- 1Research Group BIONUC (Biotechnology of Nutraceuticals and Bioactive Compounds), Departamento de Biología Funcional, Área de Microbiología, Universidad de Oviedo, Oviedo, Spain
- 2IUOPA (Instituto Universitario de Oncología del Principado de Asturias), Oviedo, Spain
- 3ISPA (Instituto de Investigación Sanitaria del Principado de Asturias), Oviedo, Spain
- 4Research Group Intestinal Microbiology, German Institute of Human Nutrition Potsdam-Rehbruecke, Nuthetal, Germany
Aromadendrin and taxifolin are two flavanonols (derived from the precursor naringenin) displaying diverse beneficial properties for humans. The carbon skeleton of these flavonoids may be transformed by the human gastrointestinal microbiota into other compounds, like auronols, which exert different and interesting biological activities. While research in flavonoids has become a certainly extensive field, studies about auronols are still scarce. In this work, different versions of the key plant enzyme for flavanonols biosynthesis, The flavanone 3-hydroxylase (F3H), has been screened for selecting the best one for the de novo production of these compounds in the bacterial factory Streptomyces albidoflavus UO-FLAV-004-NAR, a naringenin overproducer strain. This screening has rendered 2.6 μg/L of aromadendrin and 2.1 mg/L of taxifolin final production titers. Finally, the expression of the chalcone isomerase (CHI) from the gut bacterium Eubacterium ramulus has rendered a direct conversion (after feeding experiments) of 38.1% of (+)-aromadendrin into maesopsin and 74.6% of (+)-taxifolin into alphitonin. Moreover, de novo heterologous biosynthesis of 1.9 mg/L of alphitonin was accomplished by means of a co-culture strategy of a taxifolin producer S. albidoflavus and a CHI-expressing Escherichia coli, after the observation of the high instability of alphitonin in the culture medium. This study addresses the significance of culture time optimization and selection of appropriate enzymes depending on the desired final product. To our knowledge, this is the first time that alphitonin de novo production has been accomplished.
1 Introduction
Flavonoids are a family of polyphenolic compounds derived from plants (Kumar and Pandey, 2013; Das et al., 2021). These secondary metabolites are essential for the morphology and physiology of plants, displaying a wide range of functions, like cell growth regulation, pollinator insect attraction or protection against both biotic and abiotic stresses (Dias et al., 2021). Humans can benefit from flavonoid intake as these compounds show diverse bioactive properties, such as antioxidant (Chen et al., 2023; Liu et al., 2023), anti-inflammatory (Chen et al., 2023; Liu et al., 2023), antitumor (Redondo-Blanco et al., 2017) and cardioprotective (Espírito-Santo et al., 2023), among many others.
The chemical structure of flavonoids is composed of 15 carbon atoms (C6-C3-C6) forming two benzene rings (rings A and B) connected by a heterocyclic pyran ring (ring C) (Maleki et al., 2019; Marín et al., 2021). Flavonoids are grouped into different subclasses depending on the connection of rings B and C, the final conformation and oxidative status of ring C, and the total hydroxylation pattern. This structural variability of flavonoids is responsible for their diverse pharmacological and therapeutic potential (Maleki et al., 2019).
Flavonoids are present in small quantities in plants and accumulate only under certain environmental conditions. Consequently, their extraction constitutes a laborious and time-consuming process. On the other hand, their chemical synthesis is expensive and involves the use of toxic chemicals (Marín et al., 2021). For this reason, the heterologous production of flavonoids by microorganisms is becoming a valuable strategy as a fast and economic way of producing these compounds. In this context, the genus Streptomyces has been proven as a promising host for the enhanced production of flavonoids, given its ability to express multiple secondary metabolite biosynthetic gene clusters (Park et al., 2009, 2010, 2011; Marín et al., 2017, 2018, 2021; Hwang et al., 2021).
Aromadendrin (Figure 1), or dihydrokaempferol, is a flavanonol that exerts anti-inflammatory, antioxidant, and anti-diabetic properties (Lee et al., 2013). It has been reported to attenuate, in vivo, induced hepatic injury and hepatic fibrosis (Huang et al., 2023), and it shows a protective effect against experimental cardiac hypertrophy (Cui et al., 2018).
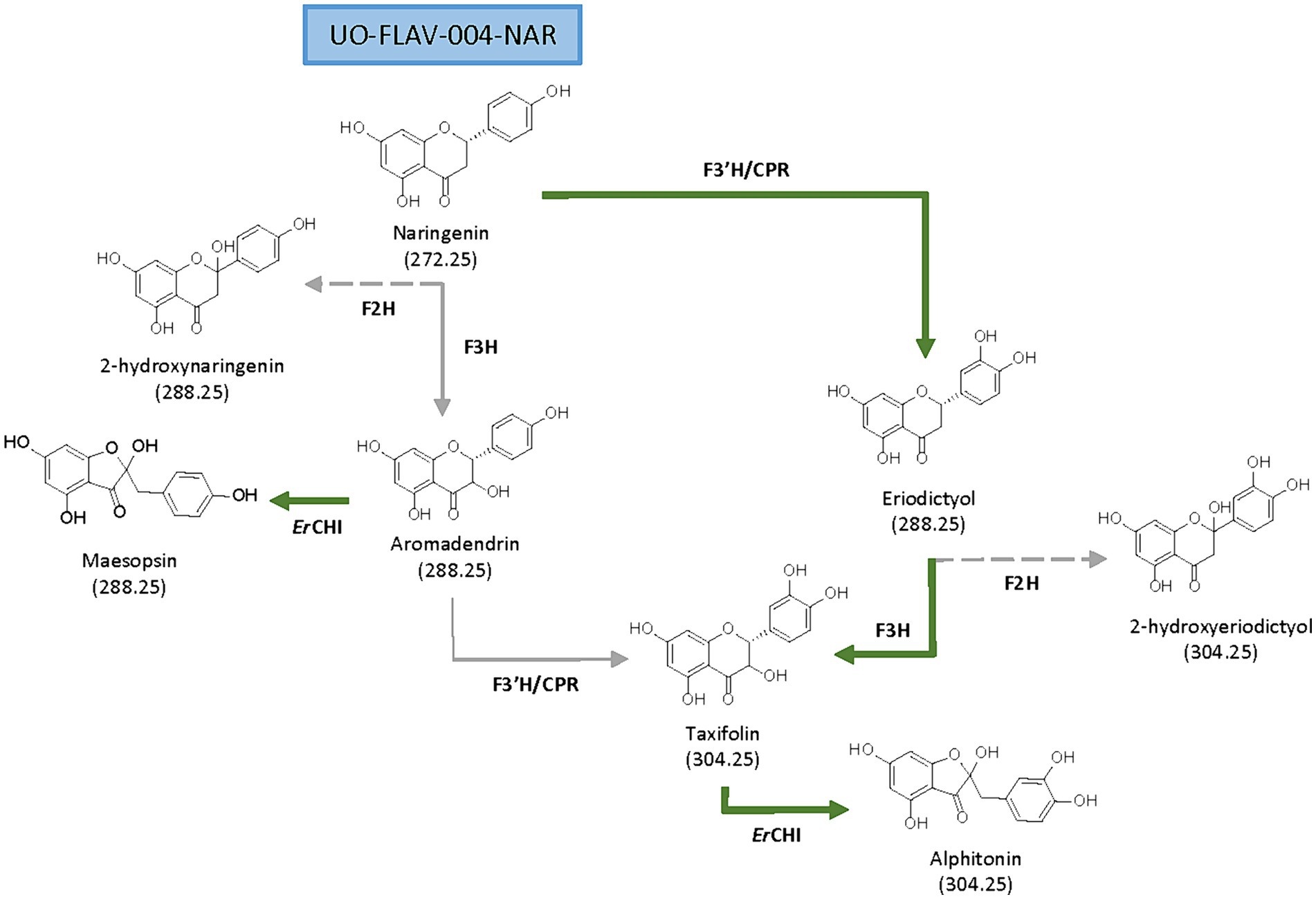
Figure 1. Proposed biosynthetic pathways for de novo production of flavanonols and auronols from naringenin. Main (green solid arrows) and secondary biosynthetic pathways (gray solid arrows), as well as side reactions (gray dashed arrows) are shown.
Taxifolin (Figure 1), also known as dihydroquercetin, is a flavanonol that, as aromadendrin, contains two stereocenters giving rise to four stereoisomers (Bernatova and Liskova, 2021; Das et al., 2021). Many pharmacological properties are attributed to taxifolin, such as anti-inflammatory, antioxidant (Liu et al., 2023), anti-Alzheimer, hepatoprotective, antiangiogenic, antimicrobial (Bernatova and Liskova, 2021; Das et al., 2021), and also cosmetic ones (Chen et al., 2023). Taxifolin has a higher antioxidant potential compared to other flavonoids, based on the disposition of its phenol groups and the elevated number of hydroxyl groups in its structure (Das et al., 2021).
Aurone derivates, auronols (Figure 1), are very interesting compounds due to their remarkable therapeutic potential as antiviral, immunosuppressive and chemopreventive agents, and also as inhibitors of multidrug resistance mechanisms (Elsinghorst et al., 2011). These auronols are flavonoid derivatives originating from a carbon skeleton reorganization in ring C, where this moiety is converted to a 5-carbon heterocyclic ring (Figure 1). In particular, alphitonin revealed significant antidiabetic and antioxidant properties (Kim et al., 2017), even more efficient than quercetin (Amić and Mastiľák Cagardová, 2023); and a maesopsin glycoside, maesopsin 4-O-β-glucoside, exhibited antitumor activity, both in vitro and in vivo, by inhibiting the growth of cancer cells (Pozzesi et al., 2011; Thuy et al., 2016). However, although auronols constitute a very promising group of compounds, yet few studies have been carried out on their therapeutic potential and their heterologous production.
The flavanone naringenin constitutes a central metabolite in flavonoid biosynthesis, being the common precursor for the biosynthesis of all the abovementioned compounds. From naringenin, the additional required genes constitute the main rate-limiting steps for both aromadendrin and taxifolin heterologous production (Lv et al., 2019; Gao et al., 2020; Yu et al., 2022). A flavanone 3-hydroxylase (F3H) is needed to convert naringenin into aromadendrin. This F3H, belonging to the 2-oxoglutarate-dependent dioxygenase family, exerts a low catalytic efficiency (Gao et al., 2020) and shows a 2-hydroxylase side activity (Marín et al., 2021). Subsequent conversion of aromadendrin into taxifolin is catalyzed by a flavonoid 3′-hydroxylase (F3’H). This is a membrane-bound cytochrome P450 monooxygenase that requires a cytochrome P450 reductase (CPR) for electron transfer (Leonard et al., 2006; Marín et al., 2017; Wu et al., 2022). In a previous work from our research group, the low activity of F3’H, due to its cytoplasmic insolubility and the need for reducing power, was addressed in Streptomyces albidoflavus J1074 by constructing a chimeric enzyme of F3’H and a reductase (Magadán-Corpas et al., 2023).
Flavonoids may be transformed by bacteria in the human gastrointestinal tract as part of the bacterial metabolism. In this way, aromadendrin and taxifolin are transformed into their respective auronol derivatives by the action of a chalcone isomerase from Eubacterium ramulus (ErCHI) (Braune et al., 2001, 2016). This reaction is a coenzyme-independent isomerization taking place in the presence or absence of oxygen (Braune et al., 2001). The ErCHI has been proven as enantioselective toward (+)-aromadendrin and (+)-taxifolin, catalyzing its C-ring contraction to maesopsin or alphitonin, respectively, by a ring opening-recyclation mechanism, with the corresponding chalcone and its di-keto tautomer as reaction intermediates (Elsinghorst et al., 2011; Braune et al., 2016).
Regarding this, it has been described that the presence of the biosynthetic precursor eriodictyol reduces the ErCHI-catalyzed isomerization of (+)-taxifolin into alphitonin to 51%, due to a competition for binding to the ErCHI active site. Also, the (+)-aromadendrin conversion into maesopsin is reduced to 84% in the presence of naringenin. This inhibition is reported to occur through a blockage of the ErCHI active site, with no further transformation of neither naringenin nor eriodictyol (Braune et al., 2016).
In this study, the biosynthesis of aromadendrin, taxifolin, maesopsin and alphitonin has been addressed in a naringenin overproducer S. albidoflavus J1074 strain. In this context, the auronols instability in the culture conditions has been identified as a major drawback for their heterologous production in S. albidoflavus and has been overcome by means of feeding with precursors for both maesopsin and alphitonin, and the co-culture of a taxifolin-producer S. albidoflavus strain with a CHI-expressing E. coli strain, after optimal F3H selection, for de novo alphitonin production.
2 Materials and methods
2.1 Genes, enzymes, antibiotics, and primers
Restriction enzymes and T4 DNA ligase were purchased from Thermo Fisher Scientific. Herculase II Fusion DNA polymerase was purchased from Agilent Technologies. NEBuilder® HiFi DNA Assembly Master Mix for Gibson assembly was purchased from Thermo Fisher Scientific. The antibiotics used were ampicillin (100 μg/mL) (Sigma Aldrich; Madrid, Spain); apramycin (50 μg/mL for S. albidoflavus or 100 μg/mL for E. coli) (Thermo Fisher Scientific; MA, United States); nalidixic acid (50 μg/mL) (Acros Organics; Belgium) and thiostrepton (50 μg/mL) (Cayman Chemical; MI, United States).
Primers were synthesized by IDT Technologies (Leuven, Belgium). Sequences of primers used in this work are listed in Supplementary Table S1.
This work is based on the F3H from Petroselinum crispum (Genbank accession no. AY230248, and OR820614 for the optimized gene), the F3H from Malus domestica (Genbank accession no. AAX89397, and OR820613 for the optimized gene), the F3H from Camellia sinensis (Genbank accession no. AY641730, and OR820612for the optimized gene), and the CHI from Eubacterium ramulus (GenBank accession no. AIS36173, and OR820615 for the optimized gene). The encoding gene sequences were synthesized by Explora Biotech (Venice, Italy) after codon optimization for Streptomyces codon usage and removal of unique restriction sites for SEVA platform and Golden Standard cloning, as Golden Standard level 0 plasmids.
2.2 Plasmid construction
All the plasmids developed in this study have been constructed based in the plasmid library for Streptomyces developed in our group (Magadán-Corpas et al., 2023), following the Golden Standard Assembly (Blázquez et al., 2023) and listed in Supplementary Table S2. The details on the construction of each plasmid in this work are included in the Supplementary data file (Plasmid Construction section, page 4). All plasmids described were verified by restriction enzyme digestions and sequencing.
2.3 Strain generation
Exogenous DNA was introduced into S. albidoflavus by either protoplast transformation or intergeneric conjugation (Kieser et al., 2000). Strains containing integrative plasmids were selected based on their resistance to the corresponding antibiotics and/or by PCR.
The strain S. albidoflavus UO-FLAV-004-NAR carries the naringenin biosynthetic gene cluster (BGC) integrated into its ՓC31 chromosomal site (Pérez-Valero et al., 2023). Plasmids pSEVAUO-M22106-PcF3H, pSEVAUO-M22106-MdF3H and pSEVAUO-M22106-CsF3H, pSEVAUO-M21503-PcF3H-F3’H/CPR, pSEVAUO-M21503-CsF3H-F3’H/CPR and pSEVAUO-M21603-PcF3H-F3’H/CPR-ErCHI [Supplementary data file (Plasmid Construction section, page 4)] were independently integrated into the ՓBT1 integration site of the S. albidoflavus UO-FLAV-004-NAR strain, yielding S. albidoflavus UO-FLAV-004-PcARO, S. albidoflavus UO-FLAV-004-MdARO, S. albidoflavus UO-FLAV-004-CsARO, S. albidoflavus UO-FLAV-004-PcTAX, S. albidoflavus UO-FLAV-004-CsTAX and S. albidoflavus UO-FLAV-004-ALPH strains, respectively.
Plasmid pSEVAUO-M21302-ErCHI [Supplementary data file (Plasmid Construction section, page 4)] was integrated into the ՓBT1 integration site of S. albidoflavus UO-FLAV-004 strain, the same improved strain used in previous experiments, but lacking naringenin BGC (Pérez-Valero et al., 2023), yielding the strain S. albidoflavus UO-FLAV-004-ErCHI.
2.4 Bacterial strains and culture conditions
All strains used in this study are listed in Supplementary Table S2. E. coli strains were grown as previously specified (Magadán-Corpas et al., 2023). S. albidoflavus was grown at 30°C in yeast extract-malt extract (YEME) supplemented with 17% (w/v) sucrose for the preparation of protoplasts, and MA medium was used for conjugation (Fernández et al., 1998). S. albidoflavus was sporulated in Bennett medium (Kieser et al., 2000) supplemented with the corresponding antibiotics when necessary.
For flavonoid production, S. albidoflavus spores were quantified, and 107 spores/ml were inoculated in shake flasks with 25 mL of NL333 pH 7.2 medium (Myronovskyi et al., 2014) and incubated at 30°C and 250 revolutions per minute (rpm) for 5 days, unless otherwise specified. Feedings with precursors were performed after 24 h of culture at a final concentration of 100 μM, and samples were collected after 5 days of total culture time (unless otherwise specified). Cultures were performed in triplicate.
Regarding the co-cultures of S. albidoflavus and E. coli, 107 spores/mL of S. albidoflavus UO-FLAV-004-CsTAX strain were cultivated in NL333 pH 7.2 medium for 48 h at 30°C and 250 rpm. An inoculum of 350 μL from an E. coli JM109 pTI2 overnight culture was added to 35 mL of TSB with ampicillin and cultivated at 37°C and 250 rpm until OD reached 0.6. Then, IPTG 1 mM was added, and the culture was grown for another 4 h at 37°C and 250 rpm. Following this, 10 mL of the E. coli culture were centrifuged, the cell pellet resuspended in 1 mL of S. albidoflavus UO-FLAV-004-CsTAX culture aliquot and added to the S. albidoflavus flask. Samples were taken from the S. albidoflavus UO-FLAV-004-CsTAX culture immediately before adding E. coli as a control, and immediately after placing the co-culture. This process was carried out in triplicate.
2.5 Reagents and biochemicals
All solvents used for solid-phase extraction, High-Performance Liquid Chromatography with Diode Array Detection (HPLC-DAD) and High Performance Liquid Chromatography-High-Resolution Electrospray Ionization Mass Spectrometry (HPLC-HRESIMS) analysis were LC–MS grade from either Sigma-Aldrich or VWR Chemicals. Authentic standard compounds for identification and quantification by HPLC-HRESIMS and HPLC-DAD analyses were provided by Extrasynthese (Genay, France).
2.6 Flavonoid extraction
Spores from the different S. albidoflavus strains were incubated as previously described. Samples of 1 mL were taken from flasks. Flavonoids were recovered by an organic extraction with acetone (cellular pellet) and ethyl acetate (culture supernatant) as previously described (Magadán-Corpas et al., 2023). The final dry extract was reconstituted in 100 μL DMSO/MeOH (1:1, v/v), and the samples were centrifuged prior to HPLC injection.
For alphitonin and maesopsin experiments, sample extraction was performed immediately after their collection, with fresh solutions of organic solvents (acetone and ethyl acetate) acidified (Braune et al., 2016) with 0.1% formic acid.
2.7 HPLC analysis
Flavonoid identification was performed using either HPLC-DAD or HPLC-HRESIMS. The HPLC-DAD separation was conducted in a 1,260 Infinity (Agilent Technologies) HPLC system equipped with an analytical Pursuit XRs C18 column (50 × 4.0 mm, 5 μm, Agilent Technologies). Samples were run by an isocratic elution of 90% de-ionized water and 10% MeCN, both acidified with 0.1% formic acid, during 5.44 min, followed by a linear gradient from 10 to 35% of MeCN until min. 21.77, then maintained until min. 27.21. Next, a linear gradient from 35 to 100% MeCN was performed up to min. 43.54, followed by an isocratic elution until min. 55. Afterwards, a linear gradient from 100 to 10% MeCN was applied up to min. 56, and prolonged until the end of the program (min. 61).
The identification and quantification of the compounds by HPLC–HRESIMS and tandem mass spectrometry (MS/MS) was performed in an Ultra Performance Liquid Chromatography (UPLC) system (Dionex Ultimate 3000, Thermo Scientific, Madrid, Spain) equipped with an analytical RP-18 HPLC column (50 × 2.1 mm, Zorbax® Eclipse Plus, 1.8 μm, Agilent Technologies, Madrid, Spain) as previously described (Marín et al., 2021). The obtained base peak chromatograms (BPCs) were extracted for the deprotonated ions of a set of flavonoids (271.0611 [M-H]− (calculated for C15H12O5) corresponding to naringenin; 287.0561 [M-H]− (calculated for C15H12O6) for eriodictyol, aromadendrin, 2-hydroxynaringenin and maesopsin; 303.0510 [M-H]− (calculated for C15H12O7), corresponding to taxifolin and alphitonin) with a mass error range of 0.005 milli mass units (mmu) and the obtained extracted ion chromatograms (EICs) were compared with authentic commercial standards.
Compounds were quantified by comparing the peak area with that of a known amount of an authentic compound through a calibration curve. The production titers are given in μg/L or mg/L, and the mean value was calculated from three biological replicates.
2.8 Flavonoids stability tests
For alphitonin, 10 mL NL333 pH 7.2 in a 100 mL flask was spiked with 50 μM of commercial alphitonin, and 1 mL samples were taken immediately after the feeding, 4 h and 24 h post-feeding. The samples were immediately extracted with 800 μL of ethyl acetate freshly acidified with 0.1% formic acid, and this extraction was repeated. The collected organic phases were dried in a vacuum centrifuge. The dry extract obtained was reconstituted in 100 μL DMSO/MeOH 1:1 (v/v), and the samples were centrifuged prior to HPLC-DAD injection.
For taxifolin stability tests with cells, 25 mL NL333 pH 7.2 in a 250 mL flask were inoculated with 107 UFC/mL of S. albidoflavus UO-FLAV-004 strain, incubated for 48 h at 250 rpm and 30°C, and then fed with 10 μM of commercial taxifolin. Samples of 1 mL were taken after the feeding every 24 h for 5 days and extracted afterwards. The dry extract obtained was reconstituted in 100 μL DMSO/MeOH 1:1 (v/v), and 5 μL were used for HPLC-HRESIMS analysis. For taxifolin stability tests without cells, identical culture conditions were used, but samples were taken every 24 h for 4 days and extracted afterwards.
2.9 Accession numbers
Sequence data have been deposited to the GenBank databases under accession numbers OR820614 for Petroselinum crispum F3H, OR820613 for Malus domestica F3H, OR820612 for Camellia sinensis F3H, OR820615 for Eubacterium ramulus CHI and OR820616 for the Golden Standard level 1 receptor plasmid pSEVAUO-M22106, assembled for this study as part of the Golden Standard library developed for its use in actinomycetes (Magadán-Corpas et al., 2023).
2.10 Statistical analysis
A two-way ANOVA was used to compare cultivation data between different strains. A Sidak correction was used. The alpha threshold (and confidence level) selected for the data were 0.05 (95% confidence level). The data were expressed as the mean value ± standard error of mean (S.E.M.). The graphic representation of all cultivation data was carried out using GRAPHPAD PRISM software (version 9, GraphPad Software, San Diego, CA, United States).
3 Results
3.1 Heterologous biosynthesis and optimization of aromadendrin
Aromadendrin is produced directly from naringenin by a F3H-catalyzed hydroxylation at the position 3 of ring C (Figure 1). In a preceding work from our research group, the Petroselinum crispum F3H enzyme (PcF3H), previously named as N3DOX, was used in S. albidoflavus J1074 for the biosynthesis of kaempferol, myricetin and quercetin (Marín et al., 2018), sharing all of them aromadendrin as a pathway precursor. For this study, the same PcF3H enzyme was used, however, the assembly strategy was approached differently, having each gene its own promoter, RBS and terminator [Supplementary data file (Plasmid Construction section)]. The strain S. albidoflavus UO-FLAV-004-NAR, a genetic engineered S. albidoflavus J1074 strain improved for naringenin production and able to reach 3.4 mg/L of this compound (Pérez-Valero et al., 2023), was used for further modifications.
The resulting aromadendrin producer strain, S. albidoflavus UO-FLAV-004-PcARO [see Material and Methods; Supplementary data file (Plasmid Construction section)], was cultivated, and its extracts were analyzed by HPLC-HRESIMS. A strain carrying the empty vector pSEVAUO-M22106, S. albidoflavus UO-FLAV-004-NAR-M22106, was used as negative control.
The obtained base peak chromatograms (BPCs) were extracted for the aromadendrin mass peak. A differential peak [retention time (rt): 5.5 min] for the proposed m/z, that perfectly coelutes with the pure commercial standard of aromadendrin, was detected in the samples from the producer strain (Figure 2A). The quantification of aromadendrin production titers in the S. albidoflavus UO-FLAV-004-PcARO strain was 0.2 ± 0.09 μg/L. The BPCs were additionally extracted for the precursor naringenin mass peak (rt: 6.9 min). Albeit naringenin is partially transformed to aromadendrin, large quantities of naringenin remained in the extracts (Figure 2A), revealing the PcF3H activity as the main bottleneck for aromadendrin production. The presence of the derivative product 2-hydroxynaringenin, generated due to the 2-hydroxylase (F2H) side activity of the F3H enzyme over naringenin, can also be detected in the chromatogram (rt: 4.2 min) (Figure 2A).
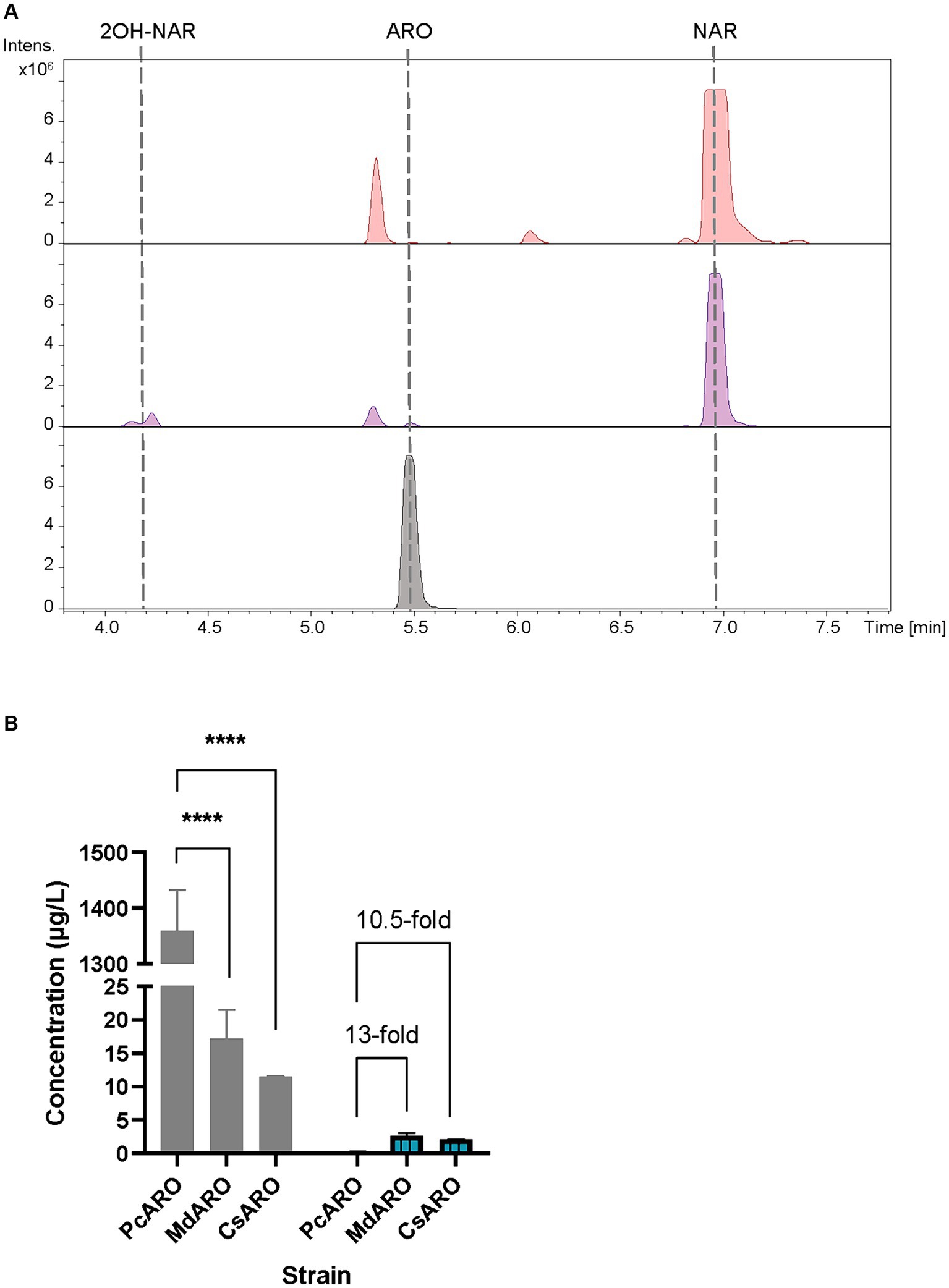
Figure 2. (A) HPLC-HRESIMS chromatograms of control strain S. albidoflavus UO-FLAV-004-NAR-M22106 (red) and the aromadendrin de novo producer strain S. albidoflavus UO-FLAV-004-PcARO (purple). Extracted ion chromatograms for naringenin, 2-hydroxynaringenin and aromadendrin. Aromadendrin commercial standard (black). (B) Compared de novo aromadendrin biosynthesis (blue/lines) and precursor naringenin accumulation (gray) after F3H screening of S. albidoflavus UO-FLAV-004-PcARO, UO-FLAV-004-MdARO, and UO-FLAV-004-CsARO strains. NAR, naringenin; ARO, aromadendrin; 2OH-NAR, 2-hydroxynaringenin. Asterisks indicate statistically significant differences (*p < 0.05; **p < 0.005; ***p < 0.0005; ****p < 0.0001).
Therefore, two other F3Hs were selected from different plant species, Malus domestica (MdF3H) and Camellia sinensis (CsF3H), and these were compared regarding their performance with PcF3H. These two new constructions were generated using the same promoter than the previous one, as a way to achieve the same transcription levels for the three genes. Strains S. albidoflavus UO-FLAV-004-MdARO and S. albidoflavus UO-FLAV-004-CsARO [see Material and Methods; Supplementary data file (Plasmid Construction section)] were cultivated, and the extracts were compared for aromadendrin production together with the S. albidoflavus UO-FLAV-004-PcARO strain.
The obtained BPCs were extracted for aromadendrin and naringenin mass peaks. The recombinant strain expressing CsF3H yielded 2.1 ± 0.03 μg/L of aromadendrin, similar to the titers reached with the MdF3H-expressing strain of 2.6 ± 0.65 μg/L (Figure 2B). This constitutes a remarkable 13-fold increase in aromadendrin production compared to the PcF3H-containing strain (0.2 ± 0.09 μg/L) (Figure 2B). However, the precursor naringenin was still accumulated (Figure 2B) and the side product 2-hydroxynaringenin was detected (data not shown) in incubations with all strains, meaning that F3H remains as the main bottleneck for aromadendrin production.
3.2 Heterologous biosynthesis and optimization of taxifolin
Taxifolin can be obtained from aromadendrin through the action of the F3’H hydroxylase. This enzyme is responsible for the hydroxylation of aromadendrin B-ring at the 3′ position (Figure 1). F3’H from Arabidopsis thaliana was successfully tested in our group fused to a reductase from the same plant as a chimera (F3’H/CPR) for the enhanced production of eriodictyol from naringenin (Magadán-Corpas et al., 2023).
In parallel to the aromadendrin producer strain S. albidoflavus UO-FLAV-004-PcARO, the taxifolin producer strain S. albidoflavus UO-FLAV-004-PcTAX was also generated [see Material and Methods; Supplementary data file (Plasmid Construction section)]. To confirm the heterologous production of taxifolin, the strain S. albidoflavus UO-FLAV-004-PcTAX was cultivated, and extracts were analyzed by HPLC-HRESIMS. The strain S. albidoflavus UO-FLAV-004-NAR-M21503, carrying the empty vector, was used as the negative control.
The obtained BPCs were extracted for the naringenin (rt: 6.9 min), eriodictyol (rt: 6.1 min), aromadendrin (rt: 5.5 min) and taxifolin mass peaks. The obtained extracted ion chromatograms (EICs) revealed the presence of a peak (rt: 4.9 min) for the proposed m/z that was absent in the negative control S. albidoflavus UO-FLAV-004-NAR-M21503 strain, and that perfectly coeluted with the pure commercial standard of taxifolin (Figure 3A). Neither naringenin nor aromadendrin were detected in this strain. However, a peak corresponding to eriodictyol was detected resulting from the F3’H/CPR hydroxylation of naringenin (Figure 3A). The quantification of taxifolin production titers in the S. albidoflavus UO-FLAV-004-PcTAX led to 2.1 ± 0.11 mg/L. Considering the aromadendrin production titers of this study (0.2 ± 0.09 μg/L ≈ 7×10−4 μM), the amount of taxifolin achieved (2.1 ± 0.11 mg/L ≈ 6.9 μM) is much higher than the expected to be formed by direct conversion from aromadendrin. In addition to its established role in catalyzing the conversion of naringenin, F3H enzyme has been documented as capable of hydroxylating eriodictyol to produce the corresponding (2R/3R)-dihydroflavonol, taxifolin (Cheng et al., 2014). This finding, in conjunction with the prior observation of the substrate versatility of F3’H enzyme (converting naringenin to eriodictyol or aromadendrin into taxifolin) (Magadán-Corpas et al., 2023), has prompted us to propose an alternative pathway for the production of taxifolin from eriodictyol (Figure 1). The accumulation of the precursor eriodictyol in S. albidoflavus UO-FLAV-004-PcTAX, suggests that analogous to the situation observed in aromadendrin production, the primary limiting factor for taxifolin production, is associated with the enzymatic activity of the PcF3H enzyme.
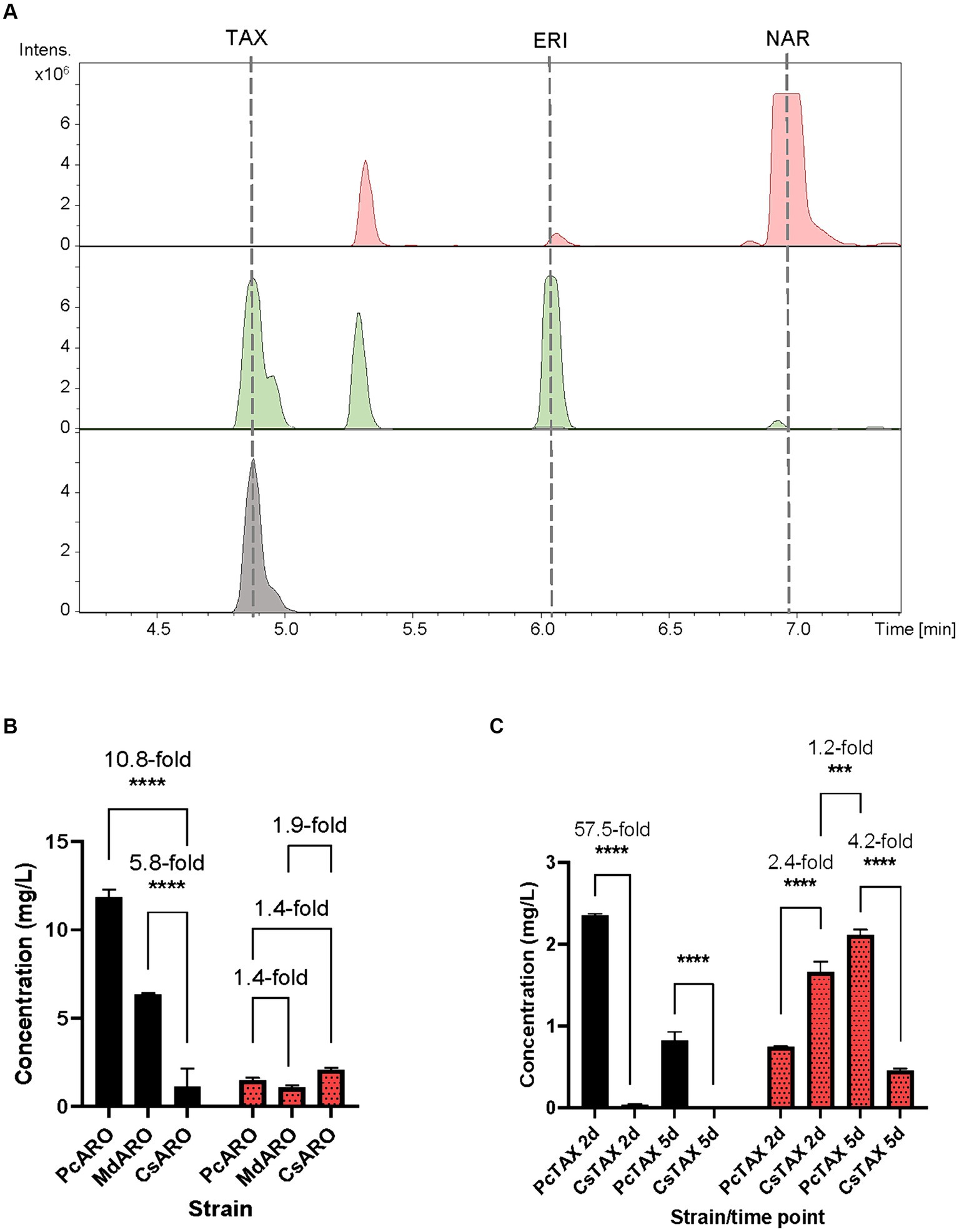
Figure 3. (A) HPLC-HRESIMS chromatograms of the control strain S. albidoflavus UO-FLAV-004-NAR-M21503 (red) and the taxifolin de novo producer strain S. albidoflavus UO-FLAV-004-PcTAX (green). Extracted ion chromatograms for naringenin, eriodictyol, aromadendrin and taxifolin. Taxifolin commercial standard (black). (B) Compared taxifolin biosynthesis (red/dots) and precursor eriodictyol accumulation (black) after feeding with 100 μM eriodictyol to S. albidoflavus UO-FLAV-004-PcARO, S. albidoflavus UO-FLAV-004-MdARO and S. albidoflavus UO-FLAV-004-CsARO. (C) Compared de novo taxifolin biosynthesis (red/dots) and precursor eriodictyol accumulation (black) of S. albidoflavus UO-FLAV-004-PcTAX and S. albidoflavus UO-FLAV-004-CsTAX. Samples taken 2 and 5 days after inoculation. The folds of the increased production are depicted. NAR, naringenin; TAX, taxifolin; ERI, eriodictyol. Asterisks indicate statistically significant differences (*p < 0.05; **p < 0.005; ***p < 0.0005; ****p < 0.0001).
Henceforth, a feeding study involving the utilization of a 100 μM concentration of eriodictyol was conducted on three distinct strains, namely, S. albidoflavus UO-FLAV-004-MdARO, S. albidoflavus UO-FLAV-004-CsARO, and S. albidoflavus UO-FLAV-004-PcARO. This investigation aimed to assess the comparative capabilities of the respective F3H enzymes in these strains with regard to the synthesis of taxifolin following eriodictyol supplementation. Samples were analyzed by HPLC-DAD. The highest taxifolin titer was 2.1 ± 0.1 mg/L, yielded by CsF3H, and constituting a 1.4-fold increased production compared to PcF3H (1.5 ± 0.2 mg/L) and a 1.9-fold compared to MdF3H (1.1 ± 0.2 mg/L) (Figure 3B). A remarkable 10.8-fold decrease in the amount of eriodictyol that remained to be converted could be observed when the CsF3H was compared with the PcF3H, while a 5.8-fold decrease in the remained eriodictyol was detected when the comparison was made with the MdF3H (Figure 3B).
Subsequent to these results, a taxifolin-producer strain of S. albidoflavus was engineered, designated as S. albidoflavus UO-FLAV-004-CsTAX, in which the CsF3H enzyme was integrated instead of the PcF3H enzyme. A comparative evaluation was conducted between this newly created strain and the previously assayed S. albidoflavus UO-FLAV-004-PcTAX strain to assess their respective abilities in achieving enhanced taxifolin production. Samples were taken after 2 and 5 days of cultivation. Taxifolin and eriodictyol levels were measured by HPLC-HRESIMS. Divergent outcomes were noted contingent on the duration of the cultivation period. Specifically, after a 2-day cultivation period, strain S. albidoflavus UO-FLAV-004-CsTAX exhibited a taxifolin production that was 2.4-fold greater than that of strain S. albidoflavus UO-FLAV-004-PcTAX (1.7 ± 0.2 mg/L vs. 0.7 ± 0.005 mg/L) (Figure 3C), aligning with the results from the feeding experiments. Conversely, after 5 days of cultivation, strain S. albidoflavus UO-FLAV-004-PcTAX yielded a taxifolin production 4.2-fold higher than that of strain S. albidoflavus UO-FLAV-004-CsTAX (2.1 ± 0.1 mg/L vs. 0.5 ± 0.04 mg/L) (Figure 3C). Notably, the highest levels of residual eriodictyol were detected in the presence of the PcF3H enzyme, amounting to 2.3 ± 0.04 mg/L and 0.8 ± 0.19 mg/L after 2 and 5 days of incubation, respectively. These levels displayed a decreasing trend over time, congruent with the observed trend of increasing taxifolin production. In contrast, minimal levels of eriodictyol were detected with the CsF3H enzyme, registering at 0.04 ± 0.01 mg/L after 2 days of incubation, this indicating a 57.5-fold lower concentration of unconsumed precursor compared to PcF3H. After 5 days, eriodictyol levels regarding the CsF3H enzyme were below the detection limit (Figure 3C). These observations in eriodictyol consumption correlated with the earliest attainment of maximum taxifolin yield achieved by the CsF3H enzyme. Following this rationale, it would be expected that taxifolin levels would remain relatively stable over time. However, a subsequent decrease in taxifolin levels was observed after 5 days of incubation in the case of the CsF3H enzyme, which may be attributed to potential taxifolin degradation within the culture.
To substantiate this hypothesis, additional experiments were conducted wherein a 10 μM taxifolin supplement was added to the NL333 pH 7.2 culture medium, both in the presence and absence of S. albidoflavus UO-FLAV-004 cells [the same improved strain used in previous experiments, but lacking naringenin BGC (Pérez-Valero et al., 2023)]. The outcomes of these experiments demonstrated that taxifolin was indeed undergoing degradation or conversion by S. albidoflavus UO-FLAV-004. This assertion is supported by the conspicuous reduction in taxifolin levels, which was exclusively observed when the taxifolin supplementation occurred in the presence of the microbial cells (Supplementary Figure S1A), whereas taxifolin levels remained largely stable in the unseeded medium (Supplementary Figure S1B).
In summary, the optimal strategy for taxifolin production involved a 5-day incubation period of the S. albidoflavus UO-FLAV-004-PcTAX strain. However, it is important to note that this approach yielded only a modest 1.2-fold enhancement in taxifolin production compared to the highest production level achieved with CsF3H enzyme, which occurred after a 2-day incubation period and exhibited substantially reduced precursor accumulation (Figure 3C).
3.3 Heterologous biosynthesis of alphitonin
A chalcone isomerase (CHI) is needed to catalyze the C-ring contraction of (+)-aromadendrin and (+)-taxifolin to the auronols maesopsin and alphitonin, respectively (Figure 1). In this study, the CHI from Eubacterium ramulus (ErCHI) was selected to carry out this conversion (Braune et al., 2016).
Following the development of the previous strains, the alphitonin producer S. albidoflavus UO-FLAV-004-ALPH strain was generated [see Material and Methods; Supplementary data file (Plasmid Construction section)]. To confirm the heterologous production of alphitonin, the strain S. albidoflavus UO-FLAV-004-ALPH was cultivated, its samples extracted with acidified organic solvents (see Materials and Methods) and analyzed by HPLC-HRESIMS. The strain S. albidoflavus UO-FLAV-004-PcTAX was used as the negative control.
The obtained BPCs were extracted for the mass peaks corresponding to taxifolin and alphitonin. The obtained EICs unveiled the presence of a peak (rt: 3.1 min) corresponding to the proposed m/z value, which co-eluted with the pure commercial standard of alphitonin. However, it is noteworthy that the same peak was also identified in the negative control, the S. albidoflavus UO-FLAV-004-PcTAX strain (Figure 4). This observation suggests the absence of alphitonin production. Conversely, the peak corresponding to the precursor taxifolin (rt: 4.9 min) was conspicuously absent in the extracts obtained from the strain S. albidoflavus UO-FLAV-004-ALPH (Figure 4). Moreover, no additional new peaks were detected, indicating the consumption of taxifolin as a consequence of ErCHI heterologous expression in this strain.
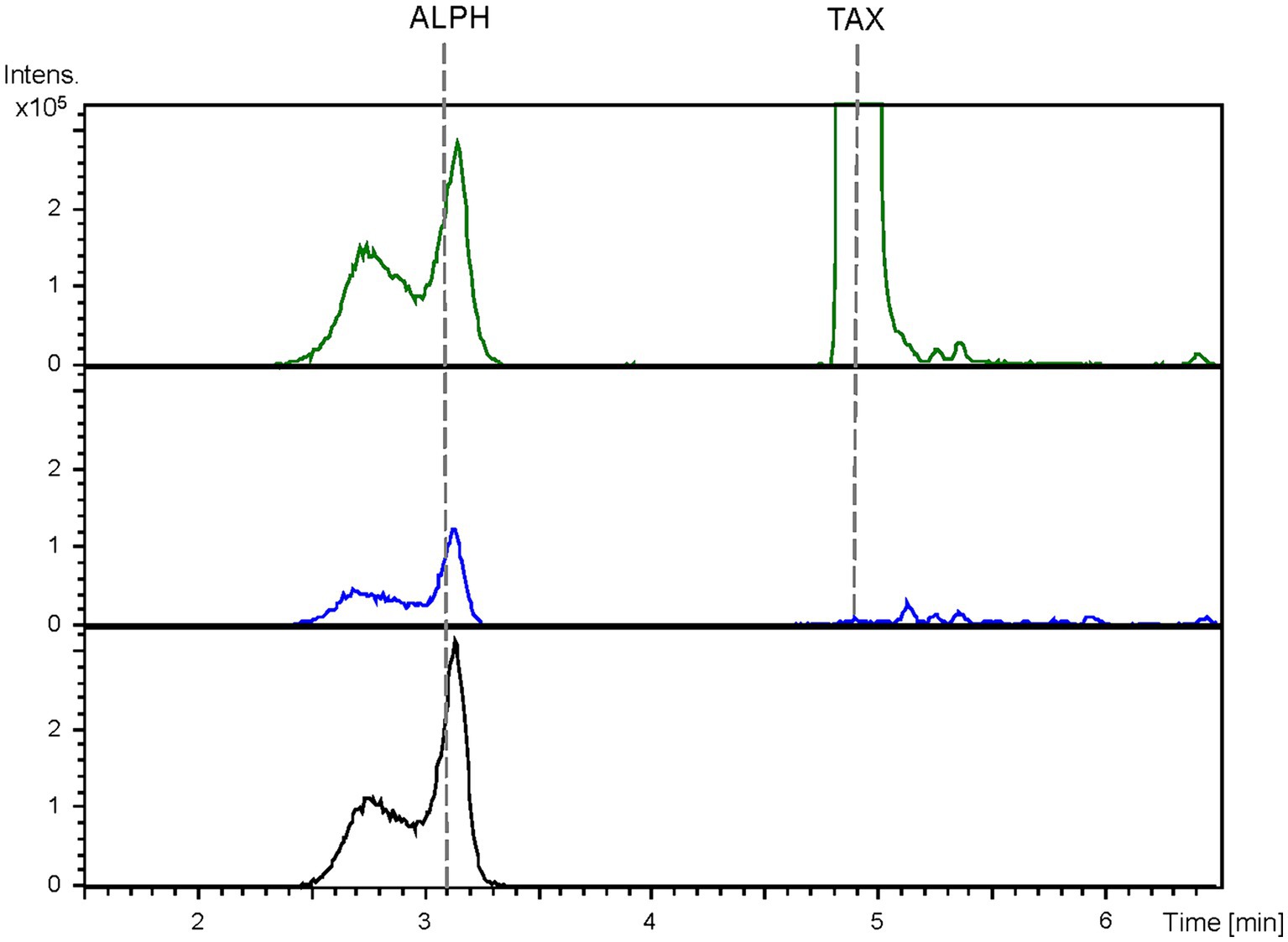
Figure 4. HPLC-HRESIMS chromatograms of control strain S. albidoflavus UO-FLAV-004-PcTAX (green) and the alphitonin de novo producer strain S. albidoflavus UO-FLAV-004-ALPH (blue). Extracted ion chromatograms for taxifolin and alphitonin. Alphitonin commercial standard (black). TAX, taxifolin; ALPH, alphitonin.
To investigate this matter, an initial examination of alphitonin stability under our specified culture conditions was undertaken. In these experiments, fresh culture medium devoid of bacterial presence was supplemented with 50 μM of commercial alphitonin. Subsequently, it was subjected to incubation conditions identical to those employed in the production cultures, and the concentration of alphitonin was monitored at time intervals of 0, 4, and 24 h. The outcomes of this investigation confirmed the spontaneous degradation of alphitonin in culture medium, as evidenced by a progressive decline in alphitonin concentrations over time, ultimately culminating in its complete disappearance after 24 h of incubation (Supplementary Figure S2).
Subsequent stability assays aimed to mitigate alphitonin decomposition during culture were conducted. These efforts encompassed attempts to enhance alphitonin stability by altering the extraction conditions, specifically by employing higher concentrations of formic acid (0.1, 0.2, and 0.5%) in the organic solvents. Additionally, the supplementation of vitamin C (0.5 mM and 5 mM) was explored as a potential stabilizer in the NL333 medium (Zhang et al., 2022). Furthermore, investigations involved incubation of alphitonin in different media (R5A, TSB, and LB) and modifying the pH of NL333 medium from 7.2 to 6.8 (Zhang et al., 2022). Regrettably, none of these strategies yielded improved recoveries of alphitonin, as the results did not show any significant enhancement (data not shown).
3.4 Conversion of taxifolin and aromadendrin into alphitonin and maesopsin, respectively, by an ErCHI-expressing Streptomyces albidoflavus strain
Given the established instability of alphitonin under our culture conditions, an inquiry arose regarding whether the absence of discernible distinctions between the alphitonin-producing strain and the control strain, in relation to the alphitonin peak, could be attributed more to the alphitonin decomposition rather than its non-conversion. In pursuit of clarifying this matter, an experimental approach was employed. Specifically, a strain of S. albidoflavus carrying only the ErCHI transcription unit, designated as strain S. albidoflavus UO-FLAV-004-ErCHI, was utilized.
To elucidate the matter, strain S. albidoflavus UO-FLAV-004-ErCHI was employed in feeding cultures with a 100 μM concentration of taxifolin. As negative controls, cultures of the same strain were performed without taxifolin supplementation, and the strain S. albidoflavus UO-FLAV-004 was also cultured under both conditions. Samples were collected at designated time points of 0, 0.5, 1.5, 4, and 24 h post-feeding, promptly subjected to extraction, and subsequently analyzed using HPLC-DAD.
In the resulting UV chromatograms, a distinctive peak corresponding to alphitonin (rt: 13.1 min) was consistently identified subsequent to taxifolin feeding in all samples derived from the S. albidoflavus UO-FLAV-004-ErCHI strain (Figure 5). This alphitonin peak exhibited a maximal conversion rate of 74.6% from the total taxifolin provided at time 0 h, with declining concentrations at subsequent time points: 63.4, 59.5, 46.3, and 27% at 0.5, 1.5, 4, and 24 h, respectively. No remaining taxifolin was detected in any of the samples obtained from the strain S. albidoflavus UO-FLAV-004-ErCHI after the feeding. Conversely, in all samples overtime from the strain S. albidoflavus UO-FLAV-004, taxifolin (rt: 19.6 min) was found to accumulate, with no alphitonin production (Figure 5). These findings indicate the immediate conversion of taxifolin into alphitonin catalyzed by ErCHI, followed by rapid degradation of alphitonin within the culture.
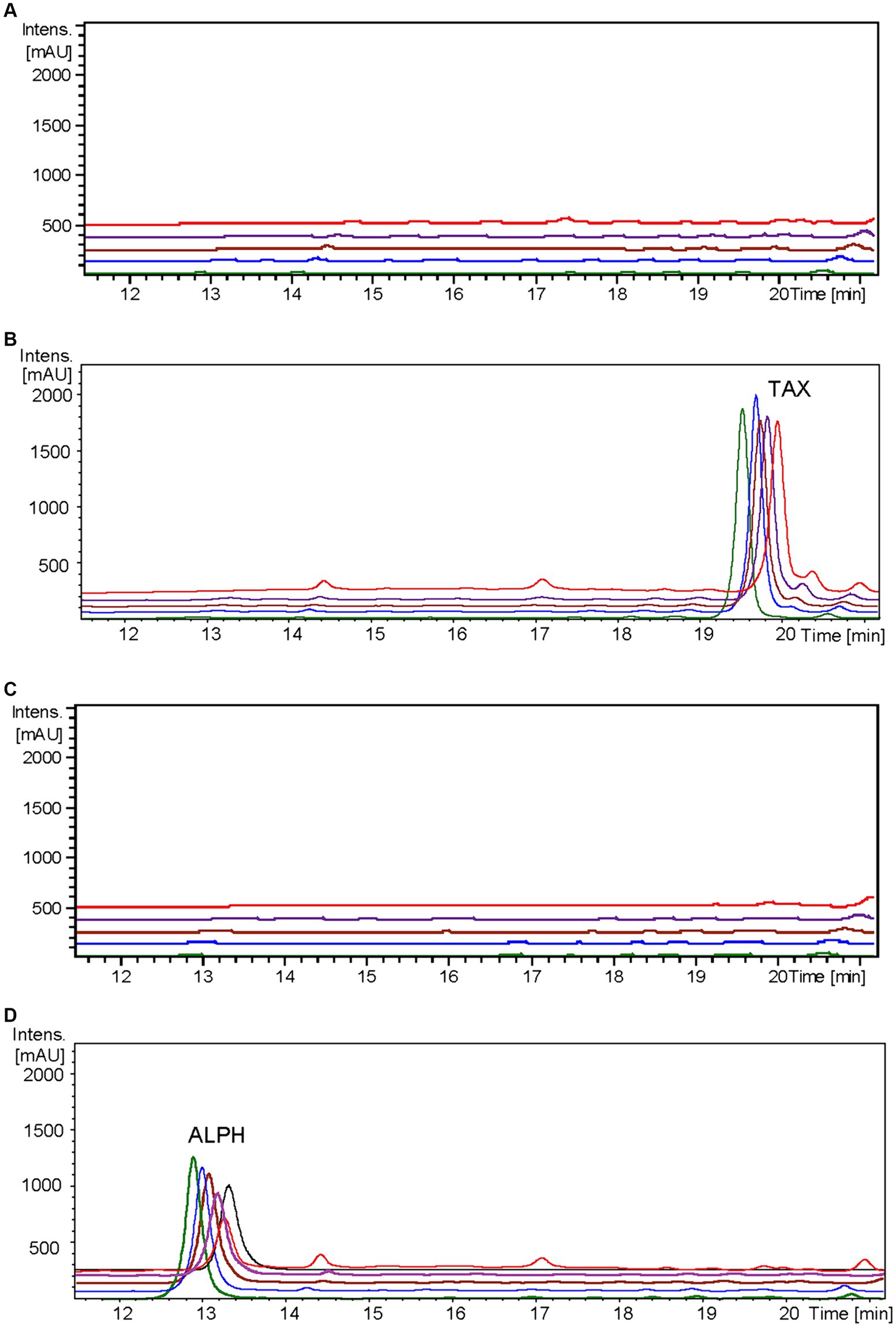
Figure 5. HPLC-DAD chromatograms at 279–281 nm of the time course for alphitonin biosynthesis after taxifolin feeding to S. albidoflavus UO-FLAV-004-ErCHI strain. (A) UO-FLAV-004 with DMSO, (B) UO-FLAV-004 with 100 μM taxifolin feeding, (C) UO-FLAV-004-ErCHI strain with DMSO, and (D) UO-FLAV-004-ErCHI with 100 μM taxifolin feeding. Samples are taken at 0 h (green), 0.5 h (blue), 1.5 h (brown), 4 h (purple), and 24 h (red) post-feeding. Alphitonin commercial standard (black). ALPH, alphitonin; TAX, taxifolin.
Significantly, when samples from the control conditions were subjected to HPLC-HRESIMS analysis, the peak detected in the previous section of this study in both the control and the alphitonin-producing strains, coeluting with alphitonin standard, was conspicuously absent (Supplementary Figure S3). The potential identity of this peak will be discussed further in the subsequent sections.
It has been previously documented that ErCHI has the capacity to catalyze reactions involving (+)-aromadendrin, yielding the auronol maesopsin (Braune et al., 2016). Consequently, an analogous experiment was conducted, but with the addition of 100 μM of aromadendrin to the cultures. In this investigation, a distinct peak corresponding to maesopsin (rt: 16.4 min) was consistently identified following aromadendrin supplementation in all samples derived from the S. albidoflavus UO-FLAV-004-ErCHI strain (Figure 6). Maesopsin production reached a maximum conversion rate of 38.1% at time 0 h, with subsequent reductions in maesopsin concentrations observed over time: 32.2, 26.8, 19.3, and 11.7% at 0.5, 1.5, 4, and 24 h, respectively. However, in this particular instance, aromadendrin (rt: 22.4 min) was detected in all samples from the S. albidoflavus UO-FLAV-004-ErCHI strain following the feeding experiment (Figure 6). This represented 44, 43.7, 43, 42.6, and 41% of the unutilized precursor. Consequently, ErCHI expressed in S. albidoflavus demonstrated the capability to convert (+)-aromadendrin into maesopsin, albeit with a lesser degree of efficiency compared to the conversion of taxifolin into alphitonin.
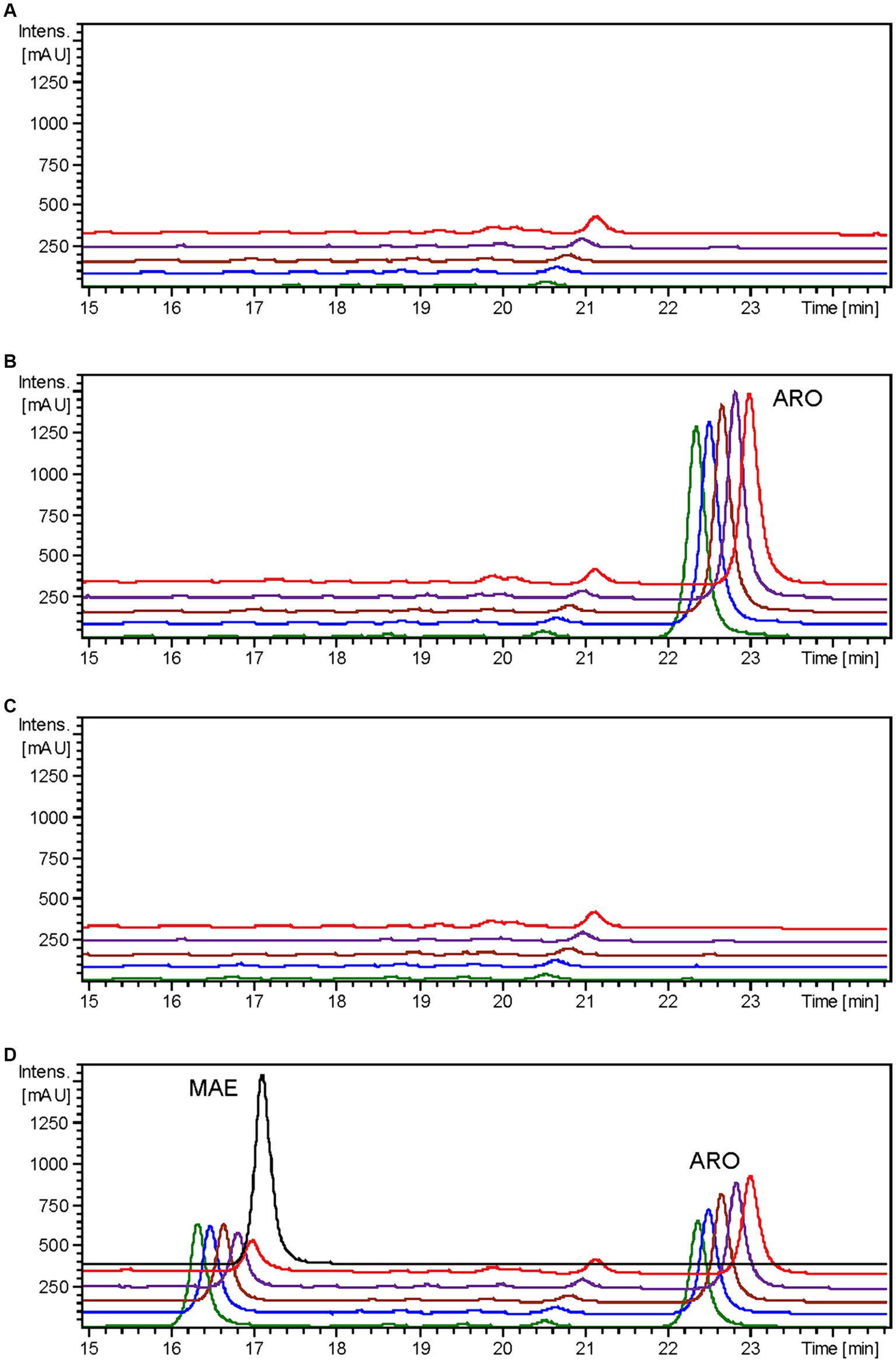
Figure 6. HPLC-DAD chromatograms at 279–281 nm of the time course for maesopsin biosynthesis after aromadendrin feeding to S. albidoflavus UO-FLAV-004-ErCHI strain. (A) UO-FLAV-004 with DMSO, (B) UO-FLAV-004 with 100 μM aromadendrin feeding, (C) UO-FLAV-004-ErCHI strain with DMSO, and (D) UO-FLAV-004-ErCHI with 100 μM aromadendrin feeding. Samples are taken at 0 h (green), 0.5 h (blue), 1.5 h (brown), 4 h (purple), and 24 h (red) post-feeding. Maesopsin commercial standard (black). MAE, maesopsin; ARO, aromadendrin.
3.5 Streptomyces albidoflavus/Escherichia coli co-cultures for heterologous de novo alphitonin biosynthesis
In summary of the previous experiments conducted in this study, it was observed that the de novo alphitonin generated by a single bacterial strain underwent degradation within the culture before becoming detectable. To overcome this issue, a single input of taxifolin is needed, as this taxifolin is promptly transformed into alphitonin, only to be subsequently degraded at a rapid pace. Consequently, in order to facilitate de novo alphitonin production, a co-culture system was established. This system involved a taxifolin-producing strain of S. albidoflavus in conjunction with E. coli JM109 pTI2 strain, the latter carrying the ErCHI gene (Braune et al., 2016).
The selection of the S. albidoflavus UO-FLAV-004-CsTAX strain for the co-culture was based on its demonstrated proficiency as an early-stage taxifolin producer (within 2 days) with minimal accumulation of the precursor eriodictyol. This choice was made considering that eriodictyol competes with taxifolin for binding to the active site of ErCHI enzyme. Both the S. albidoflavus UO-FLAV-004-CsTAX strain and the E. coli JM109 pTI2 strain were cultured separately and subsequently combined in a single flask, as detailed in the Materials and Methods section. Samples for production analysis were obtained from individual cultures as controls and immediately following the establishment of the co-culture, as the available taxifolin was promptly converted into alphitonin.
The extracted samples were subjected to analysis using HPLC-HRESIMS. BPCs were extracted for the mass peak corresponding to taxifolin and alphitonin. In the case of the strain S. albidoflavus UO-FLAV-004-CsTAX cultivated as a single culture, the taxifolin concentration reached 1.4 ± 0.3 mg/L, while alphitonin production was not detected (Figure 7). However, upon the introduction of the E. coli strain into the culture and subsequent immediate sampling, taxifolin became undetectable, and alphitonin was identified at a concentration of 1.9 ± 0.7 mg/L (Figure 7). This outcome unequivocally confirmed the conversion of taxifolin into alphitonin.
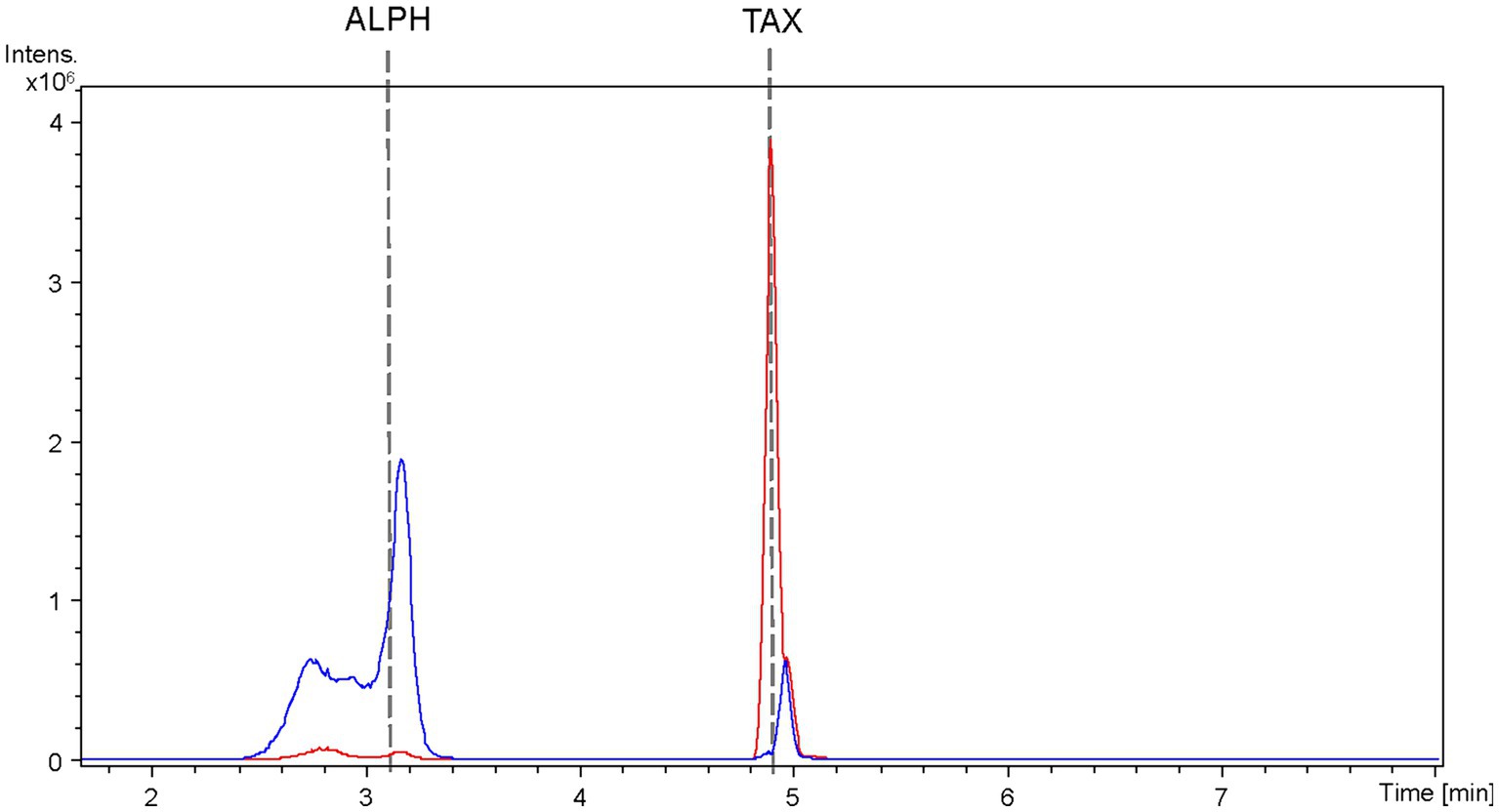
Figure 7. HPLC-HRESIMS extracted ion chromatograms of samples from the co-culture between the taxifolin producer strain S. albidoflavus UO-FLAV-004-CsF3H and the E. coli ErCHI-expressing strain JM109 pTI2, for the de novo alphitonin biosynthesis. Samples were taken from the S. albidoflavus culture before establishing the co-culture (red) and immediately after (blue) and chromatograms extracted for taxifolin and alphitonin m/z. TAX, taxifolin; ALPH: alphitonin.
4 Discussion
In previous investigations, the de novo biosynthesis of several flavonoids originating from naringenin in S. albidoflavus has been established (Marín et al., 2017, 2018, 2021; Pérez-Valero et al., 2023). In the present study, a genetically engineered S. albidoflavus J1074 strain, optimized for the production of 3.4 mg/L of naringenin (designated as S. albidoflavus UO-FLAV-004-NAR) (Pérez-Valero et al., 2023), was utilized, alongside a modular cloning platform. Consequently, this approach led to enhancements in the de novo synthesis of aromadendrin and taxifolin, as well as the production of two auronols, maesopsin and alphitonin, with the latter being produced de novo as well.
In this study, the reconstruction of the biosynthesis pathway for the flavanonols aromadendrin and taxifolin was undertaken in Streptomyces albidoflavus J1074 (Figure 1). During the course of this investigation, it was observed that both the enzymes, F3H and the chimeric enzyme F3’H/CPR, exhibited substrate flexibility. Consequently, it was demonstrated the capacity to produce taxifolin via two distinct enzymatic pathways, contingent upon the order of enzymatic reactions. In the primary pathway, the chimeric F3’H/CPR catalyzes the initial conversion of naringenin to eriodictyol, followed by the subsequent hydroxylation of eriodictyol by F3H to generate taxifolin. Conversely, in the secondary route, F3H catalyzes the initial conversion of naringenin to aromadendrin, which is then further converted into taxifolin by F3’H/CPR (Figure 1). This dual pathway arrangement allows for the maximization of taxifolin production.
The chimeric enzyme F3’H/CPR demonstrates high efficiency when acting upon both substrates, as evidenced by the absence of naringenin or aromadendrin accumulation in the cultures of the taxifolin-producing strain (Figure 3A). Nonetheless, our findings establish that the enzyme F3H serves as the primary limiting factor in the production of aromadendrin and taxifolin. This conclusion is affirmed by the substantial accumulation of precursor compounds, namely naringenin and eriodictyol, detected in the strains engineered for aromadendrin and taxifolin production, respectively (Figures 2A, 3A).
In an effort to enhance the production of aromadendrin and taxifolin, two additional F3Hs sourced from Malus domestica (MdF3H) and Camellia sinensis (CsF3H) were assessed for their potential to exhibit increased activity and reduced 2-hydroxylase side activity. In the context of aromadendrin production, both CsF3H and MdF3H demonstrated a similarly enhanced performance when acting upon naringenin, with MdF3H outperforming PcF3H by yielding a 13-fold greater quantity of aromadendrin (Figure 2B).
Notwithstanding the improvements achieved in aromadendrin production, F3H still emerged as the limiting factor, as evidenced by the accumulation of naringenin and 2-hydroxynaringenin. Therefore, further efforts would be needed to optimize F3H functionality. It has been previously documented in the literature that F3H activity has evolved from flavonol synthase (FLS) in plants, potentially through a transitional phase involving bifunctional FLS/F3H intermediate, concurrently with the existence of bifunctional FLS/F2H intermediate (Li et al., 2020). Studies have reported on site-directed mutagenesis efforts targeting key amino acids within canonical FLS, F3H, and bifunctional enzymes, leading to shifts from F3H activity to either F2H or FLS activities (Li et al., 2020; Fu et al., 2023). Numerous other approaches have been explored, involving site-directed mutagenesis that resulted in alterations in catalytic efficiency, substrate affinity, or regio/stereoselectivity (Choi et al., 2015; Zhou et al., 2022; Sui et al., 2023; Xu et al., 2023).
In this context, it is advisable to conduct structural studies at the protein level focusing on F3H, with the aim of identifying critical amino acid residues that can be modified to enhance F3H activity while concurrently diminishing its F2H side activity. Such modifications would hold promise for future applications in the biosynthesis of flavonoids.
In the context of taxifolin production, the strain S. albidoflavus UO-FLAV-004-CsTAX, carrying the CsF3H enzyme, generated 1.7 ± 0.2 mg/L of taxifolin. This quantity represented a 2.4-fold increase in taxifolin production compared to its counterpart harboring the PcF3H enzyme, and this difference was observed after a cultivation period of 2 days (Figure 3C). Conversely, the strain S. albidoflavus UO-FLAV-004-PcTAX yielded 2.1 ± 0.1 mg/L of taxifolin after an additional 3 days of cultivation, marking the highest recorded production of taxifolin de novo in the course of this study (Figure 3C). Therefore, while there was no enhancement in taxifolin production, the screening of F3H enzymes proved valuable in reducing the cultivation time and precursor accumulation, while maintaining comparable final levels of taxifolin production.
Given the observed trends of taxifolin degradation subsequent to the complete consumption of precursors, coupled with the achievement of maximum taxifolin yield in strain S. albidoflavus UO-FLAV-004-CsTAX (Figure 3C), and the challenges encountered in de novo alphitonin production using a single strain (Figure 4), an investigation into the stability of these target compounds within the culture environment was conducted. Experiments involving the addition of taxifolin to both seeded and unseeded cultures revealed that, unlike auronols, taxifolin exhibited stability under the culture conditions, however, it was susceptible to conversion or degradation by S. albidoflavus cells (Supplementary Figure S1). Nevertheless, this issue was successfully mitigated by optimizing the cultivation duration, which varied depending on the specific F3H variant employed (Figure 3C). In contrast, auronols exhibited pronounced instability within cell-free culture conditions (Supplementary Figure S2).
Confirmation of the conversion of aromadendrin into maesopsin and taxifolin into alphitonin by ErCHI was obtained through feeding experiments conducted on a strain of S. albidoflavus exclusively expressing the ErCHI enzyme. These experiments provided evidence of the immediate conversion of both precursor compounds into their respective auronols, subsequently followed by the rapid degradation of these auronols (Figures 5, 6). Furthermore, the results from these experiments indicated that a S. albidoflavus strain expressing ErCHI displayed greater efficiency in converting taxifolin into alphitonin compared to aromadendrin conversion into maesopsin. Specifically, when equal amounts (100 μM) of the respective substrate were introduced into the culture, ErCHI achieved a 74.6% conversion of taxifolin into alphitonin, whereas only 38.1% of aromadendrin was transformed into maesopsin. This finding aligns with earlier findings involving cell-free extracts of ErCHI expressed in E. coli, which demonstrated 60% activity with aromadendrin in comparison to taxifolin (Braune et al., 2016). ErCHI exhibited its highest activity within very short and early time frames, with maximum activity observed at the time of substrate feeding and no further activity subsequently recorded. This was evidenced by the complete conversion of taxifolin immediately after feeding (Figure 5) and the consistent presence of essentially the same quantity of unconverted aromadendrin throughout the corresponding experiment (Figure 6).
As all the attempts to stabilize alphitonin through modifications in culture and extraction conditions proved unsuccessful, a co-culture strategy was implemented between the strain S. albidoflavus UO-FLAV-004-CsTAX and an E. coli strain expressing the ErCHI (Braune et al., 2016). This approach ultimately yielded successful results, achieving de novo production of alphitonin at a concentration of 1.9 ± 0.7 mg/L, with no accumulation of its precursor, (+)-taxifolin (Figure 7). Optimization in the selection of the F3H enzyme and culture duration ensured a substantial taxifolin supply with negligible precursor accumulation (57.5-fold lower eriodictyol accumulation compared to its counterpart PcF3H) (Figure 3C). Consequently, the absence of the precursor prevented inhibition of ErCHI during the catalysis of taxifolin conversion into alphitonin.
For maesopsin, the limited titers of aromadendrin generated by the respective producer strains, coupled with precursor accumulation resulting from diminished F3H activity, will require further improvement at the enzyme level prior to embarking on the de novo production attempts.
Finally, it is pertinent to acknowledge and provide a plausible rationale for the conspicuous peak observed in Figure 4 and again in Figure 7, which co-elutes with alphitonin standard in the control taxifolin producer strain. Considering the previously reported F2H side activity of F3H (Marín et al., 2021), a product generated from naringenin associated with this activity was detected in the aromadendrin producer strain, leading to the production of the derivative 2-hydroxynaringenin (Figures 1, 2A). Notably, the 2-hydroxynaringenin peak was absent in samples from the control S. albidoflavus UO-FLAV-004-NAR-M22106 strain (Figure 2A), which lacks F3H. Its identity was further confirmed through comparison with a pure commercial standard of 2-hydroxynaringenin. Intriguingly, both 2-hydroxynaringenin and maesopsin standards exhibited co-elution in both HPLC-HRESIMS and HPLC-DAD analyses, and they shared identical m/z, MS/MS, and UV/Vis spectra (Supplementary Figure S4).
In light of these findings, it is proposed that in the taxifolin producer strain, the F2H side activity associated with F3H may also act upon eriodictyol, leading to the formation of a corresponding 2-hydroxylated intermediate compound, 2-hydroxyeriodictyol (Figure 1). This side intermediate compound would co-elute with alphitonin, mirroring the co-elution of 2-hydroxynaringenin with maesopsin. Unfortunately, a 2-hydroxyeriodictyol standard is not commercially available. However, the observation that these peaks, corresponding to 2-hydroxynaringenin and 2-hydroxyeriodictyol, exclusively appear in chromatograms when F3H and the corresponding precursor (naringenin and/or eriodictyol) are present strongly supports this hypothesis. Consequently, this unequivocally associates this activity with the F3H enzyme (Supplementary Figure S5).
In this study, we have comprehensively addressed the significance of culture time optimization and enzyme selection based on the desired final product. Effective optimization strategies were employed to achieve complete consumption of precursors during the production of taxifolin and alphitonin. Nevertheless, additional optimization methodologies need to be explored to maximize the production of aromadendrin and maesopsin, particularly in light of the limited efficiency exhibited by F3H and ErCHI enzymes for their respective substrates. Moreover, this study represents the first report of de novo heterologous biosynthesis of alphitonin accomplished through the judicious selection of optimal F3H enzyme and the establishment of a co-culture between S. albidoflavus J1074 and E. coli, owing to the observed high instability of this compound in cell-free culture.
Data availability statement
The datasets presented in this study can be found in online repositories. The names of the repository/repositories and accession number(s) can be found in the article/Supplementary material.
Author contributions
PM-C: Investigation, Writing – original draft. SY: Supervision, Writing – review & editing. AB: Writing – review & editing. CV: Supervision, Writing – review & editing. FL: Funding acquisition, Supervision, Writing – review & editing.
Funding
The author(s) declare that financial support was received for the research, authorship, and/or publication of this article. This research was funded by Principado de Asturias (Spain) through the program “Ayudas a organismos públicos para apoyar las actividades de I+D+I de sus grupos de investigación” (grant AYUD/2021/51347), as well as by “Programa Severo Ochoa de Ayudas Predoctorales para la investigación y docencia” from Principado de Asturias (grant PA-20-PF-BP19-058 to PM-C), the research project PID2021-127812OB-I00 from MICINN (Spanish Ministry of Science and Innovation), and the European Union’s Horizon 2020 Research and Innovation Program under Grant Agreement no. 814650 for the project SynBio4Flav.
Acknowledgments
Authors thank Explora Biotech SrL (Venice, Italy) for providing the synthetic genes, and Extrasynthese (Genay, France) for providing flavonoid pure standards. Authors thank Servicios Científico-Técnicos at the University of Oviedo (HPLC-HRESIMS Unit) for exact mass analyses.
Conflict of interest
The authors declare that the research was conducted in the absence of any commercial or financial relationships that could be construed as a potential conflict of interest.
Publisher’s note
All claims expressed in this article are solely those of the authors and do not necessarily represent those of their affiliated organizations, or those of the publisher, the editors and the reviewers. Any product that may be evaluated in this article, or claim that may be made by its manufacturer, is not guaranteed or endorsed by the publisher.
Supplementary material
The Supplementary material for this article can be found online at: https://www.frontiersin.org/articles/10.3389/fmicb.2024.1378235/full#supplementary-material
References
Amić, A., and Mastiľák Cagardová, D. (2023). A DFT study on the kinetics of HOO•, CH3OO•, and O2•− scavenging by quercetin and flavonoid Catecholic metabolites. Antioxidants 12:1154. doi: 10.3390/antiox12061154
Bernatova, I., and Liskova, S. (2021). Mechanisms modified by (−)-Epicatechin and Taxifolin relevant for the treatment of hypertension and viral infection: knowledge from preclinical studies. Antioxidants 10:467. doi: 10.3390/antiox10030467
Blázquez, B., León, D. S., Torres-Bacete, J., Gómez-Luengo, Á., Kniewel, R., Martínez, I., et al. (2023). Golden standard: a complete standard, portable, and interoperative MoClo tool for model and non-model proteobacteria. Nucleic Acids Res. 51:e98. doi: 10.1093/nar/gkad758
Braune, A., Engst, W., Elsinghorst, P. W., Furtmann, N., Bajorath, J., Gütschow, M., et al. (2016). Chalcone isomerase from Eubacterium ramulus catalyzes the ring contraction of Flavanonols. J. Bacteriol. 198, 2965–2974. doi: 10.1128/JB.00490-16
Braune, A., Gütschow, M., Engst, W., and Blaut, M. (2001). Degradation of quercetin and Luteolin by Eubacterium ramulus. Appl. Environ. Microbiol. 67, 5558–5567. doi: 10.1128/AEM.67.12.5558-5567.2001
Chen, S., Wang, X., Cheng, Y., Gao, H., and Chen, X. (2023). A review of classification, biosynthesis, biological activities and potential applications of flavonoids. Molecules 28:4982. doi: 10.3390/molecules28134982
Cheng, A.-X., Han, X.-J., Wu, Y.-F., and Lou, H.-X. (2014). The function and catalysis of 2-Oxoglutarate-dependent Oxygenases involved in plant flavonoid biosynthesis. Int. J. Mol. Sci. 15, 1080–1095. doi: 10.3390/ijms15011080
Choi, K.-Y., Yang, Y.-H., and Kim, B. (2015). Regioselectivity-driven evolution of CYP102D1 for improved synthesis of 3′-ortho-dihydroxyisoflavone. Enzym. Microb. Technol. 71, 20–27. doi: 10.1016/j.enzmictec.2015.01.004
Cui, S., Cui, Y., Li, Y., Zhang, Y., Wang, H., Qin, W., et al. (2018). Inhibition of cardiac hypertrophy by aromadendrin through down-regulating NFAT and MAPKs pathways. Biochem. Biophys. Res. Commun. 506, 805–811. doi: 10.1016/j.bbrc.2018.10.143
Das, A., Baidya, R., Chakraborty, T., Samanta, A. K., and Roy, S. (2021). Pharmacological basis and new insights of taxifolin: a comprehensive review. Biomed. Pharmacother. 142:112004. doi: 10.1016/j.biopha.2021.112004
Dias, M. C., Pinto, D. C. G. A., and Silva, A. M. S. (2021). Plant flavonoids: chemical characteristics and biological activity. Molecules 26:5377. doi: 10.3390/molecules26175377
Elsinghorst, P. W., Cavlar, T., Müller, A., Braune, A., Blaut, M., and Gütschow, M. (2011). The thermal and enzymatic Taxifolin–Alphitonin rearrangement. J. Nat. Prod. 74, 2243–2249. doi: 10.1021/np200639s
Espírito-Santo, D. A., Cordeiro, G. S., Santos, L. S., Silva, R. T., Pereira, M. U., Matos, R. J. B., et al. (2023). Cardioprotective effect of the quercetin on cardiovascular remodeling and atherosclerosis in rodents fed a high-fat diet: a systematic review. Chem. Biol. Interact. 384:110700. doi: 10.1016/j.cbi.2023.110700
Fernández, E., Weißbach, U., Reillo, C. S., Braña, A. F., Méndez, C., Rohr, J., et al. (1998). Identification of two genes from Streptomyces argillaceus encoding glycosyltransferases involved in transfer of a disaccharide during biosynthesis of the antitumor drug mithramycin. J. Bacteriol. 180, 4929–4937. doi: 10.1128/JB.180.18.4929-4937.1998
Fu, J., Wang, P.-Y., Ni, R., Zhang, J.-Z., Zhu, T.-T., Tan, H., et al. (2023). Molecular identification of a flavone synthase I/flavanone 3β-hydroxylase bifunctional enzyme from fern species Psilotum nudum. Plant Sci. 329:111599. doi: 10.1016/j.plantsci.2023.111599
Gao, S., Zhou, J., and Chen, J. (2020). Identification of flavonoids 3-hydroxylase from [Silybum marianum (L.) Gaertn] and its application in enhanced production of taxifolin. Sheng Wu Gong Cheng Xue Bao 36, 2838–2849. doi: 10.13345/j.cjb.200178
Huang, H., Wei, S., Wu, X., Zhang, M., Zhou, B., Huang, D., et al. (2023). Dihydrokaempferol attenuates CCl4-induced hepatic fibrosis by inhibiting PARP-1 to affect multiple downstream pathways and cytokines. Toxicol. Appl. Pharmacol. 464:116438. doi: 10.1016/j.taap.2023.116438
Hwang, S., Lee, Y., Kim, J. H., Kim, G., Kim, H., Kim, W., et al. (2021). Streptomyces as microbial chassis for heterologous protein expression. Front. Bioeng. Biotechnol. 9:804295. doi: 10.3389/fbioe.2021.804295
Kieser, T., Bibb, M. J., Buttner, M. J., Chater, K. F., and Hopwood, D. A. (2000). Practical Streptomyces genetics, John Innes Foundation, Norwich. Available at: https://www.scirp.org/(S(351jmbntvnsjt1aadkposzje))/reference/referencespapers.aspx?referenceid=578365(Accessed April 18, 2023).
Kim, T. H., Lee, J., Kim, H.-J., and Jo, C. (2017). Plasma-induced degradation of quercetin associated with the enhancement of biological activities. J. Agric. Food Chem. 65, 6929–6935. doi: 10.1021/acs.jafc.7b00987
Kumar, S., and Pandey, A. K. (2013). Chemistry and biological activities of flavonoids: an overview. Sci. World J. 2013, 1–16. doi: 10.1155/2013/162750
Lee, J.-W., Kim, N. H., Kim, J.-Y., Park, J.-H., Shin, S.-Y., Kwon, Y.-S., et al. (2013). Aromadendrin inhibits lipopolysaccharide-induced nuclear translocation of NF-κB and phosphorylation of JNK in RAW 264.7 macrophage cells. Biomol. Ther. (Seoul) 21, 216–221. doi: 10.4062/biomolther.2013.023
Leonard, E., Yan, Y., and Koffas, M. (2006). Functional expression of a P450 flavonoid hydroxylase for the biosynthesis of plant-specific hydroxylated flavonols in Escherichia coli. Metab. Eng. 8, 172–181. doi: 10.1016/j.ymben.2005.11.001
Li, D.-D., Ni, R., Wang, P.-P., Zhang, X.-S., Wang, P.-Y., Zhu, T.-T., et al. (2020). Molecular basis for chemical evolution of flavones to Flavonols and anthocyanins in land plants. Plant Physiol. 184, 1731–1743. doi: 10.1104/pp.20.01185
Liu, Y., Shi, X., Tian, Y., Zhai, S., Liu, Y., Xiong, Z., et al. (2023). An insight into novel therapeutic potentials of taxifolin. Front. Pharmacol. 14:1173855. doi: 10.3389/fphar.2023.1173855
Lv, Y., Edwards, H., Zhou, J., and Xu, P. (2019). Combining 26s rDNA and the Cre-lox P system for iterative gene integration and efficient marker curation in Yarrowia lipolytica. ACS Synth. Biol. 8, 568–576. doi: 10.1021/acssynbio.8b00535
Magadán-Corpas, P., Ye, S., Pérez-Valero, Á., McAlpine, P. L., Valdés-Chiara, P., Torres-Bacete, J., et al. (2023). Optimized De novo Eriodictyol biosynthesis in Streptomyces albidoflavus using an expansion of the Golden standard toolkit for its use in Actinomycetes. Int. J. Mol. Sci. 24:8879. doi: 10.3390/ijms24108879
Maleki, S. J., Crespo, J. F., and Cabanillas, B. (2019). Anti-inflammatory effects of flavonoids. Food Chem. 299:125124. doi: 10.1016/j.foodchem.2019.125124
Marín, L., Gutiérrez-del-Río, I., Entrialgo-Cadierno, R., Villar, C. J., and Lombó, F. (2018). De novo biosynthesis of myricetin, kaempferol and quercetin in Streptomyces albus and Streptomyces coelicolor. PLoS One 13:e0207278. doi: 10.1371/journal.pone.0207278
Marín, L., Gutiérrez-del-Río, I., Villar, C. J., and Lombó, F. (2021). De novo biosynthesis of garbanzol and fustin in Streptomyces albus based on a potential flavanone 3-hydroxylase with 2-hydroxylase side activity. Microb. Biotechnol. 14, 2009–2024. doi: 10.1111/1751-7915.13874
Marín, L., Gutiérrez-del-Río, I., Yagüe, P., Manteca, Á., Villar, C. J., and Lombó, F. (2017). De novo biosynthesis of Apigenin, Luteolin, and Eriodictyol in the Actinomycete Streptomyces albus and production improvement by feeding and spore conditioning. Front. Microbiol. 8:921. doi: 10.3389/fmicb.2017.00921
Myronovskyi, M., Tokovenko, B., Brötz, E., Rückert, C., Kalinowski, J., and Luzhetskyy, A. (2014). Genome rearrangements of Streptomyces albus J1074 lead to the carotenoid gene cluster activation. Appl. Microbiol. Biotechnol. 98, 795–806. doi: 10.1007/s00253-013-5440-6
Park, S. R., Ahn, M. S., Han, A. R., Park, J. W., and Yoon, Y. J. (2011). Enhanced flavonoid production in Streptomyces venezuelae via metabolic engineering. J. Microbiol. Biotechnol. 21, 1143–1146. doi: 10.4014/jmb.1108.08012
Park, S. R., Paik, J. H., Ahn, M. S., Park, J. W., and Yoon, Y. J. (2010). Biosynthesis of plant-specific flavones and Flavonols in Streptomyces venezuelae. J. Microbiol. Biotechnol. 20, 1295–1299. doi: 10.4014/jmb.1005.05038
Park, S. R., Yoon, J. A., Paik, J. H., Park, J. W., Jung, W. S., Ban, Y.-H., et al. (2009). Engineering of plant-specific phenylpropanoids biosynthesis in Streptomyces venezuelae. J. Biotechnol. 141, 181–188. doi: 10.1016/j.jbiotec.2009.03.013
Pérez-Valero, Á., Ye, S., Magadán-Corpas, P., Villar, C. J., and Lombó, F. (2023). Metabolic engineering in Streptomyces albidoflavus for the biosynthesis of the methylated flavonoids sakuranetin, acacetin, and genkwanin. Microb. Cell Factories 22:234. doi: 10.1186/s12934-023-02247-3
Pozzesi, N., Pierangeli, S., Vacca, C., Falchi, L., Pettorossi, V., Martelli, M. P., et al. (2011). Maesopsin 4-O-β-D-glucoside, a natural compound isolated from the leaves of Artocarpus tonkinensis, inhibits proliferation and up-regulates HMOX1, SRXN1 and BCAS3 in acute myeloid leukemia. J. Chemother. 23, 150–157. doi: 10.1179/joc.2011.23.3.150
Redondo-Blanco, S., Fernández, J., Gutiérrez-del-Río, I., Villar, C. J., and Lombó, F. (2017). New insights toward colorectal cancer chemotherapy using natural bioactive compounds. Front. Pharmacol. 8:109. doi: 10.3389/fphar.2017.00109
Sui, S., Xie, K., Guo, R., Dai, J., and Yang, L. (2023). Molecular characterization of a Stereoselective and promiscuous flavanone 3-hydroxylase from Carthamus tinctorius L. J. Agric. Food Chem. 71, 1679–1689. doi: 10.1021/acs.jafc.2c07202
Thuy, T. T., Thien, D. D., Quang Hung, T., Tam, N. T., Anh, N. T. H., Nga, N. T., et al. (2016). In vivo anticancer activity of maesopsin 4- O - β -glucoside isolated from leaves of Artocarpus tonkinensis a. Chev. Ex Gagnep. Asian Pac J Trop Med 9, 351–356. doi: 10.1016/j.apjtm.2016.03.012
Wu, X., Liu, J., Liu, D., Yuwen, M., Koffas, M. A. G., and Zha, J. (2022). Biosynthesis of eriodictyol from tyrosine by Corynebacterium glutamicum. Microb. Cell Factories 21:86. doi: 10.1186/s12934-022-01815-3
Xu, P., Li, X., Fan, J., Tian, S., Cao, M., Lin, A., et al. (2023). An arginine-to-histidine mutation in flavanone-3-hydroxylase results in pink strawberry fruits. Plant Physiol. 193, 1849–1865. doi: 10.1093/plphys/kiad424
Yu, S., Li, M., Gao, S., and Zhou, J. (2022). Engineering Saccharomyces cerevisiae for the production of dihydroquercetin from naringenin. Microb. Cell Factories 21:213. doi: 10.1186/s12934-022-01937-8
Zhang, H. L., Wang, M. L., Yi, L. Z., Högger, P., Arroo, R., Bajpai, V. K., et al. (2022). Stability profiling and degradation products of dihydromyricetin in Dulbecco’s modified eagle’s medium. Food Chem. 378:132033. doi: 10.1016/j.foodchem.2021.132033
Keywords: aromadendrin, taxifolin, maesopsin, alphitonin, co-culture, flavanone 3-hydroxylase
Citation: Magadán-Corpas P, Ye S, Braune A, Villar CJ and Lombó F (2024) Optimization of flavanonols heterologous biosynthesis in Streptomyces albidoflavus, and generation of auronols. Front. Microbiol. 15:1378235. doi: 10.3389/fmicb.2024.1378235
Edited by:
Félix López De Felipe, Spanish National Research Council (CSIC), SpainReviewed by:
Eduardo Rodriguez, CONICET Instituto de Biología Molecular y Celular de Rosario (IBR), ArgentinaMaria Carolina Rodriguez Daza, Wageningen University and Research, Netherlands
Copyright © 2024 Magadán-Corpas, Ye, Braune, Villar and Lombó. This is an open-access article distributed under the terms of the Creative Commons Attribution License (CC BY). The use, distribution or reproduction in other forums is permitted, provided the original author(s) and the copyright owner(s) are credited and that the original publication in this journal is cited, in accordance with accepted academic practice. No use, distribution or reproduction is permitted which does not comply with these terms.
*Correspondence: Suhui Ye, yesuhui@uniovi.es; Felipe Lombó, lombofelipe@uniovi.es