- 1Department of Clinical Laboratory Sciences, College of Nursing and Allied Health Sciences, Howard University, Washington, DC, United States
- 2Department of Nutritional Sciences, College of Nursing and Allied Health Sciences, Howard University, Washington, DC, United States
- 3Department of Psychiatry, Howard University Hospital, Washington, DC, United States
- 4Department of Nursing, College of Nursing and Allied Health Sciences, Howard University, Washington, DC, United States
- 5Division of Geriatrics, Department of Medicine, Howard University Hospital, Washington, DC, United States
The ubiquitin proteasome system (UPS) and FOXOs transcription factors play a pivotal role in cellular clearance and minimizing the accumulation of Aβ in neurodegeneration (ND). In African Americans (AAs) with mild cognitive impairment (MCI), the role of components of UPS and FOXOs; and whether they are amenable to exercise effects is unknown. We hypothesized that exercise can enhance cellular clearance systems during aging and ND by increasing expressions of FBXO32 and FOXO1. To test this hypothesis, we used TaqMan gene expression analysis in peripheral blood (PB) to investigate the component of UPS and FOXOs; and provide mechanistic insight at baseline, during exercise, and in both genders. At baseline, levels of FBXO32 were higher in women than in men. In our attempt to discern gender-specific exercise-related changes, we observed that levels of FBXO32 increased in men but not in women. Similarly, levels of FOXO1 increased in men only. These data suggest that a graded dose of FBXO32 and FOXO1 may be beneficial when PB cells carrying FBXO32 and FOXO1 summon into the brain in response to Alzheimer’s disease (AD) perturbation (docking station PB cells). Our observation is consistent with emerging studies that exercise allows the trafficking of blood factors. Given the significance of FBXO32 and FOXO1 to ND and associated muscle integrity, our findings may explain, at least in part, the benefits of exercise on memory, associated gait, and balance perturbation acknowledged to herald the emergence of MCI.
Introduction
Early detection of Alzheimer’s diseases in an asymptomatic patient is a daunting task, as clinical symptoms develop long after preclinical pathogenic processes (Dubois et al., 2016; Wojsiat et al., 2017). The success of therapeutic methods relies on early diagnosis before the loss of neurons and dementia occur (Wojsiat et al., 2017). Conversely, the etiology of Alzheimer’s disease (AD) is linked with several manifestations that are physical, peripheral, and systemic (Morris et al., 2014). As a multifactorial disease, AD affects the CNS and peripheral systems (Morris et al., 2014), making one size fits all therapeutic strategies ineffective. These challenges call for a preventative approach, such as exercise intervention.
Evidence indicates that immune cells may play a role in AD pathogenesis (Hohsfield and Humpel, 2015; Wojsiat et al., 2017). Consequently, blood-derived cells and their infiltration (homing) into the CNS towards Aβ plaques have been proposed as therapeutic strategies against AD (Hohsfield and Humpel, 2015). For example, by eliminating Aβ deposits and preventing plaque formation, the anti-inflammatory subset of peripheral monocytes can enhance neurogenesis and produce reparative growth factors like insulin-like growth factor I (IGF-1; Gate et al., 2010; Malm et al., 2010; Schwartz and Shechter, 2010). This process is reminiscent of exercise training-induced increases in brain-derived neurotrophic factor (BDNF; Liu and Nusslock, 2018) and IGF-1 (Carro et al., 2001; Trejo et al., 2001) levels in the brain, and rejuvenate the brain, and improve memory. Further, recent reports implicate exercise to promote the trafficking of blood factors to the brain (Małkiewicz et al., 2019).
Prior studies have described the role of FBXO32 in muscle protein regulation, protein turnover (Kriegenburg et al., 2012), muscle atrophy (Attaix et al., 2005), and autophagy (Kostin et al., 2003) in skeletal muscles and cardiac myocytes (Zeng et al., 2013). However, whether this perturbation exists at the Mild Cognitive Impairment (MCI) phenotypic stage of AD needs elucidation. Although mechanisms linking gait and balance to neurodegeneration (ND) are unknown, decreased muscle coordination and deficits are now acknowledged early clinical phenotypes of MCI and prodromal AD (Gras et al., 2015; Goyal et al., 2019). Thus, it is unclear if FBXO32 is perturbed in older adults because of aging and pathogenesis of ND in older African Americans (AAs) with MCI; and if fitness adaptation mitigates these processes. This is important because exercise is acknowledged to improve muscle growth and quality and we have reported that Interleukin-4 (IL-4) promoted muscle fusion and growth via the IL-4α receptor on myoblasts (Schulze et al., 2005). The latter further suggest a link between exercise, blood factors and muscle dynamics.
The second focus was to gain insight into FOXO1 transcription factor. FOXO transcription factors are considered conserved regulators of longevity (Martins et al., 2016; Hwang et al., 2018), and coordinate genes involved in cellular metabolism and resistance to oxidative stress (Webb and Brunet, 2014). FOXOs promote the expression of genes involved in autophagy and the Ubiquitin Proteasome System (UPS; Webb and Brunet, 2014). FOXO and UPS play a pivotal role in preventing the accumulation of cells with damaged DNA and cancer (Webb and Brunet, 2014; Farhan et al., 2017). Several members of the FOXO transcription family regulate FBXO32 (Webb and Brunet, 2014; Dang et al., 2016). However, whether fitness adaptation exerts some of its advantageous effects through augmentation of cellular and protein clearance via the expression of FBXO32 and FOXO1 needs an investigation. Also, the concerted role of FBXO32 and FOXO1 in mitigating aging processes and ND has not been investigated in Peripheral Blood (PB) of AAs with MCI. Importantly, the cumulative incidence rate of neurodegenerative diseases like AD was twice among AA than Caucasians (Tang et al., 2001), while proteins and damaged organelles accumulate during neurodegenerative disorders and aging (Metaxakis et al., 2018). As age-related decline in the expression of FOXO could promote ND, perturbed gait, and balance (Hwang et al., 2018), we hypothesized that exercise can enhance the cellular clearance system, and mitigate much of the harm that accumulates in the cell over time during aging and ND by increasing the expression of FBXO32 and FOXO1.
Materials and Methods
The Howard University Institutional Review Board (IRB) approved the protocols used in this study. As required for studies involving human subjects, all participants completed a signed informed consent document before enrollment.
Randomization and Blinding
By design, the study employed a single-blind controlled approach. All staff, except those who directly monitored exercise-training, were blinded to group assignments. While impractical to blind the subjects, staff who performed cognitive assessments were blinded to group assignments. Of the 42 participants who completed baseline visits, 29 participants successfully proceeded beyond randomization; 23 participants completed the 6-month visit, but RNA was successfully extracted from 21 participants as indicated in the statistics and figure legend.
Study Participants
Study participants in this study were enrolled, as described in the GEMS-1 study (Iyalomhe et al., 2015). The sample for this analysis included 42 participants who had baseline data. Because a low level of physical activity was an inclusion criterion for the study, all participants were sedentary before enrollment. Other inclusion criteria included age ≥50 years and MCI diagnosis status (Petersen criteria; Petersen, 2004). Volunteers taking medications and or have neurological conditions that may affect memory, and those unable to exercise vigorously without causing harm to self were ineligible to participate. Detailed inclusion and exclusion criteria have been previously published (Iyalomhe et al., 2015). While we did not use the MoCA assessment tool in the study, MMSE tool was used for screening but not a part of baseline measures. We are yet to analyze or associate the expression data with their cognitive score.
Briefly, eligibility and inclusion criteria for the study subjects include age <55 years; ability to exercise vigorously without causing harm to self (Iyalomhe et al., 2015); MCI diagnostic category according to Petersen criteria (Petersen, 2004) and in good general health. Diagnosis of MCI was made using the established criteria that include memory complaints, education adjusted Mini-Mental State Examination (MMSE) scores, as described (Mungas et al., 1996; Iyalomhe et al., 2015).
Exercise Training Protocol
Qualified and willing volunteers underwent a maximal treadmill exercise test for 3-month and 6-month using the Bruce protocol (Bruce and Hornsten, 1969) and as previously detailed (Iyalomhe et al., 2015).
Study Sample and Processing
The sample for this analysis included those who completed baseline, 3-month, and 6-month follow-up visits and have data on the variables that informed the scientific focus of this analysis.
Fasting blood samples were collected aseptically from all eligible volunteers at baseline, 3-months, and 6-months and allowed to clot. Serum samples were separated from clotted blood and immediately kept both at −80°C until further analysis.
Total RNA Extraction and Reverse Transcription
To assess gene expression, total RNA was extracted from clotted blood samples using Trizol-Reagent according to the manufacturer’s protocol, followed by determination of RNA concentration using the Nanodrop 1,000 Spectrophotometer (Thermo Fisher Scientific, Waltham, MA, USA). The ratio between A260/A280 was in the range of 1.75–1.95 for all samples. cDNA first strand was generated from 300 ng RNA using the High-Capacity cDNA Reverse Transcription (RT) Kit in 20 μl reaction buffer (Thermo Fisher Scientific, Waltham, MA, USA). The RT protocol consisted of 10 min at 25°C for primer annealing, 120 min at 37°C for DNA polymerization, 5 min at 85°C for enzyme deactivation, and finally cooled to 4°C indefinitely. The cDNA was stored at −20°C until further analysis.
Gene Expression by Quantitative Real Time-Polymerase Chain Reaction (qRT-PCR)
Gene expression level was assessed using the TaqMan gene expression assay system using 2× TaqMan Fast Advanced Master Mix and 20× FAM-MGM labeled probe sets (Applied Biosystems). The 20× FAM-MGM labeled target gene-specific probes sets have the following Assay IDs: Hs01041408_m1 for FBXO32, Hs00231106_m1 for FOXO1, and Hs99999905_m1 for GAPDH (Thermo Fisher Scientific, Waltham, MA, USA). The TaqMan Fast Advanced Master Mix contains AmpliTaq Fast DNA Polymerase, uracil-N glycosylase (UNG), dNTPs with dUTP, ROX™ dye (passive reference), and optimized buffer components. All probe sets were used at a final concentration of 100 nM. The PCR conditions include UNG incubation for 2 min at 50°C; polymerase activation at 92°C for 10 min; 40-cycles of denaturation at 97°C for 1 s; and annealing/extension at 62°C for 20 s. Data were normalized to GAPDH to compensate for the efficiency of the reverse transcription and differences in the amount of input RNA. Reactions were monitored on Applied Biosystems ViiA™ 7 Real-Time PCR System, and data analyzed with corresponding ViiA7 RUO software. qRT-PCR assays were performed in duplicate on all control and experimental group and normalized against GAPDH and baseline control. Relative quantification of gene expression was conducted according to the 2(−ΔΔCT) relative expression method as described (Livak and Schmittgen, 2001; Bedada et al., 2014).
Gel Electrophoresis of PCR Products
To analyze reaction quality and yield of PCR product, we used the Invitrogen E-Gel Electrophoresis System and precast 2% E-Gel agarose gels with SYBR Safe and E-Gel 1 Kb Plus DNA Ladder (Thermo Fisher Scientific, Waltham, MA, USA). Gel imaging was done using Bio-Rad imager, a gel documentation system (Bio-Rad, Irvine, CA, USA).
Statistical Methods
For baseline screening of FBXO32 and FOXO1, threshold cycle (Ct) value of FBXO32 amplicons for each participant at baseline was averaged and normalized to average Ct value of corresponding GAPDH and used as calibrator. Next, the Ct value of FBXO32 amplicons for individual participants was normalized to the Ct value of the corresponding GAPDH and compared to calibrator value (average of Ct value of FBXO32 for all participants normalized to Ct value of corresponding GAPDH at baseline). For the calculation of specific expression of FOXO1, a similar approach was used.
To assess the differential effect of exercise on gene expression, Ct value of FBXO32 amplicons for all individual (separately for male and female) participants at baseline were averaged and normalized to average Ct value of corresponding GAPDH and were used as calibrator. Then, the average Ct value of FBXO32 amplicon at the 3-month exercise time point for all male or female participants was normalized to the average Ct value of corresponding GAPDH at 3-month exercise time point and was then compared to the male or female baseline calibrator sample (average of Ct value of FBXO32 for all (separately for male and female) participants normalized to Ct value of corresponding GAPDH at baseline). A similar approach was used to calculate the specific expression of FBXO32 at 6-month in male or female participants. A similar approach was used for FOXO1 as well.
To determine statistical significance, data were analyzed by either t-test or one-way ANOVA to compare intervention groups and expressed as mean ± SEM. P < 0.05 was considered statistically significant. For baseline subjects, n = 42 where, female = 27 and male = 15. For 3-month exercise time points, n = 15 while female = 10 and male = 5. For 6-month exercise time points n = 21 while female = 15 and male = 6.
Results
Baseline Expression of FBXO32 and FOXO1
As an initial quality control check, we analyzed reaction quality, RNA integrity, cDNA first stand synthesis, and yield of PCR product using the Invitrogen E-Gel Electrophoresis System. Accordingly, gel electrophoresis-based detection of qRT-PCR products for FBXO32 showed a consistent and specific band corresponding to FBXO32 and GAPDH (Figure 1A), suggesting the cDNAs first strands were synthesized properly, and the RNAs were intact. Since FOXO transcription factors initiate transcription of FBXO32 by binding to its promoter elements (Bodine and Baehr, 2014), we developed schematics depicting FBXO32 transcription process (Figure 1B). To investigate the role of FBXO32 and FOXO1 in immune cells, we determined their expression levels in RNA samples isolated from clotted PB of AA with MCI. Next, we quantified FBXO32 and FOXO1 in real-time using the qRT-PCR. Accordingly, both genes were expressed in a cohort of 42 subjects tested at baseline (Figure 1C). In all cases, GAPDH was used as an internal control and for normalization purposes. Collectively, these expression data suggest that FBXO32 and FOXO1 might have an important role in systemic circulation and can play a therapeutic role when these PB cells carrying FBXO32 and FOXO1 trafficked into the brain. Specifically, recent reports implicate exercise to increase BBB permeability and promote the trafficking of blood factors to the brain (Małkiewicz et al., 2019); this view prompted us to investigate further the role of fitness adaptation on the expression level of FBXO32 and FOXO1 in AA with MCI.
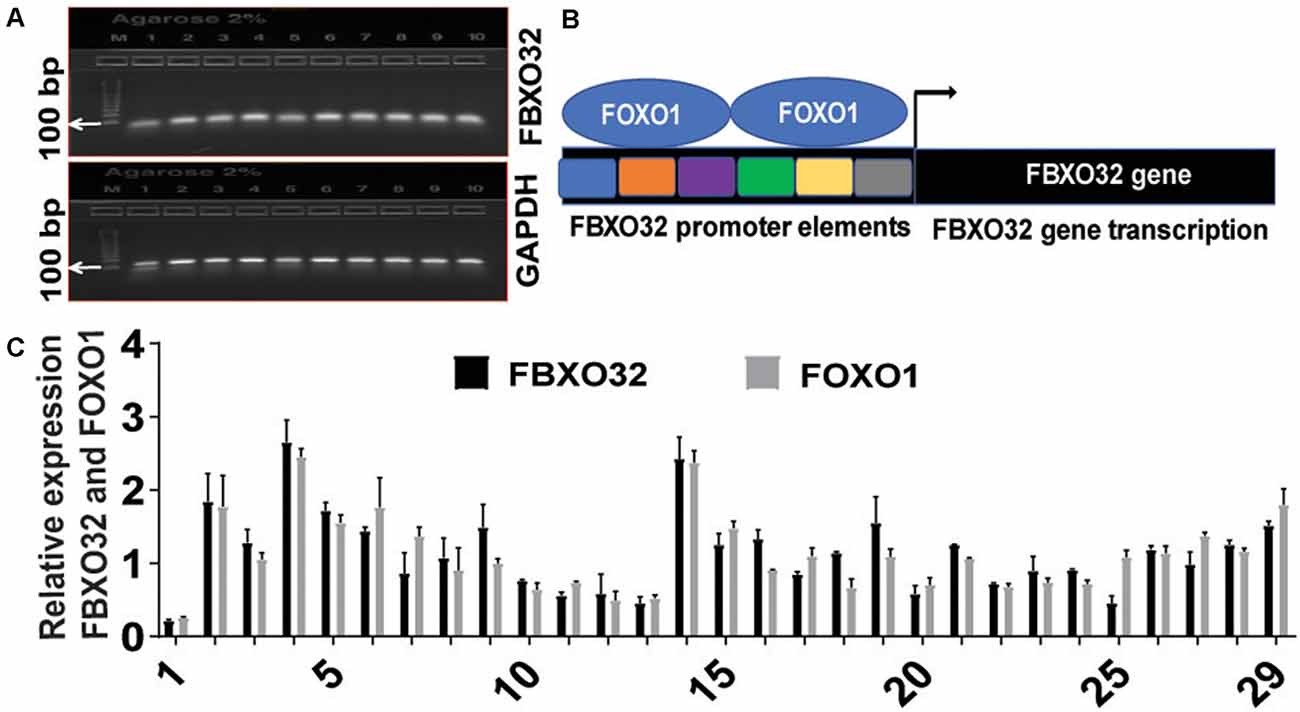
Figure 1. Baseline expression of FBXO32 and FOXO1. (A) Gel electrophoresis-based detection of quantitative real time-polymerase chain reaction (qRT-PCR) products showing consistent detection of FBXO32 and the corresponding housekeeping gene, GAPDH. (B) Schematics depicting promoter elements of FBXO32 and the binding of FOXO1 transcription factor on the promoter region. (C) Baseline quantification of FBXO32 and FOXO1 in real-time using the qRT-PCR. In all cases, GAPDH was used as an internal control and for normalization purposes. Data were run in duplicate and analyzed by one-way ANOVA and expressed as mean ± SEM, n = 42 for baseline subjects.
Gender-Dependent Expression of FBXO32 and FOXO1 at Baseline and Following Exercise Intervention
Age and gender are leading risk factors for developing AD, and female subjects tend to have a higher incidence of the disease than men (Filon et al., 2016). First, we analyzed levels of FBOX32 and FOXO1 at baseline in both genders. Data at baseline show that higher levels of FBXO32 and FOXO1 expression in female (Figure 2A and gray bar) than in male subjects (Figure 2A and black bar), suggesting females may have high reserves of FBXO32 and FOXO1 at baseline (Figure 2A).
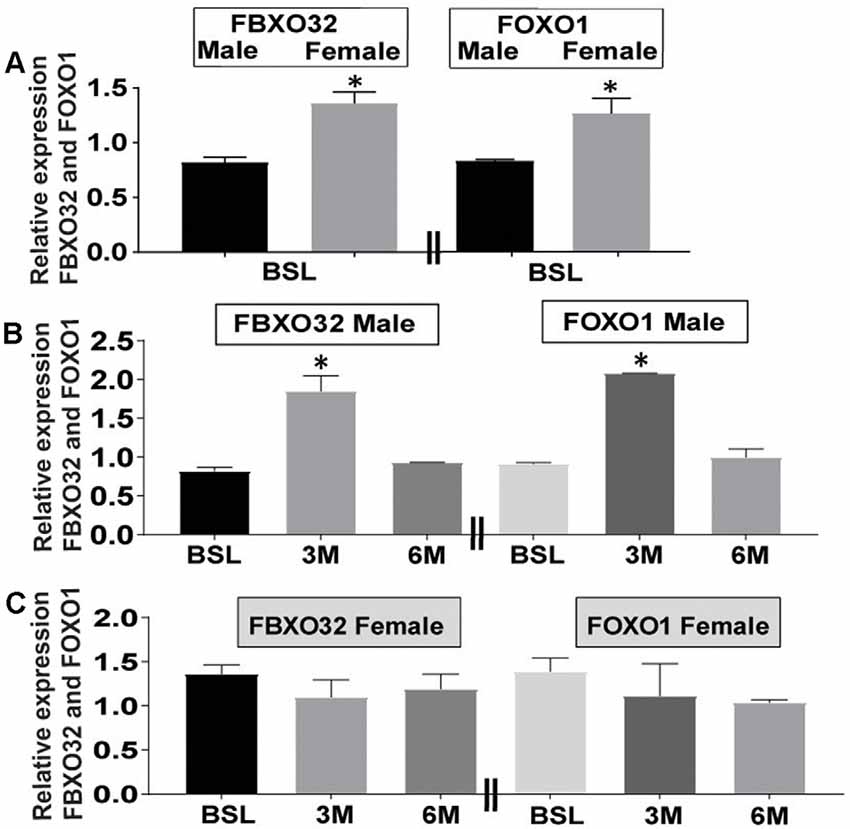
Figure 2. Differential expression of FBXO32 and FOXO1 at baseline and effect of exercise intervention by gender. (A) Representative data showing increased expression of FBXO32 and FOXO1 at baseline in female subjects (Gray bar) compared with male counterparts (Black bar). (B) Representative data showing increased expression of FBXO32 in male subjects at the 3-month exercise time point (left panel) and increased expression of FOXO1 in male subjects at the 3-month exercise time point (right panel). (C) Representative data showing lack of effect of exercise on the expression of FBXO32 in female subjects at all time points (left panel) and lack of exercise effects on the expression of FOXO1 in female subjects at all time points (right panel). Data were run in duplicate and analyzed by either t-test or one-way ANOVA and expressed as mean ± SEM. For baseline subjects, n = 42 where, female = 27 and male = 15. For 3-month exercise time points, n = 15 where female = 10 and male = 5. For 6-month exercise time points, n = 21 were female = 15 and male = 6. *P < 0.05 for panels (A,B). Limitation: because the collection of 3-month blood samples was conceived after protocol initiation, this time point included fewer volunteers than the 6-month visit.
Next, in our attempt to evaluate the differential effects of exercise-related changes by gender, we analyzed the level of FBXO32 and FOXO1 expression following exercise intervention in both genders (Figures 2B,C). Our data indicate that exercise-induced significant changes in the expression level of FBXO32 (Figure 2B and left panel) and FOXO1 (Figure 2B and right panel) in male subjects (Figure 2B). We evidenced an acute increase at the 3-month exercise-training time point, which dialed back to basal level following a 6-month exercise intervention (Figure 2B).
On the other hand, fitness adaptation did not induce significant changes in the expression level of FBXO32 (Figure 2C and left panel) and FOXO1 (Figure 2C and right panel) in female subjects (Figure 2C). Collectively, these data suggest a differential effect of gender on the expression level of FBXO32 and FOXO1 at baseline (Figure 2A) and following exercise intervention (Figures 2B,C).
Discussion
The complexity of AD pathology and the challenge of early diagnosis hinder the likelihood of therapeutic success. Thus, targeting exercise intervention can be an invaluable alternative to mitigate the progression of MCI to AD. Of note, recent work establishes a link between exercise and increased permeability of BBB, facilitating the migration of therapeutic blood factors to the brain (Małkiewicz et al., 2019).
In this study, we determined if fitness adaptation can increase the expression of FBXO32 and FOXO1, and therefore, potentially have favorable effects on ND, perturbed balance, and aging. Our finding has two components. First, we observed gender-based differences at baseline in the expression level of FBXO32 and FOXO1. Second, we evidenced the differential effect of exercise, where a 3-month exercise intervention increased levels of FBXO32 and FOXO1 in males compared to females. Given the significance of FBXO32 and FOXO1 in ND, and muscle function, our findings, may at least in part, inform the advantageous effects of exercise on memory, declining muscle coordination, and progression of ND in AA MCI participants.Perturbed muscle coordination, gait, and balance have been suggested to herald the early phase of cognitive deterioration (Gras et al., 2015; Goyal et al., 2019). Given the role of FBXO32 in muscle dynamics and function, and the acknowledged effects of exercise on muscle, gait and balance, we explored the effects of exercise on FBXO32 expression. A prior study showed that IL-4, a blood factor, served as myoblast recruitment factor during muscle growth (Horsley et al., 2003). New evidence in mice demonstrates that the up-regulation of IL-4 contributes to aerobic exercise-induced insulin sensitivity (Chen et al., 2020). These observations likely suggest a link between exercise, blood factors, muscle dynamics, and metabolism. Our observation that FBXO32 expression increased following a 3-month exercise (Figure 2B), suggests that exercise-training effects may peak at 3-month and then trend towards equilibrium at 6-month. We attribute the apparent effect at the 3-month time point to the exercise regimen. Briefly, each participant initially trained for 20 min at an intensity targeted at 50% VO2Max. We then increased training duration by 5 min each week until each participant reached 40 min of exercise at 50% VO2Max, and then increased training intensity by 5% VO2Max/week until reaching 70% VO2Max. Notably, this peaking of exercise frequency, intensity, and duration coincided with the 3-month time point. This implies that fitness level may have plateaued at 3-month, thereby motivating less changes in biomarkers. The remaining 3-month of training occurred in the context of physiologic adjustments, feedback mechanisms, and ensuring biologic adjustments. Therefore, counter regulatory mechanisms may underly the blunting, at least in part, some exercise effects at the 6-month time point. Of note, the apparent high baseline level of FBXO32 and FOXO1 in female subjects can potentially mount negative feedback responses to avoid further saturation of the UPS system. Therefore, female participants may not have responded to exercise intervention analogously to male participants who have a lower level of FBXO32 and FOXO1 at baseline.
This beneficial effect of exercise-induced increase in FBXO32 is analogous to impaired autophagy/lysosome system in FBXO32 deficient zebrafish (Buhler et al., 2016). Further, genetic deletion of FBXO32 in aging rodents shortened their lifespan and caused significant muscle atrophy, corroborating the need for FBXO32 in normal protein turnover during aging (Sandri, 2013; Sandri et al., 2013).
UPS plays an essential role in maintaining cellular quality and homeostasis (Sandri, 2013). In an experimentally induced DNA damage such as genotoxic stress, stabilization of FBXO32 led to the activation of NF-κB via polyubiquitination and proteasome-mediated degradation of an inhibitor IκBα (Meshram et al., 2017). Thus, FBXO32 is a potential activator of NF-κB signaling during genotoxic stress and inflammatory response (Meshram et al., 2017). Notably, AD is an inflammatory disorder; and reduced levels of nuclear NF-κB immunoreactivity have been observed around plaques in human AD brains (Kaltschmidt et al., 1999). Inhibition of NF-κB potentiates Aβ-mediated apoptosis in primary rat neurons (Kaltschmidt et al., 1999). FBXO32-mediated activation of NF-κB protects the cells, maintains their genomic integrity, and allow restoration of a healthy life cycle (Ryan et al., 2000; Kawahara et al., 2009; Meshram et al., 2017). Similarly, the beneficial effect of fitness adaptation through the induction of FBXO32, and therefore, proteolysis may counter the negative effect of c-myc, a proto-oncogene (Mei et al., 2015). Recently, transgenic expression of c-myc in neuronal cells induced significant cognitive deficits and ND (Lee et al., 2009). Since cell cycle disturbances may precede neuronal death in AD (Wojsiat et al., 2015), the exercise-induced increase in FBXO32 in PB can counter cell cycle disturbances mitigating the progression of MCI to AD. Thus, exercise training-induced increase of FBXO32 in our AA MCI sample may reduce inflammation, ND, and cell cycle disturbance. This is consistent with recent studies that showed a favorable effect of exercise on BBB permeability (Małkiewicz et al., 2019), facilitating the trafficking of neuroprotective blood factors to the brain (Carro et al., 2001; Trejo et al., 2001; Liu and Nusslock, 2018).
Our gender-dependent changes in FBXO32 at baseline and after exercise (Figures 2A,B) could be linked to gender hormones such as dihydrotestosterone (DHT), estradiol (E2), and estrogen receptor (Svensson et al., 2010). For instance, reduced expression of FBXO32 was associated with gonadectomy in male mice (Svensson et al., 2010), and treatment with DHT or E2 normalized the reduced level (Svensson et al., 2010). Gender hormones also influence FOXO expression as revealed by subcutaneous E2 implant, which improved insulin sensitivity and suppressed gluconeogenesis in both male and ovariectomized female mice (Yan et al., 2019), and insulin resistance is linked to the pathogenesis of AD (Kellar and Craft, 2020). However, these effects of E2 were abolished in FOXO1 knockout mice, implying an interaction between FOXO1 and E2 (Yan et al., 2019). Further, the deletion of ERα exhibited reduced levels of FBXO32 and FOXO1 (Ribas et al., 2016), underscoring the importance of gender hormones for maintaining the levels of FBXO32 and FOXO1. Further, FBXO32 RNA seq expression data from human protein atlas (HPA) data set and genotype tissue expression (GTEx) data set shows higher expression of FBXO32 in several female tissues such as the endometrium, fallopian tube, breast, ovary, cervix uteri, placenta, and vagina compared with limited male tissues such as seminal vesicles, prostate, epididymis, and testis1. Similarly, higher expression of FOXO1 is documented in several female tissues compared with male tissues2.
The FOXO transcription family members regulate FBXO32 (Webb and Brunet, 2014; Dang et al., 2016; Yin et al., 2018). Concordant with our findings for FOXO1, the baseline levels of FOXO3 mRNA were higher in older women (Raue et al., 2007), and FBXO32 expression is also regulated by FOXO3A (Zheng et al., 2010; Webb and Brunet, 2014). Others have identified FOXO as a guardian of neuronal integrity, protecting against age-progressive axonal degeneration (Hwang et al., 2018). FOXOs played a role as a regulator of longevity and genes involved in cellular metabolism and resistance to oxidative stress (Webb and Brunet, 2014; Martins et al., 2016; Hwang et al., 2018). Collectively, these observations put female subjects at a unique advantage to have a large pool of FBXO32 and FOXO1 compared with male subjects. We contend that the apparent high level of FBXO32 and FOXO1 can mount negative feedback responses to avoid further saturation of the UPS system and creating imbalance. As a result, female subjects did not respond to exercise training compared with male subjects who have lower levels of FBXO32 and FOXO1 at baseline. We plan to further inform additional proteolytic mechanisms in conjunction with FBXO32 following exercise intervention involving males and females in future studies.
Neurodegeneration is a complex and idiopathic disease and is an inevitable consequence of aging (Hwang et al., 2018). A prior study linked age-related decline in the expression of FOXOs to ND, suggesting FOXOs play a central role in neuroprotection during mammalian aging and ND (Hwang et al., 2018). Central to NDs are UPS and autophagy, two major intracellular clearing systems, the impairment of which is widely implicated in protein aggregation and neurotoxicity (Pan et al., 2008; Bedford et al., 2009; Jaeger and Wyss-Coray, 2009). Since these neurodegenerative proteinopathies operate at the crossroad between UPS and autophagy, the interplay between these degradation and clearance systems need to be balanced to ensure effective protein homeostasis (Limanaqi et al., 2020). Accordingly, recent work shows that FOXO/FBXO32/endophilin network may play an important role in balancing autophagy and UPS and thereby maintain neuronal health in the mammalian brain (Murdoch et al., 2016). The overall protein homeostasis and health of neurons are maintained by the interplay between FOXO/FBXO32/endophilin (Murdoch et al., 2016). Previously, FBXO32 has been described as a mediator between the UPS and autophagy (Zaglia et al., 2014; Mei et al., 2015; Buhler et al., 2016; Meshram et al., 2017); and along with endophilin-A necessary for autophagosome formation in neurons and the mammalian brain (Murdoch et al., 2016). Taken together, our observations and others highlight the relationship between FBXO32/FOXO1 and NDs.
Collectively, these studies support our observation that exercise training-induced changes in FBXO32 and FOXO1 expressions may, at least in part, explain the advantageous effects of exercise on neurodegenerative processes such as in MCI.
Concluding Remarks
In this study, we evaluated the effect of fitness adaptation on gene expression in PB by investigating E3 ubiquitin ligase components of the UPS; and their regulator FOXO1. We demonstrate that exercise intervention increases FBXO32 and FOXO1 in a gender-dependent manner. However, gene expression analysis performed in this study may not capture the full spectrum of the biologic effects of exercise in AA with MCI. Further investigations that include additional genes, protein levels, and cytokines at similar time points are, therefore, required. We will inform this in future analysis.
Data Availability Statement
The raw data supporting the conclusions of this article will be made available by the authors, without undue reservation.
Ethics Statement
This study involved human participants and was reviewed and approved by Howard University IRB. The participants provided their written informed consent to participate in this study.
Author Contributions
FB and TO conceived, planned, and carried out unique experiments and wrote the manuscript. ON contributed to the study, sample preparation, RNA isolation, cDNA preparation, and manuscript preparation. EN, TF, and SN contributed to the study design, study implementation, and manuscript review. TO conceived the design and implemented the overall study. All authors contributed to the article and approved the submitted version.
Funding
This work was supported by the National Institute on Aging at the National Institutes of Health (NIH) grant R01 5R01AG031517 and 5R01AG045058 to TO, and in part by Grant #UL1TR000101 from National Center for Advancing Translational Sciences/NIH, through the Clinical and Translational Science Award Program (CTSA). The content is solely the responsibility of the authors and does not necessarily represent the official views of the National Institutes of Health.
Conflict of Interest
The authors declare that the research was conducted in the absence of any commercial or financial relationships that could be construed as a potential conflict of interest.
Acknowledgments
We acknowledge the contributions of the Howard University Cognitive Aging Group for their roles in the exercise study. We also thank Dr. Sergei Nekhai (Howard University) for providing the tool for imaging gel electrophoresis.
Footnotes
- ^ https://www.proteinatlas.org/ENSG00000156804-FBXO32/tissue
- ^ https://www.proteinatlas.org/ENSG00000150907-FOXO1/tissue
References
Attaix, D., Ventadour, S., Codran, A., Bechet, D., Taillandier, D., and Combaret, L. (2005). The ubiquitin-proteasome system and skeletal muscle wasting. Essays Biochem. 41, 173–186. doi: 10.1042/EB0410173
Bedada, F. B., Chan, S. S.-K., Metzger, S. K., Zhang, L., Zhang, J., Garry, D. J., et al. (2014). Acquisition of a quantitative, stoichiometrically conserved ratiometric marker of maturation status in stem cell-derived cardiac myocytes. Stem Cell Rep. 3, 594–605. doi: 10.1016/j.stemcr.2014.07.012
Bedford, L., Paine, S., Rezvani, N., Mee, M., Lowe, J., and Mayer, R. J. (2009). The UPS and autophagy in chronic neurodegenerative disease: six of one and half a dozen of the other—or not? Autophagy 5, 224–227. doi: 10.4161/auto.5.2.7389
Bodine, S. C., and Baehr, L. M. (2014). Skeletal muscle atrophy and the E3 ubiquitin ligases MuRF1 and MAFbx/atrogin-1. Am. J. Physiol. Endocrinol. Metab. 307, E469–E484. doi: 10.1152/ajpendo.00204.2014
Bruce, R. A., and Hornsten, T. R. (1969). Exercise stress testing in evaluation of patients with ischemic heart disease. Prog. Cardiovasc. Dis. 11, 371–390. doi: 10.1016/0033-0620(69)90027-9
Buhler, A., Kustermann, M., Bummer, T., Rottbauer, W., Sandri, M., and Just, S. (2016). Atrogin-1 deficiency leads to myopathy and heart failure in zebrafish. Int. J. Mol. Sci. 17:187. doi: 10.3390/ijms17020187
Carro, E., Trejo, J. L., Busiguina, S., and Torres-Aleman, I. (2001). Circulating insulin-like growth factor I mediates the protective effects of physical exercise against brain insults of different etiology and anatomy. J. Neurosci. 21, 5678–5684. doi: 10.1523/JNEUROSCI.21-15-05678.2001
Chen, Z., Qin, X., Zhang, X., Liu, B., and Chen, M. (2020). Upregulation of IL-4 signaling contributes to aerobic exercise-induced insulin sensitivity. Biochem. Biophys. Res. Commun. 525, 662–667. doi: 10.1016/j.bbrc.2020.02.103
Dang, K., Li, Y.-Z., Gong, L.-C., Xue, W., Wang, H.-P., Goswami, N., et al. (2016). Stable atrogin-1 (Fbxo32) and MuRF1 (Trim63) gene expression is involved in the protective mechanism in soleus muscle of hibernating Daurian ground squirrels (Spermophilus dauricus). Biol. Open 5, 62–71. doi: 10.1242/bio.015776
Dubois, B., Hampel, H., Feldman, H. H., Scheltens, P., Aisen, P., Andrieu, S., et al. (2016). Preclinical Alzheimer’s disease: definition, natural history, and diagnostic criteria. Alzheimers Dement. 12, 292–323. doi: 10.1016/j.jalz.2016.02.002
Farhan, M., Wang, H., Gaur, U., Little, P. J., Xu, J., and Zheng, W. (2017). FOXO signaling pathways as therapeutic targets in cancer. Int. J. Biol. Sci. 13, 815–827. doi: 10.7150/ijbs.20052
Filon, J. R., Intorcia, A. J., Sue, L. I., Arreola, E. V., Wilson, J., Davis, K. J., et al. (2016). Gender differences in Alzheimer disease: brain atrophy, histopathology burden, and cognition. J. Neuropathol. Exp. Neurol. 75, 748–754. doi: 10.1093/jnen/nlw047
Gate, D., Rezai-Zadeh, K., Jodry, D., Rentsendorj, A., and Town, T. (2010). Macrophages in Alzheimer’s disease: the blood-borne identity. J. Neural Transm. 117, 961–970. doi: 10.1007/s00702-010-0422-7
Goyal, N., Luna, G., Curuk, E., and Aruin, A. S. (2019). Role of motor and cognitive tasks in gait of individuals with mild cognitive impairment. Int. J. Rehabil. Res. 42, 174–179. doi: 10.1097/MRR.0000000000000341
Gras, L. Z., Kanaan, S. F., McDowd, J. M., Colgrove, Y. M., Burns, J., and Pohl, P. S. (2015). Balance and gait of adults with very mild Alzheimer disease. J. Geriatr. Phys. Ther. 38, 1–7. doi: 10.1519/JPT.0000000000000020
Hohsfield, L. A., and Humpel, C. (2015). Migration of blood cells to beta-amyloid plaques in Alzheimer’s disease. Exp. Gerontol. 65, 8–15. doi: 10.1016/j.exger.2015.03.002
Horsley, V., Jansen, K. M., Mills, S. T., and Pavlath, G. K. (2003). IL-4 acts as a myoblast recruitment factor during mammalian muscle growth. Cell 113, 483–494. doi: 10.1016/s0092-8674(03)00319-2
Hwang, I., Oh, H., Santo, E., Kim, D. Y., Chen, J. W., Bronson, R. T., et al. (2018). FOXO protects against age-progressive axonal degeneration. Aging Cell 17:e12701. doi: 10.1111/acel.12701
Iyalomhe, O., Chen, Y., Allard, J., Ntekim, O., Johnson, S., Bond, V., et al. (2015). A standardized randomized 6-month aerobic exercise-training down-regulated pro-inflammatory genes, but up-regulated anti-inflammatory, neuron survival and axon growth-related genes. Exp. Gerontol. 69, 159–169. doi: 10.1016/j.exger.2015.05.005
Jaeger, P. A., and Wyss-Coray, T. (2009). All-you-can-eat: autophagy in neurodegeneration and neuroprotection. Mol. Neurodegener. 4:16. doi: 10.1186/1750-1326-4-16
Kaltschmidt, B., Uherek, M., Wellmann, H., Volk, B., and Kaltschmidt, C. (1999). Inhibition of NF-κB potentiates amyloid β-mediated neuronal apoptosis. Proc. Natl. Acad. Sci. U S A 96, 9409–9414. doi: 10.1073/pnas.96.16.9409
Kawahara, T. L., Michishita, E., Adler, A. S., Damian, M., Berber, E., Lin, M., et al. (2009). SIRT6 links histone H3 lysine 9 deacetylation to NF-κB-dependent gene expression and organismal life span. Cell 136, 62–74. doi: 10.1016/j.cell.2008.10.052
Kellar, D., and Craft, S. (2020). Brain insulin resistance in Alzheimer’s disease and related disorders: mechanisms and therapeutic approaches. Lancet Neurol. 19, 758–766. doi: 10.1016/S1474-4422(20)30231-3
Kostin, S., Pool, L., Elsässer, A., Hein, S., Drexler, H. C., Arnon, E., et al. (2003). Myocytes die by multiple mechanisms in failing human hearts. Circ. Res. 92, 715–724. doi: 10.1161/01.RES.0000067471.95890.5C
Kriegenburg, F., Ellgaard, L., and Hartmann-Petersen, R. (2012). Molecular chaperones in targeting misfolded proteins for ubiquitin-dependent degradation. FEBS J. 279, 532–542. doi: 10.1111/j.1742-4658.2011.08456.x
Lee, H.-G., Casadesus, G., Nunomura, A., Zhu, X., Castellani, R. J., Richardson, S. L., et al. (2009). The neuronal expression of MYC causes a neurodegenerative phenotype in a novel transgenic mouse. Am. J. Pathol. 174, 891–897. doi: 10.2353/ajpath.2009.080583
Limanaqi, F., Biagioni, F., Gambardella, S., Familiari, P., Frati, A., and Fornai, F. (2020). Promiscuous roles of autophagy and proteasome in neurodegenerative proteinopathies. Int. J. Mol. Sci. 21:3028. doi: 10.3390/ijms21083028
Liu, P. Z., and Nusslock, R. (2018). Exercise-mediated neurogenesis in the hippocampus via BDNF. Front. Neurosci. 12:52. doi: 10.3389/fnins.2018.00052
Livak, K. J., and Schmittgen, T. D. (2001). Analysis of relative gene expression data using real-time quantitative PCR and the 2ΔΔCT method. Methods 25, 402–408. doi: 10.1006/meth.2001.1262
Małkiewicz, M. A., Szarmach, A., Sabisz, A., Cubala, W. J., Szurowska, E., and Winklewski, P. J. (2019). Blood-brain barrier permeability and physical exercise. J. Neuroinflammation 16:15. doi: 10.1186/s12974-019-1403-x
Malm, T., Koistinaho, M., Muona, A., Magga, J., and Koistinaho, J. (2010). The role and therapeutic potential of monocytic cells in Alzheimer’s disease. Glia 58, 889–900. doi: 10.1002/glia.20973
Martins, R., Lithgow, G. J., and Link, W. (2016). Long live FOXO: unraveling the role of FOXO proteins in aging and longevity. Aging Cell 15, 196–207. doi: 10.1111/acel.12427
Mei, Z., Zhang, D., Hu, B., Wang, J., Shen, X., and Xiao, W. (2015). FBXO32 targets c-Myc for proteasomal degradation and inhibits c-Myc activity. J. Biol. Chem. 290, 16202–16214. doi: 10.1074/jbc.M115.645978
Meshram, S. N., Paul, D., Manne, R., Choppara, S., Sankaran, G., Agrawal, Y., et al. (2017). FBXO32 activates NF-κB through IκBα degradation in inflammatory and genotoxic stress. Int. J. Biochem. Cell Biol. 92, 134–140. doi: 10.1016/j.biocel.2017.09.021
Metaxakis, A., Ploumi, C., and Tavernarakis, N. (2018). Autophagy in age-associated neurodegeneration. Cells 7:37. doi: 10.3390/cells7050037
Morris, J. K., Honea, R. A., Vidoni, E. D., Swerdlow, R. H., and Burns, J. M. (2014). Is Alzheimer’s disease a systemic disease? Biochim. Biophys. Acta 1842, 1340–1349. doi: 10.1016/j.bbadis.2014.04.012
Mungas, D., Marshall, S. C., Weldon, M., Haan, M., and Reed, B. R. (1996). Age and education correction of Mini-Mental State Examination for English and Spanish-speaking elderly. Neurology 46, 700–706. doi: 10.1212/wnl.46.3.700
Murdoch, J. D., Rostosky, C. M., Gowrisankaran, S., Arora, A. S., Soukup, S. F., Vidal, R., et al. (2016). Endophilin-A deficiency induces the Foxo3a-Fbxo32 network in the brain and causes dysregulation of autophagy and the ubiquitin-proteasome system. Cell Rep. 17, 1071–1086. doi: 10.1016/j.celrep.2016.09.058
Pan, T., Kondo, S., Le, W., and Jankovic, J. (2008). The role of autophagy-lysosome pathway in neurodegeneration associated with Parkinson’s disease. Brain 131, 1969–1978. doi: 10.1093/brain/awm318
Petersen, R. C. (2004). Mild cognitive impairment as a diagnostic entity. J. Intern. Med. 256, 183–194. doi: 10.1111/j.1365-2796.2004.01388.x
Raue, U., Slivka, D., Jemiolo, B., Hollon, C., and Trappe, S. (2007). Proteolytic gene expression differs at rest and after resistance exercise between young and old women. J. Gerontol. A Biol. Sci. Med. Sci. 62, 1407–1412. doi: 10.1093/gerona/62.12.1407
Ribas, V., Drew, B. G., Zhou, Z., Phun, J., Kalajian, N. Y., Soleymani, T., et al. (2016). Skeletal muscle action of estrogen receptor alpha is critical for the maintenance of mitochondrial function and metabolic homeostasis in females. Sci. Transl. Med. 8:334ra54. doi: 10.1126/scitranslmed.aad3815
Ryan, K. M., Ernst, M. K., Rice, N. R., and Vousden, K. H. (2000). Role of NF-κB in p53-mediated programmed cell death. Nature 404, 892–897. doi: 10.1038/35009130
Sandri, M. (2013). Protein breakdown in muscle wasting: role of autophagy-lysosome and ubiquitin-proteasome. Int. J. Biochem. Cell Biol. 45, 2121–2129. doi: 10.1016/j.biocel.2013.04.023
Sandri, M., Barberi, L., Bijlsma, A. Y., Blaauw, B., Dyar, K. A., Milan, G., et al. (2013). Signalling pathways regulating muscle mass in ageing skeletal muscle: the role of the IGF1-Akt-mTOR-FoxO pathway. Biogerontology 14, 303–323. doi: 10.1007/s10522-013-9432-9
Schulze, M., Belema-Bedada, F., Technau, A., and Braun, T. (2005). Mesenchymal stem cells are recruited to striated muscle by NFAT/IL-4-mediated cell fusion. Genes Dev. 19, 1787–1798. doi: 10.1101/gad.339305
Schwartz, M., and Shechter, R. (2010). Systemic inflammatory cells fight off neurodegenerative disease. Nat. Rev. Neurol. 6, 405–410. doi: 10.1038/nrneurol.2010.71
Svensson, J., Movérare-Skrtic, S., Windahl, S., Swanson, C., and Sjogren, K. (2010). Stimulation of both estrogen and androgen receptors maintains skeletal muscle mass in gonadectomized male mice but mainly via different pathways. J. Mol. Endocrinol. 45, 45–57. doi: 10.1677/JME-09-0165
Tang, M. X., Cross, P., Andrews, H., Jacobs, D. M., Small, S., Bell, K., et al. (2001). Incidence of AD in african-americans, caribbean hispanics, and caucasians in northern manhattan. Neurology 56, 49–56. doi: 10.1212/wnl.56.1.49
Trejo, J. L., Carro, E., and Torres-Aleman, I. (2001). Circulating insulin-like growth factor I mediates exercise-induced increases in the number of new neurons in the adult hippocampus. J. Neurosci. 21, 1628–1634. doi: 10.1523/JNEUROSCI.21-05-01628.2001
Webb, A. E., and Brunet, A. (2014). FOXO transcription factors: key regulators of cellular quality control. Trends Biochem. Sci. 39, 159–169. doi: 10.1016/j.tibs.2014.02.003
Wojsiat, J., Laskowska-Kaszub, K., Mietelska-Porowska, A., and Wojda, U. (2017). Search for Alzheimer’s disease biomarkers in blood cells: hypotheses-driven approach. Biomark. Med. 11, 917–931. doi: 10.2217/bmm-2017-0041
Wojsiat, J., Prandelli, C., Laskowska-Kaszub, K., Martin-Requero, A., and Wojda, U. (2015). Oxidative stress and aberrant cell cycle in Alzheimer’s disease lymphocytes: diagnostic prospects. J. Alzheimers Dis. 46, 329–350. doi: 10.3233/JAD-141977
Yan, H., Yang, W., Zhou, F., Li, X., Pan, Q., Shen, Z., et al. (2019). Estrogen improves insulin sensitivity and suppresses gluconeogenesis via the transcription factor foxo1. Diabetes 68, 291–304. doi: 10.2337/db18-0638
Yin, J., Yang, L., Xie, Y., Liu, Y., Li, S., Yang, W., et al. (2018). Dkk3 dependent transcriptional regulation controls age related skeletal muscle atrophy. Nat. Commun. 9:1752. doi: 10.1038/s41467-018-04038-6
Zaglia, T., Milan, G., Ruhs, A., Franzoso, M., Bertaggia, E., Pianca, N., et al. (2014). Atrogin-1 deficiency promotes cardiomyopathy and premature death via impaired autophagy. J. Clin. Invest. 124, 2410–2424. doi: 10.1172/JCI66339
Zeng, Y., Li, J., Wang, H.-X., Guo, S.-B., Yang, H., Zeng, X.-J., et al. (2013). Transcriptional effects of E3 ligase atrogin-1/MAFbx on apoptosis, hypertrophy and inflammation in neonatal rat cardiomyocytes. PLoS One 8:e53831. doi: 10.1371/journal.pone.0053831
Keywords: ubiquitin proteasome system (UPS), FBXO32/atrogin-1, FoxO transcription factor, cellular clearance system, aging, immune cells, mild cognitive impairment, neurodegeneration
Citation: Bedada FB, Ntekim OE, Nwulia EO, Fungwe TV, Nadarajah SR and Obisesan TO (2021) Exercise Training-Increased FBXO32 and FOXO1 in a Gender-Dependent Manner in Mild Cognitively Impaired African Americans: GEMS-1 Study. Front. Aging Neurosci. 13:641758. doi: 10.3389/fnagi.2021.641758
Received: 14 December 2020; Accepted: 18 March 2021;
Published: 14 April 2021.
Edited by:
Zhexing Wen, Emory University, United StatesReviewed by:
Murali Vijayan, Texas Tech University Health Sciences Center, United StatesFerdinando Franzoni, University of Pisa, Italy
Copyright © 2021 Bedada, Ntekim, Nwulia, Fungwe, Nadarajah and Obisesan. This is an open-access article distributed under the terms of the Creative Commons Attribution License (CC BY). The use, distribution or reproduction in other forums is permitted, provided the original author(s) and the copyright owner(s) are credited and that the original publication in this journal is cited, in accordance with accepted academic practice. No use, distribution or reproduction is permitted which does not comply with these terms.
*Correspondence: Thomas O. Obisesan, dG9iaXNlc2FuQGhvd2FyZC5lZHU=