- 1Department of Marine Microbiology and Biogeochemistry (MMB), NIOZ Royal Netherlands Institute for Sea Research, Den Burg, Netherlands
- 2Department of Earth Sciences, Faculty of Geosciences, Utrecht University, Utrecht, Netherlands
Heterocytous cyanobacteria are important players in the carbon and nitrogen cycle. They can fix dinitrogen by using heterocytes, specialized cells containing the oxygen-sensitive nitrogenase enzyme surrounded by a thick polysaccharide and glycolipid layer which prevents oxygen diffusion and nitrogenase inactivation. Heterocyte glycolipids can be used to detect the presence of heterocytous cyanobacteria in present-day and past environments, providing insight into the functioning of the studied ecosystems. However, due to their good preservation throughout time, heterocyte glycolipids are not ideal to detect and study living communities, instead methods based on DNA are preferred. Currently cyanobacteria can be detected using untargeted genomic approaches such as metagenomics, or they can be specifically targeted by, for example, the use of primers that preferentially amplify their 16S rRNA gene or their nifH gene in the case of nitrogen fixing cyanobacteria. However, since not all cyanobacterial nitrogen fixers are heterocytous, there is currently no fast gene-based method to specifically detect and distinguish heterocytous cyanobacteria. Here, we developed a PCR-based method to specifically detect heterocytous cyanobacteria by designing primers targeting the gene (hglT) encoding the enzyme responsible for the last step in the biosynthesis of heterocyte glycolipid (i.e., a glycosyltransferase). We designed several primer sets using the publicly available sequences of 23 heterocytous cyanobacteria, after testing them on DNA extracts of 21 heterocyte-forming and 7 non-heterocyte forming freshwater cyanobacteria. The best primer set was chosen and successfully used to confirm the presence of heterocytous cyanobacteria in a marine environmental sample.
Introduction
Cyanobacteria are cosmopolitan photosynthetic organisms with a central role in earth’s biogeochemical cycles. Besides their role as primary producers, fixing carbon through photosynthesis using only sunlight and CO2, a variety of cyanobacterial species are also capable of fixing atmospheric nitrogen (N2), which also confers them an important role in the nitrogen cycle (Zehr, 2011; Mishra et al., 2021).
Nitrogen fixation is catalyzed by the nitrogenase enzyme which is easily inactivated in presence of oxygen. Hence, cyanobacteria have developed two main strategies to protect the nitrogenase from the oxygen generated during photosynthesis. One strategy is to separate both processes in time; in most nitrogen-fixing unicellular cyanobacteria N2 fixation only occurs at night, when the photosynthetic machinery is not in use and oxygen is not being produced (Millineaux et al., 1981). Filamentous cyanobacteria developed an alternative strategy, i.e., the spatial separation of both processes. Heterocytous cyanobacteria can develop heterocytes, a type of specialized cell where, when in absence of combined nitrogen, nitrogen fixation takes place (Klugkist and Haaker, 1984; Knutson et al., 2018). Heterocytes protect the nitrogenase enzyme from oxygen using several strategies such as (1) providing spatial separation of nitrogen and carbon fixation by confining nitrogen fixation to specialized cells surrounded by a thick-walled outer membrane composed of polysaccharide (heterocyte envelope polysaccharide, HEP) and an inner layer composed of heterocyte glycolipids (HGs) (Haselkorn, 1978), (2) inactivation of photosystem II in heterocytes to avoid production of oxygen (Tel-Or et al., 1997), and (3) enhanced respiration inside the heterocytes to consume internal oxygen (Wolk et al., 1994).
Currently, the presence of cyanobacteria in the environment can be confirmed by using cyanobacteria-specific 16S rRNA gene primers (Nübel et al., 1997), and the presence of dinitrogen-fixing cyanobacteria by applying primers targeting nifH (Olson et al., 1998), a key gene involved in dinitrogen fixation. However, there are no screening techniques based on genetic methods allowing the detection of heterocytous cyanobacteria in the environment, which could be used to evaluate the potential for cyanobacterial N2 fixation. Thus far, we can only determine the presence of heterocytous cyanobacteria using time and resource intensive methods such as microscopy and lipid analysis, or by using untargeted metagenomic methods. Taxonomic classification of cyanobacteria via microscopic analysis requires expertise and relies solely in morphological traits (Castenholz et al., 2001).
Cyanobacterial taxonomy is complex and has undergone extensive restructuring and revisions in recent years. It has transformed from being a hierarchical system that simply placed morphologically similar taxa into a classification system based on evolutionary relationships. Most of the studies mentioned in this work used the classification system proposed by Rippka et al. (1979), later adopted as the classification standard in the Bergey’s Manual of Systematic Bacteriology (Castenholz et al., 2001). This classification method recognized five subsections or orders, which in turn contained “families,” divided mainly according to their morphology. Heterocytous cyanobacteria occur only in subsections IV (Nostocales) and V (Stigonematales). Although this nomenclature did not reflect evolutionary relationships, it was adopted as convenient and temporary method to classify cyanobacterial strains (Castenholz et al., 2001). However, a recent revision of cyanobacterial taxonomy by Komarek et al. (2014) proposed a system that left aside classification based solely on morphology and focused on a polyphasic approach focusing on gene phylogeny (e.g., 16S rRNA) to achieve monophyletic clusters representing “orders.”
The chemical structure of the HGs and their distribution (Figure 1) has chemotaxonomical value and can potentially also be used to distinguish between cyanobacteria of different orders and families (Table 1; Supplementary Table S1; Gambacorta et al., 1998; Bauersachs et al., 2009a, 2013). For example, hexose-sugar HGs composed of C26 (HG26) diol and keto-ol are mostly present in species of the Nostocaceae family (Nostocaceae and Aphanizomenonaceae orders according to the new 16S rRNA-based classification; Gambacorta et al., 1998; Bauersachs et al., 2009a; Komarek et al., 2014; Table 1; Supplementary Table S1), while hexose C28 HGs (HG28) containing three functional groups (keto-diol and triol) are predominant in members of the Rivulariaceae (Calotrichaceae) family. Likewise, hexose C30 HG (HG30) triols and keto-diols are characteristic of members of the Scytonemataceae (Scytonemataceae and Tolypothrichaceae) family, while members of the Stigonemataceae (Stigonemataceae, Chlorogloeopsidaceae and Hapalosiphonaceae) mostly synthesize hexose C32 HGs (HG32) with three functional groups (Gambacorta et al., 1998). However, there are some exceptions to these general trends. For example, the Nostocaceae (Nostocaceae and Aphanizomenonaceae) family mostly produces hexose HG26 diol and keto-ol, but there are members of this family that also produce hexose HG28 triol and keto-diol (Supplementary Table S1). Although they are usually produced in small amounts, in some cases they are synthesized in larger amounts, such as in Aphanizomenon sp. TR83 (12% of the total HGs) and in Dolichospermum sp. BIR169 (33%) (Bauersachs et al., 2017; Supplementary Table S1). Moreover, Stigonema ocellatum SAG 48.90, a strain belonging to the Stigonematales order (Stigonemataceae), was recently shown to produce mostly hexose HG28 keto-diol (67%) and triol (27%), HGs thought to be characteristic of the Rivulariaceae (Calotrichaceae) family (Supplementary Table S1; Bauersachs et al., 2019).
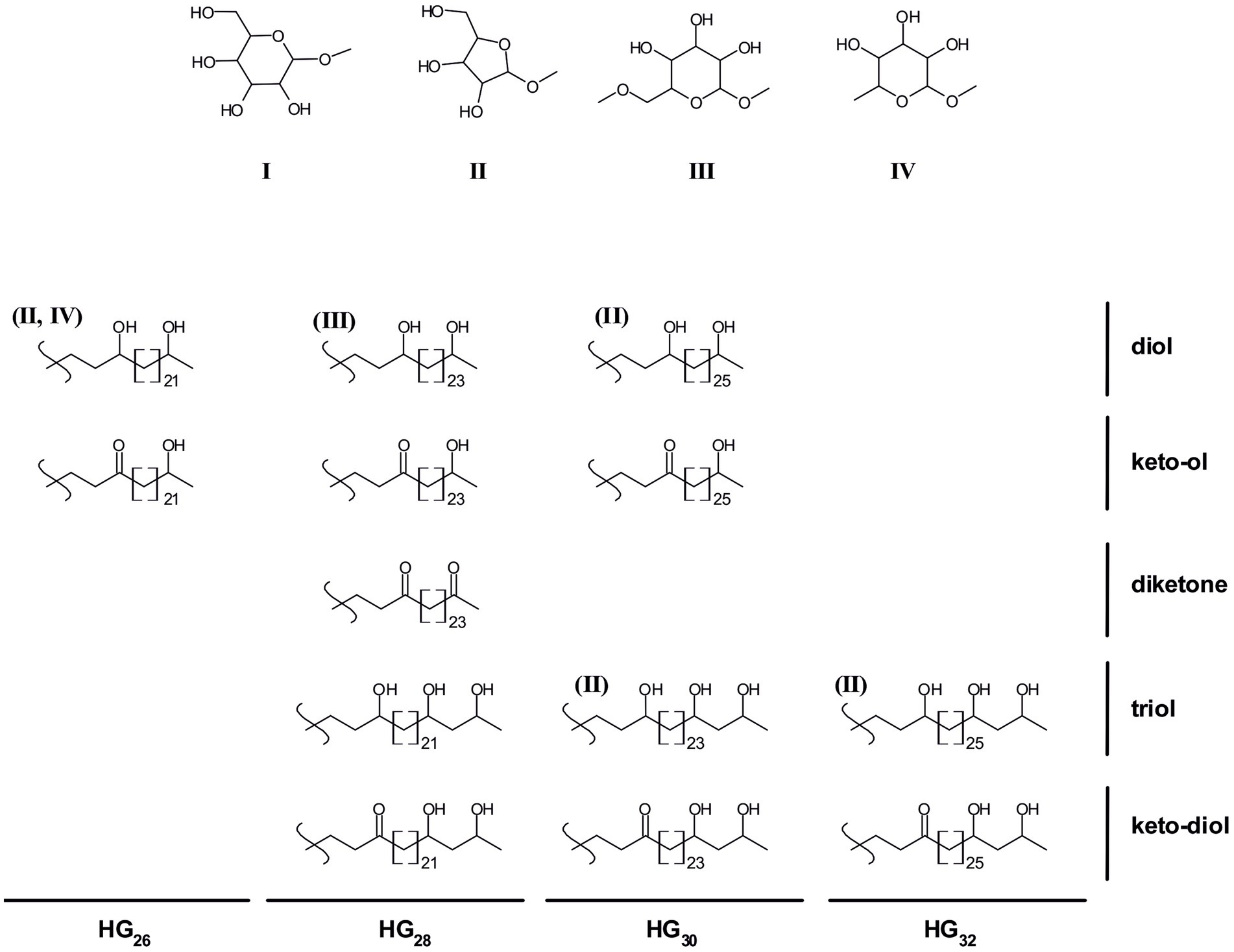
Figure 1. Chemical structures of heterocyte glycolipids found in cyanobacteria. I-IV depict the potential HG headgroups: hexose (I), pentose (II), methyl hexose (III), deoxyhexose (IV). All depicted HG structures usually contain a hexose headgroup (I), some HGs have also been observed containing alternative headgroups (shown in brackets above the HG structure).
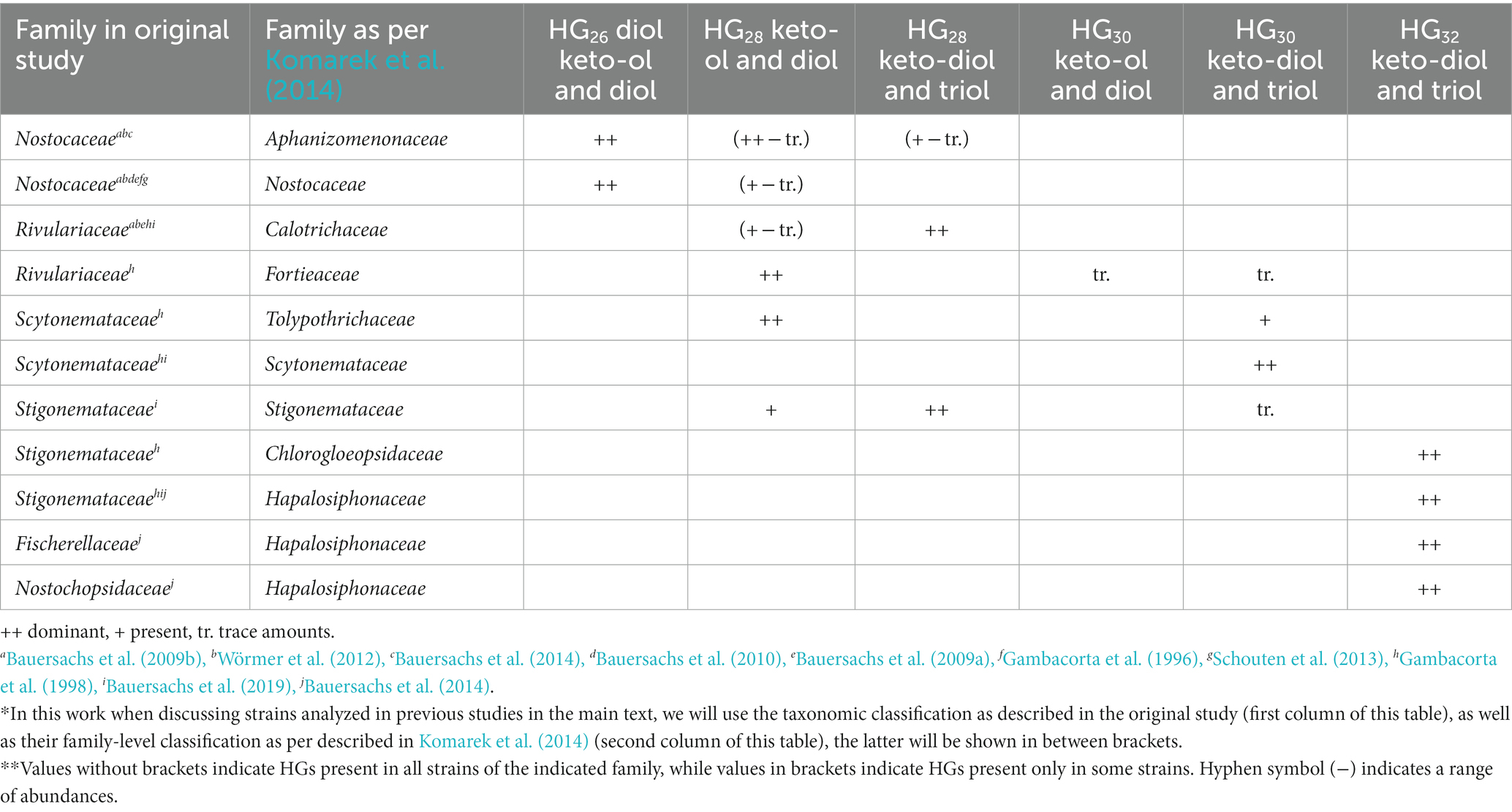
Table 1. Summary of HGs produced by different cyanobacterial families according to the classification described in Komarek et al. (2014) and to the nomenclature in the original studies.*,**
Additionally, the type of headgroup of HGs (Figure 1) can also be an indicator of the lifestyle and habitat of the producing heterocytous cyanobacterial strain. Most HG-producing cyanobacteria biosynthesize HGs with a hexose (C6) as headgroup, however some cyanobacterial species also produce HGs with alternative headgroups such as pentoses (C5), deoxyhexoses (C6) or methyl-hexoses (C6) (Schouten et al., 2013; Bale et al., 2018a; Figure 1; Supplementary Table S1). Most of the heterocytous cyanobacterial strains studied to date that synthesize HGs with a hexose headgroup are free-living and inhabit freshwater and terrestrial environments, with a few exceptions, i.e., Nostoc muscorum UTEX 1933 and Calothrix sp. UTEX 2589 synthesize HG26 and HG28 with hexose as headgroup but were enriched from marine environments (Schouten et al., 2013). In contrast, heterocytous cyanobacteria that are symbionts of marine diatoms, synthesize HG30 and HG32 HGs containing a pentose headgroup, and were, consequently, proposed as biomarkers for tracing endosymbiotic heterocytous cyanobacteria in present and past marine environments (Schouten et al., 2013; Bale et al., 2018b).
Due to their potential preservation over geological time scales, HGs have also been used as biomarkers to study the occurrence of nitrogen fixation in ancient sediments (Bauersachs et al., 2010). In addition, changes in the abundances of HG26 diols and keto-ols can be used to reconstruct surface water temperatures in freshwater environments using the HDI26 index (Bauersachs et al., 2015). However, our current understanding of HG production among heterocytous cyanobacteria is restricted, because only a limited amount of HG-producing cyanobacterial cultures has been examined. A solution would be to study the heterocytous cyanobacterial community occurring in the environment using both approaches, i.e., HG and genetic analyses. One could use untargeted genetic approaches such as 16S rRNA and nifH gene analysis using primers that specially target cyanobacteria. However, these approaches might not be specific enough, since they will target all cyanobacteria and N2 fixing cyanobacteria (among other N2 fixing organisms; Olson et al., 1998). Hence, if the species of interest are sparse, there is the risk of overseeing them and detecting only the more abundant organisms. Therefore, ideally, the genetic analysis should target genes exclusive to heterocytous cyanobacteria such as those involved in HG biosynthesis (Figure 2).
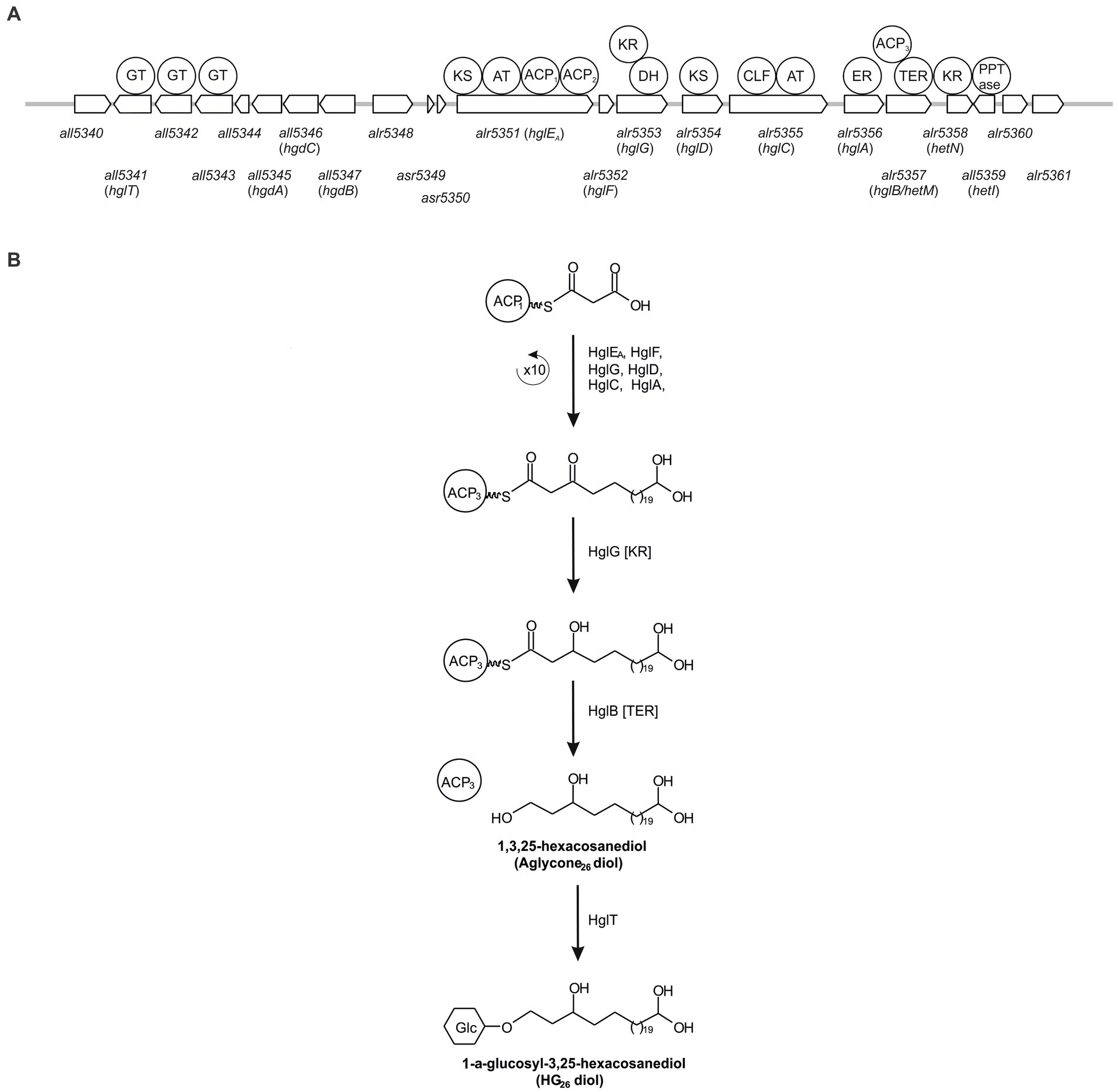
Figure 2. Schematic representation of the genes involved in HG biosynthesis in Anabaena sp. PCC 7120 (A) and simplified representation of the biosynthesis pathway of HG26 diols (B). ACP, acyl carrier protein; AT, acyl transferase; CLF, chain length factor; DH, dehydrase; ER, enoyl reductase; GT, Glycosyltransferase; KR, ketoacyl reductase; KS, β-ketoacyl synthase; TER, thioester reductase.
In this study, we developed a specific PCR-based molecular approach designed to specifically target heterocytous cyanobacteria. To this end, we developed primers targeting all5341 (also known as hglT), a gene essential for HG biosynthesis but not essential for survival, encoding the glycotransferase involved in the last step of the synthesis of HGs in Anabaena sp. PCC 7120 (Awai and Wolk, 2007; Halimatul et al., 2014) using genomes available from public databases. We validated them using 28 freshwater cyanobacterial cultures, optimized the PCR conditions, and tested them in the environment.
Materials and methods
Nucleic acids extraction
Cyanobacterial cultures
Cyanobacterial biomass from strains listed in Table 2 was obtained from frozen (−80°C) pellets stored in the former Culture Collection Yerseke (CCY) and starter cultures provided by the Pasteur Culture Collection (PCC). Except Chysosporum ovalisporum strains UAM290 and UAM292, kindly provided by Dr. Samuel Cirés and Anabaena sp. PCC 7120, kindly provided by Dr. Koichiro Awai. Genomic DNA was extracted using the DNeasy PowerSoil kit (Qiagen, Hilden, Germany) according to the manufacturer’s instructions with minor modifications, namely: samples were disrupted using a mixture of 0.2 mL 0.5 mm diameter and 0.2 mL 0.1 mm diameter glass beads (BioSpec Products, Bartlesville, OK, USA) on the bead mill homogenizer (Bead Ruptor Elite, Omni International, Kennesaw, GA, USA) running twice for 10 s at a speed of 3.55 m s−1 with a 30 s dwell. Concentration of DNA extracts was analyzed using a Nanodrop ND-1000 Spectrophotometer (NanoDrop Technologies Inc. Wilmington, DE, USA).
Environmental samples
DNA from a microbial mat collected from Schiermonnikoog station 3 (29′ 28.3626″, 6° 8′ 20.9646″) on 10th April (Fan et al., 2015), was extracted from freeze dried homogenized sample using the same extraction kit and sample homogenization protocol as described above for cyanobacterial cultures. A DNA extract of the seaweed Sargassum fluitans was kindly provided by Tom Theirlynck and Linda Amaral-Zettler. The S. fluitans sample was collected from the Central Atlantic Ocean (station 24: 8° 26′ 6.1548″, −49° 44′ 27.2142″) during cruise 64PE455 on board of the R/V Pelagia during July–August 2019 as described in Theirlynck et al. (2023). DNA extraction of S. fluitans sample number 38 is described in Theirlynck et al. (2023).
Lipid extraction and analysis
Lipids present in the microbial mat sample were extracted from freeze dried aliquots using a modified Bligh-Dyer procedure as described by Bauersachs et al. (2011 and references within). The extracted intact polar lipids were analyzed by high performance liquid chromatography coupled to electrospray ionization tandem mass spectrometry (HPLC/ESI-MS2) as described in Bauersachs et al. (2009b).
Polymerase chain reaction (PCR) and sequencing
Validation of the DNA extracted from cyanobacterial cultures
To ensure that the DNA extracted from cyanobacterial cultures was of good quality and suitable for our study, we performed a PCR using universal primers 515F-Y and 806RB targeting the 16S rRNA gene (Supplementary Table S2; Walters et al., 2015), resulting in the expected ~300 bp band. All PCR reaction mixes and cycles used in this study were as described in Besseling et al. (2018) with minor modifications, i.e., PCR annealing temperature was 50°C, extension time was 30″ and it was repeated for 30 cycles (hereafter these specifications will be shown within parenthesis in the main text).
Amplification and sequencing of hglT
Upon optimisation of the PCR conditions (see Results), amplification of hglT using the primers designed in this study was carried out using an annealing temperature 56.2°C, 1′20″ extension time, for 30 cycles, and an equimolar mixture of primers, for example Rv1 refers to an equimolar mixture of Rv1_G1, Rv1_G2, Rv1_G3, Rv1_G4 and Rv1_G5.
Upon amplification of hglT with primer mixes Fw1 mixB and Rv1, the presence of a single or multiple PCR products was analyzed via electrophoresis on an agarose gel (1%) stained with ethidium bromide or GelRed® (Biotium, Hayward, CA, USA), using SmartLadder (Eurogentec, Seraing, Belgium) as molecular weight marker. If only one band was observed the PCR product was purified using a PCR purification kit (QIAquick PCR Purification kit, Qiagen, Hilden, Germany). If more than one band was observed, then the PCR product was run on 1% or 1.5% agarose gels stained with SYBR Safe® (ThermoFisher Scientific, USA), bands were then excised and purified using the QIAquick gel extraction kit (Qiagen, Hilden, Germany). To confirm the purity of the purified bands a small aliquot was run again on an agarose gel (1%) as described above. If the amount of the PCR product was not sufficient for direct sequencing, the amount was increased via concentration of the product of several PCR reactions using a PCR purification kit (QIAquick PCR Purification kit, Qiagen, Hilden, Germany). Purified PCR products were sequenced via Sanger sequencing at Macrogen Europe (Amsterdam, The Netherlands) using primer mixes Fw1 mixB and Rv1.
If after concentration the amount of PCR product was still not sufficient for direct sequencing, it was then cloned into the pCR™4-TOPO™ vector (Invitrogen, Carlsbad, CA, USA) using the TOPO-TA cloning kit for sequencing (Invitrogen, Carlsbad, CA, USA) according to the manufacturer’s instructions. The ligation product was subsequently transformed into TOP10 competent cells (Invitrogen, Carlsbad, CA, USA) and plated in LB plates containing ampicillin (100 μg/mL), kanamycin (20 μg/mL), IPTG (0.1 mM) (UltraPure™ IPTG, Invitrogen Europe, manufactured in Italy) and X-Gal (0,2 mg/mL) (Thermo Scientific, Vilnius, Lithuania). The resulting white colonies were collected using a sterile toothpick, dissolved in 50 μL TE 1x buffer and lysed by incubation at 95°C for 10 min. Upon centrifugation at 2000 rpm for 1 min, 1 μL supernatant was used as template in 25 μL PCR reactions using M13 primers (Supplementary Table S2) and the PCR reaction mix and amplification program described above. PCR products were sent to Macrogen Europe for PCR purification and Sanger sequencing using primers T3 and T7 (Supplementary Table S2).
Amplification and sequencing of 16S rRNA
16S rRNA V4-V5 regions from DNA present in the microbial mat sample was amplified using barcoded primers 515F-Y and 926Rbc (Parada et al., 2016) via PCR reaction using Phusion Taq Polymerase (Thermo Fischer Scientific). And the following PCR program: 30″ at 98°C, 30 cycles of 10″ at 98°C, 20″ at 50°C, 30″ at 72°C; final extension at 72°C for 7′ and storage at 4°C. Samples were amplified in triplicate and pooled together after positive confirmation via electrophoresis on an agarose gel (2%). Positive controls (E. coli lysate) and negative controls (PCR water without DNA) were included. Concentration of each PCR product was quantified on an agarose gel (2%) using a quantification standard. PCR products from all samples were pooled in equimolar concentrations, ran on an agarose gel (2%) and the resulting ~400 bp band was cut off the gel and purified using QIAquick gel extraction kit (Qiagen, Hilden, Germany) according to the manufacturer’s instructions. Sequencing was performed by Macrogen Europe (Amsterdam, The Netherlands) using an Illumina MiSeq 2 × 300 bp V3 kit (Illumina, CA, USA). Amplification and analysis of 16S rRNA gene of the S. fluitans sample is described in Theirlynck et al. (2023).
Bioinformatic analysis
Search of HglT homologs and primer design
Homologs of the protein HglT of Anabaena sp. PCC 7120, encoded by gene all5351 (IMG gene ID 637235753 hereafter, hglT) were found via BLASTx (translated nucleotide sequence searched against protein sequences, accessed on May 2020) (Altschul et al., 1990; Camacho et al., 2009) against 23 genomes (Supplementary Table S3 and Supplementary Figure S1). The resulting gene sequences (Supplementary Table S4) were aligned in MEGA (v11.0.8) using the in-built MUSCLE (Edgar, 2004) sequence aligner with default parameters [gap open penalties: −400, gap extend penalties: 0, max iterations: 16, Cluster Method: UPGMA, Min Diag Length (Lambda): 24]. To select suitable regions for the design of our primers we used the following guidelines: (1) Binding region should be 17–22 base pairs (bp) long; (2) Binding region Tm’s should be 50–65°C; (3) Binding region should have moderate GC content (<50%); (4) Repetitive poly-N regions should be avoided; (5) Regions prone to secondary structure formation (hairpins, self-dimerization or primer dimer), such as regions containing complementary sequences (palindromes) within themselves should be avoided; (6) Primer pairs should have similar Tm’s (±4°C); and (7) Distance between the two binding regions (Fw and Rv) should be as large as possible. The regions comprised between the base pairs 112..131 and 299..320, were selected as suitable regions for the design of forward primers, and regions 949..968 and 973..989 were selected as targets for the design of reverse primers.
Primers were designed according to the general primer design guidelines described by Abd-Elsalam (2003), which we applied by setting the following rules: (1) length of the primer should be between 17 and 22 bp (2) degenerate nucleotides should not be consecutive (3) degenerate nucleotides should be as far apart from each other as possible (4) each primer sequence should have the least number of degenerate nucleotides possible but no more than four. Primer properties such as melting temperature (Tm) and percentage of GC content were also taken into consideration, aiming to design primers with similar Tm and moderate GC content (<50%). The potential of each primer to create hairpins and primer dimers due to potential self-annealing was also checked by using OligoCalc (Kibbe, 2007; see Supplementary Table S5). All primers were synthesized by Integrated DNA Technologies (IDT, Leuven, Belgium).
Analysis of hglT amplicons
Nucleotide sequences obtained via Sanger sequencing were processed using Geneious Prime (v 2023.0.4). Sequences were trimmed using an error probability limit of 0.01. Potential heterozygous bases in single reads were identified using a 50% peak similarity cutoff, peak detection height was set at 10%. When available, the consensus sequence was obtained by aligning forward and reverse reads with Geneious assembler using the highest sensitivity settings. When appropriate, sequences belonging to the pCR™4-TOPO™ vector (Invitrogen, Carlsbad, CA, USA) were identified and removed.
To determine their origin, the resulting sequences were queried using Geneious built-in blastN (BLAST+ v 2.12.0, accessed in March 2023) (Altschul et al., 1990; Camacho et al., 2009) with default parameters (low complexity filter, mask for lookup table, max e-value: 0.05, word size: 11, open gap cost: 5, extend gap cost: 2, scoring match: 2, scoring mismatch: 2–3).
To generate the phylogenetic trees sequences were aligned using MAFFT (v7.407) with L-INS-i iterative refinement method (Katoh and Standley, 2013) and poorly aligned regions were removed using trimAl (Capella-Gutiérrez et al., 2009). Phylogenetic trees were built using IQ-tree (v1.6.7) and its in-built nucleotide substitution model finder (Kalyaanamoorthy et al., 2017), using 1,000 replicates to perform SH-like approximate likelihood ratio test (SH-aLRT) (Guindon et al., 2010) and 1,000 bootstrap replicates. Phylogenetic trees were visualized using iTOL (v6.7.4) (Letunic and Bork, 2021).
Analysis of 16S rRNA amplicons
The resulting amplicon sequencing data was processed using the Cascabel pipeline (Abdala Asbun et al., 2020). Within the Cascabel pipeline raw reads were filtered, trimmed and ASVs identified using the dada2 R package (v 1.19.1) (Callahan et al., 2016). Taxonomy was assigned using RDP (Wang et al., 2007) classifier within dada2, using SILVA SSU database 138 Ref NR 99 (Quast et al., 2013) and setting the minimum bootstrapping support required to return a taxonomic classification to 45% (minBoot = 45). Resulting taxa with a minimum count of two observations were summarized using QIIME (using summarize_taxa.py v 1.9.1 and filter_otus_from_otu_table.py v 1.9.1) (Caporaso et al., 2010) and BIOM tables were converted using BIOM tool (v 2.1.6) (McDonald et al., 2012). Processing and analysis of 16S rRNA amplicon sequencing data of the S. fluitans sample is described in Theirlynck et al. (2023).
Results and discussion
Assessing the potential of hglT as phylogenetic marker gene
To assess the phylogenetic potential of hglT, we generated a phylogenetic tree using complete hglT sequences from genomes available on the Integrated Microbial Genomes & Microbiomes system (IMG/M1) (Chen et al., 2021). We searched for homologs of the protein HglT of Anabaena sp. PCC 7120, encoded by gene all5351 (IMG gene ID 637235753 hereafter, hglT) against 23 genomes (Supplementary Table S3) using BLASTx (translated nucleotide sequence searched against protein sequences), accessed on May 2020 (Altschul et al., 1990; Camacho et al., 2009). These included the genomes of 11 strains known to produce HGs, and 12 genomes of strains closely related to strains known to produce HGs. For example, Chrysosporum ovalisporum UAM 290 has been described to synthesize HGs (Wörmer et al., 2012) but there is no genome available for this strain, so we chose the genome of Chrysosporum ovalisporum UAM-MAO. In the BLASTx search, we selected for each genome, the protein sequence with the highest percentage of homology (average 75%; n = 23) and highest bit-score (average 421, n = 23) (Supplementary Table S4). These sequences were used to build the hglT phylogenetic tree (Figure 3A).
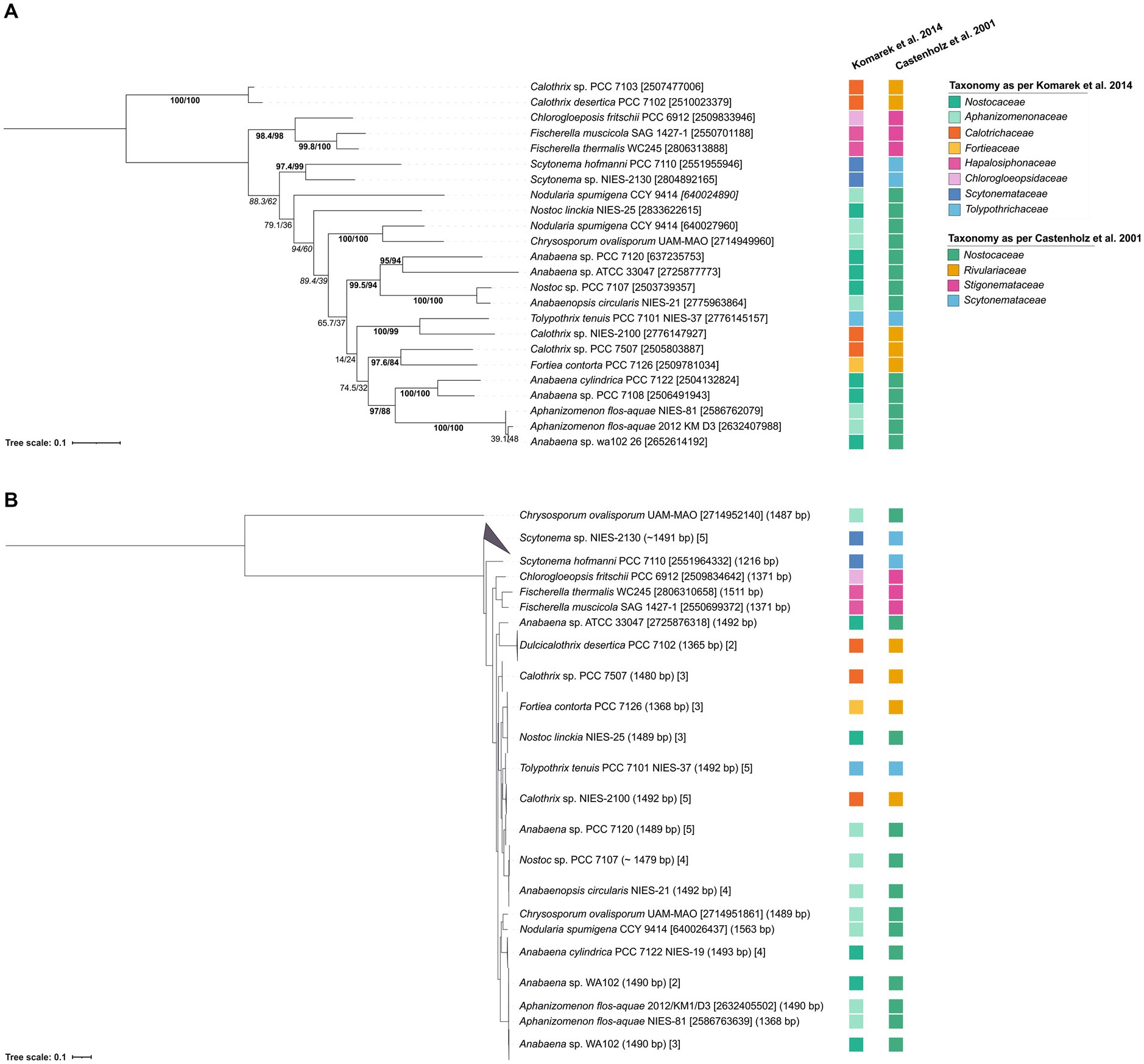
Figure 3. Tree containing (A) hglT gene sequences and (B) 16S rRNA sequences from selected heterocytous cyanobacteria (Supplementary Table S6). (A) hglT sequences (gene number shown in between brackets) (Supplementary Table S4) were obtained from selected genomes of heterocytous cyanobacteria (Supplementary Table S3). For each genome, we chose the sequence with the highest percentage of homology and highest bit-score (Supplementary Table S4). For the Nodularia spumigena CCY 9414 genome, two sequences with similar bit-score (439 and 420) and homologies of 78 and 64%, were included. (B) 16S rRNA gene sequences were obtained from IMG (Supplementary Table S6), numbers between parentheses indicate the gene length, while numbers between brackets indicate either the gene id (10 digit numbers) or the number of gene sequences included in the branch. In tree (A) values on branches indicate the SH-aLRT (left side of the slash) and the standard non-parametric bootstrap supports (right side of the slash). High SH-alrt and bootstrap supports (≥85 and ≥ 70, respectively) (Hillis and Bull, 1993; Anisimova et al., 2011) are shown in bold, when only SH-alrt support is high, values are shown in italics. Bootstrap values of tree (B) are shown in Supplementary Figure 2. Scale bar represents the mean number of substitutions per site.
To ensure that the hglT gene tree provides enough resolution, we compared it to a 16S rRNA tree generated using sequences obtained from the same (or closely related) genomes as hglT (Figure 3B; Supplementary Figure S2; Supplementary Table S6). Cyanobacteria can possess multiple copies of the 16S rRNA gene, oftentimes also of different sequence length. Thus, to avoid potential misalignments caused by large differences in sequence length we only included sequences which were at least 1,000 bp long. Figure 3B reveals that, in most cases, the 16S rRNA sequences of the examined cyanobacteria cluster together, with a few exceptions, such as the two sequences of Crysosporum ovalisporum UAM-MAO.
The results from the hglT and 16S rRNA tree do not always show the same evolutionary history for a given genome. However, both trees agree with observations of previous studies, such as the polyphyletic origin of nearly all cyanobacterial families present in the trees, confirming previous reports in literature about the polyphyletic origin of Nostoc, Anabaena, Calothrix, Tolypothrix and Scytonema (Komárek et al., 2013; Komarek et al., 2014; Berrendero Gómez et al., 2016 and references cited therein), and the monophyletic origin of the Stigonemataceae family (Chlorogloeopsidaceae and Hapalosiphonaceae) (Castenholz et al., 2001 and references within). In general, it can be concluded that the hglT tree provides enough resolution to use this gene as a marker for HG-producing cyanobacteria.
Primer design and validation in pure cultures
To design primers targeting hglT, we used all sequences used to generate the hglT tree (Figure 4; Supplementary Table S4), aligned them in MEGA (v 11.0.8) using the in-built MUSCLE (Edgar, 2004) sequence aligner with default parameters, and manually identified regions suitable as primer targets (see Supplementary Figure S1 for details).
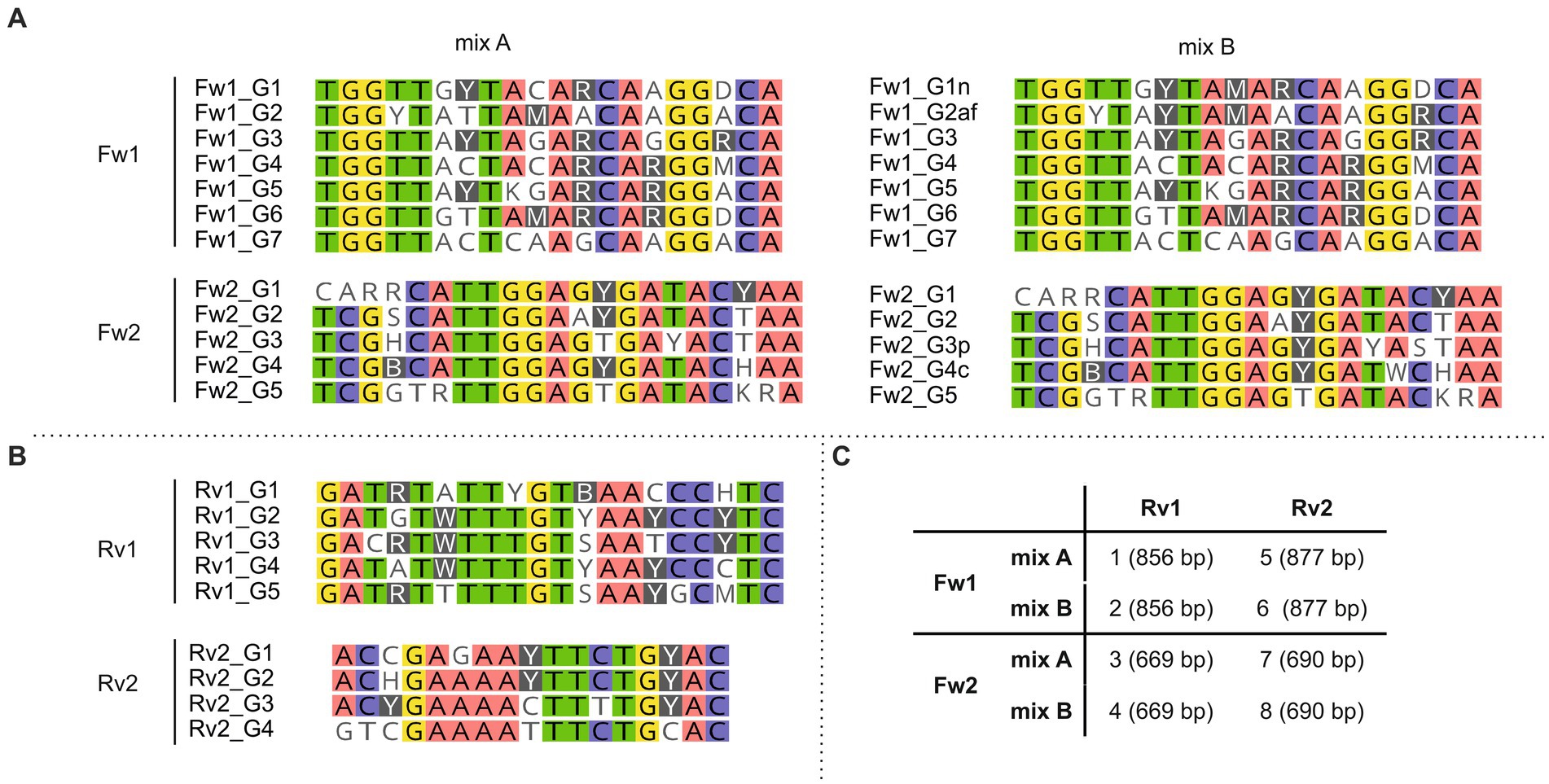
Figure 4. Primer sequences included in each forward (A) and reverse (B) primer mix. (C) Primer mixes included in primer sets 1–8 and in brackets the expected size of the resulting PCR products. Background colors show bases conserved (threshold: 75%) across the primer sequences. Primers were designed to target the most conserved gene regions.
To be able to target the largest number of cyanobacterial species, while still following the rules considered for the design of the degenerate primers (i.e., only a maximum of four degenerate nucleotides should be included; see Material and Methods for details), we decided to design several primers targeting the same binding region but containing different combinations of degenerate bases (Figure 4; Supplementary Tables S2, S5), and use them in equimolar amounts in the PCR reaction. For some primers (Fw1_G1, Fw1_G2, Fw2_G3 and Fw2_G4), we also designed an alternative variant (Fw1_G1n, Fw1_G2af, Fw2_G3p and Fw2_G4c, respectively) (Supplementary Table S5) containing one additional degenerate nucleotide that would allow to increase the number of targeted strains (Supplementary Table S7). For example, Fw1_G1n contains 4 degenerate bases and targets 8 of the 24 strains, while Fw1_G1 contains 3 degenerate bases and targets only 6 strains. Both primer variants were tested in parallel and in combination with primers targeting the same region for which only one variant was designed (i.e., Fw1_G3 to Fw1_G7). For example, primer variants with the least number of degenerations (i.e., Fw1_G1 and Fw1_G2) were tested in combination with Fw1_G3 to Fw1_G7 (hereafter referred to as “mix A”), and primer variants with the greatest number of degenerations (i.e., Fw1_G1n and Fw1_G2af) were also tested in combination with Fw1_G3 to Fw1_G7 (hereafter referred to as “mix B”) (Figure 4).
The designed primers were tested using DNA extracted from 21 heterocyte-forming cyanobacterial strains (Table 2; Supplementary Table S8). Next, in order to develop the best suited primer set and its optimal annealing temperature, we performed a PCR with an annealing temperature gradient (52–63°C annealing temperature, 1′20″ extension time, 30 cycles) on two selected strains (Calothrix CCY 9923 and Chlorogloeopsis sp. CCY 9924) using all possible primer combinations (Supplementary Figure S3). All primer combinations successfully targeted the selected strains, and primer combinations containing a larger number of degenerate bases (mix B) did not show more unspecific bands than those containing the more conservative primers (mix A) (Supplementary Figure S3). Consequently, we decided to only use primer mix B to be able to target the largest number of cyanobacterial strains, and thus reduce the total number of primer combinations from eight to four. For strain Calothrix CCY 9923 primer combinations containing Fw1 primers resulted in an additional smaller (~400 bp) and unexpected band in the agarose gel when using an annealing temperature of 56.2°C or lower (Supplementary Figure S3). However, in some cases using an annealing temperature higher than 56.2°C resulted in much lower concentrations of PCR product, as was observed, for example, when primers Fw1 mixB and Rv2 and an annealing temperature of 58.2°C were used on Chlorogloeopsis sp. CCY 9924 (Supplementary Figure S3). Hence, despite the appearance of the unspecific band, we chose in favor of higher PCR product concentrations and chose to use an annealing temperature of 56.2°C in all subsequent PCR reactions. Next, we checked which of the four remaining primer pairs could amplify the hglT gene from the largest number of strains (Supplementary Figure S4). Primer pair 2 (Fw1 mix B + Rv1) successfully generated a PCR product of the expected size (~850 bp) for most of the tested strains (Supplementary Figure S4) and was selected as the best primer pair to potentially detect C6 HG producers in the environment.
To check the specificity of this primer pair (Fw1 mix B + Rv1) we also tested it in non-heterocyte forming cyanobacterial strains (Table 2; Supplementary Table S8). In four out of seven of these strains (Synechococcus strains CCY 0011, CCY 9201, CCY 9503 and CCY 9506) PCR resulted in the production of two very faint bands (~1,000 bp and ~ 600 bp) (Supplementary Figure S5), which upon cloning and sequencing showed some homology to sequences present in Synechococcus and Cyanobium, indicating that the primers are not fully specific for heterocytous cyanobacteria. Nonetheless, this unspecific and inefficient amplification using the designed primers can be discerned by the size of the PCR product and ultimately by sequencing the amplified products (Supplementary Tables S9, S10).
To check if the selected primer pair (Fw1 mix B + Rv1) actually amplified hglT, the most abundant PCR fragments obtained from the tested cyanobacterial cultures (shown as ++ in Supplementary Figure S4) were purified and sequenced. Initially, to ensure that all the PCR fragments obtained corresponded to heterocytous cyanobacteria, we carried out an NCBI blastN search and confirmed that all analyzed PCR fragments corresponded to HG-producing cyanobacterial strains (Supplementary Table S9). However, the genera (or species) of the hit with the highest bit-score did not always correspond to genera assigned to the tested (query) strain.
Next, we wanted to see if the amplified fragments corresponding to hglT could be used as phylogenetic marker and assist during strain identification and naming. To do so we generated a phylogenetic tree containing the PCR fragments (700–870 bp) obtained from the cultures analyzed in this study and PCR fragments generated in silico (Figure 5). In silico PCR fragments were obtained from the hglT sequences initially used to design the primers (Supplementary Table S4) by selecting the DNA fragment of hglT contained between primers Fw1 mixB and Rv1. This tree (Figure 5) shows that the targeted hglT fragment has potential as a phylogenetic marker up to the genus level. We observe that the sequences obtained using our primers, in most cases, cluster with hglT fragments of strains of the same genera (Figure 5). For example, Calothrix CCY 9923 clusters with Calothrix sp. PCC 7103 and Calothrix desertica PCC 7102 (Clade 1), confirming its affiliation to the genus Calothrix, while Scytonema sp. PCC 10023 clusters with Scytonema sp. NIES-2130 and Scytonema hofmanmi PCC 7110 (Clade 4), also confirming its affiliation to the genera Scytonema. Clade 12 contains closely related sequences that are identified with different names and strain identifiers, including Anabaena CCY 9910, Anabaena cylindrica CCY 9921 and three Anabaena/Nostoc sp. PCC 7120. The latter is also shown in the tree as Anabaena sp. CCY 9626, which refers to strain PCC 7120 after being kept in the former Culture Collection Yerseke (CCY). Strain PCC 7120 has been renamed several times, underscoring the difficulties faced by taxonomists during cyanobacterial classification. It was known first as Nostoc muscorum, it was then classified as Anabaena (Rippka et al., 1979) and later renamed as Nostoc although this reassignment has been questioned by some studies (Svenning et al., 2005 and references within).
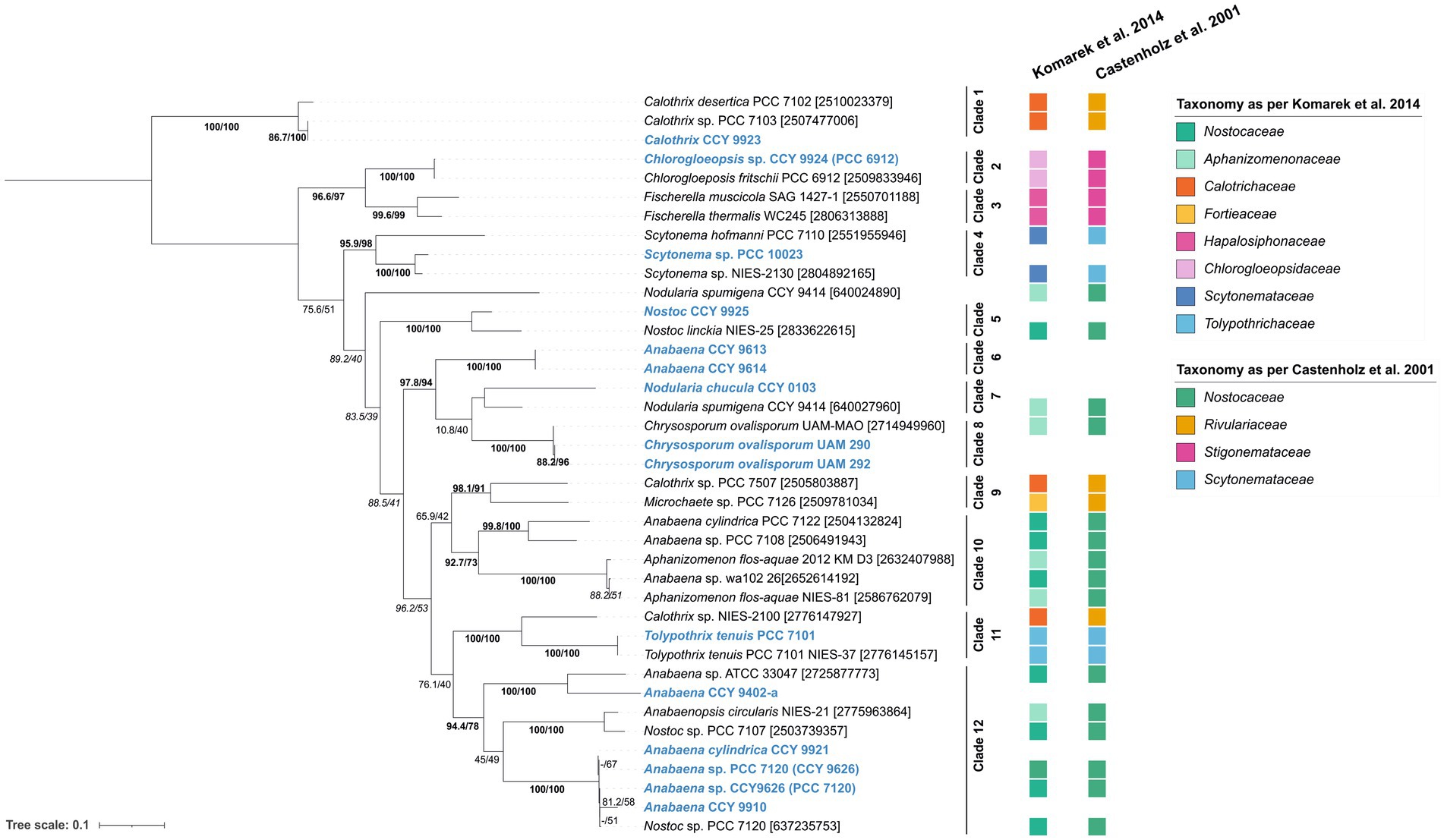
Figure 5. Tree containing the hglT gene fragments obtained via PCR and direct sequencing using primer set 2 (Fw1 mix B + Rv1) on the heterocytous cyanobacterial cultures tested in this study (sequences in blue) and in-silico PCR fragments obtained from the genomes used during primer design (Table 1). Tree was generated using substitution model TPM3 + F + I + G4 on IQ-tree (v1.6.7). Values on branches indicate the SH-aLRT (right side of the slash) and the standard non-parametric bootstrap supports (left side of the slash). High SH-alrt and bootstrap supports (≥85 and ≥ 70, respectively) (Hillis and Bull, 1993; Anisimova et al., 2011) are shown in bold, when only SH-alrt support is high, values are shown in italics. Gene ID is indicated between brackets. Alternative strain numbers are indicated in between parentheses. Scale bar represents the mean number of substitutions per site.
Overall, these results show that hglT-primer set 2 is sensitive enough to detect the gene of the glycosyltransferase involved in HG biosynthesis in heterocyte-forming cyanobacteria and that upon sequencing the resulting hglT fragments can be used as taxonomic marker.
Application of the developed genetic approach to the environment
After confirmation that the primer set 2 (Fw mix B and Rv1) successfully targeted hglT in heterocytous cyanobacterial cultures, it was tested in the environment using a microbial mat collected from the Dutch Wadden island of Schiermonnikoog. The lipid and microbial composition of microbial mats from this location had been subject of several studies in the past and were shown to contain cyanobacterial communities and heterocyte glycolipids, suggesting the presence of heterocytous cyanobacteria (Severin and Stal, 2008; Severin et al., 2010; Bauersachs et al., 2011; Fan et al., 2015).
Lipid analysis of the microbial mat confirmed the presence of heterocyte glycolipids, albeit at very low concentrations. We detected presence of 1-(O-hexose)-3,25-hexacosanediol (HG26 diol), 1-(O-hexose)-3-keto-25-hexacosanol (HG26 keto-ol), and 1-(O-hexose)-27-keto-3,25-octacosanediol (HG28 keto-diol), and also small amounts of 1-(O-hexose)-3,27-octacosanediol (HG28 diol) and 1-(O-hexose)-3,25,27-octacosanetriol (HG28 triol) (Table 3). Detection of HG26 keto-ol and diol suggest the presence of cyanobacterial members of the Nostocaceae family (Nostocaceae and Aphanizomenonaceae), and presence of HG28 keto-diol and triol suggested the presence of members of the Rivulariaceae (Calotrichaceae) family (Table 1; Supplementary Table S1). While traces of HG28 diol could also indicate the presence of organisms belonging to other cyanobacterial families such as Fortiea contorta PCC 7126 (formerly known as Microchaete sp.), Stigonema ocellatum SAG 48.90 or Tolypothrix tenuis PCC 7101. (Supplementary Table S1).
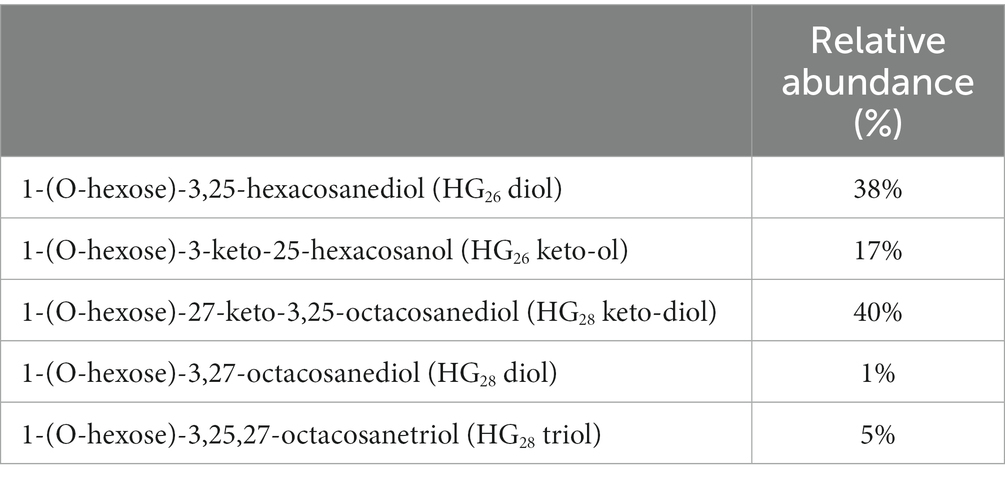
Table 3. Heterocyte glycolipids present in the microbial mat collected from Schiermonnikoog (53°29.445′N, 6°8.342′E) in April 2010.
These HG results agree with the study of Bauersachs et al. (2011). These authors reported the presence of 1-(O-hexose)3,25-hexacosanediol (HG26 diol), suggesting the presence of members of the Nostocaceae family (Nostocaceae and Aphanizomenonaceae), in supratidal microbial mats collected near our sampling site. Additionally, Bauersachs et al. (2011) also detected the presence of Calothrix sp. and Nodularia sp. (Aphanizomenaceae) in nearby sampling sites using 16S rRNA gene libraries, although HGs generally associated with Calothrix were not detected.
Next, we wanted to detect potential HG-producing heterocytous cyanobacteria in the microbial mat using our newly developed hglT primers. DNA from the freeze-dried microbial mat was extracted as described previously for the cyanobacterial cultures (Table 2). We carried out a PCR reaction on the microbial mat DNA extract using primer pair 2 (Fw1 mixB + Rv2) and the same annealing temperature that was selected for PCR on DNA extracts of cyanobacterial cultures (56°C annealing temperature, 1′ 30″ extension time, 30 cycles). Unfortunately, however, no PCR products were obtained from the microbial mat (Supplementary Figure S6). We carried out additional tests to rule out the presence of inhibitors that could seriously hinder the reaction, and to ensure that the selected sample contained DNA belonging to cyanobacteria and nitrogen fixing bacteria (See Supplementary Results and Supplementary Figures S7–S9).
We performed a gradient PCR (48–58°C annealing temperature, 1′10″ extension time, 37 cycles) on our microbial mat sample using the hglT-primer mix 2 (Fw1 mixB and Rv1) and increased the number of amplification cycles to try to obtain a PCR product. However, no clear band was obtained at any of the annealing temperatures tested (Supplementary Figure S10). Previous genetic studies showed that microbial mats collected from this location contained diazotrophic cyanobacteria, and lipid analysis indicated that heterocytous cyanobacteria were present in the microbial mat (Severin et al., 2010; Bauersachs et al., 2011). However, our primers designed for the amplification of hglT gene fragments of heterocyte cyanobacteria did not yield any band when applied on the DNA extract of the microbial mat. This can be explained in two ways: (1) no heterocytous cyanobacteria were present in the microbial mat or they were present in very low abundances (2) our primers cannot target the heterocytous cyanobacterial species present in this microbial mat.
To answer this question, we carried out 16S rRNA gene amplicon sequencing analysis. Sequencing results showed that nearly half of the reads (54%) corresponded to Amplicon Sequence Variants (ASVs) (Table 4) of cyanobacteria. Coleofasciculus chthonoplastes PCC 7420, a non-heterocytous diazotroph, was the most relatively abundant (ra) cyanobacteria (43.7% ra). Other cyanobacterial species such as the nitrogen fixers Lyngbya aestuarii PCC-7419 (2.8% ra) and Trichodesmium IMS101 (0.28% ra) were also detected but no heterocytous cyanobacteria were detected (Table 4). Hence, we conclude that the amplification of hglT using our primers failed simply because heterocytous cyanobacteria were absent or present in very low abundances. Since the use of PCR-based approaches (including the use of universal 16S rRNA primers) typically favors amplification of more abundant microbial species, potentially masking the presence of those present in lower proportion, it is possible that the heterocytous cyanobacteria were present in the microbial mat but in too low abundances to be detected by our PCR methods. Alternatively, given the excellent preservation of heterocyte glycolipids over time (Schaefer et al., 2020), and the relatively short life of DNA when released in the environment after cell death, it is also plausible that the heterocyte glycolipids detected in the microbial mat were preserved remnants of dead heterocytous cyanobacteria whose DNA had been degraded. Therefore, both explanations would be consistent with the very low levels of HG lipids detected in the microbial mat and the absence of DNA from heterocytous cyanobacteria.
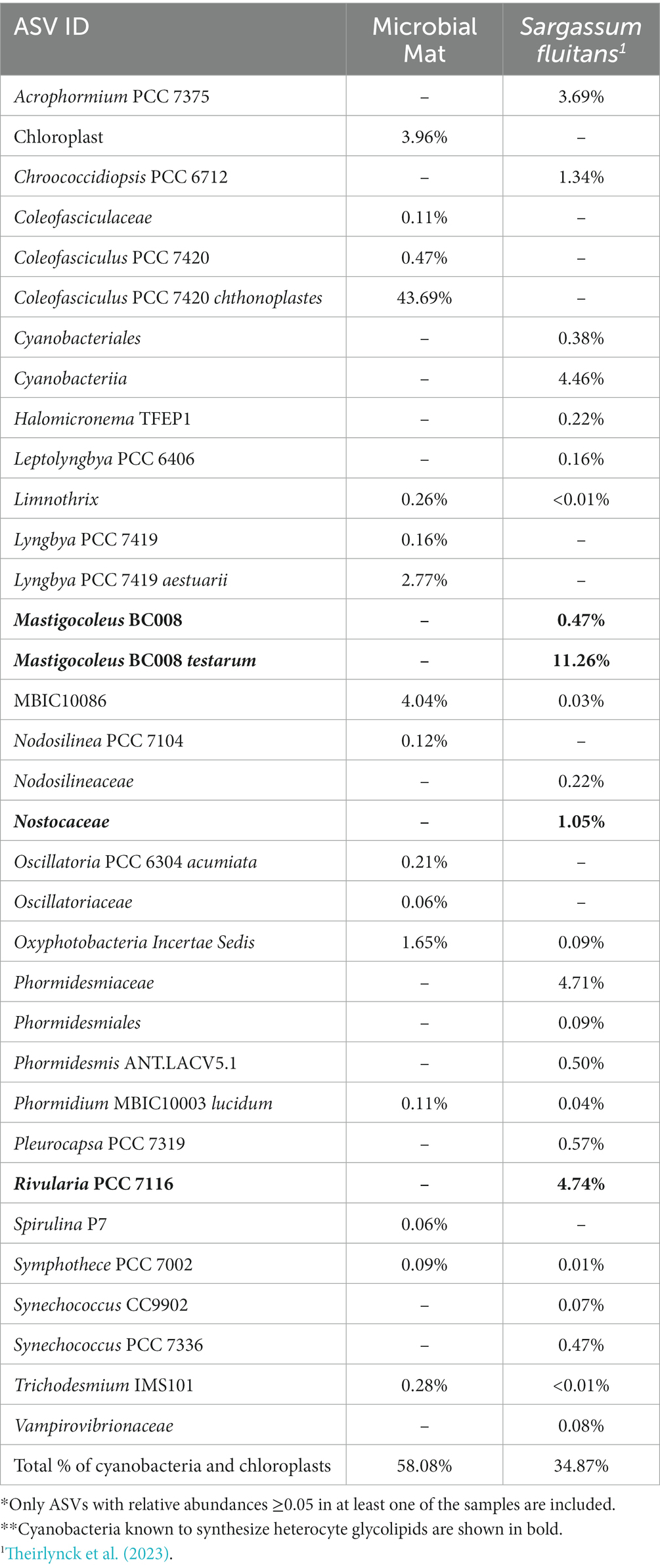
Table 4. Relative abundances of the cyanobacterial ASVs* present in the microbial mat and S. fluitans sample, obained after runing the Cascabel pipeline (Abdala Asbun et al., 2020).**
Next, we used a specimen of the floating macroalgae S. fluitans collected in the Central Atlantic Ocean. Its microbiome consists of 35.9% (ra) cyanobacteria, including several heterocytous cyanobacteria such as Mastigocoleus (11.7% ra) and Rivularia PCC 7116 (4.74% ra) (Table 4; Theirlynck et al., 2023), making it a good candidate to test our primers. Accordingly, we performed a gradient PCR (48–58°C annealing temperature, 1′10″ extension time, 37 cycles) on this specimen using the hglT-primer mix 2 (Fw1 mixB and Rv1). This led to successful amplification of the partial hglT gene when using annealing temperatures above 54.2°C. The most intense bands were observed at 54.2 and 56°C (Supplementary Figure S11), these results are in line with previous results obtained from testing the primer mix on cyanobacterial cultures and support our choice to use 56°C (instead of 58.2°C) as the preferred annealing temperature for all cyanobacterial cultures.
Thus, we have shown that the primers designed in this study can target and amplify the hglT gene of a wide variety of cyanobacterial species in the environment. This includes species not considered during primer design, such as Mastigocoleous and Rivularia PCC 7116, present in the microbiome of S. fluitans.
Conclusion
We conclude that the primer set 2 designed in this study and consisting of an equimolar mix of seven forward primers (Fw1 mixB) and five reverse primers (Rv1) can successfully target and partially amplify the hglT gene of heterocytous cyanobacteria in cultures and the environment. However, it may also target non-heterocytous cyanobacteria such as Synechococcus albeit to a lesser extent. We conclude that our primers can be used qualitatively to assess the presence of heterocytous cyanobacteria. However, to ensure accurate results we suggest that (1) a temperature gradient PCR should be performed to ensure that the best annealing temperature for the sample analyzed is used, and (2) the PCR product should be sequenced to confirm the presence of the suspected heterocytous cyanobacteria in the sample.
Data availability statement
The original contributions presented in the study are included in the article/Supplementary material. The 16S rRNA amplicon sequencing data presented in the study was deposited in the European Nucleotide Archive (ENA) repository, accession number PRJEB65747. Partial hglT sequences and phylogenetic trees presented in the study were deposited in the Zenodo repository, accession https://doi.org/10.5281/zenodo.8309792. Further inquiries can be directed to the corresponding author.
Author contributions
RP: Conceptualization, Formal analysis, Investigation, Resources, Visualization, Writing – original draft, Writing – review & editing. NB: Formal analysis, Resources, Writing – review & editing. JS: Funding acquisition, Writing – review & editing. LV: Resources, Supervision, Writing – original draft, Writing – review & editing.
Funding
The authors declare financial support was received for the research, authorship, and/or publication of this article. This work was supported by the European Research Council (ERC) under the European Union’s Horizon 2020 Research and Innovation Program (grant agreement no. 694569-MICROLIPIDS) to JS. LV and JS received funding from the Soehngen Institute for Anaerobic Microbiology (SIAM) through a Gravitation Grant (024.002.002) from the Dutch Ministry of Education, Culture, and Science (OCW).
Acknowledgments
We would like to thank Samuel Cirés for providing biomass of the C. ovalisporum strains UAM290 and UAM292, Koichiro Awai for providing the strain Anabaena sp. PCC 7120, Hoaxin Fan and Lucas Stal for providing the microbial mat samples, Tom Theirlynck and Linda Amaral-Zettler for providing the S. fluitans sample, Michele Grego for assistance handling the cyanobacterial cultures, Dina Castillo Boukhchtaber for her assistance preparing the microbial mat samples for 16S rRNA gene amplicon sequencing, and for carrying out the PCR on the S. fluitans sample, Lora Strack van Schijndel for her assistance extracting DNA from the microbial mat sample, and Bastiaan von Meijenfeldt for helpful discussions. We would also like to thank two referees for comments on an earlier version of this paper.
Conflict of interest
The authors declare that the research was conducted in the absence of any commercial or financial relationships that could be construed as a potential conflict of interest.
The author(s) declared that they were an editorial board member of Frontiers, at the time of submission. This had no impact on the peer review process and the final decision.
Publisher’s note
All claims expressed in this article are solely those of the authors and do not necessarily represent those of their affiliated organizations, or those of the publisher, the editors and the reviewers. Any product that may be evaluated in this article, or claim that may be made by its manufacturer, is not guaranteed or endorsed by the publisher.
Supplementary material
The Supplementary material for this article can be found online at: https://www.frontiersin.org/articles/10.3389/fmicb.2023.1257040/full#supplementary-material
Footnotes
References
Abdala Asbun, A., Besseling, M. A., Balzano, S., van Bleijswijk, J. D. L., Witte, H. J., Villanueva, L., et al. (2020). Cascabel: a scalable and versatile amplicon sequence data analysis pipeline delivering reproducible and documented results. Front. Genet. 11:489357. doi: 10.3389/fgene.2020.489357
Abd-Elsalam, K. A. (2003). Bioinformatic tools and guideline for PCR primer design. Afr. J. Biotechnol. 2, 91–95. doi: 10.4314/ajb.v2i5.14794
Altschul, S. F., Gish, W., Miller, W., Myers, E. W., and Lipman, D. J. (1990). Basic local alignment search tool. J. Mol. Biol. 215, 403–410. doi: 10.1016/S0022-2836(05)80360-2
Anisimova, M., Gil, M., Dufayard, J.-F., Dessimoz, C., and Gascuel, O. (2011). Survey of Branch Support Methods Demonstrates Accuracy, Power, and Robustness of Fast Likelihood-based Approximation Schemes. Syst Biol 60, 685–699. doi: 10.1093/sysbio/syr041
Awai, K., and Wolk, C. P. (2007). Identification of the glycosyl transferase required for synthesis of the principal glycolipid characteristic of heterocysts of anabaena sp. strain PCC 7120. FEMS Microbiol. Lett. 266, 98–102. doi: 10.1111/j.1574-6968.2006.00512.x
Bale, N. J., Hopmans, E. C., Dorhout, D., Stal, L. J., Grego, M., van Bleijswijk, J., et al. (2018a). A novel heterocyst glycolipid detected in a pelagic N2-fixing cyanobacterium of the genus Calothrix. Org. Geochem. 123, 44–47. doi: 10.1016/j.orggeochem.2018.06.009
Bale, N. J., Villareal, T. A., Hopmans, E. C., Brussaard, C. P. D., Besseling, M., Dorhout, D., et al. (2018b). C5 glycolipids of heterocystous cyanobacteria track symbiont abundance in the diatom Hemiaulus hauckii across the tropical North Atlantic. Biogeosciences 15, 1229–1241. doi: 10.5194/bg-15-1229-2018
Bauersachs, T., Compaoré, J., Hopmans, E. C., Stal, L. J., Schouten, S., and Sinninghe Damsté, J. S. (2009a). Distribution of heterocyst glycolipids in cyanobacteria. Phytochemistry 70, 2034–2039. doi: 10.1016/j.phytochem.2009.08.014
Bauersachs, T., Compaoré, J., Severin, I., Hopmans, E. C., Schouten, S., Stal, L. J., et al. (2011). Diazotrophic microbial community of coastal microbial mats of the southern North Sea. Geobiology 9, 349–359. doi: 10.1111/j.1472-4669.2011.00280.x
Bauersachs, T., Hopmans, E. C., Compaoré, J., Stal, L. J., Schouten, S., and Damsté, J. S. S. (2009b). Rapid analysis of long-chain glycolipids in heterocystous cyanobacteria using high-performance liquid chromatography coupled to electrospray ionization tandem mass spectrometry. Rapid Commun. Mass Spectrom. 23, 1387–1394. doi: 10.1002/rcm.4009
Bauersachs, T., Miller, S. R., Gugger, M., Mudimu, O., Friedl, T., and Schwark, L. (2019). Heterocyte glycolipids indicate polyphyly of stigonematalean cyanobacteria. Phytochemistry 166:112059. doi: 10.1016/j.phytochem.2019.112059
Bauersachs, T., Miller, S. R., van der Meer, M. T. J., Hopmans, E. C., Schouten, S., and Sinninghe Damsté, J. S. (2013). Distribution of long chain heterocyst glycolipids in cultures of the thermophilic cyanobacterium Mastigocladus laminosus and a hot spring microbial mat. Org. Geochem. 56, 19–24. doi: 10.1016/j.orggeochem.2012.11.013
Bauersachs, T., Mudimu, O., Schulz, R., and Schwark, L. (2014). Distribution of long chain heterocyst glycolipids in N2-fixing cyanobacteria of the order Stigonematales. Phytochemistry 98, 145–150. doi: 10.1016/j.phytochem.2013.11.007
Bauersachs, T., Rochelmeier, J., and Schwark, L. (2015). Seasonal lake surface water temperature trends reflected by heterocyst glycolipid-based molecular thermometers. Biogeosciences 12, 3741–3751. doi: 10.5194/bg-12-3741-2015
Bauersachs, T., Speelman, E. N., Hopmans, E. C., Reichart, G.-J., Schouten, S., and Damsté, J. S. S. (2010). Fossilized glycolipids reveal past oceanic N2 fixation by heterocystous cyanobacteria. Proc. Natl. Acad. Sci. 107, 19190–19194. doi: 10.1073/pnas.1007526107
Bauersachs, T., Talbot, H. M., Sidgwick, F., Sivonen, K., and Schwark, L. (2017). Lipid biomarker signatures as tracers for harmful cyanobacterial blooms in the Baltic Sea. PLoS One 12:e0186360. doi: 10.1371/journal.pone.0186360
Berrendero Gómez, E., Johansen, J. R., Kaštovský, J., Bohunická, M., and Čapková, K. (2016). Macrochaete gen. Nov. (Nostocales, cyanobacteria), a taxon morphologically and molecularly distinct from Calothrix. J. Phycol. 52, 638–655. doi: 10.1111/jpy.12425
Besseling, M. A., Hopmans, E. C., Boschman, R. C., Sinninghe Damsté, J. S., and Villanueva, L. (2018). Benthic archaea as potential sources of tetraether membrane lipids in sediments across an oxygen minimum zone. Biogeosciences 15, 4047–4064. doi: 10.5194/bg-15-4047-2018
Callahan, B. J., McMurdie, P. J., Rosen, M. J., Han, A. W., Johnson, A. J. A., and Holmes, S. P. (2016). DADA2: high-resolution sample inference from Illumina amplicon data. Nat. Methods 13, 581–583. doi: 10.1038/nmeth.3869
Camacho, C., Coulouris, G., Avagyan, V., Ma, N., Papadopoulos, J., Bealer, K., et al. (2009). BLAST+: architecture and applications. BMC Bioinformatics 10:421. doi: 10.1186/1471-2105-10-421
Capella-Gutiérrez, S., Silla-Martínez, J. M., and Gabaldón, T. (2009). trimAl: a tool for automated alignment trimming in large-scale phylogenetic analyses. Bioinformatics 25, 1972–1973. doi: 10.1093/bioinformatics/btp348
Caporaso, J. G., Kuczynski, J., Stombaugh, J., Bittinger, K., Bushman, F. D., Costello, E. K., et al. (2010). QIIME allows analysis of high-throughput community sequencing data. Nat. Methods 7, 335–336. doi: 10.1038/nmeth.f.303
Castenholz, R. W., Wilmotte, A., Herdman, M., Rippka, R., Waterbury, J. B., Iteman, I., et al. (2001). “Phylum BX. Cyanobacteria” in Bergey’s manual® of systematic bacteriology: Volume one: the archaea and the deeply branching and phototrophic bacteria. eds. D. R. Boone, R. W. Castenholz, and G. M. Garrity (New York, NY: Springer), 473–599.
Chen, I.-M. A., Chu, K., Palaniappan, K., Ratner, A., Huang, J., Huntemann, M., et al. (2021). The IMG/M data management and analysis system v.6.0: new tools and advanced capabilities. Nucleic Acids Res. 49, D751–D763. doi: 10.1093/nar/gkaa939
Edgar, R. C. (2004). MUSCLE: multiple sequence alignment with high accuracy and high throughput. Nucleic Acids Res. 32, 1792–1797. doi: 10.1093/nar/gkh340
Fan, H., Bolhuis, H., and Stal, L. J. (2015). Nitrification and nitrifying bacteria in a coastal microbial mat. Front. Microbiol. 6:1367. doi: 10.3389/fmicb.2015.01367
Gambacorta, A., Pagnotta, E., Romano, I., Sodano, G., and Trincone, A. (1998). Heterocyst glycolipids from nitrogen-fixing cyanobacteria other than nostocaceae. Phytochemistry 48, 801–805. doi: 10.1016/S0031-9422(97)00954-0
Gambacorta, A., Romano, I., Trincone, A., Soriente, A., Giordano, M., and Sodano, G. (1996). Heterocyst glycolipids from five nitrogen-fixing cyanobacteria. Gazz. Chim. Ital. 126, 653–656.
Guindon, S., Dufayard, J.-F., Lefort, V., Anisimova, M., Hordijk, W., and Gascuel, O. (2010). New algorithms and methods to estimate maximum-likelihood phylogenies: assessing the performance of PhyML 3.0. Syst. Biol. 59, 307–321. doi: 10.1093/sysbio/syq010
Halimatul, H. S. M., Ehira, S., and Awai, K. (2014). Fatty alcohols can complement functions of heterocyst specific glycolipids in Anabaena sp. PCC 7120. Biochem. Biophys. Res. Commun. 450, 178–183. doi: 10.1016/j.bbrc.2014.05.093
Haselkorn, R. (1978). Heterocysts. Annu. Rev. Plant Physiol. 29, 319–344. doi: 10.1146/annurev.pp.29.060178.001535
Hillis, D. M., and Bull, J. J. (1993). An Empirical Test of Bootstrapping as a Method for Assessing Confidence in Phylogenetic Analysis. Syst. Biol. 42, 182–192. doi: 10.1093/sysbio/42.2.182
Kalyaanamoorthy, S., Minh, B. Q., Wong, T. K. F., von Haeseler, A., and Jermiin, L. S. (2017). ModelFinder: fast model selection for accurate phylogenetic estimates. Nat. Methods 14, 587–589. doi: 10.1038/nmeth.4285
Katoh, K., and Standley, D. M. (2013). MAFFT multiple sequence alignment software version 7: improvements in performance and usability. Mol. Biol. Evol. 30, 772–780. doi: 10.1093/molbev/mst010
Kibbe, W. A. (2007). OligoCalc: an online oligonucleotide properties calculator. Nucleic Acids Res 35, W43–46. doi: 10.1093/nar/gkm234
Klugkist, J., and Haaker, H. (1984). Inhibition of nitrogenase activity by ammonium chloride in Azotobacter vinelandii. J. Bacteriol. 157, 148–151.
Knutson, C. M., Plunkett, M. H., Liming, R. A., and Barney, B. M. (2018). Efforts toward optimization of aerobic biohydrogen reveal details of secondary regulation of biological nitrogen fixation by nitrogenous compounds in Azotobacter vinelandii. Appl. Microbiol. Biotechnol. 102, 10315–10325. doi: 10.1007/s00253-018-9363-0
Komarek, J., Kastovsky, J., Mares, J., and Johansen, J. (2014). Taxonomic classification of cyanoprokaryotes (cyanobacterial genera) 2014, using a polyphasic approach. Preslia 86, 295–335.
Komárek, J., Santanna, C. L., Bohunická, M., Mareš, J., Hentschke, G. S., Rigonato, J., et al. (2013). Phenotype diversity and phylogeny of selected Scytonema-species (Cyanoprokaryota) from SE Brazil. Fottea 13, 173–200. doi: 10.5507/fot.2013.015
Letunic, I., and Bork, P. (2021). Interactive tree of life (iTOL) v5: an online tool for phylogenetic tree display and annotation. Nucleic Acids Res. 49, W293–W296. doi: 10.1093/nar/gkab301
McDonald, D., Clemente, J. C., Kuczynski, J., Rideout, J. R., Stombaugh, J., Wendel, D., et al. (2012). The biological observation matrix (BIOM) format or: how I learned to stop worrying and love the ome-ome. Gigascience 1:7. doi: 10.1186/2047-217X-1-7
Millineaux, P. M., Gallon, J. R., and Chaplin, A. E. (1981). Acetylene reduction (nitrogen fixation) by cyanobacteria grown under alternating light-dark cycles. FEMS Microbiol. Lett. 10, 245–247. doi: 10.1111/j.1574-6968.1981.tb06249.x
Mishra, A., Rajput, S., Gupta, P. S., Goyal, V., Singh, S., Sharma, S., et al. (2021). “Role of cyanobacteria in Rhizospheric nitrogen fixation” in Soil nitrogen ecology soil biology. eds. C. Cruz, K. Vishwakarma, D. K. Choudhary, and A. Varma (Cham: Springer International Publishing), 497–519.
Nübel, U., Garcia-Pichel, F., and Muyzer, G. (1997). PCR primers to amplify 16S rRNA genes from cyanobacteria. Appl. Environ. Microbiol. 63, 3327–3332.
Olson, J. B., Steppe, T. F., Litaker, R. W., and Paerl, H. W. (1998). N 2 -fixing microbial consortia associated with the ice cover of Lake Bonney, Antarctica. Microb. Ecol. 36, 231–238. doi: 10.1007/s002489900110
Parada, A. E., Needham, D. M., and Fuhrman, J. A. (2016). Every base matters: assessing small subunit rRNA primers for marine microbiomes with mock communities, time series and global field samples. Environ. Microbiol. 18, 1403–1414. doi: 10.1111/1462-2920.13023
Quast, C., Pruesse, E., Yilmaz, P., Gerken, J., Schweer, T., Yarza, P., et al. (2013). The SILVA ribosomal RNA gene database project: improved data processing and web-based tools. Nucleic Acids Res. 41, D590–D596. doi: 10.1093/nar/gks1219
Rippka, R., Deruelles, J., Waterbury, J. B., Herdman, M., and Stanier, R. Y. (1979). Generic assignments, strain histories and properties of pure cultures of cyanobacteria. Microbiology 111, 1–61. doi: 10.1099/00221287-111-1-1
Schaefer, B., Grice, K., Coolen, M. J. L., Summons, R. E., Cui, X., Bauersachs, T., et al. (2020). Microbial life in the nascent Chicxulub crater. Geology 48, 328–332. doi: 10.1130/G46799.1
Schouten, S., Villareal, T. A., Hopmans, E. C., Mets, A., Swanson, K. M., and Sinninghe Damsté, J. S. (2013). Endosymbiotic heterocystous cyanobacteria synthesize different heterocyst glycolipids than free-living heterocystous cyanobacteria. Phytochemistry 85, 115–121. doi: 10.1016/j.phytochem.2012.09.002
Severin, I., Acinas, S. G., and Stal, L. J. (2010). Diversity of nitrogen-fixing bacteria in cyanobacterial mats. FEMS Microbiol. Ecol. 73, 514–525. doi: 10.1111/j.1574-6941.2010.00925.x
Severin, I., and Stal, L. J. (2008). Light dependency of nitrogen fixation in a coastal cyanobacterial mat. ISME J. 2, 1077–1088. doi: 10.1038/ismej.2008.63
Svenning, M. M., Eriksson, T., and Rasmussen, U. (2005). Phylogeny of symbiotic cyanobacteria within the genus Nostoc based on 16S rDNA sequence analyses. Arch. Microbiol. 183, 19–26. doi: 10.1007/s00203-004-0740-y
Tel-Or, E., Stewart, W. D. P., and Fogg, G. E. (1997). Photosynthetic components and activities of nitrogen-fixing isolated heterocysts of Anabaena cylindrica. Proc. R. Soc. Lond. Ser. B Biol. Sci. 198, 61–86. doi: 10.1098/rspb.1977.0086
Theirlynck, T., Mendonça, I. R. W., Engelen, A. H., Bolhuis, H., Collado-Vides, L., van Tussenbroek, B. I., et al. (2023). Diversity of the holopelagic Sargassum microbiome from the great Atlantic Sargassum Belt to coastal stranding locations. Harmful Algae 122:102369. doi: 10.1016/j.hal.2022.102369
Walters, W., Hyde, E. R., Berg-Lyons, D., Ackermann, G., Humphrey, G., Parada, A., et al. (2015). Improved Bacterial 16S rRNA Gene (V4 and V4-5) and Fungal Internal Transcribed Spacer Marker Gene Primers for Microbial Community Surveys. mSystems 1:e00009–15. doi: 10.1128/mSystems.00009-15
Wang, Q., Garrity, G. M., Tiedje, J. M., and Cole, J. R. (2007). Naive Bayesian classifier for rapid assignment of rRNA sequences into the new bacterial taxonomy. Appl. Environ. Microbiol. 73, 5261–5267. doi: 10.1128/AEM.00062-07
Wolk, C. P., Ernst, A., and Elhai, J. (1994). “Heterocyst metabolism and development” in The molecular biology of cyanobacteria advances in photosynthesis. ed. D. A. Bryant (Dordrecht: Springer Netherlands), 769–823.
Wörmer, L., CirÉs, S., VelÁzquez, D., Quesada, A., and Hinrichs, K.-U. (2012). Cyanobacterial heterocyst glycolipids in cultures and environmental samples: diversity and biomarker potential. Limnol. Oceanogr. 57, 1775–1788. doi: 10.4319/lo.2012.57.6.1775
Keywords: heterocytous cyanobacteria, N2 fixation, heterocyte glycolipids, glycosyltransferase, lipid biosynthesis, polymerase chain reaction, primer design, environmental detection
Citation: Pérez Gallego R, Bale NJ, Sinninghe Damste JS and Villanueva L (2023) Developing a genetic approach to target cyanobacterial producers of heterocyte glycolipids in the environment. Front. Microbiol. 14:1257040. doi: 10.3389/fmicb.2023.1257040
Edited by:
Richard Allen White III, University of North Carolina at Charlotte, United StatesReviewed by:
Paraskevi Mara, Woods Hole Oceanographic Institution, United StatesMercedes Nieves Morión, Spanish National Research Council (CSIC), Spain
Copyright © 2023 Pérez Gallego, Bale, Sinninghe Damste and Villanueva. This is an open-access article distributed under the terms of the Creative Commons Attribution License (CC BY). The use, distribution or reproduction in other forums is permitted, provided the original author(s) and the copyright owner(s) are credited and that the original publication in this journal is cited, in accordance with accepted academic practice. No use, distribution or reproduction is permitted which does not comply with these terms.
*Correspondence: Ruth Pérez Gallego, ruth.perez.gallego@nioz.nl