- 1Department of Food Science, Dairy at Guelph, Ontario Agricultural College, University of Guelph, Guelph, ON, Canada
- 2Independent Researcher, Saint-Jean-sur-Richelieu, QC, Canada
- 3Agriculture and Agri-Food Canada, Southern Crop Protection and Food Research Center, Ottawa, ON, Canada
- 4Lallemand SAS, Animal Nutrition, Blagnac, France
The effects of farm management practices and seasonal variation on the microbial community and chemical composition of corn and grass-legume silage are largely understudied due to the advantages of controlled mini-silo experiments. This study aims to investigate the effects that some key farm factors (use of an inoculant, farm region, and bunker or tower silo) and seasonal variations have on corn and grass-legume silage from farms across Ontario, Quebec, and New York. The silage was either treated with a commercial inoculant (Lallemand Biotal Buchneri 500® or Chr Hansen SiloSolve FC®) or left untreated. The bacterial communities of silage were compared to those of raw bulk tank milk from the same farm to determine if they were similarly affected by management practices or seasonal variations. Family level analysis of the 16S rRNA V3-V4 gene amplicon bacterial community, the ITS1 amplicon fungal community, NMR water soluble metabolome, and mycotoxin LC–MS were performed on silage over a two-year period. Chemical compounds associated with the use of inoculants in corn and grass-legume silage were higher in inoculated corn (acetate, propane-1,2-diol, γ-aminobutyrate; p < 0.001) and grass-legume (propionate; p = 0.011). However, there was no significant difference in the relative abundance (RA) of Lactobacillaceae in either silage type. Leuconostocaceae was higher in non-inoculated corn (p < 0.001) and grass-legume (p < 0.001) silage than in inoculated silage. Tower silos had higher RA of Leuconostocaceae (p < 0.001) and higher pH (p < 0.001) in corn and grass-legume silage. The one farm that used liquid manure with no other fertilizer type had higher RA of Clostridiaceae (p = 0.045) and other rumen/fecal (p < 0.006) bacteria in grass-legume silage than all other farms. Seasonal variation affected most of the key silage microbial families, however the trends were rarely visible across both years. Few trends in microbial variation could be observed in both silage and bulk tank milk: two farms had higher Moraxellaceae (p < 0.001) in milk and either corn or grass-legume silage. In farms using an inoculant, lower Staphylococcaceae was observed in the raw bulk tank milk.
1. Introduction
Corn, grasses, and legumes are common forage crops used as dairy cattle feed across the globe, and ensiling is the most common form of feed preservation. The temperature variation throughout the year with a particular impact of the cold season in the northern regions of North America, Europe, and Asia highlight the importance of silage preservation (Kennang Ouamba et al., 2022). Epiphytic bacteria from the field forage, particularly lactic acid bacteria (LAB), will often dominate the microbial community of ensiled forage. Those LAB drive silage quality and the presence of potentially harmful microorganisms both to cattle health and dairy processing (Borreani et al., 2018; Driehuis et al., 2018). Epiphytic LAB can drop the pH of silage rapidly within the first week of ensiling (Borreani et al., 2018). However, depending on the specific LAB present in the silage and conditions of fermentation (such as forage buffering capacity, dry matter at ensiling, available sugar, packing density, among others) the pH decrease may be slow, leaving time for other epiphytic bacteria to compete such as Enterobacteriaceae (Borreani et al., 2018), Clostridiaceae (Li et al., 2020), Bacillaceae, and potentially pathogenic bacteria (Ávila and Carvalho, 2020).
The use of LAB as silage inoculants is common on dairy farms throughout North America to increase aerobic stability, preserve forage, increase feed efficiency, and even improve milk production when feeding common forage crops such as grasses, legumes, and corn (Muck et al., 2018). Inoculants are often a combination of obligate homofermentative strains responsible for rapid pH drop through lactic acid production (Pediococcus pentosaceus, Pediococcus acidilactici, Enterococcus faecium, Lactococcus lactis), facultative heterofermentative strains (Lactiplantibacillus plantarum), and obligate heterofermentative strains (Lentilactobacillus buchneri, Lentilactobacillus hilgardii, Lentilactobacillus diolivorans) of LAB producing organics acids with antifungal properties and enhancing aerobic stability (Weinberg and Muck, 1996). Their effects on the microbiota of silage and aerobic deterioration have been shown in mini-silo operations to be effective at controlling epiphytic bacteria and fungi (Drouin et al., 2021).
In controlled silage experiments such as mini-silos, exposure to air, humidity, and temperature changes are intentionally controlled (Kim and Adesogan, 2006), so single factors can be explored to measure individual effects. This is not the case in large scale silage operations on farms, where silage fermentation conditions vary from farm to farm depending on specific conditions such as silo type, forage growing conditions, packing density, ensiling temperature, storage periods, regional considerations, and individual practices (Filya et al., 2007; Muck et al., 2015; Windle and Kung, 2016; Randby et al., 2020). However, because of the nature of silage research and the use of mini silos, there is little to no information on most of these specific effects, due to the difficulties in studying these factors experimentally in commercial operations.
The purpose of this study was to determine the effects of seasonal factors (temperature) and farm specific factors (epiphytic bacteria, fertilizers) on the microbial community and metabolomic composition of corn and mixed grass-legume silage in commercial management systems on seven farms spanning Ontario, Québec, and northern New York state. Furthermore, the samples were divided by silo type (varying in density and exposure to air) and the use of an inoculant or not (impacting pH drop and acetic acid content), to determine if these factors were able to affect any of the microbial variations linked to season of feed-out and geographical location.
2. Materials and methods
2.1. On-site farm sampling
The seven farms included 2 farms in Southwestern Ontario, Canada, 4 farms in the Montérégie region, Québec, Canada, and 1 farm in Clinton County in northeastern New York state, USA who accepted to participate in this study. Of the seven farms, three stored the silage in tower silos and four stored the silage in bunker silos. Four farms used an inoculant (INO; two bunkers, two towers), and three farms did not use a microbial additive on the forage (NIS; two bunkers, one tower; Table 1). Farms L05, Q02, and Q03 used Biotal Buchneri 500® (Pediococcus pentosaceus 12455 and Lentilactobacillus buchneri 40788) as inoculant for the corn and the grass-legume silage, while farm E01 used Chr Hansen SiloSolve FC® (Lentilactobacillus buchneri LB1819 and Lactococcus lactis O224). Samples of corn and mixed grass-legume forage and silage as well as the milk were taken every 2 months for 2 years starting in September of 2018 and ending in July of 2020. Sampling of the silage was performed as soon as possible after the morning preparation of the total mixed ration on the farms. The sampling procedure of bunker silos was to remove 5 cm of the face layer that was exposed to oxygen and sample around 500 grams of silage, collected from five different points across the bunker face and then pooled into one sample. This procedure was done three times on each sampling day, for a total of three pooled samples per silage type per sampling day. For tower silos, the feed was run for 30 s to clear silage that had been exposed to oxygen, and then around 500 grams of sample were taken directly from the feed chute three times. A total of 42 samples of grass-legume and 42 samples of corn per farm were collected and analyzed for a total of 252 grass-legume and 252 corn silage samples across both years.
Fresh forage of mixed grass-legume and corn was taken in triplicate from the forage truck on-farm directly after cutting at 2 cm theoretical length of cut. There were three cuts of mixed grass-legume forage per year (spring, summer, fall), and one cut of corn per year (fall; Table 1). Total samples collected and analyzed from forage harvests were 18 for grass-legume per farm (total of 126 samples for grass-legume forage across both years) and 6 for corn per farm (total of 42 samples for corn forage across both years). All samples were placed on ice for transportation to the laboratory and placed into a − 20°C freezer until further analysis.
Milk sampling was done in single replicate at each silage sampling period across 2 years for a total of 12 milk samples per farm and 84 for all farms across both years. Samples were taken by stirring the bulk milk tank for 30 s before running a small amount of milk into the drain from the bottom tank valve and then placing a 200 ml container under the running milk. All samples were placed on ice for transportation to the laboratory and placed into a −20°C freezer until further analysis.
2.2. DNA extraction and 16S rRNA gene and ITS amplicon sequencing
DNA extraction for the forage and silage samples was performed according to the methodology described by Drouin et al. (2019). Overall, 5 g of sample was placed into a 50 ml conical tube and filled with 10 ml of sterile water. Tubes were then placed in a sonicator bath (FB-11203, Thermo Fisher, Waltham, MA, USA) and sonicated for 10 min at 50°C, after which they were vortexed for 1 min at high speed. Three milliliters of supernatant were then transferred to two sterile microcentrifuge tubes while avoiding as much plant debris as possible. Microtubes were centrifuged at 20.65 × g for 30 s to precipitate any large plant debris in the supernatant. Avoiding the pellet containing plant debris, the supernatant was then transferred to a clean microtube and centrifuged for 1 min at 10,000 × g. The supernatant was removed, and the pellets were treated using the protocol provided in the Quick-DNA Fecal/Soil Microbe Miniprep DNA extraction kit (Zymo Research, Irvine, CA, USA).
Preparation of the milk samples for DNA extraction was performed according to the methodology described by Julien et al. (2008). A 1.5 ml solution of 25% w/v sodium citrate was heated up to 45°C and mixed with 25 ml of raw milk in a stomacher bag. The bag was then homogenized in a stomacher at 200 rpm for 5 min, and the contents were then transferred to a 50 ml conical tube. The tube was centrifuged at 15,000 × g for 15 min. The supernatant and cream were poured out of the falcon tube and fat was removed with a cotton swab, leaving a cell pellet. The cell pellet was then washed three times by suspending in 1 ml of 2% sodium citrate using a pipette and centrifuged at 10,000 × g for 5 min before removing the supernatant. The cell pellet was then treated using the protocol provided in the Quick-DNA Fecal/Soil Microbe Miniprep DNA extraction kit (Zymo Research, Irvine, CA, USA).
DNA concentration was measured using Qubit Fluorometric Quantitation (Qubit 4, Invitrogen, Waltham, MA, USA) and then diluted to 5 ng/ml and placed into 96-well microplates. Two sets of samples were then submitted to the University of Guelph Genomics Facility for library preparation and sequencing of the 16S V3-V4 rRNA gene region for bacterial amplicons and the ITS1 region for fungal amplicons using the Illumina Miseq platform. Briefly, the 16S V3-V4 rRNA and ITS1 regions were amplified separately using a limited cycle standard PCR. Primers used for the initial amplification of the 16S amplicons were S-D-Bact-0341-b-S-17 (5′-TCG TCG GCA GCG TCA GAT GTG TAT AAG AGA CAG CCT ACG GGN GGC WGC AG) and S-D-Bact-0785-a-A-21 (5′-GTC TCG TGG GCT CGG AGA TGT GTA TAA GAG ACA GGA CTA CHV GGG TAT CTA ATC C) to produce amplicons under 550 bp (Klindworth et al., 2013). For the ITS1, initial amplification of a set of 8 forward primers and 7 reverse primers were pooled to ensure that gaps in taxonomic coverage that may be present with the use of a single ITS1 amplification primer set were covered (Bellemain et al., 2010; Deshpande et al., 2016). ITS1 amplification was designed to amplify regions usually ranging from 145 and 695 bp. The PCR products were then ligated with Illumina flow cell adapters, gene specific adapters, and index primers to prepare for sequencing. Universal flow cell adapter sequences were forward (5′-AAT GAT ACG GCG ACC ACC GAG ATC TAC AC) and reverse (5’-CAA GCA GAA GAC GGC ATA CGA GAT). Gene specific adapter sequences were forward (5’-TCG TCG GCA GCG TC) and reverse (5’-GTC TCG TGG GCT CGG). There were 8 unique forward index primers and 12 unique reverse index primers to cover all 96 samples in each MiSeq run.
2.3. Quantification of water-soluble chemical compounds
Water soluble chemical compounds were quantified using nuclear magnetic resonance at the University of Guelph NMR Center. One hundred and eighty milliliters of distilled water were added to 20 g of mixed grass-legume or corn silage in a blender and mixed for 1 min. Using a large funnel with gauze, the blended sample was poured into a 250 ml Erlenmeyer flask. After the liquid was filtered with 4 × 4 medical gauze pads (AMD Medicom Inc., Montréal, QC, Canada), 50 ml was moved to a conical tube and the pH was read using a pH meter. The remainder of the liquid was further filtered using Grade 1 circular filter paper (Whatman plc, Maidstone, UK) into a 15 ml conical tube. The filtered liquid was then further filtered into microcentrifuge tubes using 0.22 mm syringe filters (MilliporeSigma, Burlington, MA, USA). A final volume of 630 μl was then mixed thoroughly with 70 μl of ChenomX DSS NMR internal standard (Chenomx, Edmonton, AB, Canada) and submitted to the NMR center of the University of Guelph for analysis.
Chenomx NMR Mixture Analysis software was used to measure the molar concentration of peaks of 43 of the most common compounds found across silage samples identified using the Chenomx proprietary reference database. Molar concentrations were then converted into grams/kilogram of dry matter.
2.4. Mycotoxin analysis
The multi-mycotoxin method of Sulyok et al. (2006) was used to extract mycotoxins from forage and silage samples. Briefly, 0.2 ± 0.02 g of silage ground to 1 mm was extracted with 1.3 ml of 79/20/1 (v/v/v) acetonitrile/water/acetic acid. The solutions were first vortexed for 30 s, sonicated at 35°C for 30 min, and shaken on a thermomixer (35°C, 1400 rpm) for 30 min. The samples were then centrifuged and 140 μl were removed. The 140 μl extracts were diluted in 60 μl of water and placed at 4°C for 30 min. The samples were centrifuged, and the supernatants transferred to 250 μl polypropylene vials prior to LC–MS/MS analysis. The samples were analyzed using a targeted, muti-residue MS/MS method, analyzed by a Thermo Vanquish Duo Tandem UHPLC System coupled to a TSQ Altis triple quadrupole mass spectrometer (Thermo Fisher Scientific). Samples were stored in an autosampler at 10°C, and 5 μl was injected onto a Zorbax Eclipse Plus RRHD C18 column (2.1 × 100 mm, 1.8 μm; Agilent) maintained at 35°C with a flow rate of 300 μl/min. Mobile phase A (Optima LC–MS grade water +0.1% formic acid) was held at 98% for 1.0 min. Subsequently, mobile phase B (Optima LC–MS grade acetonitrile +0.1% formic acid) was increased to 22% over 0.25 min, then increased to 35% over 2.75 min. Mobile phase B was increased again to 100% over 3.5 min and held for 2.5 min before returning to 2% over 0.5 min. The OptaMax NG H-ESI source was operated with capillary voltage of 3.5 kV in positive mode and 3.25 kV in negative mode, an ion transfer tube temperature of 325°C, and vaporizer temperature of 295°C. The sheath, auxiliary, and sweep gases were set to 25, 20, and 1 arbitrary units, respectively. The Altis mass spectrometer monitored the transitions with a Q1 and Q3 resolution of 0.7 and 1.2 FWHM resolution, respectively. The argon collision gas was maintained at 1.5 mTorr in the collision cell.
2.5. Bioinformatics analysis
For taxonomic classification of 16S V3-V4 rRNA gene amplicons in corn, grass-legume, and milk, raw Miseq data were run through the phyloseq DADA2 pipeline (filtering, denoising forward and reverse reads, merging forward and reverse reads, and removing chimeras) and rarefaction curves were calculated. The majority of samples adequately covered the microbial community based on rarefaction curves at over 5,600 reads. Samples were then normalized to the samples with the lowest remaining read count. Taxonomy was classified using the Silva 136 database for 16S amplicons and UNITE for ITS amplicons. Data was agglomerated to family level and filtered based on a 0.1% relative abundance threshold present in at least 15% of samples to account for rare taxa unique to a single farm. Sampling periods were grouped into seasons by combining September/November as Fall, January/March as Winter, and May/July as Summer.
Alpha and beta diversity were calculated using the phyloseq pipeline using filtered ASV tables and classified by silo type, inoculation, farm, and season. Standard alpha diversity metrics for microbial community analysis were performed (Shannon, Simpson, Chao1) and Shannon was chosen as the most relevant index in this study, as it considers both presence/absence and abundance of taxa. Weighted and unweighted UniFrac and Bray–Curtis dissimilarity were used to report beta diversity indices.
2.6. Sparse partial least square discriminant analysis and correlations
Family level taxonomic data generated from the phyloseq pipeline as well as NMR water soluble compound quantification were submitted to the sPLS-DA pipeline of the mixOmics package in R.1 The sPLS-DA for all factors were tuned to 2 components after running 10-fold cross-validation 100 times using perf and keepX functions to determine the lowest classification error rate for each factor. sPLS-DA were then plotted separately by either 16S rRNA gene amplicons or NMR compounds using components 1 and 2, and contributions to the axes were plotted to determine families and NMR compounds with significant values within factors (significance was calculated as described in the following Statistical Analysis section). Correlation plots between bacterial families and physicochemical parameters (pH and dry matter) for corn and grass-legume silage were generated using the corplot package in R.
2.7. Statistical analysis
All statistical significance was calculated using either Wilcoxon for two variable comparisons or Kruskall-Wallis one-way analysis of variance for multi-variable comparisons using the FSA package in R by either silo type, inoculation, farm, or season. Forage samples were separated by forage type and statistical analysis was done by farm only. Kruskal-Wallis one-way analysis of variance supplemented with Dunn’s test for post-hoc pairwise comparisons was performed on each individual variable.
3. Results
3.1. Bacterial diversity of the forage
At harvest, the relative abundance (RA) of the 10 main bacterial families and four bacterial family groupings (plant-based, rumen-based, facultative anaerobic spore formers, and potentially pathogenic) in the corn ranged between 44.14 and 90.14% (Table 2). RA of Moraxellaceae was higher in forage from farm L05 than from all other farms. RA of Rhizobiaceae was significantly higher in farms E01 and Q04 than in farm L01 (p = 0.008). RA of endophytic bacteria (p = 0.005) was significantly higher in forage from farm E01 and Q04 than L01 and L05. There were no other significant differences between farms in family level RA in fresh corn forage, but trends for higher Leuconostocaceae and Enterobacteriaceae RA were observed in forage from farm L01 while trends for higher Lactobacillaceae and Pseudomonadaceae RA were noted in forage from farms L05 and Q03, respectively.
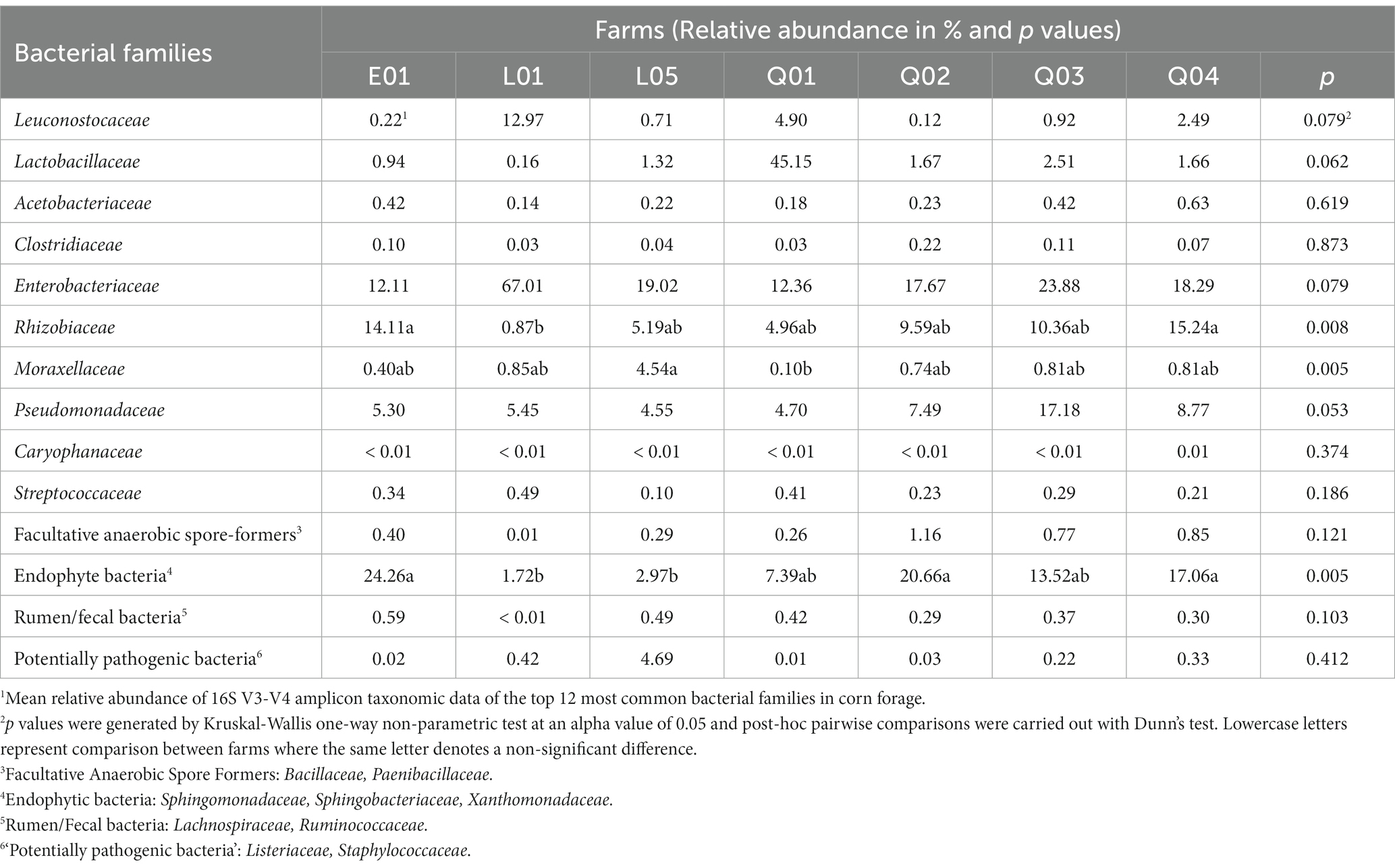
Table 2. Relative abundance (%) of the most common bacterial families from the 16S rRNA gene amplicon sequencing of DNA extracted from the freshly cut corn.
The RA of the 10 main bacterial families and four groups in mixed grass-legume forage ranged from 51.96 to 71.89% (Table 3). Relative abundance of Lactobacillaceae in mixed grass-legume forage was significantly higher in farm Q02 (p < 0.001). RA of Caryophanaceae (formerly Planococcaceae) was significantly higher in farm Q01 than all other farms, except L05 (p = 0.045). RA of Pseudomonadaceae was significantly lower in forage from farms E01, Q02, and Q04 than all other farms except farm Q03 (p = 0.007). There were no other significant differences in family level RA between farms in fresh mixed grass-legume forage except a trend for a lower RA of Enterobacteriaceae in forage from the Q1 farm.
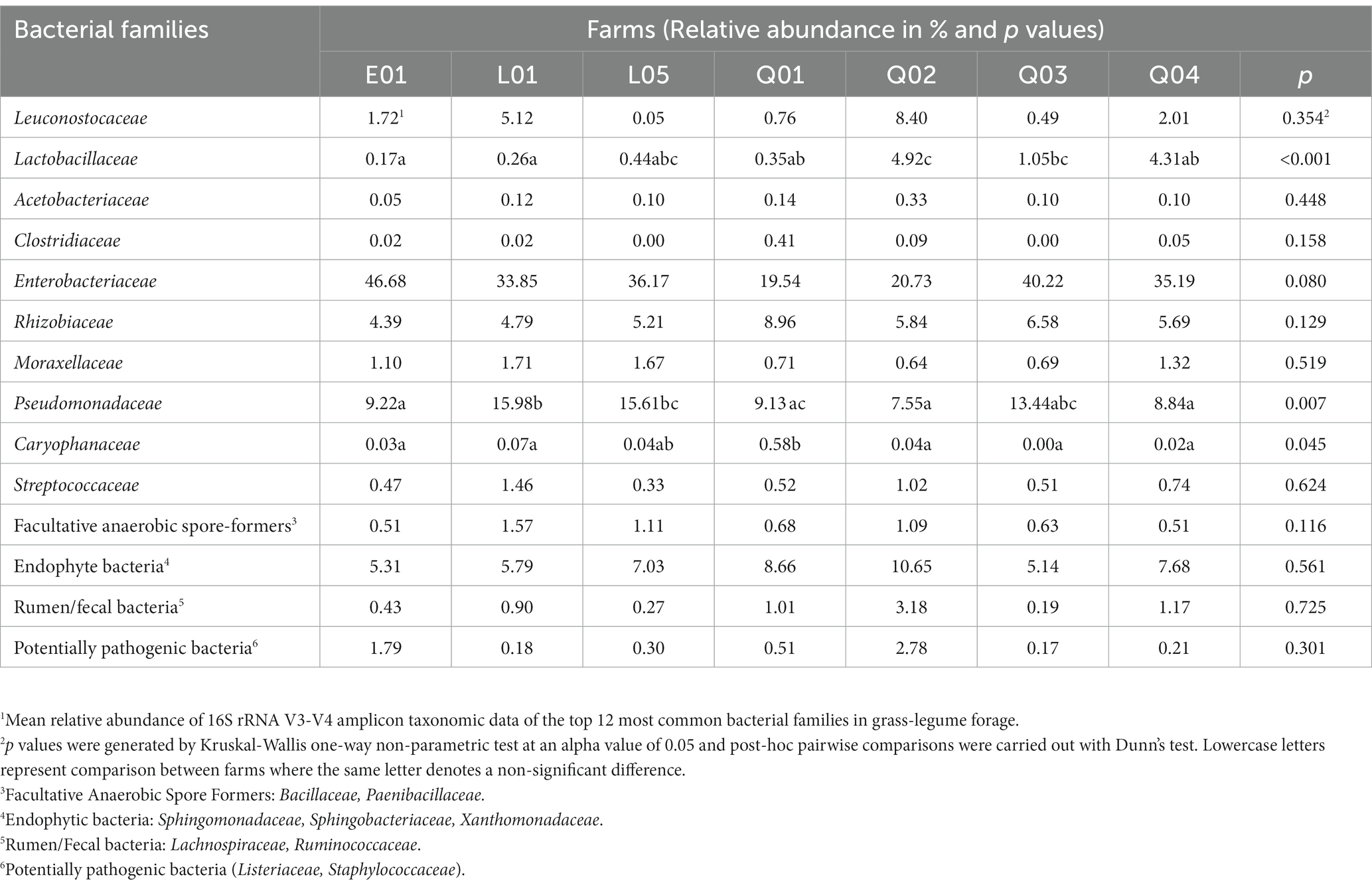
Table 3. Relative abundance (%) of the most common bacterial families from the 16S rRNA gene amplicon sequencing of DNA extracted from the freshly cut grass-legume forage.
3.2. Bacterial diversity of the silage
The alpha diversity (Shannon index) for corn silage (Table 4) showed a significant difference for farms only (L01 and L05 > all other farms; p = 0.004). For grass-legume silage (Table 4), there was a higher Shannon index for INOC vs. NIS (p = 0.029), and significant differences for farms (L05 > all other farms; p = 0.021) and seasons (no visible trends; p < 0.001).
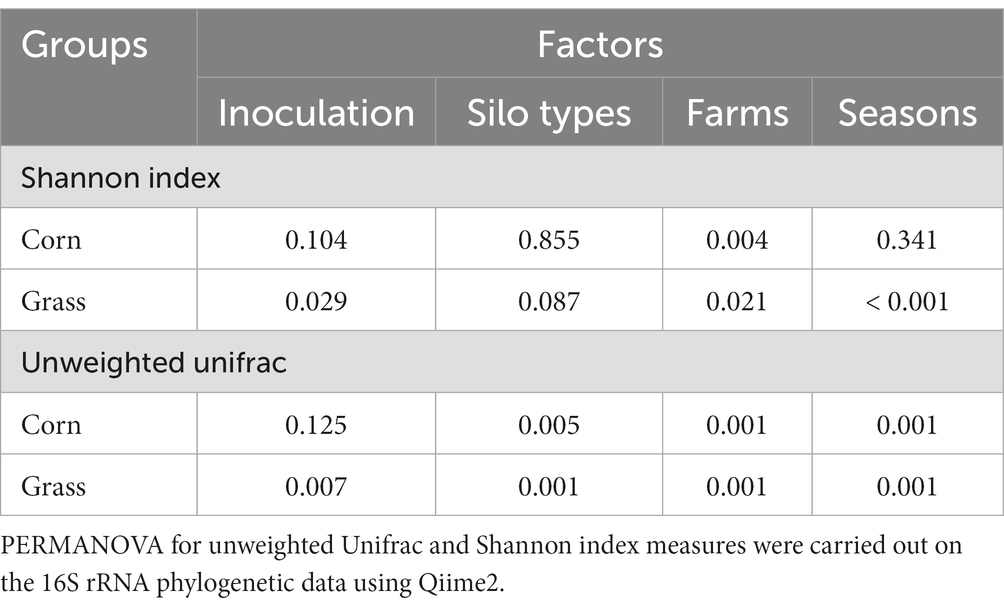
Table 4. Significance (p values) of the effect of four factors on the Unweighted UniFrac (PERMANOVA) in corn and grass-legume silage.
For corn silage, PERMANOVA for unweighted UniFrac on bacteria (Table 4) showed a significant difference between bunkers and towers (p = 0.005). Separation by farm showed a significant difference between farms (p < 0.001). Separation by sampling period showed a significant difference across each period.
Unweighted UniFrac of the bacterial community of the mixed grass-legume silage (Table 4) showed a significant difference between INOC and NIS (p = 0.007), between tower and bunker silo types (p < 0.001), among farm (p < 0.001) and across periods (p < 0.001).
3.3. Difference in bacterial population of silage across experimental factors
The bacterial families in corn silage (Figure 1) separated by inoculation (Figure 1A) with the highest contribution to sPLS-DA loadings of NIS samples on axis 1 were the Leuconostocaceae (mean RA of 4.0% for NIS and 2.5% for INOC). This observation is confirmed by the p value obtained through Wilcoxon test (Table 5) for Leuconostacaceae depending on inoculation factor (p < 0.001). The families contributing to axis 2 were not significantly different between NIS and INOC.
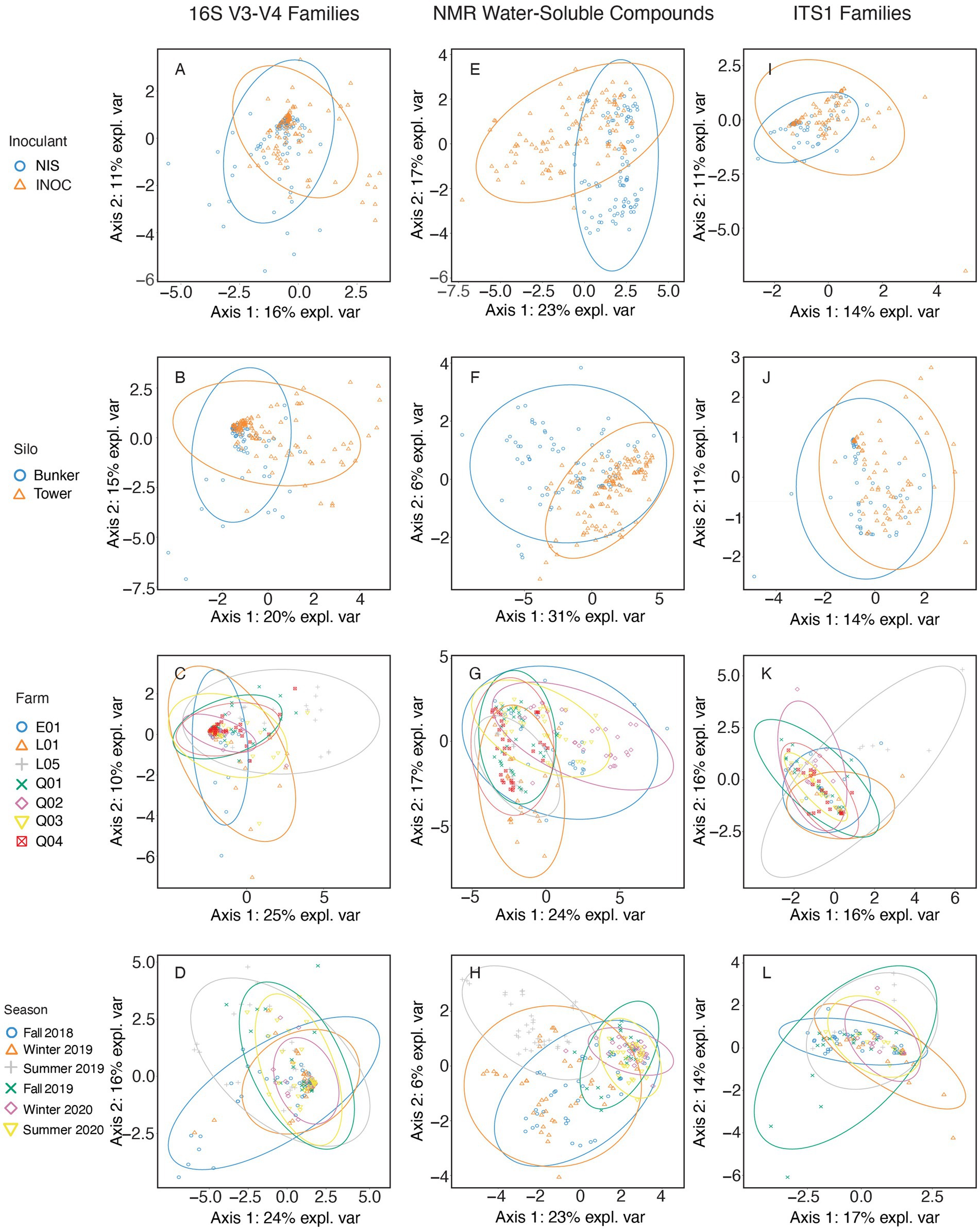
Figure 1. sPLS-DA of corn samples spanning all years and farms using 16S V3-V4 rRNA gene amplicon taxonomic data at a family level (A–D), NMR metabolomic data in grams/kilogram of dry matter (E–H), and ITS1 amplicon taxonomic data at a family level (I–L). Samples are separated by either Inoculant (A,E,I), Silo (B,F,J), Farm (C,G,K), or Season (D,H,L).
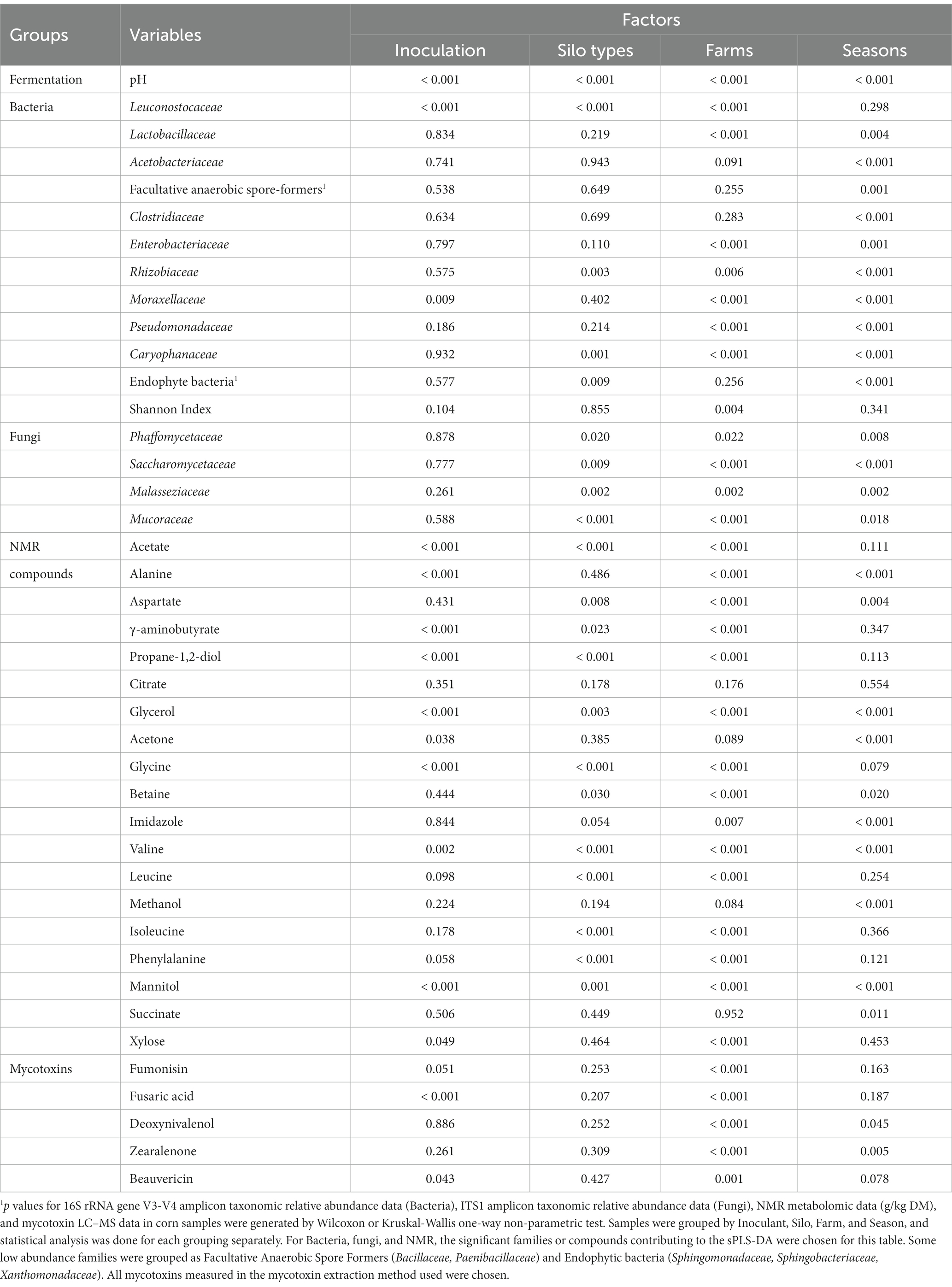
Table 5. Significance (p values) of the effect of four factors on the variation in fermentation parameters, family level taxonomic relative abundance from 16S rRNA gene and ITS1 family level amplicon sequencing of the most abundant families, concentration of the main NMR compounds, and mycotoxins in corn silage.
The families in samples separated by silo type (Figure 1B) with the highest contribution to loadings of tower silo samples on axis 1 were Leuconostocaceae (mean RA of 4.3% for towers and 1.5% for bunkers, p < 0.001 Table 5), Caryophanaceae (0.8% for towers and 0.3% for bunkers, p = 0.001 Table 5), Rhizobiaceae (0.2% for towers and nd for bunkers, p = 0.003, Table 5), and plant related bacterial families (0.6% for towers and 0.2% for bunkers, p = 0.009, Table 5). No families contributing to axis 2 had significant differences through the Wilcoxon test.
The families separated by farm (Figure 1C) with the highest contribution to loadings of farm L05 samples across axis 1 were Caryophanaceae (mean RA of 1.9% for L05 and 0.4% for other farms; Supplementary Table S1) and Moraxellaceae (5.5% for L05 and 0.7% for other farms), and for farm Q02 were Lactobacillaceae (88.6% for farm Q02 and 77.4% for other farms). Families with the highest contribution to loadings of farm L01 samples on axis 2 were Enterobacteriaceae (4.5% for farm L01 and 0.9% for all other farms). Contribution to loadings of farm Q02 samples across axis 2 were Lactobacillaceae, and for farm L05 were Leuconostocaceae (5.9% for farm L05 and 2.7% for other farms). The p values for all these families RA among farms were < 0.001 (Table 5).
The family separated by sampling period (Figure 1D) with the highest contribution to loadings of Summer 2019 samples across axis 1 was the Acetobacteraceae (4.5% for Summer 2019 and 1.8% for other seasons). Contributions to loadings of Fall 2018 samples across axis 1 were the grouping of families for facultative anaerobic spore formers (7.8% for Fall 2018 and 1.5% for other seasons) and Caryophanaceae (1.4% for Fall 2018 and 0.4% for other seasons). The family with the highest contribution to loadings of Fall 2018 samples across axis 2 was Clostridiaceae (0.7% for Fall 2018 and 0.2% for other seasons). For Summer 2018 samples across axis 2, families with the highest contributions to loadings were the families grouped as plant-based bacteria (1.0% for Summer 2018 and 0.2% for other seasons), and Moraxellaceae (2.1% for Summer 2018 and 1.2% for other seasons). The p values for all these families RA across seasons were < 0.001 (Table 5).
The bacterial families from mixed grass-legume silage (Figures 2A–D) with the highest contribution to sPLS-DA loadings of NIS samples on axis 1 (Figure 2A) were Leuconostocaceae (mean RA of 15.6% for NIS and 8.4% for INOC, p < 0.01, Table 6), and Lactobacillaceae (70.0% for NIS and 56.3% for INOC, p = 0.005, Table 6). The family with the highest contribution to loadings of INOC samples across axis 1 was Caryophanaceae (0.7% for INOC and 0.3% for NIS, p = 0.006, Table 6). Families with the highest contribution to loadings of INOC samples across axis 2 were Pseudomonadaceae (0.9% for INOC and 0.2% for NIS, p = 0.012, Table 6) and Planoccocaceae.
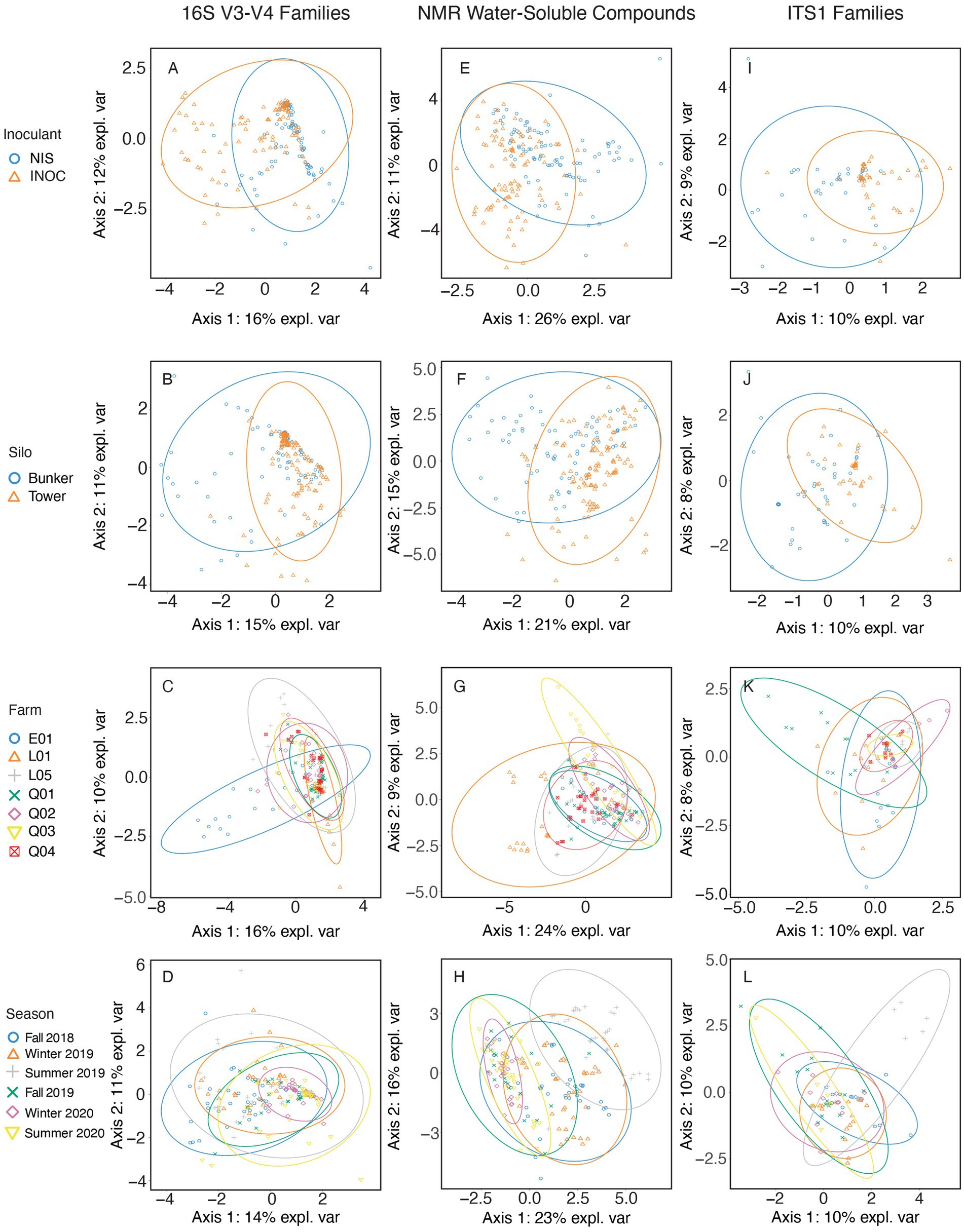
Figure 2. sPLS-DA of mixed grass-legume samples spanning all years and farms using 16S V3-V4 rRNA gene amplicon taxonomic data at a family level (A–D), NMR metabolomic data in grams/kilogram of dry matter (E–H), and ITS1 amplicon taxonomic data at a family level (I–L). Samples are separated by either Inoculant (A,E,I), Silo (B,F,J), Farm (C,G,K), or Season (D,H,L).
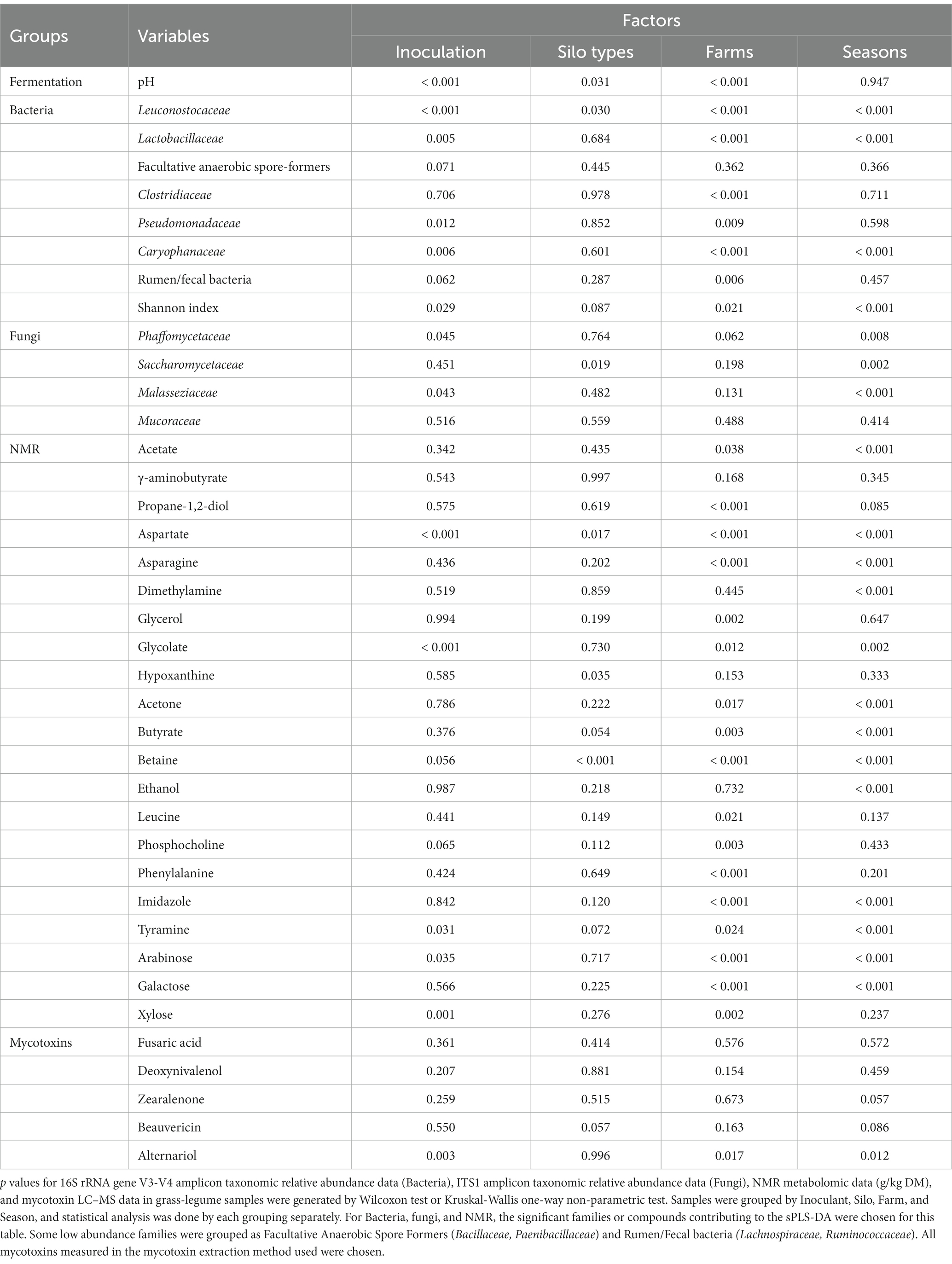
Table 6. Significance (p values) of the effect of four factors on the variation in fermentation parameters, family level taxonomic relative abundance from 16S rRNA gene and ITS1 family level amplicon sequencing of the most abundant families, concentration of the main NMR compounds, and mycotoxins in grass-legume silage.
The family in grass-legume separated by silo (Figure 2B) with the highest contribution to loadings of tower silo samples across axis 1 was Leuconostocaceae (mean RA of 13.6% for towers and 9.2% for bunkers, p = 0.03, Table 6).
The families in mixed grass-legume silage separated by farm (Figure 2C) with the highest contribution to loadings of farm E01 samples across axis 1 were Clostridiaceae (mean RA of 3.7% for farm E01 and 0.3% for other farms, p < 0.001, Supplementary Table S2) and Caryophanaceae (1.2% for farm E01 and 0.2% for other farms, p < 0.001, Table 6) and for farm L01 was Lactobacillaceae (77.6% for farm L01 and 59.6% for other farms, p < 0.001, Table 6). The family with the highest contribution to loadings of farm E01 samples across axis 2 was the grouping of rumen-based bacteria (3.0% for farm E01 and 1.7% for other farms, p = 0.006, Table 6).
The family in mixed grass-legume silage separated by season (Figure 2D) with the highest contribution to loadings of Winter 2020 samples across axis 1 was Lactobacillaceae (72.9% for Winter 2020 and 61.2% for other seasons, p < 0.001, Table 5) and for Fall 2018 was Leuconostocaceae (16.5% for Fall 2018 and 10.5% for other seasons, p < 0.001, Table 6). There were no families with significant differences that contributed to axis 2.
3.4. Correlations between pH, dry matter, and bacterial families
The correlation matrix between pH, dry matter, and bacterial families in corn silage (Supplementary Figure S1) showed positive correlations between dry matter and pH, and a negative correlation between dry matter and Peptostreptococcaceae, Bifidobacteriaceae, Prevotellaceae, and Listeriaceae. The pH was positively correlated with Leuconostocaceae, Brevibacteriaceae, Burkholderiaceae, Clostridiaceae, Bifidobacteriaceae, and Prevotellaceae.
The correlation matrix between pH, dry matter, and bacterial families in grass-legume silage (Supplementary Figure S2) showed a positive correlation between dry matter and Acetobacteraceae, Lachnospiraceae, and Bifidobacteriaceae. The pH was positively correlated with Leuconostocaceae only.
3.5. Discriminant analysis of NMR compounds from silage
The NMR compounds in corn silage (Figures 1E–H) separated by inoculation (Figure 1E) with the highest contribution to sPLS-DA loadings of INOC samples were glycerol (mean concentration of 2.3 g/kg DM for INOC and 1.7 g/kg DM for NIS), propane-1,2-diol (7.0 g/kg DM for INOC and 1.4 g/kg DM for NIS), γ-aminobutyrate (1.6 g/kg DM for INOC and 1.1 g/kg DM for NIS), and acetate (15.5 g/kg DM for INOC and 8.5 g/kg DM for NIS). The compound with the highest contribution to loadings of NIS samples across axis 1 was mannitol (9.7 g/kg DM for NIS and 4.2 g/kg DM for INOC). All compounds presented p values <0.001 between inoculation factors (Table 5).
The compounds in corn silage separated by silo type (Figure 1F) with the highest contribution to loadings of bunker silo samples across axis 1 were leucine (3.0 g/kg DM for bunkers and 2.0 g/kg DM for towers, p < 0.001, Table 5), and isoleucine (1.2 g/kg DM for bunkers and 0.8 g/kg DM for towers, p < 0.001, Table 5). The compounds with the highest contribution to loadings of bunker silo samples across axis 2 was betaine (0.4 g/kg DM for bunkers and 0.3 g/kg DM for towers, p = 0.030, Table 5) and contribution to loadings of tower silo samples across axis 2 was valine (1.5 g/kg DM for towers and 1.1 g/kg DM for bunkers, p < 0.001, Table 5).
Compounds in corn silage separated by farm (Figure 1G) with the highest contribution to loadings of farm Q02 samples across axis 1 were glycine (mean concentration of 1.7 g/kg DM for Q02 and 0.5 g/kg DM for other farms), propane-1,2-diol (13.3 g/kg DM for farm Q02 and 2.0 g/kg DM for other farms), acetate (19.5 g/kg DM for farm Q02 and 10.9 g/kg DM for other farms), and γ-aminobutyrate (2.0 g/kg DM for farm Q02 and 1.3 g/kg DM for other farms). All compounds presented p values <0.001 among farms (Table 5). There were no significant differences in chemical compounds contributing to axis 2.
Compounds in corn silage separated by sampling periods (Figure 1H) with the highest contribution to loadings of Summer 2019 samples across axis 1 were acetone (2.1 g/kg DM for Summer 2019 and 0.4 g/kg DM for other seasons) and imidazole (0.6 g/kg DM for Summer 2019 and 0.4 g/kg DM for other seasons). All compounds presented p values <0.001 across seasons (Table 5).
The NMR compound in mixed grass-legume silage (Figures 2E–H) separated by inoculant (Figure 2E) with the highest contribution to sPLS-DA loadings of NIS samples across axis 1 was xylose (mean concentration of 0.7 g/kg DM for NIS and 0.4 g/kg DM for INOC, p = 0.001; Table 6). There were no significant differences for chemical compounds contributing to axis 2.
The compound in grass-legume silage separated by silo type (Figure 2F) with the highest contribution to loadings of bunker silo samples across axis 1 was betaine (mean concentration of 1.0 g/kg DM for bunkers and 0.6 g/kg DM for towers, p < 0.001, Table 6). There were no significant compound contributing to tower silos across axis 2.
The compound in mixed grass-legume silage separated by farm (Figure 2G) with the highest contribution to loadings of L01 samples across axis 1 was aspartate (mean concentration of 5.4 g/kg DM for farm L01 and 2.1 g/kg DM for other farms; Supplementary Table S2). Compounds with the highest contribution to loadings of farm Q03 samples across axis 2 were propane-1,2-diol (4.5 g/kg DM for farm Q01 and 1.6 g/kg DM for other farms) and asparagine (3.2 g/kg DM for farm Q03 and 2.0 g/kg DM for other farms). All compounds presented p values <0.001 among farms (Table 5).
Compounds in mixed grass-legume silage separated by sampling periods (Figure 2H) with the highest contribution to loadings of Summer 2019 samples across axis 1 were acetone (2.1 g/kg DM for Summer 2019 and 0.7 g/kg DM for other seasons) and ethanol (7.2 g/kg DM for Summer 2019 and 3.2 g/kg DM for other seasons). Compounds with the highest contribution to loadings of Fall 2018 samples across axis 2 were arabinose (1.0 g/kg DM for Fall 2018 and 0.6 g/kg DM for other farms) and galactose (1.6 g/kg DM for Fall 2018 and 1.1 g/kg DM for other seasons). All compounds presented p values <0.001 across seasons (Table 6).
3.6. Compositional variation in the fungal community of silage
There were no significant differences in any fungal family in the sPLS-DA of corn silage separated by inoculation (Figure 1I).
The family in corn silage separated by silo type (Figure 1J) with the highest contribution to loadings of tower silo samples across axis 1 was Mucoraceae (mean RA of 4.8% for towers and 2.7% for bunkers, p < 0.001, Table 5) and for bunkers across axis 1 was Malasseziaceae (6.0% for bunkers and 2.1% for towers, p = 0.002; Table 5).
Fungal families of the corn silage separated by farm (Figure 1K) with the highest contribution to loadings of farm L05 samples across axis 1 was Phaffomycetaceae (mean RA of 14.0% for farm L05 and 2.27% for other farms, p = 0.020, Supplementary Table S1) and for farm L01 was Saccharomycetaceae (70.68% for farm L01 and 40.5% for other farms, p = 0.009, Table 5). The family with the highest contribution to loadings of farm L05 samples across axis 2 was Phaffomycetaceae. The family with the highest contribution to loadings of farm L01 samples across axis 2 was Saccharomycetaceae, and for farm Q01 across axis 2 was Mucoraceae (7.5% for farm Q01 and 3.5% for other farms, p < 0.001, Table 5).
Fungal families of the corn silage separated by season (Figure 1L) with the highest contribution to loadings of Winter 2020 samples across axis 1 was Saccharomycetaceae (mean RA of 65.9% for Winter 2020 and 38.5% for other seasons, p < 0.001, Table 5), and for Winter 2019 across axis 1 was Malasseziaceae (4.9% for Winter 2019 and 3.1% for other seasons, p = 0.002, Table 5). The family with the highest contribution to loadings of Summer 2019 samples across axis 2 was Phaffomycetaceae (1.8% for Summer 2019 and 0.3% for other seasons, p = 0.022, Table 5).
The fungal family in mixed grass-legume silage (Figures 2I–L) separated by inoculation (Figure 2I) with the highest contribution to sPLS-DA loadings on axis 1 was Phaffomycetaceae (mean RA of 5.7% for NIS and 1.6% for INOC, p = 0.045, Table 6). The fungal family of the mixed grass-legume silage by silo (Figure 2J) with the highest contribution to loadings of bunker samples across axis 1 was Saccharomycetaceae (mean RA of 32.5% for bunkers and 16.7% for silos, p = 0.019, Table 6). There were no significant differences in fungal families of the mixed grass-legume silage separated by farm (Figure 2K).
3.7. Quantification of mycotoxins in silage
Mycotoxins in corn silage (Table 5) analyzed by inoculant showed a higher concentration of beauvericin (mean concentration of 342.1 mg/kg DM for INOC and 223.2 mg/kg DM for NIS; p = 0.043), fumonisin (166.7 mg/kg DM for INOC and 88.7 mg/kg DM for NIS; p = 0.051), and fusaric acid (1212.2 mg/kg DM for INOC and 437.4 mg/kg DM for NIS; p < 0.001) content in INOC over NIS. There were no significant differences when separated by silo type. Separation by farm shows a lower concentration of DON (mean concentration of 161.5 mg/kg DM for farm Q02 and 587.3 mg/kg DM for other farms; p < 0.001) and zearalenone (0.7 mg/kg DM for farm Q02 and 27.0 mg/kg DM for other farms; p < 0.001) in Q02 compared to all other farms. The concentration of fumonisin (261.1 mg/kg DM for farm E01 and 103.0 mg/kg DM for other farms; p < 0.001) and fusaric acid (2325.2 mg/kg DM for farm E01 and 620.9 mg/kg DM for other farms; p < 0.001) were higher in silage from farm E01 than all other farms. Separation by period shows a higher concentration of DON (mean concentration of 3730.0 mg/kg DM for Fall 2018 and 372.4 for other seasons; p < 0.001) and zearalenone (195.5 mg/kg DM for Fall 2018 and 11.1 mg/kg DM for other seasons; p < 0.001) in Fall 2018 than all other periods.
In mixed grass-legume silage, there was a higher concentration of alternariol in NIS compared to INOC (mean concentration of 14.1 mg/kg DM for NIS and 4.4 mg/kg DM for INOC; p = 0.018).
3.8. Variation in composition of the microbial community and NMR compounds in milk
The bacterial family in milk (Figure 3) separated by inoculation of silage (Figure 3A) with the highest contribution to sPLS-DA loadings of NIS samples on axis 1 (7% explained variance) was Staphylococcaceae (mean RA of 10.2% for NIS and 4.9% for INOC; Table 7).
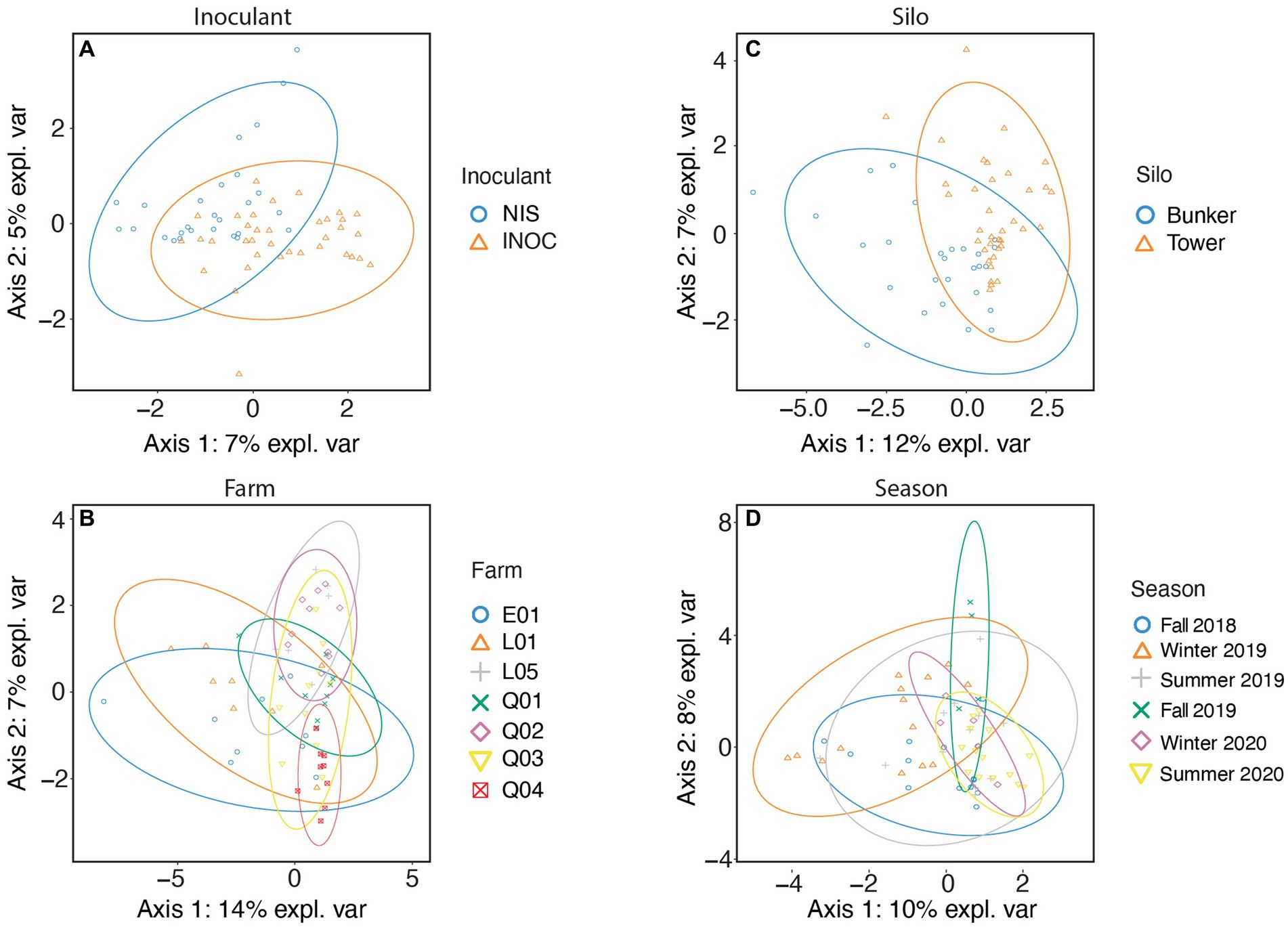
Figure 3. sPLS-DA of milk samples spanning all years and farms using 16S rRNA gene V3-V4 amplicon taxonomic data at a family level. Samples are separated by either Inoculant (A), Farm (B), Silo (C), or Season (D).
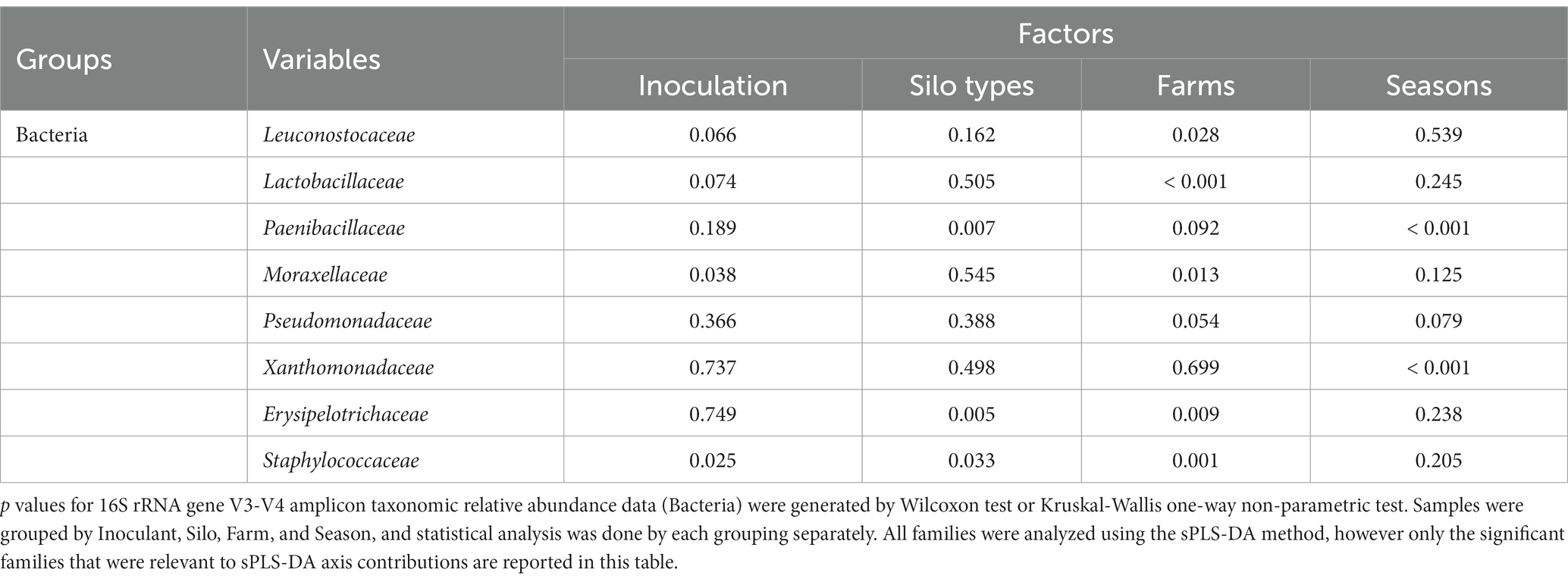
Table 7. Significance (p values) of the effect of four factors on the variation in family level taxonomic relative abundance from 16S rRNA gene family level amplicon sequencing of the main contributing families to sPLS-DAs in bulk tank milk.
The bacterial family in milk (Figure 3C) with the highest contribution to loadings of bunker silo samples on axis 1 was Erysipelotrichaceae (mean RA of 3.1% for bunkers and 1.1% for towers, p = 0.005, Table 7) and for tower samples across axis 1 was Paenibacillaceae (11.6% for towers and 4.1% for bunkers, p = 0.007, Table 7).
The bacterial families in milk separated by farm (Figure 3B) with the highest contribution to loadings of farm L05 samples across axis 2 were Moraxellaceae (11.1% for farm L05 and 3.8% for other farms, p = 0.013, Supplementary Table S3) and (20.1% for farm L05 and 3.5% for other farms, p = 0.054, Table 7) and for farm Q04 across axis 2 was Staphylococcaceae (mean RA of 21.6% for farm Q04 and 4.7% for other farms).
The bacterial family in milk (Figure 3D) with the highest contribution to loadings of Winter 2019 samples across axis 1 were Xanthomonadaceae (mean RA of 13.3% for Winter 2019 and 3.2% for other seasons, p < 0.001, Table 7) and for Fall 2019 across axis 1 was Paenibacillaceae (15.0% for Fall 2019 and 9.2 for other seasons, p < 0.001 Table 7).
4. Discussion
4.1. Diversity of forage bacteria
The epiphytic microbial profile of forage is affected by farm management practices and environmental conditions such as temperature and humidity variations, organic fertilization or soil contamination during harvesting. Nazar et al. (2022) showed that the diversity of the epiphytic microbial community differs between the type of forage (grasses, legumes), but also between species within a type, such as within the grasses (napier and sudan grass). The farms that we studied did indeed use different types of grass (timothy, brome, canary, orchard, fescue, festulolium) which could explain part of the variation in relative abundance of certain bacterial families (Lactobacillaceae, Pseudomonadaceae, and Caryophanaceae). No studies have been published on the difference in diversity of the epiphytic microbiota on the specific grasses and mixtures of grasses used in our study. Due to the high overlap between single grass types across multiple farms, we were unable to observe any key findings in the microbiota of farm silage based on individual grass types. Furthermore, Nazar et al. (2022) showed that, although there were important differences in beta diversity of the epiphytic microbiota between grasses, a short ensiling period of 3 days reduced this difference.
The use of fertilizers affects forage microbiota, as the addition of manure instead of mineral fertilizers can change the microbial diversity of forages, including increased clostridial counts during fermentation (Müller et al., 2014; Drouin et al., 2022). In our study, the Clostridiaceae may have been affected by the use of liquid manure fertilizer, as the only farm to use strictly liquid manure (farm E01) had a higher RA of Clostridiaceae and rumen-based bacteria in grass-legume silage. However, this trend was not observed before the forage was ensiled and was not observed in corn forage or silage from this farm.
4.2. Variation in microbial diversity and metabolome of corn silage
Silo type is an important but understudied aspect of farm management that could have an important role in shaping the microbiota of silage. In corn silage, the RA of Leuconostocaceae was higher in tower silos than in bunker silos. Leuconostocaceae are not adapted to the low pH encountered in low buffering capacity silage, so the abundance of bacteria from this family is generally low in corn silage, which often has a lower pH compared to grass-legume silage (Zhang et al., 2017). In the current study, the higher RA of Leuconostocaceae was correlated with higher pH in the corn silage samples and grass-legume, which aligned with the higher Leuconostocaceae in towers compared to bunkers in corn silage. This higher pH can be due to a lower density common to silage from tower silos, specifically closer to the upper section (Muck et al., 2015) that was collected, which could explain why the Leuconostocaceae are higher in tower silos of corn silage. Furthermore, the higher pH and lower density attributed to tower silos may have led to a higher RA of Saccharomycetaceae. Saccharomycetaceae are common in corn silage and generally dominate the fungal community, particularly in the presence of oxygen which favors yeast growth (Drouin et al., 2021). Although our study did not count yeast cells, it is likely that tower silos had higher total yeast count as tower silos are associated with a higher abundance of yeast (McCallum, 2020) likely due to the increased oxygen from lower density silage (Muck et al., 2015).
Leucine and isoleucine, particularly in corn silage, were higher in bunker silos. These compounds can contribute to pre-weaning growth in early life of dairy calves, which can have long term beneficial effects on milk production (Reiners et al., 2022). To our knowledge, there is no literature explaining the variation of leucine and isoleucine levels in silage. However, Park et al. (2017) compared leucine catabolism between two Lactobacillaceae and Leuconostocaceae isolates from kimchi and determined that the Lactobacillaceae isolates produced more leucine than the Leuconostocaceae, particularly at a lower pH. Although Park et al. (2017) studied isolates instead of the microbial community, it is possible that the higher RA of the Leuconostocaceae and higher pH in tower silos would be linked to the lower leucine and isoleucine in corn silage.
Due to the restricted geographic utilization and the security challenges posed for sampling tower silos (Bahloul et al., 2012), less reliable details on the fermentation parameters of this type of silo are available, although they are recognized as having lower DM losses (Wilkinson and Rinne, 2018). More studies are required to describe the microbial populations and metabolome of this silo type.
The use of the lactic acid bacteria species L. buchneri as an inoculant has been shown to increase RA of Lactobacillaceae in silage compared to NIS and to reduce Leuconostocaceae (Romero et al., 2018). For both corn and grass-legume silage, L. buchneri inoculants have been shown to increase the concentrations of acetic acid, propane-1,2-diol, propionic acid, and γ-aminobutyrate. In our study, the farms that used an inoculant had significantly higher levels of these compounds in corn silage than in silage from farms not using a microbial silage additive. However, on a farm-to-farm basis, mainly farms Q02 and Q03 (located in the same geographical area of Montérégie, Québec), showed higher content of all four of those compounds alongside higher RA of Lactobacillaceae and lower RA of Leuconostocaceae. This highlights the differences due to either specific farm practices or region. A regional difference in the microbial community of corn silage was previously shown in Iran by Gharechahi et al. (2017). The community diversity (alpha diversity) was shown to be higher in the farms from Southwestern Ontario (L01 and L05) than the other farms. This may point to a potential need for a higher inoculation rate for plant material from some farms due to a more diverse natural microbiota of corn, as RA of Lactobacillaceae was lower in these farms after ensiling compared to other farms.
Other factors that affect inoculation success include the size of the inoculant population compared to the epiphytic population, which could have antagonistic activity toward successful fermentation (Kurkjian et al., 2021). It is therefore important for farmers to closely adhere to the application methods provided by inoculant companies to achieve the desired efficacy, particularly in relation to the rate of application, as they have been strictly optimized to obtain ideal performance from each of the bacterial species.
Farm management has a strong impact on the overall quality of silage. Silage from farm Q02 showed a very typical bacterial community and metabolome for silage that has been inoculated. Silage from farm Q02 had higher Lactobacillaceae than other farms, a trend that has been observed both with inoculation of L. plantarum and L. buchneri (Han et al., 2022), and even more so as fermentation time increases (da Silva et al., 2021). The higher amounts of γ-aminobutyrate, 1,2-propanediol, and acetate in silage from farm Q02 are also strong indicators of a successful fermentation and high quality silage (Arriola et al., 2021). Furthermore, farm Q02 showed a lower amount of deoxynivalenol and zearalenone in corn silage, suggesting lower fungal activity or the successful destruction of mycotoxins during fermentation (Ogunade et al., 2018). The indicators of a successful fermentation were not visible in all inoculated silage from farms, however, and some NIS farms (Q01 in particular) showed indicators of good fermentation, such as high Lactobacillaceae, 1,2-propanediol, propionate, and acetate in grass-legume silage. This indicates that regional epiphytic bacteria could produce a high-quality silage with proper fermentation.
The metabolite profile of silage shows a very clear separation of Summer 2019 samples from the other seasons. The amount of acetone was higher in Summer 2019 than all other seasons. Ethanol and butyrate were also higher, but only in grass-legume silage. Generally, a high concentration of butyrate in silage is an indicator of clostridial fermentation (Ávila and Carvalho, 2020). Acetone and ethanol are also associated with clostridial fermentation from the acetone-butanol-ethanol pathway (Luo et al., 2016). Interestingly, the relative abundance of Clostridiaceae in grass-legume silage from the summer of 2019 was not higher than for the other seasons, even though the metabolites seem to indicate clostridial activity. This is likely due to the presence of DNA from dead or sporulated Clostridium cells, which may mask the variation in live cultivable populations. This trend was not visible in the 2020 summer season, which may indicate a higher temperature in the weeks prior to the 2019 summer sampling period. Borreani and Tabacco (2010) showed that temperature differences between 25 and 35°C showed significant increases in butyrate content and clostridia at each ascending temperature interval in corn silage.
Studies suggest that corn is much more susceptible to mycotoxin contamination than other forage types (Křížová et al., 2021). The toxins beauvericin, fumonisin, and fusaric acid are all indicators of common silage molds, particularly Fusarium, often present in silage (Shimshoni et al., 2013). The LAB inoculant species L. buchneri does not reduce mycotoxins produced by common silage molds (Ogunade et al., 2018), while inoculants L. plantarum and P. pentosaceus have been associated with higher levels of DON in corn silage in a trial by Wang et al. (2018). The inoculants used in the silage in our study did not have any effect on mycotoxin limitation with some mycotoxins even reaching higher levels in INOC corn silage. The mechanisms behind the increased mycotoxin content of inoculated corn silage are unclear but may be indirectly due to preservation of the mycotoxins because of lower temperatures of the silage core through reduction of aerobic activity brought on by the use of an inoculant (Wang et al., 2018). That being said, farm Q02 (successful silage fermentation) had lower mycotoxins than other farms. The inoculated silage from this farm had a community profile and metabolome of corn and grass-legume silage that could be associated with inoculation (Arriola et al., 2021). This could indicate that regional effects or proper use of an inoculant could reduce the concentrations of mycotoxin in inoculated silage (Ogunade et al., 2018).
Seasonal effects on the bacterial diversity at the family taxonomic level following fermentation of corn silage were much less impactful than farm or region, but there was a visible trend with samples from Fall 2018 (grouping September and November). Samples from the fall period generally include corn silage harvested the previous year and stored for around 12 months. Fall samples presented higher RA of facultative anaerobic spore formers (Bacillus and Paenibacillus) than all other seasons. Growth and presence of these bacteria are associated with higher pH and oxygen content (Ávila and Carvalho, 2020), conditions associated with the early stage of fermentation or the outer area of bunker silos. Since our sample collection started at silage from the previous year, the presence of these spore formers is likely because of repeated exposure to oxygen and gradual increase in pH over a full year of bunker opening and feed-out. The DON and zearalenone contents were significantly higher in the Fall 2018 season than all other seasons. Corn silage feed-out from the fall generally comes from silage that was put into silos the previous year. The production of DON and zearalenone is produced by field mold, and the mycotoxins are shown to increase over time during ensiling (Jensen et al., 2020). This may explain the high levels of DON during fall seasons. The high level of these two mycotoxins observed only in Fall 2018 line up with other aerobic activity or markers of poor-quality silage of these samples, such as the growth of facultative anaerobic and strict anaerobic spore formers. This indicates that conditions for ensiling were less ideal in the year prior to the trial, reflected in lower quality silage for Fall 2018, but not for Fall 2019.
4.3. Variation in the microbial diversity and metabolome of grass-legume silage
Important differences in the management of the harvest frequency and the type of fertilizer applied during the growing season were present between the farms selected for this study. These factors influenced the microbiota profiles and their evolution over time. Most of the farms applied mainly mineral based fertilizer during the growing season, but one of the farms used liquid manure. The grass-legume silage from this farm presented the 2nd highest RA from families that can be grouped as rumen/fecal related bacteria in our study, the higher RA being observed for another farm using liquid manure and mineral as fertilizers. Specific rumen families have been shown to concentrate in manure, including Ruminococcaceae, Lachnospiraceae, Rikanellaceae, and Clostridiaceae (Ozbayram et al., 2018). This link may be evident in grass-legume silage because the rumen/fecal bacteria are generally sensitive to low pH (Li et al., 2021). and grass-legume silage has a higher pH in comparison to corn silage.
Muck et al. (2015) showed that the use of a bunker silo compared to a tower silo could result in lower DM and higher clostridial fermentation of alfalfa silage. Our study has shown that there is a difference in the microbial diversity of towers and bunker silos, but the absence of a difference in the RA of the Clostridiaceae between the two silo types does not point to any advantage of using tower or bunker silos. The higher RA of Clostridiaceae in grass-legume silage from farm E01 (bunker silo) suggests that the combination of liquid manure as fertilizer, together with the use of a bunker silo, may be the cause of increased Clostridiaceae in silage samples (Müller et al., 2014; Muck et al., 2015). As mentioned for the corn silage, the volume of lower quality silage in bunker silos is observed mainly below the plastic cover and the side walls while the complete surface of the feed-out zone of tower silos could be at a lower density and prone to deterioration, specifically the upper section of the silo (Spörndly, 2018).
The use of an inoculant by some of the farms was clearly visible in corn silage, particularly through the metabolome. However, this trend was not as clear in mixed grass-legume silage. There were significantly less Lactobacillaceae in inoculated silage compared to non-inoculated except for one of the farms in the Montérégie region, and there were no significant differences in the contents of acetic acid, propionic acid, and of γ-aminobutyrate. Furthermore, the microbial diversity was not classified by farm region as seen for corn silage. There was, however, the same trend in propane-1,2-diol in grass-legume as with corn, which was numerically higher in the inoculated farms of Montérégie than all other farms. Da Silva et al. (2017) showed that inoculation with a strain of L. buchneri was correlated with higher propane-1,2-diol but no correlation was observed with acetic acid content. The presence of higher RA of some genera from the Leuconostocaceae, which can also produce acetic acid (Paillart et al., 2016) could potentially have higher metabolic impact in the first months of ensiling over the strain of L. buchneri inoculated on the forage. Furthermore, the RA of Lactobacillaceae does not equate to the relative abundance of the inoculant strain of L. buchneri (Drouin et al., 2021), so it is possible that the RA of Lactobacillaceae observed in the mixed grass-legume silage was not necessarily a direct indicator of inoculant efficacy. We did, however, also observe a relationship between inoculation and higher RA of the Lactobacillaceae in grass-legume silage. Overall, the specific farm or region seems to have a more pronounced effect on the bacterial community and the NMR metabolome of corn silage than it does on mixed grass-legume silage.
The inoculated samples of grass-legume silage from farm E01, which was stored in a bunker, had higher levels of Caryophanaceae (formerly Planococcaceae). Lysinibacillus is a genus of Caryophanaceae that is commonly found in silage and indeed represented close to 100% of the Caryophanaceae in our silage samples. The Lysinibacillus may be associated with late fermented silage (Wang et al., 2022) as well as silage that has been exposed to air (Liu et al., 2013; Duniere et al., 2017). Although the Caryophanaceae may be an indicator of aerobically compromised silage, there is no known negative or positive side effects of higher abundance of this bacterial family in silage or milk.
Alternariol was the only mycotoxin that showed a higher amount in NIS compared to INOC with grass-legume silage. Alternariol is produced by species from the fungal genus Alternaria, which are described as field fungi common in grass forages (Cheli et al., 2013). Alternariol is a genotoxic (Miao et al., 2022) mycotoxin (observed on bacterial and mammalian cells in vitro), however little is known about its in vivo effects on dairy cattle (Gallo et al., 2015). The inoculation of grass-legume silages may prevent the accumulation of alternariol, although more research must be done into the specific effects of alternariol on dairy cattle.
4.4. Microbiota of raw milk
Staphylococcus in milk is associated both with human infection primarily from raw milk cheeses (Rola et al., 2016), and mastitis in dairy cows (Adesiyun et al., 1998). In the current study, the Staphylococcaceae were lower in raw milk from farms that used INOC compared to NIS. Several genera from the Lactobacillaceae family have been shown to inhibit the growth of Staphylococcus aureus in milk. The suggested mechanisms for inhibitory antimicrobial effects by Lactobacillaceae against Staphylococcus aureus are the production of bacteriocins, hydrogen peroxide, lactic acid, and acetic acid (Karska-Wysocki et al., 2010). However, no link between the RA of Staphylococcaceae and Lactobacillaceae in milk was observed. Moreover, our study did not identify any correlation between Lactobacillaceae in silage and Staphylocaccaceae in milk, thus the potential impact of silage inoculants on milk microbiota remains inconclusive. It may very well be that the farms with the practice of inoculation also had a lower incidence of clinical mastitis in their herd.
Spore formers observed in silage have also been detected in raw milk (te Giffel et al., 2002). Our study shows a higher abundance of Paenibacillaceae in raw milk from farms that used tower silos. However, there was no difference in facultative anaerobic spore formers (Bacillaceae, Paenibacillaceae) by silo type in either corn or grass-legume silage.
The RA of Moraxellaceae was higher in raw milk from farms L05 and Q02. Acinetobacter is the dominant genus from the family Moraxellaceae in silage studies (Bai et al., 2021) and was the main genus of Moraxellaceae ASVs in both silage and milk in our study. Acinetobacter are occasionally pathogenic (Towner, 2009), and can cause lipolysis in milk, causing spoilage through rancidity and off-flavors (Hantsis-Zacharov and Halpern, 2007). The RA of Moraxellaceae in the corn silage of farm L05 was higher than all other farms, and it was higher in the grass-legume silage of Q02 compared to all other farms. This points to the possible transfer of Moraxellaceae from both corn and grass-legume silage to the milk, which is then collected in the bulk tank. However, no correlation was observed between the Moraxellaceae family from silage and milk.
The effects of season on milk microbiota were less pronounced than farm management practices. The presence of Xanthomonadaceae was higher during the winter of 2020 which was also observed by Celano et al. (2022). In their study, Celano et al. (2022) correlated the higher presence of Xanthomonadaceae with Enterobacteriaceae and the bovine ketosis marker β-hydroxybutyrate in milk samples. They suggested that higher Xanthomonadaceae and Enterobacteriaceae may represent a shift towards a microbial niche that leads to lower milk quality, however our study did not observe any significant differences in Enterobacteriaceae. On its own, there is no evidence that Xanthomonadaceae could be linked to bovine ketosis or lower milk quality. In the winter seasons, the RA of Xanthomonadaceae was higher (but not significant) in NIS than in INOC, suggesting that the use of an inoculant on silage may contribute to lowering the relative abundance of Xanthomonadaceae in raw milk.
5. Conclusion
Season, farm, silo type, and inoculation clearly affected the chemical variation of both corn and grass-legume silage, while the family level microbiota was not as clear. Individual farm practices may have obscured and dampened some of the observed effects of season and inoculation, suggesting that farm management practices and location may be equally as important. The use of an inoculant was not able to fully control the variations in silage microbiota as we see in mini-silos or from single farm experiments. In this study we did not observe changes in silage microbiota that were correlated with variations in milk microbiota although it is likely that silage management practices including use of an inoculant, silo type, and farm geographical location can affect the microbiota of bulk tank raw milk, but more studies must be done to confirm and expand upon these observations.
Data availability statement
The data presented in this study are deposited in the Borealis repository, and is accessible through this link: https://borealisdata.ca/dataset.xhtml?persistentId=doi:10.5683/SP3/C8CZBK.
Author contributions
PD and GL contributed to the conception and experimental design of the study, project administration and obtained the funding. JH collected and analyzed the samples, performed statistical analysis, prepared the figures, and wrote the first draft. PD organized the database and generated the metadata sheet. JR performed the mycotoxin analysis and wrote the related materials and methods section. PD, GL, and LD reviewed and edited the manuscript. All authors contributed to the article and approved the submitted version.
Funding
Funding for this study was provided by the NSERC Collaborative Research and Development Grant (CRDPJ 529498–18) as well as the NSERC/DFO Industrial Research Chair in Dairy Microbiology (490979–15) held by GL.
Acknowledgments
The authors would like to acknowledge the help received from senior lab technician Jeffrey Gross (University of Guelph Genomics Center) for 16S rRNA gene and ITS amplicon sequencing, and facility manager Sameer Al-Abdul-Wahid for 1H-NMR compound analysis at the University of Guelph Advanced Analysis Center.
Conflict of interest
JH, PD, JR, and GL declare that the research was conducted in the absence of any commercial or financial relationships that could be construed as a potential conflict of interest. LD is employed by Lallemand Inc., however, their affiliation did not impede their ability to follow journal guidelines or to remain impartial during the preparation of this manuscript.
Publisher’s note
All claims expressed in this article are solely those of the authors and do not necessarily represent those of their affiliated organizations, or those of the publisher, the editors and the reviewers. Any product that may be evaluated in this article, or claim that may be made by its manufacturer, is not guaranteed or endorsed by the publisher.
Supplementary material
The Supplementary material for this article can be found online at: https://www.frontiersin.org/articles/10.3389/fmicb.2023.1214915/full#supplementary-material
Footnotes
References
Adesiyun, A. A., Webb, L. A., and Romain, H. T. (1998). Prevalence and characteristics of Staphylococcus aureus strains isolated from bulk and composite milk and cattle handlers. J. Food Prot. 61, 629–632. doi: 10.4315/0362-028x-61.5.629
Arriola, K. G., Oliveira, A. S., Jiang, Y., Kim, D., Silva, H. M., Kim, S. C., et al. (2021). Meta-analysis of effects of inoculation with Lactobacillus buchneri, with or without other bacteria, on silage fermentation, aerobic stability, and performance of dairy cows. J. Dairy Sci. 104, 7653–7670. doi: 10.3168/jds.2020-19647
Ávila, C. S., and Carvalho, B. F. (2020). Silage fermentation—updates focusing on the performance of micro-organisms. J. Appl. Microbiol. 128, 966–984. doi: 10.1111/jam.14450
Bahloul, A., Chavez, M., Reggio, M., Roberge, B., and Goyer, N. (2012). Modeling ventilation time in forage tower silos. J. Agric. Saf. Health 18, 259–272. doi: 10.13031/2013.42328
Bai, C., Wang, C., Sun, L., Xu, H., Jiang, Y., Na, N., et al. (2021). Dynamics of bacterial and fungal communities and metabolites during aerobic exposure in whole-plant corn silages with two different moisture levels. Front. Microbiol. 12:663895. doi: 10.3389/fmicb.2021.663895
Bellemain, E., Carlsen, T., Brochmann, C., Coissac, E., Taberlet, P., and Kauserud, H. (2010). ITS as an environmental DNA barcode for fungi: an in silico approach reveals potential PCR biases. BMC Microbiol. 10:189. doi: 10.1186/1471-2180-10-189
Borreani, G., and Tabacco, E. (2010). The relationship of silage temperature with the microbiological status of the face of corn silage bunkers. J. Dairy Sci. 93, 2620–2629. doi: 10.3168/jds.2009-2919
Borreani, G., Tabacco, E., Schmidt, R. J., Holmes, B. J., and Muck, R. E. (2018). Silage review: factors affecting dry matter and quality losses in silages. J. Dairy Sci. 101, 3952–3979. doi: 10.3168/jds.2017-13837
Celano, G., Calasso, M., Costantino, G., Vacca, M., Ressa, A., Nikoloudaki, O., et al. (2022). Effect of seasonality on microbiological variability of raw cow milk from apulian dairy farms in Italy. Microbiol. Spectr. 10, e00514–e00522. doi: 10.1128/spectrum.00514-22
Cheli, F., Campagnoli, A., and Dell’Orto, V. (2013). Fungal populations and mycotoxins in silages: from occurrence to analysis. Anim. Feed Sci. Technol. 183, 1–16. doi: 10.1016/j.anifeedsci.2013.01.013
da Silva, E. B., Smith, M. L., Savage, R. M., Polukis, S. A., Drouin, P., and And Kung, L. Jr. (2021). Effects of lactobacillus hilgardii 4785 and lactobacillus buchneri 40788 on the bacterial community, fermentation and aerobic stability of high-moisture corn silage. J. Appl. Microbiol. 130, 1481–1493. doi: 10.1111/jam.14892
Da Silva, J., Winckler, J. P. P., Pasetti, M. H. De O., Salvo, P. A. R., Kristensen, N. B., Daniel, J. L. P., et al. (2017). Effects of Lactobacillus buchneri inoculation or 1-propanol supplementation to corn silage on the performance of lactating Holstein cows. Rev. Bras. Zootec. 46, 591–598. doi: 10.1590/S1806-92902017000700006
Deshpande, V., Wang, Q., Greenfield, P., Charleston, M., Porras-Alfaro, A., Kuske, C. R., et al. (2016). Fungal identification using a Bayesian classifier and the Warcup training set of internal transcribed spacer sequences. Mycologia 108, 1–5. doi: 10.3852/14-293
Driehuis, F., Wilkinson, J. M., Jiang, Y., Ogunade, I., and Adesogan, A. T. (2018). Silage review: animal and human health risks from silage. J. Dairy Sci. 101, 4093–4110. doi: 10.3168/jds.2017-13836
Drouin, P., Tremblay, J., and Chaucheyras-Durand, F. (2019). Dynamic succession of microbiota during ensiling of whole plant corn following inoculation with lactobacillus buchneri and Lactobacillus hilgardii alone or in combination. Microorganisms 7:595. doi: 10.3390/microorganisms7120595
Drouin, P., Tremblay, J., da Silva, É. B., and Apper, E. (2022). Changes to the microbiome of alfalfa during the growing season and after ensiling with Lentilactobacillus buchneri and Lentilactobacillus hilgardii inoculant. J. Appl. Microbiol. 133, 2331–2347. doi: 10.1111/jam.15641
Drouin, P., Tremblay, J., Renaud, J., and Apper, E. (2021). Microbiota succession during aerobic stability of maize silage inoculated with Lentilactobacillus buchneri NCIMB 40788 and Lentilactobacillus hilgardii CNCM-I-4785. Microbiol. Open 10:e1153. doi: 10.1002/mbo3.1153
Duniere, L., Xu, S., Long, J., Elekwachi, C., Wang, Y., Turkington, K., et al. (2017). Bacterial and fungal core microbiomes associated with small grain silages during ensiling and aerobic spoilage. BMC Microbiol. 17:50. doi: 10.1186/s12866-017-0947-0
Filya, I., Muck, R. E., and Contreras-Govea, F. E. (2007). Inoculant effects on alfalfa silage: fermentation products and nutritive value. J. Dairy Sci. 90, 5108–5114. doi: 10.3168/jds.2006-877
Gallo, A., Giuberti, G., Frisvad, J. C., Bertuzzi, T., and Nielsen, K. F. (2015). Review on mycotoxin issues in ruminants: occurrence in forages, effects of mycotoxin ingestion on health status and animal performance and practical strategies to counteract their negative effects. Toxins 7, 3057–3111. doi: 10.3390/toxins7083057
Gharechahi, J., Kharazian, Z. A., Sarikhan, S., Jouzani, G. S., Aghdasi, M., and Hosseini Salekdeh, G. (2017). The dynamics of the bacterial communities developed in maize silage. Microb. Biotechnol. 10, 1663–1676. doi: 10.111/1751/7915.12751
Han, H., Wang, C., Huang, Z., Zhang, Y., Sun, L., Xue, Y., et al. (2022). Effects of lactic acid bacteria-inoculated corn silage on bacterial communities and metabolites of digestive tract of sheep. Fermentation 8:320. doi: 10.3390/fermentation8070320
Hantsis-Zacharov, E., and Halpern, M. (2007). Culturable psychrotrophic bacterial communities in raw milk and their proteolytic and lipolytic traits. Appl. Environ. Microbiol. 73, 7162–7168. doi: 10.1128/AEM.00866-07
Jensen, T., De Boevre, M., De Saeger, S., Preußke, N., Sönnichsen, F. D., Kramer, E., et al. (2020). Effect of ensiling duration on the fate of deoxynivalenol, zearalenone and their derivatives in maize silage. Mycotoxin Res. 36, 127–136. doi: 10.1007/s12550-019-00378-4
Julien, M.-C., Dion, P., Lafrenière, C., Antoun, H., and Drouin, P. (2008). Sources of Clostridia in raw milk on farms. Appl. Environ. Microbiol. 74, 6348–6357. doi: 10.1128/AEM.00913-08
Karska-Wysocki, B., Bazo, M., and Smoragiewicz, W. (2010). Antibacterial activity of lactobacillus acidophilus and Lactobacillus casei against methicillin-resistant Staphylococcus aureus (MRSA). Microbiol. Res. 165, 674–686. doi: 10.1016/j.micres.2009.11.008
Kennang Ouamba, A. J., Gagnon, M., Varin, T., Chouinard, P. Y., LaPointe, G., and Roy, D. (2022). Metataxonomic insights into the microbial ecology of farm-scale hay, grass or legume, and corn silage produced with and without inoculants. Front. Syst. Biol. 2:955611. doi: 10.3389/fsysb.2022.955611
Kim, S. C., and Adesogan, A. T. (2006). Influence of ensiling temperature, simulated rainfall, and delayed sealing on fermentation characteristics and aerobic stability of corn silage. J. Dairy Sci. 89: 3122–3132. doi: 10.3168/jds.S0022-0302(06)72586-3
Klindworth, A., Pruesse, E., Schweer, T., Peplies, J., Quast, C., Horn, M., et al. (2013). Evaluation of general 16S ribosomal RNA gene PCR primers for classical and next-generation sequencing-based diversity studies. Nucleic Acids Res. 41:e1. doi: 10.1093/nar/gks808
Křížová, L., Dadáková, K., Dvořáčková, M., and Kašparovský, T. (2021). Feedborne mycotoxins beauvericin and enniatins and livestock animals. Toxins 13:32. doi: 10.3390/toxins13010032
Kurkjian, H. M., Akbari, M. J., and Momeni, B. (2021). The impact of interactions on invasion and colonization resistance in microbial communities. PLoS Comput. Biol. 17:e1008643. doi: 10.1371/journal.pcbi.1008643
Li, R., Jiang, D., Zheng, M., Tian, P., Zheng, M., and Xu, C. (2020). Microbial community dynamics during alfalfa silage with or without clostridial fermentation. Sci. Rep. 10:17782. doi: 10.1038/s41598-020-74958-1
Li, M. M., White, R. R., Guan, L. L., Harthan, L., and Hanigan, M. D. (2021). Metatranscriptomic analyses reveal ruminal pH regulates fiber degradation and fermentation by shifting the microbial community and gene expression of carbohydrate-active enzymes. Anim. Microbiome 3:32. doi: 10.1186/s42523-021-00092-6
Liu, Q. H., Shao, T., and Zhang, J. G. (2013). Determination of aerobic deterioration of corn stalk silage caused by aerobic bacteria. Anim. Feed Sci. Technol. 183, 124–131. doi: 10.1016/j.anifeedsci.2013.05.012
Luo, H., Ge, L., Zhang, J., Ding, J., Chen, R., and Shi, Z. (2016). Enhancing acetone biosynthesis and acetone–butanol–ethanol fermentation performance by co-culturing Clostridium acetobutylicum/Saccharomyces cerevisiae integrated with exogenous acetate addition. Bioresour. Technol. 200, 111–120. doi: 10.1016/j.biortech.2015.09.116
McCallum, (2020). Management practices and quality of first-cut alfalfa-grass silage on Canadian dairy farms. MSc Thesis, Dalhousie University. http://hdl.handle.net/10222/80139
Miao, Y., Wang, D., Chen, Y., Zhu, X., Tang, X., Zhang, J., et al. (2022). General toxicity and genotoxicity of alternariol: a novel 28-day multi-endpoint assessment in male Sprague–Dawley rats. Mycotoxin Res. 38, 231–241. doi: 10.1007/s12550-022-00466-y
Muck, R. E., Brink, G. E., and Broderick, G. A. (2015). Effects of silo type on ensiling alfalfa. Appl. Eng. Agric. 31, 479–486. doi: 10.13031/aea.31.10994
Muck, R. E., Nadeau, E. M. G., McAllister, T. A., Contreras-Govea, F. E., Santos, M. C., and Kung, L. (2018). Silage review: recent advances and future uses of silage additives. J. Dairy Sci. 101, 3980–4000. doi: 10.3168/jds.2017-13839
Müller, C. E., Johansson, M., Salomonsson, A.-C., and Albihn, A. (2014). Effect of anaerobic digestion residue vs. livestock manure and inorganic fertilizer on the hygienic quality of silage and haylage in bales. Grass Forage Sci. 69, 74–89. doi: 10.1111/gfs.12046
Nazar, M., Ullah, M. W., Wang, S., Zhao, J., Dong, Z., Li, J., et al. (2022). Exploring the epiphytic microbial community structure of forage crops: their adaptation and contribution to the fermentation quality of forage sorghum during ensiling. Bioengineering 9:428. doi: 10.3390/bioengineering9090428
Ogunade, I. M., Martinez-Tuppia, C., Queiroz, O. C. M., Jiang, Y., Drouin, P., Wu, F., et al. (2018). Silage review: mycotoxins in silage: occurrence, effects, prevention, and mitigation. J. Dairy Sci. 101, 4034–4059. doi: 10.3168/jds.2017-13788
Ozbayram, E. G., Ince, O., Ince, B., Harms, H., and Kleinsteuber, S. (2018). Comparison of rumen and manure microbiomes and implications for the inoculation of anaerobic digesters. Microorganisms 6:15. doi: 10.3390/microorganisms6010015
Paillart, M. J. M., Vossen, J. M. B. M., Van Der, L. E., Levin, E., Otma, E. C., Snels, J. C. M. A., et al. (2016). Organic acids produced by lactic acid bacteria (Leuconostoc sp.) contribute to sensorial quality loss in modified-atmosphere-packed fresh-cut iceberg lettuce. Acta Hortic. 1141, 289–296. doi: 10.17660/ActaHortic.2016.1141.36
Park, B., Hwang, H., Chang, J. Y., Hong, S. W., Lee, S. H., Jung, M. Y., et al. (2017). Identification of 2-hydroxyisocaproic acid production in lactic acid bacteria and evaluation of microbial dynamics during kimchi ripening. Sci. Rep. 7:10904. doi: 10.1038/s41598-017-10948-0
Randby, Å. T., Halvorsen, H. N., and Bakken, A. K. (2020). Losses and grass silage quality in bunker silos compacted by tractor versus wheel loader. Anim. Feed Sci. Technol. 266:114523. doi: 10.1016/j.anifeedsci.2020.114523
Reiners, J. N., Steele, M. A., Vonnahme, K. A., Maddock Carlin, K. R., and Swanson, K. C. (2022). Effects of supplemental leucine on growth, nutrient use, and muscle and visceral tissue mass in Holstein bull calves fed Milk replacer. Front. Anim. Sci. 3:817173. doi: 10.3389/fanim.2022.817173
Rola, J. G., Czubkowska, A., Korpysa-Dzirba, W., and Osek, J. (2016). Occurrence of Staphylococcus aureus on farms with small scale production of raw milk cheeses in Poland. Toxins 8:62. doi: 10.3390/toxins8030062
Romero, J. J., Joo, Y., Park, J., Tiezzi, F., Gutierrez-Rodriguez, E., and Castillo, M. S. (2018). Bacterial and fungal communities, fermentation, and aerobic stability of conventional hybrids and brown midrib hybrids ensiled at low moisture with or without a homo- and heterofermentative inoculant. J. Dairy Sci. 101, 3057–3076. doi: 10.3168/jds.2017-13754
Shimshoni, J. A., Cuneah, O., Sulyok, M., Krska, R., Galon, N., Sharir, B., et al. (2013). Mycotoxins in corn and wheat silage in Israel. Food Addit. Contam. Part A 30, 1614–1625. doi: 10.1080/19440049.2013.802840
Spörndly, R. (2018). Dry matter losses from different silo structures. Rapp. Sver. Lantbruksuniversitet Institutionen För Husdjurens Utfodr. Och Vård. Available at: https://res.slu.se/id/publ/97898 [Accessed January 4, 2023].
Sulyok, M., Berthiller, F., Krska, R., and Schuhmacher, R. (2006). Development and validation of a liquid chromatography/tandem mass spectrometric method for the determination of 39 mycotoxins in wheat and maize. Rapid Commun. Mass Spectrom. 20, 2649–2659. doi: 10.1002/rcm.2640
te Giffel, M. C., Wagendorp, A., Herrewegh, A., and Driehuis, F. (2002). Bacterial spores in silage and raw milk. Antonie Van Leeuwenhoek 81, 625–630. doi: 10.1023/A:1020578110353
Towner, K. J. (2009). Acinetobacter: an old friend, but a new enemy. J. Hosp. Infect. 73, 355–363. doi: 10.1016/j.jhin.2009.03.032
Wang, Y.-L., Wang, W.-K., Wu, Q.-C., Zhang, F., Li, W.-J., Yang, Z.-M., et al. (2022). The effect of different lactic acid bacteria inoculants on silage quality, phenolic acid profiles, bacterial community and in vitro rumen fermentation characteristic of whole corn silage. Fermentation 8:285. doi: 10.3390/fermentation8060285
Wang, M., Xu, S., Wang, T., Jia, T., Xu, Z., Wang, X., et al. (2018). Effect of inoculants and storage temperature on the microbial, chemical and mycotoxin composition of corn silage. Asian-Australas. J. Anim. Sci. 31, 1903–1912. doi: 10.5713/ajas.17.0801
Weinberg, Z. G., and Muck, R. E. (1996). New trends and opportunities in the development and use of inoculants for silage. FEMS Microbiol. Rev. 19, 53–68. doi: 10.1111/j.1574-6976.1996.tb00253.x
Wilkinson, J. M., and Rinne, M. (2018). Highlights of progress in silage conservation and future perspectives. Grass Forage Sci. 73, 40–52. doi: 10.1111/gfs.12327
Windle, M. C., and Kung, L. (2016). Factors affecting the numbers of expected viable lactic acid bacteria in inoculant applicator tanks. J. Dairy Sci. 99, 9334–9338. doi: 10.3168/jds.2016-10881
Keywords: silage, inoculant, corn, legume, metabolites, mycotoxins, bacteria, fungi
Citation: Huffman J, Drouin P, Renaud JB, Dunière L and LaPointe G (2023) Farm management practices and season dependent factors affect the microbial community and chemical profile of corn and grass-legume silages of farms in Ontario, Québec, and Northern New York. Front. Microbiol. 14:1214915. doi: 10.3389/fmicb.2023.1214915
Edited by:
Marta Laranjo, University of Evora, PortugalCopyright © 2023 Huffman, Drouin, Renaud, Dunière and LaPointe. This is an open-access article distributed under the terms of the Creative Commons Attribution License (CC BY). The use, distribution or reproduction in other forums is permitted, provided the original author(s) and the copyright owner(s) are credited and that the original publication in this journal is cited, in accordance with accepted academic practice. No use, distribution or reproduction is permitted which does not comply with these terms.
*Correspondence: Gisèle LaPointe, glapoint@uoguelph.ca