- 1Center for Cognitive Neurology, New York University Langone Health, New York, NY, United States
- 2Department of Neurology, New York University Langone Health, New York, NY, United States
- 3Department of Psychiatry, New York University Langone Health, New York, NY, United States
- 4Department of Physiology & Neuroscience, New York University Langone Medical Center, New York, NY, United States
- 5Department of Protein Science, School of Engineering Sciences in Chemistry, Biotechnology and Health, KTH Royal Institute of Technology, Stockholm, Sweden
- 6School of Medicine, King Abdulaziz University, Jeddah, Saudi Arabia
- 7Affibody AB, Solna, Sweden
- 8Department of Chemistry and Biotechnology, Swedish University of Agricultural Sciences (SLU), Uppsala, Sweden
- 9Department of Pathology, New York University School of Medicine, New York, NY, United States
Different strategies for treatment and prevention of Alzheimer’s disease (AD) are currently under investigation, including passive immunization with anti-amyloid β (anti-Aβ) monoclonal antibodies (mAbs). Here, we investigate the therapeutic potential of a novel type of Aβ-targeting agent based on an affibody molecule with fundamentally different properties to mAbs. We generated a therapeutic candidate, denoted ZSYM73-albumin-binding domain (ABD; 16.8 kDa), by genetic linkage of the dimeric ZSYM73 affibody for sequestering of monomeric Aβ-peptides and an ABD for extension of its in vivo half-life. Amyloid precursor protein (APP)/PS1 transgenic AD mice were administered with ZSYM73-ABD, followed by behavioral examination and immunohistochemistry. Results demonstrated rescued cognitive functions and significantly lower amyloid burden in the treated animals compared to controls. No toxicological symptoms or immunology-related side-effects were observed. To our knowledge, this is the first reported in vivo investigation of a systemically delivered scaffold protein against monomeric Aβ, demonstrating a therapeutic potential for prevention of AD.
Introduction
Alzheimer’s disease (AD) is the most common cause of dementia and is defined by a gradual onset and progression of deficits in several areas of cognition. More than 45 million people worldwide are affected and the associated costs on the society are enormous (Wisniewski and Goñi, 2015). Historically, AD has been characterized by its pathological signatures that include e.g., extracellular deposits of amyloid β (Aβ) and intracellular neurofibrillary tangles (NFTs; Nelson et al., 2012). Aβ peptides of various lengths are formed through sequential cleavage of the amyloid precursor protein (APP) by β- and γ-secretases. An important event in AD involves aggregation of soluble monomeric peptides into neurotoxic and insoluble β-sheet-rich inclusions in the brains of patients (Hardy and Selkoe, 2002). Present therapies for AD have either no or minimal disease-modifying effect, and thus, there is an urgent need for new effective treatments. Numerous therapeutic strategies are under investigation to delay the onset or slow progression of the disease (Wisniewski and Goñi, 2015; van Dyck, 2018). Active and passive immunotherapeutic approaches have been suggested to improve clinical progression and cognitive impairment through different mechanisms: (i) inhibition of Aβ production; (ii) interference with the formation of toxic aggregation intermediates; and (iii) accelerated clearance of Aβ from the CNS into the periphery (Citron, 2010; Karran et al., 2011). Several anti-Aβ antibodies have demonstrated effective clearance of Aβ together with cognitive improvements in transgenic animal models (Bard et al., 2000; DeMattos et al., 2001; Wilcock et al., 2004) and consequently progressed to clinical trials (Sevigny et al., 2016; Siemers et al., 2016; Herline et al., 2018b). However, translation to safe and efficacious therapies for humans has been challenging as AD clinical trials have failed to show sufficient clinical benefits (Doody et al., 2014; Salloway et al., 2014; Herline et al., 2018b). Recently, the monoclonal antibody (mAb) Solanezumab, that binds monomeric Aβ, was extensively evaluated in a phase III prevention trial in patients with mild AD. The study was however terminated due to failure in showing cognitive improvements. A recent phase Ib trial with the protofibril-binding mAb Aducanumab has demonstrated promising results, which has motivated an on-going phase III trial (Sevigny et al., 2016).
It has been proposed that challenges related to the failure in showing overall clinical improvement or clear disease-modifying results of these mAbs could be addressed to some of the inherent properties of antibodies (Pul et al., 2011; Herline et al., 2018b). Thus, new approaches based on engineered antibody domains or alternative scaffold-proteins that generally lack immunoglobulin-related effector functions are now investigated and moving into clinical development, as they might provide safer and more effective treatments (Robert and Wark, 2012; Nisbet et al., 2013; Vazquez-Lombardi et al., 2015). Antibody derivatives and non-immunoglobulin affinity proteins are in general smaller than full-length antibodies, and can typically be engineered into multivalent and multispecific constructs with an overall size that is still much smaller than a full-sized antibody (Löfblom et al., 2010; Robert and Wark, 2012; Vazquez-Lombardi et al., 2015; Ståhl et al., 2017). Their smaller size could potentially result in a different in vivo biodistribution profile as well as simplified administration routes, which could be important in the treatment of e.g., AD.
Affibody molecules represent a class of promising alternative scaffold proteins that have been investigated for various applications (Ståhl et al., 2017). Affibody molecules are small (6.5 kDa), three-helical bundle proteins, typically with high solubility, high expression yields in bacteria, the possibility of chemical synthesis as an alternative production strategy, and straightforward engineering of bispecific and bivalent constructs (Bass et al., 2017; Ståhl et al., 2017), which is often valuable for development of therapeutic constructs. Affibody molecules have been generated to numerous target proteins, with typical affinities in the low nanomolar to picomolar range (Ståhl et al., 2017). They have demonstrated significant potential as medical imaging agents, and have been generated to several different cancer biomarkers (Ståhl et al., 2017). A human epidermal growth factor receptor 2-targeting affibody molecule has been extensively evaluated in clinical trials as a breast cancer-imaging agent (Sörensen et al., 2014, 2016), which proved to be safe and efficacious.
For therapeutic applications, extended in vivo circulation times are generally required. Affibody molecules can be genetically fused to a 46 amino acid (5.2 kDa) albumin-binding domain (ABD), that has been deimmunized (Andersen et al., 2011; Frejd, 2012) and engineered to femtomolar affinity (Jonsson et al., 2008). This concept of half-life extension has been successfully explored in several preclinical affibody-based therapy studies (Tolmachev et al., 2007; Ståhl et al., 2017) and is currently being evaluated in a phase II clinical study where the half-life extension is used in combination with an IL-17 specific affibody for treatment of plaque psoriasis (Ståhl et al., 2017).
We have previously reported on the generation of an affibody molecule (denoted ZAb3) that binds to monomeric Aβ with a 17 nM affinity (Grönwall et al., 2007; Hoyer et al., 2008). This binder was evolved to adopt a unique structure upon binding of monomeric Aβ by engaging two identical disulfide-linked affibody units to sequester the aggregation-prone residues of the peptide in a tunnel-like cavity (Hoyer et al., 2008). Upon binding, both affibody units and the Aβ peptide undergo structural rearrangement and form an internal stabilizing β-sheet conformation (Hoyer and Härd, 2008), which might be more efficient for interactions with aggregation-prone peptides. This Aβ-sequestering affibody molecule has demonstrated efficient inhibition of formation of Aβ aggregates in an in vivo Drosophila AD model, and abolished the neurotoxic effects as well as restored the life span of the flies (Luheshi et al., 2010). The affibody molecule was further engineered into a truncated genetic dimer, thus reducing the overall size to 11.2 kDa, and increasing the affinity to Aβ (340 pM; Lindberg et al., 2015). In an in vitro binding assay, this second-generation Aβ-capturing affibody molecule (denoted ZSYM73), genetically linked to an ABD at the C-terminal, demonstrated efficient capture of physiological concentrations of monomeric Aβ from a complex mixture of proteins while simultaneously binding to serum albumin via the ABD, an important feature in a potential therapeutic setting (Lindberg et al., 2015). It was speculated that ZSYM73 could be an interesting candidate to assess as a prevention drug for AD in relevant animal models (De Genst and Muyldermans, 2015).
Encouraged by these positive results, we here investigate the efficacy of ZSYM73-ABD (total size 16.8 kDa) as a therapeutic candidate to prevent the development of AD-related pathology in transgenic AD mice. ZSYM73-ABD and a negative control protein (a dimeric variant of a Taq polymerase-binding affibody molecule genetically linked to ABD) were produced in E. coli and recovered to high purity, and the preventive efficacy was assessed in APP/PS1 double transgenic mice (Holcomb et al., 1998; Puzzo et al., 2015). The animals received three weekly injections of 100 μg therapeutic protein or negative control protein during 13 weeks, starting at the expected onset of pathology development. Extensive behavioral assessment together with histological evaluation demonstrated a significantly lower amyloid burden in both cortex and hippocampus, as well as rescued cognitive functions of the ZSYM73-ABD treated mice relative to controls. This study is the first in vivo investigation of a systemically delivered scaffold protein binding to monomeric Aβ, which demonstrates a preventive therapeutic efficacy on the development of disease-related pathology in a mouse model of AD.
Materials and Methods
Production of Affibody Fusion Proteins
Gene fragments encoding ZSYM73 (Lindberg et al., 2015) or a dimer of the control affibody molecule ZTaq (Gunneriusson et al., 1999) were fused to the gene for a deimmunized high-affinity ABD (Jonsson et al., 2008; Frejd, 2012; Malm et al., 2013) and inserted into expression vectors containing a T7 promoter system and a kanamycin resistance gene. The vectors thus encoded the fusion proteins (ZSYM73)-GAPG4STS-ABD (hereinafter denoted ZSYM73-ABD) and (ZTaq)2-GAPG4STS-ABD [hereinafter denoted (ZTaq)2-ABD]. DNA sequence verification was performed using BigDye Thermo Cycle Sequencing reactions with an ABI Prism 3700 instrument (Applied Biosystems, Foster City, CA, USA). The affibody-fusion proteins were produced in E. coli BL21 DE3 cells (Novagen, Madison, WI, USA) with protein expression induced by 0.2 mM isopropyl β-D-1-thiogalactopyranoside (IPTG), followed by cultivation in a multi-fermentor system (GRETA, Belach Bioteknik AB, Solna, Sweden). The cells were harvested, disrupted by sonication, and purified, in principle as described previously (Lindberg et al., 2015) however using an affinity chromatography column with an anti-ABD Sepharose matrix (Affibody AB, Solna, Sweden). Additional purification steps were performed using reverse phase chromatography on an ÄKTA Explorer 100 system, and size exclusion chromatography using a HiLoad 16/60 column together with an ÄKTA system (GE-Healthcare, Uppsala, Sweden) and using PBS as running buffer. After purification, potential residual endotoxins were removed by passing the proteins through 1-mL EndoTrap columns (Hyglos), according to the supplier’s recommendations. The proteins were eluted in DPBS (Gibco, Life Technologies), followed by determination of endotoxin levels (APJ, Stockholm). A hexahistidine-tagged ZSYM73 affibody molecule (hereinafter denoted ZSYM73-His6) was also produced and purified as previously described (Lindberg et al., 2015).
Characterization of Affibody Proteins
The molecular masses of the proteins were analyzed using MALDI mass spectrometry (4800 MALDI TOF-TOF, Sciex) and SDS-PAGE under both reducing and non-reducing conditions. The secondary structure content was analyzed at a concentration of 0.5 mg/mL in PBS using circular dichroism (CD) spectroscopy on a Chirascan spectropolarimeter (Applied Photophysics, United Kingdom) in a quartz cell with an optical path-length of 1 mm. CD spectra were collected from 250 to 195 nm at 20°C, before and after variable temperature measurement. The thermal stability (variable temperature measurement) was measured at 221 nm while heating the proteins from 20 to 90°C (1°C/min). In-solution kinetics and affinity between ZSYM73-ABD and Aβ40, Aβ42 [both from AnaSpec, San Jose, CA, USA; in the presence of human serum albumin (HSA)] and HSA (Albucult, Sigma-Aldrich), respectively, were determined using a kinetic exclusion assay (KinExA, Sapidyne Instruments Inc., Boise, ID, USA). In addition, the binding ability of ZSYM73-His6 (Lindberg et al., 2015) to Aβ40 and Aβ42 (AnaSpec, San Jose, CA, USA), respectively, were measured. The ka and the KD of the different interactions were measured in separate analyses. Briefly, the ka values were determined by incubating constant concentrations of affibody molecule and target protein and measuring the free amount of affibody molecule after different incubation times. The KD values were measured by incubation of varying concentrations (two-fold dilutions) of the target protein with constant concentrations of affibody molecule and measuring the free amount of affibody molecule after a constant incubation time. In both types of measurements, free amount of affibody molecule was quantified using streptavidin-coated PMMA beads (Sapidyne Instruments), immobilized with biotinylated Aβ40 or biotinylated HSA depending on the binding event that was measured. Detection was achieved using a mouse anti-His6 IgG (Sapidyne Instruments) for ZSYM73-His6 analyses, a mouse anti-affibody mAb (Affibody AB) for HSA-binding and a mouse anti-HSA mAb (Abcam, Cambridge, UK) for Aβ40 and Aβ42-binding for ZSYM73-ABD analyses. In all cases, a goat anti-mouse polyclonal antibody (pAb) conjugated with Daylight 650 (Sapidyne Instruments) was used as a secondary step in the detection. Serum stability of ZSYM73-ABD was investigated by incubation of ZSYM73-ABD in a human plasma pool at 37°C during 72 h at a concentration of 10 μg/mL. Retained affinity to Aβ was investigated in a conventional ELISA with biotinylated Aβ40 bound to streptavidin in ELISA wells. Two-step dilutions series of ZSYM73-ABD incubated in plasma for 28 days, compared to an untreated sample, were detected using an HRP-conjugated mouse anti-Z mAb (Affibody AB) followed by a one-step ultra-tetramethyl benzidine (TMB; Thermo Fisher Scientific, Rockford, IL, USA) and measured at 450 nm.
AD Mouse Model and Treatment
This study was carried out in accordance with the recommendations of the New York University School of Medicine Institutional Animal Care and Use Committee. The protocol was approved by the New York University School of Medicine Institutional Animal Care and Use Committee. APP/PS1 (APPK670N/M671L and PS1M146V transgenes) have been extensively used as a model of amyloid plaque deposition (Holcomb et al., 1998; Puzzo et al., 2015). The mice were bred at the NYU School of Medicine and were maintained on a 12 h light/dark cycle. Animals were injected intraperitoneally (i.p.) with either the Aβ-binding ZSYM73-ABD or the negative control (ZTaq)2-ABD affibody protein. Twenty mice were divided into two study groups with 10 animals in each that received three weekly injections of 100 μg affibody molecule (corresponding to a plasma concentration of ~1.5 μM that is estimated to fall to 0.2 μM after 3 days) for 13 weeks starting at the age of 4 months. In this model, amyloid plaque pathology starts at about 3 1/2 months of age; hence treatment was initiated at the beginning of pathology development (Holcomb et al., 1998; Drummond and Wisniewski, 2017). During the treatment, the mice were weighed and examined for general health indicators.
Plasma Levels of Affibody Molecule
The mice were bled before the commencement of the study (T0) and periodically throughout the experiment as follows: 24 h after the 39th injection (T1), 7 days after the 39th injection (T2), and 14 days after the 39th injection (T3). Plasma levels of affibody molecule were detected using ELISA. Aβ peptide was coated onto microtiter wells (Immulon 2HB; Thermo Electron Corp., Milford, MA, USA), following detection of affibody molecules in plasma using a goat α-affibody IgG (1:1,000 dilution, ADIDAS, Affibody AB). Bound α-affibody antibodies were detected using biotinylated anti-goat IgG (Amersham Biosciences, Piscataway, NJ, USA), followed by a Streptavidin HRP. TMB (Pierce, Rockford, IL, USA) was used as a color developing substrate and measured at 450 nm.
Sensorimotor Activity
Prior to cognitive assessment, the locomotor activity and motor coordination of the mice were tested to verify that any treatment-related effects observed in the cognitive tasks could not be explained by differences in sensorimotor abilities. Before testing, the animals were adapted to the room with lights on for 15 min.
For exploratory locomotor assessment, each mouse was habituated in a circular open field chamber (70 × 9 × 70 cm) for 15 min during which they were allowed to explore the environment. Horizontal movements in each dimension of the open field (i.e., x, y, and two z planes) were automatically recorded by a video camera (Hamilton-Kinder Smart-frame Photobeam System) mounted above the chamber. Results were reported as distance traveled (cm), average and maximum travel velocity (cm/s) and mean resting time (s) of the mouse. After each session, the field was cleaned with water and 30% ethanol.
To assess motor behavior in terms of forelimb and hindlimb coordination as well as balance, each animal was placed onto a 3.6 cm diameter rod (Rotarod 7650 accelerating model; Ugo Basile, 114 Biological Research Apparatus, Varese, Italy). First, the mice received two training trials to reach a baseline level of performance, where after they were tested in three additional trials with an initial speed of 1.5 rpm that was gradually increased by 0.5 rpm every 30 s to a maximum speed of 40.0 rpm. In each trial, the animal was tested in three sessions, each separated by 15 min. A soft foam cushion was placed under the rod to prevent injury from falling. To assess the performance, total time on the rod and rotation speed in rounds per minute were recorded when the animal fell onto a soft foam cushion or inverted (by clinging) from the top of the rotating barrel. The rod was cleaned with water and 30% ethanol after each session.
Radial Arm Maze
Spatial learning (working memory) was assessed using a radial maze with eight 30-cm arms originating from the central space, with a water-well at the end of each arm (Liu et al., 2014). An age-match group of non-transgenic mice was also tested in the radial arm maze. Clear Plexiglas guillotine doors were operated by a remote pulley system, which controlled access to the arms from the central area from where the animals were allowed to enter and exit all arms of the apparatus. Before testing, the mice were deprived of water for 24 h and then their access to water was restricted to 1 h per day for the duration of testing. After 2 days of adaptation, the mice were subjected to testing for nine consecutive days. This relatively long period of adaptation has been found important as these transgenic mice tend to be anxious and will not run the maze well otherwise. Prior to each testing day, the mice were adapted to the room with lights on for 15 min. For each session, all arms were baited with 0.1% saccharine solution and the animals were permitted to enter all arms until the eight rewards had been consumed. The number of errors, i.e., entries into previously visited arms and time to complete each session were recorded. An individual that was blinded to the status of the animal’s treatment performed the behavioral testing.
Histology
Following behavioral testing, the mice were anesthetized with sodium pentobarbital (150 mg/kg, i.p.), perfused transaortically with 0.1 M PBS, pH 7.4. The brains were immediately removed and processed. The right hemisphere was immersion-fixed overnight in periodate-lysine-paraformaldehyde. Serial coronal brain sections (40 μm) were cut (approximately 40 sections in total), of which 10 were stained for histological and immunohistochemical analysis with: (i) a mixture of anti-Aβ mAbs 6E10 (Biosource, Camarillo, CA, USA) and 4G8 (Biosource); (ii) polyclonal anti-glial fibrillary acidic protein (anti-GFAP) antibody; or (iii) anti-Iba-1 antibody. 6E10 and 4G8 are mAbs that recognize Aβ and stain both pre-amyloid and Aβ plaques. The staining was performed with a combination of the antibodies, as each labels a portion of amyloid plaques, while the combination labels all plaques. GFAP is a component of the glial intermediate filaments that form part of the cytoskeleton in astrocytes and is often employed as a marker of astrocyte activation. Iba-1 is a commonly used marker for microglial activation at both early and later stages of plaque development. All procedures were performed by an individual blinded to the experimental study and conducted on free-floating sections, in principle as previously described (Boutajangout et al., 2009, 2017; Scholtzova et al., 2009, 2014, 2017; Liu et al., 2014). Briefly, sections were incubated with primary mouse monoclonal anti-Aβ antibodies 6E10 and 4G8 (Covance Research Products Inc., Denver, PA, USA) at 1:1,000 dilution for 3 h, secondary biotinylated mouse anti-mouse IgG antibody for 1 h at 1:2,000 dilution, and subsequent avidin-peroxidase complex for 30 min at the same dilution. The sections were thereafter reacted in 3, 3-diaminobenzidine tetrahydrochloride with nickel ammonium sulfate (Mallinckrodt, Paris, KY, USA) color intensification solution. GFAP immunostaining was performed by incubation with primary polyclonal anti-GFAP (Dako Inc., Carpinteria, CA, USA) at a 1:1,000 diluent composed of 0.3% Triton X-100, 0.1% sodium azide, 0.01% bacitracin, 1% bovine serum albumin (BSA), and 10% normal goat serum in PBS for 3 h, followed by secondary biotinylated goat anti-rabbit antibody (Vector Laboratories Inc., Burlingame, CA, USA) for 1 h at 1:1,000 dilution. Iba-1 immunohistochemistry was performed similarly to that for GFAP staining with the exception that a secondary goat anti-rat antibody was used (1:1,000, Vector Laboratories Inc., Burlingame, CA, USA). Equally spaced sections were mounted and stained in a solution containing 10% potassium ferrocyanide and 20% hydrochloric acid for 45 min. Stained sections were examined for each mouse and the average number of iron positive profiles per section was calculated.
Perl’s iron stain was performed on another set of sections to detect cerebral microhemorrhages/bleeding. Sections were stained in a solution containing 5% potassium ferrocyanide and 10% hydrochloric acid for 30 min. This method has been used in previous reports that showed that passive immunization against Aβ increased the frequency of microhemorrhages in AD model mice (Pfeifer L. A. et al., 2002; Wilcock et al., 2004; Racke et al., 2005). Diamino benzidine intensification of the iron staining, which is useful for detecting low levels of iron in Aβ plaques, did not appear to improve sensitivity for detecting the microhemorrhages and was therefore not employed.
Image Analysis—Quantification of Amyloid Burden
Amyloid burden was quantified by a Bioquant stereology image analysis system (BIOQUANT Image Analysis Corporation, Nashville, TN, USA), using a random unbiased sampling scheme. Approximately 10 cortical and hippocampal sections, respectively, were analyzed per animal. Total Aβ burden (defined as the percentage of test area occupied by Aβ immunoreactivity) was quantified on coronal plane sections stained with the mixture of anti-Aβ antibodies 6E10/4G8 as previously described (Scholtzova et al., 2017; Liu et al., 2017; Goñi et al., 2018; Herline et al., 2018a). Intensification with nickel ammonium sulfate resulted in Aβ deposits being labeled black with minimal background staining that facilitated thresholding. Reactive astrocytosis was rated on a scale of 0–4. The rating was based on a semi-quantitative analysis of the extent of GFAP immunoreactivity (number of GFAP immunoreactive cells and complexity of astrocytic branching), as previously published (Goñi et al., 2013). The assessment of the Iba-1 immunostained sections was based on a semiquantitative analysis of the extent of microgliosis (0, none; 1, a few resting microglia; 2, moderate number; 3, numerous ramified/phagocytic microglia; 4, high number of microglia) in increments of 0.5, as previously reported (Scholtzova et al., 2009; Liu et al., 2014). Sections were analyzed per animal by an investigator who was blinded to the treatment status of the mice.
Statistical Analysis
Data from the accelerating rotor rod and locomotor test were analyzed by one-way ANOVA. The data collected from the radial arm maze test was analyzed by two-way measures ANOVA followed by a Neuman-Leuls post hoc test. Differences between groups in total amyloid burden, activated microglia (Iba-1), GFAP astrogliosis, brain microhemorrhages, were analyzed using a Student’s unpaired two-tailed t-test or one-tailed t-test. All statistical tests were performed using Prism 6.0 (Graphpad, San Diego, CA, USA).
CSF Bioavailability in Naïve Rats
Plasma and cerebrospinal fluid (CSF) profiles of the Aβ-binding affibody molecule were assessed to determine the CSF bioavailability in naïve rats. Rat care and experimental procedures were granted by the regional animal experimental ethics committee in Stockholm (North, N81/14). The rats were kept at Adlego Biomedical AB and maintained on a 12 h light/dark cycle. Sixteen male Sprague-Dawley rats were divided into two study groups that received intravenous injections of equimolar amounts (119 nmol/kg) of either ZSYM73-ABD or an Aβ-binding mAb (U-Protein Express, Utrecht, Netherlands) for comparison (both at 5 mL/kg). Prior to sampling of blood and CSF (at times 0, 3, 24, and 120 h), the animals were anesthetized with Ketaminol (75 mg/kg) + Rompun (10 mg/kg). CSF was sampled from Cisterna magna under anesthesia by puncturing the membrane between the occipital bone and the C1 cervical vertebrae using a needle. The membrane was exposed by opening the skin that covers the occipital bone with a minimal incision and the underlying muscles were separated by tweezers. Serum samples were prepared from blood by centrifugation. During the treatment, the rats were weighed and examined for general health indicators at each day of blood sampling. CSF and plasma samples were analyzed for ZSYM73-ABD or the Aβ-binding mAb by ELISA. Briefly, a mouse monoclonal anti-affibody was coated at 2 μg/mL in a 96 well PS microplate (Costar) overnight. Wells were washed in PBST (PBS with 0.05% Tween 20), and subsequently blocked in BlockerCasein-PBS (BCP, ThermoScientific) for 1.5 h at RT. Samples (at 20,000 pg/mL) were added to the wells, followed by addition of serum diluted 100- or 1,000-fold. For detection, primary antibody polyclonal rabbit anti-ABD antibodies were added to each well at 3 μg/mL, and incubated for 1.5 h at RT. Secondary reagent Jackson anti-rabbit IgG-HRP was added at 100 ng/mL for 1 h at RT. To develop the ELISA, ImmunoPure TMB was added to each well according to the manufacturer’s recommendations.
Results
Production of ZSYM73-ABD and (ZTaq)2-ABD
The therapeutic candidate was designed as a bispecific fusion protein, consisting of a genetic linkage between the high-affinity Aβ-capturing ZSYM73 affibody molecule and an ABD (Figure 1; Lindberg et al., 2015). In this format, ZSYM73-ABD (16.8 kDa) would be able to capture soluble Aβ peptides while simultaneously binding and circulating with HSA and thus extending the in vivo half-life of the protein (Andersen et al., 2011; Frejd, 2012). The ABD-moiety used in this study was a deimmunized variant with an engineered femtomolar affinity for HSA. The ABD also binds to serum albumin from other species, including murine serum albumin (MSA) to allow relevant preclinical evaluations (Jonsson et al., 2008; Frejd, 2012). A size-matched dimeric (ZTaq)2-ABD affibody molecule binding to DNA polymerase from Thermus aquaticus, was included as a negative control protein. The two fusion proteins were produced in E. coli and purified by affinity chromatography, reverse phase chromatography and size exclusion chromatography. Endotoxin content was analyzed and found to be below 0.15 EU/mL. In addition to this, a hexahistidine-tagged ZSYM73 protein was also produced and purified, as described elsewhere (Lindberg et al., 2015).
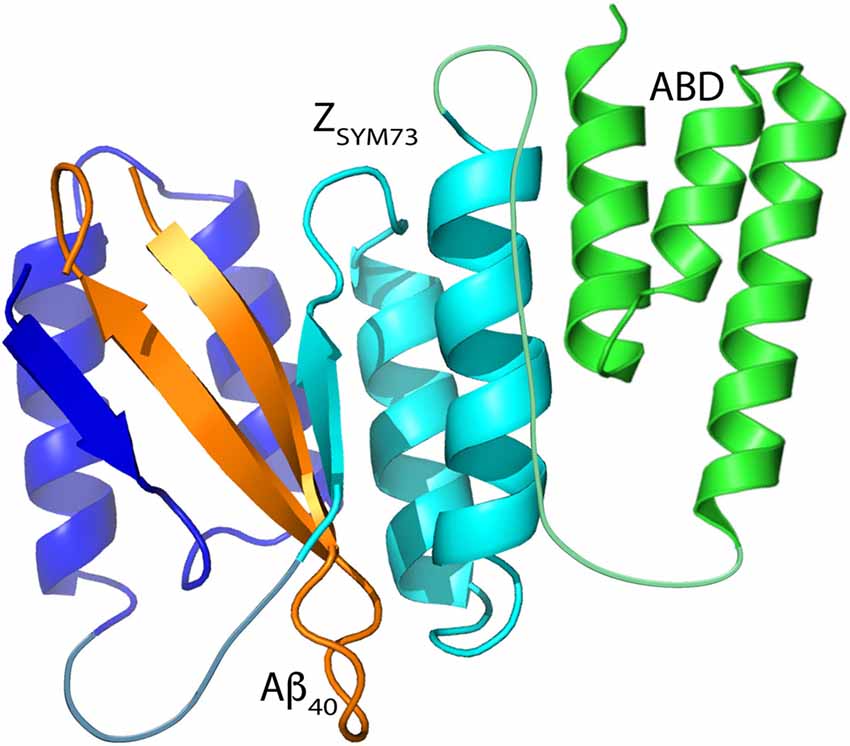
Figure 1. Schematic representation of ZSYM73-albumin-binding domain (ABD) in complex with amyloid β1–40 (Aβ)1–40. Figure adapted from Protein Data Bank entry 2OTK (ZSYM73:Aβ1–40) and Protein Data Bank entry 1GJS (ABD). The affibody subunits are illustrated in blue and cyan, the β-hairpin forming Aβ peptide is illustrated in orange, and the ABD is illustrated in green.
Characterization of the ZSYM73 Affibody Fusion Protein
The purified proteins were analyzed by SDS-PAGE and MALDI-MS. The molecular masses were measured to 16780.3 Da for ZSYM73-ABD and 18662.8 Da for (ZTaq)2-ABD (Supplementary Figure S1A,B), which was in agreement with the expected theoretical values of 16782 Da and 18672 Da, respectively. The secondary structure content and refolding of ZSYM73-ABD was investigated using CD spectroscopy. CD spectra were recorded before and after the variable temperature measurement and showed almost complete overlap, suggesting reversible folding after heat-induced denaturation (Supplementary Figure S1C). The equilibrium dissociation constants and kinetic association rate constants of both ZSYM73-ABD and ZSYM73-His6 were investigated using a KinExA. The results demonstrated that the hexahistidine-fused ZSYM73 binds Aβ40 with a KD ~12 pM and Aβ42 with a KD ~21 pM (Table 1), corresponding to approximately 28-fold higher affinity than previously reported using a surface-based biosensor assay (Lindberg et al., 2015). ZSYM73-ABD demonstrated affinities of ~60 pM to both Aβ40 and Aβ42 while simultaneously binding HSA with an equilibrium dissociation constant of ~50 pM (Table 1). The ability of ZSYM73-ABD to bind Aβ from a plasma sample at 37°C was investigated over time in an ELISA (Supplementary Figure S2). The results showed that ZSYM73-ABD retained its binding activity for at least 3 days in human plasma, compared to a non-treated sample.

Table 1. Equilibrium dissociation constants (KD), association rate constants (ka), and dissociation rate constants (kd) for the amyloid β (Aβ)-binding Affibody molecules, determined by KinExA.
Preventive Treatment of 2xTg Mice and Safety Assessment
Mice with APP/PS1 (APPK670N/M671L and PS1M146V) transgenes develop early and progressive vascular amyloid plaque pathology and were thus used to examine the preventive efficacy of the high-affinity ZSYM73-ABD candidate on the development of Aβ pathology. A scheme for the treatment strategy is outlined in Figure 2. At the onset of pathology (at approximately 3 months of age) and continuing for 13 weeks, each of 10 mice received three intraperitoneal (i.p.) injections of 100 μg ZSYM73-ABD (corresponding to a plasma concentration of ~1.5 μM that is estimated to fall to ~0.2 μM after 3 days) or the negative control protein (ZTaq)2-ABD per week. This amount was calculated to yield an equal or somewhat better exposure to that of concurrent mAbs when evaluated in mouse models. No differences in general health measures or any treatment-induced side-effects were observed across the experimental groups after the treatment (data not shown), indicating that the affibody fusion proteins were well tolerated and non-toxic. No antibodies that were reactive with ZSYM73-ABD could be detected after 39 i.p. injections (data not shown). These results correlate with results from a precursor of ZSYM73 that was previously evaluated as an ABD-fusion in rats, showing that no antibodies were detected to the fusion protein after 10 subcutaneous administrations over a period of 10 months (Fredrik Frejd, personal communication, International Publication Number WO 2005. /097202 A2).
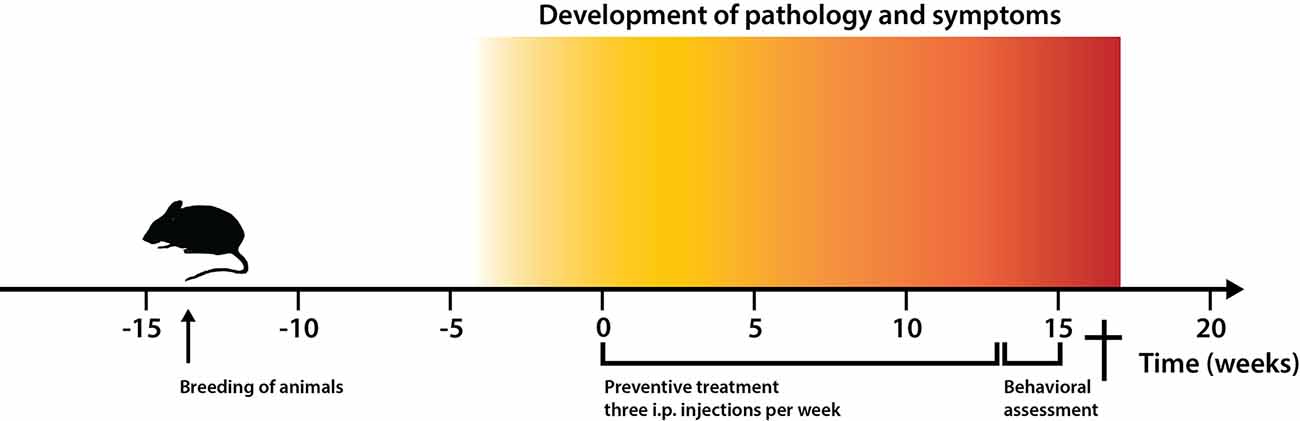
Figure 2. Experimental timeline for the treatment strategy of 2xTg Alzheimer’s disease (AD) mice. Timeline showing time points for: (i) breeding of animals with double transgenes amyloid precursor protein (APPK670N/M671L and PS1M146V) for vascular amyloid deposition; (ii) pathology development (3 months); (iii) start and duration of preventive treatment with the Aβ-binding ZSYM73-ABD affibody molecule and control (ZTaq)2-ABD; and (iv) behavioral assessment of animals.
Circulatory Half-Life of ZSYM73-ABD
Plasma levels of ZSYM73-ABD were measured using ELISA prior to the first injection (T0), 24 h after the 39th injection (T1), then 7 (T2) and 14 (T3) days after the 39th injection. Based on this, the circulatory half-life of ZSYM73-ABD was estimated to approximately 35 h, which is in accordance with previous studies on ABD-fused affibody molecules in mice (Tolmachev et al., 2007; Supplementary Figure S3).
Behavioral Studies
After the treatment period, both groups were subjected to behavioral testing. To verify that the results from the cognitive tests were not confounded by differences in sensorimotor abilities between the animals, sensorimotor testing was first conducted. No statistical differences were observed between the groups in rotarod (Figure 3A) or locomotor activity in terms of distance traveled, maximum speed, mean velocity and rest time (Figure 3B). The two groups, and an age-matched non-transgenic control group were subsequently tested in an eight-arm radial maze. The ZSYM73-ABD treated animals showed significant cognitive rescue as they navigated the maze with fewer errors than mice treated with the (ZTaq)2-ABD control protein (p < 0.0001 ANOVA two-way repeated measures for treatment effect). In addition, their performance was equal to that of the age-matched wild-type control group (Figure 3C), demonstrating that the ZSYM73-ABD treatment had a significant beneficial effect on the cognitive functions in this mouse model.
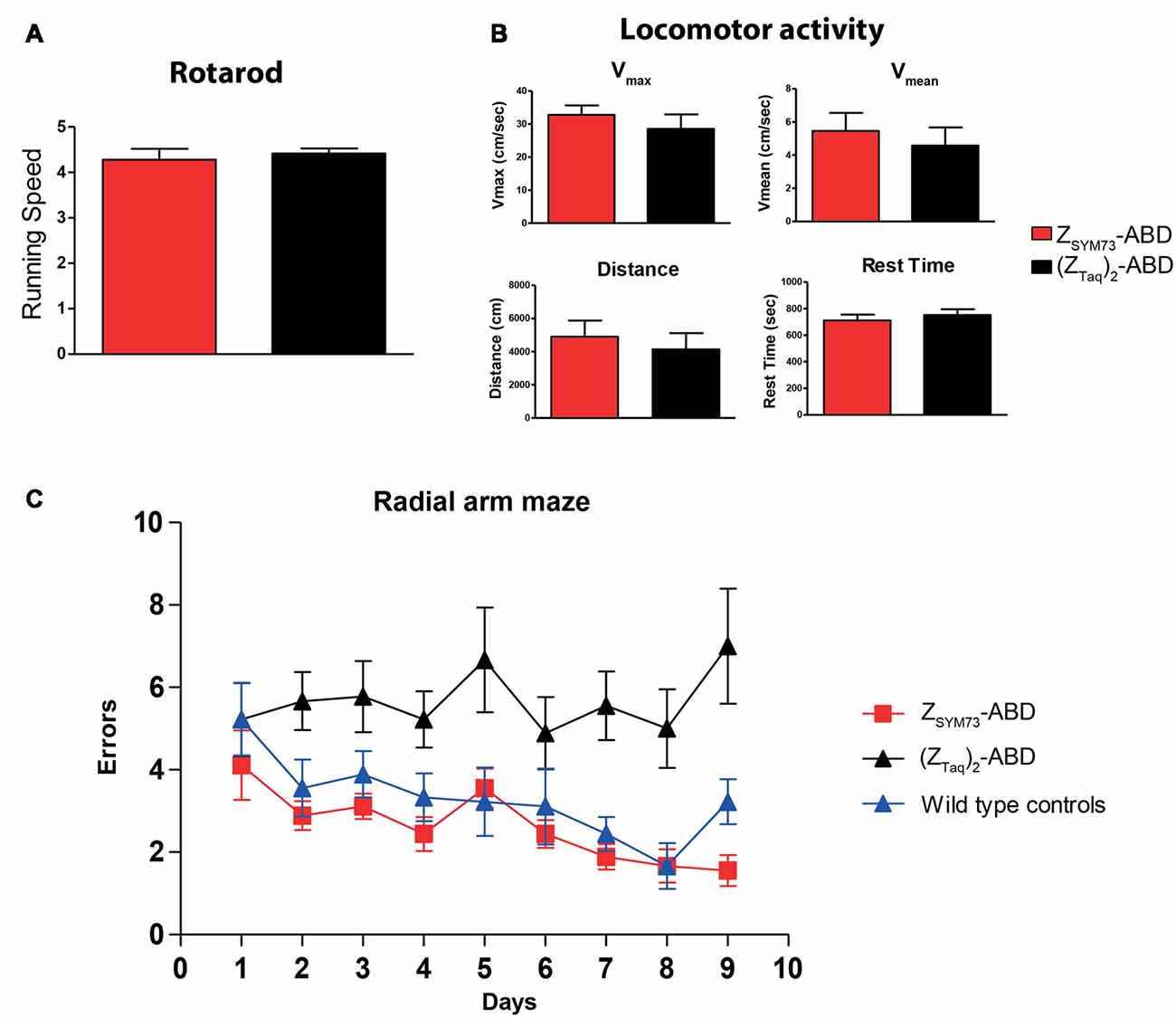
Figure 3. Cognitive testing and locomotor assessment of the affibody-administered APP/PS1 mice. (A,B) Assessment of sensorimotor abilities in 2xTg mice administered with the ZSYM73-ABD affibody molecule (red) or control (ZTaq)2-ABD (black) in (A) rotarod and (B) open field locomotor tests in which distance traveled, resting time, maximum velocity and average speed of mice were tested. (C) Radial arm maze testing (working memory assessment) of the ZSYM73-ABD (red) and (ZTaq)2-ABD (black) treated 2xTg mice. A group of wild type healthy control mice was included for comparison (blue). Animals were allowed to navigate the maze once per day for nine consecutive days. The number of errors that the mice performed on each testing day is plotted vs. the days of testing (p < 0.0001, by two-way ANOVA for treatment effect).
Histological Quantification of Amyloid Burden
The preventive effect of ZSYM73-ABD was further assessed in terms of total amounts of amyloid burden in the regions of cortex and hippocampus (Figures 4A–H). The mice were sacrificed and their brains were processed for histology. Quantification of stained brain slices showed significantly lower amyloid burden (percentage area occupied by 4G8/6E10 immunoreactivity) for the ZSYM73-ABD treated APP/PS1 Tg mice compared to the control group in both the cortex (46% lower, p = 0.03 one-tailed t-test, Figures 4C,F,H) and hippocampus (37% lower, p = 0.008 one-tailed t-test, Figures 4B,E,G).
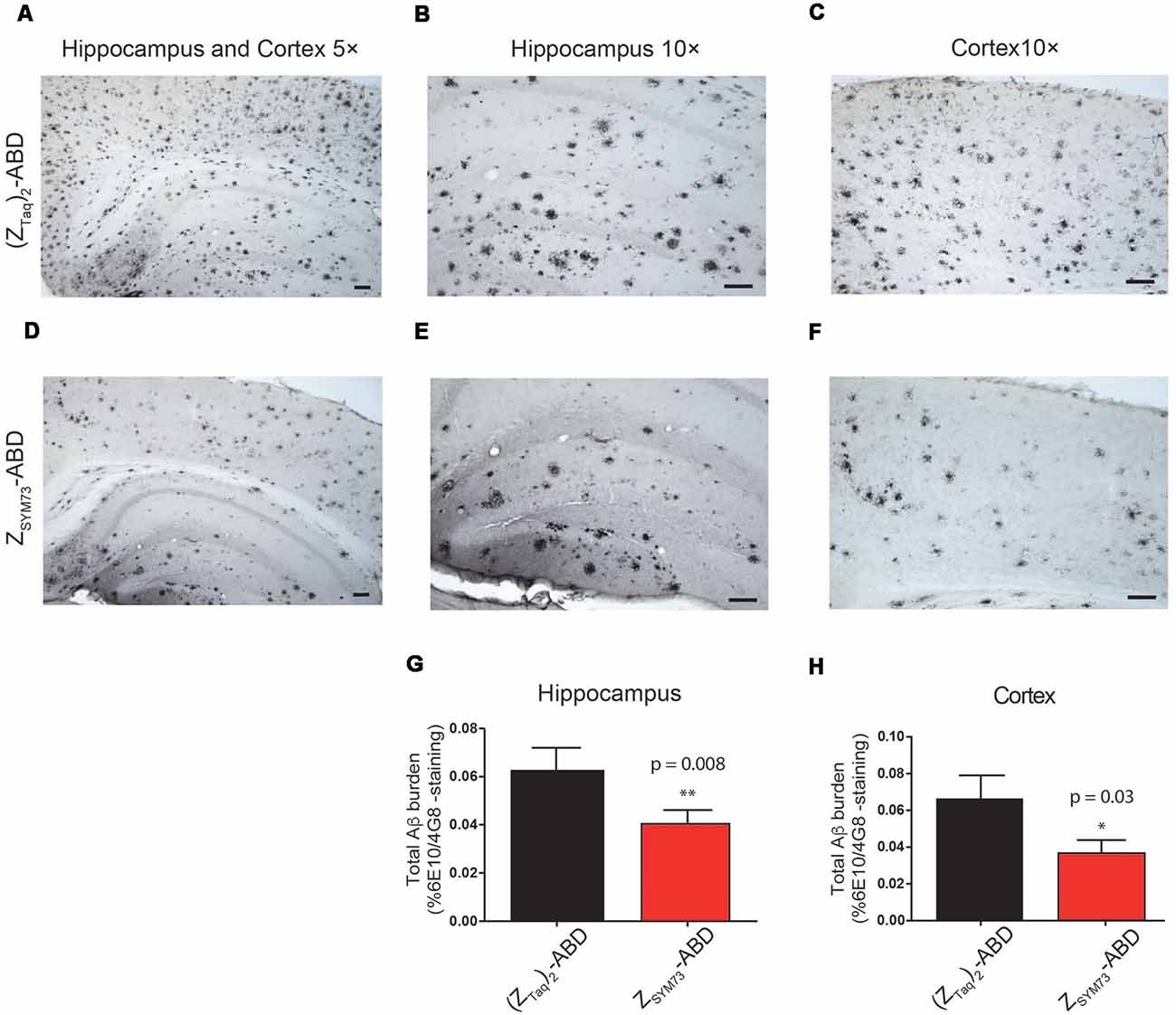
Figure 4. Histological evaluation of total amyloid brain burden. Representative immunohistochemical images and quantitative serological analysis of total amyloid burden using Aβ-binding antibodies 6E10/4G8 on brain sections from ZSYM73-ABD (red) or (ZTaq)2-ABD (black) control-treated mice, at 5× magnification (A,D). Amyloid reduction in cortical (46% reduction, *p = 0.03, one-tailed t-test; C,F,H) and hippocampal (37% reduction, **p = 0.008, one-tailed t-test; B,E,G) brain sections at 10× magnification, scale bar (100 μm).
Neuroinflammatory Response and Brain Microhemorrhages After Treatment
To evaluate the effect of the ZSYM73-ABD treatment on brain inflammation, serial sections were stained with anti-GFAP (Figures 5A–L) or anti-Iba-1 antibodies (Figures 5M–X), reactive to astrocytes and both active and resting microglia, respectively. Semi-quantitative analysis of GFAP immunoreactive cells and complexity of astrocytic branching showed no significant difference between treated and control groups in either the cortex (Figures 5B,G) or hippocampus (Figures 5C,H; p = 0.11 and p = 0.06, respectively; one-tailed t-test). Furthermore, administration of ZSYM73-ABD did not alter the microglia Iba-1 immunoreactivity in the cortex (Figures 5N,S) or hippocampus (Figures 5O,T) in the mice. Cerebral microhemorrhages were analyzed in brain sections stained with Perl’s stain and using semi-quantitative analysis. No significant differences between the ZSYM73-ABD treated animals and controls were observed in the amount of detected iron-positive profiles per brain section (p = 0.29; one-tailed t-test; Figures 6A–C). Taken together, this indicates that treatment with the Aβ-sequestering affibody did not result in neuroinflammatory responses or brain microhemorrhages.
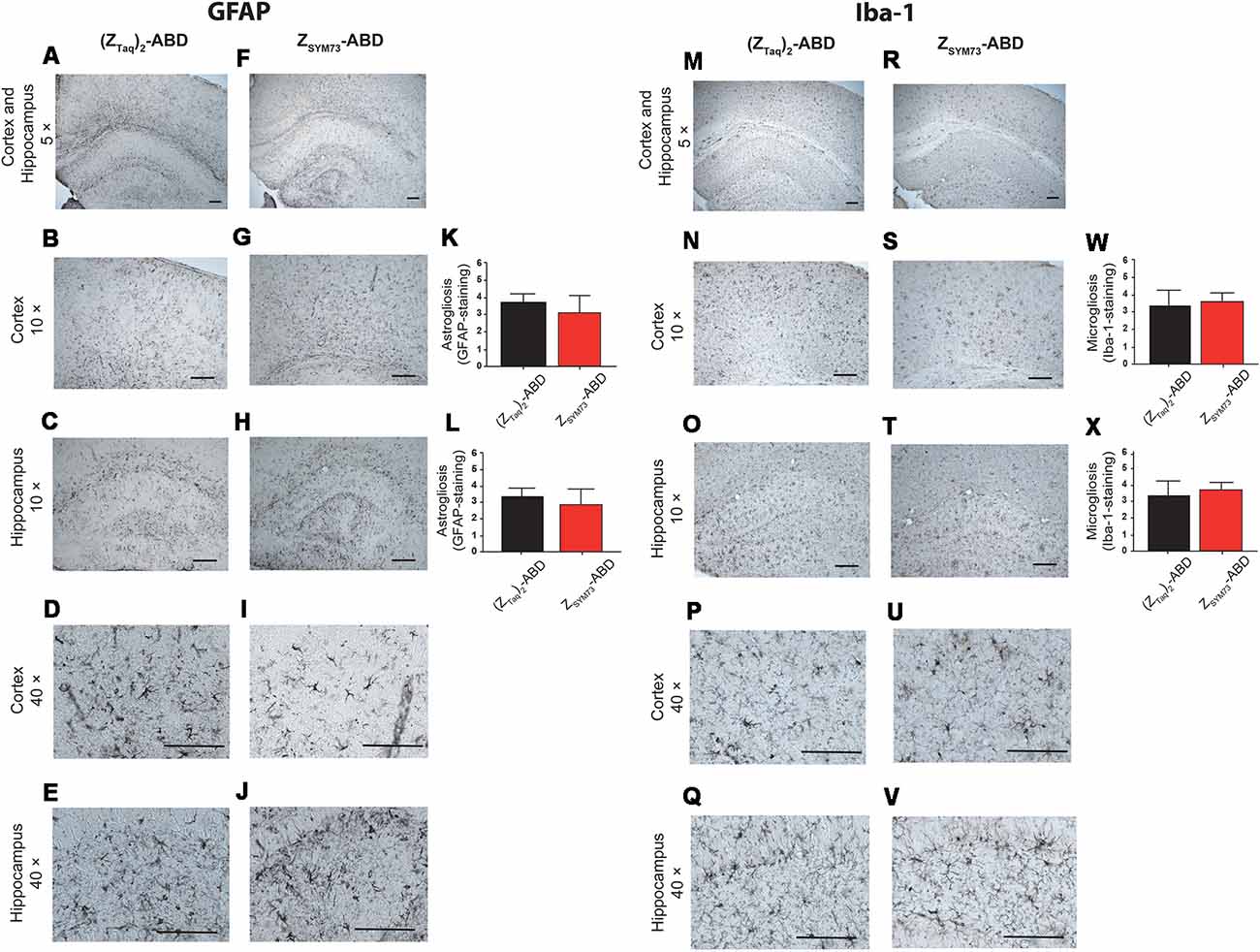
Figure 5. Analysis of glial fibrillary acidic protein (GFAP) and Iba-1 immunoreactivity post administration. Histological observations and semiquantitative rating of astrocyte activation by GFAP (A,F) and microglial cell activation by Iba-1 (M,R) reactivity on brain sections from ZSYM73-ABD (red) or (ZTaq)2-ABD (black) control-treated mice, at 5× magnification. GFAP-reactivity on cortical (B,G,K) and hippocampal (C,H,L) sections (p = 0.11 and p = 0.06, respectively); one-tailed t-test at 10× magnification, and 40× magnification (D–J), respectively. Iba-1 microglia cell reactivity on brain sections at 5× magnification. Iba-1 reactivity on cortical (p = 0.25, one-tailed t-test; N,S,W) and hippocampal (p = 0.15, one-tailed t-test; O,T,X) sections at 10× magnification, and at 40x magnifications (P–V), respectively. Scale bar (100 μm).
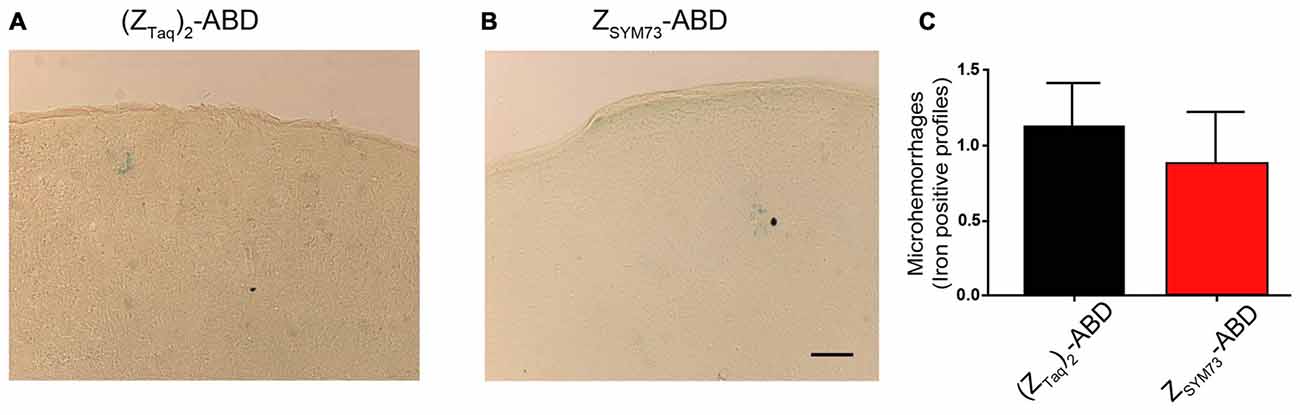
Figure 6. Semi-quantitative analysis of cerebral microhemorrhages. Representative brain sections demonstrating the degree of cerebral microhemorrhages in from (ZTaq)2-ABD (A) control or ZSYM73-ABD (B) treated mice by staining with Perl’s stain for ferric iron in hemosiderin. Semi-quantitation of iron positive profiles is shown in (C) (p = 0.29, one-tailed t-test). Scale bar (50 μm).
CSF Bioavailability in Naïve Rats
The potential site of action for affibody-mediated Aβ-sequestering was next investigated in a small pilot study. The uptake of ZSYM73-ABD to the CSF after administration was quantified in naïve rats, as CSF is difficult to extract from mice. Briefly, rats were administered with molar equivalents of either ZSYM73-ABD or an Aβ-binding mAb (U-Protein Express, Utrecht, Netherlands) as a control. CSF and plasma samples were extracted 0, 3, 24, and 120 h after the treatment, and the concentration measured using ELISA. The bioavailability in the CSF was determined to 0.13% and 0.12% for ZSYM73-ABD and the Aβ-binding mAb, respectively (Supplementary Figure S4), indicating that only a small fraction of the affibody molecule passes into the CSF. Based on this, it can be speculated that the preventive effect that was observed in mice was partly due to the sequestering of Aβ in blood as a complement to acting within the brain. However, further investigations are needed to determine the mode and site of action in detail.
Discussion
We present a novel approach to prevent the development of Aβ-related pathology, using the Aβ-sequestering affibody molecule ZSYM73. The affibody molecule was previously engineered to sequester the aggregation-prone residues of monomeric Aβ by encapsulating the peptide in a tunnel-like cavity and thus preventing the peptide from forming neurotoxic aggregates.
To test the therapeutic potential of the ZSYM73 in vivo, the therapeutic candidate ZSYM73-ABD was first produced and characterized in terms of Aβ-binding activity and affinity in solution. In solution, the affibody molecule bound Aβ peptides with a 60 pM affinity while simultaneously binding to HSA via the ABD (also with picomolar affinity; Lindberg et al., 2015).
We show that ZSYM73-ABD can prevent the development and progression of Aβ pathology in APP/PS1 double transgenic mice with an early onset of pathology. Following three i.p. injections of 100 μg ZSYM73-ABD per week for 13 weeks, the treated animals exhibited significant cognitive rescue compared to mice treated with a size-matched (ZTaq)2-ABD control protein. The improved cognitive function was associated with a marked reduction in Aβ pathology. In addition, no affibody-specific antibody responses or immune-related side-effects were observed following the repeated administrations of the affibody protein. It has been speculated that Fc-activated immune cells can cause local inflammatory responses in the form of microhemorrhages (Pfeifer M. et al., 2002). However, affibody molecules lack the Fc domain of mAbs, and no such inflammatory responses were observed after the ZSYM73-ABD treatment.
It has been speculated that the small size of ZSYM73 (approximately 10-fold smaller than IgG) could possibly result in more efficient uptake in CSF compared to antibodies upon peripheral administration. To assess this, we investigated the CSF-uptake upon i.v. administration of ZSYM73-ABD or an Aβ-binding mAb in naïve rats. The bioavailability in the CSF was determined to 0.13% for ZSYM73-ABD and 0.12% for the Aβ-binding mAb, thus indicating a similar brain-uptake for both proteins. These results suggest that the size difference of the proteins had no dramatic influence on the passage over BBB, which leads us to further speculate whether the observed therapeutic effect in mice is a consequence of Aβ sequestration within the brain or through a peripheral sink effect (DeMattos et al., 2001; Citron, 2010). An important advantage for a significantly smaller protein drug is that a much higher molar dose can be administered in the same volume, which potentially could allow for subcutaneous injections and thus omit the demand for administration at an infusion center. Further studies are needed to elucidate the site of action. In future studies, it would be interesting to explore if antibody-based transferrin receptor-mediated transcytosis (Hultqvist et al., 2017) could improve BBB transfer of ZSYM73-ABD, and thus make it even more efficient in preventing the development of AD. mAbs to proteins such as α-synuclein and tau that are associated with other neurodegenerative diseases are also showing promise therapeutically (Herline et al., 2018a; Jankovic et al., 2018). Our affibody approach can also be designed to target these other pathology-associated proteins, with the same advantages as discussed above. Hence, the findings discussed here, have potential applicability to multiple neurodegenerative disorders.
To our knowledge, this is the first in vivo investigation of a systemically delivered scaffold protein targeting monomeric Aβ, which demonstrates a therapeutic potential for treatment of AD. With the recent set backs in clinical trials using Aβ-targeting mAbs, the path forward is indeed challenging (Herline et al., 2018b; Schott et al., 2019). However, this study supports further evaluations on non-immunoglobulin-based biopharmaceutical candidates for AD to assess differences and opportunities in the mode of action, biodistribution, toxicity profile et cetera compared to antibodies. Moreover, given that AD patients would most likely require long preventive treatment regimens for a relatively large part of the population, the small size of affibody molecules should be important, allowing for subcutaneous injections without assistance from an intravenous infusion center.
Data Availability
All datasets generated for this study are included in the manuscript and/or the Supplementary Files.
Author Contributions
AB: overall responsibility for the mouse studies, including breeding of mice, injections, behavioral tasks, histological analysis and plasma studies, involved in planning of the study, evaluation of the results, writing the manuscript. HL: generation of the ZSYM73-ABD and the control protein, production, purification and characterization, took part in the mouse studies performed at NYU, involved in evaluating the results, writing the manuscript. AA: performed a majority of the brain processing and some of the quantification of the histology. IA: performed the brain processing and some of the quantification of the histology. AP: assisted in the mice studies by quantitation of the findings on brain sections. RB: assisted in the mice studies and preparing reagents. IH-G: performed the CSF bioavailability study. EG: responsible for the KinExA study, writing part of the manuscript. FF: as an expert in the affibody technology, overall responsibility for the production and characterization of the affibody-fusion proteins. TH: as an expert in protein structures, responsibility for drug design and structural considerations. JL: as an expert in protein engineering, the design of the candidate drug, evaluation of the results, writing the manuscript. SS: involved in planning of the study, evaluation of the results, writing the manuscript. TW: overall planning of the study, evaluation of the results, writing the manuscript.
Funding
This work was supported by NIH grants NS073502 and AG008051, the Saudi Arabia Cultural Mission, the Wallenberg Center for Protein Technology, the Swedish FTD Initiative and the Schörling foundation, and the Swedish Brain Foundation (FO2017-0121; FO2018-0094).
Conflict of Interest Statement
IH-G, EG, and FF are employees at Affibody AB. JL and SS are members of the technical advisory board at Affibody AB.
The remaining authors declare that the research was conducted in the absence of any commercial or financial relationships that could be construed as a potential conflict of interest.
Acknowledgments
Caroline Ekblad, Karin Dillner Bergstedt, Per Jonasson, Finn Dunås, Elisabet Wahlberg, Lindvi Gudmundsdotter and Jenny Fohlstedt (Affibody AB, Solna, Sweden) are acknowledged for assistance in protein production and valuable advice.
Supplementary Material
The Supplementary Material for this article can be found online at: https://www.frontiersin.org/articles/10.3389/fnagi.2019.00064/full#supplementary-material
References
Andersen, J. T., Pehrson, R., Tolmachev, V., Daba, M. B., Abrahmsén, L., and Ekblad, C. (2011). Extending half-life by indirect targeting of the neonatal Fc receptor (FcRn) using a minimal albumin binding domain. J. Biol. Chem. 286, 5234–5241. doi: 10.1074/jbc.M110.164848
Bard, F., Cannon, C., Barbour, R., Burke, R. L., Games, D., Grajeda, H., et al. (2000). Peripherally administered antibodies against amyloid β-peptide enter the central nervous system and reduce pathology in a mouse model of Alzheimer disease. Nat. Med. 6, 916–919. doi: 10.1038/78682
Bass, T. Z., Rosestedt, M., Mitran, B., Frejd, F. Y., Löfblom, J., Tolmachev, V., et al. (2017). In vivo evaluation of a novel format of a bivalent HER3-targeting and albumin-binding therapeutic affibody construct. Sci. Rep. 7:43118. doi: 10.1038/srep43118
Boutajangout, A., Noorwali, A., Atta, H., and Wisniewski, T. (2017). Human umbilical cord stem cell xenografts improve cognitive decline and reduce the amyloid burden in a mouse model of Alzheimer’s disease. Curr. Alzheimer Res. 14, 104–111. doi: 10.2174/1567205013666161004151416
Boutajangout, A., Goni, F., Knudsen, E., Schreiber, F., Asuni, A., Quartermain, D., et al. (2009). Diminished amyloid-beta burden in Tg2576 mice following a prophylactic oral immunization with a salmonella-based amyloid-beta derivative vaccine. J. Alzheimers Dis. 18, 961–972. doi: 10.3233/jad-2009-1204
Citron, M. (2010). Alzheimer’s disease: strategies for disease modification. Nat. Rev. Drug Discov. 9, 387–398. doi: 10.1038/nrd2896
De Genst, E., and Muyldermans, S. (2015). Development of a high affinity affibody-derived protein against amyloid β-peptide for future Alzheimer’s disease therapy. Biotechnol. J. 10, 1668–1669. doi: 10.1002/biot.201500405
DeMattos, R. B., Bales, K. R., Cummins, D. J., Dodart, J. C., Paul, S. M., and Holtzman, D. M. (2001). Peripheral anti-A beta antibody alters CNS and plasma A beta clearance and decreases brain A beta burden in a mouse model of Alzheimer’s disease. Proc. Natl. Acad. Sci. U S A 98, 8850–8855. doi: 10.1073/pnas.151261398
Doody, R. S., Thomas, R. G., Farlow, M., Iwatsubo, T., Vellas, B., Joffe, S., et al. (2014). Phase 3 trials of solanezumab for mild-to-moderate Alzheimer’s disease. N. Engl. J. Med. 370, 311–321. doi: 10.1056/NEJMoa1312889
Drummond, E., and Wisniewski, T. (2017). Alzheimer’s disease: experimental models and reality. Acta Neuropathol. 133, 155–175. doi: 10.1007/s00401-016-1662-x
Frejd, F. Y. (2012). “Half-Life extension by binding to albumin through an albumin binding domain,” in Therapeutic Proteins: Strategies to Modulate Their Plasma Half-Lives, ed. R. Kontermann, Weinheim: Wiley-VCH Verlag GmbH and Co. KGaA
Goñi, F., Martá-Ariza, M., Herline, K., Peyser, D., Boutajangout, A., Mehta, P., et al. (2018). Anti-β-sheet conformation monoclonal antibody reduces tau and Aβ oligomer pathology in an Alzheimer’s disease model. Alzheimers Res. Ther. 10:10. doi: 10.1186/s13195-018-0337-3
Goñi, F., Herline, K., Peyser, D., Wong, K., Ji, Y., Sun, Y., et al. (2013). Immunomodulation targeting of both Aβ and tau pathological conformers ameliorates Alzheimer’s disease pathology in TgSwDI and 3xTg mouse models. J. Neuroinflammation 10:150. doi: 10.1186/1742-2094-10-150
Grönwall, C., Jonsson, A., Lindström, S., Gunneriusson, E., Ståhl, S., and Herne, N. (2007). Selection and characterization of affibody ligands binding to Alzheimer amyloid beta peptides. J. Biotechnol. 128, 162–183. doi: 10.1016/j.jbiotec.2006.09.013
Gunneriusson, E., Nord, K., Uhlén, M., and Nygren, P. (1999). Affinity maturation of a Taq DNA polymerase specific affibody by helix shuffling. Protein Eng. 12, 873–878. doi: 10.1093/protein/12.10.873
Hardy, J., and Selkoe, D. J. (2002). The amyloid hypothesis of Alzheimer’s disease: progress and problems on the road to therapeutics. Science 297, 353–356. doi: 10.1126/science.1072994
Herline, K., Drummond, E., and Wisniewski, T. (2018a). Recent advancements toward therapeutic vaccines against Alzheimer’s disease. Expert Rev. Vaccines 17, 707–721. doi: 10.1080/14760584.2018.1500905
Herline, K., Prelli, F., Mehta, P., MacMurray, C., Goñi, F., and Wisniewski, T. (2018b). Immunotherapy to improve cognitive and reduce pathological species in an Alzheimer’s Disease mouse model. Alzheimers Res. Ther. 10:54. doi: 10.1186/s13195-018-0384-9
Holcomb, L., Gordon, M. N., McGowan, E., Yu, X., Benkovic, S., Jantzen, P., et al. (1998). Accelerated Alzheimer-type phenotype in transgenic mice carrying both mutant amyloid precursor protein and presenilin 1 transgenes. Nat. Med. 4, 97–100. doi: 10.1038/nm0198-097
Hoyer, W., and Härd, T. (2008). Interaction of Alzheimer’s A beta peptide with an engineered binding protein–thermodynamics and kinetics of coupled folding-binding. J. Mol. Biol. 378, 398–411. doi: 10.1016/j.jmb.2008.02.040
Hoyer, W., Grönwall, C., Jonsson, A., Ståhl, S., and Härd, T. (2008). Stabilization of a beta-hairpin in monomeric Alzheimer’s amyloid-beta peptide inhibits amyloid formation. Proc. Natl. Acad. Sci. U S A 105, 5099–5104. doi: 10.1073/pnas.0711731105
Hultqvist, G., Syvänen, S., Fang, X. T., Lannfelt, L., and Sehlin, D. (2017). Bivalent brain shuttle increases antibody uptake by monovalent binding to the transferrin receptor. Theranostics 7, 308–318. doi: 10.7150/thno.17155
Jankovic, J., Goodman, I., Safirstein, B., Marmon, T. K., Schenk, D. B., Koller, M., et al. (2018). Safety and tolerability of multiple ascending doses of PRX002/RG7935, an anti-α-synuclein monoclonal antibody, in patients with parkinson disease: a randomized clinical trial. JAMA Neurol. 75, 1206–1214. doi: 10.1001/jamaneurol.2018.1487
Jonsson, A., Dogan, J., Herne, N., Abrahmsén, L., and Nygren, P. A. (2008). Engineering of a femtomolar affinity binding protein to human serum albumin. Protein Eng. Des. Sel. 21, 515–527. doi: 10.1093/protein/gzn028
Karran, E., Mercken, M., and De Strooper, B. (2011). The amyloid cascade hypothesis for Alzheimer’s disease: an appraisal for the development of therapeutics. Nat. Rev. Drug Discov. 10, 698–712. doi: 10.1038/nrd3505
Lindberg, H., Härd, T., Löfblom, L., and Ståhl, S. (2015). A truncated and dimeric format of an affibody library on bacteria enables FACS-mediated isolation of amyloid-beta aggregation inhibitors with subnanomolar affinity. Biotechnol. J. 10, 1707–1718. doi: 10.1002/biot.201500131
Liu, S., Park, S., Allington, G., Prelli, F., Sun, Y., Martá-Ariza, M., et al. (2017). Targeting apolipoprotein E/Amyloid β binding by peptoid CPO_Aβ17–21 P ameliorates Alzheimer’s disease related pathology and cognitive decline. Sci. Rep. 7:8009. doi: 10.1038/s41598-017-08604-8
Liu, S., Breitbart, A., Sun, Y., Mehta, P. D., Boutajangout, A., Scholtzova, H., et al. (2014). Blocking the apolipoprotein E/amyloid β interaction in triple transgenic mice ameliorates Alzheimer’s disease related amyloid β and tau pathology. J. Neurochem. 128, 577–591. doi: 10.1111/jnc.12484
Löfblom, J., Feldwisch, J., Tolmachev, V., Carlsson, J., Ståhl, S., and Frejd, F. Y. (2010). Affibody molecules: engineered proteins for therapeutic, diagnostic and biotechnological applications. FEBS Lett. 584, 2670–2680. doi: 10.1016/j.febslet.2010.04.014
Luheshi, L. M., Hoyer, W., de Barros, T. P., van Dijk Härd, I., Brorsson, A. C., Macao, B., et al. (2010). Sequestration of the Abeta peptide prevents toxicity and promotes degradation in vivo. PLoS Biol. 8:e1000334. doi: 10.1371/journal.pbio.1000334
Malm, M., Kronqvist, N., Lindberg, H., Gudmundsdotter, L., Bass, T., Frejd, F. Y., et al. (2013). Inhibiting HER3-mediated tumor cell growth with affibody molecules engineered to low picomolar affinity by position-directed error-prone PCR-like diversification. PLoS One. 8:e62791. doi: 10.1371/journal.pone.0062791
Nelson, P. T., Alafuzoff, I., Bigio, E. H., Bouras, C., Braak, H., Cairns, N. J., et al. (2012). Correlation of Alzheimer disease neuropathologic changes with cognitive status: a review of the literature. J. Neuropathol. Exp. Neurol. 71, 362–381. doi: 10.1097/NEN.0b013e31825018f7
Nisbet, R. M., Nigro, J., Breheney, K., Caine, J., Hattarki, M. K., and Nuttall, S. D. (2013). Central amyloid-β-specific single chain variable fragment ameliorates Aβ aggregation and neurotoxicity. Protein Eng. Des. Sel. 26, 571–580. doi: 10.1093/protein/gzt025
Pfeifer, M., Boncristiano, S., Bondolfi, L., Stalder, A., Deller, T., Staufenbiel, M., et al. (2002). Cerebral hemorrhage after passive anti-Abeta immunotherapy. Science 298:1379. doi: 10.1126/science.1078259
Pfeifer, L. A., White, L. R., Ross, G. W., Petrovitch, H., and Launer, L. J. (2002). Cerebral amyloid angiopathy and cognitive function: the HAAS autopsy study. Neurology 58, 1629–1634. doi: 10.1212/wnl.58.11.1629
Pul, R., Dodel, R., and Stangel, M. (2011). Antibody-based therapy in Alzheimer’s disease. Expert Opin. Biol. Ther. 11, 343–357. doi: 10.1517/14712598.2011.552884
Puzzo, D., Gulisano, W., Palmeri, A., and Arancio, O. (2015). Rodent models for Alzheimer’s disease drug discovery. Expert Opin. Drug Discov. 10, 703–711. doi: 10.1517/17460441.2015.1041913
Racke, M. M., Boone, L. I., Hepburn, D. L., Parsadanian, M., Bryan, M. T., Ness, D. K., et al. (2005). Exacerbation of cerebral amyloid angiopathy-associated microhemorrhages in amyloid precursor protein transgenic mice by immunotherapy is dependent on antibody recognition of deposited forms of amyloid β. J. Neurosci 25, 629–636. doi: 10.1523/jneurosci.4337-04.2005
Robert, R., and Wark, K. L. (2012). Engineered antibody approaches for Alzheimer’s disease immunotherapy. Arch. Biochem. Biophys. 526, 132–138. doi: 10.1016/j.abb.2012.02.022
Salloway, S., Sperling, R., Fox, N. C., Blennow, K., Klunk, W., Raskind, M., et al. (2014). Two phase 3 trials of bapineuzumab in mild-to-moderate Alzheimer’s disease. N. Engl. J. Med. 370, 322–333. doi: 10.1056/NEJMoa1304839
Scholtzova, H., Chianchiano, P., Pan, J., Sun, Y., Goñi, F., Mehta, P. D., et al. (2014). Amyloid β and Tau Alzheimer’s disease related pathology is reduced by Toll-like receptor 9 stimulation. Acta Neuropathol. Commun. 2:101. doi: 10.1186/s40478-014-0101-2
Scholtzova, H., Do, E., Dhakal, S., Sun, Y., Liu, S., Mehta, P. D., et al. (2017). Innate immunity stimulation via toll-like receptor 9 ameliorates vascular amyloid pathology in Tg-SwDI mice with associated cognitive benefits. J. Neurosci. 37, 936–959. doi: 10.1523/jneurosci.1967-16.2017
Scholtzova, H., Kascsak, R. J., Bates, K. A., Boutajangout, A., Kerr, D. J., Meeker, H. C., et al. (2009). Induction of toll-like receptor 9 signaling as a method for ameliorating Alzheimer’s disease-related pathology. J. Neurosci. 29, 1846–1854. doi: 10.1523/jneurosci.5715-08.2009
Schott, J. M., Aisen, P. S., Cummings, J. L., Howard, R. J., and Fox, N. C. (2019). Unsuccessful trials of therapies for Alzheimer’s disease. Lancet 393:29. doi: 10.1016/S0140-6736(18)31896-8
Sevigny, J., Chiao, P., Bussière, T., Weinreb, P. H., Williams, L., Maier, M., et al. (2016). The antibody aducanumab reduces Aβ plaques in Alzheimer’s disease. Nature 537, 50–56. doi: 10.1038/nature19323
Siemers, E. R., Sundell, K. L., Carlson, C., Case, M., Sethuraman, G., Liu-Seifert, H., et al. (2016). Phase 3 solanezumab trials: Secondary outcomes in mild Alzheimer’s disease patients. Alzheimers Dement. 12, 110–120. doi: 10.1016/j.jalz.2015.06.1893
Sörensen, J., Sandberg, D., Sandström, M., Wennborg, A., Feldwisch, J., Tolmachev, V., et al. (2014). First-in-human molecular imaging of HER2 expression in breast cancer metastases using the 111In-ABY-025 affibody molecule. J. Nucl. Med. 55, 730–735. doi: 10.2967/jnumed.113.131243
Sörensen, J., Velikyan, I., Sandberg, D., Wennborg, A., Feldwisch, J., Tolmachev, V., et al. (2016). Measuring HER2-receptor expression in metastatic breast cancer using [68Ga]ABY-025 affibody PET/CT. Theranostics 6, 262–271. doi: 10.7150/thno.13502
Ståhl, S., Gräslund, T., Eriksson Karlström, A., Frejd, F. Y., Nygren, P. A., and Löfblom, J. (2017). Affibody molecules in biotechnological and medical applications. Trends Biotechnol. 35, 691–712. doi: 10.1016/j.tibtech.2017.04.007
Tolmachev, V., Orlova, A., Pehrson, R., Galli, J., Baastrup, B., Andersson, K., et al. (2007). Radionuclide therapy of HER2-positive microxenografts using a 177Lu-labeled HER2-specific affibody molecule. Cancer Res. 67, 2773–2782. doi: 10.1158/0008-5472.can-06-1630
van Dyck, C. H. (2018). Anti-Amyloid-β monoclonal antibodies for Alzheimer’s disease: pitfalls and promise. Biol. Psychiatry 83, 311–319. doi: 10.1016/j.biopsych.2017.08.010
Vazquez-Lombardi, R., Phan, T. G., Zimmermann, C., Lowe, D., Jermutus, L., and Christ, D. (2015). Challenges and opportunities for non-antibody scaffold drugs. Drug Discov. Today 20, 1271–1283. doi: 10.1016/j.drudis.2015.09.004
Wilcock, D. M., Rojiani, A., Rosenthal, A., Levkowitz, G., Subbarao, S., Alamed, J., et al. (2004). Passive amyloid immunotherapy clears amyloid and transiently activates microglia in a transgenic mouse model of amyloid deposition. J. Neurosci. 24, 6144–6151. doi: 10.1523/jneurosci.1090-04.2004
Keywords: Alzheimer’s disease, affibody molecule, amyloid beta (Aβ), behavior, histology, immunotherapy, transgenic mice
Citation: Boutajangout A, Lindberg H, Awwad A, Paul A, Baitalmal R, Almokyad I, Höidén-Guthenberg I, Gunneriusson E, Frejd FY, Härd T, Löfblom J, Ståhl S and Wisniewski T (2019) Affibody-Mediated Sequestration of Amyloid β Demonstrates Preventive Efficacy in a Transgenic Alzheimer’s Disease Mouse Model. Front. Aging Neurosci. 11:64. doi: 10.3389/fnagi.2019.00064
Received: 25 October 2018; Accepted: 06 March 2019;
Published: 22 March 2019.
Edited by:
Robert Petersen, College of Medicine, Central Michigan University, United StatesReviewed by:
P. Hemachandra Reddy, Texas Tech University Health Sciences Center, United StatesResham Chhabra, School of Medicine, Johns Hopkins University, United States
Copyright © 2019 Boutajangout, Lindberg, Awwad, Paul, Baitalmal, Almokyad, Höidén-Guthenberg, Gunneriusson, Frejd, Härd, Löfblom, Ståhl and Wisniewski. This is an open-access article distributed under the terms of the Creative Commons Attribution License (CC BY). The use, distribution or reproduction in other forums is permitted, provided the original author(s) and the copyright owner(s) are credited and that the original publication in this journal is cited, in accordance with accepted academic practice. No use, distribution or reproduction is permitted which does not comply with these terms.
*Correspondence: Stefan Ståhl, c3N0YUBrdGguc2U=
Thomas Wisniewski, dGhvbWFzLndpc25pZXdza2lAbnl1bGFuZ29uZS5vcmc=
† These authors have contributed equally to this work