- 1Key Laboratory of Flexible Electronics – Institute of Advanced Materials, Jiangsu National Synergistic Innovation Center for Advanced Materials, Nanjing Tech University, Nanjing, China
- 2Neurodegeneration Research Laboratory, National Neuroscience Institute, Singapore, Singapore
- 3Department of Physiology, Yong Loo Lin School of Medicine, National University of Singapore, Singapore, Singapore
Parkinson’s disease (PD) is the second most common neurodegenerative disease after Alzheimer’s disease, affecting about 7–10 million patients worldwide. The major pathological features of PD include loss of dopaminergic (DA) neurons in the substantia nigra pars compacta (SNpc) of the midbrain and the presence of α-synuclein-enriched Lewy bodies. Although the mechanism underlying PD pathogenesis remains to be elucidated, oxidative stress induced by the overproduction of reactive oxygen species (ROS) is widely accepted to be a key pathogenic factors. ROS cause oxidative damage to proteins, lipids, and DNA, which subsequently lead to neurodegeneration. Great efforts have been made to slow or stop the progress of PD. Unfortunately there is no effective cure for PD till now. Compounds with good antioxidant activity represent the promising candidates for therapeutics of PD. Some natural molecules from Chinese herbs are found to have good antioxidant activity. Both in vitro and in vivo studies demonstrate that these natural molecules could mitigate the oxidative stress and rescue the neuronal cell death in PD models. In present review, we summarized the reported natural molecules that displayed protective effects in PD. We also addressed the possible signal pathway through which natural molecules achieved their antioxidative effects and mitigate PD phenotypes. Hopefully it will pave the way to better recognize and utilize Chinese herbs for the treatment of PD.
Introduction
Parkinson’s disease (PD) is a devastating neurodegenerative disorder characterized by progressive loss of DA neurons in the SNpc of the midbrain, affecting 1–3% of the elderly population over 60 years (De Lau and Breteler, 2006). Currently, PD remains incurable and exerts heavy socio-economic burden to the society (Whetten-Goldstein et al., 1997; Lindgren et al., 2005; Winter et al., 2010). Although the precise molecular events underlying the pathogenesis of PD remain to be elucidated, the etiology of PD is found to involve environmental factors as well as genetic predisposition (Thomas and Beal, 2007). Regardless of exogenous or endogenous factors, oxidative stress is thought to play a pivotal role in the pathogenesis of PD (Jenner, 2003; Przedborski, 2017).
Oxidative stress is caused by imbalance of pro-oxidants and anti-oxidants in the cells (Barnham et al., 2004). Major reactive oxygen species (ROS) are produced in the process of adenosine triphosphate (ATP) synthesis which occurs in the mitochondria (Shadel and Horvath, 2015). DA neurons need relatively high amount of ATP to synthesize and release dopamine (Mamelak, 2018). Hence, more ROS are produced in DA neurons compared to other types of neurons. Additionally, mitochondria are believed to contribute to the generation of ROS as a result of the accumulation of mitochondrial DNA (mtDNA) mutations during the aging process (Gredilla et al., 2012). The accumulation of mtDNA mutations could decrease the capability of the electron transport chain (ETC), triggering decreased ATP production and increased ROS production (Cha et al., 2015). Rotenone, a selective inhibitor of complex I of mitochondrial respiratory chain, was been proved to cause mitochondrial dysfunction as well as ROS accumulation. Moreover, reduced level or activity of antioxidants such as superoxide dismutase (SOD), catalase (CAT), glutathione (GSH), and glutathione peroxidase (Gpx) is another contributor to the build-up of oxidative stress (Koppula et al., 2012). Mutation of mitochondria homeostasis-related genes, including parkin, DJ-1, PTEN-induced putative kinase 1 (PINK1), peroxisome proliferator-activated receptor gamma coactivator-1α (PGC-1α), and leucine-rich repeat kinase 2 (LRRK2) has also been reported to lead to familial PD (Finck and Kelly, 2006; Handschin and Spiegelman, 2006; Aquilano et al., 2008; Schapira, 2008). Taken together, compelling evidence implicates the involvement of ROS-related stress in the pathogenesis of PD.
Currently there is no effective treatment for PD. Although L-DOPA, as the substitute of dopamine, has been widely used in clinic, the poisonous and side effect over time limits its application. Chinese herbs, which have been used for thousands of years to treat various diseases in China, represent an alternative strategy given their higher efficacy and relatively modest side effects. In PD treatment, the reported effects of Chinese herbs including antioxidant, anti-inflammatory, free radicals-scavenging, anti-apoptosis, and chelating harmful metals (Fu et al., 2015). In this review, we will discuss the role of oxidative stress in PD pathogenesis, summarize the anti-ROS effects of natural molecules from Chinese herbs and its possible mechanisms, with the view to position Chinese herbs as an alternative or complementary approach in treating PD patients.
Oxidative Stress and PD
Oxygen is the prerequisite for nearly all forms of living organisms, but it is the source of oxidative stress also (Uttara et al., 2009). Oxidative stress results from excessive ROS, which is the consequence of imbalance between pro-oxidant and anti-oxidant homeostasis. ROS mainly comprise hydrogen peroxide (H2O2), superoxide anions (O2−) and the highly reactive hydroxyl radicals (OH•). Normally, the generation and elimination of ROS is well coordinated to maintain the redox status. Once the balance broken, oxidative stress will be induced, and subsequently diseases such as PD might occur. The concept of ROS involving the PD pathogenesis has been supported by multiple evidences (Figure 1).
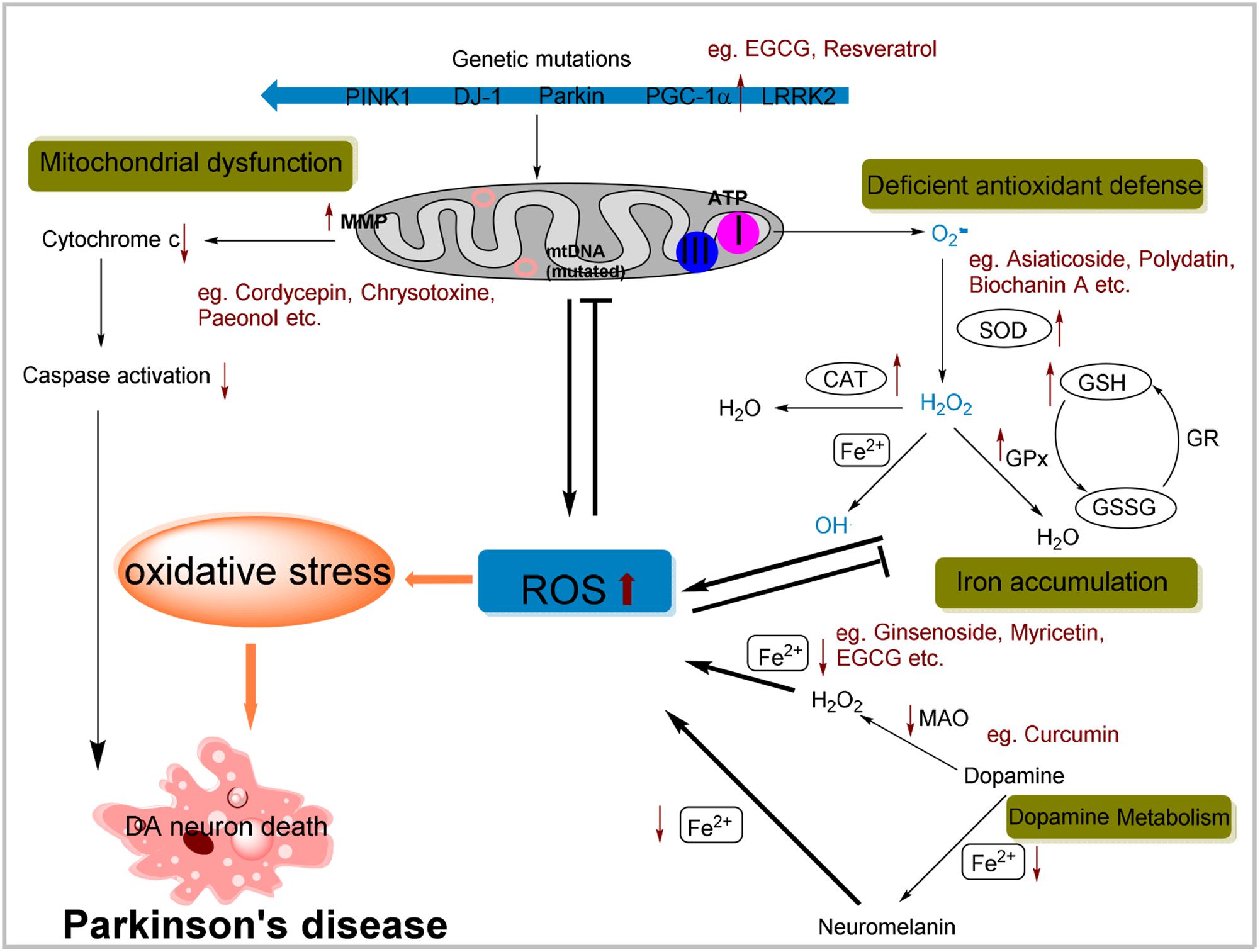
FIGURE 1. Possible cellular mechanisms attributed to oxidative stress in Parkinson’s disease (PD) and possible effects of natural antioxidants on specific pathway. Mitochondrial dysfunction by complex I inhibition and mtDNA mutation resulting in the ROS overproduction or mutation of genes involved in mitochondrial homeostasis can lead to an increased oxidative stress. ROS in turn results in the collapse of MMP and then initiates apoptosis signaling pathways contributing to DA neurons death. Also, the deficiency of antioxidant defense system may contribute to increased level of ROS. Additionally, dopamine metabolism can generate some active molecules contributing to ROS generation. Iron aggregation also enhances oxidative stress through the Fenton and Haber-Weiss reactions. In summary, all these cellular mechanisms due to the oxidative stress are implicated in the selective degeneration of dopaminergic neurons. Moreover, anti-oxidative effects of a portion of natural compounds mentioned above have been listed within specific pathway.
Aging, iron accumulations, mitochondrial dysfunction, dopamine metabolism, which are all the established PD causative factors, meanwhile render oxidative stress. For example, incidence of PD is increased with aging, especially in elders. This is in accordance with that aged neuronal cells show hypersensitive toward oxidative stress (Floyd and Carney, 1992). The surveillance of antioxidants was also found compromised with aging (Lepoivre et al., 1994; Schulz et al., 2000). Active iron metals involved in generation of ROS through Fenton reaction and iron accumulation was attributed to PD pathogenesis (Takahashi et al., 2001; Maynard et al., 2002). Metabolism of dopamine produced many reactive molecules hence DA neurons were particularly susceptible to oxidative stress (Segura-Aguilar et al., 2014). Dopamine was synthesized in the cytoplasm and immediately sequestered into monoaminergic vesicles (Herrera et al., 2017). If not secreted, dopamine could be auto-oxidized or deaminated by monoamine oxidase (MAO), during which several cytotoxic molecules, including dopamine–quinone species (SQ•), OH•, and H2O2 are generated (Graham, 1978; Maker et al., 1981). Notably, 1-methyl-4-phenyl-1,2,3,6-tetrahydropyridine (MPTP) is commonly used to induce oxidative stress-related Parkinsonism in animal models. The active metabolite of MPTP, MPP+, was selectively taken up into dopaminergic terminals by the plasma-membrane dopamine transporter (DAT) (Choi et al., 2015). MPP+ would block complex I activity of the mitochondrial respiratory chain and result in the oxidative stress (Del Zompo et al., 1992; Peter et al., 1994; Lotharius and O’Malley, 2000).
Mitochondrial dysfunction, resulting from various malgenic factors, has been implicated in ROS generation and oxidative stress in PD. mtDNA mutations contributed to mitochondrial dysfunction due to impaired capability of the ETC, triggering ROS production (Gredilla et al., 2012; Yan et al., 2013; Cha et al., 2015). ROS in turn resulted in the collapse of the mitochondrial membrane potential (MMP) and disruption of the mitochondrial respiratory chain complex I, which ultimately leaded to increased cytosolic concentrations of Ca2+ and mitochondrial cytochrome c that initiated apoptosis signaling pathways (Du et al., 2010). Mutation of genes involved in mitochondrial homeostasis was known to induce familial PD (Schapira, 2007). Parkin, an E3 ubiquitin ligase, was mitochondria key regulator of mitophagy (Narendra et al., 2008). Not surprisingly, Drosophila deficient in parkin, exhibited mitochondrial dysfunction and higher vulnerability to oxidative stress (Whitworth et al., 2005; Ng et al., 2012). Parkin knockout mice showed decreased amounts of several proteins involved in mitochondrial function and enhanced oxidative stress (Palacino et al., 2004). PINK1, together with Parkin, were tightly coordinated to the controlling of mitochondrial dynamics (Scarffe et al., 2014). PINK1 accumulates on the outer membrane of damaged mitochondria and recruits Parkin to the dysfunctional mitochondrion (Pickrell and Youle, 2015). It was showed that the lack of PINK1 resulted in the mitochondrial defects and loss of SNpc DA neurons, and these phenomena could be ameliorated by the enhanced expression of Parkin (Yang et al., 2006; Gautier et al., 2008). Many lines of evidence suggested that DJ-1, another gene reported to cause a familial early onset PD, functions as an antioxidant protein. DJ-1 bonded to subunits of mitochondrial complex I and regulates its activity (Hayashi et al., 2009). DJ-1 mutation promoted its accumulation in mitochondria and was implicated as a cellular monitor of oxidative stress (Mitsumoto and Nakagawa, 2001; Bonifati et al., 2002). DJ-1 knockout mice displayed compromised mitochondrial function and then nigrostriatal DA neuron loss (Goldberg et al., 2005; Giaime et al., 2012). Furthermore, dysfunction of PGC-1α, the key transcriptional modulator of mitochondrial biogenesis and oxidative metabolism, was also implicated in PD (Arany et al., 2005; Lin et al., 2005). PGC-1α regulated the mitochondrial function and provides homeostatic control of cellular ATP (Rohas et al., 2007). It was revealed that inhibited expression of PGC-1α resulting from methylation of its gene contributed to the mitochondrial defects in substantia nigra of PD patients (Su et al., 2015). Dominant mutations in LRRK2 are currently recognized to be the most prevalent cause of late-onset familial PD (Kumari and Tan, 2009). Actually LRRK2 patient-derived cells exhibited altered mitochondrial dynamics that was accompanied by reduction in MMP and intracellular ATP levels (Mortiboys et al., 2010). Moreover, Drosophila with LRRK2 G2019S mutant induced marked mitochondrial pathological alternation both in flight muscles and DA neurons (Ng et al., 2012).
Natural Anti-Oxidant Molecules and their Applications in PD Models
Vast territory of China has brought about abundant natural resources. One precious natural resource is Chinese herb, which has been used throughout history to improve quality of human life. In recent years, traditional Chinese herbs attract more interests due to its impressive curative effect for a variety of diseases, coupled with lower toxicity and side effects (Fu et al., 2015). As mentioned above, oxidative stress damage is one of the most important characteristics of PD. Natural antioxidants, which are enriched in Chinese herbs, provide neuroprotective effects in PD through a variety of biological pathways (Obrenovich et al., 2010; Soobrattee et al., 2010). In this review, polyphenols, flavone (baicalein), flavonols (quercetin, kaempferol, morin, and myricetin), dihydroflavones (hesperetin and naringenin), isoflavone (biochanin A), and flavane (epigallocatechin gallate), and other non-flavonoids phenolic compounds (resveratrol, curcumin, and paeonol) from Chinese herbs against PD will be discussed. Moreover, the well-known glycoside derivatives [mangiferin, salidroside, asiaticoside, polydatin, gypenoside (GP), and ginsenoside], and other compounds (nerolidol, chrysotoxine, DL-3-n-butylphthalide, cordycepin, and ursolic acid) from natural resources with good antioxidant properties will also be addressed to discuss their possible mechanisms of against PD (Table 1).
Natural Molecules Modulating Mitochondrial Function
Mitochondrial dysfunction-induced oxidative stress is widely accepted to be the key driver of PD. Epigallocatechin gallate (EGCG) is an important component of green tea and it has lots of biological effects, such as antioxidation, scavenging of free radicals and anti-apoptosis. In MPP+-treated PC12 cells, EGCG caused up-regulation of PGC-1α, resulting in improved mitochondrial function and DA neuronal survival (Ye et al., 2012). In addition, EGCG was reported to act as a suppressor of mitochondrial dysfunction in both mutant LRRK2 and parkin-null flies through activation of the AMP-activated protein kinase (AMPK) signaling pathway (Ng et al., 2012). Resveratrol, a polyphenolic compound enriched in grapes, was shown to improve mitochondrial activity via affecting energy metabolic sensors through mediation of autophagy signals and activation of NAD-dependent deacetylase sirtuin-1 (SIRT1) and PGC-1α (Lagouge et al., 2006; Jeong et al., 2012). Resveratrol could also enhanced the mRNA level of a number of PGC-1α target genes such as mitochondrial transcription factor A (TFAM), cyclooxygenase-1 (COX I), resulting in mitochondrial biogenesis (Ferretta et al., 2014). Cordycepin, a nucleoside isolated from Cordyceps species displayed antioxidant property. Pretreatment of cordycepin helped to maintain MMP and reduced activation of caspase-3 in 6-OHDA induced PD model (Olatunji et al., 2016). The flavone baicalein, isolated from Scutellaria baicalensis, was reported to protect against 6-OHDA-induced neurotoxicity both in vivo and in vitro via confronting mitochondrial dysfunction, oxidative injury, JNK activation and caspase activation (Lee et al., 2005). Curcumin, the natural polyphenol compound derived from the curry spice turmeric, displayed neuroprotective effects on the MPTP induced PD cellular model through mediating Bcl-2-mitochondria-ROS-iNOS pathway (Chen et al., 2006). Chrysotoxine, a bioactive bibenzyl compounds isolated from medicinal Dendrobium species, was reported to be free radical scavengers (Zhang et al., 2007). Pretreatment with Chrysotoxine protected against 6-OHDA-induced intracellular generation of ROS and mitochondrial dysfunctions, including the decrease of MMP, increase of intracellular free Ca2+, release of cytochrome c, and imbalance of Bax/Bcl-2 ratio (Song et al., 2010). DL-3-n-butylphthalide (NBP), derived from l-3-n-butylphthalidec extracted from the seeds of Apium graveolens Linn (Chinese celery), was found to be a natural free radical scavenger (Li et al., 2009). In a cellular PD model, pretreatment with NBP mitigated the toxicity of MPP+ by retaining the mitochondrial function, and suppressing ROS generation (Huang et al., 2010). Two polyphenols, mangiferin and morin, specifically enriched in fruit, vegetables, plant extracts, wine, and tea, were reported to reduce the formation of ROS by restoring the MMP in excitotoxic induced cell model (Campos-Esparza et al., 2009; Kavitha et al., 2014). Paeonol, a major phenolic compound of the Chinese herb, Cortex Moutan, is known for its antioxidant, anti-inflammatory and antitumor properties. Paeonol has been shown to attenuate the intracellular ROS accumulation and associated mitochondrial cell death pathway including MMP disruption, cytochrome c release and caspase-3 activation in MPP+-induced cellular PD model (Lu et al., 2015). From high throughput screening, the natural compound ursolic acid, a pentacyclic triterpenoid, was found to rescue mitochondrial function in parkin-mutant fibroblasts via the activation of the glucocorticoid receptor that is associated with increased phosphorylation of Akt (Mortiboys et al., 2013). Salidroside (Sal), a phenylpropanoid glycoside isolated from Rhodiola rosea L., had potent antioxidant properties. Sal pretreatment protected DA neurons against MPP+-induced toxicity by reducing the production of ROS or NO, regulating the ratio of Bcl-2/Bax, decreasing cytochrome c and Smac release, and inhibiting caspase activation (Wang J. et al., 2015).
Natural Molecules Regulating Endogenous Antioxidants and Dopamine Metabolism
Cells have endogenous defense mechanisms against oxidative stress, including enzymatic and non-enzymatic systems (Gilgun-Sherki et al., 2001). The capacity of antioxidant defenses declined with aging and in pathological state (Sohal and Weindruch, 1996; Lotharius et al., 2002). It was reported that polyphenols could modulate the activity of enzymes involved in oxidative stress (Ebrahimi and Schluesener, 2012). Xu et al. (2012) showed that asiaticoside, a triterpenoid saponin isolated from Centella asiatica attenuated the MPTP-induced the reduction of GSH in a rat model of Parkinsonism. Polydatin, a glycosylated derivative of resveratrol, significantly prevented the rotenone-induced decreased levels of GSH and the manganese superoxide dismutases (MnSOD) in the striatum of rodent models of PD (Chen Y. et al., 2015). Biochanin A, an O-methylated isoflavone found in chickpea, increased SOD and Gpx activities in lipopolysaccharide (LPS) induced rat PD model (Wang J. et al., 2015). Another research group reported that pretreatment with biochanin A could lead to the increase in the total GSH level in the L-glutamate-treated PC12 cells (Tan et al., 2012). Treatment with GPs, saponins extracted from Gynostemma pentaphyllum, attenuated MPTP-induced decrease of GSH and reduced SOD activity in the SNpc of the mice (Wang et al., 2010). Pretreatment with mangiferin protected N2A cells against MPP+-induced cytotoxicity, restored the GSH, and down-regulated both SOD1 and CAT mRNA expression (Amazzal et al., 2007). Nerolidol, a sesquiterpene alcohol, significantly increased the level of SOD, CAT, and GSH in a rotenone-induced PD experimental model (Javed et al., 2016). Quercetin, enriched in abundance in fruits and vegetables, onions, red wine and olive oil, restored level of GSH in the striatum of rats induced by 6-OHDA (Miean and Mohamed, 2001; Haleagrahara et al., 2011). In H2O2-induced PC12 cells, pretreatment with quercetin markedly reduced the antioxidant enzyme SOD and Gpx level (Chen L. et al., 2015). Kaempferol, a prototype flavonol presented in tea, broccoli, grapefruit, brussel sprouts and apple, was reported to have strong antioxidant and anti-inflammatory properties, and enhanced SOD and Gpx activity in the mouse model of PD (Żuk et al., 2011; Li and Pu, 2011). Cordycepin, resveratrol, hesperetin rendered up-regulation of the level and activity of antioxidants such as, SOD, GPx, CAT, GSH in 6-OHDA induced PD models (Khan et al., 2010; Kiasalari et al., 2016; Olatunji et al., 2016). Treatment with paeonol improved the MPTP-induced the oxidative stress, as determined by enhancing the activity levels of SOD, CAT, and GSH in the mouse PD model (Shi et al., 2016). Gastrodin, the major active component in the Gastrodia elata, has been demonstrated to have many pharmacological effects, such as antioxidative and neuroprotective properties. In MPP+-induced oxidative cellular PD model, pretreatment with gastrodin increased antioxidant enzyme heme oxygenase-1 (HO-1) expression (Jiang et al., 2014) and activation of HO-1 resulted in increased levels of antioxidant substrates such as biliverdin, bilirubin, and ferritin (Doré et al., 1999). Curcumin and its metabolite tetrahydrocurcumin (ThC) exerted neuroprotection against MPTP induced neurotoxicity via inhibiting MAO-B activity (Rajeswari and Sabesan, 2008).
Natural Molecules Chelating Metal Iron
Iron, which accumulated in the aging brain especially the SNpc, is thought to promote PD pathogenesis (Devos et al., 2014). Iron enhances ROS generation through the Fenton and Haber-Weiss reactions (Barnham et al., 2004). Curcumin pretreatment reversed iron-induced degeneration of nigral DA neurons by its iron chelating activity (Du et al., 2012). Ginsenoside, the active component isolated from ginseng, was reported to decrease the 6-OHDA-induced iron influx by inhibiting up-regulation of an iron importer protein divalent metal transporter 1 with iron responsive element (DMT1+IRE), which was rendered via its antioxidant effect (Xu et al., 2010). Myricetin, a natural flavonoid found in fruits, vegetables, and herbs (Kang et al., 2010), was reported to suppress iron induced toxicity in the 6-OHDA induced PD model (Ma et al., 2007). EGCG served as iron chelator inhibiting the formation of transition metal catalyzed free radicals and displaying its antioxidant and neuroprotective effects (Weinreb et al., 2009; Singh et al., 2016). EGCG could also alleviate the iron accumulation in PD through affecting the iron responsive element (Reznichenko et al., 2006). Administration of naringenin (NGEN), a natural flavonoid compound, attenuated oxidative damages in the cerebral cortex of iron treatment induced PD model, due to its iron chelating activity (Chtourou et al., 2014).
Conclusion and Perspectives
In recent years, given the limitations of current PD treatment, more attention has been given to the potential therapeutic effects of Chinese herbs (Song et al., 2012). In this review, we summarized natural antioxidants from Chinese herbs that were reported to protect against toxins-induced PD in preclinical animal models. These natural antioxidants achieve their protective effects mainly through regulating cellular oxidative homeostasis either directly or indirectly. Of them, resveratrol and EGCG, showed prominent antioxidative effect in PD models and could be a promising candidate for treating PD. Notwithstanding that, there are several challenges to overcome before natural molecules from Chinese Herbs could serve as alternative medicine for PD. How to efficiently screen and select the candidates that can be used for treatment of PD from the massive number of Chinese herbs available? What are the intracellular targets of the natural molecules? How to help the molecules pass through the blood–brain barrier (BBB) in order to directly protect the DA neurons in the SNpc? Clearly, it is promising but a lot of work needs to be done to ultimately achieve the goals of making natural molecules to be accepted and applied in PD patient treatment.
Author Contributions
YD prepared the manuscript. CX, HZ, ZF, and WH provided help in the manuscript preparation. CW-Z, LL, and K-LL did the revision.
Funding
This work was financially supported by the National Natural Science Foundation of China (81672508 and 61505076), Jiangsu Provincial Foundation for Distinguished Young Scholars (BK20170041), Key University Science Research Project of Jiangsu Province (Grant 16KJA180004), and China-Sweden Joint Mobility Project (51661145021).
Conflict of Interest Statement
The authors declare that the research was conducted in the absence of any commercial or financial relationships that could be construed as a potential conflict of interest.
References
Amazzal, L., Lapôtre, A., Quignon, F., and Bagrel, D. (2007). Mangiferin protects against 1-methyl-4-phenylpyridinium toxicity mediated by oxidative stress in N2A cells. Neurosci. Lett. 418, 159–164. doi: 10.1016/j.neulet.2007.03.025
Aquilano, K., Baldelli, S., Rotilio, G., and Ciriolo, M. R. (2008). Role of nitric oxide synthases in Parkinson’s disease: a review on the antioxidant and anti-inflammatory activity of polyphenols. Neurochem. Res. 33, 2416–2426. doi: 10.1007/s11064-008-9697-6
Arany, Z., He, H., Lin, J., Hoyer, K., Handschin, C., Toka, O., et al. (2005). Transcriptional coactivator PGC-1 alpha controls the energy state and contractile function of cardiac muscle. Cell Metab. 1, 259–271. doi: 10.1016/j.cmet.2005.03.002
Barnham, K. J., Masters, C. L., and Bush, A. I. (2004). Neurodegenerative diseases and oxidative stress. Nat. Rev. Drug Discov. 3, 205–214. doi: 10.1038/nrd1330
Bonifati, V., Rizzu, P., van, Baren MJ, Schaap, O., Breedveld, G. J., Krieger, E., et al. (2002). Mutations in the DJ-1 gene associated with autosomal recessive early-onset parkinsonism. Science 299, 256–259. doi: 10.1126/science.1077209
Campos-Esparza, M. R., Sánchez-Gómez, M. V., and Matute, C. (2009). Molecular mechanisms of neuroprotection by two natural antioxidant polyphenols. Cell Calcium 45, 358–368. doi: 10.1016/j.ceca.2008.12.007
Cha, M. Y., Kim, D. K., and Mook-Jung, I. (2015). The role of mitochondrial DNA mutation on neurodegenerative diseases. Exp. Mol. Med. 47:e150. doi: 10.1038/emm.2014.122
Chen, J., Tang, X. Q., Zhi, J. L., Cui, Y., Yu, H. M., Tang, E. H., et al. (2006). Curcumin protects PC12 cells against 1-methyl-4-phenylpyridinium ion-induced apoptosis by bcl-2-mitochondria-ROS-iNOS pathway. Apoptosis 11, 943–953. doi: 10.1007/s10495-006-6715-5
Chen, L., Sun, L., Liu, Z., Wang, H., and Xu, C. (2015). Protection afforded by quercetin against H2O2-induced apoptosis on PC12 cells via activating PI3K/Akt signal pathway. J. Recept. Signal Transduct. Res. 36, 98–102. doi: 10.3109/10799893.2015.1049363
Chen, Y., Zhang, D. Q., Liao, Z., Wang, B., Gong, S., Wang, C., et al. (2015). Anti-oxidant polydatin (piceid) protects against substantia nigral motor degeneration in multiple rodent models of Parkinson’s disease. Mol. Neurodegener. 10:4. doi: 10.1186/1750-1326-10-4
Choi, S. J., Panhelainen, A., Schmitz, Y., Larsen, K. E., Kanter, E., Wu, M., et al. (2015). Changes in neuronal dopamine homeostasis following 1-methyl-4-phenylpyridinium (MPP+) exposure. J. Biol. Chem. 290, 6799–6809. doi: 10.1074/jbc.M114.631556
Chtourou, Y., Fetoui, H., and Gdoura, R. (2014). Protective effects of naringenin on iron-overload-induced cerebral cortex neurotoxicity correlated with oxidative Stress. Biol. Trace Elem. Res. 158, 376–383. doi: 10.1007/s12011-014-9948-0
De Lau, L. M., and Breteler, M. M. (2006). Epidemiology of Parkinson’s disease. Lancet Neurol. 5, 525–535. doi: 10.1016/S1474-4422(06)70471-9
Del Zompo, M., Piccardi, M. P., Ruiu, S., Corsini, G. U., and Vaccari, A. (1992). Characterization of a putatively vesicular binding site for [3H]MPP+ in mouse striatal membranes. Brain Res. 571, 354–357. doi: 10.1016/0006-8993(92)90677-2
Devos, D., Moreau, C., Devedjian, J. C., Kluza, J., Petrault, M., Laloux, C., et al. (2014). Targeting chelatable iron as a therapeutic modality in Parkinson’s disease. Antioxid. Redox Signal. 2, 195–210. doi: 10.1089/ars.2013.5593
Doré, S., Takahashi, M., Ferris, C. D., Zakhary, R., Hester, L. D., Guastella, D., et al. (1999). Bilirubin, formed by activation of heme oxygenase-2, protects neurons against oxidative stress injury. Proc. Natl. Acad. Sci. U.S.A. 96, 2445–2450. doi: 10.1073/pnas.96.5.2445
Du, T., Li, L., Song, N., Xie, J., and Jiang, H. (2010). Rosmarinic acid antagonized 1-Methyl-4-phenylpyridinium(MPP+)-induced neurotoxicity in MES23.5 dopaminergic cells. Int. J. Toxicol. 29, 625–633. doi: 10.1177/1091581810383705
Du, X. X., Xu, H. M., Jiang, H., Song, N., Wang, J., and Xie, J. X. (2012). Curcumin protects nigral dopaminergic neurons by iron-chelation in the 6-hydroxydopamine rat model of Parkinson’s disease. Neurosci. Bull. 28, 253–258. doi: 10.1007/s12264-012-1238-2
Ebrahimi, A., and Schluesener, H. (2012). Natural polyphenols against neurodegenerative disorders: potentials and pitfalls. Ageing Res. Rev. 11, 329–345. doi: 10.1016/j.arr.2012.01.006
Ferretta, A., Gaballo, A., Tanzarella, P., Piccoli, C., Capitanio, N., Nico, B., et al. (2014). Effect of resveratrol on mitochondrial function: implications inparkin-associated familiar Parkinson’s disease. Biochim. Biophys. Acta 1842, 902–915. doi: 10.1016/j.bbadis.2014.02.010
Finck, B. N., and Kelly, D. P. (2006). PGC-1 coactivators: inducible regulators of energy metabolism in health and disease. J. Clin. Invest. 116, 615–622. doi: 10.1172/JCI27794
Floyd, R. A., and Carney, J. M. (1992). Free radical damage to protein and DNA: mechanisms involved and relevant observations on brain undergoing oxidative stress. Ann. Neurol. 32, 22–27. doi: 10.1002/ana.410320706
Fu, W., Zhuang, W., Zhou, S., and Wang, X. (2015). Plant-derived neuroprotective agents in Parkinson’s disease. Am. J. Transl. Res. 7, 1189–1202.
Gautier, C. A., Kitada, T., and Shen, J. (2008). Loss of PINK1 causes mitochondrial functional defects and increased sensitivity to oxidative stress. Proc. Natl. Acad. Sci. U.S.A. 105, 11364–11369. doi: 10.1073/pnas.0802076105
Giaime, E., Yamaguchi, H., Gautier, C. A., Kitada, T., and Shen, J. (2012). Loss of DJ-1 does not affect mitochondrial respiration but increases ROS production and mitochondrial permeability transition pore opening. PLoS One 7:e40501. doi: 10.1371/journal.pone.0040501
Gilgun-Sherki, Y., Melamed, E., and Offen, D. (2001). Oxidative stress induced-neurodegenerative diseases: the need for antioxidants that penetrate the blood brain barrier. Neuropharmacology 40, 959–975. doi: 10.1016/S0028-3908(01)00019-3
Goldberg, M. S., Pisani, A., Haburcak, M., Vortherms, T. A., Kitada, T., Costa, C., et al. (2005). Nigrostriatal dopaminergic deficits and hypokinesia caused by inactivation of the familial Parkinsonism-linked gene DJ-1. Neuron 45,489–496. doi: 10.1016/j.neuron.2005.01.041
Graham, D. G. (1978). Oxidative pathways for catecholamines in the genesis of neuromelanin and cytotoxic quinones. Mol. Pharmacol. 14, 633–643.
Gredilla, R., Weissman, L., Yang, J. L., Bohr, V. A., and Stevnsner, T. (2012). Mitochondrial base excision repair in mouse synaptosomes during normal aging and in a model of Alzheimer’s disease. Neurobiol. Aging 33, 694–707. doi: 10.1016/j.neurobiolaging.2010.06.019
Haleagrahara, N., Siew, C. J., Mitra, N. K., and Kumari, M. (2011). Neuroprotective effect of bioflavonoid quercetin in 6-hydroxydopamine-induced oxidative stress biomarkers in the rat striatum. Neurosci. Lett. 500, 139–143. doi: 10.1016/j.neulet.2011.06.021
Handschin, C., and Spiegelman, B. M. (2006). Peroxisome proliferator-activated receptor γ coactivator 1 coactivators, energy homeostasis, and metabolism. Endocr. Rev. 27, 728–735. doi: 10.1210/er.2006-0037
Hayashi, T., Ishimori, C., Takahashi-Niki, K., Taira, T., Kim, Y., Maita, H., et al. (2009). DJ-1 binds to mitochondrial complex I and maintains its activity. Biochem. Biophys. Res. Commun. 390, 667–672. doi: 10.1016/j.bbrc.2009.10.025
Herrera, A., Muñoz, P., Steinbusch, H. W. M., and Segura-Aguilar, J. (2017). Are dopamine oxidation metabolites involved in the loss of dopaminergic neurons in the nigrostriatal system in Parkinson’s disease? ACS Chem. Neurosci. 8, 702–711. doi: 10.1021/acschemneuro.7b00034
Huang, J. Z., Chen, Y. Z., Su, M., Zheng, H. F., Yang, Y. P., Chen, J., et al. (2010). DL-3-n-butylphthalide prevents oxidative damage and reduces mitochondrial dysfunction in an MPP+-induced cellular model of Parkinson’s disease. Neurosci. Lett. 475, 89–94. doi: 10.1016/j.neulet.2010.03.053
Javed, H., Azimullah, S., AbulKhair, S. B., Ojha, S., and Haque, M. E. (2016). Neuroprotective effect of nerolidol against neuroinflammation and oxidative stress induced by rotenone. BMC Neurosci. 17:58. doi: 10.1186/s12868-016-0293-4
Jenner, P. (2003). Oxidative stress in Parkinson’s disease. Ann. Neurol. 53, 26–38. doi: 10.1002/ana.10483
Jeong, J. K., Moon, M. H., Bae, B. C., Lee, Y. J., Seol, J. W., Kang, H. S., et al. (2012). Autophagy induced by resveratrol prevents human prion protein-mediated neurotoxicity. Neurosci. Res. 73, 99–105. doi: 10.1016/j.neures.2012.03.005
Jiang, G., Hu, Y., Liu, L., Cai, J., Peng, C., and Li, Q. (2014). Gastrodin protects against MPP+ -induced oxidative stress by up regulates heme oxygenase-1 expression through p38 MAPK/Nrf2 pathway in human dopaminergic cells. Neurochem. Int. 75, 79–88. doi: 10.1016/j.neuint.2014.06.003
Kang, K. A., Wang, Z. H., Zhang, R., Piao, M. J., Kim, K. C., Kang, S., et al. (2010). Myricetin protects cells against oxidative stress-induced apoptosis via regulation of PI3K/Akt and MAPK signaling pathways. Int. J. Mol. Sci. 11, 4348–4360. doi: 10.3390/ijms11114348
Kavitha, M., Manivasagam, T., Essa, M. M., Tamilselvam, K., Selvakumar, G. P., Karthikeyan, S., et al. (2014). Mangiferin antagonizes rotenone: induced apoptosis through attenuating mitochondrial dysfunction and oxidative stress in SK-N-SH neuroblastoma cells. Neurochem. Res. 39, 668–676. doi: 10.1007/s11064-014-1249-7
Khan, M. M., Ahmad, A., Ishrat, T., Khan, M. B., Hoda, M. N., Khuwaja, G., et al. (2010). Resveratrol attenuates 6-hydroxydopamine-induced oxidative damage and dopamine depletion in rat model of Parkinson’s disease. Brain Res. 1328, 139–151. doi: 10.1016/j.brainres.2010.02.031
Kiasalari, Z., Khalili, M., Baluchnejadmojarad, T., and Roghani, M. (2016). Protective effect of oral hesperetin against unilateral striatal 6-hydroxydopamine damage in the rat. Neurochem. Res. 41, 1065–1072. doi: 10.1007/s11064-015-1796-6
Koppula, S., Kumar, H., More, S. V., Lim, H. W., Hong, S. M., and Choi, D. K. (2012). Recent updates in redox regulation and free radical scavenging effects by herbal products in experimental models of Parkinson’s disease. Molecules 17, 11391–11420. doi: 10.3390/molecules171011391
Kumari, U., and Tan, E. K. (2009). LRRK2 in Parkinson’s disease: genetic and clinical studies from patients. FEBS J. 276, 6455–6463. doi: 10.1111/j.1742-4658.2009.07344.x
Lagouge, M., Argmann, C., Gerhart-Hines, Z., Meziane, H., Lerin, C., Daussin, F., et al. (2006). Resveratrol improves mitochondrial function and protects against metabolic disease by activating SIRT1 and PGC-1α. Cell 127, 1109–1122. doi: 10.1016/j.cell.2006.11.013
Lee, H. J., Noh, Y. H., Lee, D. Y., Kim, Y. S., Kim, K. Y., Chung, Y., et al. (2005). Baicalein attenuates 6-hydroxydopamine-induced neurotoxicity in SH-SY5Y cells. Eur. J. Cell. Biol. 84, 897–905. doi: 10.1016/j.ejcb.2005.07.003
Lepoivre, M., Flaman, J. M., Bobé, P., Lemaire, G., and Henry, Y. (1994). Quenching of the tyrosyl free radical of ribonucleotide reductase by nitric oxide. Relationship to cytostasis induced in tumor cells by cytotoxic macrophages. J. Biol. Chem. 269, 21891–21897.
Li, L., Zhang, B., Tao, Y., Wang, Y., Wei, H., Zhao, J., et al. (2009). DL-3-n-butylphthalide protects endothelial cells against oxidative/nitrosative stress, mitochondrial damage and subsequent cell death after oxygen glucose deprivation in vitro. Brain Res. 1290, 91–101. doi: 10.1016/j.brainres.2009.07.020
Li, S., and Pu, X. P. (2011). Neuroprotective effect of kaempferol against a 1-methyl-4-phenyl-1,2,3,6- tetrahydropyridine-induced mouse model of Parkinson’s disease. Biol. Pharm. Bull. 34, 1291–1296. doi: 10.1248/bpb.34.1291
Lin, J., Handschin, C., and Spiegelman, B. M. (2005). Metabolic control through the PGC-1 family of transcription coactivators. Cell Metab. 1, 361–370. doi: 10.1016/j.cmet.2005.05.004
Lindgren, P., Von Campenhausen, S., Spottke, E., Siebert, U., and Dodel, R. (2005). Cost of Parkinson’s disease in Europe. Eur. J. Neurol. 12, 68–73. doi: 10.1111/j.1468-1331.2005.01197.x
Lotharius, J., Barg, S., Wiekop, P., Lundberg, C., Raymon, H. K., and Brundin, P. (2002). Effect of mutant α-synuclein on dopamine homeostasis in a new human mesencephalic cell line. J. Biol. Chem. 277, 38884–38894. doi: 10.1074/jbc.M205518200
Lotharius, J., and O’Malley, K. L. (2000). The Parkinsonism-inducing drug 1-Methyl-4-phenylpyridinium triggers intracellular dopamine oxidation. J. Biol. Chem. 275, 38581–38588. doi: 10.1074/jbc.M005385200
Lu, X. L., Lin, Y. H., Wu, Q., Su, F. J., Ye, C. H., Shi, L., et al. (2015). Paeonolum protects against MPP+-induced neurotoxicity in zebrafish and PC12 cells. BMC Complement. Altern. Med. 15:137. doi: 10.1186/s12906-015-0661-0
Ma, Z. G., Wang, J., Jiang, H., Liu, T. W., and Xie, J. X. (2007). Myricetin reduces 6-hydroxydopamine-induced dopamine neuron degeneration in rats. Neuroreport 18, 1181–1185. doi: 10.1097/WNR.0b013e32821c51fe
Maker, H. S., Weiss, C., Silides, D. J., and Cohen, G. (1981). Coupling of dopamine oxidation (monoamine oxidase activity) to glutathione oxidation via the generation of hydrogen peroxide in rat brain homogenates. J. Neurochem. 36, 589–593. doi: 10.1111/j.1471-4159.1981.tb01631.x
Mamelak, M. (2018). Parkinson’s disease, the dopaminergic neuron and gammahydroxybutyrate. Neurol. Ther. 7, 5–11. doi: 10.1007/s40120-018-0091-2
Maynard, C. J., Cappai, R., Volitakis, I., Cherny, R. A., White, A. R., Beyreuther, K., et al. (2002). Overexpression of Alzheimer’s disease amyloid- β opposes the age-dependent elevations of brain copper and iron. J. Biol. Chem. 277, 44670–44676. doi: 10.1074/jbc.M204379200
Miean, K. H., and Mohamed, S. (2001). Flavonoid (myricetin, quercetin, kaempferol, luteolin, and apigenin) content of edible tropical plants. J. Agric. Food Chem. 49, 3106–3112. doi: 10.1021/jf000892m
Mitsumoto, A., and Nakagawa, Y. (2001). DJ-1 is an indicator for endogenous reactive oxygen species elicited by endotoxin. Free Radic. Res. 35, 885–893. doi: 10.1080/10715760100301381
Mortiboys, H., Aasly, J., and Bandmann, O. (2013). Ursocholanic acid rescues mitochondrial function in common forms of familial Parkinson’s disease. Brain 136, 3038–3050. doi: 10.1093/brain/awt224
Mortiboys, H., Johansen, K. K., Aasly, J. O., and Bandmann, O. (2010). Mitochondrial impairment inpatients with Parkinson disease with the G2019S mutation in LRRK2. Neurology 75, 2017–2020. doi: 10.1212/WNL.0b013e3181ff9685
Narendra, D., Tanaka, A., Suen, D. F., and Youle, R. J. (2008). Parkin is recruited selectively to impaired mitochondria and promotes their autophagy. J. Cell. Biol. 183, 795–803. doi: 10.1083/jcb.200809125
Ng, C. H., Guan, M. S., Koh, C., Ouyang, X., Yu, F., Tan, E. K., et al. (2012). AMP kinase activation mitigates dopaminergic dysfunction and mitochondrial abnormalities in drosophila models of Parkinson’s disease. J. Neurosci. 32, 14311–14317. doi: 10.1523/Jneurosci.0499-12.2012
Obrenovich, M. E., Nair, N. G., Beyaz, A., Aliev, G., and Reddy, V. P. (2010). The role of polyphenolic antioxidants in health, disease, and aging. Rejuvenation Res. 13, 631–643. doi: 10.1089/rej.2010.1043
Olatunji, O. J., Feng, Y., Olatunji, O. O., Tang, J., Ouyang, Z., and Su, Z. (2016). Cordycepin protects PC12 cells against 6-hydroxydopamine induced neurotoxicity via its antioxidant properties. Biomed. Pharmacother. 81, 7–14. doi: 10.1016/j.biopha.2016.03.009
Palacino, J. J., Sagi, D., Goldberg, M. S., Krauss, S., Motz, C., Wacker, M., et al. (2004). Mitochondrial dysfunction and oxidative damage in parkin-deficient mice. J. Biol. Chem. 279, 18614–18622. doi: 10.1074/jbc.M401135200
Peter, D., Jimenez, J., Liu, Y., Kim, J., and Edwards, R. H. (1994). The chromaffin granule and synaptic vesicle amine transporters differ in substrate recognition and sensitivity to inhibitors. J. Biol. Chem. 269, 7231–7237.
Pickrell, A. M., and Youle, R. J. (2015). The roles of PINK 1, parkin, and mitochondrial fidelity in Parkinson’s disease. Neuron 85, 257–273. doi: 10.1016/j.neuron.2014.12.007
Przedborski, S. (2017). The two-centry journey of Parkinson disease research. Nat. Rev. Neurosci. 18, 251–259. doi: 10.1038/nrn.2017.25
Rajeswari, A., and Sabesan, M. (2008). Inhibition of monoamine oxidase-B by the polyphenolic compound, curcumin and its metabolite tetrahydrocurcumin in a model of Parkinson’s disease induced by MPTP neurodegeneration in mice. Inflammopharmacology 16, 96–99. doi: 10.1007/s10787-007-1614-0
Reznichenko, L., Amit, T., Zheng, H., Avramovich-Tirosh, Y., Youdim, M. B., Weinreb, O., et al. (2006). Reduction of iron-regulated amyloid precursor protein and β-amyloid peptide by (-)-epigallocatechin-3-gallate in cell cultures: implications for iron chelation in Alzheimer’s disease. J. Neurochem. 97,527–536. doi: 10.1111/j.1471-4159.2006.03770.x
Rohas, L. M., St-Pierre, J., Uldry, M., Jäger, S., Handschin, C., and Spiegelman, B. M. (2007). A fundamental system of cellular energy homeostasis regulated by PGC-1alpha. Proc. Natl. Acad. Sci. U.S.A. 104, 7933–7938. doi: 10.1073/pnas.0702683104
Scarffe, L. A., Stevens, D. A., Dawson, V. L., and Dawson, T. M. (2014). Parkin and PINK1: much more than mitophagy. Trends Neurosci. 37, 315–324. doi: 10.1016/j.tins.2014.03.004
Schapira, A. H. (2007). Mitochondrial dysfunction in Parkinson’s disease. Cell Death Differ. 14, 1261–1266. doi: 10.1038/sj.cdd.4402160
Schapira, A. H. (2008). Mitochondria in the aetiology and pathogenesis of Parkinson’s disease. Lancet Neurol. 7, 97–109. doi: 10.1016/S1474-4422(07)70327-7
Schulz, J. B., Lindenau, J., Seyfried, J., and Dichgans, J. (2000). Glutathione, oxidative stress and neurodegeneration. Eur. J. Biochem. 267, 4904–4911. doi: 10.1046/j.1432-1327.2000.01595.x
Segura-Aguilar, J., Paris, I., Muñoz, P., Ferrari, E., Zecca, L., and Zucca, F. A. (2014). Protective and toxic roles of dopamine in Parkinson’s disease. J. Neurochem. 129, 898–915. doi: 10.1111/jnc.12686
Shadel, G. S., and Horvath, T. L. (2015). Mitochondrial ROS signaling in organismal homeostasis. Cell 163, 560–569. doi: 10.1016/j.cell.2015.10.001
Shi, X., Chen, Y. H., Liu, H., and Qu, H. D. (2016). Therapeutic effects of paeonol on methyl-4-phenyl-1,2,3,6-tetrahydropyridine/probenecid-induced Parkinson’s disease in mice. Mol. Med. Rep. 14, 2397–2404. doi: 10.3892/mmr.2016.5573
Singh, N. A., Mandal, A. K., and Khan, Z. A. (2016). Potential neuroprotective properties of epigallocatechin-3-gallate (EGCG). Nutr. J. 15:60. doi: 10.1186/s12937-016-0179-4
Sohal, R. S., and Weindruch, R. (1996). Oxidative stress, caloric restriction, and aging. Science 273, 59–63. doi: 10.1126/science.273.5271.59
Song, J. X., Shaw, P. C., Sze, C. W., Tong, Y., Yao, X. S., Ng, T. B., et al. (2010). Chrysotoxine, a novel bibenzyl compound, inhibits 6-hydroxydopamine induced apoptosis in SH-SY5Y cells via mitochondria protection and NF-kB modulation. Neurochem. Int. 57, 676–689. doi: 10.1016/j.neuint.2010.08.007
Song, J. X., Sze, S. C., Ng, T. B., Lee, C. K., Leung, G. P., Shaw, P. C., et al. (2012). Anti-Parkinsonian drug discovery from herbal medicines: what have we got from neurotoxic models. J. Ethnopharmacol. 139, 698–711. doi: 10.1016/j.jep.2011.12.030
Soobrattee, M. A., Bahorun, T., and Aruoma, O. I. (2010). Chemopreventive actions of polyphenolic compounds in cancer. Biofactors 27, 19–35. doi: 10.1002/biof.5520270103
Su, X., Chu, Y., Kordower, J. H., Li, B., Cao, H., Huang, L., et al. (2015). PGC-1α promoter methylation in Parkinson’s disease. PLoS One 8:e0134087. doi: 10.1371/journal.pone.0134087
Takahashi, S., Takahashi, I., Sato, H., Kubota, Y., Yoshida, S., and Muramatsu, Y. (2001). Age-related changes in the concentrations of major and trace elements in the brain of rats and mice. Biol. Trace Elem. Res. 80, 145–158. doi: 10.1385/BTER:80:2:145
Tan, J. W., Tham, C. L., Israf, D. A., Lee, S. H., and Kim, M. K. (2012). Neuroprotective effects of biochanin A against glutamate-induced cytotoxicity in PC12 cells via apoptosis inhibition. Neurochem. Res. 38, 512–518. doi: 10.1007/s11064-012-0943-6
Thomas, B., and Beal, M. F. (2007). Parkinson’s disease. Hum. Mol. Genet. 16, 183–194. doi: 10.1093/hmg/ddm159
Uttara, B., Singh, A. V., Zamboni, P., and Mahajan, R. T. (2009). Oxidative stress and neurodegenerative diseases: A review of upstream and downstream antioxidant therapeutic options. Curr. Neuropharmacol. 7, 65–74. doi: 10.2174/157015909787602823
Wang, J., He, C., Wu, W. Y., Chen, F., Wu, Y. Y., Li, W. Z., et al. (2015). Biochanin A protects dopaminergic neurons against lipopolysaccharide-induced damage and oxidative stress in a rat model of Parkinson’s disease. Pharmacol. Biochem. Behav. 138, 96–103. doi: 10.1016/j.pbb.2015.09.013
Wang, P., Niu, L., Gao, L., Li, W. X., Jia, D., Wang, X. L., et al. (2010). Neuroprotective effect of gypenosides against oxidative injury in the substantia nigra of a mouse model of Parkinson’s disease. J. Int. Med. Res. 38, 1084–1092. doi: 10.1177/147323001003800336
Wang, S., He, H., Chen, L., Zhang, W., Zhang, X., and Chen, J. (2015). Protective effects of salidroside in the MPTP/MPP+-induced model of Parkinson’s disease through ROS–NO-Related mitochondrion pathway. Mol. Neurobiol. 51,718–728. doi: 10.1007/s12035-014-8755-0
Weinreb, O., Amit, T., Mandel, S., and Youdim, M. B. (2009). Neuroprotective molecular mechanisms of (2)-epigallocatechin- 3-gallate: a reflective outcome of its antioxidant, iron chelating and neuritogenic properties. Genes Nutr. 4, 283–296. doi: 10.1007/s12263-009-0143-4
Whetten-Goldstein, K., Sloan, F., Kulas, E., Cutson, T., and Schenkman, M. (1997). The burden of Parkinson’s disease on society, family, and the individual. J. Am Geriatr. Soc. 45, 844–849. doi: 10.1111/j.1532-5415.1997.tb01512.x
Whitworth, A. J., Theodore, D. A., Greene, J. C., Benes, H., Wes, P. D., and Pallanck, L. J. (2005). Increased glutathione S-transferase activity rescues dopaminergic neuron loss in a Drosophila model of Parkinson’s disease. Proc. Natl. Acad. Sci. U.S.A. 102, 8024–8029. doi: 10.1073/pnas.0501078102
Winter, Y., von Campenhausen, S., Brozova, H., Skoupa, J., Reese, J. P., Bötzel, K., et al. (2010). Costs of Parkinson’s disease in Eastern Europe: a Czech cohort study. Parkinsonism Relat. Disord. 16, 51–56. doi: 10.1016/j.parkreldis.2009.07.005
Xu, C. L., Wang, Q. Z., Sun, L. M., Li, X. M., Deng, J. M., Li, L. F., et al. (2012). Asiaticoside: attenuation of neurotoxicity induced by MPTP in a rat model of Parkinsonism via maintaining redox balance and up-regulating the ratio of Bcl-2/Bax. Pharmacol. Biochem. Behav. 100, 413–418. doi: 10.1016/j.pbb.2011.09.014
Xu, H., Jiang, H., Wang, J., and Xie, J. (2010). Rg1 protects iron-induced neurotoxicity through antioxidant and iron regulatory proteins in 6-OHDA-treated MES23.5 cells. J. Cell. Biochem. 111, 1537–1545. doi: 10.1002/jcb.22885
Yan, M. H., Wang, X., and Zhu, X. (2013). Mitochondrial defects and oxidative stress in Alzheimer disease and Parkinson disease. Free Radic. Biol. Med. 62, 90–101. doi: 10.1016/j.freeradbiomed.2012.11.014
Yang, Y., Gehrke, S., Imai, Y., Huang, Z., Ouyang, Y., Wang, J.-W., et al. (2006). Mitochondrial pathology and muscle and dopaminergic neuron degeneration caused by inactivation of Drosophila Pink1 is rescued by Parkin. Proc. Natl. Acad. Sci. U.S.A. 103, 10793–10798. doi: 10.1073/pnas.0602493103
Ye, Q., Ye, L., Xu, X., Huang, B., Zhang, X., Zhu, Y., et al. (2012). Epigallocatechin-3-gallate suppresses 1-methyl-4-phenyl-pyridine-induced oxidative stress in PC12 cells via the SIRT1/PGC-1alpha signaling pathway. BMC Complement. Altern. Med. 12:82. doi: 10.1186/1472-6882-12-82
Zhang, X., Xu, J. K., Wang, J., Wang, N. L., Kurihara, H., Kitanaka, S., et al. (2007). Bioactive bibenzyl derivatives and fluorenones from dendrobium nobile. J. Nat. Prod. 70, 24–28. doi: 10.1021/np060449r
Keywords: Parkinson’s disease, dopaminergic neurons, oxidative stress, natural molecules, Chinese herb
Citation: Ding Y, Xin C, Zhang C-W, Lim K-L, Zhang H, Fu Z, Li L and Huang W (2018) Natural Molecules From Chinese Herbs Protecting Against Parkinson’s Disease via Anti-oxidative Stress. Front. Aging Neurosci. 10:246. doi: 10.3389/fnagi.2018.00246
Received: 25 December 2017; Accepted: 26 July 2018;
Published: 28 August 2018.
Edited by:
Hi-Joon Park, Kyung Hee University, South KoreaReviewed by:
Yu Tang, Central South University, ChinaMaria Shadrina, Institute of Molecular Genetics (RAS), Russia
Copyright © 2018 Ding, Xin, Zhang, Lim, Zhang, Fu, Li and Huang. This is an open-access article distributed under the terms of the Creative Commons Attribution License (CC BY). The use, distribution or reproduction in other forums is permitted, provided the original author(s) and the copyright owner(s) are credited and that the original publication in this journal is cited, in accordance with accepted academic practice. No use, distribution or reproduction is permitted which does not comply with these terms.
*Correspondence: Cheng-Wu Zhang, aWFtY3d6aGFuZ0BuanRlY2guZWR1LmNu; Lin Li, aWFtbGxpQG5qdGVjaC5lZHUuY24=