- 1Richter Lab, Basil Hetzel Institute for Translational Health Research, Department of Surgery, University of Adelaide, Adelaide, SA, Australia
- 2Department of Pharmaceutical Technology and Biopharmacy, Institute of Pharmaceutical Sciences, University of Freiburg, Freiburg, Germany
- 3Faculty of Health and Medical Sciences, Adelaide Medical School, University of Adelaide, Adelaide, SA, Australia
- 4Laboratory of Pharmaceutical Microbiology, Ghent University, Gent, Belgium
- 5Department of Preventive Dentistry, Academic Center for Dentistry Amsterdam (ACTA), University of Amsterdam and Vrije Universiteit Amsterdam, Amsterdam, Netherlands
- 6Precision Medicine Theme, South Australian Health & Medical Research Institute, Adelaide, SA, Australia
- 7Central Adelaide Local Health Network, Adelaide, SA, Australia
- 8Institute for Photonics and Advanced Sensing, University of Adelaide, Adelaide, SA, Australia
Staphylococcus aureus and Staphylococcus epidermidis are associated with life-threatening infections. Despite the best medical care, these infections frequently occur due to antibiotic resistance and the formation of biofilms of these two bacteria (i.e., clusters of bacteria embedded in a matrix). As a consequence, there is an urgent need for effective anti-biofilm treatments. Here, we describe the antibacterial properties of a combination treatment of diethyldithiocarbamate (DDC) and copper ions (Cu2+) and their low toxicity in vitro and in vivo. The antibacterial activity of DDC and Cu2+ was assessed in vitro against both planktonic and biofilm cultures of S. aureus and S. epidermidis using viability assays, microscopy, and attachment assays. Cytotoxicity of DDC and Cu2+ (DDC-Cu2+) was determined using a human fibroblast cell line. In vivo antimicrobial activity and toxicity were monitored in Galleria mellonella larvae. DDC-Cu2+ concentrations of 8 μg/ml DDC and 32 μg/ml Cu2+ resulted in over 80% MRSA and S. epidermidis biofilm killing, showed synergistic and additive effects in both planktonic and biofilm cultures of S. aureus and S. epidermidis, and synergized multiple antibiotics. DDC-Cu2+ inhibited MRSA and S. epidermidis attachment and biofilm formation in the xCELLigence and Bioflux systems. In vitro and in vivo toxicity of DDC, Cu2+ and DDC-Cu2+ resulted in > 70% fibroblast viability and > 90% G. mellonella survival. Treatment with DDC-Cu2+ significantly increased the survival of infected larvae (87% survival of infected, treated larvae vs. 47% survival of infected, untreated larvae, p < 0.001). Therefore, DDC-Cu2+ is a promising new antimicrobial with activity against planktonic and biofilm cultures of S. epidermidis and S. aureus and low cytotoxicity in vitro. This gives us high confidence to progress to mammalian animal studies, testing the antimicrobial efficacy and safety of DDC-Cu2+.
Introduction
The Gram-positive bacteria Staphylococcus aureus and Staphylococcus epidermidis are notable human pathogens, causing infections ranging from mild skin infection to life-threatening bacteremia (Kleinschmidt et al., 2015; López-Cortés et al., 2020), endocarditis (Cahill et al., 2017), osteoarticular (Kaushik and Kest, 2018) and medical device related infections (Otto, 2008; Zheng et al., 2018; Patiniott et al., 2022). Furthermore, S. aureus is the most common pathogen isolated from surgical site infections (Tong et al., 2015). Typically, a bacterial infection is treated with antibiotics (Stevens et al., 2005), e.g., intervention against S. aureus infections is executed with either β-lactams, lincosamides, lipopeptides, tetracyclines, glycopeptides, linezolid, or adjunct trimethoprim-sulfamethoxazole therapy (Liu et al., 2011). However, these therapies are frequently failing due to the rise of antibiotic resistance and the formation of biofilms (Santajit and Indrawattana, 2016).
Biofilms are aggregates of bacteria embedded in a protective matrix (Costerton et al., 1999) and are known to be up to 1,000-fold more tolerant to antimicrobial agents compared to planktonic cells (Mah and O’Toole, 2001). The biofilm matrix, a conglomeration of extracellular polymeric substances, prevents diffusion of the drug and modulates or reduces their metabolic activity (Crabbé et al., 2019). In addition, staphylococci developed penicillin-resistance, including methicillin-resistant S. aureus (MRSA) with rates varying between 1.5 and over 50% in different parts of the world (Australian Commission on Safety and Quality in Health Care (ACSQHC), 2019; Craft et al., 2019; European Centre for Disease Prevention and Control, 2022) and methicillin-resistant S. epidermidis with reported rates over 70% (Lee et al., 2018). The implications of antimicrobial resistance are devastating, as exemplified by MRSA-associated surgical site infections, which is associated with 2- to 11-fold increased patient mortality (Anderson et al., 2007). Therefore, S. aureus is listed as a high priority pathogen for research and development by the World Health Organization, emphasizing the urgency for new treatments (World Health Organization, 2017).
Innovative strategies against S. aureus and S. epidermidis in the research and development pipeline include newly synthesized compounds (Dinarvand et al., 2020; Sovari et al., 2020; Wang et al., 2021), bacteriophages (Feng et al., 2021; Walsh et al., 2021), metals (Richter et al., 2017a; Sánchez-López et al., 2020) and repurposed drugs (Thangamani et al., 2015; Richter, 2019). Repurposing of drugs has a history of multiple benefits and safe uses, allowing for a faster bench to bedside translation and lower drug development costs (Pushpakom et al., 2018).
An excellent candidate for drug repurposing is diethyldithiocarbamate (DDC). DDC is the metabolite of disulfiram, an FDA-approved drug for the treatment of chronic alcoholism, which have both recently resurfaced as potentially useful in other medical fields, such as cancer, cocaine addiction, or infections with fungi, parasites, viruses and bacteria (Kaul et al., 2021). DDC showed high antifungal activity against Candida albicans and Candida tropicalis biofilms (Harrison et al., 2007), reduced the load of Leishmania braziliensis (Khouri et al., 2010; Celes et al., 2016) and, in combination with copper ions (Cu2+), showed anti-SARS-CoV-2 activity by targeted oxidation strategies (Xu et al., 2021). The suggested mechanisms behind the antimicrobial activity of DDC is based on chelating vital metals and inhibiting enzymes (Phillips et al., 1991), such as the carbonic anhydrases present in Legionella pneumophila (Nishimori et al., 2014) or the superoxide dismutase present in Candida albicans (De Brucker et al., 2013), Leishmania braziliensis (Khouri et al., 2010) or Bacillus anthracis (Frazier et al., 2019). An additional advantage of DDC is a lack of teratogenic, mutagenic or carcinogenic effects in animal models (Gessner and Gessner, 1992).
Based on the anti-cancer activity of DDC being linked to the addition of Cu2+ and on limited activity against Gram-positive bacteria of DDC as monotherapy, DDC was combined with copper ions (Cu2+) and showed promising results against mycobacteria and streptococci (Dalecki et al., 2015; Menghani et al., 2021). However, the combination of DDC with metal ions, such as Cu2+ has not been further investigated against staphylococci and their biofilms. Thus, this study presents the antibacterial activity of DDC and Cu2+ against planktonic and biofilm S. aureus and S. epidermidis including in vivo safety and efficacy in an infected Galleria mellonella model.
Materials and methods
Bacterial strains and cell cultures
Staphylococcus epidermidis ATCC 35984 and ATCC 14990, S. aureus ATCC 25923 and ATCC 700699 (also known as MRSA Mu50), and Escherichia coli ATCC 25922 were purchased from the American Type Culture Collection (Manassas, VA, United States). Three clinical isolates, i.e., MRSA 1, 2 and 3 were obtained from Adelaide Pathology Partners (Mile End, Australia). Pseudomonas aeruginosa PAO1 was obtained from the School of Molecular Medical Sciences, University of Nottingham (Nottingham, United Kingdom). Unless stated otherwise, bacterial suspensions were prepared by dissolving colonies in 0.9% saline and adjusted to the appropriate McFarland units before being further diluted in broth and incubated at 37°C under aerobic conditions. Cell culture studies were carried out using control human fibroblast cells (Coriell Cat# GM00038, RRID: CVCL_7271) obtained from the Coriell Institute for Medical Research (Camden, NJ, United States). Unless stated otherwise, all experiments were carried out at least in triplicate and all chemicals, media and supplements were purchased from Sigma-Aldrich (Steinheim, Germany).
Minimal inhibitory concentration and checkerboard analysis
The MIC values of DDC (Carl Roth, Karlsruhe, Germany) and the antibiotics methicillin (Meth), ceftazidime (Ceft), vancomycin (Van), ciprofloxacin (Cip), doxycycline (Doxy), amikacin (Amik) and erythromycin (Erythro) towards the staphylococci S. aureus and S. epidermidis and the Gram-negative bacteria E. coli and P. aeruginosa were determined in a 96-well microtiter plate using the broth microdilution method (Wiegand et al., 2008). Bacterial suspensions were adjusted to 0.5 ± 0.1 McFarland units, further 1: 100 diluted in Mueller-Hinton broth (Thermo Fisher) and mixed with equal volumes of treatments or antibiotics. Treatment concentrations of DDC ranged from 0.5 to 128 μg/ml and for antibiotics from 0.125 to 64 μg/ml. Furthermore, the broth microdilution method was adapted to investigate the MIC of gallium nitrate hydrate (Ga3+), iron sulphate heptahydrate (Fe2+), calcium chloride dihydrate (Ca2+), magnesium sulphate (Mg2+), zinc sulphate heptahydrate (Zn2+) and copper sulphate pentahydrate (Cu2+) alone or in combination with DDC. The MIC was determined as the lowest concentration of treatment required to inhibit visual growth by the unaided eye (Wiegand et al., 2008).
Biofilm checkerboard assay
Black 96-well microtiter plates (Costar, Corning Incorporated, NY, United States) were inoculated with 100 μl of a 1: 100 diluted S. aureus, MRSA or S. epidermidis bacterial suspension in nutrient broth, adjusted to 0.5 ± 0.1 McFarland units, and incubated at 37°C for 24 h on a rotating platform at 70 rpm (3D Gyratory Mixer, Ratek Instruments, Boronia, Australia). After washing once with sterile 0.9% w/v saline to remove planktonic bacteria, biofilms were exposed to serial dilutions of (i) 1 to 256 μg/ml DDC, (ii) 4 to 256 μg/ml Cu2+, (iii) mixture of DDC and Cu2+, (iv) antibiotics with concentrations ranging from 0.5 to 128 μg/ml, including Meth, Ceft, Van, Cip, Doxy, Amik and Erythro or (v) a mixture of DDC-Cu2+ and antibiotics, and further incubated at 37°C on a rotating platform for 24 h. After a second washing step to remove the treatments, bacterial viability was assessed by the AlamarBlue cell viability assay (Peeters et al., 2008; Richter et al., 2016). Briefly, 100 μl of a freshly prepared 10% v/v AlamarBlue (Thermo Fisher, MA, United States) solution in nutrient broth (Thermo Fisher) were added to each well and incubated, protected from light, for up to 5 h at 37°C on a rotating platform. The fluorescence was determined hourly using a FLUOstar OPTIMA plate reader (BMG LABTECH, Offenburg, Germany) at λexcitation/λemission = 530/590 nm. After reaching maximum fluorescence the relative biofilm killing efficacy was quantified according to Equation 1.
Antibiofilm activity of the different treatments was determined as percentage of biofilm killing, where the fluorescence intensity of treated and untreated biofilms is represented by Itreatment and Iuntreated, respectively, and Iblank represents the background fluorescence of the 10% v/v AlamarBlue solution (Richter et al., 2016).
Synergy of compounds
The fractional inhibitory concentration index (FICi) was used to describe synergistic, additive, and antagonistic effects between DDC and Cu2+, or between DDC-Cu2+ and antibiotics. The equation for calculating the sum of FICi (ΣFICi) is based on the planktonic and biofilm checkerboard assay and exemplified for planktonic bacteria in Equation 2 using the MICs.
MICab = MIC of compound a in combination with b; MICa = MIC of compound a; MICba = MIC of compound b in combination with a; MICb = MIC of compound b (Khouri et al., 2010). Similarly, the equation for biofilms was adapted by replacing the MIC with the minimum biofilm inhibitory concentration, correlating to a minimum of 80% biofilm killing. According to previous literature, the ΣFICi was interpreted as: (i) synergy; ΣFICi ≤0.5, (ii) additivity; ΣFICi between 0.5 and 1, (iii) indifference; ΣFICi ≥1 and ≤4, and (iv) antagonism; ΣFICi ≥4 (Sopirala et al., 2010).
Confocal microscopy
An 8-well chamber slide (μ-Slide, Ibidi, Gräfelfing, Germany) was inoculated with 300 μl of a 1: 100 dilution of a bacterial suspension of MRSA Mu50 or S. epidermidis ATCC 35984 adjusted to 0.5 ± 0.1 McFarland units in nutrient broth and incubated for 24 h at 37°C on a rotating platform at 70 rpm (3D Gyratory Mixer, Ratek Instruments, Boronia, Australia). Biofilms were rinsed with phosphate buffered saline, followed by exposure to DDC-Cu2+ (8 μg/ml DDC + 32 μg/ml Cu2+) or nutrient broth alone for 24 h at 37°C on a rotating platform. After a second washing step, a 1: 1000 dilution of LIVE/DEAD BacLight staining (SYTO 9/propidium iodide; Life Technologies, Scoresby, Australia) was incubated in the dark for 30 min, then imaged by confocal laser scanning microscopy (Olympus FV3000, Shinjuku, Japan) using a 20 × and 100 × objective. The excitation/emission wavelengths of the LIVE/DEAD BacLight staining were 488/520 nm and 543/619 nm, respectively. The images were quantified using ImageJ Software (1.53q, NIH, University of Wisconsin, WI, United States). Due to the number of layers of cells in the biofilm and the magnification objective, live/dead cell count was not possible. Instead, measurement of total red and green fluorescence ratio was used to semi-quantitatively calculate the live/dead cell ratio.
Prevention of bacterial attachment
The activity of DDC-Cu2+ to inhibit bacterial attachment was determined using the xCELLigence real-time cell analysis (RTCA; Agilent, CA, United States). This technology measures the impedance through gold electrode sensors placed on the bottom of each well of the RTCA E-plate 16 (Agilent, CA, United States). When cells attach onto the electrodes, a larger impedance is detected, leading to an increase of the cell index (CI) compared to the baselines.
To measure the baselines, 50 μl of nutrient broth and 100 μl of 8 μg/ml DDC, 32 μg/ml Cu2+, DDC-Cu2+ (8 μg/ml DDC + 32 μg/ml Cu2+) dissolved in nutrient broth or media alone were added to each well. A bacterial overnight culture in nutrient broth was adjusted to OD600 of 0.4 for MRSA Mu50 and S. epidermidis ATCC 35984. A 1: 4 dilution of the bacterial suspension was added to the appropriate wells. The impedance of the cells was continuously and automatically measured every 15 min for 48 h while statically incubated at 37°C. Wells with bacterial suspension in broth (100% bacterial attachment), wells with broth alone (background) and wells with compounds in broth (0% bacterial attachment, reflecting the compounds’ influence on impedance) were assessed as controls.
Bioflux
The Bioflux system (Fluxion, United States) was used to determine inhibition of biofilm growth under flow conditions, as previously described (Hoogenkamp, 2021). All media was pre-warmed to 37°C before use. Bioflux plates were primed with 350 μl half-strength tryptone soy broth (TSB, BD, Sparks, MD, United States) and inoculated with 70 μl of a bacterial overnight culture (either MRSA Mu50 or S. epidermidis ATCC 35984) adjusted to OD600 of 0.2. Following bacterial attachment for 30 min at 37°C and no flow, bacteria were exposed to either half-strength TSB or half-strength TSB supplemented with DDC-Cu2+ (8 μg/ml DDC + 32 μg/ml Cu2+) for 24 h at 37°C under steady nutrient flow (0.5 dyne/cm2). Biofilm growth was monitored through brightfield microscopy (20× objective), and images were automatically taken every 15 min.
In vitro cytotoxicity
The GM00038 normal human skin fibroblast cell line was cultured in Eagle’s Minimum Essential Medium with Earle’s salts and non-essential amino acids supplemented with 15% fetal bovine serum (Biochrom, Berlin, Germany) and 2.2 g/l sodium bicarbonate anhydrous. Fibroblasts were seeded at 5 × 104 cells/100 μl culture medium per well in black 96-well flat-bottom plates and incubated at 37°C in 5% CO2 for 24 h to allow attachment. Cells were separately treated with either 8 μg/ml DDC, 32 μg/ml Cu2+ or DDC-Cu2+ (8 μg/ml DDC + 32 μg/ml Cu2+) for 18 h. The effect of the compounds on fibroblast viability was assessed with the CellTiter-Glo® Luminescent Viability Assay (Promega Corporation, WI, United States) according to the manufacturer’s instructions and luminescence was measured on a FLUOstar OPTIMA plate reader. Equation 3 was used to quantify the percentage of fibroblast viability, where the luminescence intensity of treated and untreated fibroblast cells is represented by Itreatment and Iuntreated, respectively, and Iblank represents the background luminescence of the CellTiter-Glo® reagent.
In vivo cytotoxicity and efficacy
Galleria mellonella larvae (Hengelsport De Poorterwere, Ghent, Belgium) were stored in the dark at 13°C and used within 3 days of receipt. Each treatment group was assigned 30 larvae. Larvae were injected in the last proleg with micro-fine (30 gauge) needle insulin syringes (BD, Franklin Lakes, NJ, United States). Three control groups were included, (i) larvae injected with 0.9% saline (uninfected, untreated control), (ii) larvae injected with treatment (uninfected, treated control to determine treatment toxicity) and (iii) larvae injected with a bacterial suspension (infected, untreated control). To determine treatment efficacy, larvae were injected with a bacterial suspension (either MRSA Mu50 or S. epidermidis ATCC 35984) and with DDC, Cu2+or DDC-Cu2+. Considering the dilution factor within the larvae, the concentrations of the DDC-Cu2+ were increased a 10-fold and based on the average weight of the larvae (250 mg) was determined as 6.4 mg/kg DDC and 25.6 mg/kg. A total volume of 20 μl was injected comprising treatment or saline in a 1:1 mix with a bacterial suspension in nutrient broth. The final bacterial density was OD600 0.05. Larvae were housed in petri dishes in the dark at 37°C and the larvae mortality was monitored daily over 4 days.
Statistical analysis
Results were statistically analyzed using GraphPad Prism (RRID:SCR_002798) version 9.00 for Windows (GraphPad Software, CA, United States) and statistical significance was determined with an α = 0.05. Parametric data (MIC, biofilm killing and cytotoxicity) are represented by the mean ± standard deviation (SD), which was analyzed using one-way analysis of variance (ANOVA) with Dunnett’s (for MICs, biofilm checkerboard, microscopy) or Tukey’s (for xCELLigence) multiple comparison test for finding statistical differences between treatment groups. G. mellonella survival data was analyzed using Kaplan–Meier survival curves with significant differences between groups determined by log-rank test, significance was Bonferroni-Holm-corrected for multiple comparisons.
Results
Minimal inhibitory concentration
As shown in Table 1, DDC displayed low antibacterial activity against S. epidermidis ATCC 35984 with a MIC of 64 μg/ml. To increase the antibacterial activity of DDC, a selection of metal salts was evaluated against S. epidermidis ATCC 35984 in the presence or absence of DDC. The MIC of the metal salts alone was 128 μg/ml for Cu2+ and above 128 μg/ml for all other metal ions (Table 1). In combination with Ga3+, Fe2+ and Ca2+, the MIC of DDC was not reduced. In contrast, the MIC of DDC was reduced to 16 μg/ml in the presence of Mg2+ and Zn2+ and to 1 μg/ml when combined with Cu2+ (Table 1).
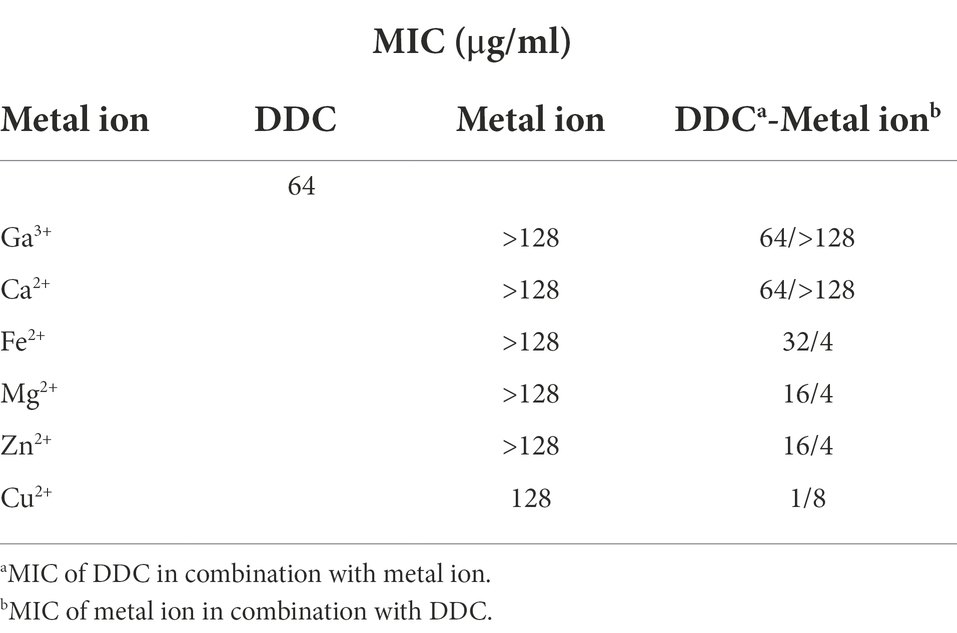
Table 1. Minimum inhibitory concentration (MIC) of diethyldithiocarbamate (DDC), metal ions and the combination of both against S. epidermidis ATCC 35984.
Since the DDC combination with Cu2+ resulted in a substantial MIC reduction in S. epidermidis ATCC 35984, the MIC of DDC in the presence or absence of Cu2+ was further investigated in a range of bacteria. In S. aureus, MRSA and S. epidermidis, the MICs of DDC ranged from 32 to 128 μg/ml. The MIC of DDC against E. coli and P. aeruginosa was above 128 μg/ml. The extensive MIC reduction of DDC in the presence of Cu2+ was also observed with other S. aureus, MRSA and S. epidermidis strains (Table 2). Both the MIC of DDC in the presence of Cu2+ and the MIC of Cu2+ in the presence of DDC were reduced in all S. aureus and S. epidermidis strains tested. Interestingly, the MIC values of the combination were the highest with 4 μg/ml DDC and 64 μg/ml Cu2+ in S. aureus ATCC 25923, the most antibiotic susceptible strain, while the MIC values of the combination were lowest, with 0.5 μg/ml DDC and 2 μg/ml Cu2+ in MRSA 2 and MRSA Mu50, the strain with the highest antibiotic MICs. In all strains tested, the lowest concentration of Cu2+ required to inhibit S. aureus and S. epidermidis growth exceeded the lowest DDC concentration.
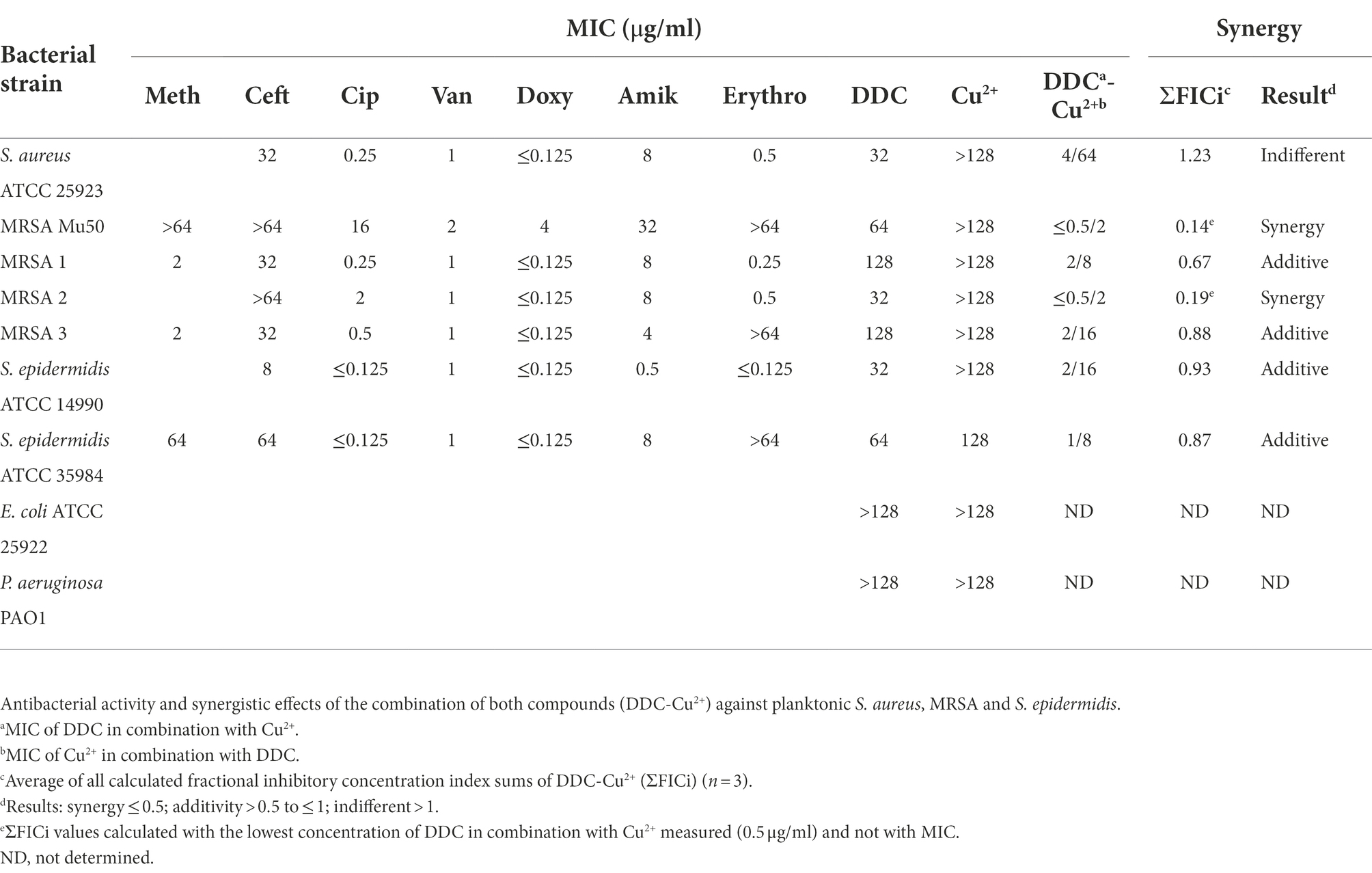
Table 2. Minimal inhibitory concentration of the antibiotics methicillin (Meth), ceftazidime (Ceft), ciprofloxacin (Cip), vancomycin (Van), doxycycline (Doxy), amikacin (Amik), erythromycin (Erythro) and the compounds diethyldithiocarbamate (DDC) and Cu2+ towards planktonic S. aureus, MRSA, S. epidermidis, E. coli and P. aeruginosa.
Effect of different DDC and Cu2+ concentrations on biofilms
MRSA and S. epidermidis biofilms were exposed to combined treatments of DDC (1 to 256 μg/ml) and Cu2+ (4 to 256 μg/ml). In Figure 1, the MRSA Mu50, MRSA 2, S. epidermidis ATCC 35984 and S. epidermidis ATCC 14990 biofilm killing of different DDC and Cu2+ combination (DDC-Cu2+) ratios were compared to the effect of single Cu2+ treatment. Overall, treatment with DDC alone, Cu2+ alone and combinations involving Cu2+ concentrations below 16 μg/ml resulted in low antibiofilm activity against S. aureus and S. epidermidis with less than 31.2% biofilm killing, except for Cu2+ 256 μg/ml against S. epidermidis ATCC 14990 resulting in 70.8% biofilm killing (Figure 1D). The highest biofilm killing was 95.8, 99.6, 99.3 and > 99.9% with 256 μg/ml Cu2+ in combination with 8 μg/ml DDC in MRSA Mu50 (Figure 1A), MRSA 2 (Figure 1B), S. epidermidis ATCC 35984 (Figure 1C) and S. epidermidis ATCC 14990 (Figure 1D), respectively. The minimal concentrations of DDC-Cu2+ that resulted in above 80.0% biofilm killing were 8 μg/ml DDC and 16 μg/ml Cu2+ in MRSA Mu50 (81.0% biofilm killing, p ≤ 0.001; Figure 1A), 4 μg/ml DDC and 32 μg/ml Cu2+ in MRSA 2 (98.6% biofilm killing, p ≤ 0.001, Figure 1B), 4 μg/ml DDC and 16 μg/ml Cu2+ in S. epidermidis ATCC 35984 (85.0% biofilm killing; p ≤ 0.001; Figure 1C) and 8 μg/ml DDC and 32 μg/ml Cu2+ in S. epidermidis ATCC 14990 (83.7% biofilm killing, p ≤ 0.01; Figure 1D). Complementing the results obtained against planktonic MRSA and S. epidermidis, low antibiofilm activity was observed when DDC concentrations exceeded Cu2+ concentrations, suggesting the importance of a DDC-Cu2+ ratio range. The lowest concentration of DDC and Cu2+ with over 80.0% biofilm killing in all strains tested was 8 μg/ml and 32 μg/ml Cu2+, therefore this concentration was chosen for further experiments. This concentration was also effective against S. aureus ATCC 25923, MRSA 1 and MRSA 3 biofilms (data not shown).
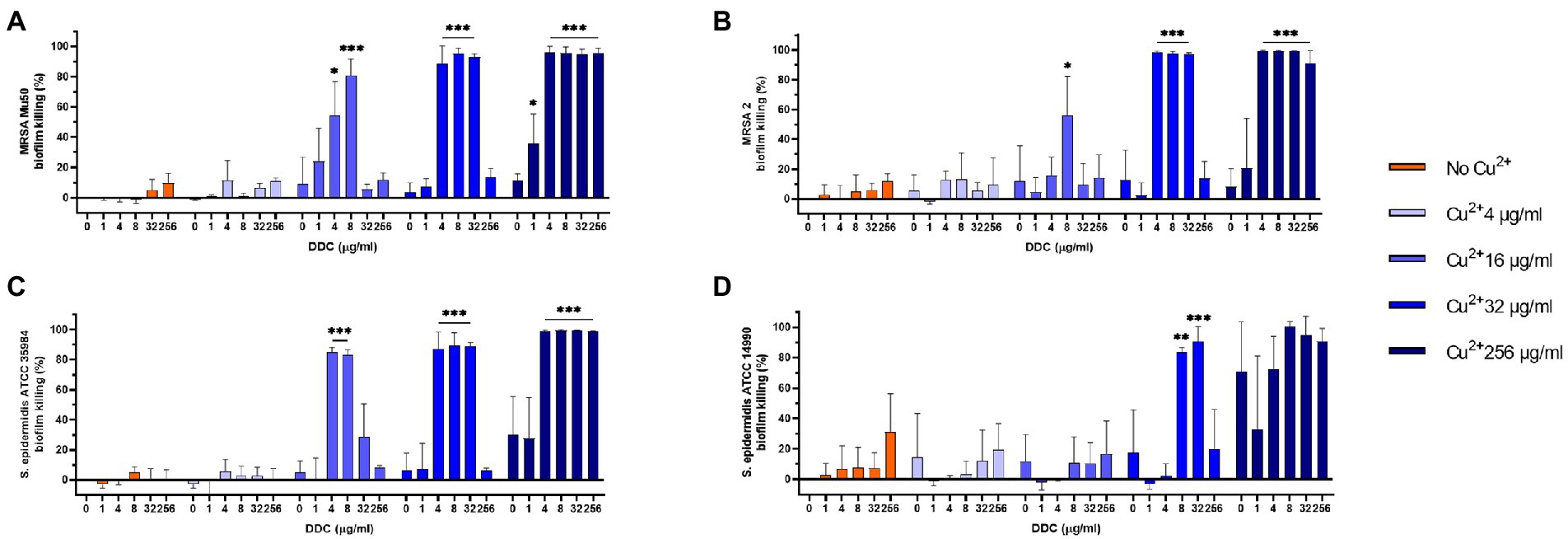
Figure 1. Effect of diethyldithiocarbamate (DDC) and Cu2+ concentrations (in μg/ml) on the viability of (A) MRSA Mu50, (B) MRSA 2, (C) Staphylococcus epidermidis ATCC 35984 and (D) S. epidermidis ATCC 14990 biofilms compared to monotherapy with Cu2+ (n = 3; *p < 0.05; **p < 0.01; ***p < 0.001).
Synergistic effects of DDC and Cu2+ in combination with different antibiotics
Synergistic and additive effects of DDC and Cu2+ were observed against all planktonic MRSA (ΣFICi: MRSA Mu50 = 0.14; MRSA 1 = 0.67; MRSA 2 = 0.19; MRSA 3 = 0.88) and S. epidermidis strains (ΣFICi: S. epidermidis ATCC 14990 = 0.93; S. epidermidis ATCC 35984 = 0.63), except for S. aureus ATCC 25923 (ΣFICi = 1.23; Table 2). Against the biofilm form of the same strains, the ΣFICi of DDC-Cu2+ was reduced in most strains (Table 3). Synergistic effects of the combination were reached against MRSA Mu50 (ΣFICi = 0.26), and additive effects were reached against both S. epidermidis strains (ΣFICi: S. epidermidis ATCC 14990 = 0.86; S. epidermidis ATCC 35984 = 0.58), S. aureus ATCC 25923 (ΣFICi = 0.80) and the other MRSA strains (ΣFICi: MRSA 1 = 0.53; MRSA 2 = 0.64; MRSA 3 = 0.66). The synergistic effects of DDC-Cu2+ in planktonic MRSA 2 and planktonic and biofilm MRSA Mu50 were not observed in the other MRSA strains tested, which showed additive effects of DDC-Cu2+. This difference should be investigated based on the phenotype and genotype of the different strains tested. As the MICs of multiple antibiotics were the highest for MRSA Mu50 and S. epidermidis ATCC 35984, respectively, these strains were chosen as representatives for S. aureus and S. epidermidis in the following experiments.
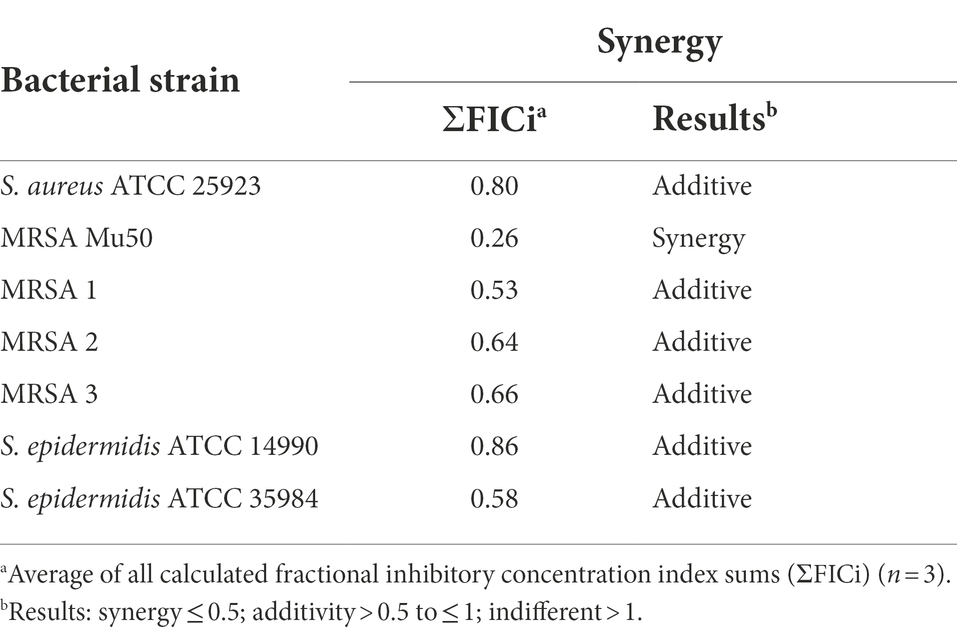
Table 3. Synergistic effects of diethyldithiocarbamate in combination with Cu2+ against S. aureus, MRSA and S. epidermidis biofilms.
The ΣFICi of the DDC-Cu2+ combination was further investigated with representatives of different classes of antibiotics against MRSA Mu50 biofilms (Table 4). The MRSA Mu50 strain was chosen based on the high antibiotics MICs in the planktonic form and on the biofilms not inhibited by antibiotics at concentrations of 128 μg/ml or lower, except for the tetracycline representative Doxy and the cell wall synthesis inhibitor Van (over 70% biofilm killing with concentrations of 16 μg/ml). When the antibiotics were combined with DDC-Cu2+, the minimum concentration to kill at least 80% of bacteria within the biofilm, was reduced at least 16-fold, except for the combination of Erythro with DDC-Cu2+ (no change). In addition, DDC-Cu2+ showed additive effect with Amik and the β-lactam antibiotics Meth and Ceft. Synergistic effects were observed when DDC-Cu2+ was combined with Cip, Doxy, and Van. However, no difference was observed with Erythro.
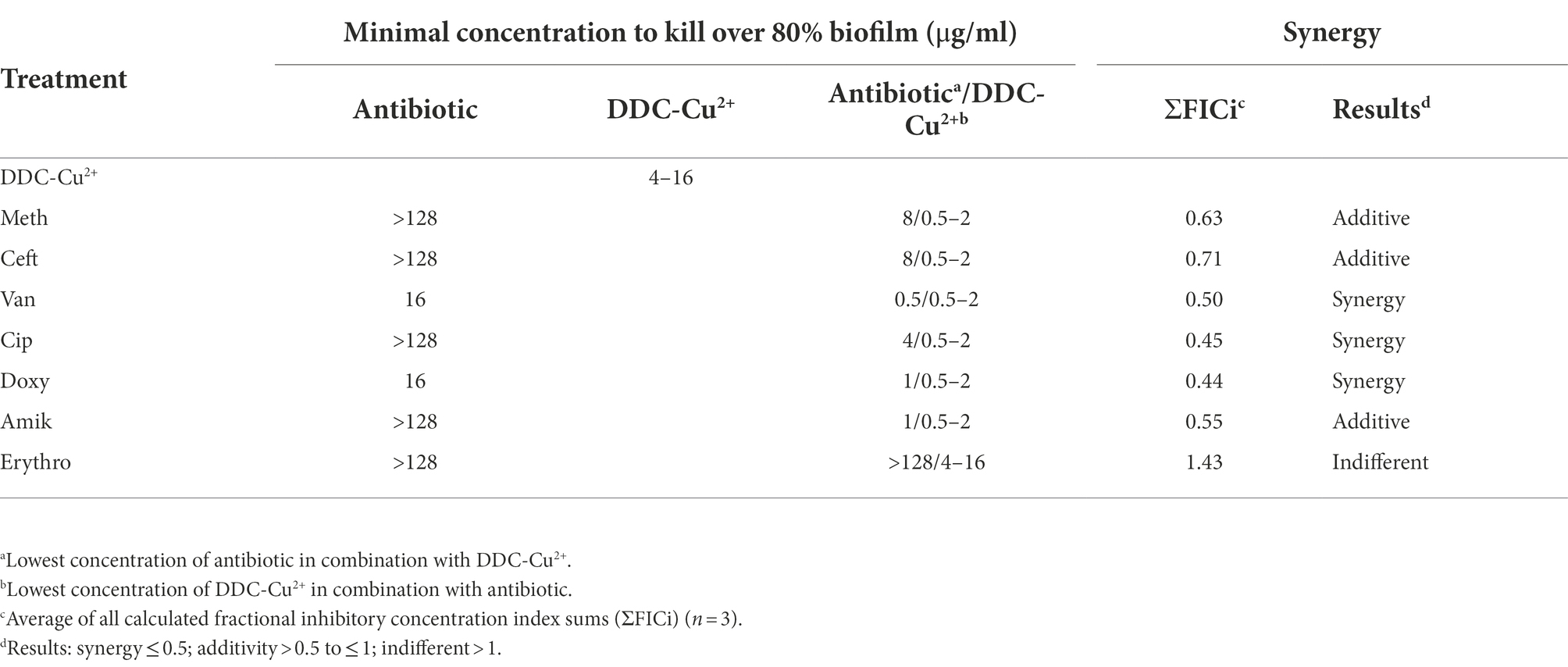
Table 4. Minimal concentration to kill over 80% biofilm and synergistic effects of antibiotics, diethyldithiocarbamate and Cu2+ (DDC-Cu2+) and the combination against MRSA Mu50 (n = 3).
Visualizing biofilms after DDC-Cu2+ treatment
Confocal microscopy images of the untreated control of S. epidermidis ATCC 35984 biofilms were characterized by a large, dense, and undisturbed biofilm with mostly viable bacteria (Figure 2A). After exposure to DDC-Cu2+ (8 μg/ml DDC + 32 μg/ml Cu2+), the biofilm structure was disturbed and less dense. In addition, an increase in number of red, indicating dead bacteria was observed (Figure 2B). Similar observations were made in MRSA Mu50 biofilm images (Supplementary Figure 1). The quantification of the fluorescence showed a significant decrease of the green/red ratio between untreated biofilm and biofilm treated with DDC-Cu2+ (Figure 2C). This ratio was also observed when using a 100 × objective on a DDC-Cu2+ treated S. epidermidis ATCC 35984 biofilm that showed dead bacteria with only few viable bacteria (Figure 2D).
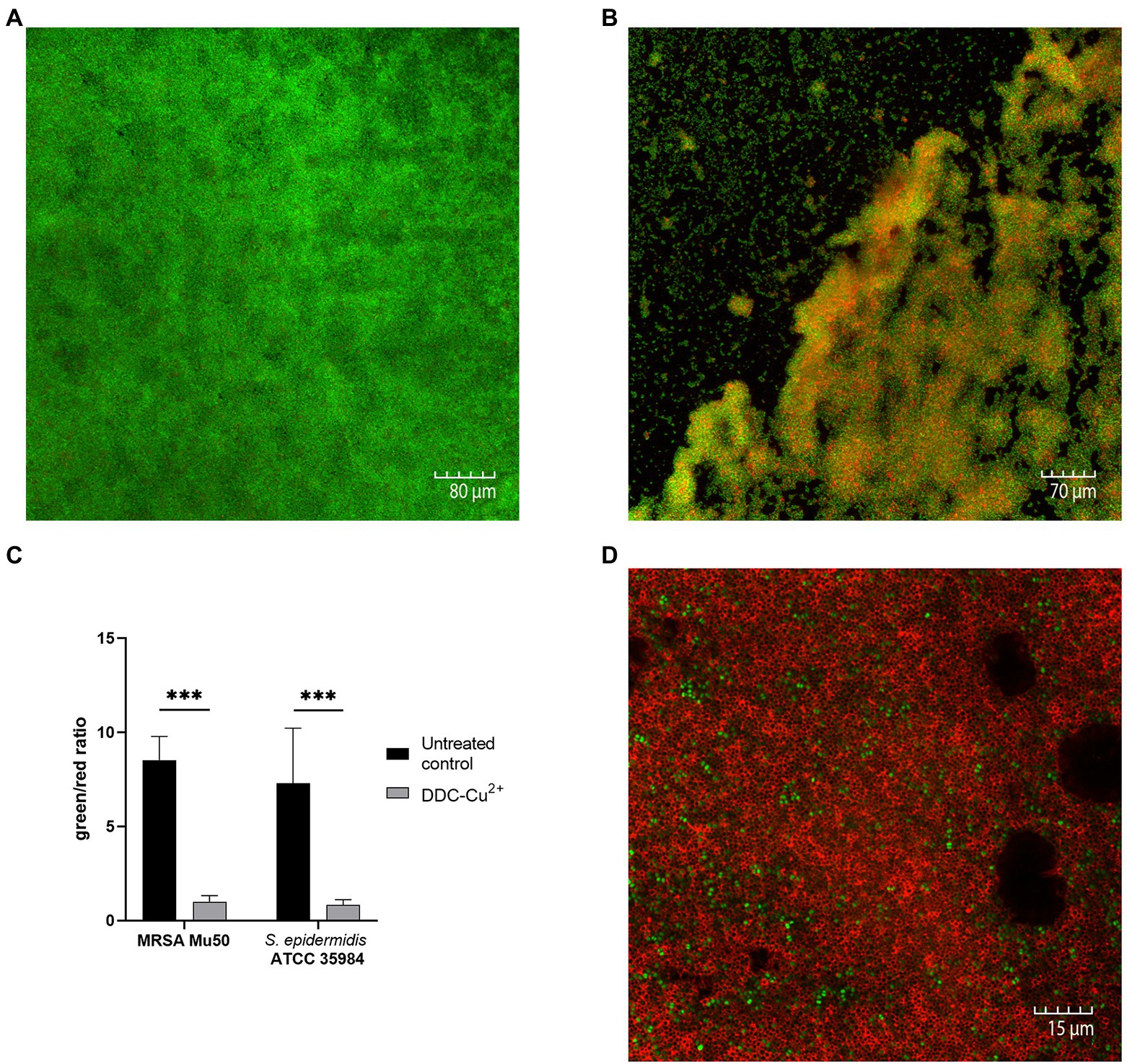
Figure 2. Comparison of stained MRSA Mu50 and S. epidermidis ATCC 35984 biofilms with LIVE/DEAD BacLight staining after treatment with 8 μg/ml diethyldithiocarbamate and 32 μg/ml Cu2+ (DDC-Cu2+). Confocal microscopy images results: green = viable bacteria; red = dead bacteria. (A) Untreated S. epidermidis ATCC 35984 biofilm at 20×. S. epidermidis ATCC 35984 biofilm after treatment with DDC-Cu2+ at (B) 20× and (D) 100×. (C) Quantification of images as green/red ratio of untreated control (black) and treatment with DDC-Cu2+ (grey) of MRSA and S. epidermidis ATCC 35984 biofilms (n = 3–8; ***p < 0.001).
DDC-Cu2+ inhibits bacterial attachment
Prevention of biofilm growth was examined in MRSA Mu50 (Figures 3A,B) and S. epidermidis ATCC 35984 (Figures 3C,D) with the xCELLigence RTCA system over 48 h. A high cell index (CI) correlates with bacteria attaching to the gold electrodes located at the bottom of the well (Abrantes and Africa, 2020). For both S. aureus and S. epidermidis, the untreated control showed a high increase in CI within the first 12 h, reaching a CI of 0.32 in MRSA Mu50 (Figure 3A) and 0.25 in S. epidermidis ATCC 35984 (Figure 3C), before steadily increasing at a slower rate to reach 0.5 in MRSA Mu50 and 0.45 in S. epidermidis ATCC 35984 after 48 h. Monotherapy of DDC (8 μg/ml) and Cu2+ (32 μg/ml) resulted in a faster CI increase compared to the untreated control, reaching a maximum after 5 h in MRSA Mu50 (CI: DDC = 0.40; Cu2+ = 0.19) and 6 h in S. epidermidis ATCC 35984 (CI: DDC = 0.35; Cu2+ = 0.18). The fast CI increase of DDC or Cu2+ treated bacteria should not be a result of DDC or Cu2+ salts interacting with the gold electrodes or the impedance, as these were assessed with the baselines. The initial increased bacterial attachment when treated with DDC or Cu2+ can be explained by the subinhibitory concentration of DDC or Cu2+ alone used in this experiment. Treatment with DDC and Cu2+ can induce oxidative stress and the production of reactive oxygen species in S. aureus, which play a role in the control of different cellular processes, such as biofilm formation (Seixas et al., 2021). Treatment with DDC alone showed no significant difference from the mean CI (12–48 h) compared to the untreated control (CI: 0.44 MRSA Mu50 and 0.38 S. epidermidis ATCC 35984). Treatment with Cu2+ alone resulted in approximately half the CI compared to untreated control (CI: 0.22 MRSA Mu50 and 0.18 S. epidermidis ATCC 35984), translating in less bacteria attaching to the bottom of the well and forming biofilms. Lastly, treatment with DDC-Cu2+ (8 μg/ml DDC + 32 μg/ml Cu2+) resulted in a CI of 0 after 12 h and a mean CI of 0.04 and 0.03 after 48 h in MRSA Mu50 (Figure 3B) and S. epidermidis ATCC 35984 (Figure 3D), respectively. Therefore, treatment with DDC-Cu2+ prevented the attachment of bacteria over 48 h, which can be a result of high bacterial killing at the tested concentrations. To determine if bacterial killing was responsible for prevention of bacterial attachment, lower DDC-Cu2+ concentrations can be investigated.
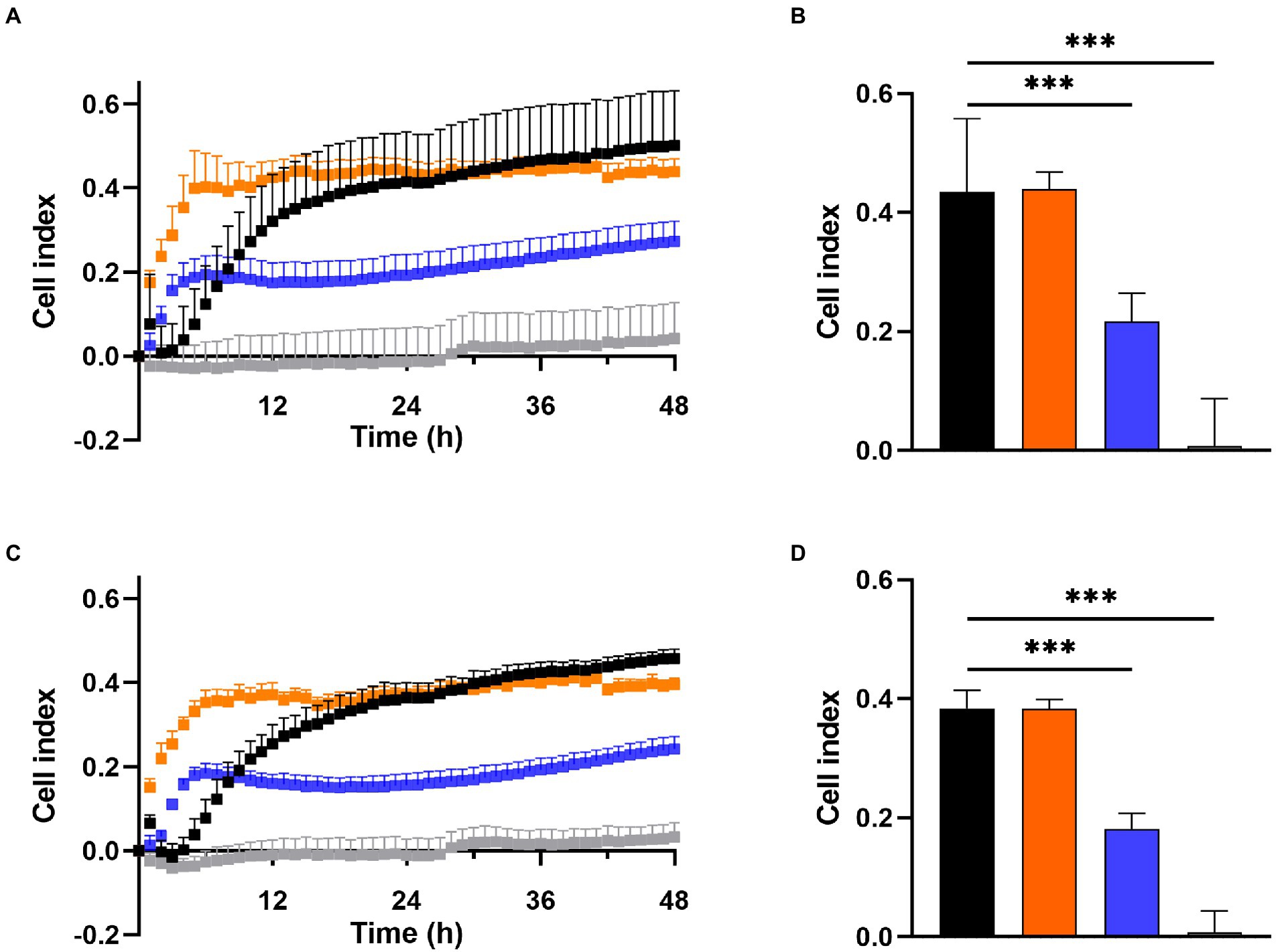
Figure 3. Effect of 8 μg/ml diethyldithiocarbamate (DDC; orange), 32 μg/ml Cu2+ (blue) and combined DDC-Cu2+ (grey) on (A) the cell index of MRSA Mu50 and (C) S. epidermidis ATCC 35984 over 48 h compared to the untreated control (black). Comparison of the mean cell index between 12 and 48 h for each treatment of (B) MRSA Mu50 and (D) S. epidermidis ATCC 35984 (n > 3; ***p < 0.001).
DDC-Cu2+ inhibits biofilm growth
Similar results were observed with the Bioflux system (Figure 4). In the untreated control, under constant nutrient flow, MRSA Mu50 bacteria started to aggregate within 8 h, formed biofilms within 16 h that continuously increased in size within 24 h (Figure 4, top time lapse). When DDC-Cu2+ was added to the constant nutrient flow, inhibition of biofilm growth was achieved over 24 h (Figure 4; bottom time lapse. Supplementary File 1: Video footage). Similar observations were made in S. epidermidis ATCC 35984 biofilms (data not shown).
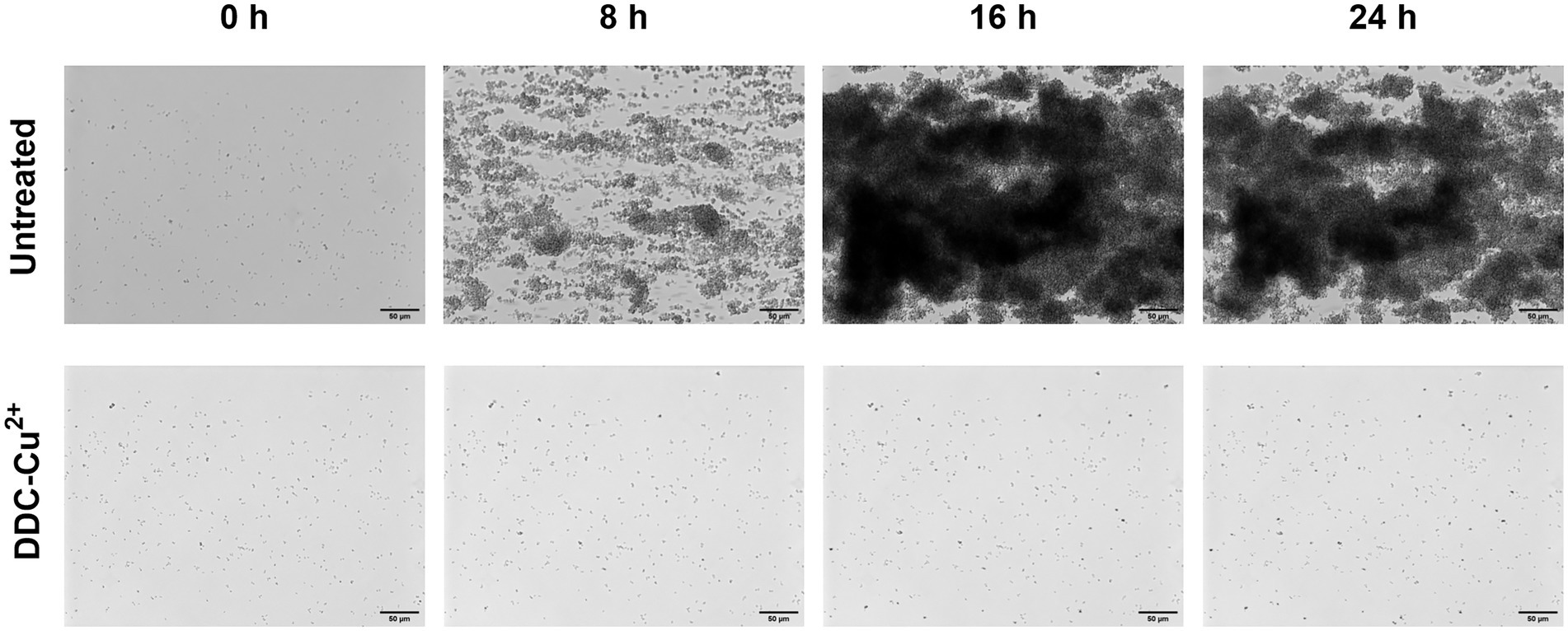
Figure 4. Monitoring of MRSA Mu50 biofilm formation over 24 h when left untreated or treated with a combination of 8 μg/ml diethyldithiocarbamate and 32 μg/ml Cu2+ combination (DDC-Cu2+) using the Bioflux system. Scale bar represents 50 μm.
Cytotoxicity of DDC-Cu2+ in vitro
The in vitro cytotoxicity of the compounds was investigated in fibroblast cells over 18 h (Figure 5A). Monotherapy with DDC and Cu2+ showed 70 and 94% fibroblast viability, respectively. Treatment with DDC-Cu2+ resulted in 75% fibroblast viability, showing no difference compared to DDC monotherapy.
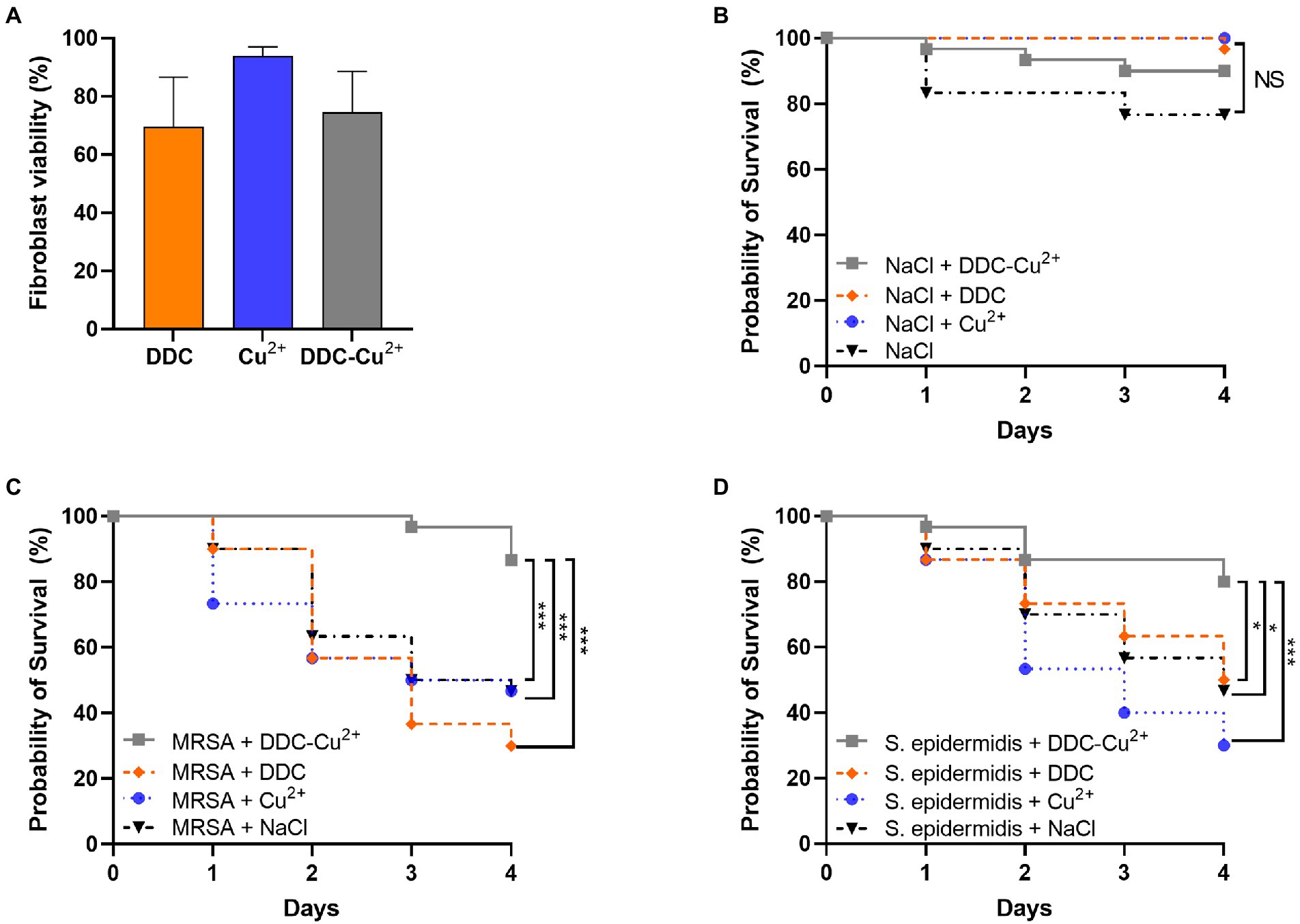
Figure 5. Effect of diethyldithiocarbamate [DDC; orange; 8 μg/ml (A), 6.4 mg/kg (B–D)], Cu2+ [blue; 32 μg/ml (A), 25.6 mg/kg (B–D)] and DDC-Cu2+ (grey) on (A) fibroblast viability (n = 3), on (B) probability of Galleria mellonella survival (30/group; n = 120), on the probability of survival of Galleria mellonella infected with (C) MRSA Mu50 (30/group; n = 120), and (D) infected with S. epidermidis ATCC 35984 (30/group; n = 120; NS = not significant; *p < 0.05; ***p < 0.001).
Toxicity and efficacy of DDC-Cu2+ in vivo using Galleria mellonella larvae
To investigate potential toxic treatment effects in vivo, G. mellonella larvae were injected with DDC, Cu2+, DDC-Cu2+ or vehicle control (saline) and the survival was monitored over 4 days. DDC, Cu2+ and DDC-Cu2+ showed similar survival rates as the vehicle control, indicating no treatment toxicity in G. mellonella (Figure 5B).
To assess the antimicrobial activity of DDC-Cu2+ in vivo, the survival of MRSA- or S. epidermidis-infected G. mellonella was examined over 4 days. In infected larvae, treatment with DDC or Cu2+ resulted in a poor survival rate, similar to the vehicle control for both MRSA- and S. epidermidis-infected G. mellonella (p > 0.05; Figures 5C,D, respectively). However, MRSA-infected and DDC-Cu2+ treated larvae, displayed a significantly higher survival rate of 87% (26/30 larvae) compared to MRSA-infected, vehicle control larvae that showed 47% survival (14/30 larvae, p = 0.0004; Figure 5C). Moreover, the survival rate of MRSA-infected, DDC-Cu2+ treated larvae was significantly higher compared to treatment with DDC alone (9/30 larvae; p = 0.0003) or Cu2+ alone (14/30 larvae; p = 0.0003). Similar results were found in S. epidermidis-infected G. mellonella, which showed a significantly higher survival rate of 80% (24/30 larvae) for S. epidermidis-infected, DDC-Cu2+ treated larvae compared to 47% survival (14/30 larvae) for S. epidermidis-infected, vehicle control larvae (p = 0.0152; Figure 5D). Survival of S. epidermidis-infected, DDC-Cu2+ treated G. mellonella (26/30 larvae) was also significantly higher compared to mono treatment with DDC (15/30 larvae; p = 0.0152) or Cu2+ (9/30 larvae; p = 0.0003).
Discussion
DDC is the metabolite of disulfiram, an FDA-approved drug for the oral treatment of chronic alcoholism, that has been previously investigated for its activity against fungi (Harrison et al., 2007; De Brucker et al., 2013), parasites (Khouri et al., 2010; Celes et al., 2016) and bacteria (Taylor et al., 1987; Dalecki et al., 2015; Long, 2017; Sheppard et al., 2018; Frazier et al., 2019). In the current study, DDC was repurposed and combined with Cu2+ for pre-clinical validation as a novel antibacterial treatment. Confirming previous results, DDC showed limited antibacterial activity against S. aureus and S. epidermidis, with MICs ranging from 16 to above 32 μg/ml and no growth inhibition of Gram-negative bacteria with MICs above 64 μg/ml. The lack of antibacterial activity of DDC against E. coli and P. aeruginosa was explained by the elevated presence of glutathione in Gram-negative bacteria. Cellular glutathione interacts with DDC and disulfiram by thiol-disulfide exchange reaction (Long, 2017; Frazier et al., 2019). While monotherapy with disulfiram showed antibacterial and antibiofilm activity against S. aureus in vitro and in vivo and synergized with multiple antibiotics (Long, 2017; Thakare et al., 2019), these results were not observed with the in vivo formed metabolites of disulfiram (Frazier et al., 2019). As disulfiram is hypothesized to form disulfides with thiophilic residues of bacterial cofactors, metabolites and enzymes (Long, 2017; Sheppard et al., 2018), the lack of antibacterial activity of DDC and other metabolites can be explained by lack of thiol-disulfide exchange. In addition, disulfiram and DDC differentiate in their chemical and physical properties (Gessner and Gessner, 1992). While disulfiram shows poor water solubility and physiological instability, therefore limiting local clinical applications (Xie et al., 2022), DDC is highly water soluble (Gessner and Gessner, 1992), a labile molecule and a very strong metal chelator (Butcher et al., 2018). Specifically, Cu2+ was investigated, as disulfiram dissociates in the presence of Cu2+, to form DDC, which chelates the metal ion and forms the stable Cu(DDC)2 complex that can be visualized by a color change (Dalecki et al., 2015) and has been shown to result in anticancer activity (Viola-Rhenals et al., 2018).
Dalecki et al. (2015) were the first to reveal that disulfiram and DDC displayed antimycobacterial effects only in the presence of Cu2+, as the presence of iron and zinc ions did not increase the antimicrobial activity of DDC against Mycobacterium tuberculosis. In addition, 90% of Mycobacterium tuberculosis inhibition occurred with 0.3 μM disulfiram, equivalent to 0.6 μM DDC and 0.3 μM Cu2+, which is consistent with the molar ratio of 2:1 and consequently the formation of the Cu(DDC)2 complex (Dalecki et al., 2015). Based on these results, Saputo et al. (2018) investigated the effect of disulfiram with Cu2+ on Streptococcus mutans and observed a reduction of disulfiram MIC from 16 μg/ml to 4 μg/ml (equivalent to 8 μg/ml DDC) in the presence of 106.6 μg/ml Cu2+. The concentration of disulfiram required to inhibit S. mutans biofilm formation was even lower with 2 μg/ml (equivalent to 4 μg/ml DDC) in the presence of 106.6 μg/ml Cu2+, resulting in synergistic effects of disulfiram and Cu2+ against both the planktonic and biofilm forms. We obtained comparable results against S. aureus and S. epidermidis, with concentrations as low as 0.5 μg/ml DDC and 2 μg/ml Cu2+ against planktonic MRSA Mu50 and 4 μg/ml DDC and 16 μg/ml Cu2+ against biofilm MRSA Mu50, respectively, reaching synergistic effects in both forms. In contrast to the concentrations required for the antimycobacterial activity, the Cu2+ concentrations necessary to enhance the activity of DDC against S. mutans, S. aureus and S. epidermidis exceeded the DDC concentration. This concentration-dependent antibacterial activity was also observed by Menghani et al. (2021) against Streptococcus pneumoniae.
The concentrations of DDC and Cu2+ play an important role in the proposed mode of action for the antibacterial activity of DDC-Cu2+. The mechanism of DDC can in part be explained by inhibition of the S. aureus carbonic anhydrase (Urbanski et al., 2021) and the chelation and extraction of required metal cofactors, including Cu2+ from metallo-enzymes, such as superoxide dismutase, rendering bacteria more susceptible to oxidative stress (Frazier et al., 2019). In addition, at high levels Cu2+ is toxic by the generation of reactive oxygen species through the Cu+/Cu2+ redox cycle and by competing with other metals at the enzymatic binding sites, leading to the inactivation and oxidation of free thiol groups of various proteins (Baker et al., 2010; Dupont et al., 2011). Therefore, bacteria have developed mechanisms to regulate the intracellular copper concentration and to evade copper induced toxicity, staphylococci have efflux systems in form of a P1-type ATPase transporter, copper-binding chaperones and copper-responsive regulators (Solioz, 2018), explaining the low antimicrobial activity of Cu2+ with a MIC above 128 μg/ml.
To explain the mode of action behind the antimycobacterial activity of DDC and Cu2+, Dalecki et al. (2015) proposed a Trojan Horse model, where the Cu(DDC)2 complex transports Cu2+ into the cytoplasm, thereby protecting Cu2+ from the bacterial copper resistance mechanisms, which in turn, allows access to targets that usually are not available to free Cu2+ (Dalecki et al., 2015). However, in the present study the concentrations closest to corresponding to the Cu(DDC)2 complex, 8 μg/ml DDC with 4 μg/ml Cu2+ and 32 μg/ml DDC with 16 μg/ml Cu2+, resulted in less than 25% S. aureus and S. epidermidis biofilm killing. Therefore, the antibiofilm activity of DDC and Cu2+ against S. aureus and S. epidermidis could not exclusively be associated to the Cu(DDC)2-complex. The lowest concentration of the mix leading to a statistical increase in S. aureus and S. epidermidis biofilm killing compared to monotherapy with Cu2+ was 8 μg/ml DDC in combination with 32 μg/ml Cu2+. Hence, the antibacterial activity of DDC-Cu2+ against S. aureus and S. epidermidis seems to be based on the formation of the Cu(DDC)2 complex and an excess of free Cu2+. Based on these results, we hypothesize that the Cu(DDC)2 complex inhibits at least one of the copper homeostasis components such as the efflux transporter, allowing for the additional Cu2+ to accumulate within the bacteria and cause copper induced toxicity (Figure 6). In addition, the extensive inhibition of MRSA biofilm attachment and aggregation by DDC-Cu2+ observed with the xCELLigence and the Bioflux systems depended on the combination of DDC and Cu2+ and can be caused by excess Cu2+ that represses the expression of positive biofilm formation regulators, such as agr and sae (Baker et al., 2010).
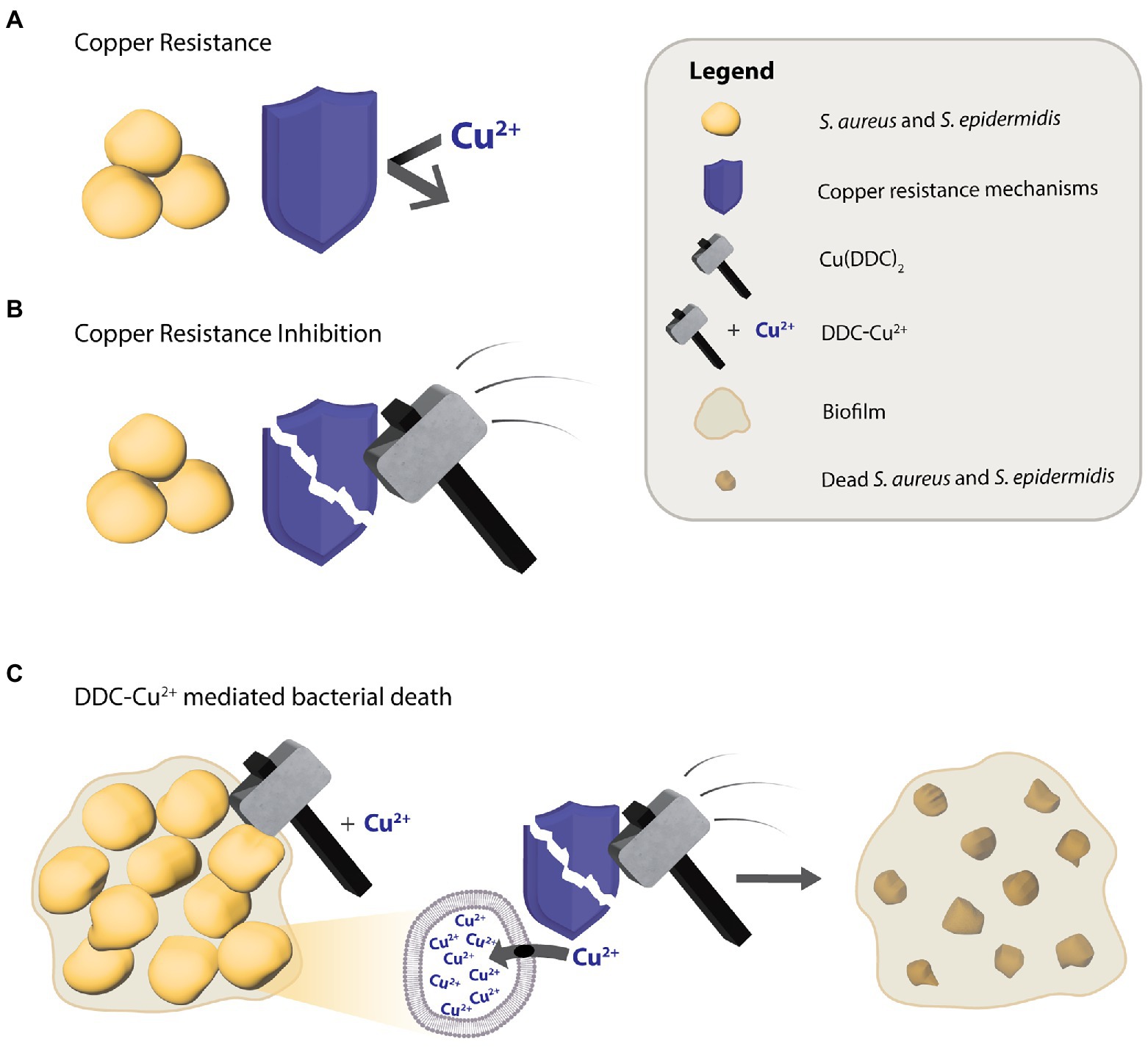
Figure 6. Putative diethyldithiocarbamate and copper (DDC-Cu2+) mode of action against S. aureus and S. epidermidis. (A) The antibacterial activity of Cu2+ is limited by copper resistance mechanisms of bacteria. (B) The Cu(DDC)2 complex inhibits the bacterial copper resistance mechanism but does not kill bacteria. (C) The combination of Cu(DDC)2 complex and excessive Cu2+ (called DDC-Cu2+) effectively kills bacteria, as the Cu(DDC)2 complex inhibits the copper resistance mechanisms, allowing for the excess Cu2+ to increase copper induced toxicity.
While the DDC-Cu2+ combination of 8 μg/ml DDC and 32 μg/ml Cu2+ inhibited planktonic S. aureus and S. epidermidis growth and biofilm formation, the same concentrations showed low cytotoxic effects against fibroblasts. As antimicrobial and cytotoxic results obtained in vitro do not always accurately predict activity under in vivo conditions (Tsai et al., 2016), both the antibacterial activity and the toxicity of DDC-Cu2+ was investigated using the G. mellonella model. These larvae have been shown to be good models to assess the safety and efficacy of antimicrobial agents against S. aureus (Brackman et al., 2011; Desbois and Coote, 2011; Tsai et al., 2016). The high survival rate of uninfected, treated larvae confirmed the non-toxicity of DDC-Cu2+ and the significant increase of survival of MRSA- and S. epidermidis-infected, DDC-Cu2+ treated G. mellonella confirmed the in vitro antibacterial activity. To the best of our knowledge, this is the first study to report the antibacterial activity and non-toxicity of DDC in combination with Cu2+ in the G. mellonella model. The promising results obtained with the G. mellonella model pre-screening experiment increases the confidence in the performance of Cu(DDC)2 and excess Cu2+ to progress to preclinical mammalian models. A pharmaceutical development of the DDC-Cu2+ combination is ongoing to provide a drug delivery platform for the treatment of infected wounds and for surgical applications. A DDC-Cu2+ formulation has potential to synergistically enhance standard-of-care with oral or topical antibiotics and reduce the pressure on resistance development.
In conclusion, the combination of DDC-Cu2+ showed considerable in vitro antimicrobial activity against planktonic and biofilm cultures of S. aureus and S. epidermidis. By enhancing multiple antibiotic classes, preventing biofilm formation, showing non-toxicity and antibacterial activity in vivo, the DDC-Cu2+ combination represents an effective novel treatment strategy to control S. aureus and S. epidermidis biofilms. Ongoing studies are focused on developing drug delivery platforms containing the DDC-Cu2+ combination for clinical application and to determine whether similar safety and antimicrobial efficacy can be observed in other in vivo models of infection.
Data availability statement
The raw data supporting the conclusions of this article will be made available by the authors, without undue reservation.
Author contributions
LK designed and conducted the experiments, analyzed data, and wrote the manuscript. KR designed and conducted the experiments and analyzed data. AA analyzed data, contributed to the result section, and reviewed the manuscript. TC, BK, and MH provided technical assistance for some of the experiments and reviewed the manuscript. AZ, RS, and KR supervised the study, reviewed the manuscript and are ranked in ascending order of contribution with KR as senior author. All authors contributed to the article and approved the submitted version.
Funding
This work was supported by the National Health and Medical Research Council (GNT1163634 and GNT2004036), the University of Adelaide (Joint PhD Scholarship held by LK) and The Hospital Research Foundation, Australia. Bioflux Z1000 setup was (partially) funded by Netherlands Scientific Organisation (NWO) Earth and Life Sciences (ALW), grant 834.13.006 to BK.
Acknowledgments
The authors kindly acknowledge Animate Your Science (www.animateyour.science) for professional graphics.
Conflict of interest
KR holds intellectual property on the DDC-Cu2+ treatment (PCT/AU2020/050661).
The remaining authors declare that the research was conducted in the absence of any commercial or financial relationships that could be construed as a potential conflict of interest.
Publisher’s note
All claims expressed in this article are solely those of the authors and do not necessarily represent those of their affiliated organizations, or those of the publisher, the editors and the reviewers. Any product that may be evaluated in this article, or claim that may be made by its manufacturer, is not guaranteed or endorsed by the publisher.
Supplementary material
The Supplementary material for this article can be found online at: https://www.frontiersin.org/articles/10.3389/fmicb.2022.999893/full#supplementary-material
Supplementary Figure 1
Confocal microscopy images of stained MRSA Mu50 biofilms with LIVE/DEAD BacLight staining after treatment with 8 μg/ml diethyldithiocarbamate and 32 μg/ml Cu2+. Confocal microscopy images results: green = viable bacteria; red = dead bacteria. (A) Untreated MRSA Mu50 biofilm at 20 ×. (B) MRSA Mu50 biofilm after treatment with DDC-Cu2+ at 20 ×.
Supplementary File 1
Video footage of MRSA Mu50 biofilm formation over 24 h when exposed to constant nutrient flow or when nutrient flow is supplemented with a combination of diethyldithiocarbamate and Cu2+ combination (DDC-Cu2+) using the Bioflux system. Scale bar represents 50 μm, 8 FPS corresponding to 2 h/s.
References
Abrantes, P., and Africa, C. W. J. (2020). Measuring Streptococcus mutans, Streptococcus sanguinis and Candida albicans biofilm formation using a real-time impedance-based system. J. Microbiol. Methods 169:105815. doi: 10.1016/j.mimet.2019.105815
Anderson, D. J., Sexton, D. J., Kanafani, Z. A., Auten, G., and Kaye, K. S. (2007). Severe surgical site infection in community hospitals: epidemiology, key procedures, and the changing prevalence of methicillin-resistant Staphylococcus aureus. Infect. Control Hosp. Epidemiol. 28, 1047–1053. doi: 10.1086/520731
Australian Commission on Safety and Quality in Health Care (ACSQHC). (2019). AURA 2019: Third Australian Report on Antimicrobial Use and Resistance in Human Health. Sydney: ACSQHC.
Baker, J., Sitthisak, S., Sengupta, M., Johnson, M., Jayaswal, R. K., and Morrissey, J. A. (2010). Copper stress induces a global stress response in Staphylococcus aureus and represses sae and agr expression and biofilm formation. Appl. Environ. Microbiol. 76, 150–160. doi: 10.1128/AEM.02268-09
Brackman, G., Cos, P., Maes, L., Nelis, H. J., and Coenye, T. (2011). Quorum sensing inhibitors increase the susceptibility of bacterial biofilms to antibiotics in vitro and in vivo. Antimicrob. Agents Chemother. 55, 2655–2661. doi: 10.1128/AAC.00045-11
Butcher, K., Kannappan, V., Kilari, R. S., Morris, M. R., McConville, C., Armesilla, A. L., et al. (2018). Investigation of the key chemical structures involved in the anticancer activity of disulfiram in A549 non-small cell lung cancer cell line. BMC Cancer 18:753. doi: 10.1186/s12885-018-4617-x
Cahill, T. J., Baddour, L. M., Habib, G., Hoen, B., Salaun, E., Pettersson, G. B., et al. (2017). Challenges in infective endocarditis. J. Am. Coll. Cardiol. 69, 325–344. doi: 10.1016/j.jacc.2016.10.066
Celes, F. S., Trovatti, E., Khouri, R., Van Weyenbergh, J., Ribeiro, S. J., Borges, V. M., et al. (2016). DETC-based bacterial cellulose bio-curatives for topical treatment of cutaneous leishmaniasis. Sci. Rep. 6:38330. doi: 10.1038/srep38330
Costerton, J. W., Stewart, P. S., and Greenberg, E. P. (1999). Bacterial biofilms: a common cause of persistent infections. Science 284, 1318–1322. doi: 10.1126/science.284.5418.1318
Crabbé, A., Jensen, P. Ø., Bjarnsholt, T., and Coenye, T. (2019). Antimicrobial tolerance and metabolic adaptations in microbial biofilms. Trends Microbiol. 27, 850–863. doi: 10.1016/j.tim.2019.05.003
Craft, K. M., Nguyen, J. M., Berg, L. J., and Townsend, S. D. (2019). Methicillin-resistant Staphylococcus aureus (MRSA): antibiotic-resistance and the biofilm phenotype. Medchemcomm. 10, 1231–1241. doi: 10.1039/C9MD00044E
Dalecki, A. G., Haeili, M., Shah, S., Speer, A., Niederweis, M., Kutsch, O., et al. (2015). Disulfiram and copper ions kill mycobacterium tuberculosis in a synergistic manner. Antimicrob. Agents Chemother. 59, 4835–4844. doi: 10.1128/AAC.00692-15
De Brucker, K., Bink, A., Meert, E., Cammue, B. P., and Thevissen, K. (2013). Potentiation of antibiofilm activity of amphotericin B by superoxide dismutase inhibition. Oxidative Med. Cell. Longev. 2013:704654, 1–7. doi: 10.1155/2013/704654
Desbois, A. P., and Coote, P. J. (2011). Wax moth larva (Galleria mellonella): an in vivo model for assessing the efficacy of antistaphylococcal agents. J. Antimicrob. Chemother. 66, 1785–1790. doi: 10.1093/jac/dkr198
Dinarvand, M., Spain, M. P., and Vafaee, F. (2020). Pharmacodynamic functions of synthetic derivatives for treatment of methicillin-resistant Staphylococcus aureus (MRSA) and mycobacterium tuberculosis. Front. Microbiol. 11:551189. doi: 10.3389/fmicb.2020.551189
Dupont, C. L., Grass, G., and Rensing, C. (2011). Copper toxicity and the origin of bacterial resistance - new insights and applications. Metallomics 3, 1109–1118. doi: 10.1039/c1mt00107h
European Centre for Disease Prevention and Control (2022). Antimicrobial Resistance in the EU/EEA (EARS-Net) – Annual Epidemiological Report 2020. Stockholm: ECDC.
Feng, T., Leptihn, S., Dong, K., Loh, B., Zhang, Y., Stefan, M. I., et al. (2021). JD419, a Staphylococcus aureus phage with a unique morphology and broad host range. Front. Microbiol. 12:602902. doi: 10.3389/fmicb.2021.602902
Frazier, K. R., Moore, J. A., and Long, T. E. (2019). Antibacterial activity of disulfiram and its metabolites. J. Appl. Microbiol. 126, 79–86. doi: 10.1111/jam.14094
Gessner, P. K., and Gessner, T. (1992). Toxicology. Disulfiram and its metabolite diethyldithiocarbamate pharmacology and status in the treatment of alcoholism, HIV infections, AIDS and heavy metal toxicity. London, United Kingdom: Chapman and Hall, 335–345
Gessner, P. K., and Gessner, T. (1992). “Relevant physical and chemical properties” in Disulfiram and its metabolite, diethyldithiocarbamate: pharmacology and status in the treatment of alcoholism, HIV infections, AIDS and heavy metal toxicity. eds. P. K. Gessner and T. Gessner (Springer Netherlands: Dordrecht), 7–12.
Harrison, J. J., Turner, R. J., and Ceri, H. (2007). A subpopulation of Candida albicans and Candida tropicalis biofilm cells are highly tolerant to chelating agents. FEMS Microbiol. Lett. 272, 172–181. doi: 10.1111/j.1574-6968.2007.00745.x
Hoogenkamp, M.A. (2021). Challenging dental unit water biofilms. Amsterdam, The Netherlands: University of Amsterdam.
Kaul, L., Süss, R., Zannettino, A., and Richter, K. (2021). The revival of dithiocarbamates: from pesticides to innovative medical treatments. iScience 24:102092. doi: 10.1016/j.isci.2021.102092
Kaushik, A., and Kest, H. (2018). Pediatric methicillin-resistant Staphylococcus aureus osteoarticular infections. Microorganisms 6:40. doi: 10.3390/microorganisms6020040
Khouri, R., Novais, F., Santana, G., de Oliveira, C. I., Vannier dos Santos, M. A., Barral, A., et al. (2010). DETC induces leishmania parasite killing in human in vitro and murine in vivo models: a promising therapeutic alternative in leishmaniasis. PLoS One 5:e14394. doi: 10.1371/journal.pone.0014394
Kleinschmidt, S., Huygens, F., Faoagali, J., Rathnayake, I. U., and Hafner, L. M. (2015). Staphylococcus epidermidis as a cause of bacteremia. Future Microbiol. 10, 1859–1879. doi: 10.2217/fmb.15.98
Lee, J. Y. H., Monk, I. R., Gonçalves da Silva, A., Seemann, T., Chua, K. Y. L., Kearns, A., et al. (2018). Global spread of three multidrug-resistant lineages of Staphylococcus epidermidis. Nat. Microbiol. 3, 1175–1185. doi: 10.1038/s41564-018-0230-7
Liu, C., Bayer, A., Cosgrove, S. E., Daum, R. S., Fridkin, S. K., Gorwitz, R. J., et al. (2011). Clinical practice guidelines by the Infectious Diseases Society of America for the treatment of methicillin-resistant Staphylococcus aureus infections in adults and children. Clin. Infect. Dis. 52, e18–e55. doi: 10.1093/cid/ciq146
Long, T. E. (2017). Repurposing thiram and disulfiram as antibacterial agents for multidrug-resistant Staphylococcus aureus infections. Antimicrob. Agents Chemother. 61, e00898-17. doi: 10.1128/AAC.00898-17
López-Cortés, L. E., Gálvez-Acebal, J., and Rodríguez-Baño, J. (2020). Therapy of Staphylococcus aureus bacteremia: evidences and challenges. Enferm. Infecc. Microbiol. Clin. (Engl. Ed). 38, 489–497. doi: 10.1016/j.eimc.2020.01.018
Mah, T. F., and O'Toole, G. A. (2001). Mechanisms of biofilm resistance to antimicrobial agents. Trends Microbiol. 9, 34–39. doi: 10.1016/S0966-842X(00)01913-2
Menghani, S. V., Rivera, A., Neubert, M., Hagerty, J. R., Lewis, L., Galgiani, J. N., et al. (2021). Demonstration of N,N-dimethyldithiocarbamate as a copper-dependent antibiotic against multiple upper respiratory tract pathogens. Microbiol. Spectr. 9:e0077821. doi: 10.1128/Spectrum.00778-21
Nishimori, I., Vullo, D., Minakuchi, T., Scozzafava, A., Osman, S. M., AlOthman, Z., et al. (2014). Anion inhibition studies of two new β-carbonic anhydrases from the bacterial pathogen legionella pneumophila. Bioorg. Med. Chem. Lett. 24, 1127–1132. doi: 10.1016/j.bmcl.2013.12.124
Otto, M. (2008). Staphylococcal biofilms. Curr. Top. Microbiol. Immunol. 322, 207–228. doi: 10.1007/978-3-540-75418-3_10
Patiniott, P., Jacombs, A., Kaul, L., Hu, H., Warner, M., Klosterhalfen, B., et al. (2022). Are late hernia mesh complications linked to staphylococci biofilms? Hernia. doi: 10.1007/s10029-022-02583-0 [Epub ahead of print].
Peeters, E., Nelis, H. J., and Coenye, T. (2008). Comparison of multiple methods for quantification of microbial biofilms grown in microtiter plates. J. Microbiol. Methods 72, 157–165. doi: 10.1016/j.mimet.2007.11.010
Phillips, M. G. M., Nedunchezian, D., Lukrec, A., and Howard, R. G. (1991). Disulfiram inhibits the in vitro growth of methicillin-resistant Staphylococcus aureus. Antimicrob. Agents Chemother. 35, 785–787. doi: 10.1128/AAC.35.4.785
Pushpakom, S., Iorio, F., Eyers, P. A., Escott, K. J., Hopper, S., Wells, A., et al. (2018). Drug repurposing: progress, challenges and recommendations. Nat. Rev. Drug Discov. 18, 41–58. doi: 10.1038/nrd.2018.168
Richter, K. (2019). Tackling superbugs in their slime castles: innovative approaches against antimicrobial-resistant biofilm infections. Microbiol. Aust. 40, 165–168. doi: 10.1071/MA19049
Richter, K., Facal, P., Thomas, N., Vandecandelaere, I., Ramezanpour, M., Cooksley, C., et al. (2017a). Taking the silver bullet colloidal silver particles for the topical treatment of biofilm-related infections. ACS Appl. Mater. Interfaces 9, 21631–21638. doi: 10.1021/acsami.7b03672
Richter, K., Ramezanpour, M., Thomas, N., Prestidge, C. A., Wormald, P. J., and Vreugde, S. (2016). Mind “De GaPP”: in vitro efficacy of deferiprone and gallium-protoporphyrin against Staphylococcus aureus biofilms. Int. Forum. Allergy Rhinol. 6, 737–743. doi: 10.1002/alr.21735
Richter, K., Thomas, N., Claeys, J., McGuane, J., Prestidge, C. A., Coenye, T., et al. (2017b). A topical hydrogel with deferiprone and gallium-protoporphyrin targets bacterial iron metabolism and has antibiofilm activity. Antimicrob. Agents Chemother. 61, e00481-17. doi: 10.1128/AAC.00481-17
Sánchez-López, E., Gomes, D., Esteruelas, G., Bonilla, L., Lopez-Machado, A. L., Galindo, R., et al. (2020). Metal-based nanoparticles as antimicrobial agents: an overview. Nano 10:292. doi: 10.3390/nano10020292
Santajit, S., and Indrawattana, N. (2016). Mechanisms of antimicrobial resistance in ESKAPE pathogens. Biomed. Res. Int. 2016, 1–8. doi: 10.1155/2016/2475067
Saputo, S., Faustoferri, R. C., and Quivey, R. G. Jr. (2018). A drug repositioning approach reveals that Streptococcus mutans is susceptible to a diverse range of established antimicrobials and nonantibiotics. Antimicrob. Agents Chemother. 62, e01674-17. doi: 10.1128/AAC.01674-17
Seixas, A. F., Quendera, A. P., Sousa, J. P., Silva, A. F. Q., Arraiano, C. M., and Andrade, J. M. (2021). Bacterial response to oxidative stress and RNA oxidation. Front. Genet. 12:821535. doi: 10.3389/fgene.2021.821535
Sheppard, J. G., Frazier, K. R., Saralkar, P., Hossain, M. F., Geldenhuys, W. J., and Long, T. E. (2018). Disulfiram-based disulfides as narrow-spectrum antibacterial agents. Bioorg. Med. Chem. Lett. 28, 1298–1302. doi: 10.1016/j.bmcl.2018.03.023
Solioz, M. (2018). Copper homeostasis in gram-positive bacteria. Copper and bacteria. London: Springer Briefs in Biometals, 21–48.
Sopirala, M. M., Mangino, J. E., Gebreyes, W. A., Biller, B., Bannerman, T., Balada-Llasat, J. M., et al. (2010). Synergy testing by Etest, microdilution checkerboard, and time-kill methods for pan-drug-resistant Acinetobacter baumannii. Antimicrob. Agents Chemother. 54, 4678–4683. doi: 10.1128/AAC.00497-10
Sovari, S. N., Vojnovic, S., Bogojevic, S. S., Crochet, A., Pavic, A., Nikodinovic-Runic, J., et al. (2020). Design, synthesis and in vivo evaluation of 3-arylcoumarin derivatives of rhenium(I) tricarbonyl complexes as potent antibacterial agents against methicillin-resistant Staphylococcus aureus (MRSA). Eur. J. Med. Chem. 205:112533. doi: 10.1016/j.ejmech.2020.112533
Stevens, D. L., Bisno, A. L., Chambers, H. F., Everett, E. D., Dellinger, P., Goldstein, E. J., et al. (2005). Practice guidelines for the diagnosis and management of skin and soft-tissue infections. Clin. Infect. Dis. 41, 1373–1406. doi: 10.1086/497143
Taylor, E. H., Walker, E., Bartelt, M., Day, S., and Pappas, A. (1987). In vitro antimicrobial activity of diethyldithiocarbamate and dimethyldithiocarbamate against methicillin-resistant Staphylococcus. Ann. Clin. Lab. Sci. 17, 171–177.
Thakare, R., Shukla, M., Kaul, G., Dasgupta, A., and Chopra, S. (2019). Repurposing disulfiram for treatment of Staphylococcus aureus infections. Int. J. Antimicrob. Agents 53, 709–715. doi: 10.1016/j.ijantimicag.2019.03.024
Thangamani, S., Mohammad, H., Younis, W., and Seleem, M. N. (2015). Drug repurposing for the treatment of staphylococcal infections. Curr. Pharm. Des. 21, 2089–2100. doi: 10.2174/1381612821666150310104416
Tong, S. Y. C., Davis, J. S., Eichenberger, E., Holland, T. L., and Fowler, V. G. Jr. (2015). Staphylococcus aureus infections: epidemiology, pathophysiology, clinical manifestations, and management. Clin. Microbiol. Rev. 28, 603–661. doi: 10.1128/CMR.00134-14
Tsai, C. J.-Y., Loh, J. M. S., and Proft, T. (2016). Galleria mellonella infection models for the study of bacterial diseases and for antimicrobial drug testing. Virulence 7, 214–229. doi: 10.1080/21505594.2015.1135289
Urbanski, L. J., Vullo, D., Parkkila, S., and Supuran, C. T. (2021). An anion and small molecule inhibition study of the β-carbonic anhydrase from Staphylococcus aureus. J. Enzyme Inhib. Med. Chem. 36, 1088–1092. doi: 10.1080/14756366.2021.1931863
Viola-Rhenals, M., Patel, K. R., Jaimes-Santamaria, L., Wu, G., Liu, J., and Dou, Q. P. (2018). Recent advances in antabuse (disulfiram): the importance of its metal-binding ability to its anticancer activity. Curr. Med. Chem. 25, 506–524. doi: 10.2174/0929867324666171023161121
Walsh, L., Johnson, C. N., Hill, C., and Ross, R. P. (2021). Efficacy of phage- and bacteriocin-based therapies in combatting nosocomial MRSA infections. Front. Mol. Biosci. 8:654038. doi: 10.3389/fmolb.2021.654038
Wang, X., Bittner, T., Milanov, M., Kaul, L., Mundinger, S., Koch, H.-G., et al. (2021). Pyridinium modified anthracenes and their endoperoxides provide a tunable scaffold with activity against gram-positive and gram-negative bacteria. ACS Infect. Dis. 7, 2073–2080. doi: 10.1021/acsinfecdis.1c00263
Wiegand, I., Hilpert, K., and Hancock, R. E. (2008). Agar and broth dilution methods to determine the minimal inhibitory concentration (MIC) of antimicrobial substances. Nat. Protoc. 3, 163–175. doi: 10.1038/nprot.2007.521
World Health Organization (2017). Antibacterial agents in clinical development: an analysis of the antibacterial clinical development pipeline, including tuberculosis. Geneva: WHO.
Xie, C., Yan, J., Cao, S., Liu, R., Sun, B., Xie, Y., et al. (2022). Bi-layered disulfiram-loaded fiber membranes with antibacterial properties for wound dressing. Appl. Biochem. Biotechnol. 194, 1359–1372. doi: 10.1007/s12010-021-03663-0
Xu, L., Tong, J., Wu, Y., Zhao, S., and Lin, B. L. (2021). A computational evaluation of targeted oxidation strategy (TOS) for potential inhibition of SARS-CoV-2 by disulfiram and analogues. Biophys. Chem. 276:106610. doi: 10.1016/j.bpc.2021.106610
Keywords: biofilm, antibacterial, diethyldithiocarbamate, copper ions, Staphylococcus aureus, Staphylococcus epidermidis, new treatment
Citation: Kaul L, Abdo AI, Coenye T, Krom BP, Hoogenkamp MA, Zannettino ACW, Süss R and Richter K (2022) The combination of diethyldithiocarbamate and copper ions is active against Staphylococcus aureus and Staphylococcus epidermidis biofilms in vitro and in vivo. Front. Microbiol. 13:999893. doi: 10.3389/fmicb.2022.999893
Edited by:
Nagendran Tharmalingam, Rhode Island Hospital, United StatesReviewed by:
Arshad Rizvi, Emory University, United StatesSoojin Jang, Korea Pasteur Institute, South Korea
Charuta Agashe, Icahn School of Medicine at Mount Sinai, United States
Copyright © 2022 Kaul, Abdo, Coenye, Krom, Hoogenkamp, Zannettino, Süss and Richter. This is an open-access article distributed under the terms of the Creative Commons Attribution License (CC BY). The use, distribution or reproduction in other forums is permitted, provided the original author(s) and the copyright owner(s) are credited and that the original publication in this journal is cited, in accordance with accepted academic practice. No use, distribution or reproduction is permitted which does not comply with these terms.
*Correspondence: Katharina Richter, katharina.richter@adelaide.edu.au