- 1Department of Anesthesiology, The First Affiliated Hospital, College of Medicine, Zhejiang University, Hangzhou, China
- 2Central Laboratory, Renmin Hospital of Wuhan University, Wuhan, China
Stroke remains a leading cause of death, disability, and medical care burden worldwide. However, transformation from laboratory findings toward effective pharmacological interventions for clinical stroke has been unsatisfactory. Novel evidence has been gained on the underlying mechanisms and therapeutic potential related to the transient receptor potential (TRP) channels in several disorders. The TRP superfamily consists of a diverse group of Ca2+ permeable non-selective cation channels. In particular, the members of TRP subfamilies, TRP canonical (TRPC) channels and TRPC6, have been found in different cell types in the whole body and have high levels of expression in the central nervous system (CNS). Notably, the TRPCs and TRPC6 channel have been implicated in neurite outgrowth and neuronal survival during normal development and in a range of CNS pathological conditions. Recent studies have shown that suppression of TRPC6 channel degradation prevents ischemic neuronal cell death in experimental stroke. Accumulating evidence supports the important functions of TRPC6 in brain ischemia. We have highlighted some crucial advancement that points toward an important involvement of TRPCs and TRPC6 in ischemic stroke. This review will make an overview of the TRP and TRPC channels due to their roles as targets for clinical trials and CNS disorders. Besides, the primary goal is to discuss and update the critical role of TRPC6 channels in stroke and provide a promising target for stroke prevention and therapy.
Introduction
Ischemic stroke is induced by the obstruction of an artery or multiple arteries leading to the brain. Focal impairment or occlusion of blood circulation to the brain impairs the normal function of neurons. The mechanisms underlying ischemic stroke are complex, and include excitotoxicity, oxidative and nitrosative stress, Ca2+ overload, inflammation, and apoptosis (Szydlowska and Tymianski, 2010; Khoshnam et al., 2017). Among these mechanisms, intracellular Ca2+ overload remains a vital role in neuronal injury associated with ischemic stroke (Choi, 1995). Glutamate receptors, such as N-methyl-D-aspartate receptor (NMDAR), are thought to be major pathways for intracellular Ca2+ influx in the central nervous system (CNS) after cerebral ischemia-reperfusion (IR) injury. Excessive NMDARs activation and the following Ca2+ influx through NMDARs are crucial steps required for initiating ischemic cell death (Szydlowska and Tymianski, 2010; Lai et al., 2011). To date, pre-clinical studies have provided substantial evidences for the neuroprotective effect of NMDAR antagonists in experimental ischemic stroke (Ginsberg, 2008). However, for several decades, clinical trials of NMDAR antagonists have all ended up with failure to show beneficial effects due to their narrow therapeutic windows and adverse effects (Wu and Tymianski, 2018). Thus, effective therapeutic interventions for ischemic stroke are urgently required.
Despite the pivotal functions of NMDARs, non-glutamate mechanisms have drawn attention as promising Ca2+ influx pathways involved in brain ischemia. In this respect, researchers shifted focus toward the transient receptor potential (TRP) channels (Szydlowska and Tymianski, 2010). TRPs are non-selective cationic channels which have key functions in different disorders (Moran, 2018). The TRP canonical (TRPC) subfamily was proved to be extensively distributed in CNS and have important functions in neuronal development (Tai et al., 2009). Understanding of these channels may drive the researchers to make a significant breakthrough in CNS diseases therapy. Recently, growing evidence indicates that TRPC6 channel has been involved in Ca2+ homeostasis and shown to participate in the molecular pathophysiology of ischemic stroke. TRPC6 was reported to have an critical role in neuroprotection in both in vitro and in vivo models of ischemic stroke (Du et al., 2010). In this review, we present a general description of the current understanding of TRPs and TRPC subfamily, with an emphasis on their involvement in clinical trials and CNS dysfunctions. Furthermore, this review concentrates on evidence-based advancements of TRPC6 in CNS disorders and cerebral ischemia. The primary aim is to clarify the relationship between TRPC6 and ischemic stroke and discuss future perspectives.
The TRP Ion Channel Family
The TRP channels comprise a big family of cation channels that are involved in various physiological and pathological processes. TRPs were first discovered in Drosophila in 1960s as a conditional phototransduction mutant (Minke, 1977; Montell et al., 1985). TRPs are commonly distributed in different cell types and tissues, and possess many vital functions in ion homeostasis, sensory transduction, inflammatory responses, innate and adaptive immune responses, and cell survival (Clapham, 2003; Nilius et al., 2007; Ramirez et al., 2018). The channel subunits consist of six transmembrane domains (TDs) that assemble as cation-permeable tetramers (Clapham et al., 2001). However, TRP channels have relatively low selectivity for the transport of cations, such as Na+ and Ca2+, into the cytoplasm.
The TRPs are divided into seven subfamilies, TRPC (canonical), TRPV (vanilloid), TRPM (melastatin), TRPP (polycystin), TRPML (mucolipin), TRPA (ankyrin), and TRPN (NO-mechano-potential), based on amino acid homology (Nilius et al., 2007). These channels can receive multiple types of intracellular and extracellular information, which in turn can induce a series of different responses. Dysfunctions of these proteins are related to many disorders (Kaneko and Szallasi, 2014); e.g., progressive kidney diseases (TRPC5 and TRPC6) (Winn et al., 2005; Zhou et al., 2017), pulmonary edema (TRPC6) (Weissmann et al., 2012), stroke (TRPC6) (Du et al., 2010), myocardial IR injury (TRPC3/6/7) (He et al., 2017), Huntington’s disease (HD) (TRPC5) (Hong et al., 2015), pruritus (TRPV1,TRPA1) (Moran, 2018), lower urinary tract disorders (TRPV4), pain (TRPV1, TRPA1, TRPM8, and TRPM3), and type 2 diabetes (TRPM5) (Voets et al., 2019), idiopathic rhinitis (TRPA1 and TRPV1) (Van Gerven et al., 2017), irritable bowel syndrome (TRPV1) (Wouters et al., 2016), and genetic diseases (TRPA1, TRPC6, TRPV3/4, TRPM1/4/6, TRPML1, TRPP2) (Moran, 2018).
There have been a number of clinical trials of compounds that regulate TRPV1, TRPV3, TRPV4, TRPA1, and TRPM8 (Moran, 2018). The vanilloid receptor, TRPV1, is identified as an important detector of pain, including heat hyperalgesia, postherpetic neuralgia, and osteoarthritic pain (Moran, 2018). Small molecule antagonists and agonists targeting TRPV1, such as NEO6860, V116517, and capsaicin, have attracted attention in research on multiple pain pathways and have been shown to have clinical potential for use in sustained pain relief (Szallasi et al., 2006; Arendt-Nielsen et al., 2016; Brown et al., 2017; Blair, 2018). However, safety issues, such as impaired noxious heat sensation and hyperthermia, require special consideration. Although several recent clinical trials suggested no increase in body temperature in humans (Arendt-Nielsen et al., 2016; Brown et al., 2017), most TRPV1 antagonists examined previously showed on-target adverse effects (Lee et al., 2017; Manitpisitkul et al., 2018), thus limiting their clinical acceptance. The balance between drug efficacy and side effects in clinical trials remains to be explored. TRPV3 is sensitive to warm temperatures (>33°C) and is predominantly found in skin keratinocytes (Xu et al., 2002; Moqrich et al., 2005). The TRPV3 antagonist, GRC 15300, has successfully finished a phase 1 clinical trial and appears to have potential for pain treatment (Pharmaceuticals, 2018). TRPV3 is also an emerging target for itch and skin diseases in animal models; further studies are required to confirm its utility in treatment of clinical skin diseases. TRPV4 was originally characterized in 2000 as an osmosensor that responds to extracellular osmolarity (Liedtke et al., 2000; Strotmann et al., 2000). TRPV4 performs essential roles in various diseases by regulating Ca2+ influx. A clinical trial of TRPV4 blockers and their promising roles in the treatment of dyspnea and pulmonary edema in heart failure and acute decompensated heart failure cases is currently finished in phase 1 stage (GlaxoSmithKline, 2019). Similarly, another single-center study designed to estimate the effects of GSK2798745, a novel TRPV4 inhibitor, on pulmonary gas exchange and pulmonary function in participants with heart failure has already completed a phase 2 clinical trial (GlaxoSmithKline, 2018). TRPA1 has been regarded as a gatekeeper of chronic inflammatory diseases (Bautista et al., 2013). A phase 2a clinical study yielded data indicating that the TRPA1 antagonist, GRC 17536, alleviates pain with no other drug-related side effects in patients with painful diabetic neuropathy, which supports the promising therapeutic efficacy of TRPA1 blockade in pain management (Pharmaceuticals, 2014). PF-05105679 is a TRPM8 antagonist that was shown to reverse cold pain sensation in the cold presser test in humans (Andrews et al., 2015; Gosset et al., 2017). Importantly, blockade of TRPM8 by PF-05105679 showed no effects on core body temperature.
Overall, TRPs have good potential as therapeutic targets for various diseases. Further efforts are still required to explore the specific and detailed functions of TRPs in different disorders.
The TRPC Subfamily and Their Roles in CNS
The mammalian C-type TRP channels (TRPC proteins) are most closely related to Drosophila TRPs. In terms of amino acid similarity, TRPCs can be classified into four subsets: TRPC1, TRPC2, TRPC3/6/7, and TRPC4/5 (Clapham et al., 2001). At a molecular level, all TRPCs consist of a pore–loop motif, three to four NH2-terminal ankyrin repeat domains (ARDs), and a COOH-terminal TRP domain (Venkatachalam and Montell, 2007). The ARD is a common protein–protein interaction module, typically consisting of 33 amino acid residues, which forms a canonical helix-loop-helix fold followed by a β-hairpin loop (Islam et al., 2018). ARDs perform crucial roles in various cellular processes, including thetranscription, signal transduction, inflammatory responses, cell development, and cell cycle (Islam et al., 2018). Confocal Förster resonance energy transfer (FRET) measurements revealed that the first ARD in TRPC5 is crucial for homomultimerization (Schindl et al., 2008). ADRs are also closely involved in TRPC4 tetramer assembly (Lepage et al., 2009). In addition, ARDs in TRPC3 in skeletal muscle could mediate TRPC3/1 heteromerization, thus regulating resting cytosolic Ca2+ levels (Woo et al., 2014). The novel membrane RING-H2 protein, RNF24, binds with the ARDs of TRPC6 in the Golgi apparatus and results in intracellular retention of TRPC6 without affecting channel activity (Lussier et al., 2008). Thus, ARDs of TRPCs likely participate in their channel heteromerization and trafficking. The TRP domain is a highly conserved sequence of 25 amino acids containing a proline-rich motif and an invariant sequence called the TRP box (Glu-Trp-Lys-Phe-Ala-Arg) (Montell, 2001). A previous study indicated that the TRP domain in TRPC3 is an essential element in regulating the cell response to erythropoietin (Hirschler-Laszkiewicz et al., 2011). The pore region of TRPCs, situated between the fifth and sixth TDs, is associated with an intracellular gate and an extracellular selectivity filter. The conserved LFW motif is a key feature of the TRPC pore loop, which has a pivotal role in hydrophobic interactions and in sustaining the appropriate structure of the ion pore (Li J. et al., 2019).
Transient receptor potential canonical channels are non-selective cation channels with different Ca2+ and Na+ permeability ratios. TRPCs activation is involved in changes in [Ca2+]i, which governs diverse complex and crucial cellular functions. Evidence suggests that all TRPCs can be commonly activated by phospholipase C (PLC) within numerous stimulation, like inflammatory and IR injury, subsequently mediating Ca2+ entry into the cell (Weissmann et al., 2012; Wu et al., 2015). TRPCs are closely connected to PLC activity controlled by modulation of the endogenous agonists diacylglycerols (DAGs) and phosphoinositides. TRPCs were originally identified in sensory nerve endings followed by axons and dendrites of central neurons in the CNS. There have been shown that TRPCs are involved in nerve-growth-cone guidance, neuronal survival, synapse formation, synaptic transmission, and sensory transduction (Li et al., 2005; Jia et al., 2007; Zhou et al., 2008; Quick et al., 2012; Hartmann and Konnerth, 2015). Notably, TPRCs are responsible for inflammation, IR injury, and excitotoxicity (Ramirez et al., 2018).
Alzheimer’s disease (AD) is driven by the cerebral deposition of amyloid-β protein (Aβ), the primary element of the senile plaques seen in the pathological brains. Loss of TRPC1 aggravates memory deficits and cell apoptosis induced by Aβ (Li et al., 2018). It is widely recognized that Aβ generation is closely related to amyloid precursor protein cleavage by γ-secretase. Wang et al. (2015) demonstrated that TRPC6 regulates γ-secretase of amyloid precursor protein. Importantly, a recent clinical case-control research revealed that the TRPC6 mRNA level in peripheral leukocytes is markedly reduced in patients with AD, which provides new insight for clinical diagnosis of AD (Chen et al., 2019). In addition, piperazine, a novel TRPC6 agonist, was shown to decrease long-term potentiation in the 5xFAD mouse, suggesting that piperazine may have potential for AD therapy (Popugaeva et al., 2019). Heteromultimeric channels of TRPC1/4/5 subunits regulate flexible relearning and working memory in mice (Broker-Lai et al., 2017). A study in TRPC5 transgenic mice identified an essential role of TRPC5, activated by G protein-coupled neuronal receptors, in the modulation of innate fear. This result provided strong evidence that TRPC5 channels may be a potential target for the alleviation of fear behavior (Riccio et al., 2009). Previous study has shown that TRPC1 aggravates hippocampal neuronal cell death (Narayanan et al., 2008). A subsequent research suggested that the TRPC1 mediates store-operated Ca2+ influx in neurons with mutant Huntingtin protein, suggesting a novel treatment for HD (Wu et al., 2011). Inhibition of TRPC1 is a potentially useful neuroprotective and therapeutic strategy against HD (Wu et al., 2018). In addition, generation of endogenous TRPC5 is increased in both transgenic mice and patients with HD. Knockdown or blockage of TRPC5 promotes neuronal survival and improved neurodegeneration in HD (Hong et al., 2015). The novel TRPC4/5 blocker, M084, was reported to show rapid antidepressant and anxiolytic-like effects in male C57BL/6 mice (Yang et al., 2015). Furthermore, TRPC channels have gained focus on the treatment of ischemic stroke. Decreased TRPC1 expression was shown in ischemic brain tissues. Overexpression of TRPC1 inhibits cerebral I/R injury, whereas TRPC1 knockout has the opposite effects. The underlying mechanism refers to the generation of reactive oxygen species via nicotinamide adenine dinucleotide phosphate (NADPH) oxidase family 4-containing-NADPH oxidase (Xu et al., 2018). Additionally, TRPC6 also holds important roles in stroke therapy, which will be discussed in further detail below.
Taken together, the observations outlined above indicating the importance of TRPCs in CNS disorders suggest that agents targeting TRPCs have great potential for clinical use. While highly selective and specific agonists and antagonists of TRPCs are needed for future study to deeply explore their roles in the treatment of CNS diseases. Even though there is limited evidence, specific channel characters of TRPC channels make them possible to be novel targets for brain ischemia. Therefore, further research efforts are required to find the possible mechanisms underlying the roles of TRPCs in CNS diseases and particularly in ischemic stroke.
Structure and Characteristics of TRPC6
The TRPC6 channel protein is composed of six TDs, intracellular NH2-ARDs, and the COOH-terminal TRPC6 domain (Figure 1). TRPC6 has a molecular weight of approximately 110 kDa and consists of 930 and 931 amino acid residues in mice and humans, respectively. The intracellular cytoplasmic domain and TD are indispensable for TRPC6 assembly (Tang et al., 2018). The TRPC6 channel is permeable to several cations, including Ca2+, Na+, K+, Cs+, and Ba2+ (Hofmann et al., 1999; Estacion et al., 2006). The relative ion permeability ratio (PCa/PNa) of TRPC6 is about 5 (Hofmann et al., 1999; Owsianik et al., 2006). Particularly, TRPC6 governs the function and fate of Ca2+ homeostasis in various cells and tissues. TRPC6 channel activation in neurons usually occurs in parallel with cytosolic entry of Ca2+ ions and the elevation of intracellular Ca2+ (Jia et al., 2007; Bouron et al., 2016). TRPC6 is widely expressed in different anatomical regions of the CNS, and is also highly distributed in placenta, lung, pancreas, adipose, heart, kidney, muscle, and other tissues (Riccio et al., 2002). TRPC6 can be activated by DAG, store-depletion, Hyperforin (St. John’s wort extract), or H2O2 (Bouron et al., 2016). DAG is a second messenger that acts as a regulator of key signaling molecules, cellular membrane constituents, and fatty acid metabolism (Massart and Zierath, 2019). It is widely accepted that activation of TRPC6 channels by DAG promotes various cellular responses, although the precise underlying mechanisms remain to be elucidated. By contrast, several studies have indicated that TRPC6 channels could also be store-operated (Yu et al., 2003; El Boustany et al., 2008). Hyperforin has been shown to specifically activate TRPC6 channels, which is mainly responsible for the antidepressant effect of St. John’s wort (Yang et al., 2018). There is also a close relation between TRPC6 and reactive oxygen species. H2O2 was reported to directly activate TRPC6 channels in different cell types (Bouron et al., 2016).
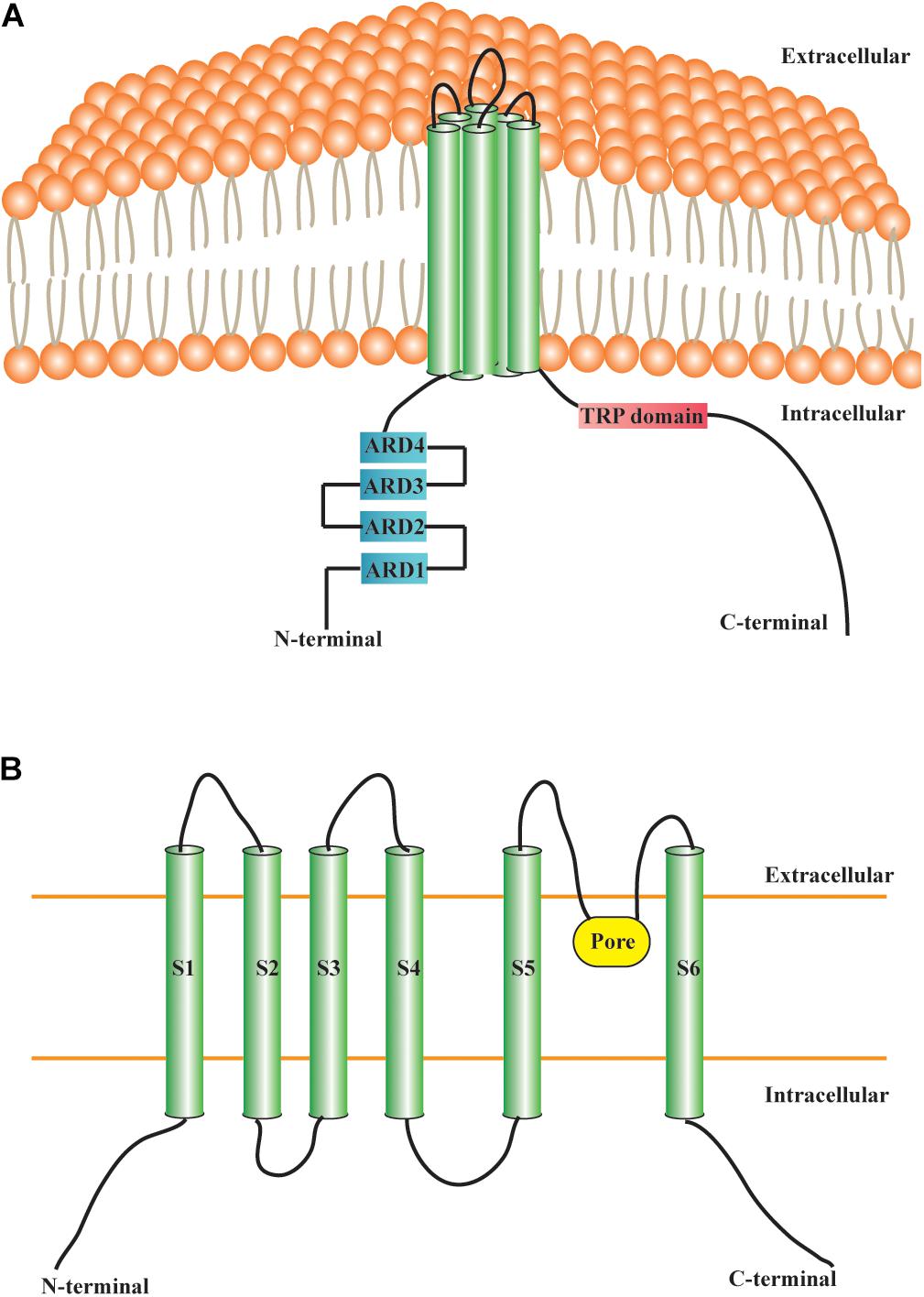
Figure 1. Structural features of TRPC6 channel. (A) Transmembrane protein TRPC6 contains six TDs, four NH2-terminal ARDs, and a COOH-terminal TRP domain. (B) S1–S6 are TDs. The pore region of TRPC6, situated between the S5 and S6, allows the passage of cations. TRP: transient receptor potential; TRPC6: transient receptor potential canonical channel 6; TD: transmembrane domain; ARD: ankyrin repeat domain; S1–S6: S1–S6 are the abbreviations of the first to sixth transmembrane domains.
The Biological Function of TRPC6 in CNS
In the postnatal rat cerebellum, TRPC6 is identified on Purkinje cell bodies, on interneurons in the molecular layer and mature granule cells in the internal granule cell layer, and is required for postnatal cerebellar neuron development (Huang et al., 2007). In cultured cerebellar granule cells, brain-derived neurotrophic factor (BDNF) induces Ca2+ elevation and nerve growth-cone extension through TRPC3/6 channels (Li et al., 2005). Besides, TRPC3 and TRPC6 contribute to BDNF-induced cerebellar granule neuron survival (Jia et al., 2007). siRNAs against TRPC3 and TRPC6 reduce BDNF-mediated intracellular Ca2+ elevation, neuroprotection, and cAMP-response element binding protein (CREB) activation in cerebellar granule neurons. By contrast, upregulation of TRPC3 or TRPC6 was shown to suppress neuronal apoptosis and promote the transcription of CREB-dependent reporter gene. Additionally, Ca2+ influx-dependent TRPC6 channel function is involved in hippocampal neuron dendritic growth via the Ca2+/calmodulin-dependent kinase IV (CaMKIV) and CREB pathway (Tai et al., 2008). In particular, the CaMKIV-CREB pathway seems to be a vital downstream molecular mechanism of TRPC6 function in the CNS. Another study showed that TRPC6 is predominantly presented in excitatory synapses and plays important roles in promoting synapse and dendritic spine formation, spatial memory, and learning through the CaMKIV-CREB pathway (Zhou et al., 2008). As an important intracellular second messenger, Ca2+ contributes to neuronal development and survival. The interaction between TRPC3/6 channels and the Na+/Ca2+ exchanger could regulate Ca2+ influx in neuronal cells, suggesting that TRPC3/6 may govern the fate of neurons by modulating Ca2+ influx (Louhivuori et al., 2010). TRPC6 also acts as a negative regulator that suppresses NMDA-induced Ca2+ currents in hippocampal neurons (Shen et al., 2013). Interestingly, NMDAR was shown to regulate transcription and degradation of TRPC6 in neurons in a bidirectional manner through NR2A or NR2B activation (Qu et al., 2017). Hyperforin, a specific agonist of TRPC6, regulates dendritic spine morphology in the hippocampus via TRPC6 activation and increasing intracellular Ca2+ transients (Leuner et al., 2013). However, the underlying signaling cascade has not yet been clarified. The same research team also reported the involvement of phosphatidylinositide 3-kinase (PI3K)/protein kinase B (PKB), Ras/mitogen-activated protein kinase/extracellular signal-regulated kinases (Ras/MAPK/ERK), and CaMKIV-CREB pathways (Heiser et al., 2013). Moreover, hyperforin also raises the number of excitatory synapses, dendritic spine density, and dendritic length in experimentally depressed rats by rescuing the decline of TRPC6 expression (Liu et al., 2015). High-throughput sequencing based on TRPC6 in autism spectrum disorder (ASD) patients and controls revealed the presence of more non-synonymous mutations in ASD individuals, suggesting that TRPC6 may carry out as a potential predisposing factor for ASD (Griesi-Oliveira et al., 2015). Furthermore, TRPC3 and TRPC6 could promote normal sensory mechanotransduction in touch and hearing neurons, and therefore knocking out both TRPC3 and TRPC6 resulted in deficits in sensory conduction (Quick et al., 2012). TRPC6 and TRPC7 channels also function in phototransduction in murine retinal ganglion cells (Jiang et al., 2018).
In general, TRPC6 channels are extensively expressed in the CNS and have various crucial functions. Therefore, agents targeting TRPC6 may have potential for the cure of brain disorders.
TRPC6 and Ischemic Stroke
During the process of ischemic stroke, increasing evidences support that TRPC6 seems to be a protective pathway. In comparison with TRPC1/3/4/5, TRPC6 is significantly degraded in neurons following exposure to ischemia, resulting in ischemic neuronal death. The maintenance of TRPC6 expression via CREB-dependent mechanisms has a neuroprotective effect, and has potential as a protective strategy against ischemic stroke (Du et al., 2010). In addition, TRPC6 suppresses NMDAR-induced Ca2+ elevation and neuronal excitotoxicity, and protects neurons from ischemic brain injury (Li et al., 2012). Resveratrol, neuroprotectin D1, and the main compound of green tea, (−)-epigallocatechin-3-gallate, inhibit calpain-mediated TRPC6 proteolysis and activate MEK/ERK or CaMKIV-dependent CREB pathways, therefore improving neurological status in experimental stroke (Lin et al., 2013a; Yao et al., 2013, 2014). Particularly, the TRPC6 activator, hyperforin, also contributes to neuroprotection after ischemic stroke by blocking TRPC6 degradation accompanied by elevation of phosphorylated CREB in CaMKIV and Ras/MEK/ERK-dependent mechanisms (Lin et al., 2013b). Furthermore, calycosin increases neuronal TRPC6 and phosphorylated CREB expression in response to brain ischemic damage (Guo et al., 2017). We believe that the TRPC6-CaMKIV-CREB pathway may be an important mechanism of ischemic stroke (Figure 2). There is increasing research interest in stem cell therapy for ischemic stroke. Animal researches have indicated that bone marrow-derived stromal cells (BMSCs) improve outcomes in stroke, although the regulatory mechanisms have yet to be clarified (Yang et al., 2014). Surprisingly, TRPC6 channels have been shown to participate in the neuroprotective effect of BMSCs and oxiracetam combination therapies in acute brain IR damage (Wang et al., 2019). Furthermore, overexpression of TRPC6 in BMSCs improves neuronal functions in rats after ischemic stroke, which is associated with BDNF/CREB pathway (Li W. et al., 2019). The inflammatory response plays a critical role in the pathophysiology of ischemic stroke. The proinflammatory cytokine, interleukin (IL)-17A, was shown to be linked with brain IR injury (Gelderblom et al., 2012). Another study suggested that TRPC6 may act downstream of IL-17A, and indicated that IL-17A could promote degradation of TRPC6, thus exacerbating cerebral IR injury (Zhang et al., 2014). Taken together, the evidences outlined above indicate that TRPC6 has a positive function in neuroprotection and that TRPC6 expression is reduced during ischemic stroke. However, it should be noted that TRPC6 may also have harmful effects on neurons following cerebral IR injury. In contrast to previous studies, TRPC6 expression was proved to be increased in wild-type mice neurons after ischemic stroke. Increased TRPC6 levels or overexpression of TRPC6 exacerbated IR injury-induced infarct volume, Ca2+ elevation, and neuronal death (Chen J. et al., 2017). Deletion of TRPC3/6/7 was shown to decrease activation of the proapoptotic factor and nuclear factor (NF)-κB, attenuate NF-κB nuclear translocation, and enhance activation of PKB, finally leading to resistance against cerebral IR injury (Chen X. et al., 2017). The causes for these discrepancies among studies remain unclear; however, they might have been due to species differences, distinct animal ischemic stroke models, dissimilar experimental environments, and researchers. Therefore, more studies are required to clarify the specific function of the TRPC6 channel in ischemic stroke.
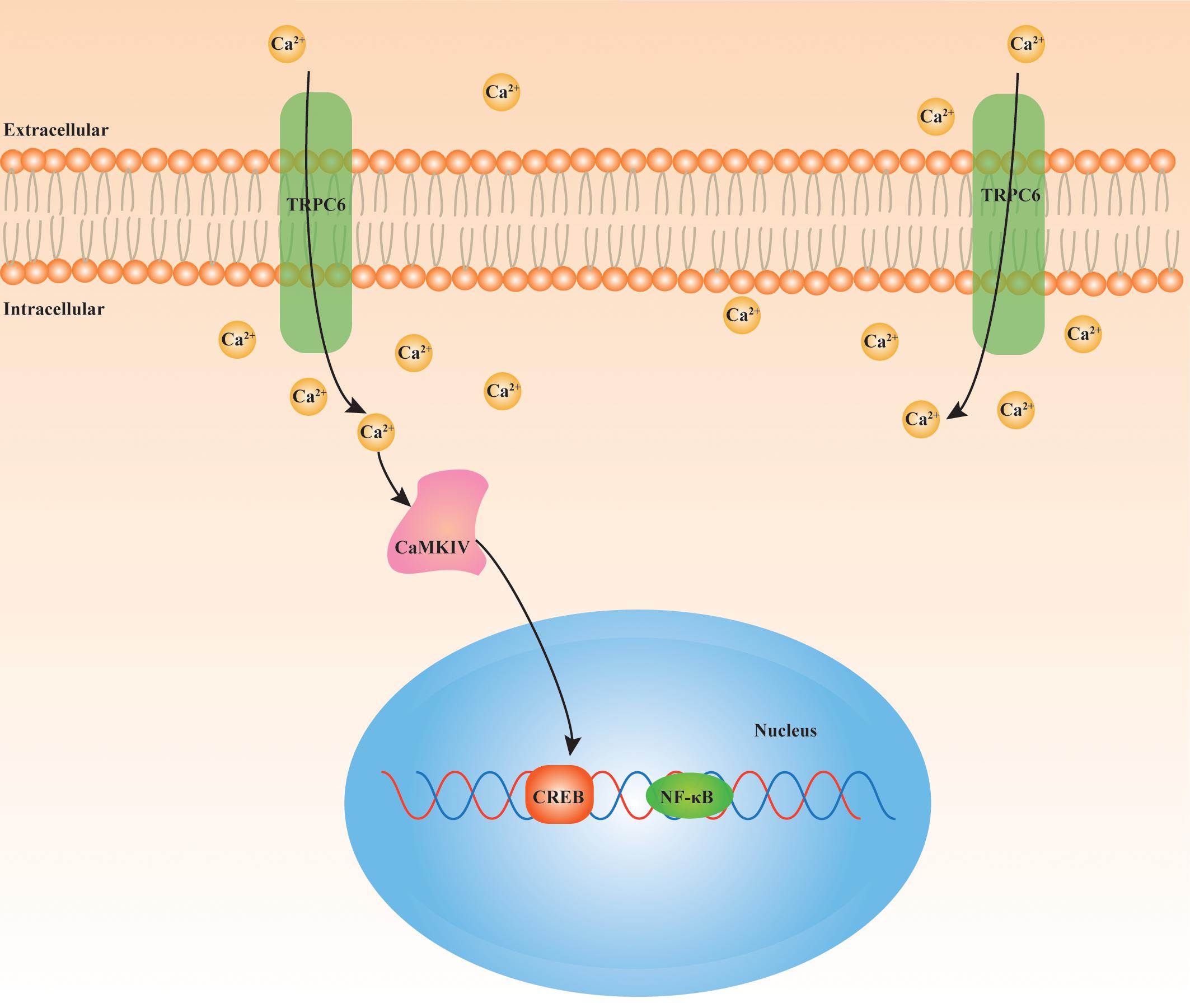
Figure 2. Underlying signaling cascade of TRPC6 in ischemic stroke. TRPC6/Ca2+/CAMKIV/CREB pathway is closely involved in the pathophysiological mechanism of cerebral IR injury. TRPC6: transient receptor potential canonical channel 6; Ca2+: calcium; CAMKIV: Ca2+/calmodulin-dependent kinase IV; CREB: cAMP-response element binding protein; NF-κB: nuclear factor (NF)-κB.
The evidence discussed here indicates that compounds targeting TRPC6 may have clinical potential as novel agents for prevention and treatment of ischemic stroke. It should be noted that interventions for most of the experiments mentioned above are performed under intracerebroventricular injection or in vitro cell condition. However, taking into consideration that TRPC6 is broadly distributed in human tissues (Riccio et al., 2002), both agonists and antagonists of TRPC6 might bring some systematic adverse effects. Besides, hyperforin had been shown to induce TRPC6-dependent Na+ currents and entry and subsequently decrease the Na+ gradients across the membrane thus may inhibit the neuronal amine uptake (Leuner et al., 2007; Wang et al., 2020). Furthermore, hyperforin could also function as a protonophore and generate H+ currents in a TRPC6-independent way in several cells (cortical microglial cells, chromaffin cells, lipid bilayers, and HEK-293 cells), and thereby suppress the neurotransmitter uptake through sodium-proton exchangers (Sell et al., 2014). Thus, many factors should be thoroughly considered before studies are conducted to explore the effect TRPC6 on ischemic stroke.
Conclusion and Perspective
Due to its high prevalence adjuvant and novel treatments for ischemic stroke are all under active investigation; e.g., digital therapeutics, microRNA-based therapeutics, cell therapy, and exosome therapy (Sun et al., 2018; Venkat et al., 2018a, b; Bayraktutan, 2019; Choi et al., 2019). Despite advances in therapy, stroke remains a major health issue. The studies discussed in this review suggest that investigation of the TRPC and TRPC6 channels may hold promise for the development of novel directions in ischemic stroke therapies. We have emphasized some important clues regarding the involved mechanisms of TRPC6 in physiology and pathophysiology of cerebral ischemia. More data and solid evidence of TRPCs and TRPC6 in stroke therapy are urgently needed to bridge the gap between current pre-clinical medical researches and clinical practice. Further studies are required to support the speculations regarding the promising therapeutic roles of TRPCs and TRPC6 in ischemic stroke, and to determine the mechanisms underlying these roles.
Author Contributions
LL wrote this manuscript. SZ and XX designed the general idea. All authors edited the drafts of the manuscript.
Funding
This study was supported by the National Natural Science Foundation of China No. 81771194 to SZ and No. 81870939 to XX.
Conflict of Interest
The authors declare that the research was conducted in the absence of any commercial or financial relationships that could be construed as a potential conflict of interest.
References
Andrews, M. D., Af Forselles, K., Beaumont, K., Galan, S. R., Glossop, P. A., Grenie, M., et al. (2015). Discovery of a selective TRPM8 antagonist with clinical efficacy in cold-related pain. ACS Med. Chem. Lett. 6, 419–424. doi: 10.1021/ml500479v
Arendt-Nielsen, L., Harris, S., Whiteside, G. T., Hummel, M., Knappenberger, T., O’Keefe, S., et al. (2016). A randomized, double-blind, positive-controlled, 3-way cross-over human experimental pain study of a TRPV1 antagonist (V116517) in healthy volunteers and comparison with preclinical profile. Pain 157, 2057–2067. doi: 10.1097/j.pain.0000000000000610
Bautista, D. M., Pellegrino, M., and Tsunozaki, M. (2013). TRPA1: a gatekeeper for inflammation. Annu. Rev. Physiol. 75, 181–200. doi: 10.1146/annurev-physiol-030212-183811
Bayraktutan, U. (2019). Endothelial progenitor cells: potential novel therapeutics for ischaemic stroke. Pharmacol. Res. 144, 181–191. doi: 10.1016/j.phrs.2019.04.017
Blair, H. A. (2018). Capsaicin 8% dermal patch: a review in peripheral neuropathic pain. Drugs 78, 1489–1500. doi: 10.1007/s40265-018-0982-7
Bouron, A., Chauvet, S., Dryer, S., and Rosado, J. A. (2016). Second messenger-operated calcium entry through TRPC6. Adv. Exp. Med. Biol. 898, 201–249. doi: 10.1007/978-3-319-26974-0_10
Broker-Lai, J., Kollewe, A., Schindeldecker, B., Pohle, J., Nguyen Chi, V., Mathar, I., et al. (2017). Heteromeric channels formed by TRPC1, TRPC4 and TRPC5 define hippocampal synaptic transmission and working memory. EMBO J. 36, 2770–2789. doi: 10.15252/embj.201696369
Brown, W., Leff, R. L., Griffin, A., Hossack, S., Aubray, R., Walker, P., et al. (2017). Safety, pharmacokinetics, and Pharmacodynamics study in healthy subjects of oral NEO6860, a modality selective transient receptor potential vanilloid subtype 1 antagonist. J. Pain 18, 726–738. doi: 10.1016/j.jpain.2017.01.009
Chen, J., Li, Z., Hatcher, J. T., Chen, Q. H., Chen, L., Wurster, R. D., et al. (2017). Deletion of TRPC6 attenuates NMDA receptor-mediated Ca2+ Entry and Ca2+-induced neurotoxicity following cerebral ischemia and oxygen-glucose deprivation. Front. Neurosci. 11:138. doi: 10.3389/fnins.2017.00138
Chen, J. M., Li, Q. W., Liu, J. S., Jiang, G. X., Liu, J. R., Chen, S. D., et al. (2019). TRPC6 mRNA levels in peripheral leucocytes of patients with Alzheimer’s disease and mild cognitive impairment: a case-control study. Prog. Neuropsychopharmacol. Biol. Psychiatry 92, 279–284. doi: 10.1016/j.pnpbp.2019.01.009
Chen, X., Lu, M., He, X., Ma, L., Birnbaumer, L., and Liao, Y. (2017). TRPC3/6/7 knockdown protects the brain from cerebral ischemia injury via astrocyte apoptosis inhibition and effects on NF-small ka. CyrillicB Translocation. Mol. Neurobiol. 54, 7555–7566. doi: 10.1007/s12035-016-0227-2
Choi, D. W. (1995). Calcium: still center-stage in hypoxic-ischemic neuronal death. Trends Neurosci. 18, 58–60. doi: 10.1016/0166-2236(95)80018-w
Choi, M. J., Kim, H., Nah, H. W., and Kang, D. W. (2019). Digital therapeutics: emerging new therapy for neurologic deficits after stroke. J. Stroke 21, 242–258. doi: 10.5853/jos.2019.01963
Clapham, D. E. (2003). TRP channels as cellular sensors. Nature 426, 517–524. doi: 10.1038/nature02196
Clapham, D. E., Runnels, L. W., and Strubing, C. (2001). The TRP ion channel family. Nat. Rev. Neurosci. 2, 387–396. doi: 10.1038/35077544
Du, W., Huang, J., Yao, H., Zhou, K., Duan, B., and Wang, Y. (2010). Inhibition of TRPC6 degradation suppresses ischemic brain damage in rats. J. Clin. Investig. 120, 3480–3492. doi: 10.1172/jci43165
El Boustany, C., Bidaux, G., Enfissi, A., Delcourt, P., Prevarskaya, N., and Capiod, T. (2008). Capacitative calcium entry and transient receptor potential canonical 6 expression control human hepatoma cell proliferation. Hepatology 47, 2068–2077. doi: 10.1002/hep.22263
Estacion, M., Sinkins, W. G., Jones, S. W., Applegate, M. A., and Schilling, W. P. (2006). Human TRPC6 expressed in HEK 293 cells forms non-selective cation channels with limited Ca2+ permeability. J. Physiol. 572, 359–377. doi: 10.1113/jphysiol.2005.103143
Gelderblom, M., Weymar, A., Bernreuther, C., Velden, J., Arunachalam, P., Steinbach, K., et al. (2012). Neutralization of the IL-17 axis diminishes neutrophil invasion and protects from ischemic stroke. Blood 120, 3793–3802. doi: 10.1182/blood-2012-02-412726
Ginsberg, M. D. (2008). Neuroprotection for ischemic stroke: past, present and future. Neuropharmacology 55, 363–389. doi: 10.1016/j.neuropharm.2007.12.007
GlaxoSmithKline (2018). A Study to Evaluate the Effect of the Transient Receptor Potential Vanilloid 4 (TRPV4) Channel Blocker, GSK2798745, on Pulmonary Gas Transfer and Respiration in Patients With Congestive Heart Failure. Available online at: https://clinicaltrials.gov/ct2/show/NCT02497937 (accessed October 25, 2019).
GlaxoSmithKline (2019). A Method Validation Study for Evaluation of Novel Treatments Limiting Pulmonary Oedema in Cardiac Failure. Available online at: https://clinicaltrials.gov/ct2/show/NCT02135861?term=trpv4&rank=2 (accessed October 25, 2019).
Gosset, J. R., Beaumont, K., Matsuura, T., Winchester, W., Attkins, N., Glatt, S., et al. (2017). A cross-species translational pharmacokinetic-pharmacodynamic evaluation of core body temperature reduction by the TRPM8 blocker PF-05105679. Eur. J. Pharm. 109S, S161–S167. doi: 10.1016/j.ejps.2017.06.009
Griesi-Oliveira, K., Acab, A., Gupta, A. R., Sunaga, D. Y., Chailangkarn, T., Nicol, X., et al. (2015). Modeling non-syndromic autism and the impact of TRPC6 disruption in human neurons. Mol. Psychiatry 20, 1350–1365. doi: 10.1038/mp.2014.141
Guo, C., Ma, Y., Ma, S., Mu, F., Deng, J., Duan, J., et al. (2017). The role of TRPC6 in the neuroprotection of calycosin against cerebral ischemic injury. Sci. Rep. 7:3039. doi: 10.1038/s41598-017-03404-6
Hartmann, J., and Konnerth, A. (2015). TRPC3-dependent synaptic transmission in central mammalian neurons. J. Mol. Med. 93, 983–989. doi: 10.1007/s00109-015-1298-7
He, X., Li, S., Liu, B., Susperreguy, S., Formoso, K., Yao, J., et al. (2017). Major contribution of the 3/6/7 class of TRPC channels to myocardial ischemia/reperfusion and cellular hypoxia/reoxygenation injuries. Proc. Natl, Acad. Sci. U.S.A. 114, E4582–E4591. doi: 10.1073/pnas.1621384114
Heiser, J. H., Schuwald, A. M., Sillani, G., Ye, L., Muller, W. E., and Leuner, K. (2013). TRPC6 channel-mediated neurite outgrowth in PC12 cells and hippocampal neurons involves activation of RAS/MEK/ERK, PI3K, and CAMKIV signaling. J. Neurochem. 127, 303–313. doi: 10.1111/jnc.12376
Hirschler-Laszkiewicz, I., Tong, Q., Waybill, K., Conrad, K., Keefer, K., Zhang, W., et al. (2011). The transient receptor potential (TRP) channel TRPC3 TRP domain and AMP-activated protein kinase binding site are required for TRPC3 activation by erythropoietin. J. Biol. Chem. 286, 30636–30646. doi: 10.1074/jbc.M111.238360
Hofmann, T., Obukhov, A. G., Schaefer, M., Harteneck, C., Gudermann, T., and Schultz, G. (1999). Direct activation of human TRPC6 and TRPC3 channels by diacylglycerol. Nature 397, 259–263. doi: 10.1038/16711
Hong, C., Seo, H., Kwak, M., Jeon, J., Jang, J., Jeong, E. M., et al. (2015). Increased TRPC5 glutathionylation contributes to striatal neuron loss in Huntington’s disease. Brain 138, 3030–3047. doi: 10.1093/brain/awv188
Huang, W. C., Young, J. S., and Glitsch, M. D. (2007). Changes in TRPC channel expression during postnatal development of cerebellar neurons. Cell Calcium 42, 1–10. doi: 10.1016/j.ceca.2006.11.002
Islam, Z., Nagampalli, R. S. K., Fatima, M. T., and Ashraf, G. M. (2018). New paradigm in ankyrin repeats: beyond protein-protein interaction module. Int. J. Biol. Macromol. 109, 1164–1173. doi: 10.1016/j.ijbiomac.2017.11.101
Jia, Y., Zhou, J., Tai, Y., and Wang, Y. (2007). TRPC channels promote cerebellar granule neuron survival. Nat. Neurosci. 10, 559–567. doi: 10.1038/nn1870
Jiang, Z., Yue, W. W. S., Chen, L., Sheng, Y., and Yau, K. W. (2018). Cyclic-nucleotide- and HCN-channel-mediated phototransduction in intrinsically photosensitive retinal ganglion cells. Cell 175, 652–664.e12. doi: 10.1016/j.cell.2018.08.055
Kaneko, Y., and Szallasi, A. (2014). Transient receptor potential (TRP) channels: a clinical perspective. Br. J. Pharmacol. 171, 2474–2507. doi: 10.1111/bph.12414
Khoshnam, S. E., Winlow, W., Farzaneh, M., Farbood, Y., and Moghaddam, H. F. (2017). Pathogenic mechanisms following ischemic stroke. Neurol. Sci. 38, 1167–1186. doi: 10.1007/s10072-017-2938-1
Lai, T. W., Shyu, W. C., and Wang, Y. T. (2011). Stroke intervention pathways: NMDA receptors and beyond. Trends Mol. Med. 17, 266–275. doi: 10.1016/j.molmed.2010.12.008
Lee, J., Kim, B. H., Yu, K. S., Kim, H. S., Kim, J. D., Cho, J. Y., et al. (2017). A first-in-human, double-blind, placebo-controlled, randomized, dose escalation study of DWP05195, a novel TRPV1 antagonist, in healthy volunteers. Drug Des. Dev. Ther. 11, 1301–1313. doi: 10.2147/dddt.S128727
Lepage, P. K., Lussier, M. P., McDuff, F. O., Lavigne, P., and Boulay, G. (2009). The self-association of two N-terminal interaction domains plays an important role in the tetramerization of TRPC4. Cell Calcium 45, 251–259. doi: 10.1016/j.ceca.2008.11.002
Leuner, K., Kazanski, V., Muller, M., Essin, K., Henke, B., Gollasch, M., et al. (2007). Hyperforin–a key constituent of St. John’s wort specifically activates TRPC6 channels. FASEB J. 21, 4101–4111. doi: 10.1096/fj.07-8110com
Leuner, K., Li, W., Amaral, M. D., Rudolph, S., Calfa, G., Schuwald, A. M., et al. (2013). Hyperforin modulates dendritic spine morphology in hippocampal pyramidal neurons by activating Ca2+ -permeable TRPC6 channels. Hippocampus 23, 40–52. doi: 10.1002/hipo.22052
Li, H., Huang, J., Du, W., Jia, C., Yao, H., and Wang, Y. (2012). TRPC6 inhibited NMDA receptor activities and protected neurons from ischemic excitotoxicity. J. Neurochem. 123, 1010–1018. doi: 10.1111/jnc.12045
Li, J., Zhang, X., Song, X., Liu, R., Zhang, J., and Li, Z. (2019). The structure of TRPC ion channels. Cell Calcium 80, 25–28. doi: 10.1016/j.ceca.2019.03.005
Li, M., Liu, E., Zhou, Q., Li, S., Wang, X., Liu, Y., et al. (2018). TRPC1 null exacerbates memory deficit and apoptosis induced by amyloid-beta. J. Alzheimers Dis. 63, 761–772. doi: 10.3233/jad-180077
Li, W., Yang, F., Gao, J., Tang, Y., Wang, J., and Pan, Y. (2019). Over-expression of TRPC6 via CRISPR based synergistic activation mediator in BMSCs ameliorates brain injury in a rat model of cerebral ischemia/reperfusion. Neuroscience 415, 147–160. doi: 10.1016/j.neuroscience.2019.06.041
Li, Y., Jia, Y. C., Cui, K., Li, N., Zheng, Z. Y., Wang, Y. Z., et al. (2005). Essential role of TRPC channels in the guidance of nerve growth cones by brain-derived neurotrophic factor. Nature 434, 894–898. doi: 10.1038/nature03477
Liedtke, W., Choe, Y., Marti-Renom, M. A., Bell, A. M., Denis, C. S., Sali, A., et al. (2000). Vanilloid receptor-related osmotically activated channel (VR-OAC), a candidate vertebrate osmoreceptor. Cell 103, 525–535. doi: 10.1016/s0092-8674(00)00143-4
Lin, Y., Chen, F., Zhang, J., Wang, T., Wei, X., Wu, J., et al. (2013a). Neuroprotective effect of resveratrol on ischemia/reperfusion injury in rats through TRPC6/CREB pathways. J. Mol. Neurosci. 50, 504–513. doi: 10.1007/s12031-013-9977-8
Liu, Y., Liu, C., Qin, X., Zhu, M., and Yang, Z. (2015). The change of spatial cognition ability in depression rat model and the possible association with down-regulated protein expression of TRPC6. Behav. Brain Res. 294, 186–193. doi: 10.1016/j.bbr.2015.07.062
Lin, Y., Zhang, J. C., Fu, J., Chen, F., Wang, J., Wu, Z. L., et al. (2013b). Hyperforin attenuates brain damage induced by transient middle cerebral artery occlusion (MCAO) in rats via inhibition of TRPC6 channels degradation. J. Cereb. Blood Flow Metab. 33, 253–262. doi: 10.1038/jcbfm.2012.164
Louhivuori, L. M., Jansson, L., Nordstrom, T., Bart, G., Nasman, J., and Akerman, K. E. (2010). Selective interference with TRPC3/6 channels disrupts OX1 receptor signalling via NCX and reveals a distinct calcium influx pathway. Cell Calcium 48, 114–123. doi: 10.1016/j.ceca.2010.07.005
Lussier, M. P., Lepage, P. K., Bousquet, S. M., and Boulay, G. (2008). RNF24, a new TRPC interacting protein, causes the intracellular retention of TRPC. Cell Calcium 43, 432–443. doi: 10.1016/j.ceca.2007.07.009
Manitpisitkul, P., Shalayda, K., Russell, L., Sanga, P., Solanki, B., Caruso, J., et al. (2018). Pharmacokinetics and Safety of Mavatrep (JNJ-39439335), a TRPV1 antagonist in healthy japanese and caucasian men: a double-blind, randomized, placebo-controlled, sequential-group phase 1 study. Clin. Pharmacol. Drug Dev. 7, 712–726. doi: 10.1002/cpdd.413
Massart, J., and Zierath, J. R. (2019). Role of diacylglycerol kinases in glucose and energy homeostasis. Trends Endocrinol. Metab. 30, 603–617. doi: 10.1016/j.tem.2019.06.003
Minke, B. (1977). Drosophila mutant with a transducer defect. Biophys. Struct. Mech. 3, 59–64. doi: 10.1007/bf00536455
Montell, C. (2001). Physiology, phylogeny, and functions of the TRP superfamily of cation channels. Sciences STKE 2001:re1. doi: 10.1126/stke.2001.90.re1
Montell, C., Jones, K., Hafen, E., and Rubin, G. (1985). Rescue of the Drosophila phototransduction mutation trp by germline transformation. Science 230, 1040–1043. doi: 10.1126/science.3933112
Moqrich, A., Hwang, S. W., Earley, T. J., Petrus, M. J., Murray, A. N., Spencer, K. S., et al. (2005). Impaired thermosensation in mice lacking TRPV3, a heat and camphor sensor in the skin. Science 307, 1468–1472. doi: 10.1126/science.1108609
Moran, M. M. (2018). TRP channels as potential drug targets. Annu. Rev. Pharmacol. Toxicol. 58, 309–330. doi: 10.1146/annurev-pharmtox-010617-052832
Narayanan, K. L., Irmady, K., Subramaniam, S., Unsicker, K., and von Bohlen und Halbach, O. (2008). Evidence that TRPC1 is involved in hippocampal glutamate-induced cell death. Neurosci. Lett. 446, 117–122. doi: 10.1016/j.neulet.2008.09.034
Nilius, B., Owsianik, G., Voets, T., and Peters, J. A. (2007). Transient receptor potential cation channels in disease. Physiol. Rev. 87, 165–217. doi: 10.1152/physrev.00021.2006
Owsianik, G., Talavera, K., Voets, T., and Nilius, B. (2006). Permeation and selectivity of TRP channels. Annu. Rev. Physiol. 68, 685–717. doi: 10.1146/annurev.physiol.68.040204.101406
Pharmaceuticals, G. (2014). Glenmark’s TRPA1 Antagonist ‘GRC 17536’ Shows Positive Data in a Proof of Concept Study. Available online at: https://www.prnewswire.com/news-releases/glenmarks-trpa1-antagonist-grc-17536-shows-positive-data-in-a-proof-of-concept-study-275445961.html (accessed October 25, 2019).
Pharmaceuticals, G. (2018). Glenmark Pharma Completes ‘GRC 15300’ Phase-I Clinical Trials. Available online at: http://www.thehindubusinessline.com/companies/glenmark-pharma-completes-grc15300-phasei-clinical-trials/article2292593.ece (accessed October 25, 2019).
Popugaeva, E., Chernyuk, D., Zhang, H., Postnikova, T. Y., Pats, K., Fedorova, E., et al. (2019). Derivatives of Piperazines as potential therapeutic agents for Alzheimer’s disease. Mol. Pharmacol. 95, 337–348. doi: 10.1124/mol.118.114348
Qu, Z., Wang, Y., Li, X., Wu, L., and Wang, Y. (2017). TRPC6 expression in neurons is differentially regulated by NR2A- and NR2B-containing NMDA receptors. J. Neurochem. 143, 282–293. doi: 10.1111/jnc.14215
Quick, K., Zhao, J., Eijkelkamp, N., Linley, J. E., Rugiero, F., Cox, J. J., et al. (2012). TRPC3 and TRPC6 are essential for normal mechanotransduction in subsets of sensory neurons and cochlear hair cells. Open Biol. 2:120068. doi: 10.1098/rsob.120068
Ramirez, G. A., Coletto, L. A., Sciorati, C., Bozzolo, E. P., Manunta, P., Rovere-Querini, P., et al. (2018). Ion channels and transporters in inflammation: special focus on TRP channels and TRPC6. Cells 7:E70. doi: 10.3390/cells7070070
Riccio, A., Li, Y., Moon, J., Kim, K. S., Smith, K. S., Rudolph, U., et al. (2009). Essential role for TRPC5 in amygdala function and fear-related behavior. Cell 137, 761–772. doi: 10.1016/j.cell.2009.03.039
Riccio, A., Medhurst, A. D., Mattei, C., Kelsell, R. E., Calver, A. R., Randall, A. D., et al. (2002). mRNA distribution analysis of human TRPC family in CNS and peripheral tissues. Brain Res. 109, 95–104. doi: 10.1016/s0169-328x(02)00527-2
Schindl, R., Frischauf, I., Kahr, H., Fritsch, R., Krenn, M., Derndl, A., et al. (2008). The first ankyrin-like repeat is the minimum indispensable key structure for functional assembly of homo- and heteromeric TRPC4/TRPC5 channels. Cell Calcium 43, 260–269. doi: 10.1016/j.ceca.2007.05.015
Sell, T. S., Belkacemi, T., Flockerzi, V., and Beck, A. (2014). Protonophore properties of hyperforin are essential for its pharmacological activity. Sci. Rep. 4:7500. doi: 10.1038/srep07500
Shen, H., Pan, J., Pan, L., and Zhang, N. (2013). TRPC6 inhibited NMDA current in cultured hippocampal neurons. Neuromol. Med. 15, 389–395. doi: 10.1007/s12017-013-8226-1
Strotmann, R., Harteneck, C., Nunnenmacher, K., Schultz, G., and Plant, T. D. (2000). OTRPC4, a nonselective cation channel that confers sensitivity to extracellular osmolarity. Nat. Cell Biol. 2, 695–702. doi: 10.1038/35036318
Sun, P., Liu, D. Z., Jickling, G. C., Sharp, F. R., and Yin, K. J. (2018). MicroRNA-based therapeutics in central nervous system injuries. J. Cereb. Blood Flow Metab. 38, 1125–1148. doi: 10.1177/0271678x18773871
Szallasi, A., Cruz, F., and Geppetti, P. (2006). TRPV1: a therapeutic target for novel analgesic drugs? Trends Mol. Med. 12, 545–554. doi: 10.1016/j.molmed.2006.09.001
Szydlowska, K., and Tymianski, M. (2010). Calcium, ischemia and excitotoxicity. Cell Calcium 47, 122–129. doi: 10.1016/j.ceca.2010.01.003
Tai, Y., Feng, S., Du, W., and Wang, Y. (2009). Functional roles of TRPC channels in the developing brain. Pflugers Arch. 458, 283–289. doi: 10.1007/s00424-008-0618-y
Tai, Y., Feng, S., Ge, R., Du, W., Zhang, X., He, Z., et al. (2008). TRPC6 channels promote dendritic growth via the CaMKIV-CREB pathway. J. Cell Sci. 121, 2301–2307. doi: 10.1242/jcs.026906
Tang, Q., Guo, W., Zheng, L., Wu, J. X., Liu, M., Zhou, X., et al. (2018). Structure of the receptor-activated human TRPC6 and TRPC3 ion channels. Cell Res. 28, 746–755. doi: 10.1038/s41422-018-0038-2
Van Gerven, L., Alpizar, Y. A., Steelant, B., Callebaut, I., Kortekaas Krohn, I., Wouters, M., et al. (2017). Enhanced chemosensory sensitivity in patients with idiopathic rhinitis and its reversal by nasal capsaicin treatment. J. Allergy Clin. Immunol. 140, 437–446.e2. doi: 10.1016/j.jaci.2017.03.014
Venkat, P., Chen, J., and Chopp, M. (2018a). Exosome-mediated amplification of endogenous brain repair mechanisms and brain and systemic organ interaction in modulating neurological outcome after stroke. J. Cereb. Blood Flow Metab. 38, 2165–2178. doi: 10.1177/0271678x18782789
Venkat, P., Shen, Y., Chopp, M., and Chen, J. (2018b). Cell-based and pharmacological neurorestorative therapies for ischemic stroke. Neuropharmacology 134, 310–322. doi: 10.1016/j.neuropharm.2017.08.036
Venkatachalam, K., and Montell, C. (2007). TRP channels. Annu. Rev. Biochem. 76, 387–417. doi: 10.1146/annurev.biochem.75.103004.142819
Voets, T., Vriens, J., and Vennekens, R. (2019). Targeting TRP channels - valuable alternatives to combat pain, lower urinary tract disorders, and type 2 diabetes? Trends Pharmacol. Sci. 40, 669–683. doi: 10.1016/j.tips.2019.07.004
Wang, H., Cheng, X., Tian, J., Xiao, Y., Tian, T., Xu, F., et al. (2020). TRPC channels: structure, function, regulation and recent advances in small molecular probes. Pharmacol. Ther. doi: 10.1016/j.pharmthera.2020.107497 [Epub ahead of print].
Wang, J., Lu, R., Yang, J., Li, H., He, Z., Jing, N., et al. (2015). TRPC6 specifically interacts with APP to inhibit its cleavage by gamma-secretase and reduce Abeta production. Nat. Commun. 6:8876. doi: 10.1038/ncomms9876
Wang, J., Sun, R., Li, Z., and Pan, Y. (2019). Combined bone marrow stromal cells and oxiracetam treatments ameliorates acute cerebral ischemia/reperfusion injury through TRPC6. Acta Biochim. Biophys. Sin. 51, 767–777. doi: 10.1093/abbs/gmz059
Weissmann, N., Sydykov, A., Kalwa, H., Storch, U., Fuchs, B., Mederos y Schnitzler, M., et al. (2012). Activation of TRPC6 channels is essential for lung ischaemia-reperfusion induced oedema in mice. Nat. Commun. 3:649. doi: 10.1038/ncomms1660
Winn, M. P., Conlon, P. J., Lynn, K. L., Farrington, M. K., Creazzo, T., Hawkins, A. F., et al. (2005). A mutation in the TRPC6 cation channel causes familial focal segmental glomerulosclerosis. Science 308, 1801–1804. doi: 10.1126/science.1106215
Woo, J. S., Lee, K. J., Huang, M., Cho, C. H., and Lee, E. H. (2014). Heteromeric TRPC3 with TRPC1 formed via its ankyrin repeats regulates the resting cytosolic Ca2+ levels in skeletal muscle. Biochem. Biophys. Res. Commun. 446, 454–459. doi: 10.1016/j.bbrc.2014.02.127
Wouters, M. M., Balemans, D., Van Wanrooy, S., Dooley, J., Cibert-Goton, V., Alpizar, Y. A., et al. (2016). Histamine receptor H1-mediated sensitization of TRPV1 mediates visceral hypersensitivity and symptoms in patients with irritable bowel syndrome. Gastroenterology 150, 875–887.e9. doi: 10.1053/j.gastro.2015.12.034
Wu, J., Ryskamp, D., Birnbaumer, L., and Bezprozvanny, I. (2018). Inhibition of TRPC1-dependent store-operated calcium entry improves synaptic stability and motor performance in a mouse model of Huntington’s disease. J. Huntingtons Dis. 7, 35–50. doi: 10.3233/jhd-170266
Wu, J., Shih, H. P., Vigont, V., Hrdlicka, L., Diggins, L., Singh, C., et al. (2011). Neuronal store-operated calcium entry pathway as a novel therapeutic target for Huntington’s disease treatment. Chem. Biol. 18, 777–793. doi: 10.1016/j.chembiol.2011.04.012
Wu, Q. J., and Tymianski, M. (2018). Targeting NMDA receptors in stroke: new hope in neuroprotection. Mol. Brain 11:15. doi: 10.1186/s13041-018-0357-8
Wu, Q. Y., Sun, M. R., Wu, C. L., Li, Y., Du, J. J., Zeng, J. Y., et al. (2015). Activation of calcium-sensing receptor increases TRPC3/6 expression in T lymphocyte in sepsis. Mol. Immunol. 64, 18–25. doi: 10.1016/j.molimm.2014.10.018
Xu, H., Ramsey, I. S., Kotecha, S. A., Moran, M. M., Chong, J. A., Lawson, D., et al. (2002). TRPV3 is a calcium-permeable temperature-sensitive cation channel. Nature 418, 181–186. doi: 10.1038/nature00882
Xu, N., Meng, H., Liu, T., Feng, Y., Qi, Y., and Wang, H. (2018). TRPC1 deficiency exacerbates cerebral ischemia/reperfusion-induced neurological injury by potentiating Nox4-derived reactive oxygen species generation. Cell. Physiol. Biochem. 51, 1723–1738. doi: 10.1159/000495676
Yang, L. P., Jiang, F. J., Wu, G. S., Deng, K., Wen, M., Zhou, X., et al. (2015). Acute treatment with a novel TRPC4/C5 channel inhibitor produces antidepressant and anxiolytic-like effects in mice. PLoS One 10:e0136255. doi: 10.1371/journal.pone.0136255
Yang, X. W., Grossman, R. B., and Xu, G. (2018). Research progress of polycyclic polyprenylated acylphloroglucinols. Chem. Rev. 118, 3508–3558. doi: 10.1021/acs.chemrev.7b00551
Yang, Z., Zhu, L., Li, F., Wang, J., Wan, H., and Pan, Y. (2014). Bone marrow stromal cells as a therapeutic treatment for ischemic stroke. Neurosci. Bull. 30, 524–534. doi: 10.1007/s12264-013-1431-y
Yao, C., Zhang, J., Chen, F., and Lin, Y. (2013). Neuroprotectin D1 attenuates brain damage induced by transient middle cerebral artery occlusion in rats through TRPC6/CREB pathways. Mol. Med. Rep. 8, 543–550. doi: 10.3892/mmr.2013.1543
Yao, C., Zhang, J., Liu, G., Chen, F., and Lin, Y. (2014). Neuroprotection by (-)-epigallocatechin-3-gallate in a rat model of stroke is mediated through inhibition of endoplasmic reticulum stress. Mol. Med. Rep. 9, 69–76. doi: 10.3892/mmr.2013.1778
Yu, Y., Sweeney, M., Zhang, S., Platoshyn, O., Landsberg, J., Rothman, A., et al. (2003). PDGF stimulates pulmonary vascular smooth muscle cell proliferation by upregulating TRPC6 expression. Am. J. Physiol. Cell Physiol. 284, C316–C330. doi: 10.1152/ajpcell.00125.2002
Zhang, J., Mao, X., Zhou, T., Cheng, X., and Lin, Y. (2014). IL-17A contributes to brain ischemia reperfusion injury through calpain-TRPC6 pathway in mice. Neuroscience 274, 419–428. doi: 10.1016/j.neuroscience.2014.06.001
Zhou, J., Du, W., Zhou, K., Tai, Y., Yao, H., Jia, Y., et al. (2008). Critical role of TRPC6 channels in the formation of excitatory synapses. Nat. Neurosci. 11, 741–743. doi: 10.1038/nn.2127
Keywords: TRP ion channels, TRPCs, TRPC6 channel, neuronal survival, ischemic stroke
Citation: Liu L, Gu L, Chen M, Zheng Y, Xiong X and Zhu S (2020) Novel Targets for Stroke Therapy: Special Focus on TRPC Channels and TRPC6. Front. Aging Neurosci. 12:70. doi: 10.3389/fnagi.2020.00070
Received: 19 November 2019; Accepted: 26 February 2020;
Published: 18 March 2020.
Edited by:
George E. Barreto, University of Limerick, IrelandReviewed by:
Dafin F. Muresanu, Iuliu Haţieganu University of Medicine and Pharmacy, RomaniaStanislava Pankratova, University of Copenhagen, Denmark
Abraham Martin, Achucarro Basque Center for Neuroscience, Spain
Copyright © 2020 Liu, Gu, Chen, Zheng, Xiong and Zhu. This is an open-access article distributed under the terms of the Creative Commons Attribution License (CC BY). The use, distribution or reproduction in other forums is permitted, provided the original author(s) and the copyright owner(s) are credited and that the original publication in this journal is cited, in accordance with accepted academic practice. No use, distribution or reproduction is permitted which does not comply with these terms.
*Correspondence: Shengmei Zhu, c216aHUyMDA4OEB6anUuZWR1LmNu; Xiaoxing Xiong, eGlhb3hpbmd4aW9uZ0B3aHUuZWR1LmNu