- Massachusetts Institute for Neurodegenerative Disease, Department of Neurology, Massachusetts General Hospital, Harvard Medical School, Harvard University, Boston, MA, United States
The physiological function of the neurovascular unit is critically dependent upon the complex structure and functions of astrocytes for optimal preservation of cerebral homeostasis. While it has been shown that astrocytes exhibit aberrant changes in both structure and function in transgenic murine models of Alzheimer’s disease (AD), it is not fully understood how this altered phenotype contributes to the pathogenesis of AD or whether this alteration predicts a therapeutic target in AD. The mechanisms underlying the spatiotemporal relationship between astrocytes, neurons and the vasculature in their orchestrated regulation of local cerebral flow in active brain regions has not been fully elucidated in brain physiology and in AD. As there is an incredible urgency to identify therapeutic targets that are well-tolerated and efficacious in protecting the brain against the pathological impact of AD, here we use the current body of literature to evaluate the hypothesis that pathological changes in astrocytes are central to the pathogenesis of AD. We also examine the current tools available to assess astrocytic calcium signaling in the living murine brain as it has an important role in the complex interaction between astrocytes, neurons and the vasculature. Furthermore, we discuss the altered function of astrocytes in their interaction with neurons in the preservation of glutamate homeostasis and additionally address the role of astrocytes at the vascular interface and their contribution to functional hyperemia within the living murine brain in health and in AD.
Introduction
Recent global estimates indicate that over 46 million individuals are living with dementia (Prince, 2017) and current clinical therapies are unable to slow progressive neurodegeneration. It is expected that by the year 2050, over 131 million people will have dementia (Prince, 2017). Alzheimer’s disease (AD) is the most prevalent form of dementia and aging is the strongest risk factor and driver of disease progression (De Strooper and Karran, 2016). Synaptic dysfunction is an important neuropathological correlate of cognitive impairment in individuals with AD (Terry et al., 1991; DeKosky et al., 1996; Serrano-Pozo et al., 2011; Selkoe and Hardy, 2016) but causative mechanisms remain elusive. An unbiased stereological analyses of a large cohort of human AD brain tissues asserted that the number of reactive astrocytes increased linearly with increasing disease severity and amyloid plaque burden reached a plateau early in the pathogenesis of AD (Serrano-Pozo et al., 2011). Reactive astrocytes undergo a complex cascade of morphological alterations that include hypertrophy and the upregulation of cytoskeleton proteins such as glial fibrillary acidic protein (GFAP) and vimentin, which may interfere with the multitude of complex physiological homeostatic functions conducted by astrocytes and contribute to the evolution of AD. Indeed, position emission tomography (PET) imaging studies using the tracer 11C-deuterium-L-deprenyl, directed towards the monoamine oxidase B enzyme, reported that reactive astrocytes are present in patients at early stages of AD, particularly those patients with Mild Cognitive Impairment (MCI) (Carter et al., 2012) and that reactive astrocytes precede amyloid plaques in a mouse model of AD (Rodriguez-Vieitez et al., 2015).
In Vivo Imaging of Astrocytes Within the Intact Murine Brain
The normal morphology of astrocytes within the murine brain consists of a central core, occupying approximately 25 percent of the volume of the entire cell, which can range from 3000–35,000 μm3 (Bindocci et al., 2017). The perivascular endfoot processes occupy only 3.5 percent of cellular volume and contribute to a continuous lining on the abluminal surface of cerebral vessels (McCaslin et al., 2011). Perivascular endfoot processes express a rich complement of membrane molecules that include potassium and water channels that are important for ionic and osmotic regulation (Yang et al., 2011). The remaining cellular volume is occupied by approximately 3–9 processes radiating from the soma, each with an estimated length ranging between 5–64 μm, in addition to a peripheral region densely populated by very fine and optically unresolvable processes (Bindocci et al., 2017). Astrocytes have been studied extensively in vitro, thus providing a useful proxy for astrocytes in vivo (Cahoy et al., 2008), however astrocytes exist within artificial experimental conditions in vitro and may express immune system genes not expressed by astrocytes in vivo (Cahoy et al., 2008). Therefore, experiments testing hypotheses pertaining to the structure and function of astrocytes in health and in AD should aim to preserve the intricate interrelations between astrocytes, neurons and the vasculature by examining astrocytic signaling in vivo, which permits discrimination between physiological and pharmacological effects (Khakh and McCarthy, 2015).
Two photon excitation laser scanning microscopy is especially well suited to the in vivo study of the morphology and function of fluorescently labeled astrocytes, throughout hundreds of microns of intact cortex within rodent brain (Denk et al., 1990; Svoboda and Yasuda, 2006). Two photon microscopy offers a tight laser focus of highly localized excitation in contrast to using either wide-field or confocal microscopy, in which a scattering induced loss of excitation photons can greatly reduce imaging depth (Svoboda and Yasuda, 2006). Furthermore, mice can be trained to become habituated to head-restraint so that they are awake during two-photon imaging, thus circumventing the need for using general anesthesia (Shih et al., 2012a).
The in vivo imaging of astrocytes within the living mouse brain by two-photon microscopy requires an aseptic surgical implantation of a chronic cranial window in which a portion of skull bone (0.8–12 mm in diameter; Holtmaat et al., 2009) is replaced with a coverslip, that can be tightly sealed using dental cement. The degree of surgical skill elicited during preparation of the cranial window will largely determine the quality and duration of optical clarity of the window, which has been shown to be suited for repeated imaging for at least 6 months (Hefendehl et al., 2011). Alternatively, a small area of murine skull bone (2 mm in diameter; Shih et al., 2012b) can be thinned using a microsurgical blade to a final thickness of approximately 10–20 μm (Holtmaat et al., 2009; Drew et al., 2010; Shih et al., 2012b). Additional skull polishing, cryanoacrylate adhesive and a coverslip can help stabilize the weakened skull bone and prolong optical access to thinned skull preparations, permitting repeated two-photon microscopic examination of astrocytes within the living murine cortex for up to 3 months, without need for repeated surgery (Shih et al., 2012b). The chronic cranial window and thinned skull preparation are each technically challenging but both procedures, once mastered, can be conducted without inducing astrogliosis (Drew et al., 2010; Hefendehl et al., 2011; Zhang et al., 2015).
In Vivo Imaging of Astrocytic Calcium Dynamics in AD Mouse Models
The physiological function of astrocytes is intricately coupled with a multitude of spontaneous oscillations in the concentration of intracellular calcium ions that have considerable spatial and temporal heterogeneity throughout the astrocyte (see Supplementary Figure S1; Bazargani and Attwell, 2016; Bindocci et al., 2017). It remains incompletely understood how the spontaneous calcium activity of astrocytes is decoded into local and global neurovascular function in brain health and in AD (Bazargani and Attwell, 2016; Bindocci et al., 2017). Fluorescent calcium indicators such as fura-2, fluo-3, fluo-4 and Oregon Green 488 BAPTA-1 (OGB-1) permit observation of the complex and asynchronous calcium signaling occurring within and between murine astrocytes in vitro (Cornell-Bell et al., 1990; Glaum et al., 1990; Jensen and Chiu, 1990; Charles et al., 1991), in situ (Dani et al., 1992) and in vivo (Hirase et al., 2004; Kuchibhotla et al., 2009). In particular, the acetoxymethyl ester form of OGB-1 (OGB-1AM) can be delivered by pressurized ejection from a micropipette at a depth of approximately 100–200 μm below the murine cortical surface (Stosiek et al., 2003). Concomitant use of the fluorescent dye, sulforhodamine 101 (SR-101) provides a method for discriminating astrocytes from other cells within the living mouse cortex because the multi-cell bolus loading of calcium sensitive dyes results in a universal labeling of all cells (Nimmerjahn et al., 2004). SR-101 can be delivered to astrocytes within the living brain of mice under anesthesia by either carefully perforating the dura, ensuring minimal damage to cortical blood vessels, or by intracortical injection using a micropipette (Nimmerjahn and Helmchen, 2012). The SR-101 dye is actively taken up by astrocytes and diffusional spread occurs through the astrocytic syncytium via astrocytic gap junctions, permitting in vivo imaging over several hours (see Figure 1A). Experimental protocols requiring chronic in vivo imaging of astrocytes over days or weeks can be technically challenging considering the requirement to deliver SR-101 into each mouse brain prior to each imaging session (Nimmerjahn et al., 2004; Nimmerjahn and Helmchen, 2012), which involves the re-opening of the cranial window.
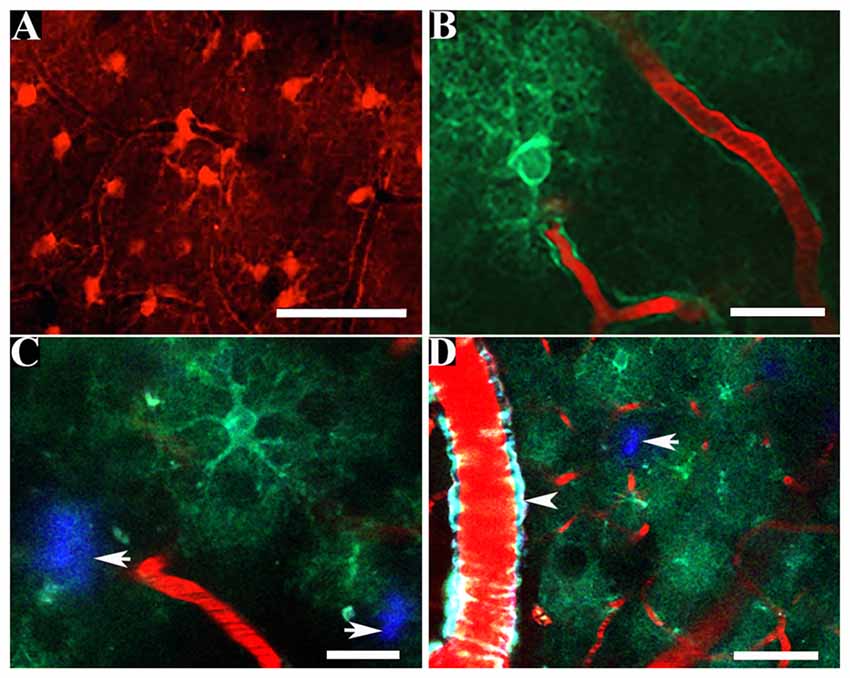
Figure 1. In vivo multiphoton imaging of astrocytic structure within the living mouse brain. Astrocytes fluorescently labeled by topical application of sulforhodamine 101 (SR-101) reveal astrocytic somas, proximal processes and perivascular endfoot processes (A) whereas the intracortical injection of a virally transduced genetically encoded calcium indicator (GECI) can be used to reveal the finer astrocytic processes in vivo (B). GECIs can be transduced by astrocytes within the amyloid plaque containing brain of a murine model of Alzheimer’s disease (C,D). Amyloid plaques are fluorescently labeled by methoxy-X04 (white arrows; C,D). The presence of a vessel affected by cerebral amyloid angiopathy (CAA) (positive for methoxy-X04) is clearly visible (white arrowhead; D). Scale bar = 50 μm (A,D), 20 μm (B,C).
The topical application of SR-101 onto the brain of 5–9 months old APPswe/PS1dE9 (APP/PS1) transgenic AD mice (Jankowsky et al., 2001) with subsequent in vivo imaging by two-photon microscopy revealed that astrocytes retain their exclusive and highly organized domains in the presence of amyloid plaques, thus supporting the hypothesis that amyloid plaques do not exert chemotactic effects on astrocytes within the living murine brain (Galea et al., 2015). Additionally, a concomitant multi-cell bolus loading of OGB-1AM with a subsequent intracortical injection of SR-101 following surgical implantation of a cranial window provided optical access to measure calcium activity in astrocytes within the somatosensory cortex of the living brain of APP/PS1 transgenic and age-matched wild-type mice (Kuchibhotla et al., 2009). This study demonstrated that in the living brain of 6–8 months old APP/PS1 transgenic mice, which progressively accumulates amyloid plaques from 4–5 months of age (Jankowsky et al., 2001; Garcia-Alloza et al., 2006), there is a >80% increase in spontaneous intracellular calcium activity within astrocytes when compared to age-matched wild-type mice (Kuchibhotla et al., 2009). Additionally, astrocytes within amyloid plaque containing brain regions in the intact brain of APP/PS1 transgenic mice exhibit spontaneous intercellular propagating calcium waves that occur independently of neuronal activity (Kuchibhotla et al., 2009). Reactive astrocytes associated with amyloid plaques exhibit an increased expression of the purinergic P2Y1 receptor (P2Y1R; Delekate et al., 2014) and transgenic mice that overexpress the P2Y1R exhibit spontaneous intercellular propagating calcium waves with increased amplitude that are independent of local neuronal activity (Shigetomi et al., 2018). These findings suggest that astrocytes spatially associated with amyloid plaques may propagate global astrocytic calcium waves that are at least partly mediated by purinergic signaling and occur independently of synaptic transmission.
The finer perisynaptic processes of astrocytes (see Figure 1B) are the predominant intracellular sites of initiation for the spontaneous astrocytic calcium waves and are thus important for interrogating the interrelationship between astrocytic calcium signaling and synaptic function in health and in the course of AD (Shigetomi et al., 2018; see Figure 1C). A Sholl morphological analyses of astrocytes bulk loaded with the commonly used calcium sensitive dyes reported that the detection of astrocytic calcium activity was limited to the soma and proximal processes to a radial distance of approximately 20 μm, with no detection of the calcium dynamics in the finer processes (Reeves et al., 2011). Genetically encoded calcium indicators (GECIs), such as the Fluorescence Resonance Energy Transfer (FRET)-based Cameleons (Miyawaki et al., 1997; Nagai et al., 2004) and the single wavelength GCaMP series (Nakai et al., 2001) offer a uniform, stable and specific fluorescent labeling of astrocytes and their processes to a radial distance of approximately 50 μm, thus resolving the finer perisynaptic processes (Shigetomi et al., 2013).
Cameleon (yellow cameleon; yc2.60 or yc3.60) consist of a calmodulin moiety fused to a M13 calmodulin binding peptide and two fluorescent proteins (cyan-yellow FRET pair) (Miyawaki et al., 1997). The binding of free calcium to calmodulin results in an intramolecular rearrangement which aids the energy transfer between both fluorescent proteins leading to a change in fluorescence ratio (Pérez Koldenkova and Nagai, 2013). These GECIs are known as ratiometric because the ratio between the fluorescence emission intensities of both fluorescent proteins reflects the concentration of free cytosolic calcium (Pérez Koldenkova and Nagai, 2013). The GCaMP series (GCaMP3, GCaMP5, GCaMP6) comprise an intensiometric chimeric construct consisting of a circularly permutated green fluorescent protein that exhibits an altered emission fluorescence intensity upon the binding of free calcium (Nakai et al., 2001). Thus, they are useful for measuring dynamic changes in intracellular calcium but not for quantitative measures of concentration. The astrocytic-specific expression of GECIs can be achieved by the transduction of an adeno-associated virus of the 2/5 serotype with a promoter such as gfaABC1D (Shigetomi et al., 2013) that can be injected into the brain region of interest of anesthetized mice (Jiang et al., 2014). GECIs can also be targeted to the astrocytic plasma membrane by an additional membrane-tethering domain thus increasing exposure to subcellular calcium dynamics resulting in the detection of a significantly greater number of calcium signals within astrocytic somas and processes when compared to the bulk loading of Fluo-4 AM (Shigetomi et al., 2010, 2013).
In Vivo Imaging of Astrocytes in Functional Hyperemia
Physiological brain function is heavily dependent upon a continuous and uninterrupted blood supply that can adapt to meet the metabolic needs of active brain regions (Girouard and Iadecola, 2006). This complex phenomenon, termed functional hyperemia, involves a sophisticated spatiotemporally correlated interplay between neurons, astrocytes and local hemodynamic responses, mediated by smooth muscle cells and endothelial cells (Zonta et al., 2003; Girouard and Iadecola, 2006; MacVicar and Newman, 2015; Tarantini et al., 2017). Impaired functional hyperemia is evident in individuals with AD at mild severity (Janik et al., 2016) and in transgenic AD mice (Niwa et al., 2000; Park et al., 2004). Therefore, deciphering the functional relationship between neurons, astrocytes and the vasculature is critical to further understanding of the pathogenesis of AD. Acute brain slices have provided useful demonstration of evoked synaptic release of glutamate which triggers propagating oscillations of intracellular calcium ions in astrocytic endfeet with temporally correlated release of vasoactive metabolites that elicit hemodynamic responses (Zonta et al., 2003; Mulligan and MacVicar, 2004; Bazargani and Attwell, 2016). However, acute brain slices are devoid of intraluminal flow required for removing vasoactive agents and for preserving myogenic tone, thus the complexities underlying the role of astrocytes in functional hyperemia may best be resolved in vivo using two photon microscopy, independently of the use of anesthesia (Zonta et al., 2003; Girouard and Iadecola, 2006; Takano et al., 2006; Bazargani and Attwell, 2016).
Sensory stimulation of the living murine barrel cortex in vivo evokes calcium signaling in neurons and astrocytes with instantaneous hemodynamic responses that correlate with elevated calcium activity in astrocytic somas and perivascular endfeet but not within parenchymal astrocytic processes or neuronal somas (Lind et al., 2013). Independent and isolated calcium responses in astrocytic perivascular endfeet, elicited by sensory stimulation in mouse brain in vivo, was most closely associated with the vasodilatory hemodynamic responses to neuronal activity (Lind et al., 2018). Additionally, evoked perivascular endfeet calcium responses in vivo was similar at penetrating arterioles and capillaries and the temporal dynamics were similar when labeled with either OGB-1AM/SR-101 or the GECI GCaMP6f (Lind et al., 2018). An in vivo examination of the spatiotemporal oscillations in calcium signaling in astrocytic perivascular endfeet concomitantly with changes in cerebral vascular diameters and/or cerebral blood flow requires a robust fluorescent labeling of perivascular endfeet that can be difficult to achieve when using the bulk loading of calcium sensitive indicators, such as OGB-1AM (Delekate et al., 2014).
In Vivo Imaging of Glutamate Dynamics in AD
Glutamatergic signaling dysfunction is a significant pathological component within the human AD brain (Jacob et al., 2007) and in transgenic murine models of AD (Masliah et al., 2000; Hefendehl et al., 2016). Optimal physiological glutamatergic signaling is mediated by the interplay between neurons and astrocytes thus protecting post-synaptic glutamate receptors from chronic overstimulation, which can lead to neuronal injury and death. The tightly controlled calcium-dependent release of glutamate from neurons activates post-synaptic N-methyl-D-aspartate (NMDA) and alpha-amino-3-hydroxy-5-methyl-4-isoxazolepropionic acid (AMPA) glutamate receptors to increase intracellular calcium and sodium and evoke action potentials (Lin et al., 2012). Astrocytes predominantly clear glutamate via functional excitatory amino-acid transporters (EAATs) and convert glutamate to glutamine for neurons which convert glutamine to glutamate for storage and release from synaptic vesicles (Lin et al., 2012). Significant gene and protein alterations of the NMDA and AMPA glutamate receptors and EAAT1 and EAAT2 glutamate transporters was detected in the hippocampus and frontal cortex of human AD brain tissues (Jacob et al., 2007). Immunohistochemical analyses of human AD brain tissues reported loss of astrocytic EAAT receptor expression in close proximity to amyloid plaques (Jacob et al., 2007) with a trend for decreased immunoreactivity of EAAT2 with increasing Braak stage, which was inversely correlated with increasing GFAP immunoreactivity in astrocytes with disease duration (Simpson et al., 2010).
Spontaneous or sensory evoked local glutamate dynamics within the intact murine brain can be evaluated using two-photon imaging of the genetically encoded intensity-based glutamate sensing fluorescent reporter (iGluSnFR; Marvin et al., 2013; Hefendehl et al., 2016), which has greater temporal resolution than the microdialysis technique (Marvin et al., 2013; Cifuentes Castro et al., 2014). Multiphoton microscopic examination of the iGluSnFR within the intact brain of APP/PS1 transgenic mice (Radde et al., 2006) reported that the plaque microenvironment had aberrant spontaneous fluctuations in the local glutamate concentration, reduced glutamate clearance following sensory stimulation and loss of the murine glutamate receptor-1 (GLT-1/EAAT2; Hefendehl et al., 2016). The selective deletion of GLT-1 in murine astrocytes resulted in spontaneous seizures and premature death, which was not evident following the selective deletion of GLT-1 in neurons (Petr et al., 2015). The administration of the beta-lactam antibiotic, ceftriaxone to APP/PS1 mice partly restored GLT-1 expression and improved the glutamatergic activity in vivo (Hefendehl et al., 2016). This suggests that aberrant astrocytic function contributes to the toxic microenvironment of amyloid plaques in AD and may be amenable to pharmacological intervention.
Future Perspectives
In 1909, Santiago Ramón y Cajal proposed that the physiological role of glia was incompletely understood because physiologists did not have the necessary tools to examine glial function (Somjen, 1988; Barres et al., 1990). The advent of new tools for the in vivo imaging of astrocytes within the intact murine brain may accelerate the generation of new hypotheses for elucidating the roles of astrocytes within normal physiology and in the pathogenesis of AD. The segmental accumulation of amyloid peptide in the walls of cerebral arteries and capillaries is termed cerebral amyloid angiopathy (CAA; see Figure 1D) and is associated with intracerebral hemorrhages and degenerative morphological changes to amyloid peptide laden vessels (Biffi and Greenberg, 2011). Post-mortem confirmed AD brain tissues exhibit CAA in >90% of cases (Kalaria and Ballard, 1999; Jellinger, 2002) and astrocytic perivascular endfeet that are spatially associated with vessels moderately affected by CAA exhibit reduced expression of the bidirectional water channel, aquaporin-4 (AQP4), in the medial temporal lobe of human AD brain tissues (Wilcock et al., 2009). Transmission electron microscopic examination of brain sections from the Tg-ArcSwe transgenic mouse model of AD (Lord et al., 2006) showed close spatial association between astrocytic perivascular endfeet and amyloid peptide on cerebral vessels, which disrupts the close interaction between astrocytic endfeet and endothelium (Yang et al., 2011). Correspondingly, immunohistochemical analysis of brain sections from the arcAβ transgenic mouse model of AD (Knobloch et al., 2007) showed the retraction and loss of astrocytic perivascular endfoot processes at amyloid peptide laden vessels and parenchymal amyloid plaques at early stages of pathology (Merlini et al., 2011). The development of new probes to specifically target AQP4 in vivo would permit the discrimination of perivascular endfeet from astrocytic processes in vivo. Additionally, the combined use of a specific fluorescent probe for AQP4 with the systemic administration of methoxy-X04 (Klunk et al., 2002) may permit a longitudinal examination of the spatial association between the morphology of astrocytic perivascular endfeet and CAA within the intact brain of murine models of AD. The development of fluorescent probes to target astrocytic cytoskeletal proteins such as GFAP and/or vimentin would permit the reactive phenotype of astrocytes and their spatial association with amyloid plaques and CAA to be examined longitudinally within murine models of AD and should prove to be powerful. The reactive astrocytic phenotype is heterogeneous and the nature of the cerebral insult can determine whether astrocytes adopt a neurotoxic reactive phenotype (termed A1) or adopt the A2 reactive subtype, which may promote neuronal health (Zamanian et al., 2012; Liddelow et al., 2017). The development of fluorescent labeling tools capable of discriminating between reactive subtypes may provide an excellent opportunity to assess the reactive phenotype in astrocytes in AD murine models so that the pharmacological effects of modulating astrocytes may be assessed longitudinally. A previous in vivo two-photon microscopy study demonstrated that the hyperactive calcium signaling phenotype exhibited by astrocytes within the cortex of APP/PS1 mice is amenable to pharmacological modulation (Delekate et al., 2014). The frequency of astrocytes exhibiting hyperactive calcium signaling was reduced following topical application of antagonists of purinergic signaling and increased by elevating the concentration of ADP nucleotides within the cortex of anesthetized APP/PS1 mice (Delekate et al., 2014). Additionally, an in vivo two photon microscopy study that examined the effects of chronic antagonism of the purinergic signaling within the intact brain of APP/PS1 mice reported a reduction in the fraction of hyperactive astrocytes associated with preservation of synaptic structure and function (Reichenbach et al., 2018). These original research findings collectively support the current relevance of using an in vivo two-photon imaging-based interrogation of astrocytes in AD which enables the testing of candidate therapies directed towards protecting the integrity of the neurovascular unit against the pathological impact of AD.
Author Contributions
PK, EH, SH and BB prepared the manuscript and figures.
Funding
This work was supported by National Institutes of Health (NIH) R01 AG054598.
Conflict of Interest Statement
The authors declare that the research was conducted in the absence of any commercial or financial relationships that could be construed as a potential conflict of interest.
Supplementary Material
The Supplementary Material for this article can be found online at: https://www.frontiersin.org/articles/10.3389/fnagi.2018.00219/full#supplementary-material
FIGURE S1 | In vivo multiphoton imaging of the spatiotemporal intracellular astrocytic calcium dynamics within the living brain of a mouse model of Alzheimer’s disease (AD). The astrocyte expresses the virally transduced genetically encoded calcium indicator (GECI) YC3.6 delivered by intracortical injection of the AAV followed by surgical implantation of a cranial window. The 10-min time course video demonstrates spontaneous oscillations in calcium throughout an astrocyte with the ratiometric changes in calcium concentration pseudo colored from blues to red.
References
Barres, B. A., Chun, L. L., and Corey, D. P. (1990). Ion channels in vertebrate glia. Annu. Rev. Neurosci. 13, 441–474. doi: 10.1146/annurev.ne.13.030190.002301
Bazargani, N., and Attwell, D. (2016). Astrocyte calcium signaling: the third wave. Nat. Neurosci. 19, 182–189. doi: 10.1038/nn.4201
Biffi, A., and Greenberg, S. M. (2011). Cerebral amyloid angiopathy: a systematic review. J. Clin. Neurol. 7, 1–9. doi: 10.3988/jcn.2011.7.1.1
Bindocci, E., Savtchouk, I., Liaudet, N., Becker, D., Carriero, G., and Volterra, A. (2017). Three-dimensional Ca2+ imaging advances understanding of astrocyte biology. Science 356:eaai8185. doi: 10.1126/science.aai8185
Cahoy, J. D., Emery, B., Kaushal, A., Foo, L. C., Zamanian, J. L., Christopherson, K. S., et al. (2008). A transcriptome database for astrocytes, neurons, and oligodendrocytes: a new resource for understanding brain development and function. J. Neurosci. 28, 264–278. doi: 10.1523/JNEUROSCI.4178-07.2008
Carter, S. F., Schöll, M., Almkvist, O., Wall, A., Engler, H., Långström, B., et al. (2012). Evidence for astrogliosis in prodromal Alzheimer disease provided by 11C-deuterium-L-deprenyl: a multitracer PET paradigm combining 11C-Pittsburgh compound B and 18F-FDG. J. Nucl. Med. 53, 37–46. doi: 10.2967/jnumed.110.087031
Charles, A. C., Merrill, J. E., Dirksen, E. R., and Sanderson, M. J. (1991). Intercellular signaling in glial cells: calcium waves and oscillations in response to mechanical stimulation and glutamate. Neuron 6, 983–992. doi: 10.1016/0896-6273(91)90238-u
Cifuentes Castro, V. H., López Valenzuela, C. L., Salazar Sánchez, J. C., Peńa, K. P., López Pérez, S. J., Ibarra, J. O., et al. (2014). An update of the classical and novel methods used for measuring fast neurotransmitters during normal and brain altered function. Curr. Neuropharmacol. 12, 490–508. doi: 10.2174/1570159x13666141223223657
Cornell-Bell, A. H., Finkbeiner, S. M., Cooper, M. S., and Smith, S. J. (1990). Glutamate induces calcium waves in cultured astrocytes: long-range glial signaling. Science 247, 470–473. doi: 10.1126/science.1967852
Dani, J. W., Chernjavsky, A., and Smith, S. J. (1992). Neuronal activity triggers calcium waves in hippocampal astrocyte networks. Neuron 8, 429–440. doi: 10.1016/0896-6273(92)90271-e
DeKosky, S. T., Scheff, S. W., and Styren, S. D. (1996). Structural correlates of cognition in dementia: quantification and assessment of synapse change. Neurodegeneration 5, 417–421. doi: 10.1006/neur.1996.0056
Delekate, A., Füchtemeier, M., Schumacher, T., Ulbrich, C., Foddis, M., and Petzold, G. C. (2014). Metabotropic P2Y1 receptor signalling mediates astrocytic hyperactivity in vivo in an Alzheimer’s disease mouse model. Nat. Commun. 5:5422. doi: 10.1038/ncomms6422
Denk, W., Strickler, J. H., and Webb, W. W. (1990). Two-photon laser scanning fluorescence microscopy. Science 248, 73–76. doi: 10.1126/science.2321027
De Strooper, B., and Karran, E. (2016). The cellular phase of Alzheimer’s disease. Cell 164, 603–615. doi: 10.1016/j.cell.2015.12.056
Drew, P. J., Shih, A. Y., Driscoll, J. D., Knutsen, P. M., Blinder, P., Davalos, D., et al. (2010). Chronic optical access through a polished and reinforced thinned skull. Nat. Methods 7, 981–984. doi: 10.1038/nmeth.1530
Galea, E., Morrison, W., Hudry, E., Arbel-Ornath, M., Bacskai, B. J., Gómez-Isla, T., et al. (2015). Topological analyses in APP/PS1 mice reveal that astrocytes do not migrate to amyloid-β plaques. Proc. Natl. Acad. Sci. U S A 112, 15556–15561. doi: 10.1073/pnas.1516779112
Garcia-Alloza, M., Robbins, E. M., Zhang-Nunes, S. X., Purcell, S. M., Betensky, R. A., Raju, S., et al. (2006). Characterization of amyloid deposition in the APPswe/PS1dE9 mouse model of Alzheimer disease. Neurobiol. Dis. 24, 516–524. doi: 10.1016/j.nbd.2006.08.017
Girouard, H., and Iadecola, C. (2006). Neurovascular coupling in the normal brain and in hypertension, stroke, and Alzheimer’s disease. J. Appl. Physiol. 100, 328–335. doi: 10.1152/japplphysiol.00966.2005
Glaum, S. R., Holzwarth, J. A., and Miller, R. J. (1990). Glutamate receptors activate Ca2+ mobilization and Ca2+ influx into astrocytes. Proc. Natl. Acad. Sci. U S A 87, 3454–3458. doi: 10.1073/pnas.87.9.3454
Hefendehl, J. K., LeDue, J., Ko, R. W., Mahler, J., Murphy, T. H., and MacVicar, B. A. (2016). Mapping synaptic glutamate transporter dysfunction in vivo to regions surrounding Aβ plaques by iGluSnFR two-photon imaging. Nat. Commun. 7:13441. doi: 10.1038/ncomms13441
Hefendehl, J. K., Wegenast-Braun, B. M., Liebig, C., Eicke, D., Milford, D., Calhoun, M. E., et al. (2011). Long-term in vivo imaging of β-amyloid plaque appearance and growth in a mouse model of cerebral β-amyloidosis. J. Neurosci. 31, 624–629. doi: 10.1523/JNEUROSCI.5147-10.2011
Hirase, H., Qian, L., Barthó, P., and Buzsáki, G. (2004). Calcium dynamics of cortical astrocytic networks in vivo. PLoS Biol. 4:e96. doi: 10.1371/journal.pbio.0020096
Holtmaat, A., Bonhoeffer, T., Chow, D. K., Chuckowree, J., DePaola, V., Hofer, S. B., et al. (2009). Long-term, high-resolution imaging in the mouse neocortex through a chronic cranial window. Nat. Protoc. 4, 1128–1144. doi: 10.1038/nprot.2009.89
Jacob, C. P., Koutsilieri, E., Bartl, J., Neuen-Jacob, E., Arzberger, T., Zander, N., et al. (2007). Alterations in expression of glutamatergic transporters and receptors in sporadic Alzheimer’s disease. J. Alzheimers Dis. 11, 97–116. doi: 10.3233/jad-2007-11113
Janik, R., Thomason, L. A., Chaudhary, S., Dorr, A., Scouten, A., Schwindt, G., et al. (2016). Attenuation of functional hyperemia to visual stimulation in mild Alzheimer’s disease and its sensitivity to cholinesterase inhibition. Biochim. Biophys. Acta 1862, 957–965. doi: 10.1016/j.bbadis.2015.10.023
Jankowsky, J. L., Slunt, H. H., Ratovitski, T., Jenkins, N. A., Copeland, N. G., and Borchelt, D. R. (2001). Co-expression of multiple transgenes in mouse CNS: a comparison of strategies. Biomol. Eng. 17, 157–165. doi: 10.1016/s1389-0344(01)00067-3
Jellinger, K. A. (2002). Alzheimer disease and cerebrovascular pathology: an update. J. Neural Transm. 109, 813–836. doi: 10.1007/s007020200068
Jensen, A. M., and Chiu, S. Y. (1990). Fluorescence measurement of changes in intracellular calcium induced by excitatory amino acids in cultured cortical astrocytes. J. Neurosci. 10, 1165–1175. doi: 10.1523/JNEUROSCI.10-04-01165.1990
Jiang, R., Haustein, M. D., Sofroniew, M. V., and Khakh, B. S. (2014). Imaging intracellular Ca2+ signals in striatal astrocytes from adult mice using genetically-encoded calcium indicators. J. Vis. Exp. 93:e51972. doi: 10.3791/51972
Kalaria, R. N., and Ballard, C. (1999). Overlap between pathology of Alzheimer disease and vascular dementia. Alzheimer Dis. Assoc. Disord. 3, 115–123. doi: 10.1097/00002093-199912003-00017
Khakh, B. S., and McCarthy, K. D. (2015). Astrocyte calcium signaling: from observations to functions and the challenges therein. Cold Spring Harb. Perspect. Biol. 7:a020404. doi: 10.1101/cshperspect.a020404
Klunk, W. E., Bacskai, B. J., Mathis, C. A., Kajdasz, S. T., McLellan, M. E., Frosch, M. P., et al. (2002). Imaging Abeta plaques in living transgenic mice with multiphoton microscopy and methoxy-X04, a systemically administered Congo Red derivative. J. Neuropathol. Exp. Neurol. 61, 797–805. doi: 10.1093/jnen/61.9.797
Knobloch, M., Konietzko, U., Krebs, D. C., and Nitsch, R. M. (2007). Intracellular Aβ and cognitive deficits precede beta-amyloid deposition in transgenic arcAbeta mice. Neurobiol. Aging 28, 1297–1306. doi: 10.1016/j.neurobiolaging.2006.06.019
Kuchibhotla, K. V., Lattarulo, C. R., Hyman, B. T., and Bacskai, B. J. (2009). Synchronous hyperactivity and intercellular calcium waves in astrocytes in Alzheimer mice. Science 323, 1211–1215. doi: 10.1126/science.1169096
Liddelow, S. A., Guttenplan, K. A., Clarke, L. E., Bennett, F. C., Bohlen, C. J., Schirmer, L., et al. (2017). Neurotoxic reactive astrocytes are induced by activated microglia. Nature 541, 481–487. doi: 10.1038/nature21029
Lin, C. L., Kong, Q., Cuny, G. D., and Glicksman, M. A. (2012). Glutamate transporter EAAT2: a new target for the treatment of neurodegenerative diseases. Future Med. Chem. 4, 1689–1700. doi: 10.4155/fmc.12.122
Lind, B. L., Brazhe, A. R., Jessen, S. B., Tan, F. C., and Lauritzen, M. J. (2013). Rapid stimulation-evoked astrocyte Ca2+ elevations and hemodynamic responses in mouse somatosensory cortex in vivo. Proc. Natl. Acad. Sci. U S A 110, E4678–E4687. doi: 10.1073/pnas.1310065110
Lind, B. L., Jessen, S. B., Lønstrup, M., Joséphine, C., Bonvento, G., and Lauritzen, M. (2018). Fast Ca2+ responses in astrocyte end-feet and neurovascular coupling in mice. Glia 66, 348–358. doi: 10.1002/glia.23246
Lord, A., Kalimo, H., Eckman, C., Zhang, X. Q., Lannfelt, L., and Nilsson, L. N. (2006). The Arctic Alzheimer mutation facilitates early intraneuronal Abeta aggregation and senile plaque formation in transgenic mice. Neurobiol. Aging 27, 67–77. doi: 10.1016/j.neurobiolaging.2004.12.007
MacVicar, B. A., and Newman, E. A. (2015). Astrocyte regulation of blood flow in the brain. Cold Spring Harb. Perspect. Biol. 7:a020388. doi: 10.1101/cshperspect.a020388
Marvin, J. S., Borghuis, B. G., Tian, L., Cichon, J., Harnett, M. T., Akerboom, J., et al. (2013). An optimized fluorescent probe for visualizing glutamate neurotransmission. Nat. Methods 10, 162–170. doi: 10.1038/nmeth.2333
Masliah, E., Alford, M., Mallory, M., Rockenstein, E., Moechars, D., and Van Leuven, F. (2000). Abnormal glutamate transport function in mutant amyloid precursor protein transgenic mice. Exp. Neurol. 163, 381–387. doi: 10.1006/exnr.2000.7386
McCaslin, A. F., Chen, B. R., Radosevich, A. J., Cauli, B., and Hillman, E. M. (2011). In vivo 3D morphology of astrocyte-vasculature interactions in the somatosensory cortex: implications for neurovascular coupling. J. Cereb. Blood Flow Metab. 31, 795–806. doi: 10.1038/jcbfm.2010.204
Merlini, M., Meyer, E. P., Ulmann-Schuler, A., and Nitsch, R. M. (2011). Vascular β-amyloid and early astrocyte alterations impair cerebrovascular function and cerebral metabolism in transgenic arcAβ mice. Acta Neuropathol. 122, 293–311. doi: 10.1007/s00401-011-0834-y
Miyawaki, A., Llopis, J., Heim, R., McCaffery, J. M., Adams, J. A., Ikura, M., et al. (1997). Fluorescent indicators for Ca2+ based on green fluorescent proteins and calmodulin. Nature 388, 882–887. doi: 10.1038/42264
Mulligan, S. J., and MacVicar, B. A. (2004). Calcium transients in astrocyte endfeet cause cerebrovascular constrictions. Nature 431, 195–199. doi: 10.1038/nature02827
Nagai, T., Yamada, S., Tominaga, T., Ichikawa, M., and Miyawaki, A. (2004). Expanded dynamic range of fluorescent indicators for Ca2+ by circularly permuted yellow fluorescent proteins. Proc. Natl. Acad. Sci. U S A 101, 10554–10559. doi: 10.1073/pnas.0400417101
Nakai, J., Ohkura, M., and Imoto, K. (2001). A high signal-to-noise Ca2+ probe composed of a single green fluorescent protein. Nat. Biotechnol. 19, 137–141. doi: 10.1038/84397
Nimmerjahn, A., and Helmchen, F. (2012). In vivo labeling of cortical astrocytes with sulforhodamine 101 (SR101). Cold Spring Harb. Protoc. 2012, 326–334. doi: 10.1101/pdb.prot068155
Nimmerjahn, A., Kirchhoff, F., Kerr, J. N., and Helmchen, F. (2004). Sulforhodamine 101 as a specific marker of astroglia in the neocortex in vivo. Nat. Methods 1, 31–37. doi: 10.1038/nmeth706
Niwa, K., Younkin, L., Ebeling, C., Turner, S. K., Westaway, D., Younkin, S., et al. (2000). Aβ1–40-related reduction in functional hyperemia in mouse neocortex during somatosensory activation. Proc. Natl. Acad. Sci. U S A 97, 9735–9740. doi: 10.1073/pnas.97.17.9735
Park, L., Anrather, J., Forster, C., Kazama, K., Carlson, G. A., and Iadecola, C. (2004). Aβ-induced vascular oxidative stress and attenuation of functional hyperemia in mouse somatosensory cortex. J. Cereb. Blood Flow Metab. 24, 334–342. doi: 10.1097/01.wcb.0000105800.49957.1e
Pérez Koldenkova, V., and Nagai, T. (2013). Genetically encoded Ca2+ indicators: properties and evaluation. Biochim. Biophys. Acta 1833, 1787–1797. doi: 10.1016/j.bbamcr.2013.01.011
Petr, G. T., Sun, Y., Fredrick, N. M., Zhou, Y., Dhamne, S. C., Hameed, M. Q., et al. (2015). Conditional deletion of the glutamate transporter GLT-1 reveals that astrocytic GLT-1 protects against fatal epilepsy while neuronal GLT-1 contributes significantly to glutamate uptake into synaptosomes. J. Neurosci. 35, 5187–5201. doi: 10.1523/JNEUROSCI.4255-14.2015
Prince, M. (2017). Progress on dementia-leaving no one behind. Lancet 390, e51–e53. doi: 10.1016/s0140-6736(17)31757-9
Radde, R., Bolmont, T., Kaeser, S. A., Coomaraswamy, J., Lindau, D., Stoltze, L., et al. (2006). Abeta42-driven cerebral amyloidosis in transgenic mice reveals early and robust pathology. EMBO Rep. 7, 940–946. doi: 10.1038/sj.embor.7400784
Reeves, A. M., Shigetomi, E., and Khakh, B. S. (2011). Bulk loading of calcium indicator dyes to study astrocyte physiology: key limitations and improvements using morphological maps. J. Neurosci. 31, 9353–9358. doi: 10.1523/JNEUROSCI.0127-11.2011
Reichenbach, N., Delekate, A., Breithausen, B., Keppler, K., Poll, S., Schulte, T., et al. (2018). P2Y1 receptor blockade normalizes network dysfunction and cognition in an Alzheimer’s disease model. J. Exp. Med. 215, 1649–1663. doi: 10.1084/jem.20171487
Rodriguez-Vieitez, E., Ni, R., Gulyás, B., Tóth, M., Häggkvist, J., Halldin, C., et al. (2015). Astrocytosis precedes amyloid plaque deposition in Alzheimer APPswe transgenic mouse brain: a correlative positron emission tomography and in vitro imaging study. Eur. J. Nucl. Med. Mol. Imaging 42, 1119–1132. doi: 10.1007/s00259-015-3047-0
Selkoe, D. J., and Hardy, J. (2016). The amyloid hypothesis of Alzheimer’s disease at 25 years. EMBO Mol. Med. 8, 595–608. doi: 10.15252/emmm.201606210
Serrano-Pozo, A., Mielke, M. L., Gómez-Isla, T., Betensky, R. A., Growdon, J. H., Frosch, M. P., et al. (2011). Reactive glia not only associates with plaques but also parallels tangles in Alzheimer’s disease. Am. J. Pathol. 179, 1371–1384. doi: 10.1016/j.ajpath.2011.05.047
Shigetomi, E., Bushong, E. A., Haustein, M. D., Tong, X., Jackson-Weaver, O., Kracun, S., et al. (2013). Imaging calcium microdomains within entire astrocyte territories and endfeet with GCaMPs expressed using adeno-associated viruses. J. Gen. Physiol. 141, 633–647. doi: 10.1085/jgp.201210949
Shigetomi, E., Hirayama, Y. J., Ikenaka, K., Tanaka, K. F., and Koizumi, S. (2018). Role of purinergic receptor P2Y1 in spatiotemporal Ca2+ dynamics in astrocytes. J. Neurosci. 38, 1383–1395. doi: 10.1523/JNEUROSCI.2625-17.2017
Shigetomi, E., Kracun, S., Sofroniew, M. V., and Khakh, B. S. (2010). A genetically targeted optical sensor to monitor calcium signals in astrocyte processes. Nat. Neurosci. 13, 759–766. doi: 10.1038/nn.2557
Shih, A. Y., Driscoll, J. D., Drew, P. J., Nishimura, N., Schaffer, C. B., and Kleinfeld, D. (2012a). Two-photon microscopy as a tool to study blood flow and neurovascular coupling in the rodent brain. J. Cereb. Blood Flow Metab. 32, 1277–1309. doi: 10.1038/jcbfm.2011.196
Shih, A. Y., Mateo, C., Drew, P. J., Tsai, P. S., and Kleinfeld, D. (2012b). A polished and reinforced thinned-skull window for long-term imaging of the mouse brain. J. Vis. Exp. 61:3742. doi: 10.3791/3742
Simpson, J. E., Ince, P. G., Lace, G., Forster, G., Shaw, P. J., Matthews, F., et al. (2010). Astrocyte phenotype in relation to Alzheimer-type pathology in the ageing brain. Neurobiol. Aging 31, 578–590. doi: 10.1016/j.neurobiolaging.2008.05.015
Somjen, G. G. (1988). Nervenkitt: notes on the history of the concept of neuroglia. Glia 1, 2–9. doi: 10.1002/glia.440010103
Stosiek, C., Garaschuk, O., Holthoff, K., and Konnerth, A. (2003). In vivo two-photon calcium imaging of neuronal networks. Proc. Natl. Acad. Sci. U S A 100, 7319–7324. doi: 10.1073/pnas.1232232100
Svoboda, K., and Yasuda, R. (2006). Principles of two-photon excitation microscopy and its applications to neuroscience. Neuron 50, 823–839. doi: 10.1016/j.neuron.2006.05.019
Takano, T., Tian, G. F., Peng, W., Lou, N., Libionka, W., Han, X., et al. (2006). Astrocyte-mediated control of cerebral blood flow. Nat. Neurosci. 9, 260–267. doi: 10.1038/nn1623
Tarantini, S., Tran, C. H. T., Gordon, G. R., Ungvari, Z., and Csiszar, A. (2017). Impaired neurovascular coupling in aging and Alzheimer’s disease: contribution of astrocyte dysfunction and endothelial impairment to cognitive decline. Exp. Gerontol. 94, 52–58. doi: 10.1016/j.exger.2016.11.004
Terry, R. D., Masliah, E., Salmon, D. P., Butters, N., DeTeresa, R., Hill, R., et al. (1991). Physical basis of cognitive alterations in Alzheimer’s disease: synapse loss is the major correlate of cognitive impairment. Ann. Neurol. 30, 572–580. doi: 10.1002/ana.410300410
Wilcock, D. M., Vitek, M. P., and Colton, C. A. (2009). Vascular amyloid alters astrocytic water and potassium channels in mouse models and humans with Alzheimer’s disease. Neuroscience 159, 1055–1069. doi: 10.1016/j.neuroscience.2009.01.023
Yang, J., Lunde, L. K., Nuntagij, P., Oguchi, T., Camassa, L. M., Nilsson, L. N., et al. (2011). Loss of astrocyte polarization in the Tg-ArcSwe mouse model of Alzheimer’s disease. J. Alzheimers Dis. 27, 711–722. doi: 10.3233/JAD-2011-110725
Zamanian, J. L., Xu, L., Foo, L. C., Nouri, N., Zhou, L., Giffard, R. G., et al. (2012). Genomic analysis of reactive astrogliosis. J. Neurosci. 32, 6391–6410. doi: 10.1523/JNEUROSCI.6221-11.2012
Zhang, Y., Cudmore, R. H., Lin, D. T., Linden, D. J., and Huganir, R. L. (2015). Visualization of NMDA receptor-dependent AMPA receptor synaptic plasticity in vivo. Nat. Neurosci. 18, 402–407. doi: 10.1038/nn.3936
Keywords: Alzheimer’s disease, astrocytes, two-photon microscopy, in vivo imaging, calcium
Citation: Kelly P, Hudry E, Hou SS and Bacskai BJ (2018) In Vivo Two Photon Imaging of Astrocytic Structure and Function in Alzheimer’s Disease. Front. Aging Neurosci. 10:219. doi: 10.3389/fnagi.2018.00219
Received: 17 April 2018; Accepted: 26 June 2018;
Published: 19 July 2018.
Edited by:
George E. Barreto, Pontifical Xavierian University, ColombiaReviewed by:
Antonia Gutiérrez, Universidad de Málaga, SpainAgneta Nordberg, Karolinska Institutet (KI), Sweden
Anna Maria Colangelo, Università degli Studi di Milano Bicocca, Italy
Copyright © 2018 Kelly, Hudry, Hou and Bacskai. This is an open-access article distributed under the terms of the Creative Commons Attribution License (CC BY). The use, distribution or reproduction in other forums is permitted, provided the original author(s) and the copyright owner(s) are credited and that the original publication in this journal is cited, in accordance with accepted academic practice. No use, distribution or reproduction is permitted which does not comply with these terms.
*Correspondence: Brian J. Bacskai, YmJhY3NrYWlAbWdoLmhhcnZhcmQuZWR1