. A., Niehoff, B., Schoo, K. L., Franco-Santos, R. M., and Meunier, C. L. (2016). Temperature driven changes in the diet preference of omnivorous copepods: no more meat when it’s hot? Ecol. Lett. 19, 45–53. doi: 10.1111/ele.12541
Chen, B., Landry, M. R., Huang, B., and Liu, H. (2012). Does warming enhance the effect of microzooplankton grazing on marine phytoplankton in the ocean? Limnol. Oceanogr. 57, 519–526. doi: 10.4319/lo.2012.57.2.0519
Chesson, J. (1978). Measuring preference in selective predation. Ecology 59, 211–215. doi: 10.2307/1936364
Chesson, J. (1983). The estimation and analysis of preference and its relationship to foraging models. Ecology 64, 1297–1304. doi: 10.2307/1937838
Cruz-Rivera, E., and Hay, M. E. (2000). Can quantity replace quality? Food choice, compensatory feeding, and fitness of marine mesograzers. Ecology 81, 201–219. doi: 10.1890/0012-9658(2000)081[0201:CQRQFC]2.0.CO;2
DeMott, W. R. (1998). Utilization of a cyanobacterium and a phosphorus deficient green alga as complementary resources by daphnids. Ecology 79, 2463–2481. doi: 10.1890/0012-9658(1998)079[2463:UOACAA]2.0.CO;2
Elser, J. J., and Urabe, J. (1999). The stoichiometry of consumer driven nutrient recycling: theory, observations, and consequences. Ecology 80, 735–751. doi: 10.1890/0012-9658(1999)080[0735:TSOCDN]2.0.CO;2
Flynn, K. J., and Mitra, A. (2009). Building the “perfect beast”: modelling mixotrophic plankton. J. Plankton Res. 31, 965–992. doi: 10.1093/plankt/fbp044
Frost, P. C., Evans-White, M. A., Finkel, Z. V., Jensen, T. C., and Matzek, V. (2005). Are you what you eat? Physiological constraints on organismal stoichiometry in an elementally imbalanced world. Oikos 109, 18–28. doi: 10.1111/j.0030-1299.2005.14049.x
Gerea, M., Queimaliños, C., and Unrein, F. (2019). Grazing impact and prey selectivity of picoplanktonic cells by mixotrophic flagellates in oligotrophic lakes. Hydrobiologia 831, 5–21. doi: 10.1007/s10750-018-3610-3
Glibert, P. M., and Burkholder, J. M. (2011). Harmful algal blooms and eutrophication: “strategies” for nutrient uptake and growth outside the Redfield comfort zone. Chin. J. Oceanogr. Limnol. 29, 724–738. doi: 10.1007/s00343-011-0502-z
Glibert, P. M., Kana, T. M., and Brown, K. (2013). From limitation to excess: consequences of substrate excess and stoichiometry for phytoplankton physiology, trophodynamics and biogeochemistry, and implications for modeling. J. Mar. Syst. 125, 14–28. doi: 10.1016/j.jmarsys.2012.10.004
Glibert, P. M., Maranger, R., Sobota, D. J., and Bouwman, L. (2014). The Haber Bosch –harmful algal bloom (HB-HAB) link. Environ. Res. Lett. 9:105001. doi: 10.1088/1748-9326/9/10/105001
Hansen, P. J., and Calado, A. J. (1999). Phagotrophic mechanisms and prey selection in free-living dinoflagellates1. J. Eukaryot. Microbiol. 46, 382–389. doi: 10.1111/j.1550-7408.1999.tb04617.x
Huang, X., Huang, L., and Yue, W. (2003). The characteristics of nutrients and eutrophication in the Pearl River estuary, South China. Mar. Pollut. Bull. 47, 30–36. doi: 10.1016/S0025-326X(02)00474-5
John, E., and Davidson, K. (2001). Prey selectivity and the influence of prey carbon: nitrogen ratio on microflagellate grazing. J. Exp. Mar. Biol. Ecol. 260, 93–111. doi: 10.1016/S0022-0981(01)00244-1
Klecka, J., and Boukal, D. S. (2012). Who eats whom in a pool? A comparative study of prey selectivity by predatory aquatic insects. PLoS One 7:e37741. doi: 10.1371/journal.pone.0037741
Knisely, K., and Geller, W. (1986). Selective feeding of four zooplankton species on natural lake phytoplankton. Oecologia 69, 86–94. doi: 10.1007/BF00399042
Legrand, C., Graneli, E., and Carlsson, P. (1998). Induced phagotrophy in the photosynthetic dinoflagellate Heterocapsa triquetra. Aquat. Microb. Ecol. 15, 65–75. doi: 10.3354/ame015065
Leles, S. G., Polimene, L., Bruggeman, J., Blackford, J., Ciavatta, S., Mitra, A., et al. (2018). Modelling mixotrophic functional diversity and implications for ecosystem function. J. Plankton Res. 40, 627–642. doi: 10.1093/plankt/fby044
Li, A., Stoecker, D. K., and Coats, D. W. (2000). Mixotrophy ingyrodinium galatheanum(DINOPHYCEAE): grazing responses to light intensity and inorganic nutrients*. J. Phycol. 36, 33–45. doi: 10.1046/j.1529-8817.2000.98076.x
Lin, C.-H., Flynn, K. J., Mitra, A., and Glibert, P. M. (2018). Simulating effects of variable stoichiometry and temperature on mixotrophy in the harmful dinoflagellate Karlodinium veneficum. Front. Mar. Sci. 5:320. doi: 10.3389/fmars.2018.00320
Liu, K., Chen, B., Zhang, S., Sato, M., Shi, Z., and Liu, H. (2019). Marine phytoplankton in subtropical coastal waters showing lower thermal sensitivity than microzooplankton. Limnol. Oceanogr. 64, 1103–1119. doi: 10.1002/lno.11101
Liu, K., Ng, Y. H., Zhang, S., and Liu, H. (2021). Effects of temperature on a mixotrophic dinoflagellate (Lepidodinium sp.) under different nutritional strategies. Mar. Ecol. Prog. Ser. 678, 37–49. doi: 10.3354/meps13865
Lundgren, V. M., Glibert, P. M., Granéli, E., Vidyarathna, N. K., Fiori, E., Ou, L., et al. (2016). Metabolic and physiological changes in Prymnesium parvum when grown under, and grazing on prey of, variable nitrogen: phosphorus stoichiometry. Harmful Algae 55, 1–12. doi: 10.1016/j.hal.2016.01.002
Manly, B. (1974). A model for certain types of selection experiments. Biometrics 30, 281–294. doi: 10.2307/2529649
Meunier, C. L., Boersma, M., Wiltshire, K. H., and Malzahn, A. M. (2016). Zooplankton eat what they need: copepod selective feeding and potential consequences for marine systems. Oikos 125, 50–58. doi: 10.1111/oik.02072
Meunier, C. L., Hantzsche, F. M., Cunha-Dupont, A. Ö., Haafke, J., Oppermann, B., Malzahn, A. M., et al. (2012). Intraspecific selectivity, compensatory feeding and flexible homeostasis in the phagotrophic flagellate Oxyrrhis marina: three ways to handle food quality fluctuations. Hydrobiologia 680, 53–62. doi: 10.1007/s10750-011-0900-4
Mitra, A., Flynn, K. J., Burkholder, J. M., Berge, T., Calbet, A., Raven, J. A., et al. (2014). The role of mixotrophic protists in the biological carbon pump. Biogeosciences 11, 995–1005. doi: 10.5194/bg-11-995-2014
Mitra, A., Flynn, K. J., Tillmann, U., Raven, J. A., Caron, D., Stoecker, D. K., et al. (2016). Defining planktonic Protist functional groups on mechanisms for energy and nutrient acquisition: incorporation of diverse Mixotrophic strategies. Protist 167, 106–120. doi: 10.1016/j.protis.2016.01.003
Moeller, H. V., Neubert, M. G., and Johnson, M. D. (2019). Intraguild predation enables coexistence of competing phytoplankton in a well-mixed water column. Ecology 100:e02874. doi: 10.1002/ecy.2874
Montagnes, D. J., Barbosa, A. B., Boenigk, J., Davidson, K., Jürgens, K., Macek, M., et al. (2008). Selective feeding behaviour of key free-living protists: avenues for continued study. Aquat. Microb. Ecol. 53, 83–98. doi: 10.3354/ame01229
Moorthi, S. D., Ptacnik, R., Sanders, R. W., Fischer, R., Busch, M., and Hillebrand, H. (2017). The functional role of planktonic mixotrophs in altering seston stoichiometry. Aquat. Microb. Ecol. 79, 235–245. doi: 10.3354/ame01832
Moreno, A. R., and Martiny, A. C. (2018). Ecological stoichiometry of ocean plankton. Annu. Rev. Mar. Sci. 10, 43–69. doi: 10.1146/annurev-marine-121916-063126
Ng, W. A., Liu, H., and Zhang, S. (2017). Diel variation of grazing of the dinoflagellate Lepidodinium sp. and ciliate Euplotes sp. on algal prey: the effect of prey cell properties. J. Plankton Res. 39, 450–462. doi: 10.1093/plankt/fbx020
R Core Team (2017). R: A language and environment for statistical computing. Vienna, Austria: R Foundation for Statistical Computing. Available at: https://www.r-project.org
Raubenheimer, D., and Jones, S. A. (2006). Nutritional imbalance in an extreme generalist omnivore: tolerance and recovery through complementary food selection. Anim. Behav. 71, 1253–1262. doi: 10.1016/j.anbehav.2005.07.024
Raven, J. A. (1997). Phagotrophy in phototrophs. Limnol. Oceanogr. 42, 198–205. doi: 10.4319/lo.1997.42.1.0198
Smalley, G. W., and Coats, D. W. (2002). Ecology of the red-tide dinoflagellate Ceratium furca: distribution, mixotrophy, and grazing impact on ciliate populations of Chesapeake Bay. J. Eukaryot. Microbiol. 49, 63–73. doi: 10.1111/j.1550-7408.2002.tb00343.x
Sterner, R. W., and Elser, J. J. (2002). Ecological Stoichiometry: The Biology of Elements from Molecules to the Biosphere. Princeton University Press, New Jersy.
Stoecker, D. K. (1998). Conceptual models of mixotrophy in planktonic protists and some ecological and evolutionary implications. Eur. J. Protistol. 34, 281–290. doi: 10.1016/s0932-4739(98)80055-2
Stoecker, D. K., Hansen, P. J., Caron, D. A., and Mitra, A. (2017). Mixotrophy in the marine plankton. Annu. Rev. Mar. Sci. 9, 311–335. doi: 10.1146/annurev-marine-010816-060617
Thrane, J. E., Hessen, D. O., and Andersen, T. (2017). Plasticity in algal stoichiometry: experimental evidence of a temperature-induced shift in optimal supply N: P ratio. Limnol. Oceanogr. 62, 1346–1354. doi: 10.1002/lno.10500
Vanni, M. J. (2002). Nutrient cycling by animals in freshwater ecosystems. Annu. Rev. Ecol. Syst. 33, 341–370. doi: 10.1146/annurev.ecolsys.33.010802.150519
Wickham, S. A., and Wimmer, R. (2019). Does Mixotrophy in ciliates compensate for poor-quality prey? Experiments with heterotrophic–mixotrophic species pairs. J. Plankton Res. 41, 583–593. doi: 10.1093/plankt/fbz052
Wilken, S., Huisman, J., Naus-Wiezer, S., and Van Donk, E. (2013). Mixotrophic organisms become more heterotrophic with rising temperature. Ecol. Lett. 16, 225–233. doi: 10.1111/ele.12033
Keywords: mixotrophs, ingestion, warming, nutrient imbalance, prey quality
Citation: Liu K, Ng HY-T, Gao Z and Liu H (2022) Selective Feeding of a Mixotrophic Dinoflagellate (Lepidodinium sp.) in Response to Experimental Warming and Inorganic Nutrient Imbalance. Front. Microbiol. 13:805306. doi: 10.3389/fmicb.2022.805306
Edited by:
Yuanyuan Feng, Shanghai Jiao Tong University, ChinaReviewed by:
Qian Li, Shanghai Jiao Tong University, ChinaCasey Michael Godwin, University of Michigan, United States
Copyright © 2022 Liu, Ng, Gao and Liu. This is an open-access article distributed under the terms of the Creative Commons Attribution License (CC BY). The use, distribution or reproduction in other forums is permitted, provided the original author(s) and the copyright owner(s) are credited and that the original publication in this journal is cited, in accordance with accepted academic practice. No use, distribution or reproduction is permitted which does not comply with these terms.
*Correspondence: Hongbin Liu, liuhb@ust.hk