- 1Department of Biomedical Sciences, College of Veterinary Medicine, Iowa State University, Ames, IA, United States
- 2Department of Biomedical Engineering, University of Texas at Austin, Austin, TX, United States
- 3Department of Food Science and Human Nutrition, College of Agriculture and Life Sciences, Iowa State University, Ames, IA, United States
- 4Department of Veterinary Clinical Sciences, Iowa State University, Ames, IA, United States
Identifying appropriate animal models is critical in developing translatable in vitro and in vivo systems for therapeutic drug development and investigating disease pathophysiology. These animal models should have direct biological and translational relevance to the underlying disease they are supposed to mimic. Aging dogs not only naturally develop a cognitive decline in many aspects including learning and memory deficits, but they also exhibit human-like individual variability in the aging process. Neurodegenerative processes that can be observed in both human and canine brains include the progressive accumulation of β-amyloid (Aβ) found as diffuse plaques in the prefrontal cortex (PFC), including the gyrus proreus (i.e., medial orbital PFC), as well as the hippocampus and the cerebral vasculature. Tau pathology, a marker of neurodegeneration and dementia progression, was also found in canine hippocampal synapses. Various epidemiological data show that human patients with neurodegenerative diseases have concurrent intestinal lesions, and histopathological changes in the gastrointestinal (GI) tract occurs decades before neurodegenerative changes. Gut microbiome alterations have also been reported in many neurodegenerative diseases including Alzheimer’s (AD) and Parkinson’s diseases, as well as inflammatory central nervous system (CNS) diseases. Interestingly, the dog gut microbiome more closely resembles human gut microbiome in composition and functional overlap compared to rodent models. This article reviews the physiology of the gut-brain axis (GBA) and its involvement with neurodegenerative diseases in humans. Additionally, we outline the advantages and weaknesses of current in vitro and in vivo models and discuss future research directions investigating major human neurodegenerative diseases such as AD and Parkinson’s diseases using dogs.
Introduction
The gut-brain axis (GBA) is a highly complex interactive network between the gut and the brain, composed of endocrinological, immunological and neural mediators, as summarized in Figure 1 (Rhee et al., 2009). The GBA is largely mediated by the central nervous system (CNS), the enteric nervous system (ENS), and the intestinal microbiota (Grenham et al., 2011). The extrinsic nerves of the gastrointestinal (GI) tract connect the gut to the brain through vagal and spinal afferent fibers, while the brain sends efferent sympathetic and parasympathetic fibers to the GI tract (Grenham et al., 2011; Browning and Travagli, 2014; Foster et al., 2017). The hypothalamic pituitary adrenal (HPA)-axis is known as the main modulator of the physiological stress response but it also modulates alimentary function during digestion (Tsigos and Chrousos, 2002) to facilitate gluconeogenesis. The hypothalamus releases corticotrophin-releasing factor (CRF) and different proteins within this family (e.g., CRF, urocortin 1–3) are also known to affect GI tract function, i.e., intestinal motility (Kihara et al., 2001), permeability (Zheng et al., 2013), and inflammation (Dinan et al., 2006). Specifically, changes in the GI motility induced by urocortin administration were noted in conscious rats, and this study also suggested that the vagal pathway could regulate the central action of urocortin (Kihara et al., 2001). Rats experiencing psychological stress showed decreased level of intestinal epithelial tight junction (TJ) proteins concurrent with increased intestinal permeability in the colon (Zheng et al., 2013). In addition, among patients with irritable bowel syndrome (IBS), the levels of proinflammatory cytokines including interleukin (IL)-6 and IL-8 were elevated as a result of adrenocorticotropic hormone (ACTH) stimulation (i.e., cortisol release; Dinan et al., 2006).
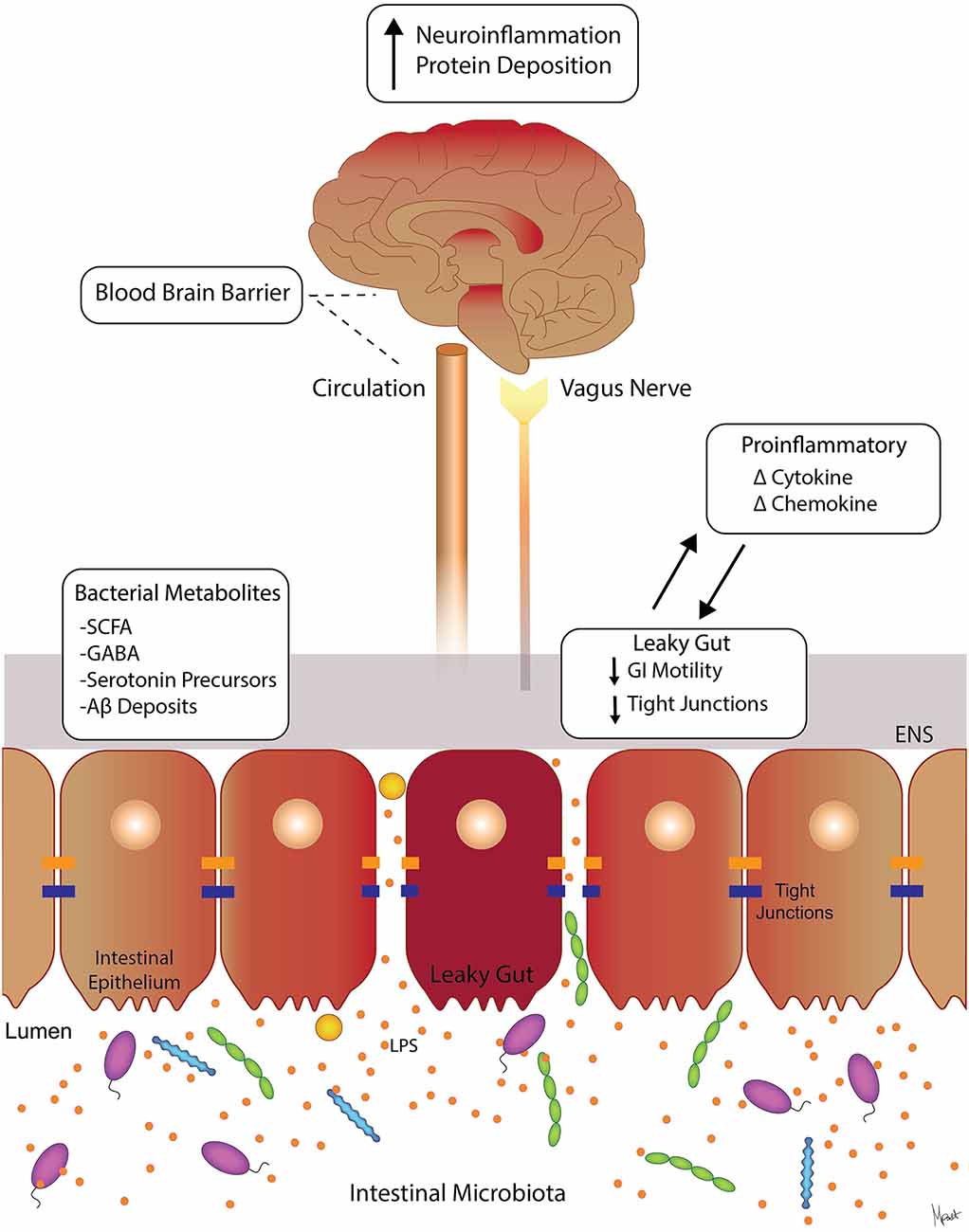
Figure 1. Molecular pathways involved in gut-brain axis (GBA). Suggested signaling pathways and cross-talk between the intestinal microbiota, the intestinal barrier, immune modulators, and neural (brain, vagus, and ENS) systems. The intestinal microbiota can affect the levels of circulating local cytokines, cause “leaky gut” with increase GI permeability, and ultimately affect brain function (Moloney et al., 2014; Carabotti et al., 2015). Intestinal bacterial metabolites such as SCFA, GABA, and serotonin precursors are neuroactive and can affect ENS and the brain (Grider and Piland, 2007; Kimura et al., 2011). Abbreviations: ENS, enteric nervous system; SCFAs, short-chain fatty acids; GABA, γ-aminobutyric acid; LPS, lipopolysaccharide; GI, gastrointestinal.
Various studies suggest that intestinal health has a significant impact on neurodegeneration despite the anatomical distance between the gut and the brain (Houser and Tansey, 2017; Zhang et al., 2018). Specifically, dysregulation of GBA cross-talk has been associated with metabolic syndrome (de Lartigue et al., 2011; Grasset et al., 2017) psychiatric disorders such as depression, anxiety, autism, as well as neurodegenerative diseases such as Parkinson’s disease (PD), and Alzheimer’s disease (AD; Sampson et al., 2016; Zhang et al., 2018). In reverse, these neurologic disorders are often times linked to altered intestinal health characterized by changes in the intestinal microbiota composition, which may disrupt the interplay between the gut and the brain (Esteve et al., 2011; O’Mahony et al., 2011). Many studies suggest that the intestinal microbiota contributes not only to modulating the communication and function of the GBA but also to modulating immune response through stimulation of cytokines and chemokines (Moloney et al., 2014). Similarly, the GBA interacts with intestinal cells and the ENS, as well as the CNS through neuroendocrine and metabolic pathways (Carabotti et al., 2015). Furthermore, ENS function can be influenced by the gut microbiota when they locally produce neurotransmitters, including γ-aminobutyric acid (GABA), amino-acid derivatives (e.g., serotonin, melatonin, and histamine) and fatty-acid derivatives (e.g., acetylcholine; Iyer et al., 2004) or biologically active catecholamines (i.e., dopamine and norepinephrine) in the gut lumen (Asano et al., 2012). The ENS is also targeted by bacterial metabolites such as short-chain fatty acids (SCFAs), including acetic acid, butyric acid, and propionic acid, which stimulate the sympathetic nervous system (Grider and Piland, 2007; Kimura et al., 2011), with downstream effects on learning and memory (Vecsey et al., 2007; Stefanko et al., 2009).
GBA in Neurodegenerative Diseases
Dysfunction of the GBA has been associated with psychiatric disorders including depression and anxiety, as well as neurodegenerative disorders including PD and AD (Sampson et al., 2016; Jiang et al., 2017). The following section will focus on recent findings of GBA involvement in PD and AD and their clinical features, as summarized in Figure 2.
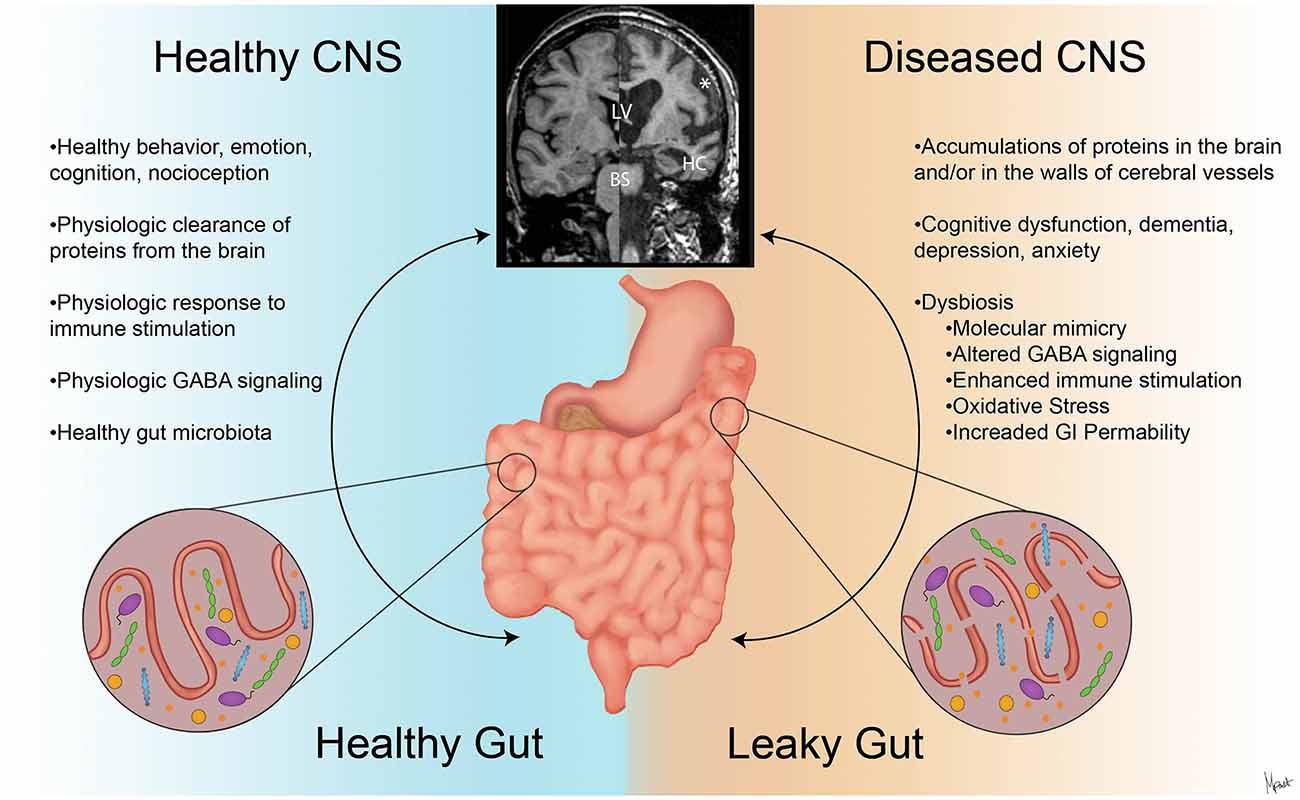
Figure 2. The contrasts of clinical presentations on the GBA in health and neurodegenerative diseases. A stable intestinal microbiota is essential for healthy gut physiology and contributes to appropriate signaling along the GBA, promoting healthy physiologic status as well as central nervous system (CNS) status (left). Intestinal dysbiosis can negatively influence gut physiology and lead to abnormal GBA signaling (Friedland, 2015), resulting in accumulation of misfolded amyloid species (Galloway et al., 2007; de Lartigue et al., 2011). This can ultimately alter CNS functions and anatomy (Wu et al., 2017) as shown with magnetic resonance imaging (MRI) volumetric scans (upper middle). In the diseased CNS and gut state (right), cortical atrophy with the widening of the subarachnoid space (*), enlargement of the lateral ventricles (LV), hippocampus atrophy (HC), and brainstem (BS) volume reduction are seen with clinical and cognitive dysfunction (Johnson et al., 2012; Lee et al., 2015).
Alzheimer’s Disease
AD is a progressive neurodegenerative disease characterized by senile plaques consisting of misfolded β-amyloid (Aβ) fibrils and oligomers (Iadanza et al., 2018), as well as hyperphosphorylated tau protein in the various regions of the brain including cerebral cortex, locus coeruleus, and hippocampus (Llorens et al., 2017). Although such protein aggregation in the brain as well as non-neural tissues (i.e., the blood vessels, skin, subcutaneous tissue, and intestine) is a histological feature of AD, such deposition could simply be a consequence of various (epi) genetic alterations triggered by environmental exposures such as sociological, or medical nutritional stress (Lemche, 2018). In fact, synthetic Aß42 peptide aggregation has been reported in Caenorhabditis elegans aging models (Patel et al., 2017). Aβ fibrillar accumulation can coincide with clinical signs of cognitive dysfunction (Attems et al., 2005; Herzig et al., 2006; Pistollato et al., 2016), however, it is noteworthy that there is a high degree of variation in the extent of Aβ accumulation among patients with cognitive decline (Monsell et al., 2015). Although almost 100 years have passed since the very first diagnosis of AD, the exact pathogenesis of the disease is still largely unknown (Iadanza et al., 2018). Likewise, no effective therapy for modulation of AD is currently available.
One hypothesis for the involvement of the GBA in the pathophysiology of neurodegenerative diseases is microbial dysbiosis, which occurs as a result of antibiotic exposure (Vangay et al., 2015), dietary changes (Muegge et al., 2011), probiotics (Delzenne et al., 2011), or a variety of other disease conditions (Tilg and Moschen, 2014; Rosenfeld, 2015). Specifically, various studies have shown an association between gut microbiome dysbiosis and the aggregation of Aβ peptides in intestinal epithelial cells (Galloway et al., 2007, 2009) and the CNS (Nam et al., 2017; Lin et al., 2016) after high-fat diet feeding. Different components of the microbiota, such as bacteria, can excrete an immunogenic mixture of functional lipopolysaccharides (LPS), amyloid species, and exudates from their outer membranes into the local intestinal environment (Oli et al., 2012; Schwartz and Boles, 2013). Amyloid species and LPS are usually soluble, although they can polymerize and form insoluble fibrous protein aggregates, leading to stimulation of oxidative stress and cross-seeding of further protein aggregation (Morales et al., 2013; Friedland, 2015; Iadanza et al., 2018). For example, the endotoxin from Escherichia coli was shown to enhance the Aβ fibril formation in an in vitro model (Asti and Gioglio, 2014). Also, co-incubation of Aβ peptide with LPS was shown to potentiate amyloids fibrillogenesis (Asti and Gioglio, 2014), and systemic injection of LPS in a transgenic AD mouse model resulted in severe amyloid deposition and tau pathology (Aziz et al., 2013; Mitew et al., 2013; Paula-Lima et al., 2013; Saulnier et al., 2013). Moreover, recent studies suggest that the structural overlap between bacterial amyloid proteins to human Aβ could induce molecular mimicry, an immune response against the self-antigens stimulated by a foreign antigen sharing structural similarities, and ultimately causing greater inflammatory responses to cerebral Aβ due to altered gut microbiota (Delzenne et al., 2011; Muegge et al., 2011; Rosenfeld, 2015).
Another hypothesis for the pathogenesis of misfolded protein aggregation is the “Prion Concept.” This hypothesis states that many neurodegenerative diseases exhibit accumulation of fibrillary, misfolded proteins similar to the propagation of prionopathies in the CNS (Goedert, 2015). Prionopathy also involves the GBA and the local immune system, where prions accumulate in dendritic cells in the Peyer’s patches and other lymphoid follicles once entering the intestinal epithelium layer (Ano et al., 2009). Interestingly, earlier studies in a senescence-accelerated mouse model identified systemic senile amyloid proteins in Peyer’s patches (Yoshioka et al., 1990). By interacting with dendritic cells, the misfolded protein might be transported to the ENS, and ultimately spread to the CNS compartment (Ano et al., 2009). A significant amount of functional amyloid protein was shown to be generated by certain bacteria, such as E. coli, Bacillus subtilis, Salmonella enterica, Salmonella typhimurium, and Staphylococcus aureus, and may contribute to the pathology of AD through the accumulation of misfolded Aβ oligomers and fibrils (Hufnagel et al., 2013; Schwartz and Boles, 2013). Some bacterial species, such as Lactobacillus spp. and Bifidobacterium spp. (both gram-positive bacteria) are known to possess the ability to metabolize glutamate, a well-known primary excitatory neurotransmitter, to produce GABA, a well-known primary inhibitory neurotransmitter (Paula-Lima et al., 2013). These observations suggest that alteration of the gut microbiota can compromise the endogenous production of GABA (Saulnier et al., 2013). In turn, alteration of GABA signaling in the brain has been linked to cognitive impairment, AD, anxiety, and depression (Aziz et al., 2013; Hornig, 2013; Mitew et al., 2013; Paula-Lima et al., 2013). Alternatively, gut bacteria can affect peripheral nerve functions through the production of neuromodulatory metabolites such as short-chain fatty acid (SCFAs; Kimura et al., 2011). SCFAs, i.e., acetic acid, butyric acid, and propionic acid, are produced by bacterial fermentation of dietary fiber in the colon (Kimura et al., 2011). SCFAs can stimulate the sympathetic nervous system to release serotonin, ultimately influencing the CNS cognitive processes such as learning and memory (Grider and Piland, 2007). Catabolism of SCFAs to ketone bodies may also provide an alternative source of ATP to the brain, which could be beneficial given that progressive glucose dysmetabolism has been reported in patients with AD (Sokoloff, 1973). Importantly, lower levels of SCFAs have also been shown to negatively affect immune responses, epithelial cell growth, and possibly affect the function of both the central and peripheral nervous systems (Kimura et al., 2011; Bienenstock et al., 2015).
Parkinson’s Disease
Patients with PD present with classic motor symptoms, such as asymmetric resting tremor, that are caused by progressive dopaminergic neuronal death in the substantia nigra pars compacta and loss of dopaminergic signaling (Houser and Tansey, 2017). The pathophysiology of neurodegeneration in PD has not been established. However, abundant evidence suggests that neuroinflammation and glial cell activation could play a significant role in PD etiopathogenesis (Rocha et al., 2015). Proinflammatory signaling molecules, including cytokines (i.e., IL-1β, IL-6, and TNF-α; Mogi et al., 1996) or enzymes [i.e., nitric oxide synthase (NOS) and cyclooxygenase-2 (COX-2); Prigione et al., 2009], and oxidative stress are considered major contributing factors to neurodegeneration and cell death in PD.
One of the leading hypotheses for the pathogenesis of PD is the abnormal accumulation of α-synuclein (αSYN; Wong and Krainc, 2017). This protein is present in various cell types in the body, and PD patients show increased expression of αSYN at presynaptic terminals of neurons and neurite projections (Wong and Krainc, 2017). This protein is highly soluble and regulates the presynaptic release of important neurotransmitters such as dopamine (Wong and Krainc, 2017). The αSYN protein is also expressed within the ENS and can be detected in intestinal submucosal neuronal structures from neurologically healthy individuals (Böttner et al., 2012; Shannon et al., 2012; Gold et al., 2013). However, through interactions with environmental factors and other proteins and small molecules (Hasegawa et al., 2002; Breydo et al., 2012), αSYN follows a β-sheet structure formation and loses its physiologic membrane-binding capacity, leading to the aggregation of misfolded proteins forming so-called Lewy neurites and Lewy bodies in dopaminergic neurons of substantia nigra and noradrenergic neurons of the locus coeruleus (Hasegawa et al., 2002). Aggregates of misfolded αSYN proteins decrease mitochondrial complex I activity, thus reducing the physiologic functions of mitochondria, which ultimately leads to oxidative stress in the neuron (Jenner, 2003; Prigione et al., 2009). Individuals with mutations in the αSYN gene SNCA or duplication of the wild-type SNCA allele are known to develop early-onset, rapidly-progressive PD (Klein and Westenberger, 2012). The spread of αSYN proteins from the ENS to the CNS by transsynaptic cell-to-cell transmission in both sympathetic and parasympathetic nervous systems (Danzer et al., 2012) is the foundation for the “Prion Concept” in PD pathophysiology (Brundin et al., 2016). Multiple studies have demonstrated the presence of αSYN aggregates in intestinal biopsies from clinically normal individuals who would develop PD later in their lives (Braak et al., 2006; Shannon et al., 2012; Hilton et al., 2014). This finding indicates that intestinal αSYN precedes sufficient CNS neurodegeneration to produce motor dysfunctions (Houser and Tansey, 2017). Various clinical GI signs or the characteristic PD ENS pathology often occur before brain functions are actually affected, with constipation being the most common GI complaint in PD (Sakakibara et al., 2003). This is likely due to an increased intestinal transit time both in the small and large intestines of PD patients (Sakakibara et al., 2003). In fact, it has been shown that constipation can be a pre-motor symptom of PD years before the patients present with the clinical signs consistent with CNS degeneration (Gao et al., 2011; Lesser, 2002). In addition, an increased intestinal permeability was shown in PD patients compared to healthy controls (Schwiertz et al., 2018). Other studies suggest that there is an increased risk of developing dementia (Chen et al., 2016) or PD (Lai et al., 2014) in patients with IBS.
Similar to the trend in AD research, the relationships between the intestinal microbiota and PD pathophysiology and their association with disturbed GI motility have been studied extensively and some of the reported differences include a decrease in fecal numbers of Prevotella spp. and Clostridium spp. in PD patients (Tan et al., 2014; Scheperjans et al., 2015). These intestinal bacteria are a major source of SCFAs, particularly butyrate, folate (vitamin B9), and thiamine (vitamin B1), which are critical for the long-term maintenance of the epithelial barrier function (Tan et al., 2014; Scheperjans et al., 2015). Interestingly, chronic exposure to these SCFAs has been associated with clinical improvement in patients with PD [i.e., decreased dopaminergic degeneration and disruption of blood-brain barrier (BBB)] and clinical symptoms (Luong and Nguyen, 2013; Scheperjans et al., 2015; Liu et al., 2017), possibly due to ketogenesis. Finally, there are a few anecdotal reports suggesting a role for the Tobacco Mosaic virus (TMV; Friedland, 2015) in the pathophysiology of PD, but these preliminary findings need to be consolidated by additional studies on the topic.
Experimental Approaches to Investigating the GBA
Both static and dynamic in vitro models have been utilized to advance the understanding of the role of the GBA in neurodegenerative diseases. In addition, novel primary intestinal stem cell (ISC) culture systems have been utilized to mimic both physiologic and pathophysiologic intestinal conditions in vitro contributing to defining gut-cross talk with local environment (Gonzalez et al., 2013; Sato and Clevers, 2013; Chandra et al., 2018). The benefits and disadvantages of two current in vitro models are summarized in Figure 3. Importantly, cognitive dysfunction is highly prevalent not only in AD patients but also in approximately one-third of patients with PD (herein referred to as “non-motor symptom” of PD; Chaudhuri et al., 2006). In the “In Vitro Models” section and “In Vivo Animal Models” section, our main focus will be on AD. However, findings from these in vivo models for investigating mechanisms of cognitive impairment would be relevant to PD as well. The similarities and differences of clinical and histological observations in humans, dogs, and rodents are further summarized in Figure 4.
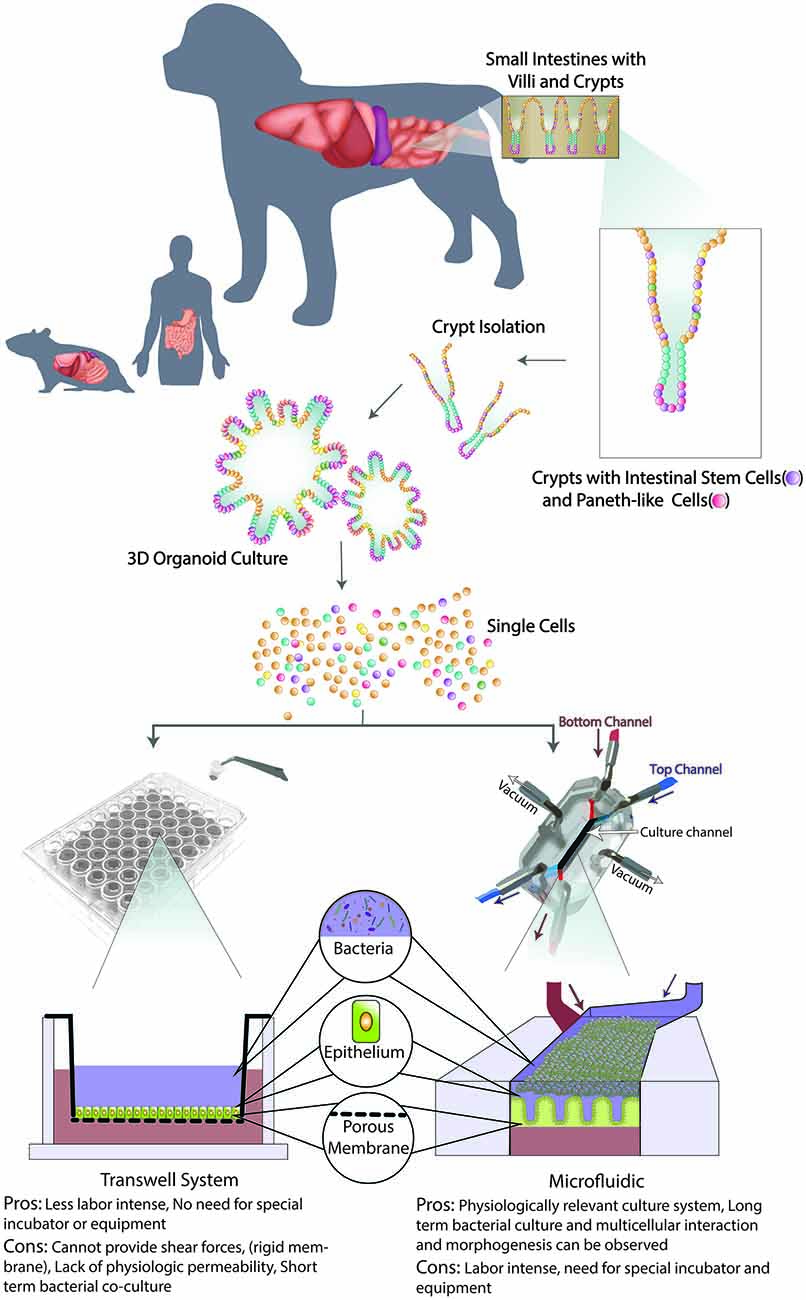
Figure 3. Schematic of organoid 3D culture development and integration into Transwell and Microfluidic systems. First, the intestinal biopsy is obtained via endoscopically or surgically, then villi and crypts are isolated with intestinal stem cells (ISCs) and Paneth-like cells. When cultured in an extracellular matrix with appropriate microenvironment factors, long-term culture of 3D canine enteroids/colonoids (ENT/COL) is accomplished. Second, a single cell suspension from such 3D culture system will be integrated with Transwell (left) and microfluidic (right) systems. On the transwell insert, 3D ENT/COL is cultured on top of the porous membrane with culture medium in the apical (blue) side and then submerged in culture medium in the basolateral (red) wells. A schematic of a Gut-on-a-chip (GOAC) microdevice allows a closed system with microtubing. Arrows indicate the direction of the flow of culture medium in the apical (blue) and basolateral (red) microchannels.
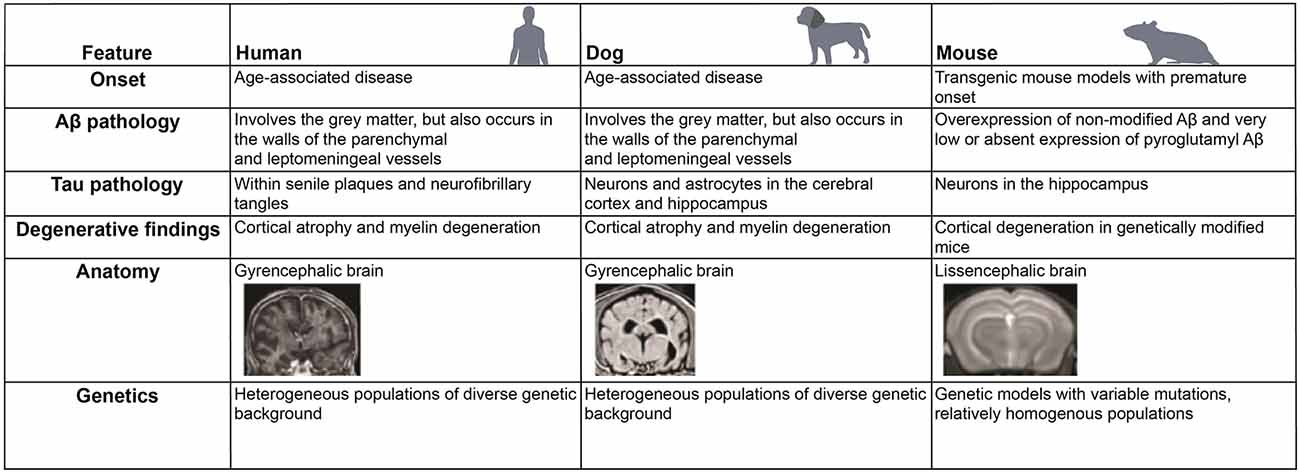
Figure 4. Comparative features of neurodegenerative changes and anatomy in different mammalian species. Similarities and differences in the development of neurodegenerative diseases, such as Alzheimer’s disease (AD), in human, dog, and mouse are listed.
In vitro Models
Static Systems
Development of translatable in vitro models is critical for elucidating disease pathophysiology and developing effective therapies for neurodegenerative diseases. Currently, only about 7% of investigational compounds tested in phase III clinical trials progress on to the market in neurology (Kola and Landis, 2004). This is worse than the average of 11% success rate of drugs marketed for all disease categories (Kola and Landis, 2004; Adjei et al., 2009). The BBB, a unique interface between the peripheral vascular system and the CNS, is a unique feature of the GBA (Rubin et al., 1991). The critical roles of BBB include supplying nutrients to the CNS, allowing the removal of waste products (such as urea or potassium), and preventing blood-borne pathogens and toxic products from entering into the brain (Alcendor et al., 2012). The BBB consists of TJs between capillary endothelial cells without fenestrations, and therefore allows the BBB to maintain a low level of pinocytosis, which preserves the structural integrity of BBB (Alcendor et al., 2013).
Attempts to develop an in vitro model to recapitulate the complexity of the BBB have included brain microvascular endothelial cells and astrocytes in a Transwell culture (Ahmed et al., in press). Leveraging its similarity with conventional 2-dimensional (2D) culture systems and its relative simplicity, the Transwell BBB system has been widely used in a research setting (Rubin et al., 1991). However, the maintenance of TJ function requires the application of the shear forces which traditional static Transwell systems are not able to offer (Santaguida et al., 2006). These critical shortcomings, including lower transepithelial electrical resistance (TEER) and higher endothelial permeability than reported in vivo, typically lead to an overestimation of drug permeability across the BBB (Santaguida et al., 2006).
Additionally, current in vitro models does not replicate the close physiological cross-talk between pericytes and the capillary endothelium that comprise the neurovascular unit (Jamieson et al., 2017). Successful integration of intraluminal flow for the in vitro culture of astrocytes has resulted in more physiological endothelial cell polarity and strengthening of TJs (Cucullo et al., 2011).
Attempts were made to study the GBA using a Transwell culture system as well (Haller et al., 2000). However, this system included only a few components of the GBA, and it is important to note that Caco-2 cells, an immortalized cell line derived from human colorectal adenocarcinoma, are used to model the enteric epithelial cells in this system. Given these collective limitations, as well as the lack of integration of microbiome/ENS in the in vitro system, the results derived from these studies may not be readily indicative of translational efficacy.
Importantly, our group recently established canine primary enteroid and colonoid (ENT/COL) culture systems (Kingsbury et al., 2017; Mochel et al., 2017). This is a canine ISC culture system which closely mimics the physiologic structure and function of in vivo intestines from both healthy and diseased individuals (Chandra et al., 2018), and allows for the investigation of pathophysiology and treatment effects. Of note, canine cognitive dysfunction (CCD) is a well-studied clinical analog of AD (Kol et al., 2015; Schütt et al., 2015; Hoffman et al., 2018; Wang et al., 2018). Since dogs and humans share an anatomically and physiologically very similar GI tract and harbor a taxonomically and functionally largely overlapping microbiome, the dog provides unique features as a spontaneous model of disease (Coelho et al., 2018; Alessandri et al., 2019). Overall, this canine model may hold promise with its translational relevance for exploration of avenues of novel therapeutics for neurologic disease in the near future (Mochel et al., 2017).
Dynamic Model Systems Using Microfluidics
Only recently, a novel ex vivo model offering dynamic shear forces to mimic physiologic conditions called organ-on-a-chip (organ-OAC) has emerged (Kimura et al., 2008; Sung et al., 2011). This microfluidic device contains microtubing that allows for continuous flow of media and is comprised of multiple cell culture channels enabling co-culture of different cell types (Kim and Ingber, 2013; Kim et al., 2016a). Specifically, the Gut-on-a-chip (GOAC) models the complex human intestinal anatomy into a two-microchannel device where volumetric flow rate, mechanical deformations, and fluid shear stress can be adjusted to reproduce the in vivo physiology of the gut (Kim and Ingber, 2013; Kim et al., 2016a). This biomimetic approach allows for the growth of the villous microarchitecture in Caco-2 cells, while proliferative cells from the intestinal crypt spontaneously migrate toward the villous tip similar to intestinal cells in vivo (Kim and Ingber, 2013). Also, differentiation of intestinal epithelial cell lines into four lineage-dependent subtypes (absorptive, mucus-secretory, enteroendocrine, and Paneth) is observed in this microfluidic system and presents a clear advantage over traditional 2D static culture systems (Kim and Ingber, 2013). When Caco-2 cells form 3-dimensional (3D) villi in the GOAC, they typically show enhanced epithelial barrier integrity, increased mucus production, and elevated drug-metabolizing P450 activity with augmented surface area and glucose reuptake, which are all relevant factors for modeling human intestinal physiology (Kim and Ingber, 2013). The 3D microarchitecture and increased mucus production are beneficial to grow live bacteria comprising the human gut microbiota using controlled flow and shear forces mimicking intestinal peristalsis (Kim et al., 2012; Kim et al., 2016a; Shin and Kim, 2018). Steady-state culture conditions inside the microchannels prevent the depletion of nutrients and the overgrowth of microbes (Kim et al., 2012). Critically, overgrowth of bacteria was seen only when manipulation to the shear stress was applied in the GOAC, which emulates the pathophysiological feature of the ileus (Kim et al., 2016a). By leveraging the innovative features of the GOAC, studies on the complex interactions between the host intestinal epithelium and the gut microbiome were also made possible (Kim et al., 2012; Kim et al., 2016b; Shin and Kim, 2018). For example, interactions between the intestinal epithelium, immune cell components, and intestinal bacteria (including non-pathogenic, pathogenic, or probiotic strains) were characterized by adding individual components one-at-a-time in a spatiotemporal manner (Kim et al., 2016a; Shin and Kim, 2018). This approach will enable researchers to evaluate the role of gut microbiome-brain axis in the development and progression of numerous intestinal diseases, such as inflammatory bowel disease (IBD) or colorectal cancer (CRC). Furthermore, the anoxic-oxic interface (AOI) of the oxygen gradient inside a modified GOAC was successfully recreated in a recent report, allowing for the co-culture of strict anaerobic intestinal bacteria and members of the fecal microbiome (Shin et al., 2019). This technology can be used to investigate the cross-talk between the gut microbiome and probiotics on intestinal health.
Recently, a BBB-OAC was established and showed physiological barrier functions (Wang et al., 2017), using ENS and enteroendocrine cells (EEC)-OAC combined to assess the GBA microenvironment (Ahmed et al., in press). Advancement in bioengineering techniques will allow incorporating multiple compartments in one in vitro system such as a GBA-OAC (Choe et al., 2017; Ahmed et al., in press; Lee and Sung, 2018). Despite the great promise of the Organ Chip technology, the transfer of cells from a macroscopic environment (e.g., well-plates) to a microfluidic system requires significant revision and optimization of cell culture protocols. Multiple factors differentiate microfluidic from macroscopic cell cultures. Microfluidic systems, for instance, harbor different culture channel surfaces and require fewer media volume as compared with macroscopic cultures (Sung and Shuler, 2009). Despite these limiting factors including the technology being labor-intensive, GOACs are a fast-growing model system that holds greater potential to investigate primary GI diseases. By extension, this system may be able to model GBA microenvironment and brain associations to better understand the role of enteric dysbiosis and neurodegenerative diseases.
In vivo Animal Models
While transgenic rodent models have been utilized to address targeted mechanistic questions relating to neuropathology and altered behavior (Hall and Roberson, 2012, 201), it is important to realize the inherent limitations of these in vivo models. Since mouse studies are used in the initial stages of drug discovery, the limitations in this animal model likely contribute to the poor success rate of AD drug discovery over the last 10 years (Kola and Landis, 2004; Adjei et al., 2009). One major limitation in studying the human GBA is a lack of an accurate animal model system that successfully replicates human ENS-microbiome interactions in health and disease. Investigation into the role of GBA with therapeutic interventions may require animal studies with tissues derived from animals that develop naturally occurring disease, including the dog. Since rodent diets differ substantially from that of humans, and diets are an important environmental factor shaping composition of the microbiome, comparing the effect of diet between species that harbor different microbial compositions (and likely functions) is difficult (Flint, 2011; Ravussin et al., 2012). For example, mice preferentially consume grains and cereals which contain relatively low ascorbic acid but have evolved their ability to synthesize this essential cofactor while humans have lost the ability to do so (Perlman, 2016) since they are omnivores. Different cytochrome P450 enzymes exist in mice compared to those in humans, thus each species has unique xenobiotic metabolism pathways that contribute to detoxification in each species (Martignoni et al., 2006; Anderson et al., 2009). These differing means of detoxification may be another reason why toxicology testing in mice has poor translatability to human toxicity (Olson et al., 2000).
Another factor explaining why rodent models do not mirror aspects of human pathophysiology is related to the limited tendency of some of these induced models to develop amyloidosis. As discussed before, AD is histologically characterized by the presence of Aβ aggregates in the walls of cerebral vessels (Attems, 2005; Herzig et al., 2006). Rodent models do not produce human sequence Aβ naturally (Shepherd et al., 2018), which limits their investigative utility as a translational model. Transgenic mouse models overexpressing mutant human amyloid precursor protein (APP) alone, or combined with transgenic presenilin 1 (PS1) and presenilin 2 (PS2), do have secondary Aβ plaque formation in the brain, histologically mimicking AD (Götz et al., 2008). However, these transgenic mouse models naturally have molecular and systemic resistance to Aβ pathology and therefore do not develop the extensive neuronal loss and clinical signs seen in human AD patients (Martin et al., 2011). Lastly, there are fundamental differences in the anatomic folding of the cerebral cortex, with humans having a gyrencephalic brain and rodents having a lissencephalic brain (Sun and Hevner, 2014). A recent meta-analysis study demonstrates that various transgenic mouse models of AD show different characteristics compared to what have observed in the human AD (Hargis and Blalock, 2017). Specifically, the findings from spontaneous AD people were not consistent with those in transgenic AD mouse models, while human studies hold similar findings across different studies (Hargis and Blalock, 2017). The study also found that among the major transgenic AD mouse the findings were not similar to one another (Hargis and Blalock, 2017).
Accumulated data shows that the dog provides a superior model system to transgenic mouse models for investigating the influence of aging in the development and treatment of neurologic disease (Head, 2013). The dog is a more translationally relevant species because of the environmental, genomic, and intestinal physiologic features they share with humans (Cummings et al., 1996). Dogs are an ideal aging model since they show a parallel aging process to humans as evidenced by beagles between 5 and 9 years old showing cognitive dysfunction similar to humans between 40 and 60 years old (Patronek et al., 1997). In addition, brain vs. body size compares favorably between humans and dogs as compared with mice (Roth and Dicke, 2005), which is another advantage of using the dog as a disease animal model for neurologic diseases as canine brains undergo similar stress as humans (Roth and Dicke, 2005). Canine spontaneous disease models also offer additional predictive value for treatment efficacy before transitioning to human clinical trials (Kol et al., 2015; Schütt et al., 2016). Finally, dog genes have adapted to a starch-rich diet during domestication similar to humans, which suggests that studying such adaptations may improve our understanding of human evolution and disease (Axelsson et al., 2013).
Canine Models as Natural Models for Neurodegenerative Diseases: Similarities and Differences
Many human chronic disorders with a mixed genetic-environmental etiology (e.g., Diabetes Mellitus, IBD, CRC), including AD and PD, have well-studied clinical analogs in dogs (Kol et al., 2015; Schütt et al., 2016; Hoffman et al., 2018). Particularly relevant to AD, aged dogs with CCD spontaneously develop a progressive decline in cognitive function associated with advanced imaging abnormalities and histopathological features similar to AD (Davis and Head, 2014). For example, CCD dogs display progressive AD-like cortical atrophy (Rofina et al., 2006; Pugliese et al., 2010) in areas of the hippocampus that may be accompanied by ventricular enlargement (Su et al., 2005). Further, aged dog brains show other neuropathological and degenerative features similar to AD, including diffuse Aβ plaque deposition (Cummings et al., 1996; Borràs et al., 1999) with cortical amyloid angiopathy (CAA; Ishihara et al., 1991), neuronal loss in temporal regions first affected by AD (Colle et al., 2000), and dysfunction of neurotransmitter systems (Insua et al., 2012). Other neuropathological abnormalities shared between dogs and humans include hyperphosphorylated tau proteins in the brain (Yu et al., 2011; Böttner et al., 2012; Smolek et al., 2016) and increased plasma Aβ1–42 levels, one of the biomarkers of AD (Schütt et al., 2015).
In addition to CCD as a model for AD, certain dog breeds are considered spontaneous models for PD. Canine multiple system degeneration (CMSD) is a fatal, inheritable movement disorder first described in Kerry Blue Terriers (deLahunta and Averill, 1976), then in Chinese Crested dogs (O’Brien et al., 2005), and these breeds are considered as natural models for PD. Dogs with CMSD are clinically normal until 3–6 months of age when they first develop the clinical signs of cerebellar ataxia (O’Brien et al., 2005). This progresses to akinesia (i.e., impairment in voluntary movement) and severe postural instability ultimately leading to euthanasia by 1–2 years of age due to a severe decline in quality of life (O’Brien et al., 2005). The histological hallmark of CMSD includes the loss of cerebellar Purkinje cells with degeneration of the olivary nucleus, substantia nigra, and caudate nucleus (deLahunta and Averill, 1976; Montgomery and Storts, 1983), areas of which are relevant to PD etiopathogenesis. Interestingly, the CMSD locus includes a segment that contains PARK2, the gene for parkin, and mutations in human PARK2 is known to cause familial PD, which has clinical and pathological similarities to CMSD (O’Brien et al., 2005).
We acknowledge that there is no perfect animal model for investigating neurodegenerative disorders, and it should be recognized that the canine model also has limitations. For example, it has been recently shown that dogs lack aldehyde oxidases (AOXs) which catalyze the oxidation of aldehydes or N-heterocycles (Terao et al., 2006). This fact has physiological, pharmacological, and toxicological relevance since AOXs represent an important metabolic pathway that oxidizes numerous endogenous and exogenous substrates of biologic importance (Garattini et al., 2003). Also, humans and dogs have different CYP3A isoforms (i.e., canine CYP3A12 is equivalent to human CYP3A4) which impact species-specific differences in permeability, toxicity, and metabolism analysis between in vitro and in vivo systems (Zhang et al., 2001). A detailed assessment of drug transporters and metabolic enzymes expression in vitro is key to establish the predictive performance of these in systems recapitulating in vivo drug absorption and metabolism. Also, it is possible that differences in activity and substrate specificity/inhibitors and inducers are observed in the dog; therefore, utilizing in vitro systems from multiple different species would allow the supplementation of other in vitro systems that do not fully mimic human physiology on their own (Zhang et al., 2001).
Conclusion
Recent analyses suggest that one of the most expensive therapeutic areas having poor success rate in terms of drug research and discovery (R&D) is neurology (Kola and Landis, 2004). One barrier to achieving lower attrition rates in neurology drug R&D is the lack of utilization of appropriate naturally occurring models of disease, such as CCD as a model for human AD. The dog is a particularly relevant species since it shares multiple epidemiologic features with humans, including similarities in diet and their intestinal microbiomes. Furthermore, CCD dogs can be used as a natural model for both AD, as well as PD, since clinical trials can be performed in dogs to assess the efficacy of novel treatments prior to human trials (i.e., reverse extrapolation). Importantly, since organoids are derived from individuals with different genotypes and environmental exposures, they are a highly relevant model system for ex vivo studies, and are of value in “precision medicine.” Integration of organoid culture systems with GOAC technology will maintain patient-specific genetic and epigenetic disease characteristics influencing inter-patient drug screening during the early exploratory R&D phase. We predict that it will be possible to predict the outcome of novel therapeutics prior to human trials by combining data from GOAC models and clinical trials with dogs serving as a model for naturally occurring neurodegenerative diseases.
Author Contributions
YA and JM conceived the idea for the review. YA searched and reviewed the literature, drafted and revised the manuscript. AW further searched and reviewed the AD and PD literature and revised the manuscript. JM, DB, KA, AJ, AK, and HK reviewed and edited the manuscript. All authors read and approved the final manuscript.
Funding
This work was supported in part by the ACVIM Advanced Research Training Fellowship, Bio & Medical Technology Development Program of the National Research Foundation funded by the Ministry of Science and ICT (2018M3A9H3025030 to HK), Cancer Research Institute (UTA18-000889 to HK), Alternative Research and Development Foundation (UTA18-001198 to HK).
Conflict of Interest Statement
JM, AJ, KA, and HK are founders of a company, 3D Health Solutions. JM, AJ, and KA are founders of a company, LEAH (Life Engine Animal Health, Inc.) located in Rochester, MN, USA. AK has an equity interest in PK Biosciences Corporation located in Ames, IA, USA. The terms of this arrangement have been reviewed and approved by Iowa State University in accordance with its conflict of interest policies.
The remaining authors declare that the research was conducted in the absence of any commercial or financial relationships that could be construed as a potential conflict of interest.
Acknowledgments
The earlier version of this manuscript has been released as a Pre-Print at www.Preprints.org (Ambrosini et al., 2019).
References
Adjei, A. A., Christian, M., and Ivy, P. (2009). Novel designs and end points for phase II clinical trials. Clin. Cancer Res. 15, 1866–1872. doi: 10.1158/1078-0432.ccr-08-2035
Ahmed, M., Puzan, M., and Koppes, D. A. (in press). Gut-brain-axis on a chip: a microfluidic model of the enteroendocrine-enteric nervous system interface:1. Available online at: https://www.northeastern.edu/rise/presentations/gut-brain-axis-on-a-chip-a-microfluidic-model-of-the-enteroendocrine-enteric-nervous-system-interface/
Alcendor, D. J., Charest, A. M., Zhu, W. Q., Vigil, H. E., and Knobel, S. M. (2012). Infection and upregulation of proinflammatory cytokines in human brain vascular pericytes by human cytomegalovirus. J. Neuroinflammation 9:95. doi: 10.1186/1742-2094-9-95
Alcendor, D. J., Block, F. E. III., Cliffel, D. E., Daniels, J. S., Ellacott, K. L. J., Goodwin, C. R., et al. (2013). Neurovascular unit on a chip: implications for translational applications. Stem Cell Res. Ther. 4:S18. doi: 10.1186/scrt379
Alessandri, G., Milani, C., Mancabelli, L., Mangifesta, M., Lugli, G. A., Viappiani, A., et al. (2019). Metagenomic dissection of the canine gut microbiota: insights into taxonomic, metabolic and nutritional features. Environ. Microbiol. 21, 1331–1343. doi: 10.1111/1462-2920.14540
Ambrosini, Y., Borcherding, D., Kanthasamy, A., Kim, H. J., Jergens, A., Allenspach, K., et al. (2019). The role of the gut-brain axis in neurodegenerative diseases and relevance of the canine model: a review. Preprints 2019:2019010275. doi: 10.20944/preprints201901.0275.v1
Anderson, S., Luffer-Atlas, D., and Knadler, M. P. (2009). Predicting circulating human metabolites: how good are we? Chem. Res. Toxicol. 22, 243–256. doi: 10.1021/tx8004086
Ano, Y., Sakudo, A., Nakayama, H., and Onodera, T. (2009). Uptake and dynamics of infectious prion protein in the intestine. Protein Pept. Lett. 16, 247–255. doi: 10.2174/092986609787601642
Asano, Y., Hiramoto, T., Nishino, R., Aiba, Y., Kimura, T., Yoshihara, K., et al. (2012). Critical role of gut microbiota in the production of biologically active, free catecholamines in the gut lumen of mice. Am. J. Physiol. Gastrointest. Liver Physiol. 303, G1288–G1295. doi: 10.1152/ajpgi.00341.2012
Asti, A., and Gioglio, L. (2014). Can a bacterial endotoxin be a key factor in the kinetics of amyloid fibril formation? J. Alzheimers Dis. 39, 169–179. doi: 10.3233/jad-131394
Attems, J., Jellinger, K. A., and Lintner, F. (2005). Alzheimer’s disease pathology influences severity and topographical distribution of cerebral amyloid angiopathy. Acta Neuropathol. 110, 222–231. doi: 10.1007/s00401-005-1064-y
Attems, J. (2005). Sporadic cerebral amyloid angiopathy: pathology, clinical implications, and possible pathomechanisms. Acta Neuropathol. 110, 345–359. doi: 10.1007/s00401-005-1074-9
Axelsson, E., Ratnakumar, A., Arendt, M.-L., Maqbool, K., Webster, M. T., Perloski, M., et al. (2013). The genomic signature of dog domestication reveals adaptation to a starch-rich diet. Nature 495, 360–364. doi: 10.1038/nature11837
Aziz, Q., Doré, J., Emmanuel, A., Guarner, F., and Quigley, E. M. M. (2013). Gut microbiota and gastrointestinal health: current concepts and future directions. Neurogastroenterol. Motil. 25, 4–15. doi: 10.1111/nmo.12046
Bienenstock, J., Kunze, W., and Forsythe, P. (2015). Microbiota and the gut-brain axis. Nutr. Rev. 73, 28–31. doi: 10.1093/nutrit/nuv019
Borràs, D., Ferrer, I., and Pumarola, M. (1999). Age-related changes in the brain of the dog. Vet. Pathol. 36, 202–211. doi: 10.1354/vp.36-3-202
Böttner, M., Zorenkov, D., Hellwig, I., Barrenschee, M., Harde, J., Fricke, T., et al. (2012). Expression pattern and localization of α-synuclein in the human enteric nervous system. Neurobiol. Dis. 48, 474–480. doi: 10.1016/j.nbd.2012.07.018
Braak, H., de Vos, R. A. I., Bohl, J., and Tredici, K. D. (2006). Gastric α-synuclein immunoreactive inclusions in meissner’s and auerbach’s plexuses in cases staged for Parkinson’s disease-related brain pathology. Neurosci. Lett. 396, 67–72. doi: 10.1016/j.neulet.2005.11.012
Breydo, L., Wu, J. W., and Uversky, V. N. (2012). α-synuclein misfolding and Parkinson’s disease. Biochim. Biophys. Acta 1822, 261–285. doi: 10.1016/j.bbadis.2011.10.002
Browning, K. N., and Travagli, R. A. (2014). Central nervous system control of gastrointestinal motility and secretion and modulation of gastrointestinal functions. Compr. Physiol. 4, 1339–1368. doi: 10.1002/cphy.c130055
Brundin, P., Ma, J., and Kordower, J. H. (2016). How strong is the evidence that Parkinson’s disease is a prion disorder? Curr. Opin. Neurol. 29, 459–466. doi: 10.1097/WCO.0000000000000349
Carabotti, M., Scirocco, A., Maselli, M. A., and Severi, C. (2015). The gut-brain axis: interactions between enteric microbiota, central and enteric nervous systems. Ann. Gastroenterol. 28, 203–209.
Chandra, L., Borcherding, D. C., Kingsbury, D., Atherly, T., Ambrosini, Y. M., Bourgois-Mochel, A., et al. (2018). Adult canine intestinal derived organoids: a novel in vitro system for translational research in comparative gastroenterology. bioRxiv [Preprint]. doi: 10.1101/466409
Chaudhuri, K. R., Healy, D. G., and Schapira, A. H. V. (2006). Non-motor symptoms of Parkinson’s disease: diagnosis and management. Lancet Neurol. 5, 235–245. doi: 10.1016/S1474-4422(06)70373-8
Chen, C.-H., Lin, C.-L., and Kao, C.-H. (2016). Irritable bowel syndrome is associated with an increased risk of dementia: a nationwide population-based study. PLoS One 11:e0144589. doi: 10.1371/journal.pone.0144589
Choe, A., Ha, S. K., Choi, I., Choi, N., and Sung, J. H. (2017). Microfluidic gut-liver chip for reproducing the first pass metabolism. Biomed. Microdevices 19:4. doi: 10.1007/s10544-016-0143-2
Coelho, L. P., Kultima, J. R., Costea, P. I., Fournier, C., Pan, Y., Czarnecki-Maulden, G., et al. (2018). Similarity of the dog and human gut microbiomes in gene content and response to diet. Microbiome 6:72. doi: 10.1186/s40168-018-0450-3
Colle, M.-A., Hauw, J.-J., Crespeau, F., Uchihara, T., Akiyama, H., Checler, F., et al. (2000). Vascular and parenchymal Aβ deposition in the aging dog: correlation with behavior. Neurobiol. Aging 21, 695–704. doi: 10.1016/S0197-4580(00)00113-5
Cucullo, L., Hossain, M., Puvenna, V., Marchi, N., and Janigro, D. (2011). The role of shear stress in blood-brain barrier endothelial physiology. BMC Neurosci. 12:40. doi: 10.1186/1471-2202-12-40
Cummings, B. J., Pike, C. J., Shankle, R., and Cotman, C. W. (1996). β-amyloid deposition and other measures of neuropathology predict cognitive status in Alzheimer’s disease. Neurobiol. Aging 17, 921–933. doi: 10.1016/s0197-4580(96)00170-4
Danzer, K. M., Kranich, L. R., Ruf, W. P., Cagsal-Getkin, O., Winslow, A. R., Zhu, L., et al. (2012). Exosomal cell-to-cell transmission of α synuclein oligomers. Mol. Neurodegener. 7:42. doi: 10.1186/1750-1326-7-42
Davis, P. R., and Head, E. (2014). Prevention approaches in a preclinical canine model of Alzheimer’s disease: benefits and challenges. Front. Pharmacol. 5:47. doi: 10.3389/fphar.2014.00047
deLahunta, A., and Averill, D. R. Jr. (1976). Hereditary cerebellar cortical and extrapyramidal nuclear abiotrophy in kerry blue terriers. J. Am. Vet. Med. Assoc. 168, 1119–1124.
de Lartigue, G., de La Serre, C. B., and Raybould, H. E. (2011). Vagal afferent neurons in high fat diet-induced obesity; intestinal microflora, gut inflammation and cholecystokinin. Physiol. Behav. 105, 100–105. doi: 10.1016/j.physbeh.2011.02.040
Delzenne, N. M., Neyrinck, A. M., and Cani, P. D. (2011). Modulation of the gut microbiota by nutrients with prebiotic properties: consequences for host health in the context of obesity and metabolic syndrome. Microb. Cell Fact. 10:S10. doi: 10.1186/1475-2859-10-S1-S10
Dinan, T. G., Quigley, E. M. M., Ahmed, S. M. M., Scully, P., O’Brien, S., O’Mahony, L., et al. (2006). Hypothalamic-pituitary-gut axis dysregulation in irritable bowel syndrome: plasma cytokines as a potential biomarker? Gastroenterology 130, 304–311. doi: 10.1053/j.gastro.2005.11.033
Esteve, E., Ricart, W., and Fernández-real, J.-M. (2011). Gut microbiota interactions with obesity, insulin resistance and type 2 diabetes: did gut microbiote co-evolve with insulin resistance? Curr. Opin. Clin. Nutr. Metab. Care 14, 483–490. doi: 10.1097/mco.0b013e328348c06d
Flint, H. J. (2011). Obesity and the gut microbiota. J. Clin. Gastroenterol. 45, S128–S132. doi: 10.1097/MCG.0b013e31821f44c4
Foster, J. A., Rinaman, L., and Cryan, J. F. (2017). Stress and the gut-brain axis: regulation by the microbiome. Neurobiol. Stress 7, 124–136. doi: 10.1016/j.ynstr.2017.03.001
Friedland, R. P. (2015). Mechanisms of molecular mimicry involving the microbiota in neurodegeneration. J. Alzheimers Dis. 45, 349–362. doi: 10.3233/jad-142841
Galloway, S., Jian, L., Johnsen, R., Chew, S., and Mamo, J. C. L. (2007). β-amyloid or its precursor protein is found in epithelial cells of the small intestine and is stimulated by high-fat feeding. J. Nutr. Biochem. 18, 279–284. doi: 10.1016/j.jnutbio.2006.07.003
Galloway, S., Takechi, R., Pallebage-Gamarallage, M. M. S., Dhaliwal, S. S., and Mamo, J. C. L. (2009). Amyloid-β colocalizes with apolipoprotein B in absorptive cells of the small intestine. Lipids Health Dis. 8:46. doi: 10.1186/1476-511X-8-46
Gao, X., Chen, H., Schwarzschild, M. A., and Ascherio, A. (2011). A prospective study of bowel movement frequency and risk of Parkinson’s disease. Am. J. Epidemiol. 174, 546–551. doi: 10.1093/aje/kwr119
Garattini, E., Mendel, R., Romão, M. J., Wright, R., and Terao, M. (2003). Mammalian molybdo-flavoenzymes, an expanding family of proteins: structure, genetics, regulation, function and pathophysiology. Biochem. J. 372, 15–32. doi: 10.1042/bj20030121
Goedert, M. (2015). Alzheimer’s and Parkinson’s diseases: the prion concept in relation to assembled Aβ, tau, and α-synuclein. Science 349:1255555. doi: 10.1126/science.1255555
Gold, A., Turkalp, Z. T., and Munoz, D. G. (2013). Enteric α-synuclein expression is increased in Parkinson’s disease but not Alzheimer’s disease. Mov. Disord. 28, 237–240. doi: 10.1002/mds.25298
Gonzalez, L. M., Williamson, I., Piedrahita, J. A., Blikslager, A. T., and Magness, S. T. (2013). Cell lineage identification and stem cell culture in a porcine model for the study of intestinal epithelial regeneration. PLoS One 8:e66465. doi: 10.1371/journal.pone.0066465
Götz, J., Ittner, L. M., Schonrock, N., and Cappai, R. (2008). An update on the toxicity of Aβ in Alzheimer’s disease. Neuropsychiatr. Dis. Treat. 4, 1033–1042. doi: 10.2147/ndt.s3016
Grasset, E., Puel, A., Charpentier, J., Collet, X., Christensen, J. E., Tercé, F., et al. (2017). A specific gut microbiota dysbiosis of type 2 diabetic mice induces GLP-1 resistance through an enteric NO-dependent and gut-brain axis mechanism. Cell Metab. 25, 1075.e5–1090.e5. doi: 10.1016/j.cmet.2017.04.013
Grenham, S., Clarke, G., Cryan, J. F., and Dinan, T. G. (2011). Brain-gut-microbe communication in health and disease. Front. Physiol. 2:94. doi: 10.3389/fphys.2011.00094
Grider, J. R., and Piland, B. E. (2007). The peristaltic reflex induced by short-chain fatty acids is mediated by sequential release of 5-HT and neuronal CGRP but not BDNF. Am. J. Physiol. Gastrointest. Liver Physiol. 292, G429–G437. doi: 10.1152/ajpgi.00376.2006
Hall, A. M., and Roberson, E. D. (2012). Mouse models of Alzheimer’s disease. Brain Res. Bull. 88, 3–12. doi: 10.1016/j.brainresbull.2011.11.017
Haller, D., Bode, C., Hammes, W. P., Pfeifer, A. M. A., Schiffrin, E. J., and Blum, S. (2000). Non-pathogenic bacteria elicit a differential cytokine response by intestinal epithelial cell/leucocyte co-cultures. Gut 47, 79–87. doi: 10.1136/gut.47.1.79
Hargis, K. E., and Blalock, E. M. (2017). Transcriptional signatures of brain aging and Alzheimer’s disease: what are our rodent models telling us? Behav. Brain Res. 322, 311–328. doi: 10.1016/j.bbr.2016.05.007
Hasegawa, M., Fujiwara, H., Nonaka, T., Wakabayashi, K., Takahashi, H., Lee, V. M.-Y., et al. (2002). Phosphorylated α-synuclein is ubiquitinated in α-synucleinopathy lesions. J. Biol. Chem. 277, 49071–49076. doi: 10.1074/jbc.M208046200
Head, E. (2013). A canine model of human aging and Alzheimer’s disease. Biochim. Biophys. Acta 1832, 1384–1389. doi: 10.1016/j.bbadis.2013.03.016
Herzig, M. C., van Nostrand, W. E., and Jucker, M. (2006). Mechanism of cerebral β-amyloid angiopathy: murine and cellular models. Brain Pathol. 16, 40–54. doi: 10.1111/j.1750-3639.2006.tb00560.x
Hilton, D., Stephens, M., Kirk, L., Edwards, P., Potter, R., Zajicek, J., et al. (2014). Accumulation of α-synuclein in the bowel of patients in the pre-clinical phase of Parkinson’s disease. Acta Neuropathol. 127, 235–241. doi: 10.1007/s00401-013-1214-6
Hoffman, J. M., Creevy, K. E., Franks, A., O’Neill, D. G., and Promislow, D. E. L. (2018). The companion dog as a model for human aging and mortality. Aging Cell 17:e12737. doi: 10.1111/acel.12737
Hornig, M. (2013). The role of microbes and autoimmunity in the pathogenesis of neuropsychiatric illness. Curr. Opin. Rheumatol. 25, 488–795. doi: 10.1097/bor.0b013e32836208de
Houser, M. C., and Tansey, M. G. (2017). The gut-brain axis: is intestinal inflammation a silent driver of Parkinson’s disease pathogenesis? NPJ Parkinsons Dis. 3:3. doi: 10.1038/s41531-016-0002-0
Hufnagel, D. A., Tükel, Ç., and Chapman, M. R. (2013). Disease to dirt: the biology of microbial amyloids. PLoS Pathog. 9:e1003740. doi: 10.1371/journal.ppat.1003740
Iadanza, M. G., Jackson, M. P., Hewitt, E. W., Ranson, N. A., and Radford, S. E. (2018). A new era for understanding amyloid structures and disease. Nat. Rev. Mol. Cell Biol. 19, 755–773. doi: 10.1038/s41580-018-0060-8
Insua, D., Corredoira, A., González-Martínez, Á., Suárez, M.-L., Santamarina, G., Sarasa, M., et al. (2012). Expression of P75 NTR, a marker for basal forebrain cholinergic neurons, in young and aged dogs with or without cognitive dysfunction syndrome. J. Alzheimers Dis. 28, 291–296. doi: 10.3233/jad-2011-110905
Ishihara, T., Gondo, T., Takahashi, M., Uchino, F., Ikeda, S.-I., Allsop, D., et al. (1991). Immunohistochemical and immunoelectron microscopical characterization of cerebrovascular and senile plaque amyloid in aged dogs’ brains. Brain Res. 548, 196–205. doi: 10.1016/0006-8993(91)91122-h
Iyer, L. M., Aravind, L., Coon, S. L., Klein, D. C., and Koonin, E. V. (2004). Evolution of cell-cell signaling in animals: did late horizontal gene transfer from bacteria have a role? Trends Genet. 20, 292–299. doi: 10.1016/j.tig.2004.05.007
Jamieson, J. J., Searson, P. C., and Gerecht, S. (2017). Engineering the human blood-brain barrier in vitro. J. Biol. Eng. 11:37. doi: 10.1186/s13036-017-0076-1
Jenner, P. (2003). Oxidative stress in Parkinson’s disease. Ann. Neurol. 53, S26–S38. doi: 10.1002/ana.10483
Jiang, C., Li, G., Huang, P., Liu, Z., and Zhao, B. (2017). The gut microbiota and Alzheimer’s disease. J. Alzheimers Dis. 58, 1–15. doi: 10.3233/JAD-161141
Johnson, K. A., Fox, N. C., Sperling, R. A., and Klunk, W. E. (2012). Brain imaging in Alzheimer disease. Cold Spring Harb. Perspect. Med. 2:a006213. doi: 10.1101/cshperspect.a006213
Kihara, N., Fujimura, M., Yamamoto, I., Itoh, E., Inui, A., and Fujimiya, M. (2001). Effects of central and peripheral urocortin on fed and fasted gastroduodenal motor activity in conscious rats. Am. J. Physiol. Gastrointest. Liver Physiol. 280, G406–G419. doi: 10.1152/ajpgi.2001.280.3.g406
Kim, H. J., Huh, D., Hamilton, G., and Ingber, D. E. (2012). Human gut-on-a-chip inhabited by microbial flora that experiences intestinal peristalsis-like motions and flow. Lab Chip 12, 2165–2174. doi: 10.1039/c2lc40074j
Kim, H. J., and Ingber, D. E. (2013). Gut-on-a-chip microenvironment induces human intestinal cells to undergo villus differentiation. Integr. Biol. 5, 1130–1140. doi: 10.1039/c3ib40126j
Kim, H. J., Li, H., Collins, J. J., and Ingber, D. E. (2016a). Contributions of microbiome and mechanical deformation to intestinal bacterial overgrowth and inflammation in a human gut-on-a-chip. Proc. Natl. Acad. Sci. U S A 113, E7–E15. doi: 10.1073/pnas.1522193112
Kim, H. J., Lee, J., Choi, J.-H., Bahinski, A., and Ingber, D. E. (2016b). Co-culture of living microbiome with microengineered human intestinal villi in a gut-on-a-chip microfluidic device. J. Vis. Exp. 114:e54344. doi: 10.3791/54344
Kimura, I., Inoue, D., Maeda, T., Hara, T., Ichimura, A., Miyauchi, S., et al. (2011). Short-chain fatty acids and ketones directly regulate sympathetic nervous system via G protein-coupled receptor 41 (GPR41). Proc. Natl. Acad. Sci. U S A 108, 8030–8035. doi: 10.1073/pnas.1016088108
Kimura, H., Yamamoto, T., Sakai, H., Sakai, Y., and Fujii, T. (2008). An integrated microfluidic system for long-term perfusion culture and on-line monitoring of intestinal tissue models. Lab Chip 8, 741–746. doi: 10.1039/b717091b
Kingsbury, D. D., Sun, L., Qi, Y., Fredericks, J., Wang, Q., M Wannemuehler, J., et al. (2017). Optimizing the development and characterization of canine small intestinal crypt organoids as a research model. Gastroenterology 152:S353. doi: 10.1016/s0016-5085(17)31441-5
Klein, C., and Westenberger, A. (2012). Genetics of Parkinson’s disease. Cold Spring Harb. Perspect. Med. 2:a008888. doi: 10.1101/cshperspect.a008888
Kol, A., Arzi, B., Athanasiou, K. A., Farmer, D. L., Nolta, J. A., Rebhun, R. B., et al. (2015). Companion animals: translational scientist’s new best friends. Sci. Transl. Med. 7:308ps21. doi: 10.1126/scitranslmed.aaa9116
Kola, I., and Landis, J. (2004). Can the pharmaceutical industry reduce attrition rates? Nat. Rev. Drug Discov. 3, 711–716. doi: 10.1038/nrd1470
Lai, S.-W., Liao, K.-F., Lin, C.-L., and Sung, F.-C. (2014). Irritable bowel syndrome correlates with increased risk of Parkinson’s disease in taiwan. Eur. J. Epidemiol. 29, 57–62. doi: 10.1007/s10654-014-9878-3
Lee, J. H., Ryan, J., Andreescu, C., Aizenstein, H., and Lim, H. K. (2015). Brainstem morphological changes in Alzheimer’s disease. Neuroreport 26, 411–415. doi: 10.1097/wnr.0000000000000362
Lee, S. Y., and Sung, J. H. (2018). Gut-liver on a chip toward an in vitro model of hepatic steatosis. Biotechnol. Bioeng. 115, 2817–2827. doi: 10.1002/bit.26793
Lemche, E. (2018). Early life stress and epigenetics in late-Onset Alzheimer’s dementia: a systematic review. Curr. Genomics 19, 522–602. doi: 10.2174/1389202919666171229145156
Lesser, G. T. (2002). Frequency of bowel movements and the future risk of Parkinson’s disease. Neurology 58:838. doi: 10.1212/wnl.58.5.838-a
Lin, B., Hasegawa, Y., Takane, K., Koibuchi, N., Cao, C., and Kim-Mitsuyama, S. (2016). High-fat-diet intake enhances cerebral amyloid angiopathy and cognitive impairment in a mouse model of Alzheimer’s disease, independently of metabolic disorders. J. Am. Heart Assoc. 5:e003154. doi: 10.1161/JAHA.115.003154
Liu, J., Wang, F., Liu, S., Du, J., Hu, X., Xiong, J., et al. (2017). Sodium butyrate exerts protective effect against Parkinson’s disease in mice via stimulation of glucagon like peptide-1. J. Neurol. Sci. 381, 176–181. doi: 10.1016/j.jns.2017.08.3235
Llorens, F., Thüne, K., Andrés-Benito, P., Tahir, W., Ansoleaga, B., Hernández-Ortega, K., et al. (2017). MicroRNA expression in the locus coeruleus, entorhinal cortex, and hippocampus at early and middle stages of braak neurofibrillary tangle pathology. J. Mol. Neurosci. 63, 206–215. doi: 10.1007/s12031-017-0971-4
Luong, K. V. Q., and Nguyen, L. T. H. (2013). The beneficial role of thiamine in parkinson disease. CNS Neurosci. Ther. 19, 461–468. doi: 10.1111/cns.12078
Martignoni, M., Groothuis, G. M. M., and de Kanter, R. (2006). Species differences between mouse, rat, dog, monkey and human CYP-mediated drug metabolism, inhibition and induction. Expert Opin. Drug Metab. Toxicol. 2, 875–894. doi: 10.1517/17425255.2.6.875
Martin, S. B., Dowling, A. L. S., and Head, E. (2011). Therapeutic interventions targeting β amyloid pathogenesis in an aging dog model. Curr. Neuropharmacol. 9, 651–661. doi: 10.2174/157015911798376217
Mitew, S., Kirkcaldie, M. T. K., Dickson, T. C., and Vickers, J. C. (2013). Altered synapses and gliotransmission in Alzheimer’s disease and AD model mice. Neurobiol. Aging 34, 2341–2351. doi: 10.1016/j.neurobiolaging.2013.04.010
Mochel, J. P., Jergens, A. E., Kingsbury, D., Kim, H. J., Martín, M. G., and Allenspach, K. (2017). Intestinal stem cells to advance drug development, precision, and regenerative medicine: a paradigm shift in translational research. AAPS J. 20:17. doi: 10.1208/s12248-017-0178-1
Mogi, M., Harada, M., Narabayashi, H., Inagaki, H., Minami, M., and Nagatsu, T. (1996). Interleukin (IL)-1β, IL-2, IL-4, IL-6 and transforming growth factor-α levels are elevated in ventricular cerebrospinal fluid in juvenile Parkinsonism and Parkinson’s disease. Neurosci. Lett. 211, 13–16. doi: 10.1016/0304-3940(96)12706-3
Moloney, R. D., Desbonnet, L., Clarke, G., Dinan, T. G., and Cryan, J. F. (2014). The microbiome: stress, health and disease. Mamm. Genome 25, 49–74. doi: 10.1007/s00335-013-9488-5
Monsell, S. E., Kukull, W. A., Roher, A. E., Maarouf, C. L., Serrano, G., Beach, T. G., et al. (2015). Characterizing apolipoprotein E ɛ4 carriers and noncarriers with the clinical diagnosis of mild to moderate Alzheimer dementia and minimal β-amyloid peptide plaques. JAMA Neurol. 72, 1124–1131. doi: 10.1001/jamaneurol.2015.1721
Montgomery, D. L., and Storts, R. W. (1983). Hereditary striatonigral and cerebello-olivary degeneration of the kerry blue terrier. I. Gross and light microscopic central nervous system lesions. Vet. Pathol. 20, 143–159. doi: 10.1177/030098588302000202
Morales, R., Moreno-Gonzalez, I., and Soto, C. (2013). Cross-seeding of misfolded proteins: implications for etiology and pathogenesis of protein misfolding diseases. PLoS Pathog. 9:e1003537. doi: 10.1371/journal.ppat.1003537
Muegge, B. D., Kuczynski, J., Knights, D., Clemente, J. C., González, A., Fontana, L., et al. (2011). Diet drives convergence in gut microbiome functions across mammalian phylogeny and within humans. Science 332, 970–974. doi: 10.1126/science.1198719
Nam, K. N., Mounier, A., Wolfe, C. M., Fitz, N. F., Carter, A. Y., Castranio, E. L., et al. (2017). Effect of high fat diet on phenotype, brain transcriptome and lipidome in Alzheimer’s model mice. Sci. Rep. 7:4307. doi: 10.1038/s41598-017-04412-2
O’Brien, D. P., Johnson, G. S., Schnabel, R. D., Khan, S., Coates, J. R., Johnson, G. C., et al. (2005). Genetic mapping of canine multiple system degeneration and ectodermal dysplasia loci. J. Hered. 96, 727–734. doi: 10.1093/jhered/esi086
O’Mahony, S. M., Hyland, N. P., Dinan, T. G., and Cryan, J. F. (2011). Maternal separation as a model of brain-gut axis dysfunction. Psychopharmacology 214, 71–88. doi: 10.1007/s00213-010-2010-9
Oli, M. W., Otoo, H. N., Crowley, P. J., Heim, K. P., Nascimento, M. M., Ramsook, C. B., et al. (2012). Functional amyloid formation by streptococcus mutans. Microbiology 158, 2903–2916. doi: 10.1099/mic.0.060855-0
Olson, H., Betton, G., Robinson, D., Thomas, K., Monro, A., Kolaja, G., et al. (2000). Concordance of the toxicity of pharmaceuticals in humans and in animals. Regul. Toxicol. Pharmacol. 32, 56–67. doi: 10.1006/rtph.2000.1399
Patel, A., Malinovska, L., Saha, S., Wang, J., Alberti, S., Krishnan, Y., et al. (2017). ATP as a biological hydrotrope. Science 356, 753–756. doi: 10.1126/science.aaf6846
Patronek, G. J., Waters, D. J., and Glickman, L. T. (1997). Comparative longevity of pet dogs and humans: implications for gerontology research. J. Gerontol. A Biol. Sci. Med. Sci. 52, B171–B178. doi: 10.1093/gerona/52a.3.b171
Paula-Lima, A. C., Brito-Moreira, J., and Ferreira, S. T. (2013). Deregulation of excitatory neurotransmission underlying synapse failure in Alzheimer’s disease. J. Neurochem. 126, 191–202. doi: 10.1111/jnc.12304
Perlman, R. L. (2016). Mouse models of human disease. Evol. Med. Public Health 2016, 170–176. doi: 10.1093/emph/eow014
Pistollato, F., Cano, S. S., Elio, I., Vergara, M. M., Giampieri, F., and Battino, M. (2016). Role of gut microbiota and nutrients in amyloid formation and pathogenesis of Alzheimer disease. Nutr. Rev. 74, 624–634. doi: 10.1093/nutrit/nuw023
Prigione, A., Isaias, I. U., Galbussera, A., Brighina, L., Begni, B., Andreoni, S., et al. (2009). Increased oxidative stress in lymphocytes from untreated Parkinson’s disease patients. Parkinsonism Relat. Disord. 15, 327–328. doi: 10.1016/j.parkreldis.2008.05.013
Pugliese, M., Carrasco, J. L., Gomez-Anson, B., Andrade, C., Zamora, A., Rodríguez, M. J., et al. (2010). Magnetic resonance imaging of cerebral involutional changes in dogs as markers of aging: an innovative tool adapted from a human visual rating scale. Vet. J. 186, 166–171. doi: 10.1016/j.tvjl.2009.08.009
Ravussin, Y., Koren, O., Spor, A., LeDuc, C., Gutman, R., Stombaugh, J., et al. (2012). Responses of gut microbiota to diet composition and weight loss in lean and obese mice. Obesity Silver Spring 20, 738–747. doi: 10.1038/oby.2011.111
Rhee, S. H., Pothoulakis, C., and Mayer, E. A. (2009). Principles and clinical implications of the brain-gut-enteric microbiota axis. Nat. Rev. Gastroenterol. Hepatol. 6, 306–314. doi: 10.1038/nrgastro.2009.35
Rocha, N. P., de Miranda, A. S., and Teixeira, A. L. (2015). Insights into neuroinflammation in Parkinson’s disease: from biomarkers to anti-inflammatory based therapies. Biomed Res. Int. 2015:628192. doi: 10.1155/2015/628192
Rofina, J. E., van Ederen, A. M., Toussaint, M. J. M., Secrève, M., van der Spek, A., van der Meer, I., et al. (2006). Cognitive disturbances in old dogs suffering from the canine counterpart of Alzheimer’s disease. Brain Res. 1069, 216–226. doi: 10.1016/j.brainres.2005.11.021
Rosenfeld, C. S. (2015). Microbiome disturbances and autism spectrum disorders. Drug Metab. Dispos. 43, 1557–1571. doi: 10.1124/dmd.115.063826
Roth, G., and Dicke, U. (2005). Evolution of the brain and intelligence. Trends Cogn. Sci. 9, 250–257. doi: 10.1016/j.tics.2005.03.005
Rubin, L. L., Hall, D. E., Porter, S., Barbu, K., Cannon, C., Horner, H. C., et al. (1991). A cell culture model of the blood-brain barrier. J. Cell Biol. 115, 1725–1735. doi: 10.1083/jcb.115.6.1725
Sakakibara, R., Odaka, T., Uchiyama, T., Asahina, M., Yamaguchi, K., Yamaguchi, T., et al. (2003). Colonic transit time and rectoanal videomanometry in Parkinson’s disease. J. Neurol. Neurosurg. Psychiatry 74, 268–272. doi: 10.1136/jnnp.74.2.268
Sampson, T. R., Debelius, J. W., Thron, T., Janssen, S., Shastri, G. G., Ilhan, Z. E., et al. (2016). Gut Microbiota regulate motor deficits and neuroinflammation in a model of Parkinson’s disease. Cell 167, 1469.e12–1480.e12. doi: 10.1016/j.cell.2016.11.018
Santaguida, S., Janigro, D., Hossain, M., Oby, E., Rapp, E., and Cucullo, L. (2006). Side by side comparison between dynamic versus static models of blood-brain barrier in vitro: a permeability study. Brain Res. 1109, 1–13. doi: 10.1016/j.brainres.2006.06.027
Sato, T., and Clevers, H. (2013). Growing self-organizing mini-guts from a single intestinal stem cell: mechanism and applications. Science 340, 1190–1194. doi: 10.1126/science.1234852
Saulnier, D. M., Ringel, Y., Heyman, M. B., Foster, J. A., Bercik, P., Shulman, R. J., et al. (2013). The intestinal microbiome, probiotics and prebiotics in neurogastroenterology. Gut Microbes 4, 17–27. doi: 10.4161/gmic.22973
Scheperjans, F., Aho, V., Pereira, P. A. B., Koskinen, K., Paulin, L., Pekkonen, E., et al. (2015). Gut microbiota are related to Parkinson’s disease and clinical phenotype. Mov. Disord. 30, 350–358. doi: 10.1002/mds.26069
Schütt, T., Helboe, L., Pedersen, L. Ø., Waldemar, G., Berendt, M., and Pedersen, J. T. (2016). Dogs with cognitive dysfunction as a spontaneous model for early Alzheimer’s disease: a translational study of neuropathological and inflammatory markers. J. Alzheimers Dis. 52, 433–449. doi: 10.3233/JAD-151085
Schütt, T., Toft, N., and Berendt, M. (2015). Cognitive function, progression of age-related behavioral changes, biomarkers, and survival in dogs more than 8 years old. J. Vet. Intern. Med. 29, 1569–1577. doi: 10.1111/jvim.13633
Schwartz, K., and Boles, B. R. (2013). Microbial amyloids—functions and interactions within the host. Curr. Opin. Microbiol. 16, 93–99. doi: 10.1016/j.mib.2012.12.001
Schwiertz, A., Spiegel, J., Dillmann, U., Grundmann, D., Bürmann, J., Faßbender, K., et al. (2018). Fecal markers of intestinal inflammation and intestinal permeability are elevated in Parkinson’s disease. Parkinsonism Relat. Disord. 50, 104–107. doi: 10.1016/j.parkreldis.2018.02.022
Shannon, K. M., Keshavarzian, A., Mutlu, E., Dodiya, H. B., Daian, D., Jaglin, J. A., et al. (2012). α-synuclein in colonic submucosa in early untreated Parkinson’s disease. Mov. Disord. 27, 709–715. doi: 10.1002/mds.23838
Shepherd, A., Zhang, T. D., Zeleznikow-Johnston, A. M., Hannan, A. J., and Burrows, E. L. (2018). Transgenic mouse models as tools for understanding how increased cognitive and physical stimulation can improve cognition in Alzheimer’s disease. Brain Plast. 4, 127–150. doi: 10.3233/bpl-180076
Shin, W., Wu, A., Massidda, M. W., Foster, C., Thomas, N., Lee, D.-W., et al. (2019). A robust longitudinal co-culture of obligate anaerobic gut microbiome with human intestinal epithelium in an anoxic-oxic interface-on-a-chip. Front. Bioeng. Biotechnol. 7:13. doi: 10.3389/fbioe.2019.00013
Shin, W., and Kim, H. J. (2018). Intestinal barrier dysfunction orchestrates the onset of inflammatory host-microbiome cross-talk in a human gut inflammation-on-a-chip. Proc. Natl. Acad. Sci. U S A 115, E10539–E10547. doi: 10.1073/pnas.1810819115
Smolek, T., Madari, A., Farbakova, J., Kandrac, O., Jadhav, S., Cente, M., et al. (2016). Tau hyperphosphorylation in synaptosomes and neuroinflammation are associated with canine cognitive impairment. J. Comp. Neurol. 524, 874–895. doi: 10.1002/cne.23877
Sokoloff, L. (1973). Metabolism of ketone bodies by the brain. Annu. Rev. Med. 24, 271–280. doi: 10.1146/annurev.me.24.020173.001415
Stefanko, D. P., Barrett, R. M., Ly, A. R., Reolon, G. K., and Wood, M. A. (2009). Modulation of long-term memory for object recognition via HDAC inhibition. Proc. Natl. Acad. Sci. U S A 106, 9447–9452. doi: 10.1073/pnas.0903964106
Sun, T., and Hevner, R. F. (2014). Growth and folding of the mammalian cerebral cortex: from molecules to malformations. Nat. Rev. Neurosci. 15, 217–232. doi: 10.1038/nrn3707
Su, M.-Y., Tapp, P. D., Vu, L., Chen, Y.-F., Chu, Y., Muggenburg, B., et al. (2005). A longitudinal study of brain morphometrics using serial magnetic resonance imaging analysis in a canine model of aging. Prog. Neuropsychopharmacol. Biol. Psychiatry 29, 389–397. doi: 10.1016/j.pnpbp.2004.12.005
Sung, J. H., and Shuler, M. L. (2009). Prevention of air bubble formation in a microfluidic perfusion cell culture system using a microscale bubble trap. Biomed. Microdevices 11, 731–738. doi: 10.1007/s10544-009-9286-8
Sung, J. H., Yu, J., Luo, D., Shuler, M. L., and March, J. C. (2011). Microscale 3-D hydrogel scaffold for biomimetic gastrointestinal (GI) tract model. Lab Chip 11, 389–392. doi: 10.1039/c0lc00273a
Tan, A. H., Mahadeva, S., Thalha, A. M., Gibson, P. R., Kiew, C. K., Yeat, C. M., et al. (2014). Small intestinal bacterial overgrowth in Parkinson’s disease. Parkinsonism Relat. Disord. 20, 535–540. doi: 10.1016/j.parkreldis.2014.02.019
Terao, M., Kurosaki, M., Barzago, M. M., Varasano, E., Boldetti, A., Bastone, A., et al. (2006). Avian and canine aldehyde oxidases. Novel insights into the biology and evolution of molybdo-flavoenzymes. J. Biol. Chem. 281, 19748–19761. doi: 10.1074/jbc.m600850200
Tilg, H., and Moschen, A. R. (2014). Microbiota and diabetes: an evolving relationship. Gut 63, 1513–1521. doi: 10.1136/gutjnl-2014-306928
Tsigos, C., and Chrousos, G. P. (2002). Hypothalamic-pituitary-adrenal axis, neuroendocrine factors and stress. J. Psychosom. Res. 53, 865–871. doi: 10.1016/S0022-3999(02)00429-4
Vangay, P., Ward, T., Gerber, J. S., and Knights, D. (2015). Antibiotics, pediatric dysbiosis, and disease. Cell Host Microbe 17, 553–564. doi: 10.1016/j.chom.2015.04.006
Vecsey, C. G., Hawk, J. D., Lattal, K. M., Stein, J. M., Fabian, S. A., Attner, M. A., et al. (2007). Histone deacetylase inhibitors enhance memory and synaptic plasticity via CREB: CBP-dependent transcriptional activation. J. Neurosci. 27, 6128–6140. doi: 10.1523/jneurosci.0296-07.2007
Wang, Y. I., Abaci, H. E., and Shuler, M. L. (2017). Microfluidic blood-brain barrier model provides in vivo-like barrier properties for drug permeability screening. Biotechnol. Bioeng. 114, 184–194. doi: 10.1002/bit.26045
Wong, Y. C., and Krainc, D. (2017). α-synuclein toxicity in neurodegeneration: mechanism and therapeutic strategies. Nat. Med. 23, 1–13. doi: 10.1038/nm.4269
Wang, J., Wang, T., Sun, Y., Feng, Y., Kisseberth, W. C., Henry, C. J., et al. (2018). Proliferative and invasive colorectal tumors in pet dogs provide unique insights into human colorectal cancer. Cancers 10:E330. doi: 10.3390/cancers10090330
Wu, S.-C., Cao, Z.-S., Chang, K.-M., and Juang, J.-L. (2017). Intestinal microbial dysbiosis aggravates the progression of Alzheimer’s disease in Drosophila. Nat. Commun. 8:24. doi: 10.1038/s41467-017-00040-6
Yoshioka, H., Takeda, T., Higuchi, K., Ohshio, G., Miyake, T., Sugiyama, T., et al. (1990). Immunohistochemical examination of Peyer’s patches in senescence-accelerated mice. Autoimmunity 8, 25–35. doi: 10.3109/08916939008998429
Yu, C.-H., Song, G.-S., Yhee, J.-Y., Kim, J.-H., Im, K.-S., Nho, W.-G., et al. (2011). Histopathological and immunohistochemical comparison of the brain of human patients with Alzheimer’s disease and the brain of aged dogs with cognitive dysfunction. J. Comp. Pathol. 145, 45–58. doi: 10.1016/j.jcpa.2010.11.004
Zhang, L., Fitzloff, J. F., Engel, L. C., and Cook, C. S. (2001). Species difference in stereoselective involvement of CYP3A in the mono-N-dealkylation of disopyramide. Xenobiotica 31, 73–83. doi: 10.1080/00498250110037488
Zhang, T., Han, Y., Wang, J., Hou, D., Deng, H., Deng, Y. L., et al. (2018). Comparative epidemiological investigation of Alzheimer’s disease and colorectal cancer: the possible role of gastrointestinal conditions in the pathogenesis of AD. Front. Aging Neurosci. 10:176. doi: 10.3389/fnagi.2018.00176
Keywords: gut-brain axis, neurodegenerative disease, canine, translational, animal models, review
Citation: Ambrosini YM, Borcherding D, Kanthasamy A, Kim HJ, Willette AA, Jergens A, Allenspach K and Mochel JP (2019) The Gut-Brain Axis in Neurodegenerative Diseases and Relevance of the Canine Model: A Review. Front. Aging Neurosci. 11:130. doi: 10.3389/fnagi.2019.00130
Received: 25 March 2019; Accepted: 16 May 2019;
Published: 18 June 2019.
Edited by:
Jorge Busciglio, University of California, Irvine, United StatesReviewed by:
Julien Rossignol, Central Michigan University, United StatesMichael Lardelli, University of Adelaide, Australia
Copyright © 2019 Ambrosini, Borcherding, Kanthasamy, Kim, Willette, Jergens, Allenspach and Mochel. This is an open-access article distributed under the terms of the Creative Commons Attribution License (CC BY). The use, distribution or reproduction in other forums is permitted, provided the original author(s) and the copyright owner(s) are credited and that the original publication in this journal is cited, in accordance with accepted academic practice. No use, distribution or reproduction is permitted which does not comply with these terms.
*Correspondence: Jonathan P. Mochel, am1vY2hlbEBpYXN0YXRlLmVkdQ==