- Institute of Bio- and Geosciences, IBG-1: Biotechnology, Forschungszentrum Jülich GmbH, Jülich, Germany
For regulatable target gene expression in the acetic acid bacterium (AAB) Gluconobacter oxydans only recently the first plasmids became available. These systems solely enable AraC- and TetR-dependent induction. In this study we showed that the L-rhamnose-dependent regulator RhaS from Escherichia coli and its target promoters PrhaBAD, PrhaT, and PrhaSR could also be used in G. oxydans for regulatable target gene expression. Interestingly, in contrast to the responsiveness in E. coli, in G. oxydans RhaS increased the expression from PrhaBAD in the absence of L-rhamnose and repressed PrhaBAD in the presence of L-rhamnose. Inserting an additional RhaS binding site directly downstream from the −10 region generating promoter variant PrhaBAD(+RhaS-BS) almost doubled the apparent RhaS-dependent promoter strength. Plasmid-based PrhaBAD and PrhaBAD(+RhaS-BS) activity could be reduced up to 90% by RhaS and L-rhamnose, while a genomic copy of PrhaBAD(+RhaS-BS) appeared fully repressed. The RhaS-dependent repression was largely tunable by L-rhamnose concentrations between 0% and only 0.3% (w/v). The RhaS-PrhaBAD and the RhaS-PrhaBAD(+RhaS-BS) systems represent the first heterologous repressible expression systems for G. oxydans. In contrast to PrhaBAD, the E. coli promoter PrhaT was almost inactive in the absence of RhaS. In the presence of RhaS, the PrhaT activity in the absence of L-rhamnose was weak, but could be induced up to 10-fold by addition of L-rhamnose, resulting in a moderate expression level. Therefore, the RhaS-PrhaT system could be suitable for tunable low-level expression of difficult enzymes or membrane proteins in G. oxydans. The insertion of an additional RhaS binding site directly downstream from the E. coli PrhaT −10 region increased the non-induced expression strength and reversed the regulation by RhaS and L-rhamnose from inducible to repressible. The PrhaSR promoter appeared to be positively auto-regulated by RhaS and this activation was increased by L-rhamnose. In summary, the interplay of the L-rhamnose-binding RhaS transcriptional regulator from E. coli with its target promoters PrhaBAD, PrhaT, PrhaSR and variants thereof provide new opportunities for regulatable gene expression in G. oxydans and possibly also for simultaneous L-rhamnose-triggered repression and activation of target genes, which is a highly interesting possibility in metabolic engineering approaches requiring redirection of carbon fluxes.
Introduction
The acetic acid bacterium (AAB) Gluconobacter oxydans harbors the beneficial ability of regio- and stereoselective incomplete oxidation of a variety of sugars, sugar alcohols and other substrates in the periplasm by membrane-bound dehydrogenases (mDHs) and release of resulting products into the cultivation medium (Mamlouk and Gullo, 2013; Pappenberger and Hohmann, 2014; Mientus et al., 2017). Therefore, G. oxydans is industrially used for oxidative biotransformations of carbohydrates to produce, e.g., the tanning lotion additive dihydroxyacetone, the vitamin C precursor L-sorbose, and 6-amino-L-sorbose used for production of the antidiabetic drug miglitol (Ameyama et al., 1981; Saito et al., 1997; Gupta et al., 2001; Tkac et al., 2001; Hekmat et al., 2003; Wang et al., 2016). The industrial versatility of G. oxydans, current applications and future perspectives have been reviewed recently (da Silva et al., 2022).
For target gene expression in G. oxydans, only constitutive promoters were used in the past due to the lack of a regulatable promoter. For expression, derivatives of the pBBR1MCS plasmid family obtained from the endogenous plasmid pBBR1 from Bordetella bronchiseptica were the most successful shuttle and expression vectors used (reviewed in Fricke et al., 2021a). Since pBBR1MCS-2 conferring kanamycine resistance typically results in an abnormal cell morphology of G. oxydans in the presence of kanamycin and potentially also in reduced expression performance, pBBR1MCS-5 and the use of gentamicin is advantageous (Fricke et al., 2021b). However, both plasmid backbones recently enabled high functionality of transferred heterologous expression systems for regulatable target gene expression in G. oxydans for the first time. Firstly, the L-arabinose-dependent AraC-ParaBAD system from Escherichia coli MC4100, which exhibits a better araC codon usage in G. oxydans than araC from E. coli MG1655, was tunable and inducible up to 480-fold (Fricke et al., 2020). Interestingly, in G. oxydans the AraC target promoter ParaBAD from E. coli was not active in the absence of AraC. This indicated that ParaBAD alone is not recognized by the G. oxydans RNA polymerase. Therefore, the typical repression of ParaBAD by AraC in the absence of the inducer L-arabinose was not required to ensure non-induced tightness of ParaBAD in G. oxydans. Secondly, the TetR-Ptet system in its native divergent organization as present in the E. coli transposon Tn10 exhibited extremely low basal expression in G. oxydans and achieved more than 3,500-fold induction according to reporter assays using the fluorescence protein mNeonGreen (Fricke et al., 2021b). In contrast to ParaBAD and AraC, Ptet highly required the repression by its regulator TetR for tightness of the system, otherwise the expression from Ptet was very strong in G. oxydans without TetR. Moreover, in cases where the native divergent organization tetR-PtetR-Ptet-gene-of-interest is leaky, modifying the genetic organization that the target gene and tetR expression both are under control of Ptet and therefore expressed as an operon and auto-regulated by TetR, can improve the non-induced tightness and the resulting inducibility of Ptet in G. oxydans (Bertucci et al., 2022).
In this study, to expand the still very limited genetic toolbox for regulatable target gene expression in G. oxydans we chose to test the L-rhamnose-dependent RhaSR system from E. coli (Baldoma et al., 1990; Egan and Schleif, 1993, 1994; Vía et al., 1996; Bhende and Egan, 1999; Wickstrum et al., 2010). Compared to the AraC-, TetR-, and LacI-based systems from E. coli, the RhaRS system offers special features that could be particularly interesting and useful for applications in G. oxydans or AAB in general (Supplementary Figure S1). Firstly, the system comprises not only one, but two transcriptional regulators, RhaR and RhaS, both responding to L-rhamnose. They are encoded by the rhaSR operon and are expressed from the promoter PrhaSR. In E. coli, basal expression from PrhaSR is positively auto-regulated by RhaR in the presence of L-rhamnose, resulting in increased expression of the rhaSR operon and in turn PrhaSR is negatively auto-regulated by RhaS since RhaS is also able to bind to the RhaR binding site at PrhaSR, competing with RhaR and blocking rhaSR expression. Secondly, the major target promoters of RhaS are PrhaBAD and PrhaT. PrhaBAD drives transcription of the structural rhaBAD genes encoding the L-rhamnose catabolic enzymes L-rhamnulose kinase, L-rhamnose isomerase and L-rhamnulose-1-phosphate aldolase. PrhaT drives transcription of rhaT encoding an L-rhamnose transport system. In E. coli, RhaS activates transcription from PrhaBAD and PrhaT in the presence of L-rhamnose. Furthermore, in E. coli the L-rhamnose metabolism is under catabolite repression by glucose, which is overcome by the binding of the cAMP receptor protein (CRP) to consensus recognition sequences found in all three Prha promoters and interaction of CRP with the RNA polymerase, which depends on the bending of the promoter DNA by RhaS or RhaR. In G. oxydans CRP is absent since the predicted CRP gene (GOX0974/GOX_RS06010) was shown to encode an iron–sulfur cluster protein termed GoxR, an FNR-type transcriptional regulator of genes involved in respiration and redox metabolism (Schweikert et al., 2021). Overall, it seemed very interesting to analyze how RhaS, RhaR, and the promoters PrhaBAD, PrhaT, and PrhaSR perform in G. oxydans and if they could be useful for regulatable gene expression in this AAB.
We found that in G. oxydans the RhaS-dependent regulation of PrhaBAD surprisingly was reversed compared to E. coli. In the absence of L-rhamnose, RhaS increased expression from PrhaBAD and in the presence of L-rhamnose RhaS repressed PrhaBAD enabling complete repression of a genomically encoded PrhaBAD promoter variant, thereby potentially providing a dynamic knock-down system for genes in G. oxydans. The effects and properties of the L-rhamnose-binding RhaS regulator and the promoters PrhaBAD, PrhaT, and PrhaSR from E. coli exhibit very interesting characteristics in G. oxydans and provide new opportunities for regulatable gene expression, both in fundamental research and metabolic engineering approaches.
Materials and methods
Bacterial strains, plasmids, media and growth conditions
Bacterial strains and plasmids used in this study and their relevant characteristics are listed in Table 1. G. oxydans cells were routinely cultivated in D-mannitol complex medium containing 40 g L−1 D-mannitol, 5 g L−1 yeast extract, 1 g L−1 KH2PO4, 1 g L−1 (NH4)2SO4, and 2.5 g L−1 MgSO4 × 7 H2O at 30°C. The initial pH of the medium was set to 6 by the addition of KOH (5 M stock). Because G. oxydans possesses a natural resistance toward cefoxitin, 50 μg ml−1 of the antibiotic was routinely added to the medium as a precaution to prevent bacterial contaminations. Stock solutions of cefoxitin (50 mg ml−1) and D-mannitol (200 g L−1) were sterile-filtered and added to autoclaved medium. Unless stated otherwise, for shake flask cultivations cells from 10 ml overnight pre-cultures were used to inoculate 50 ml D-mannitol medium in 500 ml shaking flasks with three baffles to an initial optical density at 600 nm (OD600) of 0.3 (UV-1800, Shimadzu). All shake flasks cultures were grown on a rotary shaker at an agitation speed of 180 rpm. G. oxydans cells harboring pBBR1MCS-5-based plasmids were supplemented with 10 μg ml−1 gentamicin (Kovach et al., 1994). Escherichia coli strains were cultivated at 37°C and 160 rpm in lysogeny broth (LB) medium. Medium of E. coli carrying pBBR1MCS-5-based plasmids was supplemented with 10 μg ml−1 gentamicin. Escherichia coli S17-1 was used as donor strain to transform G. oxydans by conjugation (Kiefler et al., 2017). Competent E. coli S17-1 were prepared and transformed by CaCl2 procedure as described (Hanahan, 1983).
Recombinant DNA work
All DNA oligonucleotides used in this study were obtained from Eurofins Genomics and are listed in Supplementary Table S1. All enzymes required for recombinant DNA work were purchased from Thermo Scientific. Polymerase chain reactions (PCR) used for DNA manipulation and plasmid verification followed standard protocols as described (Sambrook et al., 1989). For amplification of DNA fragments Q5 DNA polymerases was utilized as recommended by the manufacturer (New England Biolabs). All reporter plasmids were constructed in a one-step isothermal Gibson assembly (50°C, 1 h) by integrating amplified DNA fragments into the restricted broad-host vector derivative pBBR1MCS-5-TgdhM-MCS-TGOX0028 (Gibson et al., 2009). All DNA modifications to create the desired plasmids were conducted in E. coli S17-1. For plasmid isolation a QIAprep spin miniprep kit (Qiagen) was used according to the manufacturer’s protocol. The correctness of the plasmid inserts was checked by DNA sequencing (Eurofins MWG).
Construction of plasmids
In this study, all plasmids were constructed using the vector pBBR1MCS-5-TgdhM-MCS-TGOX0028 that we created previously for the TetR-Ptet system (Fricke et al., 2021b). The terminator sequences of GOX0265 (TgdhM) and GOX0028 (TGOX0028) flank the multiple cloning site (MCS) to reduce potential interferences caused by genetic elements on the plasmid backbone. Unless stated otherwise, pBBR1MCS-5-TgdhM-MCS-TGOX0028 was restricted for insert integration with the restriction endonucleases XbaI and EcoRI. Furthermore, in all constructs using the promoters PrhaBAD, PrhaSR, PrhaT, PGOX0264, or PGOX0452 to express the reporter gene mNeonGreen (mNG), the ribosome binding site (RBS) AGGAGA was placed upstream from mNG and downstream from the naturally occurring RBS of the respective promoter region.
For construction of plasmid pBBR1MCS-5-rhaSR-PrhaSR-PrhaBAD-mNG, two DNA fragments were inserted in pBBR1MCS-5-TgdhM-MCS-TGOX0028: DNA fragment with rhaSR-PrhaSR-PrhaBAD-RBS amplified with the primer pair PF1/PF2 from the genome of E. coli LJ110 and DNA fragment with mNG-TBBa_B1002 amplified with the primer pair PF3/PF4 from pBBR1MCS-5-araC-PBAD-mNG (Fricke et al., 2020). The latter DNA fragment included the terminator BBa_B1002 from the iGEM parts library directly downstream from the reporter gene mNG.
The plasmid pBBR1MCS-5-rhaS-PrhaSR-PrhaBAD-mNG lacking rhaR was constructed with a DNA fragment amplified with the primer pair PF5/PF4 from pBBR1MCS-5-rhaSR-PrhaSR-PrhaBAD-mNG resulting in fragment rhaS-PrhaSR-PrhaBAD-RBS-mNG-TBBa_B1002 and subsequent integration of this fragment into pBBR1MCS-5-TgdhM-MCS-TGOX0028.
The plasmid pBBR1MCS-5-rhaR-PrhaSR-PrhaBAD-mNG lacking rhaS was constructed with a DNA fragment containing rhaR and a DNA fragment containing PrhaSR-PrhaBAD-RBS-mNG-TBBa_B1002 amplified with the primer pairs PF1/PF6 and PF7/PF4 from template pBBR1MCS-5-rhaSR-PrhaSR-PrhaBAD-mNG. Due to the design of the primers, rhaS, being the first gene of the rhaSR operon, was deleted in such a way that the first and last three codons of rhaS remained in frame in the plasmid, thereby in principle maintaining the original operon structure.
The plasmid pBBR1MCS-5-PrhaSR-PrhaBAD-mNG lacking the whole rhaSR operon was constructed with a DNA fragment comprising PrhaSR-PrhaBAD-RBS-mNG-TBBa_B1002 generated with the primer pair PF8/PF4 from pBBR1MCS-5-rhaSR-PrhaSR-PrhaBAD-mNG by inserting it into pBBR1MCS-5-TgdhM-MCS-TGOX0028.
The plasmid pBBR1MCS-5-rhaS-PGOX0264-PrhaBAD-mNG was constructed using three DNA fragments: The first fragment contained rhaS and was amplified with the primer pair PF5/PF9 from pBBR1MCS-5-rhaS-PrhaSR-PrhaBAD-mNG. The second fragment contained RBS-PGOX0264 and was amplified with primer pair PF10/PF11 from the genome of G. oxydans 621H. The third fragment contained PrhaBAD-RBS-mNG-TBBa_B1002 and was amplified with primer pair PF12/PF4 from pBBR1MCS-5-rhaS-PrhaSR-PrhaBAD-mNG.
Similarly, plasmid pBBR1MCS-5-rhaS-PGOX0452-PrhaBAD-mNG was constructed from three DNA fragments: Again, the first fragment contained rhaS and was amplified with the primer pair PF5/PF9 from pBBR1MCS-5-rhaS-PrhaSR-PrhaBAD-mNG. The second fragment contained RBS-PGOX0452 and was amplified with the primer pair PF13/PF14 from the genome of G. oxydans 621H. The third fragment contained PrhaBAD-RBS-mNG-TBBa_B1002 and was amplified with the primer pair PF15/PF4 from pBBR1MCS-5-rhaS-PrhaSR-PrhaBAD-mNG.
The plasmid pBBR1MCS-5-mNG-PrhaSR-PGOX0264-rhaS was created with the DNA fragment TBBa_B1002-mNG-RBS-PrhaBAD amplified with the primer pair PF16/PF17 from pBBR1MCS-5-rhaS-PrhaSR-PrhaBAD-mNG and with fragment PGOX0264-RBS-rhaS amplified with the primer pair PF18/PF19 from pBBR1MCS-5-rhaS-PGOX0264-PrhaBAD-mNG.
Similarly, plasmid pBBR1MCS-5-mNG-PrhaSR-PGOX0452-rhaS was generated from DNA fragment TBBa_B1002-mNG-RBS-PrhaBAD amplified with the primer pair PF16/PF20 from template pBBR1MCS-5-rhaS-PrhaSR-PrhaBAD-mNG and from DNA fragment PGOX0452-RBS-rhaS amplified with the primer pair PF21/PF19 from template pBBR1MCS-5-rhaS-PGOX0452-PrhaBAD-mNG.
The plasmid pBBR1MCS-5-mNG-PrhaSR was constructed with DNA fragment TBBa_B1002-mNG amplified with the primer pair PF16/PF22 and DNA fragment RBS-PrhaSR-PrhaBAD amplified with the primer pair PF23/PF24, both fragments generated from plasmid pBBR1MCS-5-rhaS-PrhaSR-PrhaBAD-mNG as PCR template. In the resulting construct pBBR1MCS-5-mNG-PrhaSR, the PrhaBAD region next to PrhaSR was included to retain the native PrhaSR upstream region.
For construction of plasmid pBBR1MCS-5-rhaS-PrhaSR-PrhaBAD(+RhaS-BS)-mNG containing an additional RhaS binding site (+RhaS-BS) directly downstream from the −10 region of PrhaBAD, a DNA fragment consisting of (+RhaS-BS)-RBS-mNG-TBBa_B1002 was amplified with the primer pair PF25/PF4 from pBBR1MCS-5-rhaS-PrhaSR-PrhaBAD-mNG and integrated into the EcoRI-restricted plasmid pBBR1MCS-5-rhaS-PrhaSR-PrhaBAD-mNG. The additional RhaS binding site was introduced by primer PF25.
The plasmid pBBR1MCS-5-PrhaSR-rhaS was constructed from plasmid pBBR1MCS-5-rhaS-PrhaSR-PrhaBAD-mNG by EcoRI digestion and religation. Two EcoRI sites were located perfectly to remove mNG without the need of further cloning steps.
The plasmid pBBR1MCS-5-PrhaT-mNG lacking rhaS was constructed with the DNA fragments PrhaT-RBS generated with the primer pair PF26/PF27 from the genome of E. coli LJ110 and the fragment mNG-TBBa_B1002 generated with the primer pair PF28/PF4 from plasmid pBBR1MCS-5-rhaS-PrhaSR-PrhaBAD-mNG.
The plasmid pBBR1MCS-5-rhaS-PrhaSR-PrhaT-mNG was created with two DNA fragments. The first DNA fragment contained rhaS-PrhaSR amplified with the primer pair PF5/PF29 from pBBR1MCS-5-rhaS-PrhaSR-PrhaBAD-mNG. The second DNA fragment contained PrhaT-RBS-mNG-TBBa_B1002 amplified with the primer pair PF30/PF4 from pBBR1MCS-5-PrhaT-mNG.
The plasmid pBBR1MCS-5-rhaS-PGOX0264-PrhaT-mNG was constructed using two fragments. The fragment containing rhaS-PGOX0264 was amplified from template pBBR1MCS-5-rhaS-PGOX0264-PrhaBAD-mNG with the primer pair PF5/PF34. The fragment containing PrhaT-mNG was amplified from template pBBR1MCS-5-rhaS-PrhaSR-PrhaT-mNG with the primer pair PF4/PF37.
The plasmid pBBR1MCS-5-rhaS-PGOX0452-PrhaT-mNG was constructed using two fragments. The fragment containing rhaS-PGOX0452 was amplified from template pBBR1MCS-5-rhaS-PGOX0452-PrhaBAD-mNG with the primer pair PF5/PF38. The fragment containing PrhaT-mNG was amplified from template pBBR1MCS-5-rhaS-PrhaSR-PrhaT-mNG with the primer pair PF4/PF39.
The plasmid pBBR1MCS-5-rhaS-PGOX0264-PrhaT(−10-RhaS-BS)-mNG was constructed using two fragments. The fragment containing rhaS-PGOX0264 and the 5′ part of PrhaT was amplified from template pBBR1MCS-5-rhaS-PGOX0264-PrhaT-mNG with the primer pair MM14/PF5. The fragment containing the remaining 3′ part of PrhaT followed by mNG was amplified from template pBBR1MCS-5-rhaS-PGOX0264-PrhaT-mNG with the primer pair MM13/PF4. The additional RhaS binding site directly downstream from the −10 region of PrhaT was created and introduced by the primers MM13 and MM14.
The plasmid pBBR1MCS-5-rhaS-PGOX0452-PrhaT(−10-RhaS-BS)-mNG was constructed using two fragments. The fragment containing rhaS-PGOX0452 and the 5′ part of PrhaT was amplified from template pBBR1MCS-5-rhaS-PGOX0452-PrhaT-mNG with the primer pair MM14/PF5. The fragment containing the remaining 3′ part of PrhaT followed by mNG was amplified from template pBBR1MCS-5-rhaS-PGOX0452-PrhaT-mNG with the primer pair MM13/PF4. The additional RhaS binding site directly downstream from the −10 region of PrhaT was created and introduced by the primers MM13 and MM14.
The plasmid pBBR1MCS-5-rhaS-PGOX0264-PrhaT(TSS-RhaS-BS)-mNG was constructed using two fragments. The fragment containing rhaS-PGOX0264 and the 5′ part of PrhaT was amplified from template pBBR1MCS-5-rhaS-PGOX0264-PrhaT-mNG with the primer pair MM16/PF5. The fragment containing the remaining 3′ part of PrhaT followed by mNG was amplified from template pBBR1MCS-5-rhaS-PGOX0264-PrhaT-mNG with the primer pair MM15/PF4. The additional RhaS binding site directly downstream from the E. coli transcriptional start of PrhaT was created and introduced by the primers MM15 and MM16.
The plasmid pBBR1MCS-5-rhaS-PGOX0452-PrhaT(TSS-RhaS-BS)-mNG was constructed using two fragments. The fragment containing rhaS-PGOX0452 and the 5′ part of PrhaT was amplified from template pBBR1MCS-5-rhaS-PGOX0452-PrhaT-mNG with the primer pair MM16/PF5. The fragment containing the remaining 3′ part of PrhaT followed by mNG was amplified from template pBBR1MCS-5-rhaS-PGOX0452-PrhaT-mNG with the primer pair MM15/PF4. The additional RhaS binding site directly downstream from the E. coli transcriptional start of PrhaT was created and introduced by the primers MM15 and MM16.
The plasmid pKOS6b-igr3::mNG for genomic labelling of G. oxydans 621H by mNG under control of PrhaBAD(+RhaS-BS) in igr3 was constructed with three fragments. The upstream and downstream flanking regions of igr3 were amplified from genomic DNA of G. oxydans 621H with the primer pairs PF31/PF32 and PF33/PF34, respectively. The fragment containing PrhaBAD(+RhaS-BS)-mNG was amplified from plasmid pBBR1MCS-5-rhaS-PrhaSR-PrhaBAD(+RhaS-BS)-mNG with the primer pair PF35/PF36.
The plasmid pKOS6b-igr2::PGOX0264-rhaS for genomic integration of a PGOX0264-rhaS copy into igr2 of G. oxydans mNG was constructed with three fragments. The upstream and downstream flanking regions of igr2 were amplified from genomic DNA of G. oxydans 621H with the primer pairs PF40/PF41 and PF42/PF43, respectively. The fragment containing PGOX0264-rhaS was amplified from plasmid pBBR1MCS-5-rhaS-PGOX0264-PrhaBAD-mNG with the primer pair PF44/PF45.
The plasmid pKOS6b-igr2::PrhaSR-rhaS for genomic integration of a PrhaSR-rhaS copy into igr2 of G. oxydans mNG was constructed with three fragments. The upstream and downstream flanking regions of igr2 were amplified from genomic DNA of G. oxydans 621H with the primer pairs PF40/PF46 and PF42/PF43, respectively. The fragment containing PrhaSR-rhaS was amplified from plasmid pBBR1MCS-5-rhaS-PrhaSR-PrhaBAD-mNG with the primer pair PF44/PF47.
The plasmid pKOS6b-igr1::PGOX0264-rhaS for genomic integration of a PGOX0264-rhaS copy into igr1 of G. oxydans mNG igr2::PGOX0264-rhaS was constructed with three fragments. The upstream and downstream flanking regions of igr1 were amplified from genomic DNA of G. oxydans 621H with the primer pairs MH3/MH10 and MH6/MH9, respectively. The fragment containing PGOX0264-rhaS was amplified from plasmid pBBR1MCS-5-rhaS-PGOX0264-PrhaBAD-mNG with the primer pair MH4/MH5.
The plasmid pKOS6b-igr1::PrhaSR-rhaS for genomic integration of a PrhaSR-rhaS copy into igr1 of G. oxydans mNG igr2::PGOX0264-rhaS was constructed with three fragments. The upstream and downstream flanking regions of igr1 were amplified from genomic DNA of G. oxydans 621H with the primer pairs MH7/MH10 and MH6/MH9, respectively. The fragment containing PrhaSR-rhaS was amplified from plasmid pBBR1MCS-5-rhaS-PrhaSR-PrhaBAD-mNG with the primer pair MH5/MH8.
Construction and selection of genomically modified Gluconobacter oxydans strains
Integrations of expression cassettes into the genome of G. oxydans 621H and selection of excised plasmid backbones were carried out using pKOS6b plasmid derivatives and counterselection by cytosine deaminase, encoded by codA from E. coli, in the presence of the fluorinated pyrimidine analogue 5-fluorocytosine (FC). The cytosine deaminase converts nontoxic FC to toxic 5-fluorouracil, which is channeled into the metabolism by the uracil phosphoribosyltransferase, encoded by the chromosomal upp gene of Gluconobacter. The details of the method are described elsewhere (Kostner et al., 2013). According to this method, strain G. oxydans mNG was constructed and selected from G. oxydans 621H using the plasmid pKOS6b-igr3::mNG. The G. oxydans strains mNG igr2::PGOX0264-rhaS and mNG igr2::PrhaSR-rhaS were constructed and selected from G. oxydans mNG using the plasmids pKOS6b-igr2::PGOX0264-rhaS and pKOS6b-igr2::PrhaSR-rhaS, respectively. The G. oxydans strains mNG igr1::PGOX0264-rhaS igr2::rhaS and mNG igr1::PrhaSR-rhaS igr2::rhaS were constructed and selected from G. oxydans mNG igr2::PGOX0264-rhaS using the plasmids pKOS6b-igr1::PGOX0264-rhaS and pKOS6b-igr1::PrhaSR-rhaS, respectively.
Measurements of fluorescence protein
The regulation and relative strength of the promoters on constructed plasmids was monitored in G. oxydans by means of expressing mNG encoding the fluorescent reporter protein mNG (Shaner et al., 2013). For analysis of mNG expression with various promoters by mNG signals, G. oxydans cultures were supplemented with L-rhamnose at the indicated concentrations (w/v) using a 40% (w/v) stock solution. Equal volumes of medium were added to non-supplemented reference cultures. Throughout the cultivation, growth (OD600) and fluorescence emission were monitored in intervals using a spectrophotometer (UV-1800, Shimadzu) and an Infinite M1000 PRO Tecan reader (λex 504 nm/λem 517 nm, ex/em bandwidth 5 nm, infinite M1000 PRO Tecan). For microscale BioLector cultivations, overnight starter cultures were used to inoculate 800 μl batches of D-mannitol medium in 48-well Flowerplates® (m2p-labs) to an initial OD600 of 0.3. Sealed with disposable foil (m2p-labs), plates were cultivated for 24 h at 1,200 rpm, 85% humidity and 30°C. Growth was monitored in each well as backscattered light at 620 nm (A620 nm) and protein fluorescence was monitored as emission (λex 510 nm/λem 532 nm). For backscatter signal amplification, gain 20 was applied. Signal amplification of fluorescence emission varied (gain 40–70) and is indicated in the figure legends. All BioLector data shown in a diagram were measured in the same run of a growth experiment.
Cell flow cytometer analysis
For single cell analysis, a FACSAria™ cell sorter controlled by FACSDiva 8.0.3 software (BD Biosciences) was used to analyze the mNG reporter protein signals in G. oxydans 621H harboring either plasmid pBBR1MCS-5-rhaS-PrhaSR-PrhaBAD-mNG or pBBR1MCS-5-rhaS-PrhaSR-PrhaT-mNG. The FACS was operated with a 70 μm nozzle and run with a sheath pressure of 70 psi. The forward scatter (FSC) and side scatter (SSC) were recorded as small-angle scatter and orthogonal scatter, respectively, by means of a 488 nm solid blue laser beam. For analysis, only particles/events above 200 a.u. for FSC-H and above 300 a.u. for SSC-H as the thresholds were considered. The mNG fluorescence emission was detected from the SSC through the combination of a 502 nm long-pass and 530/30 nm band-pass filter. Prior to data acquisition, the FSC-A vs. SSC-A plot was employed to gate the population and to exclude signals originating from cell debris or electronic noise. In a second and third gating step, from the resulting population, the SSC-H signal was plotted against the SSC-W signal and this population was subsequently gated in a FSC-H vs. FSC-W plot to exclude doublets. From this resulting singlet population, 100,000 events were recorded at a rate of <10,000 events/s for fluorescence data acquisition. For data analysis and visualization of all gated events (n = 100,000) FlowJo 10.7.2 for Windows (FlowJo, LLC) was applied.
L-Rhamnose biotransformation test assay and GC-TOF-MS analysis
G. oxydans cells were grown to an OD600 of 1.3, centrifuged (4,000 × g, 5 min) and washed twice with 50 mM phosphate buffer (pH 6). After the second washing step, cells were resuspended in biotransformation buffer (6.6 g L−1 Na2HPO4, 3 g L−1 KH2PO4, 1 g L−1 NH4Cl, 0.5 g L−1 NaCl, 0.49 g L−1 MgSO4, 0.02 g L−1 CaCl2) supplemented with 2% (w/v) L-rhamnose and incubated for 24 h at 30°C and 200 rpm. Then, the cells were removed from the buffer (4,000 × g, 5 min) and the supernatant was used for analysis by gas chromatography (Agilent 6,890 N, Agilent Technologies) coupled to a Waters Micromass GCT Premier high-resolution time-of-flight mass spectrometer (Waters). Sample handling for derivatization, GC-TOF-MS operation, and peak identification were carried out as described (Paczia et al., 2012). As a control, samples from biotransformation buffer with L-rhamnose and without cells as well as biotransformation buffer without L-rhamnose yet with cells were prepared.
Total DNA extraction, library preparation, illumina sequencing, and data analysis
Total DNA was purified from a culture aliquot using a NucleoSpin Microbial DNA Mini kit (MACHEREY–NAGEL). DNA concentrations were measured using a Qubit 2.0 fluorometer (Thermo Fisher Scientific). Illumina sequencing and data analysis of the indicated PrhaBAD DNA sample was carried out as described (Fricke et al., 2021b). For the read mapping, the improved genome sequence from G. oxydans 621H and the indicated PrhaBAD plasmid sequence were used (Kranz et al., 2017).
Determination of transcriptional starts
G. oxydans cells carrying plasmid pBBR1MCS-5-rhaS-PrhaSR-PrhaBAD(+RhaS-BS)-mNG were cultivated in shake flasks with 50 ml complex D-mannitol medium. Cells were harvested at OD600 of 1.5 in the mid-exponential phase and total RNA was extracted as described (Kranz et al., 2018). The RNA sample was sent to the company Vertis Biotechnology AG (Germany) for further sample processing and data generation. For Cappable-seq RNA, the RNA sample was enriched by capping of the 5′ triphosphorylated RNA with 3′-desthiobiotin-TEG-guanosine 5′ triphosphate (DTBGTP; NEB) using the vaccinia capping enzyme (VCE; NEB) for reversible binding of biotinylated RNA species to streptavidin. Then, streptavidin beads were used to capture biotinylated RNA species followed by elution to obtain highly enriched 5′ fragment of the primary transcripts. The Cappable enriched RNA sample was poly(A)-tailed using poly(A) polymerase. In order to remove residual 5’-P-ends, the RNA was treated with Antarctic Phosphatase (NEB). Then, the 5’-PPP cap structures were converted to 5’-P using the RppH enzyme (NEB). Afterwards, an RNA adapter was ligated to the newly formed 5′-monophosphate structures. First-strand cDNA synthesis was performed using an oligo(dT)-adapter primer and the MMLV reverse transcriptase. The resulting cDNA was PCR-amplified to about 10–20 ng/μl using a high fidelity DNA polymerase. For Illumina sequencing, 100–300 bp long 5′ fragments were isolated from the full-length cDNA. For this purpose the cDNA preparation was fragmented and the 5’-cDNA fragments were then bound to streptavidin magnetic beads. The bound cDNAs were blunted and the 3’ Illumina sequencing adapter was ligated to the 3′ ends of the cDNA fragments. The bead-bound cDNAs were finally PCR-amplified. The library was sequenced on an Illumina NextSeq 500 system using 75 bp read length. The fastq file output was used for data analysis with CLC Genomics Workbench (v21.0.3). Imported reads were trimmed and quality filtered. Passed reads were used for strand-specific mapping to the G. oxydans genome and the pBBR1MCS-5-rhaS-PrhaSR-PrhaBAD(+RhaS-BS)-mNG plasmid sequence using the RNA-seq analysis tool implemented in the CLC software. Read mapping settings used were 80% length fraction and 80% similarity fraction. The starts of mapped reads and total nucleotide coverage according to the mappings were used to assess transcriptional starts on the expression plasmid with the promoters PrhaSR and PrhaBAD(+RhaS-BS).
Results
L-Rhamnose does not affect growth and is not oxidized by Gluconobacter oxydans 621H
The RhaSR-PrhaBAD system from E. coli responds to the monosaccharide rhamnose in the uncommon L conformation, which is similar to the AraC-ParaBAD system and its effector L-arabinose. Like L-arabinose, the inducer L-rhamnose needs to enter the cell to interact with its targeted regulators RhaR and RhaS (Tobin and Schleif, 1987). In contrast to L-arabinose, which is readily oxidized by Gluconobacter already in the periplasm (Peters et al., 2013; Fricke et al., 2022), for more than 90% of the strains of the genus Gluconobacter no acid formation from L-rhamnose has been reported (Kersters et al., 1990). G. oxydans 621H whole-cell enzyme activity assays using the artificial electron acceptor DCPIP also revealed no detectable activity with L-rhamnose as substrate (Peters et al., 2013). To exclude a hitherto unrecognized consumption or oxidation of the inducer L-rhamnose by G. oxydans 621H, we carried out biotransformation assays followed by GC-TOF-MS analysis, and a growth experiment.
The results confirmed that G. oxydans does not consume or oxidize L-rhamnose. In the GC-TOF-MS analysis, no new peaks were detected in 24 h samples, and the areas of the GC-TOF peaks assigned to L-rhamnose were very similar for the samples at 0 h and after 24 h (Supplementary Table S2; Supplementary Figure S2). Hence, if at all, L-rhamnose is degraded or converted by strain 621H so slowly that this effector is hardly diminished during potential applications. To check if L-rhamnose somehow affects the growth of G. oxydans 621H, we added L-rhamnose to the complex medium. With 1% (w/v) L-rhamnose instead of D-mannitol, there was no growth of G. oxydans 621H and the initial start OD600 of 0.04 did not change within 24 h (Supplementary Figure S3). In 4% (w/v) D-mannitol medium supplemented with 1% (w/v) L-rhamnose, the strain 621H grew very similar and without a significant difference compared to the growth in the D-mannitol complex medium without L-rhamnose supplement. Furthermore, with and without L-rhamnose the initial pH 6 of the growth medium was acidified to pH 4.3 after 24 h, suggesting no relevant oxidation of L-rhamnose to a corresponding acid. Therefore, there was no negative or supportive effect of L-rhamnose on the growth of G. oxydans 621H up to 1% (w/v).
In Gluconobacter oxydans, PrhaBAD from Escherichia coli is repressed in the presence of L-rhamnose
First, we tested the inducibility of PrhaBAD in G. oxydans by constructing a pBBR1MCS-5-based plasmid placing all the genetic elements in the same order as in E. coli. The rhaSR operon was under the control of its native promoter PrhaSR in divergent orientation to PrhaBAD. The fluorescent reporter mNeonGreen (mNG) was used to measure the PrhaBAD-controlled expression by placing the mNG gene downstream from PrhaBAD. On the plasmid, the elements rhaSR-PrhaSR-PrhaBAD-mNG were flanked by three terminators, TgdhM downstream from rhaR, and TBBa_B1002 and TGOX0028 downstream from mNG (Figure 1A). Furthermore, downstream from the native ribosome binding site (RBS) present in PrhaBAD the RBS 5′-AGGAGA was inserted upstream from mNG. This RBS appeared strong in G. oxydans and was also used in the regulatable AraC-ParaBAD and TetR-Ptet expression systems (Fricke et al., 2020, 2021b). The inducibility of the resulting plasmid pBBR1MCS-5-rhaSR-PrhaSR-PrhaBAD-mNG was tested in G. oxydans 621H with 1% (w/v) L-rhamnose. Overnight pre-cultures were split to inoculate main cultures in D-mannitol medium with and without L-rhamnose. Growth and mNG fluorescence was monitored in a BioLector.
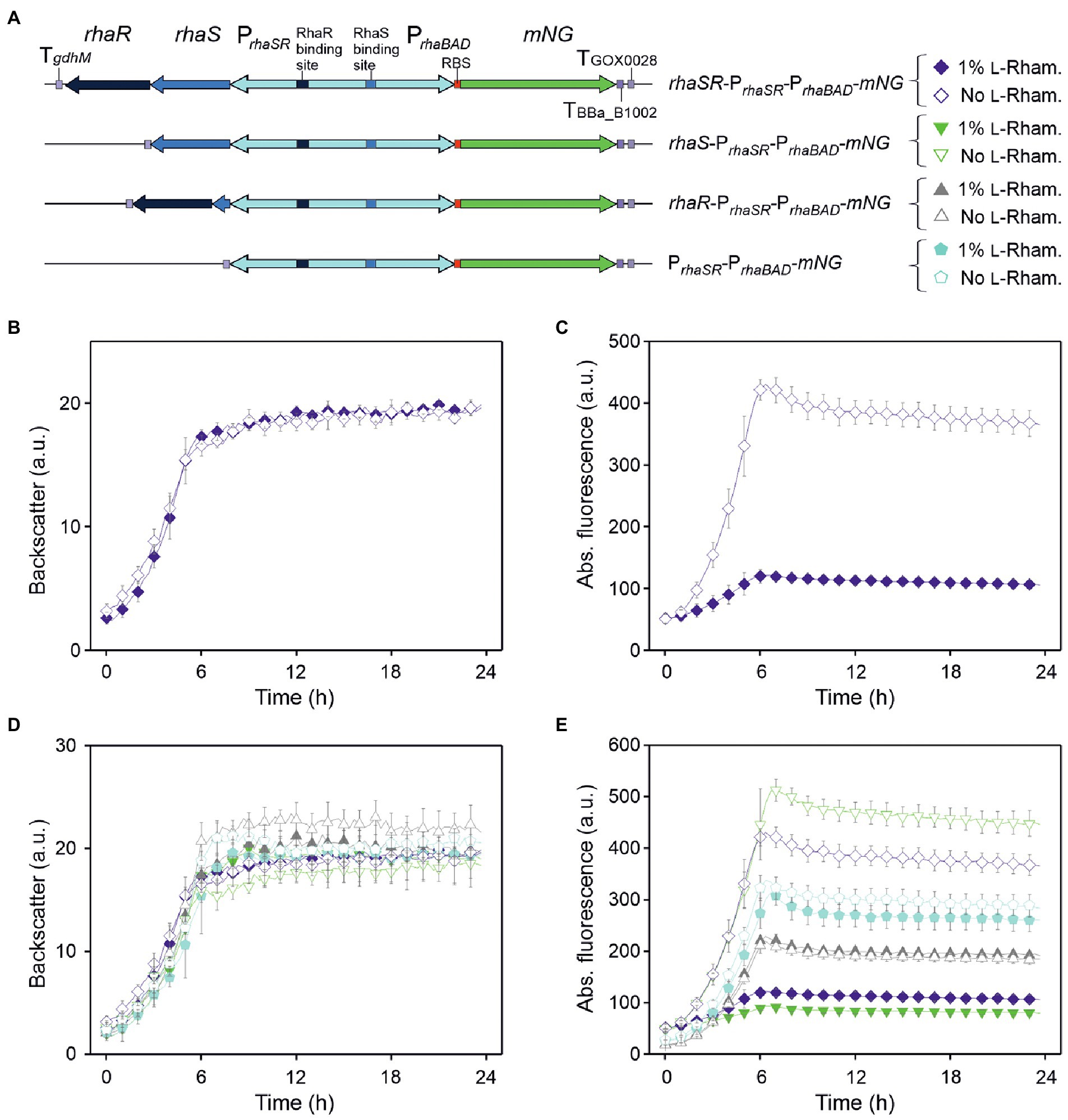
Figure 1. pBBR1MCS-5-based expression plasmids and analysis of the regulation of the RhaSR-PrhaBAD system from Escherichia coli in G. oxydans 621H. (A) Schematic illustration of the plasmid variants with reporter gene mNG used to test L-rhamnose-dependent regulation of PrhaBAD-derived expression in the presence and absence of RhaS and RhaR. TgdhM: terminator sequence of gdhM (GOX0265); TGOX0028: terminator sequence of GOX0028. The RBS 5′-AGGAGA was inserted in the 3′ region of PrhaBAD upstream from mNG. (B) Growth according to backscatter and (C) absolute mNG fluorescence in G. oxydans carrying plasmid pBBR1MCS-5-rhaSR-PrhaSR-PrhaBAD-mNG grown in D-mannitol medium without and with 1% (w/v) L-rhamnose in BioLector microscale. (D) Growth according to backscatter and (E) absolute mNG fluorescence of G. oxydans carrying either plasmid pBBR1MCS-5-rhaSR-PrhaSR-PrhaBAD-mNG, or a plasmid lacking either rhaR, or rhaS, or both rhaSR. Cells were grown in microscale (BioLector) in D-mannitol medium without and with 1% (w/v) L-rhamnose. All data represent mean values and standard deviation from two biological replicates (clones) with three technical replicates each. BioLector settings: backscatter gain 20, fluorescence gain 50.
As expected from the previous growth tests in shake flasks, all BioLector microscale cultures exhibited very similar growth regardless of L-rhamnose supplementation (Figure 1B). However, surprisingly and contrary to our expectation, the mNG fluorescence of the cultures without L-rhamnose strongly increased during growth and peaked ~6 h after inoculation when cells entered the stationary phase, while in cultures with L-rhamnose a much lower level of mNG fluorescence (~28%) was observed (Figure 1C). Thus, mNG expression from PrhaBAD appeared to be strongly repressed in the presence of L-rhamnose, suggesting that in G. oxydans the responsiveness of the RhaSR-PrhaBAD system is inverted compared to E. coli. Furthermore, according to the absolute mNG fluorescence in the absence of L-rhamnose, the promoter PrhaBAD appeared to be very strong in G. oxydans compared to ParaBAD and Ptet (Fricke et al., 2020, 2021b).
To test whether the rhaSR-PrhaBAD-mNG expression plasmid shows L-rhamnose-inducibility in E. coli, the plasmid-carrying E. coli S17-1 used for transformation of G. oxydans was tested. As expected, in LB medium supplemented with 1% (w/v) L-rhamnose, the mNG fluorescence was ~2,200-fold higher compared to the mNG fluorescence in cultures without L-rhamnose (data not shown). To verify that the reversed responsiveness of RhaSR-PrhaBAD indeed was observed in G. oxydans 621H carrying the intended plasmid without mutations possibly acquired later during growth, G. oxydans cells of an induced culture were harvested at the end of the cultivation (24 h) for isolation of total DNA and Illumina sequencing. The read data analysis excluded unexpected contamination of the culture, since 99.48% of 1,402,738 trimmed and quality-filtered reads mapped to the updated reference sequences of the G. oxydans 621H genome (88-fold coverage), the 5 endogenous plasmids, and the mNG expression plasmid with rhaSR-PrhaSR-PrhaBAD-mNG (1,011-fold coverage; Kranz et al., 2017). Besides, the sequencing results corroborated three DNA point mutations in rhaSR already observed before by Sanger sequencing when checking the insert of the plasmid after cloning in E. coli. In rhaR there was the silent mutation of CGC to CGT (Arg56). In rhaS there was the silent mutation of CTG to CTT (Leu166) and the mutation of GGG to TGG resulting in the exchange Gly136Trp in RhaS. All three mutations were present already on the plasmid when it was cloned in E. coli. To exclude an effect of these mutations on the reversed responsiveness only in G. oxydans, the plasmid was cloned again using a new rhaS DNA template from E. coli MG1655. This plasmid lacked the two point mutations in rhaS and also showed the reversed responsiveness in G. oxydans 621H with the same extent of repression (data not shown). Thus, the DNA point mutations in rhaS did not affect the regulatory properties of the system in G. oxydans. In summary, these results showed that in contrast to E. coli the PrhaBAD promoter is repressed in G. oxydans in the presence of L-rhamnose.
RhaS is responsible for L-rhamnose-dependent repression of PrhaBAD in Gluconobacter oxydans
To analyze whether RhaS and/or RhaR, or an interfering endogenous G. oxydans protein is responsible for the reversed responsiveness of the RhaSR-PrhaBAD system, we constructed derivatives of the expression plasmid either lacking in-frame a substantial part of rhaS, or lacking rhaR, or lacking both genes, yet keeping all the elements upstream and downstream from rhaS and rhaR (Figure 1A). G. oxydans clones carrying one of these plasmid derivatives were grown in D-mannitol medium without and with 1% (w/v) L-rhamnose and cultivated in a BioLector to monitor growth and mNG fluorescence. Regardless of the plasmid used, all G. oxydans cultures exhibited very similar growth with and without L-rhamnose (Figure 1D). The differences in mNG fluorescence with and without L-rhamnose clearly indicated that RhaS alone is either directly or indirectly responsible for the regulation of PrhaBAD. All clones with the plasmid lacking only rhaS exhibited a moderate maximal mNG fluorescence after ~6 h (220–228 a.u.), regardless of L-rhamnose supplementation (Figure 1E). The clones with the plasmid lacking both rhaS and rhaR also showed no response of the mNG fluorescence to L-rhamnose, yet the maximal mNG fluorescence was 50% higher compared to the plasmid still containing rhaR. Without rhaSR, the mNG signals of all clones peaked at 6 h and reached a higher intensity (314–338 a.u.), suggesting a general negative effect of RhaR on the PrhaBAD activity regardless of the presence or absence of L-rhamnose. This is in line with the observation that with the plasmid lacking only rhaR, expression from PrhaBAD increased in the absence of L-rhamnose by ~20% (513 a.u.) compared to the plasmid with both regulator genes (431 a.u.). Furthermore, with 1% (w/v) L-rhamnose the mNG expression from PrhaBAD was more reduced with the rhaS-PrhaBAD construct (94 a.u.) than with the rhaSR-PrhaBAD construct (122 a.u.).
In summary, these data indicated that RhaS activates the PrhaBAD promoter in the absence of L-rhamnose and represses PrhaBAD in the presence of L-rhamnose, and thus is exerting a dual role in G. oxydans (Figure 1E).
In the absence of L-rhamnose PrhaBAD activity is stimulated by RhaS
The clear differences in the mNG fluorescence observed with the previous plasmid derivatives with or without rhaS suggested that RhaS activates PrhaBAD in the absence of L-rhamnose in G. oxydans. If so, the apparent strength of PrhaBAD in the absence of L-rhamnose could partially be tuned by the strength of rhaS expression. To test this and the resulting down-regulation of PrhaBAD-derived mNG expression in the presence of L-rhamnose starting then from different initial expression levels, we constructed derivatives of pBBR1MCS-5-rhaS-PrhaSR-PrhaBAD-mNG expressing rhaS constitutively either from the G. oxydans promoter PGOX0264 or PGOX0452 (Figure 2A). PGOX0264 and PGOX0452 have been shown to be strong and moderate promoters in G. oxydans, respectively (Kallnik et al., 2010). With the resulting plasmids pBBR1MCS-5-rhaS-PGOX0264-PrhaBAD-mNG and pBBR1MCS-5-rhaS-PGOX0452-PrhaBAD-mNG, the mNG expression was compared to that with pBBR1MCS-5-rhaS-PrhaSR-PrhaBAD-mNG in microscale BioLector cultivations (Figure 2B). Without L-rhamnose, constitutive expression of rhaS from PGOX0264 reduced PrhaBAD-derived mNG expression by more than half and from this latter level the mNG expression was reduced by half when expressing rhaS from PGOX0452 (Figure 2C). Thus, expression of rhaS from its native promoter PrhaSR led to the highest PrhaBAD-derived mNG signals (514 a.u. after ~7 h) in the absence of L-rhamnose. These results suggested that PrhaSR is a very strong promoter per se, or because it is positively auto-regulated by RhaS. However, the RhaS protein was reported to severely aggregate when overexpressed (Wickstrum et al., 2010), and biochemical analysis of RhaS binding to the promoter DNA had not been possible due to the extreme insolubility of the overproduced RhaS protein (Egan and Schleif, 1994). Therefore, it appears more likely that PrhaSR is a weak promoter also in G. oxydans resulting in sufficient levels of functional RhaS protein activating PrhaBAD, while stronger rhaS expression via PGOX0264 and PGOX0452 likely resulted in aggregated non-functional RhaS protein.
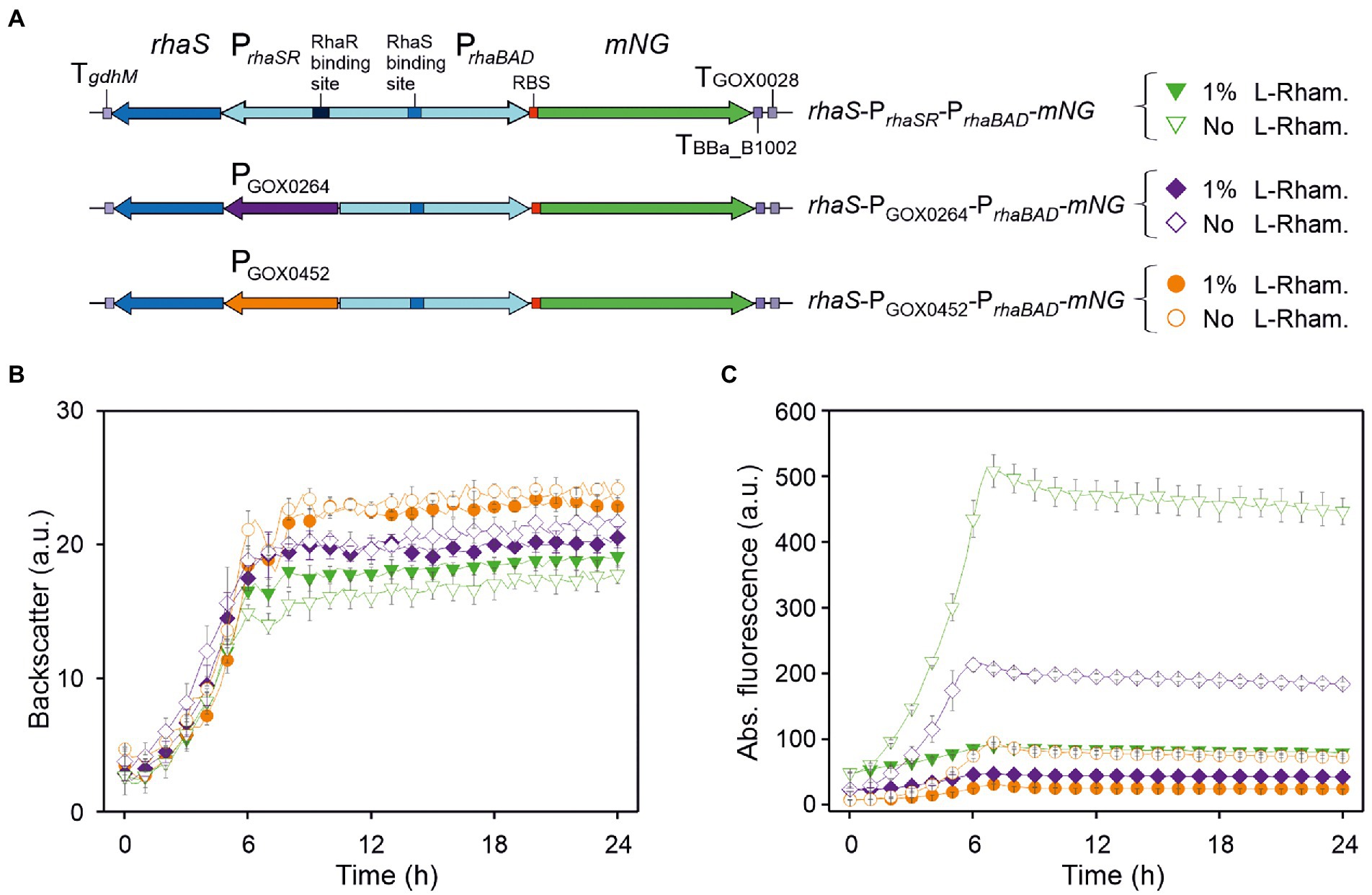
Figure 2. Performance of PrhaBAD-derived mNG expression in dependence of rhaS expression and presence of L-rhamnose. (A) Schematic illustration of the plasmid variants for constitutive expression of rhaS from the strong and moderate G. oxydans promoters PGOX0264 and PGOX0452. TgdhM: terminator sequence of gdhM (GOX0265); TGOX0028: terminator sequence of GOX0028. RBS: 5′-AGGAGA inserted in the 3′ region of PrhaBAD upstream from mNG. (B) Growth in D-mannitol medium according to backscatter and (C) absolute mNG fluorescence of G. oxydans 621H carrying either plasmid pBBR1MCS-5-rhaS-PrhaSR-PrhaBAD-mNG, or pBBR1MCS-5-rhaS-PGOX0264-PrhaBAD-mNG, or pBBR1MCS-5-rhaS-PGOX0452-PrhaBAD-mNG. Repression of mNG expression from PrhaBAD was tested with 1% (w/v) L-rhamnose. Data represent mean values and standard deviation from two biological replicates (clones) with three technical replicates each. BioLector settings: backscatter gain 20, fluorescence gain 50.
In the presence of 1% (w/v) L-rhamnose, the strong mNG expression obtained with rhaS-PrhaSR-PrhaBAD-mNG was reduced by ~82% (from 514 to 90 a.u.). The mNG expression obtained with rhaS-PGOX0264-PrhaBAD-mNG and with rhaS-PGOX0452-PrhaBAD-mNG was reduced by 77% (from 212 to 48 a.u.) and by 68% (from 95 to 30 a.u.), respectively (Figure 2C).
PrhaSR is weak in Gluconobacter oxydans, stimulated by RhaS and further stimulated by L-rhamnose
To check the strength of PrhaSR in G. oxydans and the influence of RhaS on PrhaSR activity, we created plasmids with mNG under the control of PrhaSR and with rhaS under the control of the constitutive promoters PGOX0452 or PGOX0264, or lacking rhaS (Supplementary Figure S4A). The respective G. oxydans strains were cultivated in a BioLector and showed similar growth (Supplementary Figure S4B). In the absence of L-rhamnose, moderate PGOX0452-derived rhaS expression resulted in a similar low mNG expression from PrhaSR as without rhaS, while the stronger PGOX0264-derived rhaS expression resulted in a two-fold higher mNG expression from PrhaSR, suggesting a positive effect of the RhaS level on PrhaSR activity (Supplementary Figures S4C,D). In the presence of L-rhamnose, mNG expression from PrhaSR was always increased with rhaS, while there was no effect by L-rhamnose when rhaS was absent. With moderate rhaS expression in G. oxydans harboring pBBR1MCS -5-mNG-PrhaSR-PGOX0452-rhaS, the mNG fluorescence increased ~2.5-fold from 74 to 189 a.u. with 1% (w/v) L-rhamnose. This L-rhamnose-dependent increase was less pronounced with rhaS under control of the stronger PGOX0264 where the RhaS level was expected to be higher. Here, the mNG fluorescence increased only 1.3-fold from 144 to 187 a.u. (Supplementary Figure S4C). Together, PrhaSR is also stimulated by RhaS in the absence of L-rhamnose, yet in contrast to the repressed PrhaBAD promoter, PrhaSR is further activated by RhaS in the presence of L-rhamnose.
Repression of PrhaBAD is sensitive to low L-rhamnose levels and is homogeneous
Since from all tested plasmid variants the one lacking rhaR and containing rhaS under the control of its native auto-regulated PrhaSR promoter exhibited the highest PrhaBAD activity in the absence of L-rhamnose and the highest grade of repression in the presence of L-rhamnose, the construct pBBR1MCS-5-rhaS-PrhaSR-PrhaBAD-mNG was analyzed further. The sensitivity of repression and residual mNG expression was tested in D-mannitol medium with 0.3%, 1% and 3% (w/v) L-rhamnose in a BioLector (Figures 3A,B). Already 0.3% (w/v) L-rhamnose strongly reduced the mNG fluorescence after ~7 h by 75% (225 vs. 55 a.u.). This indicated that the RhaS-PrhaBAD system is quite sensitive and already low L-rhamnose concentrations should enable a tuning of target gene repression. Supplementation with 1% and 3% (w/v) L-rhamnose reduced mNG fluorescence by 83% (38 a.u.) and 85% (34 a.u.), respectively. This suggested that already 1% (w/v) L-rhamnose was sufficient to reach almost maximal possible repression of plasmid-based PrhaBAD copies in G. oxydans.
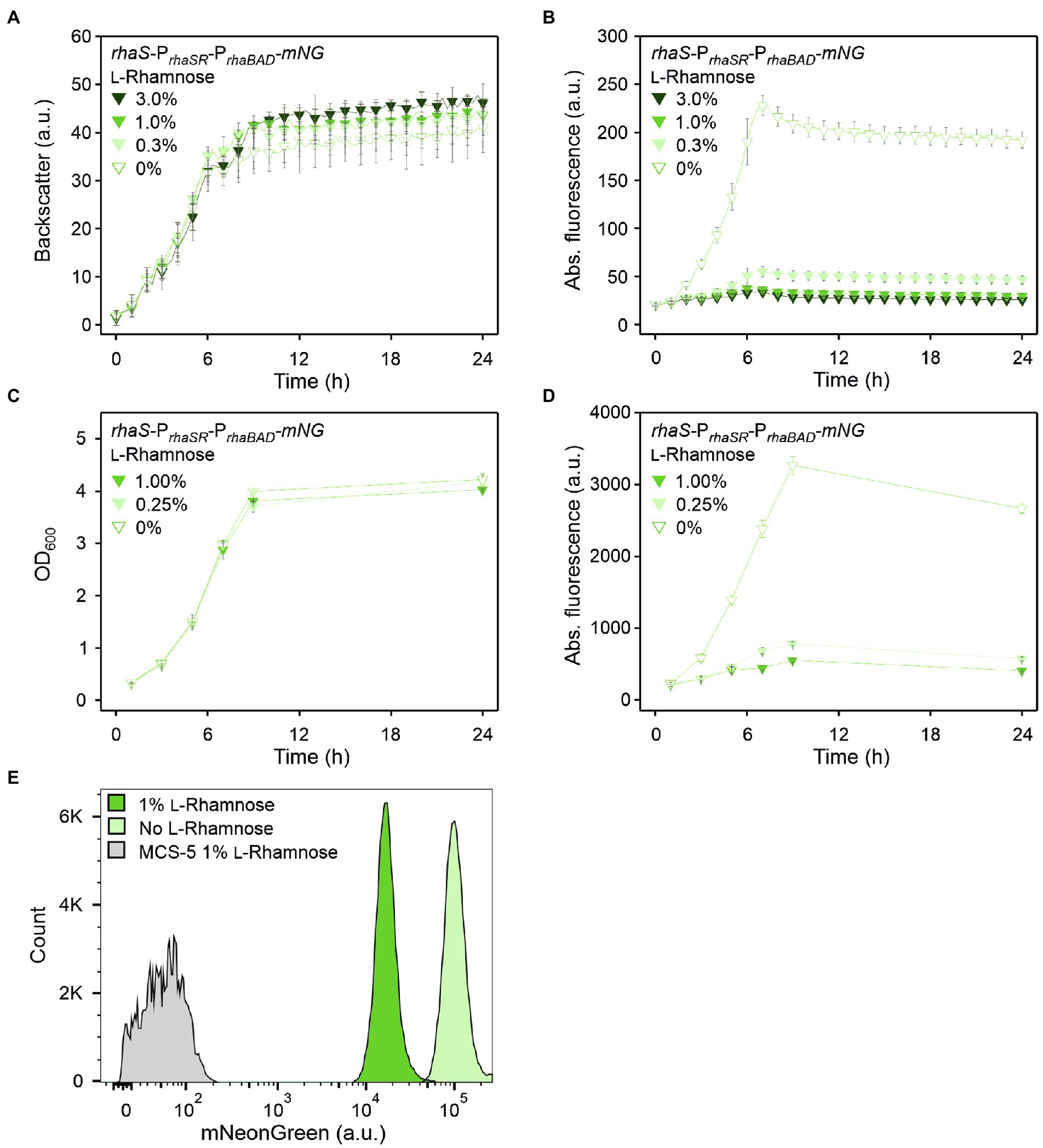
Figure 3. RhaS-dependent repression of PrhaBAD in G. oxydans in the presence of L-rhamnose. (A) Growth according to backscatter and (B) absolute mNG fluorescence in G. oxydans 621H with plasmid pBBR1MCS-5-rhaS-PrhaSR-PrhaBAD-mNG in microscale BioLector cultivations without or with L-rhamnose (w/v) as indicated. BioLector settings: backscatter gain 20, fluorescence gain 40. (C) Growth (OD600) and (D) absolute mNG fluorescence in G. oxydans 621H with plasmid pBBR1MCS-5-rhaS-PrhaSR-PrhaBAD-mNG in shake flask cultivations without and with L-rhamnose (w/v) as indicated. mNG fluorescence was measured in a Tecan reader (gain 60). Data represent mean values and standard deviation from two biological replicates (clones) with three technical replicates each. (E) FACS analysis of G. oxydans 621H with plasmid pBBR1MCS-5-rhaS-PrhaSR-PrhaBAD-mNG or empty vector pBBR1MCS-5 as a control (MCS-5). Cells were grown in shake flasks with D-mannitol medium without and with 1% (w/v) L-rhamnose. FACS analysis was performed 7 h after inoculation (induction). Total counts per sample represent 100,000 events.
This responsiveness of PrhaBAD-based expression toward relatively low L-rhamnose concentrations was also observed in shake flask cultivations. When grown in 50 ml D-mannitol medium supplemented with 0.25% L-rhamnose, the mNG fluorescence was reduced to 24% (from 3,267 to 783 a.u.) after 9 h (Figures 3C,D). In shake flask cultures with 1% (w/v) L-rhamnose, the mNG fluorescence was reduced to 17% (from 3,267 to 553 a.u.).
Flow cytometry was used to analyze the repression of PrhaBAD-derived mNG expression on the single cell level. In the absence of L-rhamnose, 7 h after inoculation 95.5% of the analyzed cells showed strong mNG fluorescence (~100,000 a.u.), while when grown with 1% (w/v) L-rhamnose, 96.4% of the analyzed cells showed a 89% reduced fluorescence (~11,000 a.u.; Figure 3E). Thus, the results of this FACS analysis are in line with the results of the BioLector and Tecan reader (shake flasks) measurements. Additionally, the FACS analysis demonstrated a high population homogeneity in both conditions.
An additional RhaS binding site directly downstream from the −10 region doubled the PrhaBAD-derived expression strength and the dynamic range of repression
In an attempt to reduce the residual expression from PrhaBAD in the presence of L-rhamnose and achieve complete repression, and to possibly lower the L-rhamnose concentrations required, we constructed and tested a plasmid with an additional RhaS binding site (+RhaS-BS) directly downstream from the annotated E. coli −10 region of PrhaBAD. Additional binding of the RhaS-L-rhamnose complex downstream from the −10 region should potentially contribute to the repression of PrhaBAD. Also, it was interesting to see the general impact of this additional RhaS BS on the PrhaBAD activity in the absence of L-rhamnose.
We used plasmid pBBR1MCS-5-rhaS-PrhaSR-PrhaBAD-mNG as template and created a copy of the 50 bp region comprising the native RhaS-BS present in PrhaBAD. This copy was inserted directly downstream from the −10 region of PrhaBAD. The resulting plasmid was termed pBBR1MCS-5-rhaS-PrhaSR-PrhaBAD(+RhaS-BS)-mNG and its expression performance was compared with that of the template plasmid (Figure 4). In D-mannitol medium without and with 1% (w/v) L-rhamnose, both strains showed similar growth independent of the plasmids or L-rhamnose supplementation (Figure 4D). Interestingly, in the absence of L-rhamnose, the maximal mNG fluorescence observed for the plasmid carrying +RhaS-BS was almost twice (405 a.u.) that of the parental plasmid (225 a.u.), suggesting additional activation of PrhaBAD by RhaS in the absence of L-rhamnose or a new transcriptional start increasing the mNG expression (Figure 4E). In the presence of 1% (w/v) L-rhamnose, the absolute residual mNG expression were similarly low for both constructs according to the mNG fluorescence. Therefore, the relative residual plasmid-based mNG expression was decreased to 11% by +RhaS-BS due to the doubled absolute expression strength in the absence of L-rhamnose (11% for +RhaS-BS: 405 a.u. reduced to 45 a.u.; 17% for parental: 225 a.u. reduced to 38 a.u.).
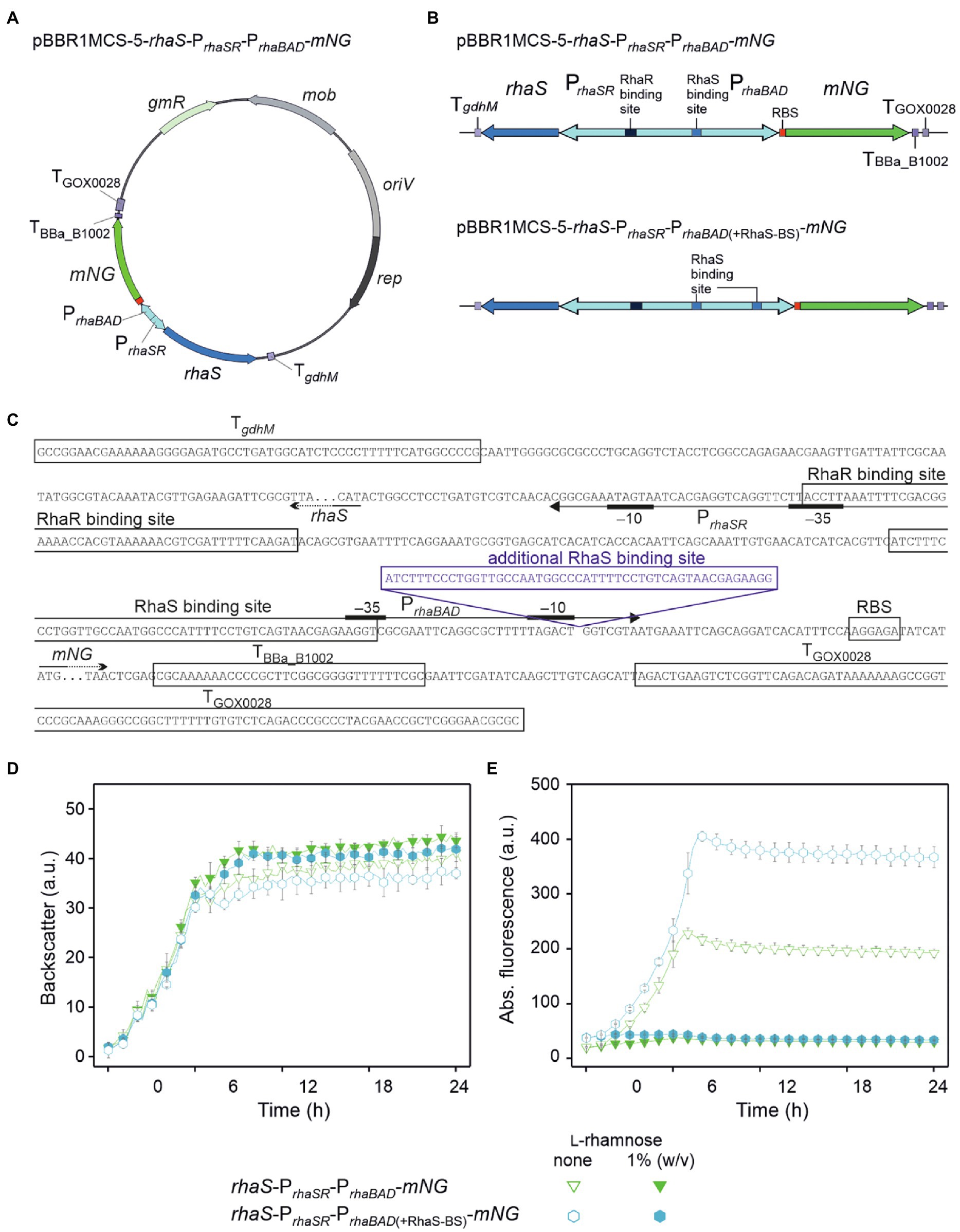
Figure 4. Insertion of an additional RhaS binding site downstream from the −10 region doubled the expression strength of PrhaBAD and the range of repression. (A) Map of plasmid pBBR1MCS-5-rhaS-PrhaSR-PrhaBAD-mNG. (B) Schematic illustration of the pBBR1MCS-5 inserts rhaS-PrhaSR-PrhaBAD-mNG and its variant rhaS-PrhaSR-PrhaBAD(+RhaS-BS)-mNG harboring an additional RhaS binding site directly downstream from the −10 region, all flanked by terminators. (C) DNA sequence details of the fragment rhaS-PrhaSR-PrhaBAD(+RhaS-BS)-mNG with RhaS and RhaR binding sites as well as terminator sequences adjacent to rhaS and mNG. The promoter elements are given according to Egan and Schleif (1993). (D) Growth according to backscatter and (E) absolute mNG fluorescence of G. oxydans 621H carrying either plasmid pBBR1MCS-5-rhaS-PrhaSR-PrhaBAD-mNG or pBBR1MCS-5-rhaS-PrhaSR-PrhaBAD(+RhaS-BS)-mNG in microscale BioLector cultivations in D-mannitol medium without and with 1% (w/v) L-rhamnose. Data represent mean values and standard deviation from two biological replicates (clones) with three technical replicates each. BioLector settings: backscatter gain 20, fluorescence gain 40.
The tunability of PrhaBAD(+RhaS-BS) repression was tested with 0.05%, 0.1%, 0.2%, 0.3%, 1%, and 3% (w/v) L-rhamnose (Figures 5A–D). With 1% and 3% (w/v), the reduction of the mNG fluorescence was similarly high (from 405 a.u. to 43 and 41 a.u., respectively), indicating that like PrhaBAD, plasmid-based PrhaBAD(+RhaS-BS) is also almost maximally repressed by 1% (w/v) L-rhamnose. The calculated residual mNG expression from PrhaBAD(+RhaS-BS) was 11% and 10%, respectively. With 0.3% (w/v) L-rhamnose, the residual mNG fluorescence was 17% (405 vs. 69 a.u.). With only 0.05% (w/v) L-rhamnose, the mNG fluorescence was reduced approximately by half (from 406 to 197 a.u.), showing the sensitivity and tunability of the system. In shake flask cultivations with 0.25% and 1% (w/v) L-rhamnose, PrhaBAD(+RhaS-BS) showed a similar repression performance as in microscale BioLector conditions. After 9 h of growth in shake flasks, the maximal mNG fluorescence without L-rhamnose (5,833 a.u.) was reduced to 1,060 and 600 a.u. in the presence of 0.25% and 1% (w/v) L-rhamnose (Figures 5E,F), representing 18% and 10% residual mNG expression.
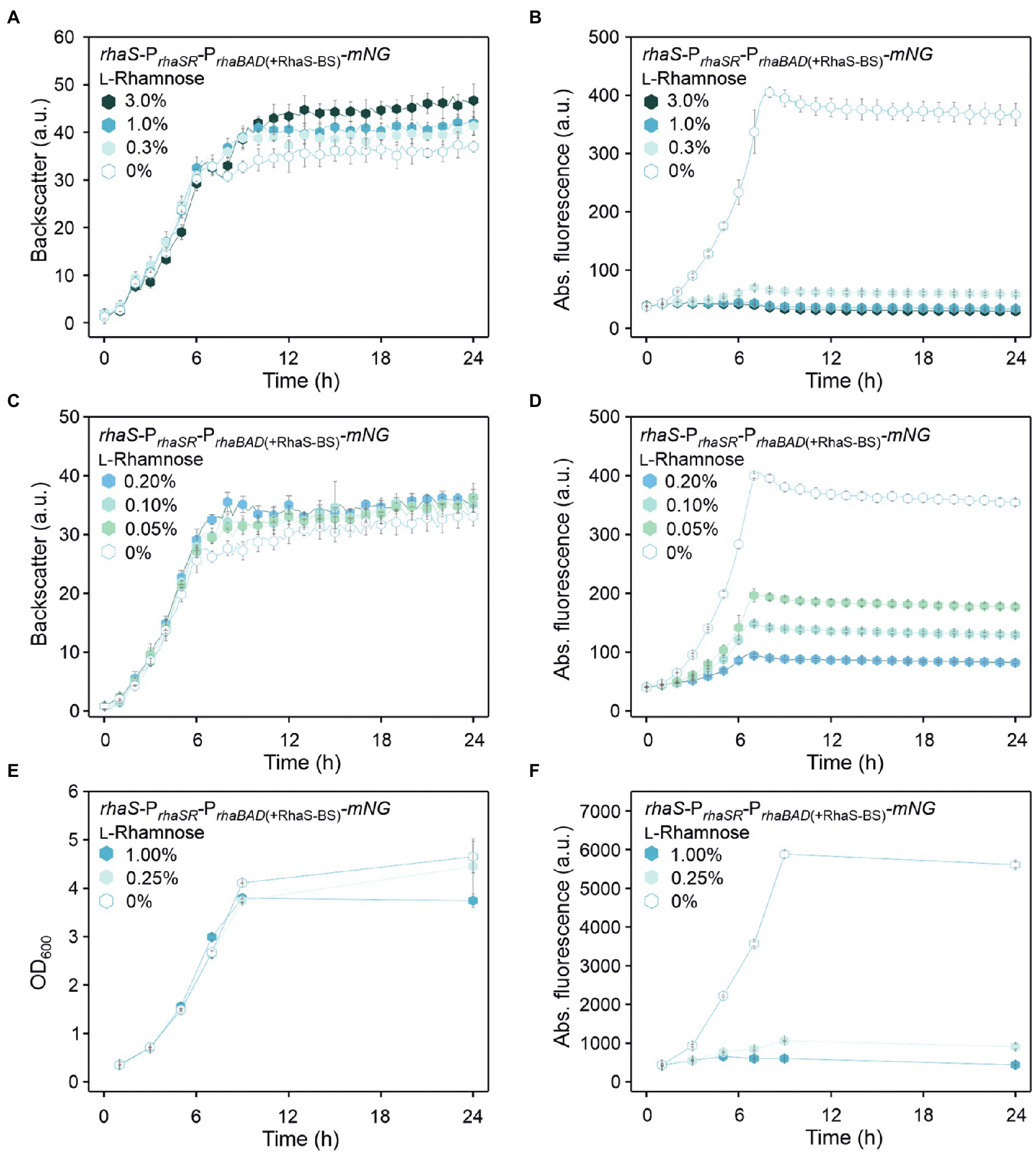
Figure 5. Tunability of the RhaS-PrhaBAD(+RhaS-BS) system in G. oxydans 621H carrying plasmid pBBR1MCS-5-rhaS-PrhaSR-PrhaBAD(+RhaS-BS)-mNG. (A,C) Growth in D-mannitol medium according to backscatter and (B,D) absolute mNG fluorescence in BioLector cultivations. L-Rhamnose was supplemented in concentrations ranging from 0.05 to 3% (w/v). All data represent mean values and standard deviation from two biological replicates (clones) with three technical replicates each. BioLector settings: backscatter gain 20, fluorescence gain 40. (E) Growth in D-mannitol medium and (F) absolute mNG fluorescence in shake flasks. The mNG fluorescence was measured in a Tecan reader (gain 60).
Plotting the relative maximal PrhaBAD- and PrhaBAD(+RhaS-BS)-derived mNG fluorescence vs. the L-rhamnose concentrations illustrates the responsiveness of both promoters toward low L-rhamnose concentrations (Figure 6). While the absolute repression of both promoters was similar and down to 10% of the maximal individual expression strength, non-repressed PrhaBAD(+RhaS-BS) was two-fold stronger than PrhaBAD and therefore offers a wider dynamic range of expression.
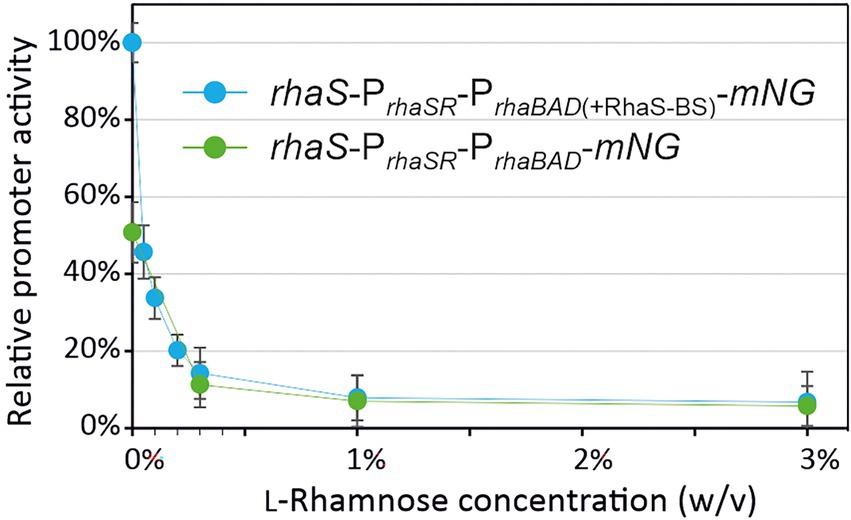
Figure 6. Responsiveness of PrhaBAD and PrhaBAD(+RhaS-BS) towards L-rhamnose. The maximal mNG fluorescence of G. oxydans 621H carrying either plasmid pBBR1MCS-5-rhaS-PrhaSR-PrhaBAD-mNG or pBBR1MCS-5-rhaS-PrhaSR-PrhaBAD(+RhaS-BS)-mNG in response to different L-rhamnose concentrations was used to calculate relative % promoter activities compared to the maximal PrhaBAD(+RhaS-BS) activity in the absence of L-rhamnose (100%).
A genomic single copy of PrhaBAD(+RhaS-BS) can be tuned and completely repressed by RhaS and L-rhamnose
We then analyzed if the stronger PrhaBAD variant can be completely repressed in a plasmid-free strain when this modified target promoter and rhaS are genomically integrated and present as a single copy instead of being present on a plasmid with medium copy number (Figure 7A). Therefore, we integrated the reporter gene mNG under control of PrhaBAD(+RhaS-BS) into the intergenic region igr3 (GOX0038/GOX_RS01330–GOX0039/GOX_RS01335). The resulting strain was termed G. oxydans mNG. For single-copy rhaS expression, we tested the promoters PrhaSR and PGOX0264 and integrated both rhaS constructs in G. oxydans mNG separately into igr2 (GOX0028/GOX_RS01280–GOX0029/GOX_RS01285). The resulting G. oxydans strains mNG igr2::PGOX0264-rhaS and mNG igr2::PrhaSR-rhaS were cultivated and analyzed in a BioLector (Figures 7B,C). As observed before with the plasmid-based approach, in the absence of L-rhamnose expression of single-copy rhaS under control of PrhaSR resulted in higher activity of PrhaBAD(+RhaS-BS) than with PGOX0264-rhaS. However, with rhaS under control of PGOX0264 a much higher extent of repression was observed with 1% (w/v) L-rhamnose. Here, the maximal mNG signals were reduced by 64% from 217 to 78 a.u. (Figure 7C). These results indicated that single-copy rhaS expression is not sufficient to completely repress PrhaBAD(+RhaS-BS). We then tested if a second genomic rhaS copy could be sufficient and integrated both PGOX0264-rhaS and PrhaSR-rhaS into strain mNG igr2::PGOX0264-rhaS separately into igr1 (GOX0013/GOX_RS01200–GOX0014/GOX_RS01205). The two resulting G. oxydans strains mNG igr1::PGOX0264-rhaS igr2::rhaS and mNG igr1::PrhaSR-rhaS igr2::rhaS were cultivated and analyzed in a BioLector (Figures 7D,E). The extent of repression in the presence of L-rhamnose was higher with two rhaS copies compared to only one copy and again PGOX0264-rhaS performed better in repression than PrhaSR-rhaS, yet two genomic rhaS copies were still not sufficient to completely repress PrhaBAD(+RhaS-BS). With one copy of PrhaSR-rhaS and one copy of PGOX0264-rhaS the maximal mNG signals were reduced by 78% from 435 to 96 a.u. With two genomic copies of PGOX0264-rhaS, the maximal mNG signals were reduced by 84% from 444 to 73 a.u. (Figure 7E).
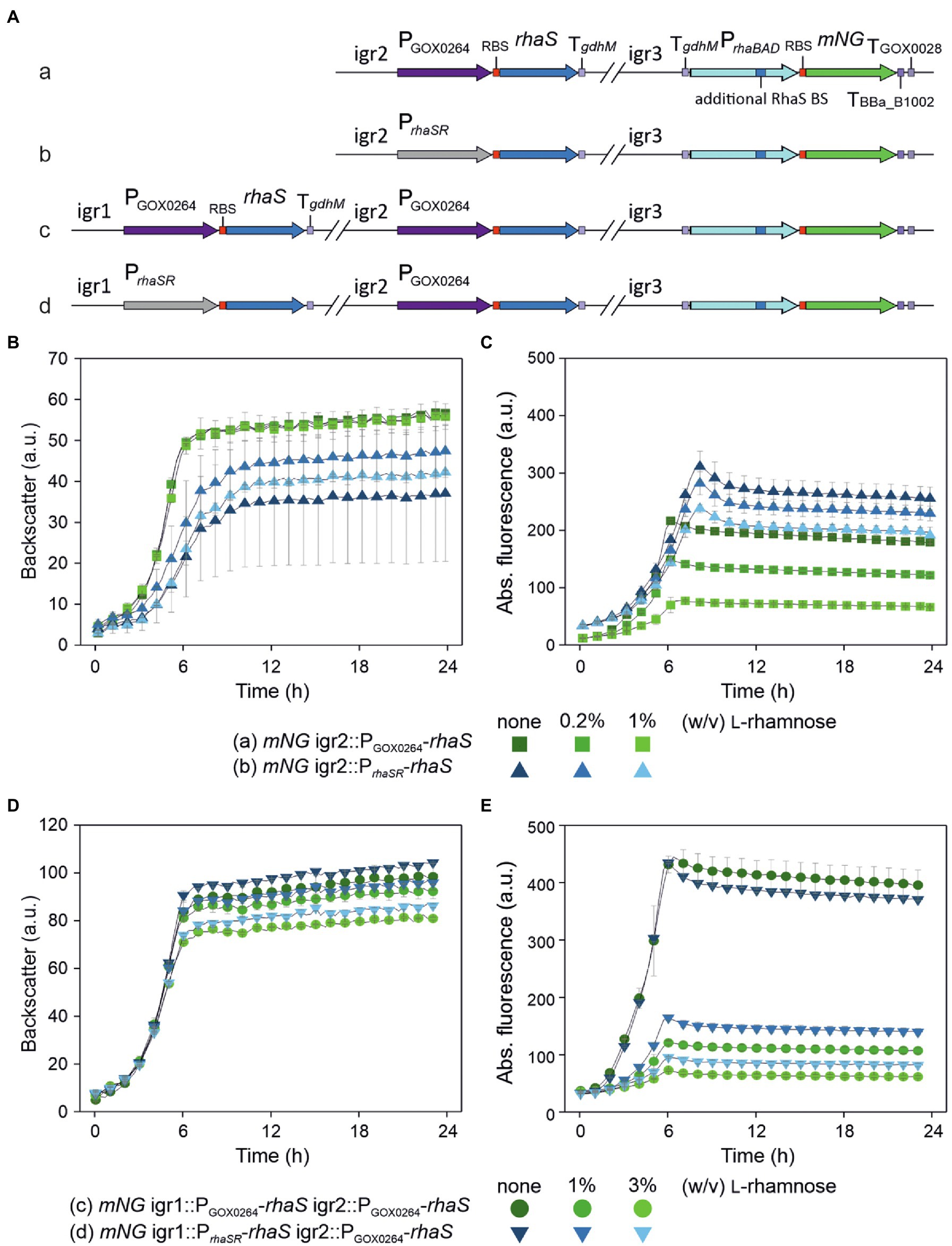
Figure 7. Partial repression of genomic single-copy PrhaBAD(+RhaS-BS)-mNG using genomically integrated copies of rhaS. (A) Schematic illustration of the genomic backgrounds of the G. oxydans 621H strains. The expression cassette PrhaBAD(+RhaS-BS)-mNG of the reporter gene was genomically integrated into the intergenic region igr3 (GOX0038/GOX_RS01330–GOX0039/GOX_RS01335). The resulting strain was termed G. oxydans mNG. For single-copy rhaS expression, a rhaS expression cassette either under control of PGOX0264 (a) or PrhaSR (b) was genomically integrated in G. oxydans mNG into igr2 (GOX0028/GOX_RS01280 - GOX0029/GOX_RS01285). A second rhaS expression cassette again either under control of PGOX0264 (c) or PrhaSR (d) was genomically integrated into igr1 (GOX0013/GOX_RS01200 - GOX0014/GOX_RS01205) in strain A with PGOX0264-rhaS in igr2. (B,D) Growth of the strains in D-mannitol medium according to backscatter and (C,E) absolute mNG fluorescence in BioLector cultivations. L-Rhamnose was supplemented as indicated. All data represent mean values and standard deviation from two biological replicates (clones) with three technical replicates each. BioLector settings: backscatter gain 20, fluorescence gain 70.
To test if a genomic single-copy PrhaBAD(+RhaS-BS) can be completely repressed at all, we constructed the rhaS expression plasmid pBBR1MCS-5-PrhaSR-rhaS and introduced it into the single-copy rhaS strain G. oxydans mNG igr2::PGOX0264-rhaS already showing 64% promoter repression (Figure 8A). The resulting plasmid-carrying strain was cultivated and analyzed in a BioLector (Figures 8B,C). According to the mNG signals, the genomic single-copy PrhaBAD(+RhaS-BS) appeared completely repressed by 3% and possibly also by 1% (w/v) L-rhamnose. To test the tunability of this repression with plasmid-based expression of rhaS, we also tested lower L-rhamnose concentrations (Supplementary Figure S5). In the presence of 0.1% (w/v) L-rhamnose, the maximal mNG signals were reduced by 64% from 216 to 78 a.u.. In the presence of 0.2% (w/v) L-rhamnose, the maximal mNG signals were reduced by 78% from 216 to 47 a.u.. These results indicated a relatively high sensitivity of the system toward lower L-rhamnose concentrations and that a genomic copy of the RhaS target promoter variant can be tuned.
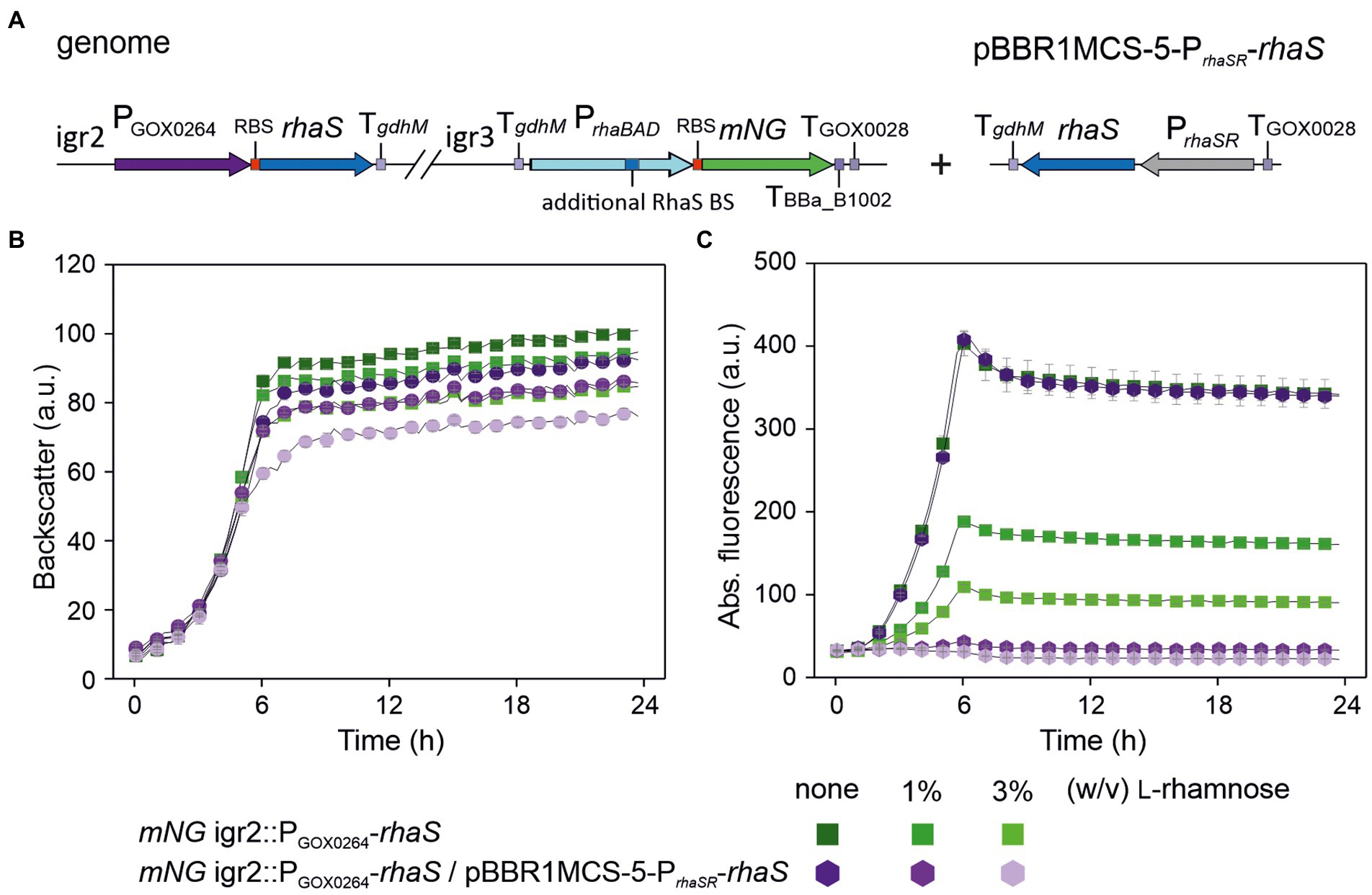
Figure 8. Complete repression of genomic single-copy PrhaBAD(+RhaS-BS)-mNG by plasmid-based expression of rhaS and L-rhamnose. (A) Schematic illustration of the genomic background as described in Figure 7A (variant a) of the plasmid-carrying G. oxydans mNG igr2::PGOX0264-rhaS strain. (B) Growth in D-mannitol medium according to backscatter and (C) absolute mNG fluorescence in BioLector cultivations. For comparison, the plasmid-free strain with only the single-copy rhaS in igr2 was included in the BioLector run. L-Rhamnose was supplemented as indicated. Data represent mean values and standard deviation from three technical replicates of one clone (mNG igr2::PGOX0264-rhaS/pBBR1-MCS-5-PrhaSR-rhaS) and two clones (mNG igr2::PGOX0264-rhaS). BioLector settings: backscatter gain 20, fluorescence gain 70.
The Escherichia coli promoter PrhaT is weak, inducible and tunable in Gluconobacter oxydans
As mentioned above, in E. coli RhaS also activates the promoter PrhaT of the L-rhamnose transporter gene rhaT. Similar to PrhaBAD, PrhaT contains two regulatory elements, one for RhaS and one for CRP binding. Contrary to PrhaBAD, the RhaS binding site on PrhaT is differently composed and slightly shifted, so that the binding site does not overlap with the −35 element of PrhaT (Vía et al., 1996; Wickstrum et al., 2010). To analyze the regulation and performance of PrhaT by RhaS in G. oxydans, we constructed reporter plasmid pBBR1MCS-5-rhaS-PrhaSR-PrhaT-mNG. As a control, plasmid pBBR1MCS-5-PrhaT-mNG lacking rhaS was constructed (Figure 9).
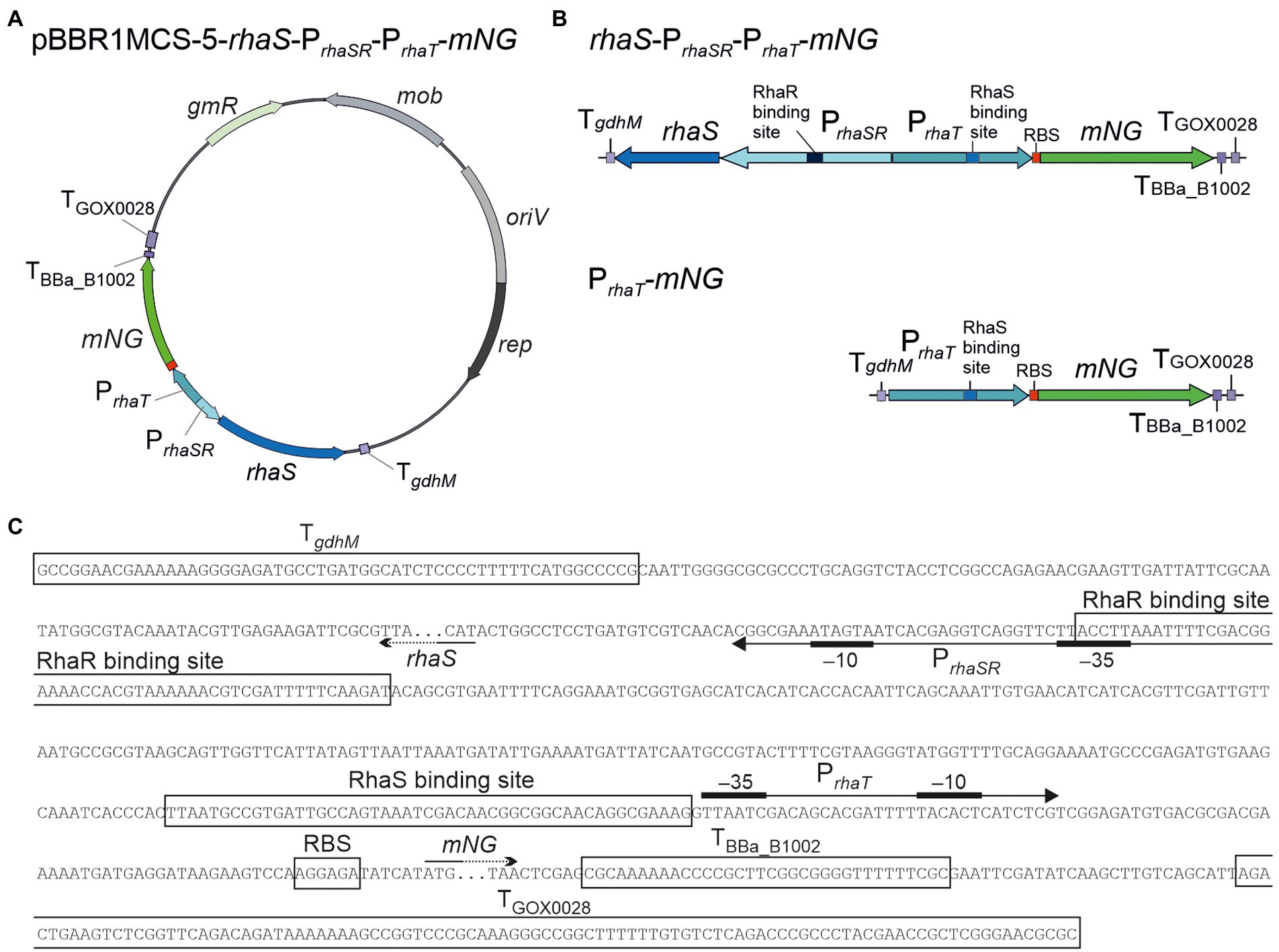
Figure 9. Reporter plasmids with PrhaT and sequence details. (A) Map of plasmid pBBR1MCS-5-rhaS-PrhaSR-PrhaT-mNG with the fluorescence reporter gene mNeonGreen (mNG) under control of the promoter PrhaT from the L-rhamnose transporter gene rhaT with the adjacent rhaS gene under control of PrhaSR, all flanked by the terminators TgdhM, TBBa_B1002 and TGOX0028. (B) Schematic illustration of the pBBR1MCS-5 inserts rhaS-PrhaSR-PrhaT-mNG and its variant PrhaT-mNG lacking rhaS-PrhaSR. (C) DNA sequence details with RhaS and RhaR binding sites and terminator sequences downstream from rhaS and mNG. PrhaT promoter elements are given according to Vía et al. (1996).
In BioLector cultivations, G. oxydans cells with pBBR1MCS-5-rhaS-PrhaSR-PrhaT-mNG or pBBR1MCS-5-PrhaT-mNG showed very similar growth independent of the presence or absence of L-rhamnose (Figure 10A). Interestingly and in contrast to PrhaBAD, mNG expression controlled by PrhaT was induced by L-rhamnose. Addition of 1% (w/v) L-rhamnose increased mNG fluorescence ~7.5-fold (from 36 to 266 a.u.) within 8 h. The values indicated a weak or moderate strength of PrhaT in G. oxydans (Figure 10B). Almost no mNG fluorescence was observed in the strain with plasmid pBBR1MCS-5-PrhaT-mNG without rhaS. Thus, on the one hand PrhaT was almost not active in G. oxydans without RhaS and an endogenous G. oxydans protein did not interfere. On the other hand, RhaS apparently weakly activated PrhaT already in the absence of L-rhamnose since with plasmid pBBR1MCS-5-rhaS-PrhaSR-PrhaT-mNG a low basal mNG fluorescence was observed also in the absence of L-rhamnose exceeding the extremely low mNG signals when rhaS was absent (Figure 10B). Alternatively, a low level of L-rhamnose could be present in the complex medium resulting in a basal RhaS-dependent induction of the system. It should be noted that due to the relatively weak expression from PrhaT compared to PrhaBAD, in these BioLector cultivations the fluorescence signals were monitored with gain 70 instead of gain 40 or 50.
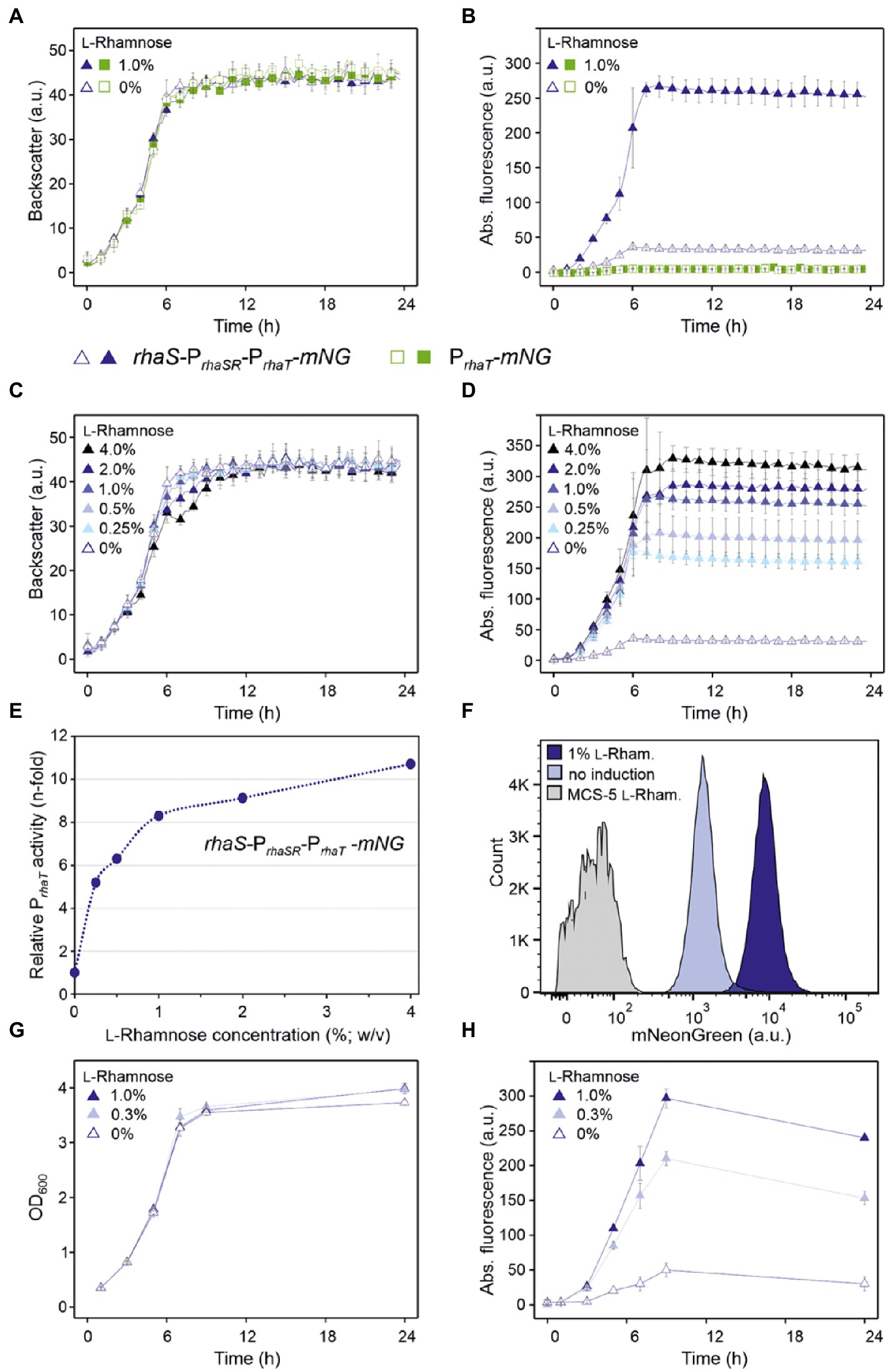
Figure 10. Performance of the RhaS-PrhaT system in G. oxydans 621H. (A) Growth according to backscatter and (B) absolute mNG fluorescence of G. oxydans 621H carrying plasmid pBBR1MCS-5-rhaS-PrhaSR-PrhaT-mNG or pBBR1MCS-5-PrhaT-mNG lacking rhaS in microscale BioLector cultivations without and with 1% (w/v) L-rhamnose. (C) Growth (backscatter) and (D) absolute mNG fluorescence of G. oxydans 621H carrying plasmid pBBR1MCS-5-rhaS-PrhaSR-PrhaT-mNG in microscale BioLector cultivations with L-rhamnose concentrations from 0.25% to 4% (w/v) as indicated. BioLector settings: backscatter gain 20, fluorescence gain 70. (E) Correlation between the relative n-fold PrhaT activity in G. oxydans 621H carrying plasmid pBBR1MCS-5-rhaS-PrhaSR-PrhaT-mNG and the L-rhamnose concentrations. For the calculation, the maximal mNG fluorescence in the absence of L-rhamnose was set to 1. (F) FACS analysis of G. oxydans 621H carrying plasmid pBBR1MCS-5-rhaS-PrhaSR-PrhaT-mNG or empty vector pBBR1MCS-5 (MCS-5) as a control. Cells were grown in shake flasks with D-mannitol medium without and with 1% (w/v) L-rhamnose. FACS analysis was performed 7 h after inoculation (induction). Total counts per sample represent 100,000 events. (G) Growth (OD600) and (H) L-Rhamnose-induced mNG fluorescence of G. oxydans 621H carrying plasmid pBBR1MCS-5-rhaS-PrhaSR-PrhaT-mNG in shake flask cultivations with D-mannitol medium. The mNG fluorescence was measured in a Tecan reader (gain 60). All data represent mean values and standard deviation from two biological replicates (clones) with three technical replicates each.
The tunability of PrhaT induction was tested with L-rhamnose concentrations ranging from 0.25% to 4% (w/v). Again, growth of G. oxydans cells with pBBR1MCS-5-rhaS-PrhaSR-PrhaT-mNG was largely unaffected by up to 2% (w/v) L-rhamnose (Figure 10C). With 4% (w/v) L-rhamnose, the backscatter data suggested a biphasic growth. The PrhaT-derived mNG expression increased gradually in an inducer-dependent manner (Figure 10D). The maximal induction observed was 9.2-fold (36 vs. 330 a.u.) and required 4% (w/v) L-rhamnose. With 0.25% (w/v) L-rhamnose already half of the maximal induction was reached showing that the weak to moderate PrhaT-derived mNG expression could be nicely tuned by low L-rhamnose concentrations (Figure 10E). The low expression strength of PrhaT and its tunability could be of particular interest for the synthesis of proteins forming inclusion bodies when expressed at higher levels.
The homogeneity of PrhaT induction was analyzed by FACS using cells harvested after 7 h of growth in D-mannitol medium without or with 1% (w/v) L-rhamnose (Figure 10F). In the absence of L-rhamnose, 97.4% of the analyzed cells with pBBR1MCS-5-rhaS-PrhaSR-PrhaT-mNG showed relatively low fluorescence signals (~1,000 a.u.). In the presence of 1% (w/v) L-rhamnose, 96.9% of the population showed approximately 9-fold higher mNG fluorescence signals (~9,000 a.u.). We also tested the inducible PrhaT-derived mNG expression in shake flask cultures with 0.3% and 1% (w/v) L-rhamnose. Under these conditions, all cultures with pBBR1MCS-5-rhaS-PrhaSR-PrhaT-mNG exhibited very similar growth (Figure 10G). The mNG expression was similarly induced as in the BioLector cultivations (Figure 10H). The maximal mNG fluorescence was reached after 9 h of growth and represented 4-fold and 6-fold induction with 0.3% (50 vs. 210 a.u.) and 1% (w/v) L-rhamnose (50 vs. 297 a.u.), respectively.
To test the influence of rhaS expression strength from different promoters on the performance of the RhaS-PrhaT system, we replaced PrhaSR and constructed plasmid variants with PGOX0264-rhaS and PGOX0452-rhaS (Figure 11A). The G. oxydans strains with either of the reporter plasmids were cultivated and analyzed in a BioLector to compare the basal expression level and the induction performance with that of cells expressing rhaS under the control of PrhaSR (Figures 11B–E). For both tested G. oxydans promoters the maximal mNG signals with 4% (w/v) L-rhamnose were ~25% lower compared to that obtained with PrhaSR-rhaS. Since the non-induced maximal mNG signals obtained with PrhaT were somewhat higher with PGOX0264-rhaS (46 a.u.) and were approximately 3-fold higher with PGOX0452-rhaS (104 a.u.) compared to PrhaSR-rhaS (36 a.u.), the maximal induction fold changes with 4% (w/v) L-rhamnose were only 5-fold with PGOX0264-rhaS and 2.4-fold with PGOX0452-rhaS. Thus, compared to PrhaSR-rhaS the non-induced basal expression level was not lowered and the induction fold changes of the RhaS-PrhaT system were not improved by using PGOX0264 or PGOX0452 for rhaS expression.
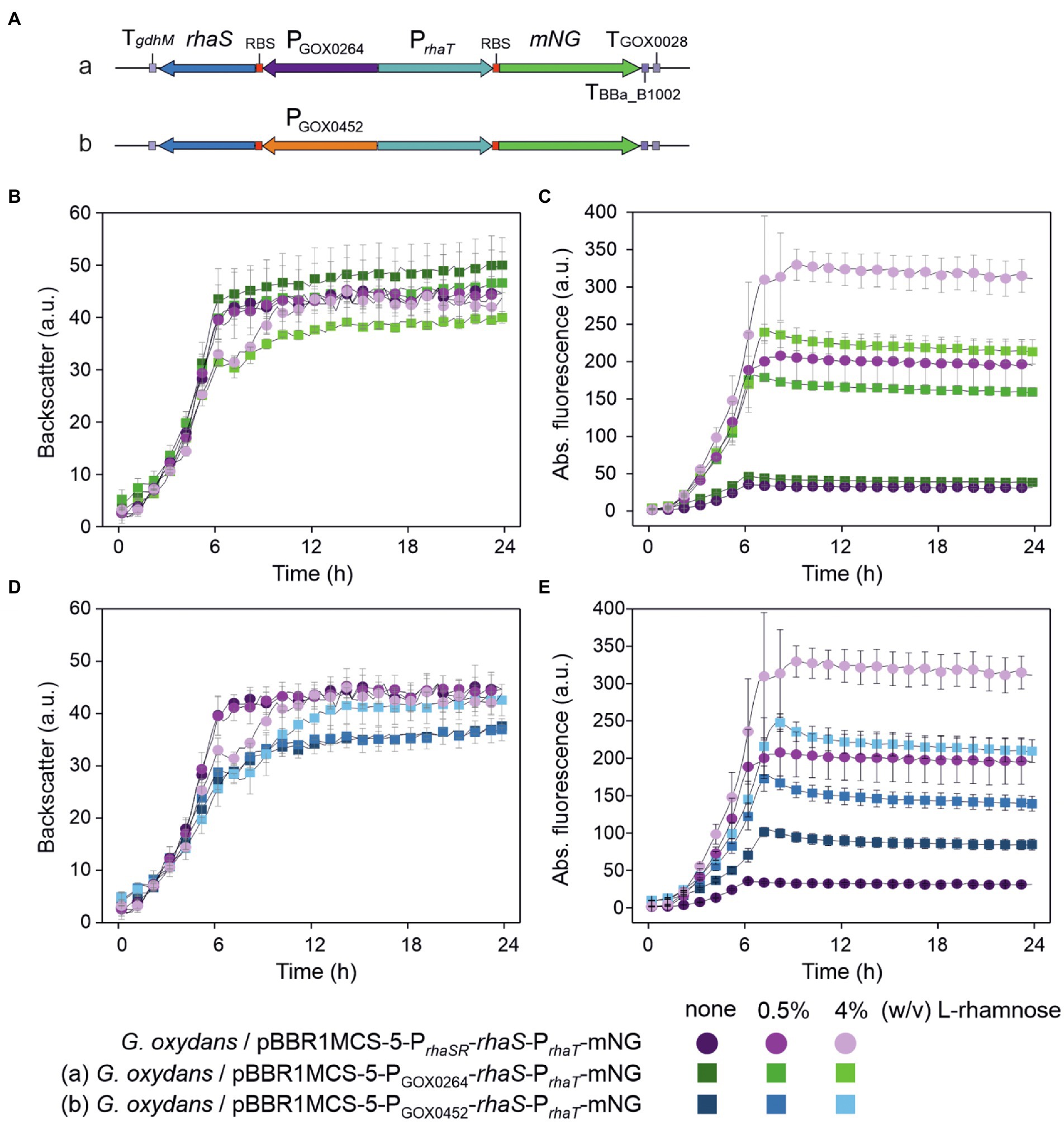
Figure 11. Performance of PrhaT-derived induction of mNG expression in dependence of rhaS expression and presence of L-rhamnose. (A) Schematic illustration of the pBBR1MCS-5 plasmid inserts to test the effects of rhaS expression. (B,D) Growth of the G. oxydans 621H strains with rhaS expression plasmid in D-mannitol medium according to backscatter and (C,E) absolute mNG fluorescence in BioLector cultivations. L-Rhamnose was supplemented as indicated. All data represent mean values and standard deviation from two biological replicates (clones) with three technical replicates each. BioLector settings: backscatter gain 20, fluorescence gain 70.
Insertion of an additional RhaS binding site can reverse the regulation making PrhaT repressible by RhaS and L-rhamnose
To test the influence of an additional RhaS binding site on the expression performance of PrhaT, we inserted the RhaS binding site sequence from PrhaBAD on the one hand directly downstream from the E. coli −10 region (−10-RhaS-BS) and on the other hand downstream from the E. coli TSS (TSS-RhaS-BS), and constructed for both PrhaT variants expression plasmids with rhaS under control of either PGOX0264 or PGOX0452 (Figures 12A,B). In case of the −10-RhaS-BS, the regulation was reversed and PrhaT(−10-RhaS-BS) was repressible. The maximal mNG signals in the absence of L-rhamnose for both rhaS constructs PGOX0264-rhaS (250 a.u.) and PGOX0452-rhaS (214 a.u.) were reduced by 65% (87 and 77 a.u.; Figures 12C,D). In contrast, the variant PrhaT(TSS-RhaS-BS) was still inducible, yet showed increased and relatively high non-induced mNG signals in the absence of L-rhamnose, which could maximally only be doubled by induction with 4% (w/v) L-rhamnose (Figures 12E,F).
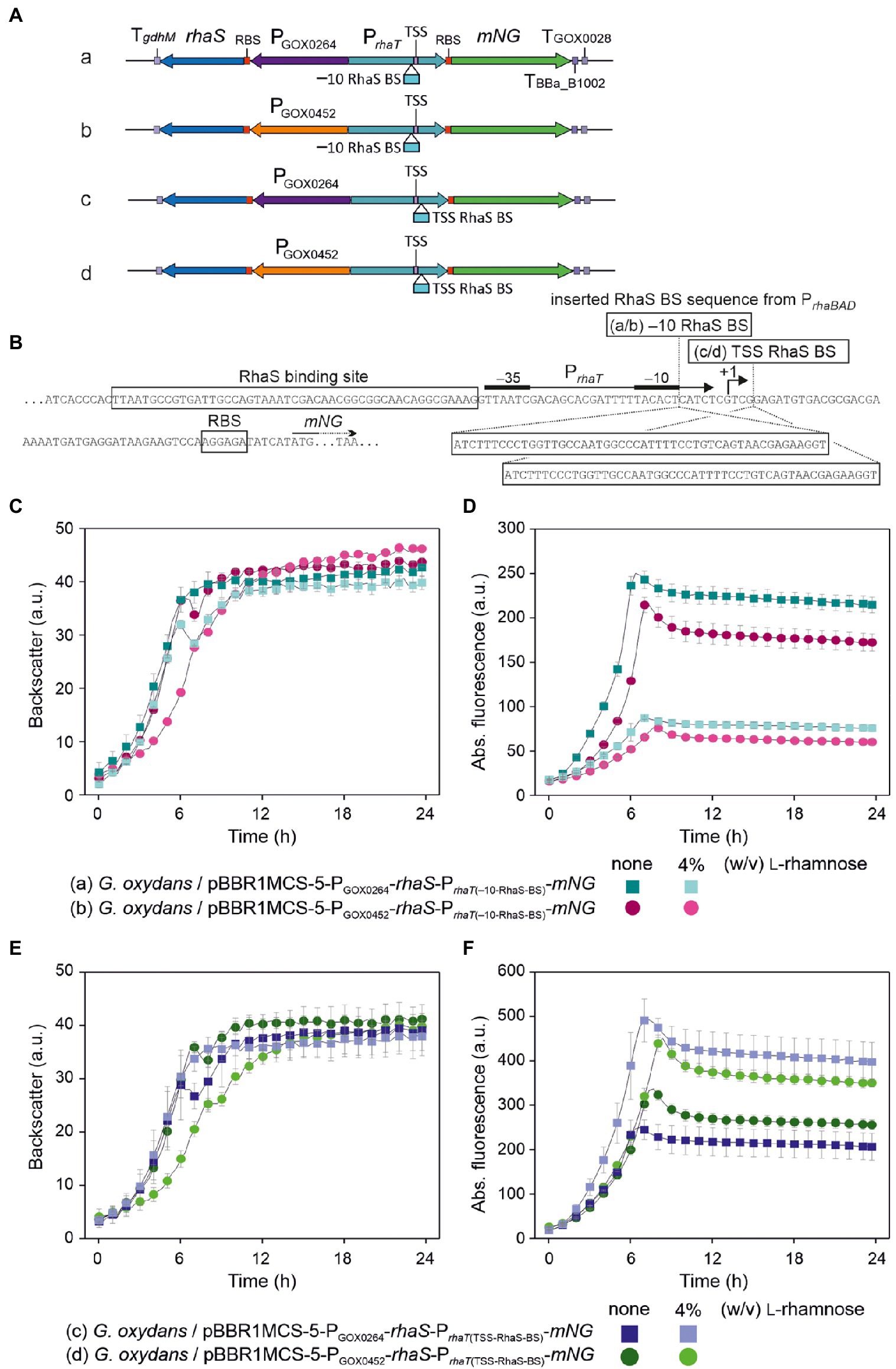
Figure 12. Insertion of an additional RhaS binding site directly downstream from the E. coli −10 region of PrhaT reversed the regulation in G. oxydans making the modified RhaS-PrhaT system repressible in the presence of L-rhamnose. (A) Schematic illustration of the pBBR1MCS-5 plasmid inserts to test the effects of an additional RhaS binding site (RhaS BS) in PrhaT directly downstream from the E. coli −10 region (−10 RhaS BS) or downstream from the E. coli PrhaT transcriptional start site (TSS RhaS BS) together with rhaS expression from PGOX0264 or PGOX0452. (B) Sequence details of PrhaT with the positions and RhaS binding site sequence from PrhaBAD inserted either directly downstream from the E. coli −10 region or downstream from the E. coli transcriptional start site (TSS +1) according to Vía et al. (1996). (C,E) Growth of the G. oxydans 621H strains with rhaS expression plasmid and modified PrhaT in D-mannitol medium according to backscatter and (D,F) absolute mNG fluorescence in BioLector cultivations. L-Rhamnose was supplemented as indicated. All data represent mean values and standard deviation from two biological replicates (clones) with three technical replicates each. BioLector settings: (C,E) backscatter gain 20, (D) fluorescence gain 60, (F) fluorescence gain 70.
Discussion
In this study, we found that the promoters PrhaBAD and PrhaT together with the transcriptional regulator RhaS, all derived from E. coli, exhibit interesting characteristics for the control of gene expression in the AAB G. oxydans. These characteristics are affected by the rhaS expression strength and additional RhaS binding sites in PrhaBAD and PrhaT. With RhaS-PrhaBAD we found the first system for G. oxydans that permits controlled down-regulation in an effector-dependent manner exhibiting tunability and enabling complete repression of a genomically encoded target gene. Furthermore, the regulation of PrhaT could be reversed from inducible to repressible by inserting an additional RhaS binding site. Altogether, these features provide novel opportunities expanding the genetic toolbox for regulatable gene expression in G. oxydans and are possibly also interesting for other AAB.
In E. coli the L-rhamnose-induced regulation of PrhaBAD requires both RhaR and RhaS (Egan and Schleif, 1993, 1994; Kelly et al., 2016). In G. oxydans, only RhaS played an effective role for the regulation of the system. In E. coli, first RhaR activates expression of the rhaSR operon in the presence of L-rhamnose, which is a prerequisite to provide sufficient RhaS levels for the induction of PrhaBAD by RhaS. This stimulation of rhaSR expression by RhaR is proposed to be achieved by bending the PrhaSR promoter DNA so that PrhaSR-bound cAMP receptor protein (CRP) can interact with the RNA polymerase (RNAP) and thereby activates transcription of rhaSR (Wickstrum et al., 2010). Activation of PrhaSR by RhaR in such a manner is not possible in G. oxydans since CRP is absent. The protein showing the highest similarity to CRP was shown to function as an iron–sulfur cluster-containing FNR-type transcriptional regulator (GOX0974/GOX_RS06010) of genes involved in respiration and redox metabolism (Schweikert et al., 2021). In G. oxydans, the presence of RhaR even decreased the RhaS-dependent PrhaBAD activity (Figure 1). This might be caused by a decreased expression of rhaSR, resulting in a lower RhaS level. In E. coli, L-rhamnose only affects the RhaR-dependent DNA bending and thereby activates transcription from PrhaSR, yet the binding of RhaR to its target DNA per se was not affected by L-rhamnose (Kolin et al., 2008). RhaS can also bind to the RhaR binding site of PrhaSR leading to lowered expression of the rhaSR operon in E. coli, thereby providing a negative feedback loop since the RhaS-dependent DNA bending of PrhaSR is different from the bending by RhaR and prevents CRP-dependent activation of rhaSR expression (Wickstrum et al., 2010). In G. oxydans, RhaS alone activated PrhaSR already in the absence of L-rhamnose and L-rhamnose further stimulated this effect (Supplementary Figures S4A,C). Therefore, in G. oxydans RhaR likely binds to PrhaSR and competes with RhaS, causing an inhibition of PrhaSR activation by RhaS and consequently lowered the RhaS level, resulting in the lower PrhaBAD activity. Alternatively, or partially, the data obtained with the constructs omitting only rhaS and both rhaSR suggested that in G. oxydans RhaR could also bind to PrhaBAD and competes with RhaS in binding to PrhaBAD, resulting in the lowered reporter signals in the absence of L-rhamnose (Figure 1). Hence, omitting rhaR and using only rhaS provides advantages when using these regulatable E. coli promoters for gene expression in G. oxydans.
A surprising outcome of this study was the reversed regulation of PrhaBAD by RhaS in G. oxydans, while PrhaT was still inducible as in E. coli. RhaS belongs to the AraC/XylS family of transcriptional regulators (Tobin and Schleif, 1987). Within this protein family most members interact with the C-terminal domain (CTD) of the α-subunit of the RNAP to activate transcription (reviewed in Ebright and Busby, 1995). It was shown that deletion of the RNAP α-CTD reduced expression 180-fold, suggesting a direct interaction of RhaS and the α-CTD of the E. coli RNAP (Holcroft and Egan, 2000). Nevertheless, some members of the AraC/XylS family may also activate transcription through interaction with the sigma 70 factor (σ70) subunit RpoD of the RNAP. This mode of activation is often indicated by regulator binding sites overlapping with the −35 element of the target promoter (Lonetto et al., 1998; Bhende and Egan, 2000). Within PrhaBAD, 4 bp of the RhaS binding site overlap with the −35 hexamer of this promoter (Figure 4), while within PrhaT the RhaS binding site does not overlap and ends 1 bp upstream from the −35 element (Figure 9). Among the family of σ70 transcription factors, the C-terminus is highly conserved as it contains DNA-binding domains and well-defined functional regions (Hakimi et al., 2000; Paget, 2015). In alanine substitution experiments, it was shown that D241 and D250 of RhaS and K593 and R599 of σ70 are likely interacting residues required for RhaS-dependent activation of PrhaBAD in E. coli (Bhende and Egan, 2000; Wickstrum and Egan, 2004). While the entire σ70 amino acid sequences from G. oxydans and from E. coli K12 exhibit only 49% identity, primarily due to little similarities in the N-terminal part, the C-terminal regions share 84% identity. In the two regions likely involved in −10 and −35 recognition, only two residues are different (Supplementary Figure S6). R448 and R599 in σ70 from E. coli correspond to K486 and K637 in σ70 from G. oxydans. R599 is involved in the recognition of the −35 hexamer and in interaction with RhaS in E. coli (Bhende and Egan, 2000; Wickstrum and Egan, 2004). Although the exchange is conservative, K637 might contribute to the reversed responsiveness in G. oxydans.
As mentioned above, in PrhaBAD the RhaS binding site overlaps with most of the −35 region by 4 bp while in PrhaT the RhaS binding site does not overlap with the −35 region and ends 1 bp upstream (Vía et al., 1996). These different distances in DNA binding positions result in different radial orientations of RhaS toward σ70-RNAP along the longitudinal DNA axis. Theoretically, with a turn of 36° per bp, a distance of 5 bp turns the radial orientation by 180°, putting RhaS (or σ70-RNAP) to the other side of the DNA strand when comparing the theoretical binding of RhaS and σ70-RNAP to PrhaBAD with the binding to PrhaT. Because of this theoretical difference in the orientation of RhaS toward σ70-RNAP, RhaS possibly interacts with the α-CTD of the RNAP in the case of PrhaT and with σ70 in the case of PrhaBAD. Since the α-CTD and σ70 from G. oxydans and E. coli differ to some extent, the conformational changes of RhaS induced by the binding of L-rhamnose may affect the interactions of RhaS with the α-CTD and with σ70 from G. oxydans differently compared to the interactions with the α-CTD and with σ70 from E. coli, finally resulting in the different modes of the regulation of PrhaBAD and PrhaT in G. oxydans. Interestingly, in the case of PrhaSR, the RhaR binding site also overlaps with the −35 region as the RhaS binding site in PrhaBAD. Moreover, one of the major groove regions of each RhaR half site on PrhaSR is nearly identical to the corresponding half site for RhaS binding on PrhaBAD and RhaS can also bind to the RhaR binding site in PrhaSR as mentioned above (Egan and Schleif, 1994). Despite these similarities between PrhaSR and PrhaBAD, in contrast to PrhaBAD, PrhaSR was still inducible by RhaS and L-rhamnose in G. oxydans. These differences in G. oxydans cannot be explained without further experimental data. For example, the recognition by and the affinity of σ70 to potential −35 and −10 regions in the absence and in the presence of RhaS and therefore the positional binding of the host RNAP to the E. coli promoter DNA relative to the RhaS binding position might differ in G. oxydans because of different DNA sequence specificities of σ70. Therefore, knowledge about the transcriptional starts sites (TSSs) within the three E. coli promoter regions PrhaBAD, PrhaT, and PrhaSR in G. oxydans is required to better explain the effects, including the activation of PrhaBAD by RhaS in the absence of L-rhamnose, the repression, and the effects of the additional RhaS binding site inserted into PrhaBAD and PrhaT.
In a first and preliminary attempt to obtain such TSS data, we prepared a total RNA sample from G. oxydans 621H with plasmid pBBR1MCS-5-rhaS-PrhaSR-PrhaBAD(+RhaS-BS)-mNG cultivated in the complex medium with D-mannitol in the absence of L-rhamnose and harvested in the mid-exponential phase. The RNA sample was sent to Vertis Biotechnologie AG for sample processing and Illumina sequencing to obtain high-quality TSS data (see Materials and Methods). The resulting fastq file comprised 10,255,084 reads (75 bp). After reads trimming and quality filtering, 1,023,259 reads mapped to the sequence of pBBR1MCS-5-rhaS-PrhaSR-PrhaBAD(+RhaS-BS)-mNG. The overall reads mapping showed three prominent reads stacks indicating the three most active transcriptional starts on the plasmid (Supplementary Figure S7). The by far highest stack (~560,000 coverage) corresponded to the annotated promoter region of gmR (aacC1) conferring gentamycin resistance and was oriented toward gmR. The second-highest stack (~175,000 coverage) was upstream from rhaS and oriented toward rhaS. In contrast to the expectation for rhaS, the start position of this stack was not within PrhaSR, but upstream from PrhaSR within the PrhaBAD region between its −35 and −10 regions from E. coli. The third-highest stack (~65,000 coverage) was found within the coding region of rhaS and oriented toward the 3′ end of rhaS. For PrhaBAD, the insertion of an additional RhaS binding site could possibly generate an additional transcriptional start site in G. oxydans enabling the two-fold increased mNG signals described above for PrhaBAD(+RhaS-BS). However, in contrast to the expectations, no one or two major TSSs with high coverage toward mNG corresponding to the reported E. coli TSS and a potential new TSS could be seen. Instead, the detailed reads mapping showed several reads stacks of only medium coverage, partially with scattering start positions, in the PrhaBAD(+RhaS-BS) region and the 5′ region of mNG (Supplementary Figure S8). Therefore, the mapping data surprisingly suggested several TSSs in this promoter region oriented toward the 3′ end of the reporter gene: 2 or more potential TSSs upstream from mNG and 3 or more potential TSSs in the 5′ region of mNG. The most upstream potential TSS for mNG was very close to the E. coli −35 region of PrhaBAD. These unexpected preliminary results require further and more detailed analysis as well as some comparisons, including the analysis of RNA samples from G. oxydans grown in the presence of L-rhamnose, from cells without rhaS, and with the other promoters PrhaSR and PrhaT.
Summing up and looking ahead, in G. oxydans the RhaS-dependent regulation of the E. coli RhaS target promoters and variants thereof provide new modes for regulatable gene expression in this AAB and possibly also in other AAB species. Inducible and repressible gene expression in response to L-rhamnose could be achieved simultaneously, which may be especially advantageous for combinatorial engineering. Tunability and complete repression of a genomic promoter copy was tested and shown only with the variant PrhaBAD(+RhaS-BS), yet it is likely that also with PrhaBAD and PrhaT(−10-RhaS-BS) complete repression of a genomic copy could be achieved. These promoters cover different ranges of expression strength, which could be selected according to the requirements of the genomic target gene. Tunable and complete promoter repression is also useful for the functional study of essential genes that cannot be deleted. Optimizing genomic rhaS expression or further increasing the genomic rhaS copy number beyond two to achieve a sufficient RhaS level may finally overcome the necessity of plasmid-based rhaS expression to achieve complete chromosomal promoter repression. Furthermore, more TSS data sets and deeper analysis are required to better understand the regulations of the target promoters by RhaS in G. oxydans. The TSS results also suggested to analyze the TSSs of heterologous promoters when they are transferred and used in G. oxydans or AAB in general. It can be expected that TSS data sets will help to better understand and overcome the difficulties in getting transferred heterologous regulatable expression systems functional and high-performant in AAB.
Data availability statement
The raw data supporting the conclusions of this article will be made available by the authors, without undue reservation. The Illumina sequencing data are available in the NCBI sequence read archive via the accession numbers PRJNA854345 and PRJNA854679.
Author contributions
TP and PF designed and supervised the study. PF, MLG, MM, and MH carried out cloning and experiments. JG performed the GC-TOF analysis. PF, MLG, MM, MH, and TP performed data analysis. PF and TP wrote the manuscript. All authors contributed to the article and approved the submitted version.
Funding
We are grateful to the Federal Ministry of Education and Research (BMBF) for financial support of the project IMPRES (031B0370B). The funding organization did not influence the design of the study or collection, analysis, and interpretation of data, or writing the manuscript.
Acknowledgments
We thank Christiane Sonntag and Ulli Degner for their technical assistance with FACS analysis and laboratory work. We thank Armin Ehrenreich for helpful discussion.
Conflict of interest
The authors declare that the research was conducted in the absence of any commercial or financial relationships that could be construed as a potential conflict of interest.
Publisher’s note
All claims expressed in this article are solely those of the authors and do not necessarily represent those of their affiliated organizations, or those of the publisher, the editors and the reviewers. Any product that may be evaluated in this article, or claim that may be made by its manufacturer, is not guaranteed or endorsed by the publisher.
Supplementary material
The supplementary material for this article can be found online at: https://www.frontiersin.org/articles/10.3389/fmicb.2022.981767/full#supplementary-material
References
Ameyama, M., Shinagawa, E., Matsushita, K., and Adachi, O. (1981). D-fructose dehydrogenase of Gluconobacter industrius: purification, characterization, and application to enzymatic microdetermination of D-fructose. J. Bacteriol. 145, 814–823. doi: 10.1128/jb.145.2.814-823.1981
Baldoma, L., Badia, J., Sweet, G., and Aguilar, J. (1990). Cloning, mapping and gene product identification of rhaT from Escherichia coli K12. FEMS Microbiol. Lett. 60, 103–107. doi: 10.1111/j.1574-6968.1990.tb03870.x
Bertucci, M., Ariano, K., Zumsteg, M., and Schweiger, P. (2022). Engineering a tunable bicistronic TetR autoregulation expression system in Gluconobacter oxydans. Peer J. 10:e13639. doi: 10.7717/peerj.13639
Bhende, P. M., and Egan, S. M. (1999). Amino acid-DNA contacts by RhaS: an AraC family transcription activator. J. Bacteriol. 181, 5185–5192. doi: 10.1128/JB.181.17.5185-5192.1999
Bhende, P. M., and Egan, S. M. (2000). Genetic evidence that transcription activation by RhaS involves specific amino acid contacts with sigma 70. J. Bacteriol. 182, 4959–4969. doi: 10.1128/JB.182.17.4959-4969.2000
da Silva, G. A. R., Oliveira, S. S. S., Lima, S. F., do Nascimento, R. P., Baptista, A. R. S., and Fiaux, S. B. (2022). The industrial versatility of Gluconobacter oxydans: current applications and future perspectives. World J. Microbiol. Biotechnol. 38:134. doi: 10.1007/s11274-022-03310-8
Ebright, R. H., and Busby, S. (1995). The Escherichia coli RNA polymerase alpha subunit: structure and function. Curr. Opin. Genet. Dev. 5, 197–203. doi: 10.1016/0959-437X(95)80008-5
Egan, S. M., and Schleif, R. F. (1993). A regulatory cascade in the induction of rhaBAD. J. Mol. Biol. 234, 87–98. doi: 10.1006/jmbi.1993.1565
Egan, S. M., and Schleif, R. F. (1994). DNA-dependent renaturation of an insoluble DNA binding protein. Identification of the RhaS binding site at rhaBAD. J. Mol. Biol. 243, 821–829. doi: 10.1006/jmbi.1994.1684
Fricke, P. M., Hartmann, R., Wirtz, A., Bott, M., and Polen, T. (2022). Production of L-arabinonic acid from L-arabinose by the acetic acid bacterium Gluconobacter oxydans. Bioresour. Technol. 17:100965. doi: 10.1016/j.biteb.2022.100965
Fricke, P. M., Klemm, A., Bott, M., and Polen, T. (2021a). On the way toward regulatable expression systems in acetic acid bacteria: target gene expression and use cases. Appl. Microbiol. Biotechnol. 105, 3423–3456. doi: 10.1007/s00253-021-11269-z
Fricke, P. M., Link, T., Gätgens, J., Sonntag, C., Otto, M., Bott, M., et al. (2020). A tunable L-arabinose-inducible expression plasmid for the acetic acid bacterium Gluconobacter oxydans. Appl. Microbiol. Biotechnol. 104, 9267–9282. doi: 10.1007/s00253-020-10905-4
Fricke, P. M., Lürkens, M., Hunnefeld, M., Sonntag, C. K., Bott, M., Davari, M. D., et al. (2021b). Highly tunable TetR-dependent target gene expression in the acetic acid bacterium Gluconobacter oxydans. Appl. Microbiol. Biotechnol. 105, 6835–6852. doi: 10.1007/s00253-021-11473-x
Gibson, D. G., Young, L., Chuang, R. Y., Venter, J. C., Hutchison, C. A., and Smith, H. O. (2009). Enzymatic assembly of DNA molecules up to several hundred kilobases. Nat. Methods 6, 343–345. doi: 10.1038/nmeth.1318
Gupta, A., Singh, V. K., Qazi, G. N., and Kumar, A. (2001). Gluconobacter oxydans: its biotechnological applications. J. Mol. Microb. Biotech. 3, 445–456.
Hakimi, M. A., Privat, I., Valay, J. G., and Lerbs-Mache, S. (2000). Evolutionary conservation of C-terminal domains of primary sigma(70)-type transcription factors between plants and bacteria. J. Biol. Chem. 275, 9215–9221. doi: 10.1074/jbc.275.13.9215
Hanahan, D. (1983). Studies on transformation of Escherichia coli with plasmids. J. Mol. Biol. 166, 557–580. doi: 10.1016/S0022-2836(83)80284-8
Hekmat, D., Bauer, R., and Fricke, J. (2003). Optimization of the microbial synthesis of dihydroxyacetone from glycerol with Gluconobacter oxydans. Bioprocess Biosyst. Eng. 26, 109–116. doi: 10.1007/s00449-003-0338-9
Holcroft, C. C., and Egan, S. M. (2000). Interdependence of activation at rhaSR by cyclic AMP receptor protein, the RNA polymerase alpha subunit C-terminal domain, and RhaR. J. Bacteriol. 182, 6774–6782. doi: 10.1128/JB.182.23.6774-6782.2000
Kallnik, V., Meyer, M., Deppenmeier, U., and Schweiger, P. (2010). Construction of expression vectors for protein production in Gluconobacter oxydans. J. Biotechnol. 150, 460–465. doi: 10.1016/j.jbiotec.2010.10.069
Kelly, C. L., Liu, Z., Yoshihara, A., Jenkinson, S. F., Wormald, M. R., Otero, J., et al. (2016). Synthetic chemical inducers and genetic decoupling enable orthogonal control of the rhaBAD promoter. ACS Synth. Biol. 5, 1136–1145. doi: 10.1021/acssynbio.6b00030
Kersters, K., Lisdiyanti, P., Komagata, K., and Swings, J. (1990). “The family Acetobacteraceae: the genera Acetobacter, Acidomonas, Asaia, Gluconacetobacter, Gluconobacter, and Kozakia,” in The Prokaryotes. eds. M. Dworkin, S. Falkow, E. Rosenberg, K. H. Schleifer, and E. Stackebrandt (New York, NY: Springer).
Kiefler, I., Bringer, S., and Bott, M. (2017). Metabolic engineering of Gluconobacter oxydans 621H for increased biomass yield. Appl. Microbiol. Biotechnol. 101, 5453–5467. doi: 10.1007/s00253-017-8308-3
Kolin, A., Balasubramaniam, V., Skredenske, J. M., Wickstrum, J. R., and Egan, S. M. (2008). Differences in the mechanism of the allosteric L-rhamnose responses of the AraC/XylS family transcription activators RhaS and RhaR. Mol. Microbiol. 68, 448–461. doi: 10.1111/j.1365-2958.2008.06164.x
Kostner, D., Peters, B., Mientus, M., Liebl, W., and Ehrenreich, A. (2013). Importance of codB for new codA-based markerless gene deletion in Gluconobacter strains. Appl. Microbiol. Biotechnol. 97, 8341–8349. doi: 10.1007/s00253-013-5164-7
Kovach, M. E., Elzer, P. H., Hill, D. S., Robertson, G. T., Farris, M. A., Roop, R. M. 2nd, et al. (1995). Four new derivatives of the broad-host-range cloning vector pBBR1MCS, carrying different antibiotic-resistance cassettes. Gene 166, 175–176. doi: 10.1016/0378-1119(95)00584-1
Kovach, M. E., Phillips, R. W., Elzer, P. H., Roop, R. M. 2nd, and Peterson, K. M. (1994). pBBR1MCS: a broad-host-range cloning vector. BioTechniques 16, 800–802.
Kranz, A., Steinmann, A., Degner, U., Mengus-Kaya, A., Matamouros, S., Bott, M., et al. (2018). Global mRNA decay and 23S rRNA fragmentation in Gluconobacter oxydans 621H. BMC Genomics 19, 753. doi: 10.1186/s12864-018-5111-1
Kranz, A., Vogel, A., Degner, U., Kiefler, I., Bott, M., Usadel, B., et al. (2017). High precision genome sequencing of engineered Gluconobacter oxydans 621H by combining long nanopore and short accurate Illumina reads. J. Biotechnol. 258, 197–205. doi: 10.1016/j.jbiotec.2017.04.016
Lonetto, M. A., Rhodius, V., Lamberg, K., Kiley, P., Busby, S., and Gross, C. (1998). Identification of a contact site for different transcription activators in region 4 of the Escherichia coli RNA polymerase sigma(70) subunit. J. Mol. Biol. 284, 1353–1365. doi: 10.1006/jmbi.1998.2268
Mamlouk, D., and Gullo, M. (2013). Acetic acid bacteria: physiology and carbon sources oxidation. Indian J. Microbiol. 53, 377–384. doi: 10.1007/s12088-013-0414-z
Mientus, M., Kostner, D., Peters, B., Liebl, W., and Ehrenreich, A. (2017). Characterization of membrane-bound dehydrogenases of Gluconobacter oxydans 621H using a new system for their functional expression. Appl. Microbiol. Biotechnol. 101, 3189–3200. doi: 10.1007/s00253-016-8069-4
Paczia, N., Nilgen, A., Lehmann, T., Gatgens, J., Wiechert, W., and Noack, S. (2012). Extensive exometabolome analysis reveals extended overflow metabolism in various microorganisms. Microb. Cell Factories 11:122. doi: 10.1186/1475-2859-11-122
Paget, M. S. (2015). Bacterial sigma factors and anti-sigma factors: structure, function and distribution. Biomol. Ther. 5, 1245–1265. doi: 10.3390/biom5031245
Pappenberger, G., and Hohmann, H. P. (2014). Industrial production of L-ascorbic acid (vitamin C) and D-isoascorbic acid. Adv. Biochem. Eng. Biotechnol. 143, 143–188. doi: 10.1007/10_2013_243
Peters, B., Mientus, M., Kostner, D., Junker, A., Liebl, W., and Ehrenreich, A. (2013). Characterization of membrane-bound dehydrogenases from Gluconobacter oxydans 621H via whole-cell activity assays using multideletion strains. Appl. Microbiol. Biotechnol. 97, 6397–6412. doi: 10.1007/s00253-013-4824-y
Saito, Y., Ishii, Y., Hayashi, H., Imao, Y., Akashi, T., Yoshikawa, K., et al. (1997). Cloning of genes coding for L-sorbose and L-sorbosone dehydrogenases from Gluconobacter oxydans and microbial production of 2-keto-L-gulonate, a precursor of L-ascorbic acid, in a recombinant G. oxydans strain. Appl. Environ. Microb. 63, 454–460. doi: 10.1128/aem.63.2.454-460.1997
Sambrook, J., Fritsch, E. F., and Maniatis, T. (1989). Molecular Cloning: A Laboratory Manual. Cold Spring Harbor, NY: Cold Spring Harbor Laboratory Press.
Schweikert, S., Kranz, A., Yakushi, T., Filipchyk, A., Polen, T., Etterich, H., et al. (2021). FNR-type regulator GoxR of the obligatorily aerobic acetic acid bacterium Gluconobacter oxydans affects expression of genes involved in respiration and redox metabolism. Appl. Environ. Microbiol. 87, 87. doi: 10.1128/AEM.00195-21
Shaner, N. C., Lambert, G. G., Chammas, A., Ni, Y., Cranfill, P. J., Baird, M. A., et al. (2013). A bright monomeric green fluorescent protein derived from Branchiostoma lanceolatum. Nat. Methods 10, 407–409. doi: 10.1038/nmeth.2413
Simon, R., Priefer, U., and Pühler, A. (1983). A broad host range mobilization system for in vivo genetic-engineering – transposon mutagenesis in gram-negative bacteria. Nature Biotechnology 1, 784–791. doi: 10.1038/nbt1183-784
Tkac, J., Navratil, M., Sturdik, E., and Gemeiner, P. (2001). Monitoring of dihydroxyacetone production during oxidation of glycerol by immobilized Gluconobacter oxydans cells with an enzyme biosensor. Enzyme Microb. Technol. 28, 383–388. doi: 10.1016/S0141-0229(00)00328-8
Tobin, J. F., and Schleif, R. F. (1987). Positive regulation of the Escherichia coli L-rhamnose operon is mediated by the products of tandemly repeated regulatory genes. J. Mol. Biol. 196, 789–799. doi: 10.1016/0022-2836(87)90405-0
Vía, P., Badia, J., Baldoma, L., Obradors, N., and Aguilar, J. (1996). Transcriptional regulation of the Escherichia coli rhaT gene. Microbiology 142, 1833–1840. doi: 10.1099/13500872-142-7-1833
Wang, E. X., Ding, M. Z., Ma, Q., Dong, X. T., and Yuan, Y. J. (2016). Reorganization of a synthetic microbial consortium for one-step vitamin C fermentation. Microb. Cell Factories 15, 21. doi: 10.1186/s12934-016-0418-6
Wickstrum, J. R., and Egan, S. M. (2004). Amino acid contacts between sigma 70 domain 4 and the transcription activators RhaS and RhaR. J. Bacteriol. 186, 6277–6285. doi: 10.1128/JB.186.18.6277-6285.2004
Keywords: Gluconobacter, rhamnose, regulation, transcription, promoter, activation, repression, acetic acid bacteria
Citation: Fricke PM, Gries ML, Mürköster M, Höninger M, Gätgens J, Bott M and Polen T (2022) The L-rhamnose-dependent regulator RhaS and its target promoters from Escherichia coli expand the genetic toolkit for regulatable gene expression in the acetic acid bacterium Gluconobacter oxydans. Front. Microbiol. 13:981767. doi: 10.3389/fmicb.2022.981767
Edited by:
Isidoro García-García, University of Cordoba, SpainReviewed by:
Uwe Deppenmeier, University of Bonn, GermanyPaul Schweiger, University of Wisconsin–La Crosse, United States
Copyright © 2022 Fricke, Gries, Mürköster, Höninger, Gätgens, Bott and Polen. This is an open-access article distributed under the terms of the Creative Commons Attribution License (CC BY). The use, distribution or reproduction in other forums is permitted, provided the original author(s) and the copyright owner(s) are credited and that the original publication in this journal is cited, in accordance with accepted academic practice. No use, distribution or reproduction is permitted which does not comply with these terms.
*Correspondence: Tino Polen, t.polen@fz-juelich.de
†ORCID: Philipp Moritz Fricke https://orcid.org/0000-0002-3507-4881
Mandy Lynn Gries https://orcid.org/0000-0002-9345-5463
Maurice Mürköster https://orcid.org/0000-0002-3589-5065
Marvin Höninger https://orcid.org/0000-0003-3733-2677
Jochem Gätgens https://orcid.org/0000-0002-9232-180X
Michael Bott https://orcid.org/0000-0002-4701-8254
Tino Polen https://orcid.org/0000-0002-0065-3007