- 1Department of Biology, American University, Washington, DC, United States
- 2Laboratory for Skin Research, Institute for Medical Research, Galilee Medical Center, Nahariya, Israel
- 3Division of Infectious Diseases, Department of Medicine, Brigham and Women’s Hospital, Harvard Medical School, Boston, MA, United States
- 4Kane Biotech Inc., Winnipeg, MB, Canada
Background: The commensal skin bacterium Cutibacterium acnes plays a role in the pathogenesis of acne vulgaris and also causes opportunistic infections of implanted medical devices due to its ability to form biofilms on biomaterial surfaces. Poly-β-(1→6)-N-acetyl-D-glucosamine (PNAG) is an extracellular polysaccharide that mediates biofilm formation and biocide resistance in a wide range of bacterial pathogens. The objective of this study was to determine whether C. acnes produces PNAG, and whether PNAG contributes to C. acnes biofilm formation and biocide resistance in vitro.
Methods: PNAG was detected on the surface of C. acnes cells by fluorescence confocal microscopy using the antigen-specific human IgG1 monoclonal antibody F598. PNAG was detected in C. acnes biofilms by measuring the ability of the PNAG-specific glycosidase dispersin B to inhibit biofilm formation and sensitize biofilms to biocide killing.
Results: Monoclonal antibody F598 bound to the surface of C. acnes cells. Dispersin B inhibited attachment of C. acnes cells to polystyrene rods, inhibited biofilm formation by C. acnes in glass and polypropylene tubes, and sensitized C. acnes biofilms to killing by benzoyl peroxide and tetracycline.
Conclusion: C. acnes produces PNAG, and PNAG contributes to C. acnes biofilm formation and biocide resistance in vitro. PNAG may play a role in C. acnes skin colonization, biocide resistance, and virulence in vivo.
Highlights
Cutibacterium acnes is a bacterium that is found on the skin of most people. C. acnes helps maintain a healthy skin microbiota but also causes acne and infections of implanted medical devices. In this study we found that C. acnes produces an adhesive extracellular polysaccharide named PNAG (poly-N-acetylglucosamine) which may help C. acnes colonize skin and medical implants. We found that PNAG protects C. acnes from killing by benzoyl peroxide and tetracycline, two drugs that are commonly used to treat acne. PNAG may represent a novel target for skin antiseptics and anti-acne drugs.
Introduction
The anaerobic Gram-positive bacterium Cutibacterium acnes is an abundant colonizer of human skin (Achermann et al., 2014). Although considered a beneficial commensal, C. acnes can cause opportunistic invasive infections of the skin, soft tissue, cardiovascular system, and implanted medical devices (Coenye et al., 2022). Cutibacterium acnes also contributes the pathogenesis of the common inflammatory dermatosis acne vulgaris (McLaughlin et al., 2019).
Poly-β-(1→6)-N-acetyl-D-glucosamine (PNAG) is an extracellular polysaccharide that mediates biofilm formation, antimicrobial resistance, host colonization, immune evasion, and stress tolerance in a wide range of Gram-negative and Gram-positive bacterial pathogens (Cywes-Bentley et al., 2013; Soliman et al., 2018). The importance of PNAG as a bacterial colonization and virulence factor has been demonstrated in numerous animal studies using PNAG mutant strains (Kropec et al., 2005; Subashchandrabose et al., 2013; Chen et al., 2014; Shanmugam et al., 2015), anti-PNAG monoclonal antibodies (Cywes-Bentley et al., 2013), a PNAG vaccine (Gening et al., 2020), and the PNAG-specific glycoside hydrolase dispersin B (Serrera et al., 2007; Darouiche et al., 2009; Ragunath et al., 2011; Gawande et al., 2014; Kaplan et al., 2018).
Previous investigations of PNAG production in C. acnes were inconclusive. Okuda et al. (2018) detected N-acetylglucosamine (GlcNAc) in the extracellular biofilm matrix of five C. acnes strains isolated from infected cardiac pacemakers using a wheat germ agglutinin dot blot assay, but dispersin B did not inhibit biofilm formation by any of the five strains in 96-well polystyrene microplates, an indicator of PNAG production. Gannesen et al. (2019) observed no nuclear magnetic resonance (NMR) spectroscopic signal for PNAG in the biofilm matrix of C. acnes strain RT5, an acne isolate. In the present study, we reexamined PNAG production in C. acnes using an anti-PNAG monoclonal antibody and the PNAG-degrading enzyme dispersin B. Here we present evidence that C. acnes produces PNAG in vitro, and that PNAG mediates C. acnes surface attachment, biofilm formation, and resistance to killing by the anti-acne agents benzoyl peroxide and tetracycline.
Materials and methods
Bacterial strains and growth conditions
The bacterial strains used in this study are listed in Table 1. Strains were maintained on Tryptic Soy agar (BD). Bacterial inocula for broth cultures were prepared by transferring a loopful of cells from a 24-h-old agar plate to a microcentrifuge tube containing 200 μL of saline, mixing the cells by vortex agitation, and diluting the cells to 106–107 CFU/mL in filter-sterilized Tryptic Soy broth (BD). Culture vessels were 13 × 100 mm glass tubes or 15-mL conical-bottom polypropylene centrifuge tubes. Tubes were filled with 1-mL of inoculum and incubated anaerobically at 37°C for 72 h. Anaerobic conditions were created using a BD GasPak EZ Anaerobe sachet system.
Antimicrobial agents and enzymes
Tetracycline hydrochloride (Sigma-Aldrich; Catalog No. T7660) was dissolved at 10 mg/mL in distilled water, filter sterilized, and diluted in broth to the indicated concentrations. Benzoyl peroxide (TCI Chemicals; Catalog No. B3152) was dissolved at 10 mg/mL in dimethyl sulfoxide and diluted directly in broth. Sodium dodecyl sulfate (SDS; Catalog No. 428018) was purchased from Merck. Deoxyribonuclease I (Catalog No. DN25) was from Sigma-Aldrich. Dispersin B was obtained from Kane Biotech (Winnipeg MB, Canada).
Fluorescence confocal microscopy
PNAG was detected on the surface of Cutibacterium spp. cells by fluorescence confocal microscopy using the PNAG-specific human IgG1 monoclonal antibody (mAb) F598 conjugated to Alexa Fluor 488 as previously described (Cywes-Bentley et al., 2013). Human IgG1 mAb F429, which binds to Pseudomonas aeruginosa alginate (Pier et al., 2004), was used as a negative control. Briefly, cells were swabbed from an agar plate onto glass microscope slides, air-dried, and covered for 1 min with ice-cold methanol. After rinsing, slides were reacted with 5.2 μg/mL mAb F598 or control mAb F429 directly conjugated to Alexa Fluor 488 along with 4 uM Syto 63 in BSA/PBS. After 2 h at room temperature, slides were washed and observed by confocal microscopy using a 63× oil objective.
Crystal violet binding assay
Biofilms cultured in glass tubes were rinsed vigorously with tap water and stained for 1 min with 1 mL of Gram’s crystal violet. Tubes were then rinsed with tap water to remove the unbound dye, air-dried, and photographed. Tubes containing sterile broth were incubated and processed along with the inoculated tubes to serve as controls. To quantitate crystal violet binding, stained tubes were filled with 1 mL of 33% acetic acid, incubated at room temperature for 30 min, and mixed by vortex agitation. A volume of 200 μL of the dissolved dye was transferred to the well of a 96-well microtiter plate and its absorbance at 595 nm (A595) was measured in a microplate reader. Biofilm inhibition by dispersin B was calculated using the formula 1 − (A595Dispersin B/A595No enzyme) × 100.
Surface attachment assay
Cutibacterium acnes cells were scraped from an agar plate and resuspended in PBS at ca. 106 CFU/mL. Cell suspensions were filtered through a 5-μm pore-size syringe filter to remove large clumps of cells and then aliquoted into three 15-mL centrifuge tubes (2.5 mL/tube). The first tube was left untreated to serve as a control. The second tube was supplemented with 20 μg/mL of dispersin B. The third tube was supplemented with 20 μg/mL of heat-inactivated dispersin B (95°C, 10 min). After 30 min at 37°C, the tubes were mixed by vortex agitation, and four 0.5-mL aliquots of each cell suspension were transferred to four separate 1.5-mL microcentrifuge tubes (0.5 mL/tube). A 25-mm long ethanol-sterilized polystyrene rod (1.5 mm diam; Plastruct Inc., Des Plaines IL, United States) was placed in each microcentrifuge tube. After 30 min, the rods were removed, rinsed with PBS to remove loosely adherent cells, and transferred to 15-mL conical centrifuge tubes containing 1 mL of PBS. Cells were detached from the rods by sonication, diluted, and plated on agar for CFU enumeration.
Autoaggregation assay
Cutibacterium acnes cells were scraped from an agar plate into 2 mL of PBS using a cell scraper. Aliquots of the cell suspension were treated with 20 μg/mL dispersin B or 10 μg/mL DNase I for 15 min. One aliquot of cells was left untreated to serve as a control. A total of 300 μL of each cell suspension was transferred to a 0.5-mL polypropylene centrifuge tube (model 6,530; Corning). The tube was then mixed by high-speed vortex agitation for 10 s, incubated statically for 20 min, and photographed.
Treatment of biofilms with enzymes and detergent
72-h-old biofilms grown in glass tubes were rinsed vigorously with water and then treated with 1 mL of 20 μg/mL dispersin B, 10 μg/mL DNase I, or 1% SDS. After 15 min, tubes were rinsed vigorously with water and stained with crystal violet as described above.
Benzoyl peroxide killing assay
72-h-old biofilms grown in glass tubes were treated directly with 20 μg/mL dispersin B in PBS for 15 min followed by 70 or 140 μg/mL benzoyl peroxide for 10 min. Biofilms were then rinsed, detached from the tubes by sonication, diluted, and plated on agar for CFU enumeration.
Tetracycline tolerance assay
Biofilms were cultured in glass tubes in broth supplemented with 100 μg/mL dispersin B and/or 0.2 μg/mL tetracycline (MIC = 0.5 μg/mL). After 72 h, biofilms were rinsed, detached from the tubes by sonication, diluted, and plated on agar for CFU enumeration.
Statistics and reproducibility of results
All experiments were performed in triplicate or quadruplicate tubes. All experiments were performed 2–3 times with similar results. The significance of differences between means was calculated using a Student’s t-test. A p-value < 0.01 was considered significant.
Results
Detection of PNAG on Cutibacterium spp. cells
Cells of Cutibacterium sp. strain KPL2009 and C. acnes strain KPL1849 were reacted with PNAG-specific mAb F598 or control mAb F429, both directly conjugated to Alexa Fluor 488, and then visualized for immunofluorescence by confocal microscopy (Figure 1). Bacteria embedded in an immunoreactive matrix of PNAG were observed with mAb F598 but not with control mAb F429, suggesting that both strains produce PNAG.
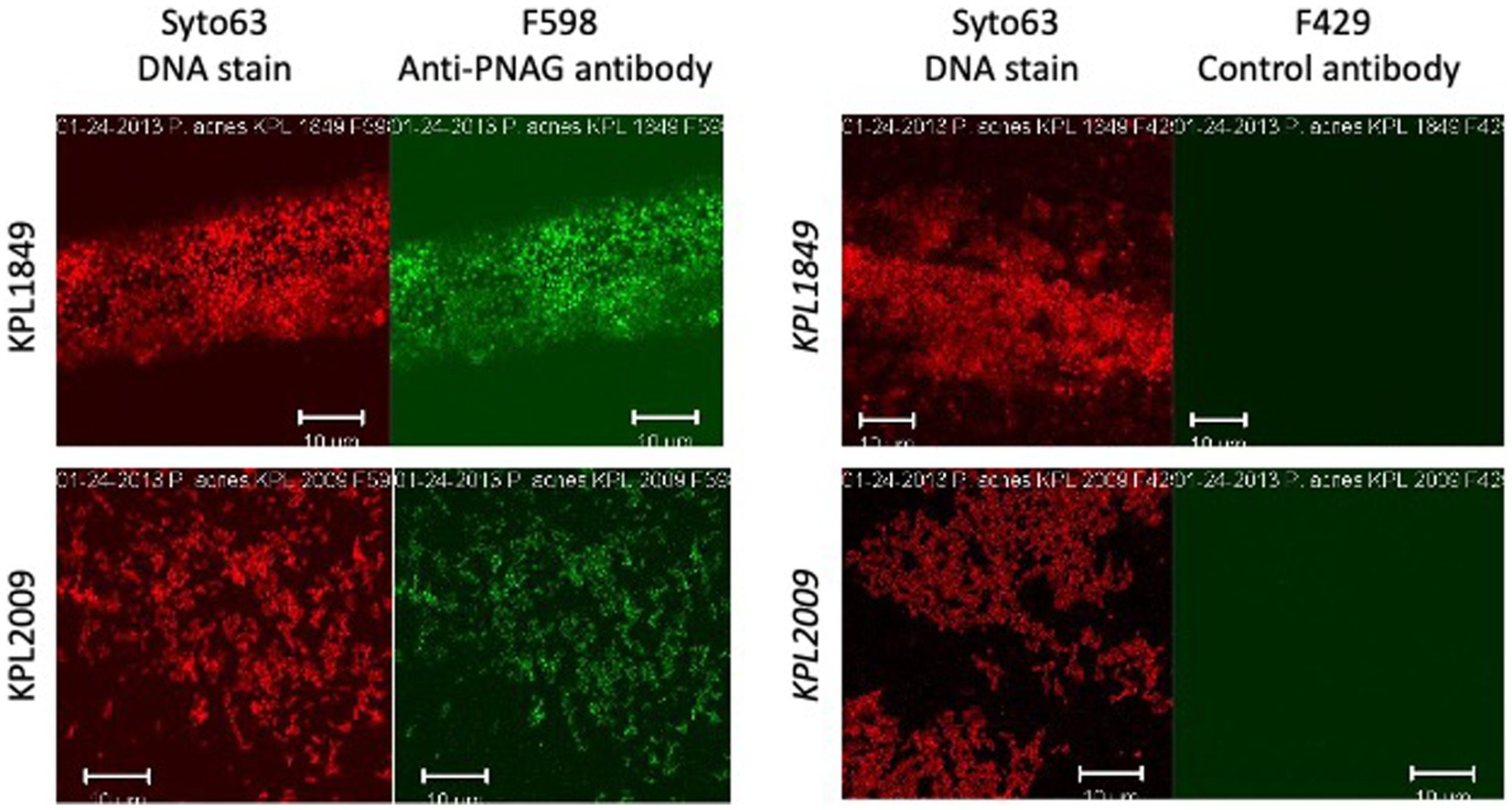
Figure 1. Fluorescence confocal microscopic images of Cutibacterium acnes strain KPL1849 (top) and Cutibacterium sp. strain KPL2009 (bottom) stained with Syto 63 and Alexa Fluor 488-conjugated anti-PNAG mAb F598 (left panels) or Syto 63 and Alexa Fluor 488-conjugated control antibody F429 which binds to Pseudomonas aeruginosa alginate (right panels). Syto 63 stains DNA red and mAb F598 stains PNAG green. Stains are indicated above. Strain names are indicated at the left. Measure bars = 10 μm.
Dispersin B inhibits Cutibacterium acnes biofilm formation in glass tubes
The ability of four C. acnes strains to form biofilms in glass culture tubes was investigated using a crystal violet binding assay (Figure 2). Two of the four strains tested (HL043PA1 and HL036PA1) formed strong biofilms as evidenced by the large amount of bound crystal violet dye at the bottom of the tube. To determine whether dispersin B inhibits C. acnes biofilm formation, biofilm-forming strains HL043PA1 and HL036PA1 were incubated in unsupplemented broth or broth supplemented with 100 μg/mL dispersin B (Figure 3). Dispersin B significantly inhibited biofilm formation by both strains as evidenced by a lower amount of bound crystal violet dye in tubes supplemented with the enzyme. Quantitation of bound dye for strain HL034PA1 yielded a biofilm inhibition value of 99% compared to the no enzyme control (p < 0.001).
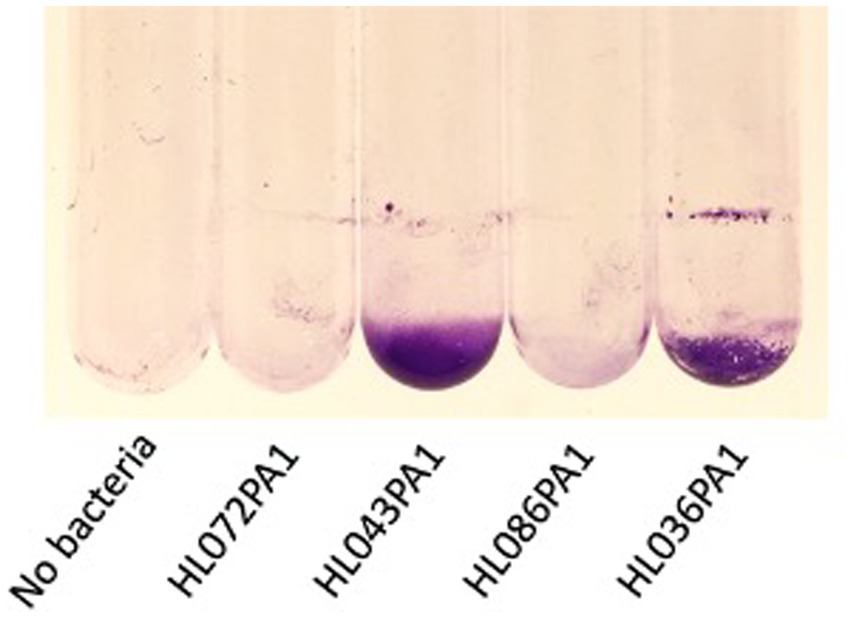
Figure 2. Biofilm formation by four Cutibacterium acnes strains in 13 × 100 mm glass tubes. Cultures were incubated for 3 days, rinsed with water, and stained with crystal violet. Strain names are indicated below. The tube at the left was incubated with sterile broth. This experiment was performed in duplicate tubes on three separate occasions with identical results. Representative tubes are shown.
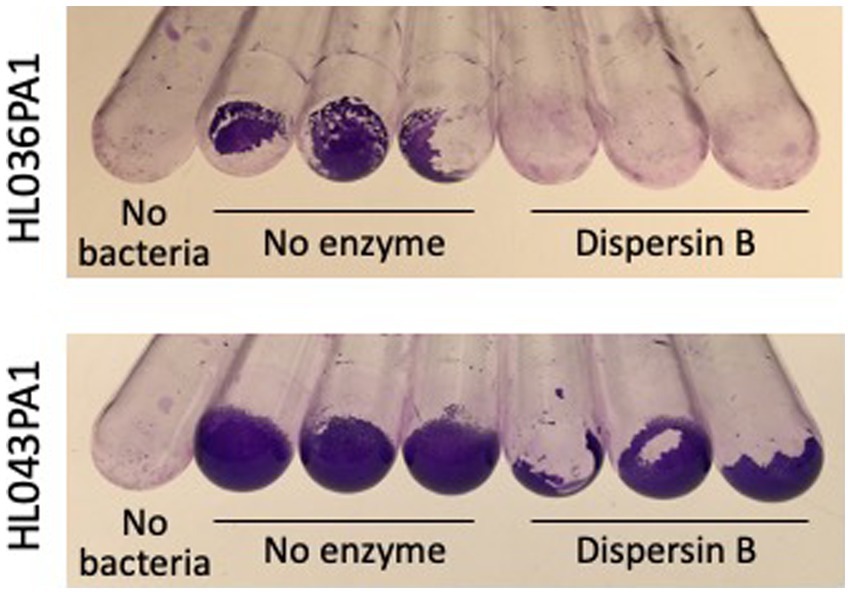
Figure 3. Biofilm formation by Cutibacterium acnes strains HL036PA1 and HL043PA1 in the absence or presence of 100 μg/mL dispersin B. Biofilms were cultured in glass tubes for 3 days, then rinsed with water and stained with crystal violet. Triplicate tubes for each condition are shown. The control tubes at the left (No bacteria) were incubated with sterile broth. These experiments were performed on two separate occasions with similar results. Tubes from representative experiments are shown.
Dispersin B inhibits attachment of Cutibacterium acnes cells to polystyrene rods
Photographs of HL043PA1 and HL036PA1 glass culture tubes taken directly after incubation (prior to rinsing and crystal violet staining) revealed the presence of thin biofilms along the sides of the tubes that were absent in tubes supplemented with dispersin B (Figure 4). This phenomenon was also evident for HL043PA1 and HL036PA1 cultured in conical-bottom polypropylene centrifuge tubes (Figure 5 and data not shown). In both types of tubes, however, a significant amount of cell clumping was observed at the bottom of the tube, even in the presence of the enzyme. These observations suggest that PNAG may promote the attachment of C. acnes cells and biofilms to surfaces. To test this hypothesis, untreated and dispersin B-treated C. acnes planktonic cells were incubated in the presence of polystyrene rods and the number of cells that attached to the rods after 30 min was enumerated (Figure 6). Significantly fewer dispersin B-treated cells attached to the rods than untreated cells, whereas cells treated with heat-inactivated dispersin B attached to the rods at the same level as untreated cells. These results suggest that PNAG contributes to C. acnes surface attachment.
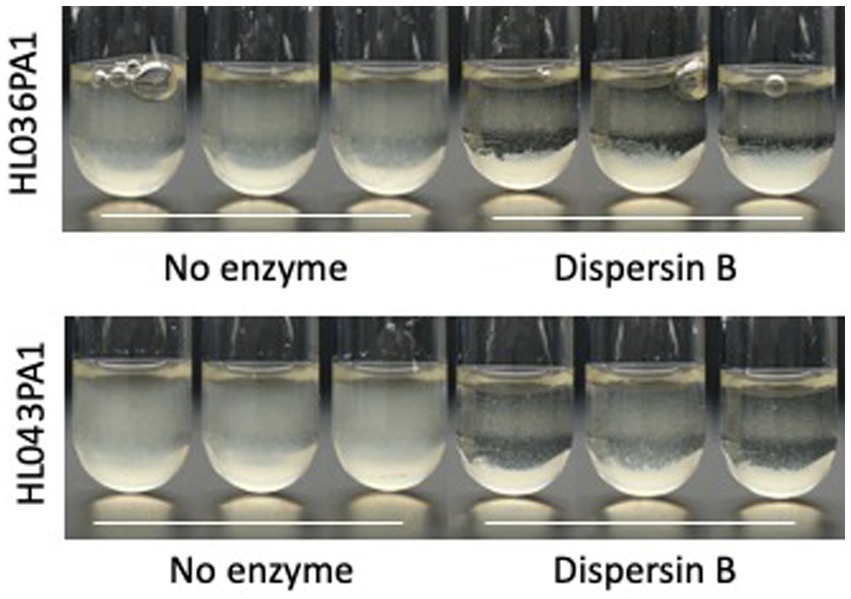
Figure 4. Growth of Cutibacterium acnes strains HL036PA1 and HL043PA1 in glass tubes in the presence of 0 or 100 μg/mL dispersin B. Tubes were photographed after 3 days of incubation. Triplicate tubes for each condition are shown. Strain names are indicated at the left. Enzyme treatments are indicated below. These experiments were performed on three separate occasions with identical results. Tubes from representative experiments are shown.
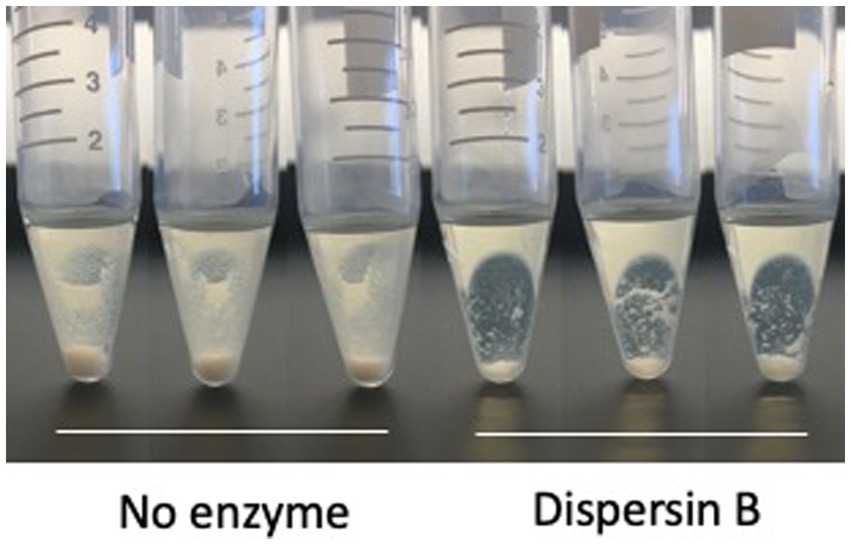
Figure 5. Growth of Cutibacterium acnes strain HL036PA1 in polypropylene tubes in the presence of 0 or 100 μg/mL dispersin B. Bacteria were photographed after 3 days of growth. Triplicate tubes for each condition are shown. This experiment was performed on two separate occasions with identical results. Tube from one representative experiment are shown.
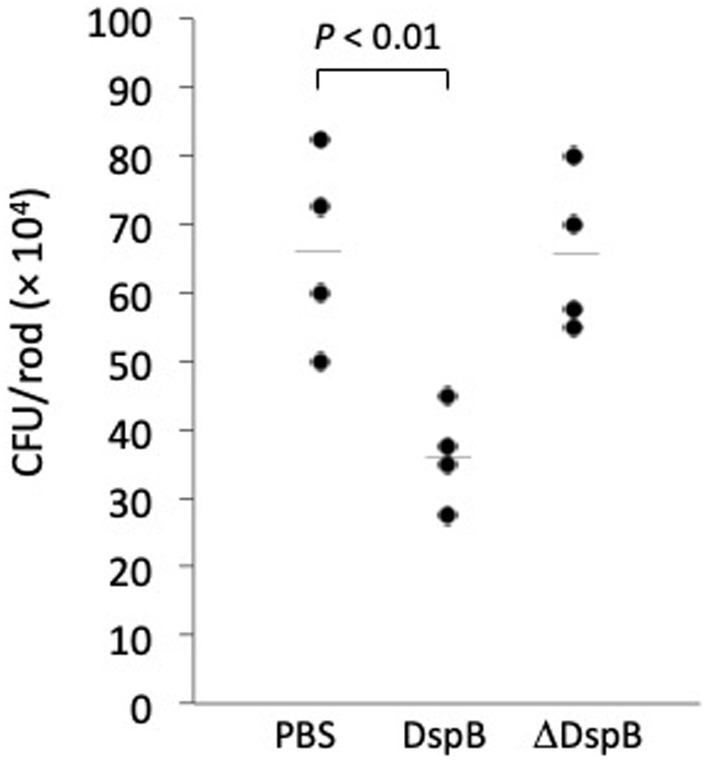
Figure 6. Attachment of Cutibacterium acnes HL043PA1 planktonic cells to polystyrene rods. Cells were treated with phosphate buffered saline (PBS), 20 μg/mL dispersin B (DspB), or 20 μg/mL heat-inactivated dispersin B (ΔDspB) for 15 min prior to contacting the rods. Each point represents one individual rod. Horizontal lines indicate mean values. This experiment was performed on two separate occasions with similar results. Results from one representative experiment are shown.
DNase I inhibits Cutibacterium acnes autoaggregation
Autoaggregation (also termed intercellular adhesion) often plays a role in biofilm formation (Trunk et al., 2018). To test whether PNAG contributes to C. acnes autoaggregation, C. acnes HL036PA1 cells were treated with dispersin B or DNase I for 30 min, mixed by vortex agitation, transferred to a microcentrifuge tube, allowed to settle for 15 min, then photographed (Figure 7). Untreated control cells and dispersin B-treated cells settled to the bottom of the tube, whereas DNase I-treated cells remained in suspension. These findings suggest that extracellular DNA, but not PNAG, contributes to C. acnes autoaggregation.
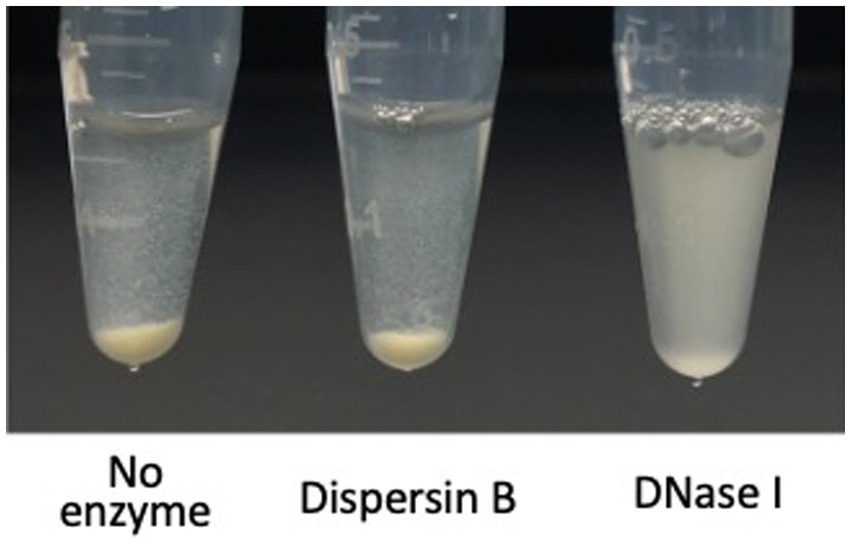
Figure 7. Autoaggregation of Cutibacterium acnes HL043PA1 cells in the presence of 20 μg/mL dispersin B or 10 μg/mL DNase I. Cell suspensions supplemented with the indicated enzyme were transferred to polypropylene tubes, incubated statically for 20 min, and then photographed. This experiment was performed in duplicate tubes on two separate occasions with identical results. Representative tubes are shown.
DNase I and SDS detach pre-formed Cutibacterium acnes biofilms from glass tubes
To further investigate the composition of C. acnes biofilms, 72-h-old HL043PA1 biofilms were treated with dispersin B, DNase I, or SDS for 15 min, and then stained with crystal violet to visualize the biofilm remaining after treatment (Figure 8). DNase I and SDS, but not dispersin B, efficiently detached the mature biofilms, suggesting that extracellular DNA and proteinaceous adhesins, but not PNAG, contribute to biofilm stability in mature C. acnes biofilms.
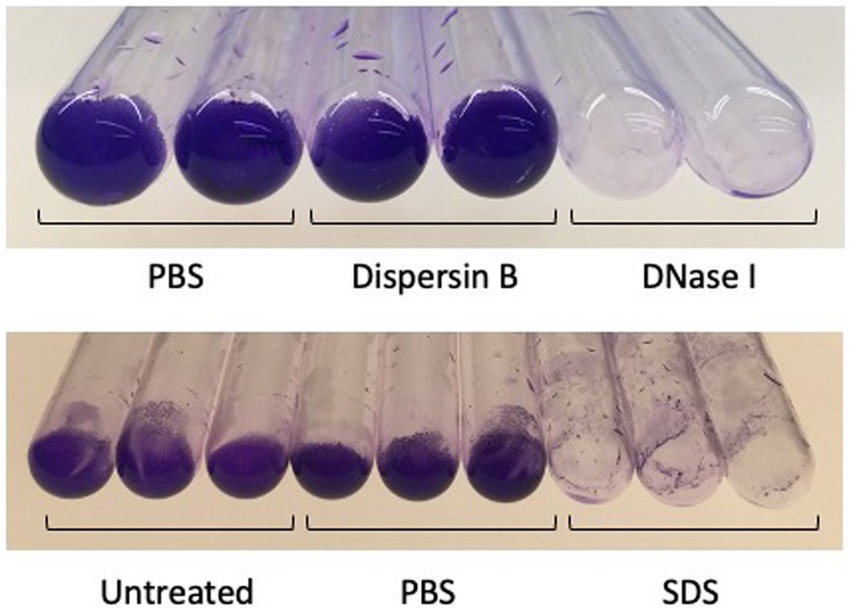
Figure 8. Detachment of 3-days-old Cutibacterium acnes HL043PA1 biofilms by enzymes and detergents. Biofilms were rinsed with water, treated with the indicated agent for 30 min at 37°C, re-rinsed, and stained with crystal violet. Dispersin B was 50 μg/mL, DNase I was 10 μg/mL, and sodium diodecyl sulfate (SDS) was at 1%. Duplicate tubes for each treatment are shown in the top panel and triplicate tubes for each treatment are shown in the bottom panel. These experiments were performed on three separate occasions with identical results. Representative experiments are shown.
Dispersin B sensitizes Cutibacterium acnes biofilms to benzoyl peroxide killing
HL043PA1 biofilms (72-h-old) were treated directly with 20 μg/mL dispersin B for 15 min followed by 70 or 140 μg/mL benzoyl peroxide for 10 min. The biofilms were then detached from the tubes by sonication, diluted, and plated on agar for CFU enumeration. Control experiments showed that dispersin B alone did not kill C. acnes cells or inhibit their growth (data not shown). Treatment of C. acnes biofilms with 70 or 140 μg/ mL benzoyl peroxide alone resulted in a 1-log reduction in C. acnes CFUs, while pre-treatment of biofilms with dispersin B increased benzoyl peroxide killing by approximately 0.5 log (p < 0.001; Figures 9A,B). These findings suggest that PNAG protects C. acnes biofilm cells from killing by benzoyl peroxide.
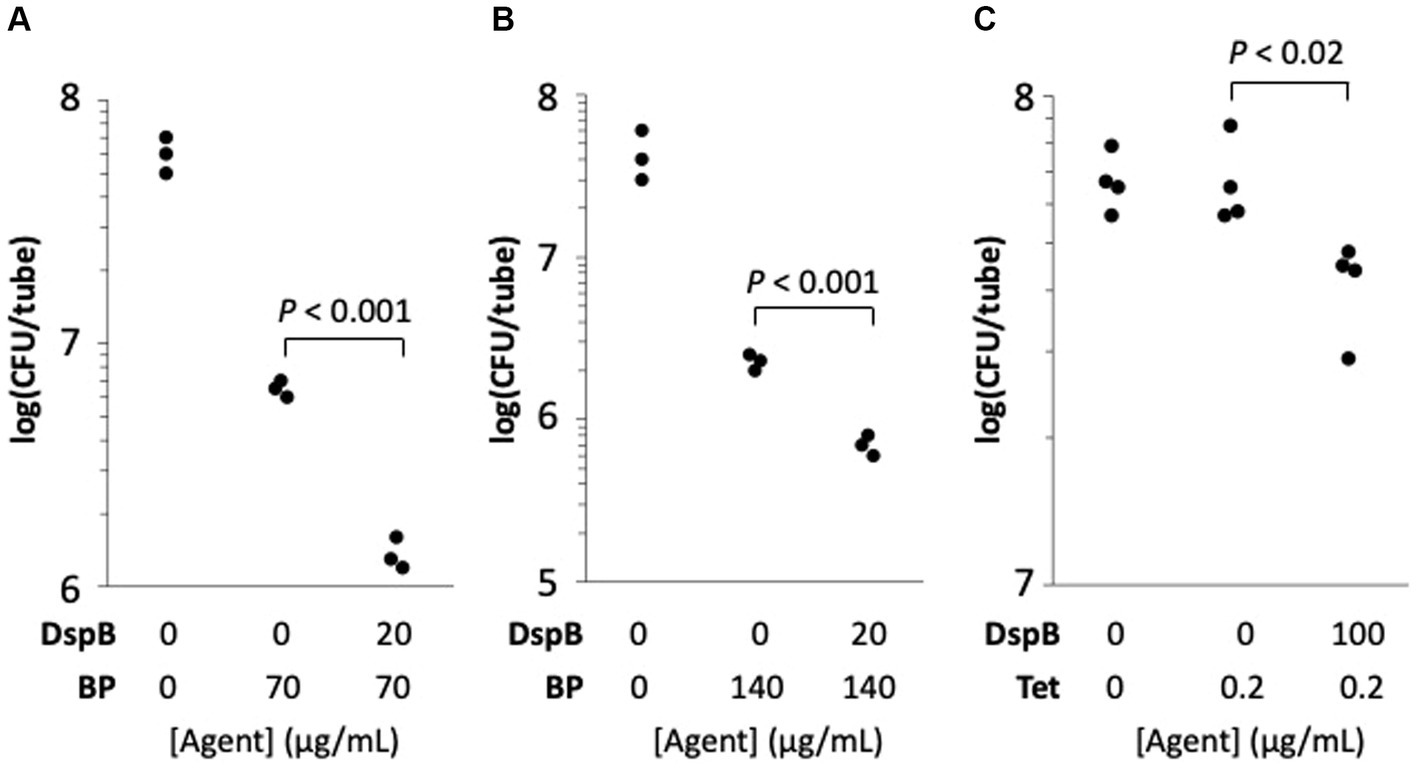
Figure 9. Dispersin B (DspB) sensitizes Cutibacterium acnes HL043PA1 biofilms to killing by benzoyl peroxide (BP) (A,B) and to growth inhibition by tetracycline (Tet) (C). Panels (A,B) show the effect of a 10-min BP treatment on 72-h-old biofilms cultured in glass tubes. Panel (A) 70 μg/mL BP; panel (B), 140 μg/mL BP. Some biofilms were treated with 20 μg/mL DspB in PBS for 15 min prior to contact with BP as indicated in the legends below. In panel (C), biofilms were cultured for 72-h in 0 or 0.2 μg/mL Tet. Some tubes were supplemented with 100 μg/mL DspB as indicated in the legend below the graph. Each dot represents one individual tube. The experiment in panel (C) was performed on two separate occasions with similarly significant differences between tetracycline alone and tetracycline + dispersin B.
Dispersin B decreases tetracycline tolerance in Cutibacterium acnes biofilms
The effect of dispersin B on the tolerance of HL043PA1 biofilms to tetracycline was measured by culturing biofilms in broth supplemented with 100 μg/mL dispersin B and/or 0.2 μg/mL tetracycline (MIC = 0.5 μg/mL). After 72 h, fewer C. acnes cells were recovered from tubes supplemented with dispersin B plus tetracycline compared to tubes supplemented with tetracycline alone (p < 0.02; Figure 9C). These findings suggest that PNAG contributes to tetracycline tolerance C. acnes biofilms.
Discussion
Cutibacterium acnes is one of the most abundant bacteria on the skin of most people (Oh et al., 2014). Cutibacterium acnes is both a beneficial commensal that helps maintain homeostasis of the skin microbiome, and an opportunistic pathogen associated with acne vulgaris and invasive infections of implanted medical devices (Achermann et al., 2014). Biofilm formation likely plays an important role in the ability of C. acnes to colonize skin and device surfaces (Coenye et al., 2022). Biofilms may also play a role in the pathogenesis of acne vulgaris (McLaughlin et al., 2019). Cutibacterium acnes biofilms have been observed in acne lesions and on implanted medical devices in vivo (Bayston et al., 2006; Jahns et al., 2012). Biofilm formation is also a common phenotype among C. acnes clinical isolates in vitro (Coenye et al., 2022). Understanding the mechanism of C. acnes biofilm formation may lead to the development of novel antibiofilm agents that can be used to treat acne or prevent invasive infections.
Bacteria in a biofilm are encased in a self-synthesized polymeric matrix that holds the cells together in a mass, attaches them to a tissue or surface, and protects them from killing by biocides and host immunity (Flemming and Wingender, 2010). Several previous studies have investigated the composition of the C. acnes biofilm matrix in vitro. Gannesen et al. (2019) found that the biofilm matrix of C. acnes acneic strain RT5 consisted of approximately 63% polysaccharides, 10% proteins, 4% DNA, and 23% other compounds. The major polysaccharide was a linear polymer of glucose, galactose, mannose, galactosamine, and diaminomannuronic acid in a molar ratio of 1:1:0.3:1:2. Jahns et al. (2016) detected similar components in the biofilm matrix of C. acnes skin isolate KPA171202 by performing fluorescent microscopy with carbohydrate-, protein-, and DNA-specific stains. Kuehnast et al. (2018) found that proteinase K significantly detached pre-formed biofilms produced by 7 of 8 C. acnes strains in flow-cells, and that DNase I detached 4 of the 8 strains tested. Similarly, Fang et al. (2021) found that cationic liposomes loaded with DNase I or proteinase K significantly detached pre-formed biofilms produced by C. acnes acneic strain ATCC6919 in 24-well microtiter plate wells, and Okuda et al. (2018) found that proteinase K and DNase I significantly inhibited biofilm formation by 2 of 5 C. acnes implant isolates in 96-well microplates when the enzymes were added to the culture medium prior to biofilm formation. Taken together, these results are consistent with the presence of polysaccharides, proteinaceous adhesins and eDNA in the biofilm matrix of some C. acnes strains. Our results demonstrating inhibition of HL043PA1 autoaggregation by DNase I (Figure 7) and detachment of C. acnes HL043PA1 biofilms by DNase I and SDS (Figure 8) are consistent with the presence of proteinaceous adhesins and eDNA in the biofilm matrix of this strain. Several studies have revealed an important role for eDNA in biofilm formation, adhesion, and structural integrity in diverse bacterial species (Panlilio and Rice, 2021).
Previous investigations of PNAG production in C. acnes were inconclusive. Gannesen et al. (2019) observed no NMR spectroscopic signal for N-acetylglucosamine, 2-acetamido-2-deoxy-galactose, or PNAG in the biofilm matrix of C. acnes strain RT5, suggesting that this strain does not produce PNAG. Okuda et al. (2018) found that dispersin B did not inhibit biofilm formation by five strains of C. acnes isolated from cardiac pacemakers when cultured in polystyrene microtiter plate wells. Since dispersin B was previously shown to inhibit biofilm formation by other PNAG-producing bacteria in vitro (Itoh et al., 2005; Parise et al., 2007; Pérez-Mendoza et al., 2011; Turk et al., 2013), these results suggested that PNAG was not a major adhesive component of biofilms produced by these five C. acnes strains.
In the present study we reinvestigated PNAG production in C. acnes using the PNAG-specific mAb F598 and the PNAG-specific glycosidase dispersin B. We found that mAb F598 reacted with cells of C. acnes strain KPL1849 and Cutibacterium sp. strain KPL2009 cultured on agar (Figure 1), suggesting that these two strains produce PNAG under some conditions. We also found that dispersin B exhibited antibiofilm activities against C. acnes strains HL036PA1 and HL043PA1 including inhibition of surface attachment (Figures 3–6) and sensitization to biocide killing (Figure 9). Since dispersin B is an accurate indicator of PNAG production (Cywes-Bentley et al., 2013; Eddenden and Nitz, 2022), these findings suggest that these two C. acnes strains also produce PNAG under some conditions. The antibiofilm activities exhibited by dispersin B against C acnes HL036PA1 and HL043PA1 are consistent with those exhibited by dispersin B against other species of bacteria (Itoh et al., 2005; Izano et al., 2007; Parise et al., 2007; Ganeshnarayan et al., 2009). The lack of a NMR signal for PNAG in the biofilm matrix of C. acnes strain RT5 (Gannesen et al., 2019) may be due to the fact that not all C. acnes strains produce PNAG, or that PNAG is a minor component of the RT5 biofilm matrix. The fact that dispersin B did not exhibit biofilm inhibiting activity against five implant-associated C. acnes strains in 96-well microplates (Okuda et al., 2018) may be due to strain differences or to the fact that dispersin B exhibits different antibiofilm activities depending on the shape and size of the culture vessel (Izano et al., 2007). It is also possible that the enzyme used by Okuda et al. (2018) was inactive because no positive control for enzyme activity was reported.
Our results suggest that PNAG mediates C. acnes surface attachment and biocide resistance, but that eDNA is the major intercellular adhesin in mature C. acnes biofilms. C. acnes be similar to Staphylococcus aureus, where double-stranded DNA is the most common extracellular component of biofilms produced by most strains (Sugimoto et al., 2018), while PNAG functions to confer resistance to killing by biocides (Serrera et al., 2007; Darouiche et al., 2009; Gawande et al., 2014) and innate host immune mediators (Kropec et al., 2005). Like C. acnes, S. aureus biofilms are readily detached by DNase I but not by dispersin B (Izano et al., 2008; Kaplan et al., 2012). More experiments are needed to determine the functions of PNAG in C. acnes cells and biofilms, and to determine whether PNAG production correlates with skin colonization, biocide resistance, immune tolerance, acne pathogenesis, and medical device infections in vivo.
Previous in vivo studies showed that PNAG is an important colonization and virulence factor for numerous other bacterial pathogens including Aggregatibacter actinomycetemcomitans (Shanmugam et al., 2015); Klebsiella pneumoniae (Chen et al., 2014); Staphylococcus epidermidis (Kaplan et al., 2018), Staphylococcus aureus (Kropec et al., 2005; Serrera et al., 2007; Darouiche et al., 2009; Gawande et al., 2014), Pectobacterium carotovorum (Ragunath et al., 2011) and Actinobacillus pleuropneumoniae (Subashchandrabose et al., 2013). PNAG may similarly contribute to C. acnes surface attachment, biofilm formation, biocide tolerance, skin colonization, acne pathogenesis and medical device infections in vivo. PNAG may enable C. acnes to form biofilms and colonize epithelial surfaces and hair follicles, to form biofilms on biomaterials, and to resist killing by antimicrobial agents and host immunity. It is also possible that PNAG functions as a biological glue that holds corneocytes together to form acne microcomedones (Burkhart and Burkhart, 2007).
Data availability statement
The original contributions presented in the study are included in the article/supplementary material, further inquiries can be directed to the corresponding author.
Author contributions
JK: Conceptualization, Data curation, Formal analysis, Funding acquisition, Investigation, Methodology, Project administration, Resources, Software, Supervision, Validation, Visualization, Writing – original draft, Writing – review & editing. CC-B: Conceptualization, Data curation, Formal analysis, Investigation, Methodology, Project administration, Resources, Software, Supervision, Validation, Visualization, Writing – original draft, Writing – review & editing. GP: Conceptualization, Data curation, Funding acquisition, Resources, Supervision, Writing – original draft, Writing – review & editing. NY: Writing – review & editing. MS: Writing – review & editing. ME: Funding acquisition, Writing – review & editing. KK: Funding acquisition, Writing – review & editing.
Funding
The author(s) declare that financial support was received for the research, authorship, and/or publication of this article. This project was funded by Kane Biotech Inc.
Acknowledgments
The authors thank Michael S. Wollenberg and Katherine P. Lemon (Baylor College of Medicine) for providing Cutibacterium sp. strain KPL2009 and C. acnes strain KPL1849. Cutibacterium acnes strains HL036PA1, HL043PA1, HL072PA1, and HL086PA1 were obtained from BEI Resources (Manassas, Virginia, United States) as part of the NIAID NIH Human Microbiome Project.
Conflict of interest
JK serves as an advisor for, owns equity in, and receives royalties from Kane Biotech Inc., Winnipeg, Canada. This company is developing antibiofilm applications related to dispersin B. GP is an inventor of intellectual properties (human monoclonal antibody to PNAG and PNAG vaccines) that are licensed by Brigham and Women’s Hospital to Alopexx, Inc., an entity in which GP also holds equity. As an inventor of intellectual properties, GP also has the right to receive a share of licensing-related income (royalties, fees) through Brigham and Women’s Hospital from Alopexx, Inc. GP’s interests were reviewed and are managed by the Brigham and Women’s Hospital and Mass General Brigham in accordance with their conflict of interest policies. CC-B is an inventor of intellectual properties (use of human monoclonal antibody to PNAG and use of PNAG vaccines) that are licensed by Brigham and Women’s Hospital to Alopexx, Inc. As an inventor of intellectual properties, CC-B also has the right to receive a share of licensing-related income (royalties, fees) through Brigham and Women’s Hospital from Alopexx, Inc. NY, MS, and ME are employees of Kane Biotech Inc., manufacturer of dispersin B (DispersinB®), and own company stocks and stock options.
The remaining author declares that the research was conducted in the absence of any commercial or financial relationships that could be construed as a potential conflict of interest.
Publisher’s note
All claims expressed in this article are solely those of the authors and do not necessarily represent those of their affiliated organizations, or those of the publisher, the editors and the reviewers. Any product that may be evaluated in this article, or claim that may be made by its manufacturer, is not guaranteed or endorsed by the publisher.
References
Achermann, Y. E., Goldstein, J., Coenye, T., and Shirtliff, M. E. (2014). Propionibacterium acnes: from commensal to opportunistic biofilm-associated implant pathogen. Clin. Microbiol. Rev. 27, 419–440. doi: 10.1128/CMR.00092-13
Bayston, R., Ashraf, W., Barker-Davies, R., Tucker, E., Clement, R., Clayton, J., et al. (2006). Biofilm formation by Propionibacterium acnes on biomaterials in vitro and in vivo: impact on diagnosis and treatment. J. Biomed. Mater. Res. A 81, 705–709. doi: 10.1002/jbm.a.31145
Burkhart, C. G., and Burkhart, C. N. (2007). Expanding the microcomedone theory and acne therapeutics: Propionibacterium acnes biofilm produces biological glue that holds corneocytes together to form plug. J. Am. Acad. Dermatol. 57, 722–724. doi: 10.1016/j.jaad.2007.05.013
Chen, K.-M., Chiang, M.-K., Wang, M., Ho, H.-C., Lu, M.-C., and Lai, Y.-C. (2014). The role of pgaC in Klebsiella pneumoniae virulence and biofilm formation. Miccrob. Pathog. 77, 89–99. doi: 10.1016/j.micpath.2014.11.005
Claesen, J., Spagnolo, J. B., Flores Ramos, S., Kurita, K. L., Byrd, A. L., Aksenov, A. A., et al. (2020). A Cutibacterium acnes antibiotic modulates human skin microbiota composition in hair follicles. Sci. Transl. Med. 12:5445. doi: 10.1126/scitranslmed.aay5445
Coenye, T., Spittaels, K.-J., and Achermann, Y. (2022). The role of biofilm formation in the pathogenesis and antimicrobial susceptibility of Cutibacterium acnes. Biofilms 4:100063. doi: 10.1016/j.bioflm.2021.100063
Cywes-Bentley, C., Skurnik, D., Zaidi, T., Roux, D., Deoliveira, R. B., Garrett, W. S., et al. (2013). Antibody to a conserved antigenic target is protective against diverse prokaryotic and eukaryotic pathogens. Proc. Natl. Acad. Sci. U. S. A. 110, E2209–E2218. doi: 10.1073/pnas.1303573110
Darouiche, R. O., Mansouri, M. D., Gawande, P. V., and Madhyastha, S. (2009). Antimicrobial and antibiofilm efficacy of triclosan and DispersinB combination. J. Antimicrob. Chemother. 64, 88–93. doi: 10.1093/jac/dkp158
Eddenden, A., and Nitz, M. (2022). Applications of an inactive Dispersin B probe to monitor biofilm polysaccharide production. Methods Enzymol. 665, 209–231. doi: 10.1016/bs.mie.2021.11.006
Fang, J.-Y., Chou, W.-L., Lin, C.-F., Sung, C. T., Alalaiwe, A., and Yang, S.-C. (2021). Facile biofilm penetration of cationic liposomes loaded with DNase I/proteinase K to eradicate Cutibacterium acnes for treating cutaneous and catheter infections. Int. J. Nanomedicine 16, 8121–8138. doi: 10.2147/IJN.S335804
Fitz-Gibbon, S., Tomida, S., Chiu, B.-H., Nguyen, L., Du, C., Liu, M., et al. (2013). Propionibacterium acnes strain populations in the human skin microbiome associated with acne. J. Invest. Dermatol. 133, 2152–2160. doi: 10.1038/jid.2013.21
Flemming, H. C., and Wingender, J. (2010). The biofilm matrix. Nat. Rev. Microbiol. 8, 623–633. doi: 10.1038/nrmicro2415
Ganeshnarayan, K., Shah, S. M., Libera, M. R., Santostefano, A., and Kaplan, J. B. (2009). Poly-N-acetylglucosamine matrix polysaccharide impedes fluid convection and transport of the cationic surfactant cetylpyridinium chloride through bacterial biofilms. Appl. Environ. Microbiol. 75, 1308–1314. doi: 10.1128/AEM.01900-08
Gannesen, A. V., Zdorovenko, E. L., Botchkova, E. A., Hardouin, J., Massier, S., Kopitsyn, D. S., et al. (2019). Composition of the biofilm matrix of Cutibacterium acnes acneic strain RT5. Front. Microbiol. 10:1284. doi: 10.3389/fmicb.2019.01284
Gawande, P. V., Leung, K. P., and Madhyastha, S. (2014). Antibiofilm and antimicrobial efficacy of DispersinB®-KSL-W peptide-based wound gel against chronic wound infection associated bacteria. Curr. Microbiol. 68, 635–641. doi: 10.1007/s00284-014-0519-6
Gening, M. L., Pier, G. B., and Nifantiev, N. E. (2020). Broadly protective semi-synthetic glycoconjugate vaccine against pathogens capable of producing poly-β-(1→6)-N-acetyl-d-glucosamine exopolysaccharide. Drug Discov. Today Technol. 35-36, 13–21. doi: 10.1016/j.ddtec.2020.09.002
Itoh, Y., Wang, X., Hinnebusch, B. J., Preston, J. F. III, and Romeo, T. (2005). Depolymerization of β-1,6-N-acetyl-D-glucosamine disrupts the integrity of diverse bacterial biofilms. J. Bacteriol. 187, 382–387. doi: 10.1128/JB.187.1.382-387.2005
Izano, E. A., Amarante, M. A., Kher, W. B., and Kaplan, J. B. (2008). Differential roles of poly-N-acetylglucosamine surface polysaccharide and extracellular DNA in Staphylococcus aureus and Staphylococcus epidermidis biofilms. Appl. Environ. Microbiol. 74, 470–476. doi: 10.1128/AEM.02073-07
Izano, E. A., Wang, H., Ragunath, C., Ramasubbu, N., and Kaplan, J. B. (2007). Detachment and killing of Aggregatibacter actinomycetemcomitans biofilms by dispersin B and SDS. J. Dent. Res. 86, 618–622. doi: 10.1177/154405910708600707
Jahns, A. C., Eilers, H., and Alexeyev, O. A. (2016). Transcriptomic analysis of Propionibacterium acnes biofilms in vitro. Anaerobe 42, 111–118. doi: 10.1016/j.anaerobe.2016.10.001
Jahns, A. C., Lundskog, B., Ganceviciene, R., Palmer, R. H., Golovleva, I., Zouboulis, C. C., et al. (2012). An increased incidence of Propionibacterium acnes biofilms in acne vulgaris: a case-control study. Br. J. Dermatol. 167, 50–58. doi: 10.1111/j.1365-2133.2012.10897.x
Kaplan, J. B., LoVetri, K., Cardona, S. T., Madhyastha, S., Sadovskaya, I., Jabbouri, S., et al. (2012). Recombinant human DNase I decreases biofilm and increases antimicrobial susceptibility in staphylococci. J. Antibiot. 65, 73–77. doi: 10.1038/ja.2011.113
Kaplan, J. B., Mlynek, K. D., Hettiarachchi, H., Alamneh, Y. A., Biggemann, L., Zurawski, D. V., et al. (2018). Extracellular polymeric substance (EPS)-degrading enzymes reduce staphylococcal surface attachment and biocide resistance on pig skin in vivo. PLoS One 13:e0205526. doi: 10.1371/journal.pone.0205526
Kropec, A., Maira-Litran, T., Jefferson, K. K., Grout, M., Cramton, S. E., Götz, F., et al. (2005). Poly-N-acetylglucosamine production in Staphylococcus aureus is essential for virulence in murine models of systemic infection. Infect. Immun. 73, 6868–6876. doi: 10.1128/IAI.73.10.6868-6876.2005
Kuehnast, T., Cakar, F., Weinhäupl, T., Pilz, A., Saja Selak, S., Schmidt, M. A., et al. (2018). Comparative analyses of biofilm formation among different Cutibacterium acnes isolates. Int. J. Med. Microbiol. 308, 1027–1035. doi: 10.1016/j.ijmm.2018.09.005
McLaughlin, J., Watterson, S., Layton, A. M., Bjourson, A. J., Barnard, E., and McDowell, A. (2019). Propionibacterium acnes and acne vulgaris: new insights from the integration of population genetic, multi-omic, biochemical and host-microbe studies. Microorganisms 7:128. doi: 10.3390/microorganisms7050128
Oh, J., Byrd, A. L., Deming, C., Conlan, S., NISC Comparative Sequencing ProgramKong, H. H., et al. (2014). Biogeography and individuality shape function in the human skin metagenome. Nature 514, 59–64. doi: 10.1038/nature13786
Okuda, K. I., Nagahori, R., Yamada, S., Sugimoto, S., Sato, C., Sato, M., et al. (2018). The composition and structure of biofilms developed by Propionibacterium acnes isolated from cardiac pace-maker devices. Front. Microbiol. 9:182. doi: 10.3389/fmicb.2018.00182
Panlilio, H., and Rice, C. V. (2021). The role of extracellular DNA in the formation, architecture, stability, and treatment of bacterial biofilms. Biotechnol. Bioeng. 118, 2129–2141. doi: 10.1002/bit.27760
Parise, G., Mishra, M., Itoh, Y., Romeo, T., and Deora, R. (2007). Role of a putative polysaccharide locus in Bordetella biofilm development. J. Bacteriol. 189, 750–760. doi: 10.1128/JB.00953-06
Pérez-Mendoza, D., Coulthurst, S. J., Sanjuán, J., and Salmond, G. P. C. (2011). N-acetylglucosamine-dependent biofilm formation in Pectobacterium atrosepticum is cryptic and activated by elevated c-di-GMP levels. Microbiology 157, 3340–3348. doi: 10.1099/mic.0.050450-0
Pier, G. B., Boyer, D., Preston, M., Coleman, F. T., Llosa, N., Mueschenborn-Koglin, S., et al. (2004). Human monoclonal antibodies to Pseudomonas aeruginosa alginate that protect against infection by both mucoid and nonmucoid strains. J. Immunol. 173, 5671–5678. doi: 10.4049/jimmunol.173.9.5671
Ragunath, C., Shanmugam, M., Bendaoud, M., Kaplan, J. B., and Ramasubbu, N. (2011). Effect of a biofilm-degrading enzyme from an oral pathogen in transgenic tobacco on the pathogenicity of Pectobacterium carotovorum subsp. carotovorum. Plant Pathol. 61, 346–354. doi: 10.1111/j.1365-3059.2011.02509.x
Serrera, A., del Pozo, J. L., Martinez, A., Alonso, M., Gonzalez, R., Leiva, J., et al. (2007). Dispersin B therapy of Staphylococcus aureus experimental port-related bloodstream infection. In: 17th European Congress of Clinical Microbiology and Infectious Disease. Abstract P1786. Munich, Germany. 29, S508.
Shanmugam, M., Gopal, P., El Abbar, F., Schreiner, H. C., Kaplan, J. B., Fine, D. H., et al. (2015). Role of exopolysaccharide in Aggregatibacter actinomycetemcomitans-induced bone resorption in a rat model for periodontal disease. PLoS One 10:e117487. doi: 10.1371/journal.pone.0117487
Soliman, C., Walduck, A. K., Yuriev, E., Richards, J. S., Cywes-Bentley, C., Pier, G. B., et al. (2018). Structural basis for antibody targeting of the broadly expressed microbial polysaccharide poly-N-acetylglucosamine. J. Biol. Chem. 293, 5079–5089. doi: 10.1074/jbc.RA117.001170
Subashchandrabose, S., Leveque, R. M., Kirkwood, R. N., Kiupel, M., and Mulks, M. H. (2013). The RNA chaperone Hfq promotes fitness of Actinobacillus pleuropneumoniae during porcine pleuropneumonia. Infect. Immun. 81, 2952–2961. doi: 10.1128/IAI.00392-13
Sugimoto, S., Sato, F., Miyakawa, R., Chiba, A., Onodera, S., Hori, S., et al. (2018). Broad impact of extracellular DNA on biofilm formation by clinically isolated methicillin-resistant and -sensitive strains of Staphylococcus aureus. Sci. Rep. 8:2254. doi: 10.1038/s41598-018-20485-z
Trunk, T., Khalil, H. S., and Leo, J. C. (2018). Bacterial autoaggregation. AIMS Microbiol. 4, 140–164. doi: 10.3934/microbiol.2018.1.140
Turk, R., Singh, A., Rousseau, J., and Weese, J. S. (2013). In vitro evaluation of DispersinB on methicillin-resistant Staphylococcus pseudintermedius biofilm. Vet. Microbiol. 166, 576–579. doi: 10.1016/j.vetmic.2013.07.011
Keywords: acne vulgaris, antibiofilm, antibiotic tolerance, benzoyl peroxide, biofilm matrix, dispersin B, DNase I, extracellular DNA
Citation: Kaplan JB, Cywes-Bentley C, Pier GB, Yakandawala N, Sailer M, Edwards MS and Kridin K (2024) Poly-β-(1→6)-N-acetyl-D-glucosamine mediates surface attachment, biofilm formation, and biocide resistance in Cutibacterium acnes. Front. Microbiol. 15:1386017. doi: 10.3389/fmicb.2024.1386017
Edited by:
Michal Letek, University of León, SpainReviewed by:
Jakub Kwiecinski, Jagiellonian University, PolandMegan R. Kiedrowski, University of Alabama at Birmingham, United States
Copyright © 2024 Kaplan, Cywes-Bentley, Pier, Yakandawala, Sailer, Edwards and Kridin. This is an open-access article distributed under the terms of the Creative Commons Attribution License (CC BY). The use, distribution or reproduction in other forums is permitted, provided the original author(s) and the copyright owner(s) are credited and that the original publication in this journal is cited, in accordance with accepted academic practice. No use, distribution or reproduction is permitted which does not comply with these terms.
*Correspondence: Jeffrey B. Kaplan, kaplanjb@american.edu