- GFZ German Research Centre for Geosciences, Section Geomicrobiology, Potsdam, Germany
Productive oxygen minimum zones are regions dominated by heterotrophic denitrification fueled by sinking organic matter. Microbial redox-sensitive transformations therein result in the loss and overall geochemical deficit in inorganic fixed nitrogen in the water column, thereby impacting global climate in terms of nutrient equilibrium and greenhouse gases. Here, geochemical data are combined with metagenomes, metatranscriptomes, and stable-isotope probing incubations from the water column and subseafloor of the Benguela upwelling system. The taxonomic composition of 16S rRNA genes and relative expression of functional marker genes are used to explore metabolic activities by nitrifiers and denitrifiers under decreased stratification and increased lateral ventilation in Namibian coastal waters. Active planktonic nitrifiers were affiliated with Candidatus Nitrosopumilus and Candidatus Nitrosopelagicus among Archaea, and Nitrospina, Nitrosomonas, Nitrosococcus, and Nitrospira among Bacteria. Concurrent evidence from taxonomic and functional marker genes shows that populations of Nitrososphaeria and Nitrospinota were highly active under dysoxic conditions, coupling ammonia and nitrite oxidation with respiratory nitrite reduction, but minor metabolic activity toward mixotrophic use of simple nitrogen compounds. Although active reduction of nitric oxide to nitrous oxide by Nitrospirota, Gammaproteobacteria, and Desulfobacterota was tractable in bottom waters, the produced nitrous oxide was apparently scavenged at the ocean surface by Bacteroidota. Planctomycetota involved in anaerobic ammonia oxidation were identified in dysoxic waters and their underlying sediments, but were not found to be metabolically active due to limited availability of nitrite. Consistent with water column geochemical profiles, metatranscriptomic data demonstrate that nitrifier denitrification is fueled by fixed and organic nitrogen dissolved in dysoxic waters, and prevails over canonical denitrification and anaerobic oxidation of ammonia when the Namibian coastal waters and sediment–water interface on the shelf are ventilated by lateral currents during austral winter.
1. Introduction
Oxygen minimum zones (OMZs) represent hotspots for oxygen-sensitive nitrogen microbial transformations (Codispoti and Christensen, 1985) and are traditionally viewed as productive areas dominated by heterotrophic denitrification fueled by algal organic matter (OM) sinking from the sunlit ocean surface down to the seafloor (Ulloa et al., 2013). In coastal waters such as the eastern tropical Pacific (Lam et al., 2009), the Arabian Sea (Orsi et al., 2017), and the Benguela upwelling (Lam et al., 2009; Callbeck et al., 2021), oxygen drawdown in OMZ waters initiate a dynamic nitrogen cycle (Kuypers et al., 2005), in which nitrate serves as the main terminal electron acceptor for the oxidation of OM, is actively reduced to nitrite (Lam and Kuypers, 2011), and is successively converted to nitrogen (N2) and nitrous oxide (N2O) gases through processes of heterotrophic denitrification (Tyrrell and Lucas, 2002) and autotrophic anaerobic ammonium oxidation (anammox; Woebken et al., 2007). Dissimilatory nitrate reduction to ammonium (DNRA), or “ammonification” (Dong et al., 2009), is considered more nitrogen conservative (Jensen et al., 2011) as the resulting release of ammonium (NH4+) regenerates nitrate via microaerobic microbial respiration in the upper part of the OMZ (Behrendt et al., 2013) and surface oxygenated waters (Füssel et al., 2012). Although all three microbial processes (i.e., denitrification, DNRA, and anammox) compete for nitrate and nitrite (Kraft et al., 2011), the uptake of nitrate and its reduction to ammonia inside the cell and excretion via DNRA may achieve higher growth yields if pursued by ammonia oxidation and denitrification (Lam and Kuypers, 2011). In general, anaerobic processes in the water column result in an overall geochemical deficit in inorganic fixed nitrogen and its loss from the oceans globally impacts the Earth climate system in terms of nutrient equilibrium (Küster-Heins et al., 2010) and emissions of greenhouse gases (Kuypers et al., 2005; Bertagnolli and Stewart, 2018). OMZs are predicted to expand and intensify in response to global climate change and altered ocean circulation (Garçon et al., 2019), leading to increase in carbon sedimentation to the seafloor and benthic releases of greenhouse gases mediated by the resident microbial communities (Wright et al., 2012). It is therefore of utmost importance to disentangle metabolic interactions in biogeochemical cycling (Inthorn et al., 2006b) within productive coastal OMZ waters and their underlying sediments.
Due to natural eutrophic conditions, the sunlit ocean surface of the Benguela upwelling system (BUS) off Namibia is highly productive (Verheye et al., 2016), leading to oxygen depletion below 60 m water depth (mwd) and a seasonal OMZ reaching down to the seafloor in austral summer (Lavik et al., 2009; Callbeck et al., 2021). Anoxic conditions extending down to the sediment–water interface (SWI) in austral summer trigger benthic releases of sulfide (H2S), methane (CH4), and NH4+ from the seafloor that seasonally intensifies OMZ conditions (Brüchert et al., 2009; Schunck et al., 2013). Due to the quasi-absence of sedimentary Fe to precipitate sulfide minerals (Böning et al., 2020), the H2S produced during organoclastic sulfate reduction (Ferdelman et al., 1999) diffuses back from the sediment into the anoxic bottom waters (Brüchert et al., 2003; Ohde and Dadou, 2018) with severe effects for the coastal life (Currie et al., 2018). Toxic H2S escapes are then mitigated by the activity of planktonic sulfur-oxidizing bacteria that detoxify sulfide (Lavik et al., 2009) while generating nitrite and NH4+ that can either augment nitrification with complete ammonia oxidation (comammox), or anammox to induce N2O emissions (Heiss and Fulweiler, 2016; Long et al., 2021). Trophic equilibrium in coastal waters is then rescued by the re-oxygenation of the SWI after the seasonal period of stratification. In austral winter 2018, water column and sediment samples suitable for microbiology analyses were obtained in the framework of an oceanographic sampling expedition to the BUS (Orsi et al., 2020b, 2022).
Here, water column geochemistry is combined with taxonomic, metagenomic, and metatranscriptomic sequencing data from the water column, seafloor sediment, as well as water and sediment samples incubated for stable-isotope probing (SIP) to detail the taxonomy and metabolic activities of microbial populations involved in the enzymology and ecology of the nitrogen cycle (Lam and Kuypers, 2011; Martínez-Espinosa et al., 2011) under decreased stratification in Namibian shelf waters. The relative expression of the corresponding functional marker genes (Suter et al., 2021) and geochemical profiles for the water column and sediment (Siccha and Kucera, 2018; Ferdelman et al., 2021a,b) show that active nitrification proceeds in two steps coupling ammonia and nitrite oxidation with respiratory nitrite reduction from oxic into dysoxic waters, and overall minor canonical denitrification in the quasi-absence of anammox (Long et al., 2021).
2. Materials and methods
2.1. Sampling
The research expedition Meteor M148-2 to the Benguela upwelling, entitled EreBUS (i.e., Processes controlling the Emissions of gREenhouse gases from the Benguela Upwelling System) took place in 2018 from 2nd to 20th July (Ferdelman et al., 2019). The water column and underlying sediments were sampled at multiple sites on the shelf and then offshore (Weatherall et al., 2021) as the ship transited from Walvis Bay, Namibia to Las Palmas, Canary Islands (Figure 1A). Water and sediment samples retrieved from the Namibian continental shelf (18.0 °S, 11.3 °E) were conditioned directly on board of the F/S Meteor vessel during the expedition (Orsi et al., 2020b, 2022). Water samples were retrieved using a Niskin rosette equipped with a Conductivity-Temperature-Depth system and a captor for light fluorescence reemitted by chlorophyll-a (chl-a; Siccha and Kucera, 2018) and water optical water quality variables (i.e., nepheloid turbidity unit; Garaba et al., 2021). Water samples from the Niskin bottles were analyzed for dissolved nutrient concentrations (i.e., nitrate, nitrite, silica, and phosphate) with a QuAAtro39 autoanalyzer (Seal Analytical) and fluorescence methods (i.e., NH4+; Ferdelman et al., 2021a). At each site, 2 L of seawater was filtered via peristaltic pumping onto an in-line 0.2 μm polycarbonate filter. Replicate filters were then stored in sterile DNA/RNA clean 15 mL Falcon tubes and frozen immediately at −80°C for further DNA and RNA analyses. A 30 cm-long gravity core was obtained from a water depth of 125 m at site 6, deploying a multicorer that yielded an intact SWI and the upper 30 cm of underlying sediment (Supplementary Figure 1). The core was sectioned into 2 cm intervals, and sediments transferred into sterile DNA/RNA free 50 ml Falcon tubes and immediately frozen at −80°C until DNA and RNA extractions (Orsi et al., 2020a,b). Pore waters were extracted using MicroRhizon moisture samplers (Rhizon CSS, Rhizosphere research products) and analyzed for phosphate, nitrogen, and sulfur species (Ferdelman et al., 2021b).
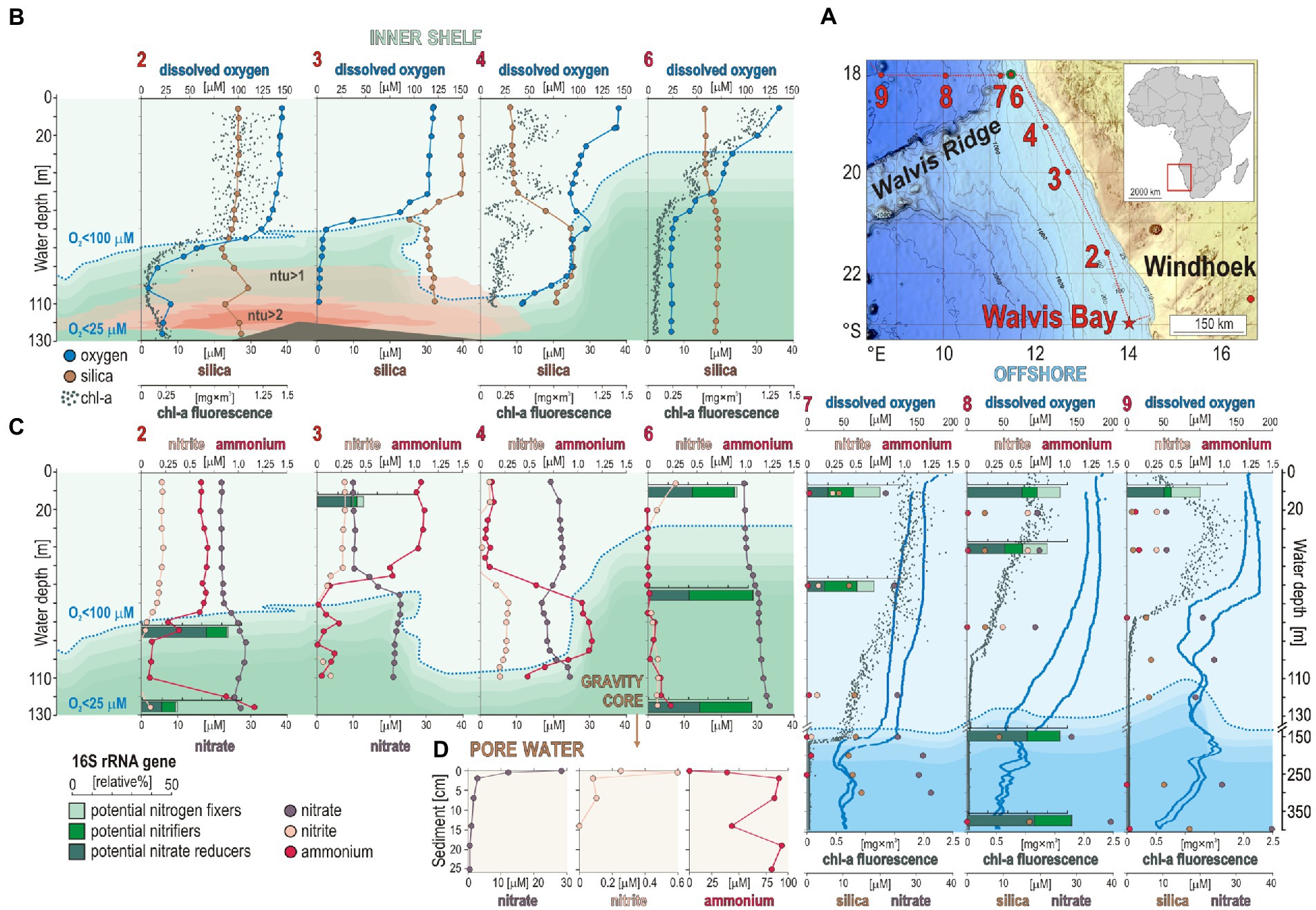
Figure 1. Sampling sites along the Namibian shelf, water column geochemical profiles, with a relative abundance of microbial guilds involved in nitrogen cycling, and pore water geochemistry. (A) Bathymetric map of the Namibian shelf (data from Weatherall et al., 2021) displaying the different sites sampled during the EreBUS cruise 2018. (B) Concentration profiles for dissolved oxygen (blue) with an oxycline defined as <100 μM (dotted line), silica (brown), chlorophyll-a (green), and turbidity in nephelometric turbidity units (ntu) at each successive sampling site. (C) Concentration profiles for nitrite (pink), ammonia (red), and nitrate (purple) in the water column at each successive site, with relative abundances (% bar charts) of potential nitrogen fixers (light green), potential nitrifiers (green), and potential nitrate reducers (dark green). (D) Pore water geochemical profiles for nitrate (purple), nitrite (pink), and ammonium (red). Geochemical data are adapted from Siccha and Kucera (2018), Garaba et al. (2021), and Ferdelman et al. (2021a,b).
Geochemical data (Siccha and Kucera, 2018; Garaba et al., 2021; Ferdelman et al., 2021a,b) are archived and publicly available from the PANGAEA® Data Publisher for Earth and Environmental Science (datasets #895640, #931090, #931097, and #928943).
2.2. Nucleic acid extractions
For size-fractionated filters, DNA was extracted by adding 850 ml of a sucrose ethylenediaminetetraacetic acid (EDTA) lysis buffer (0.75 M sucrose, 0.05 M Tris-Base, 0.02 M EDTA, 0.4 M NaCl, pH 9.0), and 100 mL of 10% sodium dodecyl sulfate to 2 mL bead-beating tubes containing the filters and 0.1 mm sterile glass beads, following a published protocol (Orsi et al., 2015). After bead beating for 1 min and heating at 99°C for 2 min, we added 25 mL of 20 mg × mL−1 proteinase K to the samples and incubated them at 55°C overnight. DNA was extracted and purified from the lysate using the DNeasy Blood and Tissue Kit (QIAGEN). The DNA from the sediments was extracted from 2 g using a sodium phosphate buffer and concentrated into 50 KDa Amicon filters, as described in previous publications (Vuillemin et al., 2019, 2020b). DNA concentrations were quantified using a Qubit 3.0 fluorometer (Thermo Fisher Scientific).
RNA was extracted from either 2 g of sediment, or from filters, using the FastRNA Pro Soil-Direct Kit (MP Biomedicals) following the manufacturer’s instructions, with final elution of templates in 40 μL PCR water (Roche) as described previously (Vuillemin et al., 2020a,b). Extraction from the filters was processed using 4 mL of RNA lysing solution together with silica glass beads from two Lysing Matrix E tubes, and homogenized using the FASTprep 5-G homogenizer (all MP Biomedicals). In order to maximize recovery of the RNA pellet, we added 4 μL glycogen at 1 μg × mL−1 prior to the 30 min isopropanol precipitation. All RNA samples were extracted in a HEPA-filtered laminar flow hood and set of pipets exclusively dedicated to RNA samples. Before and after each extraction, all surfaces were treated with RNAse-Zap and exposed to UV light for 30 min. Pipets were systematically autoclaved after use.
2.3. Stable-isotope probing incubations, 16S rRNA gene quantification
Water samples from 10 and 125 mwd at site 6 were selected for SIP incubations and amended with 13C-labeled diatom necromass produced from a culture of Chaetoceros socialis (Norwegian Culture Collection strain K1676), as previously described (Orsi et al., 2022). The concentrated mixture of dead diatom exopolysaccharides (dEPS) was used as 13C-labeled substrate (13C enrichment of >50%) for tracing in vitro heterotrophic microbial activities by amending 13C-labeled OM at a final concentration of 200 μg × g−1 (1%–3% of the in situ carbon concentrations). Sediments from 28 cm below the seafloor (cmbsf) at site 6 were selected for SIP incubations amended with 13C-bicarbonate (DIC). Flasks were incubated in triplicate in the dark for 18 h (dEPS) and 10 days (DIC), respectively, and frozen to −80°C to terminate the incubations. DNA was extracted from the incubation slurries in the home lab, as described above. DNA extracts were fractionated into 15 pools via density gradient according to published SIP protocols (Coskun et al., 2022), resuspended into 30 μL molecular grade (DEPC-treated) water, and quantified using a Qubit 3.0 fluorometer.
To determine shifts in the peak buoyant density of DNA of the incubations, qPCR assays targeting the V4 hypervariable region of 16S rRNA genes as a phylogenomic marker were carried out on the 15 density fractions. DNA templates were used in qPCR amplifications with updated 16S rRNA gene primer pair 515F (5′-GTG YCA GCM GCC GCG GTA A-3′) with 806R (5′-GGA CTA CNV GGG TWT CTA AT-3′) to increase the coverage of Archaea and marine clades (Parada et al., 2016) and run as previously described (Coskun et al., 2022). The reaction efficiencies in all qPCR assays were between 90% and 110%, with an r2 of 0.98. Gene copies were normalized to the wet weight of the sediment and volume of water filtered. The 13C-labeled fractions with highest gene copy numbers were selected and pooled for metagenomic library preparation (Supplementary Figure 2).
2.4. Library preparation
Amplicons of tagged partial 16S rRNA gene primers were run on 1.5% agarose gels; the bands were excised and purified with the QIA quick Gel Extraction Kit (QIAGEN) and the final eluted DNA quantified with the Qubit dsDNA HS Assay Kit (Thermo Fisher Scientific). Purified PCR amplicons containing unique barcodes from each sample were diluted to 1 nM solutions and pooled.
For metagenomes, initial DNA extracts were diluted to DNA concentrations of 0.2 ng × μL−1 and used in metagenomic library preparations with the Nextera XT DNA Library Prep Kit (Illumina Inc.). For metatranscriptomes, DNAse treatment, synthesis of complementary DNA, and library construction using specific barcodes were obtained from 10 μL of RNA templates by processing the Trio RNA-Seq kit protocol (NuGEN Technologies). Because the Trio RNAseq Ovation kit (NuGEN technologies) is biased against molecules with secondary structure such as ribosomal RNA and preferentially amplifies messenger RNA, performing a ribosomal RNA (rRNA) depletion step was not necessary. All libraries were quantified on an Agilent 2100 Bioanalyzer System, using the High Sensitivity DNA reagents and DNA chips (Agilent Genomics), diluted to 1 nM, and pooled according to the MiniSeq System Denature and Dilute Libraries Guide from Illumina. 500 μL library (1.8 pM) with 8 μL denatured PhiX control was sequenced in two separate runs using an paired-end Mid Output Kit (300-cycles) on the Illumina MiniSeq platform (Pichler et al., 2018).
2.5. Assembly and analysis
Demultiplexing and base calling were performed using bcl2fastq Conversion Software v. 2.18 (Illumina, Inc.). MiniSeq read trimming and assembly and OTU picking and clustering at 97% sequence identity were done using USEARCH (Edgar, 2013). Taxonomic assignments of 16S rRNA genes were generated by QIIME, version 1.9.1 (Caporaso et al., 2010), using the implemented BLAST method against the SILVA rRNA gene database, release 138 (Quast et al., 2013). All OTUs containing <10 sequences and which had no BLASTn hit were removed. Reads passing this quality control were then normalized by percentage of total sequencing depth per sample.
For metagenomic and metatranscriptomic MiniSeq reads, quality control, de novo assembly, and open reading frames (ORFs) searches were performed as described previously (Ortega-Arbulú et al., 2019). Demultiplexed reads were trimmed and paired-end reads assembled into contigs for a minimum contig length of 300 nucleotides, using CLC Genomics Workbench 9.5.4, a software toolkit for Next Generation Sequencing data analysis (QIAGEN). Paired reads were then mapped to the contigs using the aforementioned software by setting the following parameters (mismatch penalty = 3, insertion penalty = 3, deletion penalty = 3, minimum alignment length = 50% of read length, minimum percent identity = 95%). Coverage values were obtained from the number of reads mapped to a contig divided by its length (i.e., average coverage). Only contigs with an average coverage > 5 were selected for ORF searches and downstream analysis. This protocol does not assemble ribosomal RNA, and thus transcript results are only discussed in terms of messenger RNA. Protein-encoding genes and ORFs were extracted using FragGeneScan v. 1.30 (Rho et al., 2010).
For 16S rRNA gene amplicon datasets, each sample was sequenced to an average depth of 22,876 sequences per sample. Metagenomes were sequenced to an average depth of 6.3 million reads per sample. Metatranscriptomes spanning water column and seafloor habitats (n = 27) were sequenced with an average depth of 5.9 million reads, and after de novo assembly an average of 17,943 contigs per sample could be assembled. For the metagenomes prepared from the 13C-labeled SIP fractions, libraries were sequenced to an average depth of 6.6 million reads (Supplementary Tables 1, 2).
Taxonomic identifications were integrated with the functional annotations, performing BLASTp and BLASTx searches of ORFs against a large aggregated genome database of predicted proteins using the DIAMOND protein aligner version 0.9.24 (Buchfink et al., 2015). The aggregated genome database of predicted proteins includes the SEED1 and NCBI RefSeq databases updated with all predicted proteins from recently described high-quality draft subsurface metagenomic assembled genomes (MAGs) and single-cell assembled genomes (SAGs) from the NCBI protein database. The coverage of total annotated protein-encoding ORFs detected, as opposed to the number of reads mapping per kilobase per ORF (for example, RPKM), was selected to reduce potential bias from small numbers of “housekeeping” genes with potentially higher expression levels. This approach allows to assign ORFs from the metagenomic and metatranscriptomic data to higher-level taxonomic groups, thereby drawing environmental conclusions about their specific metabolic traits and activities (Breitwieser et al., 2019). The complete bioinformatics pipeline has been previously published (Orsi et al., 2020b). Statistical analyses were performed using RStudio v. 3.3.3 with the Bioconductor package (Huber et al., 2015).
All scripts and codes used to produce sequence analyses have been posted on GitHub with a link to the instructions on how to conduct the scripts.2 All metagenome, metatranscriptome, and 16S rRNA gene data are publicly accessible in NCBI through BioProject number PRJNA525353.
2.6. Phylogeny of functional genes
All 16S rRNA gene amplicon sequences were aligned with SINA online v.1.2.11 (Pruesse et al., 2007) and inserted in the SILVA 16S rRNA SSU NR99 reference database tree, release 138 (Quast et al., 2013), using the maximum parsimony algorithm without allowing changes of tree typology. Partial OTU sequences closely affiliated with known nitrifiers were selected with their environmental references and plotted in separate archaeal and bacterial Maximum Likelihood RAxML phylogenetic trees, using rapid bootstrap analysis and selecting the best trees among 100 replicates using ARB (Ludwig et al., 2004).
Phylogenetic analyses of the predicted ammonia monooxygenase (amoA) and copper-containing nitrite reductase (nirK) gene proteins were performed for all the corresponding annotated taxa in the metagenomes and metatranscriptomes, using 185 and 133 aligned amino acid sites, respectively (Garbeva et al., 2007; Alves et al., 2018). For each of the two marker gene phylogenies (amoA, nirK), all ORFs annotated to those genes from the bioinformatics pipeline were aligned against their top two BLASTp hits in the NCBI-nr and SEED databases using MUSCLE (Edgar, 2004). Conserved regions of the alignments were selected and phylogenetic analyses of the predicted proteins were performed in SeaView version 4.7 (Gouy et al., 2010) using RAxML (Stamatakis, 2014) with BLOSUM62 as the evolutionary model and 100 bootstrap replicates.
3. Results
3.1. Water column geochemistry, taxonomic, and functional gene compositions across sampling locations
Consistent with prior studies (Kuypers et al., 2005; Lavik et al., 2009), an oxycline was observed between 50 and 95 mwd that exhibited O2 concentrations spanning 100–40 μM O2 (Figure 1A). Below 65 to 95 mwd, an OMZ defined as having <60 μM O2 (Wright et al., 2012) was detected at all sites sampled along the shelf (Figure 1A). The profiles measured for chl-a fluorescence (Siccha and Kucera, 2018) display maximum concentrations (i.e., 1.25 mg × m3) in the surface ocean at site 6, with a general trend decreasing with water depth that runs in parallel to the oxygen profiles (Figure 1B). Silica covarying with chl-a concentrations may reflect productivity by siliceous plankton like diatoms (Inthorn et al., 2006b), otherwise sediment suspension in nepheloid layers (Inthorn et al., 2006a). Turbidity in the water column, measured as nephelometric turbidity units (ntu), is detectable (i.e., ntu > 1) in the shelf bottom waters between site 2 and site 4 (Figure 1B). Together with the chl-a fluorescence profile at site 4, these data indicate the presence of water eddies along the continental shelf with suspension of seafloor sediments into the water column (Figure 1B). This is typical of the winter months on the Namibian coast that present more well-mixed water conditions (Brüchert et al., 2009; Ohde and Dadou, 2018).
Concomitant trends in NH4+, nitrite, and nitrate profiles can indicate nitrification, denitrification, and anammox processes (Figure 1C). Based on measured concentrations, there appear to be different processes at sites 2 and 3 compared to sites 4 and 6 where phosphate concentrations, respectively, decrease and increase in the OMZ (Supplementary Figure 3). Site 4 displays geochemical features that are indicative of denitrification with some nitrification processes above and in the vicinity of the OMZ, i.e., respectively, a decrease in nitrate and increase in nitrite and NH4+concentrations between 50 and 100 mwd (Figure 1C), whereas sites 2 and 3 display decreasing concentrations in NH4+ and nitrite in the OMZ while nitrate increases (Figure 1C). Such concomitant decrease in nitrite and NH4+ could also be indicative of anammox processes in the upper part of the OMZ (O2 < 100 μM), i.e., below 80, 70, 110, and 30 mwd at sites 2, 3, 4, and 6, respectively. However, geochemical profiles in the water column cannot be attributed to in situ microbial processes of nitrogen cycling only, but also inform on nutrient sources and transport. NH4+ concentrations in bottom waters at site 2 reveal that benthic releases locally occur on the shelf with transport in the benthic boundary layer along the shore (Füssel et al., 2012; Neumann et al., 2016), potentially explaining the lowered water depth of the OMZ at site 4 along with a local increase in nitrite and NH4+concentrations between 50 and 100 mwd. In the sediment underlying bottom waters of the OMZ at site 6, pore water chemical analysis indicates that nitrate and nitrite were consumed quickly at the sediment surface, followed by an increased accumulation of NH4+ with depth (Figure 1D). The sediments exhibit a redox gradient spanning dysoxic conditions (~25 μM O2) at the seafloor surface to sulfidic conditions at 30 cmbsf (Supplementary Figure 4). In contrast to site 2 (Figure 1C), NH4+ does not diffuse across the SWI at site 6 (Figure 1D).
In the water column, sequencing results of 16S rRNA genes show that the microbial assemblages mostly consist of Cyanobacteria, Bacteroidota (i.e., Bacteroidia, Chlorobia, and Ignavibacteria), and Alpha- and Gammaproteobacteria. The class Nitrososphaeria (former phylum Thaumarchaeota), which represents 10% of the total microbial composition in surface waters at all sites, sums up to 30% of total 16S rRNA genes in the OMZ waters at site 6. In the sediment, the 16S rRNA gene assemblage shows a clear predominance of Chloroflexota, complemented with mostly Desulfobacterota and Gammaproteobacteria, and only 2% on average of Archaea. The copy number of 16S rRNA genes obtained via qPCR assays is highest at the SWI (i.e., log10 = 8) and remains relatively constant (i.e., log10 = 6–7) with sediment depth. The corresponding non-metric multidimensional scaling (NMDS) analysis based on 12,200 OTUs clearly indicates a significant shift in the community composition from the surface ocean across the OMZ waters down to the SWI, and further into the sediment (Supplementary Figure 5).
Analysis of the metagenomes provided a way to cross-check taxonomic assignments based on 16S rRNA primers. Comparison between the two showed a similar archaeal distribution across water column samples, whereas ORFs assigned to Proteobacteria were somehow increased in the metagenomes. In the sediment, the number of ORFs assigned to Gammaproteobacteria apparently increased at the expenses of those of Chloroflexota. In the metatranscriptomes, protein-encoding ORFs were by far more assigned to Desulfobacterota along the shelf waters (sites 2 and 4), whereas those from northward sampling sites mostly indicated Bacteroidota, Alpha- and Gammaproteobacteria, and Nitrososphaeria as the main metabolically active phyla. In the sediment, Firmicutes, Desulfobacterota, and Gammaproteobacteria represented about 50% of the expressed ORFs. The NMDS analysis based on all annotated protein-encoding ORFs obtained from our metagenomes and metatranscriptomes clearly separates all of the metagenome and metatranscriptome samples, plotting samples at different sampling locations spanning from the surface ocean across OMZ waters to sediment core top to bottom (Supplementary Figure 5).
3.2. Diversity and abundance of nitrogen cycling populations
Relative abundances based on 16S rRNA genes were established for sub-populations putatively involved in nitrogen fixation (among Actinobacteriota, Cyanobacteria, Firmicutes, and Alphaproteobacteria), nitrifiers (among Nitrososphaeria, Nitrospinota, Nitrospirota, and Gammaproteobacteria), and nitrate reducers (among Bacteroidota, Firmicutes, Nitrospirota, Alphaproteobacteria, Desulfobacterota, and Gammaproteobacteria) to determine the main taxa driving the nitrogen cycle across all sites sampled along the shelf (Figure 2). Taxa involved in nitrogen fixation in the ocean surface are more abundant at offshore stations (site 7 and 8), corresponding predominantly to cyanobacterial populations, whereas diazotrophic Alphaproteobacteria were exclusively identified at the SWI and in the uppermost sediment (Figure 2). Populations potentially carrying out nitrification increase from the surface ocean into the OMZ waters, especially at site 6 and 7 where the top part of the oxycline is located at shallower water depths (i.e., 20–40 mwd).
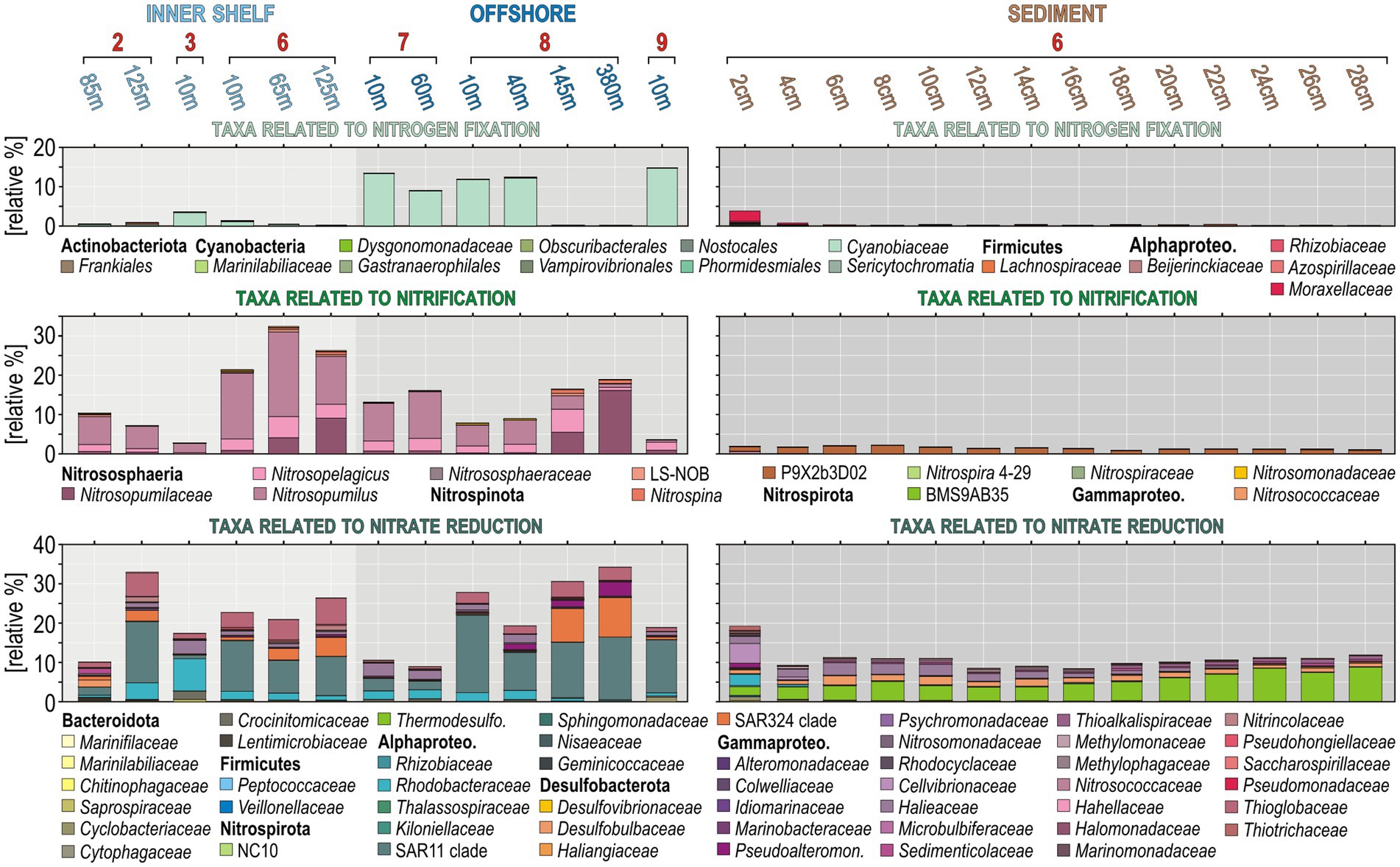
Figure 2. Quantification and taxonomy of 16S rRNA genes grouped into functional guilds for potential nitrogen fixers, nitrifiers, and nitrate reducers. (Top to bottom) Relative abundances and taxonomy of taxa potentially involved in nitrogen fixation (top), nitrification (middle), and nitrate reduction (bottom) in the water column across successive sampling sites (left) and in the sediment (right).
Ammonia-oxidizing archaea (AOA) are the most abundant nitrifiers in the water column, constituting up to 30% of the entire 16S rRNA gene assemblage in OMZ waters. The detailed phylogenetic analysis indicates the presence of Candidatus (Ca.) Nitrosopelagicus and Ca. Nitrosopumilus in the water column, and Ca. Nitrososphaera in the sediment (Figure 3A), respectively, separating planktonic (27 OTUs) and benthic (9 OTUs) populations. Nitrite-oxidizing bacteria (NOB) among Nitrospinota are present at very low 16S rRNA relative abundances (Figure 2), but are taxonomically diverse with 60 OTUs distributed among candidate clade LS-NOB and Nitrospinaceae in the water column, and candidate clade P9X2b3D02 in the sediment (Figure 3B). Other NOB and potential ammonia-oxidizing bacteria (AOB) are not abundant either (Figure 2), including diverse taxa among the Nitrospirota (8 OTUs) and Gammaproteobacteria (20 OTUs), respectively, affiliated with Nitrospira, Nitrosomonas, and Nitrosococcus (Figure 3B).
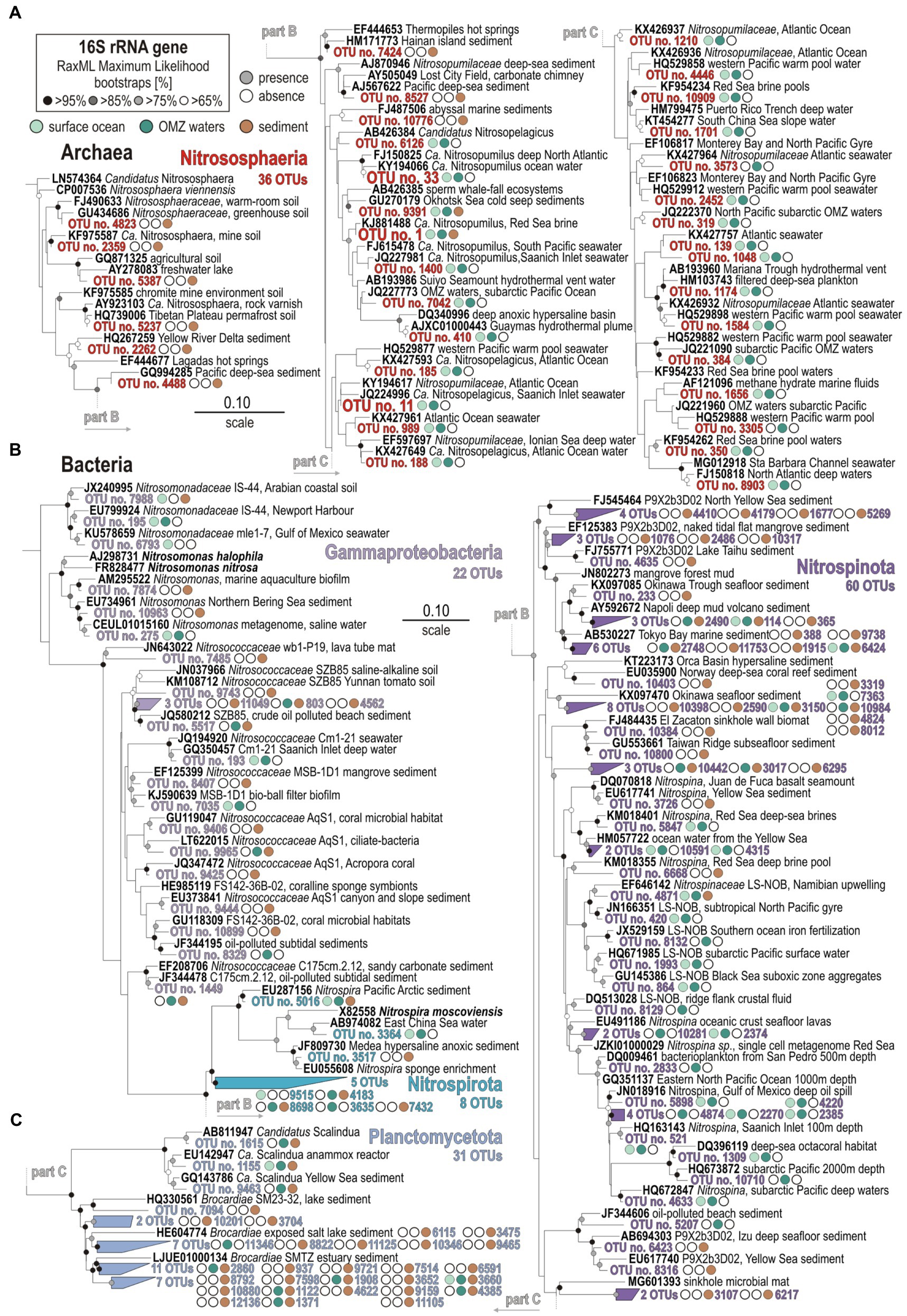
Figure 3. Phylogenetic analysis of partial 16S rRNA gene amplicons for ammonia-oxidizing archaea (A), ammonia- and nitrite-oxidizing bacteria (B), and anammox bacteria (C). RAxML Maximum Likelihood archaeal and bacterial trees selected among 100 replicates for all partial 16S rRNA transcripts (V4 hypervariable region). Presence/absence of a 16S rRNA gene with the surface ocean (light green), OMZ waters (dark green), and sediment (brown) is signified by full vs. empty circles. Bold types signify accession numbers and cultivated species, whereas regular font indicates the sequence isolation sources.
Nitrate-reducing bacteria potentially involved in denitrification and DNRA represent up to 30% of the total 16S rRNA gene assemblages in OMZ deep waters. Predominant taxa in the water column are affiliated with the alphaproteobacterial family Rhodobacteraceae and Thalassospiraceae, clade SAR11 (formerly deltaproteobacterial) clade SAR324, and gammaproteobacterial family Pseudoalteromonadaceae and Thioglobaceae (Figure 2). The SWI and surface sediment are apparently colonized by Rhodobacteraceae, Cellvibrionaceae, Halieaceae, and Thiotrichaceae, along with Thermodesulfovibrionaceae and Desulfobulbaceae, which are also known to include sulfur-oxidizing and sulfate-reducing bacteria potentially carrying out DNRA. Consistent with the depletion of pore water nitrate, the relative abundances of these taxa decrease in shallow sediment as the sediment becomes sulfidic (Supplementary Figure 4).
We also searched for 16S rRNA gene sequences affiliated with Planctomycetota known to perform anammox. We identified 31 OTUs that closely match Ca. Scalindua and Ca. Brocadia, among which only 10 OTUs are derived from OMZ water samples, whereas 21 OTUs are solely present in the sediment (Figure 3C). However, these 31 OTUs are minor in terms of relative abundances.
3.3. Stable-isotope probing incubations and 13C-labeled taxa
After 18 h of incubation in the dark at 10°C, the 13C-dEPS incubations showed 13C-labeling of 16S rRNA genes, defined by a shift in peak DNA buoyant density, in the surface ocean (10 mwd) and OMZ (125 mwd), resulting in “isotopically heavier” (i.e., 13C-enriched DNA) 16S rRNA genes compared to the controls that received the unlabeled substrate (Supplementary Figure 2). Similarly after 10 days of incubation in the dark at 10°C, the 13C-bicarbonate incubations amended with subseafloor sediment (Orsi et al., 2020b) showed an increased buoyant density of 16S rRNA genes compared to the unlabeled controls. This indicates that 13C-labeling of microbes synthesizing new DNA had occurred, which had assimilated the added 13C-dEPS and 13C-DIC into their biomass.
Metagenomic sequencing of these “isotopically heavy DNA” SIP fractions shows that most of the planktonic bacteria that had assimilated the added 13C-dEPS substrate are affiliated with Bacteroidota, both within the 10 mwd and 125 mwd (OMZ) incubations. Additionally, Nitrososphaeria increase in relative abundance at the highest CsCl densities, indicating that putative heterotrophic Nitrososphaeria had also assimilated 13C-dEPS in the SIP incubations. Taxonomic affiliations of the ORFs in the sediment heavy SIP metagenomes reveal 13C assimilation mainly by the Desulfobacterota, Gammaproteobacteria, Chloroflexota, and Actinobacteria (Supplementary Figure 2).
3.4. Potential and expression of functional marker genes involved in nitrogen cycling
Here, the relative % of total prokaryotic ORFs identified in the metatranscriptomes is compared to those present in the metagenomes in order to assess levels of metabolic expression in the enzymology of nitrogen cycling. The focus is set on ORFs encoding genes involved in nitrogen regulatory metabolism, diazotrophy, nitrification, denitrification, and simple organic nitrogen compound oxidases, reductases, and transporters (Lam and Kuypers, 2011; Martínez-Espinosa et al., 2011). The presence and expression of ORFs with correspondence to PII proteins, ammonia lyases, and ammonia ligases were used to trace nitrogen regulatory metabolism. Diazotrophy and nitrification were investigated via protein-encoding genes involved in nitrogen fixation (nif), ammonium monooxygenase (amo), nitrite oxidoreductase (nxr), nitronate monooxygenase also known as 2-nitropropane dioxygenase (nmo), along with ureases, nitrilases, and cyanases. ORFs with correspondence to gene subunits encoding the respiratory nitrate reductase (nar), periplasmic nitrate reductase (nap), nitrite reductase (nir), nitric oxide reductase (nor), nitrous oxide reductase (nos), and ammonia-forming nitrite reductase (nrf) were sorted to trace denitrification and DNRA. For organoclastic processes in link to denitrification, genes encoding nitroreductases (ntr), nitrilases (nit) and formate dehydrogenase (fhn), as well as for nitrate/nitrite, NH4+ and urea transporters (Figure 4) were investigated. In addition, for hydrazine hydrolase and hydrazine oxidoreductase (hzo) which are involved in anammox processes (Karlsson et al., 2009), a single ORF was detected in the metagenome from 50 mwd at site 4.
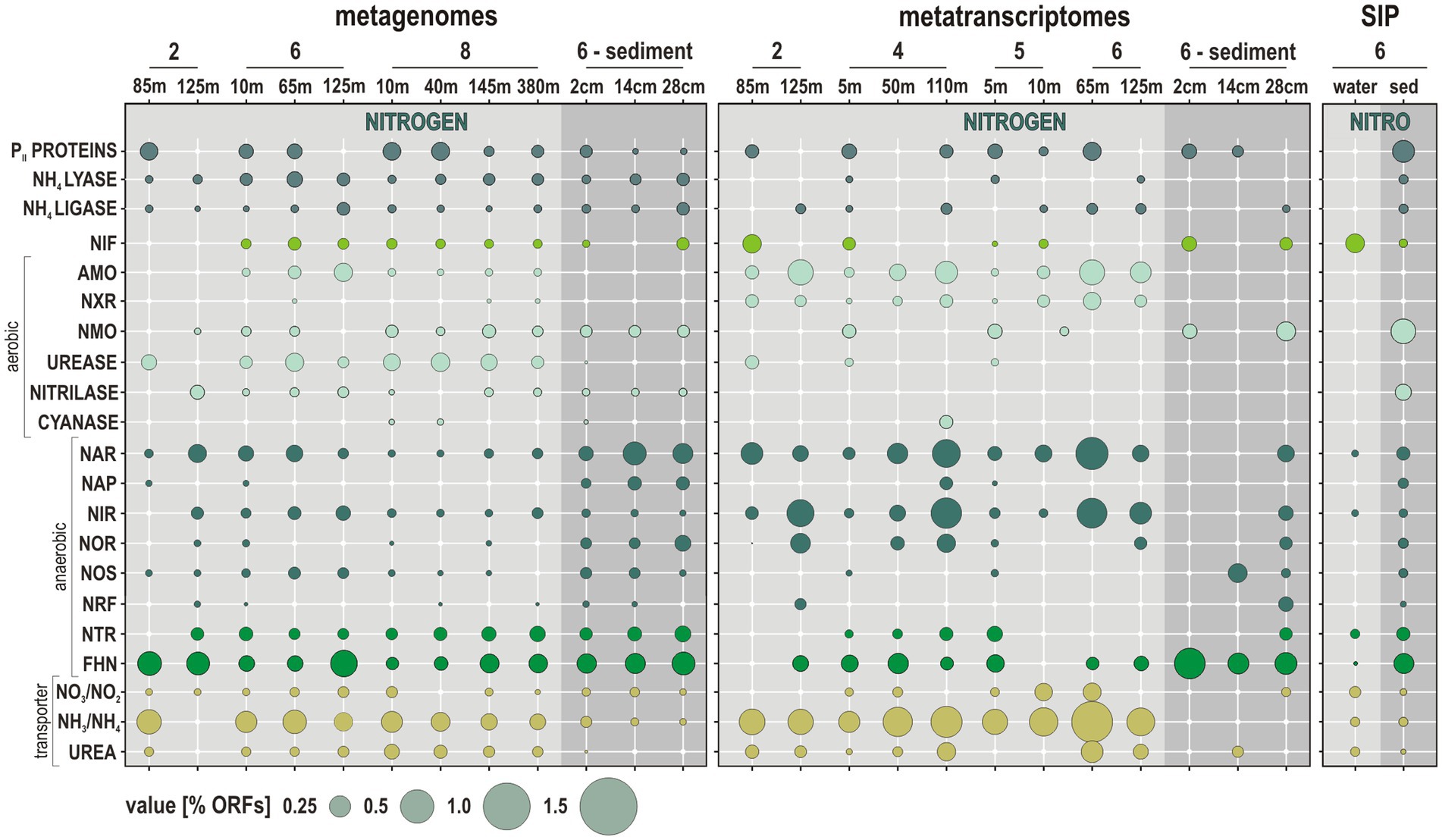
Figure 4. Metabolic potential and activities related to nitrogen cycling along the Namibian coast. Bubble plot showing the relative abundances of metabolic functions [% total ORFs] in the metagenomes, metatranscriptomes, and stable-isotope probing (SIP) sediment incubations (left to right) assigned to functional marker genes involved in nitrogen cycling. Abbreviations: PII PROTEINS: nitrogen regulatory proteins/NIF: nitrogen fixation/AMO: ammonium monooxygenase/NXR: nitrite oxidoreductase/NMO: nitronate monooxygenase/NAR: nitrate reductase/NAP: periplasmic nitrate reductase/NIR: copper-containing nitrite reductase/NOR: nitric oxide reductase/NOS: nitrous oxide reductase/NRF: ammonia-forming cytochrome nitrite reductase/NTR: nitroreductases/FHN: formate dehydrogenase.
The relative expression of ORFs attributed to PII proteins, NH4+ lyases and nif genes is indicative of related metabolic activity limited to surface waters, whereas those of NH4+ ligases tend to increase into OMZ waters. ORFs related to amo and nxr genes, with concomitant nitrite and NH4+ transporters, are expressed at relatively high % in the water column at all sites (Figure 4). The number of these ORFs shows a clear tendency to increase toward the oxycline and into OMZ waters, whereas they are below detection in the anoxic sediment. This indicates that nitrification processes are active and even increase in the vicinity of the oxycline. ORFs related to ureases and urea transporters are mostly expressed in oxic waters along the shelf. Expression of ORFs encoding nitrilases, cyanases and nmo genes is minor and only detectable in the surface ocean and oxic waters (Figure 4).
The relative % of expressed ORFs assigned to nar and nir genes increases from surface into OMZ waters at all sites (Figure 4). The relative % of ORFs assigned to nor genes follows the same trend, with expression mostly detected in OMZ waters. In comparison, expression of ORFs assigned to nos genes is minor and limited to surface waters. Although nos genes are usually considered to be unique to denitrifying bacteria (Scala and Kerkhof, 1999), their detection currently restricted to the surface ocean may indicate aerobic consumption of N2O gas emissions (Sun et al., 2017). Ammonification mediated by nrf gene transcription is only expressed in bottom waters on the shelf and in the sediment. Expressed ORFs assigned to nitroreductases were only detected at site 4, where eddies promote water column oxygenation and sediment suspension along the shore (Inthorn et al., 2006a). ORFs assigned to fhn genes, which is part of the respiratory chain of denitrification as well as of the acetogenic W-L pathway (Einsle and Kroneck, 2004), consistently increase from ocean surface into OMZ waters and underlying sediment (Figure 4). At all sites, transcripts encoding the amo, nxr, and nir genes and NH4+ transporters display the highest expression levels (calculated as % of total reads; Figure 5A), with taxonomic assignments demonstrating that Nitrososphaeria and Nitrospinota couple nitrification with denitrification (Lawton et al., 2013) in coastal OMZ waters (Figure 5B).
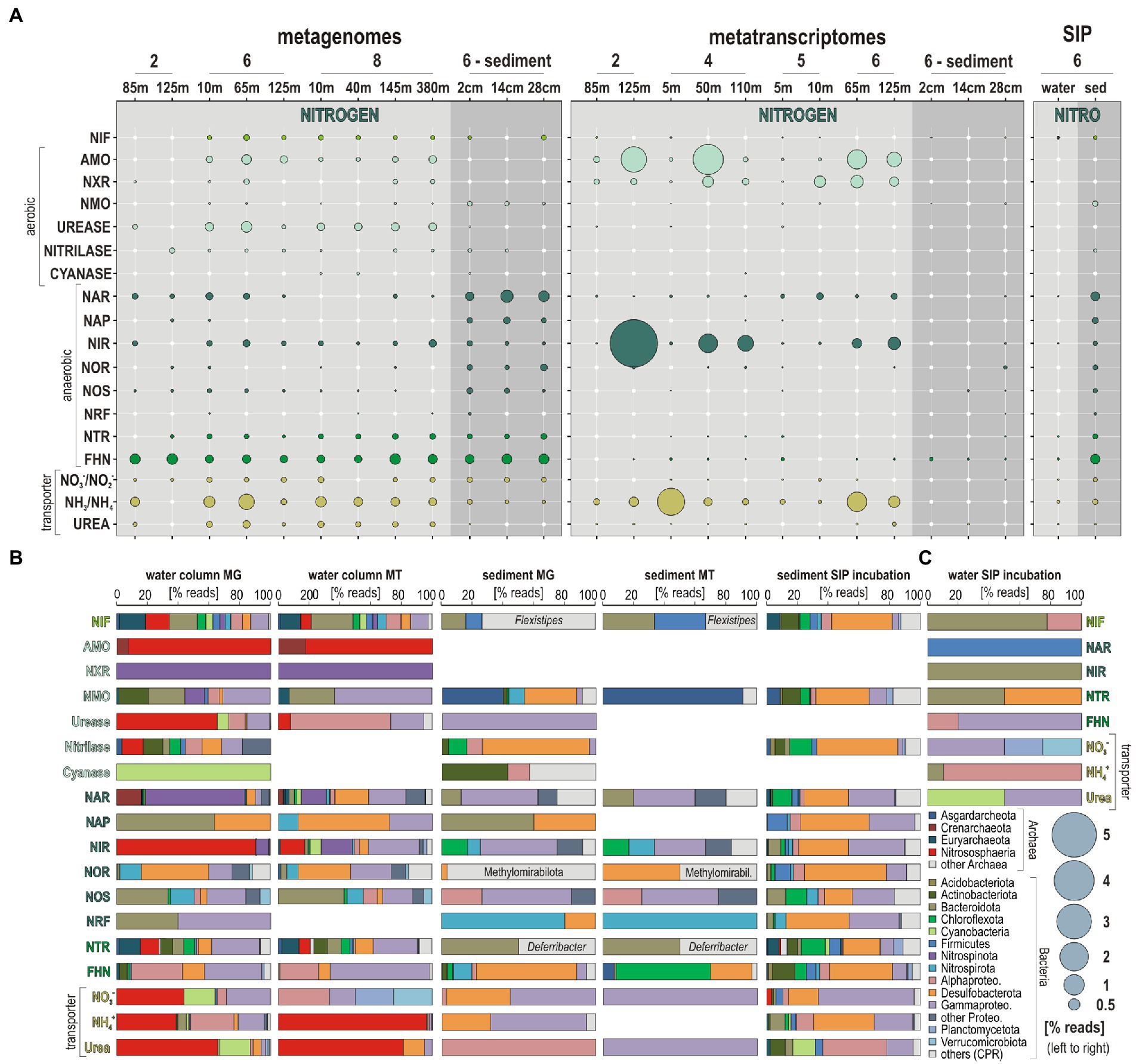
Figure 5. Metabolic functions and activities related to nitrogen cycling in the water column, sediment and SIP incubations, and the corresponding taxonomic assignments at the phylum level. (A) Bubble plot showing the relative potential and expression of metabolic functions [% total reads] assigned to nitrogen cycling in the metagenomes, metatranscriptomes, stable-isotope probing (SIP) water, and sediment incubations (left to right). (B) Taxonomic bar charts [% reads] for the corresponding functional marker genes related to nitrogen cycling at the phylum level in the metagenomes (MG), metatranscriptomes (MT), and anaerobic SIP incubations with sediment and 13C-labeled bicarbonate (DIC). The last column on the left (C) displays taxonomy of functional marker genes identified in aerobic incubations with water and 13C-labeled diatom mixture (dEPS). Abbreviations: NIF: nitrogen fixation/AMO: ammonium monooxygenase/NXR: nitrite oxidoreductase/NMO: nitronate monooxygenase/NAR: respiratory nitrate reductase/NAP: periplasmic nitrate reductase/NIR: copper-containing nitrite reductase/NOR: nitric oxide reductase/NOS: nitrous oxide reductase/NRF: ammonia-forming cytochrome nitrite reductase NTR: nitroreductases/FHN: formate dehydrogenase.
The 13C-labeled functional marker genes related to nitrogen cycling in SIP metagenomes obtained from water incubations reveal concomitant in vitro processes of nitrogen fixation (nif), denitrification (nar, nir), degradation (ntr, fhn), and uptake of nitrogen compounds (NO2−, NH4+, urea transporters) from 13C-dEPS, with denitrification initiating within 18 h (Figure 4). In SIP metagenomes from sediment incubations, the 13C-labeled functional marker genes are indicative of the complete denitrification pathway along with reduction of organic nitriles and nitronates (nitrilases, nmo). Because it is hard to discriminate ORFs genuinely occurring by isotopic enrichment in the “heavy” DNA fraction from those with high GC content (Coskun et al., 2022) and because the isotopically “light” DNA fractions were not sequenced, it may well be that the 13C-labeled ORFs with low GC content are not represented in the analysis.
3.5. Taxonomic assignment of functional marker genes, phylogenetic analysis
To identify the main microbial constituents involved in nitrogen cycling across the coastal OMZ, the taxonomic assignment of the ORFs corresponding to the aforementioned functional markers genes was summed up separately for the metagenomes, metatranscriptomes, and SIP incubations (Figure 5), according to the isolation source (i.e., water column or sediment). Briefly, populations with metabolic potential to fix nitrogen are taxonomically diverse (e.g., Bacteroidota, Cyanobacteria, and Alphaproteobacteria), but their corresponding expression levels of nif proteins are very low (Figures 5A,B). Nitrososphaeria are clearly the main actors in active transcription of amo and nir genes, nitroreductases, ureases, and urea transporters in the water column. Transcription levels of nxr, nar, and nir genes by Nitrospinota are relatively high as well (Figures 5A,B). Phyla actively encoding the complete denitrification pathway (i.e., nar, nap, nir, nor, and nos genes) in the water column include Nitrospirota, Desulfobacterota, and Gammaproteobacteria (Figure 5B). Although these processes are minor in the sediment (Figures 4, 5A), phyla that express the related ORFs are mostly identified as Proteobacteria, and accessorily as Nitrospirota and Bacteroidota (Figure 5B). Expression of fhn genes by Chloroflexota was considered indicative of acetogenesis (Vuillemin et al., 2020a).
The phylogenetic analyses of amoA and nirK gene sequences confirm that Nitrososphaeria (Figure 6A) and Nitrospinota (Figure 6B) are the main drivers of nitrifier denitrification (Fernandez and Farias, 2012; Lawton et al., 2013). Assignments of amoA genes (Figure 6A) show that only AOA among the class Nitrososphaeria are metabolically active, whereas AOB such as Nitrosomonas or Nitrosococcus were not identified. Canonical denitrifiers actively expressing nirK genes are assigned to Alpha- and Gammaproteobacteria (Figure 6B).
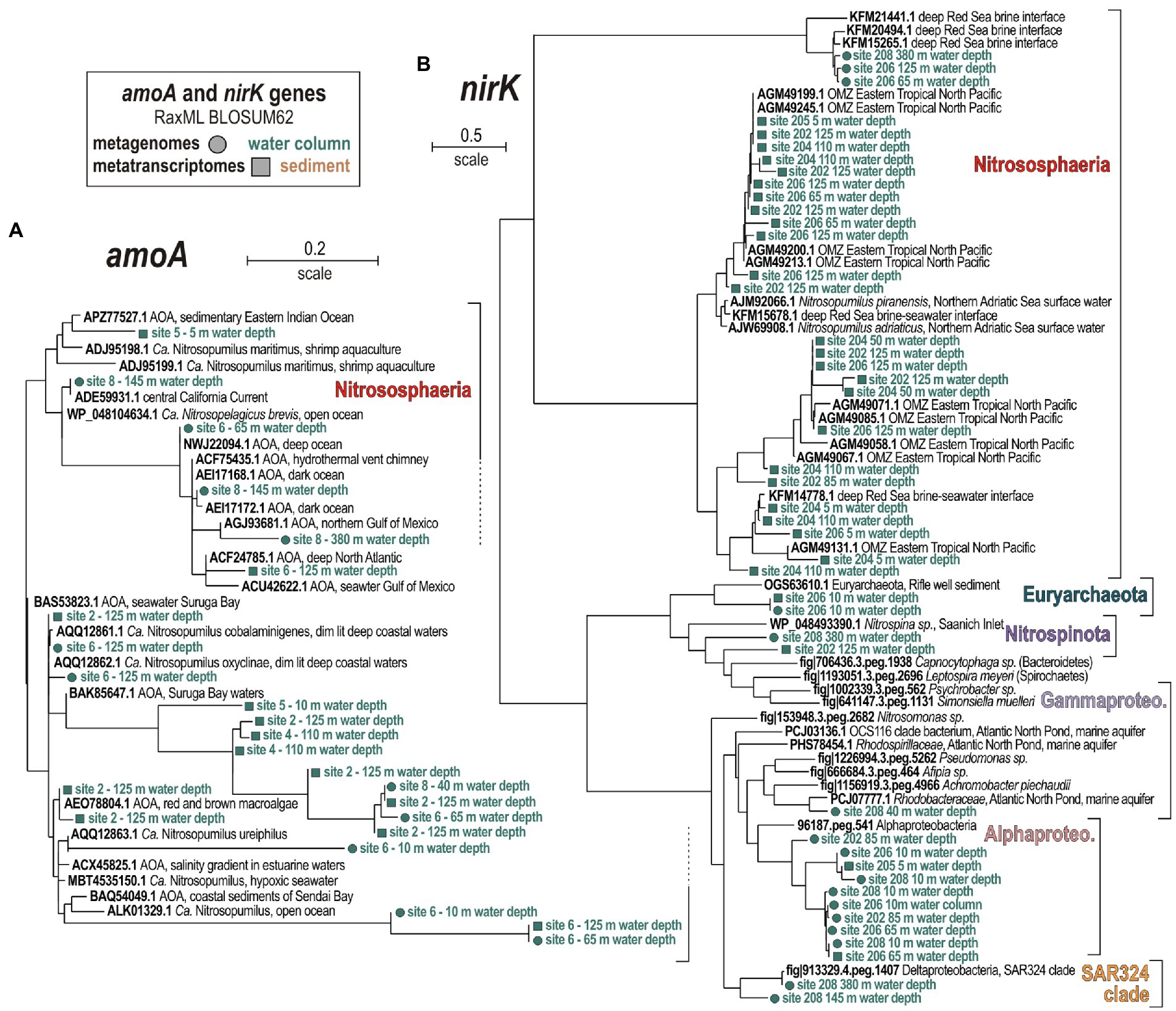
Figure 6. Phylogenetic analyses of predicted proteins encoded by (A) ammonia monooxygenase subunit A, and (B) nitrite reductase subunit K as the selected marker genes in the metagenomes and metatranscriptomes, based on RAxML using BLOSUM62 as the evolutionary model. (A) Phylogenetic tree of all amoA ORFs (185 aligned amino acid sites) detected in the metagenomes (circles) and metatranscriptomes (squares). (B) Phylogenetic tree of all nirK ORFs (133 aligned amino acid sites) detected in the metagenomes (circles) and metatranscriptomes (squares). Green and brown font, respectively, indicate water or sediment as the isolation source of the sequences.
3.6. Carbon assimilation and sensitivity to oxygen depletion
Autotrophic and heterotrophic carbon assimilation in nitrifiers was assessed, on the one hand, by looking for ORFs related to ribulose-1,5-diphosphate carboxylase (RuBisCO) in the Calvin-Benson-Bassham (CBB) cycle, ATP citrate lyase (acly) as the first step of the reductive tricarboxylic acid cycle (TCA) cycle, carbon monoxide dehydrogenase (codh) involved in CO oxidation and CO2 reduction, inclusive of the Wood-Ljungdahl (W-L) pathway, and acetyl-coenzyme A carboxylase (acc) as a step in the aerobic 3-hydroxypropionate/4-hydroxybutyrate (HP/HB) and anaerobic dicarboxylate/4-hydroxybutyrate (DC/HB) cycle as well as in anaplerotic CO2 assimilation (Erb, 2011); on the other hand by looking for acetyl-coenzyme A synthetase (acs) as the last metabolic step in glycolysis, and citrate synthase (cs) and pyruvate/lactate dehydrogenase (pdh) as the first metabolic steps in TCA cycle and lactate/pyruvate fermentation (Figure 7).
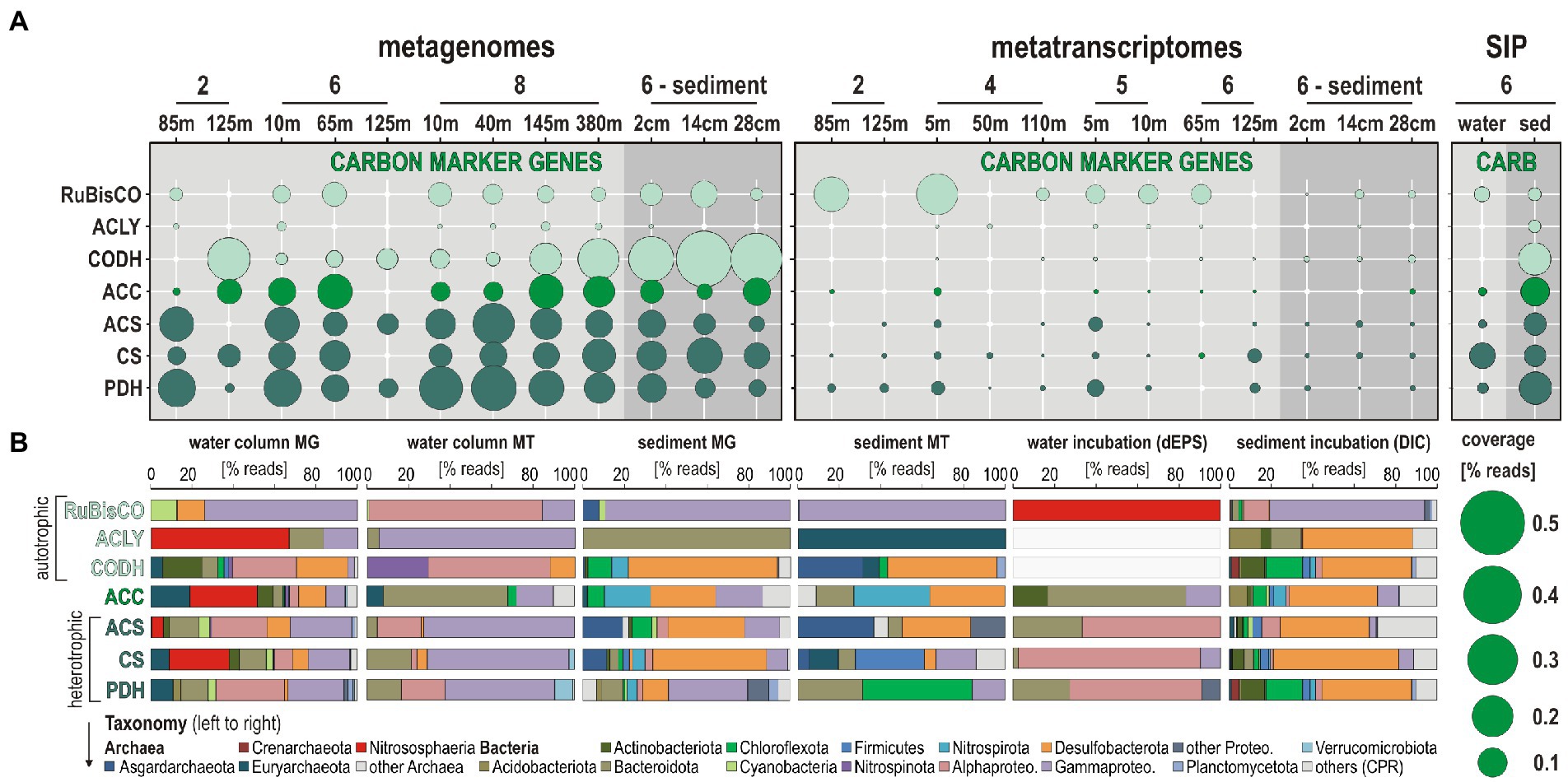
Figure 7. Metabolic functions and activities related to autotrophic and heterotrophic carbon assimilation in the water column, sediment, and SIP incubations, and the corresponding taxonomic assignments at the phylum level. (A) Bubble plot showing the relative potential and expression of metabolic functions [% total reads] assigned to marker genes involved in autotrophic-heterotrophic carbon assimilation in the metagenomes, metatranscriptomes, stable-isotope probing (SIP) water, and sediment incubations (left to right). (B) Taxonomic bar charts [% reads] for the corresponding marker genes at the phylum level in the metagenomes (MG), metatranscriptomes, (MT), and SIP incubations with water and 13C-labeled dEPS and with sediment and 13C-labeled bicarbonate (DIC). Abbreviations: RuBisCO: ribulose-1,5-diphosphate carboxylase (i.e., CBB cycle)/ACLY: ATP-citrate lyase (i.e., reductive TCA cycle)/CODH: carbon monoxide dehydrogenase (i.e., Wood-Ljungdahl pathway)/ACC: acetyl-coenzyme A carboxylase (i.e., HP/HB-DC/HP cycle)/ACS: acetyl-coenzyme A synthetase (i.e., glycolysis)/CS: citrate synthase (e.g., TCA cycle)/PDH: pyruvate dehydrogenase (i.e., lactate/pyruvate fermentation).
ORF expression levels (i.e., % total transcripts) related to RuBisCO genes are highest in both oxic and dysoxic coastal waters (Figure 7A) involving mostly the phyla Cyanobacteria and Proteobacteria (Figure 7B). In the SIP metagenomes incubated with water, RuBisCO genes are entirely assigned to Nitrososphaeria, whereas expression of RuBisCO genes in the seafloor and sediment SIP incubations is ruled by Gammaproteobacteria. In comparison, expression levels of ORFs assigned to acly, codh, and acc genes are low, but tend to slightly increase offshore (sites 5 and 6). Heterotrophic processes assessed via transcription of acs, cs, and pdh genes are detectable across all water column sampling sites and sediment, with generally higher levels of ORF expression in coastal waters than in the sediment (Figure 7A). ORFs diagnostic of aerobic autotrophic carbon fixation (acc, i.e., HP/HB cycle) are expressed by pelagic taxa among Bacteroidota and Gammaproteobacteria, with little detection of Euryarchaeota and Chloroflexota, whereas in the sediment, ORFs indicative of the anaerobic DC/HB cycle are expressed by Nitrospirota and Desulfobacterota.
In contrast, SIP metagenomes from water incubations only show 13C-labeling of the acc genes with taxonomic assignment to Bacteroidota. Assignments of ORFs related to glycolysis (acs) and TCA cycle (cs) indicate members of the Bacteroidota, Alpha- and Gammaproteobacteria as main actors of OM degradation in the water column and water incubations, and Euryarchaeota, Firmicutes, and Desulfobacterota in the seafloor and sediment incubations (Figure 7B). ORFs encoding genes for anaerobic fermentation (pdh), the W-L pathway (codh) and/or anaplerotic CO2 assimilation (Erb, 2011) are mostly expressed by Nitrospinota and Alpha- and Gammaproteobacteria in the OMZ, whereas in the sediment, such OM fermentation processes are apparently driven by Chloroflexota and Asgardarchaeota (Figure 7).
ORFs assigned to genes encoding proteins for superoxide dismutase (sod), cytochromes cbb3, bd2, and b (cyt b/d; Kalvelage et al., 2015), sensor histidine kinase (shk), and fumarate dehydrogenase (frd) were sorted to assess whether carbon assimilation pathways are associated with decreasing oxygen concentrations. Although metabolic potential based on % total reads tends to increase toward the oxycline, patterns of expression level remain inconspicuous, involving shk gene expression by Nitrososphaeria, Gammaproteobacteria, and Bacteroidota in the water column (Supplementary Figure 6).
4. Discussion
4.1. Active nitrifying and denitrifying populations along the Namibian coast
The taxonomic affiliation of 16S rRNA genes clearly separated two populations corresponding to the water column and sediment, the former being predominantly composed of diverse Proteobacteria, Cyanobacteria, Bacteroidota, and Nitrososphaeria (Ulloa et al., 2013; Ganesh et al., 2014), whereas the latter consisted mainly of Chloroflexota, Nitrospirota, and Desulfobacterota (Supplementary Figure 5).
The relative abundances of presumed nitrogen-fixing populations were most abundant offshore. Those of nitrifying populations apparently thrived in OMZ waters on the shelf toward the north where lateral current increases ventilation of bottom waters (Ohde and Dadou, 2018), whereas denitrifying populations increased with water depth at all sites (Figure 2). Consistent with this initial distribution of metabolic guilds (Figure 2), the number of expressed ORFs encoding amoA and nxr genes involved in nitrification, and nar, nir and nor genes in denitrification increased from surface ocean down into OMZ waters (Figure 4). Relative expression of NH4+, nitrite, and urea transporters also increased down into OMZ waters along with nitroreductases and formate dehydrogenase (Einsle and Kroneck, 2004), which altogether points to concomitant metabolic activities in autotrophic, heterotrophic nitrification and denitrification (Figure 4).
Based on phylogenetic analysis of 16S rRNA and amoA genes (Figures 2, 6A), pelagic AOA populations included taxa assigned to Ca. Nitrosopumilus (spp. maritimus, cobalaminigenes, oxyclinae, and ureiphilus; Ijichi and Hamasaki, 2011) and Ca. Nitrosopelagicus (sp. brevis), whereas benthic ones were clearly distinct as they were all related to Ca. Nitrososphaera (sp. viennensis). Based on 16S rRNA genes, presumed AOB populations were affiliated with Nitrosomonas sp., Nitrosococcus sp. and Nitrospira sp. among respective phyla Gammaproteobacteria and Nitrospirota, in the absence of alphaproteobacterial sequences in the assemblage, e.g., Nitrobacter sp. (Figure 3B). However, these bacteria were not identified in our phylogenetic analysis of amoA genes (Figure 6A). Assignments of 16S rRNA genes also evidenced Nitrospina-related taxa as the prevalent pelagic NOB (Sun et al., 2019). Although both AOA and NOB were identified in the taxonomic composition of 16S rRNA genes in the sediment, we could not detect any metabolic potential or activities toward benthic nitrification (Figure 5).
Several sequences were affiliated with the anammox-related taxa Ca. Scalindua and Ca. Brocadia in the OMZ (Figure 3C). However, these taxa were mostly present in the sediment and seemed not to be metabolically active as they could not be identified in the metatranscriptomes. At sites 2 and 3 where NH4+ may apparently diffuse out of the sediment, the water column profiles display intervals where NH4+ and nitrate are consumed while nitrate is being produced (Figure 1B), which constitutes at least some geochemical suggestion that anammox processes may take place. At the SWI, Gammaproteobacteria prevailed in the taxonomic composition of 16S rRNA genes (Figure 2), evidencing a benthic-pelagic transition where denitrification and DNRA can be coupled with sulfur oxidation (Callbeck et al., 2018) as sulfate reduction is the prevailing process in the underlying sediment (Vuillemin et al., 2022). Because nitrate is used as an oxidant by sulfur-oxidizing bacteria (Zhu et al., 2018) to drive DNRA, we suspect that anammox processes may be inhibited in the surface sediment (Jensen et al., 2008; Jin et al., 2013). Instead, anammox-related Planctomycetota may be more metabolically active in mixed waters (Wasmund et al., 2016) when seasonal OM production and particle sinking are highest (Karthäuser et al., 2021) and nitrite is replenished (Brüchert et al., 2003; Callbeck et al., 2021).
Altogether, variations in the related ORF expression patterns (Figure 3) suggest that trophic interactions in austral winter are more determined by aerobic remineralization of sinking OM to NH4+ (Füssel et al., 2012; Kalvelage et al., 2015) on the shelf with limited benthic releases in the benthic boundary layer. The fact that NH4+ concentrations systematically decline in the OMZ at oxygen concentrations < 60 μM (Figures 1B,C) argues for nitrifier denitrification processes in the OMZ upper waters sustained by lateral transport along the Namibian coast (Inthorn et al., 2006a,b). Although lateral ventilation creates dynamic conditions in bottom waters along the Namibian shelf, locally attenuating benthic NH4+ releases, the taxonomic and functional diversity of microbial populations and gene expression patterns were consistent with geochemical profiles in coastal and offshore waters (Figure 8).
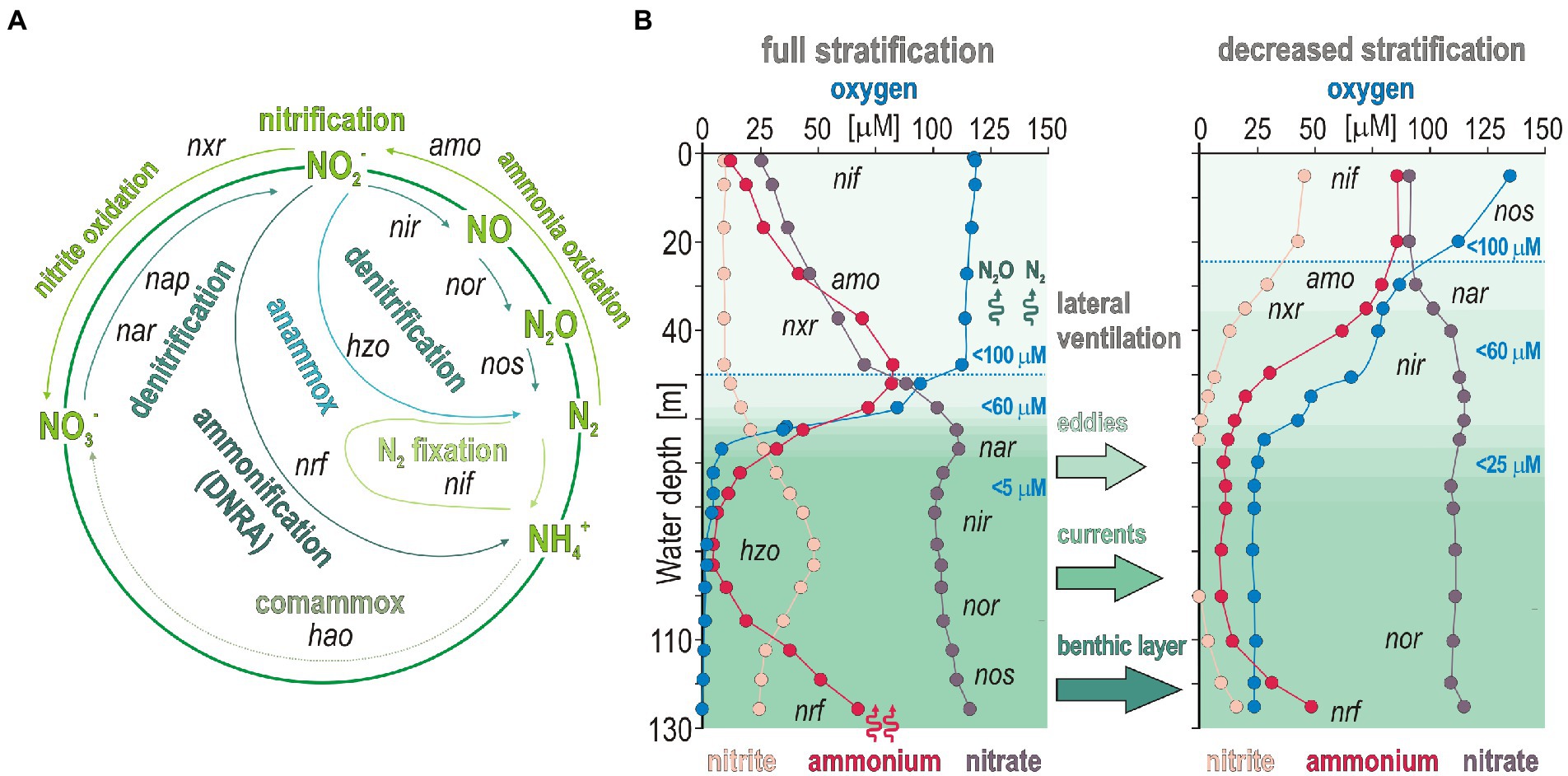
Figure 8. Pathways of nitrogen cycling with the corresponding functional marker genes, and theoretical biogeochemical gradients expected during full and partial stratification in coastal waters. (A) Nitrogen cycling functional genes related to processes of nitrogen fixation (nif), nitrification (amo and nxr), anaerobic ammonia oxidation, or anammox (hzo), dissimilatory nitrate reduction to ammonium (DNRA, nrf), and denitrification (nar, nap, nir, nor, and nos). (B) Theoretical biogeochemical gradients expected during full (left) and decreased (right) stratification. Abbreviations: nif: nitrogen fixation/amo: ammonium monooxygenase/nxr: nitrite oxidoreductase/hzo: hydrazine oxidoreductase/nrf: ammonia-forming cytochrome nitrite reductase/nar: respiratory nitrate reductase/nap: periplasmic nitrate reductase/nir: copper-containing nitrite reductase/nor: nitric oxide reductase/nos: nitrous oxide reductase/hao: hydroxylamine dehydrogenase.
4.2. Nitrifier denitrification is the prevailing process in dysoxic waters
In general, our profiling of expressed ORFs confirmed the deficit in nitrogen fixation in the sunlit ocean (Ulloa et al., 2013) as the related genes (nif) were minor and restricted to surface waters (Figure 4) even though it involved a taxonomically diverse pelagic community (Gier et al., 2016; Jayakumar and Ward, 2020), e.g., Cyanobacteria, Alpha-, Gammaproteobacteria, Firmicutes, and Euryarchaeota (Figure 5B). ORFs actively encoding aerobic amo and nxr genes consistently increased from the surface ocean down into the OMZ at all sites (Figure 4), displaying high expression levels concomitant with NH4+ transporters (Figure 5A). Metabolic use of other forms of fixed nitrogen through enzymes like nmo genes and ureases was only detectable in surface ocean of the shelf, which confirmed that urea-derived ammonia oxidation contributes little to nitrification activities in the coastal OMZ in comparison to NH4+-limited waters offshore (Kitzinger et al., 2019). Genes related to nitrilases and cyanases were identified, but not expressed (Figure 4). ORFs encoding genes for nitrification were not detected in the sediment (Figure 4). These patterns show that the upper layer of OMZ waters is critical for microaerobic nitrifying microorganisms, particularly the AOA and NOB (Kitzinger et al., 2020).
Ca. Nitrosopumilus and Ca. Nitrosopelagicus (Figures 3A, 6A) have a demonstrated capacity for growth using ammonia oxidation as an energy source (Vuillemin et al., 2019), resulting in stoichiometric production of nitrite (Könneke et al., 2005) that can be re-oxidized to nitrate (Lam and Kuypers, 2011; Füssel et al., 2012). A relatively high number of ORFs was assigned to nxr genes, whose taxonomic assignments (Figure 5B) showed that Nitrospinota is the only phylum actively involved in processes of nitrite oxidation to nitrate (Rani et al., 2017). This shows that ammonia was actively oxidized to nitrite by archaea and to nitrate by bacteria (Figures 4, 5A), leading to reciprocal feeding interactions in the vicinity of the OMZ (Figure 1C), which may limit the loss of fixed nitrogen via denitrification or anammox processes (Fernandez and Farias, 2012; Koch et al., 2015). Consistent with the presence of Nitrospira (sp. moscoviensis) in 16S rRNA genes but absence of any related bacterial amoA gene, we did not identify ORFs encoding hydroxylamine dehydrogenase (i.e., hao cluster), implying that complete nitrification did not proceed via comammox (Daims et al., 2016; Long et al., 2021). Instead, the produced nitrite appeared to be actively reduced via transcription of nirK genes (Braker et al., 2000) by the aforementioned Nitrososphaeria, and Nitrospinota, as well as Desulfobacterota in the OMZ (Figures 4). ORFs assigned to anaerobic nir genes had by far the highest expression level in terms of nitrogen reduction compared to nar genes (Figure 5A), which demonstrates that nitrifier denitrification was the prevailing process from the surface ocean into OMZ waters across all sites (Figures 4, 5) in spite of nitrate-replete OMZ waters (Figure 1C). The taxonomic assignments of the metabolic guild actively expressing nar and nir genes (Smith et al., 2007) in the water column included Nitrospinota, Nitrososphaeria, Gammaproteobacteria, and fewer Desulfobacterota (Figure 5B). Some ORFs assigned to nor genes were subsequently expressed in deep waters (Figure 4), mostly by Gammaproteobacteria, Desulfobacterota and Nitrospirota (Figure 5B) albeit at much lower levels. Interestingly, nos genes which represent the final step of denitrification (i.e., N2O reduction to N2) were only detectable in the surface ocean where they were assigned to Bacteroidota and fewer Nitrospirota and Proteobacteria (Figure 5B) known to display atypical N2O-scavenging abilities (Wyman et al., 2013; Bertagnolli et al., 2020).
These expression patterns show that nitrifiers were most active in transcribing nirK genes (Lawton et al., 2013), potentially leading to abiotic N2O production (Stein, 2011b; Ji et al., 2015) due to the necessity to detoxify the produced nitric oxide in the OMZ rather than through canonical denitrification (Stein, 2011a; Bertagnolli et al., 2020). However, the contribution of such abiotic hybrid reactions in N2O emissions is a matter of debate and likely minor (Stein et al., 2022). In addition, nitroreductases were expressed in dysoxic waters, but only locally (site 4). Thus, in spite of active recycling of metabolites (urea, R-NO2, NH4+) via microaerobic microbial respiration (Behrendt et al., 2013; Kitzinger et al., 2019), our results support the general deficit of fixed nitrogen as an electron acceptor (Kraft et al., 2011) and nitrifier denitrification (Fernandez and Farias, 2012; Lawton et al., 2013) as the main nitrogen reduction pathway in the water column at ca. 60 μM oxygen concentrations (Figure 1), potentially leading to N2 and N2O gas emissions (Wrage-Mönnig et al., 2018). While decreased stratification still allowed microaerobic oxidation of benthic NH4+ to take place, the last steps of canonical denitrification and anammox processes were apparently limited due to subsequent consumption of nitrite during nitrifier denitrification (Figure 8).
4.3. Ammonification and denitrification in sulfidic sediment
When the SWI of the Namibian inner shelf is anoxic, H2S, CH4, and NH4+ frequently diffuse out of the sediment forming sulfur plumes in the water column during austral summer (Brüchert et al., 2003, 2009). As stratification decreases along the shelf, NH4+ can be locally released from the seafloor into the benthic boundary layer (Füssel et al., 2012; Neumann et al., 2016). Seasonal variations in Namibian coastal waters may thereby promote chemolithoautotrophy with sulfur and NH4+ as successive electron donors (Farías et al., 2009; Lam and Kuypers, 2011) to drive sulfur-dependent denitrification by Gammaproteobacteria (Lipsewers et al., 2017; Vuillemin et al., 2022) pursued by nitrifier denitrification by Nitrososphaeria and Nitrospinota in the water column (Wrage-Mönnig et al., 2018; Ruiz-Fernández et al., 2020).
Taxa identified as presumed nitrifiers in the sediments were closely affiliated with Ca. Nitrososphaera viennensis (Figure 3A), Nitrosomonas nitrosa, and candidate clades among Nitrosococcaceae and Nitrospinaceae (Figure 3B). However, metabolic activities toward oxidation of nitrogen compounds only involved nmo genes by Lokiarchaeum sp. (Figure 5B), potentially resulting in minor production of nitrite (Zhang et al., 2022). Consistent with the apparent lack of metabolic expression by anammox-related Planctomycetota (Figure 3C), we did not detect any ORF related to hydrazine (i.e., H2N4) oxidoreductase (hzo) in shallow sediment (Kong et al., 2013). In spite of members of the Gammaproteobacteria, e.g., Beggiatoa, Thiothrix, and Thioploca (Vuillemin et al., 2022), being capable of coupling DNRA with sulfide oxidation (Schunck et al., 2013; Zhu et al., 2018), the abundance of ORFs expressing ammonia-forming nrf genes (i.e., DNRA) was minor (Figures 4, 5), solely involving taxa among Nitrospirota in sulfidic sediments (Murphy et al., 2020).
In highly reducing nitrate-depleted sediment (Supplementary Figure 4), the survival of denitrifiers is generally poor, and such populations are likely to employ Fe3+ reduction in their energy metabolism (Coby et al., 2011), which is theoretically absent in Namibian sediment (Böning et al., 2020), thus arguing for another pathway of NH4+ production. Expression of nitroreductases by Bacteroidota suggests active degradation of OM with release of nitro (i.e., R-NO2) compounds (Roldán et al., 2008). We deduct that benthic NH4+ production (Figure 1D) potentially released into the coastal OMZ waters (Figure 1C) results from amino acid remineralization (i.e., algal necromass) via expression of proteases by heterotrophic bacteria (Neumann et al., 2016) rather than DNRA (Pantoja and Lee, 2003; Vuillemin et al., 2022). In the bottom part of the core (28 cmbsf), ORFs assigned to nar and nir genes were mainly expressed by Proteobacteria, whereas nor and nos genes were also expressed by taxa among Methylomirabilota (former candidate NC10; Padilla et al., 2016) and Desulfobacterota, but gene expression of these final steps of denitrification was minor in the sediment (Figures 4, 5B).
Results from water incubations with dEPS over an 18-h period confirmed remineralization of algal necromass as all the genes involved in the initial reduction of fixed nitrogen (i.e., nif, nar, nir, and NH4+ transporters) were 13C-labeled in the total absence of ammonia oxidation (Figure 4). Bacteroidota appeared as the main aerobic degraders of algal necromass that rapidly grew and led to microoxic conditions under which processes of denitrification initiated, as shown by 13C-labeling of nif, nir, and ntr genes (Figure 5C). Other taxa that were 13C-labeled for nitrate, NH4+ and urea transporters were found among Planctomycetota and Proteobacteria, respectively (Figure 5C). Sediment incubations with DIC over a 10-days period resulted in the 13C-labeling of all the genes involved in denitrification and DNRA (Figures 4, 5A). The related nitrate-reducing taxa included a majority of Desulfobacterota and Gammaproteobacteria, with fewer Chloroflexota, Nitrospinota, and Actinobacteriota (Figure 5B). Under in vitro conditions, the highest number of 13C-labeled ORFs were assigned to proteases (Vuillemin et al., 2022), indicating that organic nitrogen was assimilated from algal necromass via fermentative processes. Although metabolic activities related to NH4+ oxidation in the sediment were below detection, AOA could potentially outcompete anammox bacteria in surficial sediments when lateral currents ventilate the SWI, and mitigate benthic emissions of NH4+ in the boundary layer along the shelf (Figure 1D).
4.4. Autotrophic or heterotrophic nitrifier denitrification
Under oxic conditions, AOA and NOB have metabolic potential for autotrophic carbon fixation through the HP/HB and CBB cycle (Könneke et al., 2014). In spite of being common aerobes, Nitrospinota have in theory the capability to fix carbon via the reductive TCA (Luecker et al., 2013). Expressed ORFs encoding the RuBisCO, acly, and acc genes in the water column were mostly assigned to Alpha- and Gammaproteobacteria and Bacteroidota (Figure 7). Autotrophic carbon fixation by Nitrososphaeria was either not expressed or below detection at the present sequencing depths, whereas nitrite-oxidizing Nitrospinota could apparently evolve as potential autotrophs by expressing codh genes (Zhang et al., 2020) instead of the reductive TCA as previously reported (Luecker et al., 2013). Interestingly, results from SIP metagenomes incubated with water and dEPS revealed 13C-labeling of RuBisCO genes that were exclusively assigned to Nitrososphaeria (Figure 7B). Along with our profiling of ORF expression, this confirms that chemoautotrophic ammonia and nitrite oxidizers can adapt to low concentrations of fixed nitrogen in heterotrophic food web by coupling nitrogen and carbon cycling using simple organic substrates (Kitzinger et al., 2019, 2020), e.g., urea (CH4N2O), cyanate (CH3OCN), amino acids and nitro compounds (R-NO2), for heterotrophic nitrification and nitrifier denitrification (Stein, 2011b; Dekas et al., 2019), potentially with anaplerotic CO2 assimilation mediated by RuBisCO and codh genes (Aylward and Santoro, 2020; Reji and Francis, 2020; Zhang et al., 2020).
Consistent with oxygen drawdown during heterotrophic degradation of algal OM sinking in the water column, expressed ORFs related to acs, cs, and pdh genes indicated the prevalence of fermentative glycolysis and TCA cycle for carbon assimilation under dysoxic conditions (Figure 7). In this context, ORFs assigned to formate dehydrogenase, which is also the first step of the respiratory chain in denitrification (Einsle and Kroneck, 2004), were increasingly expressed down into the OMZ by Alpha- and Gammaproteobacteria (Figures 4, 5). Although not presently expressed by nitrifiers, the electron transfer from formate to nitrate may support versatility in the use of simple carbon resources during denitrification as formate can be readily oxidized through nitrate reduction for anaerobic growth under anoxia (Koch et al., 2015; Daims et al., 2016).
Altogether metatranscriptomic profiling demonstrates that denitrification clearly outcompassed nitrogen fixation and ammonia-forming nitrate reduction, or DNRA. Nitrifiers, such as ammonia-oxidizing Nitrososphaeria and nitrite-oxidizing Nitrospinota, displayed high expression levels of amo and nxr genes in dysoxic waters in concomitance with nirK genes, thereby also performing nitrite reduction. These two groups of nitrifiers appeared to accessorize a certain degree of mixotrophy under OMZ conditions by microaerobically oxidizing simple organic compounds and anaplerotic CO2 assimilation coupled with nitrite reduction. Intriguingly, ORFs related either to anammox or comammox were not detected. Most likely, the nitrite necessary to anammox and comammox reactions was either re-oxidized to nitrate or reduced to nitric oxide by Nitrososphaeria and Nitrospinota during nitrifier denitrification. The subsequent reduction from nitric to nitrous oxide was driven by Nitrospirota and Gammaproteobacteria in OMZ waters, whereas the produced N2O was scavenged at the ocean surface. To conclude, in austral winter, the main pathways to potential N2O production stem from OM remineralization by a microbial consortium actively performing heterotrophic nitrification and nitrifier denitrification fueled by fixed and organic nitrogen dissolved in the oxycline waters.
Data availability statement
The datasets presented in this study can be found in online repositories. The names of the repository/repositories and accession number(s) can be found at: https://www.ncbi.nlm.nih.gov/, PRJNA525353.
Author contributions
AV conceived the idea for the study, extracted nucleic acids, performed quantitative PCR assays, library preparation, and Illumina sequencing, analyzed the data, designed the figures, and wrote the paper. All authors contributed to the article and approved the submitted version.
Funding
Open Access was enabled within the framework of the funding program “Open Access Publication Costs” by the German Research Foundation (DFG)—project number 491075472.
Acknowledgments
W. D. Orsi and Ö. K. Coskun are acknowledged for their help with bioinformatics and for providing feedback on the manuscript. The crew of the F/S Meteor and organizing committee of the oceanographic expedition are acknowledged in obtaining samples and producing geochemical data. A non-peer-reviewed version of this manuscript was submitted to bioRxiv.org (Vuillemin, 2022).
Conflict of interest
The author declares that the research was conducted in the absence of any commercial or financial relationships that could be construed as a potential conflict of interest.
Publisher’s note
All claims expressed in this article are solely those of the authors and do not necessarily represent those of their affiliated organizations, or those of the publisher, the editors and the reviewers. Any product that may be evaluated in this article, or claim that may be made by its manufacturer, is not guaranteed or endorsed by the publisher.
Supplementary material
The Supplementary material for this article can be found online at: https://www.frontiersin.org/articles/10.3389/fmicb.2023.1101902/full#supplementary-material
Footnotes
References
Alves, R. J. E., Minh, B. Q., Urich, T., von Haeseler, A., and Schleper, C. (2018). Unifying the global phylogeny and environmental distribution of ammonia-oxidising archaea based on amoA genes. Nat. Commun. 9:1517. doi: 10.1038/s41467-018-03861-1
Aylward, F. O., and Santoro, A. E. (2020). Heterotrophic Thaumarchaea with small genomes are widespread in the dark ocean. mSystems 5, e00415–e00420. doi: 10.1128/mSystems.00415-20
Behrendt, A., de Beer, D., and Stief, P. (2013). Vertical activity distribution of dissimilatory nitrate reduction in coastal marine sediments. Biogeosciences 10, 7509–7523. doi: 10.5194/bg-10-7509-2013
Bertagnolli, A. D., Konstantinidis, K. T., and Stewart, F. J. (2020). Non-denitrifier nitrous oxide reductases dominate marine biomes. Environ. Microbiol. Rep. 12, 681–692. doi: 10.1111/1758-2229.12879
Bertagnolli, A. D., and Stewart, F. J. (2018). Microbial niches in marine oxygen minimum zones. Nat. Rev. Microbiol. 16, 723–729. doi: 10.1038/s41579-018-0087-z
Böning, P., Schnetger, B., Belz, L., Ferdelman, T. G., Brumsack, H.-J., and Pahnke, K. (2020). Sedimentary iron cycling in the Benguela upwelling system off Namibia. Earth Planet. Sci. Lett. 538:116212. doi: 10.1016/j.epsl.2020.116212
Braker, G., Zhou, J., Wu, L., Devol, A. H., and Tiedje, J. M. (2000). Nitrite reductase genes (nirK and nirS) as functional markers to investigate diversity of denitrifying bacteria in Pacific northwest marine sediment communities. Appl. Environ. Microbiol. 66, 2096–2104. doi: 10.1128/AEM.66.5.2096-2104.2000
Breitwieser, F. P., Lu, J., and Salzberg, S. L. (2019). A review of methods and databases for metagenomic classification and assembly. Brief. Bioinform. 20, 1125–1136. doi: 10.1093/bib/bbx120
Brüchert, V., Currie, B., and Peard, K. R. (2009). Hydrogen sulphide and methane emissions on the central Namibian shelf. Prog. Oceanogr. 83, 169–179. doi: 10.1016/j.pocean.2009.07.017
Brüchert, V., Jørgensen, B. B., Neumann, K., Riechmann, D., Schlösser, M., and Schulz, H. (2003). Regulation of bacterial sulfate reduction and hydrogen sulfide fluxes in the central namibian coastal upwelling zone. Geochim. Cosmochim. Ac. 67, 4505–4518. doi: 10.1016/S0016-7037(03)00275-8
Buchfink, B., Xie, C., and Huson, D. H. (2015). Fast and sensitive protein alignment using DIAMOND. Nat. Methods 12, 59–60. doi: 10.1038/nmeth.3176
Callbeck, C. M., Canfield, D. E., Kuypers, M. M. M., Yilmaz, P., Lavik, G., Thamdrup, B., et al. (2021). Sulfur cycling in oceanic oxygen minimum zones. Limnol. Oceanogr. 66, 2360–2392. doi: 10.1002/lno.11759
Callbeck, C. M., Lavik, G., Ferdelman, T. G., Fuchs, B., Gruber-Vodicka, H. R., Hach, P. F., et al. (2018). Oxygen minimum zone cryptic sulfur cycling sustained by offshore transport of key sulfur oxidizing bacteria. Nat. Commun. 9:1729. doi: 10.1038/s41467-018-04041-x
Caporaso, J. G., Kuczynski, J., Stombaugh, J., Bittinger, K., Bushman, F. D., Costello, E. K., et al. (2010). QIIME allows analysis of high-throughput community sequencing data. Nat. Methods 7, 335–336. doi: 10.1038/nmeth.f.303
Coby, A. J., Picardal, F., Shelobolina, E., Xu, H., and Roden, E. E. (2011). Repeated anaerobic microbial redox cycling of iron. Appl. Environ. Microbiol. 77, 6036–6042. doi: 10.1128/AEM.00276-11
Codispoti, L. A., and Christensen, J. P. (1985). Nitrification, denitrification and nitrous oxide cycling in the eastern tropical South Pacific Ocean. Mar. Chem. 16, 277–300. doi: 10.1016/0304-4203(85)90051-9
Coskun, Ö. K., Vuillemin, A., Schubotz, F., Klein, F., Sichel, S. E., Eisenreich, W., et al. (2022). Quantifying the effects of hydrogen on carbon assimilation in a seafloor microbial community associated with ultramafic rocks. ISME J. 16, 257–271. doi: 10.1038/s41396-021-01066-x
Currie, B., Utne-Palm, A. C., and Salvanes, A. G. V. (2018). Winning ways with hydrogen sulphide on the Namibian shelf. Front. Mar. Sci. 5:341. doi: 10.3389/fmars.2018.00341
Daims, H., Lücker, S., and Wagner, M. (2016). A new perspective on microbes formerly known as nitrite-oxidizing bacteria. Trends Microbiol. 24, 699–712. doi: 10.1016/j.tim.2016.05.004
Dekas, A. E., Parada, A. E., Mayali, X., Fuhrman, J. A., Wollard, J., Weber, P. K., et al. (2019). Characterizing chemoautotrophy and heterotrophy in marine archaea and bacteria with single-cell multi-isotope NanoSIP. Front. Microbiol. 10:2682. doi: 10.3389/fmicb.2019.02682
Dong, L. F., Smith, C. J., Papaspyrou, S., Stott, A., Osborn, A. M., and Nedwell, D. B. (2009). Changes in benthic denitrification, nitrate ammonification, and anammox process rates and nitrate and nitrite reductase gene abundances along an estuarine nutrient gradient (the Colne estuary, United Kingdom). Appl. Environ. Microbiol. 75, 3171–3179. doi: 10.1128/AEM.02511-08
Edgar, R. C. (2004). MUSCLE: multiple sequence alignment with high accuracy and high throughput. Nucleic Acids Res. 32, 1792–1797. doi: 10.1093/nar/gkh340
Edgar, R. C. (2013). UPARSE: highly accurate OTU sequences from microbial amplicon reads. Nat. Methods 10, 996–998. doi: 10.1038/nmeth.2604
Einsle, O., and Kroneck, P. M. H. (2004). Structural basis of denitrification. Biol. Chem. 385, 875–883. doi: 10.1515/BC.2004.115
Erb, T. J. (2011). Carboxylases in natural and synthetic microbial pathways. Appl. Environ. Microbiol. 77, 8466–8477. doi: 10.1128/AEM.05702-11
Farías, L., Fernández, C., Faúndez, J., Cornejo, M., and Alcaman, M. E. (2009). Chemolithoautotrophic production mediating the cycling of the greenhouse gases N2O and CH4 in an upwelling ecosystem. Biogeosciences 6, 3053–3069. doi: 10.5194/bg-6-3053-2009
Ferdelman, T. G., Beier, S., Benito Merino, D., Böning, P., Garaba, S. P., Gomez-Saez, G. V., et al. (2019). “EreBUS: processes controlling greenhouse gas emissions from the Benguela upwelling system, cruise no. 148/2, July 2–July 20, 2018, Walvis Bay (Namibia)—Las Palmas de gran Canaria (Spain)” in METEOR-Berichte M148/2, 1–88.
Ferdelman, T. G., Fossing, H., Neumann, K., and Schulz, H. D. (1999). Sulfate reduction in surface sediments of the Southeast Atlantic continental margin between 15°38’S and 27°57’S (Angola and Namibia). Limnol. Oceanogr. 44, 650–661. doi: 10.4319/lo.1999.44.3.0650
Ferdelman, T. G., Klockgether, G., Imhoff, K., and Gomez-Saez, G. V. (2021b). Meteor expedition M18/2 EreBUS sediment porewater nutrient and sulfur from station M148/2_206–6.
Ferdelman, T. G., Klockgether, G., Imhoff, K., and Mohrholz, V. (2021a). Meteor expedition M148/2 EreBUS nutrient data from Benguela upwelling system and Angola gyre.
Fernandez, C., and Farias, L. (2012). Assimilation and regeneration of inorganic nitrogen in a coastal upwelling system: ammonium and nitrate utilization. Mar. Ecol. Prog. Ser. 451, 1–14. doi: 10.3354/meps09683
Füssel, J., Lam, P., Lavik, G., Jensen, M. M., Holtappels, M., Günter, M., et al. (2012). Nitrite oxidation in the Namibian oxygen minimum zone. ISME J. 6, 1200–1209. doi: 10.1038/ismej.2011.178
Ganesh, S., Parris, D. J., DeLong, E. F., and Stewart, F. J. (2014). Metagenomic analysis of size-fractionated picoplankton in a marine oxygen minimum zone. ISME J. 8, 187–211. doi: 10.1038/ismej.2013.144
Garaba, S. P., Thölen, C., Ferdelman, T. G., and Zielinski, O. (2021). Optical water quality variables (Secchi disk depth and Forel-Ule colour indexes) observed during cruise M148/2 aboard RV METEOR.
Garbeva, P., Baggs, E. M., and Prosser, J. I. (2007). Phylogeny of nitrite reductase (nirK) and nitric oxide reductase (norB) genes from Nitrosospira species isolated from soil. FEMS Microbiol. Lett. 266, 83–89. doi: 10.1111/j.1574-6968.2006.00517.x
Garçon, V., Karstensen, J., Palacz, A., Telszewski, M., Aparco Lara, T., Breitburg, D., et al. (2019). Multidisciplinary observing in the world ocean’s oxygen minimum zone regions: from climate to fish — the VOICE initiative. Front. Mar. Sci. 6:722. doi: 10.3389/fmars.2019.00722
Gier, J., Sommer, S., Löscher, C. R., Dale, A. W., Schmitz, R. A., and Treude, T. (2016). Nitrogen fixation in sediments along a depth transect through the Peruvian oxygen minimum zone. Biogeosciences 13, 4065–4080. doi: 10.5194/bg-13-4065-2016
Gouy, M., Guindon, S., and Gascuel, O. (2010). SeaView version 4: a multiplatform graphical user interface for sequence alignment and phylogenetic tree building. Mol. Biol. Evol. 27, 221–224. doi: 10.1093/molbev/msp259
Heiss, E. M., and Fulweiler, R. W. (2016). Coastal water column ammonium and nitrite oxidation are decoupled in summer. Estuar. Coast. Shelf Sci. 178, 110–119. doi: 10.1016/j.ecss.2016.06.002
Huber, W., Carey, V. J., Gentleman, R., Anders, S., Carlson, M., Carvalho, B. S., et al. (2015). Orchestrating high-throughput genomic analysis with Bioconductor. Nat. Methods 12, 115–121. doi: 10.1038/nmeth.3252
Ijichi, M., and Hamasaki, K. (2011). Community structure of ammonia-oxidizing marine archaea differs by depth of collection and temperature of cultivation. J. Oceanogr. 67, 739–745. doi: 10.1007/s10872-011-0066-8
Inthorn, M., Mohrholz, V., and Zabel, M. (2006a). Nepheloid layer distribution in the Benguela upwelling area offshore Namibi. Deep Sea Res. Part I: Oceanogr. Res. Pap. 53, 1423–1438. doi: 10.1016/j.dsr.2006.06.004
Inthorn, M., Wagner, T., Scheeder, G., and Zabel, M. (2006b). Lateral transport controls distribution, quality, and burial of organic matter along continental slopes in high-productivity areas. Geology 34, 205–208. doi: 10.1130/G22153.1
Jayakumar, A., and Ward, B. B. (2020). Diversity and distribution of nitrogen fixation genes in the oxygen minimum zones of the world oceans. Biogeosciences 17, 5953–5966. doi: 10.5194/bg-17-5953-2020
Jensen, M. M., Kuypers, M. M. M., Gaute, L., and Thamdrup, B. (2008). Rates and regulation of anaerobic ammonium oxidation and denitrification in the Black Sea. Limnol. Oceanogr. 53, 23–36. doi: 10.4319/lo.2008.53.1.0023
Jensen, M. M., Lam, P., Revsbech, N. P., Nagel, B., Gaye, B., Jetten, M. S., et al. (2011). Intensive nitrogen loss over the Omani shelf due to anammox coupled with dissimilatory nitrite reduction to ammonium. ISME J. 5, 1660–1670. doi: 10.1038/ismej.2011.44
Ji, Q., Babbin, A. R., Jayakumar, A., Oleynik, S., and Ward, B. B. (2015). Nitrous oxide production by nitrification and denitrification in the eastern tropical South Pacific oxygen minimum zone. Geophys. Res. Lett. 42, 10,755–10,764. doi: 10.1002/2015GL066853
Jin, R.-C., Yang, G.-F., Zhang, Q.-Q., Ma, C., Yu, J.-J., and Xing, B.-S. (2013). The effect of sulfide inhibition on the ANAMMOX process. Water Res. 47, 1459–1469. doi: 10.1016/j.watres.2012.12.018
Kalvelage, T., Lavik, G., Jensen, M. M., Revsbech, N. P., Löscher, C., Schunck, H., et al. (2015). Aerobic microbial respiration in oceanic oxygen minimum zones. PLoS One 10:e0133526. doi: 10.1371/journal.pone.0133526
Karlsson, R., Karlsson, A., Bäckman, O., Johansson, B. R., and Hulth, S. (2009). Identification of key proteins involved in the anammox reaction. FEMS Microbiol. Lett. 297, 87–94. doi: 10.1111/j.1574-6968.2009.01677.x
Karthäuser, C., Ahmerkamp, S., Marchant, H. K., Bristow, L. A., Hauss, H., Iversen, M. H., et al. (2021). Small sinking particles control anammox rates in the Peruvian oxygen minimum zone. Nat. Commun. 12:3235. doi: 10.1038/s41467-021-23340-4
Kitzinger, K., Marchant, H. K., Bristow, L. A., Herbold, C. W., Padilla, C. C., Kidane, A. T., et al. (2020). Single cell analyses reveal contrasting life strategies of the two main nitrifiers in the ocean. Nat. Commun. 11:767. doi: 10.1038/s41467-020-14542-3
Kitzinger, K., Padilla, C. C., Marchant, H. K., Hach, P. F., Herbold, C. W., Kidane, A. T., et al. (2019). Cyanate and urea are substrates for nitrification by Thaumarchaeota in the marine environment. Nat. Microbiol. 4, 234–243. doi: 10.1038/s41564-018-0316-2
Koch, H., Lücker, S., Albertsen, M., Kitzinger, K., Herbold, C., Spieck, E., et al. (2015). Expanded metabolic versatility of ubiquitous nitrite-oxidizing bacteria from the genus Nitrospira. Proc. Natl. Acad. Sci. 112, 11371–11376. doi: 10.1073/pnas.1506533112
Kong, L., Jing, H., Kataoka, T., Buchwald, C., and Liu, H. (2013). Diversity and spatial distribution of hydrazine oxidoreductase (hzo) gene in the oxygen minimum zone off Costa Rica. PLoS One 8:e78275. doi: 10.1371/journal.pone.0078275
Könneke, M., Bernhard, A. E., de la Torre, J. R., Walker, C. B., Waterbury, J. B., and Stahl, D. A. (2005). Isolation of an autotrophic ammonia-oxidizing marine archaeon. Nature 437, 543–546. doi: 10.1038/nature03911
Könneke, M., Schubert, D. M., Brown, P. C., Hügler, M., Standfest, S., Schwander, T., et al. (2014). Ammonia-oxidizing archaea use the most energy-efficient aerobic pathway for CO2 fixation. Proc. Natl. Acad. Sci. 111, 8239–8244. doi: 10.1073/pnas.1402028111
Kraft, B., Strous, M., and Tegetmeyer, H. E. (2011). Microbial nitrate respiration – Genes, enzymes and environmental distribution. J. Biotechnol. 155, 104–117. doi: 10.1016/j.jbiotec.2010.12.025
Küster-Heins, K., Steinmetz, E., de Lange, G., and Zabel, M. (2010). Phosphorus cycling in marine sediments from the continental margin off Namibia. Mar. Geol. 274, 95–106. doi: 10.1016/j.margeo.2010.03.008
Kuypers, M. M. M., Lavik, G., Woebken, D., Schmid, M., Fuchs, B. M., Amann, R., et al. (2005). Massive nitrogen loss from the Benguela upwelling system through anaerobic ammonium oxidation. Proc. Natl. Acad. Sci. 102, 6478–6483. doi: 10.1073/pnas.0502088102
Lam, P., and Kuypers, M. M. M. (2011). Microbial nitrogen cycling processes in oxygen minimum zones. Ann. Rev. Mar. Sci. 3, 317–345. doi: 10.1146/annurev-marine-120709-142814
Lam, P., Lavik, G., Jensen, M. M., van de Vossenberg, J., Schmid, M., Woebken, D., et al. (2009). Revising the nitrogen cycle in the Peruvian oxygen minimum zone. Proc. Natl. Acad. Sci. 106, 4752–4757. doi: 10.1073/pnas.0812444106
Lavik, G., Stührmann, T., Brüchert, V., Van der Plas, A., Mohrholz, V., Lam, P., et al. (2009). Detoxification of sulphidic African shelf waters by blooming chemolithotrophs. Nature 457, 581–584. doi: 10.1038/nature07588
Lawton, T. J., Bowen, K. E., Sayavedra-Soto, L. A., Arp, D. J., and Rosenzweig, A. C. (2013). Characterization of a nitrite reductase involved in nitrifier denitrification. J. Biol. Chem. 288, 25575–25583. doi: 10.1074/jbc.M113.484543
Lipsewers, Y. A., Vasquez-Cardenas, D., Seitaj, D., Schauer, R., Hidalgo-Martinez, S., Sinninghe Damsté, J. S., et al. (2017). Impact of seasonal hypoxia on activity and community structure of chemolithoautotrophic bacteria in a coastal sediment. Appl. Environ. Microbiol. 83, e03517–e03516. doi: 10.1128/AEM.03517-16
Long, A. M., Jurgensen, S. K., Petchel, A. R., Savoie, E. R., and Brum, J. R. (2021). Microbial ecology of oxygen minimum zones amidst ocean deoxygenation. Front. Microbiol. 12:748961. doi: 10.3389/fmicb.2021.748961
Ludwig, W., Strunk, O., Westram, R., Richter, L., Meier, H., Yadhukumar,, et al. (2004). ARB: a software environment for sequence data. Nucleic Acids Res. 32, 1363–1371. doi: 10.1093/nar/gkh293
Luecker, S., Nowka, B., Rattei, T., Spieck, E., and Daims, H. (2013). The genome of Nitrospina gracilis illuminates the metabolism and evolution of the major marine nitrite oxidizer. Front. Microbiol. 4:27. doi: 10.3389/fmicb.2013.00027
Martínez-Espinosa, R. M., Cole, J. A., Richardson, D. J., and Watmough, N. J. (2011). Enzymology and ecology of the nitrogen cycle. Biochem. Soc. Trans. 39, 175–178. doi: 10.1042/BST0390175
Murphy, A. E., Bulseco, A. N., Ackerman, R., Vineis, J. H., and Bowen, J. L. (2020). Sulphide addition favours respiratory ammonification (DNRA) over complete denitrification and alters the active microbial community in salt marsh sediments. Environ. Microbiol. 22, 2124–2139. doi: 10.1111/1462-2920.14969
Neumann, A., Lahajnar, N., and Emeis, K.-C. (2016). Benthic remineralisation rates in shelf and slope sediments of the northern Benguela upwelling margin. Cont. Shelf Res. 113, 47–61. doi: 10.1016/j.csr.2015.12.009
Ohde, T., and Dadou, I. (2018). Seasonal and annual variability of coastal Sulphur plumes in the northern Benguela upwelling system. PLoS One 13:e0192140. doi: 10.1371/journal.pone.0192140
Orsi, W. D., Coolen, M. J. L., Wuchter, C., He, L., More, K. D., Irigoien, X., et al. (2017). Climate oscillations reflected within the microbiome of Arabian Sea sediments. Sci. Rep. 7:6040. doi: 10.1038/s41598-017-05590-9
Orsi, W. D., Morard, R., Vuillemin, A., Eitel, M., Wörheide, G., Milucka, J., et al. (2020a). Anaerobic metabolism of foraminifera thriving below the seafloor. ISME J. 14, 2580–2594. doi: 10.1038/s41396-020-0708-1
Orsi, W. D., Smith, J. M., Wilcox, H. M., Swalwell, J. E., Carini, P., Worden, A. Z., et al. (2015). Ecophysiology of uncultivated marine euryarchaea is linked to particulate organic matter. ISME J. 9, 1747–1763. doi: 10.1038/ismej.2014.260
Orsi, W. D., Vuillemin, A., Coskun, Ö. K., Rodriguez, P., Oertel, Y., Niggemann, J., et al. (2022). Carbon assimilating fungi from surface ocean to subseafloor revealed by coupled phylogenetic and stable isotope analysis. ISME J. 16, 1245–1261. doi: 10.1038/s41396-021-01169-5
Orsi, W. D., Vuillemin, A., Rodriguez, P., Coskun, Ö. K., Gomez-Saez, G. V., Lavik, G., et al. (2020b). Metabolic activity analyses demonstrate that Lokiarchaeon exhibits homoacetogenesis in sulfidic marine sediments. Nat. Microbiol. 5, 248–255. doi: 10.1038/s41564-019-0630-3
Ortega-Arbulú, A.-S., Pichler, M., Vuillemin, A., and Orsi, W. D. (2019). Effects of organic matter and low oxygen on the mycobenthos in a coastal lagoon. Environ. Microbiol. 21, 374–388. doi: 10.1111/1462-2920.14469
Padilla, C. C., Bristow, L. A., Sarode, N., Garcia-Robledo, E., Gómez Ramírez, E., Benson, C. R., et al. (2016). NC10 bacteria in marine oxygen minimum zones. ISME J. 10, 2067–2071. doi: 10.1038/ismej.2015.262
Pantoja, S., and Lee, C. (2003). Amino acid remineralization and organic matter lability in Chilean coastal sediments. Org. Geochem. 34, 1047–1056. doi: 10.1016/S0146-6380(03)00085-8
Parada, A. E., Needham, D. M., and Fuhrman, J. A. (2016). Every base matters: assessing small subunit rRNA primers for marine microbiomes with mock communities, time series and global field samples. Environ. Microbiol. 18, 1403–1414. doi: 10.1111/1462-2920.13023
Pichler, M., Coskun, Ö. K., Ortega-Arbulú, A.-S., Conci, N., Wörheide, G., Vargas, S., et al. (2018). A 16S rRNA gene sequencing and analysis protocol for the Illumina MiniSeq platform. Microbiology 7:e00611. doi: 10.1002/mbo3.611
Pruesse, E., Quast, C., Knittel, K., Fuchs, B. M., Ludwig, W., Peplies, J., et al. (2007). SILVA: a comprehensive online resource for quality checked and aligned ribosomal RNA sequence data compatible with ARB. Nucleic Acids Res. 35, 7188–7196. doi: 10.1093/nar/gkm864
Quast, C., Pruesse, E., Yilmaz, P., Gerken, J., Schweer, T., Yarza, P., et al. (2013). The SILVA ribosomal RNA gene database project: improved data processing and web-based tools. Nucleic Acids Res. 41, D590–D596. doi: 10.1093/nar/gks1219
Rani, S., Koh, H.-W., Rhee, S.-K., Fujitani, H., and Park, S.-J. (2017). Detection and diversity of the nitrite oxidoreductase alpha subunit (nxrA) gene of Nitrospina in marine sediments. Microb. Ecol. 73, 111–122. doi: 10.1007/s00248-016-0897-3
Reji, L., and Francis, C. A. (2020). Metagenome-assembled genomes reveal unique metabolic adaptations of a basal marine Thaumarchaeota lineage. ISME J. 14, 2105–2115. doi: 10.1038/s41396-020-0675-6
Rho, M., Tang, H., and Ye, Y. (2010). FragGeneScan: predicting genes in short and error-prone reads. Nucleic Acids Res. 38:e191. doi: 10.1093/nar/gkq747
Roldán, M. D., Pérez-Reinado, E., Castillo, F., and Moreno-Vivián, C. (2008). Reduction of polynitroaromatic compounds: the bacterial nitroreductases. FEMS Microbiol. Rev. 32, 474–500. doi: 10.1111/j.1574-6976.2008.00107.x
Ruiz-Fernández, P., Ramírez-Flandes, S., Rodríguez-León, E., and Ulloa, O. (2020). Autotrophic carbon fixation pathways along the redox gradient in oxygen-depleted oceanic waters. Environ. Microbiol. Rep. 12, 334–341. doi: 10.1111/1758-2229.12837
Scala, D. J., and Kerkhof, L. J. (1999). Diversity of nitrous oxide reductase (nosZ) genes in continental shelf sediments. Appl. Environ. Microbiol. 65, 1681–1687. doi: 10.1128/AEM.65.4.1681-1687.1999
Schunck, H., Lavik, G., Desai, D. K., Großkopf, T., Kalvelage, T., Löscher, C. R., et al. (2013). Giant hydrogen sulfide plume in the oxygen minimum zone off Peru supports chemolithoautotrophy. PLoS One 8:e68661. doi: 10.1371/journal.pone.0068661
Smith, C. J., Nedwell, D. B., Dong, L. F., and Osborn, A. M. (2007). Diversity and abundance of nitrate reductase genes (narG and napA), nitrite reductase genes (nirS and nrfA), and their transcripts in estuarine sediments. Appl. Environ. Microbiol. 73, 3612–3622. doi: 10.1128/AEM.02894-06
Stamatakis, A. (2014). RAxML version 8: a tool for phylogenetic analysis and post-analysis of large phylogenies. Bioinformatics 30, 1312–1313. doi: 10.1093/bioinformatics/btu033
Stein, L. Y. (2011a). “Chapter 6—surveying N2O-producing pathways in bacteria” in Methods in enzymology research on nitrification and related processes, Part A. ed. M. G. Klotz (Academic Press), 131–152.
Stein, L. Y. (2011b). “Heterotrophic nitrification and nitrifier denitrification” in Nitrification. eds. B. B. Ward, D. J. Arp, and M. G. Klotz (John Wiley & Sons, Ltd), 95–114.
Stein, L. Y., Klotz, M. G., Lancaster, K. M., Nicol, G. W., Qin, W., Schleper, C., et al. (2022). Comment on "a critical review on nitrous oxide production by ammonia-oxidizing archaea" by Lan Wu, Xueming Chen, Wei Wei, Yiwen Liu, Dongbo Wang, and Bing-Jie Ni. Environ. Sci. Technol. 55, 797–798. doi: 10.1021/acs.est.0c06792
Sun, X., Jayakumar, A., and Ward, B. B. (2017). Community composition of nitrous oxide consuming bacteria in the oxygen minimum zone of the eastern tropical South Pacific. Front. Microbiol. 8:1183. doi: 10.3389/fmicb.2017.01183
Sun, X., Kop, L. F. M., Lau, M. C. Y., Frank, J., Jayakumar, A., Lücker, S., et al. (2019). Uncultured Nitrospina-like species are major nitrite oxidizing bacteria in oxygen minimum zones. ISME J. 13, 2391–2402. doi: 10.1038/s41396-019-0443-7
Suter, E. A., Pachiadaki, M. G., Montes, E., Edgcomb, V. P., Scranton, M. I., Taylor, C. D., et al. (2021). Diverse nitrogen cycling pathways across a marine oxygen gradient indicate nitrogen loss coupled to chemoautotrophic activity. Environ. Microbiol. 23, 2747–2764. doi: 10.1111/1462-2920.15187
Tyrrell, T., and Lucas, M. I. (2002). Geochemical evidence of denitrification in the Benguela upwelling system. Cont. Shelf Res. 22, 2497–2511. doi: 10.1016/S0278-4343(02)00077-8
Ulloa, O., Wright, J., Belmar, L., and Hallam, S. (2013). “Pelagic oxygen minimum zone microbial communities” in The Prokaryotes: Prokaryotic Communities and Ecophysiology. eds. E. Rosenberg, E. F. DeLong, S. Lory, E. Stackebrandt, and F. Thompson (Berlin: Springer), 113–122.
Verheye, H., Lamont, T., Huggett, J., Kreiner, A., and Hampton, I. (2016). Plankton productivity of the Benguela current large marine ecosystem (BCLME). Environ. Dev. 17, 75–92. doi: 10.1016/j.envdev.2015.07.011
Vuillemin, A. (2022). Nitrogen cycling activities during decreased stratification in the coastal oxygen minimum zone off Namibia. bioRxiv 516596.
Vuillemin, A., Coskun, Ö. K., and Orsi, W. D. (2022). Microbial activities and selection from surface ocean to subseafloor on the Namibian continental shelf. Appl. Environ. Microbiol. 88:e0021622. doi: 10.1128/aem.00216-22
Vuillemin, A., Kerrigan, Z., D’Hondt, S., and Orsi, W. D. (2020a). Exploring the abundance, metabolic potential and gene expression of subseafloor Chloroflexi in million-year-old oxic and anoxic abyssal clay. FEMS Microbiol. Ecol. 96:fiaa223. doi: 10.1093/femsec/fiaa223
Vuillemin, A., Vargas, S., Coşkun, Ö., Pockalny, R., Murray, R., Smith, D., et al. (2020b). Atribacteria reproducing over millions of years in the Atlantic abyssal subseafloor. MBio 11, e01937–e01920. doi: 10.1128/mBio.01937-20
Vuillemin, A., Wankel, S. D., Coskun, Ö. K., Magritsch, T., Vargas, S., Estes, E. R., et al. (2019). Archaea dominate oxic subseafloor communities over multimillion-year time scales. Sci. Adv. 5:eaaw4108. doi: 10.1126/sciadv.aaw4108
Wasmund, N., Siegel, H., Bohata, K., Flohr, A., Hansen, A., and Mohrholz, V. (2016). Phytoplankton stimulation in frontal regions of Benguela upwelling filaments by internal factors. Front. Mar. Sci. 3:211. doi: 10.3389/fmars.2016.00210
Weatherall, P., Tozer, B., Arndt, J. E., Bazhenova, E., Bringensparr, C., Castro, C. F., et al. (2021). The GEBCO_2021 grid—a continuous terrain model of the global oceans and land. Br. Oceanogr. Data Centre. doi: 10.5285/c6612cbe-50b3-0cff-e053-6c86abc09f8f
Woebken, D., Fuchs, B. M., Kuypers, M. M. M., and Amann, R. (2007). Potential interactions of particle-associated anammox bacteria with bacterial and archaeal partners in the Namibian upwelling system. Appl. Environ. Microbiol. 73, 4648–4657. doi: 10.1128/AEM.02774-06
Wrage-Mönnig, N., Horn, M. A., Well, R., Müller, C., Velthof, G., and Oenema, O. (2018). The role of nitrifier denitrification in the production of nitrous oxide revisited. Soil Biol. Biochem. 123, A3–A16. doi: 10.1016/j.soilbio.2018.03.020
Wright, J. J., Konwar, K. M., and Hallam, S. J. (2012). Microbial ecology of expanding oxygen minimum zones. Nat. Rev. Microbiol. 10, 381–394. doi: 10.1038/nrmicro2778
Wyman, M., Hodgson, S., and Bird, C. (2013). Denitrifying Alphaproteobacteria from the Arabian Sea that express nosZ, the gene encoding nitrous oxide reductase, in oxic and suboxic waters. Appl. Environ. Microbiol. 79, 2670–2681. doi: 10.1128/AEM.03705-12
Zhang, Y., Gui, H., Zhang, S., and Li, C. (2022). Diversity and potential function of prokaryotic and eukaryotic communities from different mangrove sediments. Sustainability 14:3333. doi: 10.3390/su14063333
Zhang, Y., Qin, W., Hou, L., Zakem, E. J., Wan, X., Zhao, Z., et al. (2020). Nitrifier adaptation to low energy flux controls inventory of reduced nitrogen in the dark ocean. Proc. Natl. Acad. Sci. 117, 4823–4830. doi: 10.1073/pnas.1912367117
Keywords: oxygen minimum zone, fixed nitrogen, nitrification–denitrification, Benguela upwelling system, metatranscriptome and metagenome, stable-isotope probing
Citation: Vuillemin A (2023) Nitrogen cycling activities during decreased stratification in the coastal oxygen minimum zone off Namibia. Front. Microbiol. 14:1101902. doi: 10.3389/fmicb.2023.1101902
Edited by:
Yizhi Sheng, China University of Geosciences, ChinaReviewed by:
Xiyang Dong, Third Institute of Oceanography of the Ministry of Natural Resources, ChinaLiang Guo, Xi'an University of Science and Technology, China
Copyright © 2023 Vuillemin. This is an open-access article distributed under the terms of the Creative Commons Attribution License (CC BY). The use, distribution or reproduction in other forums is permitted, provided the original author(s) and the copyright owner(s) are credited and that the original publication in this journal is cited, in accordance with accepted academic practice. No use, distribution or reproduction is permitted which does not comply with these terms.
*Correspondence: Aurèle Vuillemin, ✉ aurele.vuillemin@gfz-potsdam.de