- 1Laboratoire CHROME (EA 7352), Université de Nîmes, Nîmes, France
- 2CNRS, INSERM U1191, Institut de Génomique Fonctionnelle, University of Montpellier, Montpellier, France
- 3HiLIFE – Neuroscience Center, University of Helsinki, Helsinki, Finland
A bidirectional crosstalk between peripheral players of immunity and the central nervous system (CNS) exists. Hence, blood–brain barrier (BBB) breakdown is emerging as a participant mechanism of dysregulated peripheral–CNS interplay, promoting diseases. Here, we examine the implication of BBB damage in neurodegeneration, linking it to peripheral brain-directed autoantibodies and gut–brain axis mechanisms. As BBB breakdown is a factor contributing to, or even anticipating, neuronal dysfunction(s), we here identify contemporary pharmacological strategies that could be exploited to repair the BBB in disease conditions. Developing neurovascular, add on, therapeutic strategies may lead to a more efficacious pre-clinical to clinical transition with the goal of curbing the progression of neurodegeneration.
Brain Barriers’ Paths, Leaks, and Neurodegeneration
The term neurodegenerative describes a progressive deterioration of the central nervous system (CNS) that is frequently associated with abnormal accumulation of proteins. Importantly, neurofibrillary tau-protein tangles are not only a major sign of Alzheimer’s disease (AD) but are reported in temporal lobe epilepsy and post-traumatic encephalopathies (Tai et al., 2016). Among the emerging disease mechanisms, a peripheral–CNS pathological interplay is proposed to contribute to the neurodegenerative process (Marchi et al., 2014; Engelhardt et al., 2017; Fung et al., 2017; Pavlov and Tracey, 2017; Prinz and Priller, 2017; Le Page et al., 2018). Accordingly, harmful events occurring at the cerebrovascular interface are being examined as key determinants partaking to or even preceding neurodegeneration (Zlokovic, 2011; Nation et al., 2019; Sweeney et al., 2019). At the cerebrovasculature, specialized endothelial cells, mural cells, and astroglia constructs (Abbott et al., 2010; Giannoni et al., 2018; Sweeney et al., 2019) provide physical and biological properties governing the homeostatic–immune interactions between peripheral blood cells, or molecules, and brain neuroglia. The physiological parenchymal milieu composition ensures a healthy neuronal transmission, attainable because of the tightness of the blood–brain barrier (BBB; Zlokovic, 2008; Giannoni et al., 2018; Nation et al., 2019). At the pial arterial and venous level, the cerebrovasculature is permissive to blood cells or molecules, while it becomes impermeable at the arteriole–capillary level where barriers’ properties are fully established (Abbott et al., 2010). BBB vessels also contribute to cerebrospinal and interstitial fluid movements and the elimination of waste products from the interstitial and perivascular spaces (Noé and Marchi, 2019).
It is increasingly recognized that a BBB pathological imprint can provoke a brain pro-inflammatory disequilibrium sufficient to modify neuronal activity in the long term (Marchi et al., 2007, 2014; Nation et al., 2019). Vascular-dependent mechanisms of neurodegeneration can rapidly elicit as a consequence of peripheral infections, head trauma, ischemic stroke, or status epilepticus (Figure 1; Nation et al., 2019; Sweeney et al., 2019). These are risk factors for the development of long-term neurodegenerative sequelae and encephalopathies (e.g., post-concussion or head trauma-related chronic traumatic encephalopathy, CTE), cerebral amyloid angiopathy (CAA), AD, and epilepsy. Under conditions of increased BBB permeability, an aberrant bidirectional exchange between the neurovascular unit and the peripheral blood occurs, compounding to neurodegenerative modifications (Figure 1; Marchi et al., 2014; Engelhardt et al., 2017; Fung et al., 2017; Pavlov and Tracey, 2017; Prinz and Priller, 2017; Le Page et al., 2018). Completing a vicious cycle, beta-amyloid deposition in the brain can provoke capillaries dysfunction (Thomas et al., 1996; Zhang et al., 1997; Iadecola et al., 1999; Deane et al., 2003, 2012; Nortley et al., 2019). As an example, reactive oxygen species and endothelin-1 production were proposed to elicit vasoconstriction at pericyte locations (Nortley et al., 2019). A question remains regarding whether the endothelin-1 mechanism can directly drive neurodegeneration.
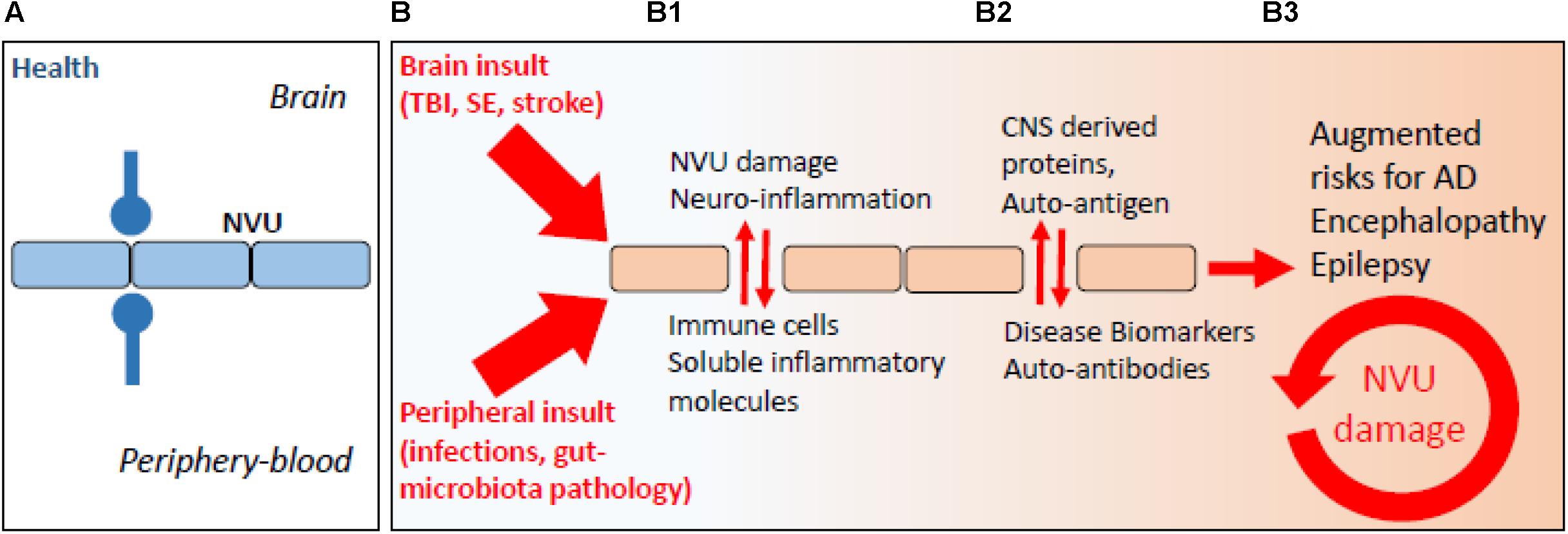
Figure 1. The periphery–brain interplay and CNS disease: the neurovascular pathological imprint. (A) Proper peripheral–brain segregation under healthy conditions (neurovascular unit, NVU; blue lines). (B) Pathological insult(s) elicited in the periphery or in the brain (traumatic brain injury, TBI; status epilepticus, SE) converge to NVU damage (e.g., BBB permeability) and neuro-inflammation, leading to temporary or prolonged loss of brain homeostatic control (B1, red arrows). (B2) Under conditions of BBB permeability, concentration gradients favor brain-derived proteins to extravasate into the peripheral blood. Under this condition, a peripheral auto-immune reaction may mount leading to the production of autoantibodies, possibly re-entering into the CNS if BBB damage endures (B3).
Autoantibodies and Neurodegeneration: Bad, Good, or Nil?
The communication between the peripheral blood and the brain occurs at preferential cerebrovascular sites (Zlokovic, 2011; Noé and Marchi, 2019), e.g., at post-capillary venules or pial vessels, and by a system of lymphatic vessels draining the cerebrospinal and interstitial fluids to cervical lymph nodes (Aspelund et al., 2015; Louveau et al., 2015a, b, 2018). At the intravascular level, moving leukocytes shape a peripheral–brain immune dialog where endothelium activation or permeability, perivascular immune cell homing, and brain entry of immune soluble factors prompt and sustain neuroglia inflammation [Figure 1; see Engelhardt et al. (2017) and Ransohoff (2016) for fundamental aspects of endothelial–leukocyte adhesion]. The implication of the cerebrovascular interface to innate and adaptive modalities of immunity is central (Schwartz and Shechter, 2010; Sommer et al., 2017). Adaptive immunity to the brain requires T- and B-cell stimulation at extra-CNS lymphatic organs and by professional antigen-presenting cells (Janeway et al., 2001), thus implying the existence of a peripheral–brain immune dialog, e.g., via the CNS vascular and lymphatic routes (Noé and Marchi, 2019).
A question exists on whether neurodegeneration may result from autoimmune-like processes (Table 1). Contingent to a prolonged or recurrent BBB permeability, specific antigens could exit the brain to reach the bloodstream, mounting a peripheral humoral response. Newly formed brain-directed autoantibodies could be neuropathological upon their entry into the brain across a continuously damaged BBB (Levin et al., 2010). Importantly, autoantibodies and autoreactive T cells were reported in the cerebrospinal fluid (CSF), sera, as well as in the brain of AD patients and experimental models of disease (Table 1; Kronimus et al., 2016; Wu and Li, 2016). Anti-Aβ antibodies (Ig type G) correlated with scores of dementia (Wilson et al., 2009). Intrathecal antibodies against tau filaments were reported in AD patients (Mruthinti et al., 2004) and were proposed as contributors of disease progression (Bartos et al., 2012). Anti-tau autoantibodies are not specific to AD as they are increased in patients suffering from other neurodegenerative diseases, e.g., multiple sclerosis (Fialová et al., 2011).
The significance of peripheral autoantibodies as biomarkers of neurodegenerative conditions also remains to be established. Autoantibodies against the glutamate receptor N-methyl-D-aspartate receptor (NMDAR) were detected in plasma of AD patients (Davydova et al., 2007). Levels of antibodies were shown to correlate with clinical severity, as patients affected by moderate and severe dementia presented a twofold autoantibody increase compared with patients suffering from mild dementia (Davydova et al., 2007). The presence of autoantibodies against 5-HT was also reported (Myagkova et al., 2001), with levels increasing during the mild phase of the disease, subsequently reaching a plateau (Myagkova et al., 2001). Similar findings were reported for autoantibodies directed against the receptor for advanced glycation end products (Wilson et al., 2009). In a transgenic model of AD, autoantibodies against the sphingolipid ceramide correlated with amyloid plaque increase (Posse de Chaves and Sipione, 2010; Dinkins et al., 2015). Autoantibodies against ATP synthase (Vacirca et al., 2012), α(1)-adrenergic, and the β(2)-adrenergic receptors were also reported (Karczewski et al., 2012). Autoantibodies against the α(1)-adrenergic and the β(2)-adrenergic receptors may contribute to vascular lesions and increased plaque formation in AD patients (Karczewski et al., 2012).
Importantly, not all autoantibodies are harmful. Brain-reactive natural autoantibodies (NAbs) are protective (Britschgi et al., 2009; Kellner et al., 2009; Dodel et al., 2011; Bach and Dodel, 2012). NAbs are mostly IgM and are spontaneously produced. NAbs are polyreactive with low affinity for self-antigens (Casali and Schettino, 1996). Physiologically, NAbs facilitate phagocytosis of apoptotic cells, inhibit inflammatory pathways, and have a role in maintaining immune tolerance (Elkon and Silverman, 2012). NAbs to Aβ can inhibit plaque aggregation, block Aβ toxicity, and catalyze Aβ clearance (Lindhagen-Persson et al., 2010). Immunotherapies using specific, or aspecific, autoantibodies were tested. Bapineuzumab is the humanized form of a monoclonal anti-Aβ antibody targeting the N-terminus of Aβ. In phase II trials, Bapineuzumab administration reduced Aβ plaques in AD brains (Salloway et al., 2009; Rinne et al., 2010) and was associated with decreased total and phospho-tau levels in the CSF (Asuni et al., 2007). Bapineuzumab was, however, discontinued after a phase III trial and showed no beneficial effects on cognitive or functional outcomes (U.S. National Library of Medicine, 2019a, b). Aducanumab (BIIB037) is a human monoclonal antibody selectively targeting aggregated Aβ (oligomers and fibrils) (Sevigny et al., 2016). An Aducanumab phase III trial was terminated as endpoints were not meet. The analysis of a larger data set is ongoing. Tau immunotherapies are also being developed, attenuating or preventing functional impairment in experimental models, as reviewed in Sigurdsson (2018).
Autoantibodies and Post-Traumatic Encephalopathy
Resulting from repeated head trauma and BBB damage, chronic traumatic encephalopathy (CTE) presents with accumulation of neurofibrillary tau-protein tangles. In TBI subjects, blood and CSF autoantibodies were suggested as etiological components or as possible biomarkers of neurodegeneration (Raad et al., 2014; Kobeissy, 2015; Table 1). Anti-glial fibrillary acidic protein (GFAP) fragments were found in the sera of TBI patients (Zhang et al., 2014). Serum autoantibodies against S100B were reported in American football players when repeated sub-concussive events were associated with BBB damage (Marchi et al., 2013). Autoantibodies against the neuronal α7-subunit of the acetylcholine receptor (Sorokina et al., 2011) as well as AMPA and NMDA receptors (Goryunova et al., 2007) were detected in TBI subjects, while IgG autoantibodies to neurons and basal lamina were reported in rat serum following experimental head trauma (Rudehill et al., 2006). Autoantibodies to the pituitary gland were reported in TBI subjects 3 years after the trauma (Tanriverdi et al., 2008, 2010). Damage to the pituitary gland is distinctive of the TBI pathology with 20–50% of patients showing some degrees of pituitary dysfunction, which affects growth hormone production (Aimaretti et al., 2005; Tanriverdi et al., 2006). An association between anti-pituitary autoantibodies and pituitary dysfunction was reported in patients suffering from mild TBI, including repetitive concussions (Tanriverdi et al., 2010).
Autoreactive antibodies have been proposed for the treatment of TBI sequelae. The presence of hyper-phosphorylated tau accumulating in neurofibrillary tangles is a characteristic of CTE (Omalu et al., 2010). Even if phospho-tau is detectable only at low levels acutely after TBI (Smith et al., 2003; Blennow et al., 2012; Goldstein et al., 2012; Mannix et al., 2013), a specific form of phospho-tau can be produced in response to TBI (cis P-tau) (Kondo et al., 2015). This protein spreads throughout the brain, harming cells and leading to post-traumatic neurodegeneration and dementia. In two animal models of TBI, administration of a monoclonal antibody discriminating between the cis and the trans forms of the protein and blocking cis P-tau prevented the onset of tauopathy and cortical atrophy. These accumulating evidence supports the possible involvement of autoantibodies in post-TBI neurodegenerative conditions, perhaps providing new disease biomarkers and therapeutic entry points.
The Gut–Brain Axis and Neurodegeneration: Is There a Barrier Implication?
Here, we discuss a specific framework where alterations of the gut microbiota (GM) could impact BBB permeability, promote neuro-inflammation, and favor neurodegenerative modifications (Figure 2; Braniste et al., 2014; Cerovic et al., 2019; Parker et al., 2019; Wang et al., 2019). Bacteria, viruses, parasites, and non-pathogenic fungi constitute the intestinal microbiota. These complex communities of microbes colonizing the gastrointestinal tract are major players in health. Modern life and diets have progressively induced changes in the composition of the GM, perhaps for the worse, as this can contribute to chronic illnesses (Lozupone et al., 2012; Myles, 2014; Kumar and Forster, 2017; Shanahan et al., 2017; Cryan et al., 2019; Pagliai et al., 2019; Reza et al., 2019). Intestinal microbes can influence brain function through a continuous dialog involving the immune, the vascular, and the nervous systems (Figure 2; Schroeder and Bäckhed, 2016; Cox and Weiner, 2018; Butler et al., 2019; Cryan et al., 2019). Modifications in the composition of the GM was reported in brain disorders, such as autism (Adams et al., 2011; Kang et al., 2019), depression (Kelly et al., 2016; Zheng et al., 2016), Parkinson’s disease (Scheperjans et al., 2015; Sampson et al., 2016), and AD (Cattaneo et al., 2017; Vogt et al., 2017; Zhuang et al., 2018). Intriguingly, the extent of the amyloid pathology in AD mice appears to be dependent of the microbial status, which is specific to the animal housing facility. APP/PS1 mice bred in a germ-free facility displays decreased amyloid plaque number compared to mice housed in non-germ-free conditions (Harach et al., 2017). Moreover, the administration of broad-spectrum, combinatorial antibiotics to APP/PS1 mice, either during the peri-natal or the adult stage, reduced brain Aβ deposition (Minter et al., 2016, 2017).
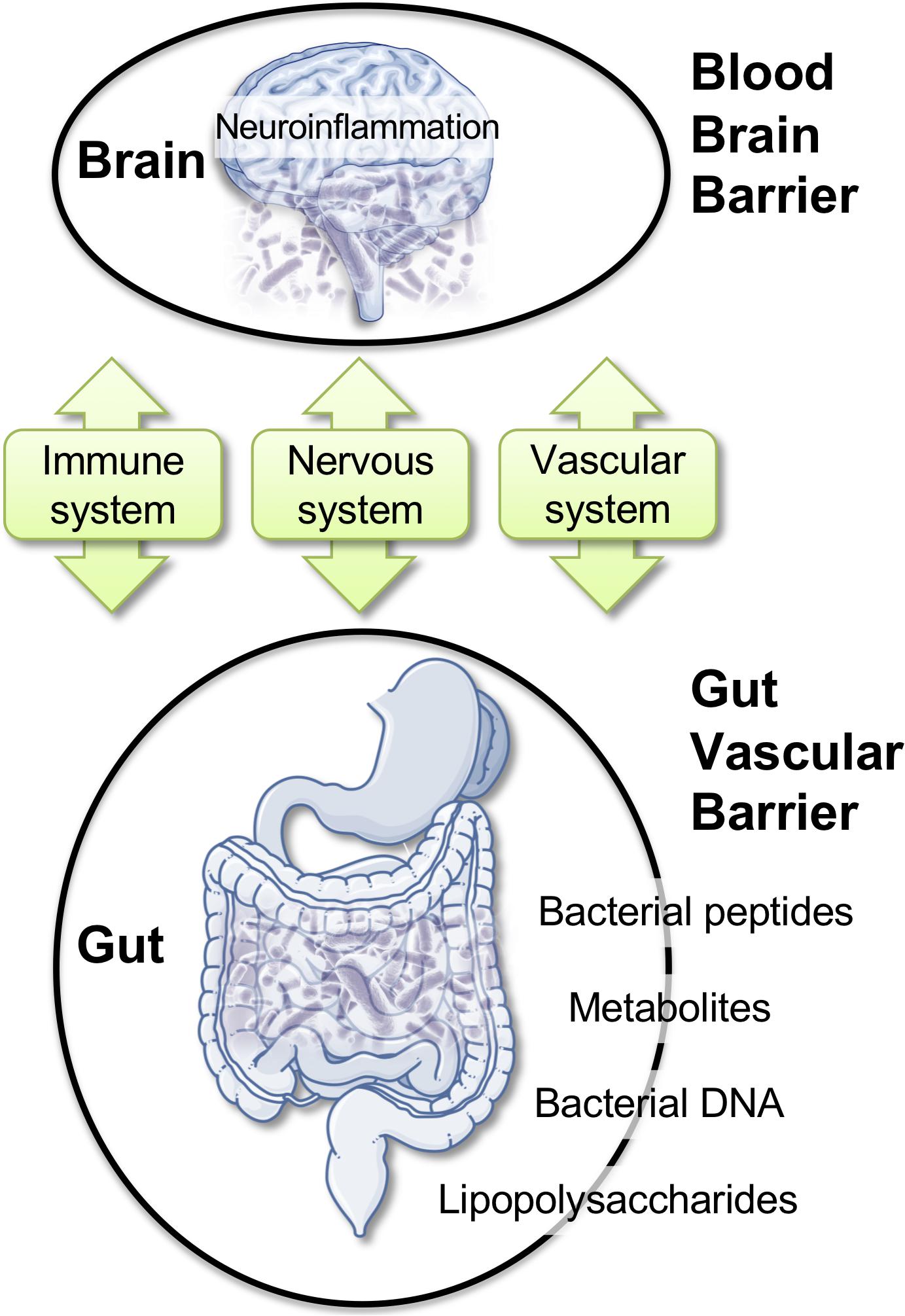
Figure 2. Gut–brain axis: communication routes and physiological barriers. A double, peripheral, and brain homeostatic control is performed by the intestinal–epithelial and blood–brain barriers under healthy conditions. Rupture of one barrier (e.g., gut) may impact the other (e.g., brain), with the blood stream and the immune system being the facilitators or the arbitrators of the pathological spread and neuro-inflammation.
Existing reports support the hypothesis of a possible infectious origin of AD. Aβ was proposed as an antimicrobial peptide (Soscia et al., 2010; Moir et al., 2018) responding to pathogens (Kumar et al., 2016; Eimer et al., 2018). Infectious agents, such as Chlamydia pneumonia, Proprionibacterium acne, Helicobacter pylori, Porphyromonas gingivalis, or spirochetes, are associated with AD (Kornhuber, 1996; Balin et al., 1998; Kountouras et al., 2006; Miklossy, 2011; Poole et al., 2015). A microbial hypothesis is supported by evidence describing the presence of viruses, such as Herpes simplex virus type I, in the brains of AD patients (Lin et al., 2002; Alonso et al., 2014; Itzhaki et al., 2016).
Within the complex interplay between the gut microbiome and the CNS, a role for brain barriers and neuroinflammation is becoming important (Braniste et al., 2014; Cerovic et al., 2019; Parker et al., 2019; Wang et al., 2019). The impact of the gut microbiome composition on CNS health was reported (Amedei and Boem, 2018; Chu et al., 2019; Sherwin et al., 2019; Virtue et al., 2019). Recent work demonstrated that GM composition controls BBB development and permeability in mice (Braniste et al., 2014). In AD, increased gut permeability due to GM dysbiosis was reported during prolonged stress. In this condition, molecules that are normally secluded in the intestine, e.g., inflammatory mediators, bacteria, or bacterial-derived agents, could leak out and reach the peripheral blood. Bacterial DNA, metabolites, or proteins circulating in the blood stream could, in turn, modify BBB permeability (Braniste et al., 2014; Myles, 2014; Kumar and Forster, 2017; Cerovic et al., 2019; Parker et al., 2019; Wang et al., 2019). Existing reports indicated bacterial DNA in human blood with a possibility for brain access (Lelouvier et al., 2016; Païssé et al., 2016; Schierwagen et al., 2018). Brain entry of P. gingivalis, a bacterium associated with periodontal disease, has been described (Dominy et al., 2019). Gingipain inhibitors reduced the bacterial load and the bacteria-induced neuro-inflammation in a mouse model (Dominy et al., 2019). Among Spirochetes, Borrelia burgdorferi is a strain associated with Lyme dementia that could enter the brain. In humans, this specific strain can form biofilms similar to senile plaques. Aβ and bacterial DNA appear as important constituents of these biofilms, suggesting that amyloid plaques may originate in association with or from the spirochetal colonies (Allen, 2016; Miklossy, 2016).
These examples highlight the need of tightly regulated intestinal and brain barriers (Rahman et al., 2018). In AD, a dysbiotic GM may enhance gut permeability and alter BBB integrity, allowing the access of infectious agents or associated molecules into the brain (Martin et al., 2018). Significantly, intestinal and brain barriers are reactive to analogous pro-inflammatory triggers. Circulating inflammatory cytokines IL-17, interferon-gamma (IFN-γ), and the small intestine epithelium protein zonulin can damage the intestinal–epithelia and BBBs (Rahman et al., 2018).
Gut Microbiota and Autoantibodies: Initial Clues
Hypotheses linking modifications of the GM and production of autoantibodies are emerging (Petta et al., 2018). Some evidence supports the concept that specific dietary components may affect B-cell maturation and activity, ultimately leading to the formation of autoantibodies (Petta et al., 2018). Obesity was associated with a systemic pro-inflammatory state, characterized by changes in the frequency of B-cell subpopulation [e.g., reduction of the anti-inflammatory IL-10+ regulatory B cell (Nishimura et al., 2013)] and by an increase in autoantibody levels (Kosaraju et al., 2017). Diets rich in polyunsaturated fatty acid are associated with the suppression of pro-inflammatory responses and a reduction of circulating autoantibodies (Pestka et al., 2014; Tomasdottir et al., 2014). Dietary components impact the composition of the gastrointestinal bacterial populations: consumption of prebiotics increases the intestinal levels of Bifidobacterium and Lactobacillus (Singh et al., 2017), with a possible link to B-cell differentiation, maturation, and activation (Ouwehand et al., 2002). Diet can impact autoantibody production, directly by promoting pro-inflammatory conditions and indirectly by altering the GM. In experimental autoimmune encephalomyelitis (EAE) it was demonstrated that the commensal microbiota composition is a pivotal factor for disease development (Lee et al., 2011) and that modifying the GM impacts the levels of T and B cells or the levels of circulating autoantibodies (Ochoa-Repáraz et al., 2009, 2010).
BBB Repairing Pharmacology: Available Options
The multi-level implication of BBB damage in neurodegenerative disorders has prompted the quest for pharmacological repairing strategies, either directed at the endothelium or by indirect targeting of the cellular players of peripheral and neuro-inflammation. Currently tested drugs are either repurposed or new (Table 2). Examples include losartan, an anti-hypertensive molecule acting as an angiotensin II antagonist. Losartan was shown to reduce BBB permeability in a rat model of hypertension (Kucuk et al., 2002; Kaya et al., 2003) and following pilocarpine-induced status epilepticus (Hong et al., 2019). BBB protection by losartan depends on angiotensin receptor type 1 (AT1) blockade. Another drug is rapamycin, a specific inhibitor of the mammalian target of rapamycin (mTOR) pathway. Rapamycin improved cerebrovascular and cognitive function in a mouse model of AD (Van Skike et al., 2018). Inhibition of mTOR preserved BBB integrity through the upregulation of tight junction proteins and downregulation of matrix metalloproteinase-9. A third option is anakinra, which is the recombinant form of the human IL-1 receptor antagonist (IL1-Ra) that inhibits IL-1α and IL-1β binding to the IL-1 receptor type 1. As inflammation comprises BBB dysfunction, the inhibition of IL-1 as proposed is a strategy enabling cerebrovascular protection (Marchi et al., 2009, 2011; Vezzani et al., 2011; Kenney-Jung et al., 2016). Recent strategies include the development of IL-1Ra molecules fused with a cell-penetrating peptide to enhance brain access (Zhang et al., 2017). After transient middle cerebral artery occlusion in rats, IL-1Ra-PEP reduced neuro-inflammation and ischemia (Zhang et al., 2017). The fourth option is IPW-5371, a small molecule blocking the transforming growth factor β receptor (TGFβR) signaling. In a recent study (Senatorov et al., 2019), IPW reduced hyperexcitability in a mouse model, protecting BBB functions. The activated protein C (APC) therapeutic analog 3K3A-APC is a fifth option. This compound has BBB and neuro-protective properties (Thiyagarajan et al., 2008; Zhong et al., 2009; Wang et al., 2016; Sinha et al., 2018; Lazic et al., 2019; Lyden et al., 2019) and it is in clinical trial for stroke treatment (Lyden et al., 2019). Next is platelet-derived growth factor subunits BB (PDGF-BB). Following an acute vascular insult, activation of the PDGF receptor beta (PDGFRβ) by PDGF-BB is beneficial, protecting the endothelium–pericyte structures. The latter was reported in mouse models of status epilepticus (Arango-Lievano et al., 2018) and cerebral ischemia (Marushima et al., 2019). Conversely, in chronic disease settings (e.g., AD, epilepsy, etc.), activation of PDGFRβ may participate to inflammation (Rustenhoven et al., 2017; Klement et al., 2019). Under this circumstance, blocking PDGFRβ signaling by using the tyrosine kinase inhibitor Imatinib could represent an anti-inflammatory strategy (Rustenhoven et al., 2017; Klement et al., 2019). In general, reducing PDGFRβ signaling could lead to contrasting effects, e.g., pericyte deficiency and BBB breakdown (Bell et al., 2010; Daneman et al., 2010; Armulik et al., 2017; Kisler et al., 2017; Montagne et al., 2018; Nikolakopoulou et al., 2019) or anti-inflammatory (Rustenhoven et al., 2017; Klement et al., 2019), depending on disease stage (acute vs. chronic). Another option, Dexamethasone, is a glucocorticoid effective in the formation and maintenance of endothelial tight junctions (Hue et al., 2015; Na et al., 2017). Dexamethasone was proposed to decrease the expression of the Jumonji Domain Containing 3 gene (JMJD3) and metallo-proteinases (MMP-2, MMP-3, and MMP-9). Finally, there is the vascular endothelial growth factor (VEGF). Amyloid accumulation is associated with endothelial apoptosis (Religa et al., 2013) in Alzheimer’s patients as well as in mouse models. In AD mice, VEGF administration rescued memory deficits by preventing Aβ-induced vascular apoptosis (Religa et al., 2013). See Table 2 for complete drug listing, mechanisms and bibliography.
Perspectives and Challenges
The importance of cerebrovascular dysfunction in neurodegenerative disorders is twofold: BBB damage is pathophysiological and it allows a diagnostic window, the latter by exploiting specific proteins that shed from the damaged or vascular wall cells to appear into accessible fluids, e.g., blood or CSF. For instance, by dosing soluble PDGFRβ in CSF and by using dynamic contrast-enhanced magnetic resonance imaging, a recent study demonstrated BBB breakdown as an early biomarker of human cognitive dysfunction (Montagne et al., 2015; Nation et al., 2019).
Tackling the complex neurodegenerative puzzle requires a continuous sharpening of pharmacological tools. This is important because no efficacious disease-modifying strategy is available to meaningfully delay or prevent disease progression. The problematics here presented may stem from semantic habits as the term neuro- indicates, for most, neurons only. Revisiting nomenclature(s) may benefit, if not legitimize, holistic, and neurovascular approaches to CNS disorders since it is evident that considering neuronal circuits insulated from the influence of glio-vascular cells is excessively reductionist.
Author Contributions
NM planned, drafted, and corrected most of the manuscript, including figures and tables. FN wrote the parts on auto-immunity and created the table. SC was responsible for the section “The Gut-Brain Axis and Neurodegeneration: Is There a Barriers’ Implication?”. PG contributed to the section on BBB drugs and to the table, and also contributed to the sections “Gut Microbiota and Autoantibodies Production: Initial Clues” and “References.”
Conflict of Interest
The authors declare that the research was conducted in the absence of any commercial or financial relationships that could be construed as a potential conflict of interest.
Acknowledgments
This work was supported by the ANR-Epicyte, ANR-HepatoBrain, Era-Net/ANR Neu-Vasc, Fondation de France, FRC, and Muse Grants to NM.
References
Abbott, N. J., Patabendige, A. A. K., Dolman, D. E. M., Yusof, S. R., and Begley, D. J. (2010). Structure and function of the blood-brain barrier. Neurobiol. Dis. 37, 13–25.
Adams, J. B., Johansen, L. J., Powell, L. D., Quig, D., and Rubin, R. A. (2011). Gastrointestinal flora and gastrointestinal status in children with autism–comparisons to typical children and correlation with autism severity. BMC Gastroenterol. 11:22. doi: 10.1186/1471-230X-11-22
Aggarwal, A., Khera, A., Singh, I., and Sandhir, R. (2015). S-nitrosoglutathione prevents blood-brain barrier disruption associated with increased matrix metalloproteinase-9 activity in experimental diabetes. J. Neurochem. 132, 595–608. doi: 10.1111/jnc.12939
Aimaretti, G., Ambrosio, M. R., Di Somma, C., Gasperi, M., Cannavò, S., Scaroni, C., et al. (2005). Residual pituitary function after brain injury-induced hypopituitarism: a prospective 12-month study. J. Clin. Endocrinol. Metab. 90, 6085–6092. doi: 10.1210/jc.2005-0504
Allen, H. B. (2016). Alzheimer’s disease: assessing the role of spirochetes, biofilms, the immune system, and amyloid-β with regard to potential treatment and prevention. J. Alzheimers Dis. 27, 1271–1276. doi: 10.3233/jad-160388
Alonso, R., Pisa, D., Marina, A. I., Morato, E., Rábano, A., and Carrasco, L. (2014). Fungal infection in patients with Alzheimer’s disease. J. Alzheimers Dis. 41, 301–311.
Amedei, A., and Boem, F. (2018). I’ve gut a feeling: microbiota impacting the conceptual and experimental perspectives of personalized medicine. Int. J. Mol. Sci. 19:E3756. doi: 10.3390/ijms19123756
Arango-Lievano, M., Boussadia, B., De Terdonck, L. D. T., Gault, C., Fontanaud, P., Lafont, C., et al. (2018). Topographic reorganization of cerebrovascular mural cells under seizure conditions. Cell Rep. 24, 1045–1059. doi: 10.1016/j.celrep.2018.03.110
Armulik, A., Genové, G., Mäe, M., Nisancioglu, M. H., Wallgard, E., Niaudet, C., et al. (2017). Pericytes regulate the blood-brain barrier. Nature 468, 557–561.
Aspelund, A., Antila, S., Proulx, S. T., Karlsen, T. V., Karaman, S., Detmar, M., et al. (2015). A dural lymphatic vascular system that drains brain interstitial fluid and macromolecules. J. Exp. Med. 212, 991–999. doi: 10.1084/jem.20142290
Asuni, A. A., Boutajangout, A., Quartermain, D., and Sigurdsson, E. M. (2007). Immunotherapy targeting pathological tau conformers in a tangle mouse model reduces brain pathology with associated functional improvements. J. Neurosci. 27, 9115–9129. doi: 10.1523/jneurosci.2361-07.2007
Bach, J.-P., and Dodel, R. (2012). Naturally occurring autoantibodies against β-Amyloid. Adv. Exp. Med. Biol. 750, 91–99. doi: 10.1007/978-1-4614-3461-0_7
Balin, B. J., Gérard, H. C., Arking, E. J., Appelt, D. M., Branigan, P. J., Abrams, J. T., et al. (1998). Identification and localization of Chlamydia pneumoniae in the Alzheimer’s brain. Med. Microbiol. Immunol. 187, 23–42.
Bartos, A., Fialová, L., Svarcová, J., and Ripova, D. (2012). Patients with Alzheimer disease have elevated intrathecal synthesis of antibodies against tau protein and heavy neurofilament. J. Neuroimmunol. 252, 100–105. doi: 10.1016/j.jneuroim.2012.08.001
Bell, R. D., Winkler, E. A., Sagare, A. P., Singh, I., LaRue, B., Deane, R., et al. (2010). Pericytes control key neurovascular functions and neuronal phenotype in the adult brain and during brain aging. Neuron 68, 409–427. doi: 10.1016/j.neuron.2010.09.043
Blennow, K., Hardy, J., and Zetterberg, H. (2012). The neuropathology and neurobiology of traumatic brain injury. Neuron 76, 886–899. doi: 10.1016/j.neuron.2012.11.021
Braniste, V., Al-Asmakh, M., Kowal, C., Anuar, F., Abbaspour, A., Tóth, M., et al. (2014). The gut microbiota influences blood-brain barrier permeability in mice. Sci. Transl. Med. 6:263ra158. doi: 10.1126/scitranslmed.3009759
Brettschneider, S., Morgenthaler, N. G., Teipel, S. J., Fischer-Schulz, C., Bürger, K., Dodel, R., et al. (2005). Decreased serum amyloid beta(1-42) autoantibody levels in Alzheimer’s disease, determined by a newly developed immuno-precipitation assay with radiolabeled amyloid beta(1-42) peptide. Biol. Psychiatry 57, 813–816. doi: 10.1016/j.biopsych.2004.12.008
Britschgi, M., Olin, C. E., Johns, H. T., Takeda-Uchimura, Y., LeMieux, M. C., Rufibach, K., et al. (2009). Neuroprotective natural antibodies to assemblies of amyloidogenic peptides decrease with normal aging and advancing Alzheimer’s disease. Proc. Natl. Acad. Sci. U.S.A. 106, 12145–12150. doi: 10.1073/pnas.0904866106
Busse, S., Busse, M., Brix, B., Probst, C., Genz, A., Bogerts, B., et al. (2014). Seroprevalence of N-methyl-D-aspartate glutamate receptor (NMDA-R) autoantibodies in aging subjects without neuropsychiatric disorders and in dementia patients. Eur. Arch. Psychiatry Clin. Neurosci. 264, 545–550. doi: 10.1007/s00406-014-0493-9
Butler, M. I., Cryan, J. F., and Dinan, T. G. (2019). Man and the microbiome: a new theory of everything? Annu. Rev. Clin. Psychol. 15, 371–398. doi: 10.1146/annurev-clinpsy-050718-095432
Casali, P., and Schettino, E. W. (1996). Structure and function of natural antibodies. Curr. Top. Microbiol. Immunol. 210, 167–179. doi: 10.1007/978-3-642-85226-8_17
Cattaneo, A., Cattane, N., Galluzzi, S., Provasi, S., Lopizzo, N., Festari, C., et al. (2017). Association of brain amyloidosis with pro-inflammatory gut bacterial taxa and peripheral inflammation markers in cognitively impaired elderly. Neurobiol. Aging 49, 60–68. doi: 10.1016/j.neurobiolaging.2016.08.019
Cerovic, M., Forloni, G., and Balducci, C. (2019). Neuroinflammation and the gut microbiota: possible alternative therapeutic targets to counteract Alzheimer’s disease? Front. Aging Neurosci. 11:284. doi: 10.3389/fnagi.2019.00284
Chu, C., Murdock, M. H., Jing, D., Won, T. H., Chung, H., Kressel, A. M., et al. (2019). The microbiota regulate neuronal function and fear extinction learning. Nature 574, 543–548. doi: 10.1038/s41586-019-1644-y
Cox, L. M., and Weiner, H. L. (2018). Microbiota signaling pathways that influence neurologic disease. Neurother. J. Am. Soc. Exp. Neurother. 15, 135–145. doi: 10.1007/s13311-017-0598-8
Cristante, E., McArthur, S., Mauro, C., Maggioli, E., Romero, I. A., Wylezinska-Arridge, M., et al. (2013). Identification of an essential endogenous regulator of blood-brain barrier integrity, and its pathological and therapeutic implications. Proc. Natl. Acad. Sci. U.S.A. 110, 832–841. doi: 10.1073/pnas.1209362110
Cryan, J. F., O’Riordan, K. J., Cowan, C. S. M., Sandhu, K. V., Bastiaanssen, T. F. S., Boehme, M., et al. (2019). The microbiota-gut-brain axis. Physiol. Rev. 99, 1877–2013.
Daneman, R., Zhou, L., Kebede, A. A., and Barres, B. A. (2010). Pericytes are required for blood-brain barrier integrity during embryogenesis. Nature 468, 562–566. doi: 10.1038/nature09513
Davydova, T. V., Voskresenskaya, N. I., Fomina, V. G., Vetrile, L. A., and Doronina, O. A. (2007). Induction of autoantibodies to glutamate in patients with Alzheimer’s disease. Bull. Exp. Biol. Med. 143, 182–183. doi: 10.1007/s10517-007-0044-8
Deane, R., Du Yan, S., Submamaryan, R. K., LaRue, B., Jovanovic, S., Hogg, E., et al. (2003). RAGE mediates amyloid-beta peptide transport across the blood-brain barrier and accumulation in brain. Nat. Med. 9, 907–913. doi: 10.1038/nm890
Deane, R., Singh, I., Sagare, A. P., Bell, R. D., Ross, N. T., LaRue, B., et al. (2012). A multimodal RAGE-specific inhibitor reduces amyloid β-mediated brain disorder in a mouse model of Alzheimer disease. J. Clin. Invest. 122, 1377–1392. doi: 10.1172/jci58642
Dinkins, M. B., Dasgupta, S., Wang, G., Zhu, G., He, Q., Kong, J. N., et al. (2015). The 5XFAD mouse model of Alzheimer’s disease exhibits an age-dependent increase in anti-ceramide IgG and exogenous administration of ceramide further increases anti-ceramide titers and amyloid plaque burden. J. Alzheimers Dis. 46, 55–61. doi: 10.3233/jad-150088
Dodel, R., Balakrishnan, K., Keyvani, K., Deuster, O., Neff, F., Andrei-Selmer, L.-C., et al. (2011). Naturally occurring autoantibodies against beta-amyloid: investigating their role in transgenic animal and in vitro models of Alzheimer’s disease. J. Neurosci. 31, 5847–5854. doi: 10.1523/jneurosci.4401-10.2011
Dominy, S. S., Lynch, C., Ermini, F., Benedyk, M., Marczyk, A., Konradi, A., et al. (2019). Porphyromonas gingivalis in Alzheimer’s disease brains: evidence for disease causation and treatment with small-molecule inhibitors. Sci. Adv. 5:eaau3333. doi: 10.1126/sciadv.aau3333
Du, Y., Dodel, R., Hampel, H., Buerger, K., Lin, S., Eastwood, B., et al. (2001). Reduced levels of amyloid beta-peptide antibody in Alzheimer disease. Neurology 57, 801–805. doi: 10.1212/wnl.57.5.801
Eimer, W. A., Vijaya Kumar, D. K., Navalpur Shanmugam, N. K., Rodriguez, A. S., Mitchell, T., Washicosky, K. J., et al. (2018). Alzheimer’s disease-associated β-amyloid is rapidly seeded by herpesviridae to protect against brain infection. Neuron 11, 56.e3–63.e3.
Elkon, K. B., and Silverman, G. J. (2012). Naturally occurring autoantibodies to apoptotic cells. Adv. Exp. Med. Biol. 750, 14–26. doi: 10.1007/978-1-4614-3461-0_2
Engelhardt, B., Vajkoczy, P., and Weller, R. O. (2017). The movers and shapers in immune privilege of the CNS. Nat. Immunol. 18, 123–131. doi: 10.1038/ni.3666
Fialová, L., Bartos, A., Svarcová, J., and Malbohan, I. (2011). Increased intrathecal high-avidity anti-tau antibodies in patients with multiple sclerosis. PLoS One 6:e27476. doi: 10.1371/journal.pone.0027476
Fung, T. C., Olson, C. A., and Hsiao, E. Y. (2017). Interactions between the microbiota, immune and nervous systems in health and disease. Nat. Neurosci. 20, 145–155. doi: 10.1038/nn.4476
Giannoni, P., Badaut, J., Dargazanli, C., De Maudave, A. F., Klement, W., Costalat, V., et al. (2018). The pericyte-glia interface at the blood-brain barrier. Clin. Sci. Lond. Engl. 14, 361–374. doi: 10.1042/CS20171634
Goldstein, L. E., Fisher, A. M., Tagge, C. A., Zhang, X.-L., Velisek, L., Sullivan, J. A., et al. (2012). Chronic traumatic encephalopathy in blast-exposed military veterans and a blast neurotrauma mouse model. Sci. Transl. Med. 4:134ra60.
Gong, P., Zhang, Z., Zou, Y., Tian, Q., Han, S., Xu, Z., et al. (2019). Tetramethylpyrazine attenuates blood-brain barrier disruption in ischemia/reperfusion injury through the JAK/STAT signaling pathway. Eur. J. Pharmacol. 854, 289–297. doi: 10.1016/j.ejphar.2019.04.028
Goryunova, A. V., Bazarnaya, N. A., Sorokina, E. G., Semenova, N. Y., Globa, O. V., Semenova, Z. B., et al. (2007). Glutamate receptor autoantibody concentrations in children with chronic post-traumatic headache. Neurosci. Behav. Physiol. 37, 761–764. doi: 10.1007/s11055-007-0079-3
Gruden, M. A., Davidova, T. B., Malisauskas, M., Sewell, R. D. E., Voskresenskaya, N. I., Wilhelm, K., et al. (2007). Differential neuroimmune markers to the onset of Alzheimer’s disease neurodegeneration and dementia: autoantibodies to Abeta((25-35)) oligomers, S100b and neurotransmitters. J. Neuroimmunol. 186, 181–192. doi: 10.1016/j.jneuroim.2007.03.023
Gustaw, K. A., Garrett, M. R., Lee, H.-G., Castellani, R. J., Zagorski, M. G., Prakasam, A., et al. (2008). Antigen-antibody dissociation in Alzheimer disease: a novel approach to diagnosis. J. Neurochem. 106, 1350–1356. doi: 10.1111/j.1471-4159.2008.05477.x
Harach, T., Marungruang, N., Duthilleul, N., Cheatham, V., Mc Coy, K. D., Frisoni, G., et al. (2017). Reduction of Abeta amyloid pathology in APPPS1 transgenic mice in the absence of gut microbiota. Sci. Rep. 08:41802.
Hind, W. H., England, T. J., and O’Sullivan, S. E. (2016). Cannabidiol protects an in vitro model of the blood-brain barrier from oxygen-glucose deprivation via PPARγ and 5-HT1A receptors. Br. J. Pharmacol. 173, 815–825. doi: 10.1111/bph.13368
Hong, S., JianCheng, H., JiaWen, W., ShuQin, Z., GuiLian, Z., HaiQin, W., et al. (2019). Losartan inhibits development of spontaneous recurrent seizures by preventing astrocyte activation and attenuating blood-brain barrier permeability following pilocarpine-induced status epilepticus. Brain Res. Bull. 149, 251–259. doi: 10.1016/j.brainresbull.2019.05.002
Hue, C. D., Cho, F. S., Cao, S., Dale Bass, C. R., Meaney, D. F., and Morrison, B. (2015). Dexamethasone potentiates in vitro blood-brain barrier recovery after primary blast injury by glucocorticoid receptor-mediated upregulation of ZO-1 tight junction protein. J. Cereb. Blood Flow Metab. 35, 1191–1198. doi: 10.1038/jcbfm.2015.38
Iadecola, C., Zhang, F., Niwa, K., Eckman, C., Turner, S. K., Fischer, E., et al. (1999). SOD1 rescues cerebral endothelial dysfunction in mice overexpressing amyloid precursor protein. Nat. Neurosci. 2, 157–161. doi: 10.1038/5715
Itzhaki, R. F., Lathe, R., Balin, B. J., Ball, M. J., Bearer, E. L., Braak, H., et al. (2016). Microbes and Alzheimer’s disease. J. Alzheimers Dis. 51, 979–984.
Janeway, C. A., Travers, P., Walport, M., and Shlomchik, M. J. Jr. (2001). Immunobiology, 5th Edn. New York, NY: Garland Science.
Kang, D.-W., Adams, J. B., Coleman, D. M., Pollard, E. L., Maldonado, J., McDonough-Means, S., et al. (2019). Long-term benefit of microbiota transfer therapy on autism symptoms and gut microbiota. Sci. Rep. 9:5821. doi: 10.1038/s41598-019-42183-0
Karczewski, P., Hempel, P., Kunze, R., and Bimmler, M. (2012). Agonistic autoantibodies to the α(1) -adrenergic receptor and the β(2) -adrenergic receptor in Alzheimer’s and vascular dementia. Scand. J. Immunol. 75, 524–530. doi: 10.1111/j.1365-3083.2012.02684.x
Kaya, M., Kalayci, R., Küçük, M., Arican, N., Elmas, I., Kudat, H., et al. (2003). Effect of losartan on the blood-brain barrier permeability in diabetic hypertensive rats. Life Sci. 73, 3235–3244. doi: 10.1016/j.lfs.2003.06.014
Kellner, A., Matschke, J., Bernreuther, C., Moch, H., Ferrer, I., and Glatzel, M. (2009). Autoantibodies against beta-amyloid are common in Alzheimer’s disease and help control plaque burden. Ann. Neurol. 65, 24–31. doi: 10.1002/ana.21475
Kelly, J. R., Borre, Y., O’ Brien, C., Patterson, E., El Aidy, S., Deane, J., et al. (2016). Transferring the blues: depression-associated gut microbiota induces neurobehavioural changes in the rat. J. Psychiatr. Res. 82, 109–118. doi: 10.1016/j.jpsychires.2016.07.019
Kenney-Jung, D. L., Vezzani, A., Kahoud, R. J., LaFrance-Corey, R. G., Ho, M.-L., Muskardin, T. W., et al. (2016). Febrile infection-related epilepsy syndrome treated with anakinra. Ann. Neurol. 80, 939–945. doi: 10.1002/ana.24806
Kisler, K., Nelson, A. R., Rege, S. V., Ramanathan, A., Wang, Y., Ahuja, A., et al. (2017). Pericyte degeneration leads to neurovascular uncoupling and limits oxygen supply to brain. Nat. Neurosci. 20, 406–416. doi: 10.1038/nn.4489
Klement, W., Blaquiere, M., Zub, E., deBock, F., Boux, F., Barbier, E., et al. (2019). A pericyte-glia scarring develops at the leaky capillaries in the hippocampus during seizure activity. Epilepsia 60, 1399–1411. doi: 10.1111/epi.16019
Kobeissy, F. H. (2015). Brain Neurotrauma: Molecular, Neuropsychological, and Rehabilitation Aspects. Boca Raton, FL: CRC Press.
Kondo, A., Shahpasand, K., Mannix, R., Qiu, J., Moncaster, J., Chen, C.-H., et al. (2015). Antibody against early driver of neurodegeneration cis P-tau blocks brain injury and tauopathy. Nature 523, 431–436. doi: 10.1038/nature14658
Kornhuber, H. H. (1996). Propionibacterium acnes in the cortex of patients with Alzheimer’s disease. Eur. Arch. Psychiatry Clin. Neurosci. 246, 108–109. doi: 10.1007/bf02274902
Kosaraju, R., Guesdon, W., Crouch, M. J., Teague, H. L., Sullivan, E. M., Karlsson, E. A., et al. (2017). B cell activity is impaired in human and mouse obesity and is responsive to an essential fatty acid upon murine influenza infection. J. Immunol. 198, 4738–4752. doi: 10.4049/jimmunol.1601031
Kountouras, J., Tsolaki, M., Gavalas, E., Boziki, M., Zavos, C., Karatzoglou, P., et al. (2006). Relationship between Helicobacter pylori infection and Alzheimer disease. Neurology 66, 938–940. doi: 10.1212/01.wnl.0000203644.68059.5f
Kronimus, Y., Albus, A., Balzer-Geldsetzer, M., Straub, S., Semler, E., Otto, M., et al. (2016). Naturally occurring autoantibodies against tau protein are reduced in Parkinson’s disease dementia. PLoS One 11:e0164953. doi: 10.1371/journal.pone.0164953
Kucuk, M., Kaya, M., Kalayci, R., Cimen, V., Kudat, H., Arican, N., et al. (2002). Effects of losartan on the blood-brain barrier permeability in long-term nitric oxide blockade-induced hypertensive rats. Life Sci. 71, 937–946. doi: 10.1016/s0024-3205(02)01772-1
Kumar, D. K. V., Choi, S. H., Washicosky, K. J., Eimer, W. A., Tucker, S., Ghofrani, J., et al. (2016). Amyloid-β peptide protects against microbial infection in mouse and worm models of Alzheimer’s disease. Sci. Transl. Med. 8:340ra72. doi: 10.1126/scitranslmed.aaf1059
Kumar, N., and Forster, S. C. (2017). Genome watch: microbiota shuns the modern world. Nat. Rev. Microbiol. 15, 710–710. doi: 10.1038/nrmicro.2017.136
Lazic, D., Sagare, A. P., Nikolakopoulou, A. M., Griffin, J. H., Vassar, R., and Zlokovic, B. V. (2019). 3K3A-activated protein C blocks amyloidogenic BACE1 pathway and improves functional outcome in mice. J. Exp. Med. 216, 279–293. doi: 10.1084/jem.20181035
Le Page, A., Dupuis, G., Frost, E. H., Larbi, A., Pawelec, G., Witkowski, J. M., et al. (2018). Role of the peripheral innate immune system in the development of Alzheimer’s disease. Exp. Gerontol. 01, 59–66.
Lee, Y. K., Menezes, J. S., Umesaki, Y., and Mazmanian, S. K. (2011). Proinflammatory T-cell responses to gut microbiota promote experimental autoimmune encephalomyelitis. Proc. Natl. Acad. Sci. U.S.A. 108(Suppl. 1), 4615–4622. doi: 10.1073/pnas.1000082107
Lelouvier, B., Servant, F., Païssé, S., Brunet, A.-C., Benyahya, S., Serino, M., et al. (2016). Changes in blood microbiota profiles associated with liver fibrosis in obese patients: a pilot analysis. Hepatology 64, 2015–2027. doi: 10.1002/hep.28829
Levin, E. C., Acharya, N. K., Han, M., Zavareh, S. B., Sedeyn, J. C., Venkataraman, V., et al. (2010). Brain-reactive autoantibodies are nearly ubiquitous in human sera and may be linked to pathology in the context of blood-brain barrier breakdown. Brain Res. 1345, 221–232. doi: 10.1016/j.brainres.2010.05.038
Lin, W.-R., Wozniak, M. A., Cooper, R. J., Wilcock, G. K., and Itzhaki, R. F. (2002). Herpesviruses in brain and Alzheimer’s disease. J. Pathol. 197, 395–402. doi: 10.1002/path.1127
Lindhagen-Persson, M., Brännström, K., Vestling, M., Steinitz, M., and Olofsson, A. (2010). Amyloid-β oligomer specificity mediated by the IgM isotype–implications for a specific protective mechanism exerted by endogenous auto-antibodies. PLoS One 5:e13928. doi: 10.1371/journal.pone.0013928
Louveau, A., Harris, T. H., and Kipnis, J. (2015a). Revisiting the mechanisms of CNS immune privilege. Trends Immunol. 36, 569–577. doi: 10.1016/j.it.2015.08.006
Louveau, A., Herz, J., Alme, M. N., Salvador, A. F., Dong, M. Q., Viar, K. E., et al. (2018). CNS lymphatic drainage and neuroinflammation are regulated by meningeal lymphatic vasculature. Nat. Neurosci. 21, 1380–1391. doi: 10.1038/s41593-018-0227-9
Louveau, A., Smirnov, I., Keyes, T. J., Eccles, J. D., Rouhani, S. J., Peske, J. D., et al. (2015b). Structural and functional features of central nervous system lymphatic vessels. Nature 523, 337–341. doi: 10.1038/nature14432
Lozupone, C. A., Stombaugh, J. I., Gordon, J. I., Jansson, J. K., and Knight, R. (2012). Diversity, stability and resilience of the human gut microbiota. Nature 489, 220–230. doi: 10.1038/nature11550
Lyden, P., Pryor, K. E., Coffey, C. S., Cudkowicz, M., Conwit, R., Jadhav, A., et al. (2019). Final results of the RHAPSODY trial: a multi-center, phase 2 trial using a continual reassessment method to determine the safety and tolerability of 3K3A-APC, a recombinant variant of human activated protein C, in combination with tissue plasminogen activator, mechanical thrombectomy or both in moderate to severe acute ischemic stroke. Ann. Neurol. 85, 125–136. doi: 10.1002/ana.25383
Maftei, M., Thurm, F., Schnack, C., Tumani, H., Otto, M., Elbert, T., et al. (2013). Increased levels of antigen-bound β-amyloid autoantibodies in serum and cerebrospinal fluid of Alzheimer’s disease patients. PLoS One 8:e68996. doi: 10.1371/journal.pone.0068996
Mannix, R., Meehan, W. P., Mandeville, J., Grant, P. E., Gray, T., Berglass, J., et al. (2013). Clinical correlates in an experimental model of repetitive mild brain injury. Ann. Neurol. 74, 65–75. doi: 10.1002/ana.23858
Marchi, N., Angelov, L., Masaryk, T., Fazio, V., Granata, T., Hernandez, N., et al. (2007). Seizure-promoting effect of blood-brain barrier disruption. Epilepsia 48, 732–742. doi: 10.1111/j.1528-1167.2007.00988.x
Marchi, N., Bazarian, J. J., Puvenna, V., Janigro, M., Ghosh, C., Zhong, J., et al. (2013). Consequences of repeated blood-brain barrier disruption in football players. PLoS One 8:e56805. doi: 10.1371/journal.pone.0056805
Marchi, N., Fan, Q., Ghosh, C., Fazio, V., Bertolini, F., Betto, G., et al. (2009). Antagonism of peripheral inflammation reduces the severity of status epilepticus. Neurobiol. Dis. 33, 171–181. doi: 10.1016/j.nbd.2008.10.002
Marchi, N., Granata, T., Freri, E., Ciusani, E., Ragona, F., Puvenna, V., et al. (2011). Efficacy of anti-inflammatory therapy in a model of acute seizures and in a population of pediatric drug resistant epileptics. PLoS One 6:e18200. doi: 10.1371/journal.pone.0018200
Marchi, N., Granata, T., and Janigro, D. (2014). Inflammatory pathways of seizure disorders. Trends Neurosci. 37, 55–65. doi: 10.1016/j.tins.2013.11.002
Martin, C. R., Osadchiy, V., Kalani, A., and Mayer, E. A. (2018). The brain-gut-microbiome axis. Cell Mol. Gastroenterol. Hepatol. 6, 133–148. doi: 10.1016/j.jcmgh.2018.04.003
Marushima, A., Nieminen, M., Kremenetskaia, I., Gianni-Barrera, R., Woitzik, J., von Degenfeld, G., et al. (2019). Balanced single-vector co-delivery of VEGF/PDGF-BB improves functional collateralization in chronic cerebral ischemia. J. Cereb. Blood Flow Metab. 9:271678X18818298. doi: 10.1177/0271678X18818298
McIntyre, J. A., Chapman, J., Shavit, E., Hamilton, R. L., and Dekosky, S. T. (2007). Redox-reactive autoantibodies in Alzheimer’s patients’ cerebrospinal fluids: preliminary studies. Autoimmunity 40, 390–396. doi: 10.1080/08916930701421020
McIntyre, J. A., Ramsey, C. J., Gitter, B. D., Saykin, A. J., Wagenknecht, D. R., Hyslop, P. A., et al. (2015). Antiphospholipid autoantibodies as blood biomarkers for detection of early stage Alzheimer’s disease. Autoimmunity 48, 344–351. doi: 10.3109/08916934.2015.1008464
McRae, A., Martins, R. N., Fonte, J., Kraftsik, R., Hirt, L., and Miklossy, J. (2007). Cerebrospinal fluid antimicroglial antibodies in Alzheimer disease: a putative marker of an ongoing inflammatory process. Exp. Gerontol. 42, 355–363. doi: 10.1016/j.exger.2006.10.015
Mecocci, P., Parnetti, L., Romano, G., Scarelli, A., Chionne, F., Cecchetti, R., et al. (1995). Serum anti-GFAP and anti-S100 autoantibodies in brain aging, Alzheimer’s disease and vascular dementia. J. Neuroimmunol. 57, 165–170. doi: 10.1016/0165-5728(94)00180-v
Miklossy, J. (2011). Alzheimer’s disease - a neurospirochetosis. Analysis of the evidence following Koch’s and Hill’s criteria. J. Neuroinflamm. 8:90. doi: 10.1186/1742-2094-8-90
Miklossy, J. (2016). Bacterial amyloid and DNA are important constituents of senile plaques: further evidence of the spirochetal and biofilm nature of senile plaques. J. Alzheimers Dis. 13, 1459–1473. doi: 10.3233/JAD-160451
Minter, M. R., Hinterleitner, R., Meisel, M., Zhang, C., Leone, V., Zhang, X., et al. (2017). Antibiotic-induced perturbations in microbial diversity during post-natal development alters amyloid pathology in an aged APPSWE/PS1ΔE9 murine model of Alzheimer’s disease. Sci. Rep. 7:10411.
Minter, M. R., Zhang, C., Leone, V., Ringus, D. L., Zhang, X., Oyler-Castrillo, P., et al. (2016). Antibiotic-induced perturbations in gut microbial diversity influences neuro-inflammation and amyloidosis in a murine model of Alzheimer’s disease. Sci. Rep. 21:30028.
Moir, R. D., Lathe, R., and Tanzi, R. E. (2018). The antimicrobial protection hypothesis of Alzheimer’s disease. Alzheimers Dement. J. Alzheimers Assoc. 14, 1602–1614. doi: 10.1016/j.jalz.2018.06.3040
Montagne, A., Barnes, S. R., Sweeney, M. D., Halliday, M. R., Sagare, A. P., Zhao, Z., et al. (2015). Blood-brain barrier breakdown in the aging human hippocampus. Neuron 85, 296–302. doi: 10.1016/j.neuron.2014.12.032
Montagne, A., Nikolakopoulou, A. M., Zhao, Z., Sagare, A. P., Si, G., Lazic, D., et al. (2018). Pericyte degeneration causes white matter dysfunction in the mouse central nervous system. Nat. Med. 24, 326–337. doi: 10.1038/nm.4482
Mruthinti, S., Buccafusco, J. J., Hill, W. D., Waller, J. L., Jackson, T. W., Zamrini, E. Y., et al. (2004). Autoimmunity in Alzheimer’s disease: increased levels of circulating IgGs binding Abeta and RAGE peptides. Neurobiol. Aging 25, 1023–1032. doi: 10.1016/j.neurobiolaging.2003.11.001
Myagkova, M. A., Gavrilova, S. I., Lermontova, N. N., Kalyn, Y. B., Selezneva, N. D., Zharikov, G. A., et al. (2001). Autoantibodies to beta-amyloid and neurotransmitters in patients with Alzheimer’s disease and senile dementia of the Alzheimer type. Bull. Exp. Biol. Med. 131, 127–129.
Myles, I. A. (2014). Fast food fever: reviewing the impacts of the Western diet on immunity. Nutr. J. 13:61. doi: 10.1186/1475-2891-13-61
Na, W., Shin, J. Y., Lee, J. Y., Jeong, S., Kim, W.-S., Yune, T. Y., et al. (2017). Dexamethasone suppresses JMJD3 gene activation via a putative negative glucocorticoid response element and maintains integrity of tight junctions in brain microvascular endothelial cells. J. Cereb. Blood Flow Metab. 37, 3695–3708. doi: 10.1177/0271678X17701156
Nation, D. A., Sweeney, M. D., Montagne, A., Sagare, A. P., D’Orazio, L. M., Pachicano, M., et al. (2019). Blood-brain barrier breakdown is an early biomarker of human cognitive dysfunction. Nat. Med. 25, 270–276. doi: 10.1038/s41591-018-0297-y
Nikolakopoulou, A. M., Zhao, Z., Montagne, A., and Zlokovic, B. V. (2019). Regional early and progressive loss of brain pericytes but not vascular smooth muscle cells in adult mice with disrupted platelet-derived growth factor receptor-β signaling. PLoS One 12:e0176225. doi: 10.1371/journal.pone.0176225
Nishimura, S., Manabe, I., Takaki, S., Nagasaki, M., Otsu, M., Yamashita, H., et al. (2013). Adipose natural regulatory B cells negatively control adipose tissue inflammation. Cell Metab. 18, 759–766. doi: 10.1016/j.cmet.2013.09.017
Noé, F. M., and Marchi, N. (2019). Central nervous system lymphatic unit, immunity, and epilepsy: is there a link? Epilepsia Open 4, 30–39. doi: 10.1002/epi4.12302
Nortley, R., Korte, N., Izquierdo, P., Hirunpattarasilp, C., Mishra, A., Jaunmuktane, Z., et al. (2019). Amyloid β oligomers constrict human capillaries in Alzheimer’s disease via signaling to pericytes. Science 365:eaav9518. doi: 10.1126/science.aav9518
Ochoa-Repáraz, J., Mielcarz, D. W., Ditrio, L. E., Burroughs, A. R., Foureau, D. M., Haque-Begum, S., et al. (2009). Role of gut commensal microflora in the development of experimental autoimmune encephalomyelitis. J. Immunol. 183, 6041–6050. doi: 10.4049/jimmunol.0900747
Ochoa-Repáraz, J., Mielcarz, D. W., Haque-Begum, S., and Kasper, L. H. (2010). Induction of a regulatory B cell population in experimental allergic encephalomyelitis by alteration of the gut commensal microflora. Gut Microbes 1, 103–108. doi: 10.4161/gmic.1.2.11515
Omalu, B. I., Hamilton, R. L., Kamboh, M. I., DeKosky, S. T., and Bailes, J. (2010). Chronic traumatic encephalopathy (CTE) in a National Football League Player: case report and emerging medicolegal practice questions. J. Forensic Nurs. 6, 40–46. doi: 10.1111/j.1939-3938.2009.01064.x
Ouwehand, A., Isolauri, E., and Salminen, S. (2002). The role of the intestinal microflora for the development of the immune system in early childhood. Eur. J. Nutr. 41(Suppl. 1), I32–I37.
Pagliai, G., Russo, E., Niccolai, E., Dinu, M., Di Pilato, V., Magrini, A., et al. (2019). Influence of a 3-month low-calorie Mediterranean diet compared to the vegetarian diet on human gut microbiota and SCFA: the CARDIVEG study. Eur. J. Nutr. doi: 10.1007/s00394-019-02050-0 [Epub ahead of print].
Païssé, S., Valle, C., Servant, F., Courtney, M., Burcelin, R., Amar, J., et al. (2016). Comprehensive description of blood microbiome from healthy donors assessed by 16S targeted metagenomic sequencing. Transfusion 56, 1138–1147. doi: 10.1111/trf.13477
Pani, F., Di Dalmazi, G., Corsello, A., Oliver, T. G., Livezey, J. R., and Caturegli, P. (2019). MON-450 pituitary antibodies in a cohort of us service members with traumatic brain injury. J. Endocr. Soc. 3(Suppl. 1):MON–450.
Parker, A., Fonseca, S., and Carding, S. R. (2019). Gut microbes and metabolites as modulators of blood-brain barrier integrity and brain health. Gut Microbes doi: 10.1080/19490976.2019.1638722 [Epub ahead of print].
Pavlov, V. A., and Tracey, K. J. (2017). Neural regulation of immunity: molecular mechanisms and clinical translation. Nat. Neurosci. 20, 156–166. doi: 10.1038/nn.4477
Pestka, J. J., Vines, L. L., Bates, M. A., He, K., and Langohr, I. (2014). Comparative effects of n-3, n-6 and n-9 unsaturated fatty acid-rich diet consumption on lupus nephritis, autoantibody production and CD4+ T cell-related gene responses in the autoimmune NZBWF1 mouse. PLoS One 9:e100255. doi: 10.1371/journal.pone.0100255
Petta, I., Fraussen, J., Somers, V., and Kleinewietfeld, M. (2018). Interrelation of diet, gut microbiome, and autoantibody production. Front. Immunol. 9:439. doi: 10.3389/fimmu.2018.00439
Poole, S., Singhrao, S. K., Chukkapalli, S., Rivera, M., Velsko, I., Kesavalu, L., et al. (2015). Active invasion of Porphyromonas gingivalis and infection-induced complement activation in ApoE-/- mice brains. J. Alzheimers Dis. 43, 67–80. doi: 10.3233/JAD-140315
Posse de Chaves, E., and Sipione, S. (2010). Sphingolipids and gangliosides of the nervous system in membrane function and dysfunction. FEBS Lett. 584, 1748–1759. doi: 10.1016/j.febslet.2009.12.010
Prinz, M., and Priller, J. (2017). The role of peripheral immune cells in the CNS in steady state and disease. Nat. Neurosci. 20, 136–144. doi: 10.1038/nn.4475
Purvis, G. S. D., Solito, E., and Thiemermann, C. (2019). Annexin-A1: therapeutic potential in microvascular disease. Front. Immunol. 10:938. doi: 10.3389/fimmu.2019.00938
Qu, B.-X., Gong, Y., Moore, C., Fu, M., German, D. C., Chang, L.-Y., et al. (2014). Beta-amyloid auto-antibodies are reduced in Alzheimer’s disease. J. Neuroimmunol. 274, 168–173. doi: 10.1016/j.jneuroim.2014.06.017
Raad, M., Nohra, E., Chams, N., Itani, M., Talih, F., Mondello, S., et al. (2014). Autoantibodies in traumatic brain injury and central nervous system trauma. Neuroscience 281, 16–23. doi: 10.1016/j.neuroscience.2014.08.045
Rahman, M. T., Ghosh, C., Hossain, M., Linfield, D., Rezaee, F., Janigro, D., et al. (2018). IFN-γ, IL-17A, or zonulin rapidly increase the permeability of the blood-brain and small intestinal epithelial barriers: relevance for neuro-inflammatory diseases. Biochem. Biophys. Res. Commun. 507, 274–279. doi: 10.1016/j.bbrc.2018.11.021
Ransohoff, R. M. (2016). How neuroinflammation contributes to neurodegeneration. Science 353, 777–783. doi: 10.1126/science.aag2590
Religa, P., Cao, R., Religa, D., Xue, Y., Bogdanovic, N., Westaway, D., et al. (2013). VEGF significantly restores impaired memory behavior in Alzheimer’s mice by improvement of vascular survival. Sci. Rep. 3, 2053.
Reza, M. M., Finlay, B. B., and Pettersson, S. (2019). Gut microbes, ageing & organ function: a chameleon in modern biology? EMBO Mol. Med. 11:e9872.
Rinne, J. O., Brooks, D. J., Rossor, M. N., Fox, N. C., Bullock, R., Klunk, W. E., et al. (2010). 11C-PiB PET assessment of change in fibrillar amyloid-beta load in patients with Alzheimer’s disease treated with bapineuzumab: a phase 2, double-blind, placebo-controlled, ascending-dose study. Lancet Neurol. 9, 363–372. doi: 10.1016/S1474-4422(10)70043-0
Rosenmann, H., Meiner, Z., Geylis, V., Abramsky, O., and Steinitz, M. (2006). Detection of circulating antibodies against tau protein in its unphosphorylated and in its neurofibrillary tangles-related phosphorylated state in Alzheimer’s disease and healthy subjects. Neurosci Lett. 410, 90–93. doi: 10.1016/j.neulet.2006.01.072
Rudehill, S., Muhallab, S., Wennersten, A., von Gertten, C., Al Nimer, F., Sandberg-Nordqvist, A. C., et al. (2006). Autoreactive antibodies against neurons and basal lamina found in serum following experimental brain contusion in rats. Acta Neurochir. 148, 199–205. doi: 10.1007/s00701-005-0673-5
Rustenhoven, J., Jansson, D., Smyth, L. C., and Dragunow, M. (2017). Brain Pericytes As Mediators of Neuroinflammation. Trends Pharmacol. Sci. 38, 291–304. doi: 10.1016/j.tips.2016.12.001
Salloway, S., Sperling, R., Gilman, S., Fox, N. C., Blennow, K., Raskind, M., et al. (2009). A phase 2 multiple ascending dose trial of bapineuzumab in mild to moderate Alzheimer disease. Neurology 73, 2061–2070. doi: 10.1212/WNL.0b013e3181c67808
Sampson, T. R., Debelius, J. W., Thron, T., Janssen, S., Shastri, G. G., Ilhan, Z. E., et al. (2016). Gut microbiota regulate motor deficits and neuroinflammation in a model of Parkinson’s disease. Cell 167, 1469.e12–1480.e12. doi: 10.1016/j.cell.2016.11.018
Scheperjans, F., Aho, V., Pereira, P. A. B., Koskinen, K., Paulin, L., Pekkonen, E., et al. (2015). Gut microbiota are related to Parkinson’s disease and clinical phenotype. Mov. Disord. 30, 350–358.
Schierwagen, R., Alvarez-Silva, C., Madsen, M. S. A., Kolbe, C. C., Meyer, C., Thomas, D., et al. (2018). Circulating microbiome in blood of different circulatory compartments. Gut doi: 10.1136/gutjnl-2018-316227 [Epub ahead of print].
Schroeder, B. O., and Bäckhed, F. (2016). Signals from the gut microbiota to distant organs in physiology and disease. Nat. Med. 22, 1079–1089. doi: 10.1038/nm.4185
Schwartz, M., and Shechter, R. (2010). Systemic inflammatory cells fight off neurodegenerative disease. Nat. Rev. Neurol. 6, 405–410. doi: 10.1038/nrneurol.2010.71
Senatorov, V. V., Friedman, A. R., Milikovsky, D. Z., Ofer, J., Saar-Ashkenazy, R., Charbash, A., et al. (2019). Blood-brain barrier dysfunction in aging induces hyper-activation of TGF-beta signaling and chronic yet reversible neural dysfunction. Sci. Transl. Med. 11:eaaw8283. doi: 10.1126/scitranslmed.aaw8283
Sevigny, J., Chiao, P., Bussière, T., Weinreb, P. H., Williams, L., Maier, M., et al. (2016). The antibody aducanumab reduces Aβ plaques in Alzheimer’s disease. Nature 01, 50–56.
Shanahan, F., van Sinderen, D., O’Toole, P. W., and Stanton, C. (2017). Feeding the microbiota: transducer of nutrient signals for the host. Gut 66, 1709–1717. doi: 10.1136/gutjnl-2017-313872
Sherwin, E., Bordenstein, S. R., Quinn, J. L., Dinan, T. G., and Cryan, J. F. (2019). Microbiota and the social brain. Science 366:eaar2016.
Sigurdsson, E. M. (2018). Tau immunotherapies for Alzheimer’s disease and related tauopathies: progress and potential pitfalls. J. Alzheimers Dis. 64(Suppl.1), S555–S565.
Singh, R. K., Chang, H.-W., Yan, D., Lee, K. M., Ucmak, D., Wong, K., et al. (2017). Influence of diet on the gut microbiome and implications for human health. J. Transl. Med. 15:73. doi: 10.1186/s12967-017-1175-y
Sinha, R. K., Wang, Y., Zhao, Z., Xu, X., Burnier, L., Gupta, N., et al. (2018). PAR1 biased signaling is required for activated protein C in vivo benefits in sepsis and stroke. Blood 15, 1163–1171. doi: 10.1182/blood-2017-10-810895
Smith, C., Graham, D. I., Murray, L. S., and Nicoll, J. A. (2003). Tau immunohistochemistry in acute brain injury. Neuropathol. Appl. Neurobiol. 29, 496–502. doi: 10.1046/j.1365-2990.2003.00488.x
Sommer, A., Winner, B., and Prots, I. (2017). The Trojan horse - neuroinflammatory impact of T cells in neurodegenerative diseases. Mol. Neurodegener. 12:78. doi: 10.1186/s13024-017-0222-8
Sorokina, E. G., Vol’pina, O. M., Semenova, Z. B., Karaseva, O. V., Koroev, D. O., Kamynina, A. V., et al. (2011). [Autoantibodies to α7-subunit of neuronal acetylcholine receptor in children with traumatic brain injury]. Zh. Nevrol. Psikhiatr. Im. S S Korsakova 111, 56–60.
Soscia, S. J., Kirby, J. E., Washicosky, K. J., Tucker, S. M., Ingelsson, M., Hyman, B., et al. (2010). The Alzheimer’s disease-associated amyloid beta-protein is an antimicrobial peptide. PLoS One 5:e9505. doi: 10.1371/journal.pone.0009505
Su, E. J., Fredriksson, L., Kanzawa, M., Moore, S., Folestad, E., Stevenson, T. K., et al. (2015). Imatinib treatment reduces brain injury in a murine model of traumatic brain injury. Front. Cell. Neurosci. 9:385. doi: 10.3389/fncel.2015.00385
Sweeney, M. D., Zhao, Z., Montagne, A., Nelson, A. R., and Zlokovic, B. V. (2019). Blood-brain barrier: from physiology to disease and back. Physiol. Rev. 01, 21–78. doi: 10.1152/physrev.00050.2017
Tai, X. Y., Koepp, M., Duncan, J. S., Fox, N., Thompson, P., Baxendale, S., et al. (2016). Hyperphosphorylated tau in patients with refractory epilepsy correlates with cognitive decline: a study of temporal lobe resections. Brain J. Neurol. 139(Pt 9), 2441–2455. doi: 10.1093/brain/aww187
Tanaka, J., Nakamura, K., Takeda, M., Tada, K., Suzuki, H., Morita, H., et al. (1989). Enzyme-linked immunosorbent assay for human autoantibody to glial fibrillary acidic protein: higher titer of the antibody is detected in serum of patients with Alzheimer’s disease. Acta Neurol. Scand. 80, 554–560. doi: 10.1111/j.1600-0404.1989.tb03926.x
Tanriverdi, F., De Bellis, A., Battaglia, M., Bellastella, G., Bizzarro, A., Sinisi, A. A., et al. (2010). Investigation of antihypothalamus and antipituitary antibodies in amateur boxers: is chronic repetitive head trauma-induced pituitary dysfunction associated with autoimmunity? Eur. J. Endocrinol. 162, 861–867. doi: 10.1530/EJE-09-1024
Tanriverdi, F., De Bellis, A., Bizzarro, A., Sinisi, A. A., Bellastella, G., Pane, E., et al. (2008). Antipituitary antibodies after traumatic brain injury: is head trauma-induced pituitary dysfunction associated with autoimmunity? Eur. J. Endocrinol. 159, 7–13. doi: 10.1530/EJE-08-0050
Tanriverdi, F., Senyurek, H., Unluhizarci, K., Selcuklu, A., Casanueva, F. F., and Kelestimur, F. (2006). High risk of hypopituitarism after traumatic brain injury: a prospective investigation of anterior pituitary function in the acute phase and 12 months after trauma. J. Clin. Endocrinol. Metab. 91, 2105–2111. doi: 10.1210/jc.2005-2476
Thiyagarajan, M., Fernández, J. A., Lane, S. M., Griffin, J. H., and Zlokovic, B. V. (2008). Activated protein C promotes neovascularization and neurogenesis in postischemic brain via protease-activated receptor 1. J. Neurosci. 28, 12788–12797. doi: 10.1523/JNEUROSCI.3485-08.2008
Thomas, T., Thomas, G., McLendon, C., Sutton, T., and Mullan, M. (1996). beta-Amyloid-mediated vasoactivity and vascular endothelial damage. Nature 380, 168–171. doi: 10.1038/380168a0
Tomasdottir, V., Thorleifsdottir, S., Vikingsson, A., Hardardottir, I., and Freysdottir, J. (2014). Dietary omega-3 fatty acids enhance the B1 but not the B2 cell immune response in mice with antigen-induced peritonitis. J. Nutr. Biochem. 25, 111–117. doi: 10.1016/j.jnutbio.2013.09.010
U.S. National Library of Medicine, (2019a). A Long-Term Safety and Tolerability Extension Study Of Bapineuzumab In Alzheimer Disease Patients. Available from: https://clinicaltrials.gov/ct2/show/NCT00998764
U.S. National Library of Medicine, (2019b). A Long-Term Safety and Tolerability Study Of Bapineuzumab In Alzheimer Disease Patients 2019. Available from: https://clinicaltrials.gov/ct2/show/NCT00996918
Vacirca, D., Delunardo, F., Matarrese, P., Colasanti, T., Margutti, P., Siracusano, A., et al. (2012). Autoantibodies to the adenosine triphosphate synthase play a pathogenetic role in Alzheimer’s disease. Neurobiol. Aging 33, 753–766. doi: 10.1016/j.neurobiolaging.2010.05.013
Van Skike, C. E., Jahrling, J. B., Olson, A. B., Sayre, N. L., Hussong, S. A., Ungvari, Z., et al. (2018). Inhibition of mTOR protects the blood-brain barrier in models of Alzheimer’s disease and vascular cognitive impairment. Am. J. Physiol. Heart Circ. Physiol. 314, H693–H703.
Vezzani, A., French, J., Bartfai, T., and Baram, T. Z. (2011). The role of inflammation in epilepsy. Nat. Rev. Neurol. 7, 31–40.
Virtue, A. T., McCright, S. J., Wright, J. M., Jimenez, M. T., Mowel, W. K., Kotzin, J. J., et al. (2019). The gut microbiota regulates white adipose tissue inflammation and obesity via a family of microRNAs. Sci. Transl. Med. 11:eaav1892. doi: 10.1126/scitranslmed.aav1892
Vogt, N. M., Kerby, R. L., Dill-McFarland, K. A., Harding, S. J., Merluzzi, A. P., Johnson, S. C., et al. (2017). Gut microbiome alterations in Alzheimer’s disease. Sci. Rep. 7:13537. doi: 10.1242/dmm.041947
Wang, X., Sun, G., Feng, T., Zhang, J., Huang, X., Wang, T., et al. (2019). Sodium oligomannate therapeutically remodels gut microbiota and suppresses gut bacterial amino acids-shaped neuroinflammation to inhibit Alzheimer’s disease progression. Cell Res. 29, 787–803. doi: 10.1038/s41422-019-0216-x
Wang, Y., Zhao, Z., Rege, S. V., Wang, M., Si, G., Zhou, Y., et al. (2016). 3K3A-activated protein C stimulates postischemic neuronal repair by human neural stem cells in mice. Nat. Med. 22, 1050–1055. doi: 10.1038/nm.4154
Weksler, M. E., Relkin, N., Turkenich, R., LaRusse, S., Zhou, L., and Szabo, P. (2002). Patients with Alzheimer disease have lower levels of serum anti-amyloid peptide antibodies than healthy elderly individuals. Exp. Gerontol. 37, 943–948. doi: 10.1016/s0531-5565(02)00029-3
Wilson, J. S., vainti, S., Buccafusco, J. J., Schade, R. F., Mitchell, M. B., Harrell, D. U., et al. (2009). Anti-RAGE and Abeta immunoglobulin levels are related to dementia level and cognitive performance. J. Gerontol. A Biol. Sci. Med. Sci. 64, 264–271. doi: 10.1093/gerona/gln002
Wu, J., and Li, L. (2016). Autoantibodies in Alzheimer’s disease: potential biomarkers, pathogenic roles, and therapeutic implications. J. Biomed. Res. 30, 361–372.
Zhang, F., Eckman, C., Younkin, S., Hsiao, K. K., and Iadecola, C. (1997). Increased susceptibility to ischemic brain damage in transgenic mice overexpressing the amyloid precursor protein. J. Neurosci. 17, 7655–7661. doi: 10.1523/jneurosci.17-20-07655.1997
Zhang, N., Yin, S., Zhang, W., Gong, X., Zhang, N., Fang, K., et al. (2017). Crystal structure and biochemical characterization of an aminopeptidase LapB from Legionella pneumophila. J. Agric. Food Chem. 65, 7569–7578. doi: 10.1021/acs.jafc.7b02849
Zhang, Z., Zoltewicz, J. S., Mondello, S., Newsom, K. J., Yang, Z., Yang, B., et al. (2014). Human traumatic brain injury induces autoantibody response against glial fibrillary acidic protein and its breakdown products. PLoS One 9:e92698. doi: 10.1371/journal.pone.0092698
Zheng, P., Zeng, B., Zhou, C., Liu, M., Fang, Z., Xu, X., et al. (2016). Gut microbiome remodeling induces depressive-like behaviors through a pathway mediated by the host’s metabolism. Mol. Psychiatry 21, 786–796. doi: 10.1038/mp.2016.44
Zhong, Z., Ilieva, H., Hallagan, L., Bell, R., Singh, I., Paquette, N., et al. (2009). Activated protein C therapy slows ALS-like disease in mice by transcriptionally inhibiting SOD1 in motor neurons and microglia cells. J. Clin. Invest. 119, 3437–3449. doi: 10.1172/JCI38476
Zhuang, Z.-Q., Shen, L.-L., Li, W.-W., Fu, X., Zeng, F., Gui, L., et al. (2018). Gut microbiota is altered in patients with Alzheimer’s disease. J. Alzheimers Dis. 63, 1337–1346. doi: 10.3233/JAD-180176
Zlokovic, B. V. (2008). The blood-brain barrier in health and chronic neurodegenerative disorders. Neuron 57, 178–201. doi: 10.1016/j.neuron.2008.01.003
Zlokovic, B. V. (2011). Neurovascular pathways to neurodegeneration in Alzheimer’s disease and other disorders. Nat. Rev. Neurosci. 12, 723–738. doi: 10.1038/nrn3114
Keywords: blood–brain barrier, neurodegeneration, peripheral immunity, traumatic brain injury, status epilepticus, autoantibodies, gut–brain axis, inflammation
Citation: Giannoni P, Claeysen S, Noe F and Marchi N (2020) Peripheral Routes to Neurodegeneration: Passing Through the Blood–Brain Barrier. Front. Aging Neurosci. 12:3. doi: 10.3389/fnagi.2020.00003
Received: 30 July 2019; Accepted: 08 January 2020;
Published: 04 February 2020.
Edited by:
Daniel Ortuño-Sahagún, Universidad de Guadalajara, MexicoReviewed by:
Annadora Bruce-Keller, Pennington Biomedical Research Center, United StatesBerislav Zlokovic, University of Southern California, United States
Copyright © 2020 Giannoni, Claeysen, Noe and Marchi. This is an open-access article distributed under the terms of the Creative Commons Attribution License (CC BY). The use, distribution or reproduction in other forums is permitted, provided the original author(s) and the copyright owner(s) are credited and that the original publication in this journal is cited, in accordance with accepted academic practice. No use, distribution or reproduction is permitted which does not comply with these terms.
*Correspondence: Nicola Marchi, bmljb2xhLm1hcmNoaUBpZ2YuY25ycy5mcg==